- 1Laboratorio de Investigación Ambiental Acuático, Centro de Estudios Avanzados, HUB-AMBIENTAL UPLA, Universidad de Playa Ancha, Valparaíso, Chile
- 2Doctorado Interdisciplinario en Ciencias Ambientales, Facultad de Ciencias Naturales y Exactas, Universidad de Playa Ancha, Valparaíso, Chile
- 3Departamento de Ciencias del Mar y Biología Aplicada, Universidad de Alicante, Alicante, Spain
- 4Departamento de Ciencias Biológicas, Universidad Autónoma de Chile, Talca, Chile
- 5Centro de Bioinnovación, Universidad de Antofagasta, Antofagasta, Chile
- 6Laboratorio de Toxicología Acuática (AQUATOX), Instituto de Ciencias Naturales Alexander von Humboldt, Universidad de Antofagasta, Antofagasta, Chile
Desalination brines from direct seawater intake that get discharged to coastal areas may produce stress responses on benthic marine communities, mostly due to its excess salinity, and especially on sessile organisms; in this context, macroalgae have been understudied in desalination ecotoxicological investigations. In this study, we assessed the short- and long-term cellular tolerance responses in two brown species of the macroalgae genus Dictyota through controlled laboratory conditions. Dictyota kunthii was collected from the eastern Pacific Ocean (average salinity, ~34 psu), whereas Dictyota dichotoma was from the Mediterranean Sea (average salinity, ~37 psu). Each macroalgae species was exposed for up to 7 days to two conditions with increased salinity values: +2 and +7 psu above their natural average salinity. Photosynthetic parameters and oxidative stress measurements were determined. The results showed that, in both Dictyota species, high salinity values induced reduced photoinhibition (Fv/Fm) but increased the primary productivity (ETRmax) and light requirement (EkETR) especially after 7 days. Conversely, the photosynthetic efficiency (αETR) decreased in hypersalinity treatments in D. dichotoma, while there were no changes in D. kunthii. The reactive oxygen species hydrogen peroxide (H2O2) was greater at high salinity values at 3 days for D. dichotoma and after 7 days in D. kunthii, while lipid peroxidation decreases under hypersalinity with time in both species. Despite the evident H2O2 accumulation in both species against hypersalinity, it did not produce oxidative damage and important impairment in the photosynthetic apparatus. These results contribute to understanding the tolerance strategies at the cellular level of Dictyota spp., which may be considered as potential candidates for biomonitoring of desalination impacts in the field.
1. Introduction
In the recent decades, seawater desalination has been promoted as a feasible solution to cover limited freshwater availability and its higher demand at a global scale (Fernández-Torquemada and Sánchez-Lizaso, 2007; Jones et al., 2019). This scarcity of water has been induced by exponential consumption in global population, increasing demand per capita, industry expansion, and contamination, together with the global climate crisis (Schewe et al., 2014; Fernández-Torquemada et al., 2019; Jones et al., 2019).
Desalination is the process by which dissolved salts and minerals from any water source, such as seawater, brackish groundwater, surface water, and even sewage, are removed (Jones et al., 2019; Fernández-Torquemada et al., 2019). Among the available technological options for desalination, reverse osmosis (RO) has become the preferred method due to its relatively low cost and energy efficiency (Fernández-Torquemada and Sánchez-Lizaso, 2007; Fernández-Torquemada et al., 2009; Shahzad et al., 2017). However, RO desalination plants may discharge residual brine into the subtidal zone, which is composed of a hypersaline effluent nearly twice as concentrated as the input seawater. The brine may also contain trace chemical residues such as coagulants, biocides, and anti-scalants (Lattemann and Höpner, 2008; Belkin et al., 2017; Fernández-Torquemada et al., 2019; Panagopoulos and Giannika, 2022; Panagopoulos, 2022). In spite of the latter, the potential detrimental effects of desalination have been observed to be mainly associated with the osmotic stress caused by salt excess (e.g. Del Pilar Ruso et al., 2015; De la Ossa Carretero et al, 2016; Cambridge et al., 2019 Fernández-Torquemada et al., 2019; Rodríguez-Rojas et al., 2020; Panagopoulos and Haralambous, 2020a; Panagopoulos and Haralambous, 2020b; Sandoval-Gil et al., 2022; Muñoz et al., 2023).
In marine photoautotrophs, hypersaline exposure induces water imbalance within the cellular environment (Kirst, 1990). An excess of Na+ and Cl- ions in the extracellular medium can cause cellular dehydration, ionic exchange disruption, and overproduction of reactive oxygen species (ROS) (Kumar et al., 2014; Garrote-Moreno et al, 2015a). In this context, most studies regarding the effects related with brine-derived hypersalinity have been carried out in marine angiosperms or seagrasses; these have demonstrated marked species-specific sensitivity and tolerance (e.g., Sandoval-Gil et al., 2014; Piro et al., 2015; Garrote-Moreno et al., 2015a; Panda et al., 2019; Shetty et al., 2019; Capó et al., 2020; Sandoval-Gil et al., 2022)—for instance, a controlled comparative study using artificial marine salts from 37 to 60 practical salinity units (psu) showed higher salinity tolerance in the seagrass Cymodocea nodosa than in Posidonia oceanica as evidenced by the higher ratios of K+/Na+ and Ca2+/Na+ (Garrote-Moreno et al., 2015b). Moreover, photosynthetic responses through in vivo chlorophyll a fluorescence in the seagrass Posidonia australis have evidenced a decrease in maximum electron transport rate (ETRmax) and saturation irradiance (EkETR) after 4 and 6 weeks of exposure to hypersalinity (46 and 54 psu) (Cambridge et al., 2017), while in Enhalus acoroides hypersalinity (40 and 50 psu) declined the maximal quantum yield (Fv/Fm) and chlorophyll content after 20 days of exposure (Kongrueang et al., 2018). Records on macroalgae and related communities are very limited, especially under brine impacts in the field or upon controlled laboratory experiments extrapolated to desalination discharges. In this context, there are some records available under high salinity values—for instance, enhanced salinity of up to 90 psu for 4 days in the intertidal green macroalga Ulva fasciata produced an intense oxidative stress condition; more specifically, hypersalinity induced H2O2 over-production and increased lipid peroxidation together with photosynthetic inhibition compared with controls (30 psu) (Lu et al., 2006). On the other hand, the intertidal macroalga Ulva compressa demonstrated decreased primary productivity and photoinhibition against hypersalinity stress (32 vs. 82 psu) after 6 days (Muñoz et al., 2020). In addition, in the only field assessment in the context of desalination impacts using macroalgae as biomonitoring organisms, transplants of the model brown macroalga Ectocarpus were subjected to brine discharges from a desalination plant in Chile (at nearly 37 vs. 34 psu at the control site) and prompted a decrease in photosynthetic activity and a significant stress condition as evidenced by the over-accumulation of ROS and antioxidant molecules (Rodríguez-Rojas et al., 2020). Thus, considering the ecological relevance of macroalgae and their abundance and wide distribution at a global scale in areas operating and projecting for the development of the industry, these make them suitable candidates for biomonitoring purposes in the frame of the potential environmental impacts of desalination.
The Dictyotales order includes 214 brown algae species, among them the genus Dictyota, which is the third most diverse order after Ectocarpales. Dictyotales has a worldwide distribution from temperate waters to the subtropical and tropical zones (Bittner et al., 2008). Moreover, Dictyota species can be found from the intertidal to the subtidal zones, being one of the most environmentally versatile and abundant marine macroalgae—thus sustaining large ecologically and economic coastal ecosystems globally (Bogaert et al., 2020). Due to the distribution of Dictyota species, they have developed different strategies to persist in adverse environments and salinity shifts (Andrade et al., 2006; Macaya and Thiel, 2008; Laib and Leghouchi, 2012). In the most ambitious and globally representative macrophyte environmental monitoring experience conducted to date in the context of desalination impacts, we evaluated the responses of two species of Dictyota—D. kunthii, from the north Chilean Pacific coast, and D. dichotoma, from the east Spain Mediterranean coast—exposed to hypersalinity conditions.
In this study, in short-term-controlled laboratory conditions, we compared photosynthetic activity and oxidative stress response in the Dictyota species under increased salinity levels similar to those found nearby brine discharges. In a complementary article (Muñoz et al., 2023), similar parameters were assessed in these species under transplantation experiments in desalination plant-impacted sites.
2. Materials and methods
2.1. Macroalgae collection and experimental design
D. kunthii was collected from Antofagasta Bay, Chile (23°38′47″ S, 70°23′53″ W) at 15-m depth. The natural salinity in Antofagasta Bay oscillates between 34.32 and 35.35 psu, and the retention of water is favored by a cyclonic twist, producing a higher superficial water temperature that varies between 14.5 and 20°C (Dávila and Valdés, 2015). On the other hand, D. dichotoma was collected from Alicante Bay, Spain (38°20′45.59″ N, 0°29′26.47″ W) at 2-m depth. High temperature and irradiance induce a negative water balance of Mediterranean Sea, producing an excess of evaporation and a minimum precipitation contribution and generating a mean salinity of 37–37.5 psu and seawater temperature of 12–28°C (Naranjo et al., 2015). After collection, the individuals were stored in a cooler and immediately transported to the laboratory to start the acclimation process.
Both species were acclimated for 24 h in plastic flasks with seawater obtained from their natural habitat and exposed to constant aeration, 50 μmol photons m−2 s−1 light, and 12:12-h light/dark photoperiod; considering the environmental conditions in their natural habitats, temperatures of 17 ± 1°C and 20 ± 1°C were used for D. kunthii and D. dichotoma, respectively, for acclimation and experimental purposes. After acclimation, both species were exposed to hypersalinity treatments, increasing in 2 (+2) and 7 (+7) psu from their natural baseline ranges (controls; CTRL). In this regard, the experiments for D. kunthii consisted in 35 (CTRL), 37, and 42 psu, each one with three biological replicates. On the other hand, the experimental conditions for D. dichotoma salinity were 37 (CTRL), 39, and 44 psu, each one with three biological replicates. Increasing values of salinity were chosen considering the real saline plume profiles generated from brine discharges of desalination plants located in Antofagasta (Rodríguez-Rojas et al., 2020) and Alicante (Fernández-Torquemada et al., 2009). The desired salinity values were obtained adding artificial sea salts (Instant Ocean® for D. kunthii and Reef Salt® for D. dichotoma) mixed with filtered seawater. Salinity monitoring was performed with a multiparametric probe (YSI model Professional Plus®). Approximately 300 g of each species (~10 of D. kunthii and ~15 individuals of D. dichotoma) was distributed separately in lid-free polycarbonate chambers with 500 ml of seawater with different salinity values in three biological replicates. Subsamples of algae thallus, avoiding the fixing disk, were collected after 3 and 7 days to analyze photosynthetic performance, while for biochemical analyses the tissue was frozen with liquid nitrogen and stored at – 80°C.
2.2. Photosynthetic performance
Photosynthetic measurements corresponding to the maximum quantum yield of photosystem II (PSII; Fv/Fm), electron transport rate (ETR), estimator of photosynthetic efficiency (αETR), saturation irradiance for ETR (EkETR), and non-photochemical quenching (NPQ) were registered with a pulse-amplitude modulated chlorophyll a fluorometer (JUNIOR PAM, Walz GmbH, Effeltrich, Germany) with the Win Control-3.2 software. All calculations were performed according to Celis-Plá et al. (2014); Celis-Plá et al (2020); Rodríguez-Rojas et al. (2020). ETR and NPQ were calculated according to Schreiber et al., 1995.
2.3. Concentration of hydrogen peroxide
The levels of H2O2 were determined using a spectrophotometer according to Rodríguez-Rojas et al. (2020) but with modifications for D. kunthii and D. dichotoma. For both species, ~100 mg fresh weight biomass was ground with liquid nitrogen and mixed with 100 µl of 10% trichloroacetic acid (TCA) in the presence of glass beads (3 mm), 100 µl of 10 mM potassium phosphate buffer (pH 7.0), and 100 µl of lysis buffer. In the case of D. kunthii, lysis buffer from E.Z.N.A. total RNA kit I was used (Omega Bio-tek), while in D. dichotoma, the lysis buffer described by Pearson et al. (2006) that contained 100 mM Tris, 50 mM EDTA (pH 7.5), 2 M NaCl, and 2% CTAB was applied. The subsequent protocol steps are described elsewhere (Rodríguez-Rojas et al., 2020).
2.4. Concentration of thiobarbituric acid reactive substances
The thiobarbituric acid reactive substance (TBARS) levels were quantified using a spectrophotometer according to Rodríguez-Rojas et al. (2020) with modifications for each species to evaluate lipid peroxidation. For D. kunthii, fresh biomass (~100 mg) was ground with liquid nitrogen and mixed with 300 µl of 10% TCA. For D. dichotoma, ~150 mg fresh biomass was ground with liquid nitrogen and mixed with 450 µl of 10% TCA. Both extracts were vortexed for 20 min. Subsequently, the mixture was centrifuged at 17,800 × g for 15 min at 4°C. The protocol steps performed further were as described elsewhere (Rodríguez-Rojas et al., 2020).
2.5. Statistical analyses
Statistical analyses related to the effects of salinity on photosynthetic activity and oxidative stress were analyzed by two-way ANOVA followed by a posteriori test of Student–Newman–Keuls test (Underwood, 1997). The level of significance used was 95% confidence interval (p = 0.05). Prior to the statistical analysis, the homogeneity of variances was tested using Cochran test and a visual inspection of the residuals in terms of normality (Underwood, 1997). Statistical software version 7 (Stat Soft Inc., Tulsa, OK, USA) and statistical package SPSS v.21 (IBM, USA) were applied. Principal component analysis (PCA) was carried out to visualize patterns among all parameters through PERMANOVA+ with PRIMER6 package. The PCA was built with all the photosynthetic and oxidative stress variables to calculate the percentage of variation by each of the axes in a multidimensional scale (Anderson et al., 2008).
2.6. Data availability
All datasets obtained to perform statistical analysis of the entire manuscript correspond to raw numerical data that is not publicly available. Nevertheless, the authors agree to share the raw data if required or when necessary.
3. Results
3.1. Photosynthetic activity
Salinity induced a significant photoinhibition (measured Fv/Fm) in D. kunthii after 7 days at +2 psu, (Figure 1A). In D. dichotoma, significant photoinhibition was observed in the highest salinity (+7 psu) only at 3 days, but it returned to baseline levels at day 7 (Figure 1B). The maximum electron transport rate (ETRmax) increased significantly in D. kunthii during exposure to +7 and +2 psu after 3 and 7 days, respectively (Figure 1C). A similar behavior was observed for D. dichotoma, in which ETRmax increased significantly with salinity at both experimental times, mostly at +7 psu (Figure 1D).
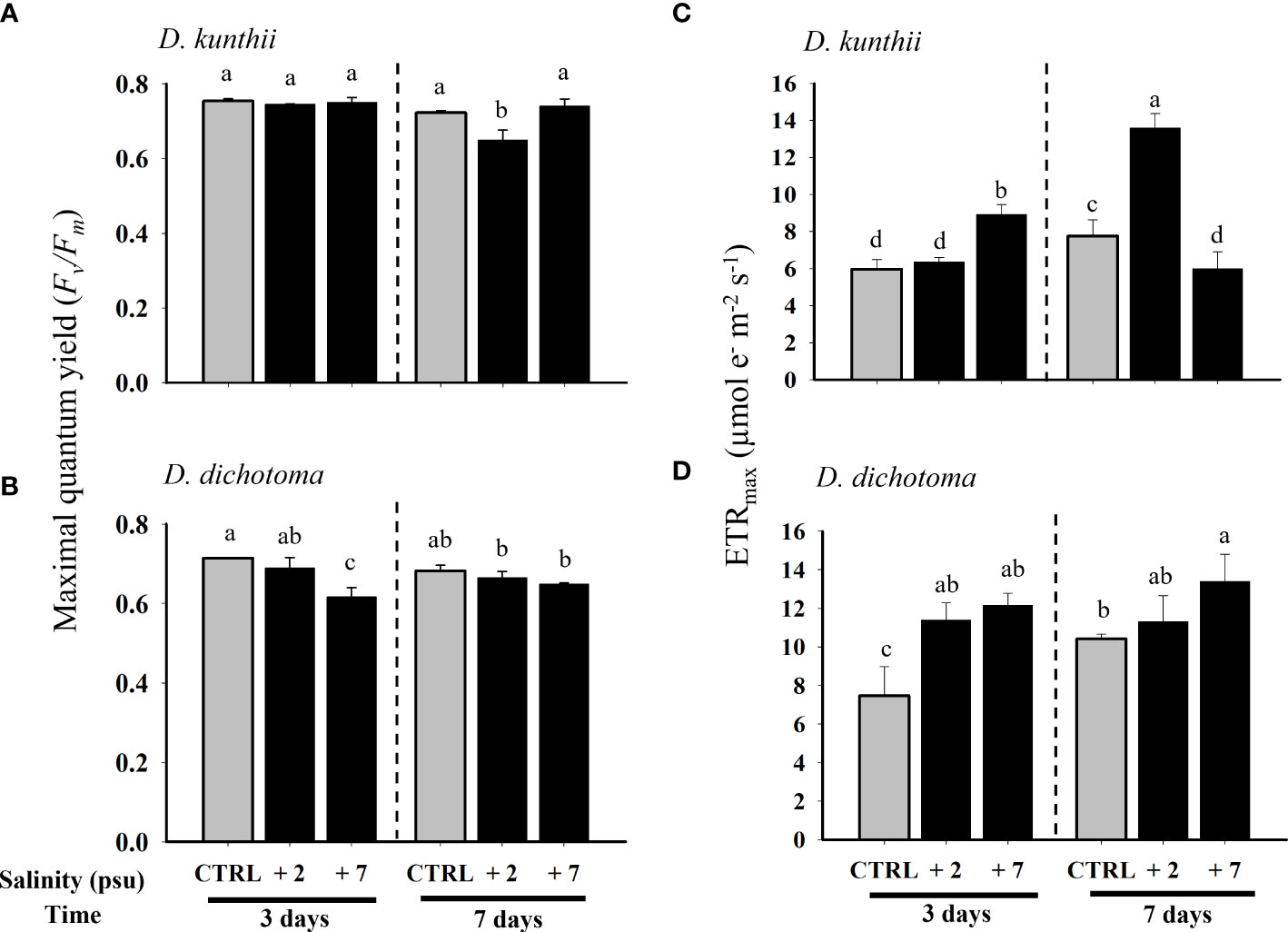
Figure 1 Maximal quantum yield (Fv/Fm) and maximum electron transport rate (ETRmax) in D. kunthii (A, C) and D. dichotoma (B, D) exposed to high salinity values. For D. kunthii, the salinity values were 35 psu (control), 37 (+2) psu, and 42 (+7) psu; for D. dichotoma, these were 37 psu (control), 39 (+2) psu, and 44 (+7) psu. The samples were analyzed after 3 and 7 days. Data corresponds to mean ± SD (n = 3). Letters represent statistical difference at 95% confidence interval (Student–Newman–Keuls test, p < 0.05).
The photosynthetic efficiency (αETR) of D. kunthii suffered no changes (Figure 2A). In contrast, αETR in D. dichotoma subjected to high salinity values always decreased compared with baseline levels, especially under +7 psu at 3 days (Figure 2B). The saturation of irradiance (EkETR) in D. kunthii only increased significantly under +2 psu at 7 days (Figure 2C). On the other hand, in D. dichotoma, EkETR significantly increased under hypersalinity at both experimental times, although with greater levels at 3 days (Figure 2D).
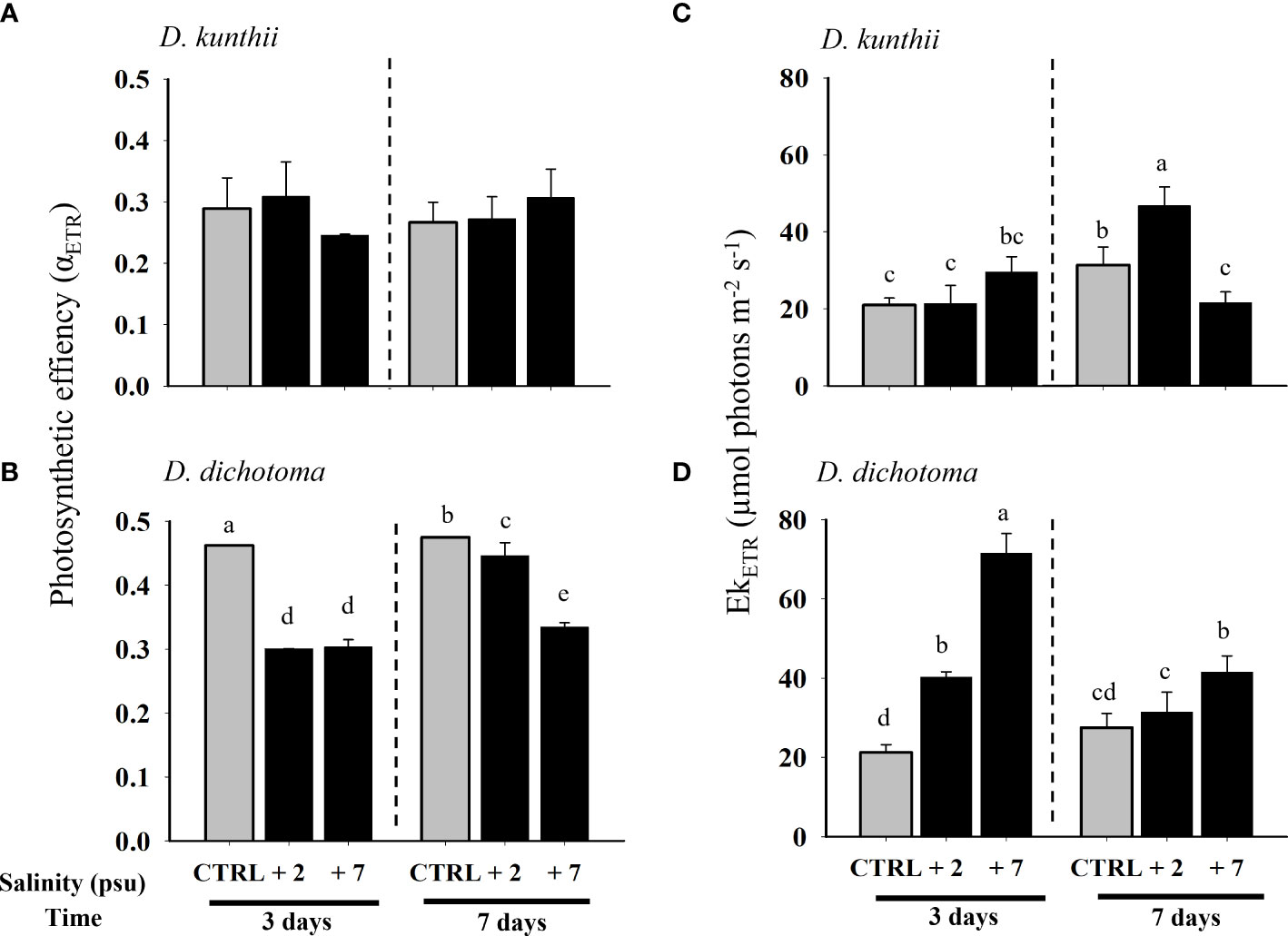
Figure 2 Photosynthetic efficiency (αETR) and saturation of irradiance (EkETR) in D. kunthii (A, C) and D. dichotoma (B, D) exposed to high salinity values. For D. kunthii, the salinity values were 35 psu (control), 37 psu (+2), and 42 (+7) psu; for D. dichotoma, these were 37 psu (control), 39 (+2) psu, and 44 (+7) psu. The samples were analyzed after 3 and 7 days. Data corresponds to mean ± SD (n = 3). Letters represent statistical difference at 95% confidence interval (Student–Newman–Keuls test, p < 0.05). No letters mean no significant differences.
On the other hand, the maximal non-photochemical quenching (NPQmax) in D. kunthii showed a sustained significant decrease with increasing salinity levels at day 3 (Figure 3A). The pattern of NPQmax was similar in D. dichotoma, although a significant decrease with respect to the controls was only detected at +7 psu after 3 days (Figure 3B).
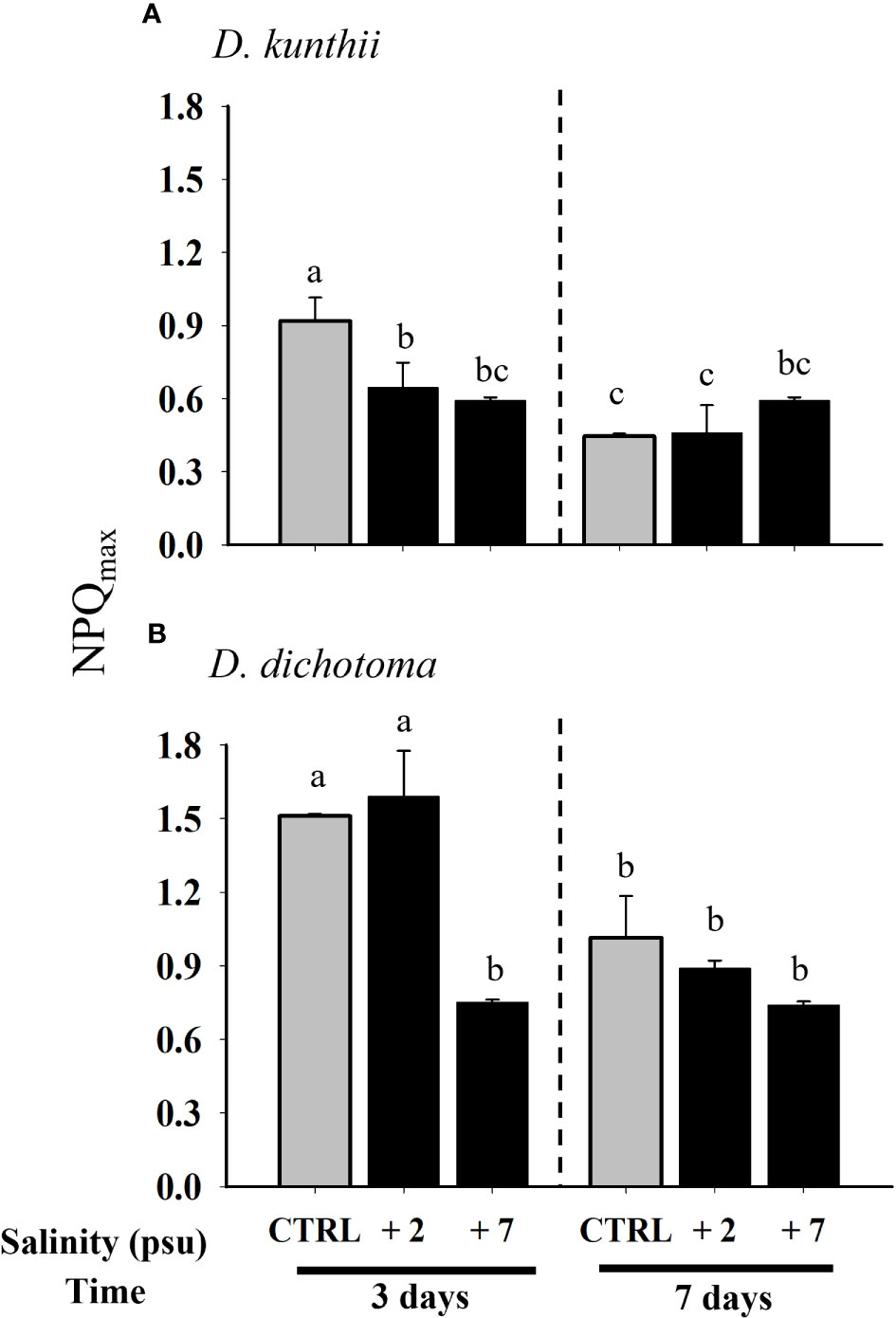
Figure 3 Maximal non-photochemical quenching (NPQmax) in D. kunthii (A) and D. dichotoma (B) exposed to high salinity values. For D. kunthii, the salinityvalues were 35 psu (control), 37 (+2) psu, and 42 (+7) psu; for D. dichotoma, these were 37 psu (control), 39 (+2) psu, and 44 (+7) psu. The sampleswere analyzed after 3 and 7 days. Data corresponds to mean ± SD (n = 3). Letters represent statistical difference at 95% confidence interval (Student–Newman–Keuls test, p < 0.05).
3.2. Quantification of H2O2 and TBARS content
While the accumulation of H2O2 in D. kunthii displayed a decrease only at +2 psu after 3 days, at 7 days there was an increase with respect to controls, especially at +2 psu, suggesting an oxidative stress condition (Figure 4A). Almost an inverted pattern compared with D. kunthii was observed in D. dichotoma, where H2O2 was largely accumulated at +2 psu and decreased significantly after 7 days in comparison to the control values, reflecting that ROS detox mechanisms were activated (Figure 4B). As in lipid peroxidation, TBARS decreased in all treatments with respect to controls at day 3 in D. kunthii, although without a significant difference among them at 7 days, suggesting that, although there was an increase in ROS, there was no oxidative damage (Figure 4C). In contrast, in D. dichotoma, no differences were detected between treatments, with the exception of a significant decrease at +7 psu after 7 days, which was in agreement with the low ROS levels (Figure 4D).
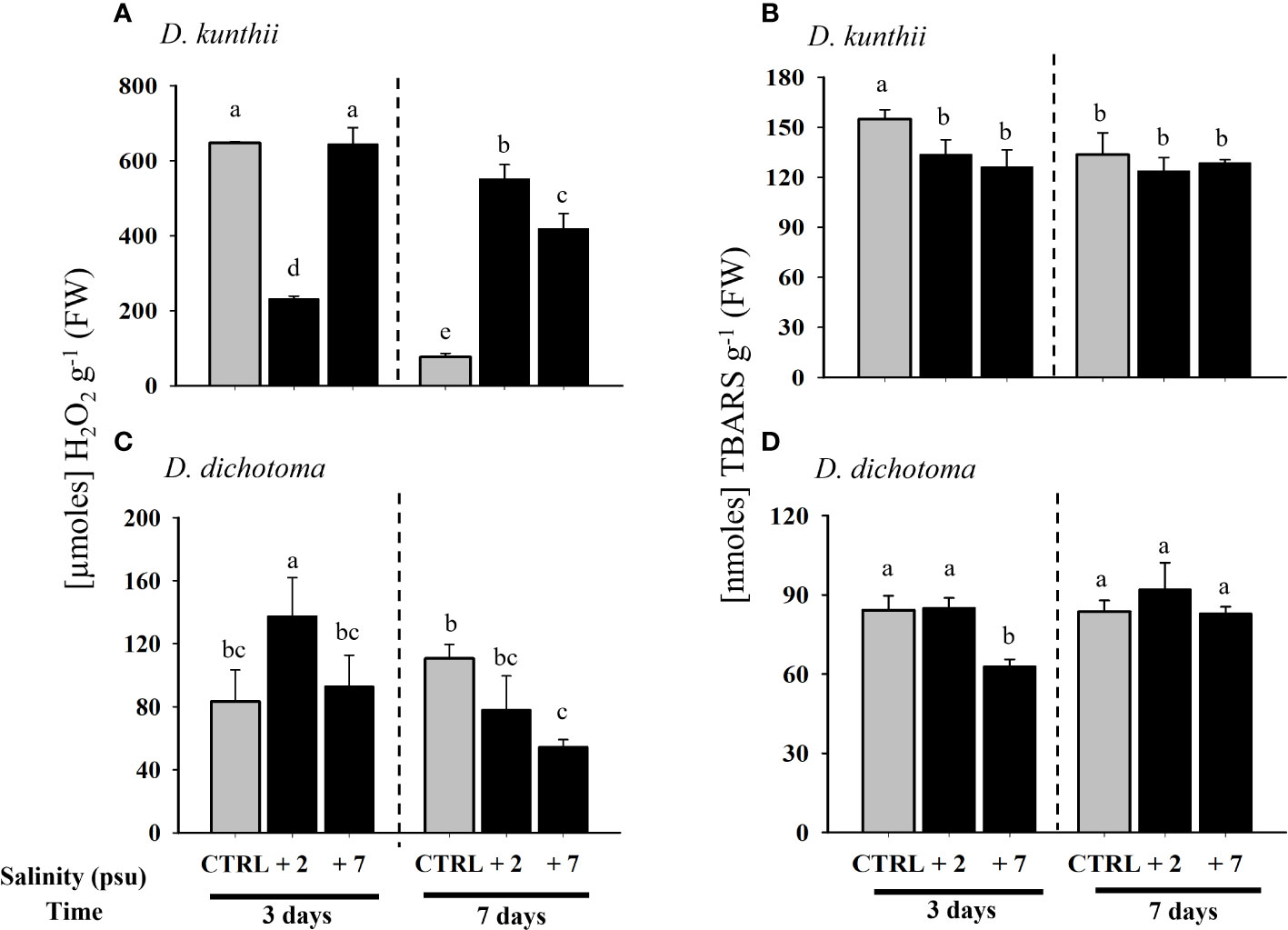
Figure 4 Concentration of H2O2 and thiobarbituric acid reactive substance in D. kunthii (A, C) and D. dichotoma (B, D) exposed to high salinity values. For D.kunthii, the salinity values were 35 psu (control), 37 (+2) psu, and 42 (+7) psu; for D. dichotoma, these were 37 psu (control), 39 (+2) psu, and 44 (+7)psu. The samples were analyzed after 3 and 7 days. Data corresponds to mean ± SD (n = 3). Letters represent statistical difference at 95% confidenceinterval (Student–Newman–Keuls test, p < 0.05).
3.3. Principal component analysis
We used PCA to identify potential patterns of response to high salinity exposure based on photosynthetic (Fv/Fm, αETR, ETRmax, EkETR, and NPQmax) and oxidative stress (H2O2 and TBARS) parameters (Figure 5). The first dimension of the PCA represented 98.9% of the total variation and was mainly characterized by H2O2. This first axis allowed to segregate the two studied species. The second dimension represented 0.9% of the total variation and mainly represented ETRmax and EkETR.
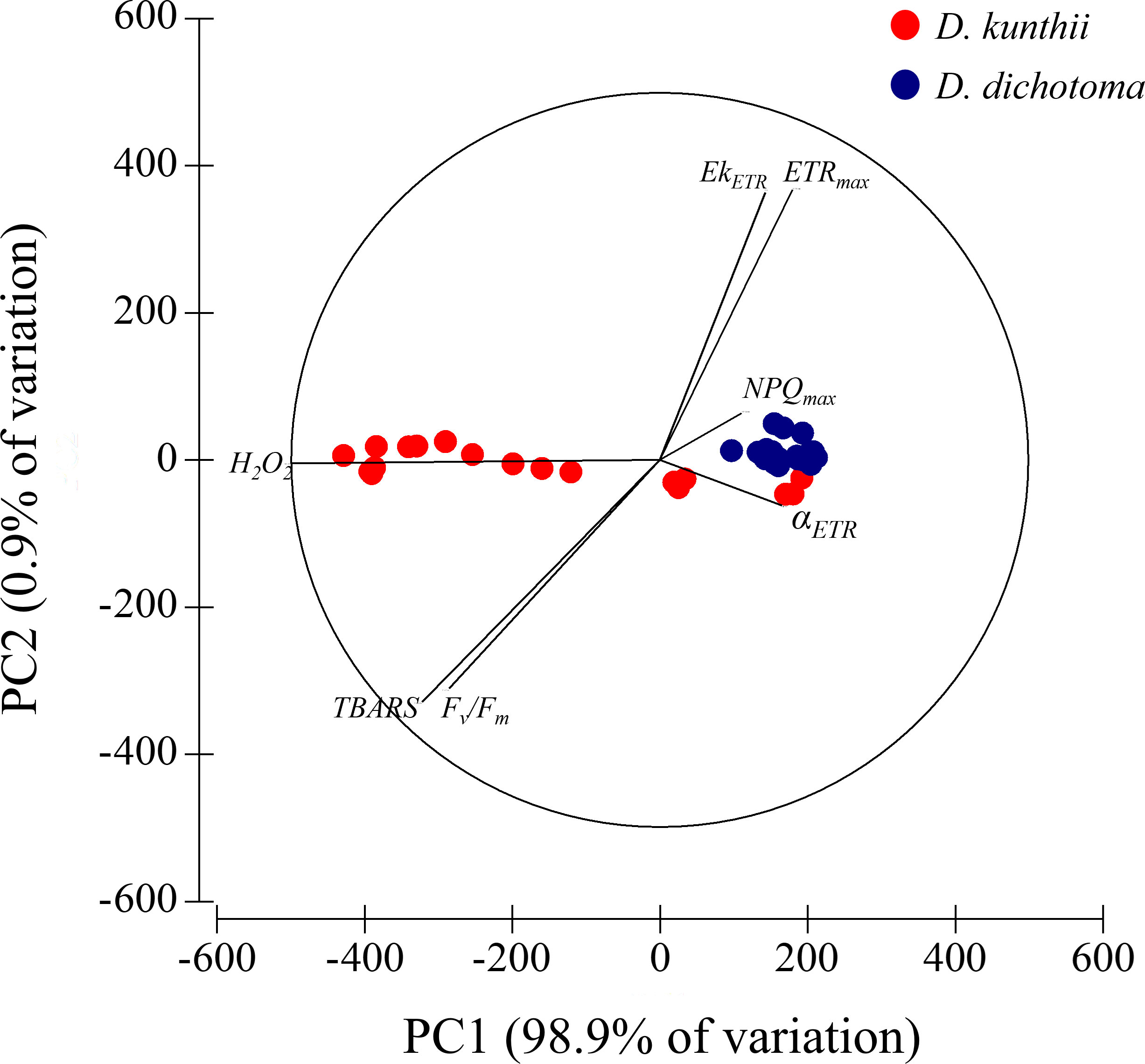
Figure 5 Principal component analysis linked to photosynthetic (Fv/Fm, αETR, ETRmax, EkETR, and NPQmax) and oxidative stress (H2O2 and thiobarbituric acid reactive substance) parameters in both times (3 and 7 days), measured in each species: D. kunthii (Sp1) and D. dichotoma (Sp2).
4. Discussion
In the present investigation, we evaluated the effects of hypersalinity under laboratory-controlled experiments emulating desalination impacts by assessing the photosynthetic and oxidative stress responses of the macroalgae D. kunthii (Chile) and D. dichotoma (Spain). Our results evidenced the effects of excess salinity in both species, although with interspecific differences.
In terms of photosynthesis, the maximal quantum yield (Fv/Fm), used as indicator of the photoinhibition of photosynthetic activity in PSII (Celis-Plá et al., 2016; Celis-Plá et al., 2020), demonstrated low impairment only in D. dichotoma at 3 days, while in D. kunthii it remained almost unaltered. These results demonstrate that excess salinity caused photoinhibition of photosynthesis only at early exposure in the case of D. dichotoma, with a recovery process at 7 days as observed. In contrast, other brown macroalgae species like Ectocarpus showed a radical decrease of Fv/Fm after 3 and 7 days with similar salinity levels, although exposed to brine at a desalination discharge site (Rodríguez-Rojas et al., 2020). Similarly, Lu et al. (2006) reported a marked decrease in Fv/Fm when U. fasciata was exposed to hypersalinity treatments (60–150 psu) compared with the controls (30 psu), while in the green macroalga U. compressa the increment of salinity (42, 62, and 82 psu) showed no effects on Fv/Fm after 7 days of exposition. ETRmax or photosynthetic productivity displayed an increase in D. dichotoma with respect to D. kunthii under hypersalinity conditions at the end of the experimental period. This is in agreement with the results observed in other macroalgae species such as U. compressa exposed to 42 psu after 6 days in laboratory conditions (Muñoz et al., 2020). Conversely, the brown macroalga Ectocarpus showed a severely impaired ETRmax after 7 days when the alga was transplanted in a brine-impacted site (36 psu) compared with the control (34 psu) (Rodríguez-Rojas et al., 2020). These ecophysiological responses are related with the mechanism for the light harvesting complex associated with PSII; as these responses were different between species, the information suggest that the size of the antenna complex was bigger in D. kunthii than in D. dichotoma (Grzymski et al., 1997). In consequence, D. dichotoma demonstrates a sun-type algal behavior, i.e., a concomitant increase of ETRmax, and irradiance of saturation or EkETR (Celis-Plá et al., 2014). Therefore, although both Dictyota species are phylogenetically related, they displayed different photoinhibition and photoacclimation capacities under excessive salinity levels, showing a more marked adapted response phenotype in D. dichotoma than in D. kunthii. In this regard, macroalgae are a highly diverse group with complex functional phenotypes with a variety of ecological roles, even within similar phylogenetic backgrounds; these aspects determine their morphological plasticity and mechanisms to withstand environmental threats, indeed also mediating their capacity to acclimate to global change scenarios (Falkenberg et al., 2013; Gao et al., 2019).
Regarding our data, the higher salinity levels had no apparent effects on the photosynthetic efficiency (αETR) of D. kunthii, while in D. dichotoma a notorious decrease was observed. Therefore, hypersalinity may have contributed to diminish the light harvesting capacity of D. dichotoma since this process has been observed to partially damage or deactivate the reaction center of the photosystems (Li et al., 2016; Cambridge et al., 2017)—for example, in the seagrass Halophila johnsonii, the increase in salinity (above 40 psu) induced the decrease of the photosynthetic efficiency (αETR) regarding the controls after 15 days (Fernández-Torquemada et al., 2005). Similar results of decreased αETR were observed in the green macroalga U. compressa exposed to 82 psu during 6 days in the laboratory (Bittner et al., 2008). Ectocarpus also exhibited a αETR reduction at 36 psu after 7 days under brine discharges in the field transplants (Rodríguez-Rojas et al., 2020). In contrast, the results of EkETR showed a positive correlation with ETRmax in both Dictyota species, in which light requirements increased as salinity was greater, thus saturating the ETR; this was reflected in the observed photoinhibition processes. On the other hand, our results showed a decrease in NPQmax at higher salinity levels in both species after 3 days, with a return to control levels at day 7. NPQ is an effective mechanism to dissipate excessive energy through heat during high light intensity or when light is paired with other present stressors, playing a role in photoprotection (Celis-Plá et al., 2016). In this sense, excess salinity can induce detrimental damage to the photosynthetic apparatus, and NPQ can aid in diminishing the adverse effects of hypersalinity (Zheng et al., 2019). However, it has been also reported that salt excess may damage the antenna complex, altering the light harvesting system of the photosystem and reducing the energy transferred to PSII, consequently inducing a decrease in NPQmax (Sudhir et al., 2005; Zheng et al., 2019); the latter may be behind the decrease in NPQmax in D. kunthii and D. dichotoma at day 3. The effects of salinity on photoprotection have already been reported—for instance, Singh et al. (2019) observed that excess salinity of up to 34 psu with respect to the control (29 psu) caused the decrease of NPQmax in the microalga Dunaliella salina. Our results also showed a recovery process in NPQmax at 7 days in both Dictyota species, probably demonstrating the activation of photoprotector processes. In this regard, the brown macroalga Sargassum polycystum showed no changes in NPQ when exposed for 2 weeks to hypersalinity of up to 40 psu vs. the controls at 32 psu, demonstrating strong photoprotection mechanisms to withstand salinity excess (Zou et al., 2018).
Although considered as a molecule with moderate reactivity, H2O2 is the precursor, through Fenton and Haber–Weiss reaction, of the highly toxic hydroxyl radical (HO·). The latter is a highly oxidizing ROS and responsible for the process of lipid peroxidation (Girotti, 2001; Lesser, 2006; Kumar et al., 2014; Moenne et al., 2016). Additionally, it has been determined that accumulation of H2O2 may cause a disruption of electron chains in the mitochondria and chloroplast, eventually affecting the photosynthetic activity (Xia et al., 2014; Hajiboland, 2014). While in D. dichotoma H2O2 accumulated mostly in +2 psu at 3 days, in D. kunthii this occurred in both hypersalinity conditions after 7 days. However, this was not reflected in an increase of lipid peroxidation or photosynthetic impairment as well, suggesting good management in the production or detoxification of this molecule within these species. Similarly to D. kunthii, the field transplants of the brown alga Ectocarpus showed a significant accumulation of H2O2, although it was not correlated with an increase in lipid peroxidation (Rodríguez-Rojas et al., 2020). On the other hand, experiments on the red macroalga Pyropia haitanensis submitted to an extremely high salinity condition (~110 psu vs. the control at ~30 psu) demonstrated an over-production of H2O2 at 30 min of exposure (Wang et al., 2020). In the green macroalga Ulva prolifera, the exposition to high salinity values of ~60 psu induced twice the H2O2 concentration in relation to the control at ~30 psu after 6 days (Luo and Liu, 2011). During the field studies by Capó et al. (2020) in P. oceanica nearby a brine discharge at nearly 41 psu, an increase of lipid peroxidation compared with the control area at 38 psu was evidenced. Moreover, in a comparative study between the green macroalga Ulva australis and red seaweed Pyropia yezoensis, inter-specific responses were observed, with higher lipid peroxidation in P. yezoensis with respect to U. australis under 55 psu vs. the controls at 30 psu after 7 days (Samanta et al., 2019). Taken together, our results suggest that the Dictyota species studied have efficient response mechanisms to cope with photodamage and oxidative stress mediated by hypersalinity conditions.
Based on our results, Dictyota species seem to have a certain tolerance to salinity increments in the short term (within 7 days), although with timeline differences and a degree of connection that may indicate ecophysiological affection mediated by oxidative stress. In this regard, it has been observed that drastic fluctuations in environmental conditions, including salinity, can induce an oxidative stress condition through different metabolic pathways, although most of them end up disrupting electron transport chains in the chloroplast and transferring excess energy to oxygen; the latter can, in turn, mediate an excess of ROS that can affect metabolic and physiological processes such as photosynthesis (Moenne et al., 2016; Muñoz et al., 2020). This process may have occurred in both species under salinity excess—for instance, when H2O2 increased in D. kunthii, it was accompanied by a decrease in Fv/Fm at 7 days; a similar pattern was observed in D. dichotoma at 3 days of exposure to excessive salinity levels. In spite of the latter, no specific effects were detected in terms of lipid peroxidation or main photosynthetic impairment, thus indicating efficient tolerance mechanisms to withstand excessive salinity levels that may be attributed to desalination discharges in both Dictyota species.
5. Conclusion
Our results showed that D. kunthii and D. dichotoma showed photoinhibition in higher salinity treatments but a gradual augmentation of primary productivity (ETRmax) and irradiance of saturation (EkETR) at all times. However, a marked decrease of photosynthetic efficiency (αETR) was observed in D. dichotoma, but there were no changes in D. kunthii. NPQ decreased in both Dictyota spp. in higher salinity levels, making the photosynthetic apparatus damage evident. Although the H2O2 accumulation was triggered by the hypersalinity condition in both species, this was not manifested in severe impairment of photosynthetic activity or oxidative damage. In contrast, lipid peroxidation diminished in both species, which demonstrated an efficient machinery of ROS scavenging. This work supports the results obtained from field experiments to determine the magnitude of the impact from the artificial increase of salinity compared with hypersalinity from brine discharge from desalination plants on both species with differing latitudinal distribution. This also contributes relevant information to understand about cellular tolerance strategies from macroalgae so as to confront hypersalinity in Dictyota spp., which, given their responses and tolerance, may be recommended as efficient candidates to address the impacts of desalination.
Data availability statement
The raw data supporting the conclusions of this article will be made available by the authors without undue reservation.
Author contributions
PM: conceptualization, field work, methodology, formal analysis, writing—original draft, and visualization. FR-R: field work, methodology, funding acquisition, and writing. PC-P: field work, methodology, and formal analysis. AL-M: field work. FB-M: manuscript review and editing. Iván Sola: manuscript review and editing. CL: writing, formal analyses, and manuscript review. FV: field work and formal analyses. RO: field work and formal analyses. JLS-L: conceptualization, formal analysis, methodology, field work, investigation, resources, review, and editing. CS: conceptualization, field work, methodology, visualization, writing—review and editing, supervision, and funding acquisition. All authors contributed to the article and approved the submitted version.
Funding
We gratefully thank the financial support from ANID FONDECYT Postdoctoral fellowship #3180394, European Commission Marie Skłodowska-Curie Actions #888415, and ANID INES I+D # INID210013. Financial support for mobility was granted from SEGIB Scholarship and Fundación Carolina of Spain to PM. We also thank TESPOST 04/19 PhD scholarship granted by Universidad de Playa Ancha to PM. The initiative was also funded by Universidad de Playa Ancha through the Concurso Regular de Investigación 2019 no. CEA 19-20.
Conflict of interest
The authors declare that the research was conducted in the absence of any commercial or financial relationships that could be construed as a potential conflict of interest.
Publisher’s note
All claims expressed in this article are solely those of the authors and do not necessarily represent those of their affiliated organizations, or those of the publisher, the editors and the reviewers. Any product that may be evaluated in this article, or claim that may be made by its manufacturer, is not guaranteed or endorsed by the publisher.
References
Anderson M., Gorley R. N., Clarke K. (2008). PERMANOVA+ for primer: Guide to software and statistical methods (Plymouth: PRIMER-E).
Andrade S., MH M., JW M., Correa J. A. (2006). Cadmium– copper antagonism in seaweeds inhabiting coastal areas affected by copper mine waste disposals. Environ. Sci. Technol. 40, 4382–4387. doi: 10.1021/es060278c
Belkin N., Rahav E., Elifantz H., Kress N., Berman-Frank I. (2017). The effect of coagulants and antiscalants discharged with seawater desalination brines on coastal microbial communities: a laboratory and in situ study from the southeastern Mediterranean. Water Res. 110, 321–331. doi: 10.1016/j.watres.2016.12.013
Benedetti-Cecchi L., Pannacciulli F., Bulleri F., Moschella P. S., Airoldi L., Relini G., et al. (2001). Predicting the consequences of anthropogenic disturbance : large-scale effects of loss of canopy algae on rocky shores. Mar. Ecol. Prog. Ser. 214, 137–150. doi: 10.3354/meps214137
Bittner L., Payri C. E., Couloux A., Cruaud C., de Reviers B., Rousseau F. (2008). Molecular phylogeny of the dictyotales and their position within the phaeophyceae, based on nuclear, plastid and mitochondrial DNA sequence data. Mol. Phylogenet. Evol. 49, 211–226. doi: 10.1016/j.ympev.2008.06.018
Bogaert K. A., Delva S., De Clerck O. (2020). Concise review of the genus Dictyota J.V. lamouroux. J. Appl. Phycology 32, 1521–1543. doi: 10.1007/s10811-020-02121-4
Cambridge M. L., Zavala-Perez A., Cawthray G. R., Mondon J., Kendrick G. A. (2017). Effects of high salinity from desalination brine on growth, photosynthesis, water relations and osmolyte concentrations of seagrass Posidonia australis. Mar. pollut. Bull. 115, 252–260. doi: 10.1016/j.marpolbul.2016.11.066
Cambridge M. L., Zavala-Perez A., Cawthray G. R., Statton J., Mondon J., Kendrick G. A. (2019). Effects of desalination brine and seawater with the same elevated salinity on growth, physiology and seedling development of the seagrass Posidonia australis. Mar. pollut. Bull. 140, 462–471. doi: 10.1016/j.marpolbul.2019.02.001
Capó X., Tejada S., Ferriol P., Pinya S., Mateu-Vicens G., Montero-González I., et al. (2020). Hypersaline water from desalinization plants causes oxidative damage in Posidonia oceanica meadows. Sci. Total Environ. 736, 139601. doi: 10.1016/j.scitotenv.2020.139601
Celis-Plá P. S. M., Bouzon Z. L., Hall-Spencer J. M., Schmidt E. C., Korbee N., Figueroa F. L. (2016). Seasonal changes in photoprotectors and antioxidant capacity of the fucoid macroalga Cystoseira tamariscifolia. Mar. Environ. Res. 115, 89–97.
Celis-Plá P., Martínez B., Quintano E., García-Sánchez M., Pedersen A., Navarro N. P., et al. (2014). Short-term ecophysiological and biochemical responses of Cystoseira tamariscifolia and Ellisolandia elongata to environmental changes. Aquat. Biol. 22, 227–243. doi: 10.3354/ab00573
Celis-Plá P. S. M., Moenne F., Rodríguez-Rojas F., Pardo D., Lavergne C., Moenne A., et al. (2020). Antarctic Intertidal macroalgae under predicted increased temperatures mediated by global climate change: Would they cope? Sci. Total Environ. 740, 140379. doi: 10.1016/j.scitotenv.2020.140379
Dávila P. M., Valdés J. (2015). Variabilidad temporal de las masas de agua costeras en bahía San Jorge, antofagasta, Chile 23°-2012). Rev. Biología Marina y Oceanografía 50, 61–80. doi: 10.4067/S0718-19572015000100006
De la Ossa Carretero J. A., Del-Pilar-Ruso Y., Loya-Fernández A., Ferrero-Vicente L. M., Marco-Méndez C., Martínez-García E., et al. (2016). Bioindicators as metrics for environmental monitoring of desalination plant discharges. Mar. pollut. Bull. 103, 313–318. doi: 10.1016/j.marpolbul.2015.12.023
Del Pilar Ruso Y., Martínez-García E., Giménez-Casalduero F., Loya-Fernández A., Ferrero-Vicente L. M., Marco-Méndez C., et al. (2015). Benthic community recovery from brine impact after the implementation of mitigation measures. Water Res. 70, 325–336. doi: 10.1016/j.watres.2014.11.036
Falkenberg L. J., Rusell B. D., Connell S. D. (2013). Contrasting resource limitations of marine primary producers: implications for competitive interactions under enriched CO2 and nutrient regimes. Oecologia 17, 575–583. doi: 10.1007/s00442-012-2507-5
Fernández-Torquemada Y., Carratalá A., Sánchez Lizaso J. L. (2019). Impact of brine on the marine environment and how it can be reduced. Desalination Water Treat 167, 27–37. doi: 10.5004/dwt.2019.24615
Fernández-Torquemada Y., Durako M. J., Sánchez Lizaso J. L. (2005). Effects of salinity and possible interactions with temperature and pH on growth and photosynthesis of Halophila johnsonii eiseman. Mar. Biol. 148, 251–260. doi: 10.1007/s00227-005-0075-5
Fernández-Torquemada Y., González-Correa J. M., Loya A., Ferrero L. M., Díaz-Valdés M., Sánchez-Lizaso J. L. (2009). Dispersion of brine discharge from seawater reverse osmosis desalination plants. Desalination Water Treat 5, 137–145. doi: 10.5004/dwt.2009.576
Fernández-Torquemada Y., Sánchez-Lizaso J. L. (2007). Monitoring of brine discharges from seawater desalination plants in the Mediterranean. Int. J. Environ. Health 1, 449–461. doi: 10.1504/IJENVH.2007.017870
Gao X., Kim J., Park S., Yu O., Kim Y., Choi H. (2019). Diverse responses of sporophytic photochemical efficiency and gametophytic growth for two edible kelps, Saccharina japonica and Undaria pinnatifida, to ocean acidification and warming. Mar. pollut. Bull. 142, 315–320. doi: 10.1016/j.marpolbul.2019.03.063
Garrote-Moreno A., McDonald A., Sherman T. D., Sánchez-Lizaso J. L., Heck K. L., Cebrian J. (2015a). Short-term impacts of salinity pulses on ionic ratios of the seagrasses Thalassia testudinum and Halodule wrightii. Aquat. Bot. 120, 315–321. doi: 10.1016/j.aquabot.2014.09.011
Garrote-Moreno A., Sandoval-Gil J. M., Ruiz J. M., Marín-Guirao L., Bernardeau-Esteller J., Muñoz R. G., et al. (2015b). Plant water relations and ion homoeostasis of Mediterranean seagrasses (Posidonia oceanica and Cymodocea nodosa) in response to hypersaline stress. Mar. Biol. 162, 55–68. doi: 10.1007/s00227-014-2565-9
Girotti A. W. (2001). Photosensitized oxidation of membrane lipids: reaction pathways, cytotoxic effects, and cytoprotective mechanisms. J. Photochem. Photobiol. B: Biol. 63, 103–113. doi: 10.1016/S1011-1344(01)00207-X
Grzymski J., Johnsen G., Sakshaug E. (1997). The significance of intracellular self-shading on the biooptical properties of brown, red and green macroalgae. J. Phycology 33, 408–414. doi: 10.1111/j.0022-3646.1997.00408.x
Hajiboland R. (2014). “Reactive oxygen species and photosynthesis,” in Oxidative damage to plants (San Diego, United States: Elsevier Academic Press), 1–63.
Jones E., Qadir M., van Vliet M. T. H., Smakhtin V. (2019). The state of desalination and brine production: A global outlook. Sci. Total Environ. 657, 1343–1356. doi: 10.1016/j.scitotenv.2018.12.076
Kirst G. (1990). Salinity tolerance of eukaryotic marine algae. Annu. Rev. Plant Biol. 41, 21–53. doi: 10.1146/annurev.pp.41.060190.000321
Kongrueang P., Buapet P., Roongsattham P. (2018). Physiological responses of Enhalus acoroides to osmotic stress. Botánica Marina 61, 257–267. doi: 10.1515/bot-2017-0108
Kumar M., Kumari P., Reddy C., Jha B. (2014). Salinity and desiccation induced oxidative stress acclimation in seaweeds. Adv. Botanical Res. 71, 91–123. doi: 10.1016/B978-0-12-408062-1.00004-4
Laib E., Leghouchi E. (2012). Cd, cr, Cu, Pb, and zn concentrations in Ulva lactuca, Codium fragile, Jania rubens, and Dictyota dichotoma from rabta bay, jijel (Algeria). Environ. Monit. Assess. 184, 1711–1718. doi: 10.1007/s10661-011-2072-0
Lattemann S., Höpner T. (2008). Environmental impact and impact assessment of seawater desalination. Desalination 220, 1–15. doi: 10.1016/j.desal.2007.03.009
Lesser M. P. (2006). Oxidative stress in marine environments: biochemistry and physiological ecology. Annu. Rev. Physiol. 68, 253–278. doi: 10.1146/annurev.physiol.68.040104.110001
Li Y., Liu J., Zhang L., Pang T. (2016). Changes of photosynthetic performances in mature thalli of the red alga Gelidium amansii (Gelidiaceae) exposed to different salinities. Mar. Biol. Res. 12, 631–639. doi: 10.1080/17451000.2016.1177654
Luo M. B., Liu F. (2011). Salinity-induced oxidative stress and regulation of antioxidant defense system in the marine macroalga Ulva prolifera. J. Exp. Mar. Biol. Ecol. 409, 223–228. doi: 10.1016/j.jembe.2011.08.023
Lu I. F., Sung M.-S., Lee T.-M. (2006). Salinity stress and hydrogen peroxide regulation of antioxidant defense system in Ulva fasciata. Mar. Biol. 150, 1–15. doi: 10.1007/s00227-006-0323-3
Macaya E. C., Thiel M. (2008). In situ tests on inducible defenses in Dictyota kunthii and Macrocystis integrifolia (Phaeophyceae) from the Chilean coast. J. Exp. Mar. Biol. Ecol. 354, 28–38. doi: 10.1016/j.jembe.2007.10.005
Moenne A., González A., Sáez C. A. (2016). Mechanisms of metal tolerance in marine macroalgae, with emphasis on copper tolerance in chlorophyta and rhodophyta. Aquat. Toxicol. 176, 30–37. doi: 10.1016/j.aquatox.2016.04.015
Muñoz P. T., Rodríguez-Rojas F., Celis-Plá P. S. M., López-Marras A., Blanco-Murillo F., Sola I., et al. (2023). Desalination effects on macroalgae (part b): transplantation experiments at brine-impacted sites with dictyota spp. from the pacific ocean and Mediterranean Sea. Front. Mar. Sci. 10, 1042799. doi: 10.3389/fmars.2023.1042799 doi: 10.3389/fmars.2023.1042799
Muñoz P. T., Rodríguez-Rojas F., Celis-Plá P., Méndez L., Pinto D., Pardo D., et al. (2020). Physiological and metabolic responses to hypersalinity reveal interpopulation tolerance in the green macroalga Ulva compressa with different pollution histories. Aquat. Toxicol. 225, 105552. doi: 10.1016/j.aquatox.2020.105552
Naranjo C., Sammartino S., García-Lafuente J., Bellanco M. J., Taupier-Letage I. (2015). Mediterranean Waters along and across the strait of Gibraltar, characterization and zonal modification. Deep Sea Res. Part I: Oceanographic Res. Papers 105, 41–52.
Panagopoulos A. (2022). Brine management (saline water & wastewater effluents): Sustainable utilization and resource recovery strategy through minimal and zero liquid discharge (MLD & ZLD) desalination systems. Chem. Eng. Process. - Process Intensification 176, 108944. doi: 10.1016/j.cep.2022.108944
Panagopoulos A., Giannika V. (2022). Comparative techno-economic and environmental analysis of minimal liquid discharge (MLD) and zero liquid discharge (ZLD) desalination systems for seawater brine treatment and valorization. Sustain. Energy Technol. Assessments 53, 102477. doi: 10.1016/j.seta.2022.102477
Panagopoulos A., Haralambous K. J. (2020a). Environmental impacts of desalination and brine treatment - challenges and mitigation measures. Mar. pollut. Bull. 161, 111773. doi: 10.1016/j.marpolbul.2020.111773
Panagopoulos A., Haralambous K. J. (2020b). Minimal liquid discharge (MLD) and zero liquid discharge (ZLD) strategies for wastewater management and resource recovery – analysis, challenges and prospects. J. Environ. Chem. Eng. 8 (5), 104418. doi: 10.1016/j.jece.2020.104418
Panda A., Rangani J., Kumar Parida A. (2019). Cross talk between ROS homeostasis and antioxidative machinery contributes to salt tolerance of the xero-halophyte Haloxylon salicornicum. Environ. Exp. Bot. 166, 103799. doi: 10.1016/j.envexpbot.2019.103799
Pearson G., Lago-Leston A., Valente M., Serrão E. (2006). Simple and rapid RNA extraction from freeze-dried tissue of brown algae and seagrasses. Eur. J. Phycology 41, 97–104. doi: 10.1080/09670260500505011
Piro A., Marín-Guirao L., Serra I. A., Spadafora A., Sandoval-Gil J. M., Bernardeau-Esteller J., et al. (2015). The modulation of leaf metabolism plays a role in salt tolerance of exposed to hypersaline stress in mesocosms. Front. Plant Sci. 6, 464. doi: 10.3389/fpls.2015.00464
Rodríguez-Rojas F., López-Marras A., Celis-Plá P. S. M., Muñoz P., García-Bartolomei E., Valenzuela F., et al. (2020). Ecophysiological and cellular stress responses in the cosmopolitan brown macroalga Ectocarpus as biomonitoring tools for assessing desalination brine impacts. Desalination 489, 114527. doi: 10.1016/j.desal.2020.114527
Samanta P., Shin S., Jang S., Kim J. K. (2019). Comparative assessment of salinity tolerance based on physiological and biochemical performances in Ulva australis and Pyropia yezoensis. Algal Res. 42, 101590. doi: 10.1016/j.algal.2019.101590
Sandoval-Gil J. M., Ruiz J. M., Marín-Guirao L. (2022). Advances in understanding multilevel responses of seagrasses to hypersalinity. Mar. Environ. Res. 183, 105809. doi: 10.1016/j.marenvres.2022.105809
Sandoval-Gil J. M., Ruiz J. M., Marín-Guirao L., Bernardeau-Esteller J., Sánchez-Lizaso J. L. (2014). Ecophysiological plasticity of shallow and deep populations of the Mediterranean seagrasses Posidonia oceanica and Cymodocea nodosa in response to hypersaline stress. Mar. Environ. Res. 95, 39–61. doi: 10.1016/j.marenvres.2013.12.011
Schewe J., Heinke J., Gerten D., Haddeland I., Arnell N. W., Clark D. B., et al. (2014). Multimodel assessment of water scarcity under climate change. Proc. Natl. Acad. Sci. 111, 3245–3250. doi: 10.1073/pnas.1222460110
Shahzad M. W., Burhan M., Ang L., Ng K. C. (2017). Energy-water-environment nexus underpinning future desalination sustainability. Desalination 413, 52–64. doi: 10.1016/j.desal.2017.03.009
Shetty P., Gitau M. M., Maróti G. (2019). Salinity stress responses and adaptation mechanisms in eukaryotic green microalgae. Cells 8, 1657. doi: 10.3390/cells8121657
Singh P., Khadim R., Singh A. K., Singh U., Maurya P., Tiwari A., et al. (2019). Biochemical and physiological characterization of a halotolerant Dunaliella salina isolated from hypersaline sambhar lake, India. J. Phycology 55, 60–73. doi: 10.1111/jpy.12777
Sudhir P. R., Pogoryelov D., Kovács L and Garab G., Murthy S. D. S. (2005). The effects of salt stress on photosynthetic electron transport and thylakoid membrane proteins in the cyanobacterium Spirulina platensis. J. Biochem. Mol. Biol. 38, 481–485. doi: 10.5483/BMBRep.2005.38.4.481
Underwood A. J. (1997). Experiments in ecology: their logical design and interpretation using analysis of variance (Cambridge, United Kingdom: Cambridge University Press).
Wang W., Xing L., Xu K., Ji D., Xu Y., Chen C., et al. (2020). Salt stress-induced H2O2 and Ca2+ mediate K+/Na+ homeostasis in Pyropia haitanensis. J. Appl. Phycology 32, 4199–4210. doi: 10.1007/s10811-020-02284-0
Zheng Z., Gao S., Wang G. (2019). High salt stress in the upper part of floating mats of Ulva prolifera, a species that causes green tides, enhances non-photochemical quenching. J. Phycology 55, 1041–1049. doi: 10.1111/jpy.12881
Keywords: desalination, hypersalinity, brown macroalgae, photosynthesis, oxidative stress
Citation: Muñoz PT, Rodríguez-Rojas F, Celis-Plá PSM, López-Marras A, Blanco-Murillo F, Sola I, Lavergne C, Valenzuela F, Orrego R, Sánchez-Lizaso JL and Sáez CA (2023) Desalination effects on macroalgae (part A): Laboratory-controlled experiments with Dictyota spp. from the Pacific Ocean and Mediterranean Sea. Front. Mar. Sci. 10:1042782. doi: 10.3389/fmars.2023.1042782
Received: 13 September 2022; Accepted: 03 January 2023;
Published: 03 February 2023.
Edited by:
Tao Li, Guangzhou Marine Geological Survey, ChinaReviewed by:
Argyris Panagopoulos, National Technical University of Athens, GreeceEyal Rahav, Israel Oceanographic and Limnological Research (IOLR), Israel
Copyright © 2023 Muñoz, Rodríguez-Rojas, Celis-Plá, López-Marras, Blanco-Murillo, Sola, Lavergne, Valenzuela, Orrego, Sánchez-Lizaso and Sáez. This is an open-access article distributed under the terms of the Creative Commons Attribution License (CC BY). The use, distribution or reproduction in other forums is permitted, provided the original author(s) and the copyright owner(s) are credited and that the original publication in this journal is cited, in accordance with accepted academic practice. No use, distribution or reproduction is permitted which does not comply with these terms.
*Correspondence: Claudio A. Sáez, Y2xhdWRpby5zYWV6QHVwbGEuY2w=