- 1Southern Seas Ecology Laboratories, University of Adelaide, School of Biological Sciences, Adelaide, SA, Australia
- 2South Australian Research and Development Institute (SARDI), Aquatic Sciences, West Beach, SA, Australia
- 3School of Life and Environmental Sciences, Deakin University, Burwood, VIC, Australia
- 4Conservation Department, Phillip Island Nature Parks, Cowes, VIC, Australia
Marine predators recovering from historic, commercial, over-harvesting can create conservation challenges when they prey on vulnerable species. Pinniped predation of seabirds presents one such challenge and identifying the source colonies experiencing seal predation are needed to inform conservation management and decision planning. Here, we present a novel application of stable isotope and trace element techniques to identify the source colony of little penguins (Eudyptula minor) predated by long-nosed fur seals (Arctocephalus forsteri). We created baseline biochemical ‘feather-prints’ from feathers for six major breeding colonies across south-east Australia to compare with feathers from predated penguins recovered from seal scats. Feeding trials of captive seals confirmed that digestion of penguin feathers did not compromise stable isotope (δ13C and δ15N) or trace element (Al, Ti, Sr and Mg) signatures. The resulting biochemical ‘feather-prints’ were found to be robust in being correctly classified to local sites (78%) and broader regions (85%). The distinguishing ‘feather-prints’ appeared to be driven by industrial inputs from land, colony-specific foraging patterns and potentially proximity to oceanographic systems (i.e. upwelling). Here, we show that 46-70% of predated feathers were assigned to ‘local’ penguin colonies. We consider that the regional penguin abundances and the proximity of their colonies to seal sites, as well as demographic-specific foraging patterns may shape their contribution to seal diet at local, regional and inter-regional scales. This diagnostic tool is powerful, having broad applications identifying seabird colonies at greatest risk to pinniped predation and informing targeted, site-specific, conservation effort.
Introduction
Many pinniped populations are undergoing sustained growth after being heavily depleted by commercial harvest between the late 1800s and early 1900s (Ling, 1999; Magera et al., 2013; Roman et al., 2015; Goldsworthy et al., 2019b). Whilst the resurgence of these top-predators represent significant conservation outcomes and are to be celebrated, they come with new challenges in understanding the importance of healthy predator populations in restoring ecosystem function and their top-down effects on their prey, including seabirds (Marshall et al., 2016). The effect of higher trophic predators (such as pinnipeds and other marine mammals) on the behaviour, distribution or abundance of their prey is also influenced by how vulnerable the prey species are to other natural or anthropogenic threats (Hunter and Price, 1992; Matson and Hunter, 1992; Hunt Jr et al., 2002). Seabirds are considered the most threatened group of birds in the world, experiencing natural and anthropogenic stressors in both their terrestrial and marine environments (Croxall et al., 2012; Dias et al., 2019) with populations globally declining by >70% since 1950 (Paleczny et al., 2015). Penguins (family Spheniscidae) provide a prime example of physiologically unique (flightless) seabirds vulnerable to both terrestrial and marine predation (Xavier and Trathan, 2020), with 10 of the 18 recognized penguin species listed as either vulnerable or endangered on the ICUN Red List 2021. At the lowest end of the penguin size range, little penguins (Eudyptula minor), averaging 30 cm in length and 1 kg in body mass, have a slower swimming speed than other penguin species (Bethge et al., 1997), potentially increasing their vulnerability to seal predation. Little penguins also share a sympatric breeding distribution with the long-nosed fur seal (Arctocephalus forsteri); co-occurring on offshore islands from the southwest coast of Western Australia, across the southern coast (including Tasmania) and up the eastern coast of mainland Australia (as far north as South Solitary Island), and on to New Zealand and the Chatham Islands (Marchant and Higgins, 1990) where penguins also breed.
Recent population declines in little penguins (hereafter penguin), a component of the long-nosed fur seal diet, has exacerbated social tensions around the recovery of the native long-nosed fur seal. Ancestral A. forsteri established populations on New Zealand and Australian coasts in the last million years (Kirkwood and Goldsworthy, 2013). Also known as the New Zealand fur seal, we prefer to use the vernacular name ‘long-nosed fur seal’ in Australia, as fishers and other community sectors argue incorrectly that the long-nosed fur seal is not native to Australia (Shaughnessy et al., 2015). In the last 2-3 decades, the population size of this species across south-eastern Australia (New South Wales – Tasmania) has been increasing (Shaughnessy et al., 2015; S. Reinhold et al., unpubl. data). Misperceptions of the ‘introduced’ status of long-nosed fur seals have in-part driven calls to cull this native top predator in Australia.
Predation of seabirds occurs amongst many seal species (Antarctic fur seals (Arctocephalus gazella): (Visser et al., 2008); Cape fur seals (Arctocephalus pusillus): (Du Toit et al., 2004); leopard seals (Hydrurga leptonyx): (Ainley et al., 2005); South American sea lions (Otaria flavescens): (Rey et al., 2012); New Zealand sea lions (Phocarctos hookeri): (Morrison et al., 2016), and long-nosed fur seals (Page et al., 2005). In South Australia, penguin remains have been detected in 0-40% of long-nosed fur seal scats (Page et al., 2005; Goldsworthy et al., 2019a) with the variance attributed to potential differences in site-specific and age-class related predation patterns. In 2006-08, 30% of long-nosed fur seal scats from Kanowna Island, in Central Bass Strait, Victoria, showed evidence of penguin predation (Hoskins et al., 2017). Recently, however, seven hot spots of penguin predation (>30% of scats with penguin remains) have been detected across south-eastern Australia, four of which occur in Bass Strait (S. Reinhold et al., unpubl. data).
Bass Strait, the shallow continental shelf area between mainland Australia and Tasmania, is a key region for Australian seabirds, supporting a large proportion of breeding populations of at least 11 species (Ross et al., 1995), including 82% of Australia’s little penguin population. On a global scale, this marine area is considered a region of low primary productivity (Gibbs et al., 1986; Gibbs et al., 1991) that occurs at the confluence of three main ocean currents; the East Australian Current (EAC), South Australian Current (SAC) and sub-Antarctic surface water (SASW). The latter two represent key sources of primary productivity, as do the Bonney Upwelling and west Tasmanian upwelling that disperse nutrient rich water into west Bass Strait (Middleton et al., 2007; Middleton and Bye, 2007; Kämpf, 2015).
In Bass Strait, during pre-moult, foraging adult penguins undertake an intensive foraging bout that lasts approximately three weeks – likely accessing prey in nutrient rich waters (i.e. areas of upwelling). This pre-moult foraging period is critical for adult penguins to increase their body mass to sustain the upcoming three week period of fasting during moult (Gales et al., 1988). Post-moult foraging also entails an extended offshore foraging period that serves to re-plenish fasting penguins in the lead up to the breeding season (McCutcheon et al., 2011). Consequently, moulted penguin feathers retain the biochemical signatures of the environmental conditions (i.e. different sources of primary production) and anthropogenic contaminants, also described as bottom-up influences, experienced by penguins during the pre-moult foraging period (Hobson and Clark, 1992; Finger et al., 2015; Kowalczyk et al., 2015). In St Kilda (Port Phillip Bay, Victoria) for example, trace metal concentrations in penguin feathers have been linked to varying levels of industrialisation adjacent to penguin foraging zones (Finger et al., 2015). Similarly, stable carbon isotopes (δ13C) from penguin feathers have been used to provide insight into the relative contributions of different production sources in a trophic network (Hobson et al., 1994; Cherel and Hobson, 2007). By contrast, nitrogen (δ15N) serves as an indicator of consumer trophic position (Vanderklift and Ponsard, 2003). Combined, trace element and stable isotope signatures in feathers therefore provide a biochemical map of bottom-up factors (toxicants, primary productivity and trophic positioning) for penguins using different pre-moult foraging zones. Hence, the potential exists to compare between penguin feathers recovered from long-nosed fur seal scats and moulted penguin feathers (Fromant et al., 2020). Complimentary to the highly synchronised life stages of penguins across Bass Strait, the different levels of coastal industrialisation and sources of primary productivity entering this system (EAC in the east and Antarctic upwelling in the west) (Gibbs et al., 1986; Gibbs et al., 1991), suggest that Bass Strait may be a good candidate for detecting biochemical variation (in feathers) between penguin colonies using different pre-moult foraging zones.
Feathers have been consistently recovered from long-nosed fur seal scats where penguin predation occurs. In terrestrial systems, predator-prey relationships have successfully been explored using stable isotope signatures from the hair of wild deer, compared to the deer hair retrieved from the scats of wolves where their ranges overlap (Derbridge et al., 2012). Similarly, we aim to develop biochemical techniques that can identify the source colony of predated penguin feathers recovered from long-nosed fur seal scats. Using a combination of stable isotope and trace element techniques, this research aims to (1) develop a biochemical map as a baseline of ‘feather-prints’ – using moulted feathers from penguin colonies across Western, Central and Eastern Bass Strait and Port Phillip Bay, (2) use these ‘feather-prints’ to determine the scale of differentiation as either colony or region specific (or both), and (3)use the baseline ‘feather-prints’ to infer the source colony and/or region of predated feathers in long-nosed fur seal scats.
Materials and Methods
Sample Collection
Every year, between November and March, adult penguins in Bass Strait experience three, highly synchronised, life stages in the lead up to the breeding season; (1) pre-moult foraging (Nov-Jan), (2) a catastrophic moult during which all feathers are shed while individuals are on land (Feb-March), and (3) post-moult foraging (March-April) (Reilly and Cullen, 1981). We collected moulted feathers from penguin colonies across four regions; Western (Cape Nelson and Deen Maar Island), Central (Phillip Island, Kanowna Island and Rabbit Island) and Eastern Bass Strait (Gabo Island), and Port Phillip Bay (St. Kilda) to inform baseline biochemical ‘feather-prints’ (Figure 1 and Table 1). Kanowna and Gabo Island feathers were opportunistically collected from satellite tracking retrieval procedures (feathers remaining on water-proof tape used to attach GPS data loggers were collected - separate study), whilst moulted feathers were collected for the other four sites. Trace element and stable isotope concentrations circulating in the blood at the time of moult are thought to be a combination of what has been consumed in the weeks of pre-moult feeding and a remobilisation of chemical sequestration from internal tissues (Furness et al., 1986; Bearhop et al., 2000). The blood supply to feathers ceases after formation of the new feathers. Therefore, the data presented from feathers moulted in 2019 are an indication of 2018/19 pre-moult foraging at all sites (Figure 2 and Table 1). Between October and November 2018, we randomly sampled fresh scats from three long-nosed fur seal sites across northern Bass Strait (Table 1). Feathers are metabolically inert after formation and, hence, penguin feathers retrieved from long-nosed fur seal scats collected between April 2018 and up to February 2019 reflect the biochemical signatures of pre-moult foraging of penguins during 2018 (Figure 2). Overall, penguin feathers were evident in 37 scats; Cape Bridgewater (Western Bass Strait) (n=11), Kanowna Island (Central Bass Strait) (n=10) and Gabo Island (Eastern Bass Strait) (n=16).
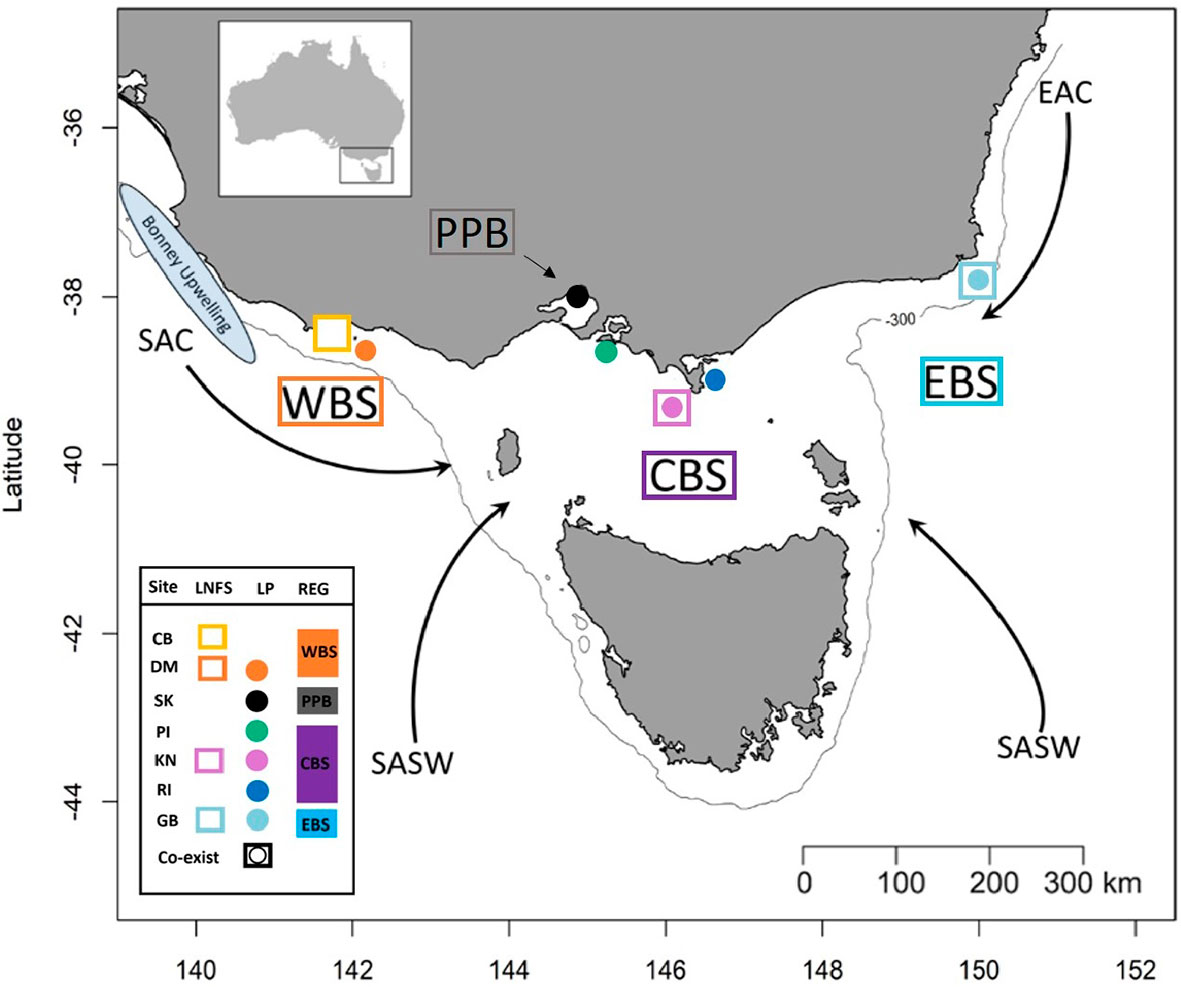
Figure 1 Simplified representation of the four sampling regions and the major water masses influencing those regions. Western Bass Strait (WBS); Port Phillip Bay (PPB); Central Bass Strait (CBS); Eastern Bass Strait (EBS); Cape Bridgewater (CB); Deen Maar Island (DM); St. Kilda (SK); Phillip Island (PI); Kanowna Island (KN); Rabbit Island (RI); Gabo Island (GB); South Australian Current (SAC); Sub-Antarctic Surface Water (SASW); and East Australian Current (EAC) from Sandery and Kämpf (2007). Circles indicate sampled penguin colonies, squares represent sample sites of scats of long-nosed fur seals and squares with circles represent locations where both species co-exist. The solid line indicates the location of the 300m isobath.
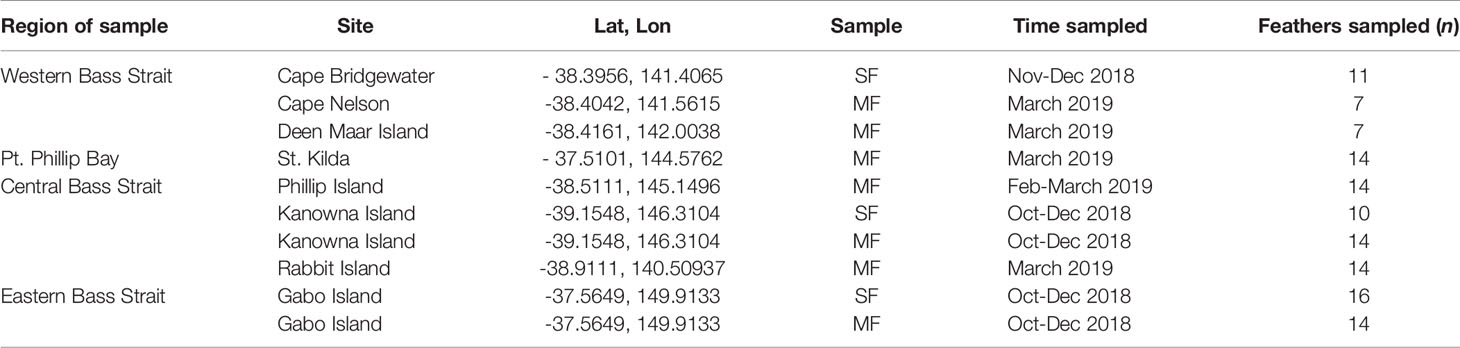
Table 1 Collection locations, seasons and sample sizes for Moulted Feathers (MF) and Scat Feathers (SF) retrieved from little penguin colonies and scats of long-nosed fur seals respectively.
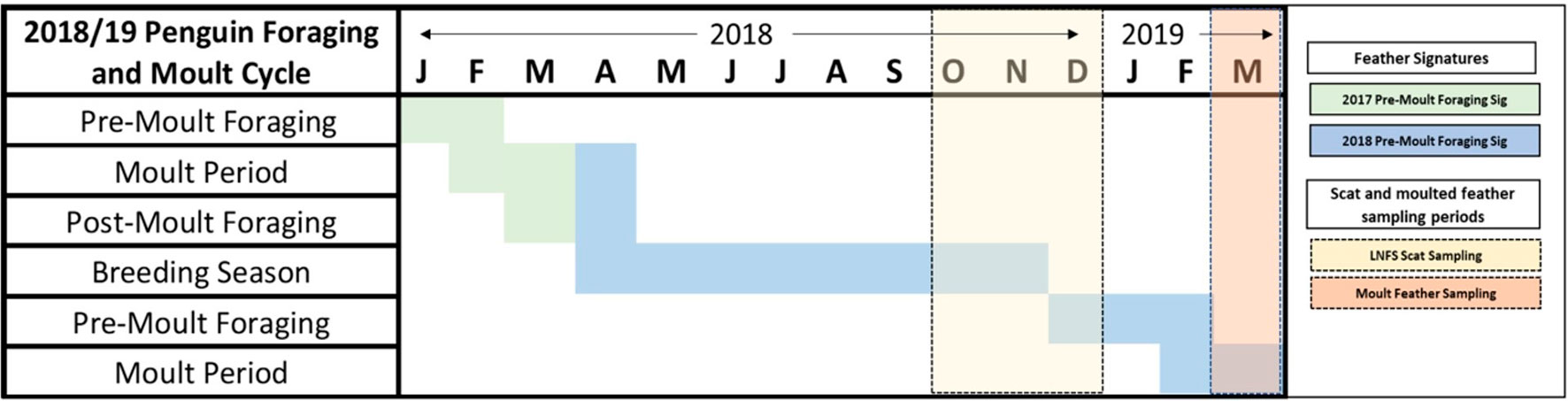
Figure 2 Foraging and moult phenology of little penguins in Bass Strait. Blocks with horizontal lines correspond to the 2017 and 2018 pre-moult foraging signatures of feathers. Yellow and orange shaded blocks correspond to the sampling periods of seal scats and moulted penguin feathers respectively.
Feeding Trial
To investigate the biochemical effect of digestion on penguin feathers and subsequent comparability between moulted and scat feathers, three feeding trials were undertaken at Melbourne Zoo, between August and November 2018. Three long-nosed fur seals (n = 1 female, n = 3 male) and one Australian fur seal (female; Arctocephalus pusillus doriferus) were fed fish tightly stuffed with little penguin feathers (approx. 50g) from two penguin carcasses from Phillip Island that died naturally as a result of heat stress. Feeding occurred between 0900-1000 h on three separate occasions (a minimum of 10 days apart). Scats were then collected daily for 72 h and frozen at -20°C until analysed. Samples were soaked in warm water in individual plastic containers for at least 24 h and washed with tap water through a nested 1.0 mm sieve to extract digested feathers. Undigested feathers were also collected from the two penguin carcasses and used as a control for comparison to digested feathers. Due to sample size limitations, feathers originating from one carcass were used for trace element signature comparisons between digested and undigested feathers. Feathers from both penguin carcasses were utilised for stable isotope comparisons.
Sample Processing
The laboratory procedures described below were used to determine stable isotope and trace element signatures in penguin feathers recovered from the feeding trial, moulted feathers and predated feathers from long-nosed fur seal scats (Table 1).
Stable Isotope Analyses
We cleaned penguin feathers in 5ml teflon vials containing 2:1 chloroform:methanol solution (5 ml) using a sonic bath with vials immersed in water for 2 minutes. Two successive methanol rinses using the sonic bath (2 min per rinse) followed initial cleaning (Cherel et al., 2014). Feathers were then oven dried (60°C) for 48 hours and cut into small fragments. We packed ~0.4mg of feathers into pre-combusted tin capsules (Elemental Microanalysis 9x5mm C10-042) and determined carbon (13C/12C, δ13C and nitrogen (15N/14N, δ15N isotope ratios using a continuous flow ratio mass spectrometer (Nu Horizon, Wrexham, UK) coupled to an elemental analyser (EA3000, EuroVector, Pavia, Italy). Isotopic results are presented in δ notation relative to Vienna PeeDee Belemnite and the atmospheric abundance for δ13C and δ15N, respectively (Coplen et al., 2006).
All samples where corrected for instrument drift and normalized according to reference values using in-house standards (n=25); glycine -31.2‰, glutamic acid -16.72‰ & triphenylamine (TPA) -29.2‰ calibrated against USGS and IAEA certified reference materials (USGS40, USGS 41, IAEA-2).
Trace Element Analysis
Penguin feathers were vigorously washed in Milli-Q water three times and then oven-dried at 45°C for 48 h with a resulting dry weight (dw) range of 1.9 – 5.4 mg dw (Finger et al., 2015). Whole feathers were initially digested in an aqua regia solution made up of (2.7ml 70% nitric acid (RCI Premium, ACI Labscan) and 0.3ml 37% hydrochloric acid (NORMAPURE, VWR CHEMICALS Analar) at 95°C for 12 h. Samples were then re-digested using 0.03 ml 69% nitric acid and diluted with Milli- Q water to a final volume of 3 mL (2% nitric concentration and ~1000ppb dilution). Feathers were assessed for concentrations of Lithium (Li), Boron (B), sodium (Na), magnesium (Mg), aluminium (Al), potassium (K), calcium (Ca), titanium (Ti), vanadium (V), cromium (Cr), manganese (Mn), iron (Fe), cobalt (Co), nickel (Ni), copper (Cu), zinc (Zn), arsenic (As), selenium (Se), strontium (Sr), cadmium (Cd), tin (Sn), antimony (Sb), barium (Ba), lead (Pb), and bismuth (Bi). Feathers were analysed at Adelaide Microscopy, University of Adelaide, with an Agilent 8900x ICP-MS/MS and limit of detection of 0.01 mg/kg. Trace elements Li, Cr, Ni, As, Cd, Sn, Sb, Pb and Bi were below the limit of detection (0.01 mg/kg) and therefore excluded from further analysis. Elements B, Na, Mg, Al, K, Fe, Sr, Se, Ti, V, Mn, Co and Ba exceeded the detection limits of the ICP-MS/MS for 100% of samples. A minimum of five procedural blanks were analysed per 60 samples and all results reported as mg/kg dry weight (dw). External machine precision (i.e. machine drift) was assessed by measuring 100ppb standards every 10 samples in the absence of Standard Reference Materials (SRM) for feather tissues.
Statistical Analyses
Feeding trial analysis of digested and undigested feathers were prioritised above the broader analysis of moulted and scat feathers to inform their biochemical comparability.
Feeding Trial
To determine whether long-nosed fur seal digestion altered the feather biochemical signatures, we performed paired t-tests for the undigested and digested feathers, for each stable isotope (δ13C and δ15N) and trace element (B, Na, Mg, Al, K, Fe, Sr, Se, Sn, Ti, V, Mn, Co and Ba) detected. Significance was taken to be p<0.05 for all statistical analyses.
Moulted and Scat Feathers
Normality of distribution for each element and stable isotope was tested using the Shapiro Wilk test. Parametric assumptions were violated for Mg and δ15N regardless of transformation type. Therefore, to apply a consistent statistical approach to all elements and stable isotopes for multivariate analyses, non-parametric tests were used. Biochemical signatures were compared between sites using a two-factor PERMANOVA design for each element and isotope individually and then all signatures combined (Anderson, 2001). Data were normalised prior to constructing resemblance matrices based on Euclidean distance dissimilarity and analysed using unrestricted permutation with 9999 random repeats. No significant differences were detected between the two sites sampled in Western Bass Strait, Deen Maar Island and Cape Nelson. Due to the close proximity of the sites and uniform Western Bass Strait regional representation, the seven samples from each site were pooled for comparison to the remaining five sites. Boxplots were used to display the median and lower (Q1) and upper (Q3) quartiles for each trace element and stable isotope per site. Outliers in the multivariate baseline data were identified using Principle Component Analysis (PCA) and feathers that were beyond a 95% confidence ellipse were excluded from further analysis (Figure S1). Multivariate data were then reduced to two-dimensions and visualized using canonical analysis of principal coordinates (CAP) (Anderson and Willis, 2003). Canonical axes (CAP1 and CAP2) represent linear combinations of the orthonormal principal coordinate axes that best discriminate feather biochemistry by site or region. Vector diagrams in each canonical plot show the influence of individual elements and stable isotopes to sample positioning in multivariate space. The relative length and direction of each vector correspond to its discriminatory ability.
Signatures of feathers collected from scats were added to the CAP plot as unknown samples and leave-one-out cross validation was used to classify a source colony based on the multi-elemental signals of each scat feather sample. Accurate source colony allocations of scat feathers relies on the assumption that all possible colony sources across Bass Strait and Port Phillip Bay have been included in the baseline data set (Campana, 1999). All statistical analyses were executed using R version 4.2.3 (R Core Team, 2020) and PRIMER (v. 7.0.13; Auckland, NZ).
Results
Feeding Trial
No significant variation was detected among stable isotope signatures between undigested and digested feathers, from two penguin carcasses; δ13C (n = 3, t = 1.47 df = 2, P = 0.28), (n = 6, t = 0.74, df = 5, P = 0.49), and δ15N (n = 3, t = 0.63, df = 2, P = 0.59), (n = 6, t = 0.92, df = 5, P = 0.40) (Figure 3). Comparison of undigested and digested feathers from one carcass also yielded similar means for trace elements Mg (t = -0.70 df = 6, P = 0.51), Al (t = -0.72 df = 6, P = 0.50), Ti (t = 0.94 df = 6, P = 0.38), Sr (t = 0.05 df = 6, P = 0.96) (Figure 4). However, we did detect a significant difference (P < 0.05 for each) in B, Na, Fe, Cu, Zn, Se, V and Mn between undigested and digested feathers and we therefore excluded these from further analyses of penguin colony or seal scat feathers.
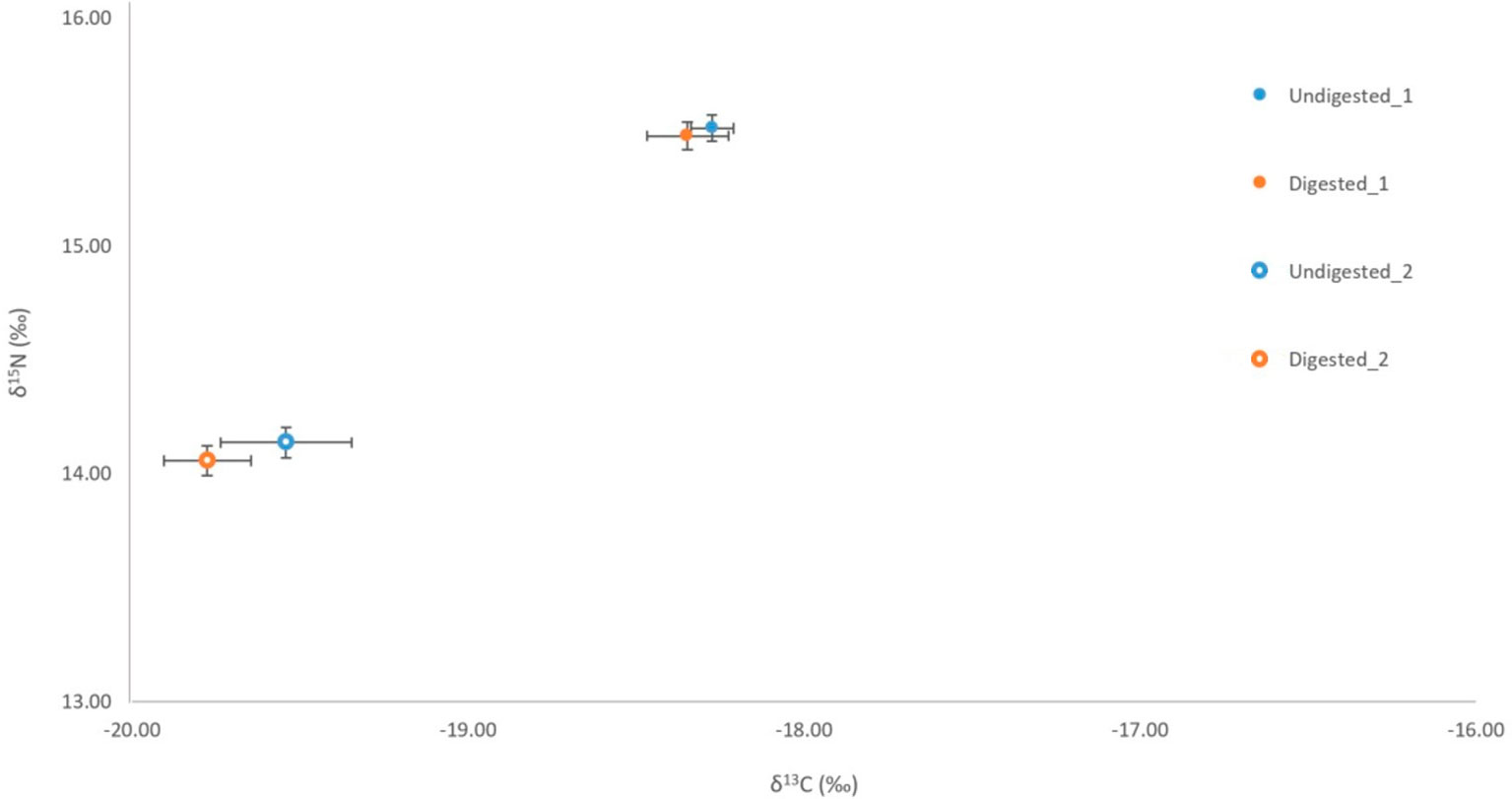
Figure 3 Comparison of δ13C and δ15N signatures for undigested (penguin 1 n= 6, penguin 2 n=6) and digested (penguin 1 n= 3, penguin 2 n=3) feathers of little penguins consumed by captive Australian and long-nosed fur seals.
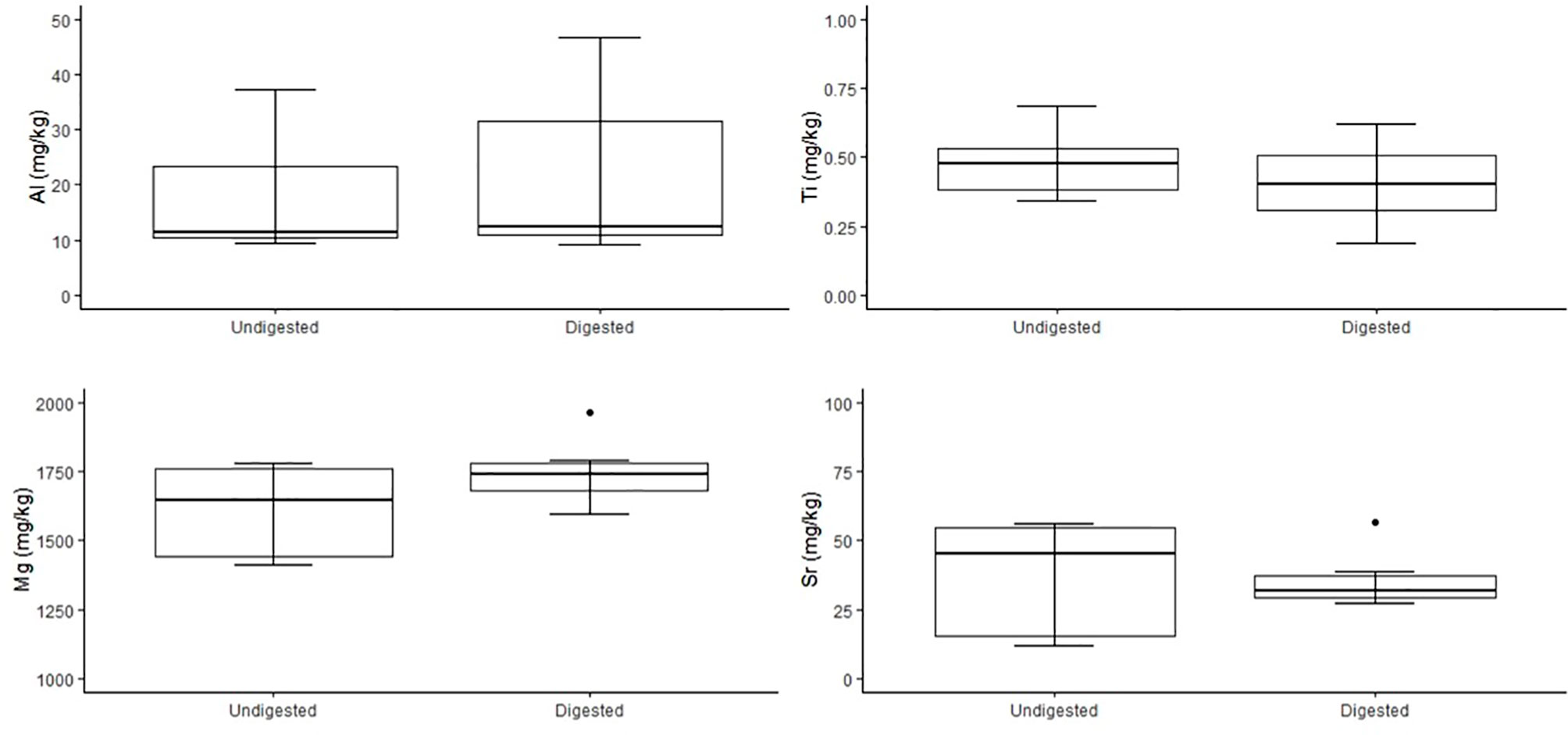
Figure 4 Comparison of concentrations of trace elements Al, Ti, Mg and Sr (mg/kg dry weight) of undigested (n = 7) and digested (n = 7) feathers from carcass of n = 1 little penguin. Box plots display median values with box edges representing lower (Q1) and upper (Q3) quartiles, defined as the 25th and 75th percentiles, whiskers representing variability outside the upper and lower quartiles and dots representing outliers.
Penguin Baseline ‘Feather-Prints’
Individual Chemistry Signatures
Trace element and stable isotope concentrations isolated for comparison with scat feathers for the six penguin locations sampled across four regions (Western, Central and Eastern Bass Strait, and Port Phillip Bay) are shown in the supplementary materials (Table S1). One-way MANOVA detected a significant difference in the distribution of mean stable isotope values and trace element concentrations in moulted feathers at both regional and site scales. Mean Ti concentrations in feathers showed the greatest difference between Western Bass Strait and other regions, with feathers containing on average 3.1, 5.4, and 8.3 times more Ti than feathers from Port Phillip Bay, Central and Eastern Bass Strait respectively. Similar mean Al concentrations were detected for feathers from Western Bass Strait sites and Port Phillip Bay (p = 0.52) with both regions demonstrating a minimum of Al levels two-fold higher than Central and Eastern Bass Strait sites (Figure 5). Western Bass Strait demonstrated similar Sr concentrations to all regions (p >0.05 for all) but significantly different Mg concentrations at a regional scale (p <0.05 for all). Port Phillip Bay δ15N and δ13C values differed significantly to all other regions (p = 0.00 for all) (Figure S1, S2), whilst regionally Western, Central and Eastern Bass Strait resulted in statistically similar values for both stable isotopes (p >0.05 for all). At a site level, Kanowna Island δ15N values differed significantly to all sites (all p <0.05) with the exception of Gabo Island (p = 0.06). For δ13C, Kanowna and Rabbit Island also differed (p = 0.00) but all other sites resulted in statistically similar values when compared to one another across the three Bass Strait regions (p >0.05 for all).
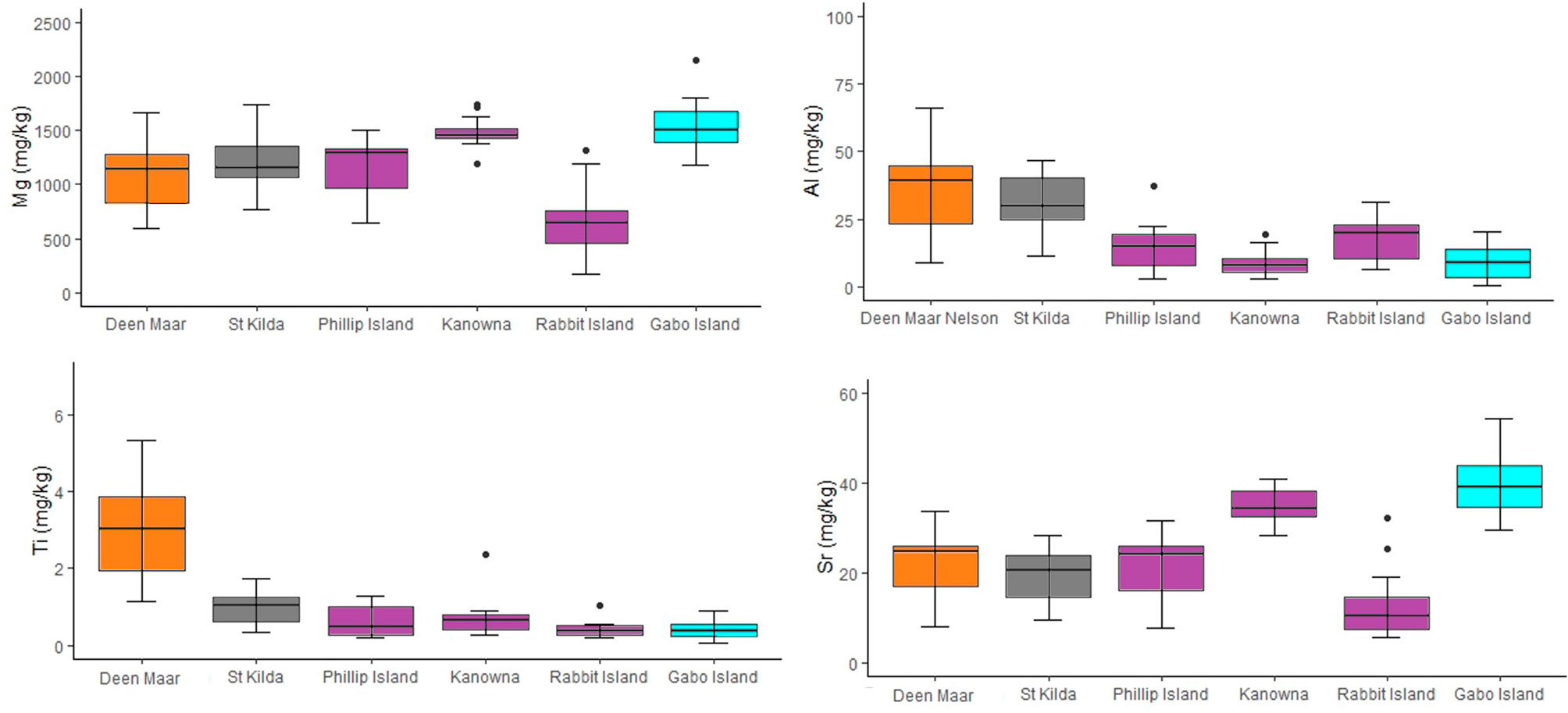
Figure 5 Concentrations for trace elements Al, Ti, Mg and Sr (mg/kg dry weight) from moulted feathers of little penguins collected from Deen Maar Island and Cape Nelson (WBS), St. Kilda (PPB), Phillip Island (CBS), Kanowna Island (CBS), Rabbit Island (CBS) and Gabo Island (EBS). Box plots display median values with box edges representing lower (Q1) and upper (Q3) quartiles, defined as the 25th and 75th percentiles, whiskers representing variability outside the upper and lower quartiles and dots representing outliers. The Y-axis for each element differs and colors of box plots correspond to colors presented in Figure 1.
Multivariate Results
There was considerable variation in the elemental concentrations and stable isotope values in baseline feathers that resulted in biochemical differentiation at both regional and site-specific scales (Figure 6). With the exception of three feathers (1 per site; Deen Maar, Rabbit Island and Gabo Island), PCO analysis resulted in the distribution of all baseline feathers within the 95% confidence ellipse (Figure S3). Overall, CAP analysis resulted in regional classification success for 85% of baseline feathers and 78% at colony-specific scales (Figure 6). Western Bass Strait (93.3% *correct classification) and Port Phillip Bay (92.9% *) resulted in the highest correct classifications with dissimilarity measures predominantly driven by high Ti and δ15N signatures respectively. Both regions also demonstrated high Al levels compared to baseline feather signatures from Central and Eastern Bass Strait (Figure 5). Central (82.1% *) and Eastern Bass Strait (76.9% *) baseline data shared similar Mg and Sr concentrations with δ13C predominantly driving variation between the two regions. The relative length and direction of each vector corresponding to the discriminatory ability of each biochemical signature remained largely consistent between site and regional CAP comparisons. For sites, St. Kilda (92.9% *) resulted in the highest proportion of correct classifications (thereafter referred to as site or regional ‘biochemical resolution’) followed by Deen Maar Island (86.7% *), Kanowna Island (78.6% *), Rabbit Island (72.7% *), Gabo Island (69.2% *) and Phillip Island (64.3% *) (Figure 6).
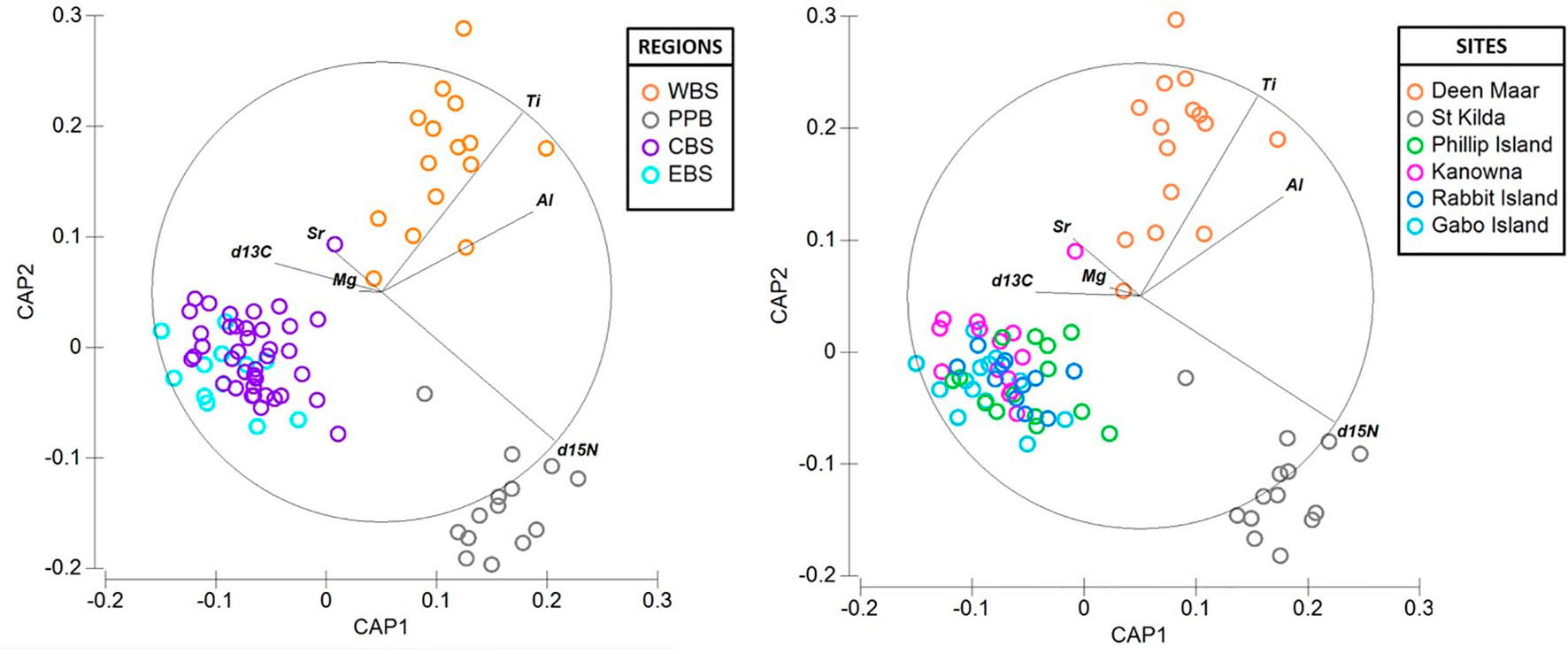
Figure 6 Canonical variate plots of the multi-elemental and isotope chemistry of feathers of little penguins sampled across four regions; (A) Western (WBS), Central (CBS) and Eastern Bass Strait (EBS) and Port Phillip Bay (PPB) and (B) sites; Deen Maar Island, St. Kilda, Phillip Island, Kanowna Island, Rabbit Island and Gabo Island. Vector diagrams show the direction and weight of individual isotopes and elements to sample distribution.
Scat Feathers – Source Penguin Colony
All signatures of scat feathers overlapped with the baseline data of which 32.4% were assigned to penguins from Kanowna Island (Central Bass Strait), followed by 27.0% from Gabo Island (Eastern Bass Strait). Rabbit Island (Central Bass Strait), Deen Maar Island (Western Bass Strait) and Phillip Island (Central Bass Strait) each made up 18.9%, 13.5% and 8.1% of scat feathers respectively. St. Kilda (Port Phillip Bay) penguins however, remained undetected from the scats collected across the three regions in this study (Figure 7). Local penguin colonies accounted for 46-70% of predated feathers. These local colonies either (1) co-exist with the long-nosed fur seals we sampled or (2) occur in closest proximity, relative to other sampled colonies, to the sampled seal site (Figure 8).
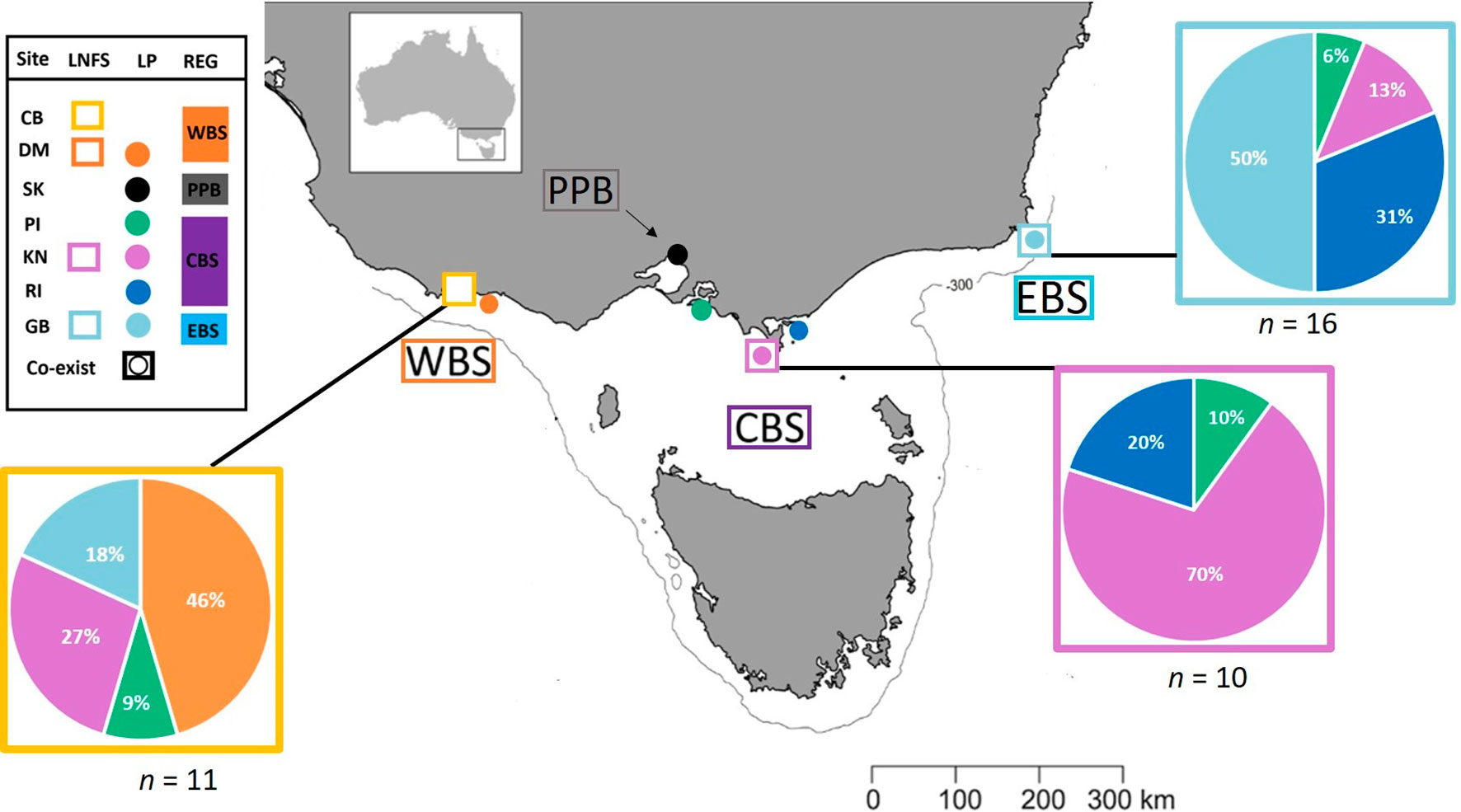
Figure 7 Proportional (%) contribution of source-colonies assigned to little penguin feathers retrieved from scats of long-nosed fur seals. Plots show results of canonical variate analysis of baseline multi-elemental and isotope signatures from penguin colonies sampled across Western (WBS), Central (CBS) and Eastern Bass Strait (EBS), and Pt. Phillip Bay (PPB); Deen Maar Island and Cape Nelson (DM); St. Kilda (SK); Phillip Island (PI); Kanowna Island (KN); Rabbit Island (RI); Gabo Island (GB). Scats of long-nosed fur seals were sampled between October – November 2018.
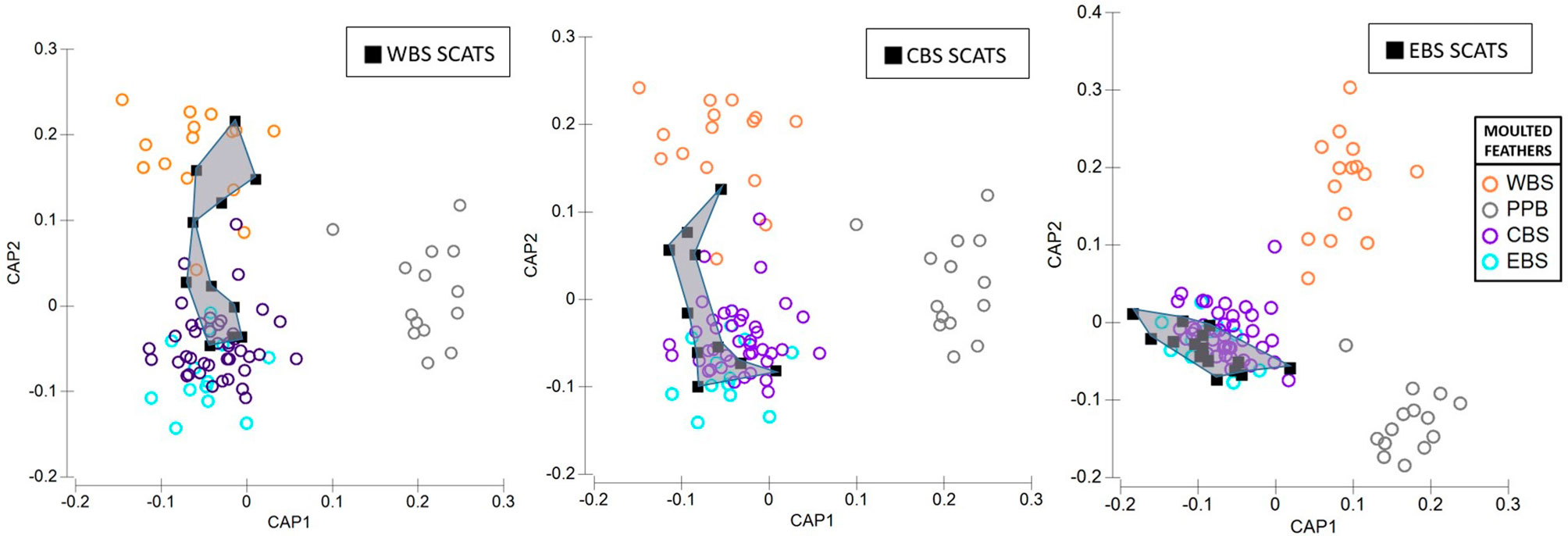
Figure 8 Canonical variate plots of the multi-elemental and isotope chemistry of feathers sampled from colonies of little penguins across Western (WBS), Central (CBS), Eastern Bass Strait (EBS), and Pt. Phillip Bay (PPB) (indicated by circles). Squares with convex hulls represent feathers retrieved from long-nosed fur seal scats sampled from (A) Cape Bridgewater (WBS), (B) Kanowna Island (CBS) and (C) Gabo Island (EBS).
The proportion of predated feathers assigned to regional and inter-regional penguin colonies, relative to the site of long-nosed fur seal scat collection, varied across the three regions (Figure 7). Whilst predated feathers from Western Bass Strait were exclusively detected in Cape Bridgewater (Western Bass Strait) scats, the remaining 54% of scat feathers resulted in the highest inter-regional diversity of penguins including Central Bass Strait colonies; Kanowna Island (27%) and Phillip Island (9%), as well as Eastern Bass Strait, Gabo Island (18%), feathers. Comparatively, scat feathers from Kanowna Island (Central Bass Strait) resulted in the highest occurrence of localised predation with 70% of feathers assigned to Kanowna Island penguins and the remaining 30% attributed to regional colonies; Rabbit Island (20%) and Phillip Island (10%) (Figure 8). Complimentary to the 50% of Gabo scat feathers allocated to local Gabo Island penguins, the remaining predated feathers were assigned to originating from inter-regional Central Bass Strait colonies; Rabbit Island (31%), Kanowna Island (13%) and Phillip Island (6%) (Figure 8).
Discussion
This study was able to chemically discriminate moulted penguin feathers among sites across Bass Strait and Port Phillip Bay. Using these baseline data, moulted feathers were assigned to their known region or colony of origin with 85% and 78% accuracy, respectively. Variance in moulted feather signatures may reflect system-specific, bottom-up influences including foraging specialisation in areas of high productivity, as well as potential industrial and oceanographic factors within or adjacent to the respective penguin foraging zones.
Across the three regions sampled for long-nosed fur seal scats, 46-70% of feathers collected from scats indicated patterns of ‘local’ penguin predation. Inter-regional predation of penguins, relative to the site of long-nosed fur seal scat collection, were detected in Eastern and Western Bass Strait scats, while Central Bass Strait scats were solely assigned to ‘local’ and regional penguins. The latter was likely explained by a higher bioavailability of penguins in Central Bass Strait. In comparison, Western Bass Strait birds were predated at a localised scale only. Whereas St. Kilda penguins, known to forage exclusively in Port Phillip Bay and situated furthest from long-nosed fur seal sites (relative to other penguin colonies sampled in this study), remained undetected in all scats. Potential drivers shaping localised and inter-regional predation (or lack thereof) are described with reference to penguin colony proximity to seal sites, areas of high productivity and demographic-specific species foraging movements.
Baseline Signatures From Moulted Penguin Feathers
Results of feeding trials supported the biochemical comparability between baseline signatures from moulted and scat feathers. Arregui et al. (2018) also reported fin whale scats as a reliable indicator of prey consumption with unaltered stable isotope ratios of krill post transit along the digestive tract. The afore mentioned biochemical comparisons between wild deer hair and predated hair from the scats of wolves, further highlights the application of such methods across marine and terrestrial predator-prey relationships (Derbridge et al., 2012).
Across Bass Strait and Port Phillip Bay, moulted feathers from St. Kilda retained stable isotope and trace element signatures with the highest overall discriminatory resolution (92.9% correct classification). Key biochemical signatures discriminating moulted feathers from St. Kilda, included elevated δ15N and Al levels. St. Kilda penguins are known to forage exclusively within PPB, specialising on juvenile anchovy and luminous bay squid with high δ15N values (Preston et al., 2008; Chiaradia et al., 2012). Consistent with the findings of this study, Kowalczyk et al. (2015) detected high δ15N levels in St. Kilda penguin feathers (Table S1). Finger et al. (2015) also detected elevated Aluminium (22% higher than this study), Arsenic and Mercury concentrations in St. Kilda penguins compared to other Central Bass Strait breeding sites (Phillip Island and Notch Island) (Table S1). Aluminum impairment in seabirds is mainly related to its disruptive effect on calcium homeostasis as well as phosphorus metabolism, ultimately leading to muscle weakness and decreased growth rates (Scheuhammer, 1987). Port Phillip Bay is host to the city of Melbourne’s business district, and the restricted currents and wave action in the bay may act as a contamination hotspot for the heavy metals subsequently reflected in elevated levels amongst St. Kilda penguin feathers (Aly et al., 2013).
Elevated Al but also Ti concentrations discriminated moulted feathers from Western Bass Strait from penguin colonies in Central and Eastern Bass Strait. The bioaccumulation of anthropogenic and natural sources of both Ti and Al represent potential risks to seabirds such as penguins that forage at higher trophic levels (Scheuhammer, 1987; Finger et al., 2015; Walsh, 2018; Hauser-Davis et al., 2020). Titanium, which is often depleted in surface waters but elevated in deep water, and which can occur over a range of at least two orders of magnitude, has been described as a potential tracer of chemical transfer processes in open oceans (Dammshäuser et al., 2011). Penguin colonies from Western Bass Strait in this study are situated within the eastern bounds of the Bonney Upwelling system – an important source of transfer for deep and nutrient rich waters to the surface (Middleton and Bye, 2007). However, very little is known about the biological function of Ti and Al, or the biogeochemical processes (like coastal upwelling) that may control its distribution in the marine environment.
We highlight two local sources (~40km from Western Bass Strait penguin colonies) of potential industrial discharge for both Ti and Al; (1) the Portland Aluminium Smelter and (2) the commercial Port of Portland. Emissions from the Portland Aluminium Smelter, with a production capacity of 345,000 tonnes of Al per year, can enter coastal waters via contaminated particulate matter and effluent discharge that potentially biomagnify up the marine trophic food chain (Radhalakshmiet al., 2014; Sun et al., 2020). For Ti, potential local entry points within Western Bass Strait include transport via the mineral sands from the Port of Portland, from which titanium dioxide (Ti0₂) is derived (Force, 1991). In 2017, for example, approximately 490,000 tonnes of mineral sands were reported as imported/exported via the Port of Portland. In its nanoparticulate (matter between 1-100nm) form, Ti02 is used in a wide range of products (i.e. sunscreen, paints, cements, care cosmetics) and considered a contaminant of emerging concern - particularly for aquatic ecosystems (Weir et al., 2012; Shi et al., 2013; Hauser-Davis et al., 2020). Miller et al. (2012) demonstrated that relatively low levels of ultraviolet light, consistent with those found in nature, can induce toxicity of Ti0₂ nanoparticles to marine phytoplankton. However, very little is known about the potential effects of Ti0₂ travelling up the trophic chain.
In comparison to Western Bass Strait and Port Phillip Bay (93% and 92% correct classification, respectively), a higher level of biochemical homogeneity was detected between Central (82.1%) and Eastern Bass Strait (76.9%) sites (Figure 6A). This may reflect overlapping foraging zones and/or the confluence of currents meeting in Central Bass Strait reducing the discriminatory biochemical resolution between the two regions (Ridgway, 1997; Sandery and Kämpf, 2007). For example, oligotrophic, low nutrient waters from the East Australian Current (EAC) flow southward along the eastern edge of Bass Strait and the South Australian Current (SAC) advects warm water from the west that flows eastward through Bass Strait (Sandery and Kämpf, 2007). Pre-moult Eastern Bass Strait penguins at Gabo Island, unconstrained by chick feeding requirements, may travel along the EAC towards other sources of nutrient rich inputs into Central Bass Strait.
Overall, biochemical variation in moulted penguin feathers between the four regions provide baseline signatures at high spatial resolution that reflect variation in distance to areas of high productivity and consequent foraging strategies, as well as natural and potentially land derived anthropogenic sources of elevated contaminants. Interestingly, these baseline signatures also highlight the complexity of threats penguins encounter, including potential terrestrial sources of contaminants, at system-specific levels.
Bioavailability and Proximity Influences Predation Pressure
Both, long-nosed fur seals and penguins are highly mobile marine predators demonstrating vastly different foraging strategies which are broadly shaped by system-specific cycles of productivity and life history constraints (Page et al., 2005; Sidhu et al., 2012; Pelletier et al., 2014; Foo et al., 2019). Feathers originating from St. Kilda penguins were undetected from scats, likely explained by the specialised foraging that occurs from this colony throughout the year within the confines of Port Phillip Bay (Preston et al., 2008; Chiaradia et al., 2012). We note that long-nosed fur seals do not commonly within Port Phillip Bay. Furthermore, St. Kilda penguins have experienced a decline since their estimated peak population size of 1061 adults in 2015, with estimates of, 998 adults in 2016, 738 adults in 2017, and 694 adults in 2018 (F. Sperring, unpublished data). Overall, between 46-70% of predated feathers retrieved from scats across Bass Strait were assigned to ‘local’ penguins (Figure 8). Most likely, this reflects overlapping marine foraging zones for the animals with shared or close terrestrial habitat. Both species utilise epipelagic coastal waters, but the long-nosed fur seals also forage in offshore waters (Collins et al., 1999; Baylis et al., 2008; Salton et al., 2021). For example, Salton et al. (2021) recently tracked a sub-adult male fur seal from Montague Island, New South Wales, to the Nee Islets, in southern New Zealand. During our study, scat sampling occurred during the penguin-breeding season (Oct-Dec 2018) when adult penguins across Victoria typically make one-day foraging trips (Chiaradia, 1999), typically within a 30 km radius of their breeding site (Collins et al., 1999). Consequently, we can expect that local breeding penguins are more bioavailable to nearby long-nosed fur seals when constrained by mate and chick feeding requirements.
The highest level of localised predation was detected for Kanowna Island (Central Bass Strait), where predated feathers were solely allocated to Kanowna penguins (70%) or other colonies within the Central Bass Strait region (30%). Relative to Eastern and Western Bass Strait, Central Bass Strait has a greater abundance of penguins - proportionally increasing the bioavailability of Central Bass Strait penguins within the region (Dann pers comm). For both Central and Eastern Bass Strait, the proportion of different feather signatures found in the scats correlated to the proximity of source penguin colonies. Both regions displayed patterns of increased predation pressure on local, and then regional or inter-regional penguins, with proximity to seal sites. For example, in addition to the birds originating from Kanowna Island (70%), the proportional contribution of Rabbit Island (20%) and Phillip Island (10%) penguins to scats collected from Central Bass Strait correlated with the colonies proximity (~45km and ~120km respectively) to Kanowna Island. For Eastern Bass Strait, local penguins comprised 50% of predated birds. The remaining birds were assigned to inter-regional Central Bass Strait penguins; Rabbit (31%), Kanowna (13%) and Phillip Island (6%). These three colonies are located ~330km, ~370km and ~500km from Gabo Island, respectively. Overall, the largest proportion of scat feathers collected across Bass Strait originated from Kanowna and Gabo Island penguin colonies, the two largest penguin colonies co-existing with long-nosed fur seals (Reinhold, S. unpublished data). The combination of high penguin bioavailability in close proximity to seal sites may therefore place Kanowna and Gabo Island colonies at greater risk of seal predation. We note however, population level inferences about penguin colonies at greatest risk to seal predation require an increased sample size of scat feathers sampled across a broad temporal spectrum.
Species and Demographic-Specific Foraging Movements
While understanding where predatory events on penguins occur is beyond the scope of this study, the presence of inter-regional penguins in scats from Western and Eastern Bass Strait may reflect sexually immature penguins (<2yrs) which are more likely to demonstrate a more versatile foraging strategy that includes occurrence in these regions (Dann et al., 1992). Unlike breeding penguins, juveniles are more likely to travel further post-fledging and during penguin breeding months. However, the exclusive occurrence of Western Bass Strait penguins in scats from Western Bass Strait at Cape Bridgewater (46% of Western Bass Strait scats feathers) may suggest that juvenile as well as breeding birds from Western Bass Strait undertake more localised foraging strategies. The nutrient rich Bonney Upwelling system extends around the Western Bass Strait penguin colonies and provides an abundance of nearby food. This constrained spatial distribution of predated penguins from Western Bass Strait is consistent with dispersal patterns described for banded penguins from Western Bass Strait colonies (Norman et al., 2017). On average, banded penguins recovered from 20 colonies between Lorne and Portland (320km range) in Western Bass Strait moved ~38km from their original banding site (Norman et al., 2012). Phillip Island penguins also travel west post fledging, attributed to the access of nutrient-rich Bonney upwelling waters (Reilly and Cullen, 1982; Dann et al., 1992). Recoveries of flipper-banded first-year birds from Phillip Island indicate that they travel several hundred kilometers west between Warrnambool and Port MacDonnell, situated in the Bonney Upwelling 250km and 420 km from Phillip Island (Dann et al., 1992).
An equally plausible explanation for the occurrence of inter-regional (adult or juvenile) penguins in seal scats is long-distance movements by long-nosed fur seals (up to 220 km per day (Salton et al., 2021). Long-nosed fur seals retain prey for an estimated gut passage half time of 51 hours – prior to defecating scats (Fea and Harcourt, 1997). This represents the amount of time it takes for half the total of recovered prey remains to reappear in scats. Consequently, seals arriving from distant foraging grounds could still be digesting penguins sourced from inter-regional predation events upon their return to the site of scat collection. However, the proportional contribution of inter-regional penguins detected in this study should be considered as a minimum - as scats defecated at sea are likely to retain a higher proportion of hard-parts originating from prey, including penguin feathers, consumed in offshore waters.
Conclusion
This is the first study to develop a diagnostic tool for identifying seabird colonies at greatest risk to pinniped predation. Interestingly, the biochemical signatures that best discriminated between penguin colonies were shaped by system-specific dynamics including proximity to areas of high ocean productivity, human development, and industrialization, thus highlighting the inter-section of terrestrial and marine threats that penguins encounter. We propose future studies also explore the resilience of Hg concentrations in feathers to seal digestion, potentially enhancing the future biochemical resolution of colony-specific baselines.
Overall, seals were more likely to prey upon the penguins breeding in closest proximity to them, consistent with the constrained foraging patterns displayed by adult penguins during the breeding season – when the seal scats were collected. Region-specific predator-prey dynamics were likely tied to the proximity of penguin colonies to seals sites and the abundance or bioavailability of penguins. Meanwhile, inter-regional predator-prey dynamics may reflect variation in the dispersion of seals or juvenile penguins.
This study also suggests that penguin colonies may still be in decline despite a lack of seal predation. In such cases, other natural and anthropogenic stressors, for example industrialisation, shown here by heavy metal loads in feathers, may warrant further research to understand their contribution to population declines. As the factors that influence predation, or lack thereof, vary across regions and among local sites, this study highlights the value and importance of identifying the source colony of seabirds predated by pinnipeds to inform effective management efforts and outcomes.
Data Availability Statement
The raw data supporting the conclusions of this article will be made available by the authors, without undue reservation.
Ethics Statement
The animal study was reviewed and approved by University of Adelaide Animal Ethics Committee (S2017-106) and the Phillip Island Nature Park Animal Ethics Committee (2.2016).
Author Contributions
S-LR, SG, BG, SC, and RM conceived and designed the study. S-LR, JA, and RM assisted with sample collection. S-LR wrote the manuscript, performed the laboratory processing and sample preparation, collected and analysed the data, and applied the statistical analyses. All authors contributed to the article and approved the submitted version.
Funding
Operating costs were largely funded by a Penguin Foundation Grant and Holsworth Endowment Grant (Ecological Society of Australia). Penguin feather chemistry analysis (ICP-MS and Mass Spectrometre) was funded by both a Holsworth Wildlife Research Endowment Grant (Ecological Society of Australia) and Nature Foundation Grand Start Grant awarded to S-LR.
Conflict of Interest
The authors declare that the research was conducted in the absence of any commercial or financial relationships that could be construed as a potential conflict of interest.
Publisher’s Note
All claims expressed in this article are solely those of the authors and do not necessarily represent those of their affiliated organizations, or those of the publisher, the editors and the reviewers. Any product that may be evaluated in this article, or claim that may be made by its manufacturer, is not guaranteed or endorsed by the publisher.
Acknowledgments
We thank Phillip Island Nature Parks (PINP) staff M. Van Polanen Petel, P. Wasiak and L. Renwick for collecting feather samples from Phillip Island. Gabo Island samples were collected by T. Mitchell and L. Op Den Bruow. For samples collected from Rabbit Island we thank A. Chiaradia (PINP), Z. Chiaradia, R. Faulkner (PINP) and I. Van Vilet (Parks Victoria) with transport provided by Geoff Boyd from Prom Adventurer Boat Charters. Feathers from St. Kilda were collected by volunteers from Earthcare St. Kilda, with special acknowledgments to Flossy Sperring. We also thank skipper Vinnie Antony from Seals by Sea tours for travel to sites across West Bass Strait. A. Formant provided support in collecting samples from Kanowna Island.
We thank S. Gilbert (Adelaide Microscopy, Australia) for operating the ICP-MS and guidance in sample preparation requirements. Dr. T Hall and Dr. R. Klaebe (University of Adelaide Biogeochemistry Facility) operated the Mass Spectrometre and provided support in sample preparation techniques. Finally, we thank J. Gomes and Justin Valentine from the Melbourne Zoo for their support in facilitating and co-ordinating the seal feeding trials. Additional logistic support was also provided by the University of Adelaide, Phillip Island Nature Parks and the South Australian Research Development Institute (Aquatic Sciences).
Supplementary Material
The Supplementary Material for this article can be found online at: https://www.frontiersin.org/articles/10.3389/fmars.2022.813106/full#supplementary-material
References
Ainley D. G., Ballard G., Karl B. J., Dugger K. M. (2005). Leopard Seal Predation Rates at Penguin Colonies of Different Size. Antarctic Sci. 17, 335–340. doi: 10.1017/S0954102005002750
Aly W., Williams I. D., Hudson M. D. (2013). Metal Contamination in Water, Sediment and Biota From a Semi-Enclosed Coastal Area. Environ. Monitoring Assess. 185, 3879–3895. doi: 10.1007/s10661-012-2837-0
Anderson M. J. (2001). A New Method for Non-Parametric Multivariate Analysis of Variance. Austral Ecol. 26, 32–46. doi: 10.1111/j.1442-9993.2001.01070.pp.x
Anderson M. J., Willis T. J. (2003). Canonical Analysis of Principal Coordinates: A Useful Method of Constrained Ordination for Ecology. Ecology 84, 511–525. doi: 10.1890/0012-9658(2003)084[0511:CAOPCA]2.0.CO;2
Arregui M., Borrell A., Víkingsson G., Ólafsdóttir D., Aguilar A. (2018). Stable Isotope Analysis of Fecal Material Provides Insight Into the Diet of Fin Whales. Marine Mammal Sci. 34, 1059–1069. doi: 10.1111/mms.12504
Baylis A. M. M., Page B., Goldsworthy S. D. (2008). Effect of Seasonal Changes in Upwelling Activity on the Foraging Locations of a Wide-Ranging Central-Place Forager, the New Zealand Fur Seal. Can. J. Zool. 86, 774–789. doi: 10.1139/Z08-055
Bearhop S., Waldron S., Thompson D., Furness R. (2000). Bioamplification of Mercury in Great Skua Catharacta Skua Chicks: The Influence of Trophic Status as Determined by Stable Isotope Signatures of Blood and Feathers. Marine Pollution Bull. 40, 181–185. doi: 10.1016/S0025-326X(99)00205-2
Bethge P., Nicol S., Culik B., Wilson R. (1997). Diving Behaviour and Energetics in Breeding Little Penguins (Eudyptula Minor). J. Zool. 242, 483–502. doi: 10.1111/j.1469-7998.1997.tb03851.x
Campana S. E. (1999). Chemistry and Composition of Fish Otoliths: Pathways, Mechanisms and Applications. Marine Ecol. Prog. Ser. 188, 263–297. doi: 10.3354/meps188263
Cherel Y., Hobson K. (2007). Geographical Variation in Carbon Stable Isotope Signatures of Marine Predators: A Tool to Investigate Their Foraging Areas in the Southern Ocean. Marine Ecol. Prog. Ser. 329, 281–287. doi: 10.3354/meps329281
Cherel Y., Jaquemet S., Maglio A., Jaeger A. (2014). Differences in Δ13c and Δ15n Values Between Feathers and Blood of Seabird Chicks: Implications for Non-Invasive Isotopic Investigations. Marine Biol. 161, 229–237. doi: 10.1007/s00227-013-2314-5
Chiaradia A. K. (1999). Daily Nest Attendance and Breeding Performance in the Little Penguin Eudyptula minor at Phillip Island, Australia. Marine Ornithol. 27, 13–20.
Chiaradia A., Forero M. G., Hobson K. A., Swearer S. E., Hume F., Renwick L., et al. (2012). Diet Segregation Between Two Colonies of Little Penguins Eudyptula minor in Southeast Australia. Austral Ecol. 37, 610–619. doi: 10.1111/j.1442-9993.2011.02323.x
Collins M., Cullen J., Dann P. (1999). Seasonal and Annual Foraging Movements of Little Penguins From Phillip Island, Victoria. Wildlife Res. 26, 705–721. doi: 10.1071/WR98003
Coplen T. B., Brand W. A., Gehre M., Gröning M., Meijer H. A., Toman B., et al. (2006). New Guidelines for Δ 13C Measurements. Analytical Chem. 78, 2439–2441. doi: 10.1021/ac052027c
Croxall J. P., Butchart S. H. M., Lascelles B. E. N., Stattersfield A. J., Sullivan B. E. N., Symes A., et al. (2012). Seabird Conservation Status, Threats and Priority Actions: A Global Assessment. Bird Conserv. Int. 22, 1–34. doi: 10.1017/S0959270912000020
Dammshäuser A., Wagener T., Croot P. L. (2011). Surface Water Dissolved Aluminum and Titanium: Tracers for Specific Time Scales of Dust Deposition to the Atlantic? Geophysical Res. Lett. 38, 24–30. doi: 10.1029/2011gl049847
Dann P., Cullen J., Thoday R., Jessop R. (1992). Movements and Patterns of Mortality at Sea of Little Penguins Eudyptula Minor From Phillip Island, Victoria. Emu-Austral Ornithol. 91, 278–286. doi: 10.1071/MU9910278
Derbridge J. J., Krausman P. R., Darimont C. T. (2012). Using Bayesian Stable Isotope Mixing Models to Estimate Wolf Diet in a Multi-Prey Ecosystem. J. Wildlife Manage. 76, 1277–1289. doi: 10.1002/jwmg.359
Dias M. P., Martin R., Pearmain E. J., Burfield I. J., Small C., Phillips R. A., et al. (2019). Threats to Seabirds: A Global Assessment. Biol. Conserv. 237, 525–537. doi: 10.1016/j.biocon.2019.06.033
Du Toit M., Bartlett P., Bester M., Roux J. (2004). Seabird Predation by Individual Seals at Ichaboe Island, Namibia. South Afr. J. Wildlife Res. 34, 45–54. doi: 10.10520/EJC117184
Fea N., Harcourt R. (1997). Assessing the Use of Faecal and Regurgitate Analysis as a Means of Determining the Diet of New Zealand Fur Seals, in Marine Mammal Research of the Southern Hemisphere, Eds. Hindell M, Kemper C. (Norton, NSW: Surrey Beatty & Sons Chipping). 143–150.
Finger A., Lavers J. L., Dann P., Nugegoda D., Orbell J. D., Robertson B., et al. (2015). The Little Penguin (Eudyptula Minor) as an Indicator of Coastal Trace Metal Pollution. Environ. Pollution 205, 365–377. doi: 10.1016/j.envpol.2015.06.022
Foo D., Mcmahon C., Hindell M., Goldsworthy S., Bailleul F. (2019). Influence of Shelf Oceanographic Variability on Alternate Foraging Strategies in Long-Nosed Fur Seals. Marine Ecol. Prog. Ser. 615, 189–204. doi: 10.3354/meps12922
Force E. R. (1991). Geology of Titanium-Mineral Deposits Geol. Soc. Am. Bull. 259, 3–6. doi: 10.1130/SPE259-p1
Fromant A., Schumann N., Dann P., Cherel Y., Arnould J. P. (2020). Trophic Niches of a Seabird Assemblage in Bass Strait, South-Eastern Australia. PeerJ 8, e8700. doi: 10.7717/peerj.8700
Furness R., Muirhead S., Woodburn M. (1986). Using Bird Feathers to Measure Mercury in the Environment: Relationships Between Mercury Content and Moult. Marine Pollution Bull. 17, 27–30. doi: 10.1016/0025-326X(86)90801-5
Gales R., Green B., Stahel C. (1988). The Energetics of Free-Living Little Penguins Eudyptula Minor (Spheniscidae), During Molt. Aust. J. Zool. 36, 159–167. doi: 10.1071/ZO9880159
Gibbs C., Arnott G., Longmore A., Marchant J. (1991). Nutrient and Plankton Distribution Near a Shelf Break Front in the Region of the Bass Strait Cascade. Marine Freshwater Res. 42, 201–217. doi: 10.1071/MF9910201
Gibbs C., Tomczak M. Jr., Longmore A. (1986). The Nutrient Regime of Bass Strait. Marine Freshwater Res. 37, 451–466. doi: 10.1071/MF9860451
Goldsworthy S. D., Bailleul F., Nursey-Bray M., Mackay A., Oxley A., Reinhold S.-L., et al. (2019a). Assessment of the Impacts of Seal Populations on the Seafood Industry in South Australia (Adelaide: South Australian Research and Development Institute (Aquatic Sciences).
Goldsworthy S. D. S., Smart J., Mackay A., Bailleul F., Reinhold S.-L., Stonnill M., et al. (2019b). “Monitoring of Seal Bay and Other Pinniped Populations on Kangaroo Island: 2017/2018,” in Report to the Department for Environment and Water (Adelaide: South Australian Research and Development Institute Aquatic Sciences). SARDI Publication No. F2014/000322-5. SARDI Research Report Series No. 1018 59pp.
Hauser-Davis R. A., Monteiro F., Da Rocha R. C. C., Lemos L., Cardoso M. D., Siciliano S. (2020). Titanium as a Contaminant of Emerging Concern in the Aquatic Environment and the Current Knowledge Gap Regarding Seabird Contamination. Ornithologia 11, 7–15.
Hobson K. A., Clark R. G. (1992). Assessing Avian Diets Using Stable Isotopes I: Turnover of 13C in Tissues. Condor 94, 181–188. doi: 10.2307/1368807
Hobson K. A., Piatt J. F., Pitocchelli J. (1994). Using Stable Isotopes to Determine Seabird Trophic Relationships. J. Anim. Ecol. 63, 786–798. doi: 10.2307/5256
Hoskins A. J., Schumann N., Costa D. P. (2017). Foraging Niche Separation in Sympatric Temperate-Latitude Fur Seal Species. Marine Ecol. Prog. Ser. 566, 229–241. doi: 10.3354/meps12024
Hunter M. D., Price P. W. (1992). Playing Chutes and Ladders: Heterogeneity and the Relative Roles of Bottom-Up and Top-Down Forces in Natural Communities. Ecology 73, 724–732. doi: 10.2307/1940152
Hunt G. L. Jr., Stabeno P., Walters G., Sinclair E., Brodeur R. D., Napp J. M., et al. (2002). Climate Change and Control of the Southeastern Bering Sea Pelagic Ecosystem. Deep Sea Res. Part II: Topical Stud. Oceanography 49, 5821–5853. doi: 10.1016/S0967-0645(02)00321-1
Kämpf J. (2015). Phytoplankton Blooms on the Western Shelf of Tasmania: Evidence of a Highly Productive Ecosystem. Ocean Sci. 11, 1–11. doi: 10.5194/os-11-1-2015
Kirkwood R., Goldsworthy S. (2013). Fur Seals and Sea Lions. (Collingwood Victoria: CSIRO Publishing). doi: 10.1071/9780643109834
Kowalczyk N. D., Chiaradia A., Preston T. J., Reina R. D. (2015). Fine-Scale Dietary Changes Between the Breeding and Non-Breeding Diet of a Resident Seabird. R. Soc. Open Sci. 2, 140–291. doi: 10.1098/rsos.140291
Ling J. (1999). Exploitation of Fur Seals and Sea Lions From Australian, New Zealand and Adjacent Subantarctic Islands During the Eighteenth, Nineteenth and Twentieth Centuries. Aust. Zoologist 31, 323–350. doi: 10.7882/AZ.1999.036
Magera A. M., Mills Flemming J. E., Kaschner K., Christensen L. B., Lotze H. K. (2013). Recovery Trends in Marine Mammal Populations. PloS One 8, e77908. doi: 10.1371/journal.pone.0077908
Marchant S., Higgins P. J. (1990). Handbook of Australian, New Zealand & Antarctic Birds. Vol. 1, Ratites to Ducks, Part B: Australian Pelicans to Ducks (Melbourne: Oxford University Press Australia and New Zealand).
Marshall K. N., Stier A. C., Samhouri J. F., Kelly R. P., Ward E. J. (2016). Conservation Challenges of Predator Recovery. Conserv. Lett. 9, 70–78. doi: 10.1111/conl.12186
Matson P., Hunter M. (1992). Special Feature: Top-Down and Bottom-Up Forces in Population and Community Ecology. Ecology 73, 723–765. doi: 10.2307/1940151
Mccutcheon C., Dann P., Salton M., Renwick L., Hoskins A., Gormley A., et al. (2011). The Foraging Range of Little Penguins (Eudyptula Minor) During Winter. Emu 111, 321–329. doi: 10.1071/MU10078
Middleton J. F., Arthur C., Van Ruth P., Ward T. M., Mcclean J. L., Maltrud M. E., et al. (2007). El Nino Effects and Upwelling Off South Australia. J. Phys. Oceanography 37, 2458–2477. doi: 10.1175/JPO3119.1
Middleton J. F., Bye J. A. (2007). A Review of the Shelf-Slope Circulation Along Australia’s Southern Shelves: Cape Leeuwin to Portland. Prog. Oceanography 75, 1–41. doi: 10.1016/j.pocean.2007.07.001
Miller R. J., Bennett S., Keller A. A., Pease S., Lenihan H. S. (2012). Tio2 Nanoparticles are Phototoxic to Marine Phytoplankton. PloS One 7, e30321. doi: 10.1371/journal.pone.0030321
Morrison K. W., Armstrong D. P., Battley P. F., Jamieson S. E., Thompson D. R. (2016). Predation by New Zealand Sea Lions and Brown Skuas is Causing the Continued Decline of an Eastern Rockhopper Penguin Colony on Campbell Island. Polar Biol. 40, 735–751. doi: 10.1007/s00300-016-1996-9
Norman F., Dann P., Du Guesclin P., Drynan D., Sutherland D. (2017). Recoveries of Little Penguins Eudyptula Minor Flipper-Banded at Colonies in Western Victoria. Corella 41, 20–26.
Norman F., Dann P., Unthank S., Montague T. (2012). Movements of Little Penguins Eudyptula Minor Banded at Rabbit Island and the Seal Island Group, Wilsons Promontory, Victoria. Corella 3, 57–62.
Page B., Mckenzie J., Goldsworthy S. D. (2005). Dietary Resource Partitioning Among Sympatric New Zealand and Australian Fur Seals. Marine Ecol. Prog. Ser. 293, 283–302. doi: 10.3354/meps293283
Paleczny M., Hammill E., Karpouzi V., Pauly D. (2015). Population Trend of the World’s Monitored Seabirds. PloS One 10, 1950–2010. doi: 10.1371/journal.pone.0129342
Pelletier L., Chiaradia A., Kato A., Ropert-Coudert Y. (2014). Fine-Scale Spatial Age Segregation in the Limited Foraging Area of an Inshore Seabird Species, the Little Penguin. Oecologia 176, 399–408. doi: 10.1007/s00442-014-3018-3
Preston T. J., Ropert-Coudert Y., Kato A., Chiaradia A., Kirkwood R., Dann P., et al. (2008). Foraging Behaviour of Little Penguins Eudyptula Minor in an Artificially Modified Environment. Endangered Species Res. 4, 95–103. doi: 10.3354/esr00069
Radhalakshmi R., Sivakumar V., Ali H. (2014). Analysis of Selected Species of Ascidians as Bioindicators of Metals in Marine Ecosystem. Internation J. Curr. Microbiol. Appl. Sci. 3, 755–764.
Reilly P., Cullen J. (1981). The Little Penguin Eudyptula Minor in Victoria, II: Breeding. Emu 81, 1–19. doi: 10.1071/MU9810001
Reilly P., Cullen J. (1982). The Little Penguin Eudyptula Minor in Victoria III. Dispersal of Chicks and Survival After Banding. Emu-Austral Ornithol. 82, 137–142. doi: 10.1071/MU9820137
Rey A. R., Samaniego R. S., Petracci P. F. (2012). New Records of South American Sea Lion Otaria Flavescens Predation on Southern Rockhopper Penguins Eudyptes Chrysocome at Staten Island, Argentina. Polar Biol. 35, 319–322. doi: 10.1007/s00300-011-1072-4
Ridgway K. (1997). Seasonal Cycle of the East Australian Current. J. Geophysical Res. Oceans 102, 921–936. J. S. doi: 10.1029/97JC00227
Roman J., Dunphy-Daly M. M., Johnston D. W., Read A. J. (2015). Lifting Baselines to Address the Consequences of Conservation Success. Trends Ecol. Evol. 30, 299–302. doi: 10.1016/j.tree.2015.04.003
Ross G., Burbidge A., Brothers N., Canty P., Dann P., Fuller P., et al. (1995). “The Status of Australia’s Seabirds,” in The State of the Marine Environment Report for Australia: Technical Annex: 1. Eds. Zann L. P., Kailola P. (Townsville, Qld: Great Barrier Reef Marine Park Authority), 167–182.
Salton M., Carr M., Tarjan L. M., Clarke J., Kirkwood R., Slip D., et al. (2021). Protected Area Use by Two Sympatric Marine Predators Repopulating Their Historical Range. Endangered Species Res. 45, 181–194. doi: 10.3354/esr01129
Sandery P. A., Kämpf J. (2007). Transport Timescales for Identifying Seasonal Variation in Bass Strait, South-Eastern Australia. Estuarine Coastal Shelf Sci. 74, 684–696. doi: 10.1016/j.ecss.2007.05.011
Scheuhammer A. (1987). The Chronic Toxicity of Aluminium, Cadmium, Mercury, and Lead in Birds: A Review. Environ. Pollution 46, 263–295. doi: 10.1016/0269-7491(87)90173-4
Shaughnessy P. D., Goldsworthy S. D., Mackay A. I. (2015). The Long-Nosed Fur Seal (Arctocephalus forsteri) in South Australia in 2013–14: Abundance, Status and Trends. Aust. J. Zool. 63, 101–110. doi: 10.1071/ZO14103
Shi H., Magaye R., Castranova V., Zhao J. (2013). Titanium Dioxide Nanoparticles: A Review of Current Toxicological Data. Particle Fibre Toxicol. 10, 1–33. doi: 10.1186/1743-8977-10-15
Sidhu L. A., Dann P., Chambers L., Catchpole E. A. (2012). Seasonal Ocean Temperature and the Survival of First-Year Little Penguins Eudyptula Minor in South-Eastern Australia. Marine Ecol. Prog. Ser. 454, 263–272. doi: 10.3354/meps09709
Sun T., Wu H., Wang X., Ji C., Shan X., Li F. (2020). Evaluation on the Biomagnification or Biodilution of Trace Metals in Global Marine Food Webs by Meta-Analysis. Environ. Pollution 264, 113856. doi: 10.1016/j.envpol.2019.113856
Vanderklift M. A., Ponsard S. (2003). Sources of Variation in Consumer-Diet δ15n Enrichment: A Meta-Analysis. Oecologia 136, 169–182. doi: 10.1007/s00442-003-1270-z
Visser I. N., Drennan M. P., White R. W., Maclean S. F., Lagerstrom L. C., Francis J. M. (2008). Antarctic Fur Seals (Arctocephalus Gazella) Observed Predating Adélie (Pygoscelis Adeliae) and Chinstrap Penguins (P. Antarctica), Antarctic Peninsula. Aquat. Mammals 34, 193–199. doi: 10.1578/AM.34.2.2008.193
Walsh P. (2018). “The Use of Seabirds as Monitors of Heavy Metals in the Marine Environment,” in Heavy Metals in the Marine Environment, vol. 183-204 . Eds. Furness R. W., Rainbow P. S. (Florida USA: CRC Press). doi: 10.1007/978-3-030-73613-2_1
Weir A., Westerhoff P., Fabricius L., Hristovski K., Von Goetz N. (2012). Titanium Dioxide Nanoparticles in Food and Personal Care Products. Environ. Sci. Technol. 46, 2242–2250. doi: 10.1021/es204168d
Keywords: wildlife conflict management, predator-prey interaction, feathers, stable isotope analysis, trace element analysis, little penguin Eudyptula minor, long-nosed fur seal, Arctocephalus forsteri
Citation: Reinhold S-L, Goldsworthy SD, Arnould JPY, Gillanders BM, Connell SD and McIntosh RR (2022) Tracing Seal Predation Back to the Source Colony of Their Penguin Prey: A Trace Element and Stable Isotope Analysis. Front. Mar. Sci. 9:813106. doi: 10.3389/fmars.2022.813106
Received: 11 November 2021; Accepted: 08 March 2022;
Published: 30 March 2022.
Edited by:
Patrick Jodice, United States Geological Survey (USGS), United StatesReviewed by:
Luis Cardona, University of Barcelona, SpainAndrea Walters, University of Tasmania, Australia
Copyright © 2022 Reinhold, Goldsworthy, Arnould, Gillanders, Connell and McIntosh. This is an open-access article distributed under the terms of the Creative Commons Attribution License (CC BY). The use, distribution or reproduction in other forums is permitted, provided the original author(s) and the copyright owner(s) are credited and that the original publication in this journal is cited, in accordance with accepted academic practice. No use, distribution or reproduction is permitted which does not comply with these terms.
*Correspondence: Sarah-Lena Reinhold, c2FyYWgtbGVuYS5yZWluaG9sZEBhZGVsYWlkZS5lZHUuYXU=