- 1Institute of Geosciences, Goethe University Frankfurt, Frankfurt am Main, Germany
- 2Frankfurt Isotope and Element Research Center (FIERCE), Goethe University Frankfurt, Frankfurt am Main, Germany
- 3National Institute of Water & Atmospheric Research, Wellington, New Zealand
- 4Institute for Environmental Physics, University of Heidelberg, Heidelberg, Germany
Recent oceanographic observations have identified significant changes of intermediate water masses characterized by increased temperatures, lowered pH and deoxygenation. In order to improve our understanding as to how these changes may impact deep-sea ecosystems one important strategy is to reconstruct past oceanic conditions. Here we examine the applicability of the scleractinian cold-water coral Solenosmilia variabilis as a marine archive for the reconstructions of past intermediate water mass temperatures by using Lithium (Li)/Magnesium (Mg) ratios. In particular, our study addresses 1) the calibration of Li/Mg ratios against in-situ temperature data, 2) the reconstruction of past intermediate water mass temperatures using scleractinian coral fossil samples from the Brazilian continental margin and 3) the identification of intraspecies variability within the coral microstructure. Results showed that Li/Mg ratios measured in the skeletons of S. variabilis fit into existing Li/Mg-T calibrations of other cold-water scleractinian. Furthermore, the coral microstructure exhibits interspecies variability of Li/Ca and Mg/Ca ratios were also similar to what has been observed in other cold-water scleractinian corals, suggesting a similar biomineralization control on the incorporation of Li and Mg into the skeleton. However, the Li/Mg based temperature reconstruction using fossil samples resulted in unexpectedly high variations >10°C, which might not be solely related to temperature variations of the intermediate water mass over the last 160 ka on the Brazilian continental margin. We speculate that such temperature variability may be caused by vertical movements of the aragonite saturation horizon and the associated seawater pH changes, which in turn influence the incorporation of Li and Mg into the coral skeleton. Based on these results it is recommended that future studies investigating past oceanic conditions need to consider the carbonate system parameters and how they might impact the mechanisms of Li and Mg being incorporated into skeletons of cold-water coral species such as S. variabilis.
1 Introduction
The reconstruction of past oceanic and environmental conditions is one important application to help predict the impact of the current climate change on ocean circulation, ocean chemistry and deep-sea ecosystems. Seawater temperature is a particularly important parameter to assess as temperature influences various physical and chemical processes within the oceanic system (Weyl, 1959). For decades, the Antarctic Intermediate water (AAIW) has experienced warming off the coast off Brazil. From 1958 to 2009, the temperature has increased to about 0.23°C (McCarthy et al., 2011).
To accurately predict how anthropogenic climate change will affect the mid-depth of the South Atlantic, it is crucial to study the sensitivity of physiochemical properties in marine systems to changes in atmospheric greenhouse gases such as CO2, as well as rising atmospheric temperatures. Already, it is known how much deep ocean waters in certain regions have changed in terms of temperature. During the last ice age and the deglacial period (about 20,000-10,000 years ago), high-resolution records from ice cores from polar regions showed rapid (centennial to decadal) changes in ocean climate (Barnola et al., 1987; Indermühle et al., 1999; Petit et al., 1999; Augustin et al., 2004). Conventional paleoceanographic reconstructions are often made from marine sediment cores (e.g. Charles et al., 1996; Raddatz et al., 2017) but these have the disadvantage of being intermixed with marine organisms, thus blurring rapid signals (e.g. Leuschner et al., 2002). In addition, the timing of deposition is difficult to determine from the sediment cores, leading to inaccurate age models. As such, there is an urgent need to combine both atmospheric and oceanic records to understand the various coupling mechanisms involved during times of rapid climate change.
In this study cold-water scleractinian stony corals present a promising new tool to reconstruct past ocean properties. In comparison to other marine proxy archives in use, this group have the advantage that they, 1) commonly occur in almost all oceans, 2) are located in great depths, living far from sunlight inn well over 1000 m depth, 3) incorporate the isotopic and element composition of the surrounding seawater in equilibrium with various ocean parameters such as temperature and pH, 4) can live for decades to millennia, and lastly 5) their skeletons can be accurately dated using radioactive isotope decay methods (Cheng et al., 2000; Houlbrèque et al., 2010; Frank et al., 2011; Montagna et al., 2014; Raddatz et al., 2014a; Robinson et al., 2014; Wefing et al., 2017; Freiwald et al., 2021).
For instance, U/Ca and boron isotope systematics are commonly used for reconstruction of parameters of the carbonate system (Sinclair et al., 2006; Blamart et al., 2007; Anagnostou et al., 2011; Anagnostou et al., 2012; McCulloch et al., 2012; Raddatz et al., 2014a; Raddatz et al., 2014b; Raddatz et al., 2016). Element ratios such as P/Ca, B/Ca and Cd/Ca in scleractinian corals are known for the nutrient concentration (Adkins et al., 1998; Montagna et al., 2006; Mangini et al., 2010; Anagnostou et al., 2011; Hemsing et al., 2018; Spooner et al., 2018). Biological processes however, need to be considered in the development of proxies in coral skeletons, as the incorporation of the elements can be affected by different pathways. The most pronounced geochemical and mineralogical variations occur in corals between the center of calcification (COC) and the theca wall (e.g. Gladfeiter, 1982; Cohen et al., 2006; Meibom et al., 2006; Sinclair et al., 2006; Gladfelter, 2007; Meibom et al., 2008; Rollion-Bard et al., 2010; Raddatz et al., 2013; Schleinkofer et al., 2019). The COC is composed of small granular crystals and the fibrous aragonite of organic mineral compounds forming long aragonite needles (Gladfeiter, 1982; Constantz, 1989; Gladfelter, 2007). Various vital effects influence geochemical properties within the calcification process, both on the pathway itself as well as in the formation of the skeletal structure (Adkins et al., 2003; Gagnon et al., 2007). It is postulated that there are several ways that the elements enter the calcifying fluid. According to Holcomb et al. (2009) and Gagnon et al. (2007) the increase of the pH in the extracellular calcifying fluid causes a higher saturation of the Element/Ca (E/Ca) ratio in the COC than in the fibrous aragonite. In contrast Rollion-Bard et al. (2010) assumes the fibrous aragonite and COC to be formed independently and therefore have different E/Ca composition. Other changes that may cause a difference in the coral chemistry and calcification process are Rayleigh fractionation and kinetic effects (Cohen et al., 2006; Montagna et al., 2006; Sinclair et al., 2006; Gagnon et al., 2007).
Recent studies developed a method to overcome such “vital effects”, by implementing Li/Mg ratio of aragonitic marine carbonates (Bryan and Marchitto, 2008; Case et al., 2010; Hathorne et al., 2013; Raddatz et al., 2013). A positive correlation between Li/Ca and Mg/Ca suggest that the uptake mechanism and the biological and physicochemical factors are in fact the same for both E/Ca (Case et al., 2010; Raddatz et al., 2013; Montagna et al., 2014). By combining Li/Ca and Mg/Ca ratios the effect of the biological processes is reduced, resulting in temperature being the primary factor to influence the coral’s Li/Mg composition. Hence, the Li/Mg ratios exhibits a strong inverse exponential relationship with temperature across both warm and cold-water corals of 0-30°C (Case et al., 2010; Hathorne et al., 2013; Raddatz et al., 2013; Montagna et al., 2014). This is also seen in benthic foraminifera (Stewart et al., 2020). Very few studies to date have applied this proxy for the reconstruction of past seawater temperatures (e.g. Montero-Serrano et al., 2013; Raddatz et al., 2014b; Fowell et al., 2016; Raddatz et al., 2016; Cuny-Guirriec et al., 2019).
The cold-water coral (CWC) Solenosmilia variabilis is one of the six most important reef building corals located in the deep sea but thus far, this group has not been the focus of paleoceanographic studies. S. variabilis occurs primarily in depths of 220-2.165 m and is often found below the dominat depths of the cold-water corals Desmophyllum pertusum (also known as Lophelia pertusa) and Madrepora oculata. The distribution of S. variabilis extends over mostly all oceans of the world however no occurrences have been recorded from the Antarctic Ocean nor in the North and East Pacific regions (Cairns, 1995; Freiwald et al., 2004; Davies and Guinotte, 2011). A possible reason for this distribution pattern is the appearance of S. variabilis in the aragonite undersaturated zones or close to the aragonite saturation horizon (Davies and Guinotte, 2011; Bostock et al., 2015), which in the North Pacific is in > 2000 m at greater depths than in the Northeast Atlantic (50-600 m) (Guinotte et al., 2006). Suitable growth areas are predicted in the Atlantic at continental slopes and the Mid ocean ridge (Davies and Guinotte, 2011). Today live and dead S. variabilis colonies are located primarily on underwater features such as seamounts in the New Zealand (NZ) region (Tracey et al., 2011) as well as in the Drake passage in the southwestern part of the Atlantic Ocean, southeastern Pacific Ocean. While the reef-like colonies in NZ do survive in waters that are deeper than 2000 m, they thrive in depths from ~800 to 1000 m (Davies and Guinotte, 2011; Tracey et al., 2011; Bostock et al., 2015; Freiwald et al., 2021).
Here we study the applicability of the scleractinian cold-water coral S. variabilis as a geochemical proxy archive for the reconstructions of past intermediate water masses in the Brazilian Continental margin, South Atlantic and in the South Pacific region. In particular, we address 1) the calibration of Li/Mg ratios against in-situ temperature, 2) the reconstruction of past intermediate water mass temperature using fossil samples and 3) discuss the intraspecies variability of Li/Mg ratios.
1.1 Study area
1.1.1 SW Pacific
Live collected S. variabilis were provided from the National Institute for Water and Atmosphere (NIWA), Wellington, New Zealand. The sampling area is restricted to offshore deep waters the NZ Exclusive Economic Zone in the Southwest Pacific (Figure 1A; Table 1). Two samples were collected from a ridge-like feature on, the Chatham Rise, an area that stretches about 1400 km to the east of New Zealand (Clark and Rowden, 2009) and one sample was gathered north of New Zealand, in the southern area of the Kermadec Ridge. All samples were located in the Antarctic intermediate water (AAIW) at a water depth of 1000-1300 m and a temperature range of 3.7-5.4°C (Locarnini et al., 2009). The samples are all located in the lower part of the AAIW, characterized by a low salinity of 34.4 g/kg (Antonov et al., 2009).
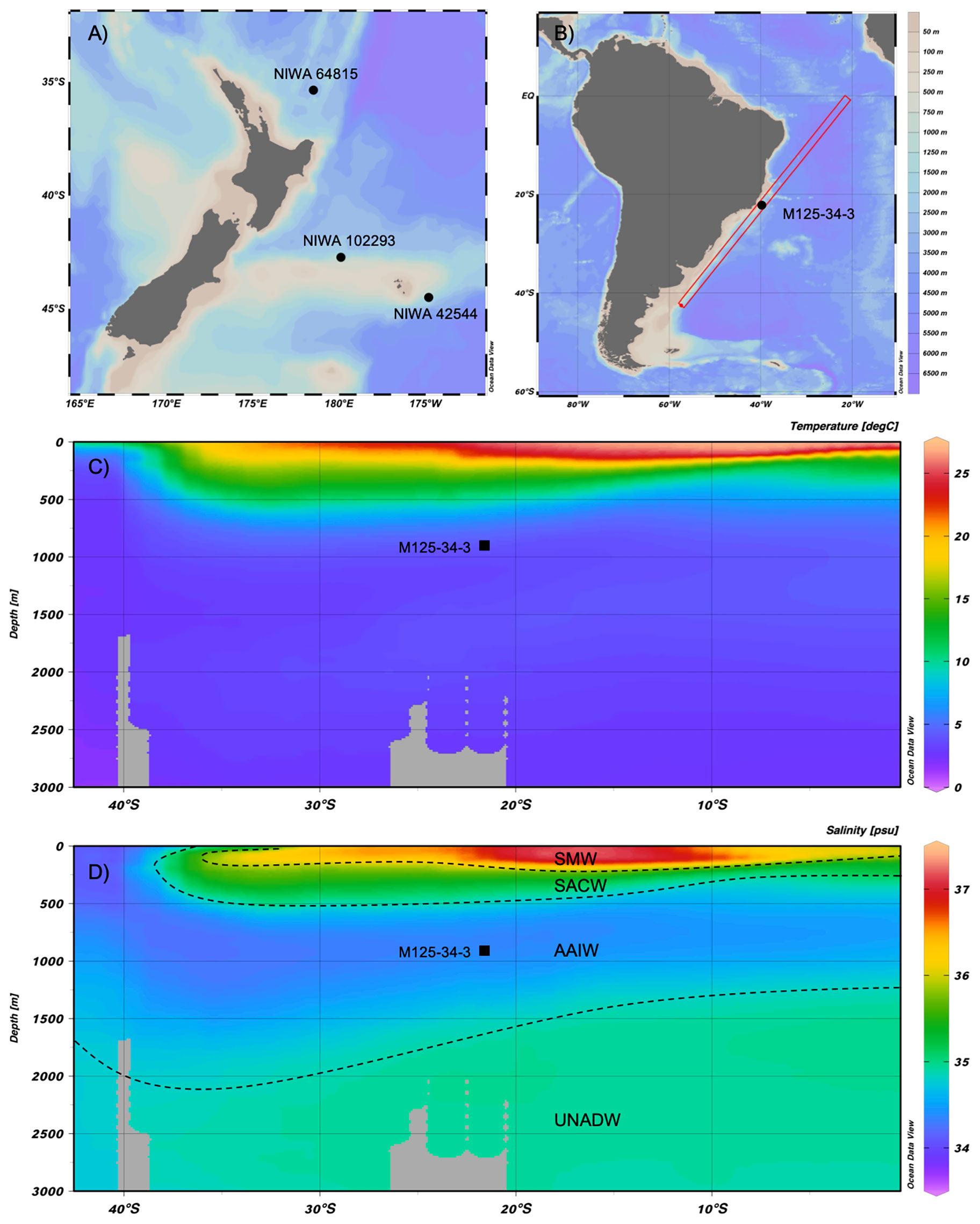
Figure 1 Map of the sampling locations: (A) live samples locations off New Zealand and (B) the location of the sediment core M125-34-3 off the coast of Brazil. (C) Temperature profile and (D) salinity profile of marked area in B, the black squares mark the position of the Bowie Mound (Schlitzer, 2021). The dashed lines show the boundary of the water masses from shallow to deep. These are: the salinity maximum waters (SMW), South Atlantic Central waters (SACW), Antarctic intermediate waters (AAIW) and Upper North Atlantic deep waters (UNADW).

Table 1 Coordinates of the analyzed samples in the Pacific (NIWA 102293; NIWA 42544; NIWA 64815) and the gravity core (M125-34-2) in the South Atlantic.
1.1.2 South Atlantic
The modern hydrography around the Bowie Mound, in the South Atlantic, to the east of Brazil is shown in Figure 1. The top water mass is the Salinity maximum water and reaches depth of up to 200 m (Mémery et al., 2000). In deeper waters, the north flowing South Atlantic Central Water (SACW) is characterized by lower temperatures and salinities. At the depth of the Bowie Mound (866 m) the AAIW follows with a transport direction to the north and low salinity (< 34.4 g/kg). At 900 m the ΩAr is ~1.2, the pH of the seawater ~7.97, the concentration 79 µmol/kg and temperatures are about 4.3°C (Raddatz et al., 2020). Beneath AAIW the Upper North Atlantic Deep Water (UNADW) flows in the southward direction with higher salinity (Antonov et al., 2009).
2 Material and methods
Three live collected samples of S. variabilis came from various research surveys in New Zealand waters, Southwest Pacific region. Sample depth and temperature details are as follows: NIWA 102293 was collected at a depth of 1020 m in 5.4 ± 0.2°C; NIWA 42544 in 1386 m at 3.8 ± 0.1°C; and NIWA 64815 in a depth of 1382 m at 3.7 ± 0.2°C (Figures 1A, 2A; Table 1).
Fossil S. variabilis (Figure 2B) samples were retrieved during cruise M125 with the German research vessel METEOR and originate from the Bowie Mound, east of Brazil in a depth of 866 m and in 4.3°C (Figure 1; Table 1; Bahr et al., 2016).
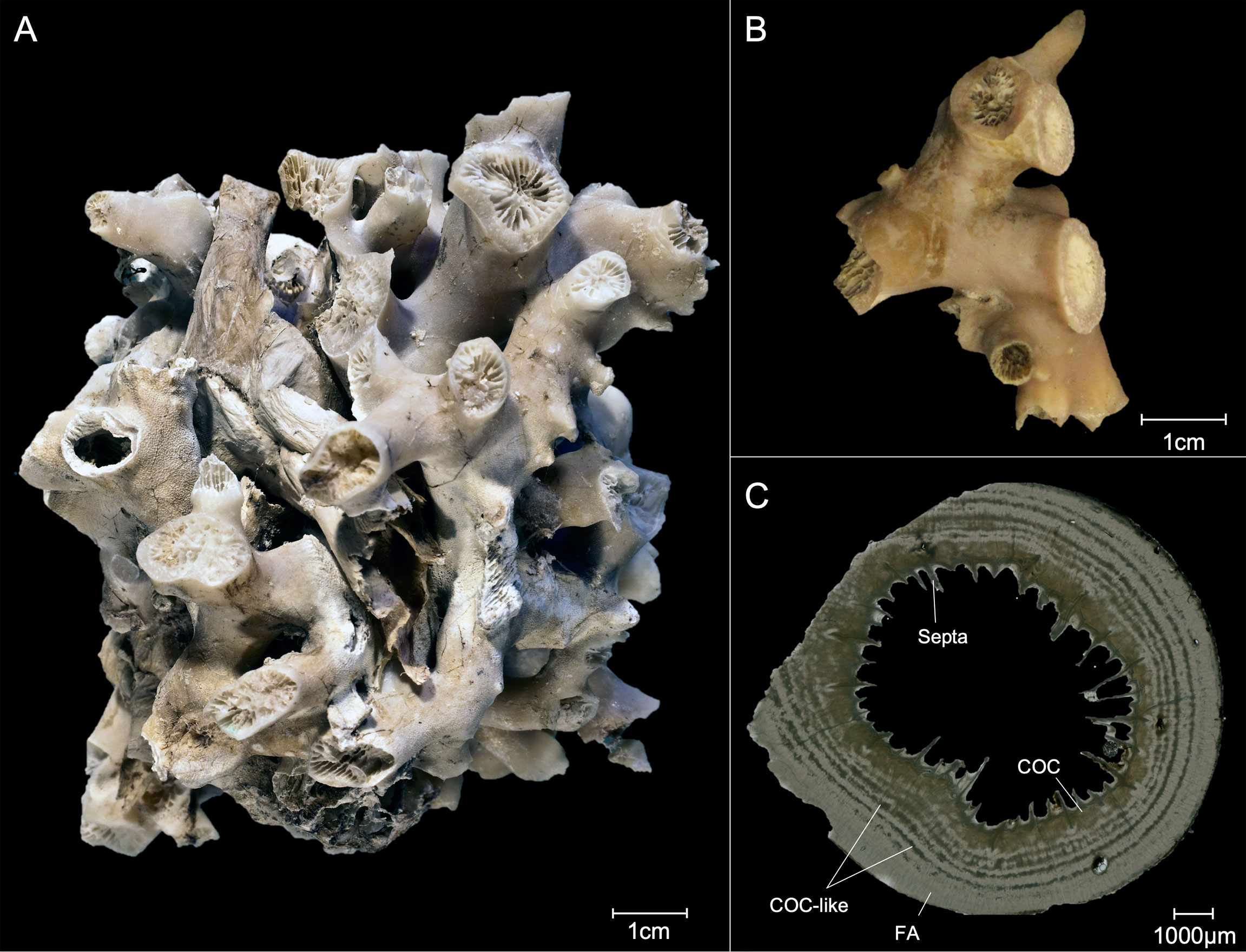
Figure 2 Different representations of the coral samples of S. variabilis: (A) Live picked sample in the Pacific near New Zealand. (B) Fossil sample of the Bowie Mound in AAIW and (C) cross section of a fossil sample; COC, center of Calcification; FA, Fibrous aragonite.
The 5.86 m gravity core retrieved from Bowie Mound covered 160 ka and was characterized by non-coral-bearing and coral-bearing intervals. The coral-bearing intervals mainly comprised fossil forms of S. variabilis cold-water corals (Raddatz et al., 2020), and these were bedded in silty clay and clay-like sand sediment (Bahr et al., 2020), providing very good preservation of the coral fragments.
The coral fragments were dated by using the 230Th/U method (seen in Raddatz et al., 2020). Further information on the structure of the sediment core can be obtained from the studies by Raddatz et al. (2020) and Bahr et al. (2020). For this study a total of 27 samples were analyzed.
2.1 Element/Ca measurements
About 1 mg of calcium-carbonate was taken from the uppermost calices extracted with a Proxxon IBS/E dremel tool out of the theca wall. Samples have been drilled by avoiding visible lines of calcification (COC), as they display different element compositions compared to the surrounding theca wall (Gagnon et al., 2007; Montagna et al., 2014). Subsequently, samples were cleaned by weak acid leach in 5 % HNO3 for 5 sec. Samples for the heterogeneity profiles (Figure 2C; N = 5-6) did not avoid COCs and were extracted using a Micromill.
Elemental ratios of CWCs were measured with an Inductively-Coupled-Plasma-Mass-Spectrometer (ICP-MS) ThermoScientific Element 2 at Fierce, Institute of Geoscience, Goethe University Frankfurt. Approximately 150 µg of homogenized carbonate powder was dissolved in 1.8 ml 2% HNO3 containing 1.2 ppm Yttrium (Y) used as an internal standard. The measured intensities were background subtracted and standardized internally to Y and normalized to Ca. External standards were made from single-element solutions to match typical element compositions of cold-water scleractinian corals (Rosenthal et al., 1999). The coral standard JCp- 1 was measured after every fifth sample in order to allow for any drift corrections. The measured JCp-1 (N = 15) are consistent with the recommended values by Hathorne et al. (2013): Li/Ca 6.185 ± 0.107 µmol/mol, Mg/Ca 4.199 ± 0.065 mmol/mol and this study Li/Ca 6.036 ± 0.701 µmol/mol (97.6 %), Mg/Ca 4.153 ± 0.374 mmol/mol (98.9 %).
3 Results and discussion
3.1 S. variabilis as a geochemical archive for Li/Mg based temperature reconstructions
3.1.1 Adding S. variabilis to the Li/Mg-T calibration
In previous studies, elemental ratios (e.g. Li/Ca, Sr/Ca, Mg/Li) have been identified to be temperature depended in scleractinian cold-water corals (Case et al., 2010; Raddatz et al., 2013; Raddatz et al., 2014a). The most robust temperature proxy, including aragonitic zooxanthellate (shallow) and azooxanthellate (deep) scleractinian, appears to be Li/Mg ratio (Case et al., 2010; Montagna et al., 2014; Cuny-Guirriec et al., 2019; Stewart et al., 2020).
To test the cold-water coral S. variabilis as a suitable archive for reconstructions of intermediate water temperatures, the Li/Mg-temperature calibration by Montagna et al. (2014), was applied:
This calibration includes a temperature range of 0-30°C for cold- temperate- and warm-water scleractinian corals. In addition, the study by Stewart et al. (2020) extended this calibration to cover other aragonitic corals, including stylasterid hydrocorals, scleractinian (including Zooxanthellate) corals, as well as foraminifera:
within uncertainty similar to the original calibration by Montagna et al. (2014).
Using the in-situ temperature data, extracted from World Ocean Atlas 2009 (Locarnini et al., 2009), the live collected samples from the SW Pacific produced expected Li/Mg ratios of 2.74 ± 0.14 mmol/mol (2SD) at 5.4 ± 0.5°C, 4.52 ± 0.78 mmol/mol at 3.8 ± 0.1°C and 3.74 ± 0.61 mmol/mol at 3.7 ± 0.2°C (Figure 3). Combining these data with published data from Case et al. (2010) and Montagna et al. (2014) results in the following empirical Li/Mg-T calibration:
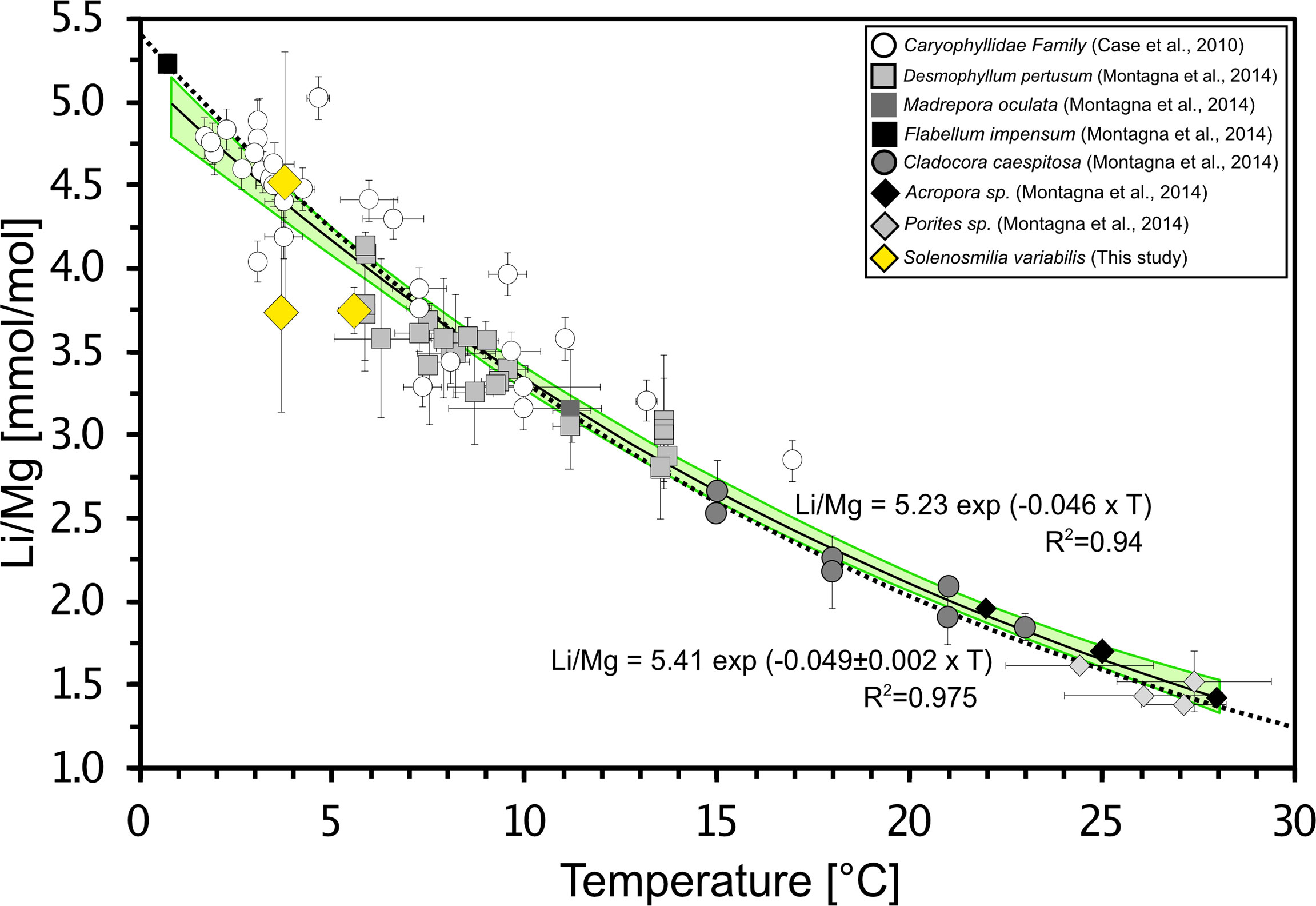
Figure 3 Li/Mg. ratio vs. seawater temperature for the published data Case et al. (2010); Montagna et al. (2014) and new data from current study (yellow diamond). The temperature range of cold-water, temperate, and warm-water corals is 0-30°C with a range of the Li/Mg of 1-5.5 mmol/mol measured by laser and solution ICP-MS. The additional measurements are 2.74 ± 0.14 mmol/mol (2SD) at 5.4 ± 0.2°C, 4.52 ± 0.78 mmol/mol at 3.8 ± 0.1°C and 3.74 ± 0.61 mmol/mol at 3.7 ± 0.2°C. The calibration of Montagna et al. (2014) is shown as black dashed line (R2 = 0.975). The black solid line indicates the new function with additional data of this study (R2 = 0.94). Light green shaded band show the 95% confidence interval.
The new samples and calibration are consistent with the original exponential function by Montagna et al. (2014), considering the confidence interval (Figure 3).
3.2 Li/Mg based temperatures using fossil S. variabilis from Bowie Mound, off Brazil
The Li/Mg measurements obtained from the Bowie Mound samples produced a variability of 2.55-5.55 ± 0.11 mmol/mol (Figure 4), resulting in a large temperature range of - 0.53 to 15.34 ± 1.55°C, when applying the Li/Mg-Temperature calibration by Montagna et al. (2014). In particular, four samples plot outside the expected temperature range of 4-15.2°C (Mienis et al., 2014; Raddatz and Rüggeberg, 2021). AAIW temperatures of up to 15°C appear very high considering the water depth of almost 900 m (Figure 5). However, Mg/Ca data based temperature reconstruction using the endobenthic foraminiferal species Uvigerina spp. also show reconstructed temperature variations of 8-9°C ( ± 1°C 2σ) in the intermediate water (IW) (852 m water depth) in the SW Atlantic (M78/1-235-1; 11°36.53’N, 60°57.86’W) over the last 24 ka (Poggemann et al., 2018).
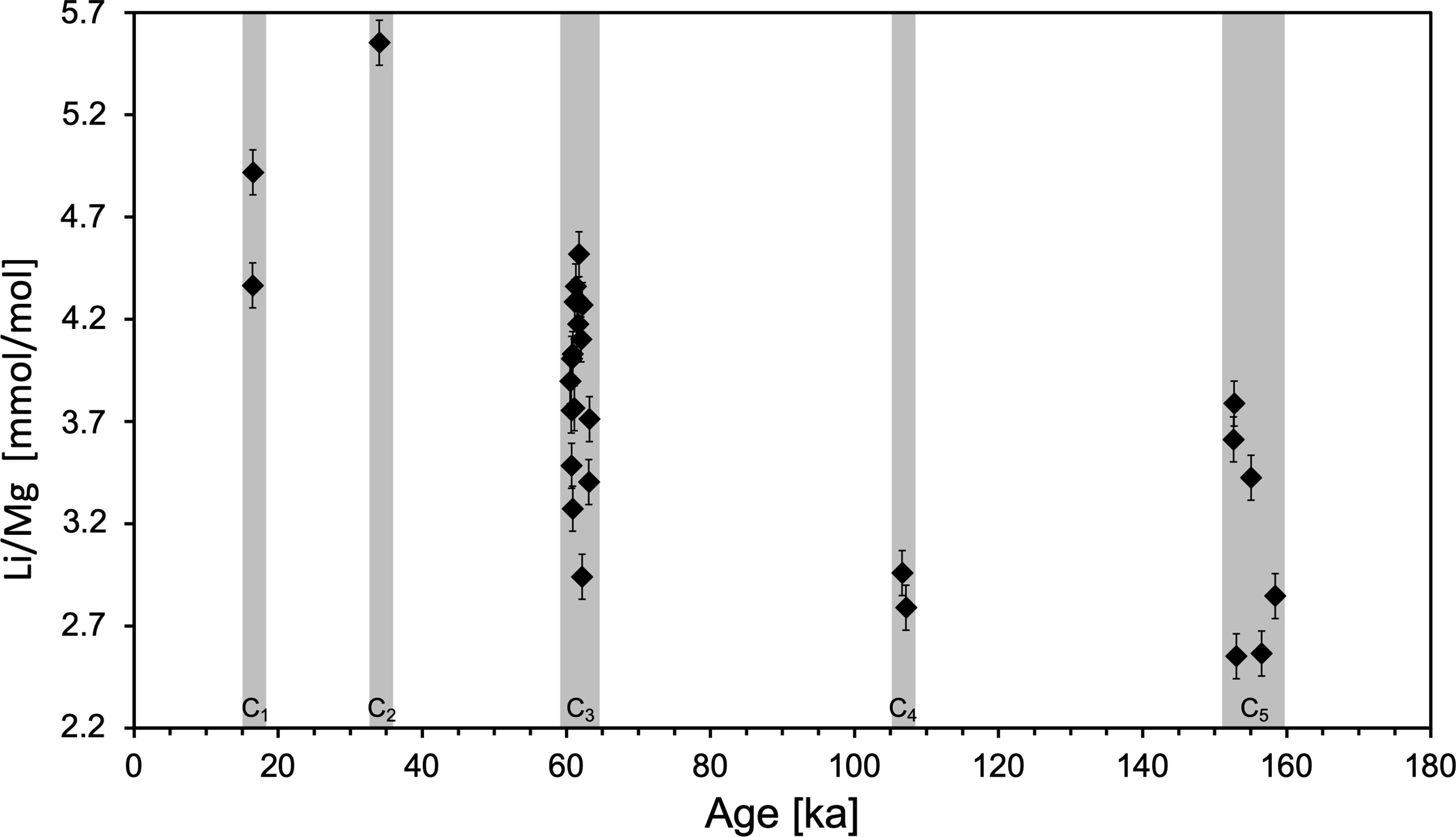
Figure 4 Li/Mg [mmol/mol] ( ± 0.11 2SD) measurements of fossil S. variabilis of the past 180 ka, subdivided in intervals C1-C5.
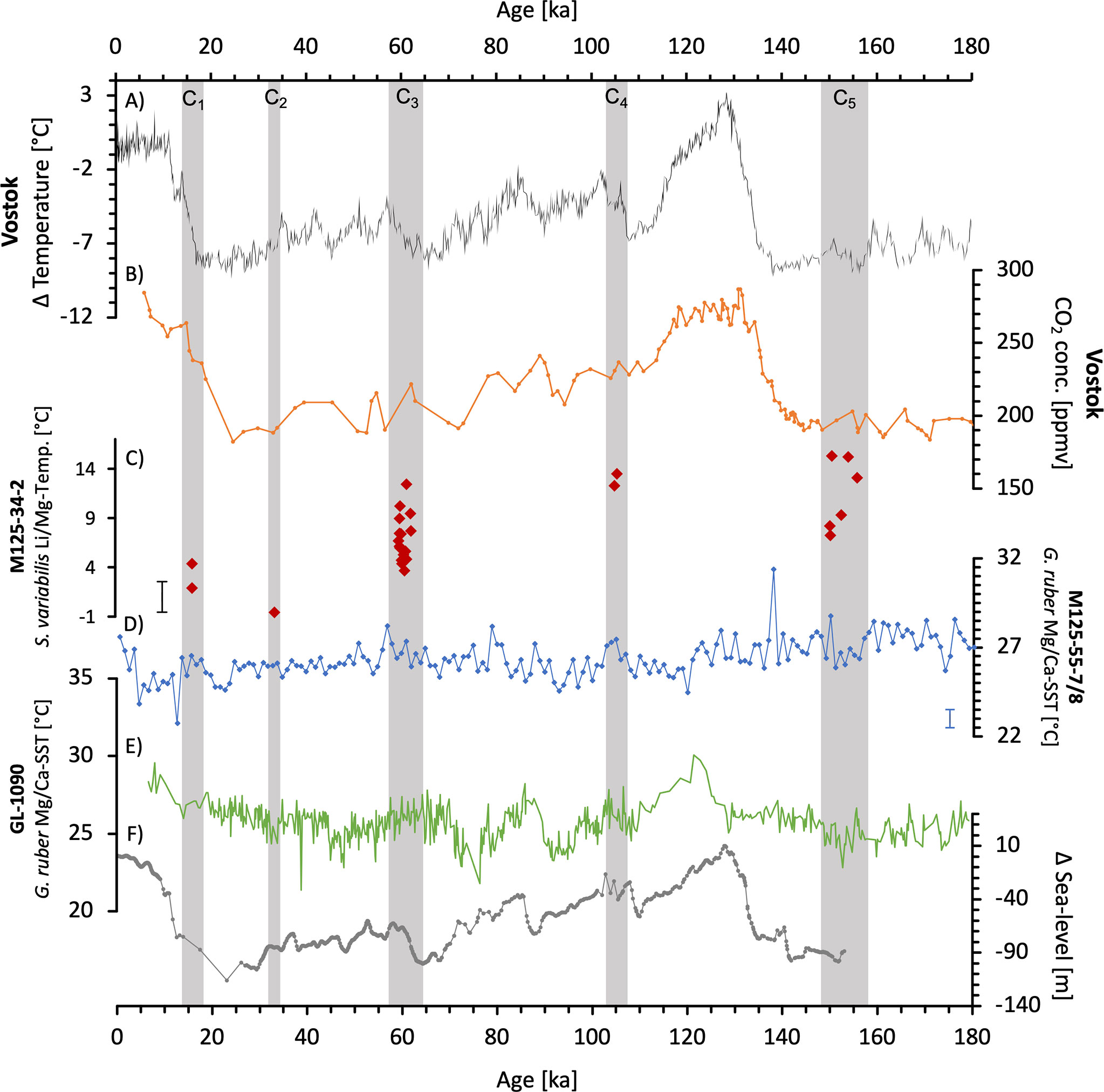
Figure 5 Evolution of enviromental parameters over the last 180 ka before present. (A) The temperaturefluctuations, averaged over the last 1,000 years, from the Vostok ice core (Petit et al., 1999). (B) CO2 concentration in ppmv reconstructed from the Vostok ice core (Petit et al., 1999). (C) Temperature reconstruction of the SAIW from sediment core M125-34-2, using the Li/Mg in the CWC S. variabilis (this study). With a 2SD of 1.55°C calculated from the JCp-1 measurements. (D) Temperature reconstruction of surface water from the southwestern tropical Atlantic (M125-55-7/8) based on the Mg/Ca of Globigerinoides ruber (G. ruber) (pink). The standard deviation is ±0.5°C (Hou et al., 2020). (E) Surface water temperatures of the subtropical western South Atlantic reconstructed from sediment core GL-1090 of the Mg/Ca of G. ruber (Santos et al., 2017). (F) Global sea level variations referenced to present-day sea level (Grant et al., 2012). The gray bars mark the intervals (C1-5) of S. variabilis.
The Bowie Mound represent five distinct coral growth periods (C1-C5) according to their occurrence between 0-160 ka (Raddatz et al., 2020) (Table 2). The calculated average Li/Mg temperature are with 7.71°C ( ± 1.55°C, 2SD), by 3.4°C higher than the present day ambient AAIW (Raddatz et al., 2020).
At about 16.5 ka, Poggemann et al. (2018) recorded an IW temperature of 4.4°C based on the Mg/Ca ratios of benthic foraminifera, which is consistent with the average temperature of 3.2°C in this study in C1 (Table 2). The cold temperatures of < 4°C retrieved from one of the two coral fragments in C1 and the one sample from C2 cannot be treated as outliers, since Roberts et al. (2016) also reconstructed temperatures below 0°C in the IW at a depth of 598 m 2-20 ka BP. Accordingly, we assume here that the cold temperatures are part of the ecological niche of S. variabilis, as they live in the South Pacific Region of New Zealand at 2-3°C (Davies and Guinotte, 2011).
Compared to average temperature of the last 160 ka, S. variabilis occurred in colder temperature periods (Figure 5A), in times when both, global sea level and atmospheric CO2 concentration were lower than they are today (Figures 5B, F; Petit et al., 1999; Grant et al., 2012). The occurrence of S. variabilis during these periods correlates with the cold events in the northern hemisphere, in particular the Heinrich stadial (HS) 1 before 14.6-18 ka, the HS4 before approx. 40 ka and the HS6 before 60 ka, as reported in the study by (Raddatz et al., 2020). During HS’s large amounts of ice collapsed from the northern ice sheets (Toggweiler and Lea, 2010), thereby reduced the temperature gradient between the two hemispheres (Toggweiler and Lea, 2010). There is an ongoing discussion as to whether the Atlantic meridional overturning circulation (AMOC) was significantly weakened during such periods or possibly completely ceased and therefore inhibited North Atlantic deep-water formation (NADW) (Böhm et al., 2015; Lund et al., 2015; Lynch-Stieglitz, 2017; Poggemann et al., 2017). The conditions for a collapse scenario could only have been fulfilled in HS1, HS2 and HS11; HS3 to HS10 resulted only to a weakening of NADW formation (Böhm et al., 2015). Poggemann et al. (2018) postulated three possible explanations for the heating of IW in HS1: (1) the exchange of the AAIW with warm water, (2) a heating of the surface water, which is transferred to the intermediate water, or (3) a warming of the AAIW itself. In the first scenario, warmer water masses from the north would have had to replace the IW or at least be involved in their warming (Poggemann et al., 2018). However, the influence of overlying or northerly water masses could not be detected by using different proxies (Poggemann et al., 2018). The second scenario can be excluded here as the SST in the South Atlantic (Figures 5D, E; Santos et al., 2017; Hou et al., 2020) was quite constant and do not correlate with the temperature at S. variabilis locations in the IW. Therefore, the present data set supports the hypothesis of the third scenario, in which the AAIW warmed by itself. Poggemann et al. (2018) demonstrated a warming of the IW in HS1 of about 1.6-10.9°C, which is consistent with the temperature ranges of 1.4-3.3°C obtained in this study. The apparently weakened or even ceased southward flowing NADW no longer influenced the formation of the AAIW in the HS1 (Böhm et al., 2015; Poggemann et al., 2017). Additionally, this contributed to an intense upwelling and release of CO2 from the Circumpolar Deepwater (CPDW) in the SO (e.g. Broecker, 1998; Burke and Robinson, 2012; Poggemann et al., 2017). The resulting increase in nutrient concentration in the SO could not be metabolized due to an insufficient iron content (e.g. Martínez-García et al., 2014). Subsequently, these excessive nutrients were transported northwards via AAIW to the tropical West Atlantic (Poggemann et al., 2017). This may have increased primary production and enhanced the biological pump, leading to a deglacial CO2 plateau in the atmosphere and an elevated export of organic matter to the deep sea (Archer et al., 2000; Sigman and Boyle, 2000; Marcott et al., 2014). The resulting higher respiration outputs of CO2 in the AAIW has the potential to lower seawater pH and drive aragonite dissolution, thereby shift the aragonite compensation depth. The study by Allen et al. (2015) presents such an effect of . decrease in the deep water and a lowering in atmospheric CO2 in the southwest Pacific during the onset of HS1 (18-20 ka). In contrast Lynch-Stieglitz et al. (2019) suggests that the high nutrient content in the water masses is not the source of the high CO2 content in the deglacial. The study assumes a direct atmosphere CO2 uptake direct by the surface and intermediate water masses in the Southern and North Atlantic.
It is typical for S. variabilis to grow in a temperature range of 3-4°C, which differs by 3°C from D. pertusum (Fallon et al., 2014; Flögel et al., 2014; Gammon et al., 2018). As D. pertusum exhibits temperature fluctuations of more than 6°C in Tisler Reef (Guihen et al., 2012), we also suggest a large tolerance range of temperature (> 8°C) and possibly other environmental parameters for S. variabilis. While D. pertusum occurs primarily in interglacial periods at higher latitudes at shallower depths, S. variabilis has already been documented to prefer more extreme environmental conditions at lower latitudes, greater depths and thus less aragonite-saturated water masses (Schröder-Ritzrau et al., 2005; Thresher et al., 2011; Flögel et al., 2014; Bostock et al., 2015; Gammon et al., 2018).
Considering the high temperature tolerance, we assume, that a secondary factor influences the incorporation of Li and Mg into the coral skeleton. Regarding the lower aragonite saturation within the occurrence of S. variabilis, we propose the aragonite saturation here as a secondary controller of Li/Mg. At a aragonite saturation(ΩAr = ([Ca2+] x [ ])/Ksp; Ksp is the solubility product of aragonite) ΩAr > 1 precipitation is induced, whereas at ΩAr< 1 dissolution of aragonite is preferred (Mucci, 1983; Doney et al., 2009).
Aragonite dissolution in the late Pleistocene South Atlantic has been reconstructed using the pteropod Limacina inflata dissolution index (LDX) (Gerhardt et al., 2000; Gerhardt and Henrich, 2001). In particular, in the western South Atlantic, LDX traced undersaturated AAIW and Upper Circumpolar Deep Water (UCDW; Henrich et al., 2003). The coral periods (C1-C4) mostly occur during such phases of low aragonite saturation (Henrich et al., 2003). Since aragonite saturation influences the calcification rate, this could also have an impact on the elemental composition of the Li/Mg. Such an effect has been demonstrated in foraminifera on Mg/Ca-temperature proxy (Gray et al., 2018; Gray and Evans, 2019). Several pathways of element incorporation into foraminifera shells have been postulated as well as vital effects that control the incorporation of Mg during calcification (Bentov and Erez, 2006). Furthermore it has been shown that also salinity and pH influence the Mg/Ca the shell composition (Gray and Evans, 2019). In addition to the temperature sensitivity, the study conducted by Gray and Evans (2019) on foraminifera revealed a salinity sensitivity of 3.6 ± 0.01 % (2SD) per g/kg and a carbonate chemistry sensitivity of -5 to -9 % per 0.1 pH unit. This may also be valid for Li/Mg with respect to aragonite saturation. To follow this up, further studies should investigate the dependence of Li/Mg on aragonite saturation using boron isotopes and U/Ca (Anagnostou et al., 2011; Anagnostou et al., 2012; McCulloch et al., 2012).
3.3 Heterogeneity profiles of Li/Ca and Mg/Ca in S. variabilis
Different fluctuations on the cross-sectional axis, with almost uniform changes are reflected in the elemental ratios of the coral skeleton (Figure 6). Here the change of E/Ca ratios are prominent in the COC and fibrous aragonite (theca wall) (Figure 2). In three of four samples, the Mg/Ca and Li/Ca ratios exhibits a decreasing trend following the direction of the COC structure to the theca-wall (Figures 6A–C), at the fourth sample (Figure 6D) the Li/Ca and Mg/Ca increase. The Mg/Ca shows a range of 0.75-4.71 ± 0.25 mmol/mol (2SD). Within the coral structure the variability is about 0.75-2.27 mmol/mol, with an average variation factor of 1.5. The Li/Ca varies between 8.48 and 19.31 ± 0.77 µmol/mol (2SD), with an internal fluctuation of ~5 µmol/mol and an average variation factor of 1.5. The corresponding Li/Mg ratios indicate an increase in the direction of the theca-wall in three samples, in the fourth sample the trend is opposite. The measured range is 2.93-5.15 ± 0.11 mmol/mol (2SD).
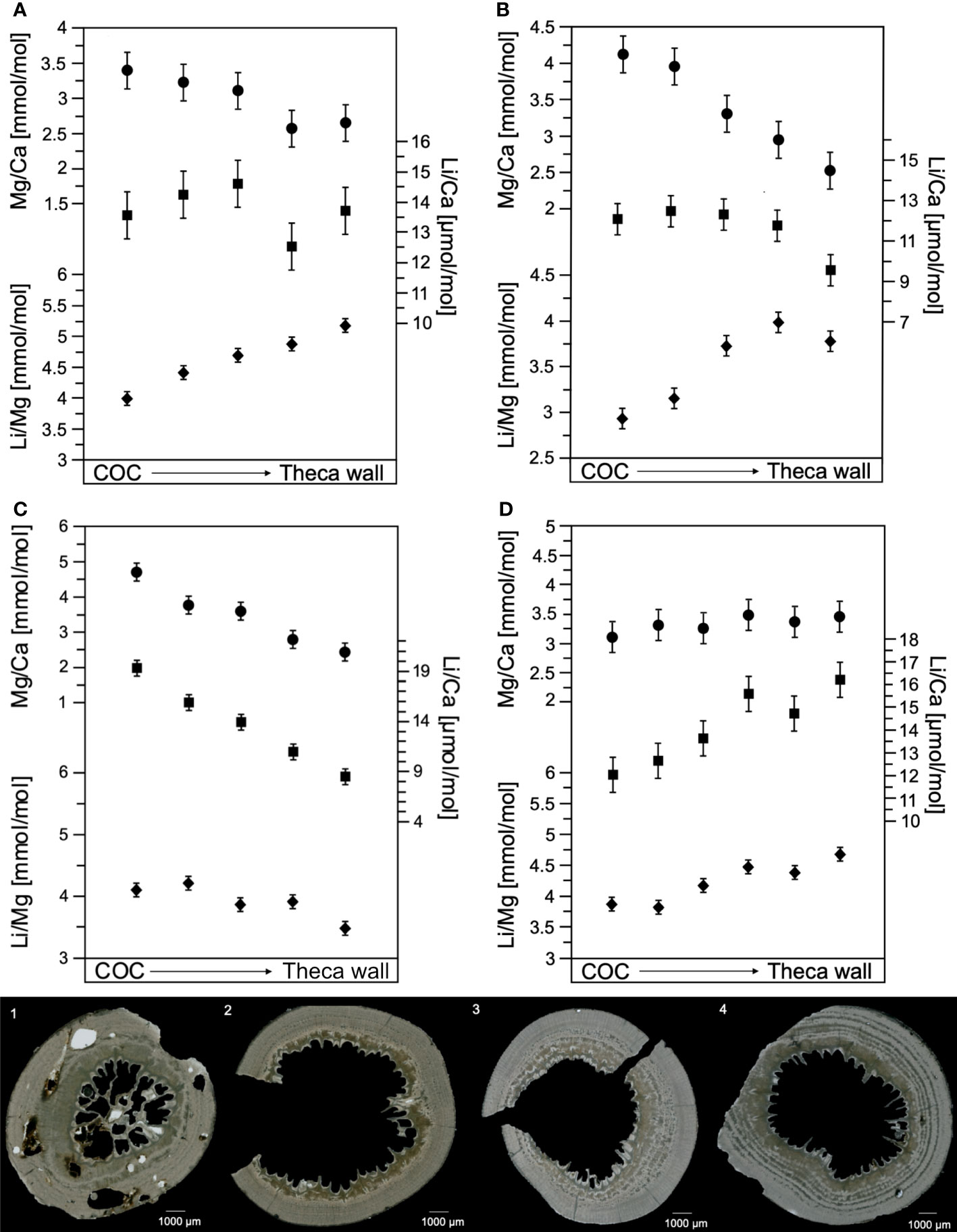
Figure 6 Elemental ratios Mg/Ca [mmol/mol], Li/Ca [μmol/mol], and Li/Mg [mmol/mol], of four samples from the South Atlantic. The doubled standard variation for Mg/Ca ±0.25 mmol/mol, Li/Ca ±0.77 µmol/mol and Li/Mg ±0.11 mmol/mol was determined by JCp-1 measurements. Figures 1–4 below, show the cross-section of the analyzed sample with an allocation of 1-A, 2-B, 3-C and 4-D. Samples were collected by micromill starting at the COC structure ongoing until the theca wall.
Previous studies also indicated internal fluctuations of Mg/Ca and Li/Ca up to a variation factor of 3 (e.g. Case et al., 2010; Raddatz et al., 2013; Montagna et al., 2014). Here we compare the S. variabilis measurements with the additional scleractinian cold-water corals: D. pertusum, Balanophyllia sp., Desmophyllum cristagalli (D. cristagalli) and Desmophyllum dianthus (D. dianthus) (Figure 7). D. pertusum exhibits a Mg/Ca range of 1.5-4.6 mmol/mol and Li/Ca about 8.0-25.3 µmol/mol with a variation factor of 1.48-2.4 and 1.68-1.8 (Raddatz et al., 2013; Rollion-Bard and Blamart, 2015 and Schleinkofer et al., 2019). Balanophyllia reveals Mg/Ca variations of 1.2-2.8 mmol/mol and 6.0-13.5 µmol/mol Li/Ca, with a variation factor over 2 on both E/Ca (Case et al., 2010). The Mg/Ca is about 2.0-3.7 mmol/mol and Li/Ca is 7.7-13.8 µmol/mol in the internal coral structure of D. cristagalli, the variation factor for both ratios is 1.8 (Rollion-Bard and Blamart, 2015). Recorded Mg/Ca in D. dianthus indicate a range of 0.9-4.0 mmol/mol and Li/Ca of 6.0-15.0 µmol/mol, with a variation factor of 2-3 and 2 (Gagnon et al., 2007; Case et al., 2010), which is higher than in S. variabilis.
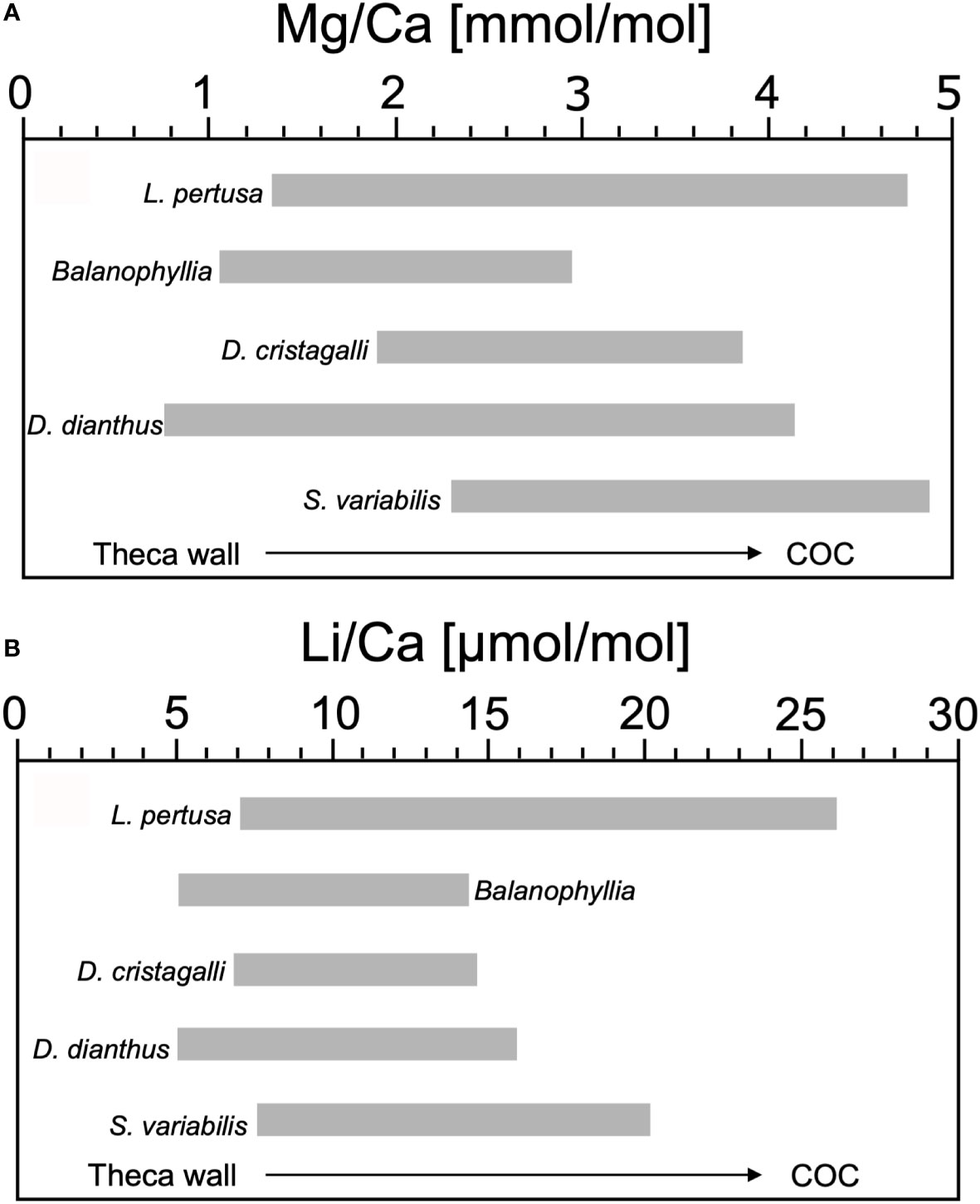
Figure 7 Comparison of the Mg/Ca [mmol/mol] and Li/Ca [µmol/mol] in the internal skeleton structure of different CWC’s: L. pertusa, Balanophyllia, D. cristagalli, D. dianthus and S. variabilis. (A) In L. pertusa, Mg/Ca vary in a range of 1.5-4.6 mmol/mol (Raddatz et al., 2013; Rollion-Bard and Blamart, 2015; Schleinkofer et al., 2019), in Balanophyllia 1.2-2.8 mmol/mol (Case et al., 2010), in D. cristagalli 2.0-3.7 mmol/mol (Rollion-Bard and Blamart, 2015), in D. dianthus the range of values extends of 0.9-4.0 mmol/mol (Gagnon et al., 2007; Case et al., 2010), and in S. variabilis between 2.4-4.7mmol/mol (this study). (B) Li/Ca range from 8.0-25.3 μmol/mol in L. pertusa (Raddatz et al., 2013; Rollion-Bard and Blamart, 2015), 6.0-13.5 μmol/mol in Balanophyllia (Case et al., 2010), in D. cristagalli 7.7-13.8 μmol/mol (Rollion-Bard and Blamart, 2015), in D. dianthus 6.0-15.0 μmol/mol (Case et al., 2010), and in S. variabilis 8.5-19.3 μmol/mol (this study).
The internal correlation of the Mg/Ca and Li/Ca ratios is apparent in all measurements, especially in sample D (Figure 6D). These internal variations are suspected to be induced by another factor than temperature. Based on the positive correlation between Mg/Ca and Li/Ca (Figure 8) (R2 = 0.48; P =1.66*10-8), it is most likely that both ratios are controlled by similar mechanism (Case et al., 2010; Raddatz et al., 2013) and diagenetic overprints appear to be unlikely. The increase in element ratios from the COC to the outer part of theca wall in sample D (Figure 6) can be explained by the occurrence of several COC-like structures within the fibrous aragonite (shown in Figure 2C). Hence, the internal fluctuations in the remaining samples can also be explained by the presence of COC-like structures.
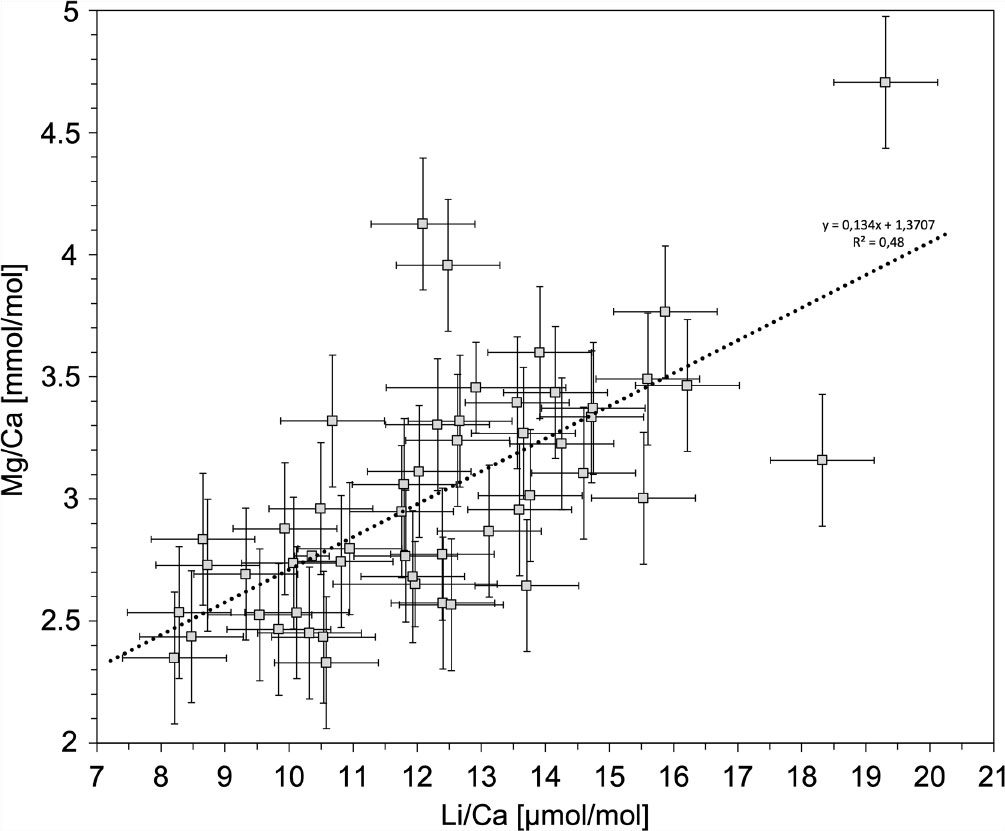
Figure 8 All samples exhibit a correlation of Mg/Ca and Li/Ca in S. variabilis, which is shown by the dotted line. Uncertainties are indicated in 2SD.
Finally, S. variabilis exhibits the same fluctuation in E/Ca ratios as other scleractinian corals that are likely induced by vital effects. We assume here that Li/Ca and Mg/Ca in S. variabilis also mainly follows the Rayleigh-fractionation model, as this is the primary model in warm, temperate and cold-water corals (Montagna et al., 2014). However, the variation factor is still smaller compared to other CWC’s, possibly due to the slow growth rate of S. variabilis (Fallon et al., 2014; Gammon et al., 2018). The E/Ca ratios of S. variabilis are most consistent with measured E/Ca ratios of D. pertusum in the North Atlantic, Mediteranean Sea and Red Sea (Figure 8; Raddatz et al., 2013; Rollion-Bard and Blamart, 2015; Schleinkofer et al., 2019). S. variabilis growth rate is about 0.8-1.25 mm/yr in natural habitats (Fallon et al., 2014) and in cultivation experiments about 0.5-3.968 mm/yr (Gammon et al., 2018) with a similar growth rate as D. pertusum about 2.44-3.77 mm/yr in the Gulf of Mexico (Brooke and Young, 2009). The gradual growth rate of S. variabilis could result in an increased variability of the element concentrations and formation of COC-like structures due to the reflection of longer time scales of elemental incorporation.
The large internal fluctuation within the coral structure represents an inaccuracy in the proxy application. Here we used bulk samples for solution-based chemistry, which might contain COC-like structures and could affect the resulting Li/Mg. However, Mg/Ca and Li/Ca show larger fluctuations, which are already reduced by using Li/Mg. To avoid a possible bias, alternative sampling strategies can be applied. Since COC-like structures can be visually identified in the skeleton of the coral, they could be excluded if sampled by a micromill. Another approach are in situ techniques using laser ICP-MS, EPMA (electron probe microanalyzer) or NanoSIMS (secondary ion mass spectrometry) to directly detect and reject variations induced by the COC or COC-like structures. High Mg/Ca ratios could be caused by high organic material content in the COC/COC-like structures as shown in other scleractinian corals (Cuif et al., 2003, Stolarski, 2003).
4 Conclusion
This study provides the first application of using S. variabilis as a geochemical archive using the Li/Mg-T paleothermometer. Our analysis of live collected samples from the South Pacific Region of New Zealand fit in the existing Li/Mg-Temperature calibrations, indicating that a species-specific calibration is not needed.
However, the reconstructed Li/Mg bases paleotemperatures of intermediate water masses from the fossil S. variabilis coral from sediment cores exhibit relatively large temperature fluctuations of up to 15°C in the AAIW off Brazil. We propose that such high temperature fluctuations can be, to a certain degree, related to intermediate water mass warming during Heinrich Stadials, but are possibly also related to change in the aragonite saturation state.
Nevertheless, the internal variation of Li/Ca and Mg/Ca in the coral microstructure is consistent with previous studies of e.g. Case et al. (2010); Raddatz et al. (2013) and Schleinkofer et al. (2019). Compared to other scleractinian CWCs, the variation factor is assumed to be related to the slow growth rate.
For more accurate reconstructions of temperature future studied should investigate other secondary factors, such as pH, aragonite saturation and salinity that might influence the Li and Mg incorporation in S. variabilis. We encourage further investigation on cold-water corals for their suitability as archives for their use in multiproxy studies. Especially with regard to studies on climate change, it is important to understand and recognize to which environmental conditions different species can adapt to and which future changes in their marine environment they can cope with.
Data availability statement
The raw data supporting the conclusions of this article will be made available by the authors, without undue reservation.
Author contributions
ES, SN and RJ contributed to conception and design of the study. ES, SA and RJ organized the database. ES wrote the first draft of the manuscript. SN, RJ, TD, FN wrote sections of the manuscript. RJ cruise participation. All authors contributed to manuscript revision, read, and approved the submitted version.
Acknowledgments
We thank the captain, crew and the scientific part of R/V Meteor cruise M125. The S. variabilis specimens off New Zealand were collected by NIWA staff as part of their various Ministry of Business, Innovation and Employment funded research programmes on R/V Tangaroa and by Fisheries New Zealand Observers. JR acknowledges funding from the Focus Track A/B programme by the Goethe University Frankfurt. This is FIERCE contribution No. 101.
Conflict of interest
The authors declare that the research was conducted in the absence of any commercial or financial relationships that could be construed as a potential conflict of interest.
Publisher’s note
All claims expressed in this article are solely those of the authors and do not necessarily represent those of their affiliated organizations, or those of the publisher, the editors and the reviewers. Any product that may be evaluated in this article, or claim that may be made by its manufacturer, is not guaranteed or endorsed by the publisher.
References
Adkins J. F., Boyle E. A., Curry W. B., Lutringer A. (2003). Stable isotopes in deep-sea corals and a new mechanism for “vital effects”. In: Geochimica Cosmochimica Acta 67 (6), 1129–1143. doi: 10.1016/S0016-7037(02)01203-6
Adkins J. F., Cheng H., Boyle E. A., Druffel E. R. M., Edwards R. L. (1998). Deep-Sea coral evidence for rapid change in ventilation of the deep north atlantic 15,400 years ago. Sci. (New York N.Y.) 280 (5364), 725–728. doi: 10.1126/science.280.5364.725
Allen K. A., Sikes E. L., Hönisch B., Elmore A. C., Guilderson T. P., Rosenthal Y., et al. (2015). Southwest pacific deep water carbonate chemistry linked to high southern latitude climate and atmospheric CO2 during the last glacial termination. Quaternary Sci. Rev. 122, 180–191. doi: 10.1016/j.quascirev.2015.05.007
Anagnostou E., Huang K.-F., You C.-F., Sikes E. L., Sherrell R. M. (2012). Evaluation of boron isotope ratio as a pH proxy in the deep sea coral desmophyllum dianthus: Evidence of physiological pH adjustment. in. Earth Planetary Sci. Lett. 349-350, 251–260. doi: 10.1016/j.epsl.2012.07.006
Anagnostou E., Sherrell R. M., Gagnon A., LaVigne M., Field M.P., McDonough W. F. (2011). Seawater nutrient and carbonate ion concentrations recorded as P/Ca, Ba/Ca, and U/Ca in the deep-sea coral desmophyllum dianthus. Geochimica Cosmochimica Acta 75 (9), 2529–2543. doi: 10.1016/j.gca.2011.02.019
Antonov J. I., Seidov D., Boyer T. P., Locarnini R. A., Mishonov A. V., Garcia H. E., et al. (2009). World ocean atlas 2009, Vol. Volume 2. 184 pp.
Archer D., Winguth A., Lea D., Mahowald N (2000). What caused the glacial/interglacial atmospheric pCO2 cycles? Reviews of Geophysics 38 (2), 159–89. doi: 10.1029/1999RG000066
Augustin L., Barbante C., Barnes P. R.F., Barnola J. M., Bigler M., Castellano E., et al. (2004). Eight glacial cycles from an Antarctic ice core. Nature 429 (6992), 623–628. doi: 10.1038/nature02599
Bahr A., Spadano Albuquerque A. L., Ardenghi N., Batenburg S., Bayer M., et al. (2016). South American hydrological balance and paleoceanography during the late pleistocene and Holocene (SAMBA) - cruise no. M125 - march 21 - April 15, 2016 - Rio de Janeiro (Brazil) - fortaleza (Brazil) (Unter Mitarbeit von TIB - Technische Informationsbibliothek Universitätsbibliothek Hannover and Alfred-Wegener-Institut für Polar- und Meeresforschung).
Bahr A., Doubrawa M., Titschack J., Austermann G., Koutsodendris A., Nürnberg D, et al (2020). Monsoonal forcing of cold-water coral growth off southeastern Brazil during the past 160 kyr. Biogeosciences 17 (23), 5883–5908. doi: 10.5194/bg-17-5883-2020
Barnola J. M., Raynaud D., Korotkevich Y. S., Lorius C. (1987). Vostok ice core provides 160,000-year record of atmospheric CO2. Nature 329 (6138), 408–414. doi: 10.1038/329408a0
Bentov S., Erez J. (2006). Impact of biomineralization processes on the mg content of foraminiferal shells: A biological perspective. Geochem. Geophys. Geosyst. 7 (1). doi: 10.1029/2005GC001015
Blamart D., Rollion-Bard C., Meibom A., Cuif J.-P., Juillet-Leclerc A., Dauphin Y. (2007). Correlation of boron isotopic composition with ultrastructure in the deep-sea coral lophelia pertusa: Implications for biomineralization and paleo-pH. Geochem. Geophys. Geosyst. 8 (12). doi: 10.1029/2007GC001686
Böhm E., Lippold J., Gutjahr M., Frank M., Blaser P., Antz B., et al. (2015). Strong and deep Atlantic meridional overturning circulation during the last glacial cycle. Nature 517 (7532), 73–76. doi: 10.1038/nature14059
Bostock H. C., Tracey D. M., Currie K. I., Dunbar G. B., Handler M. R., Mikaloff Fletcher S. E., et al. (2015). : The carbonate mineralogy and distribution of habitat-forming deep-sea corals in the southwest pacific region. Deep Sea Res. Part I: Oceanogr. Res. Papers 100, 88–104. doi: 10.1016/j.dsr.2015.02.008
Broecker W. S. (1998). Paleocean circulation during the last deglaciation: A bipolar seesaw? Paleoceanography. 13(2), 119–121. doi: 10.1029/97pa03707
Brooke S., Young C.M (2009). In situ measurement of survival and growth of Lophelia pertusa in the northern Gulf of Mexico. Marine Ecology Progress Series 397, 153–61. doi: 10.3354/meps08344
Bryan S. P., Marchitto T. M. (2008). Mg/Ca-temperature proxy in benthic foraminifera: New calibrations from the Florida straits and a hypothesis regarding Mg/Li. Paleoceanography 23 (2), n/a–n/a. doi: 10.1029/2007PA001553
Burke A., Robinson L. (2012). The southern ocean's role in carbon exchange during the last deglaciation. Science 335, 557–561. doi: 10.1126/science.1208163
Case D. H., Robinson L. F., Auro M. E., Gagnon A. C. (2010). Environmental and biological controls on mg and Li in deep-sea scleractinian corals. Earth Planetary Sci. Lett. 300 (3-4), 215–225. doi: 10.1016/j.epsl.2010.09.029
Charles C. D., Lynch-Stieglitz J., Ninnemann U. S., Fairbanks R. G. (1996). Climate connections between the hemisphere revealed by deep sea sediment core/ice core correlations. in. Earth Planetary Sci. Lett. 142 (1-2), 19–27. doi: 10.1016/0012-821X(96)00083-0
Cheng H., Adkins J., Edwards R.L., Boyle E. A. (2000). U-Th Dating of deep-sea corals. Geochimica Cosmochimica Acta 64 (14), 2401–2416. doi: 10.1016/S0016-7037(99)00422-6
Clark M. R., Rowden A. A. (2009). Effect of deepwater trawling on the macro-invertebrate assemblages of seamounts on the chatham rise, new Zealand. Deep Sea Res. Part I: Oceanogr. Res. Papers 56 (9), 1540–1554. doi: 10.1016/j.dsr.2009.04.015
Cohen A. L., Gaetani G. A., Lundälv T., Corliss B. H., George R. Y. (2006). Compositional variability in a cold-water scleractinian, lophelia pertusa: New insights into “vital effects”. Geochem. Geophys. Geosyst. 7 (12). doi: 10.1029/2006GC001354
Constantz B. R. (1989). “Skeletal organization in Caribbean acropora spp. (Lamarck),” in Rex E. crick (Hg.): Origin, evolution, and modern aspects of biomineralization in plants and animals (Boston, MA: Springer US), 175–199
Cuif J. P., Dauphin Y., Doucet J., Salome M., Susini J. (2003). XANES mapping of organic sulfate in three scleractinian coral skeletons. Geochimica et Cosmochimica Acta 67(1), 75–83. doi: 10.1016/S0016-7037(02)01041-4
Cuny-Guirriec K., Douville E., Reynaud S., Allemand D., Bordier L., Canesi M., et al. (2019). Coral Li/Mg thermometry: Caveats and constraints. Chem. Geol. 523, 162–178. doi: 10.1016/j.chemgeo.2019.03.038
Davies A. J., Guinotte J. M. (2011). Global habitat suitability for framework-forming cold-water corals. PloS One 6 (4), e18483. doi: 10.1371/journal.pone.0018483
Doney S. C., Fabry V. J., Feely R. A., Kleypas J. A. (2009). Ocean acidification: the other CO2 problem. Annu. Rev. Mar. Sci. 1, 169–192. doi: 10.1146/annurev.marine.010908.163834
Fallon S. J., Thresher R. E., Adkins J. (2014). Age and growth of the cold-water scleractinian solenosmilia variabilis and its reef on SW pacific seamounts. Coral Reefs 33 (1), 31–38. doi: 10.1007/s00338-013-1097-y
Flögel S., Dullo W.-C., Pfannkuche O., Kiriakoulakis K., Rüggeberg A. (2014). Geochemical and physical constraints for the occurrence of living cold-water corals. Deep Sea Res. Part II: Topical Stud. Oceanogr. 99, 19–26. doi: 10.1016/j.dsr2.2013.06.006
Fowell S. E., Sandford K., Stewart J. A., Castillo K. D., Ries J. B., Foster G. L. (2016). Intrareef variations in Li/Mg and Sr/Ca sea surface temperature proxies in the Caribbean reef-building coral siderastrea siderea. Paleoceanography 31 (10), 1315–1329. doi: 10.1002/2016PA002968
Frank N., Freiwald A., Correa M. L., Wienberg C., Eisele M., Hebbeln D., et al. (2011). Northeastern Atlantic cold-water coral reefs and climate. in. Geology 39 (8), 743–746. doi: 10.1130/G31825.1
Freiwald A., Fosså J., Grehan A., Koslow T., Roberts J. (2004). Cold-water coral reefs: Out of sight – no longer out of mind.
Freiwald A., Rogers A., Hall-Spencer J., Guinotte J. M., Davies A. J., Yesson C., et al. (2021). Global distribution of cold-water corals.
Gagnon A. C., Adkins J. F., Fernandez D. P., Robinson L. F. (2007). Sr/Ca and Mg/Ca vital effects correlated with skeletal architecture in a scleractinian deep-sea coral and the role of Rayleigh fractionation. Earth Planetary Sci. Lett. 261 (1-2), 280–295. doi: 10.1016/j.epsl.2007.07.013
Gammon M. J., Tracey D. M., Marriott P. M., Cummings V. J., Davy S. K. (2018). The physiological response of the deep-sea coral solenosmilia variabilis to ocean acidification. PeerJ 6, e5236. doi: 10.7717/peerj.5236
Gerhardt S., Groth H., Rühlemann C., Henrich R. (2000). Aragonite preservation in late quaternary sediment cores on the Brazilian continental slope: implications for intermediate water circulation. Int. Journ Earth Sci. 88, 607–618. doi: 10.1007/s005310050291
Gerhardt S., Henrich R. (2001). Shell preservation of Limacina inflata (Pteropoda) in surface sediments from the central and south Atlantic ocean: A new proxy to determine the aragonite saturation state of water masses. Deep Sea Res. Part I: Oceanogr. Res. Papers 48, 2051–2071. doi: 10.1016/S0967-0637(01)00005-X
Gladfeiter E. H. (1982). Skeletal development in acropora cervicornis: I. patterns of calcium carbonate accretion in the axial corallite. Coral Reefs 1 (1), 45–51. doi: 10.1007/BF00286539
Gladfelter E. H. (2007). Skeletal development in acropora palmata (Lamarck 1816): a scanning electron microscope (SEM) comparison demonstrating similar mechanisms of skeletal extension in axial versus encrusting growth. Coral Reefs 26 (4), 883–892. doi: 10.1007/s00338-007-0278-y
Grant K. M., Rohling E. J., Bar-Matthews M., Ayalon A., Medina-Elizalde M., Ramsey C.B., et al. (2012). Rapid coupling between ice volume and polar temperature over the past 150,000 years. Nature 491 (7426), 744–747. doi: 10.1038/nature11593
Gray W. R., Evans D. (2019). Nonthermal influences on Mg/Ca in planktonic foraminifera: A review of culture studies and application to the last glacial maximum. Paleoceanography Paleoclimatology 34 (3), 306–315. doi: 10.1029/2018PA003517
Gray W. R., Weldeab S., Lea D. W., Rosenthal Y., Gruber N., et al. (2018). The effects of temperature, salinity, and the carbonate system on Mg/Ca in globigerinoides ruber (white): A global sediment trap calibration. Earth Planetary Sci. Lett. 482, 607–620. doi: 10.1016/j.epsl.2017.11.026
Guihen D., White M., Lundälv T. (2012). Temperature shocks and ecological implications at a cold-water coral reef. Mar. Biodivers. Rec. 5. doi: 10.1017/S1755267212000413
Guinotte J. M., Orr J., Cairns S., Freiwald A., Morgan L., George R. (2006). Will human-induced changes in seawater chemistry alter the distribution of deep-sea scleractinian corals? in. Front. Ecol. Environ. 4 (3), 141–146. doi: 10.1890/1540-9295(2006)004[0141:WHCISC]2.0.CO;2
Hathorne E., Gagnon A., Felis T., Adkins J., Asami R., Boer W., et al. (2013). Interlaboratory study for coral Sr/Ca and other element/Ca ratio measurements. Geochem. Geophys. Geosyst. 14 (9), 3730–3750. doi: 10.1002/ggge.20230
Hemsing F., Hsieh Y.-T., Bridgestock L., Spooner P. T., Robinson L. F., Frank N., et al. (2018). Barium isotopes in cold-water corals. Earth Planetary Sci. Lett. 491, 183–192. doi: 10.1016/j.epsl.2018.03.040
Henrich R., Baumann K. H., Gerhardt S., Gröger M., Volbers A. (2003). “Carbonate preservation in deep and intermediate water masses in the south Atlantic: Evaluation and geological record (a review),” in The south Atlantic in the late quaternary. Eds. Wefer G., Mulitza S., Ratmeyer V. (Berlin, Heidelberg: Springer). doi: 10.1007/978-3-642-18917-3_28
Holcomb M., Cohen A. L., Gabitov R. I., Hutter J.L. (2009). Compositional and morphological features of aragonite precipitated experimentally from seawater and biogenically by corals. Geochimica et Cosmochimica Acta 73, 4166–79. doi: 10.1016/j.gca.2009.04.015
Hou A., Bahr A., Schmidt S., Strebl C., Albuquerque A. L., Chiessi C. M., et al. (2020). Forcing of western tropical south Atlantic sea surface temperature across three glacial-interglacial cycles. Global Planetary Change 188, 103150. doi: 10.1016/j.gloplacha.2020.103150
Houlbrèque F., McCulloch M., Roark B., Guilderson T., Meibom A., Kimball J., et al. (2010). Uranium-series dating and growth characteristics of the deep-sea scleractinian coral: Enallopsammia rostrata from the equatorial pacific. Geochimica Cosmochimica Acta 74 (8), 2380–2395. doi: 10.1016/j.gca.2010.01.017
Indermühle A., Stocker T. F., Joos F., Fischer H., Smith H. J., Wahlen M., et al. (1999). Holocene Carbon-cycle dynamics based on CO2 trapped in ice at Taylor dome, Antarctica. Nature 398 (6723), 121–126. doi: 10.1038/18158
Leuschner D. C., Sirocko F., Grootes P. M., Erlenkeuser H. (2002). Possible influence of zoophycos bioturbation on radiocarbon dating and environmental interpretation. Mar. Micropaleontol. 46 (1-2), 111–126. doi: 10.1016/S0377-8398(02)00044-0
Locarnini R. A., Mishonov A. V., Antonov J. I., Boyer T. P., Garcia H. E., Baranova O. K., et al. (2009) World ocean atlas 2009. Available at: http://www.nodc.noaa.gov/OC5/indprod.html.
Lynch-Stieglitz J. (2017). The Atlantic meridional overturning circulation and abrupt climate change. Annu. Rev. Mar. Sci 9 (585) 83–104. doi: 10.1146/annurev-marine-010816-060415
Lynch-Stieglitz J., Valley S. G., Schmidt M. W. (2019). Temperature-dependent ocean–atmosphere equilibration of carbon isotopes in surface and intermediate waters over the deglaciation. Earth Planetary Sci. Lett. 506, 466–475. doi: 10.1016/j.epsl.2018.11.024
Lund D. C., Tessin A. C., Hoffman J. L., Schmittner A (2015). Southwest Atlantic water mass evolution during the last deglaciation. Paleoceanography 30 (5), 477–94. doi: 10.1002/2014PA002657
Mangini A., Godoy J. M., Godoy M. L., Kowsmann R., Santos G. M., Ruckelshausen M. (2010). Deep sea corals off Brazil verify a poorly ventilated southern pacific ocean during H2, H1 and the younger dryas. In: Earth Planetary Sci. Lett. 293 (3-4), 269–276. doi: 10.1016/j.epsl.2010.02.041
Marcott S., Bauska T., Buizert C., Steig E. J., Rosen J. L., Cuffey K. M., et al. (2014). Centennial-scale changes in the global carbon cycle during the last deglaciation. Nature 514, 616–619. doi: 10.1038/nature13799
Martínez-García A., Sigman D. M., Ren H., Anderson R. F., Straub M., Hodell D. A., et al. (2014). Iron fertilization of the subantarctic ocean during the last ice age. Sci. (New York N.Y.) 343 (6177), 1347–1350. doi: 10.1126/science.1246848
McCarthy G., McDonagh E., King B. (2011). Decadal variability of thermocline and intermediate waters at 24°S in the south Atlantic. J. Phys. Oceanogr. 41 (1), 157–165. doi: 10.1175/2010JPO4467.1
McCulloch M., Falter J., Trotter J., Montagna P. (2012). Coral resilience to ocean acidification and global warming through pH up-regulation. Nat. Clim Change 2 (8), 623–627. doi: 10.1038/NCLIMATE1473
Meibom A., Cuif J.-P., Houlbreque F., Mostefaoui S., Dauphin Y., Meibom K. L., et al. (2008). Compositional variations at ultra-structure length scales in coral skeleton. Geochimica Cosmochimica Acta 72 (6), 1555–1569. doi: 10.1016/j.gca.2008.01.009
Meibom A., Yurimoto H., Cuif J.-P., Domart-Coulon I., Houlbreque F., Constantz B., et al. (2006). Vital effects in coral skeletal composition display strict three-dimensional control. Geophys. Res. Lett. 33 (11). doi: 10.1029/2006GL025968
Mémery L., Arhan M., Álvarez-Salgado X. A., Messias M. J., Mercier H., Castro C. G, et al. (2000). The water masses along the western boundary of the south and equatorial Atlantic. Progress in Oceanography 47 (1), 69–98. doi: 10.1016/S0079-6611(00)00032-X
Mienis F., Duineveld G. C. A., Davies A. J., Lavaleye M. M. S., Ross S. W., Seim H., et al. (2014). Cold-water coral growth under extreme environmental conditions, the cape lookout area, NW Atlantic. Biogeosciences 11 (9), 2543–2560. doi: 10.5194/bg-11-2543-2014
Montagna P., McCulloch M., Douville E., López Correa M., Trotter J., Rodolfo-Metalpa R., et al. (2014). Li/Mg systematics in scleractinian corals: Calibration of the thermometer. Geochimica Cosmochimica Acta 132, 288–310. doi: 10.1016/j.gca.2014.02.005
Montagna P., McCulloch M., Taviani M., Mazzoli C., Vendrell B. (2006). Phosphorus in cold-water corals as a proxy for seawater nutrient chemistry. Sci. (New York N.Y.) 312 (5781), 1788–1791. doi: 10.1126/science.1125781
Montero-Serrano J. C., Frank N., Tisnerat-Laborde N., Colin C., Wu C. C., Lin K, et al (2013). Decadal changes in the mid-depth water mass dynamic of the Northeastern Atlantic margin (Bay of Biscay. Earth and Planetary Science Letters 364), 134–44. doi: 10.1016/j.epsl.2013.01.012
Mucci A. (1983). The solubility of calcite and aragonite in seawater at various salinities, temperatures, and one atmosphere total pressure. Am. J. Sci. 283 (7), 780–799. doi: 10.2475/ajs.283.7.780
Petit J. R., Jouzel J., Raynaud D., Barkov N. I., Barnola J.-M., Basile I., et al. (1999). Climate and atmospheric history of the past 420,000 years from the vostok ice core, Antarctica. Nature 399 (6735), 429–436. doi: 10.1038/20859
Poggemann D.-W., Hathorne E., Nürnberg D., Frank M., Bruhn I., Reißig S., et al. (2017). Rapid deglacial injection of nutrients into the tropical Atlantic via Antarctic intermediate water. Earth Planetary Sci. Lett. 463, 118–126. doi: 10.1016/j.epsl.2017.01.030
Poggemann D.-W., Nürnberg D., Hathorne E. C., Frank M., Rath W., Reißig S., et al. (2018). Deglacial heat uptake by the southern ocean and rapid northward redistribution Via Antarctic intermediate water. Paleoceanography Paleoclimatology 33 (11), 1292–1305. doi: 10.1029/2017PA003284
Raddatz J., Liebetrau V., Rüggeberg A., Hathorne E., Krabbenhöft A., Eisenhauer A., et al. (2013). Stable Sr-isotope, Sr/Ca, Mg/Ca, Li/Ca and Mg/Li ratios in the scleractinian cold-water coral lophelia pertusa. Chem. Geol. 352, 143–152. doi: 10.1016/j.chemgeo.2013.06.013
Raddatz J., Liebetrau V., Trotter J., Rüggeberg A., Flögel S., Dullo W.-C., et al. (2016). Environmental constraints on Holocene cold-water coral reef growth off Norway: Insights from a multiproxy approach. Paleoceanography 31 (10), 1350–1367. doi: 10.1002/2016PA002974
Raddatz J., Nürnberg D., Tiedemann R., Rippert N. (2017). Southeastern marginal West pacific warm pool sea-surface and thermocline dynamics during the pleistocene (2.5–0.5 ma). Palaeogeography Palaeoclimatology Palaeoecol. 471, 144–156. doi: 10.1016/j.palaeo.2017.01.024
Raddatz J., Rüggeberg A. (2021). Constraining past environmental changes of cold-water coral mounds with geochemical proxies in corals and foraminifera. Depositional Rec 7 (2), 200–222. doi: 10.1002/dep2.98
Raddatz J., Rüggeberg A., Flögel S., Hathorne E. C., Liebetrau V., Eisenhauer A., et al. (2014a). The influence of seawater pH on U / Ca ratios in the scleractinian cold-water coral lophelia pertusa. Biogeosciences 11 (7), 1863–1871. doi: 10.5194/bg-11-1863-2014
Raddatz J., Rüggeberg A., Liebetrau V., Foubert A., Hathorne E., Fietzke J., et al. (2014b). Environmental boundary conditions of cold-water coral mound growth over the last 3 million years in the porcupine seabight, northeast Atlantic. Deep Sea Res. Part II: Topical Stud. Oceanogr. 99, 227–236. doi: 10.1016/j.dsr2.2013.06.009
Raddatz J., Titschack J., Frank N., Freiwald A., Conforti A., Osborne A., et al. (2020). Solenosmilia variabilis-bearing cold-water coral mounds off Brazil. Coral Reefs 39 (1), 69–83. doi: 10.1007/s00338-019-01882-w
Roberts J., Gottschalk J., Skinner L. C., Peck V. L., Kender S., Elderfield H., et al. (2016). Evolution of south Atlantic density and chemical stratification across the last deglaciation. Proc. Natl. Acad. Sci. United States America 113 (3), 514–519. doi: 10.1073/pnas.1511252113
Robinson L. F., Adkins J. F., Frank N., Gagnon A. C., Prouty N. G., Brendan Roark E., et al. (2014). The geochemistry of deep-sea coral skeletons: A review of vital effects and applications for palaeoceanography. Deep Sea Res. Part II: Topical Stud. Oceanogr. 99, 184–198. doi: 10.1016/j.dsr2.2013.06.005
Rollion-Bard C., Blamart D. (2015). Possible controls on Li, Na, and mg incorporation into aragonite coral skeletons. Chem. Geol. 396, 98–111. doi: 10.1016/j.chemgeo.2014.12.011
Rollion-Bard C., Blamart D., Cuif J.-P., Dauphin Y. (2010). In situ measurements of oxygen isotopic composition in deep-sea coral, lophelia pertusa: Re-examination of the current geochemical models of biomineralization. Geochimica Cosmochimica Acta 74 (4), 1338–1349. doi: 10.1016/j.gca.2009.11.011
Rosenthal Y., Field M. P., Sherrell R.M. (1999). Precise determination of element/calcium ratios in calcareous samples using sector field inductively coupled plasma mass spectrometry. Analytical chemistry 7(15), 3248–53. doi: 10.1021/ac981410x
Santos T. P., Lessa D. O., Venancio I. M., Chiessi C. M., Mulitza S., Kuhnert H., et al. (2017). Prolonged warming of the Brazil current precedes deglaciations. Earth Planetary Sci. Lett. 463, 1–12. doi: 10.1016/j.epsl.2017.01.014
Schleinkofer N., Raddatz J., Freiwald A., Evans D., Beuck L., Rüggeberg A., et al. (2019). Environmental and biological controls on Na/Ca ratios in scleractinian cold-water corals. in. Biogeosciences 16 (18), 3565–3582. doi: 10.5194/bg-16-3565-2019
Schlitzer R. (2021) Ocean data view. version 2021. Available at: http://odv.awi.de.
Schröder-Ritzrau A., Freiwald A., Mangini A. (2005). “U/Th-dating of deep-water corals from the eastern north Atlantic and the western Mediterranean Sea,” in André Freiwald and J. Murray Roberts (Hg.): Cold-Water Corals and Ecosystems. Berlin/Heidelberg: Springer-Verlag (Erlangen Earth Conference Series). 157–172.
Sigman D. M., Boyle E. A (2000). Glacial/interglacial variations in atmospheric carbon dioxide. Nature. 407 (6806), 859–69. doi: 10.1038/35038000
Sinclair D. J., Williams B., Risk M. (2006). A biological origin for climate signals in corals–trace element “vital effects” are ubiquitous in scleractinian coral skeletons. Geophys. Res. Lett. 33 (17). doi: 10.1029/2006GL027183
Spooner P. T., Robinson L. F., Hemsing F., Morris P., Stewart J. A. (2018). Extended calibration of cold-water coral Ba/Ca using multiple genera and co-located measurements of dissolved barium concentration. Chem. Geol. 499, 100–110. doi: 10.1016/j.chemgeo.2018.09.012
Stewart J. A., Robinson L. F., Day R. D., Strawson I., Burke A., Rae J. W, et al (2020). Refining trace metal temperature proxies in cold-water scleractinian and stylasterid corals. Earth and Planetary Science Letters 545, 116412. doi: 10.1016/j.epsl.2020.116412
Stolarski J (2003). Three-dimensional micro-and nanostructural characteristics of the scleractinian coral skeleton: a biocalcification proxy. Acta Palaeontologica Polonica 48 (4)
Thresher R. E., Adkins J., Fallon S. J., Gowlett-Holmes K., Althaus F., Williams A. (2011). Extraordinarily high biomass benthic community on southern ocean seamounts. Sci. Rep. 1, 119. doi: 10.1038/srep00119
Toggweiler J. R., Lea D. W. (2010). Temperature differences between the hemispheres and ice age climate variability. Paleoceanography 25 (2). doi: 10.1029/2009PA001758
Tracey D., Rowden A., Mackay K., Compton T. (2011). Habitat-forming cold-water corals show affinity for seamounts in the new Zealand region. Mar. Ecol. Prog. Series 430, 1–22. doi: 10.3354/meps09164
Wefing A.-M., Arps J., Blaser P., Wienberg C., Hebbeln D., Frank N. (2017). High precision U-series dating of scleractinian cold-water corals using an automated chromatographic U and Th extraction. Chem. Geol. 4751, 140–148. doi: 10.1016/j.chemgeo.2017.10.036
Keywords: cold water coral (CWC), south atlantic, solenosmilia variabilis, Li/Mg, intermediate water mass
Citation: Endress S, Schleinkofer N, Schmidt A, Tracey D, Frank N and Raddatz J (2022) The cold-water coral Solenosmilia variabilis as a paleoceanographic archive for the reconstruction of intermediate water mass temperature variability on the Brazilian continental margin. Front. Mar. Sci. 9:909407. doi: 10.3389/fmars.2022.909407
Received: 31 March 2022; Accepted: 04 July 2022;
Published: 02 August 2022.
Edited by:
Ravi Bhushan, Physical Research Laboratory, IndiaCopyright © 2022 Endress, Schleinkofer, Schmidt, Tracey, Frank and Raddatz. This is an open-access article distributed under the terms of the Creative Commons Attribution License (CC BY). The use, distribution or reproduction in other forums is permitted, provided the original author(s) and the copyright owner(s) are credited and that the original publication in this journal is cited, in accordance with accepted academic practice. No use, distribution or reproduction is permitted which does not comply with these terms.
*Correspondence: S. Endress, c2VyYWZpbmEuZW5kcmVzc0BnbWFpbC5jb20=