- 1School of Biological Sciences, The University of Western Australia, Crawley, WA, Australia
- 2UWA Oceans Institute, The University of Western Australia, Crawley, WA, Australia
- 3Ooid Scientific, South Fremantle, WA, Australia
- 4Department of Biodiversity, Conservation and Attractions, WA Government, Kensington, WA, Australia
Seagrasses are globally recognized as bioindicators of marine eutrophication and contamination. Seagrasses also harbor a distinct root microbial community that largely reflects the conditions of the surrounding environment as well as the condition of the seagrass. Hence monitoring changes in the root microbial community could act as an additional biological indicator that reflects both the seagrass health condition, as well as potential deterioration in coastal waters. We used 16S rRNA gene sequencing combined with analysis of seagrass nutrients (C, N, δ15N, δ13C) and tissue metal concentrations to investigate potential links between seagrass (Halophila ovalis) root bacteria and seagrass nutrient and metal concentrations within an anthropogenically influenced estuary. We found seagrass tissue nitrogen (%) and δ15N values were 2–5 times higher than global averages for this species. Seagrass root associated bacteria formed distinct communities that clustered by site and were correlated to both seagrass nutrient and metal concentration, with some putative sulfide oxidizing bacteria (Sulfurimonas and Sulfurovum) correlated with greater nutrient concentrations, and putative iron cycling bacteria (Lewinella and Woeseia) correlated with greater Fe and As concentrations. Our findings shed further light on the relationship between seagrass and their microbes, as well as provide additional assessment of the use of both seagrass and their microbes as indicators of estuarine and seagrass condition.
Introduction
Estuaries, which are transitional zones between the land and the sea, trap sediments and pollutants from rivers and streams and reduce the extent to which these pollutants enter the ocean. However, poor catchment management can lead to increased sediment, nutrient and pollutant loads to estuaries which can cause a decline in the health of the estuary and increase pollution export to the ocean (Dafforn et al., 2012; Statham, 2012). Excess nutrients and heavy metals are both common and serious pollutants of estuaries (Jiang et al., 2001; Sutherland et al., 2017). Excess nutrients in estuaries can lead to cultural eutrophication and associated deoxygenation events, which is a leading cause of impairment of freshwater and coastal ecosystems globally (Statham, 2012). Heavy metals can accumulate in estuaries naturally through the weathering of rocks and soils and anthropogenically via urban and industrial run-off, sewage, and poor agricultural practices (Birch et al., 2018b). Heavy metals can be in dissolved or particulate form and often accrue in sediments (Burton and Johnston, 2010). Under certain physicochemical conditions (e.g., water hypoxia and anoxia), these contaminated sediments can become a source of continued pollution for estuarine ecosystems (Bennett et al., 2012).
Seagrasses are foundation species within many estuaries across the globe, providing habitat, and performing many essential ecological functions (Short et al., 2007; Orth et al., 2017). Seagrasses can uptake nutrients and heavy metals from the sediment via their roots or from the water column via their shoots. The canopies of seagrass meadows also enhance particle trapping which can further facilitate the accumulation of metals in seagrass sediments (De Boer, 2007). Seagrasses have been used as valuable indicators of estuarine condition and contamination because concentrations of metals and nutrients (and their associated isotope signatures) in seagrass tissue often reflect the concentrations of the surrounding environment (Govers et al., 2014; Kilminster et al., 2014; Bonanno and Orlando-Bonaca, 2017; Orth et al., 2017; Lee et al., 2019). However, whilst seagrasses are considered foundational species of marine ecosystems, microbes are the foundational units of Earth; responsible for balancing and maintaining global biogeochemical cycles. Because of this, microbes (predominantly bacteria) have been used to assess ecological conditions of various natural and man-made systems (Birrer et al., 2019; Filippini et al., 2019) and have recently been used to also assess the health of seagrass (Martin et al., 2020a). As microbes respond rapidly to changes in their environment, developing microbial indicators enhances our capacity to detect potential ecosystem deterioration before lethal impacts have occurred on macroorganisms.
The Swan-Canning estuary flows through Perth the capital city of Western Australia and has seen increased levels of nutrients (above natural levels) due to rapid population growth and intensive agriculture in the surrounding catchment. These excess nutrients have contributed to observed signs of eutrophication (e.g., algal blooms and deoxygenation events) (Robson and Hamilton, 2003). Sediments have also continued to accumulate in the estuary which are enriched in both nutrients and some heavy metals (Gerritse et al., 1998; Rate et al., 2000; Nice, 2009). Historical practice of using riverside wetland areas as rubbish tips, then infilling for parkland or development has also left areas of increased contamination in close proximity to, and leaching into, the estuarine environment (Brearly, 2006). One area of concern is the Alfred Cove Marine Park, which is in close proximity to historic waste disposal sites. Seagrasses in the Alfred Cove Marine Park have previously been found to be in poor condition, to have low C:N ratios as well as elevated δ15N compared to seagrass in other areas of the Swan-Canning estuary, indicating potentially elevated local inputs of nitrogen to the area (Kilminster and Forbes, 2014). A subsequent groundwater sampling program at Alfred Cove Marine Park confirmed that nitrogen and metal contamination (namely iron, copper, chromium and mercury) exists in this area and that there is potential for this to impact seagrass and the wider estuarine ecology (Vogwill and Oldmeadow, 2018). The aims of this study were to; (i) assess whether concentrations of nutrients (carbon and nitrogen), stable isotopes of carbon and nitrogen, and metals of seagrass tissue correlate with estuary and/or groundwater quality, and (ii) investigate links between seagrass root bacterial communities and seagrass nutrient and seagrass metal concentrations to assess the suitability of bacteria as an additional indicator of seagrass and estuary condition.
Materials and Methods
Site Description
The study sites were located within the Swan-Canning estuary which flows through Perth, Western Australia (31.95°S, 115.86°E) and drains a catchment area of approximately 2,090 km2. The Swan-Canning estuary has a small tidal range and tributaries generally only flow during winter and spring. Halophila ovalis is the dominant seagrass growing in the Swan-Canning estuary. The main study area of Alfred Cove Marine Park is one of three marine parks within the Swan-Canning estuary and covers an area ∼ 2 square km (Figure 1 and Supplementary Table 1). The marine park encompasses mudflats, seagrass meadows and intertidal vegetation, which provides habitats for a variety of animals including internationally important migratory wading birds.
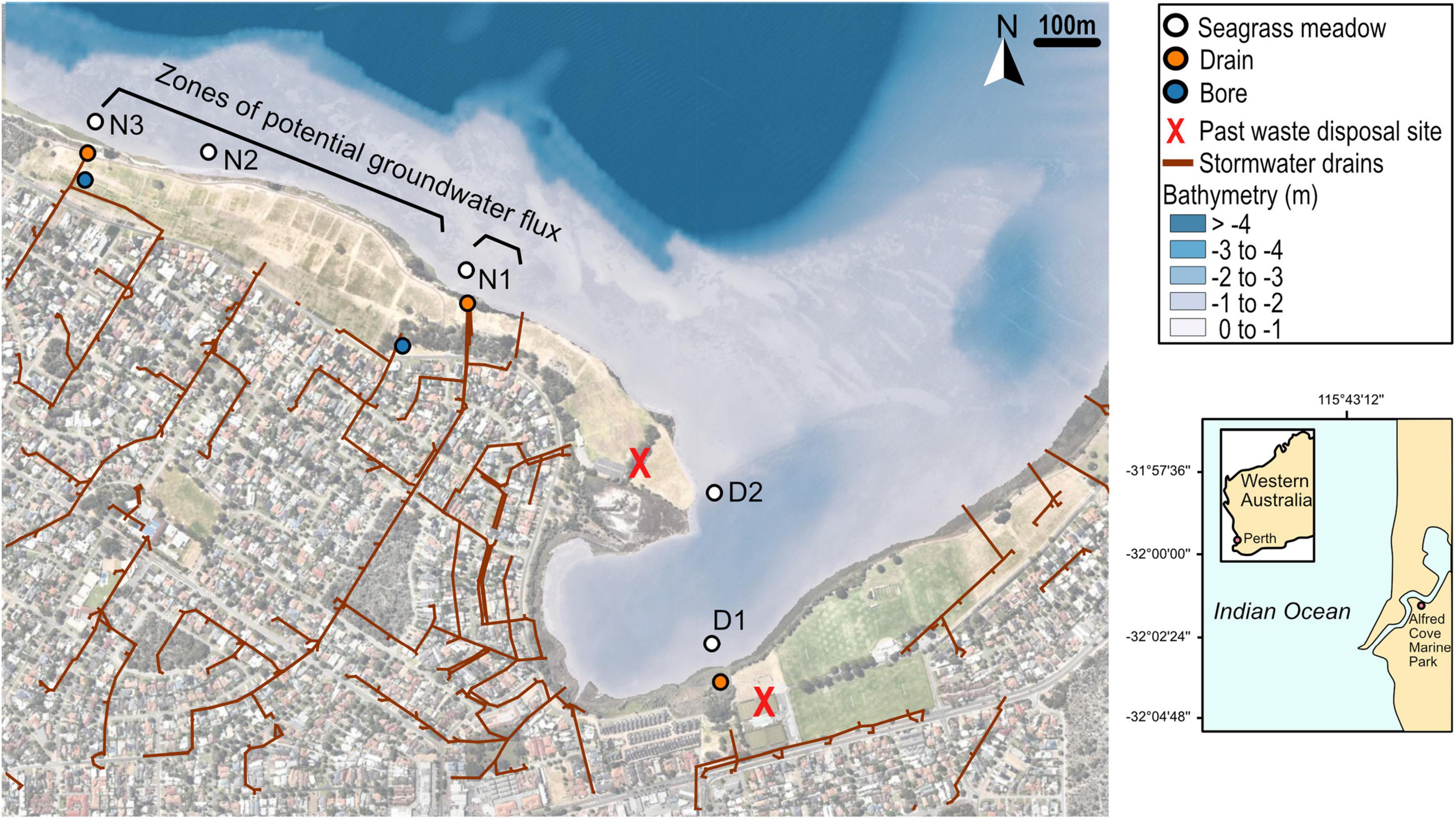
Figure 1. Site names and locations along the Alfred Cove Marine Park with stormwater drainage channels, bathymetry and aerial imagery overlain, where D1 and D2 are closest to old waste disposal sites and N1, N2, and N3 are in the line of the modeled zone of groundwater flux potential. Zones of flux potential of groundwater throughflow have been adapted from Cahill et al. (2017), which were estimated from conductivity-depth profiles. Two historical domestic and building waste disposal sites which were operating in the 1950’s are marked with red crosses. Underlying map imagery © 2022 Google, Imagery © 2022 CNES/ Airbus, Maxar Technologies.
The low topography of the area makes it subject to groundwater flow and stormwater runoff and there are several suburban drains which enter the area (Figure 1). There are two known historic waste disposal sites in the area (Figure 1, marked with red crosses) that were used for domestic and building wastes between 1952 and 1964. During this same decade (1950–1960), the foreshore was cleared of riparian vegetation, the riverbed was dredged with the spoil used to fill in the foreshore.
Sample Collection
A total of 5 seagrass sites, three stormwater drains and two groundwater bores were sampled along the foreshore in January 2019 (Austral summer). All seagrass sites were in water depths between 0.5 and 1.5 m (Figure 1). Temperature, salinity, dissolved oxygen and electrical conductivity of each of the sites (except site 4) were recorded in situ at the time of collection using a YSI Pro and YSI ProODO. Groundwater was accessed via pumping valves on bores that were purged for 5 min before retrieving a water sample. Water samples were also collected from each site and immediately filtered either through a 0.45 μm Polyethersulphone (PES) syringe filter (for δ15N-NO3 and δ18O-NO3 analysis) or PES syringe 0.2 μm (for dissolved nutrient analysis). Sampling syringes and containers were rinsed twice with deionized water and with new sample water prior to sampling. All collected water samples were cooled immediately on ice, stored at 4°C, and were analyzed within 2 weeks of collection (see below).
Four replicate seagrass tissue and sediment samples were collected at each of the seagrass sites using cores (96 mm diameter, 15 cm deep). Sediment was sieved (2 mm) in the field before cooling immediately on ice. Upon returning to the laboratory, the sediment samples were directly frozen, whilst the seagrass tissue was first rinsed in DI water, epiphytes were gently removed and then the tissue frozen. Seagrass biomass (root, rhizome and shoot) was estimated from drying (60°C) and weighing Halophila ovalis collected from cores. This dry biomass was also used for δ15N, δ13C, nutrient, and metal analysis (see below).
An additional four replicate apical shoots of H. ovalis (with roots attached) were removed from the meadows using the same cores. The two youngest roots (the first and second root) were gently washed from the sediment in situ (i.e., some sediment grains were still attached to the root hairs), cut from the rhizome using sterile scissors and gloves, placed into Whirl-paks® (Nasco, Wisconsin) then immediately into liquid nitrogen. Upon returning to the laboratory, these samples were transferred to a –80°C freezer until DNA extraction.
δ15N, δ13C, Nutrient, and Metal Analysis
Dissolved nitrate, ammonium and soluble reactive phosphorus (SRP) were analyzed from filtered (0.2 μm) water samples. Nitrate and ammonium were measured with a colorimetric detection method on a Bran-Luebbe Autoanalyzer 3 (Bran and Luebbe, Norderstedt, Germany) and digital Colorimeter (Technicon, Tarrytown, New York). SRP was determined colorimetrically using the ascorbic acid–molybdate technique (Murphy and Riley, 1962). Dissolved nitrate in water samples was also analyzed for δ15N (‰, Air) and δ18O (‰, VSMOW-SLAP) using a modification of the denitrifier method (Sigman et al., 2001; Casciotti et al., 2002) on a continuous flow stable isotope ratio mass spectrometer (Delta V Plus, Thermo-Finnigan, Germany), and the results reported after normalization vs. international standards (δ15N—N2, IAEA-NO-3, USGS32, USGS34, USGS35). The combined uncertainty of analyses (1 std dev) for δ15N and δ18O was 0.25‰.
The first and second shoot, rhizome and root from the apex of H. ovalis (youngest tissue), as well as sediment were analyzed for δ15N, δ13C,% C, and % N. Frozen seagrass tissue and sediment were dried (60°C) and ground into a fine powder using zirconia balls and a SPEX SamplePrep 2010 Geno/Grinder. Additionally, the sediments were treated with 4% HCl to remove carbonates. Ground samples were then analyzed using a continuous flow system consisting of a Delta V Plus mass spectrometer connected with a Thermo Flush 1112 via Conflo IV (Thermo-Finnigan, Germany). All values are given in per mil; δ13C (‰, VPDB) and δ15N (‰, Air) according to delta notation (Coplen et al., 2006). Multi-point normalization and international reference materials (δ13C: NBS22, USGS24, NBS19, IAEA600, USGS40; δ15N—N1, N2, USGS32, IAEA600, USGS40) were used to reduce raw values to the international scales (Skrzypek, 2013). The combined analytical uncertainty (1 std dev) for δ13C was 0.10‰ and for δ15N was 0.10‰.
The first and second shoot, rhizome and root from the apex of H. ovalis (youngest tissue) were also analyzed for Al, As, Cd, Cu, Fe, Pb, and Zn. Dried and ground tissue was digested with HNO3 and HClO4 (0.1 g 10 mL–1) according to Simmons (1975). Analysis of digests was performed using a Perkin Elmer Nexion 1000 (Shelton, Connecticut United States) Inductively Coupled Plasma—Mass Spectrometer. Detection limits (mg kg–1) for metals were as follows; Al (10), As (0.10), Cd (0.02), Cu (1.0), Fe (1.0), Pb (0.1), and Zn (0.5). These metal concentrations were also compared with metal concentrations analyzed from H. ovalis tissue and sediments in site N1 in June 2019 (austral winter) using the same method and instrument.
DNA Extraction, Sequencing, and Bioinformatics
DNA was extracted from ∼0.1 g of apical roots using the DNeasy PowerSoil DNA kit (QIAGEN) according to the manufacturer’s instructions. A FastPrep-24 bead beater (MP Biomedicals) set at 4.5 ms–1 for 60 s was used to lyse microbial cells prior to DNA extraction. Extracted DNA was then eluted in 50 μL nuclease-free water. Microbial communities were profiled using the primers 341F–806R that target the V3-V4 hypervariable region of the 16S rRNA gene. These primers predominantly amplify bacterial taxa but also amplify some members of archaea and have lower off-target amplification of plant chloroplasts (Muyzer et al., 1993; Muhling et al., 2008; Caporaso et al., 2011). Sequencing of pooled amplicons was performed by the Australian Genome Research Facility (AGRF) on the MiSeq platform, using Nextera XT v2 indices and 300 bp paired end sequencing chemistry. Primers were trimmed using cutadapt (version 1.16) (Martin, 2011) and trimmed reads were processed in DADA2 (version 1.9)1 (Callahan et al., 2016). Taxonomy was assigned following the DADA2 pipeline with the Ribosomal Database Project (RDP) classifier and the SILVA SSU Ref NR 132 database (Quast et al., 2013). Amplicon sequence variants (ASVs) identified in less than 1% of the reads were removed (filter defined by investigating prevalence plots) as well as ASVs identified as chloroplast or mitochondria. Archaea were not filtered out.
PICRUSt2 (Douglas et al., 2020) accompanied by EPA-ng (Barbera et al., 2019), gappa (Czech et al., 2020), castor (Louca and Doebeli, 2018), and MinPath (Ye and Doak, 2009) were used to assign filtered sequencing reads to functional orthologs (KEGG orthologs; KOs) and infer functional pathways. The recommended maximum NSTI cut-off of 2 was implemented in PICRUSt2 to prevent unconsidered interpretation of overly speculative inferences. The NSTI values in our dataset ranged from 0.0001 to 1.3343, indicating a well characterized microbial community in relation to isolate genomes. All pathways were subsequently classified to broader functional categories (i.e., higher pathways) using a custom mapping file created using the MetaCyc database (Caspi et al., 2016). General sequencing statistics can be found in Supplementary Table 2. All raw sequences and meta-data have been uploaded to NCBI Sequence Read Archive (SRA) under BioProject ID: PRJNA663104.
Data Analysis
Differences in seagrass biomass, seagrass and sediment δ15N, δ13C, C (%), and N (%) among sites were tested with one-way ANOVA’s. Normality and homogeneity of variances were checked, and data were log transformed where required. Tukey’s HSD was used for multi-comparisons if a significant difference among sites was found. A constrained ordination (Canonical Analysis of Principal Coordinates; CAP) using Bray-Curtis dissimilatory measure on non-rarified ASVs was used to explore the association between root bacterial communities and seagrass root parameters (∼root biomass + root metal concentrations + root nutrients) among sites. A CAP model using Bray-Curtis dissimilatory measures was also built to explore the association between predicted bacterial functions and seagrass root parameters among sites. The significance of the CAP models were assessed using permutation tests with 999 permutations. Correlation analysis between the top 40 most abundant ASVs and predicted functions (agglomerated to Genus and level 2 KEGG functional categories, respectively) and root variables was performed using Pearson’s correlation coefficient with p-values adjusted for multiple testing (Bonferroni correction).
All statistical analysis was carried out in R (version 4.0.2) (R Development Core Team, 2011) using the Phyloseq (version 1.24.2) (McMurdie and Holmes, 2013), ggplot2 (version 3.3.2) (Wickham, 2009), and vegan packages (version 2.5–6) (Oksanen et al., 2016).
Results
Water Quality
Dissolved oxygen (DO) concentration of seagrass beds ranged from 7.9 mg L–1 (99% air saturation) to 15.5 mg L–1 (202% air saturation), whilst DO in stormwater drains flowing into seagrass meadows ranged from 0.1 mg L–1 (1% air saturation) to 2.8 mg L–1 (32% air saturation). The stormwater drains tended to have high concentrations of soluble reactive phosphorus (SRP) and N-NH4 compared to the seagrass meadows in the estuary, with some of these nutrient values above the Australian and New Zealand guidelines for fresh and marine water quality (ANZECC and ARMCANZ; Table 1). The groundwater also had levels of SRP and dissolved inorganic nitrogen that exceeded guidelines (Table 1), with the bore sampled closest to site N1 having relatively high levels of N-NH4 (2.03 mg L–1) and the bore sampled closest to site N3 having high levels of N-NO3 (0.20 mg L–1). The δ15N-NO3 values (where N-NO3 was above the detection limit) ranged from 5.8 to 11.0‰, with the highest value measured in the stormwater drain at S5. The δ18O-NO3 values ranged from –2.9 to 3.7‰, with the highest value measured in the stormwater drain at S5.
Seagrass Biomass and Nutrients
Belowground biomass of Halophila ovalis recovered from cores (live roots and rhizomes) was significantly lower at site D1 than sites N2 and N3 (with a general increasing trend from D1 to N3), but aboveground biomass was not significantly different (Figure 2).
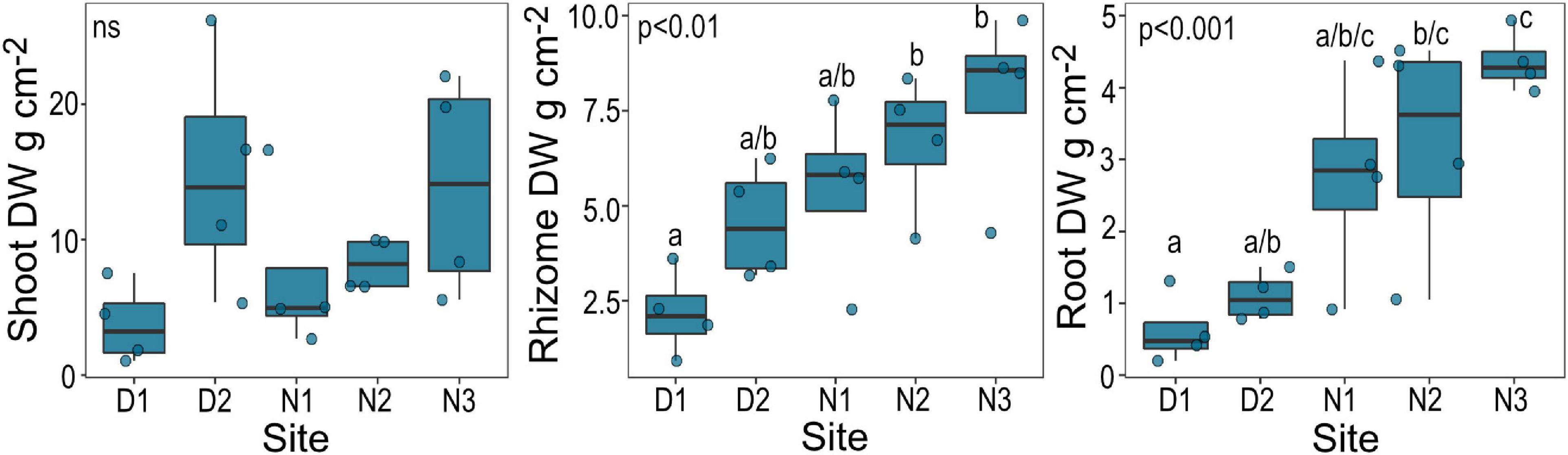
Figure 2. Biomass (DW, dry weight) of H. ovalis by site. Data are boxplots with datapoints overlain. Significant post hoc comparisons between individual sites are indicated by lowercase letters. Ns, no significant difference among sites.
The sediment at site D2 had significantly higher nitrogen ( = 0.1% DW) and carbon content ( = 0.9% DW) compared to all other sites (Figure 3). Nitrogen% dry weight (DW) was also greatest in seagrass shoots ( = 3.0% DW) and roots ( = 1.4% DW) growing at site N2 compared to other sites, with exception to shoot nitrogen at N2 which had an average high mean shoot N of 3.2% DW (Figure 3). δ13C of seagrass tissue (shoots, rhizomes and roots) was significantly higher at site D2 compared to all other sites, while δ15N was significantly lower (Figure 3). The seagrass tissue (shoots, rhizomes and roots) at sites N1 to N3 were all higher in δ15N compared to other sites; with a maximum value across these sites of 8.9‰ measured from shoot tissue at site N3 (Figure 3).
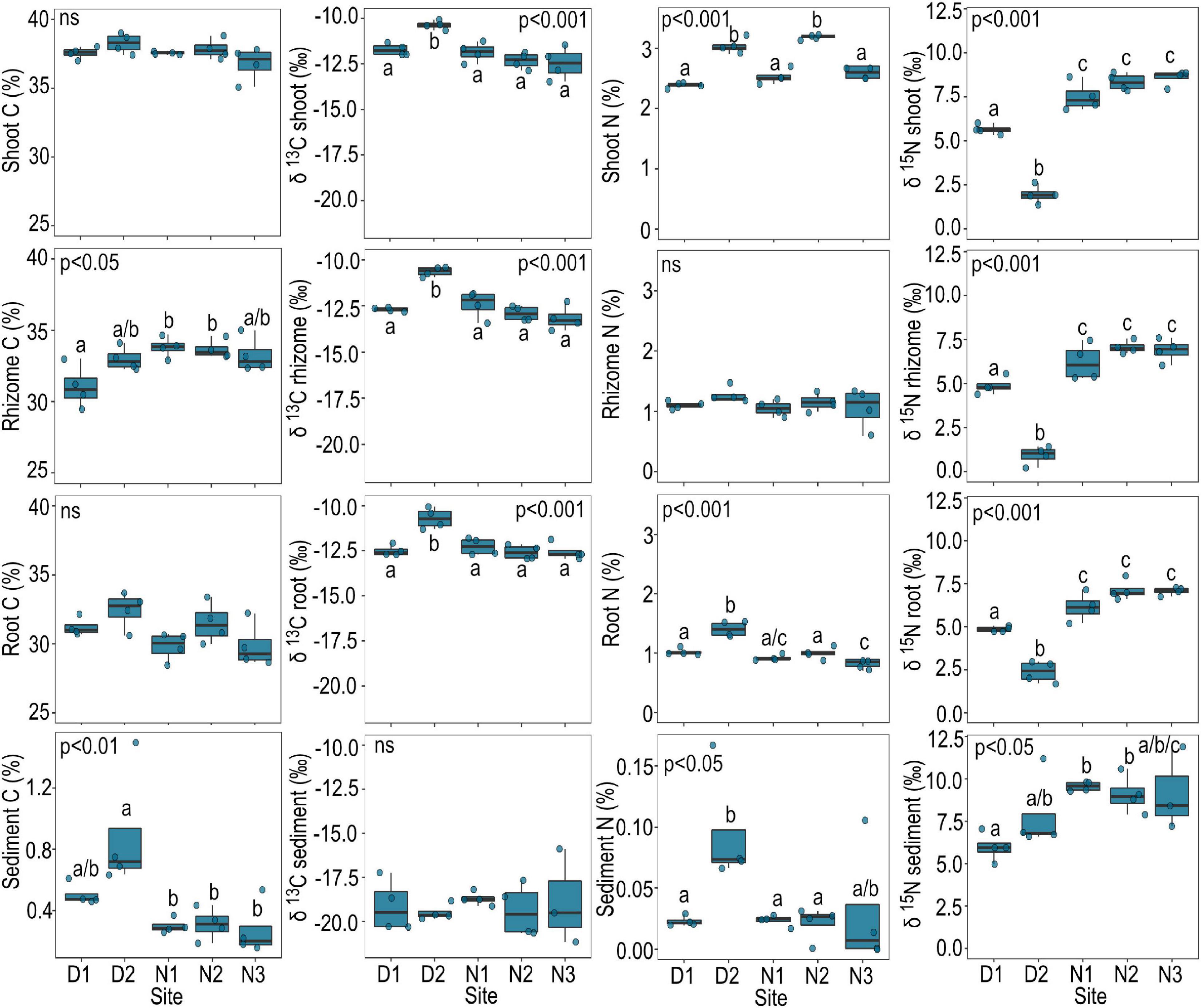
Figure 3. Carbon (%), δ13C, nitrogen (%), and δ15N of seagrass tissue and sediment by site. Data are boxplots with datapoints overlain. Significant post hoc comparisons are indicated by lowercase letters. Ns, no significant difference among sites.
Seagrass Heavy Metals
Seagrass tissue metal concentrations differed according to the metal analyzed, the tissue type, and the site (Figure 4). With exception of zinc and cadmium, metals were higher in the belowground tissue than aboveground (Figure 4). Several metal concentrations in seagrass roots were highly positively correlated, including arsenic and iron (p < 0.001, r = 0.98) and copper and lead (p < 0.001, r = 0.71).
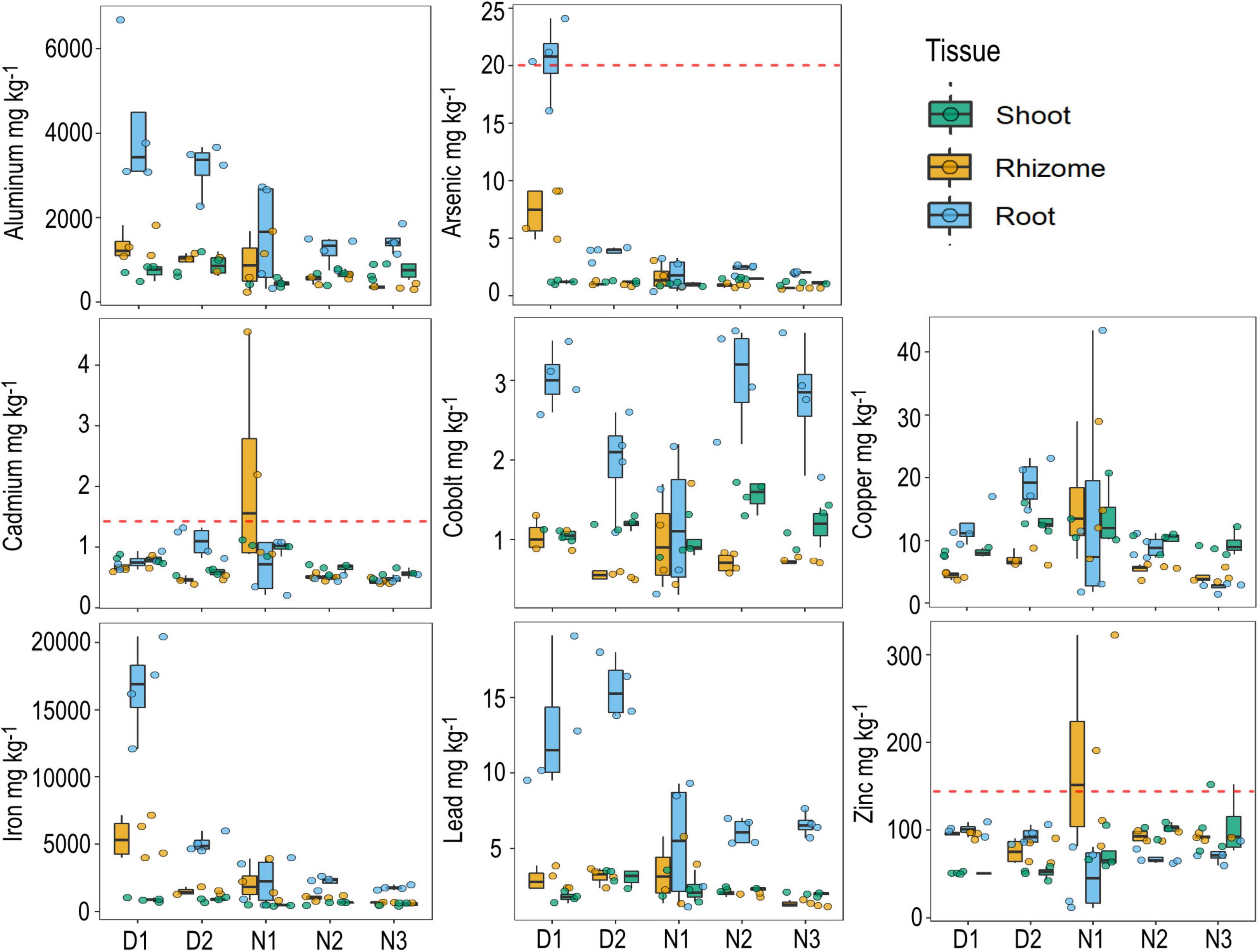
Figure 4. Metal concentrations in seagrass tissue (shoot, rhizome, and roots) by site. Data are boxplots with datapoints overlain. The dashed red line indicates the default value guideline (the concentrations below which there is a low risk of unacceptable effects occurring) for toxicants in sediment according to the Australian and New Zealand Guidelines for Fresh and marine water quality.
Site D1 had some of the highest metal tissue concentrations of all the sites, including for arsenic (As in roots = 20.5 mg kg–1), aluminum (Al in roots = 4,162 mg kg–1), and iron (Fe in roots = 16, 585 mg kg–1). Site N1 had the highest tissue concentrations of copper, cadmium and zinc, though these values were highly variable (Figure 4). There are currently no environmental guidelines for heavy metals of aquatic plants in Australia, but some of the heavy metal concentrations measured from H. ovalis tissue exceeded the sediment toxicant guidelines in Australia and New Zealand, including arsenic, cadmium and zinc (Figure 4). The tissue concentrations of cobolt and zinc when averaged across all sites within the marine park were within the range of those recorded from H. ovalis within the highly urbanized Sydney estuary, but were lower for lead, copper and arsenic (Figure 5). Regression analysis between root concentrations of heavy metals and sediment heavy metals collected in winter from the current study area showed a significant positive relationship for arsenic [(As) roots = –8.12 + 26.39*(As) Sediment; p < 0.01, R2 = 0.87] and a significant negative relationship for lead [(Pb) roots = 20.26 –4.27 *(Pb) Sediment; p < 0.01, R2 = 0.84]. Several metal concentrations in seagrass tissues were negatively correlated with total seagrass biomass including arsenic (p < 0.01, r = –0.62), iron (p < 0.01, r = –0.62) and aluminum (p < 0.05, r = –0.50).
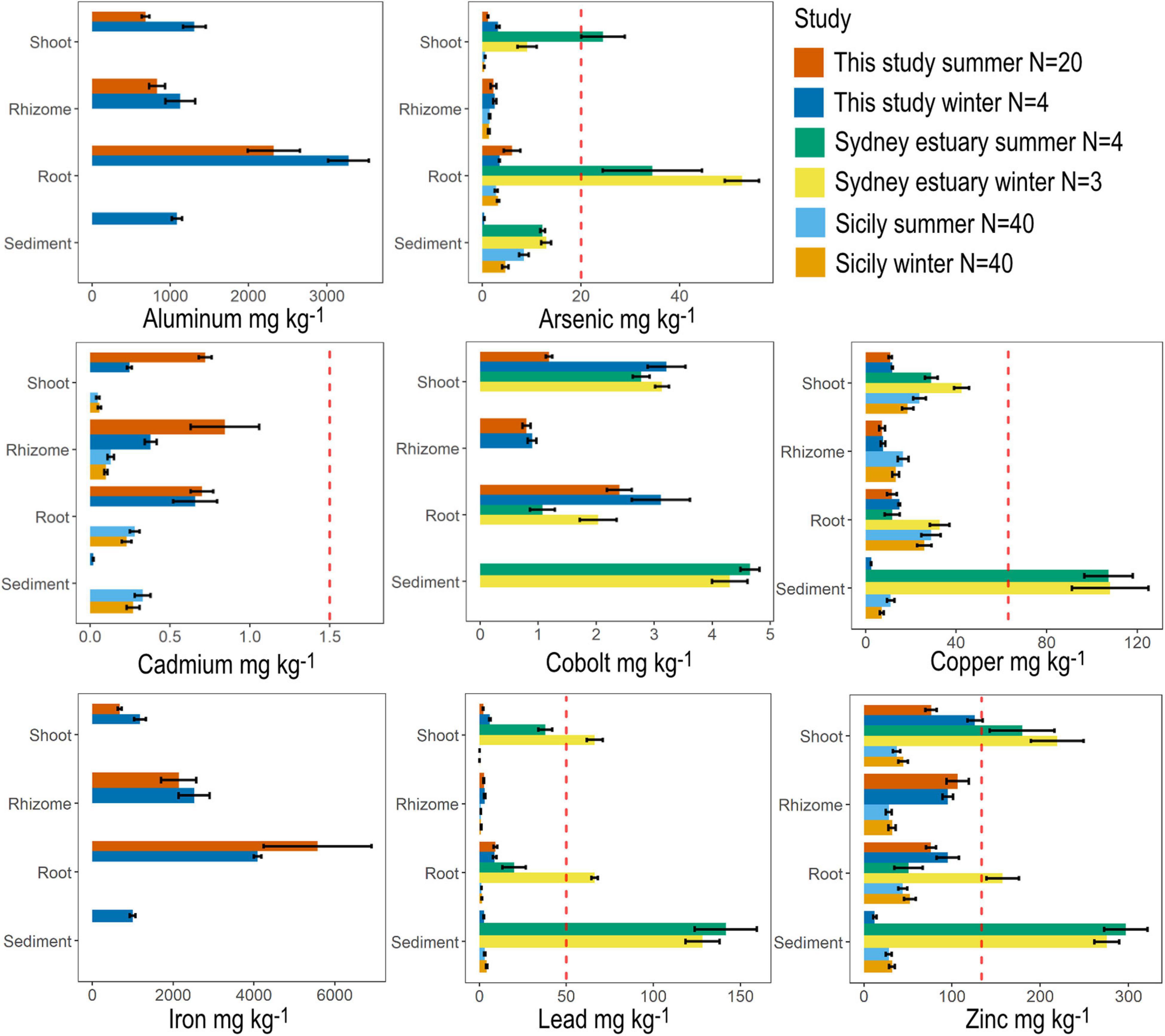
Figure 5. Metal concentrations in seagrass tissue (shoot, rhizome and roots) across all sites compared with mean values recorded from the literature for Halophila species. Seagrass tissue at site N1 was also analyzed for metals during June (winter). The dashed red line indicates the default value guideline (the concentrations below which there is a low risk of unacceptable effects occurring) for toxicants in sediment according to the Australian and New Zealand Guidelines for Fresh and marine water. Values for the Sydney estuary were taken from Birch et al. (2018a) and values from Sicily were taken from Bonanno and Raccuia (2018).
Seagrass Root Bacterial Communities
A total of 3128 ASVs were recovered from H. ovalis roots. These root bacterial communities were grouped distinctly by site, and this grouping was associated with differences in root nutrients, biomass and metal concentrations (permutation test of constrained analysis p < 0.001; Figure 6A). Root bacterial communities at Site D2 were associated with higher levels of arsenic and iron, whilst bacterial communities at site N2 and N3 were associated with higher root biomass (Figure 6A). Bacterial communities at site N3 were associated with higher δ15N root tissue values, whilst those at site D2 were mostly associated with higher N%, δ13C, and C% (Figure 6A).
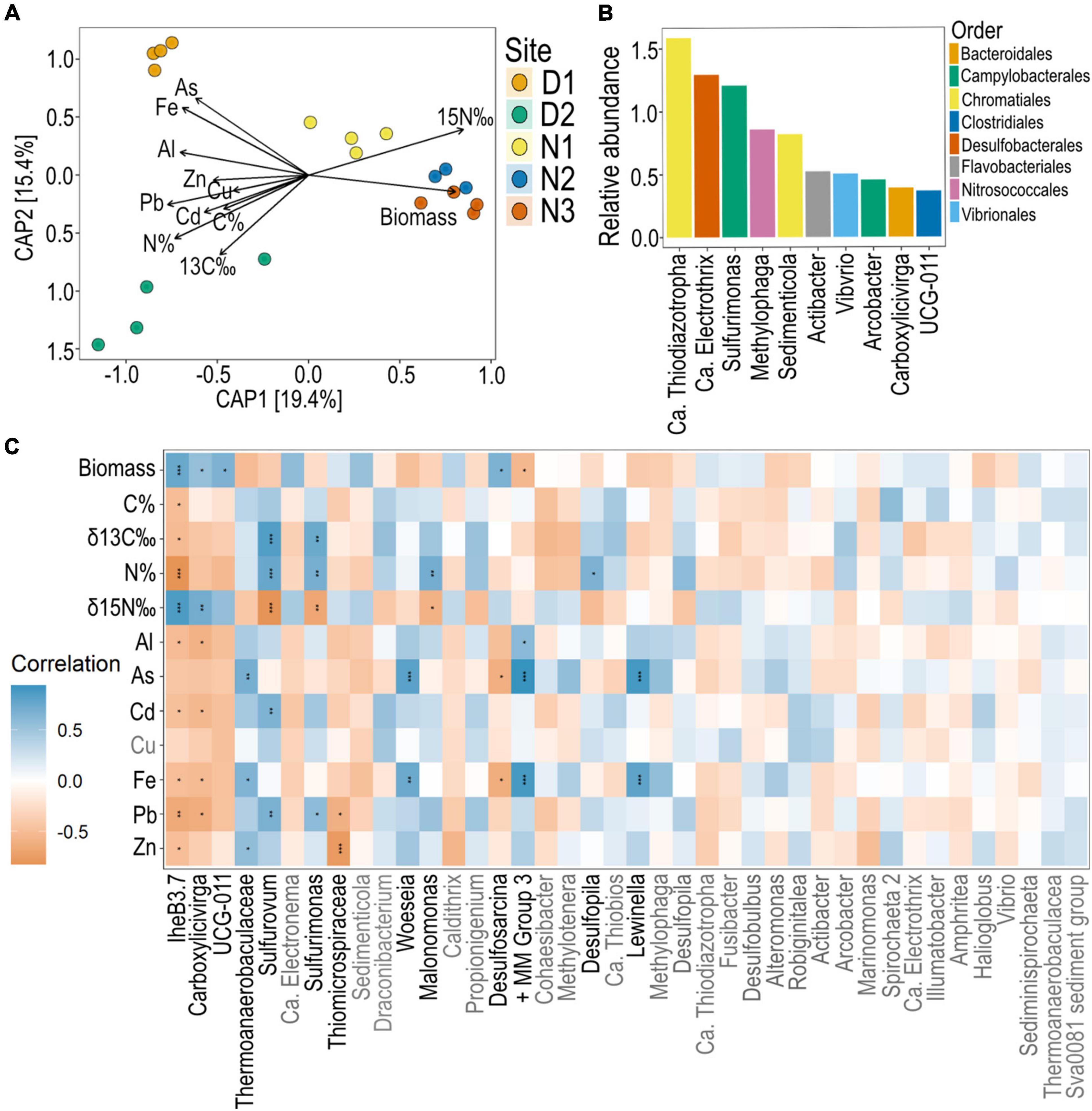
Figure 6. Seagrass root bacteria across sites and their correlation with seagrass nutrient and metal concentrations. (A) CAP of Bray-Curtis dissimilatory measures of ASVs constrained by root properties (root biomass, root nutrients and root metal concentrations). (B) Top 10 most abundant ASVs across all sites of seagrass roots (agglomerated to genus). (C) Pearson’s correlation analysis between the top 40 most abundant ASVs (agglomerated to genus) and seagrass root parameters with significant Bonferroni corrected p-values indicated with a *, where * = 0.05, ** < 0.01, *** < 0.001. All metal concentrations are mg kg–1. + MM Group 3, Marine methylotrophic group 3.
Agglomerating ASVs to the genus level reduced the number of ASVs to 462. Within this dataset, the top 40 ASVs comprised 71.4% of the community. Of these, the top three ASVs with the highest relative abundance in the H. ovalis root microbiome across all sites were related to putative sulfide oxidizing nitrogen fixers [Ca. Thiodiazotropha (Sedimenticolaceae), Ca. Electrothrix (Desulfobulbaceae), and Sulfurimonas (Sulfurimonadaceae); Figure 6B]. Of these top genera, Sulfurimonas was correlated to seagrass N%, δ13C and lead, and negatively correlated to δ15N (Figure 6C). This pattern was also mirrored by related putative sulfide oxidizers of the genus Sulfurovum (Sulfurovaceae). Several of the top 40 most abundant ASVs were positively correlated with As and Fe concentrations in seagrass roots including, Lewinella (Saprospiraceae), Marine methylotrophic group 3 (Methylophagaceae), Woeseia (Woeseiaceae), and subgroup 10 of Thermoanaerobaculaceae.
The predicted functions of the root bacterial communities also grouped by site, although less distinctly than the grouping observed for ASVs (permutation test of constrained analysis p < 0.05; Figure 7A). Predicted bacterial functions from site D2 were particularly variable (Figure 7A). The most common predicted bacterial functions of H. ovalis root microbiome across sties were related to biosynthesis, including amino acid biosynthesis and fatty acid biosynthesis (Figure 7B). Functions related to respiration were also common, as were functions related to the utilization of C1 compounds (Figure 7B). Predicted functions relating to bacterial respiration and carbohydrate biosynthesis were positively correlated to root N% and δ13C (Figure 7C). Microbial functions related to fatty acid and lipid biosynthesis were negatively correlated to root biomass, but were positively correlated with root concentrations of Al, As and Fe (Figure 7C).
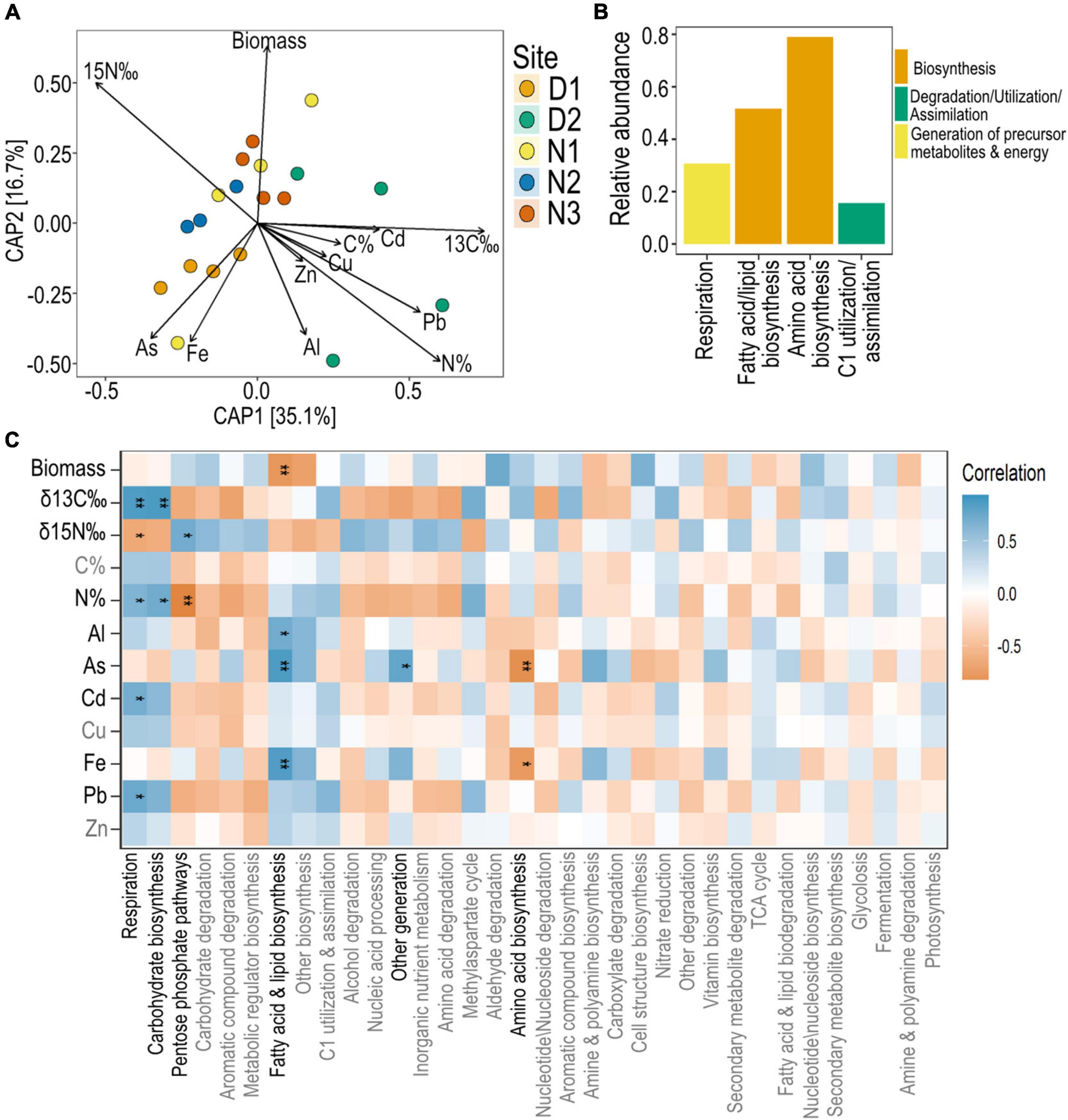
Figure 7. Predicted bacterial functions of seagrass root microbiomes and their correlation with nutrient and metal concentrations. (A) Constrained Analysis of Principal Coordinates (CAP) model of PICRUSt2 predicted functions constrained by root properties (root biomass, root nutrients and root metal concentrations). (B) Top 10 most abundant predicted functions across all sites of seagrass roots at level 2. (C) Pearson’s correlation analysis between the top 40 most abundant PICRUSt2 predicted functions (agglomerated to level 2 functions) and seagrass root parameters with significant Bonferroni corrected p-values indicated with a *, where * = 0.05, ** < 0.01.
Discussion
Biological monitoring tools (either individual organisms or communities) are increasingly used in integrated assessments of contaminant exposure and for assessing environmental degradation in ecosystems. Here we show that seagrass nutrient and metal status is reflective of an anthropogenically influenced estuary (elevated nutrients and metals). Seagrass root associated bacteria also correlated with the nutrient and metal status of seagrass, thus providing an additional potential monitoring tool for assessing both seagrass and estuarine health.
Seagrass Nutrients as Indicators for Dissolved Inorganic Nitrogen Sources
The δ15N and N% values of H. ovalis leaves in this study are among the highest recorded for this species globally, but are in line with previous reports of H. ovalis from the Swan-Canning estuary (Hillman et al., 1995). Leaf N% of H. ovalis has been previously found to range from 0.75 to 2.68% dry weight (DW) (Birch, 1975; Terrados et al., 1999; Yamamuro et al., 2001, 2003; Mellors et al., 2005), with nutrient limitation estimated to occur with leaf N < 1.8% DW (Duarte, 1990). All N% leaf values in the current study were > 2% DW, with some as high as 3.2% DW, which aligns with the nutrient enriched status of the Alfred Cove Marine Park, and more generally of the Swan-Canning estuary (Hillman et al., 1995; Gerritse et al., 1998).
δ15N values of H. ovalis leaves have been reported to range from –1.4 to 3.2‰, with a global mean of ∼ 2‰ (Yamamuro et al., 2001, 2003; Christiaen et al., 2014). The average δ15N value of H. ovalis leaves in the current study was 6.3‰, with a maximum value of 8.9‰. These δ15N values were also greater than those recorded from the Leschenault estuary (mean = 2.13 ± 0.58‰, n = 30, data unpublished), which is an estuary also dominated by H. ovalis and located 150 km south of the Swan-Canning estuary. Higher δ15N values in seagrass leaves have been used to indicate anthropogenic nitrogen [dissolved inorganic N; dissolved inorganic nitrogen (DIN)] from sources such as sewage. The δ15N isotope is typically higher in wastewater effluent compared to DIN in natural systems due to enzymatic preference for 14N by bacteria during nitrogen transformations (Lepoint et al., 2004). For example, elevated δ15N signatures in stream biota have previously been used to indicate DIN from leaking septic tanks of an urban watershed that was entering via groundwater (Steffy and Kilham, 2004). Groundwater modeling of the current study area at Alfred Cove Marine park indicated flux potential of groundwater directly over sites N1 to N3 (Figure 1; Cahill et al., 2017). These sites also had the highest δ15N in seagrass tissues and in the sediment of all the sites which indicates groundwater is a likely source of DIN for these seagrass. The groundwater N-NH4 concentrations near these same sites were between 30–60 times greater than the N-NH4 concentrations in surface water at the seagrass meadows (Table 1). Ammonium is considered the dominant form of DIN for seagrass uptake, particularly for uptake from the roots (Touchette and Burkholder, 2000). Such relatively high concentrations of N-NH4 in the groundwater are likely a result of denitrification within the aquifer or during infiltration through unsaturated sediments, which also elevates the δ15N-NO3 value as NO3 is consumed (Mariotti et al., 1988). However, the contribution of legacy DIN entering the groundwater from old septic tank systems (which are now largely disused in the region) cannot be ruled out. For this study, the only δ15N-NO3 value that could be estimated from the groundwater at Alfred Cove Marine Park was 6.8‰, but this was from the section of the aquifer that had lower N-NH4 concentrations, and so this value is likely to be higher closer to site N3. Additionally, the stormwater drain entering site N3 had a δ15N-NO3 value of 11‰, which may indicate leaching highly denitrified residual nitrogen that is mobilized from the urban catchment during high rainfall events. Several factors influence the δ15N values of seagrasses including temperature, nitrogen availability and the influence of denitrification (higher δ15N) vs. fertilizer run-off (lower δ15N) and nitrogen from biological nitrogen fixation (δ15N close to 0‰) (Lepoint et al., 2004; Glibert et al., 2019). Further investigation of fractionation effects due to denitrification processes in the aquifer and in the rhizosphere would increase confidence in the interpretation of these results and allow more definitive conclusions as to the elevated δ15N observed in the current study.
Seagrasses as Indicators for Heavy Metals
Heavy metal concentrations of the Swan-Canning estuary have been previously reported to be highly heterogenous within the estuary, with some sites around the city center contaminated with zinc, lead and copper at levels exceeding Australian and New Zealand sediment quality trigger values (Rate et al., 2000; Nice, 2009). Additionally, groundwater from disused waste disposal sites along the Swan-Canning estuary, including from the site under study, has been found to be contaminated with lead, aluminum, chromium, copper, zinc, arsenic, cadmium, manganese, nickel, and iron (Evans, 2009; Vogwill and Oldmeadow, 2018). Heavy metal analysis of mussels in the Swan-Canning estuary found that zinc was present at the highest concentrations of all metals tested (average across sites = 29.3 mg kg–1), and that these mussels had concentrations of cadmium, copper and zinc that were approximately twice as high as the nearby embayment of Cockburn Sound (Shute, 2007). Some heavy metal concentrations in H. ovalis tissue in this study were above sediment guidelines (e.g., arsenic, cadmium and zinc), but were not the highest recorded for this species. The highest literature values of heavy metal concentrations for H. ovalis tissue were recorded from Sydney estuary, which is heavily urbanized with an extensive history of industrial use and subsequent contamination events (Birch et al., 2018a). However, few studies exist with reported values of heavy metal concentrations for Halophila species (see Nienhuis, 1986; Birch et al., 2018a; Bonanno and Raccuia, 2018), which makes global comparisons limited.
There is little information on how heavy metals impact seagrass physiology and their potential to biomagnify through food chains; especially for H. ovalis. Of the few toxicity studies that have been completed, heavy metals have been found to predominantly impact the photosynthetic apparatus of seagrasses (Ralph and Burchett, 1995; Prange and Dennison, 2000). In the current study, aluminum, iron and arsenic were all negatively correlated with seagrass biomass, which could be indicative of photosystem damage. However, this correlation may also be due to other parameters known to impact seagrass biomass (e.g., sulfide and light) that could be co-correlated with metal concentration. In the case of arsenic, increased uptake of arsenic in macrophytes has been linked with phosphorus deficiency which may impact growth (Chen et al., 2015). This is because plants can uptake arsenic unintentionally via phosphate and silicone transporters. A range of freshwater plants including Vallisneria natans, which is in the same family as H. ovalis, have been shown to hyperaccumulate arsenic (Chen et al., 2015; Wagner et al., 2020), and their potential for use in phytoremediation of wetlands is being explored (Rahman and Hasegawa, 2011). In both the current study and that of Birch et al. (2018a), H. ovalis roots contained total arsenic concentrations that were between two and seven times higher than those in the sediments, which may indicate that H. ovalis is also able to accumulate arsenic from sediments. Groundwater surrounding the Perth region is thought to be enriched in arsenic due to the exposure of pyritic sediments caused by a combination of dewatering activities, groundwater abstraction, and reduced rainfall; all of which lower the water table (Appleyard et al., 2006). Iron concentration is commonly correlated with arsenic, as was the situation in the current study, as Fe (III) minerals are strong scavengers of arsenic via surface sorption and coprecipitation (Johnston et al., 2011). Additionally, the formation of Fe (III) minerals on seagrass roots (so called iron plaques) may be driven by oxygen loss from seagrass roots (Jensen et al., 2005; Brodersen et al., 2014; Martin et al., 2019), as has been shown for rice (Wang et al., 2013; Cheng et al., 2014). Reductive dissolution of Fe (III) minerals can occur in anoxic conditions (e.g., when root oxygen loss ceases at night), which can then release arsenic to the water column (Bennett et al., 2012). Plaque formation and dissolution may also be driven by the activities root associated bacteria via elemental cycling (discussed in section below).
It should be stated that the concentrations of metals in seagrass tissues presented here may not necessarily reflect the levels present in the sediment, as whilst seagrass may accumulate some metals, they may also exclude others. Indeed, using the sediment and seagrass metal data collected from the current study site in winter, only concentrations of arsenic and lead were found to be significantly related to the concentrations in the sediment. Additionally, metal bioavailability in sediments is largely controlled by sediment geochemistry. In the current study, the concentrations of cadmium and lead in seagrass roots were significantly correlated to sediment C% (cadmium: p = 0.03, r = 0.48, lead: p < 0.01, r = 0.64). However, the interpretation of this is further complicated by the fact that seagrass are also capable of uptake of some metals from the water column through their shoots (Luy et al., 2012; Malea et al., 2018). The advantage of focusing on seagrass tissue rather than sediment or water concentrations is that it provides an integrated measurement of exposure over a period of time rather than just a snapshot which is highly biased by flow, sediment re-suspension/re-working, mixing, tides and other geochemical properties of the sediment. Another benefit of measuring seagrass tissue directly, is that it provides a measure of the total metal concentrations that organisms feeding on seagrass may be exposed to. In fact, the effect of heavy metal contamination in seagrass ecosystems may have a greater impact on the organisms that feed on seagrasses if these metals biomagnify through food webs. Black swans (Cygnus atratus) are significant grazers of H. ovalis in the Swan-Canning estuary; removing up to 23% of total seagrass production (Eklof et al., 2009). Whilst heavy metal accumulation has been demonstrated for various fauna inhabiting the Swan-Canning estuary such as mussels and bony fish (Shute, 2007; Ranaldi and Gagnon, 2008), heavy metal accumulation in black swans has not been investigated. Recently, scales from wetland snakes collected across wetlands surrounding the Swan-Canning estuary showed contamination of various heavy metals including Mn, As, and Se, which suggests these metals could be accumulating to levels of concern in top predators (Lettoof et al., 2021). Further research on metal biomagnification and biotransference in seagrass ecosystems, as well as understanding the forms that these metals are in (e.g., organic vs. inorganic) is warranted to understand the environmental implications of seagrasses that may accumulate metals of concern.
Linking Seagrass Root Bacteria to Root Tissue Nutrients and Root Tissue Metals
The composition and predicted functions of the root associated bacteria of H. ovalis were linked to both seagrass root nutrients (N%, C%, δ13C, δ15N) and seagrass root metal concentrations (particularly As and Fe). For example, some putative sulfide oxidizers including Sulfurimonas and Sulfurovum were positively correlated to root N% and C%. Sulfurimonas related sequences have been recovered previously from seagrass roots, and have been shown to respond to environmental perturbations such as light and oxygen availability (Fahimipour et al., 2017; Martin et al., 2018, 2019). Their relatively high abundance in seagrass roots combined with their apparent response to environmental conditions could make them a good candidate indicator of change across different seagrass species and environments.
Several studies have also shown that metal pollution can impact microbial (bacteria and phytoplankton) communities by decreasing diversity, biomass, and activity (Chodak et al., 2013; Chen et al., 2014; Gołebiewski et al., 2014), which may affect overall nutrient cycling and productivity. Here, both As and Fe were highly correlated to the seagrass root bacterial community structure as well as predicted functions. Of the most abundant bacteria present in the seagrass root microbiome (top 40 ASVs), the genera Lewinella and the Marine methylotrophic group 3 were strongly positively correlated with seagrass root concentrations of As and Fe. Methylotrophic bacteria use reduced one-carbon compounds (such as methane, methanol and other methylated compounds) as the carbon source for their growth and are key players in the global carbon cycle, while Lewinella strains are capable of hydrolysis and utilization of complex carbon substrates (McIlroy and Nielsen, 2014). Whilst these genera are significantly correlated with these metals in the current study, this does not imply a cause-effect relationship. However, it does suggest that these bacteria are at least resistant to environments with greater arsenic and iron concentrations which implies they may prove promising candidates for tracking these metals in seagrass and coastal ecosystems.
The speciation and mobility of metals in the environment is also highly influenced by redox activities of microbes. For example, ferrous iron and arsenite can serve as electron donors for several chemolithotrophic microbes that use oxygen or nitrate as electron acceptors (Hassan et al., 2015). Sulfur-cycling bacteria and methane oxidizers can also impact arsenic and iron mobilization in sediments (Glodowska et al., 2020). As reported previously (Cúcio et al., 2018; Scholz et al., 2019; Tarquinio et al., 2019; Martin et al., 2020a,b), the seagrass root bacterial community was overwhelmingly dominated by putative sulfur cyclers, particularly sulfide oxidizers such as [Ca. Thiodiazotropha (Sedimenticolaceae), Ca. Electrothrix (Desulfobulbaceae), and Sulfurimonas (Sulfurimonadaceae). Sulfide intrusion, and sulfide tissue concentrations of H. ovalis and of sediments in the Swan-Canning estuary have previously been found to be greater than sulfide intrusion of H. ovalis in another estuary in the southwest of Australia (the Leschenault estuary) (Kilminster et al., 2014; Martin et al., 2020a). This difference is likely due to the higher degree of nutrient and organic matter enrichment in the more urbanized Swan-Canning estuary. These sulfur cycling microbes were associated with sites experiencing higher degree of sediment stress (predominantly measured as greater sulfide intrusion). Sulfide concentrations and sulfide intrusion were not measured in the current study, but would be playing an important role in influencing the composition of the root bacterial communities of H. ovalis seen here. The role of sulfur cycling bacteria in influencing seagrass health is an important ongoing research area with implications for improving both environmental monitoring and seagrass restoration (Martin et al., 2020b; Scholz et al., 2021).
Whilst the development of microbial indicators using next-generation sequencing technologies shows great promise for increasing the scope and sensitivity of environmental monitoring, their true success will only be realized when combined with experimental approaches to unravel mechanistic links between microbial taxonomy and function, which have been confirmed from isolated cultures. These mechanistic links are also necessary for predicting microbial responses to pollutants across a range of environments. However, despite the well-known limitations of sequencing technology, the integration of microbial parameters into current monitoring programs is still a worthwhile exercise for hypothesis generation regarding pollution impacts on ecosystems functions. Future advances in sequencing technology will also likely facilitate the uptake of this technology by more diverse end users; either as a result of decreased sequencing costs or increased accuracy of data (e.g., long read sequencing) and improvements in database accuracy. As such, complementary use of biochemical and molecular indicator tools shows great promise as another line of evidence in helping understand ecosystem response to environmental perturbations and ultimately aid in predicting and mitigating impacts of future change.
Data Availability Statement
The datasets presented in this study can be found in online repositories. The names of the repository/repositories and accession number(s) can be found below: https://www.ncbi.nlm.nih.gov/, PRJNA663104.
Author Contributions
BM: study design and conception, method development, data collection, data analysis, drafting the manuscript, and approval of final submission. JM: method development, data collection, data analysis, drafting the manuscript, and approval of final submission. MF, GK, and JC: study design and conception, method development, drafting the manuscript, and approval of final submission. GS: study design and conception, data analysis, drafting the manuscript, and approval of final submission. All authors contributed to the article and approved the submitted version.
Funding
This research was funded through WA Department of Biodiversity Conservation and Attractions (DBCA). MF was supported by the Robson and Robertson postdoctoral fellowship awarded by the Jock Clough Marine Foundation. This research was partly supported by the Integrated Coastal Analyses and Sensor Technology (ICoAST) project with funding from the Indian Ocean Marine Research Centre, a joint partnership between The University of Western Australia (UWA), the Australian Institute of Marine Science (AIMS), the Commonwealth Scientific and Industrial Research Organisation (CSIRO), and the Department of Primary Industries and Regional Development (DPIRD) WA.
Conflict of Interest
The authors declare that the research was conducted in the absence of any commercial or financial relationships that could be construed as a potential conflict of interest.
Publisher’s Note
All claims expressed in this article are solely those of the authors and do not necessarily represent those of their affiliated organizations, or those of the publisher, the editors and the reviewers. Any product that may be evaluated in this article, or claim that may be made by its manufacturer, is not guaranteed or endorsed by the publisher.
Acknowledgments
We thank Liam Kelly, Natalie Joyce, and City of Melville for their assistance with fieldwork and collection of data. We also thank the reviewers who have improved this manuscript through review. We also acknowledge the use of WABC and UWA-SAGE, as well as the technical assistance of Douglas Ford, Kate Bowler, and Michael Smirk for stable isotope and metal analysis. We acknowledge the use of the services and facilities of AGRF and PWIS for many fruitful discussions. We acknowledge that this work was completed on Noongar land, and that Noongar people remain the spiritual and cultural custodians of their land, and continue to practice their values, languages, beliefs and knowledge. We pay our respects to the traditional owners of the lands on which we live and work across Western Australia and Australia.
Supplementary Material
The Supplementary Material for this article can be found online at: https://www.frontiersin.org/articles/10.3389/fmars.2021.768864/full#supplementary-material
Footnotes
References
Appleyard, S. J., Angeloni, J., and Watkins, R. (2006). Arsenic-rich groundwater in an urban area experiencing drought and increasing population density, Perth, Australia. Appl. Geochem. 21, 83–97. doi: 10.1016/j.apgeochem.2005.09.008
Barbera, P., Kozlov, A. M., Czech, L., Morel, B., Darriba, D., Flouri, T., et al. (2019). EPA-ng: massively parallel evolutionary placement of genetic sequences. Syst. Biol. 68, 365–369. doi: 10.1093/sysbio/syy054
Bennett, W. W., Teasdale, P. R., Panther, J. G., Welsh, D. T., Zhao, H., and Jolley, D. F. (2012). Investigating arsenic speciation and mobilization in sediments with DGT and DET: a mesocosm evaluation of oxic-anoxic transitions. Environ. Sci. Technol. 46, 3981–3989. doi: 10.1021/es204484k
Birch, G. F., Cox, B. M., and Besley, C. H. (2018a). Metal concentrations in seagrass (Halophila ovalis) tissue and ambient sediment in a highly modified estuarine environment (Sydney estuary, Australia). Mar. Pollut. Bull. 131, 130–141. doi: 10.1016/j.marpolbul.2018.04.010
Birch, G. F., Cox, B. M., and Besley, C. H. (2018b). The relationship between metal concentrations in seagrass (Zostera capricorni) tissue and ambient fine sediment in modified and near-pristine estuaries (Sydney estuaries, Australia). Mar. Pollut. Bull. 128, 72–81. doi: 10.1016/j.marpolbul.2018.01.006
Birch, W. R. (1975). Some chemical and calorific properties of tropical marine angiosperms compared with those of other plants. J. Appl. Ecol. 12, 201–212.
Birrer, S. C., Dafforn, K. A., Sun, M. Y., Williams, R. B. H., Potts, J., Scanes, P., et al. (2019). Using meta-omics of contaminated sediments to monitor changes in pathways relevant to climate regulation. Environ. Microbiol. 21, 389–401. doi: 10.1111/1462-2920.14470
Bonanno, G., and Orlando-Bonaca, M. (2017). Trace elements in Mediterranean seagrasses: accumulation, tolerance and biomonitoring. A review. Mar. Pollut. Bull. 125, 8–18. doi: 10.1016/j.marpolbul.2017.10.078
Bonanno, G., and Raccuia, S. A. (2018). Comparative assessment of trace element accumulation and bioindication in seagrasses Posidonia oceanica, Cymodocea nodosa and Halophila stipulacea. Mar. Pollut. Bull. 131, 260–266. doi: 10.1016/j.marpolbul.2018.04.039
Brearly, A. (2006). Ernest Hodgkin’s Swanland: Estuaries and Coastal Lagoons of South-Western Australia. Perth, WA: UWA Publishing.
Brodersen, K. E., Nielsen, D. A., Ralph, P. J., and Michael, K. (2014). Oxic microshield and local pH enhancement protects Zostera muelleri from sediment derived hydrogen sulphide. New Phytol. 205, 1264–1276. doi: 10.1111/nph.13124
Burton, G. A., and Johnston, E. L. (2010). Assessing contaminated sediments in the context of multiple stressors. Environ. Toxicol. Chem. 29, 2625–2643. doi: 10.1002/etc.332
Cahill, K., Rutherford, J., Farmer, D., and Munday, T. (2017). Ground-Based Geophysics: Results from an Investigation Near Lucky Bay. Perth, WA: CSIRO.
Callahan, B. J., McMurdie, P. J., Rosen, M., Han, A. W., Johnson, A. J. A., and Holmes, S. (2016). DADA2: high resolution sample inference from Illumina amplicon data. Nat. Methods 13, 581–583. doi: 10.1038/nmeth.3869.DADA2
Caporaso, J. G., Lauber, C. L., Walters, W. A., Berg-Lyons, D., Lozupone, C. A., Turnbaugh, P. J., et al. (2011). Global patterns of 16S rRNA diversity at a depth of millions of sequences per sample. Proc. Natl. Acad. Sci. U.S.A. 108, 4516–4522. doi: 10.1073/pnas.1000080107
Casciotti, K. L., Sigman, D. M., Hastings, M. G., Böhlke, J. K., and Hilkert, A. (2002). Measurement of the oxygen isotopic composition of nitrate in seawater and freshwater using the denitrifier method. Anal. Chem. 74, 4905–4912. doi: 10.1021/ac020113w
Caspi, R., Billington, R., Ferrer, L., Foerster, H., Fulcher, C. A., Keseler, I. M., et al. (2016). The MetaCyc database of metabolic pathways and enzymes and the BioCyc collection of pathway/genome databases. Nucleic Acids Res. 44, D471—D480. doi: 10.1093/nar/gkv1164
Chen, G., Liu, X., Brookes, P. C., and Xu, J. (2015). Opportunities for phytoremediation and bioindication of arsenic contaminated water using a submerged aquatic plant: Vallisneria natans (lour.) Hara. Int. J. Phytoremediation 17, 249–255. doi: 10.1080/15226514.2014.883496
Chen, J., He, F., Zhang, X., Sun, X., Zheng, J., and Zheng, J. (2014). Heavy metal pollution decreases microbial abundance, diversity and activity within particle-size fractions of a paddy soil. FEMS Microbiol. Ecol. 87, 164–181. doi: 10.1111/1574-6941.12212
Cheng, H., Wang, M., Wong, M. H., and Ye, Z. (2014). Does radial oxygen loss and iron plaque formation on roots alter Cd and Pb uptake and distribution in rice plant tissues? Plant Soil 375, 137–148. doi: 10.1007/s11104-013-1945-0
Chodak, M., Gołebiewski, M., Morawska-Płoskonka, J., Kuduk, K., and Niklińska, M. (2013). Diversity of microorganisms from forest soils differently polluted with heavy metals. Appl. Soil Ecol. 64, 7–14. doi: 10.1016/j.apsoil.2012.11.004
Christiaen, B., Bernard, R. J., Mortazavi, B., Cebrian, J., and Ortmann, A. C. (2014). The degree of urbanization across the globe is not reflected in the δ15N of seagrass leaves. Mar. Pollut. Bull. 83, 440–445. doi: 10.1016/j.marpolbul.2013.06.024
Coplen, T. B., Brand, W. A., Gehre, M., Gröning, M., Meijer, H. A. J., Toman, B., et al. (2006). New guidelines for δ13C measurements. Anal. Chem. 78, 2439–2441. doi: 10.1021/ac052027c
Cúcio, C., Overmars, L., Engelen, A. H., and Muyzer, G. (2018). Metagenomic analysis shows the presence of bacteria related to free-living forms of sulfur-oxidizing chemolithoautotrophic symbionts in the rhizosphere of the seagrass Zostera marina. Front. Mar. Sci. 5:171. doi: 10.3389/fmars.2018.00171
Czech, L., Barbera, P., and Stamatakis, A. (2020). Genesis and Gappa: processing, analyzing and visualizing phylogenetic (placement) data. Bioinformatics 36, 3263–3265. doi: 10.1093/bioinformatics/btaa070
Dafforn, K. A., Simpson, S. L., Kelaher, B. P., Clark, G. F., Komyakova, V., Wong, C. K. C., et al. (2012). The challenge of choosing environmental indicators of anthropogenic impacts in estuaries. Environ. Pollut. 163, 207–217. doi: 10.1016/j.envpol.2011.12.029
De Boer, W. F. (2007). Seagrass-sediment interactions, positive feedbacks and critical thresholds for occurrence: a review. Hydrobiologia 591, 5–24. doi: 10.1007/s10750-007-0780-9
Douglas, G. M., Maffei, V. J., Zaneveld, J. R., Yurgel, S. N., Brown, J. R., Taylor, C. M., et al. (2020). PICRUSt2 for prediction of metagenome functions. Nat. Biotechnol. 38, 685–688. doi: 10.1038/s41587-020-0548-6
Duarte, C. (1990). Seagrass nutrient content. Mar. Ecol. Prog. Ser. 67, 201–207. doi: 10.3354/meps067201
Eklof, J. S., McMahon, K., and Lavery, P. S. (2009). Effects of multiple disturbances in seagrass meadows: shading decreases resilience to grazing. Mar. Freshw. Res. 60, 1317–1327. doi: 10.1071/MF09008
Evans, S. (2009). A Baseline Study of Contaminants in Groundwater at Disused Waste Disposal Sites in the Swan Canning Catchment. Perth, WA: Department of Water and Environmental Regulation.
Fahimipour, A. K., Kardish, M. R., Lang, J. M., Green, J. L., Eisen, J. A., and Stachowicz, J. J. (2017). Global-scale structure of the eelgrass microbiome. Appl. Environ. Microbiol. 83:e03391-16. doi: 10.1128/AEM.03391-16
Filippini, G., Bugnot, A. B., Johnston, E. L., Ruszczyk, J., Potts, J., Scanes, P., et al. (2019). Sediment bacterial communities associated with environmental factors in Intermittently Closed and Open Lakes and Lagoons (ICOLLs). Sci. Total Environ. 693:133462. doi: 10.1016/j.scitotenv.2019.07.268
Gerritse, R. G., Wallbrink, P. J., and Murray, A. S. (1998). Accumulation of phosphorus and heavy metals in the Peel-Harvey Estuary in Western Australia: results of a preliminary study. Estuar. Coast. Shelf Sci. 47, 679–693. doi: 10.1006/ecss.1998.0392
Glibert, P. M., Middelburg, J. J., Mcclelland, J. W., and Vander Zanden, M. J. (2019). Stable isotope tracers: enriching our perspectives and questions on sources, fates, rates, and pathways of major elements in aquatic systems. Limnol. Oceanogr. 66, 950–981. doi: 10.1002/lno.11087
Glodowska, M., Stopelli, E., Schneider, M., Rathi, B., Straub, D., Lightfoot, A., et al. (2020). Arsenic mobilization by anaerobic iron-dependent methane oxidation. Commun. Earth Environ. 1:42. doi: 10.1038/s43247-020-00037-y
Gołebiewski, M., Deja-Sikora, E., Cichosz, M., Tretyn, A., and Wróbel, B. (2014). 16S rDNA pyrosequencing analysis of bacterial community in heavy metals polluted soils. Microb. Ecol. 67, 635–647. doi: 10.1007/s00248-013-0344-7
Govers, L. L., Lamers, L. P. M., Bouma, T. J., Eygensteyn, J., de Brouwer, J. H. F., Hendriks, A. J., et al. (2014). Seagrasses as indicators for coastal trace metal pollution: a global meta-analysis serving as a benchmark, and a Caribbean case study. Environ. Pollut. 195, 210–217. doi: 10.1016/j.envpol.2014.08.028
Hassan, Z., Sultana, M., van Breukelen, B. M., Khan, S. I., and Röling, W. F. M. (2015). Diverse arsenic- and iron-cycling microbial communities in arsenic-contaminated aquifers used for drinking water in Bangladesh. FEMS Microbiol. Ecol. 91:fiv026. doi: 10.1093/femsec/fiv026
Hillman, K., McComb, A. J., and Walker, D. I. (1995). The distribution, biomass and primary production of the seagrass Halophila ovalis in the Swan/Canning Estuary, Western Australia. Aquat. Bot. 51, 1–54. doi: 10.1016/0304-3770(95)00466-D
Jensen, S. I., Kühl, M., Glud, R. N., Jørgensen, L. B., and Priemé, A. (2005). Oxic microzones and radial oxygen loss from roots of Zostera marina. Mar. Ecol. Prog. Ser. 293, 49–58. doi: 10.3354/meps293049
Jiang, Y., Kirkman, H., and Hua, A. (2001). Megacity development: managing impacts on marine environments. Ocean Coast. Manag. 44, 293–318. doi: 10.1016/S0964-5691(01)00052-7
Johnston, S. G., Keene, A. F., Burton, E. D., Bush, R. T., and Sullivan, L. A. (2011). Iron and arsenic cycling in intertidal surface sediments during wetland remediation. Environ. Sci. Technol. 45, 2179–2185. doi: 10.1021/es103403n
Kilminster, K., and Forbes, V. (2014). Seagrass as an Indicator of Estuary Condition in the Swan-Canning Estuary, Water Science Technical Series, Report No. 62. Perth, WA: Department of Water.
Kilminster, K., Forbes, V., and Holmer, M. (2014). Development of a ‘sediment-stress’ functional-level indicator for the seagrass Halophila ovalis. Ecol. Indic. 36, 280–289. doi: 10.1016/j.ecolind.2013.07.026
Lee, G., Suonan, Z., Kim, S. H., Hwang, D. W., and Lee, K. S. (2019). Heavy metal accumulation and phytoremediation potential by transplants of the seagrass Zostera marina in the polluted bay systems. Mar. Pollut. Bull. 149:110509. doi: 10.1016/j.marpolbul.2019.110509
Lepoint, G., Dauby, P., and Gobert, S. (2004). Applications of C and N stable isotopes to ecological and environmental studies in seagrass ecosystems. Mar. Pollut. Bull. 49, 887–891. doi: 10.1016/j.marpolbul.2004.07.005
Lettoof, D. C., Rankenburg, K., McDonald, B. J., Evans, N. J., Bateman, P. W., Aubret, F., et al. (2021). Snake scales record environmental metal(loid) contamination. Environ. Pollut. 274:116547. doi: 10.1016/j.envpol.2021.116547
Louca, S., and Doebeli, M. (2018). Efficient comparative phylogenetics on large trees. Bioinformatics 34, 1053–1055. doi: 10.1093/bioinformatics/btx701
Luy, N., Gobert, S., Sartoretto, S., Biondo, R., Bouquegneau, J. M., and Richir, J. (2012). Chemical contamination along the Mediterranean French coast using Posidonia oceanica (L.) Delile above-ground tissues: a multiple trace element study. Ecol. Indic. 18, 269–277. doi: 10.1016/j.ecolind.2011.11.005
Malea, P., Kevrekidis, T., Chatzipanagiotou, K. R., and Mogias, A. (2018). Cadmium uptake kinetics in parts of the seagrass Cymodocea nodosa at high exposure concentrations. J. Biol. Res. 25:5. doi: 10.1186/s40709-018-0076-4
Mariotti, A., Landreau, A., and Simon, B. (1988). 15N isotope biogeochemistry and natural denitrification process in groundwater: application to the chalk aquifer if northern France. Geochim. Cosmochim. Acta 52, 1869–1878. doi: 10.1016/0016-7037(88)90010-5
Martin, B. C., Alarcon, M. S., Gleeson, D., Middleton, A., Fraser, M. W., Ryan, M. H., et al. (2020a). Root microbiomes as indicators of seagrass health. FEMS Microbiol. Ecol. 96:fiz201. doi: 10.1093/femsec/fiz201
Martin, B. C., Middleton, J. A., Fraser, M. W., Marshall, I. P. G., Scholz, V. V., and Schmidt, H. (2020b). Cutting out the middle clam: lucinid endosymbiotic bacteria are also associated with seagrass roots worldwide. ISME J. 14, 2901–2905. doi: 10.1038/s41396-020-00771-3
Martin, B. C., Bougoure, J., Ryan, M. H., Bennett, W. W., Colmer, T. D., Joyce, N. K., et al. (2019). Oxygen loss from seagrass roots coincides with colonisation of sulphide-oxidising cable bacteria and reduces sulphide stress. ISME J. 13, 707–719. doi: 10.1038/s41396-018-0308-5
Martin, B. C., Gleeson, D., Statton, J., Siebers, A. R., Grierson, P., Ryan, M. H., et al. (2018). Low light availability alters root exudation and reduces putative beneficial microorganisms in seagrass roots. Front. Microbiol. 8:2667. doi: 10.3389/fmicb.2017.02667
Martin, M. (2011). Cutadapt removes adapter sequences from high-throughput sequencing reads. EMBnet J. 7, 2803–2809. doi: 10.1089/cmb.2017.0096
McIlroy, S. J., and Nielsen, P. H. (2014). “The family Saprospiraceae,” in The Prokaryotes, eds E. Rosenberg, E. F. DeLong, S. Lory, E. Stackebrandt, and F. Thompson (Berlin: Springer), 863–889. doi: 10.1007/978-3-642-38954-2_138
McMurdie, P. J., and Holmes, S. (2013). phyloseq: an R package for reproducible interactive analysis and graphics of microbiome census data. PLoS One 8:e61217. doi: 10.1371/journal.pone.0061217
Mellors, J., Waycott, M., and Marsh, H. (2005). Variation in biogeochemical parameters across intertidal seagrass meadows in the central Great Barrier Reef region. Mar. Pollut. Bull. 51, 335–342. doi: 10.1016/j.marpolbul.2004.10.046
Muhling, M., Woolven-Allen, J., Murrell, J. C., and Joint, I. (2008). Improved group-specific PCR primers for denaturing gradient gel electrophoresis analysis of the genetic diversity of complex microbial communities. ISME J. 2, 379–392. doi: 10.1038/ismej.2007.97
Murphy, J., and Riley, J. P. (1962). A modified single solution method for the determination of phosphate in natural waters. Anal. Chim. Acta 27, 31–36. doi: 10.1016/S0003-2670(00)88444-5
Muyzer, G., De Waal, E. C., and Uitterlinden, A. G. (1993). Profiling of complex microbial populations by denaturing gradient gel electrophoresis analysis of polymerase chain reaction-amplified genes coding for 16S rRNA. Appl. Environ. Microbiol. 59, 695–700. doi: 10.1128/aem.59.3.695-700.1993
Nice, H. (2009). A Baseline Study of Contaminants in the Sediments of the Swan and Canning Estuaries. Perth, WA: Department of Water and Environmental Regulation.
Nienhuis, P. H. (1986). Background levels of heavy metals in nine tropical seagrass species in Indonesia. Mar. Pollut. Bull. 17, 508–511. doi: 10.1016/0025-326X(86)90640-5
Oksanen, J., Blanchet, F., Friendly, M., Kindt, R., Legendre, P., McGlinn, D., et al. (2016). Vegan: Community Ecology Package. R package Version. 2.0-10.
Orth, R. J., Dennison, W. C., Lefcheck, J. S., Gurbisz, C., Hannam, M., Keisman, J., et al. (2017). Submersed aquatic vegetation in chesapeake bay: sentinel species in a changing world. BioScience 67, 698–712. doi: 10.1093/biosci/bix058
Prange, J. A., and Dennison, W. C. (2000). Physiological responses of five seagrass species to trace metals. Mar. Pollut. Bull. 41, 327–336. doi: 10.1016/S0025-326X(00)00126-0
Quast, C., Pruesse, E., Yilmaz, P., Gerken, J., Schweer, T., Yarza, P., et al. (2013). The SILVA ribosomal RNA gene database project: improved data processing and web-based tools. Nucleic Acids Res. 41, 590–596. doi: 10.1093/nar/gks1219
R Development Core Team (2011). R: A Language and Environment for Statistical Computing. Vienna: R Foundation for Statistical Computing.
Rahman, M. A., and Hasegawa, H. (2011). Aquatic arsenic: phytoremediation using floating macrophytes. Chemosphere 83, 633–646. doi: 10.1016/j.chemosphere.2011.02.045
Ralph, P. J., and Burchett, M. D. (1995). Photosynthetic responses of the seagrass Halophila ovalis (R. Br.) Hook. f. to high irradiance stress, using chlorophyll a fluorescence. Aquat. Bot. 51, 55–66. doi: 10.1016/0304-3770(95)00456-A
Ranaldi, M. M., and Gagnon, M. M. (2008). Trace metal incorporation in otoliths of black bream (Acanthopagrus butcheri Munro), an indicator of exposure to metal contamination. Water Air Soil Pollut. 194, 31–43. doi: 10.1007/s11270-008-9696-x
Rate, A. W., Robertson, A. E., and Borg, A. T. (2000). Distribution of heavy metals in near-shore sediments of the Swan River estuary, Western Australia. Water Air Soil Pollut. 124, 155–168. doi: 10.1023/A:1005289203825
Robson, B. J., and Hamilton, D. P. (2003). Summer flow event induces a cyanobacterial bloom in a seasonal Western Australian estuary. Mar. Freshw. Res. 54, 139–151. doi: 10.1071/MF02090
Scholz, V. V., Martin, B. C., Meyer, R., Schramm, A., Fraser, M. W., Nielsen, L. P., et al. (2021). Cable bacteria at oxygen-releasing roots of aquatic plants: a widespread and diverse plant-microbe association. New Phytol. 232, 2138–2151. doi: 10.1111/nph.17415
Scholz, V. V., Müller, H., Koren, K., Nielsen, L. P., and Meckenstock, R. U. (2019). The rhizosphere of aquatic plants is a habitat for cable bacteria. FEMS Microbiol. Ecol. 95:fiz062. doi: 10.1093/femsec/fiz062
Short, F., Carruthers, T., Dennison, W., and Waycott, M. (2007). Global seagrass distribution and diversity: a bioregional model. J. Exp. Mar. Biol. Ecol. 350, 3–20. doi: 10.1016/j.jembe.2007.06.012
Shute, S. (2007). Tributyltin and Heavy Metal Survey in the Swan River. Prepared for the Swan River Trust. Report No. 581/1. Wembley, WA: Oceanica Consulting Pty Ltd.
Sigman, D. M., Casciotti, K. L., Andreani, M., Barford, C., Galanter, M., and Böhlke, J. K. (2001). A bacterial method for the nitrogen isotopic analysis of nitrate in seawater and freshwater. Anal. Chem. 73, 4145–4153. doi: 10.1021/ac010088e
Simmons, W. J. (1975). Determination of low concentrations of cobalt in small samples of plant material by flameless atomic absorption spectrophotometry. Anal. Chem. 47, 2015–2018. doi: 10.1021/ac60362a013
Skrzypek, G. (2013). Normalization procedures and reference material selection in stable HCNOS isotope analyses: an overview. Anal. Bioanal. Chem. 405, 2815–2823. doi: 10.1007/s00216-012-6517-2
Statham, P. J. (2012). Nutrients in estuaries - an overview and the potential impacts of climate change. Sci. Total Environ. 434, 213–227. doi: 10.1016/j.scitotenv.2011.09.088
Steffy, L. Y., and Kilham, S. S. (2004). Elevated δ15N in stream biota in areas with septic tank systems in an urban watershed. Ecol. Appl. 14, 637–641. doi: 10.1890/03-5148
Sutherland, M. D., Dafforn, K. A., Scanes, P., Potts, J., Simpson, S. L., Sim, V. X. Y., et al. (2017). Links between contaminant hotspots in low flow estuarine systems and altered sediment biogeochemical processes. Estuar. Coast. Shelf Sci. 198, 497–507. doi: 10.1016/j.ecss.2016.08.029
Tarquinio, F., Hyndes, G. A., Laverock, B., Koenders, A., and Säwström, C. (2019). The seagrass holobiont: understanding seagrass-bacteria interactions and their role in seagrass ecosystem functioning. FEMS Microbiol. Lett. 366:fnz057. doi: 10.1093/femsle/fnz057
Terrados, J., Borum, J., Duarte, C. M., Fortes, M. D., Kamp-Nielsen, L., Agawin, N. S. R., et al. (1999). Nutrient and mass allocation of South-east Asian seagrasses. Aquat. Bot. 63, 203–217. doi: 10.1016/S0304-3770(99)00004-2
Touchette, B. W., and Burkholder, J. M. (2000). Review of nitrogen and phosphorus metabolism in seagrasses. J. Exp. Bot. 250, 133–167. doi: 10.1037/h0034880
Vogwill, R., and Oldmeadow, D. (2018). IF14 Groundwater Sampling and analysis Report. Harare: Prepared for Department of Parks and Wildlife.
Wagner, S., Hoefer, C., Puschenreiter, M., Wenzel, W. W., Oburger, E., Hann, S., et al. (2020). Arsenic redox transformations and cycling in the rhizosphere of Pteris vittata and Pteris quadriaurita. Environ. Exp. Bot. 177:104122. doi: 10.1016/j.envexpbot.2020.104122
Wang, X., Yao, H., Wong, M. H., and Ye, Z. (2013). Dynamic changes in radial oxygen loss and iron plaque formation and their effects on Cd and As accumulation in rice (Oryza sativa L.). Environ. Geochem. Health 35, 779–788. doi: 10.1007/s10653-013-9534-y
Yamamuro, M., Kayanne, H., and Yamano, H. (2003). δ15N of seagrass leaves for monitoring anthropogenic nutrient increases in coral reef ecosystems. Mar. Pollut. Bull. 46, 452–458. doi: 10.1016/S0025-326X(02)00463-0
Yamamuro, M., Umezawa, Y., and Koike, I. (2001). Seasonality in nutrient concentrations and stable isotope ratios of Halophila ovalis growing on the intertidal flat of SW Thailand. Limnology 2, 199–205. doi: 10.1007/s10201-001-8036-2
Keywords: microbiome, stable isotopes, nitrogen, carbon, 16S rRNA, arsenic, iron
Citation: Martin BC, Middleton JA, Skrzypek G, Kendrick GA, Cosgrove J and Fraser MW (2022) Composition of Seagrass Root Associated Bacterial Communities Are Linked to Nutrients and Heavy Metal Concentrations in an Anthropogenically Influenced Estuary. Front. Mar. Sci. 8:768864. doi: 10.3389/fmars.2021.768864
Received: 01 September 2021; Accepted: 31 December 2021;
Published: 27 January 2022.
Edited by:
Anthony William Larkum, University of Technology Sydney, AustraliaReviewed by:
Shunyan Cheung, Hong Kong University of Science and Technology, Hong Kong SAR, ChinaPerran Cook, Monash University, Australia
Copyright © 2022 Martin, Middleton, Skrzypek, Kendrick, Cosgrove and Fraser. This is an open-access article distributed under the terms of the Creative Commons Attribution License (CC BY). The use, distribution or reproduction in other forums is permitted, provided the original author(s) and the copyright owner(s) are credited and that the original publication in this journal is cited, in accordance with accepted academic practice. No use, distribution or reproduction is permitted which does not comply with these terms.
*Correspondence: Belinda C. Martin, QmVsaW5kYS5tYXJ0aW5AdXdhLmVkdS5hdQ==