- 1Key Laboratory of Submarine Geosciences and Prospecting Technology, College of Marine Geosciences, Ocean University of China, Qingdao, China
- 2Laboratory for Marine Geology, Qingdao National Laboratory for Marine Science and Technology, Qingdao, China
- 3Frontiers Science Center for Deep Ocean Multispheres and Earth System (FDOMES) and Key Laboratory of Marine Chemistry Theory and Technology, Ministry of Education, Ocean University of China, Qingdao, China
- 4School of Ocean Sciences, China University of Geosciences, Beijing, China
- 5Nanjing Center, China Geological Survey, Nanjing, China
- 6State Key Laboratory of Estuarine and Coastal Research, East China Normal University, Shanghai, China
The content and isotopic composition of pyrite sulfur are significantly affected by local depositional conditions and are sensitive to environmental evolution. Here, we use core QK11, collected from Xiapu Bay, southeast coast of China, to reveal how local depositional conditions constrained pyrite formation and sulfur isotopic composition since MIS5. Our results show that the content of pyrite sulfur is mainly controlled by the TOC content during interglacial intervals and is limited by the supplement of sulfate in glacial intervals. Therefore, the C/S ratios can effectively identify three transgressions since MIS5. The sulfur isotopic composition of pyrite ranges from -36.7 to 18.4‰ in the whole core. The occurrence of isotopically “heavy” pyrite is observed at the depth of 2.2~9.2, 27.2~33.8, and 43.5~62.5 mbsf, which is attributed to the influence of sulfate reservoir effect, depositional event, unsteady diagenetic environment, and other factors, highlighting the influence of local depositional and diagenetic processes on the isotopic composition of pyrite sulfur. Pyrite sulfur in other layers is generally depleted in 34S, as low as -36.7‰, indicating that the early-stage organiclastic sulfate reduction (OSR) plays an important role in sulfur isotopic fractionation. The results also suggest that organic carbon indicators (TOC/TN ratio and δ13C) combined with the C/S ratio can effectively distinguish between freshwater and marine environments, which is of great significance to reveal depositional evolution in deep time.
Introduction
Pyrite (FeS2) represents the main sulfur sink of marine sediments, which has been widely employed to trace the global sulfur cycle, biological evolution, and redox conditions in Earth’s surface environment (Berner, 1984; Raiswell and Canfield, 2012; Fike et al., 2015; Fakhraee et al., 2019). In the sediments deposited on the continental margins, the sulfide used to form pyrite is mainly derived from sulfate reduction. During the sulfate reduction process, microbes preferentially utilize the isotopically lighter sulfur in sulfate to produce sulfides, so the produced pyrite is depleted in 34S relative to the initial sulfate, resulting in a large sulfur isotopic fractionation (Jørgensen, 1982; Habicht and Canfield, 1997). In spite of the control of microbial metabolism, recent studies have suggested that the sulfur isotopic composition of pyrite (δ34Spy) is also affected by local depositional factors (Lang et al., 2020; Houghton et al., 2021; Liu et al., 2021a), which could be preserved in the sedimentary record. For example, recent studies showed that the high sedimentation rates are conducive to the formation of the relative “closed” diagenetic system, leading to the formation of isotopically “heavy” pyrite (Pasquier et al., 2017; Liu et al., 2019).
Other processes that may cause the enrichment of 34S in pyrite are as follows. In a system with low-sulfate concentration, when the sulfate is completely consumed by the sulfate reduction process, the produced sulfide will inherit the sulfur isotopic composition of the original sulfate pool with a minor isotopic fractionation (Gomes and Hurtgen, 2013). As sediments are buried deeper, the communication between porewater and overlying seawater is limited, resulting in a simultaneous increase in sulfur isotope of porewater sulfate and produced sulfides in a “closed” diagenetic system (Canfield, 2001; Liu et al., 2020a). In methane-rich environments, anaerobic methane oxidation (AOM) can be coupled with sulfate reduction, leading to pyrite formation with high content and heavy sulfur isotope in the sulfate-methane transition zone (Jørgensen et al., 2004; Borowski et al., 2013; Lin et al., 2017). The diffusion of hydrogen sulfide (H2S) driven by porewater sulfide concentration gradient would result in large differences in diagenetic processes and isotopic composition of pyrite sulfur between different layers (Liu et al., 2021b). Abiotic oxidation of sulfide to sulfate, due to frequent, repeated oxidative reworking in the shallow marine environment, would modulate the δ34S signals in sedimentary pyrite (Fry et al., 1988; Aller et al., 2010). However, the relationship between local depositional conditions and sulfur isotopic composition is complex, and the specific constraint mechanism between them requires further research.
The East China Sea (ECS) receives a lot of terrestrial sediment and organic matter from the Changjiang River and many other local rivers from Zhejiang, Fujiang, and Taiwan (Liu et al., 2018a). Under the influence of wave, tide, and current, several mud depocenters with high contents of organic carbon and fine-grain sediments were developed, which provide a natural laboratory to study how depositional processes control pyrite formation and its geochemical composition (Liu et al., 2018a; Zhao et al., 2018; Zhang et al., 2021). In this study, we analyzed the core QK11, collected from the southeast coast of China, to reveal how the depositional evolution constrains the pyrite formation and its sulfur isotope since MIS5.
Regional setting
The ECS is one of the typical river-dominated marginal oceans, which receives a large amount of terrestrial material input from the Changjiang River as well as numerous mountainous rivers, including Oujiang, Minjiang, and Zhuoshuixi (Figure 1A). The current system in the ECS inner shelf modulates the transport and deposition of terrestrial sediment (Zhang et al., 2019), including the Changjiang diluted water (CDW), Zhejiang-Fujian coastal current (ZFCC), and Taiwan warm current (TWC) (Figure 1A). And a mud depocenter was formed under such sedimentary conditions, which is distributed in a northeast-southwest direction along the coast of Zhejiang and Fujian (Liu et al., 2006; Liu et al., 2007; Dong et al., 2018; Dong et al., 2020; Dong et al., 2021). Xiapu Bay, the study area, is located in the northern Fujian Province along the ECS (Figure 1B). Luohanxi and Beixi Rivers are the two main rivers that flow into Xiapu Bay, but the runoff sediment flux can be almost neglected compared to sediments from the inner shelf of the ECS transported by tidal currents (Sun et al., 2022).
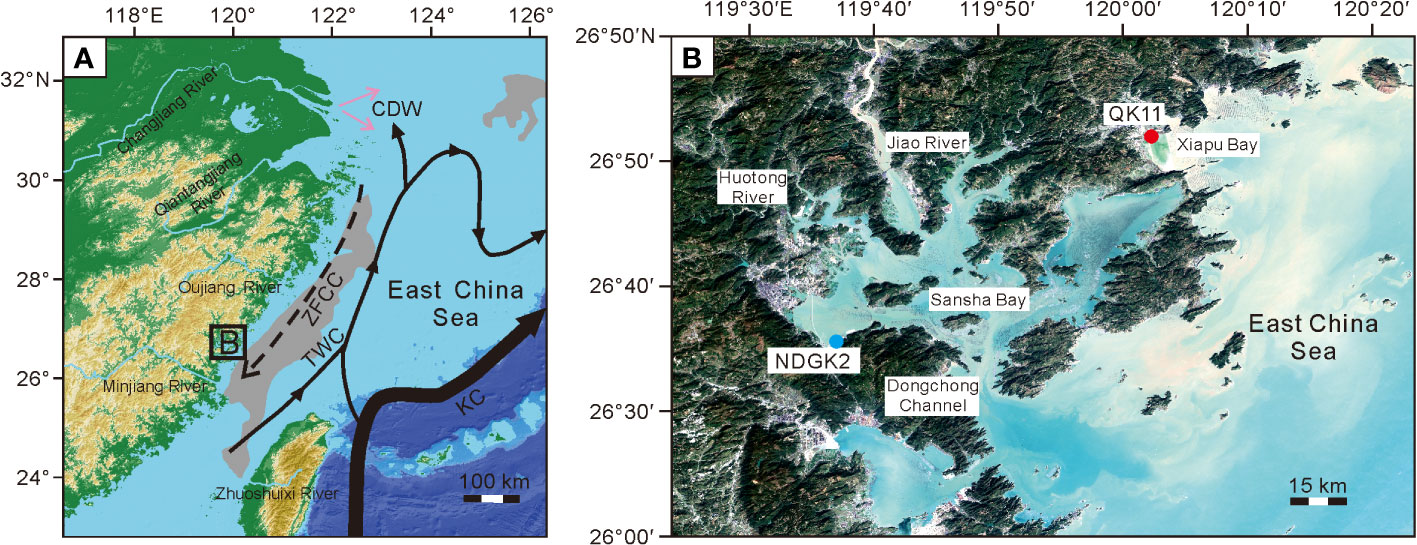
Figure 1 Regional setting and core location of core QK11. (A) The distribution of mud sediments on the East China Sea inner shelf with the current system (Liu et al., 2006). KC, Kuroshio Current; TWC, Taiwan Warm Current; ZFCC, Zhejiang-Fujian Coastal Current. (B) Xiapu Bay, the locations of core QK11 (Red dot, this study) and core NDGK2 (blue dot, Dai et al., 2021).
A series of core data from the Bohai Sea, Yellow Sea, East China Sea, and South China Sea demonstrated that the Chinese marginal seas have experienced three transgressions, corresponding to MIS5, MIS3, and MIS1, respectively (Waelbroeck et al., 2002; Lisiecki and Raymo, 2005; Wang et al., 2020). However, on the ECS coast, especially the coast area of Zhejiang and Fujian, only one or two transgressive strata were developed, and the period and magnitude of marine transgression are still a controversial topic (Geng, 1982; Zhao et al., 2008; Lin and Dai, 2012). In recent studies of cores collected from Sansha Bay and Xiapu Bay, three transgressive events since MIS5 were identified using geochemical indicators, palynology, and micropalaeontology, which established a well stratigraphic and sedimentologic framework for our study (Dai et al., 2021; Yu et al., 2021; Sun et al., 2022; Wang et al., 2022).
Materials and methods
Core QK11
The core QK11 is located in Xiapu Bay, northern Fujian Province (26.88°N, 120.05°E, Figure 1B), with a length of 70.6 m and an orifice elevation of 6 m. There is a homogeneous layer filled with clay at the depth of 70.6~69.8 mbsf (Figure 2). The sediments deposited at the depth of 69.8~37.7 mbsf are generally coarse-grained, consisting mainly of pebble-bearing sand or sand-bearing pebble layers, interspersed with several thin intervals of clay and sand. The strata at the depth of 37.7-20.8 mbsf is a set of homogeneous clay layers with shell fragments, and the sediment is dramatically coarsened at about 34-31 mbsf. At the depth of 20.8~16.1 mbsf, the sediments are composed of mainly hard clay. The deposits above 16.1 mbsf are muddy clay layers with a large amount of shell and carbonized plant fragments.
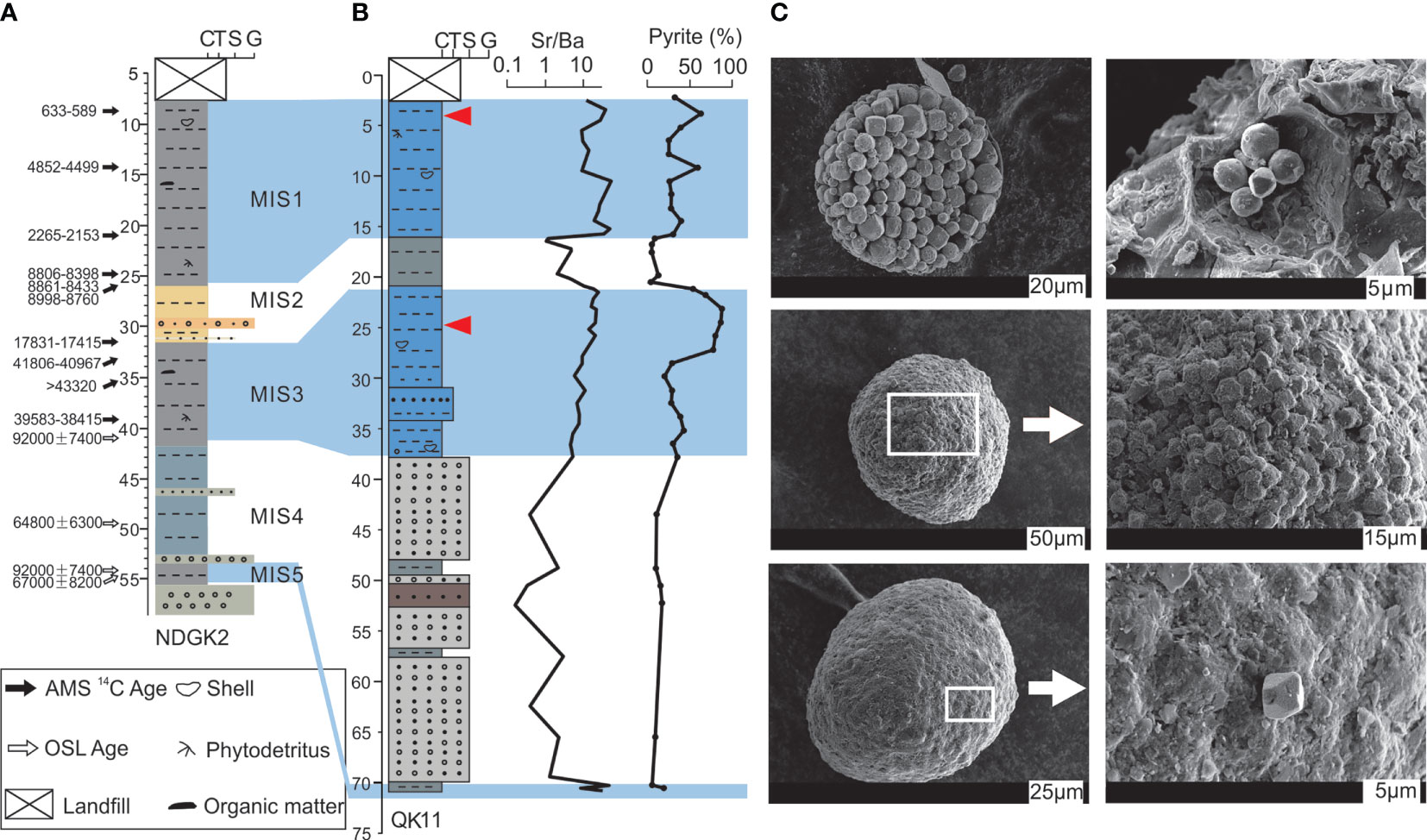
Figure 2 Chronology and pyrite of core QK11. (A) Dating of core NDGK2. (B) Sr/Ba ratio and relative content of pyrite in heavy minerals of core QK11. (C) Scanning electron microscope (SEM) photographs of pyrite aggregates. Age data are referred to Dai et al. (2021); the relative pyrite content in coarse (500~32 μm) heavy minerals are from Sun et al. (2022); The shaded regions indicate intervals of elevated relative contents of pyrite and increased Sr/Ba ratios during the interglacial MIS5, 3, and 1; The red arrows represent the sampling locations of pyrite photographs; The white rectangles represent the areas shown on the right in Figure 2C.
Pyrite sulfur analysis
Pyrite sulfur in bulk sediments was obtained by the method described by Canfield et al. (1986). Under constant flow of nitrogen or argon gas, the sediments were heated to 186°C (± 10°C) in a solution of 6 M HCl and 2 M chromium (II) chloride and stirred for 4 h. Reduced sulfur in sediments was released as hydrogen sulfide and captured as precipitation of silver sulfide in a test tube containing silver nitrate solution. After rinsing, drying, and weighing, the contents of chromium-reducible sulfur (CRS) in bulk sediment were calculated gravimetrically. In this paper, we analyzed CRS content as pyrite sulfur content.
Gas chromatography-isotope ratio mass spectrometry (GC-IRMS) was used to analyze the sulfur isotopic composition in CRS. The Instrument model: Delta V Plus GC-IRMS (Thermo Fisher Science), Sensitivity is better than 1200 M/I, error < 0.1‰; Detection error: RMs (IAEAS1, IAEAS2, IAEAS3) standard deviation < 0.3‰, analysis error < 0.1‰.
Total organic carbon and total nitrogen analysis
The contents and isotope analysis of TOC and TN were carried out in the Key Lab of Submarine Geosciences and Prospecting Techniques, MOE, China. The test instruments are an EA-IsoLink elemental analyzer with a MAS 200R autosampler coupled to a MAT253 plus isotope ratio mass spectrometer via a ConFlo IV universal interface (all from Thermo Fisher Scientific, Bremen, Germany). The Elemental Technology Co. Ltd. reference material, IVA33802180, is a soil standard sample with a total carbon content of 0.83 ± 0.05% and 0.07 ± 0.01% nitrogen, used as a routine working standard for the content determination of carbon and nitrogen. The contents of TOC and TN were calculated by standard curves and the accuracy can be estimated to be around 3% RSD. The international reference materials (RMs): USGS40 (L-glutamic), USGS64 (glycine), and USGS24 (graphite) (all from NIST, US), were used in this study. The δ13C values certified by USGS40, USGS64, and USGS24 as the mean ± 95% confidence is -26.39 ± 0.09‰, -40.81 ± 0.04‰, and -16.05 ± 0.04‰, respectively. The precision for δ13C is ± 0.06‰ (1σ) for 30 μg of carbon.
Results
Chronology and pyrite of core QK11
The chronology of core QK11 is referred to a well-established stratigraphic framework of core NDGK2 (Figure 2A), including ages from AMS14C and optically stimulated luminescence (OSL), and relative stratigraphic correlation between them (Dai et al., 2021; Sun et al., 2022). According to previous work on core QK11 (Sun et al., 2022), the relative pyrite content in heavy minerals in core QK11 increases significantly during the interglacial stages, which corresponds to the intervals with high salinity reflected by increased Sr/Ba ratios (Figure 2B). Pyrite aggregates, main framboids with a large diameter (> 50 μm), consist of octahedral and irregular pyrite microcrystals (Figure 2C). According to the relative pyrite contents and previous research results (Dai et al., 2021; Sun et al., 2022), core QK11 is divided into five sedimentary intervals (Figure 2). Three interglacial intervals at depths of 70.6~70.2, 37.5~22.8, and 15.8~2.2 mbsf correspond to MIS5, MIS3, and MIS1, respectively, whereas glacial intervals at depth of 70.2~37.5 mbsf and 22.8~15.8 mbsf correspond to MIS4 and MIS2, respectively.
Pyrite sulfur content, isotopic composition, and C/S ratio
The CRS contents, representing the pyrite sulfur in the study area (Liu et al., 2020b), show a strong fluctuation coinciding with the glacial-interglacial cycles (Figure 3A). The contents of CRS are generally less than 1.13%, and maintain high values in clay layers. The coarse-grained sediments composed of silt and pebbles present low contents of CRS, most of which are below the detection limit (0.01%). We set the CRS values below the detection limit to 0.01% for the calculation of C/S ratios.
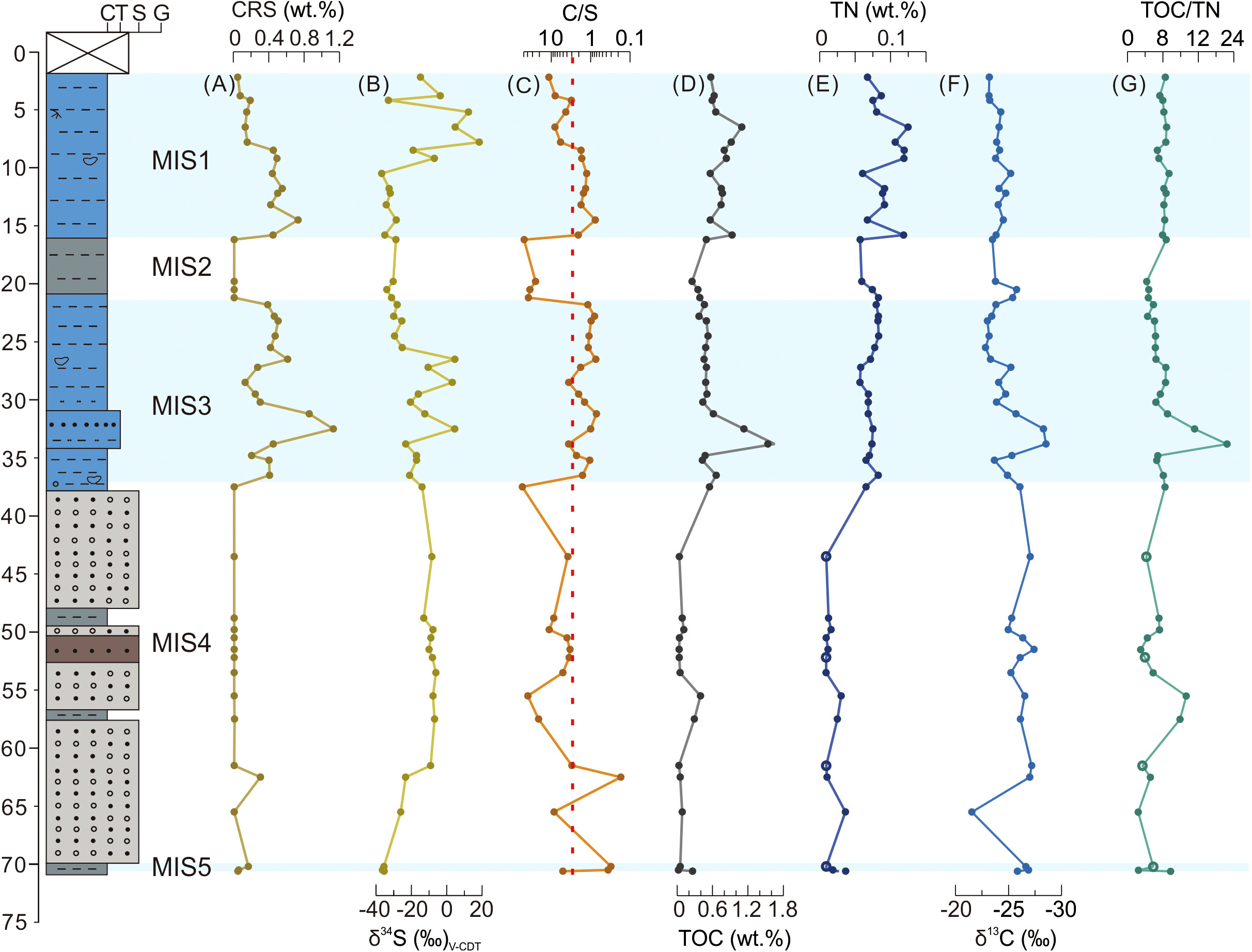
Figure 3 The lithology and contents of carbon, nitrogen, and sulfur abundance of core QK11. (A, B) The abundance of chromium-reducible sulfur (CRS) and the sulfur isotopic composition of CRS; (C) The ratio between total organic carbon and chromium-reducible sulfur (C/S); (D-F) The contents of total organic carbon (TOC) and total nitrogen (TN) and the isotopic composition of TOC; (G) The ratio between total organic carbon and total nitrogen (TOC/TN).
The δ34Spy in core QK11 ranges from -36.7‰ to 18.4‰, with Δ34S (δ34Ssulphate-δ34Spy) as high as 57.94‰, calculated from the sulfur isotopic composition of modern seawater sulfate (21‰, Tostevin et al., 2014). And the isotopically “heavy” pyrite occurs at the depth of 7.8~2.2, 33.8~27.2, and 43.5~62.5 mbsf (Figure 3B). The C/S ratio of core QK11 ranges from 0.91~38.46 and effectively divides glacial and interglacial sedimentary intervals (Figure 3C). C/S ratios of glacial intervals are high, with an average of 12.44, whereas the interglacial C/S ratios are generally less than 2.8. The average value of C/S ratios is only 1.47 in the lower part of the MIS1 interval, which increases significantly in the upper part accompanied by the decrease in CRS contents.
TOC and TN contents, δ13C of TOC, and TOC/TN ratios
The contents of TOC and TN show no obvious trends in core QK11 (Figures 3D, E). TOC content ranges from 0.02 to 1.65% with an average of 0.47%. And the maximum value occurs at the depth of 33.8 mbsf. The TN contents of several samples in the lower half of the core are too low to obtain data, which were set as 0.01% and represented as a hollow circle in Figures 3E, G. There are no trends for TOC/TN ratio and δ13C in the same way, but they all peak at the depth of 33.8 mbsf: TOC/TN ratio increases to 22.43, whereas δ13C decreases to -28.53‰ (Figures 3F, G).
Discussion
Limiting factor for the formation of sedimentary pyrite
In organic mineralization, organic carbon is consumed as an electron donor in the sulfate reduction process, with the production of hydrogen sulfide (Fike et al., 2015). Although most of the products are reoxidized to sulfate, the remaining fraction of them are able to form metastable iron sulfide with the participation of reactive iron, and ultimately convert to sedimentary pyrite which is buried as the major sulfur sink in marginal sea sediments (Jørgensen, 1982; Berner, 1984; Rickard, 2012; Fike et al., 2015). The formation of sedimentary pyrite is greatly affected by the sedimentary conditions, including the quantity and reactivity of organic carbon, the availability of reactive iron, and the supplement of sulfate (Berner, 1984). Here, we analyze the above factors to demonstrate the main factor that impacts the formation of sedimentary pyrite in the study area since MIS5.
Pyrite is sensitive to reactive iron availability which has an important influence on the early diagenetic process of sediments (Shawar et al., 2018). In the Baltic and Black Seas, for example, with high organic carbon content but limited reactive iron supply in sediments, the residual porewater hydrogen sulfide diffuses downward from the marine mud to the underlying lacustrine clay and reacts with the reactive iron to form the sulfidization front (Jørgensen et al., 2004; Holmkvist et al., 2014; Liu et al., 2020a). However, the inner shelf of the ECS receives a large amount of terrestrial supply from large and medium-sized rivers such as the Yangtze River and Qiantang River, with an adequate supply of reactive iron (Huang and Lin, 1995; Lin et al., 2002; Wei et al., 2021). The availability of reactive iron does not limit the formation of sedimentary pyrite in the study area, although strong iron reduction may inhibit the sulfate reduction process (Kao et al., 2004).
Previous studies demonstrated a significant positive correlation between the organic burial rate and sulfate reduction rate, indicating that the sedimentary pyrite formation in the ECS shelf is mainly controlled by the organic carbon content (Lin et al., 2002). This perspective is also well verified in our results by a positive correlation between CRS and TOC contents in core QK11 (R2 = 0.623) (Figure 4A), and the maximum of organic carbon contents corresponds to the peak of CRS contents (Figure 3). However, it should be noted that the contents of CRS are generally below the detection limit in glacial intervals, whereas the TOC varies between 0.1 and 0.4%, with an extremely low correlation coefficient (Figure 4A). Thus, the formation of sedimentary pyrite in glacial intervals should be affected by other factors besides the organic carbon contents (e.g., undersupply of porewater sulfate in the terrestrial environment). In the glacial intervals (MIS4 and 2), the freshwater environment caused by the low sea level could not provide enough sulfate to produce sulfide, which will finally suppress the formation of pyrite. When the study area was flooded during transgressive intervals (MIS5, 3, and 1), a decent sulfate supply would alleviate the limiting effect of sulfate on sulfide production. Therefore, the formation of sedimentary pyrite was dominated by the supplement of sulfate in the glacial intervals and mainly controlled by TOC content during interglacial intervals.
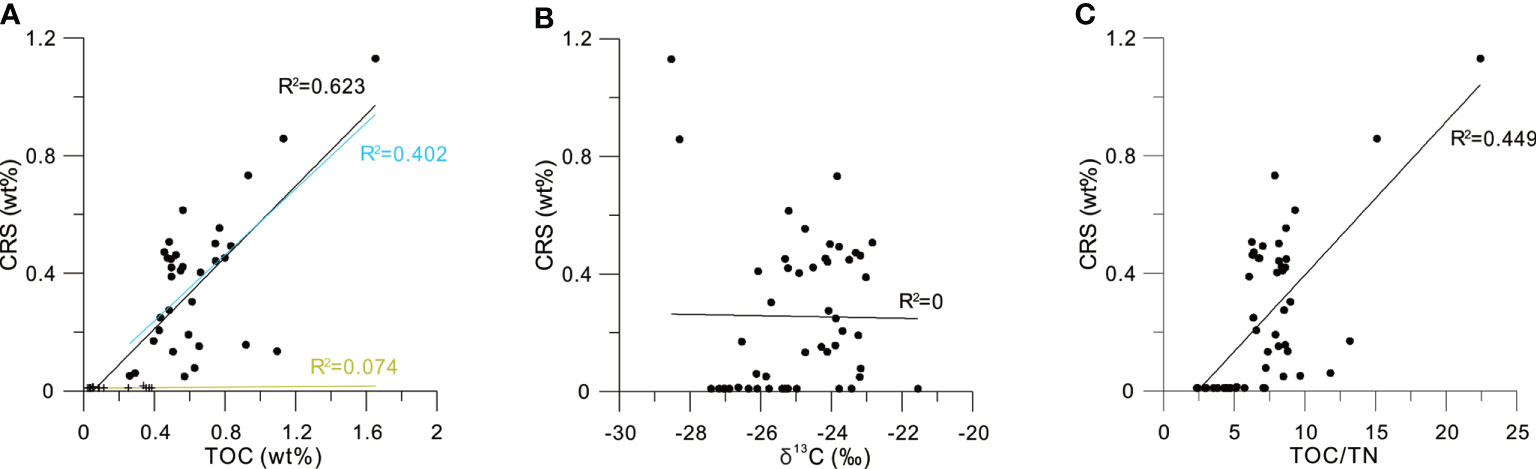
Figure 4 Sedimentary factors controlling CRS content in core QK11. (A-C) TOC content, δ13C of TOC, and TOC/TN ratio vs. CRS content. The fit line of carbon and sulfur correlation of all samples in (A) is black, the blue line is for interglacial sediments (marine environment), and the yellow line is for the glacial sediments (freshwater environment).
Previous studies suggested that the less reactive organic compounds would result in lower rates of sulfate reduction (Berner, 1984; Westrich and Berner, 1984). Thus, the source of organic carbon may potentially affect the formation of pyrite, because of the differences in reactivity between marine and terrestrial organic carbon (the latter is more refractory) (Blair and Aller, 2012). The TOC/TN ratio and δ13C of organic carbon have been widely used as classical indicators to trace the source of organic carbon in sediments deposited on global estuaries and continental shelves dominated by large rivers (Bianchi et al., 2002; Zhan et al., 2011). We attempt to assess the possible influence of variation in reactivity of organic carbon on sedimentary pyrite during transgressive cycles by using them. No positive obvious correlation is observed between δ13C and CRS content (Figure 4B). And the correlation between TOC/TN ratio and CRS is moderate, with an R2 of 0.449 (Figure 4C). Due to the influence of possible “extra nitrogen source” (e.g., adsorption of inorganic nitrogen by clay minerals), the measured values of TOC/TN ratios in terrestrial sediments are significantly different from the actual values (Meyers, 1994; Meyers, 1997; Sampei et al., 1997; Sampei and Matsumoto, 2001; Hu et al., 2014). The average values of TOC/TN ratios in the two freshwater sedimentary intervals (MIS2 and MIS4) are 4.6 and 4.4, respectively, indicating a marine origin of organic carbon. Therefore, the correlation between CRS and TOC/TN ratio in core QK11 may not truly reflect their relationships, which needs careful evaluation.
Depositional evolution since MIS5 constrained by C/S ratios
Pyrite is usually more abundant in marine sediments than in fluvial and lacustrine sediments due to the differences in supplement of sulfate between them. The statistical results show that the C/S = 2.8 ± 1.5 can be used to distinguish the marine from the freshwater sedimentary environment (Berner and Raiswell, 1984; Raiswell and Canfield, 2012; Wei and Algeo, 2020). However, C/S ratios represent high values in certain marine environments as in the freshwater environment, such as the euxinic bottom water limited by iron, organic-rich environment promoted by upwelling, and unsteady diagenetic environment (Morse and Emeis, 1990; Lyons and Berner, 1992; Aller and Blair, 1996). Thus, when applying the C/S ratio to reveal the sedimentary history, it is necessary to combine other indicators (e.g., Sr/Ba ratio) (Liu et al., 2022). Based on previous studies, we evaluate the applicability of the C/S ratio to indicate depositional evolution in the Xiapu Bay since MIS5.
The C/S ratios of glacial sedimentary intervals in core QK11 are significantly higher than 2.8 (Figure 5), indicating a typical freshwater sedimentary environment (Berner and Raiswell, 1984). But some samples of the MIS4 interval have relatively low C/S ratios, even less than 2.8. This is mainly because the contents of CRS in this interval are extremely low, and most of them are below the detection limit (0.01%), thus the actual C/S ratios should be higher than the calculated values. Sedimentary conditions during glacial intervals, such as strong weathering, undersupply of porewater sulfate, and coarse-grained sediments with less TOC, would lead to poor formation and preservation of authigenic pyrite, followed by high C/S ratios.
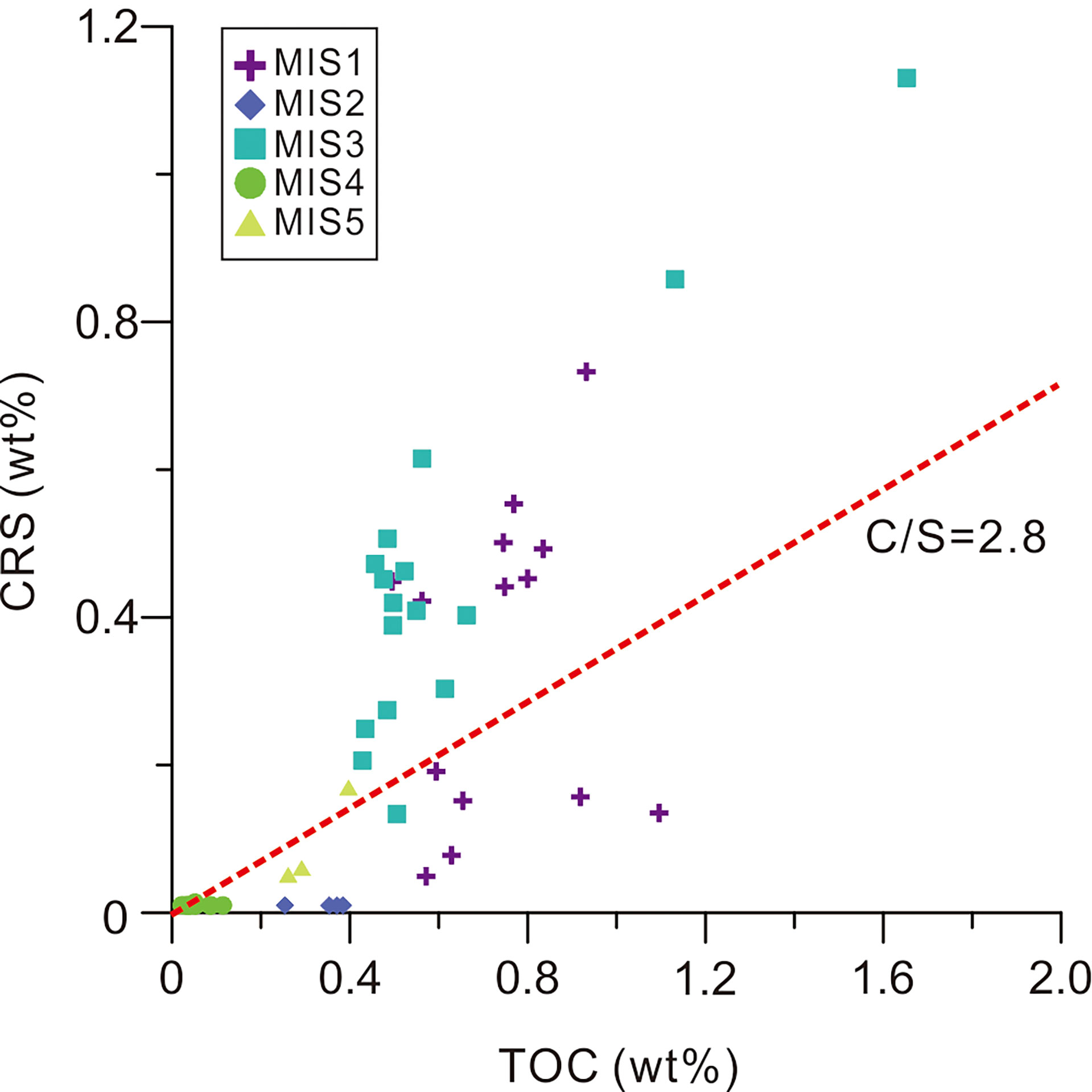
Figure 5 The correlation between the content of total organic carbon (TOC) and CRS (representing pyrite sulfur).
The C/S ratio in MIS5 ranges from 2.3 to 5.1, with an average of 4.1, and no foraminifera is observed in this interval (Sun et al., 2022). These sedimentary records seem to support that the sediments were deposited in a fluvial or lacustrine environment. However, this view needs to be questioned. In fact, C/S ratios cannot well distinguish the brackish environment from the marine environment because their ranges overlap considerably (Wei and Algeo, 2020). Compared with intervals of MIS4 and MIS2 with high C/S ratios, the C/S ratios of MIS5 interval are near the boundary values. The Sr/Ba ratio in this interval increases significantly (Figure 2B), which represents a depositional environment with increased salinity (Sun et al., 2022). In addition, the marine dinocyst record of NDGK2, located in Sansha Bay (Figure 1B), also suggests that the study area has experienced a marine incursion during MIS5 (Dai et al., 2021). We suggest that Xiapu Bay is influenced by seawater sulfate during MIS5, but the magnitude of transgression is weak so that the sedimentary environment is not conducive to the growth or later preservation of foraminifera.
The MIS1 interval is segmented in geochemical characteristics. The lower part is similar to the MIS3 interval. The evidence, including abundant pyrite aggregates, low C/S ratios, and foraminifera records, indicates a typical marine environment during this period (Sun et al., 2022). However, the upper part of MIS1 sediments is characterized by high C/S ratios and low CRS contents (Figure 3C). This phenomenon is attributed to the unsteady diagenetic environment along the ECS coast, which is consistent with previous results off the Zhejiang coast (Liu et al., 2018b; Liu et al., 2021c; Liu et al., 2022). In the unsteady diagenetic environment, the sulfate reduction is suppressed by iron reduction, and sulfides are oxidized to sulfate under strong hydrodynamic conditions and bioturbation, resulting in low CRS contents and high C/S ratios in surface sediments deposited on the ECS inner shelf (Ge et al., 2015; Zhu et al., 2016; Liu et al., 2018b).
C/S ratios show that the transgression in MIS5 is weak, whereas the transgressive events in MIS3 and MIS1 have a wider impact. Due to the unsteady diagenetic environment along the ECS coast, the sedimentary strata deposited in MIS1 is segmented in C/S ratio and CRS content. These findings demonstrate that the C/S ratio can effectively reveal the depositional evolution of Xiapu Bay since MIS5, and also emphasizes the necessity of combining different indicators in the reconstruction of the paleoenvironment (Liu et al., 2022).
Response of isotopic composition of pyrite sulfur to depositional evolution
The formation of pyrite in marine sediments plays a fundamental role in the biogeochemical carbon-sulfur-iron cycling and modulated the redox conditions on Earth’s surface over geological timescales (Algeo et al., 2015). And the evolution history of Earth’s surface environment was recorded in δ34Spy extensively (Fike et al., 2015). Recent studies have demonstrated that δ34Spy is significantly impacted by local depositional conditions in short timescales, suggesting that the isotopic composition of pyrite sulfur is very sensitive to local depositional evolution rather than the global sulfur cycle (Pasquier et al., 2017; Liu et al., 2019; Liu et al., 2020b). Here, we carry out a series of geochemical analysis work of core QK11 to study the influence of depositional evolution on δ34Spy during glacial-interglacial cycles since MIS5. On the whole, there is no obvious correlation between the δ34Spy values and other geochemical parameters (TOC, δ13C, C/S ratio, TOC/TN ratio) in core QK11 (Figures 6A–D). It probably suggests that the sulfur isotopic composition of pyrite is influenced by multiple factors rather than a certain one. Since MIS5, the study area has experienced three transgressive events, and the discrepancy of local depositional conditions such as transgressive magnitude, organic carbon input, and sedimentation rate during these periods may have varying controls on the δ34Spy.
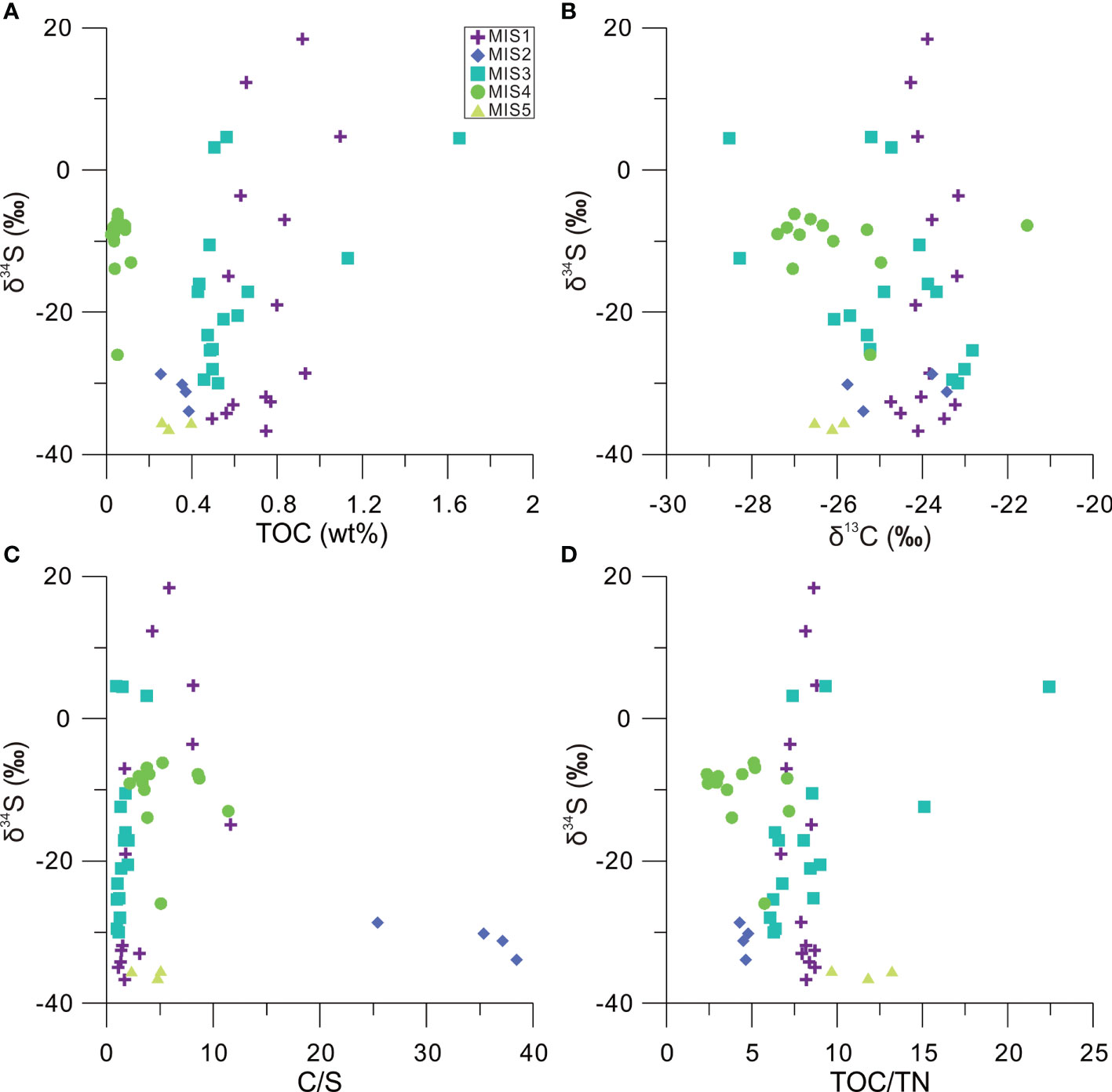
Figure 6 The correlation between δ34Spy of pyrite sulfur and TOC content (A), δ13C of TOC (B), C/S ratio (C), and TOC/TN ratio (D).
Previous studies have shown that bacterial sulfate reduction is associated with a strong isotopic fractionation, with sulfide and pyrite depleted in 34S, and possibly reaching up to 70‰ (Sim et al., 2011). The δ34Spy in sediments of Xiapu Bay deposited in MIS5 is stably negative and ranges from -35 to -40‰ generally with an offset of about 57‰. The considerable isotopic offset indicates that early-stage organiclastic sulfate reduction (OSR) plays an important role in pyrite formation, although the contribution of disproportionation in sulfur isotopic fractionation cannot be ruled out (Canfield and Thamdrup, 1994). Despite transgression being weak during MIS5, the supplement of sulfate is enough to protect δ34Spy from the sulfate reservoir effect (Gomes and Hurtgen, 2013).
Xiapu Bay presented a typical marine environment during MIS3 revealed by C/S ratios, Sr/Ba ratios, and foraminifera records (Sun et al., 2022). But at the depth of 27~34 mbsf, lithologic and geochemical characteristics change significantly, with isotopically “heavy” pyrite in sediments correspondingly (Figure 3). The sediment type changed from clay and silt to coarser sand and gravel. TOC increased from 0.5% to 1.6%, resulting in the CRS peak in the whole core. The dramatic change of TOC/TN ratio and δ13C at the depth of 32.5~33.8 mbsf indicates an important shift in the source of organic carbon, suggesting an increased input of terrestrial materials. Although not obvious, the phenomenon of source shift of organic carbon is also observed at 27 mbsf revealed by TOC/TN ratio and δ13C, where the isotopic composition of pyrite sulfur maintains a high value. We propose that the heavy sulfur isotope is related to the depositional event occurring at 27~34 mbsf of core QK11. And relevant factors, such as the availability and reactivity of organic carbon and sedimentation rates, are worth analyzing.
During the depositional event, the abrupt increased TOC/TN ratios and decreased δ13C values indicate that the organic carbon is mainly a terrigenous source, which is refractory and not conducive to being utilized by sulfate-reducing bacteria (Meyers, 1997; Blair and Aller, 2012). However, the peak values of TOC and CRS appear together, and the influence of TOC content on the sulfur isotopic composition of pyrite should be evaluated. It is suggested that a high sulfate reduction rate can significantly reduce the sulfur isotopic fractionation of OSR, while high organic carbon content can increase the sulfate reduction rate, which may lead to the positive δ34Spy value (Lin et al., 2002; Leavitt et al., 2013). However, the poor correlation between TOC content and δ34Spy in MIS3 stage sediments shows that high organic carbon content is not responsible for the positive sulfur isotopic signature of pyrite. We speculate that the high sedimentation rates associated with depositional events may be the crucial factor. High sedimentation rate would decrease the connectivity between the porewater sulfate reservoir and the overlying water column to form a relative “closed” diagenetic system (Pasquier et al., 2017; Liu et al., 2019). In this case, local porewater sulfate reservoir becomes enriched in 34S as the sulfate reduction process continues, which leads to produced sulfide and pyrites formed later becoming enriched in 34S. The preponderance of terrigenous materials also implies a great availability of reactive iron in sediments, which has important implications for early diagenetic processes. In the Bornholm Basin, the coupling of high reactive iron contents and heavy sulfur isotope was observed (Liu et al., 2021a). It is interpreted that the high sedimentation rates facilitated the burial of reactive iron and hence the subsurface availability of reactive iron for continued and progressively more 34S-enriched sediment-hosted pyrite formation.
The MIS1 strata are segmented in geochemical indicators including CRS contents, C/S ratios, and δ34Spy With 8 mbsf as the boundary, the lower part maintains a large amount of CRS which is depleted in 34S with an offset higher than 50‰. The upper member is characterized by low CRS contents, high C/S ratios, and isotopically “heavy” pyrite, due to the unsteady diagenetic environment in the ECS (Ge et al., 2015; Zhu et al., 2016). Under such depositional conditions, reoxidation of porewater hydrogen sulfide to sulfate can potentially lead to a minor and even negative value of sulfur isotopic offset between the sedimentary pyrite and original sulfate, and override in-situ signals of sulfur fractionation of sulfate reduction and other diagenetic processes (Fry et al., 1988; Fike et al., 2015). And the phenomenon of isotopically “heavy” pyrite also appears on other continental shelves with an unsteady diagenetic environment, such as the Amazon–Guianas mobile mud belt (Aller et al., 2010). In addition, given the abundance of shallow gas (CH4) buried around Sansha Bay and Xiapu Bay with a depth between 0 and 20 m (Wu et al., 2022), leakage of shallow gas may influence the sulfur isotopic composition of pyrite (Jørgensen et al., 2004; Peketi et al., 2012; Lin et al., 2016; Lin et al., 2017; Miao et al., 2021). However, the coupling of high CRS content and isotopically “heavy” pyrite is not observed in the upper part, indicating that the shallow gas is unlikely to be a significant factor.
Xiapu Bay represented a typical freshwater sedimentary environment during MIS4. The undersupply of porewater sulfate suppressed the sulfate reduction, which resulted in a positive isotopic signature of pyrite sulfur by the sulfate reservoir effect (Gomes and Hurtgen, 2013). Though the sediments of MIS2 interval deposited in a freshwater environment likewise, the sulfur isotopic composition of pyrite is negative, which cannot be explained by the sulfate reservoir effect. The possibilities include the vertical diffusion of hydrogen sulfide in adjacent layers and additional sulfate supplies. The hydrogen sulfide in the MIS1 interval mainly comes from OSR and is depleted in 34S. The hydrogen sulfide diffuses downward and combines with the reactive iron in MIS2 interval, resulting in 34S-depleted pyrite. The diffusion of hydrogen sulfide during OSR would be rather weak in an iron-sufficient environment. Therefore, the CRS contents maintain extremely low values without a sulfidization front in MIS2 interval. In addition, the two adjacent layers of the MIS2 interval were extensively affected by transgressions. After the early diagenesis, the residual porewater sulfate in adjacent layers may migrate vertically to support pyrite formation in the MIS2 interval.
Implications for classical indicators of organic carbon
The effectiveness of C/S ratios has been verified in this study as well as in previous work on the inner shelf of the ECS (Liu et al., 2021c; Liu et al., 2022). Though the C/S ratio can reveal three transgressive layers in the coastal area of southeast China since MIS5, we have to acknowledge that it is also affected by an unsteady diagenetic environment and other sedimentary factors. Therefore, when using C/S ratios to indicate paleosalinity and marine transgression, it is necessary to combine them with other indicators (such as Sr/Ba ratio and TOC/TN ratio) to reduce the risk of making a biased interpretation of depositional evolution (Liu et al., 2022).
The TOC/TN ratio has been widely utilized in the identification of organic carbon sources in estuarine and continental shelf areas (Meyers, 1994; Meyers, 1997; Yu et al., 2011; Zhan et al., 2011). In our study, however, the application of the TOC/TN ratio in terrestrial sedimentary intervals (MIS2 and MIS4) was severely disturbed due to the low organic carbon content in the sediments and the possible adsorption of inorganic nitrogen by clay minerals (Sampei and Matsumoto, 2001; Hu et al., 2014). But for transgressive strata, the combination of TOC/TN ratio and δ13C shows that the organic carbon sources in the study area experienced a transition from terrigenous source to marine and terrigenous mixed source to marine source during the three transgressions since MIS5 (Figure 7), which is consistent with the research results that the magnitude of the transgressions gradually increased as revealed by other indicators (C/S ratio, Sr/Ba ratio, etc.). This indicates that TOC/TN ratio and δ13C can effectively record sedimentary information during transgressions, and should be combined with pyrite-related indicators to indicate depositional evolution.
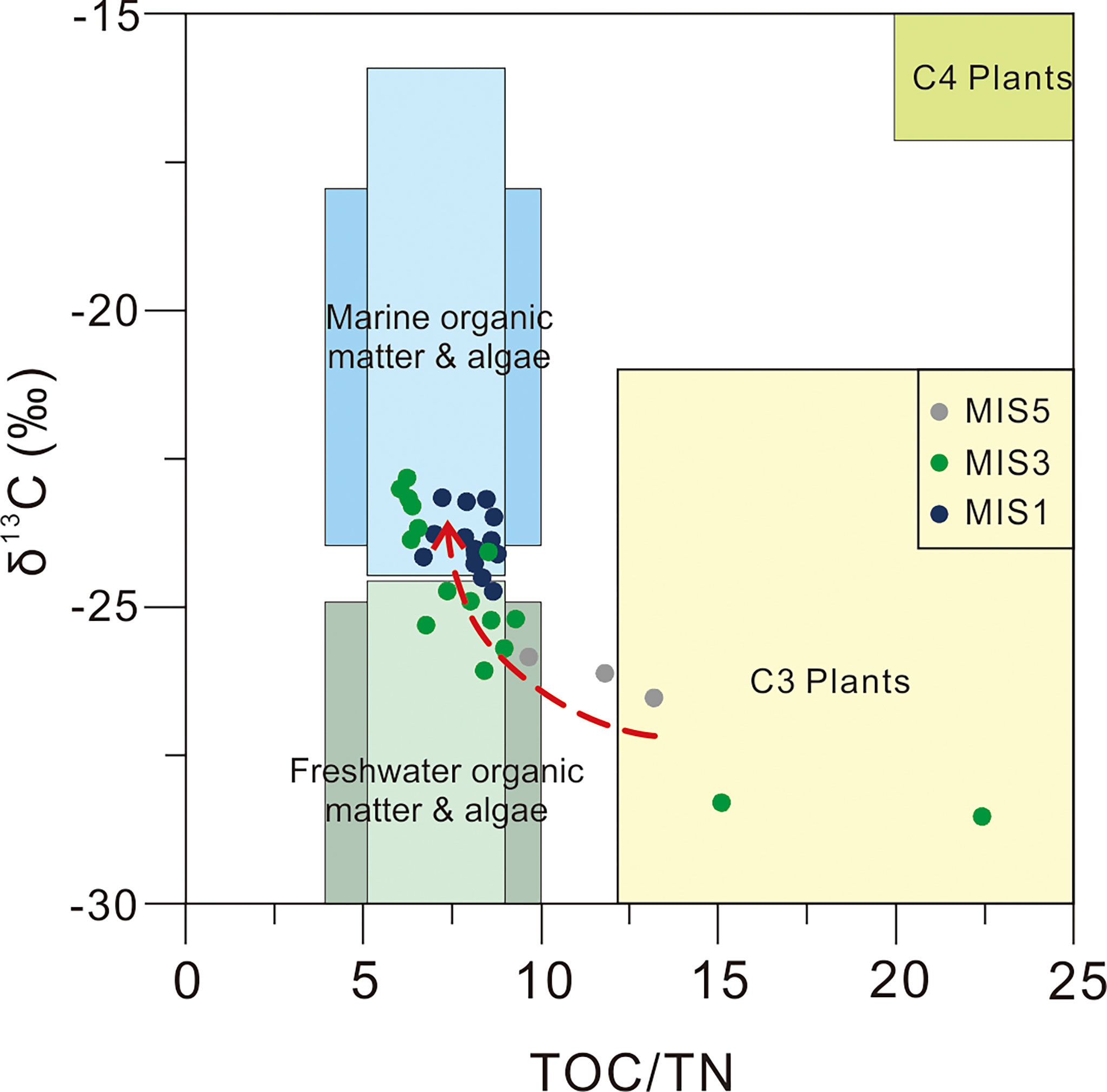
Figure 7 Source of organic matter in core QK11 indicated by TOC/TN ratios and δ13C values. The endmember values refer to Lamb et al. (2006). The red arrow indicates the evolutionary history of organic carbon sources.
Conclusions
In this study, we analyzed the depositional evolution history and its influence on the sulfur content and isotope of pyrite in the coastal area of southeast China since MIS5 according to a set of geochemical data from core QK11. The main conclusions are as follows:
1) The positive correlation between TOC and CRS contents in core QK11 indicates that sedimentary pyrite is mainly controlled by TOC in the interglacial transgressive strata. The formation of pyrite during glacial intervals is limited by the supplement of sulfate, inducing extremely low pyrite sulfur contents. Therefore, C/S ratios can effectively identify three transgressions on the Fujian coast since MIS5.
2) Local depositional conditions strongly affect the sulfur isotopic composition of authigenic pyrite in study area during transgressive cycles. The isotopically “heavy” pyrite in core QK11 is attributed to the sulfate reservoir effect, unsteady diagenetic environment, and depositional event.
3) Classical indicators, such as the TOC/TN ratio, are prone to deviation under freshwater sedimentary conditions. It is necessary to combine other geochemical indicators, such as C/S and Sr/Ba ratios, to reconstruct a more reliable history of depositional evolution.
Data availability statement
The original contributions presented in the study are included in the article/Supplementary Material. Further inquiries can be directed to the corresponding authors.
Author contributions
XC: Data curation, Writing- Original draft preparation. XL: Conceptualization, Funding acquisition, Writing- Reviewing and Editing. HW, GZ, ZM, and JY: Writing- Reviewing and Editing. JC: Funding acquisition, Writing- Reviewing and Editing. All authors contributed to the article and approved the submitted version.
Funding
This work was supported by the National Natural Science Foundation of China (41976053; 41771226) and the Natural Science Foundation of Shandong Province (ZR2021YQ26).
Conflict of interest
The authors declare that the research was conducted in the absence of any commercial or financial relationships that could be construed as a potential conflict of interest.
Publisher’s note
All claims expressed in this article are solely those of the authors and do not necessarily represent those of their affiliated organizations, or those of the publisher, the editors and the reviewers. Any product that may be evaluated in this article, or claim that may be made by its manufacturer, is not guaranteed or endorsed by the publisher.
Supplementary material
The Supplementary Material for this article can be found online at: https://www.frontiersin.org/articles/10.3389/fmars.2022.1005663/full#supplementary-material
References
Algeo T. J., Luo G. M., Song H. Y., Lyons T. W., Canfield D. E. (2015). Reconstruction of secular variation in seawater sulfate concentrations. Biogeosciences 12, 2131–2151. doi: 10.5194/bg-12-2131-2015
Aller R. C., Blair N. E. (1996). Sulfur diagenesis and burial on the Amazon shelf: Major control by physical sedimentation processes. Geo-Mar. Lett. 16, 3–10. doi: 10.1007/BF01218830
Aller R. C., Madrid V., Chistoserdov A., Aller J. Y., Heilbrun C. (2010). Unsteady diagenetic processes and sulfur biogeochemistry in tropical deltaic muds: Implications for oceanic isotope cycles and the sedimentary record. Geochim. Cosmochim. Acta 74, 4671–4692. doi: 10.1016/j.gca.2010.05.008
Berner R. A. (1984). Sedimentary pyrite formation: An update. Geochim. Cosmochim. Acta 48, 605–615. doi: 10.1016/0016-7037(84)90089-9
Berner R. A., Raiswell R. (1984). C/S method for distinguishing freshwater from marine sedimentary rocks. Geology 12, 365–368. doi: 10.1130/0091-7613(1984)12<365:CMFDFF>2.0.CO;2
Bianchi T. S., Mitra S., McKee B. A. (2002). Sources of terrestrially-derived organic carbon in lower Mississippi river and Louisiana shelf sediments: Implications for differential sedimentation and transport at the coastal margin. Mar. Chem. 77, 211–223. doi: 10.1016/S0304-4203(01)00088-3
Blair N. E., Aller R. C. (2012). The fate of terrestrial organic carbon in the marine environment. Ann. Rev. Mar. Sci. 4, 401–423. doi: 10.1146/annurev-marine-120709-142717
Borowski W. S., Rodriguez N. M., Paull C. K., Ussler W. (2013). Are 34S-enriched authigenic sulfide minerals a proxy for elevated methane flux and gas hydrates in the geologic record? Mar. Petroleum Geol. 43, 381–395. doi: 10.1016/j.marpetgeo.2012.12.009
Canfield D. E. (2001). Biogeochemistry of sulfur isotopes. Rev. Mineral. Geochem. 43, 607–636. doi: 10.2138/gsrmg.43.1.607
Canfield D. E., Raiswell R., Westrich J. T., Reaves C. M., Berner R. A. (1986). The use of chromium reduction in the analysis of reduced inorganic sulfur in sediments and shales. Chem. Geol. 54, 149–155. doi: 10.1016/0009-2541(86)90078-1
Canfield D., Thamdrup B. (1994). The production of 34S-depleted sulfide during bacterial disproportionation of elemental sulfur. Science 266, 1973–1975. doi: 10.1126/science.11540246
Dai L., Li S., Yu J., Wang J., Peng B., Wu B., et al. (2021). Palynological evidence indicates the paleoclimate evolution in southeast China since late marine isotope stage 5. Quaternary Sci. Rev. 266, 106964. doi: 10.1016/j.quascirev.2021.106964
Dong J., Li A., Liu X., Wan S., Feng X., Lu J., et al. (2018). Sea-Level oscillations in the East China Sea and their implications for global seawater redistribution during 14.0–10.0 kyr BP. Palaeogeography Palaeoclimatol. Palaeoecol. 511, 298–308. doi: 10.1016/j.palaeo.2018.08.015
Dong J., Li A., Liu X., Wan S., Xu F., Shi X. (2020). Holocene Climate modulates mud supply, transport, and sedimentation on the East China Sea shelf. J. Geophysical Res.: Earth Surface 125:e2020JF005731. doi: 10.1029/2020JF005731
Dong J., Li A., Lu Z., Liu X., Wan S., Yan H., et al. (2021). Millennial-scale interaction between the East Asian winter monsoon and El niño-related tropical pacific precipitation in the Holocene. Palaeogeography Palaeoclimatol. Palaeoecol. 573:110442. doi: 10.1016/j.palaeo.2021.110442
Fakhraee M., Hancisse O., Canfield D. E., Crowe S. A., Katsev S. (2019). Proterozoic seawater sulfate scarcity and the evolution of ocean–atmosphere chemistry. Nat. Geosci. 12, 375–380. doi: 10.1038/s41561-019-0351-5
Fike D. A., Bradley A. S., Rose C. V. (2015). Rethinking the ancient sulfur cycle. Annu. Rev. Earth Planetary Sci. 43, 593–622. doi: 10.1146/annurev-earth-060313-054802
Fry B., Ruf W., Gest H., Hayes J. M. (1988). Sulfur isotope effects associated with oxidation of sulfide by O2 in aqueous solution. Chem. Geol.: Isotope Geosci. Section 73, 205–210. doi: 10.1016/0168-9622(88)90001-2
Geng X. (1982). Transgressions and regressions in the eastern China since the late pleistocene epoch. Acta Oceanol. Sin. 1, 234–247.
Ge C., Zhang W., Dong C., Dong Y., Bai X., Liu J., et al. (2015). Magnetic mineral diagenesis in the river-dominated inner shelf of the East China Sea, China. J. Geophysical Res.: Solid Earth 120, 4720–4733. doi: 10.1002/2015JB011952
Gomes M. L., Hurtgen M. T. (2013). Sulfur isotope systematics of a euxinic, low-sulfate lake: Evaluating the importance of the reservoir effect in modern and ancient oceans. Geology 41, 663–666. doi: 10.1130/G34187.1
Habicht K. S., Canfield D. E. (1997). Sulfur isotope fractionation during bacterial sulfate reduction in organic-rich sediments. Geochim. Cosmochim. Acta 61, 5351–5361. doi: 10.1016/S0016-7037(97)00311-6
Holmkvist L., Kamyshny A., Brüchert V., Ferdelman T. G., Jørgensen B. B. (2014). Sulfidization of lacustrine glacial clay upon Holocene marine transgression (Arkona basin, Baltic Sea). Geochim. Cosmochim. Acta 142, 75–94. doi: 10.1016/j.gca.2014.07.030
Houghton J., Scarponi D., Capraro L., Fike D. A. (2021). Impact of sedimentation, climate and sea level on marine sedimentary pyrite sulfur isotopes: Insights from the Valle di manche section (Lower-middle pleistocene, southern Italy). Palaeogeography Palaeoclimatol. Palaeoecol. 585, 110730. doi: 10.1016/j.palaeo.2021.110730
Huang K.-M., Lin A. (1995). The carbon-sulfide-iron relationship and sulfate reduction rate in the East China Sea continental shelf sediments. Geochem. J. 29, 301–315. doi: 10.2343/geochemj.29.301
Hu B., Li J., Zhao J., Wei H., Yin X., Li G., et al. (2014). Late Holocene elemental and isotopic carbon and nitrogen records from the East China Sea inner shelf: Implications for monsoon and upwelling. Mar. Chem. 162, 60–70. doi: 10.1016/j.marchem.2014.03.008
Jørgensen B. B. (1982). Mineralization of organic matter in the sea bed–the role of sulphate reduction. Nature 296, 643–645. doi: 10.1038/296643a0
Jørgensen B. B., Böttcher M. E., Lüschen H., Neretin L. N., Volkov I. I. (2004). Anaerobic methane oxidation and a deep H2S sink generate isotopically heavy sulfides in black Sea sediments. Geochim. Cosmochim. Acta 68, 2095–2118. doi: 10.1016/j.gca.2003.07.017
Kao S.-J., Hsu S.-C., Horng C.-S., Liu K.-K. (2004). Carbon-sulfur-iron relationships in the rapidly accumulating marine sediments off southwestern Taiwan. Geochem. Soc. Special Publ. 9, 441–457. doi: 10.1016/S1873-9881(04)80031-2
Lamb A. L., Wilson G. P., Leng M. J. (2006). A review of coastal palaeoclimate and relative sea-level reconstructions using δ13C and C/N ratios in organic material. Earth-Sci. Rev. 75, 29–57. doi: 10.1016/j.earscirev.2005.10.003
Lang X., Tang W., Ma H., Shen B. (2020). Local environmental variation obscures the interpretation of pyrite sulfur isotope records. Earth Planetary Sci. Lett. 533, 116056. doi: 10.1016/j.epsl.2019.116056
Leavitt W. D., Halevy I., Bradley A. S., Johnston D. T. (2013). Influence of sulfate reduction rates on the phanerozoic sulfur isotope record. Proc. Natl. Acad. Sci. 110, 11244–11249. doi: 10.1073/pnas.1218874110
Lin J., Dai L. (2012). Quaternary marine transgressions in eastern China. J. Palaeogeogr. 1, 105–125. doi: 10.3724/SP.J.1261.2012.00009
Lin S., Huang K.-M., Chen S.-K. (2002). Sulfate reduction and iron sulfide mineral formation in the southern East China Sea continental slope sediment. Deep Sea Res. Part I 49, 1837–1852. doi: 10.1016/S0967-0637(02)00092-4
Lin Z., Sun X., Lu Y., Strauss H., Xu L., Gong J., et al. (2017). The enrichment of heavy iron isotopes in authigenic pyrite as a possible indicator of sulfate-driven anaerobic oxidation of methane: Insights from the south China Sea. Chem. Geol. 449, 15–29. doi: 10.1016/j.chemgeo.2016.11.032
Lin Z., Sun X., Lu Y., Xu L., Gong J., Lu H., et al. (2016). Stable isotope patterns of coexisting pyrite and gypsum indicating variable methane flow at a seep site of the shenhu area, south China Sea. J. Asian Earth Sci. 123, 213–223. doi: 10.1016/j.jseaes.2016.04.007
Lisiecki L. E., Raymo M. E. (2005). A pliocene-pleistocene stack of 57 globally distributed benthic δ18O records. Paleoceanography 20, PA1003. doi: 10.1029/2004PA001071
Liu J., Antler G., Pellerin A., Izon G., Dohrmann I., Findlay A. J., et al. (2021a). Isotopically “heavy” pyrite in marine sediments due to high sedimentation rates and non-steady-state deposition. Geology 49, 816–821. doi: 10.1130/G48415.1
Liu X., Fike D., Li A., Dong J., Xu F., Zhuang G., et al. (2019). Pyrite sulfur isotopes constrained by sedimentation rates: Evidence from sediments on the East China Sea inner shelf since the late pleistocene. Chem. Geol. 505, 66–75. doi: 10.1016/j.chemgeo.2018.12.014
Liu X., Li A., Dong J., Lu J., Huang J., Wan S. (2018a). Provenance discrimination of sediments in the zhejiang-fujian mud belt, East China Sea: Implications for the development of the mud depocenter. J. Asian Earth Sci. 151, 1–15. doi: 10.1016/j.jseaes.2017.10.017
Liu X., Li A., Dong J., Zhuang G., Xu F., Wan S. (2018b). Nonevaporative origin for gypsum in mud sediments from the East China Sea shelf. Mar. Chem. 205, 90–97. doi: 10.1016/j.marchem.2018.08.009
Liu X., Li A., Fike D. A., Dong J., Xu F., Zhuang G., et al. (2020b). Environmental evolution of the East China Sea inner shelf and its constraints on pyrite sulfur contents and isotopes since the last deglaciation. Mar. Geol. 429:106307. doi: 10.1016/j.margeo.2020.106307
Liu J. P., Li A. C., Xu K. H., Velozzi D. M., Yang Z. S., Milliman J. D., et al. (2006). Sedimentary features of the Yangtze river-derived along-shelf clinoform deposit in the East China Sea. Cont. Shelf Res. 26, 2141–2156. doi: 10.1016/j.csr.2006.07.013
Liu J., Pellerin A., Antler G., Izon G., Findlay A. J., Røy H., et al. (2021b). Early diagenesis of sulfur in bornholm basin sediments: The role of upward diffusion of isotopically “heavy” sulfide. Geochim. Cosmochim. Acta 313, 359–377. doi: 10.1016/j.gca.2021.08.018
Liu J., Pellerin A., Antler G., Kasten S., Findlay A. J., Dohrmann I., et al. (2020a). Early diagenesis of iron and sulfur in bornholm basin sediments: The role of near-surface pyrite formation. Geochim. Cosmochim. Acta 284, 43–60. doi: 10.1016/j.gca.2020.06.003
Liu J. P., Xu K. H., Li A. C., Milliman J. D., Velozzi D. M., Xiao S. B., et al. (2007). Flux and fate of Yangtze river sediment delivered to the East China Sea. Geomorphology 85, 208–224. doi: 10.1016/j.geomorph.2006.03.023
Liu X., Zhang M., Li A., Dong J., Zhang K., Gu Y., et al. (2022). Sedimentary pyrites and C/S ratios of mud sediments on the East China Sea inner shelf indicate late pleistocene-Holocene environmental evolution. Mar. Geol. 450:106854. doi: 10.1016/j.margeo.2022.106854
Liu X., Zhang M., Li A., Fan D., Dong J., Jiao C., et al. (2021c). Depositional control on carbon and sulfur preservation onshore and offshore the oujiang estuary: Implications for the C/S ratio as a salinity indicator. Cont. Shelf Res. 227, 104510. doi: 10.1016/j.csr.2021.104510
Lyons T. W., Berner R. A. (1992). Carbon-sulfur-iron systematics of the uppermost deep-water sediments of the black Sea. Chem. Geol. 99, 1–27. doi: 10.1016/0009-2541(92)90028-4
Meyers P. A. (1994). Preservation of elemental and isotopic source identification of sedimentary organic matter. Chem. Geol. 114, 289–302. doi: 10.1016/0009-2541(94)90059-0
Meyers P. A. (1997). Organic geochemical proxies of paleoceanographic, paleolimnologic, and paleoclimatic processes. Organic Geochem. 27, 213–250. doi: 10.1016/S0146-6380(97)00049-1
Miao X., Feng X., Liu X., Li J., Wei J. (2021). Effects of methane seepage activity on the morphology and geochemistry of authigenic pyrite. Mar. Petroleum Geol. 133, 105231. doi: 10.1016/j.marpetgeo.2021.105231
Morse J. W., Emeis K. C. (1990). Controls on C/S ratios in hemipelagic upwelling sediments. Am. J. Sci. 290, 1117–1135. doi: 10.2475/ajs.290.10.1117
Pasquier V., Sansjofre P., Rabineau M., Revillon S., Houghton J., Fike D. A. (2017). Pyrite sulfur isotopes reveal glacial-interglacial environmental changes. Proc. Natl. Acad. Sci. 114, 5941–5945. doi: 10.1073/pnas.1618245114
Peketi A., Mazumdar A., Joshi R. K., Patil D. J., Srinivas P. L., Dayal A. M. (2012). Tracing the paleo sulfate-methane transition zones and H2S seepage events in marine sediments: An application of c-S-Mo systematics. Geochem. Geophys. Geosystems 13:Q1007. doi: 10.1029/2012GC004288
Raiswell R., Canfield D. E. (2012). The iron biogeochemical cycle past and present. Geochem. Perspect. 1, 1–220. doi: 10.7185/geochempersp.1.1
Rickard D. (2012). Microbial sulfide oxidation in sediments Developments in Sedimentology. 65, 353–372. doi: 10.1016/B978-0-444-52989-3.00009-X
Sampei Y., Matsumoto E. (2001). C/N ratios in a sediment core from nakaumi lagoon, southwest Japan: Usefulness as an organic source indicator. Geochem. J. 35, 189–205. doi: 10.2343/geochemj.35.189
Sampei Y., Matsumoto E., Kamei T., Tokuoka T. (1997). Sulfur and organic carbon relationship in sediments from coastal brackish lakes in the shimane peninsula district, southwest Japan. Geochem. J. 31, 245–262. doi: 10.2343/geochemj.31.245
Shawar L., Halevy I., Said-Ahmad W., Feinstein S., Boyko V., Kamyshny A., et al. (2018). Dynamics of pyrite formation and organic matter sulfurization in organic-rich carbonate sediments. Geochim. Cosmochim. Acta 241, 219–239. doi: 10.1016/j.gca.2018.08.048
Sim M. S., Bosak T., Ono S. (2011). Large Sulfur isotope fractionation does not require disproportionation. Science 333, 74–77. doi: 10.1126/science.1205103
Sun D.-D., Liu P., Zhang J., Yu J.-J., Yue W., Wang J.-L., et al. (2022). Identification and significance of the late pleistocene transgressive strata in the bays if northern fujian province based on geochemical element indicators of sediments. J. Palaeogeogr. 24, 139–151. doi: 10.7605/gdlxb.2022.01.011
Tostevin R., Turchyn A. V., Farquhar J., Johnston D. T., Eldridge D. L., Bishop J. K. B., et al. (2014). Multiple sulfur isotope constraints on the modern sulfur cycle. Earth Planetary Sci. Lett. 396, 14–21. doi: 10.1016/j.epsl.2014.03.057
Waelbroeck C., Labeyrie L., Michel E., Duplessy J. C., McManus J. F., Lambeck K., et al. (2002). Sea-Level and deep water temperature changes derived from benthic foraminifera isotopic records. Quaternary Sci. Rev. 21, 295–305. doi: 10.1016/S0277-3791(01)00101-9
Wang J., Lin F., Peng B., Liu P., Zhang C., Jinxiu L. (2022). Temporal and spatial characteristics of quaternary stratigraphy in ningde area, fujian province and its indication of sea level changes. Geol. China. Available at: http://kns.cnki.net/kcms/detail/11.1167.P.20220314.20221914.20220008.html
Wang Z., Zhang J., Mei X., Chen X., Zhao L., Zhang Y., et al. (2020). The stratigraphy and depositional environments of china’s sea shelves since MIS5 (74-128) ka. Geol. China 47, 1370–1394. doi: 10.12029/gc20200506
Wei W., Algeo T. J. (2020). Elemental proxies for paleosalinity analysis of ancient shales and mudrocks. Geochim. Cosmochim. Acta 287, 341–366. doi: 10.1016/j.gca.2019.06.034
Wei G.-Y., Chen T., Poulton S. W., Lin Y.-B., He T., Shi X., et al. (2021). A chemical weathering control on the delivery of particulate iron to the continental shelf. Geochim. Cosmochim. Acta 308, 204–216. doi: 10.1016/j.gca.2021.05.058
Westrich J. T., Berner R. A. (1984). The role of sedimentary organic matter in bacterial sulfate reduction: The G model tested. Limnol. Oceanogr. 29, 236–249. doi: 10.4319/lo.1984.29.2.0236
Wu B., Lin F., Zhang Y., Bo P., Wang J. (2022). Distribution characteristics and genesis analysis of submarine shallow gas in ningde coastal area, fujian province. East China Geol. 43, 87–93. doi: 10.16788/j.hddz.32-1865/P.2022.01.009
Yu J., Peng B., Lan Y., Bin W., Jilong W., Dalin D., et al. (2021). Palynological record revealed anthropogenic deforestation, Sea level and climate changes since marine isotope stage 5a in the northeastern coast of fujian province. Earth Sci. 46, 281–292. doi: 10.3799/dqkx.2019.264
Yu F., Zong Y., Lloyd J. M., Leng M. J., Switzer A. D., Yim W. W. S., et al. (2011). Mid-Holocene variability of the East Asian monsoon based on bulk organic δ13C and C/N records from the pearl river estuary, southern China. Holocene 22, 705–715. doi: 10.1177/0959683611417740
Zhang K., Li A., Huang P., Lu J., Liu X., Zhang J. (2019). Sedimentary responses to the cross-shelf transport of terrigenous material on the East China Sea continental shelf. Sedimentary Geol. 384, 50–59. doi: 10.1016/j.sedgeo.2019.03.006
Zhang K., Li A., Liu X., Chen M.-T., Lu J., Zhang J., et al. (2021). Heavy mineral record from the east China sea inner shelf: Implications for provenance and climate changes over the past 1500 years. Cont. Shelf Res. 226, 104488. doi: 10.1016/j.csr.2021.104488
Zhan Q., Wang Z., Xie Y., Xie J., He Z. (2011). Assessing C/N and δ13C as indicators of Holocene sea level and freshwater discharge changes in the subaqueous Yangtze delta, China. Holocene 22, 697–704. doi: 10.1177/0959683611423685
Zhao B., Wang Z., Chen J., Chen Z. (2008). Marine sediment records and relative sea level change during late pleistocene in the changjiang delta area and adjacent continental shelf. Quaternary Int. 186, 164–172. doi: 10.1016/j.quaint.2007.08.006
Zhao B., Yao P., Bianchi T. S., Arellano A. R., Wang X., Yang J., et al. (2018). The remineralization of sedimentary organic carbon in different sedimentary regimes of the yellow and East China seas. Chem. Geol. 495, 104–117. doi: 10.1016/j.chemgeo.2018.08.012
Keywords: pyrite, sulfur isotope, organic carbon, sedimentary environment, East China Sea
Citation: Chang X, Liu X, Wang H, Zhuang G, Ma Z, Yu J and Chen J (2022) Depositional control on the sulfur content and isotope of sedimentary pyrite from the southeast coast of China since MIS5. Front. Mar. Sci. 9:1005663. doi: 10.3389/fmars.2022.1005663
Received: 28 July 2022; Accepted: 22 August 2022;
Published: 08 September 2022.
Edited by:
Zhiyong Lin, University of Hamburg, GermanyReviewed by:
Dong Feng, Shanghai Ocean University, ChinaJiarui Liu, University of California, Los Angeles, United States
Copyright © 2022 Chang, Liu, Wang, Zhuang, Ma, Yu and Chen. This is an open-access article distributed under the terms of the Creative Commons Attribution License (CC BY). The use, distribution or reproduction in other forums is permitted, provided the original author(s) and the copyright owner(s) are credited and that the original publication in this journal is cited, in accordance with accepted academic practice. No use, distribution or reproduction is permitted which does not comply with these terms.
*Correspondence: Xiting Liu, bGl1eGl0aW5nQG91Yy5lZHUuY24=; Jing Chen, amNoZW5AZ2VvLmVjbnUuZWR1LmNu