- 1Ecology and Biodiversity Research Group, Plant Biology and Nature Management, Vrije Universiteit Brussel (VUB), Brussels, Belgium
- 2Department of Biological, Chemical and Pharmaceutical Sciences and Technologies, University of Palermo, Palermo, Italy
Mediterranean salinas, originally built for salt production, function as alternative wetlands. A variety of accompanying lagoon, ditch, and marsh systems are suitable habitats for salt-tolerant submerged macrophytes and often characterized by monospecific beds of Ruppia. Traditionally, birds are considered the main dispersal vector of submerged macrophytes. However, Ruppia spiralis habitats are under marine influence and therefore interference of coastal currents in their connectivity might be expected. In this study, we aim to infer connectivity and spatial patterns from population genetic structures. Using nuclear microsatellite loci, the nuclear ribosomal cistron and chloroplast sequences, we investigated the genetic diversity, genetic structure, and demographic history of 10 R. spiralis populations along a 25-km coastal stretch of western Sicily encompassing a variety of saline habitats. We tested for local fine-scaled structures, hypotheses of regional isolation by distance, and migration directionality. Our results revealed a high degree of allele and gene diversity that was locally maintained by outcrossing. At the regional level, we detected isolation by distance and identified three genetically differentiated clusters, with a significant structure that matches an overall north-to-south unidirectional migration model. This directionality follows the main sea current, hence indicating the importance of hydrological connectivity in regional conservation management. Significant fine-scale spatial structures only emerged in some populations and were absent in the ‘salina fridda’ habitat that showed the largest clonal richness. The local site-dependent patterns emphasize a need to examine the influence of disturbances on seed recruitment and clonal growth over small distances.
Introduction
Salinas are artificial coastal pond systems that support a range of ecosystem services (Soares et al., 2018). In a changing climate and under conditions of elevated nutrients, they have become extremely important for nature conservation. Specifically, salinas are recognized for their function as alternative coastal wetlands (Walmsley, 1999; López et al., 2010). Their interconnected ponds create a high degree of spatial heterogeneity, which in turn attracts many typical wetland species (Evagelopoulos et al., 2008; Hamdi et al., 2008; Messina et al., 2012). In the Mediterranean Basin, which is located at a boundary between tropical and temperate zones, salinas even provide critical resting and feeding places for migratory birds (Britton and Johnson, 1987; Sadoul et al., 1998; López et al., 2004). On the other hand, salinas also present numerous stressful conditions such as high salinity, wind-exposed waters, and poorly oxygenated sediments. This provides a challenging habitat for most macrophytes to grow and reproduce (Mannino and Graziano, 2014).
Macrophytes are important structural elements in the water column that deliver conditions supporting high biomasses within a variety of aquatic organisms (Diehl and Kornijów, 1998). Mostly inhabiting freshwater, only a limited number of genera are able to tolerate high salinity. Among those is the genus Ruppia L., which tolerates the highest levels of salinity (Brock, 1982; Melack, 1988). Ruppia plants accumulate proline as an adaptation to saltwater environments (Brock, 1981). Species that belong to this genus form dense monospecific beds in shallow saline water bodies, providing spawning grounds for marine fish and habitat for invertebrates (Verhoeven, 1979; Verhoeven, 1980; Triest and Sierens, 2009). These beds can form both from sexual and vegetative (clonal) reproduction (Verhoeven, 1979; Gesti et al., 2005). In this regard, Ruppia shares a lot of similarities with closely related seagrasses (Les et al., 1997). In Europe, the common Ruppia spiralis L. ex Dum. [formerly named Ruppia cirrhosa (Petagna) Grande (Ito et al., 2017; den Hartog and Triest, 2020)] has the widest tolerance to salt and occurs in salinas, saltmarshes, estuaries, and coastal lakes (Verhoeven, 1979; Triest and Sierens, 2010; Triest and Sierens, 2013; Mannino et al., 2015; Triest et al., 2017). Although many studies attained knowledge on the ecology, productivity, and ecophysiology at the genus level, there has been an increasing attempt in understanding the population level variation (Triest and Symoens, 1991; Triest and Sierens, 2013; Triest and Sierens, 2014; Martínez-Garrido et al., 2017; Triest et al., 2017), in particular because of a need to solve taxonomic confusion in this highly variable genus (Green and Short, 2003; den Hartog and Kuo, 2006; den Hartog and Triest, 2020).
Ruppia species have a simple morphology with reduced vegetative and reproductive features and often display phenotypic plasticity (den Hartog and Kuo, 2006; Mannino et al., 2015). In addition, hybridization between species has been documented as well as the existence of polyploidy (Ito et al., 2010; Triest and Sierens, 2014; Triest and Sierens, 2015; Martínez-Garrido et al., 2016). This all complicates the identification of Ruppia species, leading to many misconceptions about their ecology, distribution, and conservation status (den Hartog and Kuo, 2006; Short et al., 2007; Triest and Sierens, 2009; Mannino et al., 2015). Similarly, the detection of evolutionary significant units (ESUs) is difficult because of morphologically variable populations (Triest and Symoens, 1991). For R. spiralis, a species that occurs in such a wide variety of saline habitats, it is possible that populations are adapting locally (Triest et al., 2018). Because this has implications for the conservation and management of the species, it is important to identify population dynamics and patterns of connectivity (Jackson, 2017).
Salinas are closed water bodies with a temporal connection to the sea. This isolation may affect the genetic diversity and structure of inhabiting R. spiralis populations by reducing gene flow, making populations less resilient to changing conditions (Ehlers et al., 2008; Wright et al., 2013; Triest et al., 2021a). Dispersal processes that influence connectivity are therefore essential to maintaining long-term viable R. spiralis populations. Traditionally, birds are considered the main dispersal vector of Ruppia, carrying and transporting seeds on their feet and feathers and in their gut (Ito et al., 2010; Triest and Sierens, 2013; Yu et al., 2014). Ruppia seeds have been found in bird feces and were able to germinate afterward (Figuerola et al., 2002; Charalambidou et al., 2003). Similarly, the genetic structure and the presence of Ruppia populations in inland brackish waterbodies suggest that zoochorous dispersal occurs (Triest and Sierens, 2015; Martínez-Garrido et al., 2017). However, because of the marine character of R. spiralis, interference of sea currents in their connectivity can be expected (Triest and Sierens, 2013). It has already been shown that sea currents shape the connectivity of primary R. spiralis habitats at a large geographic scale, suggesting that establishment originates from drifting propagules (Triest et al., 2017). The importance of hydrological connectivity for the persistence of R. spiralis populations within large lagoons was indicated by high relatedness between individuals over distances up to 20 km. At such small spatial scales, it can be expected that the genetic structure and detectable patterns of recent gene flow were at least partly influenced by local hydrological connections.
In this study, we aim to estimate the genetic structure and patterns of connectivity of R. spiralis populations along the western coast of Sicily between the Natural Oriented Reserve “Saline di Trapani e Paceco” and “Marsala” by analyzing microsatellite loci. More specifically, we aim to (i) analyze genetic diversity, (ii) test for spatial structures, and (iii) reveal the directionality of migration among populations to better understand the importance of different dispersal vectors at a regional scale. Species identity was verified from the nuclear ribosomal cistron and four chloroplast marker sequences. Because our study is done in the western Mediterranean Basin, which was previously identified as a diversity center of Ruppia (Mannino et al., 2015), we suspect high genetic diversity with less pronounced spatial genetic structure among hydrologically well-connected sites. Because of the sampling design in both salina environments and open estuaries, we hypothesize that sea currents along with birds play a role in determining the contemporary population structure. The outcomes of our study will help to understand how R. spiralis colonizes and persists in salinas and coastal lagoons.
Materials and methods
Study site description
The Natural Oriented Reserve “Saline di Trapani e Paceco” is located along the western coast of Sicily and covers approximately 1,000 ha. The reserve is characterized by regulated salinas that comprise a number of interconnected ponds, built for the production of salt. Nonetheless, the area is recognized as a Special Protection Area (SPA) as part of the NATURA 2000 network (SIC ITA010007). Different pond types can be distinguished by their positioning and the salinity of the water. At the beginning of the salt process, the first pond receives water directly from the sea or via a channel connected to the sea. This pond, referred to as ‘salina fridda’, has a salinity that is similar or slightly higher than that of seawater. In later phases, water progressively flows to the next ponds, from where it will start to evaporate and salinity levels will increase. Ruppia spiralis could be collected from the first two kinds of ponds in the salt process (‘salina fridda’ and ‘salina caure d’acqua frisca’) and the accompanying channels.
Along the coast, sea currents follow a seasonal pattern. Throughout the summer, the reserve is subject to the Tyrrhenian Sicilian Current that directs relatively high saline water from north to south (Thompson et al., 2016; Menna et al., 2019). Because of the irregular shape of the coastline, different areas experience this current at different velocities. As such, the zone between the mainland and Isola Grande becomes almost completely sheltered, forming coastal lagoons at shallow depths (Figure 1). Here, additional populations of R. spiralis were sampled.
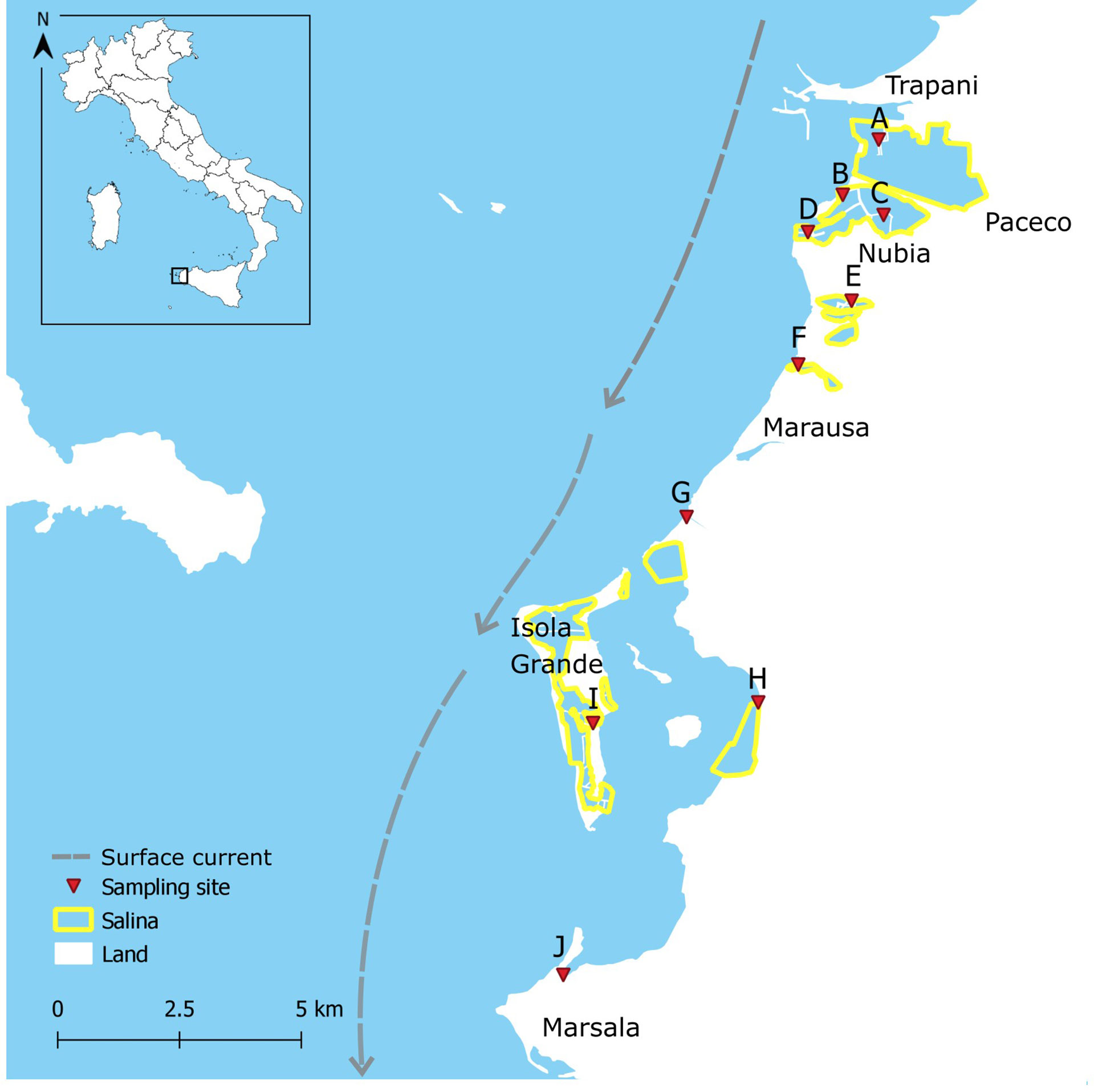
Figure 1 Map of the anylyzed western coast of Sicily with location of sampled Ruppia spiralis populations (indicated with red triangles). Dashed lines are the main surface currents.
Plant material
Ruppia spiralis plants were collected in 10 water bodies (identified by a code from A to J) during the first week of August 2020 (Table 1). At each locality, 16 to 30 individual shoots were collected following a 30-m linear transect. For sites with a low density of stands, the linear transect was extended until 30 individual shoots were collected. For population C, we sampled without transect as stands were so small and scattered. Each plant sample was individually labeled and dried, and for each population, a reference herbarium was deposited at BRVU (Herbarium of Vrije Universiteit Brussel, formally transferred in 2018 to BR, Botanic Garden, Meise, Belgium).
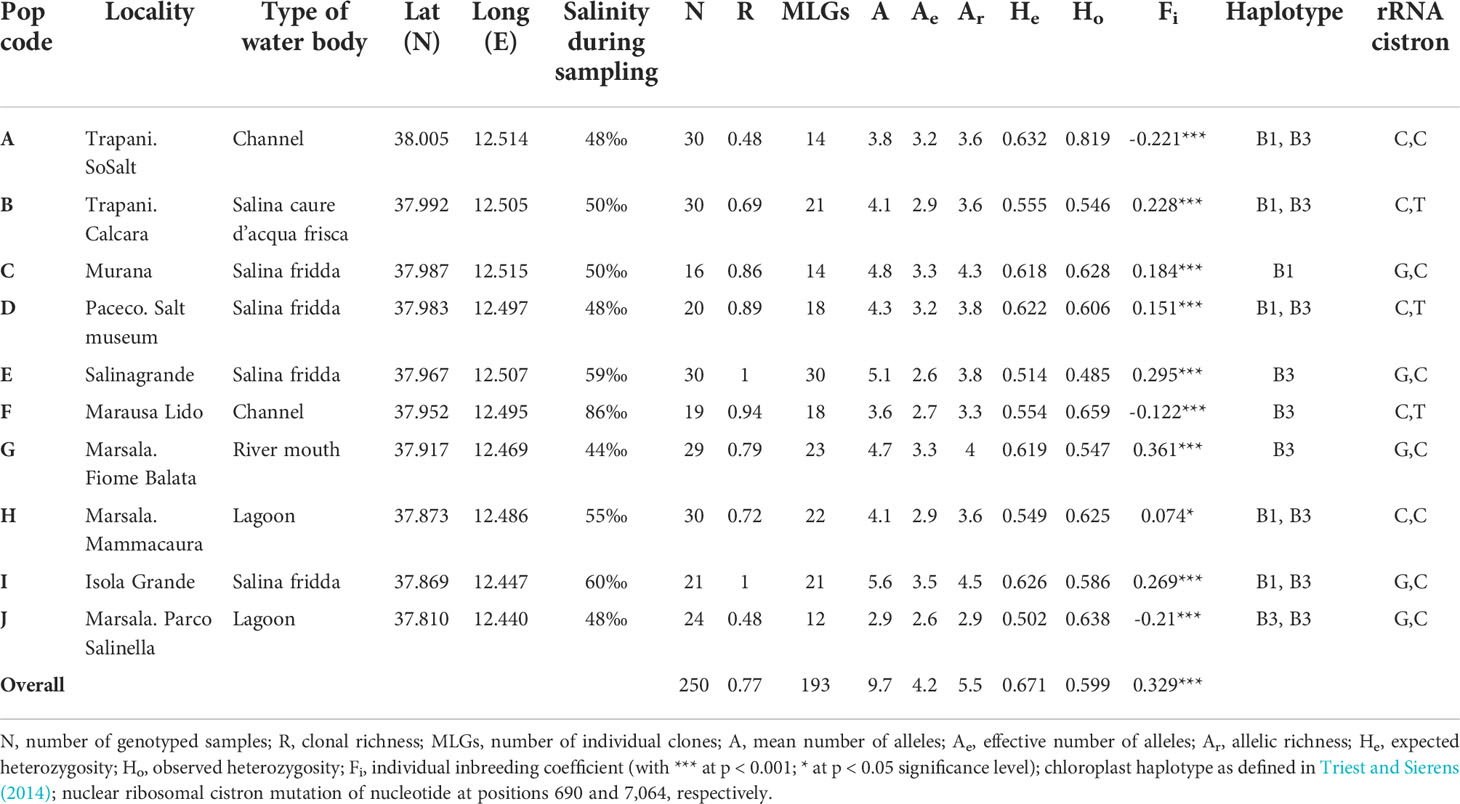
Table 1 Location details and population genetic variables of Ruppia spiralis in 10 Sicilian water bodies.
DNA extraction, sequencing, and microsatellite amplification
Genomic DNA was isolated from dry plant material using the E.Z.N.A. SP Plant DNA Mini Kit (Omega Bio‐tek, Norcross, GA, USA). Polymerase chain reaction (PCR) was used to amplify 15 microsatellite loci (Supplementary Table S1). For the total reaction mixture, 2.5 μl genomic DNA was combined with 1.25 μl primer mix (forward and reversed fluorescent labeled primers), 6.25 μl QIAGEN Multiplex PCR Master Mix, and 2.5 μl H2O. The PCR reactions were performed in a thermal cycler (Bio-Rad T100) as follows: 95°C for 15 min, 35 cycles of 95°C for 30 s (denaturation), 57°C for 1 min 30 s (annealing), 72°C for 40 s (elongation), and a final extension step of 60°C for 30 min. PCR products were run on an ABI3730XL sequencer (Macrogen, Seoul, Korea), and fragments were analyzed with GeneMarker V2.6.0 (SoftGenetics LLC®). We encountered 10 ramets with five alleles for one locus during scoring. To allow data analyses as a tetraploid taxon, the least common allele was omitted from those few cases.
To detect haplotype variation and rule out any different species identity, one sample of each population was used for a comparative analysis of chloroplast sequences. After passing quality inspection (DNA concentration between 5 and 15 ng/µl), the constructed library (TruSeq Nano DNA Kit) was sequenced by 300-bp × 2 paired-end sequencing in an Illumina MiSeq platform (Macrogen, Seoul, South Korea). Raw data were used for de novo assembling of several genes/introns from a nearly complete chloroplast genome. All assemblies used GenBank accession MN233650 of Ruppia sinensis Yu and den Hartog (Yu et al., 2019) as a reference genome (similar method as in Triest et al., 2021b), and the mapping of reads of Sicilian samples was performed in Geneious Prime v 2019.2.1 (©2005–2019 Biomatters Ltd.) (https://www.geneious.com). The assemblages averaged 12,913–57,881 reads with a mean depth of reads ranging from 24 to 109 coverage. The de novo targeted chloroplast assemblies were processed against R. spiralis sequences (under R. cirrhosa in GenBank) of the intergenic spacer trnH-psbA (JN113267; 452 bp) and three mononucleotide microsatellite regions, ccmp2 (JN113250; 202 bp), ccmp3 (JN113258; 157 bp), and ccmp10 (JN113260; 166 bp), representing haplotype B from the Mediterranean as defined by Triest and Sierens (2014). The consensus sequences were aligned with ClustalW (Thompson et al., 1994). Lastly, chloroplast microsatellite regions that showed variation among populations were amplified for individuals, to check if haplotypes occur homogeneously within a population.
The nuclear ribosomal cistron (18S, ITS1, 5.8S, ITS2, and 26S) was assembled from a Ruppia spiralis sequence of a partial nuclear ribosomal cistron (GenBank JQ937105; submitted under Ruppia maritima) containing an internal transcribed spacer 1 (partial sequence), 5.8S ribosomal RNA gene (complete sequence), and internal transcribed spacer 2 (partial sequence) and was subsequently used as a seed in Geneious Prime v 2019.2.1 to progressively enlarge the flanking regions. A base pair of 6,545 was obtained for one selected sample and further used as a reference to map every other sample, averaging 11,570–20,436 reads, with a mean depth of reads ranging from 380 to 652 coverage. The generated consensus sequences of 7,338-bp length of 10 samples (same as used for chloroplast sequences) were aligned for a comparative description of mutated positions using MAFFT v7.388 (Katoh et al., 2002; Katoh and Standley, 2013).
Population genetic variables
Prior to all analyses, the package Poppr in R v1.2.5001 (Kamvar et al., 2014; Kamvar et al., 2015; R Core Team, 2019) was used to detect repeated multilocus genotypes (MLGs) within populations. Because repeated MLGs are likely the result of clonal growth, only one representative sample per site was retained. For the calculation of population genetic variables, the software of SPAGeDi v1.5 was used, which is suitable for tetraploids (Hardy and Vekemans, 2002; Vekemans and Hardy, 2004). At each site, the number of alleles (A), effective number of alleles (Ae), allelic richness (Ar), observed heterozygosity (Ho), expected heterozygosity (He), and individual inbreeding coefficient (Fi) were calculated. For comparison purposes, allelic richness was estimated for subsamples of the smallest sample size (Ar at k = 22). Clonal richness (R) was calculated with the formula R = (G−1)/(N−1) (Dorken and Eckert, 2001).
Spatial structures
To detect isolation by distance (IBD) patterns, a one-sided Mantel test was performed on a matrix of the pairwise genotypic differentiation (pairwise Fst) between populations and a matrix of pairwise geographical distances, randomizing with 1,000 permutations using SPAGeDi v1.5. This test is based on an analysis of variance (ANOVA) and was performed separately for two different geographic distance matrices. The first included Euclidean distances between populations, the second was based on calculated geographic distances between populations following hydrological connections in salinas or coastal water flow. This was complemented with a more detailed ANOVA at the population level using a similar pairwise comparison and 1,000 permutations but dividing distance among four classes. At first, distance classes were defined by obtaining an equal number of pairwise comparisons of populations (3.42–7.79–12.88–22.58 km). Next, distance classes (4–9–14–25 km) were proposed based on the population’s respective distances from each other. The variance of the pairwise kinship coefficient (Fij) (Loiselle et al., 1995) for all populations was in the same way calculated over complete distance and divided among distance classes. The genetic structure among populations (Fst), inbreeding within populations (Fis), and overall inbreeding (Fit) were estimated with ANOVA, and significance was tested with 1,000 random permutations. To identify fine-scale spatial genetic structures (FSGS), i.e., the non-random spatial distribution of genotypes (Vekemans and Hardy, 2004), pairwise kinship coefficient Fij (Loiselle et al., 1995) was assessed between genets at the transect level. Population C was omitted from this analysis because the low coverage and fragmented distribution did not allow sufficient pairwise comparisons of individual genets within given distance classes to obtain statistical support. Pairwise kinship within sites collectively and individually was analyzed (one-sided ANOVA, 1,000 permutations) using the whole sample as a reference for allele frequencies. Five distance classes were tested, defined by an equal number of pairs (4–8–12.5–19–100 m) or based on genetic structures that have previously been found in seagrasses (1–5–10–15–100 m) (Orth et al., 1994; Phan et al., 2017; Dierick et al., 2021).
Population structure and clustering
Patterns of population genetic structure, such as groups with genetically related individuals, can be visualized in clusters. Hence, a principal component analysis (PCA) was performed at the individual level using the R package ade4 (Dray and Dufour, 2007; R Core Team, 2019). Because PCA focuses on loci containing the most variation and we wanted to maximize separability between groups, complementary discriminant analysis of principal components (DAPCs) was computed using the Adegenet package in R (Jombart, 2008; R Core Team, 2019). Both are acceptable multivariate methods to analyze genetic patterns; however, the latter is designed to identify complex genetic structures in populations (Jombart et al., 2010). Using DAPC, genetic structure was inferred from 40 principal components and populations were partitioned into clusters (K = 3). Thereafter, we calculated the genetic differentiation (ρST) among populations and clusters using an analysis of molecular variance (AMOVA) with the software GenoDive 3.05 (Meirmans and van Tienderen, 2004; Meirmans and Liu, 2018).
Gene flow
To interpret the observed genetic variation within populations, potential patterns of historical gene flow were investigated. Therefore, coalescent models were run using Migrate-n (Beerli et al., 2019) with two sets of populations based on the DAPC results. First, gene flow directionalities were explored between populations D and F, which belong to the same cluster. In the second analysis, the remaining eight populations were partitioned into a northern and southern group. Because Migrate-n is designed for diploid species (2×) and R. spiralis is polyploid (4×), our datasets first had to be restructured. As suggested by Beerli (2012), alleles of one individual were redistributed into two new diploid individuals, making sure to maximize heterozygosity and minimize effects on allele frequencies. While deduplicating is evident for diploid and tetraploid loci, it brings the challenge of determining which allele has to be duplicated from loci with three alleles. We rewrote them as two diploids using the allelic dosages. For instance, if a locus had three alleles (ABC) and we could infer which allele had the highest allelic dosage (e.g., B), this allele was duplicated (AB and BC). To analyze allelic dosages, GeneMarker V2.6.0 (SoftGenetics LLC®) was used. When the allelic dosages were unclear, the allele with the highest frequency for this population was duplicated. To ensure consistency with the original data, chi-square tests were run on every locus for each group separately (Supplementary Table S2). In addition, individuals containing missing allele values were discarded (∼28%), since using only completely genotyped individuals provided a sufficient large sample size.
Migrate-n allows to estimate the parameters θ (population size) and M (migration rate) under the assumption that they are constant through time. Uniform priors for these parameters were set (for θ, minimum = 0, maximum = 10, and delta = 0.1; for M, minimum = 0, maximum = 100, and delta = 10), and the program was run on a subsample of 24 (for populations D and F) or 30 (for north–south populations) individuals per population with the Brownian motion microsatellite model using Bayesian inference. Parameter values were searched using Markov chain Monte Carlo (MCMC) for 1 million generations with a burn-in of 10,000. Three models were explored to test different hypotheses of gene flow. The first model represented a full migration model with bidirectional migration rates. The additional two models tested unidirectional migration, each in the opposite direction. The Bezier approximation score for all loci was compared between models to find the most likely direction of gene flow. Using the estimated θ and M parameter values, the number of migrants per generation was calculated from that model by the following equation: Nm = (θ*M)/4.
Demographic history and population divergence
The software DIYABC Random Forest (RF) v1.0.14-beta (Collin et al., 2021) was used to gain insight into the demographic history of R. spiralis populations. Seven scenarios of historical divergence were investigated in a model that was designed using the three groups adopted from the DAPC results (ABCE, GHIJ, and DF). In doing so, parameter priors were assumed uniform (for NA, min = 10, max = 10,000; for N1, min = 10 max = 10,000; for N2, min = 10, max = 10,000; for N3, min = 10 max = 10,000; for td, min = 0 max = 5,000; for t1, min = 0 max = 5000) and all loci were considered dinucleotidic. A training dataset was generated by running 70,000 simulations. Hereby, all summary statistics available in DIYABC RF v1.0.14-beta were used. Thereafter, RF algorithms implemented in the program were used to predict the best scenario. The full training dataset was considered, and RF analyses were ran using 500 trees. The scenario with the highest classification vote was selected as the best-suited scenario for the target dataset. For this scenario, parameters (NA, N1, N2, N3, t1, td) were estimated.
Results
Genetic diversity and identity
Since all populations contained several individuals with more than two alleles for several loci, there is an indication of tetraploidy. In two populations (A and C), we even detected a few individuals with up to five alleles for the same locus (Supplementary Table S1). Although this might suggest the existence of hexaploid individuals, for the purpose of this study these samples were considered tetraploids, by considering the most common alleles. We observed plenty of multiallelic (three or four alleles per locus) situations in populations D and F when compared to all other populations. In total, we identified 147 alleles from 15 loci in 193 individuals (mean number A = 9.7, effective number Ae = 4.2, allelic richness Ar = 5.5), with minor differences among populations (Table 1). Population J had the lowest number of alleles (A = 2.9 and Ae = 2.6). Averaged across all populations and loci (Table 1), observed heterozygosity was lower than expected heterozygosity (Ho = 0.599, He = 0.671). The individual inbreeding Fi was significantly positive for seven out of 10 populations and significantly negative for the remaining (Table 1). Population G showed the highest level of heterozygote shortage (Fi = 0.361), whereas population A showed the highest level of heterozygote access (Fi = -0.221). We identified 57 repeated MLGs from the original dataset. The largest clonal richness (R = 0.86-1 was observed in the ‘salina fridda’ water body) (Table 1). Populations A and J featured genets that were extended along the majority of the transect, although they were intermingled with other genets.
Chloroplast sequences of samples from each population resulted had exactly similar lengths for the trnH-psbA intergenic spacer (452 bp) and of two mononucleotide microsatellite regions ccmp3 (157 bp) and ccmp10 (166 bp). For ccmp2, variants of either 202 and 203 bp were found in population C and populations E, F, and G, respectively, or were encountered mixed among individuals within the same population, namely, populations A, B, D, H, I, and J (GenBank accessions were provided in the Methods section). These sequences respectively correspond to haplotypes B1 and B3 of R. spiralis as defined in Triest and Sierens (2014).
The nuclear ribosomal cistron sequences corresponded to ITS-A of R. spiralis as defined in Triest and Sierens (2014). However, the complete cistron showed three additional variants due to mutations in position 690 (C or G)– and position 7,064 (C or T) (Table 1). An rRna-cistron variant (C–C, respectively at positions 690 and 7,064) was observed in populations A and H, a C–T variant in populations B, D, and F, and a G–C variant in populations C, E, I, and J (GenBank accession ON527176, ON527177, and ON527178). The overlapping reads were consistent in most samples, although populations D and F showed an incomplete homogenization at nucleotide position 7,064 (61%–67% of T).
Spatial structures
Global ANOVA-based F-statistics were calculated to obtain a general imprint of the genetic structure of R. spiralis along the analyzed coast of Sicily. Overall, we found moderate population differentiation (Fst = 0.185), moderate within-population inbreeding (Fis = 0.193), and higher overall inbreeding (Fit = 0.343). Mantel tests confirmed significant patterns of IBD (p < 0.01) among populations (Figure 2). Firstly, populations are significantly more differentiated (Fst) over larger geographic distance (y = 0.004x + 0.14 with R² = 0.24). Secondly, Euclidean distances reflected genetic structure slightly better (R² = 0.24) than distances following hydrological connections (R² = 0.20). More precisely, pairwise Fst permutation testing of all populations within four distance classes revealed a significantly lower differentiation (p < 0.01) below 4 km and a significantly higher differentiation (p < 0.05) beyond 14 km (Figure 2A). As expected, an opposite pattern was obtained for the relatedness among individuals between populations Fij (y = -0.0023x + 0.0019 with R² = 0.23 and p < 0.01). We found higher kinship below 4 km (p < 0.01).
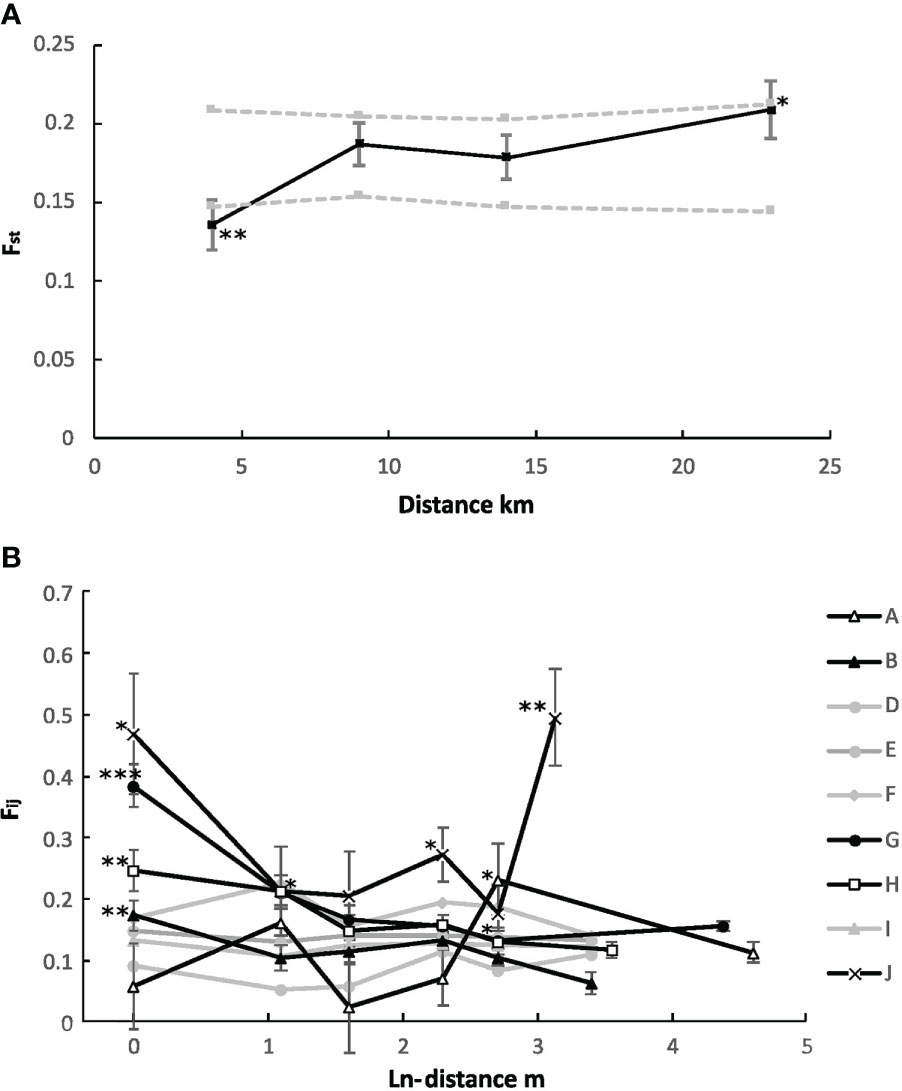
Figure 2 Spatial correlations among and within Ruppia spiralis populations along the analyzed western coast of Sicily (Italy). Rescue are displayed with discrete distance classes (with *** at p < 0.001; ** at p < 0.01; * at p < 0.05 significance level). (A) A mantel test of pairwise differention (Fst) over four distance classes. Dotted grey lines represent upper and lower confidence intervals at 95%. (B) Autocorrelation of individuals within populations as pairwise kinship (Fij) over In-distance. Black lines represent populations with significant FSGS, whereas grey lines represent non-significant structures.
A fine-scale genetic structure was detected among genets within populations. Across all populations, individual kinship over transect distance resulted in significant slopes of the regression with ln(distance) (y = -0.0091 * ln(x) + 0.162, with R² = 0.004 and p < 0.01). Divided among distance classes, genets that were less than 1 m apart had a significantly higher kinship than average (p < 0.001). However, a significant FSGS on genets was only observed in five out of nine populations (channel and lagoon populations, namely, populations A, B, G, H, J) with elevated values of kinship mostly within 1 m (Figure 2B). In populations A and J, a significantly higher value of kinship was found at higher distances (15–100 m). This is likely the result of gaps in the dataset that follow from the exclusion of pairs between ramets of the same genet. An FSGS was absent in the ‘salina fridda’ habitat.
Population structure and clustering
The DAPC showed three strongly separated groups (K = 3) (Figure 3A) and is reflected by the PCA (Supplementary Figure S3). Clusters 1 and 2, which are only separated along the y-axis of the DA, discriminate between the northern populations and southern populations. Cluster 3 exclusively contains samples of populations D and F and are clearly separated along the x-axis. Almost all populations contain low levels of admixture between clusters (Figure 3B). Individuals that were assigned to a different cluster than the majority of its population are characterized by alleles that can be considered common within the complete region of this study. The three clusters are differentiated by an AMOVA-based ρst value of 0.251 (ρst among populations = 0.279).
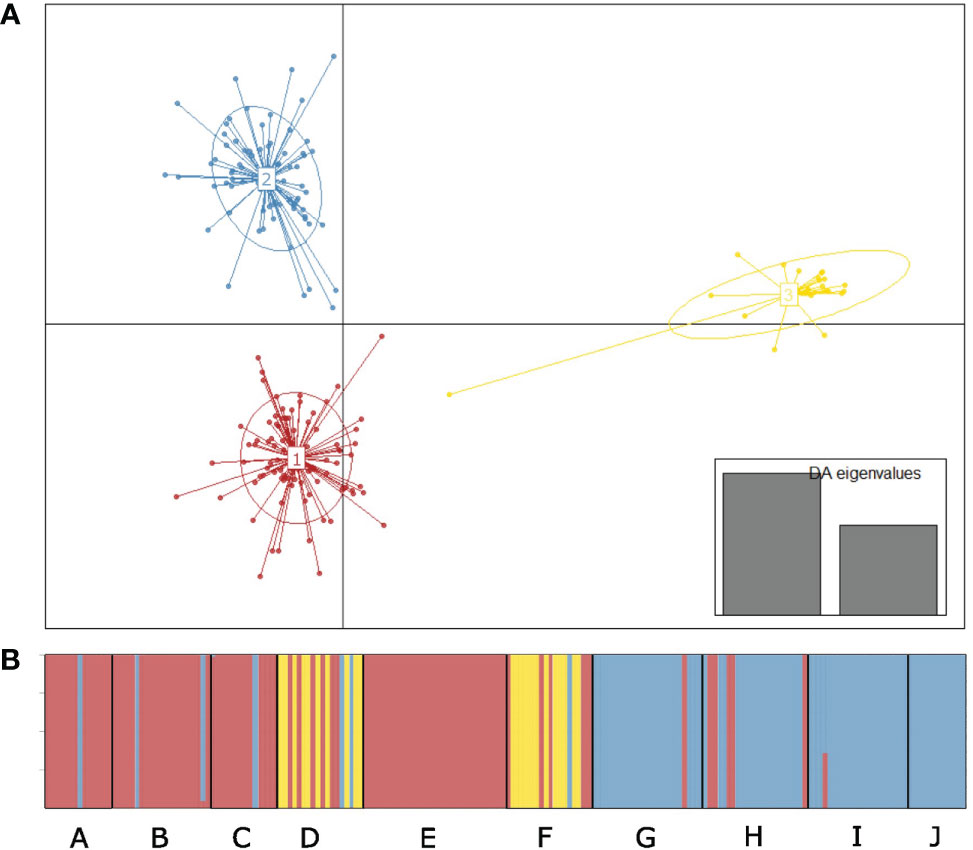
Figure 3 Discriminant analysis of principal components of ten Ruppia spiralis populations inferred for K = 3 populations. (A) Scatter plot describing the relationship between the clusters. Each colored circle represents a cluster and each dot represents an individual. Numbers represent the different clusters identified by the DAPC analysis. (B) Barplot showing the posterior probabilities of membership of individuals to the clusters. Populations are arranged from north to south (A–J) on the x-axis. Different colors correspond to the different clusters from the scatterplot.
Gene flow
The Migrate-n analyses revealed the north to south directional models to be the most likely models to predict gene flow along the western coast of Sicily, when compared to the south to north unidirectional and bidirectional models (Table 2). These models estimated a gene flow of Nem = 9.9 from the source population D to sink population F and a gene flow of Nem = 2.7 from the source populations A, B, C, and E to the sink populations G, H, I, and J.
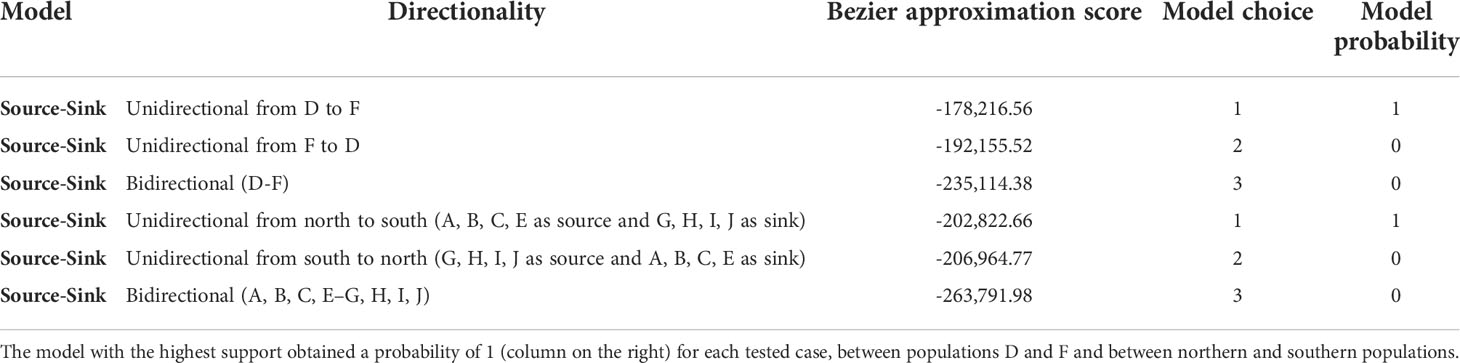
Table 2 Migrate-n models comparing gene flow directionality of Ruppia spiralis between waterbodies located along a 25-km coastal stretch of western Sicily (Italy).
Demographic history and population divergence
The ABCDIY-RF analysis suggested that northern and southern populations have diverged more recently than, but not long after, the divergence between northern populations and populations D and F (Scenario 7 in Figure 4, Table 3). Considering a generation time of 1 year, with potential overlap between generations, the divergence along this coastal stretch of the Western Sicily coastline appeared fairly recent and was estimated between 430 (lower CI at t1) and 4615 (upper CI at t1) generations.
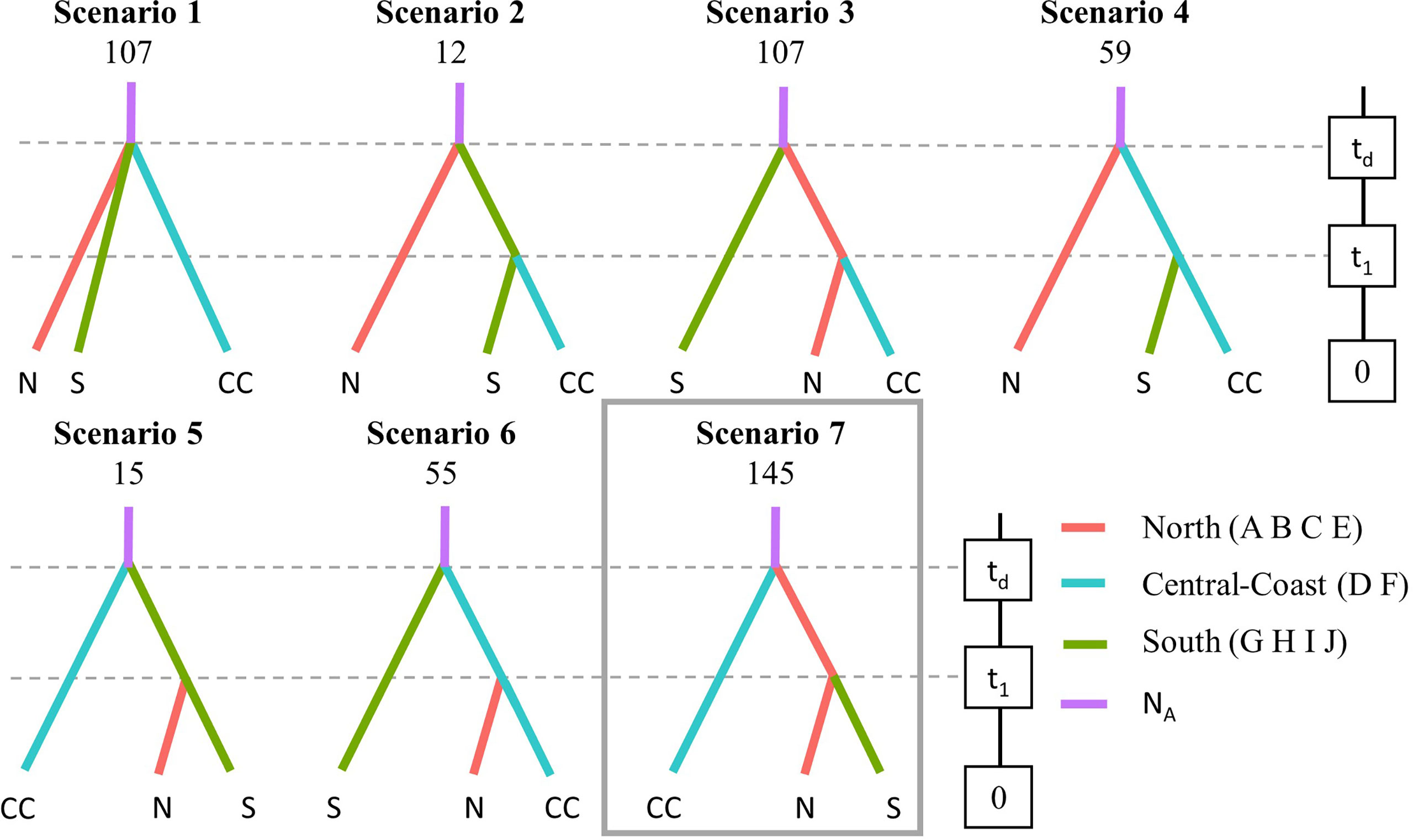
Figure 4 Design of DIYABC RF analysis to compare seven scenarios of population divergence. Scenario 1 represents an expansion event; scenario 2-7 represent all possibilities of divergence with a continuous population size and two divergence events. NA is the ancestral effective population size and td and t1 represent time events (not drawn to scale). Classifcation votes are not together with each scenario and the selected scenario (corresponding to the one with the highest vote) is indicated by a grey box (posterior probability 0.455).
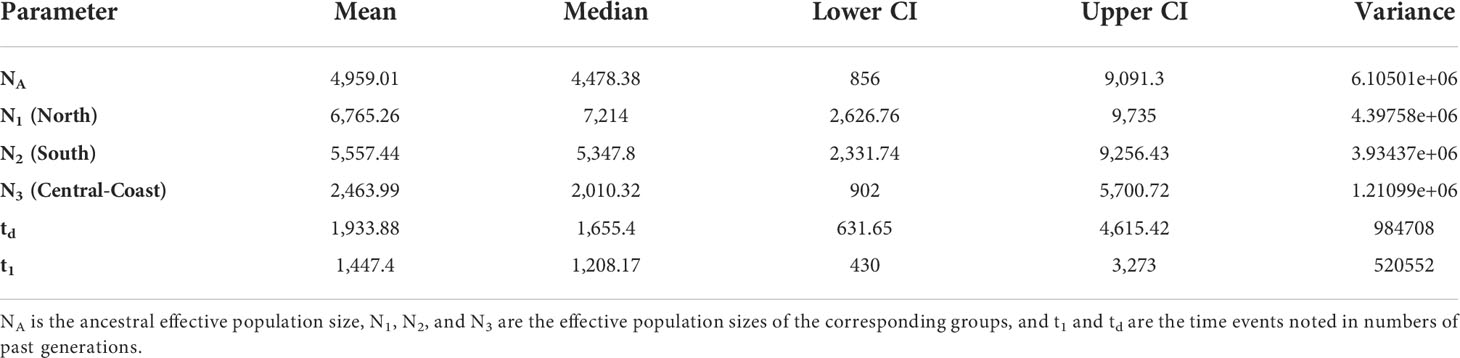
Table 3 Parameter estimations of the selected scenario (Scenario 7 in Figure 4) in the DIYABC-RF analysis.
Discussion
This study investigated the genetic structure and connectivity of Ruppia spiralis populations in various water bodies along the salinas of the western coast of Sicily. In particular, we focused on spatial genetic analyses and inferred patterns of gene flow, historical divergence, and dispersal along a 25-km stretch. The high amount of genetic diversity was similar to other studies of R. spiralis in the Mediterranean, possibly due to a glacial refugium for R. spiralis in Sicily and elsewhere in the Mediterranean (Triest and Sierens, 2014; Martínez-Garrido, et al., 2017; Triest et al., 2017). We detected spatial patterns at regional, although less at fine-scale or transect level. From these outcomes, several survival strategies as well as patterns of dispersal could be inferred.
Regional structure and connectivity
Overall, the observed genetic structure along the western coast of Sicily contains patterns of distance-related differentiation between populations. A Mantel test resulted in a significant pattern of IBD. Moreover, pairwise Fst permutation testing within distance classes revealed a significantly lower differentiation between sites that were less than 4 km apart and a significantly higher differentiation between sites that were over 14 km apart. Nevertheless, the three clusters as suggested by the DAPC indicate that genetic structure is not solely reflecting an overall IBD pattern. One of the gene pools (cluster 3) that comprise individuals of populations D and F is characterized by a greater number of multiallelic loci and many unique alleles. Although geographically closely located to cluster 1, populations D and F are genetically relatively strongly diverged from others. This high differentiation can be explained by the historical divergence and by the relatively lower effective population size (Ne) of populations D and F. According to the DIYABC-RF analysis, D and F most likely diverged from (or alternatively, currently are more related to) the northern populations before the divergence between north and south over this regional distance. Since these time events were estimated relatively close to each other, the severity of differentiation is possibly driven further by the lower Ne of populations D and F. This is possible when restricted gene flow contributed to the maintenance of only limited alleles and/or alleles with deviant lengths. The main contributing alleles of the DAPC do tie in with this hypothesis. It could be discussed that model choice was not fully unambiguous. However, this is likely the result of gene flow allowing migrants from other groups to enter the gene pool, thus not having fully separated groups in the analysis. Indeed, individuals from cluster 1 were predominantly present in the north and individuals from cluster 2 in the south, although not exclusively. Based on the Migrate-n analysis between northern and southern populations, we can exclude that their differentiation is due to effects of drift only and can provide evidence of historical gene flow (i.e., Nem >1) (Wright, 1943). However, the estimated Nem values are low, indicating limited overall connectivity between north and south. In fact, the degree of migration may still be overestimated in the calculation of Nem. This is because a tetraploid migrant of R. spiralis carries more allele copies than a diploid migrant, hence having a greater effect on the evening out of allele frequencies (Meirmans et al., 2018). Thus, the ploidy level of R. spiralis ultimately increases the effect of migration to buffer population differentiation. Migrate-n additionally revealed that gene flow most likely followed a north to south direction. Interestingly, this directionality corresponds with the direction of sea currents along the western coast of Sicily and thus supports our hypotheses that hydrological connectivity may play an important role in the colonialization of R. spiralis in new habitats. The main sea current along the western coast of Sicily moves from north to south but differs in velocity at the local scale (Pisacane et al., 2018; Menna et al., 2019; Palma et al., 2020). Local conditions such as reduced velocity between the eastern coast of Isola Grande and the mainland might partially retain dispersal and restrict gene flow even further. In fact, isolating effects of currents in genetic structure have been observed in seagrasses (Ruiz-Montoya et al., 2015; Jahnke et al., 2019).
In contrast to our results, a study of the Southern Iberian Peninsula found a stronger influence of dispersal by birds than by sea currents (Martínez-Garrido et al., 2017). Previously, it has been discussed that these findings might be an effect of repeated sampling sites within the same lagoon (Triest et al., 2017). It is important to note that birds can only be considered a main disperser when they are abundant during the period of seed production, which for R. spiralis would be around August (Verhoeven, 1979). Although the natural reserve in Trapani is the main corridor in the central-western Mediterranean for migratory birds (Sorci et al., 1991), we expect that this will not have a considerable contribution to the dispersal of R. spiralis seeds in the Mediterranean. In studies on Ruppia where bird-mediated dispersal is evidenced in isolated lagoons, a scattered genetic structure is observed (Triest and Sierens, 2015). If waterfowl had a strong impact on gene dispersal of R. spiralis, we would expect more pronounced homogenization between populations. Although microsatellite alleles were substantially different and indicated three major clusters, the salina populations of western Sicily feature mixed populations of individuals assigned to different genetic clusters. The chloroplast haplotypes B1 and B3 indicated additional traces of mixed gene pools in most populations situated most closely and connected to the coastal zone, namely, A, B, D, H, I, and J. The more isolated inland locations appeared to contain a single haplotype. Three nuclear ribosomal cistron variants further subdivided the few studied individuals of the three major clusters whereas the maternal chloroplast markers suggest mixing of variants after seed dispersal between populations. Populations D and F remain most divergent for all markers and had incomplete homogenization of the ribosomal cistron suggesting historical introgression events. This idea is also supported by their higher level of allelic diversity and larger occurrence of individuals with up to four alleles in a locus. Together with the potential occurrence of hexaploid individuals in population A, the genetic clusters and mixing of variants suggest a dynamic situation as a result of occasional dispersal between populations. Even if tests of directionality point to a historical north to south migration for the region, one should consider as well random dispersal patterns and occasions of locally mixed gene pools comparable to observations of multiple hybridization events in the Camargue region (Beirinckx et al., 2020). Therefore, it is important to note that birds will certainly have their role in seed dispersal of R. spiralis. However, our results imply that the influence of currents could be more important in leaving traces of accumulated historical gene flow. In this aspect, R. spiralis resembles largely the survival strategy of seagrasses that depend on coastal currents than of, e.g., brackish water-submerged macrophytes such as Stuckenia pectinata (L.) Böerner (syn. Potamogeton pectinatus L.) that largely depend on bird-mediated seed dispersal. No doubt that R. spiralis undergoes both ways of dispersal, although hydrodynamics appear to be explanatory.
Fine-scale structure
Spatial autocorrelation analyses on genets showed different FSGS patterns between populations. For example, significantly higher values of kinship at a close distance (<1 m) were detected in populations B, G, H, and J, whereas no significant structures were observed in four other populations (D, E, F, I). We can assume that gene dispersal remained stable over several generations in populations where FSGS was detected. Under these conditions, local seed rains might form, whereby seedlings settle in the proximity of the parental species (Triest et al., 2014). However, patterns on FSGS among genets do not allow to distinguish between the dispersal of seeds or pollen. In contrast, the absence of FSGS might be related to effects of physical disturbance that may upset local seed rains and/or may cause a high mortality rate and decrease the reproductive output. Consequently, the frequency of disturbances will influence the pattern of fine-scale gene dispersal. The fact that we could not find significant FSGS in the ‘salina fridda’ indicated that this habitat presents more disturbances for R. spiralis. It has been demonstrated that the waterbird communities in a Mediterranean salina differ between ponds and from natural salt marshes (Birtsas et al., 2011). Moreover, it was shown that flamingos (Phoenicopterus roseus P.) specifically prefer the ‘salina fridda’ (or prebasin in Birtsas et al., 2011) and were the most numerous species in that pond. The feeding behavior of flamingos has previously been brought up as a disturbance factor in Ruppia ponds (Rodriguez-Pérez and Green, 2006). Therefore, it can be hypothesized that flamingos negatively impact the formation of FSGS through random mixing of propagules in R. spiralis populations. Likewise, hydrological processes such as the opening and closing of water supply may be considered a disturbance factor.
Ruppia spiralis can reproduce sexually through outcrossing or clonally through rhizomes (Verhoeven, 1979; Gesti et al., 2005). It is not known which factors either affect clonal growth or induce flower production. It has been suggested that the stability of the habitat could explain the observed variation in clonal structure (Martínez-Garrido et al., 2017), as was observed in seagrasses (Eriksson, 1993; McMahon et al., 2017). Nearly all populations sustained a high genotypic diversity and a low frequency of clones, although much variation in clonal richness was found between populations (R = 0.48–1). In addition, we found high allelic diversity and high values of heterozygosity, suggesting that outcrossing is the preferred mode of reproduction. The flowers of R. spiralis are well adapted for outcrossing (Ackerman, 1995; Triest et al., 2018). Pollen is released at the water surface and floats upon the surface toward female flowers (Verhoeven, 1979). Because of this different reproductive ecology of R. spiralis, high inbreeding levels have not been detected within the species, whereas it has been in sister taxa R. maritima and R. brevipedunculata (Triest and Sierens, 2015; Triest et al., 2021a). Next to reproduction strategy, habitat preferences and ploidy level may be considered genetic drivers in Ruppia species. Overall, R. spiralis prefers more permanent waterbodies than R. maritima (Verhoeven, 1979; Triest et al., 2015; Beirinckx et al., 2020). Therefore, R. spiralis can form perennial populations that provide more time for mutations to accumulate within the clones. Similarly, more mutations per generation are possible in the tetraploid R. spiralis compared to the diploid R. maritima, because the amount of chromosome copies is doubled (Meirmans et al., 2018). The combination of these characteristics makes that R. spiralis can maintain a large amount of genetic diversity. Notwithstanding the overall high diversity, a higher proportion of clones was sampled in some populations (A, J, B, H, and G in decreasing order). These populations correspond to those with significant FSGS and consequently more stable habitats. It has been hypothesized that stable habitats increase the probability of rhizome survival, allowing clones to spread and colonize over a long period (Martínez-Garrido et al., 2017). Our results might support this hypothesis, although the observed high diversity is in favor of supporting a survival strategy of limited local (patchy) regrowth.
Conservation implications
Anthropogenic disturbances are threatening wetland ecosystems all over the world. Because R. spiralis has a wide tolerance from brackish water to saltwater and can persist under many disturbances, it can provide habitat for marine fish and invertebrates and resting areas for many species of migratory birds in a variety of aquatic systems (Verhoeven, 1980). Salinas have been identified as valuable surrogate wetlands for shore and migratory birds, which may buffer against the impact of natural habitat loss (Weber et al., 1999; Masero, 2003). In the Mediterranean Basin, where salinas extend over 1,000 km² in area (Sadoul et al., 1998), their importance for conservation is without a doubt. However, there has been a trend to cease salt production in many parts of the Mediterranean. As a result, many salinas are inactive and their hydrological infrastructures are collapsing (Sadoul et al., 1998; Petanidou and Dalaka, 2009). Present data on R. spiralis highlight the importance of water flow in salinas. We therefore recommend active management of salinas, i.e., ponds have to be (temporarily) connected to and supplied with seawater, for maintaining long-term viable populations. A similar management plan has been advised for the conservation of salina water-bird species with regard to the protection of their nests (Birtsas et al., 2011). Since R. spiralis is the most dominant submerged macrophyte species that can persist in these salinas and deliver conditions for high-quality food in sufficient quantities to support waterfowl (Triest and Sierens, 2009), this will be beneficial for a wide range of wildlife.
Perceptions of the diversity of Mediterranean Ruppia
The taxonomy of the Ruppia genus is complicated and has undergone many revisions over the past decades (den Hartog and Kuo, 2006; Triest and Sierens, 2014; Ito et al., 2017; den Hartog and Triest, 2020). In general, three species are recognized in the Mediterranean: R. spiralis, R. maritima, and the more restricted R. drepanensis Tineo. Several genome duplication events have characterized these species, causing different numbers of chromosomes to be observed (Triest and Symoens, 1991; Talavera et al., 1993; Mannino and Geraci, 2016; Beirinckx et al., 2020). Traditionally, R. spiralis is considered to be a tetraploid taxon. However, an earlier study on Sicilian Ruppia populations revealed the presence of a diploid R. spiralis (Mannino and Geraci, 2016). In the present study, we found, next to tetraploid R. spiralis, indications of hexaploid individuals of R. spiralis. A hexaploid R. spiralis has only once before been detected in northern Germany (Reese, 1962). In western Sicily, recent evidence also revealed the presence of R. drepanensis (De Castro et al., 2021). The diversity of Ruppia that coexists in this area may follow from the diversity gradient, observed over the whole Mediterranean Basin (Triest and Sierens, 2014). Hereby, the strait of Sicily could function as a funnel, where the high diversity of the western basin passes and accumulates. Further investigation is certainly needed to confirm this hypothesis and to confirm if hexaploid R. spiralis truly exists in Sicily.
In conclusion, high levels of allelic diversity were detected in R. spiralis at the regional scale. Distinct genetic clusters indicate that its distribution along western Sicily is shaped by different historical colonization events and geographic distance. The observed genetic structure follows an overall north-to-south migration pattern, confirming expectations of gene dispersal by currents. To allow gene dispersal between populations, managers should be aware of the importance of hydrological connectivity. Despite significant structures at a regional scale, significant FSGS were only detected in some populations. Our results suggest that salinas are valuable habitats for harboring diverse R. spiralis populations at a regional scale, although these may be subject to a local physical disturbance.
Data availability statement
The datasets presented in this study can be found in online repositories. The names of the repository/repositories and accession number(s) can be found in the article/Supplementary Material.
Author contributions
LT, LBo, LBe, and AM contributed to the conception and design of the study. LBo and LBe performed data collection. LBo and TS carried out DNA extractions and PCRs. LT de novo assembled the nuclear ribosomal cistron, aligned reads, and compared haplotype identity. TS and LT contributed reagents, materials, and analytic tools. LBo organized the database, performed the analyses, and wrote the first draft of the manuscript. All authors contributed to the manuscript revision and read and approved the submitted version.
Funding
This research was supported by BAS42 of the Vrije Universiteit Brussel.
Acknowledgments
We want to thank Gerardo Cortellaro, Silvana Piacentino, and Anna Giordano from WWF Italy for the organization of sampling trips and their helpful support in finding Ruppia populations. We also acknowledge Pepe Culcasi and all other salt workers of the Natural Reserve “Saline di Trapani e Paceco” for allowing access to the sampling sites.
Conflict of interest
The authors declare that the research was conducted in the absence of any commercial or financial relationships that could be construed as a potential conflict of interest.
Publisher’s note
All claims expressed in this article are solely those of the authors and do not necessarily represent those of their affiliated organizations, or those of the publisher, the editors and the reviewers. Any product that may be evaluated in this article, or claim that may be made by its manufacturer, is not guaranteed or endorsed by the publisher.
Supplementary material
The Supplementary Material for this article can be found online at: https://www.frontiersin.org/articles/10.3389/fmars.2022.950795/full#supplementary-material
References
Ackerman J. D. (1995). Convergence of filiform pollen morphologies in sea- grasses: Functional mechanisms. Evol. Ecol. 9, 139–153. doi: 10.1007/BF01237753
Beerli P. (2012). Migrate documentation version 3.2.1 (Tallahassee: Florida State University) 114. Dept Scientific Computing.
Beerli P., Mashayekhi S., Sadeghi M., Khodaei M., Shaw K. (2019). Population genetic inference with MIGRATE. Curr. Protoc. Bioinform. 68, 1–28. doi: 10.1002/cpbi.87
Beirinckx L., Vanschoenwinkel B., Triest L. (2020). Hidden hybridization and habitat differentiation in a Mediterranean macrophyte, the euryhaline genus Ruppia. Front. Plant Sci. 11. doi: 10.3389/fpls.2020.00830
Birtsas P. K., Sokos C. K., Papaspyropoulos K. G., Kazoglou Y. E. (2011). Comparison of waterbird communities in a Mediterranean salina - saltmarsh complex. Belg. J. Zool. 141 (1), 24–31.
Britton R. H., Johnson A. R. (1987). An ecological account of a Mediterranean salina: The salin de Giraud, camargue (S. France). Biol. Conserv. 42 (3), 185–230. doi: 10.1016/0006-3207(87)90133-9
Brock M. A. (1981). Accumulation of proline in a submerged aquatic halophyte, ruppia l. Oecologia 51, 217–219. doi: 10.1007/BF00540604
Brock M. A. (1982). Biology of the salinity tolerant genus Ruppia l. in saline lakes in south Australia i. morphological variation within and between species and ecophysiology. Aquat. Bot. 13, 219–248. doi: 10.1016/0304-3770(82)90062-6
Charalambidou I., Santamaría L., Langevoord L. (2003). Effect of ingestion by five avian dispersers on the retention time, retrieval and germination of Ruppia maritima seeds. Funct. Ecol. 17 (6), 747–753. doi: 10.1111/j.1365-2435.2003.00787.x
Collin F.-D., Durif G., Raynal L., Gautier M., Vitalis R., Lombaert E., et al. (2021). Extending approximate Bayesian computation with supervised machine learning to infer demographic history from genetic polymorphisms using DIYABC random forest. Mol. Ecol. Resources Wiley/Blackwell 21 (8), 2598–2613. doi: 10.1111/1755-0998.13413
De Castro O., Geraci A., Mannino A. M., Mormile N., Santangelo A., Troia A. (2021). A contribution to the characterization of Ruppia drepanensis (Ruppiaceae), a key species of threatened mediterranean wetlands. Ann. Missouri Bot. Gard. 106, 1–9. doi: 10.3417/2020520
den Hartog C., Kuo. J. (2006). “Taxonomy and biogeography of seagrasses“, in Seagrasses: Biology, ecology and conservation. Eds. Larkum A. W. D., Orth R. J., Duarte C. (Dordrecht: Springer) 1–23.
den Hartog C., Triest L. (2020). A profound view and discourse on the typification and status of three confused taxa: Ruppia maritima, r. spiralis and R. cirrhosa. Botanica Marina 63, 229–239. doi: 10.1515/bot-2019-0045
Diehl S., Kornijów R. (1998). “Influence of submerged macrophytes on trophic interactions among fish and macroinvertebrates” in Structuring role of submerged macrophytes in lakes. Eds. Jeppesen E., Søndergaard M., Søndergaard M., Christoffersen K. (New York: Springer), 24–46.
Dierick J., Phan T. T. H., Luong Q. D., Triest L. (2021). Persistent clones and local seed recruitment contribute to the resilience of Enhalus acoroides populations under disturbance. Front. Plant Sci. 12. doi: 10.3389/fpls.2021.658213
Dorken M. E., Eckert C. G. (2001). Severely reduced sexual reproductionin northern populations of a clonal plant, decodon verticillatus (Lythraceae). J. Ecol. 89, 339–350. doi: 10.1046/j.1365-2745.2001.00558.x
Dray S., Dufour A.-B. (2007). The ade4 package: Implementing the duality diagram for ecologists. J. Stat. Software 22 (4), 1–20. doi: 10.18637/jss.v022.i04
Ehlers A., Worm B., Reusch T. (2008). Importance of genetic diversity in eelgrass zostera marina for its resilience to global warming. Mar. Ecol. Prog. Ser. 355, 1–7. doi: 10.3354/meps07369
Eriksson O. (1993). Dynamics of genets in clonal plants. Trends Ecol. Evol. 8, 313–316. doi: 10.1016/0169-5347(93)90237-j
Evagelopoulos A., Koutsoubas D., Basset A., Pinna M., Dimitriadis C., Sangiorgio F., et al. (2008). Spatial and seasonal variability of the macrobenthic fauna in Mediterranean solar saltworks ecosystems. Aquat. Conserv. 18, 118–134. doi: 10.1002/aqc.948
Figuerola J., Green A., Santamaria L. (2002). Comparative dispersal effectiveness of wigeongrass seeds by waterfowl wintering in south- west Spain: Quantitative and qualitative aspects. J. Ecol. 90, 989–1001. doi: 10.1046/j.1365-2745.2002.00734.x
Gesti J., Badosa A., Quintana. X. D. (2005). Reproductive potential in Ruppia cirrhosa (Petagna) grande in response to water permanence. Aquat. Bot. 81, 191–198. doi: 10.1016/j.aquabot.2004.11.005
Green P., Short F. T. (2003). World atlas of seagrasses (Berkeley and Los Angeles: University of California Press). UNEP-WCMC.
Hamdi N., Charfi F., Moali A. (2008). Variation of the waterbird community relying to the ichkeul national park, Tunisia. Eur. J. Wildl. Res. 54, 417–424. doi: 10.1007/s10344-007-0162-7
Hardy O. J., Vekemans X. (2002). SPAGeDi: A versatile computer program to analyse spatial genetic structure at the individual or population levels. Mol. Ecol. Notes 2, 618–620. doi: 10.1046/j.1471-8286.2002.00305.x
Ito Y., Ohi-Toma T., Murata J., Tanaka N. (2010). Hybridization and polyploidy of an aquatic plant, Ruppia (Ruppiaceae), inferred from plastid and nuclear DNA phylogenies. Am. J. Bot. 97, 1156–1167. doi: 10.3732/ajb.0900168
Ito Y., Ohi-Toma T., Nepi C., Stinca A., Tanaka N., Murata J. (2017). Towards a better understanding of the Ruppia maritima complex (Ruppiaceae): Notes on the correct application and typification of the names R. cirrhosa and R. spiralis. Taxon 66, 167–171. doi: 10.12705/661.11
Jackson L. J. (2017). Molecular tools provide a range of powerful options for the conservationist's toolbox. Aquat. Conserv. 27, 296–302. doi: 10.1002/aqc.2766
Jahnke M., Gullström M., Larsson J., Asplund M. E., Mgeleka S., Silas M. O., et al. (2019). Population genetic structure and connectivity of the seagrass thalassia hemprichii in the Western Indian ocean is influenced by predominant ocean currents. Ecol. Evol. 9 (16), 8953–8964. doi: 10.1002/ece3.5420
Jombart T. (2008). Adegenet: A r package for the multivariate analysis of genetic markers. Bioinformatics 24, 1403–1405. doi: 10.1093/bioinformatics/btn129
Jombart T., Devillard S., Balloux F. (2010). Discriminant analysis of principal components: A new method for the analysis of genetically structured populations. BMC Genet. 11, 94. doi: 10.1186/1471-2156-11-94
Kamvar Z. N., Brooks J. C., Grünwald N. J. (2015). Novel r tools for analysis of genome-wide population genetic data with emphasis on clonality. Front. Genet. 6. doi: 10.3389/fgene.2015.00208
Kamvar Z. N., Tabima J. F., Grünwald N. J. (2014). Poppr?: An r package for genetic analysis of populations with clonal, partially clonal, and/or sexual reproduction. PeerJ 2, e281. doi: 10.7717/peerj.281
Katoh K., Misawa K., Kuma K. I., Miyata T. (2002). MAFFT: A novel method for rapid multiple sequence alignment based on fast Fourier transform. Nucleic Acids Res. 30, 3059–3066. doi: 10.1093/nar/gkf436
Katoh K., Standley D. M. (2013). MAFFT multiple sequence alignment software version 7: Improvements in performance and usability. Mol. Biol. Evol. 30, 772–780. doi: 10.1093/molbev/mst010
Les D. H., Cleland M. A., Waycott M. (1997). Phylogenetic studies in alismatidae, II: Evolution of marine angiosperms (Seagrasses) and hydrophily. Syst. Biol. 22, 443–463. doi: 10.2307/2419820
Loiselle B., Sork V. L., Nason J., Graham C. (1995). Spatial genetic structure of a tropical understory shrub, Psychotria officinalis (Rubiaceae). Am. J. Bot. 82, 1420–1425. doi: 10.1002/j.1537-2197.1995.tb12679.x
López E., Aguilera P. A., Schmitz M. F., Castro H., Pineda F. D. (2010). Selection of ecological indicators for the conservation, management and monitoring of Mediterranean coastal salinas. Environ. Monit. Assess. 166, 241–256. doi: 10.1007/s10661-009-0998-2
López E., Castro H., Aguilera P. (2004). Salinas de andalucía (Sevilla: Junta de Andalucía). Consejería de Medio Ambiente.
Mannino A. M., Geraci A. (2016). Diploid Ruppia cirrhosa populations from a southern Mediterranean shallow system. Aquat. Bot. 132, 37–40. doi: 10.1016/j.aquabot.2016.04.001
Mannino A. M., Graziano M. (2014). Differences in the growth cycle of Ruppia cirrhosa (Petagna) grande in a Mediterranean shallow system. Plant Biosyst 150 (1), 54–61. doi: 10.1080/11263504.2014.906511
Mannino A. M., Menéndez M., Obrador B., Sfriso A., Triest L. (2015). The genus Ruppia l. (Ruppiaceae) in the Mediterranean region: An overview. Aquat. Bot. 124, 1–9. doi: 10.1016/j.aquabot.2015.02.005
Martínez-Garrido J., Bermejo R., Serrão E. A., Sánchez-Lizaso J., González-Wangüemert M. (2017). Regional genetic structure in the aquatic macrophyte Ruppia cirrhosa suggests dispersal by waterbirds. Estuaries Coasts 40, 1705–1716. doi: 10.1007/s12237-017-0247-9
Martínez-Garrido J., Serrão E. A., Engelen A. H., Cox C. J., García-Murillo P., González-Wangüemert M. (2016). Multilocus genetic analyses provide insight into speciation and hybridization in aquatic grasses, genus Ruppia. Biol. J. Linn. Soc 117, 177–191. doi: 10.1111/bij.12666
Masero J. A. (2003). Assessing alternative anthropogenic habitats for conserving water- birds: Salinas as buffer areas against the impact of natural habitat loss for shorebirds. Biodivers. Conserv. 12, 1157–1173. doi: 10.1023/a:1023021320448
McMahon K. M., Evans R. D., van Dijk K., Hernawan U., Kendrick G. A., Lavery P. S., et al. (2017). Disturbance is an important driver of clonal richness in tropical seagrasses. Front. Plant Sci. 8. doi: 10.3389/fpls.2017.02026
Meirmans P. G., Liu S. (2018). Analysis of molecular variance (AMOVA) for autopolyploids. Front. Ecol. Evol. 6. doi: 10.3389/fevo.2018.00066
Meirmans P. G., Liu S., Van Tienderen P. H. (2018). The analysis of polyploid genetic data. J. Hered. 109, 283–296. doi: 10.1093/jhered/esy006
Meirmans P. G., Van Tienderen P. H. (2004). GENOTYPE and GENODIVE: two programs for the analysis of genetic diversity of asexual organisms. Mol. Ecol. Notes 4, 792–794. doi: 10.1111/j.1471-8286.2004.00770.x
Melack J. M. (1988). “Aquatic plants in extreme environments“, in Handbook of vegetation science, vol. Vol.15 14 . Ed. Symoens J. J. (Dordrecht: Springer), 341–378.
Menna M., Poulain P.-M., Ciani D., Doglioli A., Notarstefano G., Gerin R., et al. (2019). New insights of the Sicily channel and southern tyrrhenian Sea variability. Water 11 (7), 1355. doi: 10.3390/w11071355
Messina G., Pezzino E., Montesanto G., Caruso D., Lombardo B. M. (2012). The diversity of terrestrial isopods in the natural reserve “Saline di trapani e paceco” (Crustacea, isopoda, oniscidea) in northwestern Sicily. ZooKeys 176, 215–230. doi: 10.3897/zookeys.176.2367
Orth R. J., Luckenbach M., Moore K. A. (1994). Seed dispersal in a marine macrophyte: implications for colonization and restoration. Ecology 75, 1927–1939. doi: 10.2307/1941597
Palma M., Iacono R., Sannino G., Bargagli A., Carillo A., Fekete B. M., et al. (2020). Short-term, linear, and non-linear local effects of the tides on the surface dynamics in a new, high-resolution model of the Mediterranean Sea circulation. Ocean Dynamics 70 (7), 935–963. doi: 10.1007/s10236-020-01364-6
Petanidou T., Dalaka A. (2009). Mediterranean’s changing saltscapes: A study of the abandonment of saltmaking business in Greece. Global NEST J. 11, 415–433.
Phan T. T. H., De Raeymaeker M., Luong Q. D., Triest L. (2017). Clonal and genetic diversity of the threatened seagrass Halophila beccarii in a tropical lagoon: Resilience through short distance dispersal. Aquat. Bot. 142, 96–104. doi: 10.1016/j.aquabot.2017.07.006
Pisacane G., Sannino G., Carillo A., Struglia M. V., Bastianoni S. (2018). Marine energy exploitation in the Mediterranean Region<: Steps forward and challenges. Front. Energy Res. 6. doi: 10.3389/fenrg.2018.00109
R Core Team (2019). R: A language and environment for statistical computing. R Foundation Stat. Computing Vienna Austria.
Reese G. (1962). Zur intragenerischen taxonomie der gattung Ruppia l. ein cytosystematischer beitrag. Zeimchrift fur. Botanik 50, 237–264.
Rodríguez-Pérez H., Green A. J. (2006). Waterbird impacts on widgeongrass Ruppia maritima in a Mediterranean wetland: Comparing bird groups and seasonal effects. Oikos 112 (3), 525–534. doi: 10.1111/j.0030-1299.2006.14307.x
Ruiz-Montoya L., Lowe R. J., Kendrick G. A. (2015). Contemporary connectivity is sustained by wind- and current-driven seed dispersal among seagrass meadows. Movement Ecol. 3, 1. doi: 10.1186/s40462-015-0034-9
Sadoul N., Walmsley J., Charpentier B. (1998). Salinas and nature conservation. MedWet Conservation of Mediterranean Wetlands series No 9. 96p, (France: Tour du Valat, Arles)
Short F., Carruthers T., Dennison W., Waycott M. (2007). Global seagrass distribution and diversity: A bioregional model. J. Exp. Mar. Biol. Ecol. 350, 3–20. doi: 10.1016/j.jembe.2007.06.012
Soares R.H.R.d., Assunção C. A., Fernandes. de O.F., Marinho-Soriano E. (2018). Identification and analysis of ecosystem services associated with biodiversity of saltworks. Ocean Coast. Manage. 163, 278–284. doi: 10.1016/j.ocecoaman.2018.07.007
Sorci G., Sarà M., Naselli-Flores L., Surdo S. (1991). Metodologia di valutazione della qualità ambientale: Applicazione alle aree umide siciliane. Ric. Biol. Selvaggina 17, 459–464.
Talavera S., García-Murillo P., Herrera J. (1993). Chromosome numbers and a new model for karyotype evolution in Ruppia l. (Ruppiaceae). Aquat. Bot. 45, 1–13. doi: 10.1016/0304-3770(93)90048-2
Thompson J. D., Higgins D. G., Gibson. T. J. (1994). CLUSTAL W: improving the sensitivity of progressive multiple sequence alignment through sequence weighting, position-specific gap penalties and weight matrix choice. Nucleic Acids Res. 22, 4673–4680. doi: 10.1093/nar/22.22.4673
Thompson P. R., Piecuch C. G., Merrifield M. A., McCreary J. P., Firing E. (2016). The Sicily channel surface circulation revisited using a neural clustering analysis of a high-resolution simulation. J. Geophys. Research: Oceans 121, 4545–4567. doi: 10.1002/2016JC012132
Triest L., Beirinckx L., Sierens T. (2018). Lagoons and saltwater wetlands getting more diversity: A molecular approach reveals cryptic lineages of a euryhaline submerged macrophyte (Ruppia). Aquat. Conserv. Mar. Freshw. Ecosyst. 28, 370–382. doi: 10.1002/aqc.2863
Triest L., Dierick J., Phan T. T. H., Luong Q. D., Huy N. Q., Sierens T. (2021a). Low genetic connectivity of strongly inbred Ruppia brevipedunculata in aquaculture dominated lagoons (Vietnam). Front. Conserv. Sci. 2. doi: 10.3389/fcosc.2021.723376
Triest L., Sierens T. (2009). High diversity of Ruppia meadows in saline ponds and lakes of the western Mediterranean. Hydrobiologia 634, 97–105. doi: 10.1007/s10750-009-9892-8
Triest L., Sierens T. (2010). Chloroplast sequences reveal a diversity gradient in the Mediterranean Ruppia cirrhosa species complex. Aquat. Bot. 93, 68–74. doi: 10.1016/j.aquabot.2010.03.007
Triest L., Sierens T. (2013). Is the genetic structure of Mediterranean Ruppia shaped by bird-mediated dispersal or sea currents? Aquat. Bot. 104, 45–53. doi: 10.1016/j.aquabot.2014.02.004
Triest L., Sierens T. (2014). Seagrass radiation after messinian salinity crisis reflected by strong genetic structuring and out-of-Africa scenario (Ruppiaceae). PloS One 9, e104264. doi: 10.1371/journal.pone.0104264
Triest L., Sierens T. (2015). Strong bottlenecks, inbreeding and multiple hybridization of threatened European Ruppia maritima populations. Aquat. Bot. 125, 31–43. doi: 10.1016/j.aquabot.2015.05.001
Triest L., Sierens T., Menemenlis D., van der Stocken T. (2017). Inferring connectivity range in submerged aquatic populations (Ruppia l.) Along european coastal lagoons from genetic imprint and simulated dispersal trajectories. Front. Plant Sci. 9. doi: 10.3389/fpls.2018.00806
Triest L., Sierens T., Terer T. (2014). Diversity and fine-scale spatial genetic structure of Cyperus papyrus populations in lake naivasha (Kenya) using microsatellite markers. Hydrobiologia 737, 131–144. doi: 10.1007/s10750-013-1584-8
Triest L., Sierens T., van der Stocken T. (2021b). Complete chloroplast genome variants reveal discrete long-distance dispersal routes of rhizophora in the Western Indian ocean. Front. Conserv. Sci. 2. doi: 10.3389/fcosc.2021.726676
Triest L., Symoens J. J. (1991). Isozymes in water plants. Ed. Triest L. (Meise:National Botanic Garden of Belgium) 117–132. Opera Botanica Belgica.
Vekemans X., Hardy O. J. (2004). New insights from fine-scale spatial genetic structure analyses in plant populations. Mol. Ecol. 13, 921–935. doi: 10.1046/j.1365-294x.2004.02076.x
Verhoeven J. T. A. (1979). The ecology of Ruppia-dominated communities in western europe. i. distribution of Ruppia representatives in relation to their autoecology. Aquat. Bot. 6, 197–268. doi: 10.1016/0304-3770(79)90064-0
Verhoeven J. T. A. (1980). The ecology of Ruppia-dominated communities in western europe. II. synecological classification. structure and dynamics of the macroflora and macrofauna communities. Aquat. Bot. 8, 1–85. doi: 10.1016/0304-3770(80)90044-3
Walmsley J. G. (1999). The ecological importance of Mediterranean salinasKorovessis N. A., Lekkas T. D (Eds.), Proceedings of the Post Conference Symposium SALTWORKS: Preserving saline coastal ecosystem (6th Conference on Environmental Science & Technology, pp. 81–95). (Samos, Greece:Global Nest).
Weber T. P., Houston A. I., Ens B. (1999). Consequences of habitat loss at migratory stopover sites: Atheoretical investigation. J. Avian Biol. 30, 416–426. doi: 10.2307/3677014
Wright S. I., Kalisz S., Slotte T. (2013). Evolutionary consequences of self-fertilization in plants. Proc. R. Soc B Biol. Sci. 280, 20130133. doi: 10.1098/rspb.2013.0133
Yu S., Shi M.-M., Chen X.-Y. (2014). Species diversity and distribution of ruppia in China: Potential roles of long-distance dispersal and environmental factors. J. Syst. Evol. 52, 231–239. doi: 10.1111/jse.12069
Keywords: connectivity, migration, salinas, microsatellite, coastal, submerged aquatic vegetation
Citation: Bossaer L, Beirinckx L, Sierens T, Mannino AM and Triest L (2022) Spatial genetic structure reveals migration directionality in Mediterranean Ruppia spiralis (Western Sicily). Front. Mar. Sci. 9:950795. doi: 10.3389/fmars.2022.950795
Received: 23 May 2022; Accepted: 19 August 2022;
Published: 12 September 2022.
Edited by:
Lorenzo Zane, University of Padua, ItalyReviewed by:
Carlos Vergara-Chen, Universidad Tecnológica de Panamá, PanamaPablo Garcia Murillo, University of Seville, Spain
Yu Ito, Setsunan University, Japan
Copyright © 2022 Bossaer, Beirinckx, Sierens, Mannino and Triest. This is an open-access article distributed under the terms of the Creative Commons Attribution License (CC BY). The use, distribution or reproduction in other forums is permitted, provided the original author(s) and the copyright owner(s) are credited and that the original publication in this journal is cited, in accordance with accepted academic practice. No use, distribution or reproduction is permitted which does not comply with these terms.
*Correspondence: Laura Bossaer, bGF1cmEuYm9zc2FlckB2dWIuYmU=