- 1Marine Ecological and Evolutionary Physiology Laboratory, Département de Biologie, Chimie et Géographie, Université du Québec à Rimouski, Rimouski, QC, Canada
- 2Institut Maurice-Lamontagne, Fisheries and Oceans Canada, Mont-Joli, QC, Canada
- 3ECOMARE-Laboratory for Innovation and Sustainability of Marine Biological Resources, CESAM-Centre for Environmental and Marine Studies, Department of Biology, University of Aveiro, Aveiro, Portugal
Impacts of global ocean changes on species have historically been investigated at the whole-organism level. However, acquiring an in-depth understanding of the organisms’ cellular metabolic responses is paramount to better define their sensitivity to environmental challenges. This is particularly relevant for species that experience highly different environmental conditions across their distribution range as local acclimatization or adaptation can influence their responses to rapid global ocean changes. We aimed at shedding light on the cellular mechanisms underpinning the sensitivity to combined ocean warming (OW) and acidification (OA) in the northern shrimp Pandalus borealis, from four different geographic origins defined by distinctive environmental regimes in the northwest Atlantic: i.e. St. Lawrence Estuary (SLE), Eastern Scotian Shelf (ESS), Esquiman Channel (EC) and Northeast Newfoundland Coast (NNC). We characterized targeted metabolomics profiles of the muscle of shrimp exposed to three temperatures (2, 6 or 10°C) and two pH levels (7.75 or 7.40). Overall, shrimp metabolomics profiles were modulated by a significant interaction between temperature, pH and origin. Temperature drove most of the metabolomics reprogramming, confirming that P. borealis is more sensitive to OW than OA. Inter-origin differences in metabolomics profiles were also observed, with temperature*pH interactions impacting only shrimp from SLE and ESS, pH affecting only shrimp from SLE and temperature impacting shrimp from all origins. Temperature influenced metabolomics pathways related to the tricarboxylic acid cycle (TCA) and amino acid metabolism, resulting mainly in an accumulation of TCA intermediates and tyrosine. Temperature*pH and pH in isolation only affected amino acid metabolism, leading to amino acids accumulation under low pH. However, the ratio of ATP : ADP remained constant across conditions in shrimp from all origins suggesting that their energetic status is not affected by OW and OA. Still, the accumulation of TCA intermediates and tyrosine suggests the possible enhancement of immune responses under future OW and OA conditions. Our findings suggest that shrimp from SLE are more sensitive at the molecular level, compared to others, to future complex environmental conditions. This underlines the importance of investigating intraspecific variation in mechanisms of responses to combined drivers when trying to define species’ sensitivity to global ocean changes.
1 Introduction
The rising concentration of anthropogenically emitted carbon dioxide (CO2) in the atmosphere directly affects the marine environment via the decrease of global mean seawater pH and change in carbonate chemistry (ocean acidification, OA) (Caldeira and Wickett, 2003; IPCC, 2022). Indirectly, the increase of greenhouse gasses leads to rising seawater temperatures, a phenomenon known as ocean warming (OW) (IPCC, 2022). According to the most pessimistic, but also most realistic, scenario of the Intergovernmental Panel on Climate Change (IPCC, 2022), both OA and OW are predicted to worsen by the end of the century (+4°C and -0.3 pH units mean globally) posing a major threat to marine biodiversity, particularly to calcifying organisms and ectotherms (Orr et al., 2005; Paaijmans et al., 2013; Lefevre, 2016). Survival, metabolism, growth, reproduction and behaviour are some of the traits affected by OW and OA, as observed at the whole-organism level: which happen to be the most studied traits in global change biology to define species’ sensitivity to global changes. However, to better understand the mechanisms underlying the responses to environmental changes at higher levels of biological organization (from whole-organism to species, communities and ecosystems), it is necessary to elucidate and integrate the responses at lower levels of biological organization, i.e. cellular and molecular levels (Bartholomew, 1964; Harvey et al., 2014). In this context, the use of state-of-the-art molecular tools, such as -omics techniques, are of great interest as they allow the determination of which molecules and ultimately which cellular pathways play an important role in the response of organisms exposed to different climate change scenarios (Jones et al., 2013; Pinu et al., 2019; Cappello, 2020; Ebner, 2021). In particular, metabolites are the end products of cellular regulatory processes that involve multiple interactions among genes, transcripts, proteins and the environment. Therefore, metabolites are considered to be representative of cellular, organs, and organism phenotypes (Fiehn, 2002). Recently, the use of metabolomics analyses, i.e. the identification and quantification of targeted or all metabolites of a biological system (Bundy et al., 2009), has increased in studies investigating the responses of organisms to environmental changes (Williams et al., 2009; Mayor et al., 2015; Aru et al., 2017; Wu et al., 2017; Li et al., 2020a; Li et al., 2020b), and specifically to global change drivers such as OW, OA and ocean deoxygenation, in isolation or combined (e.g. Ellis et al., 2014; Wei et al., 2015; Zhang et al., 2017; Huo et al., 2019). Indeed, in multiple cases, global change drivers have been shown to induce a shift in metabolic pathways, phenomenon known as metabolic reprogramming, and to affect the energy metabolism of organisms (Lannig et al., 2010; Liu et al., 2020; Noisette et al., 2021; Dong et al., 2022; Thor et al., 2022). Elevated levels of succinate, fumarate and malate suggest a disruption of the tricarboxylic acid cycle (TCA) that, together with elevated levels of lactate, support the hypothesis of a shift from aerobic to anaerobic metabolism (Williams et al., 2009; Wei et al., 2015; Clark et al., 2017; Huo et al., 2019; Noisette et al., 2021). However, metabolism not only changes in response to the effect of ocean global drivers, but it is also shown to vary among populations of the same species. For example, populations of the common periwinkle Littorina littorea in the northeast Atlantic appeared metabolically adapted to the regional environmental conditions they experience. This was shown by differences in their metabolic rates and metabolomics profiles that influenced their whole-organism response to OA (Calosi et al., 2017). Differences in metabolic rates among populations, and in their response to OW and OA, have been shown in a number of marine species (Di Santo, 2015; Vargas et al., 2017; Thor et al., 2018). Therefore, multi-population studies need to be conducted to accurately and reliably determine species’ sensitivity to future ocean conditions, to help identifying the mechanisms underpinning known whole-organism physiological responses. This is especially true for species with a wide range of distribution and of ecological and commercial relevance. A good example is the northern shrimp Pandalus borealis. This species is widely distributed in the north Atlantic (Bergström, 2000), where it is a major prey for many fish and marine mammal predators of these ecosystems and supports important fisheries (Hammill and Stenson, 2000; Dawe et al., 2012; Jónsdóttir et al., 2012). In particular, it is the third most lucrative marine fishery in the Canadian waters of the northwest Atlantic (DFO, 2021a). The northern shrimp has been shown to be sensitive to both OW and OA (Brillon et al., 2005; Chabot and Ouellet, 2005; Daoud et al., 2007; Bechmann et al., 2011; Arnberg et al., 2013; Hammer and Pedersen, 2013; Chemel et al., 2020). In addition, it appears to be potentially long-term acclimatized or adapted to regional environmental conditions throughout the northwest Atlantic (Koeller et al., 2009; Ouellet et al., 2017). Indeed, average bottom waters, where shrimp are most abundant, are characterized by higher temperatures and lower pH levels (∼6-7°C, ∼7.6 pH units) in the Gulf of St. Lawrence compared to the deep waters outside the St. Lawrence system (∼0-3°C, ∼7.8-8 pH units) (DFO, 2021b; Bourdages et al., 2022; Cyr et al., 2022; DFO, 2022a; DFO, 2022b). Our study aimed at shedding light on the cellular mechanisms underpinning the physiological responses of the northern shrimp from different geographic origins with distinctive environmental conditions, under combined OW and OA scenarios predicted to occur at the end of the century, to characterize the intraspecific variation in metabolic pathways across conditions. To do so, we exposed female shrimp from four different geographic origins within the northwest Atlantic (i.e. St. Lawrence Estuary, Eastern Scotian Shelf, Esquiman Channel and Northeast Newfoundland Coast) to different combinations of OW and OA, and we identified key metabolites associated to the aerobic and anaerobic metabolism to perform targeted metabolomics analyses. Based on our current knowledge, we hypothesize that shrimp from different origins will show different metabolomics profiles under exposure to combined OW and OA, but all possibly showing a shift from aerobic to anaerobic metabolism.
2 Material and methods
2.1 Ethical statement
All procedures complied with Canadian legislation for animal experimentation as the Canadian Council for Animal Care does not require projects using crustaceans to be approved by an Animal Care Committee, thus an ethics approval was not required for this study.
2.2 Shrimp collection and experimental design
The analyses of key targeted metabolites associated to the aerobic and anaerobic metabolism was carried out on abdominal muscle samples of female shrimp P. borealis previously exposed to isolated and combined OW and OA scenarios (Guscelli et al. under review). Female shrimp were selected because they are the main target of the fishery, they have a greater role in defining population demography and are more sensitive compared to males to ocean global change drivers (Dupont-Prinet et al., 2013). Moreover, we selected only non-ovigerous females to avoid any potential confounding effect linked to differences in reproductive stages and the oxygen consumption of the egg mass. Briefly, shrimp were collected from four different geographic origins within the northwest Atlantic: i.e. St. Lawrence Estuary (SLE, 48° 35’ N, 68° 35’ W; May 2018), Eastern Scotian Shelf (ESS, 45° 23’ N, 61° 04’ W; February 2019), Esquiman Channel (EC, 50° 44’ N, 57°29’ W; July 2019) and Northeast Newfoundland Coast (NNC, 50° 18’ N, 54° 16’ W; November 2019) with shrimp trawls or traps. At depths where shrimp were sampled temperature and pH conditions are stable year-round, but subjected to slow inter-annual changes. Shrimp were then transported to the Maurice-Lamontagne Institute (MLI), Fisheries and Oceans Canada (Mont-Joli, QC, Canada) where they were maintained in rearing tanks (1700 L) for approximately eight weeks before the beginning of the experiment. Average conditions during this period were 4.5°C, pH 7.9, 100% oxygen saturation relative to air and salinity 32. Shrimp were then exposed for 30 d to one of six treatments (with two replicate tanks per treatment) combining temperatures and pH levels representing the favourable, actual, or future scenario in the northwest Atlantic. In detail, 2°C was chosen as favourable temperature for this species (Shumway et al., 1985), while 6°C represents the recent temperature of shrimp habitat in the Gulf of St. Lawrence (GSL) (Bourdages et al., 2022) and a +4°C predicted globally for the end of the century (RCP 8.5 scenario, IPCC, 2014) for other origins, such as ESS and NNC, and 10°C corresponds to the +4°C increase for GSL shrimp (IPCC, 2014). A pH of 7.75 was selected based on the current conditions of the deep waters of the Estuary and Gulf of St. Lawrence (Mucci et al., 2011; Mucci et al., 2018) and approximatively the -0.3 pH units decrease scenario predicted to occur globally by the year 2100 (RCP 8.5 scenario, IPCC, 2014) for other origins, such as ESS and NNC, while the pH of 7.40 was chosen to correspond to the -0.3 pH units drop for GLS shrimp (IPCC, 2014). Treatments were identified as low temperature and current pH (2C: 2°C, pH 7.75), low temperature and low pH (2A: 2°C, pH 7.40), intermediate temperature and current pH (6C: 6°C, pH 7.75), intermediate temperature and low pH (6A: 6°C, pH 7.40), elevated temperature and current pH (10C: 10°C, pH 7.75), elevated temperature and low pH (10A: 10°C, pH 7.40). At the end of the exposure period, we measured shrimp oxygen uptake as a proxy for metabolic rates via intermittent-flow respirometry on five individual per tank (10 per treatment) (results are presented in Guscelli et al. under review). At the end of the respirometry trials we rapidly dissected shrimp on ice and the abdomen muscle was carefully placed in microtubes (Eppendorf, Germany) and flash-frozen in liquid nitrogen to instantly interrupt all biochemical reactions. This procedure was conducted rigorously and rapidly, lasting less than a minute, in order to minimize the negative effects of handling and standardise them (if any) across all treatment and origin combinations. Samples were then stored at -80°C pending analyses.
Shrimp that moulted and/or died during respirometry measurements were considered as unstable samples for metabolomics analysis and they were thus removed from the dataset.
Maintenance of shrimp from all geographic origins and monitoring of temperature and pH in the experimental setup were similar to those detailed for shrimp of SLE in Chemel et al. (2020). For details on the physico-chemical parameters for the duration of the 30-d experiment see Guscelli et al. (under review).
2.3 Metabolite extraction and quantification
Metabolite extraction was carried out by Les laboratoires Iso-BioKem Inc. following the method established in their laboratory. Briefly, samples were first freeze-dried for 24 h and then divided in two centrifugal PP tubes (1.5 mL) to carry out the extraction for positive and negative analyses separately. Each part was then weighted, to later normalize metabolite concentration, and homogenized at 6000 rpm for 30 s at –4°C (Precellys 24 with cryolis cooling unit, Bertin corporation, Montigny-le-Bretonneux, France). Once the extraction solution was added, samples were vortexed for 10 s and centrifuged at 30130 ×g for 5 min at 5°C to collect and transfer 250 μL of supernatant in an amber HPLC vial with insert (Wheaton, NJ, USA) to analyse the fresh extract. Finally, 225 μL of the supernatant were injected in a high-performance liquid chromatography system (HPLC 1260 Infinity II, Agilent Technologies, Santa Clara, CA, USA) coupled to a mass spectrometer (6420 Triple Quad, Agilent Technologies) to detect targeted metabolites. The absolute quantification of these metabolites (in ng mL-1) was assessed with the MassHunter QQQ quantitative (Quant-my-Way) software (Agilent Technologies) using a calibration curve previously created using Phenylalanine-d8 and Fumarate-d4 standards (Cambridge Isotope Laboratories, Tewksbury, MA, USA).
2.4 Data pre-processing
Raw data matrices were normalized by wet weight and final concentration values were expressed as ng metabolite mg−1 wet weight. As LC-MS metabolomics datasets can contain a high amount of missing values (Wei et al., 2018), the matrices were first imported in the web tool Metlmp 1.2 (https://metabolomics.cc.hawaii.edu/software/MetImp/, accessed in September 2021) for missing data imputation. As our missing data were due to limit of quantification (LOQ) of the technique, we followed the protocol proposed by Wei et al. (2018) for the ‘Missing not at random (MNAR)’ type of missing values. Briefly, we used the QRILC algorithm (Quantile Regression Imputation of Left-Censored Data) with 40% group wise missing filtering to input missing values (seed 1234). We decided to increase the threshold to 40% missing values in order to keep more metabolites for the statistical analyses and only rejected 14 metabolites out of 48 identified. Finally, the database was imported into Metaboanalyst 5.0 (http://www.metaboanalyst.ca/, accessed in October 2021) to be log-transformed, to reduce lack of normality and heteroscedasticity in the data, and auto-scaled, to adjust for differences in fold-changes between metabolites.
2.5 Data analysis
Unsupervised principal components analysis (PCA) was applied to explore data structure (FactoMineR package, Husson et al., 2010) and one outlier was removed from the complete dataset. Then, to test the isolated and combined effects of elevated seawater temperature, low pH and geographic origin on metabolomics profiles of shrimp muscle tissue, we performed a three-way Permutational Multivariate Analysis of Variance (PERMANOVA). The PERMANOVA was applied to the data resemblance matrix (based on Euclidean distance) and was run using type III sums of squares and permutation of residuals under a reduced model (9999 permutations) using the PERMANOVA + add-on in Primer-E v6 (Anderson et al., 2008).
Multivariate and univariate analyses were performed to identify metabolites that discriminate between different temperatures and pH levels, within each geographic origin. Four PCAs were carried out to explore data structure, one per geographic origin. Additionally, to test for the effect of temperature, pH and their interaction within each geographic origin, a two-way analysis of variance (ANOVA) with a False Discovery Rate (FDR) cut-off set at 0.05 for significance was carried out for each metabolite on the database (specimen package, Costa et al., 2017). As commonly done when a high number of comparisons exists, the FDR was applied to correct p-values (separately for each origin and each factor) for 34 tests. When one of these tests was significant according to FDR, Tukey post-hoc tests were used to identify significant differences using the HSD.test or the glht function for isolated or interactive effects respectively (Hothorn et al., 2008; Bretz et al., 2016). Analyses were performed using the software R 4.1.1 version (R Core Team, 2020). Finally, significant metabolites identified by the ANOVAs were then used for pathway analysis to assess the most relevant metabolic pathways involved in the response of shrimp from different geographic origins to temperature increases and pH decreases. To do so, the chosen metabolites were imputed in the “Pathway Analysis” tool of the MetaboAnalyst software. As there are no libraries available for shrimp, we chose the pathway library of a model organism within the same phylum (Arthropoda), namely Drosophila melanogaster (fruit fly). Pathway analysis was carried out based on functional enrichment, assessed using hypergeometric tests for over-representation analysis (p < 0.05), and pathway topology analysis, implemented using the relative betweenness centrality (> 0.1). Pathways were considered relevant according to either over-representation p < 0.05 or pathway topology analysis with impact > 0.1 and most relevant according to the combination of both.
Finally, linear models were used to test the effects of isolated and combined seawater temperature and pH, and geographic origin (fixed factors) on shrimp ATP : ADP ratio, which was calculated and analysed a posteriori to verify the cellular energetic status.
3 Results
3.1 Whole metabolome
The overall PCAs, applied to explore the general data structure by factor (geographic origin, temperature, pH and temperature*pH treatments), did not show clear group separation (Supplementary Figure 1).
However, temperature and pH significantly and differently modulated the metabolomics profiles of shrimp from the four geographic origins as indicated by the presence of a significant interaction among origin, temperature and pH (Table 1).
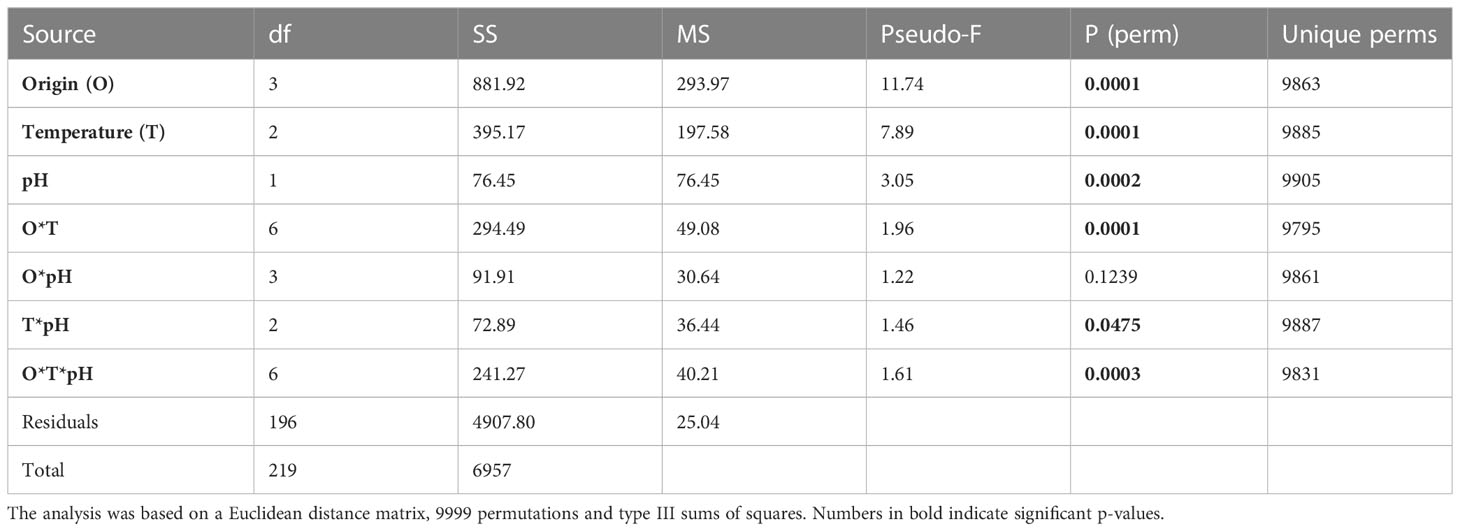
Table 1 Summary of the results of the PERMANOVA test employed to investigate the effects of origin, temperature and pH and their interactions on the northern shrimp muscle metabolomics profiles.
3.2 Origins
For each origin, the PCAs did not show a clear group separation as some overlapping was found among temperature*pH treatments (Figure 1). However, some differences in metabolomics profiles of shrimp exposed to current and low pH levels were shown for shrimp from the SLE (Figure 1), while some differences between the lowest and highest temperatures were shown for the metabolomics profiles of shrimp from the three other origins (Figure 1). Furthermore, for all geographic origins, between seven and 12 metabolites were found to change significantly among temperature, pH and temperature*pH treatments (Supplementary Table 1). Three metabolites of shrimp from the SLE and one metabolite of shrimp from ESS differed among temperature*pH treatments as indicated by the presence of a significant interaction between temperature and pH (Table 2). However, no effect of this interaction was found to be significant on metabolomics profiles of shrimp from EC and NNC (Table 2). Additionally, temperature alone was found to significantly affect the metabolomics profiles of shrimp from all geographic origins whilst pH in isolation was found to significantly affect the metabolomics profiles of shrimp from SLE (Table 2).
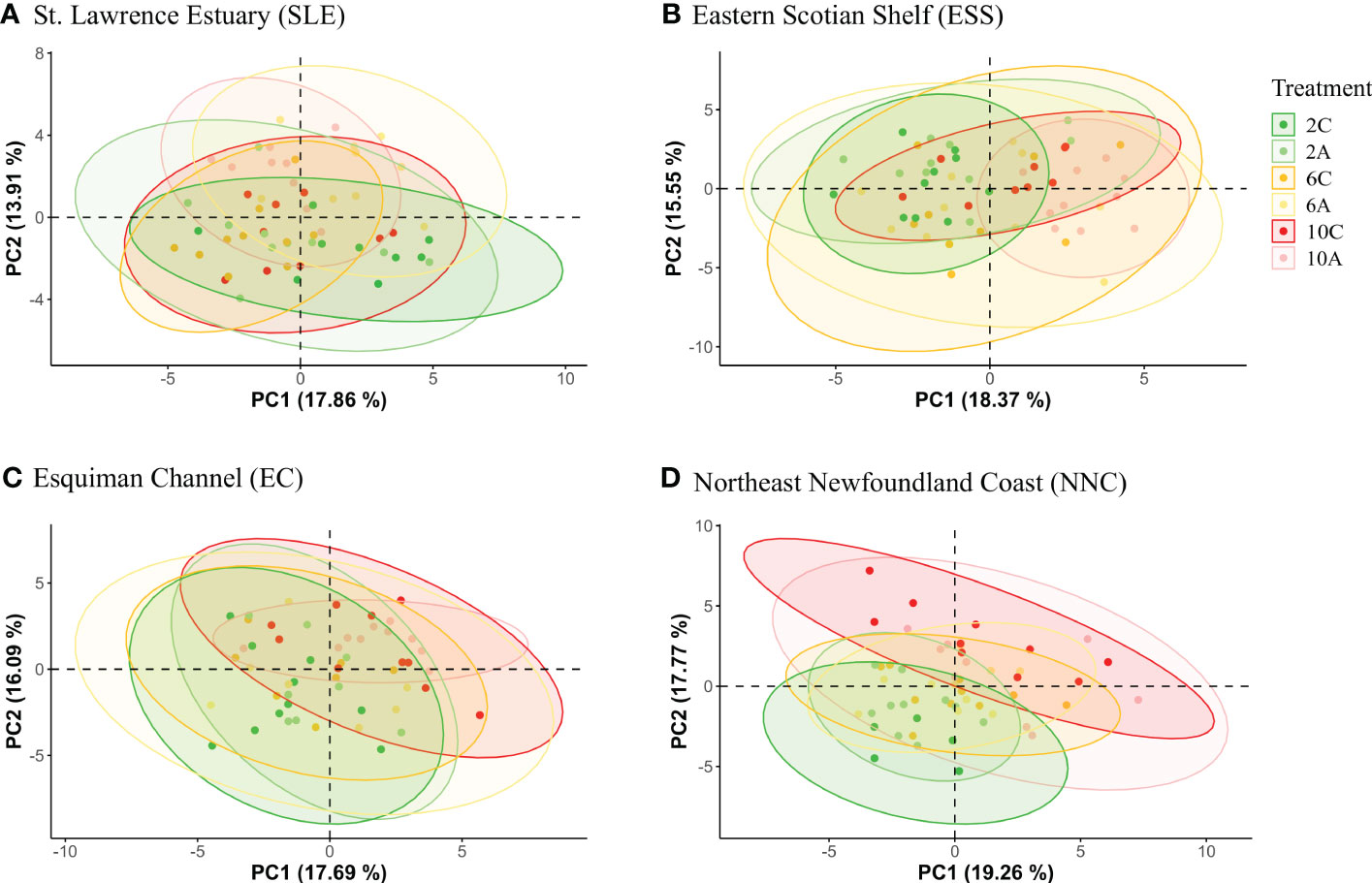
Figure 1 PCA 2D score plot with 95% confidence ellipse representing the variation in metabolomics profiles in the northern shrimp Pandalus borealis according to temperature*pH treatments: 2C (2°C, pH 7.75), 2A (2°C, pH 7.40), 6C (6°C, pH 7.75), 6A (6°C, pH 7.40), 10C (10°C, pH 7.75) and 10A (10°C, pH 7.40) for (A) St. Lawrence Estuary (SLE), (B) Eastern Scotian Shelf (ESS), (C) Esquiman Channel (EC) and (D) Northeast Newfoundland Coast (NNC).
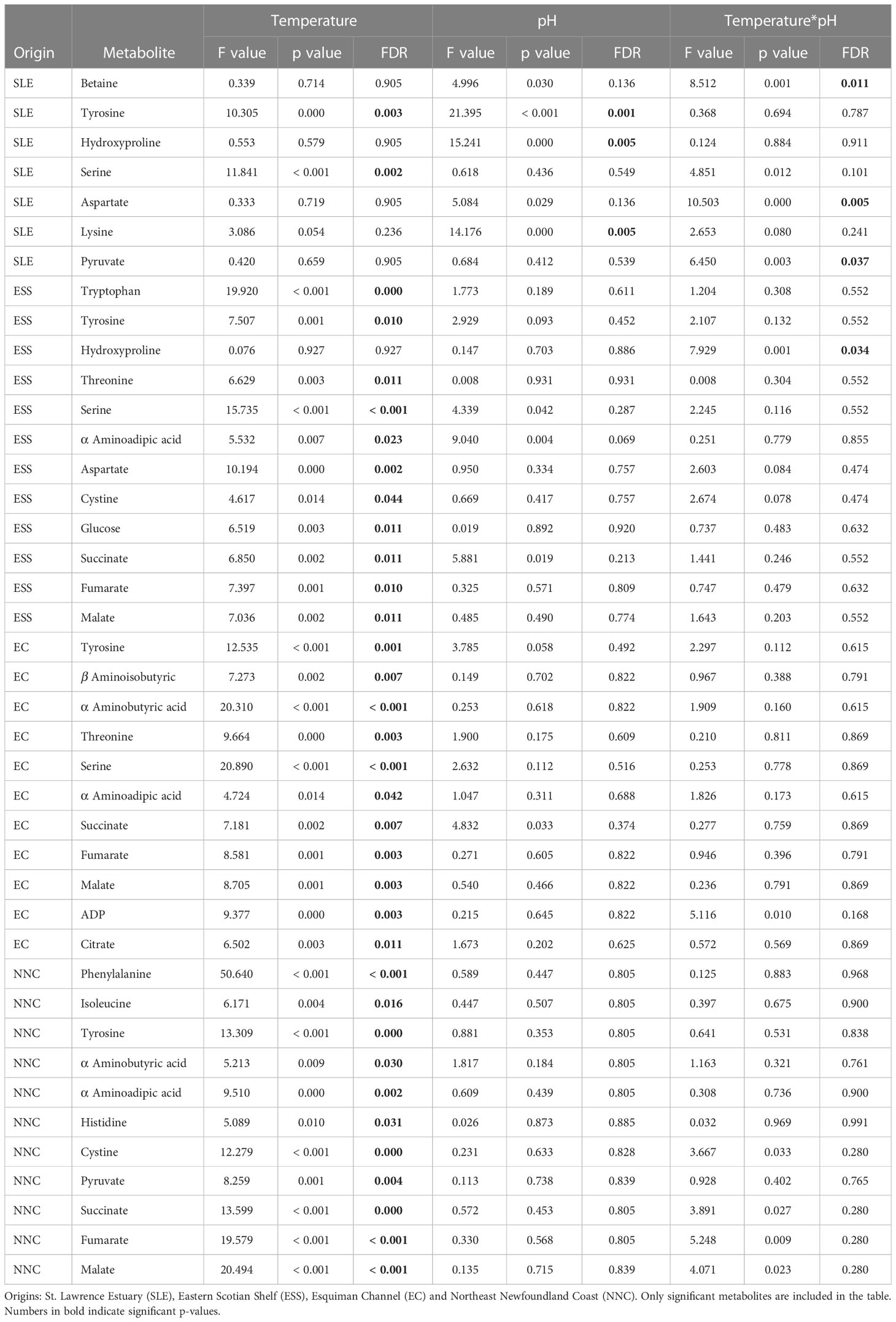
Table 2 Summary of the results of the ANOVA tests used to investigate the effects of temperature and pH and their interaction on metabolites quantified in the northern shrimp muscle samples.
3.2.1 SLE
i) The concentration of three metabolites differed significantly among temperature*pH treatments as indicated by the presence of a significant interaction between temperature and pH (Table 2). Pathway analysis showed that these metabolites were involved in five relevant pathways, including: amino acid and pyruvate metabolism and arginine biosynthesis (Figure 2A). The most relevant pathway was the alanine, aspartate and glutamate metabolism (Figure 2A). In this pathway, aspartate mean concentration increased between current and low pH at the intermediate temperature (Figure 2A). Despite the fact that significant effects were evidenced by the ANOVA on pyruvate, multiple comparisons tests between treatments failed to detect significant differences among groups.
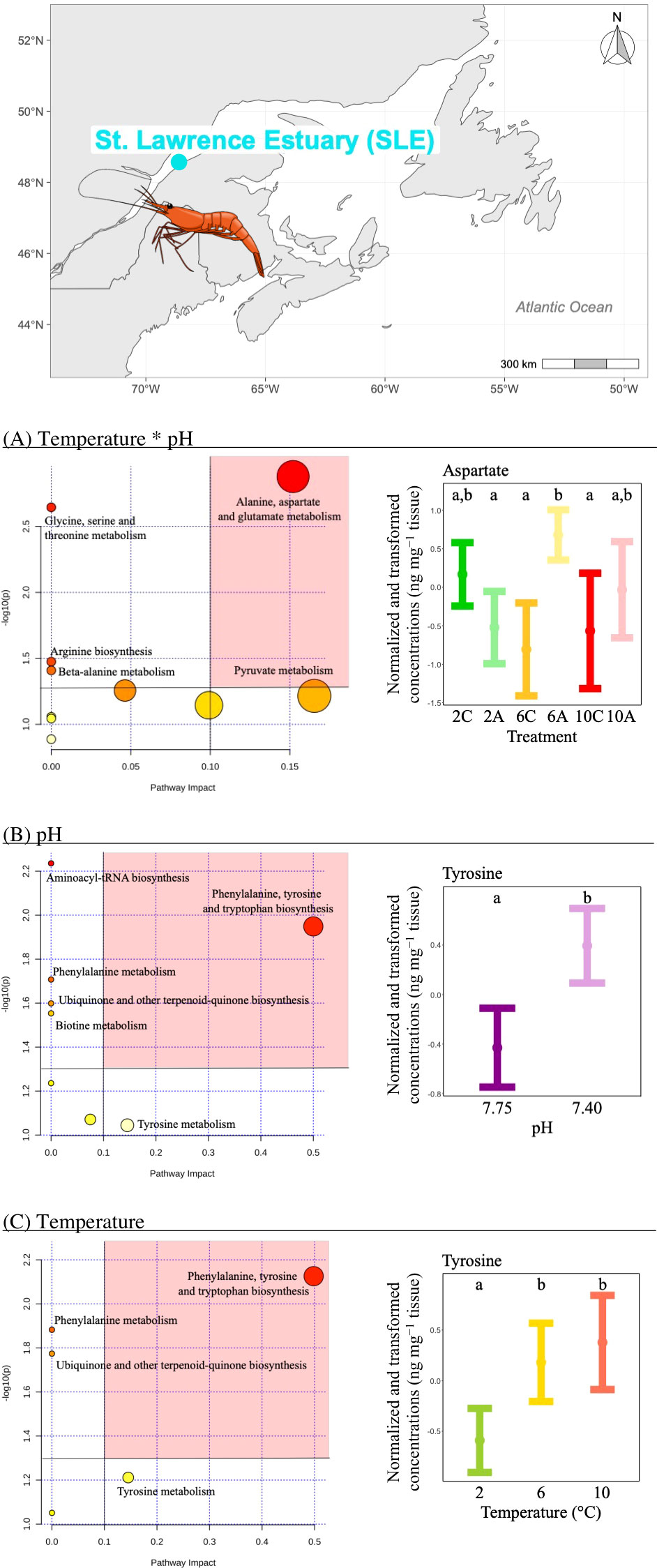
Figure 2 Metabolomics differences due to isolated and combined effects of seawater temperature and pH in the northern shrimp P. borealis from the St. Lawrence Estuary (SLE). In more detail, at the top of the figure, we show the map representing the collection site and at the left results for the pathway analysis of metabolic changes carried out with the statistically significant metabolites (ANOVA p < 0.05) due to (A) the combined effect of seawater temperature and pH, (B) the isolated effect of seawater pH and (C) the isolated effect of seawater temperature. Pathways within the red area were considered the most relevant as they have p < 0.05 and impact > 0.1. On the right, (A) mean and 95% CI for aspartate concentration for each temperature*pH treatments: 2C (green, N=9), 2A (light green, N=10), 6C (yellow, N=10), 6A (light yellow, N=9), 10C (red, N=9) and 10A (light red, N=9); (B) mean and 95% CI for tyrosine concentration for each pH treatment: 7.75 (purple, N=28) and 7.40 (light purple, N=28), combining all temperatures; (C) mean and 95% CI for tyrosine concentration for each temperature treatment: 2 (green, N=19), 6 (yellow, N=19) and 10°C (red, N=18), combining all pH levels. Lower case letters identify significant differences (p < 0.05) among treatments.
ii) The concentration of three metabolites differed significantly between pH treatments (Table 2), and pathway analysis showed that these metabolites were involved in six relevant pathways, including: amino acid biosynthesis and metabolism, aminoacyl-tRNA biosynthesis, ubiquinone and other terpenoid-quinone biosynthesis and biotin metabolism (Figure 2B). The most relevant pathway was the phenylalanine, tyrosine and tryptophan biosynthesis (Figure 2B). In this pathway, tyrosine mean concentration increased at low pH (Figure 2B).
iii) The concentration of two metabolites differed significantly among temperature treatments (Table 2), and the pathway analysis showed that these metabolites were involved in four relevant pathways, including: amino acid biosynthesis and metabolism and ubiquinone and other terpenoid-quinone biosynthesis (Figure 2C). The most relevant pathway was the phenylalanine, tyrosine and tryptophan biosynthesis (Figure 2C). Within this pathway, tyrosine mean concentration increased with increasing temperature being the highest and similar at the two highest temperature treatments (Figure 2C).
3.2.2 ESS
i) Only the concentration of hydroxyproline differed significantly among temperature*pH treatments as indicated by the presence of a significant interaction between temperature and pH (Table 2), and the pathway analysis showed that this metabolite was involved in the arginine and proline metabolism, despite the fact that MetaboAnalyst was unable to produce a pathway figure due to the input metabolite list being only one metabolite. Moreover, multiple comparisons tests between treatments failed to detect significant differences in hydroxyproline concentrations among groups.
ii) The concentration of 12 metabolites differed significantly among temperature treatments (Table 2), and the pathway analysis showed that these metabolites were involved in eight relevant pathways, including: citrate cycle, amino acid biosynthesis and metabolism, aminoacyl-tRNA biosynthesis and pyruvate metabolism (Figure 3). Among these, four were the most relevant pathways: the citrate cycle, the alanine, aspartate and glutamate metabolism, the phenylalanine, tyrosine and tryptophan biosynthesis and the tyrosine metabolism (Figure 3). Within these most relevant pathways, malate, fumarate and succinate mean concentrations increased with increasing temperature being the highest and similar at the two highest temperature treatments (Figure 3). Conversely, tyrosine mean concentrations measured at the two lowest temperatures tested were similar but lower than mean concentration at the highest temperature condition (Figure 3). Similarly, aspartate mean concentration significantly increased between 6 and 10°C and the mean concentration measured at the lowest temperature was comparable to the one measured at the two highest temperatures tested (Figure 3).
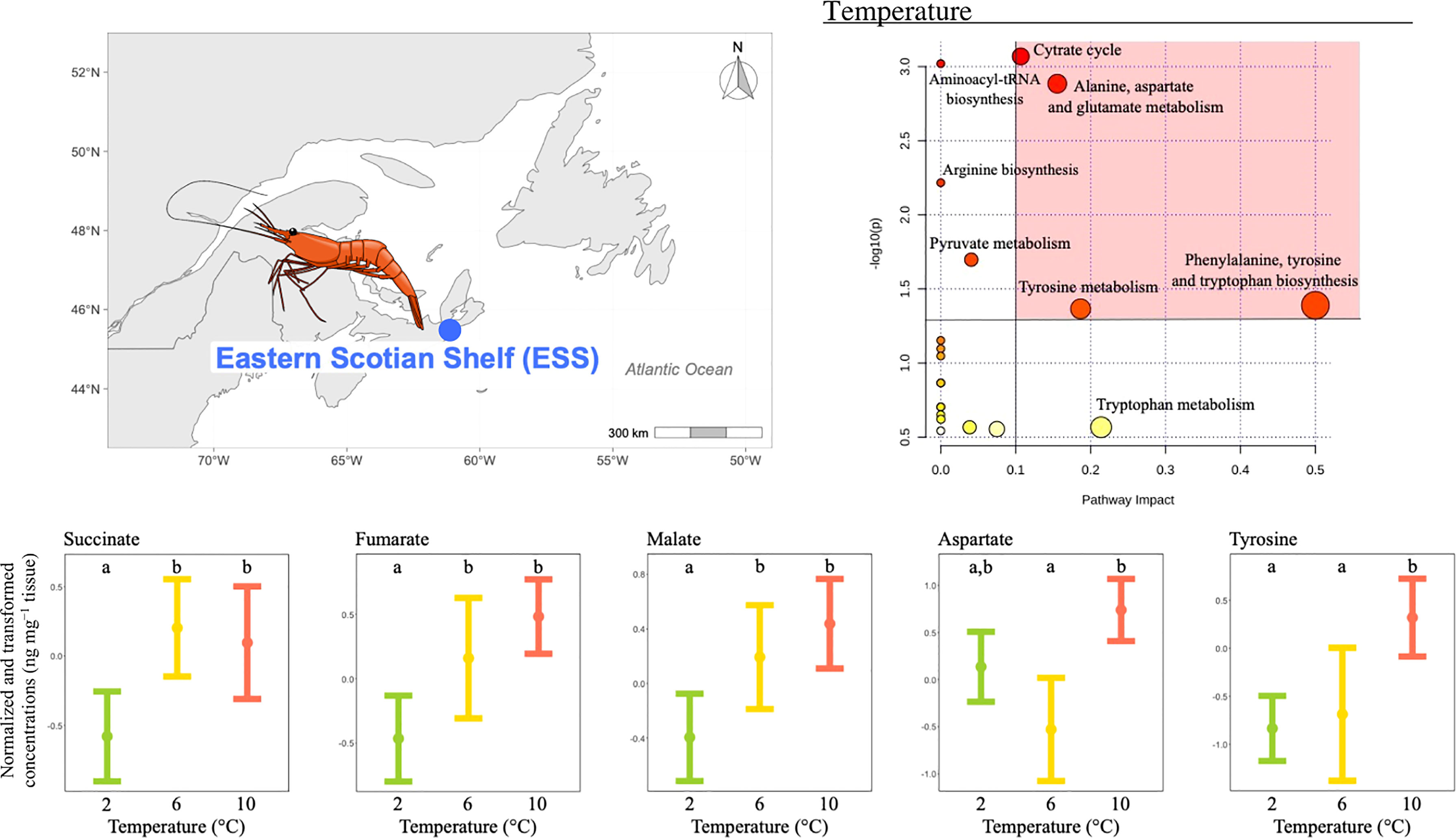
Figure 3 Metabolomics differences among temperatures in the northern shrimp P. borealis from the Eastern Scotian Shelf (ESS). In more detail, at the top left of the figure we show the map representing the collection site and at the top right of the figure we show the pathway analysis of metabolic changes carried out with the statistically significant metabolites (ANOVA p < 0.05). Pathways within the red area were considered the most relevant as they have p < 0.05 and impact > 0.1. At the bottom of the figure we show the mean concentration and 95% CI for succinate, fumarate, malate, aspartate and tyrosine for each temperature treatment: 2 (green, N=20), 6 (yellow, N=19) and 10°C (red, N=19), combining all pH levels. Lower case letters identify significant differences (p < 0.05) among treatments.
3.2.3 EC
The concentration of 11 metabolites differed significantly among temperature treatments (Table 2), and the pathway analysis showed that these metabolites were involved in six relevant pathways, including: citrate cycle, amino acid biosynthesis and metabolism, pyruvate metabolism and glyoxylate and dicarboxylate metabolism (Figure 4). Among these, three were the most relevant pathways: the citrate cycle, the phenylalanine, tyrosine and tryptophan biosynthesis and the tyrosine metabolism (Figure 4). Within these pathways, malate, fumarate, succinate, citrate and tyrosine mean concentrations all increased with increasing temperature. In more detail, malate, fumarate and citrate mean concentrations measured at the two lowest temperatures tested were similar and the lowest compared to the mean reported at the highest temperature condition (Figure 4). Differently, tyrosine mean concentrations measured at the two highest temperatures were significantly higher than that reported at the lowest temperature condition, but comparable to each other (Figure 4). Finally, succinate mean concentration measured at the highest and lowest temperatures were significantly different from each other, and both comparable to the mean measured at the intermediate temperature (Figure 4).
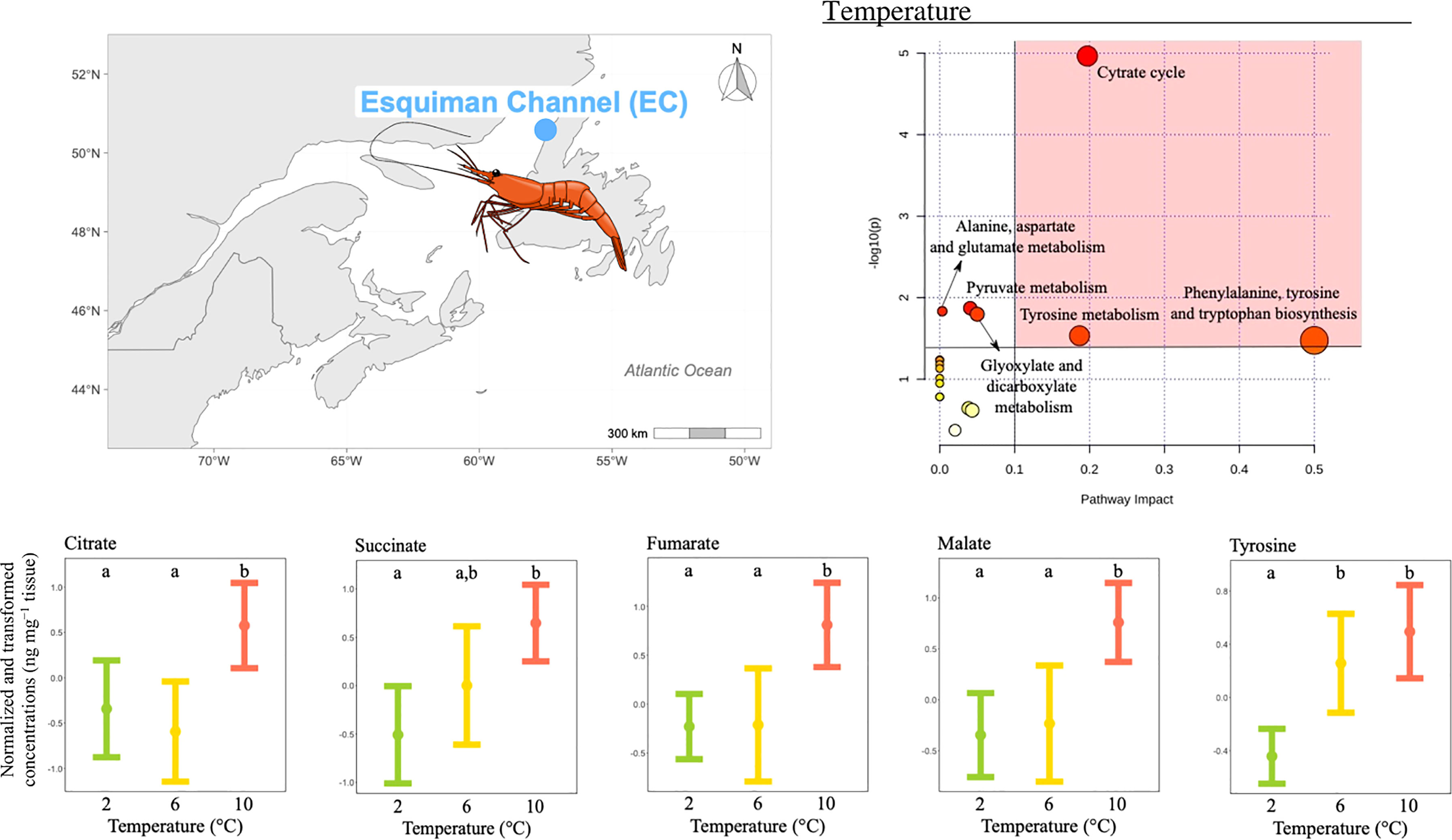
Figure 4 Metabolomics differences among temperatures in the northern shrimp P. borealis from the Esquiman Channel (EC). In more detail, at the top left of the figure we show the map representing the collection site and at the top right of the figure we show the pathway analysis of metabolic changes carried out with the statistically significant metabolites (ANOVA p < 0.05). Pathways within the red area were considered the most relevant as they have p < 0.05 and impact > 0.1. At the bottom of the figure we show the mean concentration and 95% CI for citrate, succinate, fumarate, malate and tyrosine for each temperature treatment: 2 (green, N=18), 6 (yellow, N=14) and 10°C (red, N=20), combining all pH levels. Lower case letters identify significant differences (p < 0.05) among treatments.
3.2.4 NNC
The concentration of 11 metabolites differed significantly among temperature treatments (Table 2), and the pathway analysis showed that these metabolites were involved in nine relevant pathways, including: citrate cycle, amino acid biosynthesis and metabolism, aminoacyl-tRNA biosynthesis and pyruvate metabolism (Figure 5). Among these, five were the most relevant pathways: the citrate cycle, the phenylalanine, tyrosine and tryptophan biosynthesis, the tyrosine metabolism, phenylalanine metabolism and the pyruvate metabolism (Figure 5). In these pathways, metabolites such as malate, fumarate, succinate, pyruvate, phenylalanine and tyrosine mean concentrations increased with increasing temperature. In more detail, succinate and pyruvate mean concentration were similar at the two lowest temperatures tested, but lower than the mean concentration at the highest temperature condition (Figure 5), whilst mean concentration for all other metabolites significantly differed among all temperatures tested (Figure 5).
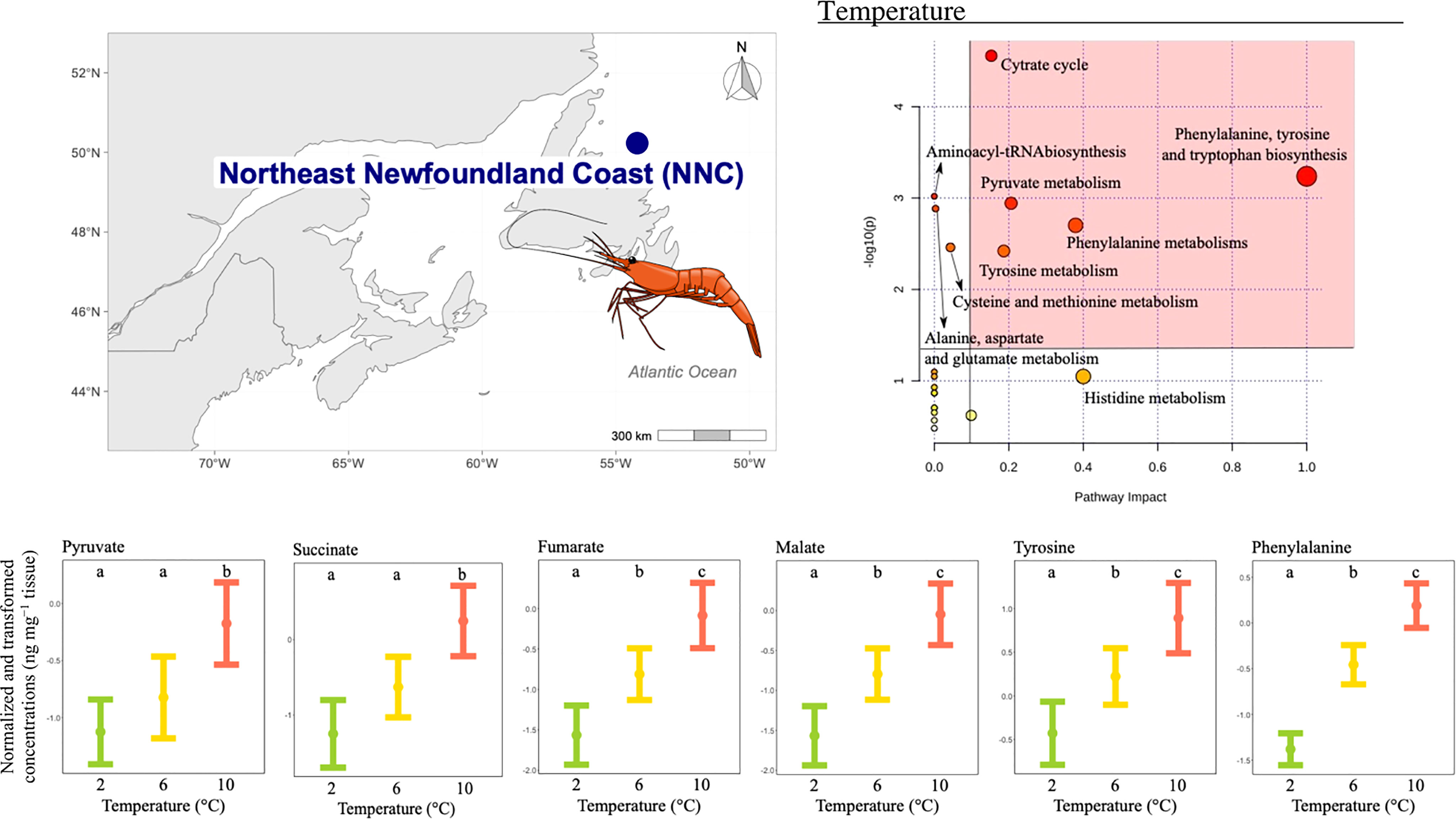
Figure 5 Metabolomics differences among temperatures in the northern shrimp P. borealis from the Northeast Newfoundland Coast (NNC). In more detail, at the top left of the figure we show the map representing the collection site and at the top right of the figure we show the pathway analysis of metabolic changes carried out with the statistically significant metabolites (ANOVA p < 0.05). Pathways within the red area were considered the most relevant as they have p < 0.05 and impact > 0.1. At the bottom of the figure we show the mean concentration and 95% CI for pyruvate, succinate, fumarate, malate, tyrosine and phenylalanine for each temperature treatment: 2 (green, N=15), 6 (yellow, N=20) and 10°C (red, N=19), combining all pH levels. Lower case letters identify significant differences (p < 0.05) among treatments. .
3.3 ATP : ADP ratio
No significant effect of temperature, pH and their interaction was found on the ATP : ADP ratio of shrimp from any origin (minimum p-value = 0.6339).
4 Discussion
Here, we show that the ecologically and economically important northern shrimp undergoes metabolomics reprogramming under future global change scenarios, shrimp from different origin responding differently to the combined exposure to temperature and pH. This said, temperature drives most of the responses, followed by a modest effect of the interaction between temperature and pH and almost no effect of pH. These results confirm that the northern shrimp is more sensitive to OW than to OA, similarly to what has been reported for other marine organisms (e.g. Schalkhausser et al., 2014; Noisette et al., 2016; Matoo et al., 2021). Additionally, inter-origin differences are observed, as combined effects of temperature and pH are only detected in SLE and ESS shrimp, and the isolated effect of pH is only evident in SLE shrimp. Despite these inter-origin differences, the metabolomics changes observed are comparable and all related to the regulation of the aerobic energy production pathway and amino acid metabolism, with environmental drivers mostly impacting metabolites of the tricarboxylic acid cycle (TCA). While these metabolites are intrinsically linked to energy metabolism, recent studies emphasize that TCA intermediates participate in a wide range of cellular processes (Martínez-Reyes and Chandel, 2020; Choi et al., 2021). Based on our previous results on P. borealis physiological responses to global change drivers (Guscelli et al. under review) and the present study, we propose that modulation of TCA intermediates and amino acids may be an important mechanism regulating immune and stress responses of shrimp under global change scenarios.
Overall, the northern shrimp metabolomics profiles are mainly affected by temperature. Indeed, only metabolites concentrations of shrimp from SLE changed under exposure to low pH or its combination with temperature, suggesting that shrimp from SLE are more sensitive to OA than shrimp from other origins, at the molecular level. These results are coherent with the results obtained previously at higher levels of biological organization, which confirm that overall adults of P. borealis are more sensitive to OW than OA (Chemel et al., 2020; Guscelli et al. under review; Hammer and Pedersen, 2013). Interestingly, the SLE shrimp response to OW and OA is the most divergent when compared to those of shrimp from all other origins. In fact, in shrimp from SLE, the alanine, aspartate and glutamate metabolism pathway is affected but the TCA pathway is not. These results suggest that the responses of shrimp from SLE differ from the ones of shrimp from other origins at multiple levels of biological organization: metabolic, cellular and whole-organism. Indeed, we previously reported that shrimp from SLE possess the worst general physiological condition as they show the highest maximum metabolic rate associated to the lowest cellular energetic capacity (Guscelli et al. under review). Moreover, the differences in metabolomics profiles of shrimp from different origins exposed to combined global change drivers, support the previous suggestion of a long-term acclimatization or adaptation of this species to different regional environmental conditions (Guscelli et al. under review). Although we recognize that inter-origin differences could be influenced by the potential effect of seasonality on the physiological responses of shrimp to global change drivers, its influence was minimized to a very marginal effect by ensuring similar (approximately eight weeks) and stable pre-exposure laboratory conditions and by selecting only non-ovigerous females. Variation in the metabolome among populations as sign of local adaptation has been shown in other marine invertebrates (e.g. Calosi et al., 2017; Vohsen et al., 2019). However, to our knowledge this is the first study that provides evidence of an inter-origin metabolomics reprogramming under combined OW and OA.
Interestingly, our results show an increase in concentration of TCA intermediates in shrimp from ESS, EC and NNC exposed to increasing temperature. Specifically, we show an increase of succinate, fumarate and malate concentrations in shrimp from these three origins and an increase in citrate and pyruvate concentrations in shrimp from EC and NNC, respectively, with increasing temperature. Usually, the accumulation of TCA metabolites suggests a disruption of the TCA cycle and a consequent shift from aerobic to anaerobic metabolism, as previously shown for example in the saltwater clam Laternula elliptica exposed to acute warming and in the olive flounder Paralichthys olivaceus, exposed to low salinity (Clark et al., 2017; Wu et al., 2017). In the olive flounder, the accumulation of lactate under low salinity exposure further supports the shift to anaerobic metabolism. However, in our study lactate concentrations are under the limit of detection, which do not allow us to confirm that shrimp shift their energy production to anaerobic metabolism, limiting our further discussion of this hypothesis. Moreover, previous results on the cellular energetic capacity of the northern shrimp exposed to combined OW and OA showed that activities of enzymes catalysing reactions of the oxidative phosphorylation pathway remained stable and that shrimp aerobic scope increased with increasing temperature between 2 and 10°C suggesting that shrimp can tolerate this range of temperatures and sustain an aerobic metabolism (Guscelli et al. under review). In the present study AMP, ADP and ATP levels remained stable and comparable among shrimp from different origins in all treatments, further suggesting that shrimp can maintain aerobic respiration even under exposure to high temperatures. This led us to a posteriori investigate if temperature, pH and/or origin affect the ATP : ADP ratio as it is a relevant indicator of energy consumption and changes in cellular energy status (Metallo and Vander Heiden, 2013; Tantama et al., 2013; Yuan et al., 2013). Higher levels of ATP : ADP ratio indicate that organisms possess enough energy to sustain oxidative metabolism while lower levels of ATP : ADP ratio indicates that glycolysis needs to be enhanced. In our study, ATP : ADP ratio is not affected by global change drivers and does not differ among origins, further suggesting that most shrimp can maintain their energy status and possibly sustain aerobic metabolism, confirming that the northern shrimp can tolerate temperatures up to 10°C and low pH conditions, but survival is reduced under these conditions (Guscelli et al. under review).
The release of TCA metabolites by mitochondria has been recently linked to the regulation of cell fate and function as they are also involved in controlling chromatin modifications, DNA methylation ultimately affecting protein functions (Martínez-Reyes and Chandel, 2020). TCA metabolites, in particular succinate, have been shown to exert signalling functions and to regulate immune and inflammatory responses, regulating tissue damage (Mills and O’Neill, 2014; Choi et al., 2021). Succinate is a substrate for reactive oxygen species (ROS) production as it provides electrons to the respiratory chain, thus its accumulation can promote the production of ROS linked to oxidative stress and immune response (Murphy, 2009; Lushchak, 2011; Li et al., 2020a; Zhang et al., 2020). However, ROS production was not measured here, and we are unable to validate whether the accumulation of TCA metabolites in the abdominal muscle is linked to oxidative stress in the northern shrimp exposed to OW and OA. Additionally, the antioxidant defence response previously measured on P. borealis exposed to low dissolved oxygen levels is unclear (Dupont-Prinet et al., 2013; Pillet et al., 2016). However, the increase in tyrosine concentration in shrimp from all origins under elevated temperature and low pH further supports the suggestion of the enhancement of the immune response. Indeed, metabolites derived from the phenylalanine, tyrosine and tryptophan biosynthesis and degradation play key roles in plants and animals’ immune responses (Parthasarathy et al., 2018). Recently, the regulation of phenylalanine, tyrosine and tryptophan biosynthesis and the tyrosine accumulation in the thick shell mussels Mytilus coruscus, exposed to OA have been suggested to potentially be linked to the immune response (Shang et al., 2022), although this needs to be investigated in the northern shrimp. We therefore suggest future studies to explore the effect of global ocean changes on P. borealis oxidative stress (for example measuring ROS production and antioxidant enzymes activity) and immune response. To do so, multiple tissues should be examined to acquire a more complete understanding of the northern shrimp response to OW and OA, especially hepatopancreas that is considered as the most sensitive tissue to oxidative stress (Ruppert et al., 2004). Nonetheless, as the northern shrimp low response of antioxidant enzymes (Dupont-Prinet et al., 2013; Pillet et al., 2016) could be due to the investigation of such response over short exposure, we suggest developing experiments employing longer exposure periods, as in the present study. Finally, to further acquire information on the potential immune response of shrimp, it would be interesting to measure the itaconate concentration as it is a key immune-responsive metabolite, stemming from diversion of TCA flux, that has been linked to disease in mussels (Li et al., 2020a). Confirming that global change drivers induce oxidative stress in the northern shrimp and enhance their immune response could lead to the re-evaluation of shrimp sensitivity and vulnerability to OW and OA. Indeed, although our results do not support a switch to anaerobiosis, the accumulation of TCA intermediates in shrimp exposed to the highest temperature and the potential enhancement of an immune response confirm that 10°C is a temperature close to the northern shrimp thermal tolerance limit (Guscelli et al. under review). This is also confirmed by the increase in free amino acid levels with increasing temperature, which can be indicative of altered protein synthesis/breakdown, possibly to use amino acids as energy sources to feed the TCA cycle. Additionally, amino acids have been increasingly recognised for their function in the immune response and tissue repair of crustaceans and other marine animals, suggesting their importance in coping with stress (Herrera et al., 2019; Huang et al., 2020).
In conclusion, our study confirms that P. borealis is overall tolerant to OW and OA conditions predicted to occur by 2100 as the cellular energetic status of shrimp from all origins is maintained. However, OW exposure, and in minor proportion OA, induce the accumulation of metabolites capable of regulating cell physiology and enhancing immune responses, suggesting signs of stress under OW and OA exposure in shrimp from all origins. Our results highlight the importance of investigating cellular mechanisms underpinning whole-organism physiological responses, to better estimate species’ sensitivity to future complex environmental conditions (Bartholomew, 1964; Harvey et al., 2014). Finally, the inter-origin differences shown in the metabolic response of shrimp exposed to OW and OA, and the intraspecific similitudes shown in form of the potential enhancement of immune response, highlight the importance of conducting macrophysiological studies to define species’ sensitivity to future environmental conditions (Chown and Gaston, 2008).
Data availability statement
The original contributions presented in this study are included in Figure S1 and Table S1 in the Supplementary Material. The dataset generated for this study will be available in the online repository PANGEA. Further inquiries can be directed to the corresponding author.
Ethics statement
Ethical review and approval was not required for the animal study because all procedures complied with Canadian legislation for animal experimentation as the Canadian Council for Animal Care does not require projects using crustaceans to be approved by an Animal Care Committee.
Author contributions
DC provided the installations for the experimental setups and the live shrimp for the study and EG carried out the experiments, the sample collection and the sample preparation for analysis. PC provided the fundings to support the samples analysis at Les laboratoires Iso-BioKem Inc. EG prepared the dataset and conducted the bioinformatics analyses with support from DM and FV, and discussions with DM and PC. EG conducted the interpretation of the results and prepared figures and tables. EG wrote the manuscript and all authors contributed to its final version. All authors contributed to the article and approved the submitted version.
Funding
Our work has been funded by: (i) an OURANOS grant (554023) to DC and PC; (ii) a DFO Strategic Program for Ecosystem-Based Research and Advice grant and an Aquatic Climate Change Adaptation Services Program grant to DC; (iii) a FIR UQAR grant, a MITACS-Ouranos Accelerate grant, a Canada Foundation for Innovation (CFI) grant and a Natural Sciences and Engineering Research Council of Canada (NSERC) Discovery grants (RGPIN-2015-06500 and RGPIN-2020-05627) to PC; (iv) a Fundação para a Ciência e Tecnologia (Portugal) through a Scientific Employment Stimulus researcher contract granted to DM (CEECIND/01250/2018) and the financial support to CESAM (UIDP/50017/2020+UIDB/50017/2020+LA/P/0094/2020); (v) a MITACS-Ouranos Accelerate grant to support EG; (vi) a Fonds de Recherche du Québec - Nature et Technologies (FRQNT) scholarship (Doctoral PBEEE, 289597) and a Réal-Decoste Ouranos scholarship (286109) to EG; and (vii) a FRQNT scholarship (Doctoral, 274427) and a Vanier Canada Graduate Scholarships (433956) to FV.
Acknowledgments
The authors are especially grateful to Bertrand Genard, Judith Savoie, Anthony Schmutz and Mathieu Millour from Les laboratoires Iso-BioKem Inc. EG, FV and PC are members of the inter-institutional strategic research network Québec-Océan. DC is member of the inter-institutional strategic research network Ressources Aquatiques Québec.
Conflict of interest
The authors declare that the research was conducted in the absence of any commercial or financial relationships that could be construed as a potential conflict of interest.
Publisher’s note
All claims expressed in this article are solely those of the authors and do not necessarily represent those of their affiliated organizations, or those of the publisher, the editors and the reviewers. Any product that may be evaluated in this article, or claim that may be made by its manufacturer, is not guaranteed or endorsed by the publisher.
Supplementary material
The Supplementary Material for this article can be found online at: https://www.frontiersin.org/articles/10.3389/fmars.2023.1170451/full#supplementary-material
References
Anderson M., Gorley R. N., Clarke R. K. (2008). Permanova+ for primer: guide to software and statisticl methods. Primer-E Limited.
Arnberg M., Calosi P., Spicer J. I., Tandberg A. H. S., Nilsen M., Westerlund S., et al. (2013). Elevated temperature elicits greater effects than decreased pH on the development, feeding and metabolism of northern shrimp (Pandalus borealis) larvae. Mar. Biol. 160, 2037–2048. doi: 10.1007/s00227-012-2072-9
Aru V., Engelsen S. B., Savorani F., Culurgioni J., Sarais G., Atzori G., et al. (2017). The effect of season on the metabolic profile of the European clam Ruditapes decussatus as studied by 1H-NMR spectroscopy. Metabolites 7, 1–14. doi: 10.3390/metabo7030036
Bartholomew G. A. (1964). The roles of physiology and behavior in the maintenance of homeostasis in the desert environment. Symp. Soc Exp. Biol. 18, 7–29.
Bechmann R. K., Taban I. C., Westerlund S., Godal B. F., Arnberg M., Vingen S., et al. (2011). Effects of ocean acidification on early life stages of shrimp (Pandalus borealis) and mussel (Mytilus edulis). J. Toxicol. Environ. Heal. - Part A 74, 424–438. doi: 10.1080/15287394.2011.550460
Bergström B. I. (2000). The biology of Pandalus. Adv. Mar. Biol. 38, 55–245. doi: 10.1016/S0065-2881(00)38003-8
Bourdages H., Roux M.-J., Marquis M.-C., Galbraith P., Isabel L. (2022). Assessment of northern shrimp stocks in the estuary and gulf of st. Lawrence in 2021: commercial fishery and research survey data. DFO Can. Sci. Advis. Sec. Res. Doc. 2022/027, xiv + 195.
Bretz F., Hothorn T., Westfall P. (2016). Multiple comparisons using R. CRC press doi: 10.1201/9781420010909-f
Brillon S., Lambert Y., Dodson J. (2005). Egg survival, embryonic development, and larval characteristics of northern shrimp (Pandalus borealis) females subject to different temperature and feeding conditions. Mar. Biol. 147, 895–911. doi: 10.1007/s00227-005-1633-6
Bundy J. G., Davey M. P., Viant M. R. (2009). Environmental metabolomics: a critical review and future perspectives. Metabolomics 5, 3–21. doi: 10.1007/s11306-008-0152-0
Caldeira K., Wickett M. E. (2003). Anthropogenic carbon and ocean pH. Nature 425, 365. doi: 10.1038/425365a
Calosi P., Melatunan S., Turner L. M., Artioli Y., Davidson R. L., Byrne J. J., et al. (2017). Regional adaptation defines sensitivity to future ocean acidification. Nat. Commun. 8 (1), 1–10. doi: 10.1038/NCOMMS13994
Cappello T. (2020). NMR-based metabolomics of aquatic organisms. EMagRes 9, 81–100. doi: 10.1002/9780470034590.emrstm1604
Chabot D., Ouellet P. (2005). Rearing Pandalus borealis larvae in the laboratory: II. routine oxygen consumption, maximum oxygen consumption and metabolic scope at three temperatures. Mar. Biol. 147, 881–894. doi: 10.1007/s00227-005-1626-5
Chemel M., Noisette F., Chabot D., Guscelli E., Leclerc L., Calosi P. (2020). Good news {{/amp]]mdash; bad news: combined ocean change drivers decrease survival but have no negative impact on nutritional value and organoleptic quality of the northern shrimp. Front. Mar. Sci. 7. doi: 10.3389/fmars.2020.00611
Choi I., Son H., Baek J. H. (2021). Tricarboxylic acid (TCA) cycle intermediates: regulators of immune responses. Life 11, 1–19. doi: 10.3390/life11010069
Chown S. L., Gaston K. J. (2008). Macrophysiology for a changing world. Proc. R. Soc B Biol. Sci. 275, 1469–1478. doi: 10.1098/rspb.2008.0137
Clark M. S., Sommer U., Sihra J. K., Thorne M. A. S., Morley S. A., King M., et al. (2017). Biodiversity in marine invertebrate responses to acute warming revealed by a comparative multi-omics approach. Glob. Change Biol. 23, 318–330. doi: 10.1111/gcb.13357
Costa C., Afonso T., Cardoso S., Maraschin M., Rocha M. (2017). Specmine: an R package for metabolomics and spectral data analysis and mining. In BOOK OF ABSTRACTS OF (p. 63)
Cyr F., Snook S., Bishop C., Galbraith P. S., Chen N., Han G. (2022). Physical oceanographic conditions on the Newfoundland and Labrador shelf during 2021. Can. Sci. Advis. Secr. Res. Doc.
Daoud D., Chabot D., Audet C., Lambert Y. (2007). Temperature induced variation in oxygen consumption of juvenile and adult stages of the northern shrimp, Pandalus borealis. J. Exp. Mar. Bio. Ecol. 347, 30–40. doi: 10.1016/j.jembe.2007.02.013
Dawe E. G., Koen-Alonso M., Chabot D., Stansbury D., Mullowney D. (2012). Trophic interactions between key predatory fishes and crustaceans: comparison of two Northwest Atlantic systems during a period of ecosystem change. Mar. Ecol. Prog. Ser. 469, 233–248. doi: 10.3354/meps10136
DFO (2021a) 2020 value of Atlantic & pacific coast commerical landings, by province. Available at: https://www.dfo-mpo.gc.ca/stats/commercial/land-debarq/sea-maritimes/s2020pv-eng.htm?
DFO (2021b). Oceanographic conditions in the Atlantic zone in 2020. Can. Sci. Advis. Secr. Sci. Advis. Rep. 2021/026.
DFO (2022a). 2021 assessment of northern shrimp on the Eastern scotian shelf (SFAS 13–15). Can. Sci. Advis. Secr. Sci. Advis. Rep. 2022/033.
DFO (2022b). Oceanographic conditions in the Atlantic zone in 2021. Can. Sci. Advis. Secr. Sci. Advis. Rep. 2022/025.
Di Santo V. (2015). Ocean acidification exacerbates the impacts of global warming on embryonic little skate, Leucoraja erinacea (Mitchill). J. Exp. Mar. Bio. Ecol. 463, 72–78. doi: 10.1016/j.jembe.2014.11.006
Dong X., Yang Z., Liu Z., Wang X., Yu H., Peng C., et al. (2022). Metabonomic analysis provides new insights into the response of zhikong scallop (Chlamys farreri) to heat stress by improving energy metabolism and antioxidant capacity. Antioxidants 11 (6), 1084. doi: 10.3390/antiox11061084
Dupont-Prinet A., Pillet M., Chabot D., Hansen T., Tremblay R., Audet C. (2013). Northern shrimp (Pandalus borealis) oxygen consumption and metabolic enzyme activities are severely constrained by hypoxia in the estuary and gulf of st. Lawrence. J. Exp. Mar. Bio. Ecol. 448, 298–307. doi: 10.1016/j.jembe.2013.07.019
Ebner J. N. (2021). Trends in the application of “omics” to ecotoxicology and stress ecology. Genes (Basel). 12 (10), 1481. doi: 10.1007/978-94-007-2072-5
Ellis R. P., Spicer J. I., Byrne J. J., Sommer U., Viant M. R., White D. A., et al. (2014). 1H NMR metabolomics reveals contrasting response by male and female mussels exposed to reduced seawater pH, increased temperature, and a pathogen. Environ. Sci. Technol. 48, 7044–7052. doi: 10.1021/es501601w
Fiehn O. (2002). Metabolomics – the link between genotypes and phenotypes. Plant Mol. Biol. 48, 155–171. doi: 10.1023/A:1013713905833
Hammer K. M., Pedersen S. A. (2013). Deep-water prawn Pandalus borealis displays a relatively high pH regulatory capacity in response to CO2-induced acidosis. Mar. Ecol. Prog. Ser. 492, 139–151. doi: 10.3354/meps10476
Hammill M. O., Stenson G. B. (2000). Estimated prey consumption by harp seals (Phoca groenlandica), hooded seals (Cystophora cristata), grey seals (Halichoerus grypus) and harbour seals (Phoca vitulina) in Atlantic Canada. J. Northwest Atl. Fish. Sci. 26, 1–23. doi: 10.2960/J.v26.a1
Harvey B. P., Al-Janabi B., Broszeit S., Cioffi R., Kumar A., Aranguren-Gassis M., et al. (2014). Evolution of marine organisms under climate change at different levels of biological organisation. Water 6, 3545–3574. doi: 10.3390/w6113545
Herrera M., Mancera J. M., Costas B. (2019). The use of dietary additives in fish stress mitigation: comparative endocrine and physiological responses. Front. Endocrinol. (Lausanne). 10. doi: 10.3389/fendo.2019.00447
Hothorn T., Bretz F., Westfall P. (2008). Simultaneous inference in general parametric models. Biometrical J. 50 (3), 346–363. doi: 10.1002/bimj.200810425
Huang Z., Aweya J. J., Zhu C., Tran N. T., Hong Y., Li S., et al. (2020). Modulation of crustacean innate immune response by amino acids and their metabolites: inferences from other species. Front. Immunol. 11. doi: 10.3389/fimmu.2020.574721
Huo D., Sun L., Zhang L., Ru X., Liu S., Yang H. (2019). Metabolome responses of the sea cucumber Apostichopus japonicus to multiple environmental stresses: heat and hypoxia. Mar. pollut. Bull. 138, 407–420. doi: 10.1016/j.marpolbul.2018.11.063
Husson F., Le S., Pages J. (2010). Exploratory multivariate analysis by example using r, Chapman and hall. CRC Computer Science & Data Analysis. CRC Press , 30, 101–102.
IPCC (2014). Summary for policy makers. (Geneva, Szwitzerland: IPCC). doi: 10.1016/j.renene.2009.11.012
IPCC (2022). The ocean and cryosphere in a changing climate. Geneva, Szwitzerland: IPCC doi: 10.1017/9781009157964
Jones O. A. H., Maguire M. L., Griffin J. L., Dias D. A., Spurgeon D. J., Svendsen C. (2013). Metabolomics and its use in ecology. Austral Ecol. 38, 713–720. doi: 10.1111/aec.12019
Jónsdóttir I. G., Björnsson H., Skúladóttir U. (2012). Predation by Atlantic cod Gadus morhua on northern shrimp Pandalus borealis in inshore and offshore areas of Iceland. Mar. Ecol. Prog. Ser. 469, 223–232. doi: 10.3354/meps09977
Koeller P., Fuentes-Yaco C., Platt T., Sathyendranath S., Richards A., Ouellet P., et al. (2009). Basin-scale coherence in phenology of shrimps and phytoplankton in the north Atlantic ocean. Sci. (80-. ). 324, 791–793. doi: 10.1126/science.1170987
Lannig G., Eilers S., Pörtner H. O., Sokolova I. M., Bock C. (2010). Impact of ocean acidification on energy metabolism of oyster, Crassostrea gigas - changes in metabolic pathways and thermal response. Mar. Drugs 8, 2318–2339. doi: 10.3390/md8082318
Lefevre S. (2016). Are global warming and ocean acidification conspiring against marine ectotherms? a meta-analysis of the respiratory effects of elevated temperature, high CO2 and their interaction. Conserv. Physiol. 4, 1–31. doi: 10.1093/conphys/cow009
Li S., Alfaro A. C., Nguyen T. V., Young T., Lulijwa R. (2020a). An integrated omics approach to investigate summer mortality of new Zealand GreenshellTM mussels. Metabolomics 16, 1–16. doi: 10.1007/s11306-020-01722-x
Li Y., Yin W., Zhan Y., Jia Y., Cui D., Zhang W., et al. (2020b). Comparative metabolome analysis provides new insights into increased larval mortality under seawater acidification in the sea urchin Strongylocentrotus intermedius. Sci. Total Environ. 747, 141206. doi: 10.1016/j.scitotenv.2020.141206
Liu Z., Zhang Y., Zhou Z., Zong Y., Zheng Y., Liu C., et al. (2020). Metabolomic and transcriptomic profiling reveals the alteration of energy metabolism in oyster larvae during initial shell formation and under experimental ocean acidification. Sci. Rep. 10, 1–11. doi: 10.1038/s41598-020-62963-3
Lushchak V. I. (2011). Environmentally induced oxidative stress in aquatic animals. Aquat. Toxicol. 101, 13–30. doi: 10.1016/j.aquatox.2010.10.006
Martínez-Reyes I., Chandel N. S. (2020). Mitochondrial TCA cycle metabolites control physiology and disease. Nat. Commun. 11, 1–11. doi: 10.1038/s41467-019-13668-3
Matoo O. B., Lannig G., Bock C., Sokolova I. M. (2021). Temperature but not ocean acidification affects energy metabolism and enzyme activities in the blue mussel, Mytilus edulis. Ecol. Evol. 11, 3366–3379. doi: 10.1002/ece3.7289
Mayor D. J., Sommer U., Cook K. B., Viant M. R. (2015). The metabolic response of marine copepods to environmental warming and ocean acidification in the absence of food. Sci. Rep. 5, 1–12. doi: 10.1038/srep13690
Metallo C. M., Vander Heiden M. G. (2013). Understanding metabolic regulation and its influence on cell physiology. Mol. Cell 49, 388–398. doi: 10.1016/j.molcel.2013.01.018
Mills E., O’Neill L. A. J. (2014). Succinate: a metabolic signal in inflammation. Trends Cell Biol. 24, 313–320. doi: 10.1016/j.tcb.2013.11.008
Mucci A., Levasseur M., Gratton Y., Martias C., Scarratt M., Gilbert D., et al. (2018). Tidally induced variations of ph at the head of the laurentian channel. Can. J. Fish. Aquat. Sci. 75, 1128–1141. doi: 10.1139/cjfas-2017-0007
Mucci A., Starr M., Gilbert D., Sundby B. (2011). Acidification of lower st. Lawrence estuary bottom waters. Atmos. - Ocean 49, 206–218. doi: 10.1080/07055900.2011.599265
Murphy M. P. (2009). How mitochondria produce reactive oxygen species. Biochem. J. 417, 1–13. doi: 10.1042/BJ20081386
Noisette F., Bordeyne F., Davoult D., Martin S. (2016). Assessing the physiological responses of the gastropod Crepidula fornicata to predicted ocean acidification and warming. Limnol. Oceanogr. 61, 430–444. doi: 10.1002/lno.10225
Noisette F., Calosi P., Madeira D., Chemel M., Menu-Courey K., Piedalue S., et al. (2021). Tolerant larvae and sensitive juveniles: integrating metabolomics and whole-organism responses to define life-stage specific sensitivity to ocean acidification in the American lobster. Metabolites 11 (9), 584. doi: 10.3390/metabo11090584
Orr J. C., Fabry V. J., Aumont O., Bopp L., Doney S., Feely R. A., et al. (2005). Anthropogenic ocean acidification over the twenty-first century and its impact on calcifying organisms. Nature 437 (7059), 681–686. doi: 10.1038/nature04095
Ouellet P., Chabot D., Calosi P., Orr D., Galbraith P. S. (2017). Regional variations in early life stages response to a temperature gradient in the northern shrimp Pandalus borealis and vulnerability of the populations to ocean warming. J. Exp. Mar. Bio. Ecol. 497, 50–60. doi: 10.1016/j.jembe.2017.09.007
Paaijmans K. P., Heinig R. L., Seliga R. A., Blanford J. I., Blanford S., Murdock C. C., et al. (2013). Temperature variation makes ectotherms more sensitive to climate change. Glob. Change Biol. 19, 2373–2380. doi: 10.1111/gcb.12240
Parthasarathy A., Cross P. J., Dobson R. C. J., Adams L. E., Savka M. A., Hudson A. O. (2018). A three-ring circus: metabolism of the three proteogenic aromatic amino acids and their role in the health of plants and animals. Front. Mol. Biosci. 5. doi: 10.3389/fmolb.2018.00029
Pillet M., Dupont-Prinet A., Chabot D., Tremblay R., Audet C. (2016). Effects of exposure to hypoxia on metabolic pathways in northern shrimp (Pandalus borealis) and Greenland halibut (Reinhardtius hippoglossoides). J. Exp. Mar. Bio. Ecol. 483, 88–96. doi: 10.1016/j.jembe.2016.07.002
Pinu F. R., Beale D. J., Paten A. M., Kouremenos K., Swarup S., Schirra H. J., et al. (2019). Systems biology and multi-omics integration: viewpoints from the metabolomics research community. Metabolites 9, 1–31. doi: 10.3390/metabo9040076
R Core Team (2020). R: a language and environment for statistical computing (Vienna, Austria: R Found. Stat. Comput).
Ruppert E. E., Fox R. S., Barnes R. D. (2004). Invertebrate zoology: a functional evolutionary approach (No. 592 RUPi).
Schalkhausser B., Bock C., Pörtner H. O., Lannig G. (2014). Escape performance of temperate king scallop, Pecten maximus under ocean warming and acidification. Mar. Biol. 161, 2819–2829. doi: 10.1007/s00227-014-2548-x
Shang Y., Wang X., Shi Y., Huang W., Sokolova I., Chang X., et al. (2022). Ocean acidification affects the bioenergetics of marine mussels as revealed by high-coverage quantitative metabolomics. Sci. Total Environ. 858, 160090. doi: 10.1016/j.scitotenv.2022.160090
Shumway S. E., Perkins H. C., Schick D. F., Stickney A. P. (1985). Synopsis of biological data on the pink shrimp, Pandalus borealis Krøyer. FAO Fisheries Synopsis No. 1838. 144, 57.
Tantama M., Martínez-François J. R., Mongeon R., Yellen G. (2013). Imaging energy status in live cells with a fluorescent biosensor of the intracellular ATP-to-ADP ratio. Nat. Commun. 4, 2550. doi: 10.1038/ncomms3550
Thor P., Bailey A., Dupont S., Calosi P., Søreide J. E., De Wit P., et al. (2018). Contrasting physiological responses to future ocean acidification among Arctic copepod populations. Glob. Change Biol. 24 (1), e365–e377. doi: 10.1111/gcb.13870
Thor P., Vermandele F., Bailey A., Guscelli E., Loubet-Sartrou L., Dupont S., et al. (2022). Ocean acidification causes fundamental changes in the cellular metabolism of the Arctic copepod Calanus glacialis as detected by metabolomic analysis. Sci. Rep. 12, 22223. doi: 10.1038/s41598-022-26480-9
Vargas C. A., Lagos N. A., Lardies M. A., Duarte C., Manríquez P. H., Aguilera V. M., et al. (2017). Species-specific responses to ocean acidification should account for local adaptation and adaptive plasticity. Nat. Ecol. Evol. 1 (4), 1–7. doi: 10.1038/s41559-017-0084
Vohsen S. A., Fisher C. R., Baums I. B. (2019). Metabolomic richness and fingerprints of deep-sea coral species and populations. Metabolomics 15, 1–13. doi: 10.1007/s11306-019-1500-y
Wei L., Wang Q., Ning X., Mu C., Wang C., Cao R., et al. (2015). Combined metabolome and proteome analysis of the mantle tissue from pacific oyster Crassostrea gigas exposed to elevated pCO2. Comp. Biochem. Physiol. - Part D Genomics Proteomics 13, 16–23. doi: 10.1016/j.cbd.2014.12.001
Wei R., Wang J., Su M., Jia E., Chen S., Chen T., et al. (2018). Missing value imputation approach for mass spectrometry-based metabolomics data. Sci. Rep. 8, 1–10. doi: 10.1038/s41598-017-19120-0
Williams T. D., Wu H., Santos E. M., Ball J., Katsiadaki I., Brown M. M., et al. (2009). Hepatic transcriptomic and metabolomic responses in the stickleback (Gasterosteus aculeatus) exposed to ethinyl-estradiol. Environ. Sci. Technol. 43 (16), 6341–6348. doi: 10.1021/es9008689
Wu H., Liu J., Lu Z., Xu L., Ji C., Wang Q., et al. (2017). Metabolite and gene expression responses in juvenile flounder Paralichthys olivaceus exposed to reduced salinities. Fish Shellfish Immunol. 63, 417–423. doi: 10.1016/j.fsi.2017.02.042
Yuan H. X., Xiong Y., Guan K. L. (2013). Nutrient sensing, metabolism, and cell growth control. Mol. Cell 49, 379–387. doi: 10.1016/j.molcel.2013.01.019
Zhang Y., Wu H., Wei L., Xie Z., Guan B. (2017). Effects of hypoxia in the gills of the Manila clam Ruditapes philippinarum using NMR-based metabolomics. Mar. pollut. Bull. 114, 84–89. doi: 10.1016/j.marpolbul.2016.08.066
Keywords: Metabolomics (OMICS), macrophysiology, conservation physiology, crustaceans, Pandalus borealis, pathways, stress, immunity
Citation: Guscelli E, Chabot D, Vermandele F, Madeira D and Calosi P (2023) All roads lead to Rome: inter-origin variation in metabolomics reprogramming of the northern shrimp exposed to global changes leads to a comparable physiological status. Front. Mar. Sci. 10:1170451. doi: 10.3389/fmars.2023.1170451
Received: 20 February 2023; Accepted: 09 May 2023;
Published: 23 May 2023.
Edited by:
Jonathan Y.S. Leung, University of Adelaide, AustraliaReviewed by:
Gisela Lannig, Alfred Wegener Institute Helmholtz Centre for Polar and Marine Research (AWI), GermanyLiqiang Zhao, Guangdong Ocean University, China
Copyright © 2023 Guscelli, Chabot, Vermandele, Madeira and Calosi. This is an open-access article distributed under the terms of the Creative Commons Attribution License (CC BY). The use, distribution or reproduction in other forums is permitted, provided the original author(s) and the copyright owner(s) are credited and that the original publication in this journal is cited, in accordance with accepted academic practice. No use, distribution or reproduction is permitted which does not comply with these terms.
*Correspondence: Ella Guscelli, ZWxsYS5ndXNjZWxsaUB1cWFyLmNh
†These authors have contributed equally to this work and share last authorship