- 1Department of Marine Zoology, Senckenberg Research Institute and Natural History Museum, Frankfurt, Germany
- 2Institute for Ecology, Diversity and Evolution, Goethe University Frankfurt, Frankfurt, Germany
- 3Senckenberg Biodiversity and Climate Research Centre, Frankfurt, Germany
- 4Biodiversity and Biocomplexity Unit, Okinawa Institute of Science and Technology Graduate University, Okinawa, Japan
Establishing management programs to preserve the benthic communities along the NW Pacific and the Arctic Ocean (AO) requires a deep understanding of the composition of communities and their responses to environmental stressors. In this study, we thus examine patterns of benthic community composition and patterns of species richness along the NW Pacific and Arctic Seas and investigate the most important environmental drivers of those patterns. Overall we found a trend of decreasing species richness toward higher latitudes and deeper waters, peaking in coastal waters of the eastern Philippines. The most dominant taxa along the entire study area were Arthropoda, Mollusca, Cnidaria, Echinodermata, and Annelida. We found that depth, not temperature, was the main driver of community composition along the NW Pacific and neighboring Arctic Seas. Depth has been previously suggested as a factor driving species distribution in benthic fauna. Following depth, the most influential environmental drivers of community composition along the NW Pacific and the Arctic Ocean were silicate, light, and currents. For example, silicate in Hexactinellida, Holothuroidea, and Ophiuroidea; and light in Cephalopoda and Gymnolaemata had the highest correlations with community composition. In this study, based on a combination of new samples and open-access data, we show that different benthic communities might respond differently to future climatic changes based on their taxon-specific biological, physiological, and ecological characteristics. International conservation efforts and habitat preservation should take an adaptive approach and apply measures that take the differences among benthic communities in responding to future climate change into account. This facilitates implementing appropriate conservation management strategies and sustainable utilization of the NW Pacific and Arctic marine ecosystems.
Introduction
Understanding trends in community composition and species richness and how different taxa respond to the environmental stressors in shaping those trends provides fundamental information for species and habitat management systems. In the last two decades, many studies reported a decline in species biodiversity toward higher latitudes and deeper areas of the ocean with lower-slope peaks (Rex et al., 2005a; Rex and Etter, 2010; Costello and Chaudhary, 2017; Jöst et al., 2019; Saeedi et al., 2019a,b, 2020), although some studies have shown opposite trends (Brandt et al., 2007a,b). In the framework of the Census of the Diversity of Abyssal Marine Life (CeDAMar), several expeditions documented that deep-sea biodiversity is very unevenly distributed in different oceans and ocean basins (Ebbe et al., 2010; Ramirez-Llodra et al., 2010). For example, species diversity in some deep-sea gastropods and nematodes increases from the continental shelf to the bathyal and abyssal zones due to increased environmental stability (Rex, 1973; Danovaro et al., 2010; Rex and Etter, 2010). However, the high species richness of some taxa in some regions might be an artifact of biases in sampling and taxonomic effort (Brandt et al., 2007a; Canonico et al., 2019; Reimer et al., 2019; Saeedi et al., 2019c). Here, we examine the patterns of benthic community composition and species richness along the NW Pacific and the AO, and document how these patterns are correlated with a suite of environmental variables.
The NW Pacific includes massive areas of continental shelves and deep-sea basins diverging in depth, hydrology, and isolation, and, thus, is one of the most productive and species-rich regions of the World Ocean (Grebmeier et al., 2006; Renema et al., 2008; Sanciangco et al., 2013; Saeedi and Brandt, 2020a; Saeedi et al., 2020). The Sea of Japan, the warm temperate zone of the NW Pacific, and the Kuril-Kamchatka Trench (KKT) receives two major currents; the warm Tsushima and cold Oyashio currents (OC). The OC then flows southward through Japanese waters from off Hokkaido along the Pacific coast (Su et al., 1990; Fujikura et al., 2010; Saeedi and Brandt, 2020a; Figure 1). The northern parts of the NW Pacific are adjacent to some of the Arctic Seas such as the northern Bering and southern Chukchi Seas, which are also highly productive regions due to the northward transport of the nutrient-rich Pacific water overflowing the shallow shelf areas (layers above 200 m) toward the Arctic Ocean (AO) (Grebmeier et al., 2006).
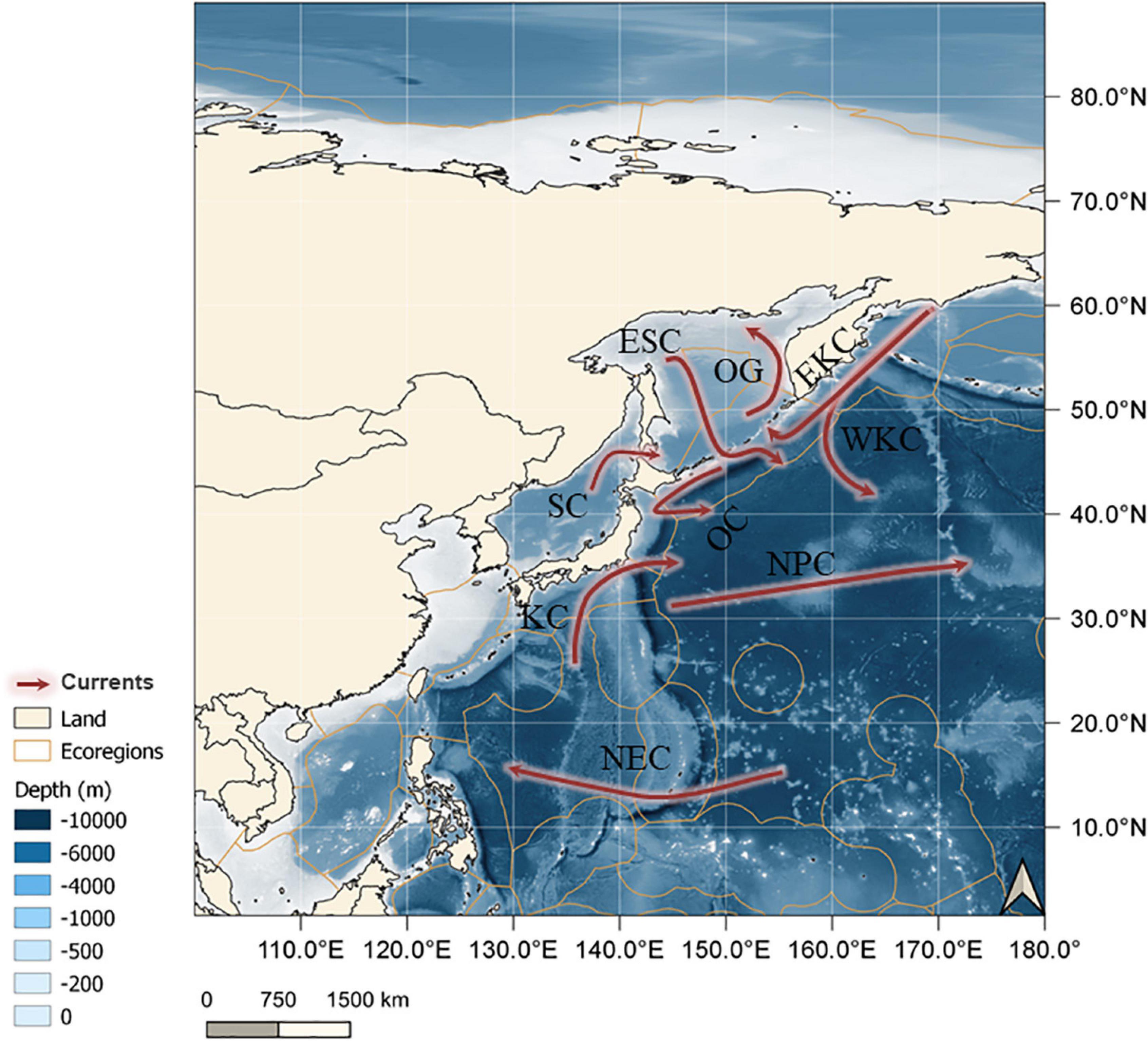
Figure 1. Study area including the marine ecoregions of the world (orange polygons) (MEOW) (Spalding et al., 2007) and its major currents (red arrows). EKC, East Kuril Current; ESC, East Sakhalin Current; KC, Kuroshio Current; NPC, North Pacific Current; OC, Oyashio Current; OG, Okhotsk Gyre; SC, Soya Warm Current; WKC, West Kuril Current, NEC, Northern Equatorial Current. QGIS 3.8.3 was used to create this map.
Depth zonation of macrofauna in different NW Pacific basins reveals that arthropods, represented by Crustacea, are dominant throughout all geographic areas; however, the community compositions of the most frequent taxa (Crustacea, Polychaeta, Echinodermata, Mollusca, and Cnidaria) varies between different geographic areas such as the Sea of Okhotsk, Sea of Japan, and Kuril-Kamchatka Trench (Brandt et al., 2019). Similarities in species composition have previously been documented between the isolated deep-sea basins of the marginal seas such as the Sea of Japan and the Sea of Okhotsk as well as the open NW Pacific (Kussakin, 1979; Tyler, 2002). As a general pattern, community composition of benthic fauna of the temperate NW Pacific shows more similarities to the Arctic Seas compared to the tropical and subtropical NW Pacific (Saeedi et al., 2019d).
Howard Sanders (Sanders, 1968; Sanders and Hessler, 1969) was the first person who described high deep-sea biodiversity, based on box-corer samples off North America, which led him to postulate the “stability-time hypothesis,” (Sanders, 1968), meaning that the deep sea harbors high species richness due to environmental stability over millions of years. Later, short-term processes were discussed as biodiversity drivers by Dayton and Hessler (1972) who suggested that “biological disturbance” could increase biodiversity (Dayton and Hessler, 1972). These ideas have been further developed, and have also been included in the theory of “contemporaneous disequilibrium” by Grassle and Sanders (1973), which makes any imbalance in the ecosystem or habitat potentially responsible for biodiversity change. Later theories were based on this assumption, such as the “predation” or “competition” theories (Rex, 1976) or “productivity” theory (Rex, 1977; Smith and Stockley, 2005; Rex and Etter, 2010), which suggests that productivity drives biodiversity patterns and taxon composition in addition to geographic “isolation” (Levin et al., 2001).
The overall number of benthic species decreases with depth, which is probably due to the exponential decrease in organic carbon flux to the benthos with increasing depth and distance from productive coastal systems, which limits ecological and evolutionary opportunities in the abyss compared to the bathyal and continental shelves (Rex et al., 2005b). However, the underlying mechanisms causing these species richness patterns are highly complex.
Many environmental parameters such as temperature, dissolved oxygen, surface productivity, seasonality of productivity, salinity, and ice cover can all play a role in shaping large-scale biodiversity patterns, but their relative importance might depend on the ecological and physiological characteristics of taxa and the oceanographic context of their ecosystems (Yasuhara et al., 2012b; Saeedi et al., 2019b). These results suggest that multiple variables might be related to community structure in our study. Benthic community structure at the taxonomic level of phyla could also be linked to multiple chemical stressors such as organic enrichment and toxic contamination in marine ecosystems (Lenihan et al., 2003). Niche dynamics were also suggested as a driver of community composition in wood-fall benthic communities in the south Atlantic Ocean (Saeedi et al., 2019a). One also needs to bear in mind that some deep-water taxa such as peracarid assemblages might vary seasonally and interannually (Ashford et al., 2019; Di Franco et al., 2020). Bottom current regimes and speeds have also been introduced as important factors affecting the composition of deep-sea communities (Roy et al., 2014; Lacharité and Metaxas, 2018), where higher diversity was observed in coarser and more heterogeneous substrates of the Gulf of Maine (Lacharité and Metaxas, 2018). Sediment type and grain size are reported to have significant correlations with driving the benthic biomass and diversity (Cummings et al., 2010; Hansen et al., 2020), for example in peracarids (Ashcroft et al., 2011), amphipods, isopods, and cumaceans (Brandt et al., 2019).
Understanding the drivers of benthic community composition is crucial to planning conservation for the ecological future of these communities. Climate change is accelerating in the NW Pacific and the Polar regions, impacting the species richness and community structure of its own and its adjacent latitudes (Renaud et al., 2019; Saeedi and Brandt, 2020a; Simões et al., 2021). Projected future distributions of crustaceans distributed along the NW Pacific and the AO showed poleward shifts and a decline of species richness at mid-latitudes under climate change, while more frequent shifts between temperate to polar regions were recovered (Simões et al., 2021). Significant increasing trends in the meridional sea level gradient and local winds suggest an increased northward seawater volume transport over the benthic species hotspots (Waga et al., 2020). This event might have contributed to the expansion of subarctic taxa into the Pacific Arctic regions that might have a strong influence in restructuring the benthic ecosystem in this region in the future (Waga et al., 2020). It is, therefore, important to study community composition and its environmental drivers in both NW Pacific and the AO to better compare the distribution range changes of the benthic phyla. Al-Habahbeh et al. (2020) observed that Arctic coastal benthic communities show slow recovery rates, and abrupt shifts in community structure document the vulnerability of Arctic coastal ecosystems to perturbations and continued effects of climate (Al-Habahbeh et al., 2020). The are several reports of mass extinction of marine species at the end of the Permian Period (c. 251 Ma) (Rampino and Caldeira, 2005; Clarkson et al., 2015; Bonifácio et al., 2020). Given the known marine calcifier species that have become extinct over the past few centuries and millennia (Barnosky et al., 2011; Henehan et al., 2019), and the predictions of potential species’ extinction in the future as a result of global warming (Saeedi et al., 2017; Barroso et al., 2018), another mass extinction may be underway.
One of the urgent action items in preventing biodiversity loss is assessing the main drivers of biodiversity and species community composition changes for multiple taxa. In this study, we thus provide a fundamental assessment of the biodiversity status and community composition of shallow-water and deep-sea benthic fauna along the NW Pacific and the AO using our sampling and open-access data. We aim to 1- uncover the patterns of community composition and species richness of benthic fauna, and 2- determine the main environmental drivers of the benthic taxa-specific community composition along the NW Pacific and the AO. We hypothesize that 1- Arthropoda and Mollusca are the most dominant taxa in the study area, and 2- environmental drivers of benthic community composition patterns are taxon-specific, with depth and temperature being the most important factors in shaping those patterns. So far, these sorts of analyses have been done for a few taxa or local regions. Here, we include all the marine benthic taxa and analyze the relationship between environmental variables and community composition using redundancy analysis (RDA). The outcome of this research is crucial for planning immediate action to reduce relevant pressures, including climate change, safeguarding places of remaining abundance, and recovering depleted populations, habitats, and ecosystems elsewhere. This would be a historic milestone in humanity’s quest to achieve a globally sustainable future on the basis of the United Nations Sustainable Development Goal 14 (UN SDG 14 or “life below water”) that aims to “conserve and sustainably use the oceans, seas and marine resources for sustainable development” (Duarte et al., 2020). This is also crucial for future environmental management and conservation, especially in areas experiencing accelerated environmental change such as the Arctic regions (Renaud et al., 2019).
Methods
Data Collection and Quality Control
Biodiversity Data
The study area covers the NW Pacific and its adjacent Arctic Ocean from 0 to 90°N, and 100 to 180°E (Figure 1). We have compiled a comprehensive dataset by merging our sampling records from the deep sea (Saeedi et al., 2019e; Saeedi and Brandt, 2020b) with open-access data retrieved from the Ocean Biodiversity Information System (OBIS)1 and Global Biodiversity Information Facility (GBIF)2 (for citations of OBIS and GBIF datasets, please see Saeedi et al., 2019b). Our sampling data included the digitized data from our four benthic deep-sea expeditions to the NW Pacific including the Sea of Japan Biodiversity Study (SoJaBio, 2010) (Malyutina and Brandt, 2013), Kuril-Kamchatka Biodiversity Study (KuramBio I and II, 2012–2016) (Brandt and Malyutina, 2015), and the Sea of Okhotsk Biodiversity Study (SokhoBio, 2015; Malyutina et al., 2018). Benthic specimens were collected using standard gear including MUC (multicorer), GKG (giant box corer), EBS (epibenthic sledge) AGT (Agassiz Trawl), WT (bottom trawl) (Brandt and Malyutina, 2014), BC (box corer) (Brandt et al., 2010), and PN (plankton net) (Chernyshev and Polyakova, 2018) and were deployed following a standardized method (Brandt and Malyutina, 2014). We have categorized the retrieved data from OBIS and GBIF to pelagic and benthic species using a combination of primary literature and open accesses databases, and only the benthic species were included in the analyses. Moreover, all species were categorized into shallow-water (0–500 m) and deep-sea groups (>500 m). We followed the World Register of Deep Sea Species (WoRDSS)3 depth classification, as 500 m is a depth at which seasonal variation in physical parameters (e.g., temperature and salinity), as well as the influence of sunlight, becomes minimal. Species recorded below 500 m, but which are known to range above 500 m, were included in our dataset (as both shallow and deep sea) as they may contribute significantly to the ecology of the deep sea.
All geographic distribution records were then merged, checked for suitability, and dubious records were either corrected (e.g., reversing latitude and longitude fields, removing duplicate records) using the R package “robis” (Provoost, 2018). All the data related to fossils or records on land were removed. Species names were finally taxon-matched against the World Register of Marine Species (WoRMS, 2018)4, synonyms were reconciled, and only accepted taxa were selected for the analysis. The final dataset consisted of 18,668 distribution records of which 11,029 belonged to accepted benthic species from 0 to 10,900 m depth (Supplementary Figure 1).
Environmental Data
Benthic layers were extracted from Bio-ORACLE5 (Tyberghein et al., 2012; Assis et al., 2018) at a 5-arcmin (∼10 km) spatial resolution corresponding to the maximum depth including average temperature (°C), dissolved oxygen (mol.m––3), current velocity (m––1), salinity (PSS), nitrate (mol.m––3), silicate (mol.m––3), phosphate (mol.m––3), light at bottom, chlorophyll (mg.m––3), phytoplankton (umol.m––3), and primary productivity (g.m––3.day––1). The depth layer (m) was extracted from the General Bathymetric Chart of the Oceans.6
Data Analyses
Community Composition and Species Richness
To present all possible logical relations between community composition of different ecological zones including tropical, subtropical, temperate, and polar regions, we created a Venn diagram using a web-based tool, InteractVenn (Heberle et al., 2015). To characterize the latitudinal community composition and benthic species distribution range accumulations, we excluded duplicate latitudinal records for species within the same phylum, and created violin plots with the R package ggplot2 (Wickham, 2016), to represent the distributions of data estimated using a kernel density approach.
We have calculated (1) alpha (number of species per each Marine Ecoregions of the World (MEOW) (Spalding et al., 2007) and per equal-sized hexagons), (2) beta (cluster similarity analysis of alpha species richness corresponding to ecoregions), and (3) gamma species richness (total number of species per 5-degree latitudinal bands and per 100 m depth intervals) for the benthic community along the NW Pacific and the AO.
To obtain the number of species unique to each corresponding ecoregion and hexagonal cell (alpha diversity), we used the spatial join function in R packages sf (Pebesma et al., 2018) and tidyverse (Wickham, 2017), to intersect each species with both equal-sized hexagonal cells and with ecoregions according to coastal and shelf waters in the marine ecoregions of the world (MEOW) polygons (Spalding et al., 2007). We applied hierarchical cluster analysis using the vegan package in R (Oksanen et al., 2010, 2015) using Euclidean distance and group-average linkage as recommended for analysis of species composition, to compare the species richness between the ecoregions (beta diversity). Bootstrap support was obtained via ordinary bootstrap resampling and multiscale bootstrap resampling (AU) (Shimodaira, 2004) using the R package Pvclust (Suzuki et al., 2015). All the unique species were assigned to each 5-degree latitudinal band and 100 m depth interval (gamma diversity) and the results were plotted in R. Rarefaction was used to control for the effect of sampling effort on a number of reported species in each 5-degree latitudinal band by repeatedly counting the number of species in a constant number of simulated random samples. We considered each sample as a unique combination of date and location where one or more species were recorded. We used ES50 in vegan package R to estimate the species richness in 50 samples per 5-degree latitudinal band (Oksanen et al., 2017).
Redundancy Analysis
We analyzed the relationship between environmental variables and community composition using redundancy analysis (RDA) with the vegan R package (Oksanen et al., 2010, 2015). RDA regresses multiple response variables on multiple predictor variables and attempts to find linear relationships in the response variables that are correlated with relationships in the predictor variables. We restricted this analysis to species for which there was more than one observation, and to sites for which there were values for all environmental predictors. In order to account for the effects of spatial autocorrelation, we conducted a principal component of neighbor matrices (PCNM) analysis and used the output vectors from the PCNM analysis along with the environmental data as explanatory variables in the RDA analysis. Finally, we conducted a permutation-based ANOVA on the RDA results to measure what portion of the variance in community composition was explained by individual environmental predictors.
We performed one RDA analysis for all species together, and then a separate one for each class for which sufficient records were available to obtain a result. There were not enough records available to do these analyses at the lower levels than class. We retained only classes for which at least 10 species had been sampled, but among these several of the less well-sampled classes did not produce results due to failure of the RDA algorithm.
Results
Benthic Community Composition
Temperate latitudinal bands of the NW Pacific (36–54°) had the highest number of shared benthic species with four other geographic zones including tropical, subtropical, and northern and the southern polar regions (48 species) (Figure 2). Both northern and southern polar regions shared species with the temperate latitudes but had no common species with the tropical and subtropical regions. The other geographical zones had no benthic species in common. Looking at the middle of the diagram, there are no species that were shared between all geographic zones (0 species).
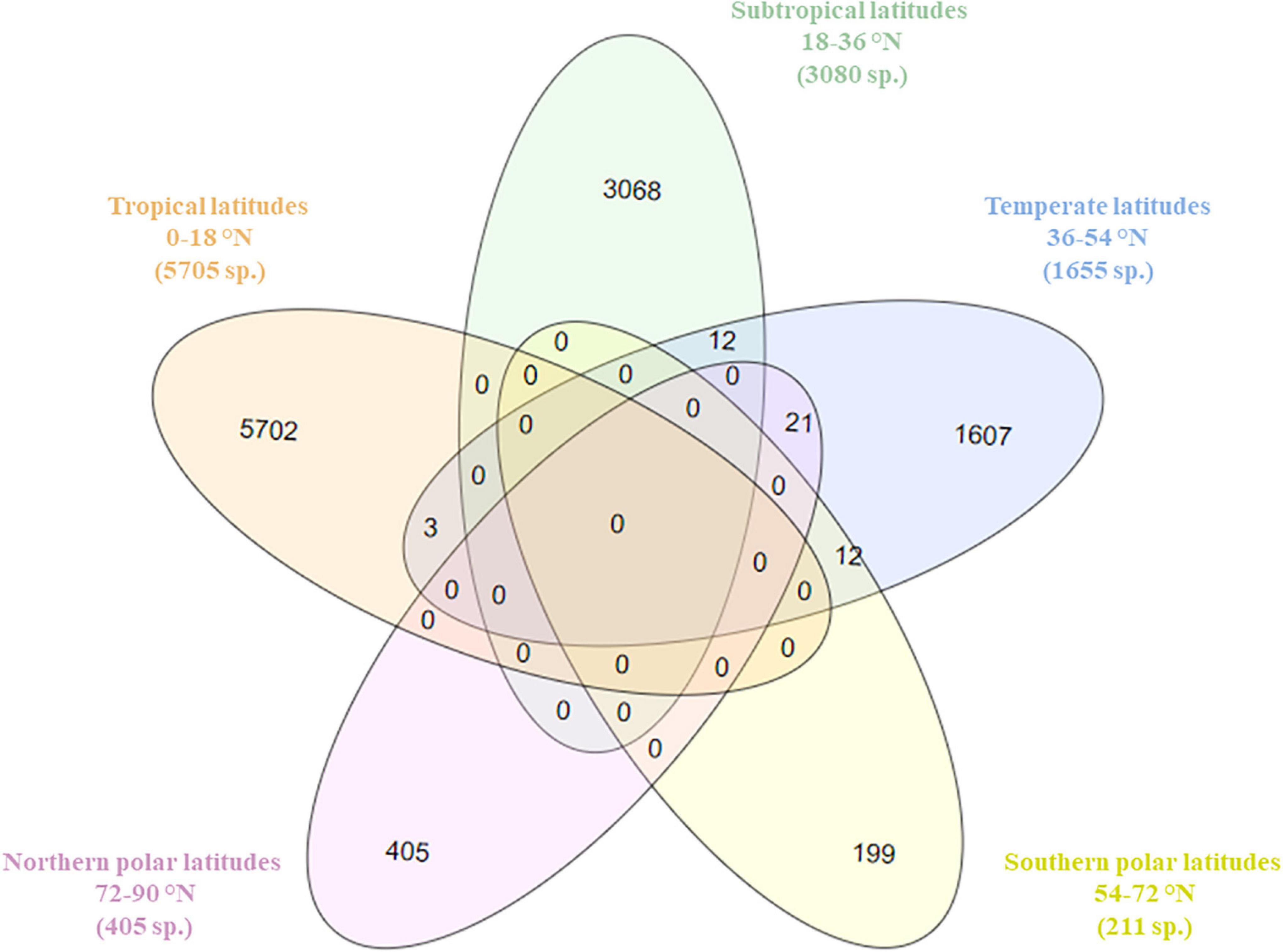
Figure 2. Venn diagram showing the possible relations of species composition (based on species presence/absence matrix per geographical zones) and five latitudinal zones, number of species shown in brackets. Different colors represent different geographical zones.
In most of the 5-degree latitudinal bands of the NW Pacific and the AO, the greatest number of species occurred in the phyla Arthropoda, Mollusca, Cnidaria, Echinodermata, and Annelida, comprising >50% of the total relative species composition in each 5-degree band (Figure 3). Latitudes 40–50°N had the highest number of phyla (20 phyla out of a total 24 phyla) of the NW Pacific (c. 83% of the total community composition). In the AO, Arthropoda and Annelida were the most dominant phyla followed by Mollusca.
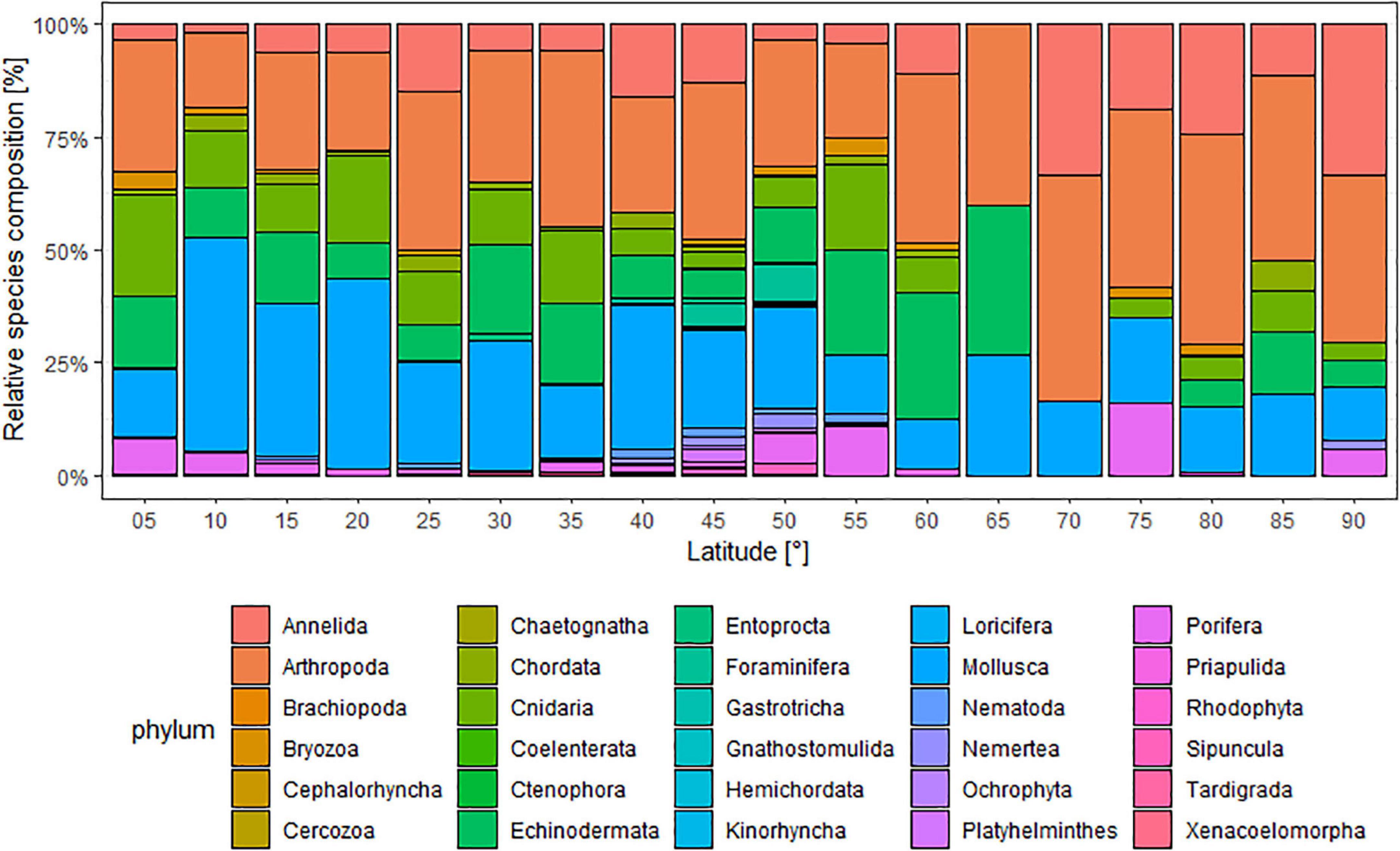
Figure 3. Relative abundance (%) of species composition of different phyla against 5-degree latitudinal bands. Different colors represent different phyla.
Most of the benthic phyla had representative species in both NW Pacific and the AO; however, some taxa such as Sipuncula or Kinorhyncha were not reported or/and the data were not published online for the AO (Figure 4). Also, no taxon was reported solely specifically to the AO. Two obvious species-density peaks are apparent in many taxa (e.g., Porifera, Cnidaria, Mollusca, Echinodermata, Chordata, and Bryozoa); one at latitudes 5–10°N and the other one at latitudes 30–50°N. More than half of the taxa had their average distribution ranges from 40 to 50°N.
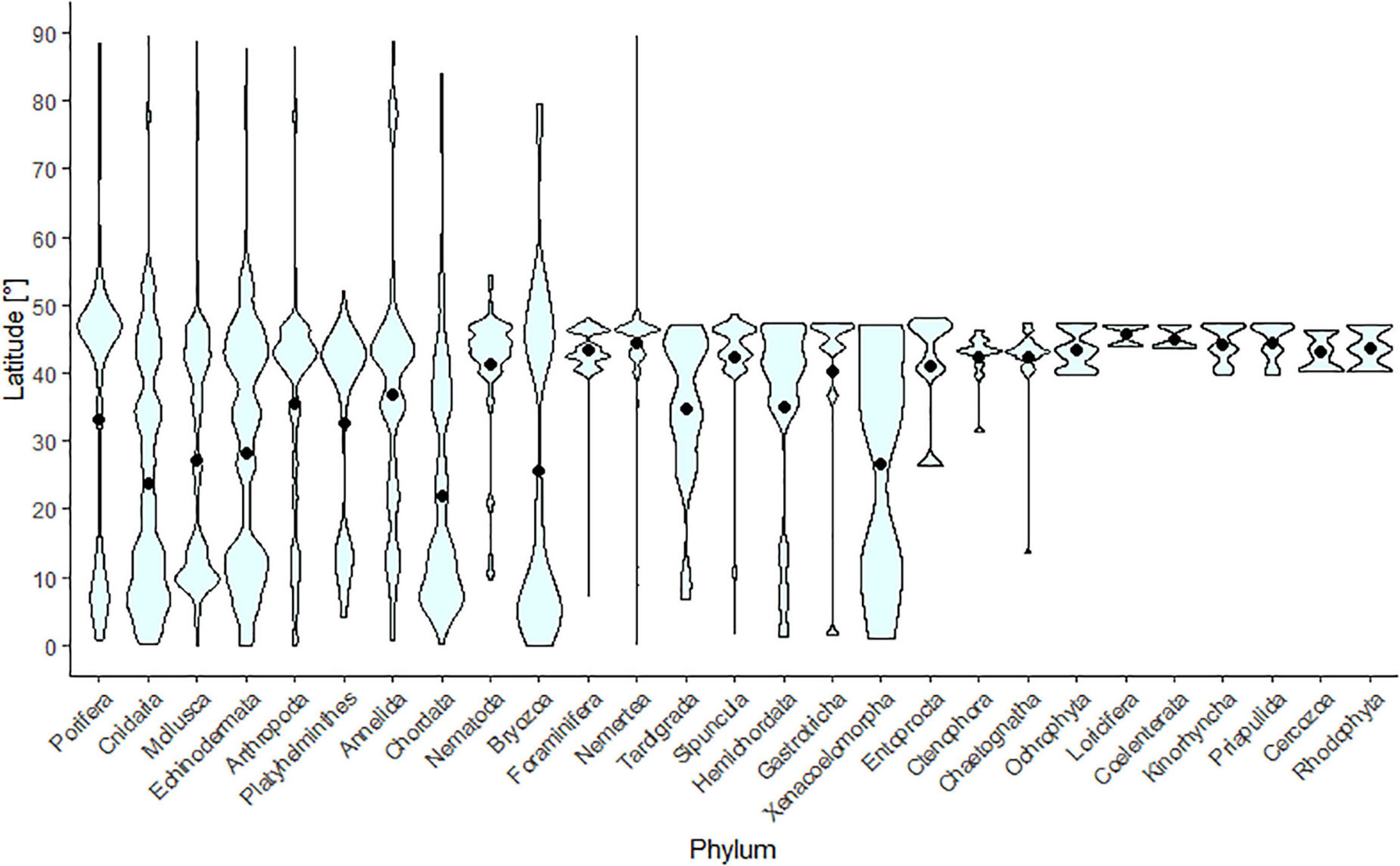
Figure 4. Violin plot representing the latitudinal distribution density range of benthic species per phylum. The black dots show the average latitude of records for each phylum. In general, there are fewer data available in latitudes above 50°N for some taxa presented here (Supplementary Figure 2).
Species Richness (Alpha, Beta, and Gamma)
The number of reported distribution records per equal-sized hexagonal cell (sampling effort) were highest in latitudes between 5 and 15°N around the Oyashio Current, and between 35 and 50°N around the eastern Philippines (c. 2,000–4,000 records) (Figure 5). However, the number of species per equal-sized hexagonal cell (alpha species richness) peaked only in the eastern Philippines with more than 3,000 species. Sampling effort or open-access data in Arctic seas were lower than the NW Pacific sea basins. For example, the East Siberian Sea had less than 200 distribution records belonging to 56 species. The Laptev Sea had more available data than the Eastern Siberian Sea.
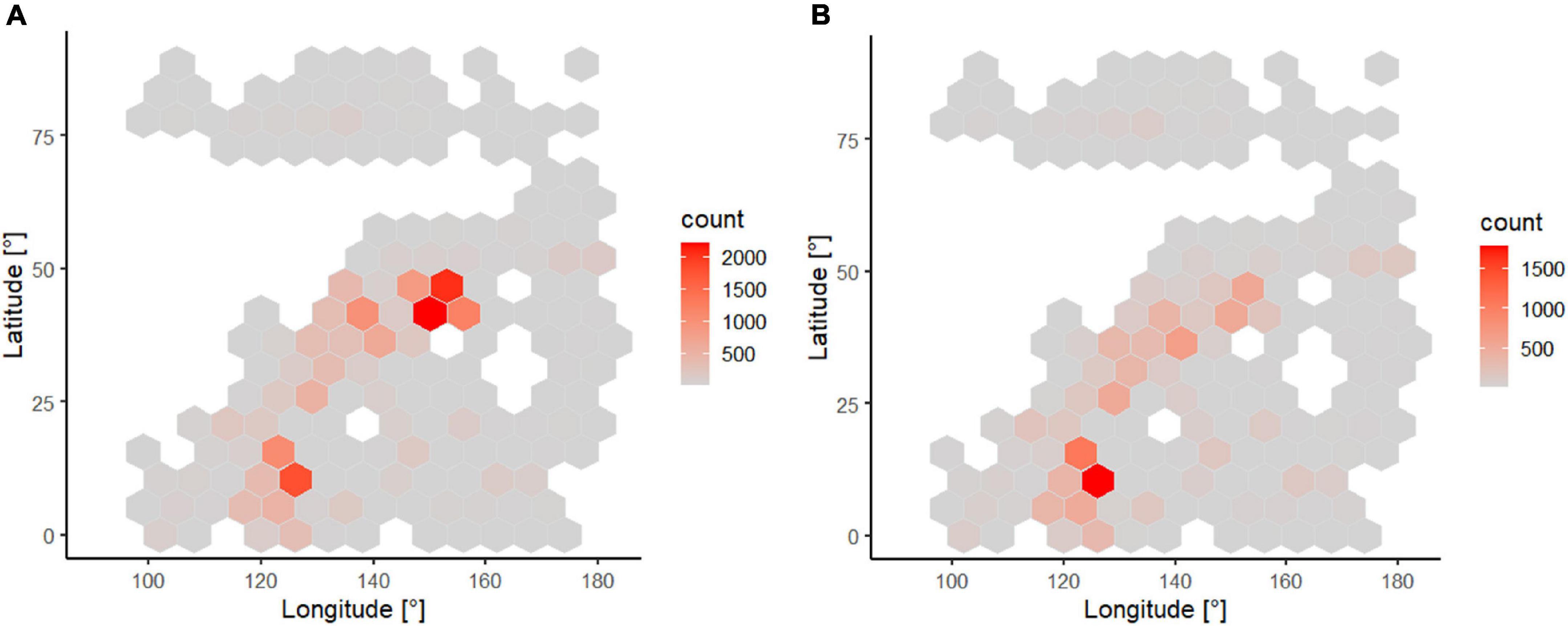
Figure 5. The number of reported distribution records (sampling effort) (A) and the number of species (alpha species richness) (B) per c. 600,000 km2 equal-sized hexagonal cells. R package hexbin (Carr et al., 2011) and ggplot2 (Wickham, 2016) were used to create this figure. Color code refers to lowest (gray) to highest (red) values.
The cluster analysis of species presence/absence per ecoregion (beta species richness) supported 10 distinct geographic clusters (AU > 0.70), which corresponded to marine provinces of MEOW including 1. Western coral triangle, 2. Cold temperate NW Pacific, 3. Warm temperate NW Pacific, 4. Tropical NW Pacific, 5. South Kuroshio, 6. The South China Sea, 7. Arctic, 8. Cold temperate NW Pacific, 9. Marshall Gilbert and Ellis Islands, and 10. Sunda Shelf (Figure 6).
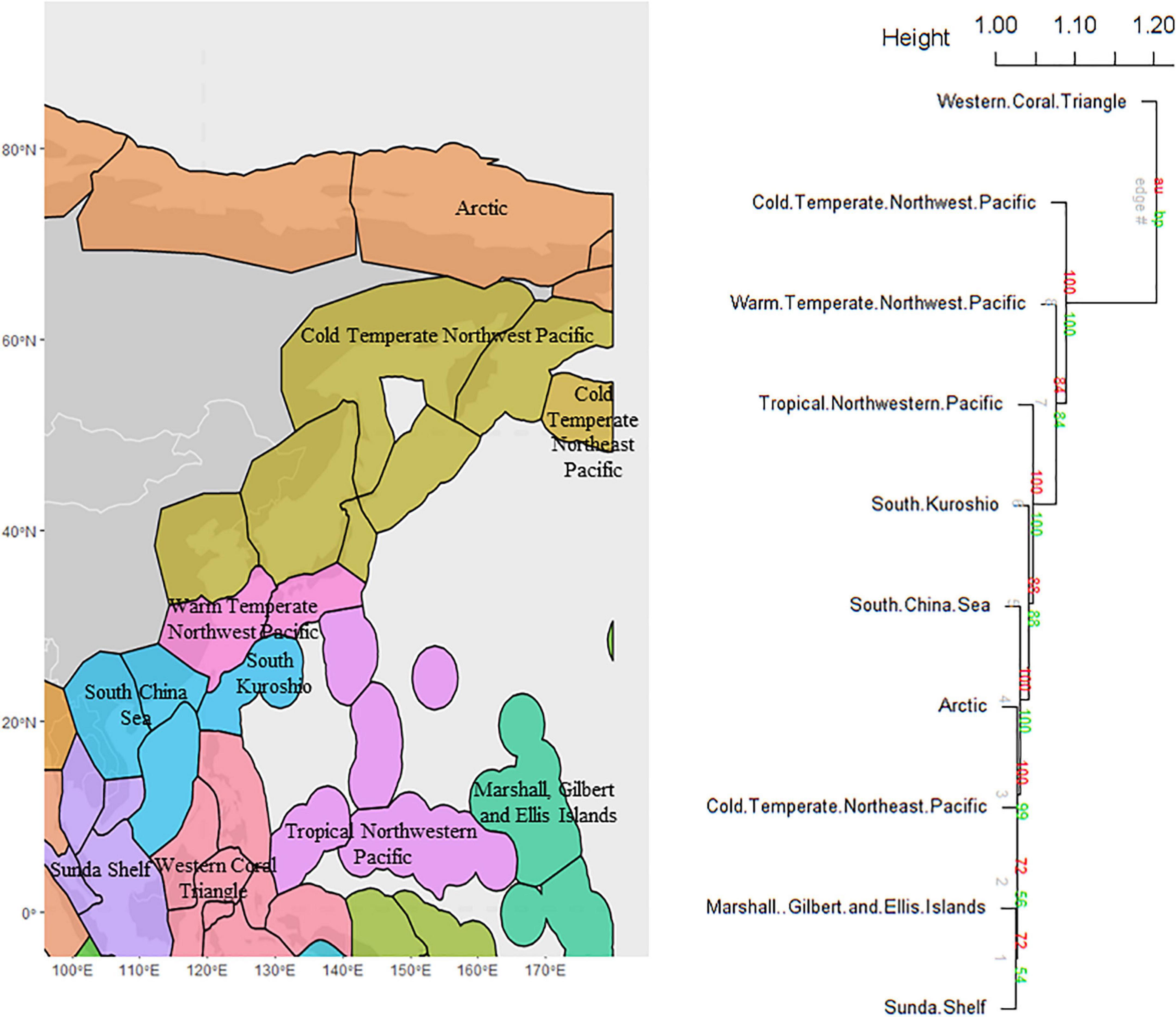
Figure 6. Cluster dendrogram of benthic fauna in ecoregions of the NW Pacific and the Arctic Ocean. The numbers above each edge on the dendrogram show the probability of nodes below that edge occurring as a cluster in resampled trees, via ordinary bootstrap resampling (BP, green) or multiscale bootstrap resampling (AU, red). Ten significantly different Marine Ecoregions of the World (MEOW) (Spalding et al., 2007) corresponded to the marine provinces shown on the map in different colors.
Latitudinal and bathymetric benthic species richness decreased toward the higher latitudes and deeper areas according to the data available for this study (Supplementary Figures 2–6). Number of distribution records per 5-degree latitudinal band and per 100 m depth interval were highest in latitudes from 40 to 50°N and depths above 500 m, with most of the records belonging to Arthropoda followed by Mollusca, Echinodermata, and Annelida (Supplementary Figures 2, 4); but the numbers of species per 5-degree latitudinal band and per 100 m depth interval (gamma species richness) were higher in latitudes between 5 and 10°N and depths above 500 m, mostly belonging to species of Mollusca and Arthropoda (Supplementary Figures 3, 5).
When the expected number of species was estimated accounting for sample size, latitudes 45 and 50°N showed the lowest species richness in relation to their high sample size, compared to the other latitudes (Supplementary Figure 7). Latitudes 5 and 10°N; however, had the highest number of expected species even after accounting for their sampling size.
Community Composition Response to Environmental Drivers
The correlation among different environmental variables is summarized in Supplementary Figure 8. For all classes taken together, 47.3% of the variance in species occurrence patterns was explained by the RDA, with 45.8% being explained by spatial autocorrelation alone (Figure 7 and Supplementary Material). Although only 1.5% of the variance was explained by environmental predictors, some of those relationships were nonetheless highly significant (p < 0.001 for depth, p < 0.01 for silicate, and p < 0.05 for dissolved oxygen content). For classes analyzed separately, the results were broadly concordant with those for the combined RDA; approximately 45–50% of the variance was explained by the analyses, with most of that being due to spatial autocorrelation (Supplementary Material). Of the environmental predictors found to be significantly correlated with patterns of benthic species occurrence and community composition, depth was the most reliable predictor in about half of the classes (20 classes out of 41 examined classes) (Supplementary Table 1). Silicate, light, currents, dissolved oxygen, phosphate, and primary productivity were important for many classes as well (Figure 8 and Supplementary Table 1).
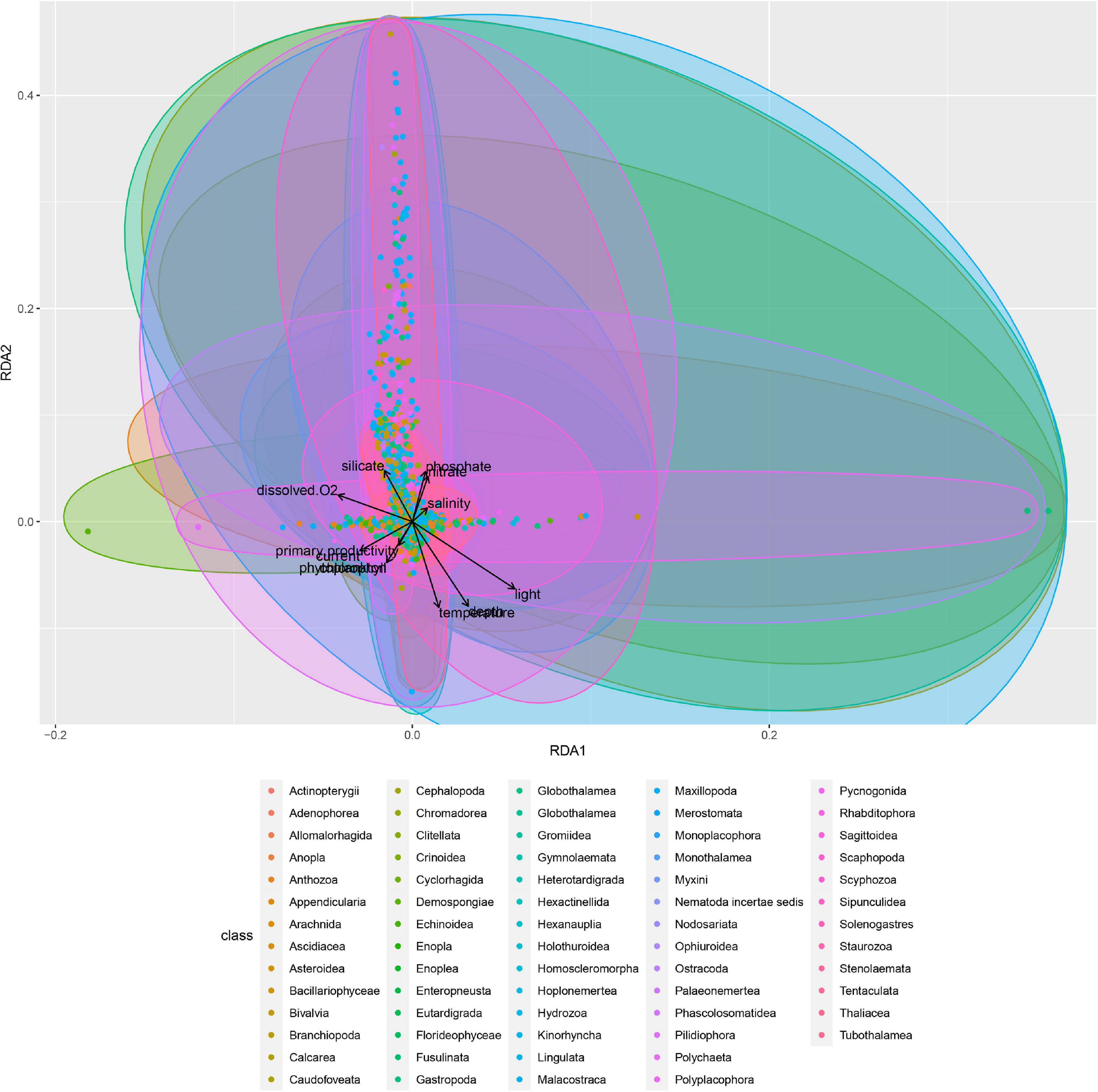
Figure 7. Redundancy analysis (RDA) of all studied classes. Different point colors represent different classes (unique species of each class are shown with the same color per class). For variation explained by each axis and all the RDA analyses and R scripts, please see the Supplementary Material.
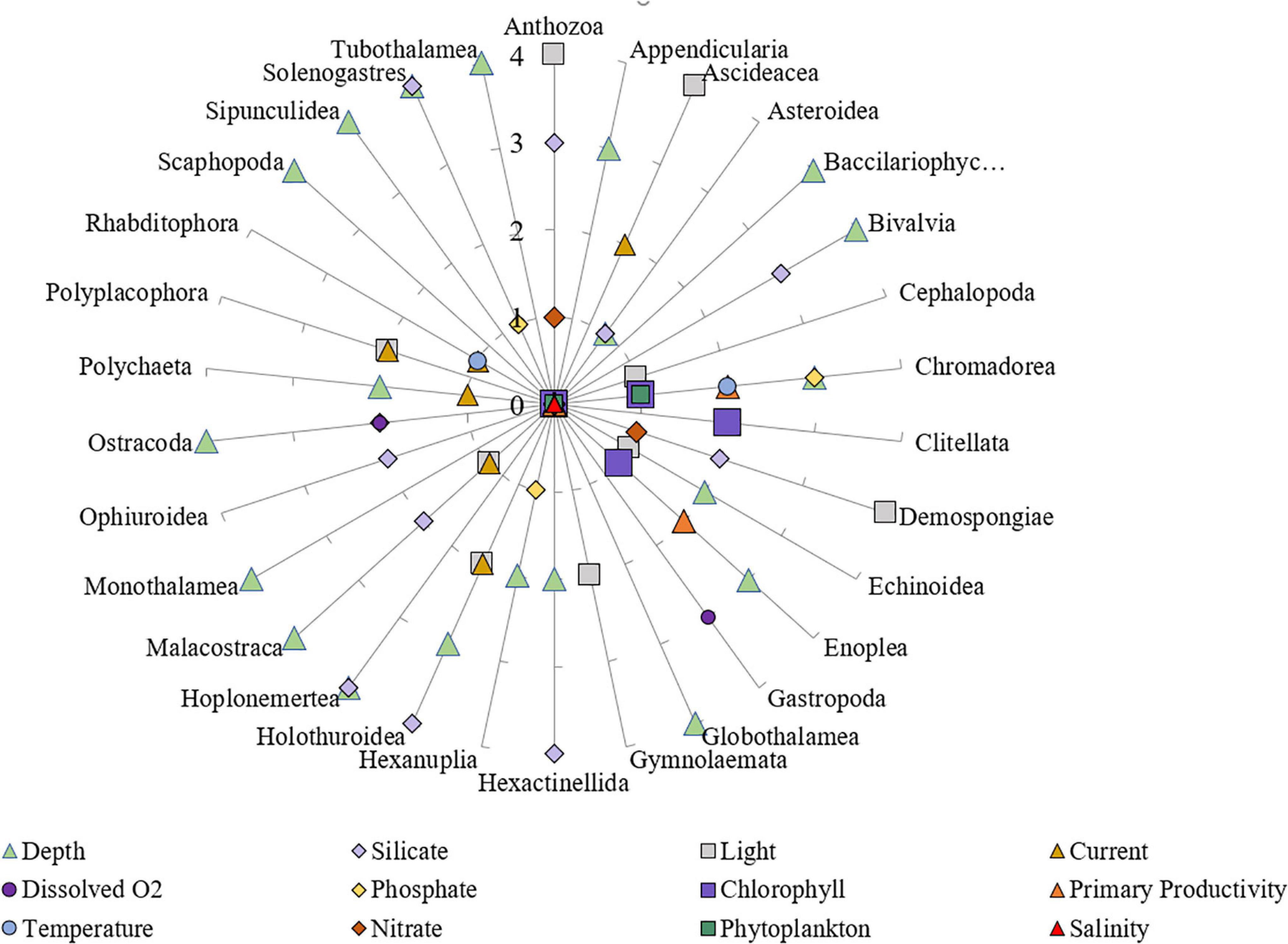
Figure 8. The significance level of importance of environmental variables in determining the species community composition was ranked from 1 to 4 for each class; p < 0.001 = 4; p < 0.01 = 3; p < 0.05 = 2; p < 0.1 = 1 and are summarized in this figure. Different environmental variables are shown with different symbols and colors.
Depth was highly correlated with the composition of classes such as Bivalvia and Scaphopoda (Mollusca), and Malacostraca and Ostracoda (Arthropoda). Silicate took over depth in importance in many classes such as Hexactinellida (Porifera), Holothuroidea and Ophiuroidea (Echinodermata). In some classes such as Cephalopoda (Mollusca), and Gymnolaemata (Bryozoa), only light was correlated with class composition; however, in other classes such as Chromadorea and Enoplea (worms), many variables such as depth, phosphate, primary productivity, temperature and other variables were significantly correlated with class composition patterns. Salinity was not a significantly important variable in determining the composition in any classes. Other environmental variables such as phytoplankton, nitrate, and temperature were not also significantly correlated with the class composition of the examined taxa.
Discussion
In this study, we hypothesized that Arthropoda and Mollusca are the most dominant taxa along the NW Pacific and the AO, and our results confirmed this hypothesis. We also hypothesized that community composition within each taxon responds differently to environmental variables, but that depth and temperature are the most important drivers of the community composition patterns overall. Our results partially confirmed our second hypothesis; different environmental variables drove composition in different taxa, with depth being the dominant driver in most taxa. However, part of our second hypothesis was rejected, as the temperature was not an important factor in shaping the community compositions of most taxa. Following depth, the most influential environmental drivers of benthic community composition along the NW Pacific and the AO were silicate, light, and currents.
Marine benthic communities along the NW Pacific and Arctic latitudes showed distinct latitudinal boundaries. Tropical latitudes (0–18°N) had the highest species richness compared to their neighboring latitudes. This might be explained by differences in sampling effort and available open access data along the Arctic seas compared to the NW Pacific. There were only three species that were shared between the tropical and subtropical latitudes in this study. However, temperate latitudes had the highest number of species shared with the subtropical and Arctic latitudes. This might be explained by range expansions of the subtropical species toward the higher temperate latitudes following widespread and spatially-uniform temperatures within the tropics (Out of the Tropics Hypothesis) (Jablonski et al., 2006, 2013). Many narrow-ranging Arctic species might have evolved from NW Pacific temperate ancestors and inhabited the Arctic latitudes as a natural phenomenon (Briggs, 2003; Vermeij et al., 2019). North Pacific marine species probably evolved over a period of c. 40 Myr from warm−temperate and eurythermic tropical species that adapted to the colder temperatures; from the Miocene onward, the North Pacific species expanded their ranges to the adjacent Arctic Ocean through Bering Strait following an increase in productivity (Briggs, 2003; Vermeij et al., 2019). More interestingly, northern Arctic latitudes (72–90°N) shared more species with the warm-temperate NW Pacific latitudes (21 species) compared to the southern Arctic latitudes and colder temperate latitudes (12 species). This is most likely driven by the fact that less ocean area is available in latitudes from 60 to 70°N, which is also reflected in the reduced number of species in this area compared to the other latitudes. On the other hand, Arctic marine faunas accumulated through cycles of glaciation, leading to unique assemblages rather than being entirely derived from temperate latitudes following glaciation (Bringloe et al., 2020). Arctic marine species diversity and endemicity are potentially born from pressures induced by isolation during Cenozoic global cooling and glaciations (Bringloe et al., 2020).
Beta species richness (presence/absence of species per ecoregions) via similarity cluster analyses showed that there are 10 significantly different geographic regions including the Arctic, temperate NW Pacific, Central Indo-Pacific, and eastern Indo-Pacific realms. These 10 geographic regions were grouped based on distributions of the benthic community in an agreement with MEOW province classifications (Spalding et al., 2007). These findings support the suggested geographic distribution classification for both shallow-water and deep-sea fauna in our study area (Saeedi et al., 2019d), and for biodiversity in general based on taxonomic configurations, evolutionary history, patterns of dispersal, geographic isolation (Spalding et al., 2007; Briggs and Bowen, 2012), and available area (Antell et al., 2020). One should also keep in mind that our knowledge about the deep Arctic fauna is very limited as Gradinger et al. (2010) demonstrated that the biodiversity of the AO is better studied in nearshore areas than the deep-sea ridge systems that extend thousands of kilometers across the Arctic seafloor (Gradinger et al., 2010). Thus, our data from the adjacent Arctic Ocean were scarce compared to the NW Pacific, which has been intensively analyzed in the last decade following extensive sampling from the board of RV Vityaz in the last century as well as an international collaboration between German and Russian scientists (Brandt and Malyutina, 2015; Brandt et al., 2018, 2020; Saeedi et al., 2019b,d, 2020; Saeedi and Brandt, 2020a).
Annelida, followed by Arthropoda, Cnidaria, Echinodermata, and Mollusca were the most dominant taxa in the NW Pacific and the Arctic Ocean. Saeedi et al. (2019d), examining both pelagic and benthic fauna, also reported that these five taxonomic groups were the most abundant and dominant taxa in both shallow water and deep sea of the NW Pacific and the Arctic Ocean. In this study, we have added more than 1,500 deep-sea records to the dataset examined by Saeedi et al. (2019b), but still, the community structure remains similar to those reported earlier. Brandt et al. (2019) examined the depth zonation of the NW Pacific deep-sea macrofauna. They found the same results for all marine species examined here; Arthropoda represented by crustaceans were dominant throughout all geographic areas of the deep NW Pacific, followed by Annelida, Echinodermata, and Mollusca (Brandt et al., 2019). Crustacea are widely reported as one of the most dominant components of species richness in the deep-water macrobenthos of the NW Pacific (Birstein, 1971; Kussakin and Vasina, 1990; Kussakin, 1999, 2003; Brandt and Malyutina, 2015; Elsner et al., 2015; Golovan et al., 2018; Brandt et al., 2020) in addition to Polychaeta (Brandt et al., 2018) and Mollusca (Kamenev, 2019). Kamenev (2020) discovered that the Thyasiridae (Mollusca: Bivalvia) is the most species-rich family of bivalves in the abyssal and hadal zones of the NW Pacific; 14 species were recently found from depths exceeding 3,000 m with the deepest report between 9,000 and 9,583 m in the Kuril-Kamchatka Trench belonging to Axinulus roseus Kamenev, 2020 (Kamenev, 2020). Polychaetes, in general, are one of the most dominant and diverse groups of the macrofauna of the Pacific Ocean and play a major role in the functioning of benthic communities (Jumars et al., 2015; Bonifácio et al., 2020). The highest coral reef diversity reported from the Coral Triangle (Bellwood and Hughes, 2001; Renema et al., 2008) is also in agreement with our findings here.
Eastern Philippines, Palawan/North Borneo, Sulawesi Sea/Makassar Strait, Sea of Japan, Central Kuroshio Current, and Oyashio Current had the highest number of reported distribution records of benthic fauna; however, only Eastern Philippines, Sulawesi Sea/Makassar Strait, and Central Kuroshio Current had the highest species richness (alpha and gamma) among all the examined ecoregions. Even when ocean area was accounted for by dividing the ocean into equal-sized hexagons, the eastern Philippines still had more than 1,500 species in about 600,000 km2. The high species richness in the Philippines could still be partially related to higher sampling. This high species richness of the benthic fauna in the Philippines has been reported for many taxa [bivalves, corals, gastropods, and crustaceans and for all taxa overall (Bellwood and Hughes, 2001; Kerswell, 2006; Saeedi and Costello, 2012; Selig et al., 2014; Asaad et al., 2017)].
Sampling, taxonomy research, and data digitization efforts could also play an important role here in biasing the results (Reimer et al., 2019; Saeedi et al., 2019c). Contrary to the well-investigated NW Pacific (Ushakov, 1952; Zenkewitch et al., 1955; Zenkevitch, 1963), we have less knowledge about the AO fauna, especially its deep sea. The lack of knowledge of the species composition of many areas in the AO is preventing the adjacent countries from establishing adequate conservation plans, which are crucial in times of rapid climate change, e.g., expressed through temperature increase, sea-level rise, and potential methane emissions (Blasiak et al., 2020). The lack of publicly available biodiversity data for Arctic taxa is obvious (e.g., Figure 4), which documents that fewer data are available in latitudes above 50°N. For example, while open-access databases did not include Sipuncula, Echiura, Hemichordata, and Priapulida, publications document their presence from the Siberian Seas, Laptev Sea, Kara Sea, and central Arctic regions (Vedenin et al., 2021). However, some taxa such as Sipuncula are generally less diverse in the AO compared to the NW Pacific (Kędra et al., 2018). Renaud et al. (2019) documented “weakness of general statements regarding the vulnerability of taxa on biogeographic or presumed physiological grounds, and suggest that more basic biological data on Arctic taxa are needed for improved projections of ecosystem responses to climate change.” Benthic Biotopes in the Arctic and Sub-Arctic Norwegian Waters have been recently classified and mapped (Buhl-Mortensen et al., 2020); however, these authors also explain that knowledge about megafauna composition and biotopes is poor for deep-water benthic habitats in the Arctic region. There are some areas along the deep Nordic Seas classified as vulnerable marine ecosystems (VMEs) including several benthic habitats such as cold-water coral reefs or deep-sea sponge aggregations (Burgos et al., 2020); however, the scarcity of Arctic deep-sea data hampers a sound classification of VMEs in the AO to establish efficient conservations. There are, in general, more sampling and data sharing efforts for some parts of the NW Pacific, but the adjacent AO stays under-sampled, or data are not mobilized and shared publically. This can make biodiversity assessments difficult and bias species richness patterns. Consequently, management efforts may not take action accurately.
Latitudinal and bathymetric sampling effort and benthic gamma species richness decreased toward higher latitudes and deeper ocean areas even when the sample size was accounted for. We found that the highest sampling effort was in latitudes from 40 to 50°N and depths shallower than 500 m, which could also highlight the importance of adding deep-sea data from our expeditions to those latitudes. However, gamma species richness was higher in latitudes between 5 and 10°N and depths shallower than 500 m, mostly with regard to species of Mollusca and Arthropoda in the NW Pacific. This is probably because most of the species originated in the tropical NW Pacific and later extended their ranges toward the higher temperate and Arctic latitudes, so the tropical NW Pacific hosts both endemic (narrow-ranging) and widespread (wide-ranging) benthic species (Jablonski et al., 2006, 2013). Moreover, in the tropical NW Pacific, high productivity and thermal energy may increase speciation rates by providing a greater range of energetic lifestyles and higher metabolism (Clarke and Gaston, 2006; Mittelbach et al., 2007; Erwin, 2009; Saeedi et al., 2019b). It has been well-documented that the warm and nutrient-rich NW Pacific might foster greater population sizes and intra-specific competition that can drive higher species richness, altering the benthic community structure (Renema et al., 2008; Leprieur et al., 2016; Saeedi et al., 2019b,d). On the other hand, the existence of many islands and deep-sea areas along the NW Pacific create a diversity of topographic and oceanographic conditions, mostly shaped by past and current geological events (e.g., glaciation and deglaciation resulting sea-level fluctuations) (Belasky, 1996; Renema et al., 2008; Leprieur et al., 2016). These processes might have led to a higher environmental heterogeneity, which could possibly increase diversity in this area by species accumulation (Belasky, 1996; Renema et al., 2008; Yasuhara et al., 2009, 2012a; Leprieur et al., 2016; Moriaki et al., 2017).
One of the most important results of this study was documenting the taxon-specific community composition patterns as a response to different environmental variables. It has been well-documented before that some mechanisms such as productivity and isolation could cause high local species concentrations of specific phyla (Levin et al., 2001; Snelgrove and Smith, 2002; Brandt et al., 2007b; Smith, 2008). Others have suggested sea surface temperature as the most important variable in driving the latitudinal species richness patterns (Yasuhara et al., 2009, 2012a; Chaudhary et al., 2016, 2017; Saeedi et al., 2017, 2019b). However, changes in community structure of deep-reef benthic communities in temperate eastern Australia were mostly correlated with primary productivity and the temperature climatology, while local-scale variability in community composition was explained only by depth (James et al., 2017). Some studies have found that high topographic heterogeneity negatively influences benthic assemblage diversity, while sediment characteristics (i.e., percent sand content) were found to influence peracarid assemblages more than sediment particle-size diversity (Ashford et al., 2019). These authors have assumed that those assemblages might vary inter-and intra-annually, and respond differently to annual environmental changes with different rates of community change. However, some studies reported that benthic community composition and feeding type was correlated with sediment grain size in the Chukchi Sea (Pisareva et al., 2015), and in the deep Kongsfjord of the AO (Fetzer et al., 2002). Bonifácio et al. (2020) studied the polychaete assemblages across the nodule province of the eastern Clarion-Clipperton Fracture Zone (equatorial Pacific) (Bonifácio et al., 2020). They found that patterns in community structure and composition of polychaetes were mainly related to variations in organic carbon fluxes to the seafloor at the regional scale and to nodule density at the local scale. We also have found that different environmental variables were responsible for shaping benthic community composition in the NW Pacific and the adjacent Arctic Ocean. Among the most important environmental drivers were depth, silicate, current, and light, but surprisingly temperature did not have a significant influence on the composition of benthic communities. The importance of depth in speciation and structuring benthic communities has been well-documented by many scientists in different areas such as the Arctic, NW Pacific, and North Atlantic Oceans (Roy et al., 2014; Lacharité and Metaxas, 2018; Molina et al., 2019; Saeedi et al., 2019b; Murillo et al., 2020). We also found that shallow-water and deep-sea ostracods’ distributions are highly correlated with depth, dissolved oxygen, and silicate. Brandão et al. (2019), using the new data from the Kuril-Kamchatka Trench and the compilation of the literature, also found that ostracod counts decreased from 3,500 m toward deeper areas at 9,000 m (Brandão et al., 2019). A correlation between ostracods’ distributions and depth (negative) was also found, but no correlation was found for primary productivity or dissolved oxygen (Hong et al., 2019). However, Yasuhara et al. (2012a) suggested that species composition in ostracods along the deep North Pacific is influenced by both temperature and productivity. The main inorganic material forming the skeletal elements in Demospongiae and Hexactinellida, the spicules, is amorphous silica. Interestingly, we have found that silicate concentration is one of the main drivers of community composition in Demospongiae and Hexactinellida. Recent studies have revealed the effect of light regimes on growth of cuttlefish (Fiorito et al., 2014; Sykes et al., 2014), distribution of Ascidiacea (Shenkar and Swalla, 2011) and symbiosis activities of Anthozoa (as the acquisition of both ammonium and nitrate in is a light-dependent process) (Furla et al., 2005), which might explain the importance of light in the distribution of these taxa in this study. Some studies have also highlighted the role of currents in shifting the community compositions and distributions of epibenthic megafauna such as echinoderms, supporting our findings in this study (Roy et al., 2014; Lacharité and Metaxas, 2018). They have found that current speeds resulted in a higher diversity of epibenthic megafauna in coarser and more heterogeneous substrates in both the deep temperate continental shelf and Canadian Arctic benthic communities (substrate type was regarded as a proxy for current velocity with coarser substrate indicating a higher near-bottom flow regime). In this study, we refer to current velocity as the vast region of our study area includes all possible combinations of sea current activities, and there are no data that would allow drawing any conclusions based on overall sea currents. Salinity was not a strong driver in structuring the benthic communities in our study. However, salinity is a crucial driver of the community composition of bacterial and phytoplankton communities in shallow waters (Langenheder et al., 2003; Larson and Belovsky, 2013; Klier et al., 2018). Salinity below 100 m depth is more uniform and controls the stratification and water column stability in the upper ocean (de Boyer Montégut et al., 2007; Smith et al., 2011).
Environmental drivers of change in Arctic benthic biota have been recently summarized by Degen (2015) and ecological drivers of and responses by Arctic benthic communities by Molis et al. (2019). These authors showed that the Arctic hard- and soft-bottom communities show fundamental differences in their ecology. Hansen et al. (2020) unravelled the effects of environmental drivers and spatial structure on benthic species distribution patterns in Eurasian-Arctic seas (Barents, Kara, and Laptev Seas) (Hansen et al., 2020). Their results suggest that “different environmental variables determine the variation of Eurasian-Arctic benthic community composition within the spatial scales considered and highlight the importance of considering the diverse spatial structure of species communities in marine ecosystems (Hansen et al., 2006).” Megabenthic community structure was also related to several environmental variables over 2,000 km of the Canadian Arctic, from the Beaufort Sea to northern Baffin Bay (Roy et al., 2014). However, besides the biodiversity data gaps in some parts of the AO, the environmental data and ocean data assimilation are missing in many regions to support any specific assumptions or predictions on how vulnerable these species are to environmental change (Renaud et al., 2019).
Changes in the composition of benthic fauna communities serve as a useful indicator of the impact of environmental stressors under future climate change. By examining community-level responses of multiple taxa to environmental drivers we provide baseline data from which future changes can be detected, which will foster the establishment and implementation of international monitoring and conservation efforts. Many studies have looked at regional benthic communities in relation to environmental drivers, but here we combined all benthic taxa along large latitudinal and bathymetric gradients (where data were available online) to develop a broad overview of how each taxon responds to different environmental drivers along the NW Pacific and the AO. We demonstrate that benthic phyla respond differently to environmental stressors, and as a result, we recommend that taxon-specific criteria should be considered in international and large-scale management efforts to establish Benthic Protected Areas (BPA) along the NW Pacific and adjacent AO regions.
Data Availability Statement
The original contributions presented in the study are included in the article/Supplementary Material, further inquiries can be directed to the corresponding author.
Author Contributions
HS designed the research, collected and quality controlled the data, ran the statistical analyses, created the figures and tables, and wrote the manuscript. DW contributed to analyzing the data and creating the figures, writing, and English proofreading the manuscript. AB designed the German-Russian expeditions, collected and shared the field data from the deep NW Pacific, and contributed to writing the manuscript. All authors contributed to the article and approved the submitted version.
Conflict of Interest
The authors declare that the research was conducted in the absence of any commercial or financial relationships that could be construed as a potential conflict of interest.
Publisher’s Note
All claims expressed in this article are solely those of the authors and do not necessarily represent those of their affiliated organizations, or those of the publisher, the editors and the reviewers. Any product that may be evaluated in this article, or claim that may be made by its manufacturer, is not guaranteed or endorsed by the publisher.
Acknowledgments
This manuscript was part of the “Biogeography of the NW Pacific deep-sea fauna and their possible future invasions into the Arctic Ocean project (Beneficial project).” Beneficial project (grant number 03F0780A) was funded by the Federal Ministry for Education and Research (BMBF: Bundesministerium für Bildung und Forschung) in Germany. We would like to thank all the data providers in OBIS and GBIF whom made their data publicly available to be used in this study. We would specially like to thank Pieter Provoost, data manager in OBIS, who gave us useful advice in curating and quality control of the data retrieved from OBIS. Finally, we would like to thank the referees of the manuscript Jan Marcin Weslawski and specially Katrin Iken whom their constructive comments improved our manuscript.
Supplementary Material
The Supplementary Material for this article can be found online at: https://www.frontiersin.org/articles/10.3389/fmars.2022.804019/full#supplementary-material
Footnotes
- ^ www.iobis.org
- ^ https://www.gbif.org
- ^ http://www.marinespecies.org/deepsea/
- ^ www.marinespecies.org
- ^ http://www.bio-oracle.org/
- ^ https://www.gebco.net/
References
Al-Habahbeh, A. K., Kortsch, S., Bluhm, B. A., Beuchel, F., Gulliksen, B., Ballantine, C., et al. (2020). Arctic coastal benthos long-term responses to perturbations under climate warming. Philos. Trans. R. Soc. Lond. A Math. Phys. Eng. Sci. 378:20190355. doi: 10.1098/rsta.2019.0355
Antell, G. S., Kiessling, W., Aberhan, M., and Saupe, E. E. (2020). Marine biodiversity and geographic distributions are independent on large scales. Curr. Biol. 30, 115–121.e5.
Asaad, I., Lundquist, C. J., Erdmann, M. V., and Costello, M. J. (2017). Ecological criteria to identify areas for biodiversity conservation. Biol. Conserv. 213, 309–316.
Ashcroft, M. B., French, K. O., and Chisholm, L. A. (2011). An evaluation of environmental factors affecting species distributions. Ecol. Model. 222, 524–531.
Ashford, O. S., Kenny, A. J., Barrio Froján, C. R., Horton, T., and Rogers, A. D. (2019). Investigating the environmental drivers of deep-seafloor biodiversity: a case study of peracarid crustacean assemblages in the Northwest Atlantic Ocean. Ecol. Evol. 9, 14167–14204. doi: 10.1002/ece3.5852
Assis, J., Tyberghein, L., Bosch, S., Verbruggen, H., Serrão, E. A., and De Clerck, O. (2018). Bio-ORACLE v2.0: extending marine data layers for bioclimatic modelling. Glob. Ecol. Biogeogr. 27, 277–284.
Barnosky, A. D., Matzke, N., Tomiya, S., Wogan, G. O., Swartz, B., Quental, T. B., et al. (2011). Has the Earth’s sixth mass extinction already arrived? Nature 471, 51–57. doi: 10.1038/nature09678
Barroso, R., Kudenov, J. D., Halanych, K. M., Saeedi, H., Sumida, P. Y. G., and Bernardino, A. F. (2018). A new species of xylophylic fireworm (Annelida: Amphinomidae: Cryptonome) from deep-sea wood falls in the SW Atlantic. Deep Sea Res. Part I Oceanogr. Res. Pap. 137, 66–75.
Belasky, P. (1996). Biogeography of Indo-Pacific larger foraminifera and scleractinian corals: a probabilistic approach to estimating taxonomic diversity, faunal similarity, and sampling bias. Palaeogeogr. Palaeoclimatol. Palaeoecol. 122, 119–141. doi: 10.1016/0031-0182(95)00092-5
Bellwood, D. R., and Hughes, T. P. (2001). Regional-scale assembly rules and biodiversity of coral reefs. Science (New York, NY) 292, 1532–1535. doi: 10.1126/science.1058635
Birstein, J. (1971). Fauna of the Kurile-Kamchatka Trench. Additions to the fauna of isopods (Crustacea, Isopoda) of the Kurile-Kamchatka Trench. Part II. Asellota 2, Vol. 92. Moscow: Trudy Instituta Okeanogiya, Akademiya Nauk SSSR, 162–238.
Blasiak, R., Wynberg, R., Grorud-Colvert, K., Thambisetty, S., Bandarra, N. M., Canario, A. V., et al. (2020). The ocean genome and future prospects for conservation and equity. Nat. Sustain. 3, 588–596. doi: 10.1038/s41893-020-0522-9
Bonifácio, P., Martínez Arbizu, P., and Menot, L. (2020). Alpha and beta diversity patterns of polychaete assemblages across the nodule province of the eastern Clarion-Clipperton fracture zone (equatorial Pacific). Biogeosciences 17, 865–886.
Brandão, S. N., Hoppema, M., Kamenev, G. M., Karanovic, I., Riehl, T., Tanaka, H., et al. (2019). Review of Ostracoda (Crustacea) living below the carbonate compensation depth and the deepest record of a calcified ostracod. Progr. Oceanogr. 178:102144.
Brandt, A., Alalykina, I., Brix, S., Brenke, N., Błażewicz, M., Golovan, O. A., et al. (2019). Depth zonation of Northwest Pacific deep-sea macrofauna. Progr. Oceanogr. 176, 102131.
Brandt, A., Alalykina, I., Fukumori, H., Golovan, O., Kniesz, K., Lavrenteva, A., et al. (2018). First insights into macrofaunal composition from the SokhoBio expedition (Sea of Okhotsk, Bussol Strait and northern slope of the Kuril-Kamchatka Trench). Deep Sea Res. Part II Top. Stud. Oceanogr. 154, 106–120. doi: 10.1016/j.dsr2.2018.05.022
Brandt, A., and Malyutina, M. (2014). “German-Russian deep-sea expedition KuramBio (Kurile Kamchatka Biodiversity Study)–results and perspectives,” in World Conference on Marine Biodiversity (Quingdao).
Brandt, A., and Malyutina, M. V. (2015). The German-Russian deep-sea expedition KuramBio (Kurile Kamchatka biodiversity studies) on board of the RV Sonne in 2012 following the footsteps of the legendary expeditions with RV Vityaz. Deep Sea Res. Part II Top. Stud. Oceanogr. 111, 1–9.
Brandt, A., Brix, S., Riehl, T., and Malyutina, M. (2020). Biodiversity and biogeography of the abyssal and hadal Kuril-Kamchatka trench and adjacent NW Pacific deep-sea regions. Progr. Oceanogr. 181:102232. doi: 10.1016/j.pocean.2019.102232
Brandt, A., De Broyer, C., De Mesel, I., Ellingsen, K., Gooday, A., Hilbig, B., et al. (2007a). The biodiversity of the deep Southern Ocean benthos. Philos. Trans. R. Soc. B Biol. Sci. 362, 39–66. doi: 10.1098/rstb.2006.1952
Brandt, A., Gooday, A. J., Brandao, S. N., Brix, S., Brökeland, W., Cedhagen, T., et al. (2007b). First insights into the biodiversity and biogeography of the Southern Ocean deep sea. Nature 447, 307–311. doi: 10.1038/nature05827
Brandt, A., Malyutina, M., Majorova, N., Bashmanov, A., Brenke, N., Chizhova, T., et al. (2010). The Russian-German Deep-Sea Expedition (SoJaBio) to the Sea of Japan onboard of the R/V Akademik Lavrentyev (51st Cruise, August 11th – September 5th, 2010). The Cruise Report. doi: 10.13140/RG.2.1.1818.2809
Briggs, J. C. (2003). Marine centres of origin as evolutionary engines. J. Biogeogr. 30, 1–18. doi: 10.1046/j.1365-2699.2003.00810.x
Briggs, J. C., and Bowen, B. W. (2012). A realignment of marine biogeographic provinces with particular reference to fish distributions. J. Biogeogr. 39, 12–30.
Bringloe, T. T., Verbruggen, H., and Saunders, G. W. (2020). Unique biodiversity in Arctic marine forests is shaped by diverse recolonization pathways and far northern glacial refugia. Proc. Natl. Acad. Sci. U.S.A. 117, 22590–22596. doi: 10.1073/pnas.2002753117
Buhl-Mortensen, P., Dolan, M. F., Ross, R. E., Gonzalez-Mirelis, G., Buhl-Mortensen, L., Bjarnadóttir, L. R., et al. (2020). Classification and mapping of Benthic Biotopes in Arctic and Sub-Arctic Norwegian waters. Front. Mar. Sci. 7:271. doi: 10.3389/fmars.2020.00271
Burgos, J. M., Buhl-Mortensen, L., Buhl-Mortensen, P., Ólafsdóttir, S. H., Steingrund, P., Ragnarsson, S. Á, et al. (2020). Predicting the distribution of indicator taxa of vulnerable marine ecosystems in the Arctic and Sub-arctic Waters of the Nordic Seas. Front. Mar. Sci. 7:131. doi: 10.3389/fmars.2020.00131
Canonico, G., Buttigieg, P. L., Montes, E., Muller-Karger, F. E., Stepien, C., Wright, D., et al. (2019). Global observational needs and resources for marine biodiversity. Front. Mar. Sci. 6:367. doi: 10.3389/fmars.2019.00367
Carr, D., Lewin-Koh, N., and Maechler, M. (2011). hexbin: Hexagonal Binning Routines. R package version 1.26.0. Available online at: http://github.com/edzer/hexbin (accessed December 15, 2020).
Chaudhary, C., Saeedi, H., and Castello, M. J. (2016). Bimodality of Latitudinal Gradients in Marine Species Richness. Trends in Ecology & Evolution. 31, 670–676. doi: 10.1016/j.tree.2016.06.001
Chaudhary, C., Saeedi, H., and Costello, M. J. (2017). Marine species richness is bimodal with latitude: a reply to Fernandez and Marques. Trends Ecol. Evol. 32, 234–237. doi: 10.1016/j.tree.2017.02.007
Chernyshev, A. V., and Polyakova, N. E. (2018). Nemerteans from deep-sea expedition SokhoBio with description of Uniporus alisae sp. nov.(Hoplonemertea: Reptantia sl) from the Sea of Okhotsk. Deep Sea Res. Part II Top. Stud. Oceanogr. 154, 121–139.
Clarke, A., and Gaston, K. J. (2006). Climate, energy and diversity. Proc. Biol. Sci. 273, 2257–2266. doi: 10.1098/rspb.2006.3545
Clarkson, M., Kasemann, S. A., Wood, R., Lenton, T., Daines, S., Richoz, S., et al. (2015). Ocean acidification and the Permo-Triassic mass extinction. Science 348, 229–232. doi: 10.1126/science.aaa0193
Costello, M. J., and Chaudhary, C. (2017). Marine biodiversity, biogeography, deep-sea gradients, and conservation. Curr. Biol. 27, R511–R527.
Cummings, V. J., Thrush, S. F., Chiantore, M., Hewitt, J. E., and Cattaneo-Vietti, R. (2010). Macrobenthic communities of the north-western Ross Sea shelf: links to depth, sediment characteristics and latitude. Antarct. Sci. 22, 793–804.
Danovaro, R., Company, J. B., Corinaldesi, C., D’Onghia, G., Galil, B., Gambi, C., et al. (2010). Deep-Sea biodiversity in the Mediterranean Sea: the known, the unknown, and the unknowable. PLoS One 5:e11832. doi: 10.1371/journal.pone.0011832
Dayton, P., and Hessler, R. (eds) (1972). Role of biological disturbance in maintaining diversity in the deep sea. Deep Sea Res. Oceanogr. Abstr. 19, 199–208. doi: 10.1016/0011-7471(72)90031-9
de Boyer Montégut, C., Mignot, J., Lazar, A., and Cravatte, S. (2007). Control of salinity on the mixed layer depth in the world ocean: 1. General description. J. Geophys. Res. Oceans 112:C06011. doi: 10.1029/2006JC003953
Degen, R. (2015). The Future Arctic Biosphere-Environmental Drivers of Change in Arctic Benthic Biota. Ph.D. thesis. Bremen: University of Bremen.
Di Franco, D., Linse, K., Griffiths, H., Haas, C., Saeedi, H., and Brandt, A. (2020). Abundance and distributional patterns of benthic peracarid crustaceans from the Atlantic sector of the Southern Ocean and Weddell Sea. Front. Mar. Sci. 7:554663. doi: 10.3389/fmars.2020.554663
Duarte, C. M., Agusti, S., Barbier, E., Britten, G. L., Castilla, J. C., Gattuso, J.-P., et al. (2020). Rebuilding marine life. Nature 580, 39–51.
Ebbe, B., Billett, D. S. M., Brandt, A., Ellingsen, K., Glover, A., Keller, S., et al. (2010). “Diversity of Abyssal Marine Life,” in Life in the World’s Oceans: Diversity, Distribution and Abundance, ed. A. McIntyre (Chichester: Wiley-Blackwell), 139–160. doi: 10.1002/9781444325508.ch8
Elsner, N. O., Malyutina, M. V., Golovan, O. A., Brenke, N., Riehl, T., and Brandt, A. (2015). Deep down: isopod biodiversity of the Kuril–Kamchatka abyssal area including a comparison with data of previous expeditions of the RV Vityaz. Deep Sea Res. Part II Top. Stud. Oceanogr. 111, 210–219.
Fetzer, I., Lønne, O., and Pearson, T. (2002). The distribution of juvenile benthic invertebrates in an arctic glacial fjord. Polar Biol. 25, 303–315. doi: 10.1007/s00300-001-0345-8
Fiorito, G., Affuso, A., Anderson, D. B., Basil, J., Bonnaud, L., Botta, G., et al. (2014). Cephalopods in neuroscience: regulations, research and the 3Rs. Invert. Neurosci. 14, 13–36. doi: 10.1007/s10158-013-0165-x
Fujikura, K., Lindsay, D., Kitazato, H., Nishida, S., and Shirayama, Y. (2010). Marine biodiversity in Japanese waters. PLoS One 5:e11836. doi: 10.1371/journal.pone.0011836
Furla, P., Allemand, D., Shick, J. M., Ferrier-Pagès, C., Richier, S., Plantivaux, A., et al. (2005). The symbiotic anthozoan: a physiological chimera between alga and animal. Integr. Comp. Biol. 45, 595–604. doi: 10.1093/icb/45.4.595
Golovan, O. A., Malyutina, M. V., and Brandt, A. (2018). First record of the deep-sea isopod family Dendrotionidae (Isopoda: Asellota) from the Northwest Pacific with description of two new species of Dendromunna. Mar. Biodivers. 48, 531–544.
Gradinger, R., Bluhm, B. A., Hopcroft, R. R., Gebruk, A. V., Kosobokova, K., Sirenko, B., et al. (2010). Marine life in the Arctic. Life in the World’s Oceans: Diversity, Distribution and Abundance. Oxford: Wiley-Blackwell, 183–202.
Grassle, J. F., and Sanders, HL (eds). (1973). Life histories and the role of disturbance. Deep Sea Res. Oceanogr. Abstr. 7, 643–659. doi: 10.1016/0011-7471(73)90032-6
Grebmeier, J. M., Cooper, L. W., Feder, H. M., and Sirenko, B. I. (2006). Ecosystem dynamics of the Pacific-influenced Northern Bering and Chukchi Seas in the Amerasian Arctic. Progr. Oceanogr. 71, 331–361.
Hansen, J., Sato, M., Ruedy, R., Lo, K., Lea, D. W., and Medina-Elizade, M. (2006). Global temperature change. Proc. Natl. Acad. Sci. U.S.A. 103, 14288–14293.
Hansen, M. L., Piepenburg, D., Pantiukhin, D., and Kraan, C. (2020). Unraveling the effects of environmental drivers and spatial structure on benthic species distribution patterns in Eurasian-Arctic seas (Barents, Kara and Laptev Seas). Polar Biol. 43, 1693–1705.
Heberle, H., Meirelles, G. V., da Silva, F. R., Telles, G. P., and Minghim, R. (2015). InteractiVenn: a web-based tool for the analysis of sets through Venn diagrams. BMC Bioinformatics 16:169. doi: 10.1186/s12859-015-0611-3
Henehan, M. J., Ridgwell, A., Thomas, E., Zhang, S., Alegret, L., Schmidt, D. N., et al. (2019). Rapid ocean acidification and protracted Earth system recovery followed the end-Cretaceous Chicxulub impact. Proc. Natl. Acad. Sci. U.S.A. 116, 22500–22504. doi: 10.1073/pnas.1905989116
Hong, Y., Yasuhara, M., Iwatani, H., and Mamo, B. (2019). Baseline for ostracod-based northwestern Pacific and Indo-Pacific shallow-marine paleoenvironmental reconstructions: ecological modeling of species distributions. Biogeosciences 16, 585–604.
Jablonski, D., Belanger, C. L., Berke, S. K., Huang, S., Krug, A. Z., Roy, K., et al. (2013). Out of the tropics, but how? Fossils, bridge species, and thermal ranges in the dynamics of the marine latitudinal diversity gradient. Proc. Natl. Acad. Sci. U.S.A. 110, 10487–10494. doi: 10.1073/pnas.1308997110
Jablonski, D., Roy, K., and Valentine, J. W. (2006). Out of the tropics: evolutionary dynamics of the latitudinal diversity gradient. Science 314, 102–106. doi: 10.1126/science.1130880
James, L. C., Marzloff, M. P., Barrett, N., Friedman, A., and Johnson, C. R. (2017). Changes in deep reef benthic community composition across a latitudinal and environmental gradient in temperate Eastern Australia. Mar. Ecol. Progr. Ser. 565, 35–52.
Jöst, A. B., Yasuhara, M., Wei, C. L., Okahashi, H., Ostmann, A., Martínez Arbizu, P., et al. (2019). North Atlantic Gateway: test bed of deep-sea macroecological patterns. J. Biogeogr. 46, 2056–2066.
Jumars, P. A., Dorgan, K. M., and Lindsay, S. M. (2015). Diet of worms emended: an update of polychaete feeding guilds. Ann. Rev. Mar. Sci. 7, 497–520. doi: 10.1146/annurev-marine-010814-020007
Kamenev, G. M. (2019). Bivalve mollusks of the Kuril-Kamchatka Trench, Northwest Pacific Ocean: species composition, distribution and taxonomic remarks. Progr. Oceanogr. 176:102127.
Kamenev, G. M. (2020). Three new deep-sea species of Thyasiridae (Mollusca: Bivalvia) from the abyssal plain of the northwestern Pacific Ocean and hadal depths of the Kuril-Kamchatka Trench. PeerJ 8:e10405. doi: 10.7717/peerj.10405
Kędra, M., Grebmeier, J. M., and Cooper, L. W. (2018). Sipunculan fauna in the Pacific Arctic region: a significant component of benthic infaunal communities. Polar Biol. 41, 163–174. doi: 10.1007/s00300-017-2179-z
Kerswell, A. P. (2006). Global biodiversity patterns of benthic marine algae. Ecology 87, 2479–2488. doi: 10.1890/0012-9658(2006)87[2479:gbpobm]2.0.co;2
Klier, J., Dellwig, O., Leipe, T., Jürgens, K., and Herlemann, D. P. R. (2018). Benthic bacterial community composition in the Oligohaline-marine transition of surface sediments in the Baltic Sea Based on rRNA analysis. Front. Microbiol. 9:236. doi: 10.3389/fmicb.2018.00236
Kussakin, O. (1979). Marine and Brackish Isopods (Isopoda) of Cold and Temperate Waters of the Northern Hemisphere, vol 1. Suborder Flabellifera. Opredeliteli po faune SSSR, Izdavaemye Zoologicheskim institutom Akademii nauk SSSR, Vol. 122. Vladivostok: Academia Nauk, 1–470.
Kussakin, O. (1999). Marine and Brackish-Water Isopods from Cold and Temperate Waters of the Northern Hemisphere Suborder Asellota: Part 2, Families Joeropsididae, Nannoniscidae, Desmosomatidae, Macrostylidae., Keys to the Fauna of the SSSR. Keys to the fauna of the SSSR. Vladivostok: Academia Nauk, 3.
Kussakin, O. (2003). Marine and Brackish-Water ISOPODA of the Cold and Temperate Waters of the Northern Hemisphere. III. Suborder Asellota. Part 3. Family Munnopsidae.(Opredeliteli po Faune, Izdavaemie Zoologicheskim Institutom Rossiyskoy Academii Nauk). Opredeliteli po Faune, Izdavaemie Zoologicheskim Institutom Rossiyskoy. Vladivostok: Academii Nauk, 1–381.
Kussakin, O., and Vasina, G. (1990). “Descriptions of isopods of the suborders Flabellifera and Valvifera of bathyal regions of the Kurile Islands,” Systematics and Marine Biology of Marine Organisms (Vladivostok: Academia Nauk), 43–63.
Lacharité, M., and Metaxas, A. (2018). Environmental drivers of epibenthic megafauna on a deep temperate continental shelf: a multiscale approach. Progr. Oceanogr. 162, 171–186.
Langenheder, S., Kisand, V., Wikner, J., and Tranvik, L. J. (2003). Salinity as a structuring factor for the composition and performance of bacterioplankton degrading riverine DOC. FEMS Microbiol. Ecol. 45, 189–202. doi: 10.1016/S0168-6496(03)00149-1
Larson, C. A., and Belovsky, G. E. (2013). Salinity and nutrients influence species richness and evenness of phytoplankton communities in microcosm experiments from Great Salt Lake, Utah, USA. J. Plankton Res. 35, 1154–1166.
Lenihan, H. S., Peterson, C. H., Kim, S. L., Conlan, K. E., Fairey, R., McDonald, C., et al. (2003). Variation in marine benthic community composition allows discrimination of multiple stressors. Mar. Ecol. Progr. Ser. 261, 63–73.
Leprieur, F., Descombes, P., Gaboriau, T., Cowman, P. F., Parravicini, V., Kulbicki, M., et al. (2016). Plate tectonics drive tropical reef biodiversity dynamics. Nat. Commun. 7:11461. doi: 10.1038/ncomms11461
Levin, L. A., Etter, R. J., Rex, M. A., Gooday, A. J., Smith, C. R., Pineda, J., et al. (2001). Environmental influences on regional deep-sea species diversity. Annu. Rev. Ecol. Syst. 32, 51–93.
Malyutina, M. V., and Brandt, A. (2013). Introduction to SoJaBio (Sea of Japan biodiversity studies). Deep Sea Res. Part II Top. Stud. Oceanogr. 86, 1–9.
Malyutina, M. V., Chernyshev, A. V., and Brandt, A. (2018). Introduction to the SokhoBio (Sea of Okhotsk Biodiversity Studies) expedition 2015. Deep Sea Res. Part II Top. Stud. Oceanogr. 154, 1–9.
Mittelbach, G. G., Schemske, D. W., Cornell, H. V., Allen, A. P., Brown, J. M., Bush, M. B., et al. (2007). Evolution and the latitudinal diversity gradient: speciation, extinction and biogeography. Ecol. Lett. 10, 315–331. doi: 10.1111/j.1461-0248.2007.01020.x
Molina, ÈJ., Silberberger, M. J., Kokarev, V., and Reiss, H. (2019). Environmental drivers of benthic community structure in a deep sub-arctic fjord system. Estuar. Coast. Shelf Sci. 225:106239. doi: 10.1016/j.ecss.2019.05.021
Molis, M., Beuchel, F., Laudien, J., Włodarska-Kowalczuk, M., and Buschbaum, C. (2019). “Ecological drivers of and responses by arctic benthic communities, with an emphasis on kongsfjorden, svalbard,” in The Ecosystem of Kongsfjorden, Svalbard. Advances in Polar Ecology, Vol. 2, eds H. Hop and C. Wiencke (Cham: Springer), 423–481.
Moriaki, Y., Iwatani, H., Hunt, G., Okahashi, H., Kase, T., Hayashi, H., et al. (2017). Cenozoic dynamics of shallow-marine biodiversity in the Western Pacific. J. Biogeogr. 44, 567–578. doi: 10.1111/jbi.12880
Murillo, F. J., Weigel, B., Bouchard Marmen, M., and Kenchington, E. (2020). Marine epibenthic functional diversity on Flemish Cap (north-west Atlantic)—Identifying trait responses to the environment and mapping ecosystem functions. Divers. Distrib. 26, 460–478. doi: 10.1111/ddi.13026
Oksanen, J., Blanchet, F. G., Friendly, M., Kindt, R., Legendre, P., McGlinn, D., et al. (2017). vegan: Community Ecology Package. R Package Version 2.4-4. Available Online at: https://CRAN.R-project.org/package=vegan (accessed November 28, 2020).
Oksanen, J., Blanchet, F. G., Kindt, R., Legendre, P., Minchin, P. R., O’hara, R., et al. (2015). Package ‘vegan’. Community Ecology Package, Version, Vol. 2.
Oksanen, J., Blanchet, F. G., Kindt, R., Legendre, P., O’hara, R., Simpson, G. L., et al. (2010). Vegan: Community Ecology Package. R Package Version 1.17-4. Available online at: http://cran r-project.org (accesed November 11, 2011).
Pebesma, E., Bivand, R., Racine, E., Sumner, M., Cook, I., and Keitt, T. (2018). sf: Simple Features for R. R Package Version 06-1.
Pisareva, M. N., Pickart, R. S., Iken, K., Ershova, E. A., Grebmeier, J. M., Cooper, L. W., et al. (2015). The relationship between patterns of benthic fauna and zooplankton in the Chukchi Sea and physical forcing. Oceanography 28, 68–83. doi: 10.5670/oceanog.2015.58
Provoost, P. B. S. (2018). “robis: R Client to Access Data from the OBIS API.” Ocean Biogeographic Information System. R Package Version 1.0, 1 Edn. Paris: Intergovernmental Oceanographic Commission of UNESCO.
Ramirez-Llodra, E., Brandt, A., Danovaro, R., Mol, B. D., Escobar, E., German, C. R., et al. (2010). Deep, diverse and definitely different: unique attributes of the world’s largest ecosystem. Biogeosciences 7, 2851–2899.
Rampino, M. R., and Caldeira, K. (2005). Major perturbation of ocean chemistry and a ‘Strangelove Ocean’after the end-Permian mass extinction. Terra Nova 17, 554–559.
Reimer, J. D., Biondi, P., Lau, Y. W., Masucci, G. D., Nguyen, X. H., Santos, M. E., et al. (2019). Marine biodiversity research in the Ryukyu Islands, Japan: current status and trends. PeerJ 7:e6532. doi: 10.7717/peerj.6532
Renaud, P. E., Wallhead, P., Kotta, J., Włodarska-Kowalczuk, M., Bellerby, R. G., Rätsep, M., et al. (2019). Arctic sensitivity? Suitable habitat for benthic taxa is surprisingly robust to climate change. Front. Mar. Sci. 6:538. doi: 10.3389/fmars.2019.00538
Renema, W., Bellwood, D. R., Braga, J. C., Bromfield, K., Hall, R., Johnson, K. G., et al. (2008). Hopping hotspots: global shifts in marine biodiversity. Science (New York, NY) 321, 654–657. doi: 10.1126/science.1155674
Rex, M. A. (1973). Deep-sea species diversity: decreased gastropod diversity at abyssal depths. Science 181, 1051–1053. doi: 10.1126/science.181.4104.1051
Rex, M. A. (1977). “Zonation in deep-sea gastropods: the importance of biological interactions to rates of zonation,” in Biology of Benthic Organisms, eds B. F. Keegan, P. O. Ceidigh, and P. J. S. Boaden (London: Elsevier), 521–530.
Rex, M. A., and Etter, R. J. (2010). Deep-Sea Biodiversity: Pattern and Scale. Cambridge, MA: Harvard University Press.
Rex, M. A., Crame, J. A., Stuart, C. T., and Clarke, A. (2005a). Large-scale biogeographic patterns in marine mollusks: a confluence of history and productivity? Ecology 86, 2288–2297.
Rex, M. A., McClain, C. R., Johnson, N. A., Etter, R. J., Allen, J. A., Bouchet, P., et al. (2005b). A source-sink hypothesis for abyssal biodiversity. Am. Nat. 165, 163–178. doi: 10.1086/427226
Rex, MA (eds) (1976). Biological accomodation in the deep-sea benthos: comparative evidence on the importance of predation and productivity. Deep Sea Res. Oceanogr. Abstr. 23, 975–987.
Roy, V., Iken, K., and Archambault, P. (2014). Environmental drivers of the Canadian Arctic Megabenthic communities. PLoS One 9:e100900. doi: 10.1371/journal.pone.0100900
Saeedi, H., and Brandt, A. (eds.). (2020a). “Biogeographic Atlas of the Deep NW Pacific Fauna,” in Advanced Books (Sofia: Pensoft Publishers), 1.
Saeedi, H., and Brandt, A. (2020b). NW Pacific Deep-Sea Benthos Biodiversity (Beneficial Project). v1.2. Deep-Sea OBIS Node. Dataset/Samplingevent.
Saeedi, H., and Costello, M. (2012). “Aspects of global distribution of six marine bivalve mollusc families,” in Clam Fisheries and Aquaculture, ed. F. da Costa (New York, NY: Nova Science Publishers Inc.), 27–44.
Saeedi, H., Bernardino, A. F., Shimabukuro, M., Falchetto, G., and Sumida, P. Y. G. (2019a). Macrofaunal community structure and biodiversity patterns based on a wood-fall experiment in the deep South-west Atlantic. Deep Sea Res. Part I Oceanogr. Res. Pap. 145, 73–82. doi: 10.1016/j.dsr.2019.01.008
Saeedi, H., Costello, M. J., Warren, D., and Brandt, A. (2019b). Latitudinal and bathymetrical species richness patterns in the NW Pacific and adjacent Arctic Ocean. Sci. Rep. 9:9303. doi: 10.1038/s41598-019-45813-9
Saeedi, H., Reimer, J. D., Brandt, M. I., Dumais, P.-O., Jażdżewska, A. M., Jeffery, N. W., et al. (2019c). Global marine biodiversity in the context of achieving the Aichi Targets: ways forward and addressing data gaps. PeerJ 7:e7221. doi: 10.7717/peerj.7221
Saeedi, H., Simões, M., and Brandt, A. (2019d). Endemicity and community composition of marine species along the NW Pacific and the adjacent Arctic Ocean. Progr. Oceanogr. 178:102199.
Saeedi, H., Winterberg, H., Alalykina, L. I., Bergmeier, S. F., Downey, R., Golovan, O., et al. (2019e). NW Pacific Deep-Sea Benthos Distribution and Abundance (Beneficial Project). Deep-Sea OBIS Node Dataset/Samplingevent, Vol. 1.
Saeedi, H., Dennis, T. E., and Costello, M. J. (2017). Bimodal latitudinal species richness and high endemicity of razor clams (Mollusca). J. Biogeogr. 44, 592–604. doi: 10.3897/BDJ.7.e31375
Saeedi, H., Simões, M., and Brandt, A. (2020). Biodiversity and distribution patterns of deep-sea fauna along the temperate NW Pacific. Progr. Oceanogr. 183:102296. doi: 10.1016/j.pocean.2020.102296
Sanciangco, J. C., Carpenter, K. E., Etnoyer, P. J., and Moretzsohn, F. (2013). Habitat availability and heterogeneity and the Indo-Pacific warm pool as predictors of marine species richness in the tropical Indo-Pacific. PLoS One 8:e56245. doi: 10.1371/journal.pone.0056245
Sanders, H. L., and Hessler, R. R. (1969). Ecology of the deep-sea benthos. Science 163, 1419–1424. doi: 10.1126/science.163.3874.1419
Selig, E. R., Turner, W. R., Troëng, S., Wallace, B. P., Halpern, B. S., Kaschner, K., et al. (2014). Global priorities for marine biodiversity conservation. PLoS One 9:e82898. doi: 10.1371/journal.pone.0082898
Shenkar, N., and Swalla, B. J. (2011). Global diversity of Ascidiacea. PLoS One 6:e20657. doi: 10.1371/journal.pone.0020657
Shimodaira, H. (2004). Approximately unbiased tests of regions using multistep-multiscale bootstrap resampling. Ann. Stat. 32, 2616–2641.
Simões, M. V., Saeedi, H., Cobos, M. E., and Brandt, A. (2021). Environmental matching reveals non-uniform range-shift patterns in benthic marine Crustacea. Clim. Change 168, 1–20.
Smith, A. B., and Stockley, B. (2005). The geological history of deep-sea colonization by echinoids: roles of surface productivity and deep-water ventilation. Proc. R. Soc. Lond. B Biol. Sci. 272, 865–869. doi: 10.1098/rspb.2004.2996
Smith, K. L., Sherman, A. D., Shaw, T. J., Murray, A. E., Vernet, M., and Cefarelli, A. O. (2011). Carbon export associated with free-drifting icebergs in the Southern Ocean. Deep Sea Res. Part II Top. Stud. Oceanogr. 58, 1485–1496. doi: 10.1146/annurev-marine-121211-172317
Smith, W. (2008). Practical understanding and use of surface enhanced Raman scattering/surface enhanced resonance Raman scattering in chemical and biological analysis. Chem. Soc. Rev. 37, 955–964. doi: 10.1039/b708841h
Snelgrove, P. V., and Smith, C. R. (2002). A Riot of Species in an Environmental Calm: The Paradox of the Species-Rich Deep-Sea Floor. Oceanography and Marine Biology, An Annual Review, Vol. 40. Boca Raton, FL: CRC Press, 319–320.
Spalding, M. D., Fox, H. E., Allen, G. R., Davidson, N., Ferdana, Z. A., Finlayson, M., et al. (2007). Marine ecoregions of the world: a bioregionalization of coastal and shelf areas. BioScience 57, 573–583. doi: 10.1111/cla.12453
Su, J., Guan, B., and Jiang, J. (1990). The Kuroshio. Part I. Physical. Oceanogr. Mar. Biol. 28, 11–71. doi: 10.1016/j.scitotenv.2017.02.208
Sykes, A. V., Quintana, D., and Andrade, J. P. (2014). The Effects of light intensity on growth and survival of cuttlefish (sepia officinalis) hatchlings and Juveniles. Aquac. Res. 45, 2032–2040.
Tyberghein, L., Verbruggen, H., Pauly, K., Troupin, C., Mineur, F., and De Clerck, O. (2012). Bio-ORACLE: a global environmental dataset for marine species distribution modelling. Glob. Ecol. Biogeogr. 21, 272–281.
Tyler, P. A. (2002). Deep-sea eukaryote ecology of the semi-isolated basins off Japan. J. Oceanogr. 58, 333–341.
Vedenin, A., Galkin, S., and Gebruk, A. (2021). List of macrobenthic species: data from the Siberian Seas and the adjacent area of the deep-sea Central Arctic. Data Brief 36:107115. doi: 10.1016/j.dib.2021.107115
Vermeij, G. J., Banker, R., Capece, L. R., Hernandez, E. S., Salley, S. O., Padilla Vriesman, V., et al. (2019). The coastal North Pacific: origins and history of a dominant marine biota. J. Biogeogr. 46, 1–18.
Waga, H., Hirawake, T., and Grebmeier, J. M. (2020). Recent change in benthic macrofaunal community composition in relation to physical forcing in the Pacific Arctic. Polar Biol. 43, 285–294.
WoRMS (2018). World Register of Marine Species 2018. Available Online at: http://www.marinespecies.org/aphia.php?p=stats*Q
Yasuhara, M., Hunt, G., Cronin, T. M., and Okahashi, H. (2009). Temporal latitudinal-gradient dynamics and tropical instability of deep-sea species diversity. Proc. Natl. Acad. Sci. U.S.A. 106, 21717–21720. doi: 10.1073/pnas.0910935106
Yasuhara, M., Hunt, G., van Dijken, G., Arrigo, K. R., Cronin, T. M., and Wollenburg, J. E. (2012b). Patterns and controlling factors of species diversity in the Arctic Ocean. J. Biogeogr. 39, 2081–2088. doi: 10.3389/fmicb.2017.00882
Yasuhara, M., Hunt, G., Cronin, T. M., Hokanishi, N., Kawahata, H., Tsujimoto, A., et al. (2012a). Climatic forcing of quaternary deep-sea benthic communities in the North Pacific Ocean. Paleobiology 38, 162–179. doi: 10.1017/s0094837300000464
Keywords: benthic fauna, shallow water, deep sea, community composition, depth, silicate, NW Pacific, Arctic Ocean
Citation: Saeedi H, Warren D and Brandt A (2022) The Environmental Drivers of Benthic Fauna Diversity and Community Composition. Front. Mar. Sci. 9:804019. doi: 10.3389/fmars.2022.804019
Received: 28 October 2021; Accepted: 17 February 2022;
Published: 10 March 2022.
Edited by:
Daniel Martin, Spanish National Research Council (CSIC), SpainReviewed by:
Jan Marcin Weslawski, Institute of Oceanology (PAN), PolandKatrin Iken, University of Alaska Fairbanks, United States
Copyright © 2022 Saeedi, Warren and Brandt. This is an open-access article distributed under the terms of the Creative Commons Attribution License (CC BY). The use, distribution or reproduction in other forums is permitted, provided the original author(s) and the copyright owner(s) are credited and that the original publication in this journal is cited, in accordance with accepted academic practice. No use, distribution or reproduction is permitted which does not comply with these terms.
*Correspondence: Hanieh Saeedi, aGFuaWVoLnNhZWVkaUBzZW5ja2VuYmVyZy5kZQ==