- Hawai'i Institute of Marine Biology, University of Hawai'i at Mānoa, Kāne'ohe, HI, United States
Global coral reef decline is largely driven by the breakdown of the coral-algal symbiosis during temperature stress. Corals can acclimatize to higher temperatures, but the cellular processes underlying this ability are poorly understood. We show that preconditioning-based improvements in thermal tolerance in Pocillopora acuta are accompanied by increases in host glutathione reductase (GR) activity and gene expression, which prevents DNA damage. A strong correlation between GR and BI-1 expressions in heat-stressed preconditioned corals and the presence of an antioxidant response element (ARE) in the GR promoter suggest BI-1 could regulate GR expression through Nrf2/ARE pathway. To fortify this link, we developed and GFP-validated an siRNA-mediated gene knockdown protocol and targeted the coral BI-1 gene. BI-1 knock-down specifically decreased GR expression and activity and increased oxidative DNA damage in heat-stressed preconditioned corals, showing that a BI-1-mediated, enhanced antioxidant response during acute heat stress is a key mechanism that prevents oxidative DNA damage after preconditioning.
Introduction
Healthy coral reefs support nearly one-third of marine species and provide shelter, nursery habitat and coastal protection across the tropical oceans (Knowlton et al., 2010; Fisher et al., 2015; Vercelloni et al., 2018). The energetic and structural foundation of this ecosystem is the coral-algal symbiosis, which is disrupted by thermal stress and is critically threatened by climate change. Climate-induced mass bleaching has impacted most of the world’s reefs and is predicted to increase in frequency and intensity, threatening the long-term persistence of these ecosystems (van Hooidonk et al., 2013; Hughes et al., 2018; Eakin et al., 2019). A deeper understanding of the cellular and molecular mechanisms underlying coral-algal symbiosis maintenance is critical for modern conservation and management (van Oppen et al., 2015; Committee on Interventions to Increase the Resilience of Coral Reefs et al., 2019).
Corals are energetically dependent on their intracellular algal symbionts (family Symbiodiniaceae) (LaJeunesse et al., 2018), which provide photosynthetically synthesized sugars and receive shelter and a supply of inorganic molecules from the host. During thermal stress, symbionts release increased reactive oxygen species (ROS), which are believed to trigger molecular cascades resulting in coral bleaching and can lead to the eventual death of the coral host organism (Lesser, 1996; Suggett et al., 2008; Saragosti et al., 2010; Roberty et al., 2015; Krueger et al., 2015a; Levin et al., 2016; Gardner et al., 2017; Oakley and Davy, 2018; Nielsen et al., 2018); however, definitive evidence to support this hypothesis is still missing (Szabó et al., 2020).
Under normal conditions, ROS are scavenged by antioxidant systems in the host and symbionts to reduce damage to cell membranes, lipids, proteins, and nucleic acids (Nesa and Hidaka, 2008; Halliwell and Gutteridge, 2015). Under increased temperatures, ROS concentrations become elevated and the coral holobiont activates first-line enzymatic antioxidants such as catalase or superoxide dismutase and maintain a reducing intracellular environment via the glutathione redox cycle (Krueger et al., 2014; Krueger et al., 2015a; Roberty et al., 2015; Diaz et al., 2016; Levin et al., 2016; Bielmyer-Fraser et al., 2018; Lopes et al., 2018; Nielsen et al., 2018). These systems are well-studied in Symbiodiniaceae, where genera with differing thermal resilience vary in antioxidant gene expression (Krueger et al., 2014; Krueger et al., 2015a; Levin et al., 2016) and thermally resilient algae generally produce more antioxidants, which are better able to maintain cellular homeostasis. Conversely, the role of host-derived antioxidants in bleaching and thermal resilience remains disputed. While pioneering studies show antioxidant activation in adult coral host tissue and larvae under heat stress (Lesser et al., 1990; Yakovleva et al., 2004; Yakovleva et al., 2009; Ross et al., 2013; Krueger et al., 2015a; Hillyer et al., 2017), more recent experiments have failed to support these findings (Nielsen et al., 2018; Lopes et al., 2018). However, maintenance of low ROS levels within the holobiont is likely a major factor maintaining the dynamic coral-algal symbiosis (Hughes et al., 2020; Szabó et al., 2020; Alderdice et al., 2022) and activation of DNA damage response pathways is a hallmark of thermal stress in corals (Dixon et al., 2020).
Intra-generational plasticity is critical for mitigating stress in corals (reviewed in (Drury, 2020)). As sedentary animals, they have only a limited ability to avoid stressful environmental conditions, thus their potential to react to dynamic changes in their surrounding is crucial for their survival. An increasing body of knowledge demonstrates various mechanisms (gene expression or morphological plasticity, i.e (Kenkel and Matz, 2017; Drury et al., 2022; Brambilla et al., 2022).) that corals employ to promote resilience. It highlights the importance of acclimatization for the long-term persistence of coral reefs to “buying time” for adaptive change (Drury, 2020). While many corals show improved thermal tolerance after pre-exposure to sublethal temperatures (Middlebrook et al., 2008; Bellantuono et al., 2012; Palumbi et al., 2014; Bay and Palumbi, 2015; Ainsworth et al., 2016; Schoepf et al., 2019; Dilworth et al., 2021; Majerova et al., 2021), the molecular triggers and consequences of this process remain poorly understood.
In this work, we aimed to identify and describe molecular pathways underlying acclimatization-based increased thermal resilience in the cosmopolitan reef-building coral Pocillopora acuta. To pursue functional analysis of this trait, we developed, validated, and conducted siRNA-mediated gene knockdown experiments in living adult corals. We hypothesize that upon thermal preconditioning, corals reinforce intracellular homeostasis via improvements in the glutathione-based antioxidant system, which in turn prevents accumulation of oxidative DNA damage. This mechanism may be mediated by the BI-1 protein via the Nrf2/ARE signaling pathway. Understanding cellular and molecular mechanisms that influence coral acclimatization or adaptation to thermal stress is an important step towards understanding resilience and trade-offs in corals and coral reef ecosystems.
Native Hawaiian culture is profoundly connected with corals and coral reefs, including those of Kāne'ohe Bay, O'ahu, where this research is based (Gregg et al., 2015). We hope to honor this bond and the unique relationship between local communities and Hawaiian land and sea and appreciate the opportunity to conduct this research.
Results
Thermal preconditioning reduces bleaching susceptibility in P. acuta
This study uses samples from the coral colonies described in the preconditioning experiment of Majerova et al. (2021), which were pre-exposed to a 3 day sublethal stress at 29°C (PC) or 26°C (NPC/control) two weeks before exposure to acute heat stress (32°C; Figure 1A). During this acute stress, preconditioned (PC) corals showed substantially prolonged symbiosis stability when compared to non-preconditioned (NPC) corals (Figure 1B). After 3 days of heat stress, NPC corals were visibly bleached while PC corals resembled control, non-treated corals. This difference was more pronounced after 5 days of heat stress. PC corals were losing symbionts significantly more slowly than in NPC corals (p< 0.001) (Majerova et al., 2021).
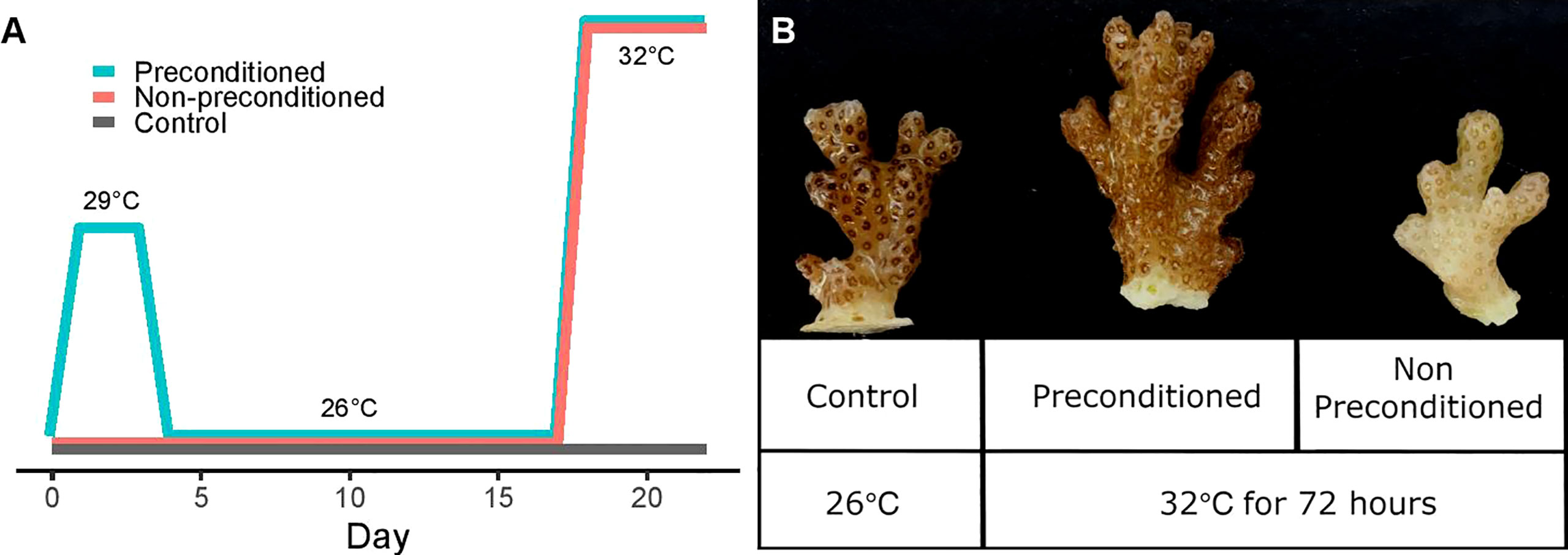
Figure 1 Experimental design. (A) Preconditioning profile. Preconditioned corals (PC) were exposed to sublethal 29°C for 72 hours and returned to 26°C for additional two weeks before undergoing acute thermal stress (32°C). Non-preconditioned (NPC) corals were exposed to acute thermal stress with no previous preconditioning. (B) Different response to acute heat stress (32°C for 72 hours) in preconditioned (PC) and non-preconditioned (NPC) corals (right). While PC corals resembled control corals, NPC corals were visibly bleached.
Preconditioning selectively increases the activity of host-derived glutathione reductase
The activity of glutathione reductase in P. acuta is influenced by acute heat stress (LMM, activity~conditioning*time + (1|colony); p(time) = 0.001) and was constitutively higher in PC corals than NPC corals (p(conditioning)< 0.001, Figure 2A). Interestingly, the activity of peroxide-scavenging antioxidants increased (p(time) = 0.009) but did not differ between PC and NPC corals. Elevated activities of two different peroxidases - catalase and glutathione peroxidase – have been previously detected in stressed corals (Yakovleva et al., 2004; Ross et al., 2013; Krueger et al., 2015a; Bielmyer-Fraser et al., 2018), but the catalase activity kit used here is not specific and may detect activity of any enzyme with peroxidase activity (Bioassay Systems, personal communication). Thus, we separately tested the activity of glutathione peroxidase with a specific kit (Bioassay Systems). There was no detectable glutathione peroxidase activity in our samples, so we conclude that the peroxidase activity is primarily catalase, although we cannot conclusively exclude technical issues with the assay.
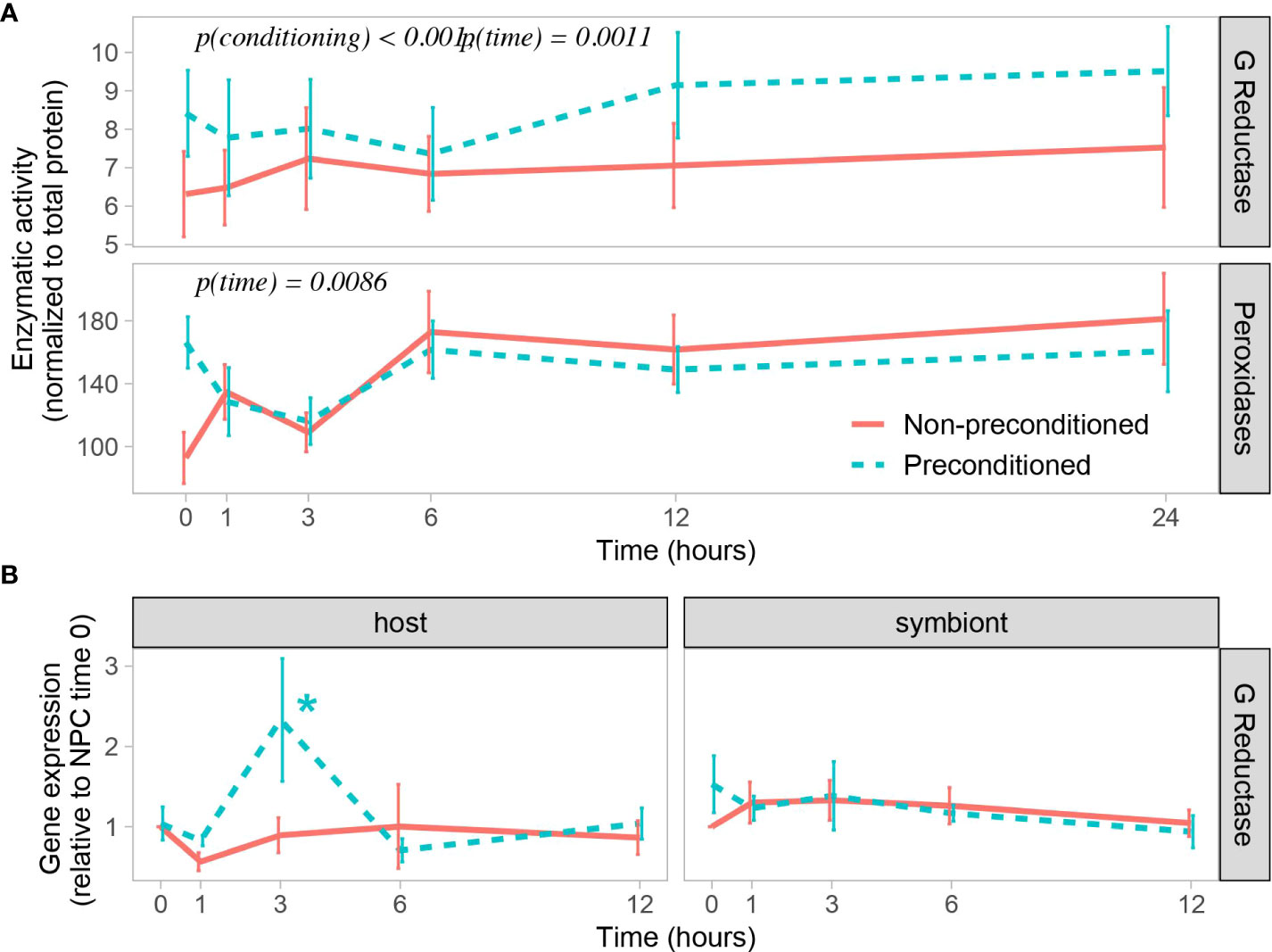
Figure 2 Enzymatic activity and gene expression during thermal stress. (A) Thermally PC corals have higher activity of glutathione reductase than NPC, but do not differ in the activity of peroxide-scavenging enzymes. Activity was measured in host cell extracts, n = 7. (B) Gene expression pattern of glutathione reductase in host and symbiont cells. Gene expression of host-derived glutathione reductase significantly increased at 3 hours of acute heat stress in PC corals, but not NPC corals. Graphs depict means with standard errors, n = 7. * represents p value < 0.05.
To examine if the observed increase in glutathione reductase activity was connected to the stimulation of gene expression and if such response is common for both partners or is host-specific, we analyzed the expression of coral host and symbiont gene in PC and NPC corals during heat stress (Figure 2B). Host glutathione reductase expression changed over time and between conditioning treatments (LMM, expression~conditioning*time + (1|coral), p(time)< 0.001, p(treatment) = 0.0098). We observed an increase in expression in PC corals shortly after the beginning of the heat stress (p(1h) = 0.0736) that peaked at 3 hours, when the expression was ~ 2-fold higher compared to NPC corals (p(3h) = 0.0015).
Symbiont glutathione reductase expression was not significantly different between conditioning treatments (p = 0.8099) and did not change over time (p = 0.1485). This suggests that glutathione reductase dynamics are driven by the coral host; however, there was no significant correlation between protein activity and gene expression for either host or symbiont cells (Figure S1), which is a common phenomenon described across the animal kingdom (reviewed in (Liu et al., 2016)).
Increased antioxidant activity protects DNA from oxidative damage
Glutathione reductase helps stabilize the reducing environment of the cell, enhancing its ROS scavenging ability and preventing cellular stress such as oxidative DNA damage (Halliwell and Gutteridge, 2015; Couto et al., 2016). To clarify whether the increase in glutathione reductase activity improves ROS protection in heat-stressed corals, we analyzed the level of oxidized guanine species (8-OHdG), markers of oxidative DNA damage (Halliwell and Gutteridge, 2015). There was a clear difference between PC and NPC corals in time (Two-Way ANOVA, 8-OHdG~conditioning*time with Tukey post-hoc testing, p(time:conditioning) = 0.0098, Figure 3). Unlike in PC corals, we observed an accumulation of 8-OHdG in NPC corals after 24 hours of acute heat stress (p(NPC) = 0.0991, p(PC) = 0.4082, p(NPC-PC, 24h) = 0.0310, p(NPC – PC, 0h) = 0.7449).
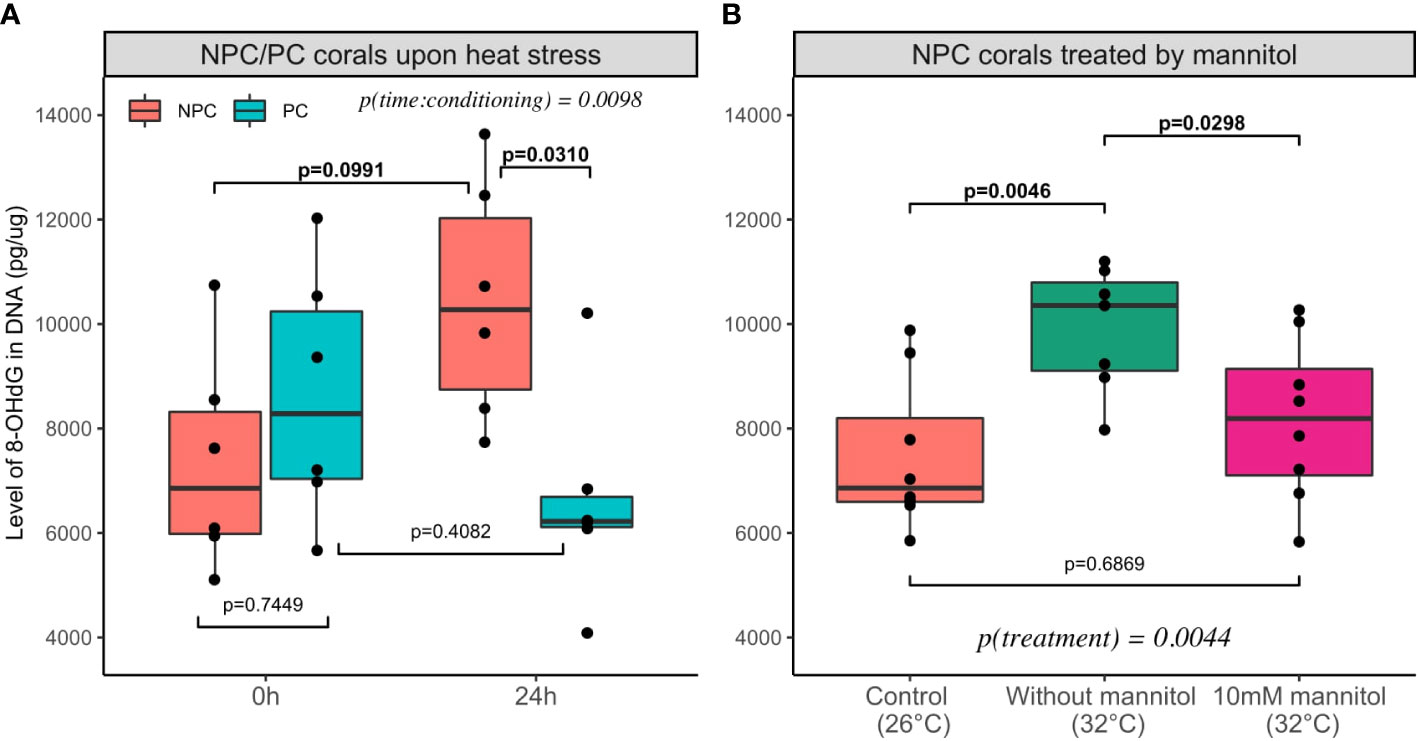
Figure 3 Oxidative DNA damage during heat stress. (A) Non-preconditioned corals accumulate DNA damage (the oxidized guanine species, 8-OHdG) but preconditioned corals do not. After 24 hours of the stress, there is a significant difference in the level of 8-OHdG between the treatments. n = 6 (B) The accumulation of 8-OHdG in non-preconditioned corals during acute heat stress is prevented by the addition of 10 mM mannitol, a non-enzymatic antioxidant. n = 8. The boxplots show median of the data, first and third quartile and respective datapoints.
To fortify the connection between antioxidants and oxidative DNA damage in corals, we treated NPC corals with 10mM mannitol during acute heat stress. Mannitol is considered a non-enzymatic antioxidant that protects plants and algae from ROS (Patel and Williamson, 2016) and prevents general DNA damage in coral tissue aggregates (Nesa and Hidaka, 2008). Again, NPC corals accumulated marks of oxidative DNA damage during heat stress (One-way ANOVA, p(treatment) = 0.0044), but the addition of 10 mM mannitol reduced this increase (One-Way ANOVA, p(no treatment:mannitol) = 0.0298, p(control:mannitol) = 0.6869, Figure 3B), demonstrating the direct connection between antioxidant defense systems and DNA damage.
Pa-BI-1 expression correlates with the expression of glutathione reductase in preconditioned corals
BI-1 (BAX inhibitor 1) is an anti-apoptotic protein that – among others – promotes cell survival by increasing the production of antioxidants through the activation of Nrf2 transcription factor in human cells (Lee et al., 2007; Harvey et al., 2009). We previously showed that PC corals increase the expression of pa-BI-1 during acute heat stress compared to NPC corals (Majerova et al., 2021). Since these observations were made on the same set of samples as our measurements of the expression of glutathione reductase (pa-GR), we examined the correlation between the gene expressions of pa-BI-1 and pa-GR. We observed a positive correlation in PC (R = 0.76, p(lm)< 0.001) but not NPC (R = 0.126, p(lm) = 0.4679) corals (Figure S2), but the relationship was not significantly different between treatments (ANCOVA, p(conditioning) = 0.2903). The strongest correlation occurred during the first 3 hours of the heat stress, where we observed a significant overexpression of both genes in PC compared to the control sample at 0h (R = 0.910, p(lm)< 0.001 but not NPC corals (R = 0.011, p(lm) = 0.9623, ANCOVA, p(conditioning) = 0.0052 (Majerova et al., 2021); and Figure 2), suggesting coral BI-1 can regulate the expression of antioxidant genes upon stress conditions in PC corals.
siRNA-mediated knockdown assay downregulates coral GFP family
We developed and validated a siRNA-mediated gene knockdown method in living adult corals by targeting proteins of the GFP family, using a common approach for developing similar technologies in non-model organisms (Cleves et al., 2018; Karabulut et al., 2019; Yuyama et al., 2021). We identified 12 predicted Pocillopora GFP-like fluorescent chromoproteins in the NCBI mRNA database and used BLOCK-iT™ RNAi Designer (Thermo Fisher Scientific) to design siRNA molecules with the best predicted success rate to inhibit expression of individual predicted GFP-like genes. We chose an siRNA duplex that had 100% sequence similarity with 4 predicted GFP-like genes, one mismatched nucleotide with 7 predicted GFP-like genes and was not complementary to 1 of the genes. We also designed RT-qPCR primers to amplify the siRNA target region of the GFP-like genes (Table S1). Both primers have either full complementarity to the predicted genes or contain one mismatched nucleotide in the central portion of the sequence. We optimized the timing of siRNA administration and coral sampling and used a control siRNA with no known target in the Pocillopora transcriptome (siNTC) to exclude the effect of the siRNA treatment itself on the studied genes and pathways. We tested several delivery methods, including PEI (Polyethylenimine, Sigma Aldrich), Lipofectamine2000 (Thermo Fisher Scientific), CaCl2 phosphate transfection, and Fuse-It (Ibidi), and found the INTERFERin (Polyplus) to be the only method which produced reliable knockdown of the target genes.
A total of 16 coral fragments from 8 different parent colonies (1-3 ramets per colony) were used for the siRNA developmental experiment. We analyzed GFP expression and fluorescence signals derived from host GFP and symbiont chlorophyl a (ChlA) for each fragment independently. We found 9 corals (representing 6 out of 8 parent colonies) in which the expression of GFP decreased by at least 20% compared to the siNTC (Figure 4A). Expression downregulation ranged from 14% to 58% and averaged 32% ± 16% (mean ± SD). Independent verification of GFP expression via confocal microscopy confirmed the GFP to ChlA ratio decreased to 73% ± 30% of controls (mean ± SD; (p(t-test) = 0.0094) and ranged from 39% to 80% (Figure 4B). Two corals showed an exception to this pattern where the GFP to ChlA ratio slightly increased despite the expression downregulation.
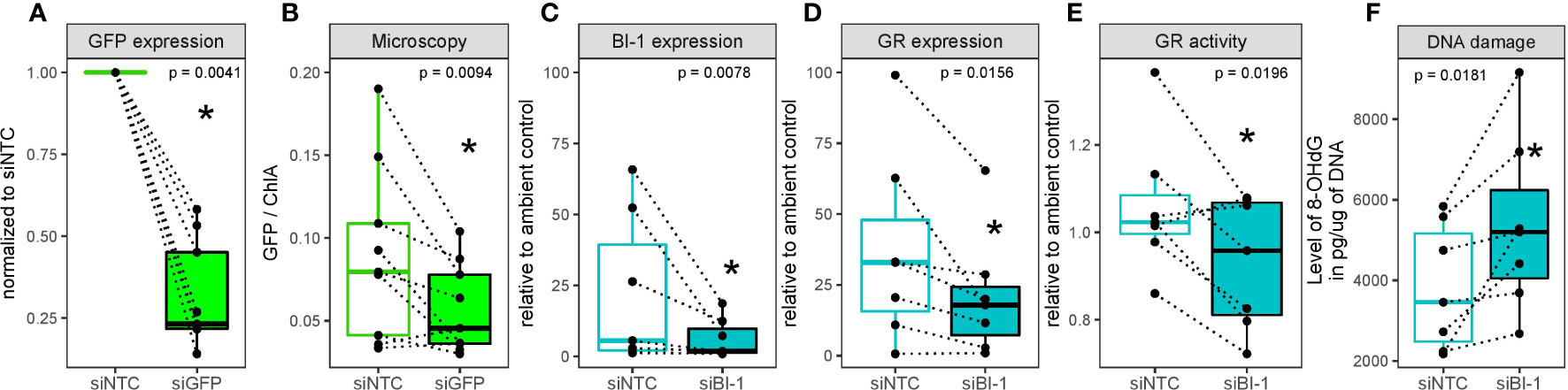
Figure 4 BI-1 overexpression in heat-stressed preconditioned corals improves DNA damage protection via regulation of glutathione reductase activity. (A) Expression of GFP in corals treated with siGPF, normalized to siNTC, n = 9 (B) Confocal microscopy analysis of GFP-derived signal normalized to symbiont-derived signal in the same corals, n = 9 (C, D) Expression of BI-1 and glutathione reductase genes, respectively, in heat-stressed PC corals is efficiently reduced after siRNA-mediated gene knockdown (siBI-1). siNTC represents PC corals treated with a negative control siRNA. Data are normalized to untreated corals at ambient temperature, n=7 (E) The activity of glutathione reductase decreases upon siBI-1 knockdown in PC corals. Data are normalized to untreated corals at ambient temperature, n = 7. (F) The level of oxidized guanine in coral DNA during heat stress increases after siBI-1 knockdown in PC corals (n = 7). Dotted lines connect paired samples from the same colony. The boxplots show median of the data, first and third quartile and respective datapoints. * represents p value < 0.05.
pa-BI-1 controls the expression of glutathione reductase in preconditioned corals
To confirm the presumed link between pa-BI-1 and pa-GR, we inhibited the expression of pa-BI-1 in heat-stressed PC corals (Figure 4C). We optimized the start of the heat stress to 48 hours post-transfection to achieve the strongest knock-down during the first hours of the acute heat stress when pa-BI-1 was most strongly overexpressed in PC corals (Majerova et al., 2021). We successfully inhibited pa-BI-1 expression in 7 out of 17 corals (similarly to siGPF, we arbitrarily set a threshold of 80% gene expression as a successful knock-down) ranging from 14% to 80% of control pa-BI-1 expression in siNTC-treated corals (47% ± 24, mean ± SD; Figure 4C). For all further analyses, we used only the 7 corals with a successful knock-down.
As expected, we observed an overexpression of both pa-BI-1 and pa-GR in heat-stressed PC corals incubated with siNTC (Figure 4C, D, siNTC) when compared to control corals (PC corals at ambient temperature). After siBI-1 knockdown (Figure 4C, siBI-1), the expression of both pa-BI-1 and pa-GR decreased (Figure 4C, D, Wilcoxon test, p(BI-1) = 0.0078, p(GR) = 0.0156), but expression was still higher than in ambient control corals (Wilcoxon test, p(BI-1) = 0.0781, p(GR) = 0.0156). There was a strong correlation between pa-BI-1 expression and pa-GR expression following the gene knockdown (lm, GR~BI-1, p = 0.0036, r (Fisher et al., 2015) = 0.48), indicating the inhibition of pa-BI-1 expression leads to a decrease in pa-GR expression.
To exclude non-specific effects of the siRNA treatment, we analyzed the correlation of pa-BI-1 expression with 4 genes (pa-HSP70, pa-Bcl-2, pa-BAK, pa-BAX) previously found to be involved in coral bleaching (Pernice et al., 2011; Tchernov et al., 2011; Majerova et al., 2021). These genes were selected because they also follow similar expression patterns as pa-BI-1 in corals upon heat stress. We also included the pa-NFKBI (NFkB inhibitor) gene that showed a differential expression profile than pa-BI-1 ( (Majerova et al., 2021), Figure S3A). pa-BAX and pa-Bcl-2 showed strong correlation with pa-BI-1 gene expression in PC corals (Figure S3B) (r(Pearson) = 0.856 and 0.726; p(lm)< 0.001) and a weaker but still considerable correlation in NPC corals (r(Pearson) = 0.642 and 0.531; p(lm)< 0.001). pa-BAK and pa-HSP70 showed a moderate correlation with pa-BI-1 in PC corals (r(Pearson) = 0.446 and 0.438; p(lm) = 0.013 and 0.0023) and – as expected – the expression of pa-NFKBI did not correlate with the expression of pa-BI-1 in any corals (r(Pearson) = 0.27 and 0.203 (for PC and NPC corals, respectively); p(lm) = 0.12 and 0.18).
We hypothesized that if the siRNA treatment is not specific to the pa-BI-1 gene or impacts the whole bleaching pathway, we would observe a shift in multiple genes involved in the coral bleaching cascade. Upon siBI-1 treatment, the expression of these genes did not change significantly (Figure S3B), supporting the specificity of the siRNA treatment to siBI-1 gene expression.
Decrease in glutathione reductase gene expression results in a decrease in enzyme activity
Changes in gene expression are not always directly mirrored in the protein level and/or activity (Liu et al., 2016), so we measured the activity of glutathione reductase in coral host tissue after siBI-1 gene knockdown. The decrease in pa-GR expression after 3 hours of heat stress was followed by a significant decrease in the enzymatic activity at 24 hours post stress (Paired t-test, p(activity) = 0.0391, Figure 4E). There was a rapid decrease in the glutathione reductase activity in 5 corals, and a very slight increase in 2 corals (Figure 4E; dotted lines connecting siNTC-siBI-1 pairs), suggesting that decreased pa-GR expression does result in decreased pa-GR activity, but there may be genotype-specific effects.
Corals with inhibited expression of pa-BI-1 are more prone to oxidative DNA damage
To examine the connection between antioxidant system and oxidative DNA damage in heat-stressed corals, we analyzed the level of oxidative DNA damage using an 8-OHdG marker in corals with pa-BI-1 knockdown and subsequent decrease in glutathione reductase expression and activity. siBI-1 treated corals accumulated significantly more oxidized guanines in DNA during acute heat stress (Figure 4F; 24 hours at 32°C, Paired t-test, p = 0.0181) when compared to the PC corals treated with control siRNA. This observation supports the hypothesis that during heat stress, corals use antioxidants to protect important cellular structures from oxidative damage.
An antioxidant responsive element lies within the promotor of coral glutathione reductase gene
In mammals, BI-1 can activate the Nrf2 transcription factor which regulates gene expressions through the cis-acting elements called antioxidant responsive elements (ARE) (Lee et al., 2007; Raghunath et al., 2018) in various Nrf2 target genes’ promoters. Nrf2 gene has not been described in reef-building corals but the Nematostella vectensis putative Nrf2 protein sequence (GenBank KU746947.1), returns blast hits (tblastn) for several uncharacterized loci in scleractinian corals (e.g., Orbicella faveolata LOC110060612, 93% query cover, 29.89% identity and 1e-43 E-value, Acropora millepora LOC114950848, 54% query cover, 33.23% identity and 2e-34 E-value, or Pocillopora damicornis, LOC113687044, 53% query cover, 30.94% identity and 1e-43 E-value) suggesting a protein with a Nrf2-like function may be present.
We searched the promoter region (within NW_020843386.1) of the glutathione reductase gene (XM_027196629.1) in Pocillopora damicornis (Cunning et al., 2018) and found an ARE-similar sequence 5’-TGACTTAGC-3’ (Banning et al., 2005; Raghunath et al., 2018) 557 bp upstream of the predicted beginning of the gene ORF. This ARE was first discovered and described in the promoter of glutathione peroxidase in human liver carcinoma cells (HepG2) at positions -76 and -387 with respect to +1 transcription start site (Banning et al., 2005). This striking resemblance suggests that the Nrf2/ARE pathway may be more evolutionarily conserved than previously thought.
Discussion
Like most organisms, corals have the ability to acclimatize to environmental change, which reduces the severity of bleaching and mortality during thermal stress (Middlebrook et al., 2008; Bellantuono et al., 2012; Palumbi et al., 2014; Bay and Palumbi, 2015; Foo and Byrne, 2016; Schoepf et al., 2019; Majerova et al., 2021). However, this natural phenomenon is genotype-specific (Dilworth et al., 2021; Drury et al., 2022) and could be lost under future climate-change scenarios (Ainsworth et al., 2016). One of the main obstacles to the application of this strategy for conservation (van Oppen et al., 2015; Committee on Interventions to Increase the Resilience of Coral Reefs et al., 2019; Drury, 2020) is our limited understanding of molecular and cellular mechanisms behind acclimatization and/or adaptation to increased temperatures. Here we show that preconditioning-based acclimatization is mediated by the interaction of the pro-life gene BI-1 and the antioxidant response, which impacts fitness via cellular phenotypes such as DNA damage.
Transcriptomic data from acclimatized corals shed light on the main gene families and cellular pathways that play a role in the bleaching process, but functional studies have been largely missing in reef-building corals (Bellantuono et al., 2012; Palumbi et al., 2014; Bay and Palumbi, 2015; Thomas et al., 2018). Recently, we showed that preconditioning in Pocillopora acuta leads to improved thermal tolerance due to modulations in the programmed cell death pathway (PCD), most likely via autophagy/symbiophagy (Majerova et al., 2021). However, the primary signals or molecular consequences of such a prolonged symbiosis maintenance under thermal stress are unclear. During heat stress, the coral host and symbionts release increased reactive oxygen species (ROS) (Suggett et al., 2008; Armoza-Zvuloni and Shaked, 2014; Roberty et al., 2015; Diaz et al., 2016; Gardner et al., 2017; Nielsen et al., 2018; Szabó et al., 2020) which can activate an array of regulatory pathways, often depending on the level of ROS accumulation (Schieber and Chandel, 2014; Redza-Dutordoir and Averill-Bates, 2016). For example, in model animals, low doses of ROS activate cell survival signaling pathways such as the unfolded protein response (UPR) or Nrf2, while high doses of ROS activate PCD (Redza-Dutordoir and Averill-Bates, 2016). We thus hypothesized that after preconditioning, the level of ROS signaling molecules in heat-stressed corals was reduced, which resulted in changes in PCD signaling.
We find that preconditioned (PC) corals with higher tolerance to thermal stress and reduced bleaching rate (Majerova et al., 2021) have higher activity of glutathione reductase, but not peroxide-scavenging enzymes, in the host tissue relative to non-preconditioned (NPC) corals. Gene expression analysis suggests that the observed increase in glutathione reductase activity is due to the specific response of the coral host but cannot be explained simply and entirely by the expression rate. The levels of mRNA do not always correlate with cellular protein levels and the relationship between the two strongly varies during dynamic transitions such as short-term acclimatization (reviewed in (Liu et al., 2016)), a pattern which was previously observed in heat-stressed Symbiodiniaceae (Krueger et al., 2015b) While posttranscriptional and posttranslational modifications may positively impact the activity, turnover rate, or localization of the antioxidant in PC corals in ambient conditions, our results suggest the rapid induction of pa-GR gene expression in PC corals is potentially a major contributor to the observed activity differences upon acute heat stress.
Glutathione is a non-enzymatic antioxidant that exists in reduced (GSH) and oxidized (GSSG) form in the cell (reviewed in (Halliwell and Gutteridge, 2015)). GSH neutralizes ROS while being oxidized to glutathione disulfide (GSSG); this oxidized state is converted back to the reduced state by glutathione reductase (GR). Under normal conditions, over 90% of the glutathione pool is maintained as GSH by GR activity, so GR is directly responsible for maintaining the reducing environment of the cell and for ROS scavenging. The level of GSH and/or the activity of enzymes involved in the glutathione redox cycle are inversely associated with the oxidative DNA damage (Lenton et al., 1999; Dannenmann et al., 2015). ROS-related oxidative DNA damage can stem from heat stress in mice, human, plant and fish cells (Heise, 2006; Purschke et al., 2010; Cvjetko et al., 2014; Liu et al., 2015; Kantidze et al., 2016; Houston et al., 2018), and increased DNA damage was observed in coral tissue explants exposed to elevated temperatures or to direct sunlight (Nesa and Hidaka, 2008; Nesa et al., 2012). Inversely, heat acclimation prevents accumulation of 8-OHdG markers in blood cells of navy boiler tenders exposed to high heat during work (Huang et al., 2012).,
We tested the level of 8-OHdG, markers of oxidative DNA damage, in PC and NPC corals after 24 hours of heat stress and found that while NPC corals accumulate these markers, PC corals do not. Previous experiments showed that the addition of the exogeneous antioxidant mannitol can reduce DNA damage in heat-stressed coral cell aggregates (Nesa and Hidaka, 2008), so we used this antioxidant on a new set of NPC corals to solidify the link between the antioxidant system and DNA damage in the whole adult coral organism, demonstrating that the antioxidant system is the major contributor to the differences in DNA damage between PC and NPC corals.
DNA damage also triggers diverse cell death pathways, including autophagy (Surova and Zhivotovsky, 2013), supporting our previous results showing preconditioning improves coral thermal tolerance via modulations in the autophagy pathway (Majerova et al., 2021). BI-1 (BAX-1 inhibitor), a pro-survival PCD gene involved in the regulation of PCD pathways, was shown to reduce ROS accumulation in vertebrates and to activate Nrf2, which is a transcription factor of various antioxidants including glutathione reductase (Lee et al., 2007; Robinson et al., 2011). Interestingly, we saw an upregulation of pa-BI-1 in PC corals upon heat stress which peaked at 3 h (Majerova et al., [[NoYear]]) in parallel with host glutathione reductase expression. We compared the expression rates of pa-BI-1 and pa-GR in PC and NPC corals and strikingly, they are highly correlated in PC corals in the early phase of the heat stress response (< 6 hours), but not in NPC corals (Figure S2). We hypothesize that preconditioning may enable pa-BI-1 to effectively regulate the expression of pa-GR through epigenetic modifications of the pa-GR regulatory elements. DNA methylation patterns vary between corals living in different environments and is dynamic over time in corals exposed to environmental changes, likely enabling fine tuning of expression in response to various conditions (Putnam et al., 2016; Liew et al., 2018; Liew et al., 2020; Rodríguez-Casariego et al., 2020). Future experiments should investigate this link between preconditioning, epigenetic modifications, and expression of particular genes in corals.
To demonstrate the functional correlation between pa-BI-1 and pa-GR, we developed a protocol for siRNA-mediated gene knockdown in living adult corals. Gene knockdown assays have shown limited efficiency in cnidarians and have only been used in a few studies, representing a major obstacle for functional studies of these non-model organisms. Existing protocols in cnidarians were not technically feasible in adult reef-building corals due to the skeletal structure, and rely on microinjection of juveniles or electroporation of anemones as the delivery technique (Yasuoka et al., 2016; Cleves et al., 2018; Karabulut et al., 2019; Yuyama et al., 2021). Other studies using liposomal compounds transferred long dsRNA into anemones or coral larva, where the animal’s RNAi machinery was allowed to process it into siRNA (Dunn et al., 2007; Dunn et al., 2007; Yuyama et al., 2021).
In this experiment, we used processed siRNA designed to specifically target the gene of interest and delivered it to the coral tissue using a lipidic vesicle INTERFERin (Polyplus) which proved to be the most efficient and the only repeatable method of those tested. We first targeted the family of green fluorescent proteins, a standard strategy to validate gene-knockdown in non-model organisms owing to its ease of monitoring success in real time (Cleves et al., 2018; Karabulut et al., 2019; Yuyama et al., 2021). We were able to achieve a significant downregulation of GFP proteins in the majority of test corals and we hypothesize that coral mucus likely interferes with the siRNA transfection procedure in some individuals, meaning that individuals undergoing siRNA-involved experiments must be first tested for the successful knockdown before further functional analyses take place. Additionally, the knock-down efficiency we achieved allowed the study of the function of genes that are upregulated during specific cellular and molecular processes (after the overexpressed gene is knocked down close to the basal level) but may not be applicable for complete loss-of-function studies.
Our siRNA methodology allowed us to functionally connect BI-1 gene expression with the coral antioxidant system by inhibiting pa-BI-1 overexpression in preconditioned corals during acute heat stress. We manipulated the gene in 8 out of 17 corals, which decreased pa-GR expression and lead to a decline in pa-GR activity, demonstrating that pa-BI-1 regulates gene expression of pa-GR in preconditioned corals. The decreased expression of pa-GR paralleled decreased glutathione reductase antioxidant activity in siBI-1 corals, leading to more oxidative damage than corals treated with a control siRNA (siRNA with no known target in P. acuta), supporting the hypothesis that antioxidants prevent cellular oxidative damage in corals.
In vertebrate models, BI-1 was also found to regulate expression of genes coding for antioxidants through the Nrf-2 transcription factor (Lee et al., 2007; Harvey et al., 2009). Although Nrf2 has not been described in reef-building corals, a homolog of the Nrf-2 gene was identified and annotated in the anemone Nematostella vectensis (GenBank KU746947.1 (Layden et al., 2016),), where the Nrf-2 mediated oxidative stress response pathway was activated in its symbiotic but not apo-symbiotic morph during thermal stress (Weizman and Levy, 2019). Nrf2 regulates the expression of antioxidant genes via binding to the so-called ARE (antioxidant response element) cis-elements located upstream of the transcription start site (Raghunath et al., 2018). In the putative promoter of coral glutathione reductase gene, we found an ARE similar to one described in the promoter of the glutathione peroxidase gene in human liver cells (Banning et al., 2005), suggesting the Nrf2/ARE pathway is conserved in cnidarians, where it controls the expression of antioxidant genes during environmental stress response. We thus propose this pathway could also connect BI-1 and glutathione reductase in Pocillopora acuta, but additional experiments are necessary to confirm this hypothesis. We propose that next steps should include i) the functional study of relevant genes’ knockdown in coral bleaching (when the loss of symbionts would be analyzed upon siRNA treatment), ii) exploration of changes in epigenetic regulation upon preconditioning, and iii) analysis of ROS release in coral cells/tissue upon preconditioning.
This work describes cellular and molecular principles of coral symbiosis maintenance under heat stress and how it is modulated during acclimatization, which is a critical ecological pathway to the long-term persistence of coral reefs. The data presented here show that pre-exposure to thermal stress improves the ability of corals to maintain cellular redox homeostasis through BI-1-mediated glutathione reductase overexpression. This prevents the accumulation of oxidative stress markers 8-OHdG, avoids the activation of programmed cell death and results in prolongated coral-algal symbiosis. Conversely, heat-stressed corals may experience high ROS, subsequent oxidative stress, activation of cell death pathways, and bleaching.
Describing and understanding resilient trails in stony corals is fundamental for the development and application of reef restauration projects based on selective principles such as (human)-assisted evolution (van Oppen et al., 2015; Committee on Interventions to Increase the Resilience of Coral Reefs et al., 2019). Invention of new methodology that allows functional studies in adult reef-building corals facilitates this goal and significantly contributes to our growing knowledge of the response of organisms to environmental stress and their ability to adapt to future climate scenarios.
Materials and methods
Collections, experimental setup, and preconditioning
Seven colonies of P. acuta were collected in spring 2018 at 3 different sites and depths ranging between 1 to 4m across Kāne’ohe Bay, Hawai’i to maximize the likelihood that distinct genotypes were collected (21.45036 N 157.79563 W; 21.44443 N 157.79536 W; 21.44007 N 157.79174 W). Colonies were held in flow-through tanks under natural light and temperature conditions, unless stated otherwise. Experimental treatments were conducted as described in Majerova et al. (2021). Briefly, corals were split in half and allocated into preconditioning (PC) and control (NPC) treatment tanks, and PC corals were exposed to a 29°C treatment for 72h before returning to ambient (26°C) while NPC corals were maintained at 26°C (Figure 1A). These temperatures represent the typical temperature peaks during summer months and average pre-summer temperature in Kāne’ohe Bay, respectively (NOAA measurements, Mokuoloe Station #1612480, and [Majerova et al., 2021)]. Corals were fragmented into ~ 5 cm nubbins, mounted onto aragonite plugs and reallocated into treatment tanks. After two weeks at 26°C, corals were exposed to a 32°C treatment or control and sampled at 0, 1, 3, 6, 12, and 24 hours (0h is a pre-exposure timepoint and was sampled at 8 am). A whole nubbin was sub-sampled and used at each timepoint. Samples were stored immediately at -80°C. Bleaching rate was assessed as the shift in symbiont-to-host signal ratio with time-lapse confocal microscopy (Zeiss LSM-710) as described in Majerova et al. (2021) One separate nubbin was used for confocal microscopy analysis repetitively at each timepoint.
Antioxidant activity assays
Samples previously stored at -80°C were homogenized in ice cold extraction buffer (100 mM Tris-HCl, 20 mM EDTA, pH 7.5) in Qiagen TissueLyser (30 s-1 for 20 s) with acid-washed glass beads (Sigma). The symbiont and host cells were separated with low-speed centrifugation (800g, 5 min, 4°C) and the supernatant was then sonicated for 3 min. Cell debris were pelleted by centrifugation (14,000g, 10 min, 4°C) and whole cell protein extract concentration was measured using Qubit Protein Assay Kit (Thermo Fisher Scientific). Protein extracts were immediately used for EnzyChrom Catalase Assay Kit and EnzyChrom Glutathione Reductase Assay Kit (BioAssay Systems), respectively. The working protein concentration was optimized prior to the experiment to ensure the measured activity was within the range of kit detection limits. We used 1340 ng and 300 ng of the whole cell protein extract in Catalase Assay Kit and Glutathione Reductase Assay Kit, respectively, to normalize the enzymatic activity to total protein. Each analysis included one sample per timepoint and conditioning per colony (n(colony)=7). Samples were extracted in small batches (up to 12 samples per run) to decrease extraction time, and control and treatment samples of the same colony were always extracted together to minimize the chance that any observed difference is due to different sample processing.
The Catalase Assay Kit is not specific to catalase and measures activity of all peroxide-scavenging antioxidants, so we used the EnzyChrom Glutathione Peroxidase Assay Kit (BioAssay Systems) to distinguish activity of different peroxide-scavenging antioxidants. All assays (total protein 2 – 10 μg) were below the detection limit of the kit.
Gene expression analysis
Gene expression was analyzed as described in Majerova et al. (2021). Briefly, RNA was extracted via RiboZol RNA Extraction Reagent (VWR Life Science) with DNase I step between phenol-chloroform extraction and ethanol precipitation. Two-step Reverse transcription quantitative PCR (RT-qPCR) analysis was executed as follows: 1μg of RNA was reversely-transcribed with High-Capacity cDNA reverse transcription kit (Thermo Fisher Scientific) and the qPCR reactions were run in 12 μl with PowerUp™ SYBR™ Green Master Mix (Applied Biosystems) for 40 cycles according to the manufacturer’s instructions. Efficiency of each primer pair was tested with a dilution curve followed by melting curve analysis. Profiles of four genes for each organism (actin, elongation factor 1a, calmodulin, and adenosylhomocysteinase for the host and actin, tubulin, ribosomal protein S4, and S-adenosyl-l-methionine synthetase for the symbiont) under different treatments were compared with Best Keeper software (Pfaffl et al., 2004) and pa-EF-1 (elongation factor 1a, P. acuta), and SAM (S-adenosyl-l-methionine synthetase, Symbiodinium sp.) showed the highest expression stability upon heat stress and were chosen as the reference genes by the software algorithm. All primer sequences are listed in Table S1. Expression of target genes was calculated relative to the non-preconditioned coral at time 0 with ΔΔCt method.
Each analysis included one sample per timepoint and conditioning treatment per colony (n(colony)=7). Sequences of all primers used in this study are listed in Table S1.
Oxidative DNA damage assay
To assess the impact of oxidative stress on DNA, we analyzed the level of 8-Hydroxy-2’-deoxyguanosine (8-OHdG) in PC and NPC corals as a proxy for cumulative oxidative DNA damage. 2 fragments from 6 corals in each treatment were sampled prior to and after 24 hours of thermal stress (as described above), frozen and kept at -80°C.
We used a custom extraction protocol to extract DNA from host cells after symbiont and host cells were separated as described above. After the low-speed centrifugation, SDS (0.5% final concentration) was added to the supernatant containing host cells and nuclei and incubated at 58°C for 15 min. Then, proteinase K (0.5 mg/ml final concentration) was added and incubated at 58°C for 2 h. After incubation, samples were cooled to room temperature and potassium acetate was added to a final concentration 0.5 M. Samples were centrifuged (14,000g, 10 min, 4°C) and the supernatant was precipitated by isopropanol (1:0.7 ratio). After precipitation, the DNA pellet was resuspended in water and the sample was treated by RNAse A for 15 min. DNA was purified by phenol-chloroform extraction followed by ethanol precipitation (overnight, -20°C) and resuspended in water. 500 ng of total genomic DNA in 20 μl was denatured at 95°C for 5 min, rapidly cooled down on ice, digested to single nucleotides by 20 U of Nuclease P1 (NEB) at 37°C for 30 min and dephosphorylated by 1 U of Shrimp Alkaline Phosphatase (NEB) for 30 min at 37°C. All the enzymes were inactivated at 75°C for 10 min. DNA was then diluted 1:5 in 1x Assay buffer and processed according to the kit instructions (DNA Damage Competitive ELISA Kit, Invitrogen). The initial DNA concentration used in the assay was optimized to ensure the resulting values fall within the optimum range of kit detection limits (between Std3 (2000 pg/ml) and Std6 (250 pg/ml)). We used MyCurveFit.com software to model the standard curve and to predict result values. The analysis was conducted using 2 samples per timepoint, treatment and conditioning per colony using 6 colonies in total. The results from biological replicates were averaged before statistical analysis.
Corals with increased glutathione reductase activity are less prone to oxidative DNA damage accumulation, so we decided to test the impact of exogenous antioxidants to heat-stressed non-preconditioned corals. For this analysis, a new set of corals was collected from Kāne’ohe Bay in spring 2020 and prepared as describe above. This new set of corals was used for the following mannitol experiment and the siRNA validation and testing experiments (see below).
To analyze the impact of exogenous antioxidant to oxidative DNA damage, we treated NPC nubbins with 10 mM mannitol (Nesa and Hidaka, 2008) or a seawater control for the duration of the heat stress experiment (6 NPC and 6 PC corals, 2 nubbins per colony per treatment) in a closed-system tank under natural light. All coral fragments were sampled after 24h of acute heat stress (32°C), frozen and kept at -80°C. DNA damage assay was conducted as described above.
siRNA design
BLOCK-iT™ RNAi Designer (Thermo Fisher Scientific) was used to design siRNA for the P. damicornis BI-1 mRNA sequence (XM_027189407.1 (Cunning et al., 2018),) and predicted GFP-like mRNA (XM_027188377.1). In order to silence the most GFP-like genes possible, we aligned all the proposed siRNA sequences to the set of 12 predicted GFP-like proteins we found in the Pocillopora transcriptome database in NCBI (XM_027191428.1, XM_027191431.1, XM_027188381.1, XM_027195143.1, XM_027188383.1, XM_027188379.1, XM_027188378.1, XM_027188377.1, XM_027188376.1, XM_027195136.1, XM_027188380.1, XM_027188372.1) and chose the sequence with the highest alignment to the most proteins. For BI-1 silencing, we chose the highest-ranking siRNA sequence containing BCD Tuschl’s patterns (Elbashir, 2001) and targeting region 194- 212 of the 1437 bp long mRNA sequence. Control siRNA (siNTC) was designed to contain the same nucleotide composition as siBI-1 but having no known target in the P. damicornis mRNA sequence database. All siRNA molecules were synthesized by Sigma-Aldrich. Sequences of all siRNA molecules are listed in Table S1.
siRNA-mediated knockdown
PC coral fragments (~ 3 cm long) were placed into 20 ml cultivation vials, completely submerged into flow-through tanks with ambient temperature seawater and left to acclimatize overnight. The next day, vials were moved into precise temperature-controlled water bath, the seawater was carefully removed without disturbing corals and the siRNA transfection was carried out according to the manufacturer’s instructions (INTERFERin® transfection reagent, Polyplus). siRNA transfection mix (20 µl of 10nM siRNA and 16 µl INTERFERin® reagent in 250 μl 0.2 nm filtered seawater) was pipetted directly on the exposed coral and after ~ 2 min, 5 ml of 0.2 nm filtered seawater was added to fully cover the nubbin and coral was incubated at ambient temperature for 6 hours. After this time, corals were fully submerged into a flow-through tank with ambient temperature seawater for two days. For siBI-1 experiment, 48 hours after the beginning of the siRNA transfection, corals were exposed to an acute heat stress (32°C, ramping speed of ~1°C per 10 min). At 3h post-stress, each coral was sampled for the gene expression analysis and at 24h hours post-stress for glutathione reductase activity and DNA damage assay, using the same fragment for both timepoints. The sampling consumed the fragment.
Confocal microscopy
Corals for the siGFP experiment were imaged as described in Majerova et al. (2021), with the following adjustments. Each coral nubbin was scanned three times with EC Plan-Neofluar 2.5x/0.075 objective using Z-Stack mode with fixed 12 layers spanning tissue thickness of approximately 0.1 mm. Images were then processed in Fiji software (ImageJ) (Schindelin et al., 2012) using Zprojection set to Sum Slices to display the sum of the symbiont and GFP-derived signals through all the tissue layers. We then measured the signal intensity in 10 circular regions of interest (ROIs) spreading to the whole fragment with the exception to polyp mouths, tentacles, and clearly injured tissue (after cutting the nubbin, e.g.) and compared the ratio of GFP signal to the symbiont-derived signal (chlorophyl a) between siGFP and siNTC treated corals. Polyp mouths and tentacles were avoided for two principal reasons, i) corals tend to actively stretch and retract tentacles during microscopy which makes scanning in z-stack mode very difficult and results in blurry Zprojections, ii) GFP signal is much higher in these areas, so it is not possible to use the same microscopy settings while achieving an appropriate signal intensity range.
Statistical analyses
All statistical analyses were conducted in R (R Core Team, 2020) using the following libraries (alphabetically): bestNormalize, car, cowplot, dplyr, egg, emmeans, ggfortify, ggplot2, ggpmisc, ggpubr, ggsignif, lme4, longpower, mgcv, plotrix, readxl, tidyr, tidyverse, viridis. Datasets were tested for normality (histogram plot) and heteroscedascity (Levene test) and normalized as needed using a logarithmic transformation or the BestNormalize function in R.
We analyzed antioxidant activity after normalizing data to the initial control activity (NPC, time 0) for each colony. We used a generalized mixed model (lmer function, package lme4) with conditioning and time as main effects and colony as a random effect with a Tukey post-hoc testing for each timepoint.
We analyzed gene expression after normalizing data to the initial control expression for each colony. We used a generalized mixed model to examine the normalized expression of each gene separately, using conditioning, treatment and time as main effects and colony as a random effect. We estimated least-squares means and compared between treatments for each timepoint using bonferroni adjusted p-values.
To analyze DNA damage, we ran a two-way ANOVA to compare the level of 8-OHdG (pg per μl DNA) of PC and NPC corals with time and conditioning treatment as main effects and a Tukey HSD for post-hoc analysis. The effect of mannitol treatment to DNA damage was tested by one-way ANOVA with treatment (control, heat stress, heat stress with mannitol) as variable followed by a Tukey post-hoc testing.
We calculated Pearson correlation coefficients and variance explained using linear regression for the relationships between a) gene expression and enzymatic activity and b) BI-1 and glutathione reductase expression. We used ANCOVA to compare the BI-1 and GR expression correlation among preconditioning treatments.
The effect of siRNA-mediated knockdown was analyzed using one-tailed t-test for the GFP expression in the siGFP experiment, two-tailed t-test for siGFP microscopy, and two-tailed paired samples Wilcoxon test on data normalized to control (ambient temperature, non-treated) coral samples for the siBI-1 experiment. Antioxidant activity data in siBI-1 experiment were also first normalized to control coral samples and then subjected to paired two-tailed t-test. DNA damage analysis in siRNA-treated corals was analyzed with paired two-tailed t-test on raw data.
Statistical analyses are summarized in Table S2.
Data availability statement
The original contributions presented in the study are included in the article/Supplementary Material. Further inquiries can be directed to the corresponding author.
Author contributions
EM conceived the experiments, conducted research, analyzed data, and wrote the manuscript. CD analyzed data and wrote the manuscript. All authors contributed to the article and approved the submitted version.
Funding
This work was funded by the Paul G. Allen Family Foundation, the Annenberg Foundation, and the National Science Foundation (IOS-2041401)
Acknowledgments
We would like to thank Shayle Matsuda, Fiona C. Carey, Filip Blaštík, and Vojtěch Prokůpek for their help with coral collection and siRNA knock-down optimization tests, and Tomáš Buryška for advice on execution of enzymatic assays. We thank the ToBo Lab (HIMB) for access to instruments. We dedicate this manuscript to Ruth Gates, who inspired us to use molecular tools to understand the coral bleaching crisis. This work was funded by the Paul G. Allen Family Foundation, the Annenberg Foundation, and the National Science Foundation (IOS-2041401). Corals were collected under SAP-2020-25 to HIMB. This is HIMB contribution 1904 and SOEST contribution 11570.
Conflict of interest
The authors declare that the research was conducted in the absence of any commercial or financial relationships that could be construed as a potential conflict of interest.
Publisher’s note
All claims expressed in this article are solely those of the authors and do not necessarily represent those of their affiliated organizations, or those of the publisher, the editors and the reviewers. Any product that may be evaluated in this article, or claim that may be made by its manufacturer, is not guaranteed or endorsed by the publisher.
Supplementary material
The Supplementary Material for this article can be found online at: https://www.frontiersin.org/articles/10.3389/fmars.2022.971332/full#supplementary-material
Supplementary Table 1 | List of primers and siRNAs used in the study.
Supplementary Table 2 | List of statistical methods and codes used in the manuscript.
Supplementary Figure 1 | Correlation of glutathione reductase activity and gene expression across all timepoints. There is no apparent correlation between the gene expression in host or symbiont cells with the activity of the enzyme. Raw expression is calculated as 2-ΔCt;ΔCt = Ct(target) – Ct(reference), raw activity is measured in U/L. n = 6.
Supplementary Figure 2 | Expressions of BI-1 and glutathione reductase correlate in corals after preconditioning at ambient temperature and during early hours of an acute heat stress. Non-preconditioned (NPC) corals are depicted in red, preconditioned (PC) corals in cyan. All expression levels are normalized to the control coral (NPC at ambient temperature) using 2-ΔΔCt formula. rP stands for Pearson correlation coefficient, p for p-value.
Supplementary Figure 3 | (A) Correlation of of pa-BI-1 gene expression with other genes involved in bleaching pathway. (B) Expression of these genes after pa-BI-1 knockdown. Non-preconditioned (NPC) corals are depicted in red, preconditioned (PC) corals in cyan. All expression levels are normalized to the control coral (NPC at ambient temperature) using 2-ΔΔCt formula. rP stands for Pearson correlation coefficient, p for p-value. The boxplots show median of the data, first and third quartile and respective datapoints.
References
Ainsworth T. D., Heron S. F., Ortiz J. C., Mumby P. J., Grech A., Ogawa D., et al. (2016). Climate change disables coral bleaching protection on the great barrier reef. Science 352, 338–342. doi: 10.1126/science.aac7125
Alderdice R., Hume B. C. C., Kühl M., Pernice M., Suggett D. J., Voolstra C. R. (2022). Disparate inventories of hypoxia gene sets across corals align with inferred environmental resilience. Front. Mar. Sci. 9, 834332. doi: 10.3389/fmars.2022.834332
Armoza-Zvuloni R., Shaked Y. (2014). Release of hydrogen peroxide and antioxidants by the coral <i<Stylophora pistillata</i< to its external <i<milieu</i<. Biogeosciences 11, 4587–4598. doi: 10.5194/bg-11-4587-2014
Banning A., Deubel S., Kluth D., Zhou Z., Brigelius-Flohé R. (2005). The GI-GPx gene is a target for Nrf2. Mol. Cell. Biol. 25, 4914–4923. doi: 10.1128/MCB.25.12.4914-4923.2005
Bay R. A., Palumbi S. R. (2015). Rapid acclimation ability mediated by transcriptome changes in reef-building corals. Genome Biol. Evol. 7, 1602–1612. doi: 10.1093/gbe/evv085
Bellantuono A. J., Granados-Cifuentes C., Miller D. J., Hoegh-Guldberg O., Rodriguez-Lanetty M. (2012). Coral thermal tolerance: Tuning gene expression to resist thermal stress. PloS One 7, e50685. doi: 10.1371/journal.pone.0050685
Bielmyer-Fraser G. K., Patel P., Capo T., Grosell M. (2018). Physiological responses of corals to ocean acidification and copper exposure. Mar. pollut. Bull. 133, 781–790. doi: 10.1016/j.marpolbul.2018.06.048
Brambilla V., Barbosa M., Dehnert I., Madin J., Maggioni D., Peddie C., et al. (2022). Shaping coral traits: Plasticity more than filtering. Mar. Ecol. Prog. Ser. 692, 53–65. doi: 10.3354/meps14080
Cleves P. A., Strader M. E., Bay L. K., Pringle J. R., Matz M. V. (2018). CRISPR/Cas9-mediated genome editing in a reef-building coral. Proc. Natl. Acad. Sci. 115, 5235–5240. doi: 10.1073/pnas.1722151115
Committee on Interventions to Increase the Resilience of Coral Reefs, Ocean Studies Board, Board on Life Sciences, Division on Earth and Life Studies, National Academies of Sciences, Engineering, and Medicine (2019). A research review of interventions to increase the persistence and resilience of coral reefs (Washington, DC: The National Academies Press). doi: 10.17226/25279
Couto N., Wood J., Barber J. (2016). The role of glutathione reductase and related enzymes on cellular redox homoeostasis network. Free Radic. Biol. Med. 95, 27–42. doi: 10.1016/j.freeradbiomed.2016.02.028
Cunning R., Bay R. A., Gillette P., Baker A. C., Traylor-Knowles N. (2018). Comparative analysis of the pocillopora damicornis genome highlights role of immune system in coral evolution. Sci. Rep. 8, 16134. doi: 10.1038/s41598-018-34459-8
Cvjetko P., Balen B., Stefanic P., Debogovic L., Pavlica M., Klobucar G. I. V.. (2014). Dynamics of heat-shock induced DNA damage and repair in senescent tobacco plants. Biol. Plant 58, 71–79. doi: 10.1007/s10535-013-0362-9
Dannenmann B., Lehle S., Hildebrand D. G., Kübler A., Grondona P., Schmid V., et al. (2015). High glutathione and glutathione peroxidase-2 levels mediate cell-Type-Specific DNA damage protection in human induced pluripotent stem cells. Stem Cell Rep. 4, 886–898. doi: 10.1016/j.stemcr.2015.04.004
Diaz J. M., Hansel C. M., Apprill A., Brighi C., Zhang T., Weber L., et al. (2016). Species-specific control of external superoxide levels by the coral holobiont during a natural bleaching event. Nat. Commun. 7, 13801. doi: 10.1038/ncomms13801
Dilworth J., Caruso C., Kahkejian V. A., Baker A. C., Drury C. (2021). Host genotype and stable differences in algal symbiont communities explain patterns of thermal stress response of montipora capitata following thermal pre-exposure and across multiple bleaching events. Coral Reefs 40, 151–163. doi: 10.1007/s00338-020-02024-3
Dixon G., Abbott E., Matz M. (2020). Meta-analysis of the coral environmental stress response: Acropora corals show opposing responses depending on stress intensity. Mol. Ecol. 29, 2855–2870. doi: 10.1111/mec.15535
Drury C. (2020). Resilience in reef-building corals: The ecological and evolutionary importance of the host response to thermal stress. Mol. Ecol. 29, 448–465. doi: 10.1111/mec.15337
Drury C., Dilworth J., Majerová E., Caruso C., Greer J. B. (2022). Expression plasticity regulates intraspecific variation in the acclimatization potential of a reef-building coral. Nat. Commun 13, 4790. doi: 10.1038/s41467-022-32452-4
Dunn S. R., Phillips W. S., Green D. R., Weis V. M. (2007). Knockdown of actin and caspase gene expression by RNA interference in the symbiotic anemone. Aiptasia pallida. Biol. Bull. 212, 250–258. doi: 10.2307/25066607
Dunn S. R., Schnitzler C. E., Weis V. M. (2007). Apoptosis and autophagy as mechanisms of dinoflagellate symbiont release during cnidarian bleaching: every which way you lose. Proc. R. Soc B Biol. Sci. 274, 3079–3085. doi: 10.1098/rspb.2007.0711
Eakin C. M., Sweatman H. P. A., Brainard R. E. (2019). The 2014–2017 global-scale coral bleaching event: insights and impacts. Coral Reefs 38, 539–545. doi: 10.1007/s00338-019-01844-2
Elbashir S. M. (2001). Functional anatomy of siRNAs for mediating efficient RNAi in drosophila melanogaster embryo lysate. EMBO J. 20, 6877–6888. doi: 10.1093/emboj/20.23.6877
Fisher R., O’Leary R. A., Low-Choy S., Mengersen K., Knowlton N., Brainard R. E., et al. (2015). Species richness on coral reefs and the pursuit of convergent global estimates. Curr. Biol. 25, 500–505. doi: 10.1016/j.cub.2014.12.022
Foo S. A., Byrne M. (2016). “Acclimatization and adaptive capacity of marine species in a changing ocean,” in Advances in marine biology, vol. 74. (Elsevier), 69–116. doi: 10.1016/bs.amb.2016.06.001
Gardner S. G., Raina J.-B., Ralph P. J., Petrou K. (2017). Reactive oxygen species (ROS) and dimethylated sulphur compounds in coral explants under acute thermal stress. J. Exp. Biol. 220, 1787–1791. doi: 10.1242/jeb.153049
Gregg T. M., Mead L., Burns J. H. R., Takabayashi M. (2015). “Puka mai he KoA’: The significance of corals in Hawaiian culture,” in Ethnobiology of corals and coral reefs. Eds. Narchi N., Price L. L. (Switzerland: Springer International Publishing), 103–115. doi: 10.1007/978-3-319-23763-3_7
Halliwell B., Gutteridge J. M. C. (2015). Free radicals in biology and medicine (United Kingdom: Oxford University Press). doi: 10.1093/acprof:oso/9780198717478.001.0001
Harvey C. J., Thimmulappa R. K., Singh A., Blake D. J., Ling G., Wakabayashi N., et al. (2009). Nrf2-regulated glutathione recycling independent of biosynthesis is critical for cell survival during oxidative stress. Free Radic. Biol. Med. 46, 443–453. doi: 10.1016/j.freeradbiomed.2008.10.040
Heise K. (2006). Oxidative stress during stressful heat exposure and recovery in the north Sea eelpout zoarces viviparus l. J. Exp. Biol. 209, 353–363. doi: 10.1242/jeb.01977
Hillyer K. E., Dias D. A., Lutz A., Wilkinson S. P., Roessner U., Davy S. K.. (2017). Metabolite profiling of symbiont and host during thermal stress and bleaching in the coral acropora aspera. Coral Reefs 36, 105–118. doi: 10.1007/s00338-016-1508-y
Houston B. J., Nixon B., Martin J. H., De Iuliis G. N., Trigg N. A., Bromfield E. G., et al. (2018). Heat exposure induces oxidative stress and DNA damage in the male germ line†. Biol. Reprod. 98, 593–606. doi: 10.1093/biolre/ioy009
Huang Y.-K., Lin C.-W., Chang C.-C., Chen P.-F., Wang C.-J., Hsueh Y.-M., et al. (2012). Heat acclimation decreased oxidative DNA damage resulting from exposure to high heat in an occupational setting. Eur. J. Appl. Physiol. 112, 4119–4126. doi: 10.1007/s00421-012-2401-1
Hughes T. P., Anderson K. D., Connolly S. R., Heron S. F., Kerry J. T., Lough J. M., et al. (2018). Spatial and temporal patterns of mass bleaching of corals in the anthropocene. Science 359, 80–83. doi: 10.1126/science.aan8048
Hughes D. J., Alderdice R., Cooney C., Kühl M., Pernice M., Voolstra C. R., et al. (2020). Coral reef survival under accelerating ocean deoxygenation. Nat. Clim. Change 10, 296–307. doi: 10.1038/s41558-020-0737-9
Kantidze O. L., Velichko A. K., Luzhin A. V., Razin S. V. (2016). Heat stress-induced DNA damage. Acta Naturae 8, 75–78. doi: 10.32607/20758251-2016-8-2-75-78
Karabulut A., He S., Chen C.-Y., McKinney S. A., Gibson M. C. (2019). Electroporation of short hairpin RNAs for rapid and efficient gene knockdown in the starlet sea anemone, nematostella vectensis. Dev. Biol. 448, 7–15. doi: 10.1016/j.ydbio.2019.01.005
Kenkel C. D., Matz M. V. (2017). Gene expression plasticity as a mechanism of coral adaptation to a variable environment. Nat. Ecol. Evol. 1, 0014. doi: 10.1038/s41559-016-0014
Knowlton N., Brainard R. E., Fisher R., Moews M., Plaisance L., Caley M. J., et al. (2010). “Coral reef biodiversity,” in Life in the world’s oceans. Ed. McIntyre A. D. (United Kingdom: Wiley-Blackwell), 65–78. doi: 10.1002/9781444325508.ch4
Krueger T., Becker S., Pontasch S., Dove S., Hoegh-Guldberg O., Leggat W., et al. (2014). Antioxidant plasticity and thermal sensitivity in four types of Symbiodinium sp. J. Phycol. 50, 1035–1047. doi: 10.1111/jpy.12232
Krueger T., Hawkins T. D., Becker S., Pontasch S., Dove S., Hoegh-Guldberg O., et al. (2015a). Differential coral bleaching–contrasting the activity and response of enzymatic antioxidants in symbiotic partners under thermal stress. Comp. Biochem. Physiol. A. Mol. Integr. Physiol. 190, 15–25. doi: 10.1016/j.cbpa.2015.08.012
Krueger T., Fisher P. L., Becker S., Pontasch S., Dove S., Hoegh-Guldberg O., et al. (2015b). Transcriptomic characterization of the enzymatic antioxidants FeSOD, MnSOD, APX and KatG in the dinoflagellate genus symbiodinium. BMC Evol. Biol. 15, 48. doi: 10.1186/s12862-015-0326-0
LaJeunesse T. C., Parkinson J. E., Gabrielson P. W., Jeong H. J., Reimer J. D., Voolstra C. R., et al. (2018). Systematic revision of symbiodiniaceae highlights the antiquity and diversity of coral endosymbionts. Curr. Biol. CB 28, 2570–2580.e6. doi: 10.1016/j.cub.2018.07.008
Layden M. J., Johnston H., Amiel A. R., Havrilak J., Steinworth B., Chock T., et al. (2016). MAPK signaling is necessary for neurogenesis in nematostella vectensis. BMC Biol. 14, 61. doi: 10.1186/s12915-016-0282-1
Lee G.-H., Kim H.-K., Chae S.-W., Kim D.-S., Ha K.-C., Cuddy M., et al. (2007). Bax inhibitor-1 regulates endoplasmic reticulum stress-associated reactive oxygen species and heme oxygenase-1 expression. J. Biol. Chem. 282, 21618–21628. doi: 10.1074/jbc.M700053200
Lenton K. J., Therriault H., Fülöp T., Payette H., Wagner J. R. (1999). Glutathione and ascorbate are negatively correlated with oxidative DNA damage in human lymphocytes. Carcinogenesis 20, 607–613. doi: 10.1093/carcin/20.4.607
Lesser M. P. (1996). Elevated temperatures and ultraviolet radiation cause oxidative stress and inhibit photosynthesis in ymbiotic dinoflagellates. Limnol. Oceanogr. 41, 271–283. doi: 10.4319/lo.1996.41.2.0271
Lesser M. P., Stochaj W. R., Tapley D. W., Shick J. M. (1990). Bleaching in coral reef anthozoans: effects of irradiance, ultraviolet radiation, and temperature on the activities of protective enzymes against active oxygen. Coral Reefs 8, 225–232. doi: 10.1007/BF00265015
Levin R. A., Beltran V. H., Hill R., Kjelleberg S., McDougald D., Steinberg P. D., et al. (2016). Sex, scavengers, and chaperones: Transcriptome secrets of divergent Symbiodinium Thermal Tolerances. Mol. Biol. Evol. 33, 2201–2215. doi: 10.1093/molbev/msw119
Liew Y. J., Zoccola D., Li Y., Tambutté E., Venn A. A., Michell C. T., et al. (2018). Epigenome-associated phenotypic acclimatization to ocean acidification in a reef-building coral. Sci. Adv. 4, eaar8028. doi: 10.1126/sciadv.aar8028
Liew Y. J., Howells E. J., Wang X., Michell C. T., Burt J. A., Idaghdour Y., et al. (2020). Intergenerational epigenetic inheritance in reef-building corals. Nat. Clim. Change 10, 254–259. doi: 10.1038/s41558-019-0687-2
Liu Y., Beyer A., Aebersold R. (2016). On the dependency of cellular protein levels on mRNA abundance. Cell 165, 535–550. doi: 10.1016/j.cell.2016.03.014
Liu F.-W., Liu F.-C., Wang Y.-R., Tsai H.-I., Yu H.-P. (2015). Aloin protects skin fibroblasts from heat stress-induced oxidative stress damage by regulating the oxidative defense system. PloS One 10, e0143528. doi: 10.1145/2818302
Lopes A. R., Faleiro F., Rosa I. C., Pimentel M. S., Trubenbach K., Repolho T., et al. (2018). Physiological resilience of a temperate soft coral to ocean warming and acidification. Cell Stress Chaperones 23, 1093–1100. doi: 10.1007/s12192-018-0919-9
Majerova E., Carey F. C., Drury C., Gates R. D. (2021). Preconditioning improves bleaching tolerance in the reef-building coral Pocillopora acuta through modulations in the programmed cell death pathways. Mol. Ecol. 30, 3560–3574. doi: 10.1111/mec.15988
Middlebrook R., Hoegh-Guldberg O., Leggat W. (2008). The effect of thermal history on the susceptibility of reef-building corals to thermal stress. J. Exp. Biol. 211, 1050–1056. doi: 10.1242/jeb.013284
Nesa B., Baird A. H., Harii S., Yakovleva I., Hidaka M. (2012). Algal symbionts increase DNA damage in coral planulae exposed to sunlight. Zool. Stud. 51, 12–17.
Nesa B., Hidaka M. (2008). Thermal stress increases oxidative DNA damage in coral cell aggregates. Proc. 11th Int. Coral Reef Symp. Sess. number 5, 144–148. Available at: https://nsuworks.nova.edu/occ_icrs/1.
Nielsen D. A., Petrou K., Gates R. D. (2018). Coral bleaching from a single cell perspective. ISME J. 12, 1558–1567. doi: 10.1038/s41396-018-0080-6
Oakley C. A., Davy S. K. (2018). “Cell biology of coral bleaching,” in Coral bleaching, vol. 233 . Eds. van Oppen M. J. H., Lough J. M. (Switzerland: Springer International Publishing), 189–211. doi: 10.1007/978-3-319-75393-5_8
Palumbi S. R., Barshis D. J., Traylor-Knowles N., Bay R. A. (2014). Mechanisms of reef coral resistance to future climate change. Science 344, 895–898. doi: 10.1126/science.1251336
Patel T. K., Williamson J. D. (2016). Mannitol in plants, fungi, and plant–fungal interactions. Trends Plant Sci. 21, 486–497. doi: 10.1016/j.tplants.2016.01.006
Pernice M., Dunn S. R., Miard T., Dufour S., Dove S., Hoegh-Guldberg O.. (2011). Regulation of apoptotic mediators reveals dynamic responses to thermal stress in the reef building coral acropora millepora. PloS One 6, e16095. doi: 10.1371/journal.pone.0016095
Pfaffl M. W., Tichopad A., Prgomet C., Neuvians T. P. (2004). Determination of stable housekeeping genes, differentially regulated target genes and sample integrity: BestKeeper – excel-based tool using pair-wise correlations. Biotechnol. Lett. 26, 509–515. doi: 10.1023/B:BILE.0000019559.84305.47
Purschke M., Laubach H.-J., Rox Anderson R., Manstein D. (2010). Thermal injury causes DNA damage and lethality in unheated surrounding cells: Active thermal bystander effect. J. Invest. Dermatol. 130, 86–92. doi: 10.1038/jid.2009.205
Putnam H. M., Davidson J. M., Gates R. D. (2016). Ocean acidification influences host DNA methylation and phenotypic plasticity in environmentally susceptible corals. Evol. Appl. 9, 1165–1178. doi: 10.1111/eva.12408
Raghunath A., Sundarraj K., Nagarajan R., Arfuso F., Bian J., Kumar A. P., et al. (2018). Antioxidant response elements: Discovery, classes, regulation and potential applications. Redox Biol. 17, 297–314. doi: 10.1016/j.redox.2018.05.002
R Core Team (2020). R: A language and environment for statistical computing (R Foundation for Statistical Computing). Available at: https://www.R-project.org/.
Redza-Dutordoir M., Averill-Bates D. A. (2016). Activation of apoptosis signalling pathways by reactive oxygen species. Biochim. Biophys. Acta BBA - Mol. Cell Res. 1863, 2977–2992. doi: 10.1016/j.bbamcr.2016.09.012
Roberty S., Fransolet D., Cardol P., Plumier J.-C., Franck F. (2015). Imbalance between oxygen photoreduction and antioxidant capacities in symbiodinium cells exposed to combined heat and high light stress. Coral Reefs 34, 1063–1073. doi: 10.1007/s00338-015-1328-5
Robinson K. S., Clements A., Williams A. C., Berger C. N., Frankel G. (2011). Bax inhibitor 1 in apoptosis and disease. Oncogene 30, 2391–2400. doi: 10.1038/onc.2010.636
Rodríguez-Casariego J. A., Mercado-Molina A. E., Garcia-Souto D., Ortiz-Rivera I. M., Lopes C., Baums I. B., et al. (2020). Genome-wide DNA methylation analysis reveals a conserved epigenetic response to seasonal environmental variation in the staghorn coral acropora cervicornis. Front. Mar. Sci. 7, 560424. doi: 10.3389/fmars.2020.560424
Ross C., Ritson-Williams R., Olsen K., Paul V. J. (2013). Short-term and latent post-settlement effects associated with elevated temperature and oxidative stress on larvae from the coral porites astreoides. Coral Reefs 32, 71–79. doi: 10.1007/s00338-012-0956-2
Saragosti E., Tchernov D., Katsir A., Shaked Y. (2010). Extracellular production and degradation of superoxide in the coral stylophora pistillata and cultured symbiodinium. PloS One 5, e12508. doi: 10.1371/journal.pone.0012508
Schieber M., Chandel N. S. (2014). ROS function in redox signaling and oxidative stress. Curr. Biol. 24, R453–R462. doi: 10.1016/j.cub.2014.03.034
Schindelin J., Arganda-Carreras I., Frise E., Kaynig V., Longair M., Pietzsch T., et al. (2012). Fiji: an open-source platform for biological-image analysis. Nat. Methods 9, 676–682. doi: 10.1038/nmeth.2019
Schoepf V., Carrion S. A., Pfeifer S. M., Naugle M., Dugal L., Bruyn J., et al. (2019). Stress-resistant corals may not acclimatize to ocean warming but maintain heat tolerance under cooler temperatures. Nat. Commun. 10, 4031. doi: 10.1038/s41467-019-12065-0
Suggett D. J., Warner M. E., Smith D. J., Davey P., Hennige S., Baker N. R., et al. (2008). Photosynthesis and production of hydrogen peroxide by symbiodinium (pyrrhophyta) phylotypes with different thermal tolerances1. J. Phycol. 44, 948–956. doi: 10.1111/j.1529-8817.2008.00537.x
Surova O., Zhivotovsky B. (2013). Various modes of cell death induced by DNA damage. Oncogene 32, 3789–3797. doi: 10.1038/onc.2012.556
Szabó M., Larkum A. W. D., Vass I. (2020). “A review: The role of reactive oxygen species in mass coral bleaching,” in Photosynthesis in algae: Biochemical and physiological mechanisms, vol. 45 . Eds. Larkum A. W. D., Grossman A. R., Raven J. A. (Switzerland: Springer International Publishing), 459–488. doi: 10.1007/978-3-030-33397-3_17
Tchernov D., Kvitt H., Haramaty L., Bibby T. S., Gorbunov M. Y., Rosenfeld H., et al. (2011). Apoptosis and the selective survival of host animals following thermal bleaching in zooxanthellate corals. Proc. Natl. Acad. Sci. 108, 9905–9909. doi: 10.1073/pnas.1106924108
Thomas L., Rose N. H., Bay R. A., López E. H., Morikawa M. K., Ruiz-Jones L., et al. (2018). Mechanisms of thermal tolerance in reef-building corals across a fine-grained environmental mosaic: Lessons from ofu, American Samoa. Front. Mar. Sci. 4. doi: 10.3389/fmars.2017.00434
van Hooidonk R., Maynard J. A., Planes S. (2013). Temporary refugia for coral reefs in a warming world. Nat. Clim. Change 3, 508–511. doi: 10.1038/nclimate1829
van Oppen M. J. H., Oliver J. K., Putnam H. M., Gates R. D. (2015). Building coral reef resilience through assisted evolution. Proc. Natl. Acad. Sci. 112, 2307–2313. doi: 10.1073/pnas.1422301112
Vercelloni J., Clifford S., Caley M. J., Pearse A. R., Brown R., James A., et al. (2018). Using virtual reality to estimate aesthetic values of coral reefs. R. Soc Open Sci. 5, 172226. doi: 10.1098/rsos.172226
Weizman E., Levy O. (2019). The role of chromatin dynamics under global warming response in the symbiotic coral model aiptasia. Commun. Biol. 2, 282. doi: 10.1038/s42003-019-0543-y
Yakovleva I., Bhagooli R., Takemura A., Hidaka M. (2004). Differential susceptibility to oxidative stress of two scleractinian corals: antioxidant functioning of mycosporine-glycine. Comp. Biochem. Physiol. B Biochem. Mol. Biol. 139, 721–730. doi: 10.1016/j.cbpc.2004.08.016
Yakovleva I., Baird A., Yamamoto H., Bhagooli R., Nonaka M., Hidaka M.. (2009). Algal symbionts increase oxidative damage and death in coral larvae at high temperatures. Mar. Ecol. Prog. Ser. 378, 105–112. doi: 10.3354/meps07857
Yasuoka Y., Shinzato C., Satoh N. (2016). The mesoderm-forming gene brachyury regulates ectoderm-endoderm demarcation in the coral acropora digitifera. Curr. Biol. 26, 2885–2892. doi: 10.1016/j.cub.2016.08.011
Keywords: coral bleaching, oxidative stress, siRNA gene knockdown, acclimatization, antioxidants
Citation: Majerová E and Drury C (2022) Thermal preconditioning in a reef-building coral alleviates oxidative damage through a BI-1-mediated antioxidant response. Front. Mar. Sci. 9:971332. doi: 10.3389/fmars.2022.971332
Received: 16 June 2022; Accepted: 15 September 2022;
Published: 03 October 2022.
Edited by:
Danwei Huang, National University of Singapore, SingaporeReviewed by:
Rachel Alderdice, University of Technology Sydney, AustraliaRosa Celia Poquita-Du, Senckenberg Biodiversity and Climate Research Centre, Germany
Copyright © 2022 Majerová and Drury. This is an open-access article distributed under the terms of the Creative Commons Attribution License (CC BY). The use, distribution or reproduction in other forums is permitted, provided the original author(s) and the copyright owner(s) are credited and that the original publication in this journal is cited, in accordance with accepted academic practice. No use, distribution or reproduction is permitted which does not comply with these terms.
*Correspondence: Eva Majerová, bWFqYS5ldmEyQGdtYWlsLmNvbQ==