- 1Bigelow Laboratory for Ocean Sciences, East Boothbay, ME, United States
- 2Department of Geosciences, Princeton University, Princeton, NJ, United States
- 3Scripps College, Claremont, CA, United States
- 4School of Marine and Atmospheric Sciences, Stony Brook University, Southampton, NY, United States
- 5Colby College, Waterville, ME, United States
A substantial component of phytoplankton production in the oceans is channeled through protistan grazers but understanding what dictates the magnitude of this process on a regional and temporal basis is limited, in part, by a shortage of experimental options. A novel saturation approach based on the functional response of planktonic grazers to increasing prey abundance was developed using laboratory cultures of the predator-prey combination of Ochromonas danica and Micromonas pusilla and tested in the coastal waters of the Gulf of Maine. In incubation series, 2 μm polystyrene microspheres were used as surrogate prey to generate increasing levels of saturation of predator ingestion rates of natural prey, resulting in increased rates of apparent growth of the picophytoplankton populations. The relationship between level of addition of surrogate prey to apparent growth, consistently provided significant estimates of maximal growth in the absence of grazing and grazing mortality for populations of picoeukaryotes and Synechococcus. Estimates of gross growth and grazing mortality were comparable to results from dilution experiments carried out in the same waters. The saturation approach represents an additional tool to investigate predator-prey interactions in planktonic communities. Further investigations may show that it can be used to quantify group-specific grazing mortality and growth rates beyond coastal waters and in multiple size classes of prey.
Introduction
Herbivory is the major fate of primary production in the oceans and the major herbivores in most pelagic communities are protists (Sherr and Sherr, 2002). These primary consumers have been loosely referred to as the microzooplankton, with the growing realization that many protists are mixotrophic, able to combine phagotrophy with photoautotrophic production (Jones, 1997; Stoecker, 1998; Zubkov and Tarran, 2008). Predation by microzooplankton influences planktonic systems at many levels. This includes structuring the phenotypic and genetic composition of prokaryote and eukaryote communities; comprising a major route of nutrient transfer between functional groups and size classes, including a conduit from primary producers to higher trophic levels outside the microbial loop; and acting as an important nutrient recycling process, increasing the bioavailability of inorganic nutrients and trace metals to bacteria and photoautotrophs (reviewed in Sherr and Sherr, 2002; Worden et al., 2015).
A variety of approaches have been used to quantify rates of grazing by microzooplankton in the natural environment. This includes tracing the ingestion of labeled or surrogate prey by grazers. Fluorescent dyes (Sherr et al., 1987; Rublee and Gallegos, 1989; Putt, 1991) and radioisotopes (Lessard and Swift, 1985; Archer et al., 1996) have been used to label algal or bacterial prey. Surrogate prey used to visually quantify ingestion rates of protists have included fluorescent microspheres, wheat starch particles and bacteria containing green fluorescent protein (Pace and Bailiff, 1987; Kivi and Setälä, 1995; Fu et al., 2003). These approaches provide useful information on particle ingestion rates by specific components of pelagic microbial communities but tend to be labor intensive, and are complicated by protists able to select between particle types, issues with preservation of fully representative components of the community and extrapolation of single cell-specific rates to a community level process (Pace and Bailiff, 1987; Putt, 1991).
By far the most commonly applied method has been the dilution approach of Landry and Hassett (1982). The near ubiquitous use of the dilution approach has provided the opportunity to compile and compare measurements conducted in multiple studies covering many regions (Sherr and Sherr, 2002; Calbet and Landry, 2004; Schmoker et al., 2013; Chen et al., 2014). Schmoker et al. (2013) compiled 1,525 data points from dilution experiments and grouped them into oceanic provinces to examine regional variability in microzooplankton grazing mortality and phytoplankton growth. High variability in grazing impact on the phytoplankton community was found between regions, with median values ranging between 0.07 d–1 in southern polar regions and 0.49 d–1 in the sub-tropical Atlantic; this is equivalent to the consumption of 53 and 70% of primary production, respectively. Despite these attempts to synthesize the vast number of measurements that have been conducted, the environmental factors underlying regional differences in microzooplankton grazing pressure are not readily apparent. This may reflect differences in how effectively the dilution approach performs in varied environments and how non-linear relationships between the level of dilution and apparent growth rates of phytoplankton are interpreted in the experiments (reviewed by Dolan and McKeon, 2005).
In situ monitoring of picoplankton populations, possible using shipboard continuous or autonomous submersible flow cytometers, provides data from which model-derived estimates of gross growth rates and mortality can be obtained without the need for experimental incubations or the manipulation of cell abundance (Sosik et al., 2003; Ribalet et al., 2015; Fowler et al., 2020). Picophytoplankton populations, which include cyanobacteria such as Synechococcus and Prochlorococcus and small eukaryotes including prymnesiophytes, prasinophytes pelagophytes and chrysophytes, make important contributions to the ecological characteristics and biochemical flux in both near-shore and oceanic waters (Jardillier et al., 2010; Fowler et al., 2020). Distinctly higher gross growth rates of picophytoplankton have been derived from the in situ data, compared to those obtained from dilution experiments (Fowler et al., 2020), emphasizing the need to better understand their growth and fate, and the appeal of additional approaches to quantify these rates. Here we introduce an experimental approach that may avoid some of the complexities of the dilution approach, while providing additional insights into predator-prey interactions among these smaller size classes in natural waters.
A fundamental feature of the behavior of planktonic grazers is a rapid increase in ingestion as prey abundance is increased, followed by saturation of uptake above some threshold value (Frost, 1972; Fenchel, 1980). Here we present an approach that exploits this functional response to determine estimates of the rate of grazing mortality imposed by microzooplankton on their prey. The fundamentals of the approach are illustrated using a single predator—prey combination in laboratory culture. This is followed by presentation of a series of experimental tests carried out in natural waters, including a comparison with the dilution approach. This series of experiments is used to illustrate that a relatively straightforward experimental design provides an additional experimental tool to estimate rates of growth and mortality due to microzooplankton grazing of specific components of natural phytoplankton communities.
Materials and Methods
Theoretical Basis of the Approach
For microzooplankton, rates of prey ingestion are influenced by the time involved in both prey capture and prey digestion. The former includes encounter rates, prey handling and prey avoidance and/or rejection, the latter depends on how quickly prey can be processed through the digestive system before the predator becomes satiated (Flynn and Mitra, 2016). Addition of surrogate prey may affect both aspects and, even if selected against by the predator, the additional handling time involved will reduce the ingestion rate of natural prey and hence their rates of grazing mortality.
Demonstrations of the functional response of protozoan and metazoan zooplankton involve measurement of ingestion rates of grazers at increasing concentrations of a single prey type, including in some cases, artificial prey such as latex beads (Fenchel, 1980; Jonsson, 1986; Verity, 1991). In a procedure that mimics determination of the functional response, the proposed saturation approach involves adding increasing abundances of surrogate prey to incubations of natural communities and measuring the observed growth response of the natural prey of interest. At increasing levels of surrogate prey addition, as grazers become “saturated” by handling and ingesting surrogate prey, the apparent growth rate (μapp) of the natural prey increases and approaches a maximum value that represents their specific growth rate in the absence of grazing (μmax). The difference between μapp with no addition of surrogate prey, effectively the net growth rate (μnet), and μmax, provides an estimate of the level of mortality due to grazing (m)
with the assumption that the specific growth rate of the prey is not affected by the addition of surrogate prey.
Analagous to plant growth in response to increasing amounts of a limiting nutrient, the increasing growth rate of prey in response to increasing surrogate prey was represented by an asymptotic regression model, of similar form to the Mitscherlich response model (Mitscherlich, 1928). The model was used to derive values of μnet, μmax and m from the relationship between μapp and level of addition of surrogate prey,
where C is the surrogate prey concentration and a is proportional to the relative rate of increase in μapp with increasing C. Note, umax is referred to as “gross growth rate” but does not account for other causes of mortality, such as viral infection. Model fitting was carried out using the non-linear least squares regression function (nls) in R (R Core Team, 2021).
Tests Using Single Prey and Predator Combinations
Proof-of-concept tests were performed using laboratory cultures of a single isolate representative of the picophytoplankton and a typical protistan predator. Specifically, the experiments used the mixotrophic chrysophyte Ochromonas danica (CCMP 1391), ∼ 6–9 μm in diameter, as a predator, and the generally abundant picoeukaryote Micromonas pusilla (CCMP 485), ∼ 1–2 μm in diameter, as prey. The cultures were maintained in sterile L1 media in 50 ml borosilicate glass test tubes in a 21°C incubator with a 14 h light/10 h dark cycle and light levels of ∼ 90 μE m–2 s–1. Prior to the start of the experiments, O. danica was transitioned from K media and rice to only sterile L1 media and then fed every other day on M. pusilla. The prey, M. pusilla was maintained in semi-continuous growth through regular transfers (2–3 days). In 3 experiments, similar ratios of M. pusilla (target 3 × 105 ml–1) and O. danica (target 2,000 ml–1) were generated in tubes containing a total volume of 40 ml. Fluorescent polystyrene microspheres (beads) of 2 μm in diameter (Fluoresbrite Plain YG microspheres, Polysciences, Inc., Warrington, PA), were used as surrogate prey.
To minimize clumping, the beads were blocked in a solution of 1% bovine serum albumin overnight then centrifuged for 5 min at 2,000 rpm, after which the pellet was resuspended in 0.2 μm filtered seawater. A new solution of beads was prepared at the start of each experiment. Duplicate tubes were prepared for 6 different surrogate prey concentrations ranging from 0 to 7.5 × 106 ml–1. During the incubations, the experimental tubes were rotated at 1.2 rpm on a plankton wheel to keep particles in suspension. Two subsamples of 1 ml were removed from each tube at the start (T0) and the final time point (TF) after ∼24 h, for flow cytometric analysis.
Picophytoplankton Rates in Natural Waters
The experimental format established in the culture experiments was then adapted for use in natural waters. From 16th July to 15th August, 2019 (days of the year 197–227) and 8th June to 26th July 2021 (days 165–207), a series of saturation experiments were performed that focused on determining the growth rates and grazing mortality of picoeukaryote and Syncechococcus sp. populations. Gulf of Maine coastal seawater was collected from the Damariscotta River Estuary at Bigelow Laboratory’s dock off East Boothbay, Maine, United States (43.8604° N, 69.5781° W). Water for all experiments was collected within 1 h of high tide at 1 m depth using a 5 l Niskin bottle and was gravity filtered through 200 μm mesh to remove zooplankton. Several Niskin casts of seawater were combined in an acid washed carboy before being siphoned into 600 ml acid washed polycarbonate bottles. For each saturation experiment, 12–14 bottles were used to generate a range of levels of surrogate prey addition. Fluorescent polystyrene microspheres of 2 μm in diameter, treated as for the laboratory experiments, were added to the bottles either as duplicate treatments or in a continuous series of abundance. Maximum surrogate prey abundance ranged from 1.7 × 106 beads ml−1 on day 197 to 3.8 × 106 beads ml−1 on day 226, in 2019.
Bottles were incubated for 24 h in a flow-through incubator on the dock under a nylon mesh that removed ∼40% of the surface PAR. In an initial test of the flow-through incubator, five 600 ml bottles were filled with seawater from the same carboy and with no further treatment, were incubated for 24 h. The counts of the T0 abundance of picoeukaryotes from the five bottles showed a coefficient of variation of 1.3%, that increased in the T24 counts to only 4.1%, indicating consistent growth among the 5 replicate bottles. Following all experiments, the seawater to which beads had been added was filtered through a 0.45 μm capsule filter to recover the beads before discarding the water.
For comparison, a series of dilution experiments was carried out in parallel to the saturation experiments, using a modification of the approach of Landry and Hassett (1982). The same water used for the saturation experiments was used to prepare triplicate bottles of the whole water (100%) and duplicate bottles at four different levels of dilution (10, 25, 50, and 75%) in 600 ml polycarbonate bottles. Diluent was prepared from the 200 μm pre-screened seawater by gentle filtration through a 0.45 μm mini-capsule filter (Pall Life Sciences). The dilution experiments were set up following the saturation incubations to allow time for flow cytometric analysis of each set of subsamples and so, T0 and T24 were delayed by ∼ 2 h relative to those of the saturation experiments.
Flow Cytometric Measurements
A ZE5 Cell Analyzer (Bio-Rad, Hercules, CA, United States) was used to measure optical properties of single cells from each sample. Particles were excited with a 488 nm blue excitation laser (100 mW). Data acquisition was triggered on forward scatter (FSC). Signals were recorded from detectors with bandpass filters for right angle light scatter and fluorescence emission in red (692 nm / 80 nm band pass) indicative of chlorophyll a, orange for phycoerythrin (593/52 nm), and green (525/35 nm). To ensure accurate calibration of the flow cytometer, ZE5 QC beads (Bio-Rad, Hercules, CA, United States) were run daily. Data files were analyzed from logarithmic dot plots based on fluorescence and characteristic light scattering properties (Durand and Olson, 1996) using FlowJo 10.6 Software (Becton Dickinson & Company, San Jose, CA, United States) (Supplementary Figure 1).
For culture and natural community experiments, surrogate prey were gated using FSC and green fluorescence. Despite the blocking procedure, some clumping of beads did occur but, in this application, doublet and triplet clumps were treated as single surrogate prey particles. For laboratory experiments, O. danica was gated on side scatter and red fluorescence and the M. pusilla populations were determined using forward scatter and red fluorescence. For field experiments, picophytoplankton were identified based upon cell size, using forward scatter as a proxy, and red fluorescence. Picophytoplankton were classified, as either Synechococcus or picoeukaryotes based on the presence or absence of the accessory pigment phycoerythrin, respectively. Using these gating criteria, the number of cells in each identified population was enumerated and converted to cell abundances based on the processed sample volume.
Results
Single Prey and Predator Interactions
The single prey and predator laboratory culture experiments confirm the theoretical prediction. Apparent growth rate (μapp) of M. pusilla increased with the addition of beads and tended towards a horizontal asymptote at increasing levels of surrogate prey addition, indicating saturation of ingestion in O. danica (Figure 1). Fits of Equation 2 to the relationship between μapp and the surrogate prey abundance, provided highly significant estimates of μmax (p-value < 0.001), μnet (p < 0.05) and a (p < 0.05) in each of the experiments (Table 1). Derived values of m decreased from 0.60 to 0.33 d–1 over the three experiments, despite the reduced prey to predator ratios. Although a distinct population of O. danica could be counted initially, as they grazed on M. pusilla and ingested beads, the population became more difficult to define in the flow cytometric signal and it was not possible to obtain final abundances and reliable growth rates for the predator.
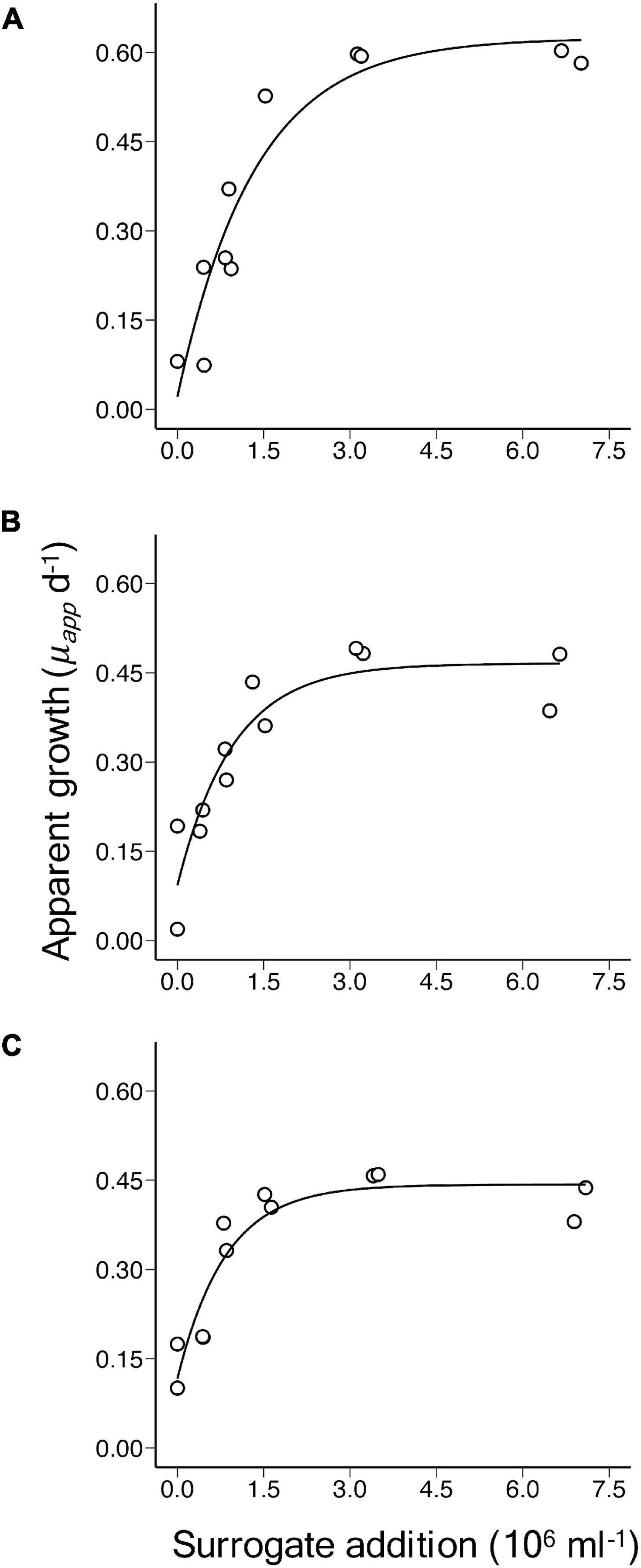
Figure 1. Laboratory culture experiments of Ochromonas danica grazing on Micromonas pusilla, showing the relationship between apparent growth rate (μapp) of M. pusilla over 24 h vs. the initial level of addition of 2 μm microspheres. The curves are significant fits of an asymptotic regression model and the corresponding details are shown in Table 1 for experiments (A–C).
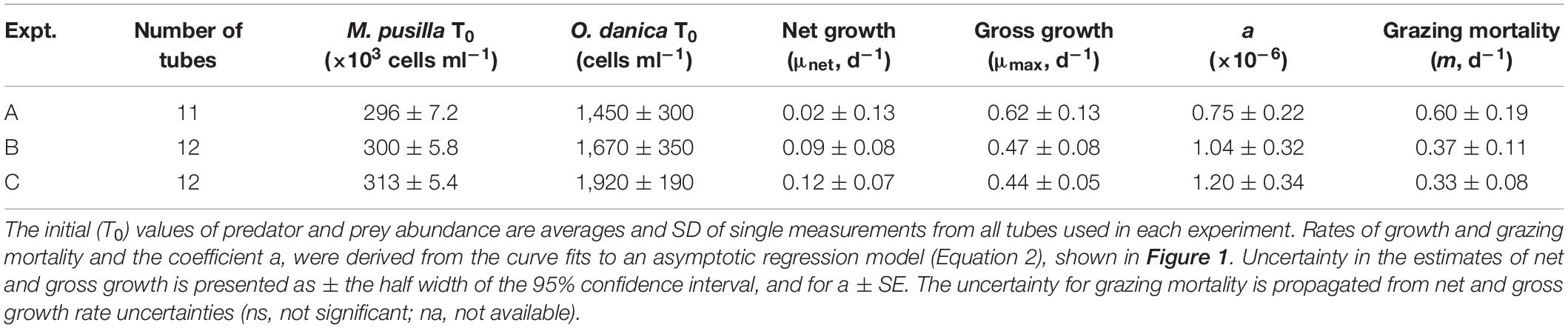
Table 1. Saturation experiments using cultures of Ochromonas danica grazing on Micromonas pusilla over 24 h.
Initial prey to predator ratios were approximately 200, 180, and 160 in experiments A, B, and C, respectively (Table 1). At the levels of abundance used, growth and grazing rates were almost balanced in the cultures with no bead addition, resulting in similar, relatively low rates of μnet for M. pusilla in the three experiments (Table 1).
In general, the abundances of freely suspended beads decreased during the incubations by an average of 25, 3, and 25% in A, B, and C, respectively. The decrease possibly results from a combination of ingestion by predators, sinking out of suspension despite the mixing during incubations and sticking to other particles. Proportionally higher losses tended to occur at higher levels of bead addition but this was not always the case.
Saturation Experiments Using Natural Waters
The dynamics of the two picophytoplankton populations showed variable patterns indicative of differences in their rates of growth and the grazing pressure they experienced. During the 2019 summer period, the abundance of the picoeukaryote population increased by approximately 50% from 43,100 cells ml–1 on day 197 to 60,600 cells ml–1 on day 211 and then gradually declined over the following 2 weeks (Figure 2A). In contrast, the abundance of Synechococcus increased more than 10-fold from 2,860 on day 197 to 30,100 on day 211 and remained at a relatively high level to day 226 (Figure 2A). Similar abundances of each group were observed in the summer of 2021 (Supplementary Tables 1, 2). Note, the mouth of the Damariscotta River Estuary experiences high levels of tidally driven water exchange and this may also have influenced population dynamics.
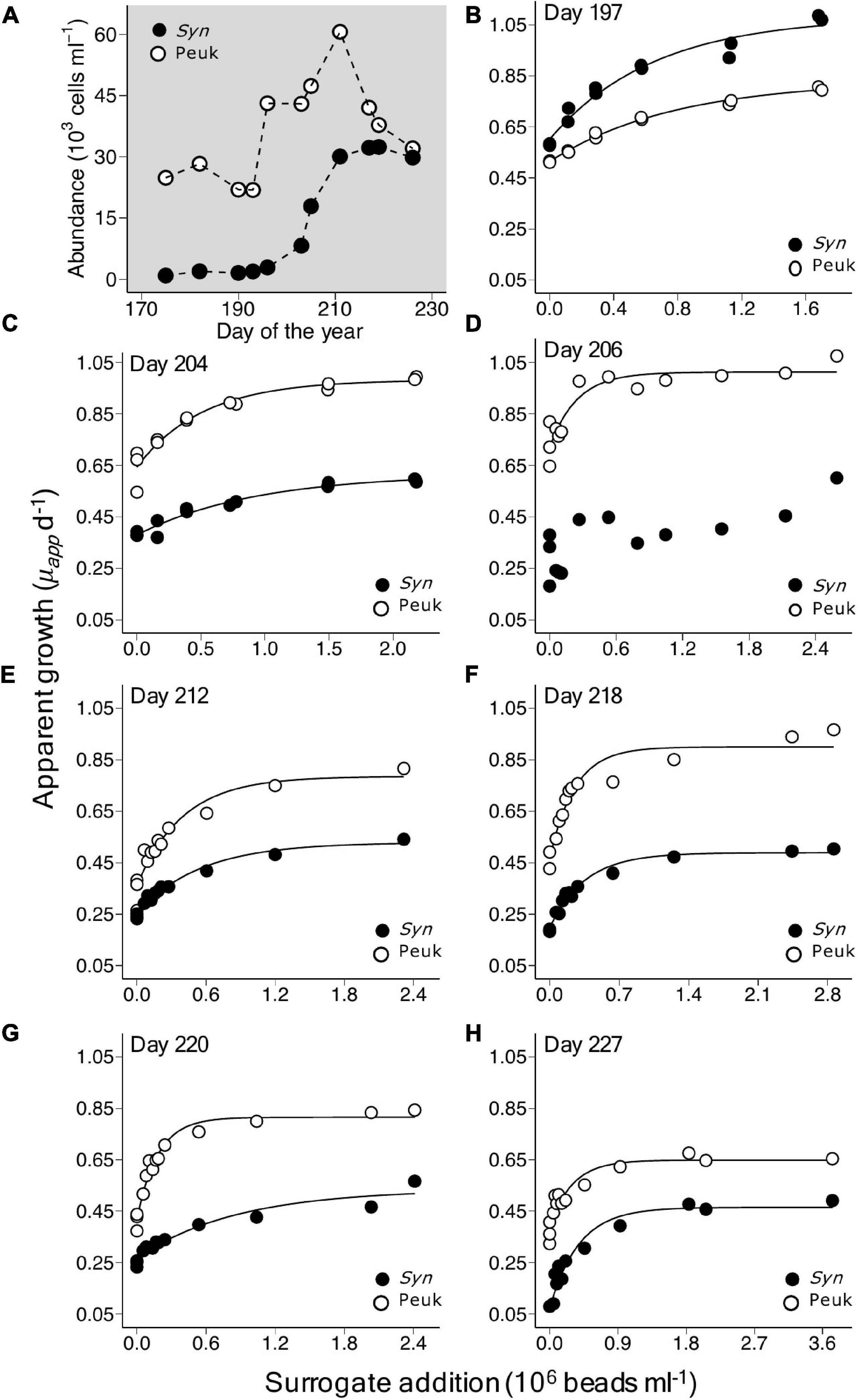
Figure 2. Growth response in populations of picoeukaryotes and Synechococcus in a series of saturation experiments using coastal waters of the Gulf of Maine during July and August, 2019. (A) Shows changes in abundance of the two taxonomic groups during this period. Plots (B–H) show the apparent growth rate (μapp) vs. surrogate prey addition, with corresponding asymptotic regression fits, in experiments conducted between days of year 197 and 227, details of which are shown in Table 2.
As illustrated for 2019, increased bead addition resulted in increased μapp, tending toward a horizontal asymptote at bead abundances that exceeded ∼2.0 × 106 ml–1 (Figures 2B–H). The growth response to bead addition indicates increasing saturation of the existing grazer population and reduction of grazing mortality in the picophytoplankton populations. Fits of Equation 2 to the relationship between μapp and the surrogate prey abundance, provided highly significant estimates of μmax (p < 0.001), μnet (p < 0.01) and a (p < 0.05), for each of the taxonomic groups in all seven experiments, except for Synechococcus on day 206 (Table 2). Values of m ranged from 0.26 to 0.43 d–1 for picoeukaryotes and 0.23 to 0.42 d–1 for Synechococcus. Estimates of gross growth in the absence of grazing (μmax) indicate a highly productive population of picoeukaryotes that grew at rates of over 1 d–1. These rates of μmax gradually declined over the period to close to 1 doubling d–1, by day 227 (Table 2). Synechococcus also showed high rates of μmax initially, that stabilized to between 0.47 and 0.53 d–1 from days 212 to 227. Similar rates of growth and mortality were measured in the four experiments successfully conducted at the same location during the summer of 2021 (Supplementary Table 2).
Paired t-tests showed significant differences in μnet (p = 3.5 × 10–5), m (p = 1.3 × 10–2) and μmax (p = 1.9 × 10–4) occurred between Synechococcus and picoeukaryotes in the combined dataset of saturation experiments. Rates of grazing mortality were the most comparable of the coefficients between the two taxonomic groups, while higher rates of gross growth among picoeukaryotes were largely responsible for the significant differences in net growth rates (Supplementary Figure 2).
Comparison to Dilution Experiments
In general, the rates from each approach show a similar range of values for μmax, μnet and m (Figure 3 and Supplementary Table 1). Successful, parallel experiments allow direct comparison on days 197 and 204 in 2019 and on days 174, 182, 196, and 207 in 2021. It should be noted that the dilution incubations were started approximately 2 h after the saturation incubations, which may explain the slight differences in μnet between experiments on the same days (Table 2 and Supplementary Tables 1, 2). In the combined dataset of comparable experiments, there was no statistical evidence that the mean difference between experimental approaches was significantly different from zero (paired t-tests, p-value < 0.05). This is apparent in the lack of clear trends between approaches when estimates of μmax and m are compared for picoeukaryotes or Synechococcus, although large variations were apparent on certain days, particularly days 197 and 182 (Figure 4).
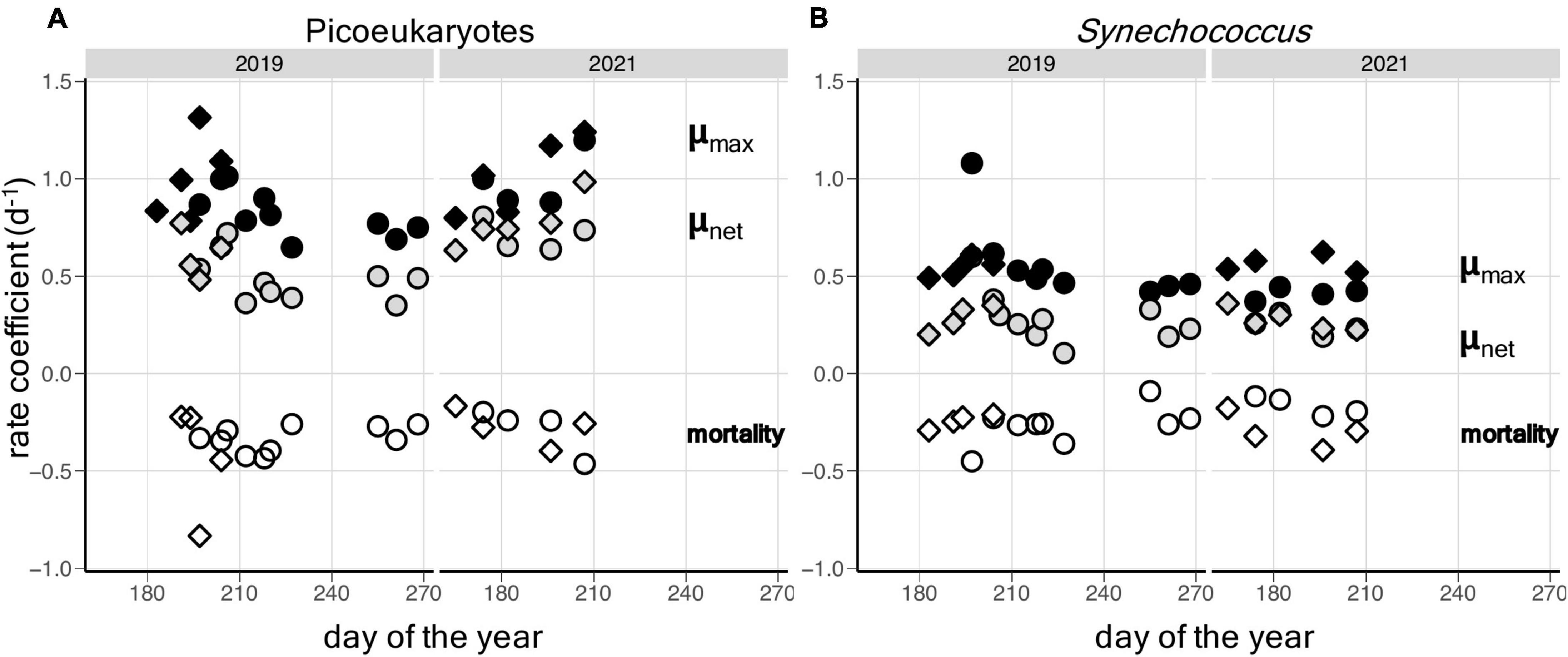
Figure 3. Temporal patterns of net (μnet) and gross growth (μmax) rates and mortality due to grazing (m) in: (A) picoeukaryote; and (B) Synechococcus populations in Gulf of Maine coastal waters during the summers of 2019 and 2021. Values derived from saturation experiments are shown as circles and values from dilution experiments are shown as diamonds.
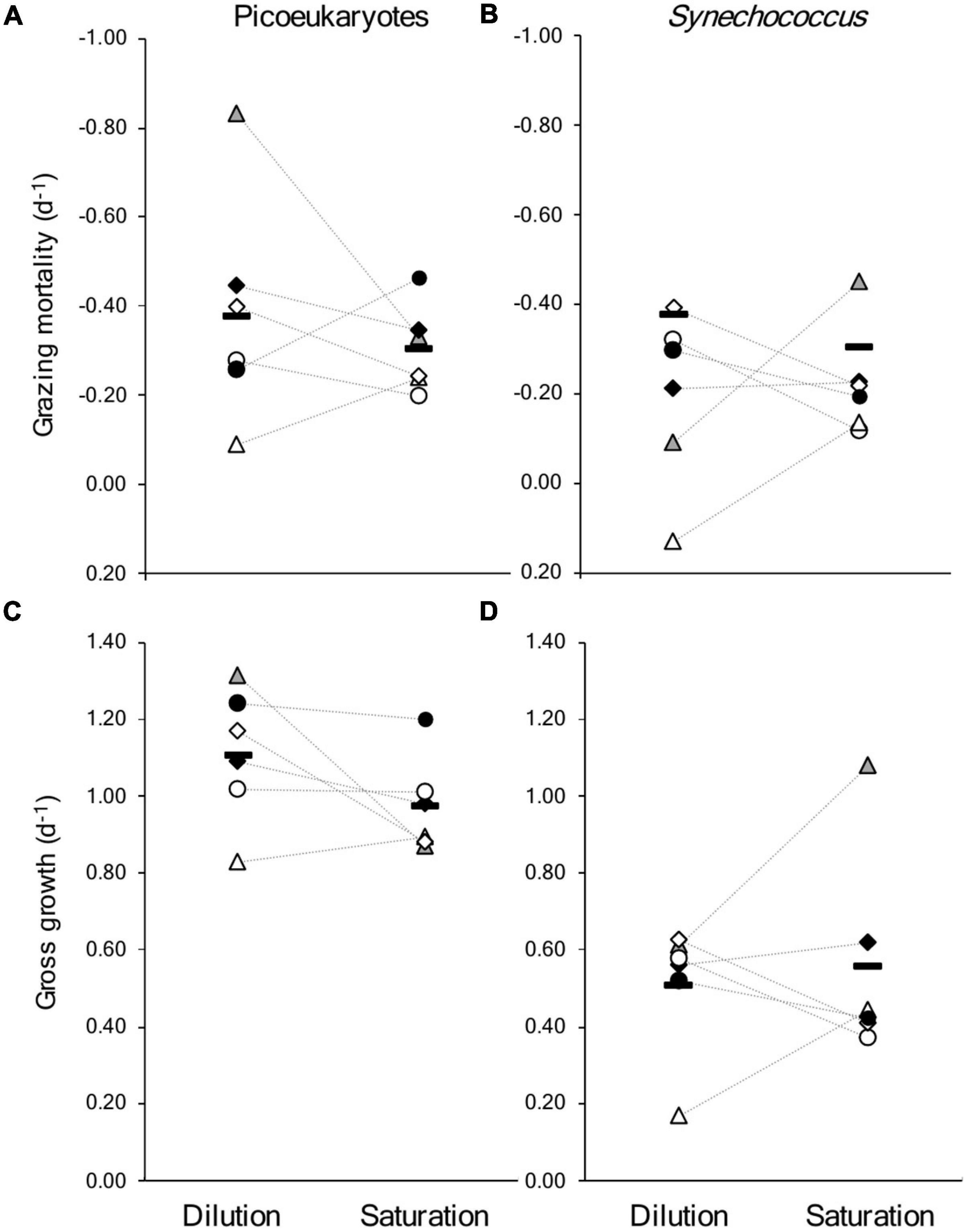
Figure 4. Comparison of results from the six saturation and dilution experiments run in parallel in coastal waters of the Gulf of Maine in the summers of 2019 and 2021. Showing rates of mortality due to grazing for (A) picoeukaryotes, and (B) Synechococcus; and gross growth rates for (C) picoeukaryote, and (D) Synechococcus. Paired symbols represent parallel experiments; gray triangle: day 197 (2019), black diamond: 204 (2019), open circle: 174 (2021), open triangle: 182 (2021), open diamond: 196 (2022), filled circle: 207 (2022); the horizontal bars are the mean of the six experiments, the dotted lines connect experiments run on the same day. The values and associated levels of uncertainty are included in Table 2 and Supplementary Tables 1, 2. The dilution approach generated a positive slope for the relationship between apparent growth and level of dilution for Synechococcus on day 182 (Supplementary Table 1).
Note, the raw data from both the saturation and dilution experiments is included in the Supplementary Material.
Discussion
The combination of laboratory culture experiments and tests in coastal waters illustrate the potential of the saturation approach to generate estimates of the mortality due to microzooplankton grazing (m) and gross growth rates of picophytoplankton in the absence of grazing (umax). The functional response theory (Holling, 1965) that underpins the saturation approach, appears to hold true in natural communities where diverse phagotrophs, with differing prey preferences and nutritional strategies, are likely to occur.
Picophytoplankton Population Dynamics
The saturation approach confirmed intriguing differences in the dynamics of co-occurring picoeukaryote and Synechococcus populations. The most notable difference being the considerably higher rates of umax in the picoeukaryote population, equivalent to between ∼1 and 2 doublings d–1 compared to generally < 1 doubling d–1 for Synechocccus (Table 2, Figures 3, 4, and Supplementary Figure 2). Similar, higher rates of gross growth and grazing mortality of picoeukaryotes compared to Synechococcus, have been observed in other temperate and sub-tropical coastal waters (Worden et al., 2004; Kimmance et al., 2007; Fowler et al., 2020).
At the light levels used for the incubations, approximately 60% surface PAR, μmax of both populations exceeded m, resulting in positive net growth rates (Figure 3). If it is assumed that the same coastal waters were sampled on each date, then the picoeukaryote population showed an increase in abundance between days ∼190 and 211 of 2019, equivalent to a net rate of growth of 0.05 d–1 (Figure 3A). The similar pattern in Synechococcus abundance lagged the picoeukaryote population by approximately 5 d but increased at a faster net rate between days 193 and 211 of 0.15 d–1 (Figure 3A). Even during these periods of maximal in situ net growth, the μnet values derived from the incubations were considerably higher for both populations (Figure 3 and Table 2). The closer balance between gross growth and grazing mortality in the water column as a whole, may be a consequence of reduced overall light levels on gross growth rates caused by mixing of photoautotrophic cells through the water column, while grazing mortality rates may be more constant with depth.
Limitations and Advantages of the Saturation Approach
The approach as presented here, was focused on specific groups of picophytoplankton whose abundance could be precisely quantified by flow cytometry. In this respect, the method may not be a suitable means of obtaining total phytoplankton grazing mortality in the way that the dilution approach has been applied. How chlorophyll concentrations changed in response to increasing bead addition was not measured but may provide estimates of grazing and growth rates when a single size spectrum dominates the phytoplankton. As illustrated previously and here, the dilution approach can also be used to generate phytoplankton group-specific information that can be directly compared to results from the saturation approach (Figures 3, 4; Landry et al., 1995; Worden and Binder, 2003).
The saturation approach relies on several assumptions that need to be considered and more thoroughly tested for applications in specific environments. The primary assumption is that the addition of surrogate prey does not influence μmax of the natural prey. In preliminary laboratory experiments, 2 μm beads were added to six tubes containing only M. pusilla over a range of 0–6 × 106 beads ml–1, to test for an effect of the microspheres on growth. Under the illumination and mixing regime generally used for the culture experiments, growth rates of M. pusilla averaged 0.48 ± 0.03 d–1 across the six tubes, with no apparent trend, indicating minimal influence of the surrogate prey on growth (not shown). Similar tests were not carried out for natural waters, where the separation of prey and predators is more difficult to accomplish.
There is the possibility that in nutrient limited waters, the reduced grazing caused by surrogate prey additions may decrease nutrient recycling rates and as a consequence, potentially reduce estimates of m and μmax. A possible advantage of the saturation approach is that the biomass of the natural population is not altered across the incubation series as part of the initial experimental set-up. Population size does vary, nonetheless, between bottles in the series over the course of the incubations. In the natural water experiments, the maximum variations in population size between incubations exhibiting net growth (zero bead addition) and gross growth (μmax) within an experiment, were 1.7-fold for picoeukaryotes on day 204 and 1.9-fold for Synechococcus on day 197 (Table 2). Thus, variation in nutrient availability between treatments of the saturation approach is likely to be lower than across the 10–5-fold difference in initial biomass that is required in dilution experiments.
A key aspect of the experimental design is judgment of the quantity of surrogate prey required to illicit a saturation response, while minimizing potential artifacts from too many additional particles. In the coastal waters of the Gulf of Maine, 2 μm bead additions of > 2 × 106 ml–1 saturated the grazers of picoeukaryote and Synechococcus populations, sufficiently to generate significant values of m and μmax (Figure 2). It seems reasonable to expect the behavioral response of phagotrophic protists to be consistent in similar water types but this remains to be established. Preliminary studies in oceanic waters suggest grazers of Prochlorococcus and Synechococcus populations may tend toward saturation at bead additions of < 1 × 106 ml–1. In theory, coefficient a of Equation 2 varies in proportion to the rate of increase in μapp relative to C, the surrogate prey concentration. It may be possible to use values of a, or an analogous coefficient, to judge how effectively different surrogate prey types or sizes lead to saturation of predators. As more experiments are carried out, it should be possible to standardize the surrogate prey type and levels of addition for different water types.
The asymptotic regression model interpretation of the response of μapp to increasing surrogate prey, provided highly significant values of μmax (p < 0.001) and μnet (p < 0.01) and hence, estimates of m (Table 2 and Supplementary Table 2). However, multiple predator types may consume picophytoplankton populations, each having a different “functional response” to increased surrogate prey abundance and the interpretation of the observed experimental results may be considered over-simplistic. Future studies could usefully examine more complex model interpretations of the saturation approach that consider, for example, predator and prey motility and encounter rates, the process and duration of prey capture and selectivity by the predator (Flynn and Mitra, 2016).
The governing role that dissolved compounds play in microbial interactions is becoming ever more apparent (Strom et al., 2007; Stocker et al., 2008). Indeed, a contributing factor to inconsistent results generated by dilution approach is the alteration of seawater chemistry through the filtration step to create the diluent required in the experimental set-up. One specific example, is the release of polyunsaturated aldehydes to the dissolved phase and the resulting increased inhibition of phytoplankton apparent growth with increased levels of dilution (Stoecker et al., 2015). An advantage of the saturation approach is that modification of seawater chemistry may be kept to a minimum. Although the bovine serum albumin treatment of beads and subsequent resuspension in filtered seawater appeared to be sufficient for these experiments, more thorough cleaning protocols for artificial surrogate prey are likely to be required for low nutrient and oceanic waters. Nonetheless, it may be possible to investigate grazer-mediated production of dissolved organic compounds, inorganic nutrients and possibly, trace elements by using the approach to generate a gradient of grazing pressure.
Conclusion
The saturation approach provides another experimental option to obtain information on the mortality due to grazing and gross growth rates of specific groups of phytoplankton. It may be particularly suited to investigations into the growth and mortality of picophytoplankton, as demonstrated in the present study. Further investigations are needed into whether the approach is equally effective in determining these rates for larger organisms but it seems reasonable to assume that by increasing the surrogate particle size, larger microzooplankton and zooplankton will also be susceptible to saturation of ingestion rates. It may even be possible to target multiple size classes of prey and predator interactions within a single set of incubations by using a size spectrum of surrogate prey. As seawater chemistry is not altered, the saturation approach may also be an appropriate way to quantify grazing mortality and growth rates of heterotrophic prokaryotes. Although the approach relies on incubations that are long enough to accurately determine changes in prey abundance and therefore growth rates, the experimental set up is less laborious than the dilution approach. In this respect, it may be possible to carry out more experiments in parallel using the saturation approach and thereby obtain greater spatial resolution or treatment-specific information. Simplifying the approach by reducing the number of levels of saturation may also allow more measurements. Several further aspects of the presented approach could be usefully improved including identifying more cost-effective and biodegradable surrogate particles, this would reduce the need to recover the particles post-incubation. It will be particularly useful to establish the numbers of surrogate prey required to generate statistically robust estimates of the rate coefficients in differing pelagic ecosystems, making routine application of the approach more reliable.
Data Availability Statement
The datasets presented in this study can be found in online repositories. The names of the repository/repositories and accession number(s) can be found below: https://www.bco-dmo.org/project/735732. The basic data for saturation and dilution experiments are also included in the Supplementary Material.
Author Contributions
The approach was conceived by SA with additional input from NP and LL. NP, LL, and KP conducted the culture experiments. LL, MK, KM, WD, and MS conducted the natural water experiments. Manuscript writing was carried out by SA with input from all other authors. All authors contributed to the article and approved the submitted version.
Funding
We gratefully acknowledge financial support from the National Science Foundation, United States (NSF project No. OCB 1738061 to SA and NP). WD was supported by the Bigelow Laboratory for Ocean Sciences – Undergraduate Research Experience in the Gulf of Maine and the World Ocean Program (NSF REU 1460861). Matthew Wade was supported by the Linde Packman Lab for Biosciences Innovation and the Champlin Science Scholars Fund at Colby College. Instrumentation was supported by NSF FSML project 1821065.
Conflict of Interest
The authors declare that the research was conducted in the absence of any commercial or financial relationships that could be construed as a potential conflict of interest.
Publisher’s Note
All claims expressed in this article are solely those of the authors and do not necessarily represent those of their affiliated organizations, or those of the publisher, the editors and the reviewers. Any product that may be evaluated in this article, or claim that may be made by its manufacturer, is not guaranteed or endorsed by the publisher.
Acknowledgments
We thank Diane Stoecker, Glen Tarran, and Susan Kimmance for advice on the approach and experimental design. The help of Matthew Wade is acknowledged for installation of the incubation facility on Bigelow Laboratory’s dock. We gratefully acknowledge the advice of the reviewers.
Supplementary Material
The Supplementary Material for this article can be found online at: https://www.frontiersin.org/articles/10.3389/fmars.2022.844620/full#supplementary-material
References
Archer, S. D., Leakey, R. J. G., Burkill, P. H., and Sleigh, M. A. (1996). Microbial dynamics in coastal waters of East Antarctica: herbivory by heterotrophic dinoflagellates. Mar. Ecol. Prog. Ser. 139, 239–255. doi: 10.3354/meps139239
Calbet, A., and Landry, M. R. (2004). Phytoplankton growth, microzooplankton grazing, and carbon cycling in marine systems. Limnol. Oceanogr. 49, 51–57.
Chen, B., Laws, E. A., Liu, H., and Huang, B. (2014). Estimating microzooplankton grazing half-saturation constants from dilution experiments with nonlinear feeding kinetics. Limnol. Oceanogr. 59, 639–644. doi: 10.4319/lo.2014.59.3.0639
Dolan, J., and McKeon, K. (2005). The reliability of grazing rate estimates from dilution experiments: have we over-estimated rates of organic carbon consumption by microzooplankton? Ocean Sci. 1, 1–7.
Durand, M. D., and Olson, R. J. (1996). Contributions of phytoplankton light scattering and cell concentration changes to diel variations in beam attenuation in the equatorial Pacific from flow cytometric measurements of pico-, ultra-and nanoplankton. Deep Sea. Res. II. 43, 891–906. doi: 10.1016/0967-0645(96)00020-3
Fenchel, T. (1980). Suspension feeding in ciliated protozoa: functional response and particle size selection. Microb. Ecol. 6, 1–11. doi: 10.1007/BF02020370
Flynn, K. J., and Mitra, A. (2016). Why plankton modelers should reconsider using rectangular hyperbolic (Michaelis-Menten, Monod) descriptions of predator-prey interactions. Front. Mar. Sci. 3:165. doi: 10.3389/fmars.2016.00165
Fowler, B. L., Neubert, M. G., Hunter-Cevera, K. R., Olson, R. J., Shalapyonok, A., Solow, A. R., et al. (2020). Dynamics and functional diversity of the smallest phytoplankton on the Northeast US Shelf. Proc. Nat. Acad. Sci. U.S.A. 117, 12215–12221. doi: 10.1073/pnas.1918439117
Frost, B. W. (1972). Effects of size and concentration of food particles on the feeding behavior of the marine planktonic copepod Calanus pacificus. Limnol. Oceanogr. 17, 805–815.
Fu, Y., O’Kelly, C., Sieracki, M., and Distel, D. L. (2003). Protistan grazing analysis by flow cytometry using prey labeled by in vivo expression of fluorescent proteins. Appl. Environ. Microbiol. 69, 6848–6855. doi: 10.1128/AEM.69.11.6848-6855.2003
Holling, C. S. (1965). The functional response of predators to prey density and its role in mimicry and population regulation. Mem. Entomol. Soc. Can. 97, 5–60.
Jardillier, L., Zubkov, M. V., Pearman, J., and Scanlan, D. J. (2010). Significant CO2 fixation by small prymnesiophytes in the subtropical and tropical Northeast Atlantic Ocean. ISME J. 4, 1180–1192. doi: 10.1038/ismej.2010.36
Jones, H. (1997). A classification of mixotrophic protists based on their behaviour. Freshw. Biol. 37, 35–43. doi: 10.1016/j.protis.2014.01.002
Jonsson, P. R. (1986). Particle size selection, feeding rates and growth dynamics of marine planktonic oligotrichous ciliates (Ciliophora: Oligotrichina). Mar. Ecol. Prog. Ser. 33, 265–277.
Kimmance, S. A., Wilson, W. H., and Archer, S. D. (2007). Modified dilution technique to estimate viral versus grazing mortality of phytoplankton: limitations associated with method sensitivity in natural waters. Aquat. Microb. Ecol. 49, 207–222. doi: 10.3354/ame01136
Kivi, K., and Setälä, O. (1995). Simultaneous measurement of food particle selection and clearance rates of planktonic oligotrich ciliates (Ciliophora: Oligotrichina). Mar. Ecol. Prog. Ser. 119, 125–137. doi: 10.3354/meps119125
Landry, M. R., and Hassett, R. (1982). Estimating the grazing impact of marine micro-zooplankton. Mar. Biol. 67, 283–288. doi: 10.1007/BF00397668
Landry, M. R., Kirshtein, J., and Constantinou, J. (1995). A refined dilution technique for measuring the community grazing impact of microzooplankton, with experimental tests in the central equatorial Pacific. Mar. Ecol. Prog. Ser. 120, 53–63. doi: 10.3354/meps120053
Lessard, E. J., and Swift, E. (1985). Species-specific grazing rates of heterotrophic dinoflagellates in oceanic waters, measured with a dual-label radioisotope technique. Mar. Biol. 87, 289–296. doi: 10.1007/BF00397808
Mitscherlich, E. A. (1928). Die zweite Annäherung des Wirkungsgesetzes der Wachstumsfaktoren. Z. Pflanzenernährung Düngung Bodenkd. 12, 273–282. doi: 10.1002/jpln.19280120502
Pace, M. L., and Bailiff, M. D. (1987). Evaluation of a fluorescent microsphere technique for measuring grazing rates of phagotrophic microorganisms. Mar. Ecol. Prog. Ser. 40, 185–193. doi: 10.3354/meps040185
Putt, M. (1991). Development and evaluation of tracer particles for use in microzooplankton herbivory studies. Mar. Ecol. Prog. Ser. 77, 27–37. doi: 10.3354/meps077027
R Core Team (2021). R: A Language and Environment for Statistical Computing. Vienna: R Foundation for Statistical Computing.
Ribalet, F., Swalwell, J., Clayton, S., Jiménez, V., Sudek, S., Lin, Y., et al. (2015). Light-driven synchrony of Prochlorococcus growth and mortality in the subtropical Pacific gyre. Proc. Nat. Acad. Sci. U.S.A. 112, 8008–8012. doi: 10.1073/pnas.1424279112
Rublee, P. A., and Gallegos, C. L. (1989). Use of fluorescently labeled algae (FLA) to estimate microzooplankton grazing. Mar. Ecol. Prog. Ser. 51, 221–227. doi: 10.3354/meps051221
Schmoker, C., Hernández-León, S., and Calbet, A. (2013). Microzooplankton grazing in the oceans: impacts, data variability, knowledge gaps and future directions. J. Plankton Res. 35, 691–706. doi: 10.1093/plankt/fbt023
Sherr, B. F., Sherr, E. B., and Fallon, R. D. (1987). Use of monodispersed, fluorescently labeled bacteria to estimate in situ protozoan bacterivory. Appl. Environ. Microbiol. 53, 958–965. doi: 10.1128/AEM.53.5.958-965.1987
Sherr, E. B., and Sherr, B. F. (2002). Significance of predation by protists in aquatic microbial food webs. Antonie Van Leeuwenhoek 81, 293–308. doi: 10.1023/A:1020591307260
Sosik, H. M., Olson, R. J., Neubert, M. G., Shalapyonok, A., and Solow, A. R. (2003). Growth rates of coastal phytoplankton from time-series measurements with a submersible flow cytometer. Limnol. Oceanogr. 48, 1756–1765. doi: 10.4319/lo.2003.48.5.1756
Stocker, R., Seymour, J. R., Samadani, A., Hunt, D. E., and Polz, M. F. (2008). Rapid chemotactic response enables marine bacteria to exploit ephemeral microscale nutrient patches. Proc. Natl. Acad. Sci. U.S.A. 105, 4209–4214. doi: 10.1073/pnas.0709765105
Stoecker, D. K. (1998). Conceptual models of mixotrophy in planktonic protists and some ecological and evolutionary implications. Eur. J. Protistol. 34, 281–290. doi: 10.1016/S0932-4739(98)80055-2
Stoecker, D. K., Nejstgaard, J. C., Madhusoodhanan, R., Pohnert, G., Wolfram, S., Jakobsen, H. H., et al. (2015). Underestimation of microzooplankton grazing in dilution experiments due to inhibition of phytoplankton growth. Limnol. Oceanogr. 60, 1426–1438. doi: 10.1002/lno.10106
Strom, S. L., Wolfe, G. V., and Bright, K. J. (2007). Responses of marine planktonic protists to amino acids: feeding inhibition and swimming behavior in the ciliate Favella sp. Aquat. Microb. Ecol. 47, 107–121. doi: 10.3354/ame047107
Verity, P. G. (1991). Measurement and simulation of prey uptake by marine planktonic ciliates fed plastidic and aplastidic nanoplankton. Limnol. Oceanogr. 36, 729–750. doi: 10.4319/lo.1991.36.4.0729
Worden, A. Z., and Binder, B. J. (2003). Application of dilution experiments for measuring growth and mortality rates among Prochlorococcus and Synechococcus populations in oligotrophic environments. Aquat. Microb. Ecol. 30, 159–174. doi: 10.3354/ame030159
Worden, A. Z., Follows, M. J., Giovannoni, S. J., Wilken, S., Zimmerman, A. E., and Keeling, P. J. (2015). Rethinking the marine carbon cycle: factoring in the multifarious lifestyles of microbes. Science 347:6223. doi: 10.1126/science.1257594
Worden, A. Z., Nolan, J. K., and Palenik, B. (2004). Assessing the dynamics and ecology of marine picophytoplankton: the importance of the eukaryotic component. Limnol. Oceanogr. 49, 168–179. doi: 10.4319/lo.2004.49.1.0168
Keywords: saturation approach, grazing rate, Synechococcus, picoeukaryote, microzooplankton
Citation: Archer SD, Lubelczyk LC, Kunes M, McPhee K, Dawydiak W, Staiger M, Posman KM and Poulton NJ (2022) Saturation Approach to Determine Grazing Mortality in Picoeukaryote and Synechococcus Populations. Front. Mar. Sci. 9:844620. doi: 10.3389/fmars.2022.844620
Received: 28 December 2021; Accepted: 08 February 2022;
Published: 17 March 2022.
Edited by:
Gordon T. Taylor, Stony Brook University, United StatesReviewed by:
Andrew J. Irwin, Dalhousie University, CanadaStephen Beckett, Georgia Institute of Technology, United States
Copyright © 2022 Archer, Lubelczyk, Kunes, McPhee, Dawydiak, Staiger, Posman and Poulton. This is an open-access article distributed under the terms of the Creative Commons Attribution License (CC BY). The use, distribution or reproduction in other forums is permitted, provided the original author(s) and the copyright owner(s) are credited and that the original publication in this journal is cited, in accordance with accepted academic practice. No use, distribution or reproduction is permitted which does not comply with these terms.
*Correspondence: Stephen D. Archer, c2FyY2hlckBiaWdlbG93Lm9yZw==