- 1Departamento Científico, Instituto Antártico Chileno, Punta Arenas, Chile
- 2Faculté de Sciences, CNRS, UMR 7144, Adaptation et Diversité en Milieu Marin, AD2M, Station Biologique de Roscoff, Sorbonne Université, Roscoff, France
- 3Faculté de Sciences, CNRS, FR 2424 CNRS, ABiMS, Station Biologique de Roscoff, Sorbonne Université, Roscoff, France
- 4Instituto de Acuicultura, Universidad Austral de Chile, Puerto Montt, Chile
- 5Centro FONDAP de Investigación de Altas Latitudes (IDEAL), Punta Arenas, Chile
The Western Antarctic Peninsula (WAP) is among the areas of the planet showing some of the most significant increases in air and water temperature. It is projected that increasing temperature will modulate coastal ecosystems at species ecological performance and molecular composition. The main way that the organisms can cope with large thermal variation is by having a reversible phenotypic plasticity, which provides the organisms with a compensatory physiological response when facing challenging conditions. The giant Antarctic isopod Glyptonotus antarcticus is one of most common species in Antarctic waters. This species has a larval development inside of the maternal marsupium, where juveniles have a short period to acclimate to environmental conditions after birth. In this sense, we hypothesize that juveniles exposed to unusual temperature increases even for short periods, would not respond adequately showing a narrow phenotypic plasticity. We experimentally assessed if early juveniles of G. antarcticus have the molecular plasticity when exposed to increased temperature at 5°C during 1, 6, 12 and 24 h. Sequenced libraries were compared between control (0°C) and each experimental treatment to detect differentially expressed transcripts. The main molecular pathways affected by thermal stress were antioxidant, proteases, endopeptidases and ubiquination transcripts which were up-regulated and mitochondrial respiratory chain, cuticle, cytoskeleton and a molt transcript which were down-regulated. Regarding the HSP transcript, only 3 were up-regulated at least in two points of the stress kinetic, without classical Hsp70 and Hsp90 transcripts. This study shows that juveniles of G. antarcticus do not show molecular phenotypic plasticity to cope with acute short-term heat stress, even for one or few hours of exposure with an absence of an eco-physiological capacity to respond. This may have consequences at the ecological population level, showing a reduced individual ability to survive decreasing population recruitment.
Introduction
The Western Antarctic Peninsula (WAP) is among the areas of the planet showing most significant increases in air and water temperature (Stenni et al., 2017), with evidence indicating that the mean atmospheric temperatures increased by 3°C (0.6°C per decade) and sea surface temperatures by 1°C (SST in the upper 100 m) between 1955 and 2004 (Moffat and Meredith, 2018). The IPCC report for Polar regions has shown that in recent years (2005–2017), the Southern Ocean was responsible for an increased proportion of the global ocean heat increase (45–62%) (high confidence) (Meredith et al., 2019). These evidences confirm that this region includes a major center of considerable, recent warming in the shallows, and this is forecast to be sustained (Barnes et al., 2021). Consequently, increased sea temperatures might cause rapid changes in the Southern Ocean and its inhabitants (Morley et al., 2020). With very few exceptions, the Antarctic marine species are more sensitive to temperature variations than other marine groups from other regions (Peck, 2011, Peck et al., 2013). The projected that rising temperatures could be modulate communities of coastal ecosystems at species ecological performance and molecular composition (Ashton et al., 2017; Peck, 2018; Morley et al., 2020).
Organisms shifts in size structure, spatial range, and seasonal abundances of populations may occurs by altered physiological function behavior, and demographic traits as consequences of exposure to directs effects of in ocean temperature (Doney et al., 2012; Griffiths et al., 2017). According to Doney et al. (2012), the main determinant of a species tolerance to environmental variability and change is their physiological performance. In fact, organisms initially respond based on physiological and behavioral adaptation to climate changes, according to their evolutionary history (Somero, 2012). Changes and establishment of new environmental conditions may be physiologically tolerable, allowing acclimatization, or may be intolerable, provoking migration, change phenology or death and local extinction if adaptation is not possible (Parmesan, 2006).
The principal way that the organisms can cope with large thermal variation is having a reversible phenotypic plasticity, providing to the organisms with a compensatory physiological response when facing challenging conditions (Clusella-Trullas et al., 2014). Species with short generation times require little phenotypic plasticity and primarily respond having genetic modifications. In contrasts, species with long generations (as most Antarctic marine invertebrates and fishes) are expected to have wide range of phenotypic plasticity to survive long enough for adaptation processes to take effect (Peck, 2011, 2018; Somero, 2012, 2015; Chown et al., 2015; Convey and Peck, 2019). However, a large proportion of these studies have been conducted in adult individuals without considering how developmental stage or age affect their responses, mainly because of the availability of tissue or depending on what animals are available (Clark et al., 2016). The response of juveniles to thermal stress has been characterized in few species of Antarctic organisms (Rinehart et al., 2006; Clark et al., 2013, 2016; Peck et al., 2013; Husmann et al., 2014). In the case of the Antarctic midge, Belgica antarctica, the heat shock proteins are continuously and strongly expressed during larval life. Results suggest that adult midges exhibit no constitutive up-regulation of their Hsps and have a lower intrinsic tolerance to high temperatures (Rinehart et al., 2006). Other studies on the Antarctic clam (Laternula elliptica) have assessed changes in gene expression levels in young and older individuals (Husmann et al., 2014; Clark et al., 2016).
Transcriptomic modulation is the main mechanism of phenotypic plasticity, being the first line of response to environmental stress. Pfennig et al. (2010) defined phenotypic plasticity as the ability the ability of a single genotype to produce multiple phenotypes in response to variation in the environment. The gene expression act as mechanisms driving most phenotypic plasticity and in several crustaceans studies detected changes in transcriptome expression and also shifts in the heat-shock proteins expression (Logan and Cox, 2020). Regarding marine Antarctic invertebrates, Clark et al. (2017) showed that a crustacean Paraceradocus miersi, a brachiopod Liothryrella uva and a bivalve mollusk Laternula elliptica showed a heat shock response with the upregulation of expression of HSP70 family genes. In the same study, the gastropod Marseniopsis mollis and the echinoderm Cucumaria georgiana did not show Heat shock response, but the expression of mitochondrial electron transport chain. In the case of the Antarctic sponge Isodictya sp., a genome-wide transcriptomic assessment to thermal stress (3 and 5°C) found up-regulated genes including HSP, ribosomal proteins, ubiquitin related genes and few genes with antioxidant activity (González-Aravena et al., 2019). This study revealed that degree and type of stress response varied little from 3 to 5°C, suggesting that even a moderate increased temperature could induce a stress at the limit of this organism capacity. Another experimental study demonstrated that genes involved in cellular stress response were differentially expressed in Laternulla elliptica individuals exposed to warming of just 2°C over summer temperatures found in the natural habitat (Truebano et al., 2010). A recent study evaluating the genome-wide transcriptomic response to ocean acidification and warming in the pelagic Antarctic gastropod Limacina helicina antarctica evidenced that temperature is the master variable driving global change of gene expression (Johnson and Hofmann, 2020). These results suggest that this species do not have enough plasticity to respond to environmental stresses reaching the limit capacities to cope its. Altogether, transcriptomic evaluation of thermal stress with crustacean and marine Antarctic invertebrates, provide valuable ecologically relevant information about eco-physiology responses and whether they possess phenotypic plasticity to cope with this stress.
Glyptonotus antarticus (Eights) is a large marine isopod, and it has been recorded from several islands of the Southern Ocean (South Georgia, South Orkneys and South Shetland Islands) as well as in the Ross Sea, at depths from the littoral level to 585 m (Janecki and Rakusa-Suszczewski, 2006). In Fildes Bay (King George Island, South Shetland) G. antarcticus is normally associated to soft bottoms between depths of 20 and 40 m. This species has a larval development inside of maternal marsupium (Janssen and Hoese, 1993), hence their juveniles have a short period to acclimate to environmental conditions after birth. Despite the highly phenotypic plasticity recorded in some Antarctic marine invertebrates (Morley et al., 2012, 2016), it is expected that Antarctic ectotherms have a specialization to a narrow range of low temperatures (Pörtner et al., 2007). In this sense, we hypothesize that if juveniles are exposed to unusual temperature even for shorts periods (few hours), they would not be able to respond adequately to warming, showing a narrow phenotypic plasticity. Genome-wide transcriptomic assessments are highly important to determine if early juveniles are eco-physiological capable to respond to ecologically relevant environmental stress, under temperature prediction for 2100 (IPCC, 2019). The aim of this study is to determine if early juveniles of the giant isopod G. antarcticus have the molecular plasticity once they are exposed to an acute (1, 6, 12 and 24 h) 5°C heat shock.
Materials and Methods
Animal Collection and Experimental Heat Stress Exposure
Forty specimens of G. antarcticus were collected by SCUBA diving at 10–20 m depth during the Austral summer at Maxwell Bay (62°12′12.2″S–58°56′41.7″ W), Fildes Peninsula, King George Island. After collections, animals transported to the laboratory and maintained in a re-circulating seawater system in a temperature-controlled room (1.0 ± 1.0°C, 34% salinity) and constant aeration. Specimens were acclimated for 1 week before experiments. Gravid females were separated and examined for juveniles within the marsupial sack. Four-day old juveniles (ca. 7 mm total length), released by a single female, were used for the experiments. Juveniles were placed in 50 mL flasks filled with 0.45 μm UV-sterilized seawater at the experimental condition within thermostated baths.
A laboratory-controlled heat stress kinetic was performed using 36 juveniles of G. antarcticus individuals exposed to 5°C temperature, whereas 18 individuals were maintained at summer normal temperature 0°C (Control). Nine heat-stressed organisms were sampled at 1, 6, 12, and 24 h to form three pools of three individuals, at each kinetic point, prior to RNA isolation. Equally, nine non-stressed organisms were sampled at 1 and 24 h to constitute three pools of three individuals at each point for RNA isolation of control conditions. No fatalities were observed during the thermal exposure.
RNA Isolation and Sequencing
Three biological replicates constituted by pools of three individuals were used for RNA isolation at each kinetic point of thermal stress (1, 6, 12, and 24 h). In addition, 2 control points of normal summer temperature (1 and 24 h) were conducted with the same criteria. All samples were placed in RNAlater (Qiagen, Hilden, Germany) and stored at −80°C on site.
The RNA isolations were performed with E.Z.N.A® Total RNA Kit II (Omega Bio-Tek Inc., Norcross, GA, United States), previously homogenized the whole bodies with Precellys® Evolution with a Cryolys cooling unit (Bertin Technologies, Montigny-Le-Bretonneux, France). RNA concentrations were measured using a Nanoquant spectrophotometer (Tecan, Switzerland). RNA samples were precipitated with ethanol and sent to the Macrogen company for libraries preparing and sequencing. RNA quality and quantity were determined on a 2100 Bioanalyzer (Agilent Technologies, Santa Clara, CA, United States). One μg of RNA from each pool was used to produce RNA libraries for sequencing, with a TruSeq Stranded mRNA kit (Illumina, San Diego, CA, United States). Sequencing was performed in a paired-end 100 bp mode using Illumina Hiseq2500 platform. The cDNA ensemble corresponding to 18 libraries was deposited at the NCBI GEO database with accession ID GSE180716.
Bioinformatic Analyses
Eighteen paired-end libraries were obtained from Illumina HiSeq 2500 sequencing. The raw sequences were analyzed under Galaxy Platform ABIMS from Biologist Station of Roscoff1, until finding the differentially expressed transcripts between control and treatment samples (Figure 1). Firstly, the raw sequences were checked for quality, passing all of them the filter criterion with all nucleotides with > 30 phred score, excepting 9 first and last one nucleotides. For these reasons, the first 9 nucleotides and the last one of all raw reads were trimmed with Trim sequences program. Then, a reference transcriptome was assembled using all libraries with Trinity (Grabherr et al., 2011) and annotated with Trinotate. Alignment analyses were performed for every sample with Bowtie2 (Langmead et al., 2009) to assess Transcriptome quality showing aligned percentage between 81.68 and 84.78% with an average of 83.64% evidencing a high accurate assembly. This reference transcriptome assembly was used for aligning and counting reads for each sample with the normalization method “Transcripts Per Millions” (TPM) using Salmon program (Patro et al., 2017). The TPM normalized counts were used to compare generate expressions matrixes of transcript expression among experimental conditions. Then, these matrixes were used to obtain the differentially expressed transcripts (DET) using EdgeR software (Robinson et al., 2009), considering differentially expressed with a fold-chage > 1.5 and False discovery rate < 0.05. The files with differentially expressed transcripts were exported from Galaxy and their list of transcripts with Suisseprot abbreviation were used to obtain interaction networks and enriched GOterms with STRING online program (Szklarczyk et al., 2019), for down and up-regulated of all comparisons together and for each time points separately (Supplementary Material 3).
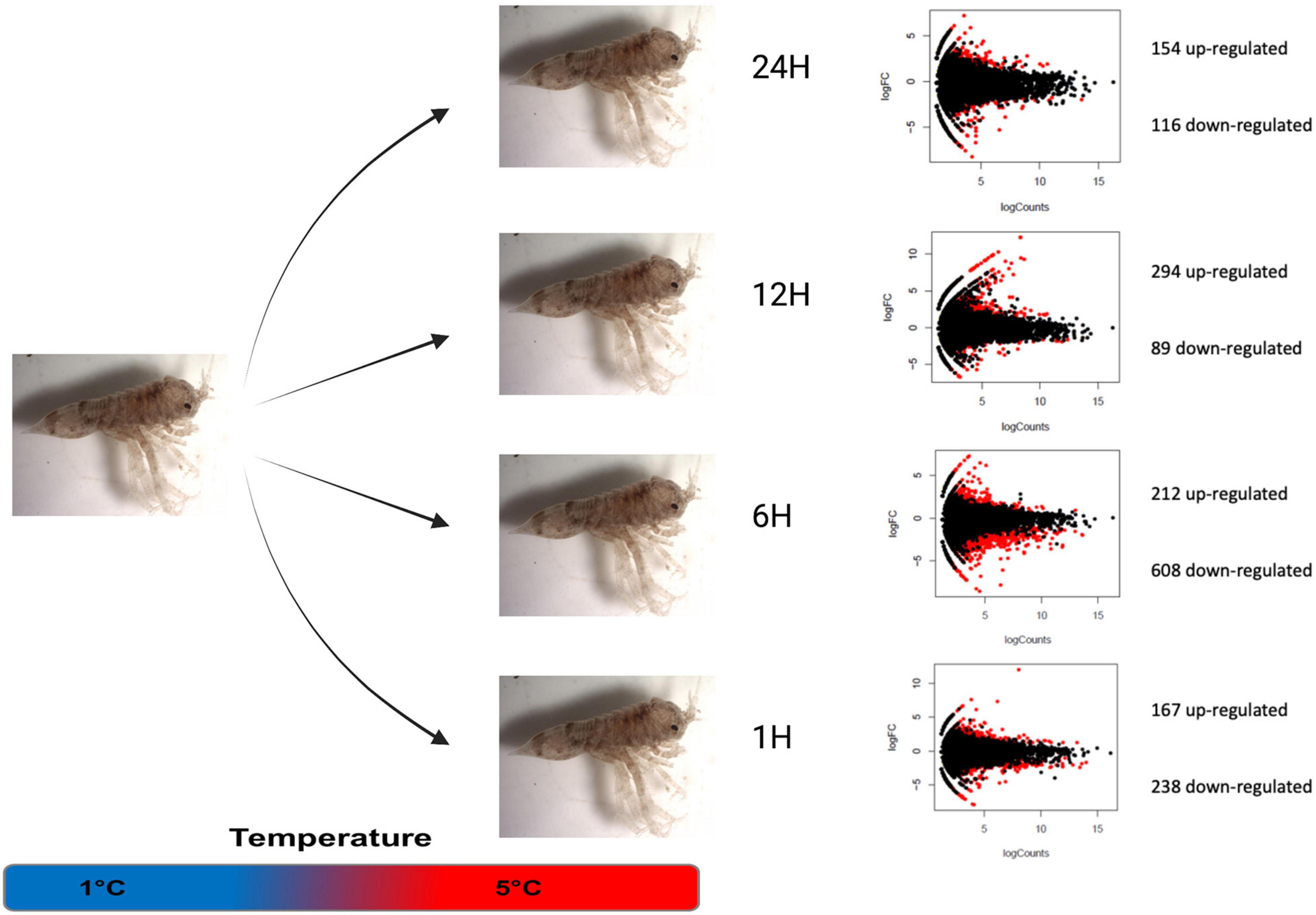
Figure 1. Differentially expressed transcripts between control (1°C) and treatment (5°C) conditions during different kinetic times. Volcano plots showing the up- and down-regulated genes between heat stress and control condition at each time point. Genes which were found to be significantly different by edgeR are indicated in red.
Results
Transcriptome Sequencing Stats
After exposure to experimental condition, RNA isolation, library preparation and sequencing 674,021,026 paired-end reads were yielded, with 37,445,612.56 reads per sample average. Using the total 18 paired-end libraries, we assembled a high-quality reference transcriptome containing 175,629 transcripts. This transcriptome has a N50 of 1,093 nucleotides and an average contig 1,071.13 nucleotides based on all transcript contigs. Regarding completeness of contigs, comparing with Arthropoda database, the BUSCO software revealed a 97% of contigs as complete (40.3% of contigs as complete and single copy and 56.7% complete and duplicated), 2% as fragmented and 1% as missing (Table 1).
Differentially Expressed Transcripts
The transcript expression level counting with normalized TPM were compared between Control 1 h (1°C 1 h) and 1°C 24 h to assess the effects of sampling time during the experiment. Differential gene expression analysis using edgeR suggests a very low effect of aquarium maintenance during the experimental work, showing only 40 differentially expressed genes 23 down-regulated and 17 upregulated. In contrast, comparisons between Control 1 h and thermal stress treatments showed that several transcripts were affected by acute thermal stress at 5°C from 1 to 24 h (Figure 1). At the 1-h 5°C acute stress, 405 transcripts were differentially expressed with 238 down- and 167 up-regulated. Following the stress kinetic at 6 h, 820 differentially expressed transcripts (DET) have been detected with 608 down and 212 up-regulated, being this point with the most transcriptional effects. Then, at the 12-h stress point, 383 differentially expressed transcripts were recorded, with 89 down and 294 up-regulated, being this experimental treatment the only one showing more up-regulated than down-regulated transcripts. Finally, at 24 h, 270 transcripts were revealed having differentially express level, with 116 down and 154 up-regulated transcripts (Figure 1 and Supplementary Material 1).
Considering all DET lists at the four experimental comparisons, we observed an overall pattern of molecular pathways affected by acute thermal stress with groups of transcripts at least in two points of the experimental kinetic (Figure 2 and Supplementary Material 2). Firstly, we detected an induction of few transcript coding for Heat Stress Proteins (HSP) including Stress-70 protein (Hsc70-5), Stress-induced-phosphoprotein 1 and Calumenin-B. However, other HSP such as Hsp83 and Hsp7D were also repressed. Interestingly, two antioxidant transcripts were differentially expressed during the entire thermal stress kinetic. This included Super oxide dismutase [SOD (Cu-Zn)], Glutathione peroxidase (GPx). In contrast, Glutathione S-transferase (GST) was differentially expressed at two points. Other two pathways that were clearly affected were the proteases and ubiquination with several transcripts induced by acute thermal stress with 20 and 4, respectively. Interestingly, a protease potentially involved in crustacean molt, the cathepsin L was down-regulated at the middle and at the end of the kinetic. Another effect of acute stress was the markedly repression of several transcript coding for cuticle proteins including cuticle proteins AM1199, CP1876, AM/CP1144, cuticlin-1 among others (Figure 2 and Supplementary Material 2), and also the energy genes such as mitochondrial respiratory chain (Cytochrome c oxidase; Cytochrome b; NADH dehydrogenase among others) and cytoskeleton transcripts (tubuline, myosin and actin).
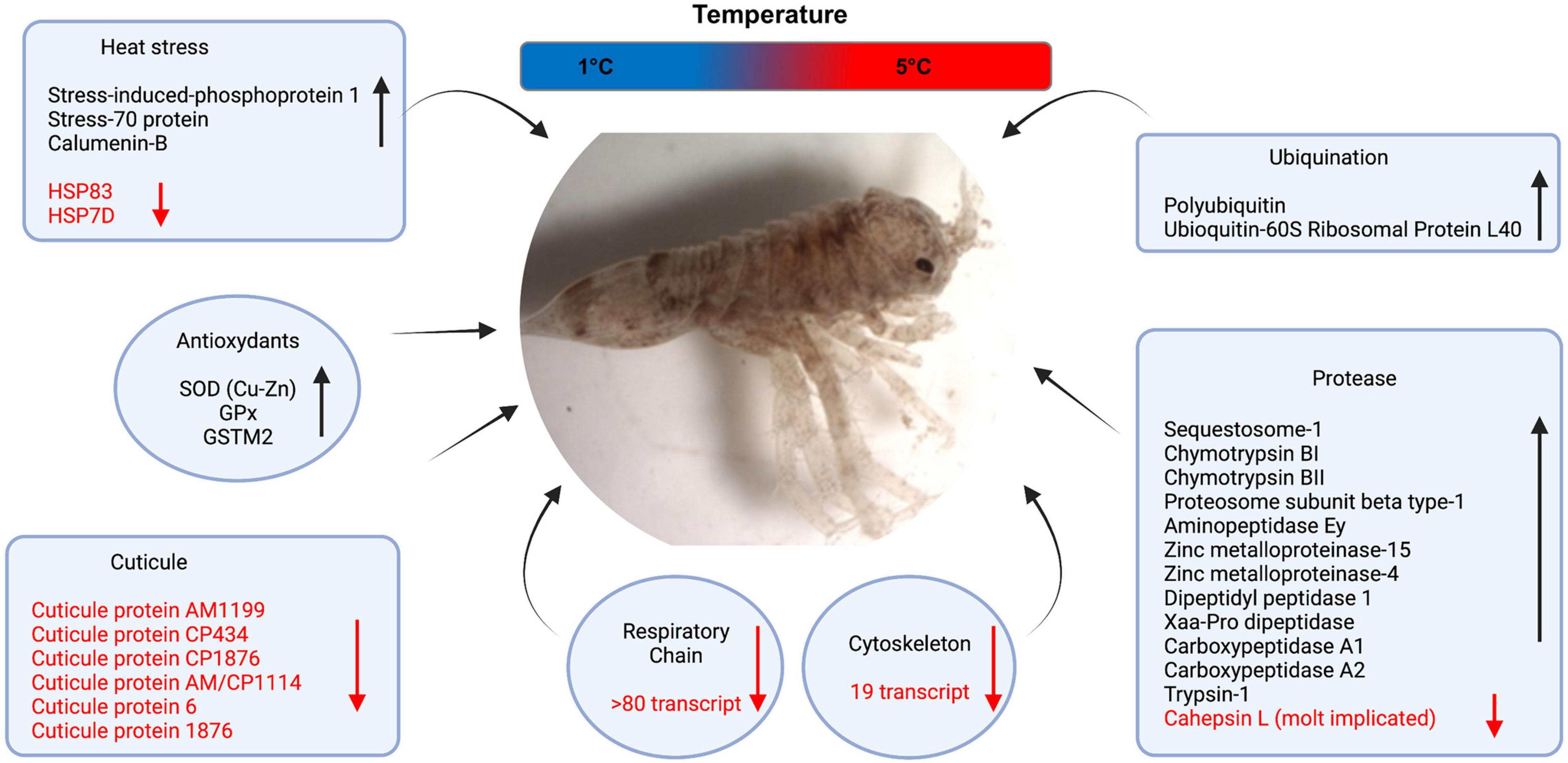
Figure 2. Relevant molecular pathways with differentially expressed transcripts. Up-regulated transcripts are shown in black and down-regulated in red.
Enrichment GO Terms Analyses
At 1 h of thermal stress (5°C) the down-regulated transcripts were enriched with molecular function implicated in translation, such as such mannose and kinase binding with potentially function in cellular signaling, respectively (Supplementary Material 3 and Supplementary Figure 1). Among up-regulated transcripts at this kinetic point, the analysis detects an enrichment with peptidase molecular functions GO terms (Supplementary Material 3 and Supplementary Figure 1). After 6-h exposure, many molecular functions were enriched with down-regulated transcript list. Between these functions, some of them are implicated in translation as such as pre-mRNA and ribosome binding, mitochondrial electron transfer activity and ATP binding, cytoskeletal and actin binding, and finally some peptidases (Supplementary Material 3 and Supplementary Figure 2). Considering up-regulated transcripts list at 6-hour exposure, most of molecular functions were related with peptidase activity and another two with oxidoreductase activity (Supplementary Material 3 and Supplementary Figure 2). The analysis of the transcripts list at 12-h exposure showed that no enriched GO terms were revealed nor for down and up-regulated lists (Supplementary Material 3 and Supplementary Figure 3). Contrary to the first 3 point of the kinetic, at the end of the experimental exposure (24 h) most of differentially transcripts were up-regulated of which are overrepresented with molecular functions as peptidases, hydrolases and phospholipase (Supplementary Material 3 and Supplementary Figure 4). Regarding the down-regulated transcript at 24 h, these were enriched with electron transfer activity, adenylate cyclase binding, and cytoskeletal protein binding molecular functions (Supplementary Material 3 and Supplementary Figure 4).
Protein-Protein Interaction Network Analyses
The visualization of the different biological processes at each point of the kinetics showed that the protein-protein interaction network were consistent with enrichment analyses. The differentially expressed transcripts between control and heat stress for down- and up regulated transcripts list at each time point (1, 6, 12, and 24 h) show several highlighted processes (Figures 3, 4). Specifically, the analyze for the down- regulated genes revealed the interaction of transcriptional, alternative splicing, translation, cytoskeletal and mitochondrial energy transcripts were recorded with interactions (Supplementary Material 3 and Supplementary Figures 7–9). The only exception was at 12-hours which has a very narrow set of transcripts with interactions (Supplementary Material 3 and Supplementary Figure 10). For up-regulated transcript lists for each time point, the most relevant interactions showed by the analyze were peptidases, ubiquination, antioxidant and co-chaperone transcripts, of which were present at the majority of kinetic points of heat-stress (Supplementary Material 3 and Supplementary Figures 11–14).
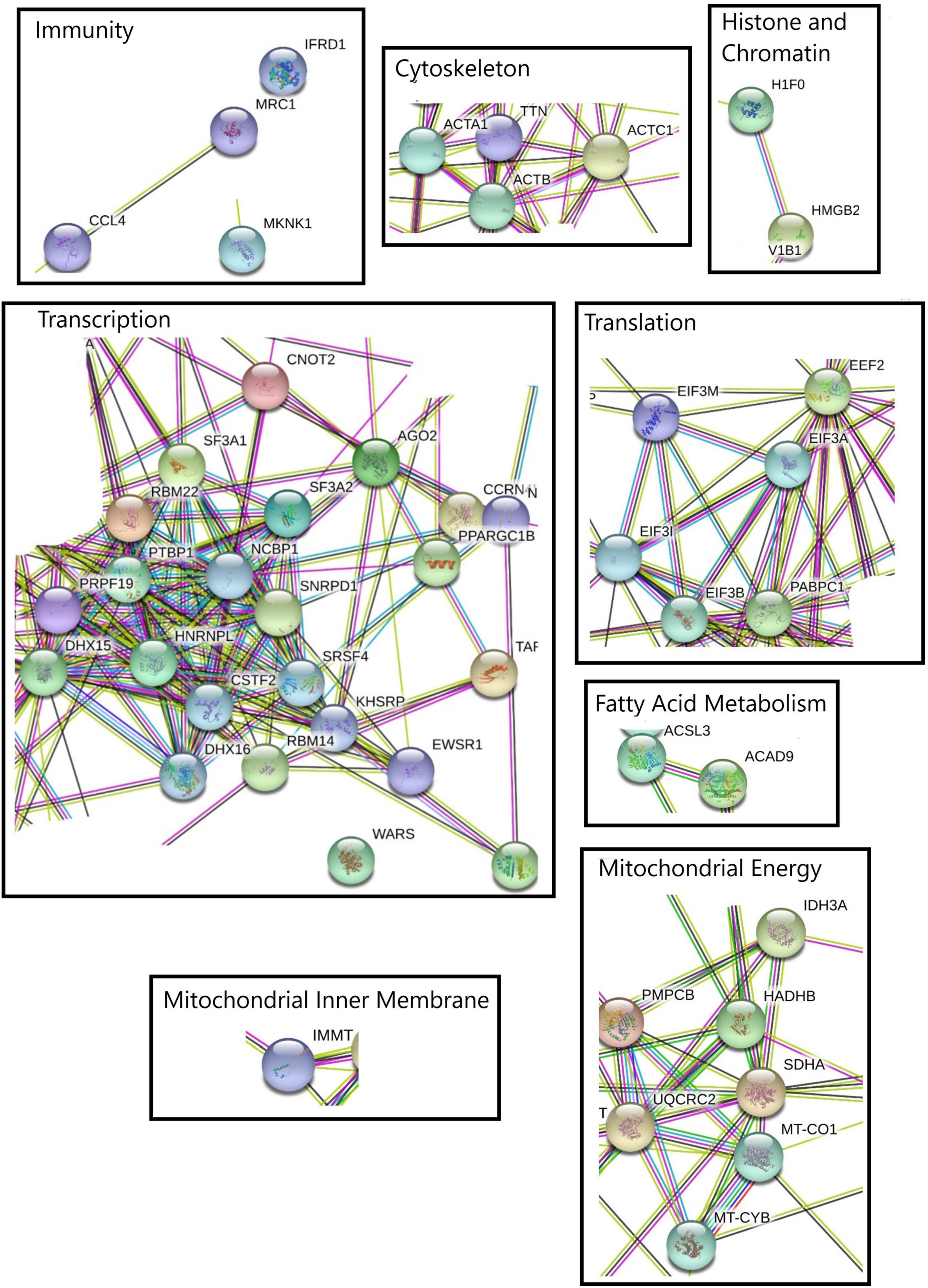
Figure 3. Protein-protein interaction network performed with STRING online software using four kinetic points for down-regulated transcripts. The relevant molecular pathways were zoomed and placed in squares indicating cluster of transcript representations of each pathway. Please see Supplementary Material 3 and Supplementary Figure 5 for a complete view of protein-protein interaction network.
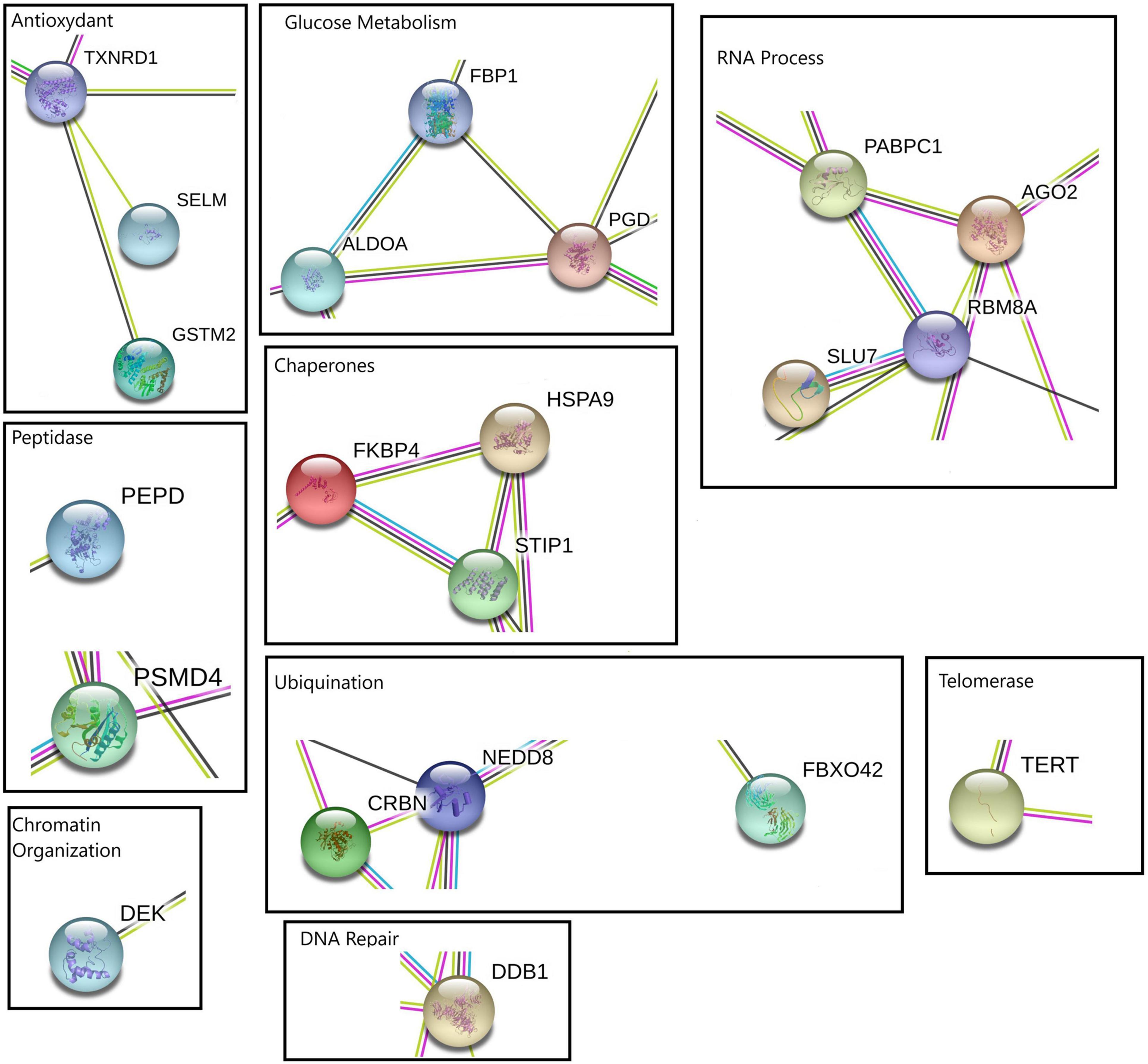
Figure 4. Protein-protein interaction network performed with STRING online software using four kinetic points for up-regulated transcripts. The relevant molecular pathways were zoomed and placed in squares indicating cluster of transcript representations of each pathway. Please see Supplementary Material 3 and Supplementary Figure 6 for a complete view of protein-protein interaction network.
Discussion
The cellular response to stress involves a variety of molecular changes at the level of different cell types and tissues. A classic response of organisms to warming is the overexpression of heat stress proteins (Collins et al., 2021). Transcriptomic techniques to assess this response have been widely used and may reflect the physiological coping mechanisms of response. Previous studies in Antarctic crustacean transcriptomes have been already sequenced and assembled improving our understanding of the stress response in this important group (Clark et al., 2011, 2017; Toullec et al., 2013; Cascella et al., 2015; Collins et al., 2021). The genome-wide transcriptomic analyses of G. antarcticus assessing the effects of acute thermal stress during an experimental kinetic showed a dynamic effect of a 5°C temperature increase. The analyses revealed that at the beginning of heat-stress (1 h to 5°C), produce the modulation of 405 transcripts, being more of them half down-regulated, suggesting that a short period of acute stress (1 h) is enough to produce an effect on gene expression. Then, longer exposure at 6-hours (5°C) the effect on gene expression increased with 820 transcripts modulated of which 608 (74.15%) were down-regulated, suggesting that studied organisms were under a negative pressure at this experimental point.
The transcriptomic profiling in Antarctic crustacean has revealed the expression of classical heat shock proteins (hsps) sequences and notable differences in induction pattern. In this context, Antarctic fish have been an important model for approaching the understanding of the role of chaperone proteins in an adaptation to survival at low temperatures. Most of the Antarctic notothenioid fishes, show constitutive expression of HSP70 (Place et al., 2004; Clark et al., 2008). However, some Antarctic zoarcid such as Lycodichthys dearborni, reveal an ability to upregulate the Hsp70 gene in response to a short exposure to 4°C (Place and Hofmann, 2005). In crustaceans the expressions of Hsp70 were measured as thermal stress markers within three species (Paraceradocus miersi, krill Euphausia superba and E. crystallorophias). The expression of Hsp70 transcript in P. miersi was up-regulated, while E. superba and E. crystallorophias in experiments using to moderate thermal shock of 3°C and 6°C, was possible identified 5 types of Hsps, constitutive and inducible forms in both species. Overall, these data confirm that juveniles of G. antarticus showed a poor response, which was similar to the Antarctic crustacean P. miersi due to that most of the differentially expressed genes were downregulated (Collins et al., 2021), however, in P. miersi the expression of chaperones such as Hsp70 is well conserved and was inducible. Moreover, the calumenin-B genes are up-regulated in response to heat shock in the scallop Pecten maximus and the clam Mya truncata (Artigaud et al., 2015; Sleight et al., 2018), however, their specific functions in crustaceans need to be exanimated.
A trend toward reduced expression of genes involved in metabolism in crustaceans with a higher thermal tolerance range has been well documented. The molecular evidence in the response of the amphipods Gammarus chevreuxi and Echinogammarus marinus showed that this animals triggering a hypometabolism in stressful conditions (Collins et al., 2019, 2020, 2021). Interestingly, a study comparing heat-resistant with heat-tolerant populations of the temperate shrimp Oratosquilla oratoria, the majority of differentially expressed genes were down-regulated for heat-sensitive population (Lou et al., 2019), similarly to what occurred in our experiment with G. antarcticus. These authors evidenced the contrary for heat-tolerant population, being the majority of differentially expressed genes up-regulated. Then, a reduction of the molecular response was observed at 12-h and 24 h, with fewer differentially expressed transcripts with a slight increase of up-regulation.
In terms of molecular pathways, the mains affected by the acute thermal stress we found mitochondrial respiratory chain enzymes, implicated in ATP production (Supplementary Material 1). Others pathways affected are antioxidant enzymes, some stress related proteins, proteases, ubiquination enzymes, cytoskeleton, protein synthesis and cuticle proteins (Supplementary Material 1). Regarding the respiratory chain proteins, them were all down-regulated during entire experimental kinetic, suggesting a possible mismatch between oxygen demand and supply system as has been describe in other metazoans (Pörtner and Knust, 2007). This could be explained by the fact that temperature increase provokes a higher ATP demand until at point of missfunction of mitochondrial respiratory chain. Increased oxygen consumption during heat stress is a known process in marine invertebrates and fish (Abele and Puntarulo, 2004), usually producing reactive species oxygen (ROS) that can cause damage at the cellular level (Dan Dunn et al., 2015). These ROS need to be neutralized by antioxidant molecules, which in juvenile G. antarcticus show a clear increase in the expression of these genes such as SOD, GPx, GST, probably to reduce ROS levels produced during the oxidative stress.
Another stress effect observed in juveniles of G. antarcticus was the large set of cytoskeleton proteins such as actin/tubulin/myosin that were down-regulated, being these kind of transcripts previously registered as differentially expressed in others marine Antarctic invertebrates (Truebano et al., 2010; Clark et al., 2017; González-Aravena et al., 2019). The molt cycle is a complex process involving many regulatory pathways and the dynamics of these proteins are closely related to the growth and molting cycle of crustaceans (Gao et al., 2017). Heat stress in juveniles of G. antarcticus could affect this transcriptional process similar to what was previously observed in P. miersi related to down-regulation of cuticule genes and repression of molting (Collins et al., 2021). Other molecular perturbations recorded were the down-regulation of a high number of cuticular proteins associated with arthropod exoskeletons and the down-regulation of a protease involved in exoskeleton construction (Cathepsin L), suggesting that growth energy was allocated to other molecular pathways as a signal of stress at molecular level. In juveniles, regulation of the molting processes is key to ensure constant growth and the repression of cuticle proteins, which are an important composition of the exoskeleton, could affected the rate of growing in this species. Further studies about the characterization of the exoskeleton-related genes and pathways will enhanced our understanding of the molecular mechanisms underlying exoskeleton development in Antarctic crustaceans.
Genes differentially expressed between control and acute heat stress were functionally enriched with processes relating to proteases, endopeptidases and ubiquination pathways. We found transcripts with significant sequence homology to genes encoding proteases such as sequestosome-1, chymotrypsin and trypsin were up regulated during acute heat stress. In addition, chymotrypsin B transcript which participate in various biological processes, including digestion, immune response and molting was detected in all times of the stress condition (Kuballa et al., 2011). Trypsin was also up-regulated but at the end of the kinetics, this enzyme has been described as part of the mechanism that acts to break down tonofibrillae, releasing the old cuticle during molting in the Antarctic krill E. superba (Seear et al., 2010). However, we cannot consider the possible involvement of both enzymes in the activation of the phenoloxidase system which is an important pathway of immune response (Kuballa et al., 2011). The absence of classical HSPs up-regulation and their remarkably up-regulation of protein degrading enzymes, suggest that cells were under acute heat stress needing to discard damaged proteins, rather than folding misfolded peptides. In addition, the increased expression of genes involved in autophagy (polyubiquitin and ubiquitin-60S ribosomal protein L40) processes was very marked and confirms the demonstrated consistency of the heat stress response with autophagy induction in juveniles.
Considering ontological differences in molecular response, nowadays it a matter of debate whether Antarctic invertebrate juveniles are more or less resistant to thermal stress than adults. A comparison of the response to warming between juveniles and adults for 4 marine Antarctic invertebrates showed that for slowly and mid temperature increases, juveniles are more resistant than adults and juveniles of one species were more resistant for fastest warming (Peck et al., 2004, 2013). In the present study, juveniles of G. antarcticus seem to be sensitive to heat stress; however, future studies with adults are needed to verify an ontogenetic differential response. The response of juveniles G. antarcticus to acute heat-stress, suggests that the organisms do not trigger an accurate molecular induction of transcripts to cope with temperature increase. This is hypothesized by the absent of clear HSPs induction, the up-regulation of proteases and endopeptidases together with the induction of antioxidant and down regulation of mitochondrial respiratory chain. The absent of HSP induction during thermal stress, has been ready found in heat-sensitive individuals of Crassostrea gigas, being clearly up-regulated in heat-resistant organisms (Kim et al., 2017). In this sense, juveniles G. antarcticus could be considered as sensitive to heat stress, with the absent of response but affected by protein denaturation and ROS production, trying to mitigate down-regulating energy production and protein synthesis.
The present study, based on a genome-wide transcriptional approach has revealed that juveniles of G. antarcticus do not show molecular phenotypic plasticity to cope to acute short-term heat-stress, without an eco-physiological capacity to respond. The down regulation of cuticle transcripts and molting implicated enzymes, could led to individuals being more susceptible to predation, reducing energy production possibly to limit ROS generation with the down-regulation of mitochondrial respiratory chain. Overall, this molecular picture could reduce individual survivability and decrease population recruitment, which would have ecological consequences at the population level. Future studies are needed to assess acute heat stress in adults and chronic exposure to warming in both juveniles and adults to gain population-level knowledge at the molecular level.
Data Availability Statement
The datasets presented in this study can be found in online repositories. The names of the repository/repositories and accession number(s) can be found below: NCBI (accession: GSE180716).
Author Contributions
MG-A conceived the experimental design and project administration. KP and MG-A carried out the experimental assays. AF carry out RNA extraction and data curation. RR, AF, J-YT, and EC carried out the bioinformatics analyses. RR, CC, and AF carried out the statistical analyses. RR, CC, and MG-A wrote—reviewed and edited the manuscript with contribution of AF. All authors read and approved the final manuscript.
Funding
The study was financed by grants from the ANID-Chile (Fondecyt 11090265, 11190802, and 1131001) and logistical support was provided for the Chilean Antarctic Institute (INACH).
Conflict of Interest
The authors declare that the research was conducted in the absence of any commercial or financial relationships that could be construed as a potential conflict of interest.
Publisher’s Note
All claims expressed in this article are solely those of the authors and do not necessarily represent those of their affiliated organizations, or those of the publisher, the editors and the reviewers. Any product that may be evaluated in this article, or claim that may be made by its manufacturer, is not guaranteed or endorsed by the publisher.
Supplementary Material
The Supplementary Material for this article can be found online at: https://www.frontiersin.org/articles/10.3389/fmars.2021.761866/full#supplementary-material
Footnotes
References
Abele, D., and Puntarulo, S. (2004). Formation of reactive species and induction of antioxidant defense systems in polar and temperate marine invertebrates and fish. Comp. Biochem. Physiol. A 138, 405–415.
Artigaud, S., Richard, J., Thorne, M. A., Lavaud, R., Flye-Sainte-Marie, J., Jean, F., et al. (2015). Deciphering the molecular adaptation of the king scallop (Pecten maximus) to heat stress using transcriptomics and proteomics. BMC Genomics 16:988. doi: 10.1186/s12864-015-2132-x
Ashton, G. V., Morley, S. A., Barnes, D. K. A., Clark, M. S., and Peck, L. S. (2017). Warming by 1°C drives species and assemblage level responses in Antarctica’s marine shallows. Curr. Biol. 27, 2698–2705. doi: 10.1016/j.cub.2017.07.048
Barnes, D. K. A., Ashton, G. V., Morley, S. A., and Peck, L. S. (2021). 1°C warming increases spatial competition frequency and complexity in Antarctic marine macrofauna. Commun. Biol. 4:208. doi: 10.1038/s42003-021-01742-w
Cascella, K., Jollivet, D., Papot, C., Leger, N., Corre, E., Ravaux, J., et al. (2015). Diversification, evolution and sub-functionalization of 70kDa heat-shock proteins in two sister species of Antarctic krill: differences in thermal habitats, responses and implications under climate change. PLoS One 10:e0121642. doi: 10.1371/journal.pone.0121642
Chown, S. L., Clarke, A., Fraser, C. I., Cary, S. C., Moon, K. L., and McGeoch, M. A. (2015). The changing form of Antarctic biodiversity. Nature 522, 431–438. doi: 10.1038/nature14505
Clark, M. S., Fraser, K. P. P., Burns, G. P., and Peck, L. S. (2008). The HSP70 heat shock response in the Antarctic fish Harpagifer antarcticus. Polar Biol. 31, 171–180. doi: 10.1007/s00300-007-0344-5
Clark, M. S., Husmann, G., Thorne, M. A. S., Burns, G., Truebano, M., Peck, L. S., et al. (2013). Hypoxia impacts large adults first: consequences in a warming world. Glob. Change Biol. 19, 2251–2263.
Clark, M. S., Sommer, U., Sihra, J. K., Thorne, M. A. S., Morley, S. A., King, M., et al. (2017). Biodiversity in marine invertebrate responses to acute warming revealed by a comparative multi-omics approach. Glob. Chang. Biol. 23, 318–330. doi: 10.1111/gcb.13357
Clark, M. S., Thorne, M., Toullec, J.-Y., Meng, Y., Luo Guan, L., Peck, L. S., et al. (2011). Antarctic krill 454 pyrosequencing reveals chaperone and stress transcriptome. PLoS One 6:e15919. doi: 10.1371/journal.pone.0015919
Clark, M. S., Thorne, M. A. S., Burns, G., and Peck, L. S. (2016). Age-related thermal response: the cellular resilience of juveniles. Cell Stress Chaperones 21, 75–85. doi: 10.1007/s12192-015-0640-x
Clusella-Trullas, S., Boardman, L., Faulkner, K. T., Peck, L. S., and Chown, S. L. (2014). Effects of temperature on heat-shock responses and survival of two species of marine invertebrates from sub-Antarctic Marion Island. Antarct. Sci. 26, 145–152. doi: 10.1017/S0954102013000473
Collins, M., Clark, M. S., Spicer, J. I., and Truebano, M. (2020). Transcriptional frontloading contributes to cross-tolerance between stressors. Evol. Appl. 14, 577–587. doi: 10.1111/eva.13142
Collins, M., Peck, L. S., and Clark, M. S. (2021). Large within, and between, species differences in marine cellular responses: unpredictability in a changing environment. Sci. Total Environ. 794:148594. doi: 10.1016/j.scitotenv.2021.148594
Collins, M., Tills, O., Turner, L. M., Clark, M. S., Spicer, J. I., and Truebano, M. (2019). Moderate reductions in dissolved oxygen may compromise performance in an ecologically important estuarine invertebrate. Sci. Total Environ. 693:133444.
Convey, P., and Peck, L. S. (2019). Antarctic environmental change and biological responses. Sci. Adv. 11:eaaz0888. doi: 10.1126/sciadv.aaz0888
Dan Dunn, J., Alvarez, L. A., Zhang, X., and Soldati, T. (2015). Reactive oxygen species and mitochondria: a nexus of cellular homeostasis. Redox Biol. 6, 472–485. doi: 10.1016/j.redox.2015.09.005
Doney, S. C., Ruckelshaus, M., Emmett Duffy, J., Barry, J. P., Chan, F., English, C. A., et al. (2012). Climate change impacts on marine ecosystems. Ann. Rev. Mar. Sci. 4, 11–37. doi: 10.1146/annurev-marine-041911-111611
Gao, Y., Wei, J., Yuan, J., Zhang, X., Li, F., and Xiang, J. (2017). Transcriptome analysis on the exoskeleton formation in early developmetal stages and reconstruction scenario in growth-moulting in Litopenaeus vannamei. Sci. Rep. 7:1098. doi: 10.1038/s41598-017-01220-6
González-Aravena, M., Kenny, N. J., Osorio, M., Font, A., Riesgo, A., and Cárdenas, C. A. (2019). Warm temperatures, cool sponges: the effect of increased temperatures on the Antarctic sponge Isodictya sp. PeerJ 7:e8088. doi: 10.7717/peerj.8088
Grabherr, M. G., Haas, B. J., Yassour, M., Levin, J. Z., Thompson, D. A., Amit, I., et al. (2011). Full-length transcriptome assembly from RNA-Seq data without a reference genome. Nat. Biotechnol. 29, 644–652. doi: 10.1038/nbt.1883
Griffiths, H. J., Meijers, A. J. S., and Bracegirdle, T. J. (2017). More losers than winners in a century of future Southern Ocean seafloor warming. Nat. Clim. Chang. 7, 749–754. doi: 10.1038/nclimate3377
Husmann, G., Abele, D., Rosenstiel, P., Clark, M. S., Kraemer, L., and Philipp, E. E. R. (2014). Age-dependent expression of stress and antimicrobial genes in the hemocytes and siphon tissue of the Antarctic bivalve, Laternula elliptica, exposed to injury and starvation. Cell Stress Chaperones 19, 15–32.
Janecki, T., and Rakusa-Suszczewski, S. (2006). Biology and metabolism of Glyptonotus antarcticus (Eights) (Crustacea: Isopoda) from Admiralty Bay, King George Island, Antarctica. Polar Biosci. 19, 29–42.
Janssen, H. H., and Hoese, B. (1993). Marsupium morphology and brooding biology of the Antarctic giant isopod, Glyptonotus antarcticus Eights 1853 (Crustacea, Isopoda, Chaetiliidae). Polar Biol. 13, 145–149.
Johnson, K. M., and Hofmann, G. E. (2020). Combined stress of ocean acidification and warming influence survival and drives differential gene expression patterns in the Antarctic pteropod, Limacina helicina antarctica. Conserv. Physiol. 8:coaa013. doi: 10.1093/conphys/coaa013
Kim, B. M., Kim, K., Choi, I. Y., and Rhee, J. S. (2017). Transcriptome response of the Pacific oyster, Crassostrea gigas susceptible to thermal stress: a comparison with the response of tolerant oyster. Mol. Cell. Toxicol. 13, 105–113. doi: 10.1007/s13273-017-0011-z
Kuballa, A. V., Holton, T. A., Paterson, B., and Elizur, A. (2011). Moult cycle specific differential gene expression profiling of the crab Portunus pelagicus. BMC Genomics 12:147. doi: 10.1186/1471-2164-12-147
Langmead, B., Trapnell, C., Pop, M., and Salzberg, S. L. (2009). Ultrafast and memory-efficient alignment of short DNA sequences to the human genome. Genome Biol. 10:R25. doi: 10.1186/gb-2009-10-3-r25
Logan, M. L., and Cox, C. L. (2020). Genetic constraints, transcriptome plasticity, and the evolutionary response to climate change. Front. Genet. 11:538226. doi: 10.3389/fgene.2020.538226
Lou, F., Han, Z., and Gao, T. (2019). Transcriptomic responses of two ecologically divergent populations of japanese mantis shrimp (Oratosquilla oratoria) under thermal stress. Animals 9:399. doi: 10.3390/ani9070399
Meredith, M. P., Sommerkorn, M., Cassotta, S., Derksen, C., Ekaykin, A., Hollowed, A., et al. (2019). “Polar regions,” in Intergovernmental Panel on Climate Change (IPCC) Special Report on the Ocean and Cryosphere in a Changing Climate, eds R. Hock, J.-P. Gattuso, and N. Abram (Geneva: IPCC).
Moffat, C., and Meredith, M. (2018). Shelf-ocean exchange and hydrography west of the Antarctic Peninsula: a review. Philos. Trans. R. Soc. A Math. Phys. Eng. Sci. 376:20170164. doi: 10.1098/rsta.2017.0164
Morley, S. A., Abele, D., Barnes, D. K. A., Cárdenas, C. A., Cotté, C., Gutt, J., et al. (2020). Global drivers on Southern Ocean ecosystems: changing physical environments and anthropogenic pressures in an earth system. Front. Mar. Sci. 7:547188. doi: 10.3389/fmars.2020.547188
Morley, S. A., Berman, J., Barnes, D. K. A., Carbonell, C., de, J., Downey, R. V., et al. (2016). Extreme phenotypic plasticity in metabolic physiology of Antarctic demosponges. Front. Ecol. Evol. 3:157. doi: 10.3389/fevo.2015.00157
Morley, S. A., Hirse, T., Thorne, M. A. S., Pörtner, H. O., and Peck, L. S. (2012). Physiological plasticity, long term resistance or acclimation to temperature, in the Antarctic bivalve, Laternula elliptica. Comp. Biochem. Physiol. A Mol. Integr. Physiol. 162, 16–21. doi: 10.1016/j.cbpa.2012.01.009
Parmesan, C. (2006). Ecological and evolutionary responses to recent climate change. Annu. Rev. Ecol. Evol. Syst. 37, 637–669. doi: 10.1146/annurev.ecolsys.37.091305.110100
Patro, R., Duggal, G., Love, M. I., Irizarry, R. A., and Kingsford, C. (2017). Salmon provides fast and bias-aware quantification of transcript expression. Nat. Methods 14, 417–419. doi: 10.1038/nmeth.4197
Peck, L. S. (2011). Organisms and responses to environmental change. Mar. Genomics 4, 237–243. doi: 10.1016/j.margen.2011.07.001
Peck, L. S. (2018). Antarctic marine biodiversity: adaptations, environments and responses to change. Oceanogr. Mar. Biol. Annu. Rev. 56, 2–133.
Peck, L. S., Souster, T., and Clark, M. S. (2013). Juveniles are more resistant to warming than adults in 4 species of antarctic marine invertebrates. PLoS One 8:e66033. doi: 10.1371/journal.pone.0066033
Peck, L. S., Webb, K. E., and Bailey, D. M. (2004). Extreme sensitivity of biological function to temperature in Antarctic marine species. Func. Ecol. 18, 625–630.
Pfennig, D. W., Wund, M. A., Snell-Rood, E. C., Cruickshank, T., Schlichting, C. D., and Moczek, A. P. (2010). Phenotypic plasticity’s impacts on diversification and speciation. Trends Ecol. Evol. 25, 459–467. doi: 10.1016/j.tree.2010.05.006
Place, S. P., and Hofmann, G. E. (2005). Constitutive expression of a stress-inducible heat shock protein gene, hsp70, in a phylogenetically distant Antarctic fish. Polar Biol. 28, 261–267.
Place, S. P., Zippay, M. L., and Hofmann, G. E. (2004). Constitutive roles for inducible genes: evidence for the alteration in expression of the inducible hsp70 gene in Antarctic notothenioid fishes. Am. J. Physiol. Regul. Integr. Comp. Physiol. 287, 429–436.
Pörtner, H. O., and Knust, R. (2007). Climate change affects marine fishes through the oxygen limitation of thermal tolerance. Science 315, 95–97. doi: 10.1126/science.1135471
Pörtner, H. O., Peck, L., and Somero, G. (2007). Thermal limits and adaptation in marine Antarctic ectotherms: an integrative view. Philos. Trans. R. Soc. B Biol. Sci. 362, 2233–2258. doi: 10.1098/rstb.2006.1947
Rinehart, J. P., Hayward, S. A., Elnitsky, M. A., Sandro, L. H., Lee, R. E., and Denlinger, D. L. (2006). Continuous upregulation of shock proteins in larvae, but not adults, of a polar insect. Proc. Natl. Acad. Sci. U.S.A. 103, 14223–14227.
Robinson, M. D., McCarthy, D. J., and Smyth, G. K. (2009). edgeR: a bioconductor package for differential expression analysis of digital gene expression data. Bioinformatics 26, 139–140. doi: 10.1093/bioinformatics/btp616
Seear, P. J., Tarling, G. A., Burns, G., Goodall-Copestake, W. P., Gaten, E., Ozkaya, O., et al. (2010). Differential gene expression during the moult cycle of Antarctic krill (Euphausia superba). BMC Genomics 11:582. doi: 10.1186/1471-2164-11-582
Sleight, V. A., Peck, L. S., Dyrynda, E. A., Smith, V. J., and Clark, M. S. (2018). Cellular stress responses to chronic heat shock and shell damage in temperate Mya truncata. Cell Stress Chaperones 23, 1003–1017. doi: 10.1007/s12192-018-0910-5
Somero, G. (2015). Temporal patterning of thermal acclimation: from behavior to membrane biophysics. J. Exp. Biol. 218, 167–169. doi: 10.1242/jeb.109843
Somero, G. N. (2012). The physiology of global change: linking patterns to mechanisms. Ann. Rev. Mar. Sci. 4, 39–61. doi: 10.1146/annurev-marine-120710-100935
Stenni, B., Curran, M. A. J., Abram, N. J., Orsi, A., Goursaud, S., Masson-Delmotte, V., et al. (2017). Antarctic climate variability on regional and continental scales over the last 2000 years. Clim. Past 13, 1609–1634. doi: 10.5194/cp-13-1609-2017
Szklarczyk, D., Gable, A. L., Lyon, D., Junge, A., Wyder, S., Huerta-Cepas, J., et al. (2019). STRING v11: protein-protein association networks with increased coverage, supporting functional discovery in genome-wide experimental datasets. Nucleic Acids Res. 47, D607–D613. doi: 10.1093/nar/gky1131
Toullec, J.-Y., Corre, E., Bernay, B., Thorne, M. A. S., Cascella, K., Ollivaux, C., et al. (2013). Transcriptome and peptidome characterisation of the main neuropeptides and peptidic hormones of a Euphausiid: the ice krill, Euphausia crystallorophias. PLoS One 8:e71609. doi: 10.1371/journal.pone.0071609
Keywords: marine invertebrates, Antarctica, warming, transcriptomic (RNA-Seq), phenotypic plasticity
Citation: González-Aravena M, Rondon R, Font A, Cárdenas CA, Toullec J-Y, Corre E and Paschke K (2021) Low Transcriptomic Plasticity of Antarctic Giant Isopod Glyptonotus antarcticus Juveniles Exposed to Acute Thermal Stress. Front. Mar. Sci. 8:761866. doi: 10.3389/fmars.2021.761866
Received: 20 August 2021; Accepted: 17 November 2021;
Published: 09 December 2021.
Edited by:
Ming Li, Ningbo University, ChinaReviewed by:
Brad Buckley, Portland State University, United StatesHaibo Jiang, Guizhou University, China
Copyright © 2021 González-Aravena, Rondon, Font, Cárdenas, Toullec, Corre and Paschke. This is an open-access article distributed under the terms of the Creative Commons Attribution License (CC BY). The use, distribution or reproduction in other forums is permitted, provided the original author(s) and the copyright owner(s) are credited and that the original publication in this journal is cited, in accordance with accepted academic practice. No use, distribution or reproduction is permitted which does not comply with these terms.
*Correspondence: Marcelo González-Aravena, bWdvbnphbGV6QGluYWNoLmNs
†These authors have contributed equally to this work