- 1Marine Ecology Department, University of Bremen, Bremen, Germany
- 2Coral Reef Ecology Research Group, Universidad del Valle, Cali, Colombia
Upwelling events can change the sea water conditions within few hours during several months on a seasonal regimen. These events are predicted to intensify due to climate change, exposing shallow benthic organisms to hypoxia and thermal stress, among other extreme conditions. Some coral reefs in the Eastern Tropical Pacific (ETP) recurrently experience upwelling events. Coral reefs off Gorgona Island, Colombia, are exposed to lower oxygen concentrations (3.0 - 3.7 mg O2 L-1) and lower temperatures (17 - 24°C) from mid-January to mid-April, when upwelling develops, compared to the rest of the year (4.9 ± 0.7 mg O2 L-1, 28.4 ± 0.3°C, mean ± SD). While no visible signs of stress have been reported for corals during upwelling, it can be hypothesized that corals would be negatively affected by these changes. Therefore, the objectives of this study were to (1) identify the effects of temperature and hypoxia on the metabolic rate (MO2) of Pocillopora corals under lab conditions, and (2) to examine the changes in skeletal growth and Symbiodiniaceae density of Pocillopora in the field between upwelling and non-upwelling seasons. Findings revealed that MO2 was significantly reduced by 20% at 24°C and significantly increased by 10% at 32°C compared to 28°C. During the upwelling season, Pocillopora corals exhibited a 52% increase in Symbiodiniaceae density, but the growth rate decreased by 50% compared to the non-upwelling season. Fast changes in water conditions during the upwelling strongly affects the metabolism of Pocillopora corals. Although conditions during upwelling were not lethal, they compromised the energy of the coral for their vital functions, indicating that upwelling pushes them toward their physiological limit. Consequently, predicted increases in upwelling events in combination with ocean warming and deoxygenation may be particularly critical for upwelling-exposed corals and the reefs they build in the Eastern Tropical Pacific.
1 Introduction
Upwelling is a process where cool deep waters are transported to shallow depths due to the displacement of a surface water mass by winds. This phenomenon can occur in the open ocean and along coastlines, and is characterized by a series of circulation cells that cause changes in pH, oxygen levels, and nutrient concentrations (Spring and Williams, 2023). Coastal upwelling in the Eastern Tropical Pacific (ETP) is initiated when wind jets exceed 10 m s−1 and pass from the Caribbean and Gulf of Mexico to the Pacific through topographic depressions at the gulfs of Tehuantepec (Mexico), Papagayo (Costa Rica), and Panama (D’Croz and O’Dea, 2007; Coria-Monter et al., 2019). The temperature decrease associated with upwelling occurs rapidly, typically within 1 hour, and is characterized by pulses that can last several hours during the first quarter of the year (Rixen et al., 2012; Stuhldreier et al., 2015).
In the Gulf of Panama seasonal upwelling is primarily driven by the migration of the Intertropical Convergence Zone (ITCZ) from 10° N to 3° N during the dry season (December to April). During this time, the northeast trade winds intensify and cross the isthmus of Panama, causing the 20°C thermocline to reach its shallowest position (< 20 m depth) (Poveda et al., 2006; D’Croz and O’Dea, 2007; Alexander et al., 2012). During the upwelling season the temperature, pH and aragonite saturation decrease in the sub-surface water column (4 – 20 m depth), while salinity, nutrients and some carbonate parameters increase, promoting phytoplankton growth near the surface (D’Croz and O’Dea, 2007; Manzello, 2010b). Also, hypoxic conditions (0.1 mg L-1) from the oxygen minimum zone (OMZ) reach the 10 m depth (Lucey et al., 2021). However, this pattern may be modified by El Niño Southern Oscillation (ENSO) events (Wang et al., 2017).
Despite seasonal abrupt changes in water conditions associated with upwelling, several coral reefs are present in the ETP region (Glynn et al., 2017a). However, at upwelling sites reef development is limited to protected areas shielded from direct oceanic influence, reef frameworks are thinner compared to those at non-upwelling sites, and Pocillopora corals exhibit low growth rates. These reef characteristics are thought to result from the low temperature, low pH, and high nutrient availability during upwelling events (Glynn and Stewart, 1973; Medellín-Maldonado et al., 2016; Tortolero-Langarica et al., 2017). Nonetheless, the rates of long-term reef accretion are similar throughout the ETP region (Toth et al., 2012; Toth et al., 2015; Toth et al., 2017).
Upwelling has been suggested to reduce warm thermal stress during El Niño events and provide a thermal refuge from ocean warming (Randall et al., 2020), and warming associated with El Niño can counteract the negative effects of low temperature on coral growth during upwelling (Vargas-Ángel et al., 2001; Jiménez and Cortés, 2003a). However, some discrepancy exists regarding trends in bioerosion and future persistence of reefs at upwelling sites (Manzello et al., 2008; Manzello, 2010a; Enochs et al., 2021; Rodriguez-Ruano et al., 2023). It has been proposed that to effectively reduce thermal stress during warming events, upwelling must occur simultaneously and provide sufficient cooling, phosphate levels must increase to alleviate nutrient starvation of Symbiodiniaceae, or corals must actively feed during plankton blooms (Chollett et al., 2010; Riegl et al., 2019). However, upwelling can also lead to the growth of benthic algae that compete with corals for space, and higher abundance of suspension-feeding macroboring fauna (Smith, 2006; Riegl et al., 2019; Palmer et al., 2022; Rodriguez-Ruano et al., 2023).
At present, climate change is causing vertical expansion of the OMZ and ocean deoxygenation (Clarke et al., 2021). The rate of ocean deoxygenation in the ETP is 49 mmol m-2 yr−1 (Stramma et al., 2008). For the near future it is predicted that upwelling events will increase in frequency and strength (Rixen et al., 2012; Sydeman et al., 2014; Bakun et al., 2015; Fiedler and Lavín, 2017). Additionally, the current low pH conditions at upwelling centers highlights the susceptibility of Pocillopora corals to ocean acidification (Manzello et al., 2008). However, predictions on the effect of future trends of ENSO and its influence on upwelling are uncertain (García-Reyes et al., 2015; Wang et al., 2017; Bograd et al., 2023).
Temperature and oxygen (O2) conditions regulate many metabolic processes, and imbalances at the organismal level can impact ecological processes, particularly for habitat-forming taxa such as scleractinian corals. Metabolic impairments occur because enzyme activity is temperature-dependent, with maximum kinetics occurring within a specific range; below or above this range, enzymes become inactivated or denatured (Fitt et al., 2001; Daniel et al., 2010; Sawall et al., 2022). Additionally, the energy for enzymatic processes depends on ATP production, which requires O2 as the terminal electron acceptor during aerobic synthesis (Salin et al., 2015). Mitochondrial activity is particularly reduced under low temperature and hypoxia (Gnaiger, 2001; Fangue et al., 2009), which occurs at dissolved O2 concentrations (DO) < 2 mg L-1, or any DO insufficient to maintain metabolic functions (Vaquer-Sunyer and Duarte, 2008; Welker et al., 2013; Hughes et al., 2020).
Thermal stress (cool or warm temperatures) induces the collapse of the symbiosis between the corals and the endosymbiotic algae (Symbiodiniaceae), leading to coral bleaching. When bleaching is not lethal, such stress increases susceptibility to diseases, and reduces growth and reproductive output (Baird et al., 2009; Kemp et al., 2011; Miller and Richardson, 2015; McLachlan et al., 2020). Even if thermal stress does not cause bleaching, at low temperatures the membrane permeability of cells is reduced, restricting the movement of glycerol from the symbiotic algae to the coral (Mayfield and Gates, 2007; Rodríguez-Troncoso et al., 2014), and the respiration rate of corals increases faster than the photosynthetic rate of the algae with increasing temperature (Coles and Jokiel, 1977; Castillo and Helmuth, 2005), both of which result in a deficiency of autotrophic energy for the corals’ needs.
Hypoxia also has multiple negative impact on corals; when it is no lethal, it causes bleaching, partial tissue detachment from the skeleton, affects the activity of calcifying cells, homeobox genes, and lipid metabolism; and it produces oxidative stress, and DNA damage (Baohua et al., 2004; Weber et al., 2012; Wijgerde et al., 2012; Haas et al., 2014; Raj et al., 2020; Alderdice et al., 2021; Johnson et al., 2021; Deleja et al., 2022; Gravinese et al., 2022). The co-occurrence of hypoxia and warming reduce the respiration rate and energy for vital functions of corals (Gravinese et al., 2022), and although corals may switch to anaerobic metabolism, this is inefficient for survival under prolonged stress (Murphy and Richmond, 2016; Linsmayer et al., 2020).
In Symbiodiniaceae, hypoxia can reduce the activity of antioxidant enzymes, the respiration rate, and the photochemical efficiency of PSII (Matta and Trench, 1991; Gardella and Edmunds, 1999; Ulstrup et al., 2005). However, hypoxia may also have no effects on the endosymbiotic algae (Osinga et al., 2017). The bleaching response is associated with the activation of the Hypoxia-Inducible Factor, a gene important for the mitigation of hypoxic stress (Alderdice et al., 2022). However, Symbiodiniaceae community association and hypoxia tolerance could be species and site-specific; for example, the bleaching resistant Acropora tenuis associates with a different Cladocopium community than the bleaching sensible A. selago (Alderdice et al., 2020); Pocillopora acuta host mainly Durusdinium in hypoxic environments (mangroves), while Cladocopium at normoxic sites (reef); but transplantation evidenced stable symbiont association (Haydon et al., 2021).
Despite the well-known negative effects of hypoxia and cool stress on scleractinian corals, and the occurrence of both conditions in the ETP during upwelling events, there is lack of research comparing the effects of hypoxia vs those of thermal stress (Rodríguez-Troncoso et al., 2014; Rodríguez-Troncoso et al., 2016; Glynn et al., 2017b; Tortolero-Langarica et al., 2017). To our knowledge, two reports have linked bleaching and mass mortality of Pocillopora corals with hypoxia after dinoflagellate blooms at Caño Island (Costa Rica) and Ensenada de Utría (Colombia) (Guzman et al., 1990; Vargas-Angel, 1996), and our previous work showed that the hypoxic threshold of Pocillopora capitata (Critical oxygen tension or Pcrit = 3.7 mg L-1) from Gorgona Island lies within the lower DO conditions reported on reefs in this island (3.0 - 3.7 mg L-1) (Castrillón-Cifuentes et al., 2023). The Pcrit, a metric for assessing the minimum DO level that maintains the metabolic rate (Negrete and Esbaugh, 2019; Regan et al., 2019), has been found to be closer to the lower DO levels recorded in the environment of different scleractinian corals, indicating their sensitivity to drops in DO below the ambient typical range (Dodds et al., 2007; Hughes et al., 2022). Therefore, our objective was to assess the physiological response of Pocillopora (the main reef builder in the ETP) to rapid changes in temperature and O2 conditions that can occur during upwelling events, with particular interest on the effect of the interaction of low temperatures and hypoxia.
We hypothesized that exposure to cool thermal stress and hypoxic conditions during upwelling events would significantly affect the physiology of Pocillopora. This is because these corals naturally experience pronounced changes in temperature and O2 conditions on a seasonal basis. To test this hypothesis, our first step was to characterize the interannual changes in temperature and DO on coral reefs of Gorgona Island, Colombia, in order to determine the average and extreme conditions that corals face during upwelling. Subsequently, we assessed the physiological response of Pocillopora corals to these conditions using a combination of field and laboratory measurements. We have already characterized the spatio-temporal changes in DO on coral reefs of Gorgona Island (data available in Castrillón-Cifuentes et al., 2023). Therefore, this research complements our previous publication and expands our knowledge of coral responses to multifactorial changes in the environment.
2 Materials and methods
2.1 Study area
Gorgona Island (2°58’10” N - 78°11’05” W, Figures 1A, B) is a National Natural Park located 30 km off the nearest point on the Colombian Pacific coast. Despite its tropical position, there is marked climatic seasonality, primarily driven by the seasonal latitudinal displacement of the ITCZ, which affects most of Panama Bight (Rodriguez-Rubio and Stuardo, 2002; Corredor-Acosta et al., 2020). From January to April, there is low precipitation (350 mm month-1) and the thermocline is at its shallowest position (7.5 m), allowing for the lifting of cool, high-salinity, nutrient rich, and low-oxygen deep waters (25°C, 30.8 PSU, 0.6 mg L-1 of Chl-a, 4.0 mg O2 L-1). For the rest of the year, precipitation increases (587 mm month-1), and the thermocline deepens (47 m), allowing for warmer and more oxygenated waters to occurs, but with lower salinity and chlorophyll-a conditions (27°C, 4.9 mg O2 L-1, 29.8 PSU, 0.1 mg L-1 of Chl-a) (Giraldo et al., 2008; Blanco, 2009; Giraldo et al., 2014; Sampson and Giraldo, 2014; Zapata, 2017; Castrillón-Cifuentes et al., 2023). Changes in pH did not reveal a temporal pattern (Ramírez-Martínez et al., 2022).
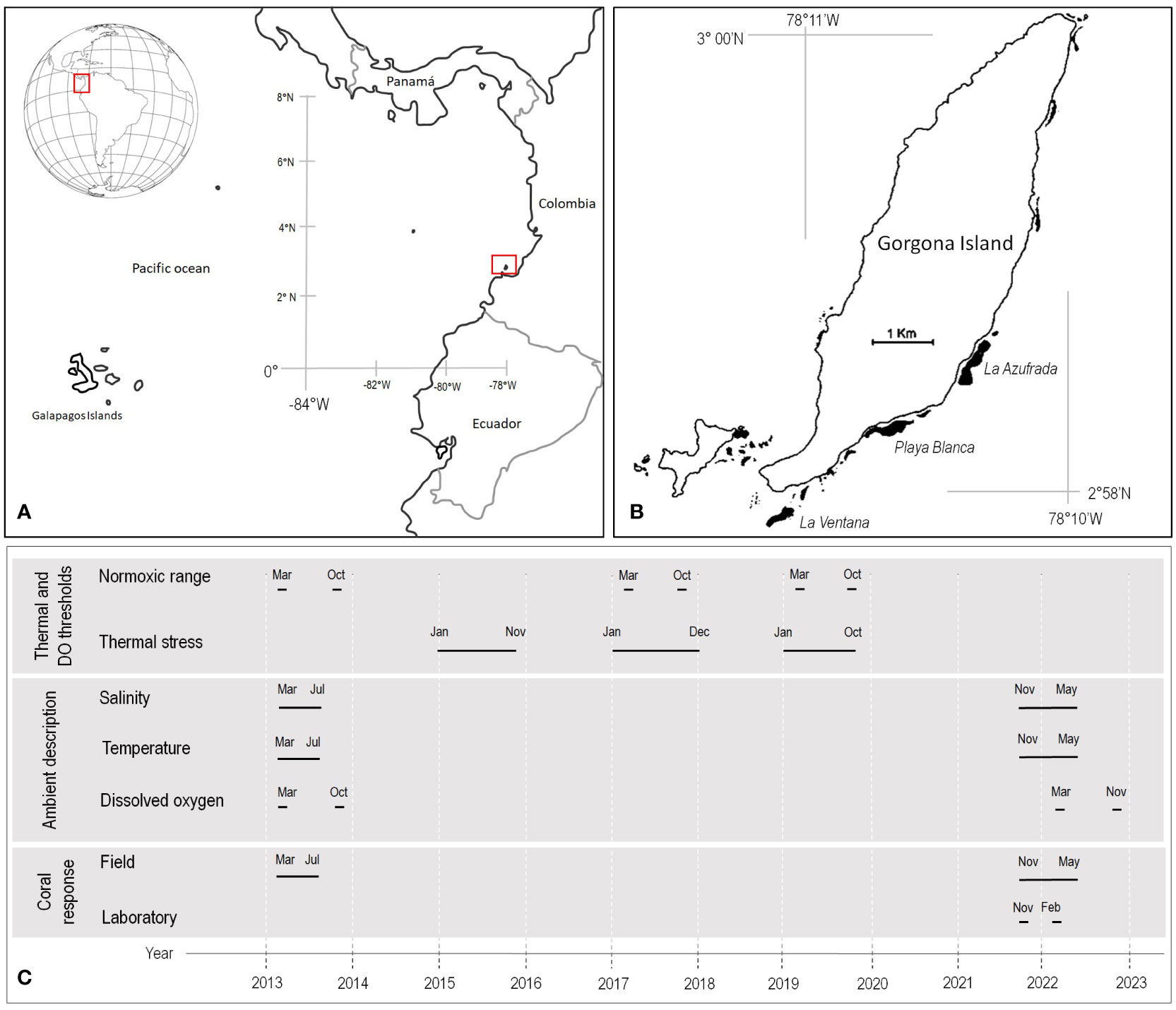
Figure 1 (A) Location of Gorgona Island in the Colombian Pacific. (B) Black areas denote the coral reefs of Gorgona Island where colonies of Pocillopora were collected for experiments. (C) Timeline for the data collection to stablish the normoxic and hypoxic conditions, and the cool and warm thermal stress that face corals at Gorgona Island; also, to show when was assessed the coral response to upwelling events in the field (growth rate and Symbiodiniaceae density) and laboratory (hypoxic threshold and respiration rate).
Interannual variability in oceanographic conditions occurs due to ENSO events. During El Niño, sea surface temperatures can increase by more than 1.7°C above the mean for several months and high oxygen conditions occur (5.1 mg O2 L-1) due to less intense upwelling events (Ledesma et al., 2022; Castrillón-Cifuentes et al., 2023). In contrast, cool temperatures as low as 18°C and hypoxic conditions (< 3.7 mg O2 L-1) have been registered on the reef slope during La Niña events, when upwelling intensifies (Vargas-Ángel et al., 2001; Mora and Ospina, 2002; Castrillón-Cifuentes et al., 2023).
2.2 Environmental conditions on coral reefs of Gorgona Island during field measurements of growth and Symbiodiniaceae density of Pocillopora
In 2013, temperature and salinity were recorded with a datalogger at La Azufrada (LA) reef between March 1 and July 31. During March temperature was 24.8 ± 1.5°C (mean ± SD), and salinity 34.8 ± 2.0 PSU. The rest of the time (April – July), temperature was 27.6 ± 0.6°C, and salinity 34.1 ± 0.9 PSU (Supplementary Information File 1). All reefs of the island exhibited the same trend of lower DO during the upwelling season (3.5 ± 0.3 mg O2 L-1) than during the non-upwelling season (4.7 ± 0.1 mg O2 L-1) (Castrillón-Cifuentes et al., 2023). Chlorophyll-a concentration (a proxy for nutrient conditions) was higher (although not statistically significantly so) during March (1.23 ± 0.056 μg L-1) than during October (1.04 ± 0.056 μg L-1) (Cuellar-Chacon, 2017). pH was 7.4 in March (Giraldo and Valencia, 2013), and 8.6 in October (Vivas Aguas et al., 2014), but values were collected by different devices and researchers. Nevertheless, no statistical differences in pH conditions at La Azufrada reef were detected between seasons in a study that was carried out from October 2010 to November 2011 (Ramírez-Martínez et al., 2022).
From November 14, 2021, to May 22, 2022, temperature was recorded with a datalogger at La Ventana (LV) and Playa Blanca (PB) reefs (Supplementary Information File 1). Salinity was recorded for the same period at PB, but only until the end of February at LV due to a device failure. Upwelling occurred from February 25 to March 31, 2022. During this period mean (± SD) temperature was 25.9 ± 1.0°C and 26.1 ± 1.1°C at LV and PB, respectively, and salinity was 32.1± 0.5 PSU at PB reef. Previous to the upwelling event (November 14, 2021 to February 24, 2022) the temperature was 27.0 ± 0.3°C at both reefs, and salinity 30.9 ± 0.3 PSU at PB and 33.5 ± 0.4 PSU at LV. After the upwelling (April to May 2022) the temperature was 28.3 ± 0.2°C at LV and 28.5 ± 0.3°C at PB, and salinity 31.2 ± 0.2 PSU at PB. Dissolved oxygen conditions at LA reef during March 2022 were 4.8 ± 1.0 mg O2 L-1 (Castrillón-Cifuentes et al., 2023), and during November 2022 they were 6.7 ± 0.7 mg O2 L-1, measured with a Firesting-GO2 (PyroScience GmbH).
2.3 Field measurements of growth and Symbiodiniaceae density of Pocillopora
2.3.1 Study organism
Species delimitation of Pocillopora corals in the ETP has not been fully resolved, particularly if based on morphological characteristics. According to molecular analyses there are three types of Pocillopora within this region: type 1 is widespread throughout the ETP; type 2 is restricted to the Clipperton Atoll, and type 3 inhabits Panama and Galapagos Islands (Pinzón and Lajeunesse, 2011; Pinzón et al., 2013). Also, Pocillopora colonies from the ETP can switch morphology (Paz-García et al., 2015), but morphospecies did not exhibit differences in growth rates (Tortolero-Langarica et al., 2017). In this text we refer to the study organism simply as Pocillopora. The colonies selected for this study had morphological features (Supplementary Information File 1) of Pocillopora damicornis (Veron et al., 2022), although Schmidt-Roach et al. (2014) state that this species is absent from the ETP.
2.3.2 Growth rate
To identify seasonal changes in Pocillopora growth at Gorgona Island, during 2013, 27 colonies from LA reef were monitored from March to July. Each colony was photographed with a size reference in March 13, May 3, and July 4, and the diameter of the colonies was calculated using the ImageJ software (National Institute of Health, USA). The growth rate of the colonies was then calculated for two periods: March to May, corresponding to the effects of upwelling season, and May to July, corresponding to the non-upwelling season. Statistical differences in growth between seasons were examined with a paired t-test after checking for the normality of the distribution of differences between paired observations (Shapiro-Wilk’s W = 0.979, p = 0.842).
From November 2021 to May 2022, the growth rate of coral fragments from LV and PB reefs were monitored (10 fragments per reef, each one from a different colony). The fragments were cemented with marine epoxy to ceramic tiles in April 2021 and allowed to grow at their respective reefs, and photographed with a size reference on November 14, 2021, and February 25 and May 22, 2022. The linear extension based on the height of the fragments was measured using the ImageJ software. The growth rate of the fragments was then calculated for two periods: November 2021 to February 2022 for the upwelling season, and February to May 2022 for the non-upwelling season. Differences in growth between seasons and reefs were compared using a Repeated Measures ANOVA, with season as a within-subject factor and reef as a between-subject factor. The normality of residuals assumption was satisfied for both seasons (Shapiro-Wilk’s W = 0.909 and 0.951, p = 0.063 and 0.384, respectively), and variances were homogeneous between reefs in November-February and in February-May (Levene’s test, F = 1.566 and 4.323, p = 0.226 and 0.052, respectively).
2.3.3 Symbiodiniaceae density
To identify seasonal changes on the Symbiodiniaceae density of Pocillopora corals at Gorgona Island, the density of algae from the 27 colonies monitored at LA reef was measured on March 13, 2013 (upwelling) and July 4, 2013 (non-upwelling). Once during each month, a 3 cm fragment was collected. The soft tissue from the fragments was fixed in Zenker’s solution, decalcified in HCL (4%), preserved in 70% ethanol, and stained with Toluidine blue. Histological slides were cut at a thickness of 5 µm. On each slide, 15 polyps were randomly selected, and all endosymbiotic algae cells within each polyp were counted. The area of the polyps in the histological slide was calculated after measuring their diameter with a micrometer scale. The density of Symbiodiniaceae was established for the polyp area (µm2) and extrapolated to cm2. No taxonomic identification of the symbionts was made.
The luminosity (amount of white) in the color of the 27 colonies monitored at LA reef was determined in the photos used to measure the growth. Luminosity is related to the density of Symbiodiniaceae in corals, and lighter colors (or high luminosity) in the coral correspond to low algal density (Siebeck et al., 2006). Differences between the upwelling and non-upwelling seasons for the density of Symbiodiniaceae and the luminosity in the color of corals were compared using a paired t-test. Both Symbiodiniaceae density and luminosity satisfied the normality of paired differences assumption (Shapiro-Wilk’s W = 0.937 and 0.930, p = 0.101 and 0.071, respectively).
Assuming that coral tissue luminosity is an indicator of Symbiodiniaceae density (Siebeck et al., 2006), the luminosity in the color of coral fragments from LV and PB reefs was measured in photos taken during February and May of 2022 (upwelling and non-upwelling, respectively). Differences in the luminosity of coral fragments at each reef were assessed between seasons using a repeated measures ANOVA. These data met the assumption of normality of residuals in both February (Shapiro-Wilk’s W = 0.973, p = 0.828) and May (Shapiro-Wilk’s W = 0.964, p = 0.646) as well as the assumption of homogeneity of variances between reefs both in February (Levene’s test F = 0.020, p = 0.888) and in May (F = 0.024, p = 0.879).
2.4 Definition of thermal conditions to evaluate the metabolic response of Pocillopora
Temperature data loggers (Onset Hobo) were located at 1 and 5 m depths on LA reef during 2015, 2017, and 2019. Data were retrieved to account for the thermal variability that may occur on coral reefs of Gorgona Island. Data loggers recorded every 30 minutes for the entire year or until battery depletion. The annual average temperature (27.5 ± 1.5, mean ± SD) was calculated between January 1 and the last record of each year: November 15, 2015, December 31, 2017, and October 9, 2019 (Figure 1C). The upwelling season was described using the data between January 19 and April 15, time at which temperatures < 26°C (annual mean – SD) begin to occur, while data after April 16 were used for characterizing the non-upwelling season (Table 1).
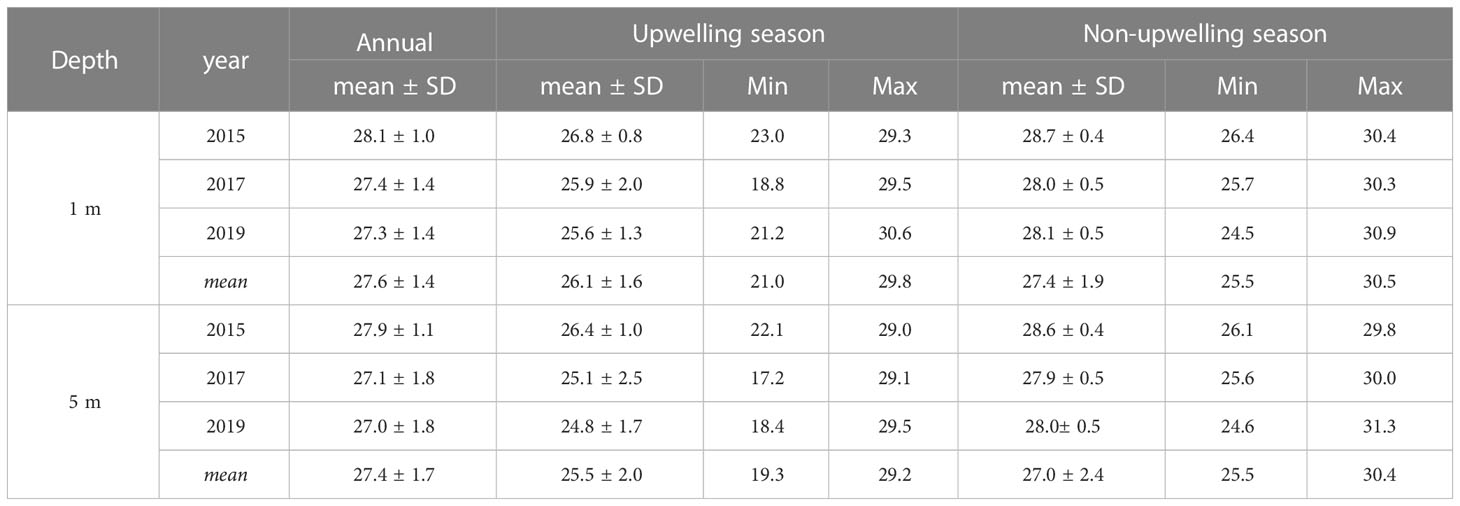
Table 1 Descriptive statistics for the thermal variation (°C) recorded by data loggers at La Azufrada reef, at 1 and 5 m depth, and during the years 2015, 2017, and 2019.
A normal thermal condition was 27.5 ± 1.5°C, which corresponds to the mean annual temperature. Cool stress conditions, which occur during the upwelling season, were defined as any temperature < the mean – SD (24.0°C). Warm stress conditions were temperatures > the annual mean + SD (29.0°C). Cool events (< 24°C) were more intense during 2019. Warm events (> 29°C) occurred through all year, but were more intense between May and July, and in specially during 2015 (Supplementary Information File 1).
2.5 Metabolic response of Pocillopora to changes in temperature and DO
On November 12, 2021, seven fragments from different colonies of Pocillopora were collected at LA reef and transported to the laboratory on the island. There, the fragments were incubated in hermetically sealed respiration chambers filled with seawater from the reef (6.2 mg O2 L-1, 30 PSU). The respiration chambers were kept at 28 ± 0.7°C (mean ± SD, a normal thermal condition), in the dark for 6 hours, including 1 hour of acclimation and 5 hours for DO measurements.
To maintain a stable temperature, all respiration chambers were incubated in a water bath equipped with a thermostat and submersible water pump to circulate the water and homogenize the temperature. A total of eight respiration chambers were used, seven containing coral fragments and one serving as a control, containing 363 ml of water and no coral to measure background or microbial respiration. All respiration chambers had internal water movement generated by peristaltic pumps. Changes in DO (mg L-1) were recorded every 20 minutes with a Firesting-GO2 (PyroScience GmbH); the sensor was calibrated according to the manufacturer’s instructions.
The process was repeated on November 17, 2021, with different colonies than those employed for the normal thermal conditions, and with a water bath at 32.0 ± 0.3°C (Warm stress), which exceeds by ~1°C the maximum temperature recorded by the loggers, and is a temperature that occurred during a previous El Niño event (Vargas-Ángel et al., 2001). On February 27, 2022, the experiment was repeated (using again different colonies) with a water bath at 24.0 ± 1.1°C (Cool stress). To maintain this low temperature, frozen gel packages were added to the water bath, and coral fragments were left in the respiration chambers for 7 h, with 1 h for acclimation and 6 h for DO measurements. This time encompassed the time required to deplete DO to hypoxic levels, which occurs during the upwelling season. All incubations ended when DO fell to ≤ 2.0 mg L-1 in at least four respiration chambers, which was after 6 h in the 28°C and 32°C incubations, and 7 h in the 24°C incubation.
The metabolic or respiration rate (MO2) was calculated using the calc_MO2 function of the Respirometry package in R (Seibel et al., 2021). We set the bin_width to 0 which computes a MO2 value from one PO2 observation to the next along the incubation period. Then the Pcrit of each coral fragment at the given temperature was calculated using the calc_pcrit function with the paired PO2 and MO2 values obtained after applying the calc_MO2 function and established according to the broken stick regression (Yeager and Ultsch, 1989). Scripts and data are presented in Supplementary Information File 2. The Pcrit of each coral fragment at the given temperature was statistically compared using a one-way ANOVA. The data met the assumptions of homoscedasticity (Cochran’s test C = 0.53, df = 2, p = 0.36) and normality of residuals (K-S d = 0.13, p > 0.20).
The MO2 values recorded on each coral from one PO2 observation to the next were grouped according to whether they were below or above the Pcrit, which corresponds to hypoxic and normoxic conditions, respectively. Then, the mean MO2 at normoxic and hypoxic conditions for each coral fragment at their respective temperature were compared statistically using a Repeated Measures ANOVA. Raw data met the assumptions of homogeneity of variances and covariances (Box’s M test= 9.2, df= 6, p= 0.2). MO2 data were standardized as the amount of O2 consumed per mass of coral tissue per minute (mg O2 g-1 min-1). The weight of coral tissue was determined by the difference between the wet and dry (ash-free) weights of the coral fragments.
3 Results
3.1 Field measurements of growth and Symbiodiniaceae density of Pocillopora
The growth rate of Pocillopora colonies during 2013 and at La Azufrada reef during the upwelling season was 43.3% lower than during the non-upwelling season (t-test: -2.1, df = 26, p = 0.04). The mean (± SD) growth rate during upwelling was 0.017 ± 0.01 cm day-1 vs. 0.030 ± 0.02 during non-upwelling. The same seasonal pattern was evidenced in coral fragments monitored from November 2021 to May 2022 at Playa Blanca and La Ventana reefs: during the upwelling season growth rate was reduced by 68% (Table 2). However, during both seasons, coral fragments at Playa Blanca reef exhibited a lower growth rate (-0.001 vs. 0.0003 cm day-1 during upwelling and non-upwelling season, respectively) compared to fragments at La Ventana reef (0.004 vs. 0.008 cm day-1 during the upwelling and non-upwelling season, respectively).
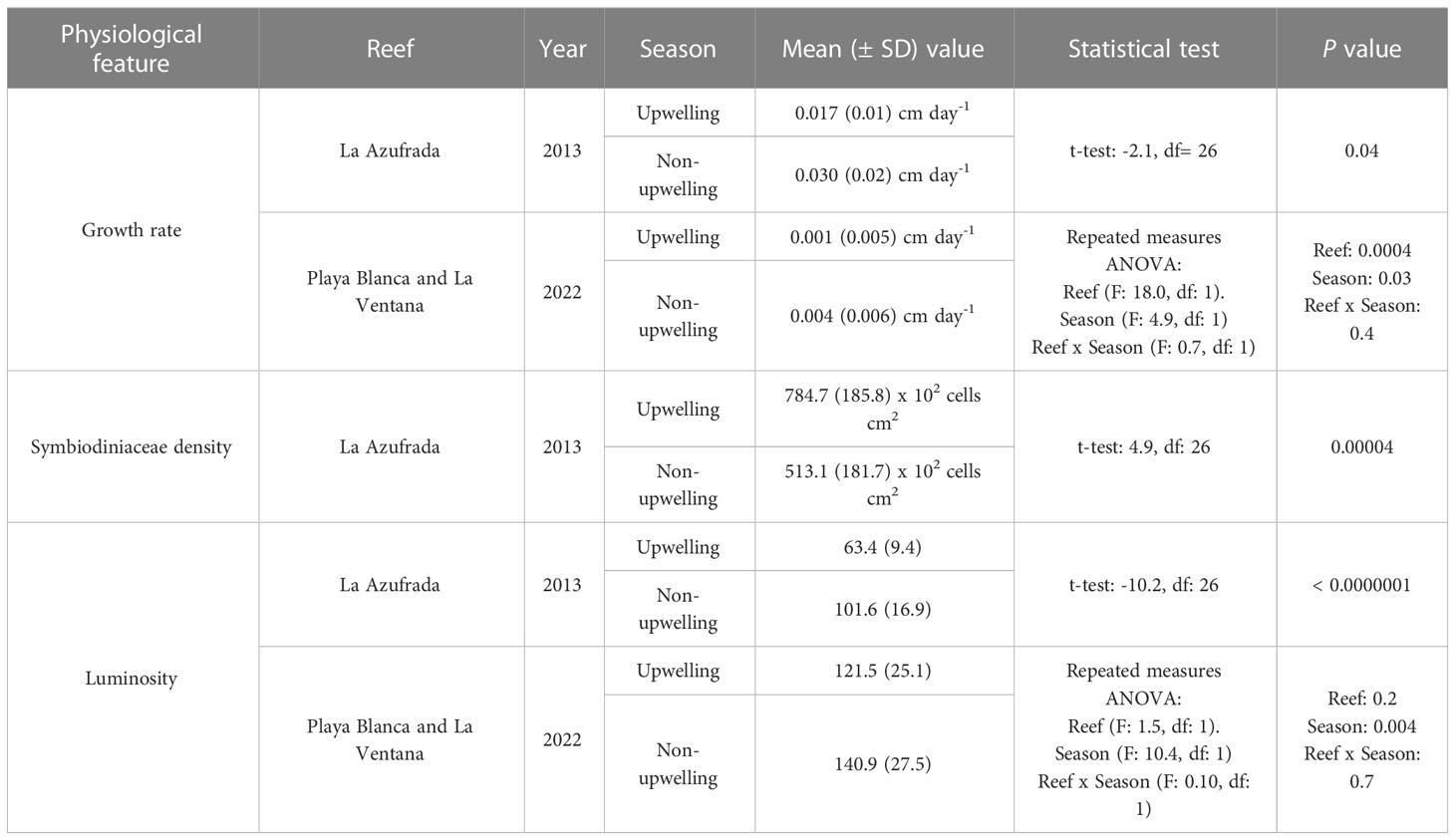
Table 2 Statistical analysis to identify the effects of upwelling and non-upwelling seasons on the physiology of Pocillopora from Gorgona Island.
Symbiodiniaceae density was significantly higher in March than in July 2013, a reduction of symbiotic algae populations from the upwelling to the non-upwelling seasons of 53% (Table 2). As expected, the luminosity of colonies was significantly lower in March than in July 2013, indicating that colonies had darker colors, and likely a higher density of Symbiodiniaceae, during the upwelling season (Figure 2). The same seasonal pattern of low luminosity during the upwelling season was found for the coral fragments at La Ventana and Playa Blanca reefs during 2022, but no statistical differences between reefs were observed (Table 2).
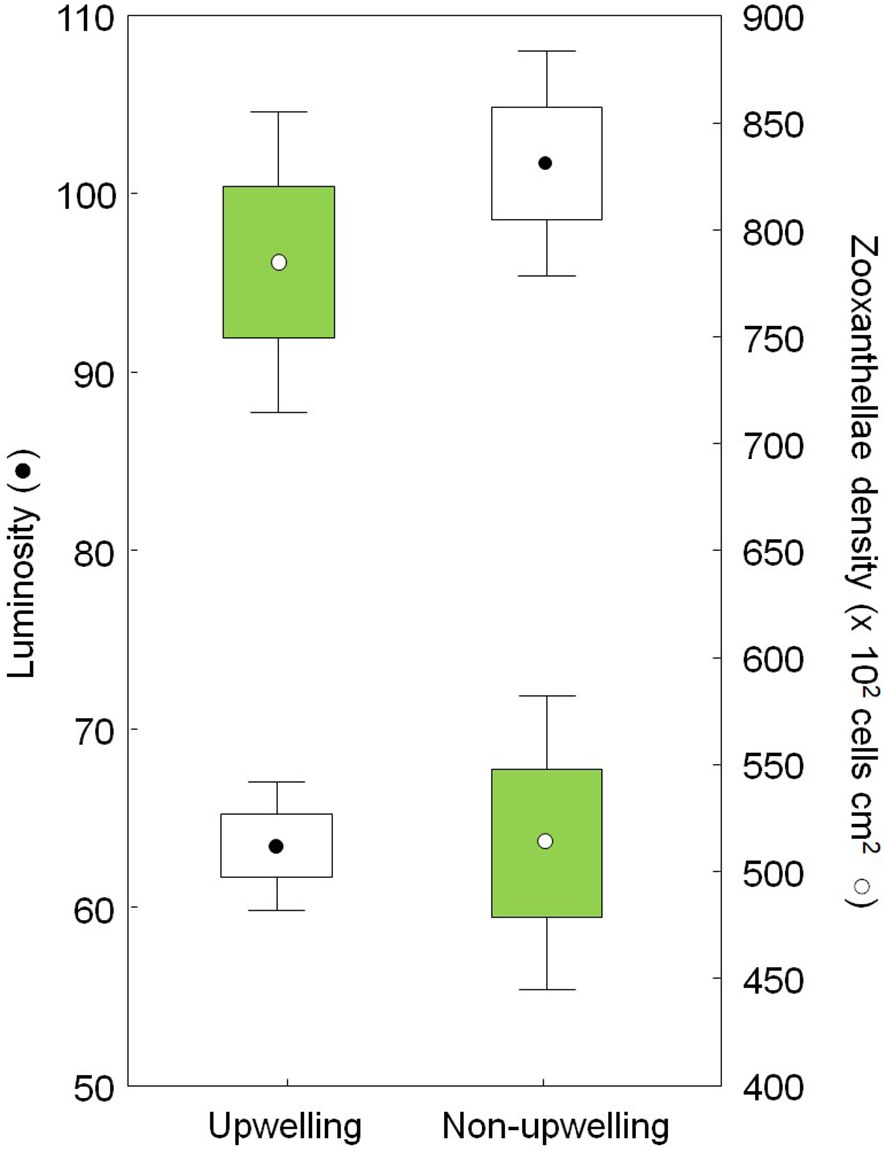
Figure 2 Seasonal changes of zooxanthellae density (green) and luminosity (white) of Pocillopora from La Azufrada reef during 2013.
3.2 Metabolic response of Pocillopora to changes in temperature and DO
The critical oxygen tension of Pocillopora was significantly influenced by temperature (one-way ANOVA, F = 7.9, df = 2, p = 0.003); specifically, Pcrit decreased as temperature increased. At 24°C the Pcrit was 4.2 ± 1.2 mg O2 L-1, at 28°C it was 3.7 ± 1.0 mg O2 L-1 and at 32°C the Pcrit was 1.9 ± 0.7 mg O2 L-1.
Temperature also had a significant effect on the MO2 (Repeated measures ANOVA, F = 4.31, df = 2, p = 0.02), such that oxygen consumption increased as temperature increased. At 24°C the MO2 was 0.46 ± 0.18 mg O2 g-1 min-1, at 28°C it was 0.74 ± 0.33 mg O2 g-1 min-1, and at 32°C it was 0.74 ± 0.55 mg O2 g-1 min-1. When corals were first exposed to normoxic conditions and then to hypoxic conditions, as expected, the MO2 decreased significantly from 0.85 ± 0.47 mg O2 g-1 min-1 to 0.45 ± 0.15 mg O2 g-1 min-1 (F = 18.0, df = 1, p = 0.0004).
There was a significant interaction between temperature and O2 conditions (i.e., normoxia and hypoxia) on the MO2 (F = 5.42, df = 2, p = 0.01). Under normoxic conditions the rate of oxygen consumption increased with temperature, and as expected the MO2 was different between the extreme temperatures, also between 24°C and 28°C, but not between 28°C and 32°C (Figure 3, Table 3). In contrast, under hypoxic conditions the MO2 tented to increase from 24°C to 28°C, but tended to decrease from 28°C to 32°C. Both at cool and warm temperatures MO2 was lower respect the normal condition (28°C). However, none of the latter differences were significant. In short, temperature had the expected effect under normoxia but not under hypoxia (Figure 3, Table 3).
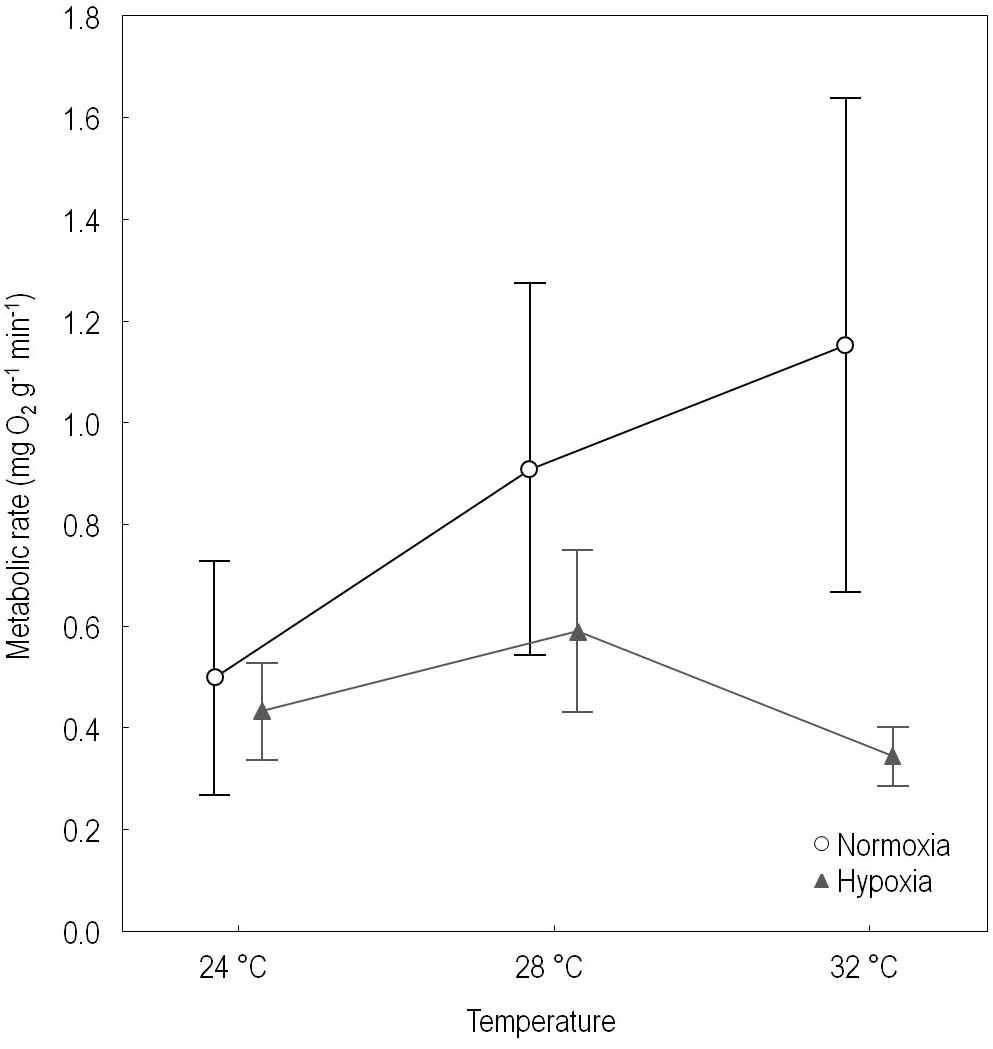
Figure 3 Effect of temperature and oxygen conditions (normoxia and hypoxia) on the metabolic rate of Pocillopora.
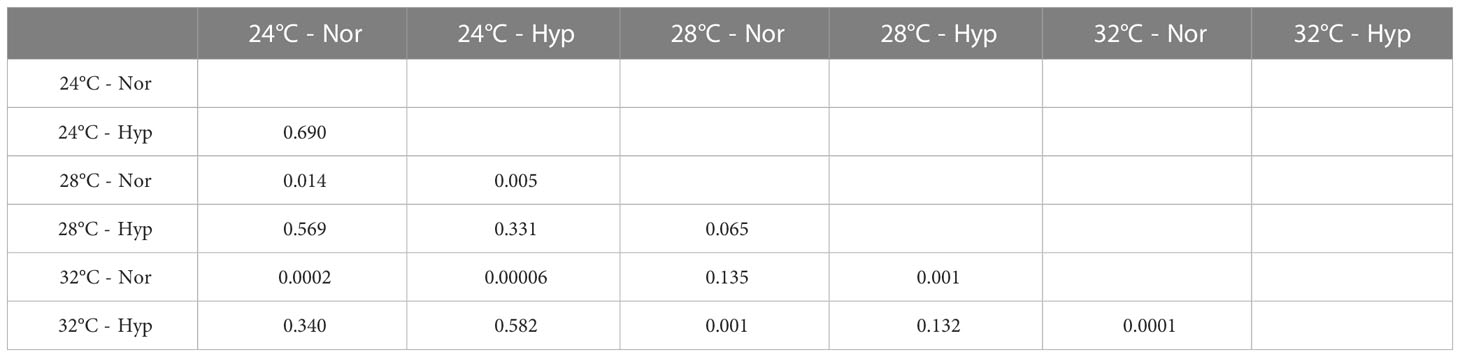
Table 3 Multiple comparison test (Fisher LSD) to determine the probability of significant differences in the metabolic rate at different temperatures (24, 28 and 32°C) and oxygen conditions (Nor, normoxia; Hyp, hypoxia).
4 Discussion
Many corals exhibit seasonal modulation of their physiology, with lower symbiont densities and tissue biomass during the warmer months than during cooler months, because warm conditions affect the symbiosis between the coral and the Symbiodiniaceae leading to energy deficits (Fagoonee et al., 1999; Fitt et al., 2001; Kemp et al., 2011; Kemp et al., 2016; Gantt et al., 2023). Our study provides evidence of plasticity in the physiology of Pocillopora in response to seasonal changes in environmental conditions. However, the pattern of reduced coral biomass during warm months due to the sensibility of Symbiodiniaceae does not hold true for corals that are influenced seasonally by upwelling, such as those at Gorgona Island, where abrupt changes in ambient conditions (mainly low temperature and dissolved oxygen conditions) significantly affect coral performance.
4.1 Growth rate
Our study reveals that both colonies and fragments of Pocillopora exhibit reduced growth rates during the upwelling season compared to the non-upwelling season. We observed a 43.3% reduction in growth rate for colonies and a 68% reduction for fragments. Our findings are consistent with the results of Glynn and Stewart (1973) in the Gulf of Panama, who described seasonal variation in the growth of Pocillopora fragments due to changes in temperature, with a drop in growth rate of approximately 80% during the upwelling season (February and March: < 0.5 mm month-1) compared to the growth rate before upwelling (2 – 3 mm month-1), and growth cessation occurring at temperatures below 20°C.
Although different methods were applied in our work and the work of Glynn and Stewart (1973), the growth rate of our fragments (Upwelling: 0.4 mm month-1; Non-upwelling: 1.3 mm month-1) was similar to the growth rate reported by these authors. Interestingly, they did not find differences between measuring the growth rate with photographic records (as we did) or staining corals with alizarin red. Previous growth rates of Pocillopora fragments reported for Gorgona Island during the non-upwelling season were between 1.9 and 4.8 cm year-1 (Lizcano-Sandoval et al., 2018; Ishida-Castañeda et al., 2020), and the growth rate of our fragments during the non-upwelling season was close to that range (1.6 cm year-1).
Additionally, as reported by Céspedes-Rodríguez and Londoño-Cruz (2021), we found low growth rates at Playa Blanca reef, where higher sediment loads may account for depressed coral growth (Zapata, 2001). However, we discard the possibility that the low growth rate at Playa Blanca was the result of differences in temperature or DO between reefs, as both abiotic conditions respond to seasonal changes and there is no between-reef variability in such parameters (SM1; Castrillón-Cifuentes et al., 2023). Although salinity was lower at Playa Blanca than at La Ventana during the non-upwelling season, conclusions cannot be made due to a lack of data for La Ventana during the upwelling season.
The negative effect of upwelling on the growth rate of Pocillopora in the ETP can also be observed when comparing localities influenced or not by these events (Manzello, 2010a; Medellín-Maldonado et al., 2016; Randall et al., 2020), as well as during ENSO events that modulate upwelling intensity. During La Niña events, upwelling intensifies and causes low growth rates in Pocillopora (Tortolero-Langarica et al., 2017), and at upwelling localities Pocillopora experiences increases in growth rates during moderate El Niño events (Vargas-Ángel et al., 2001; Jiménez and Cortés, 2003b). Additionally, tissue recovery after thermal stress has been found to be greater in response to warm stress rather to cool stress events (Glynn and Fong, 2006).
We propose that the low growth rate of Pocillopora during the upwelling season could be the result of reduced metabolic activity, particularly in the calcifying cells, due to low DO and temperature conditions. At upwelling localities, low temperatures coincide with low DO conditions (Rixen et al., 2012), and the coral reefs of Gorgona Island are no exception (Castrillón-Cifuentes et al., 2023). As a result of this stressful environment, metabolic constraints occur due to reduced ATP production under hypoxia and reduced enzymatic activity at low temperatures (Fitt et al., 2001; Gnaiger, 2001; Fangue et al., 2009; Salin et al., 2015; Sawall et al., 2022). Additionally, we suspect that negative effects of low DO in the environment could be worsened due to the development of hypoxia within coral tissues, which might result from the night respiration of Symbiodiniaceae (Altieri et al., 2017), whose population was observed to increases during the upwelling season (Table 2).
Acidifying conditions, which characterizes the ETP, also contributes to depress growth rate (Manzello, 2010a), but its seasonal variation was not evident on coral reefs at Gorgona Island (Ramírez-Martínez et al., 2022). Although there is a consensus that the decreased production of cement components in Pocillopora reefs at upwelling localities of the ETP is the result of low Ωarag (Manzello et al., 2008), we propose that O2 limitation may also play a vital role in this process. Low DO in the environment reduces ATP production by the calicoblastic mitochondria, which is required to pump calcium ions and protons for coral skeleton construction. Therefore, under conditions of low temperature, low glycerol, and low O2, it is expected that the calicoblastic mitochondria will not be able to produce skeletal components (Colombo-Pallotta et al., 2010). In fact, well-oxygenated conditions (80 – 100% oxygen saturation) are required for optimum calcification rate in Galaxea fascicularis, and neither feeding nor light conditions mitigate the negative effects of hypoxia on calcification (Wijgerde et al., 2012). In Acropora millepora, DO rather than pH controls coral growth (Wijgerde et al., 2014).
To explain how corals survive stressful conditions, like those that occurs during the upwelling in the ETP, Wooldridge (2014) has highlighted a series of counterintuitive trade-offs between linear extension rate and other traits such as skeletal density, lipid storage, immune response, and reproductive capacity. This may be the case for Pocillopora at Gorgona Island, as ambient conditions during the upwelling season depress their metabolism, but corals can survive even worsen conditions: the co-occurrence of sub-aerial exposure during spring tides that also happens during the upwelling season. To survive sub-aerial exposure, Pocillopora corals at Gorgona Island seem to maintain their growth rate while reducing reproductive output (Castrillón-Cifuentes et al., 2017). As consequence of low sexual reproduction, populations are primarily maintained through fragmentation (Muñoz et al., 2018). Hence, high growth rates during the non-upwelling season (Lizcano-Sandoval et al., 2018; Ishida-Castañeda et al., 2020; Céspedes-Rodríguez and Londoño-Cruz, 2021) is advantageous for asexual reproduction.
4.2 Symbiodiniaceae density
Symbiodiniaceae density (or luminosity as its proxy) in Pocillopora corals at Gorgona Island varied seasonally, with a 53% higher density during the upwelling season compared to the non-upwelling season. Mayfield and Gates (2007) propose that corals under stress disrupt the symbiosis, because the cell’s ability to transport molecules (mainly Glycerol and free amino acids) across membranes is affected creating osmotic stress, which in turns results in reactive oxygens species (ROS) formation, photoinhibition, apoptosis and exocytosis of Symbiodiniaceae, and host cell detachment. Most records of bleaching events (loss of Symbiodiniaceae or their pigments) are attributed to thermal stress, mainly due to warm temperatures (Hughes et al., 2017; Hughes et al., 2018); however, other physical factors (light, depth, currents) that modulates temperature (Van Oppen and Lough, 2009), as well low salinity (Kerswell and Jones, 2003; Kongjandtre et al., 2021) also contributes to bleaching.
Under no stress conditions corals also constantly expel Symbiodiniaceae (1% of the population per day; Davy et al., 2012), and the density of Symbiodiniaceae varies temporally in response to environmental conditions. In P. damicornis and Pocillopora verrucosa from Hawaii and the Red Sea, an increase in Symbiodiniaceae density during winter is promoted by an increase in nutrients rather than by changes in temperature (Stimson, 1997; Al-Sofyani and Floos, 2013; Sawall et al., 2014). Similarly, Acropora formosa has more symbiotic algae during the autumn-winter than during the spring-summer, and the temporal pattern is explained by a combination of temperature, solar radiation, nitrate, and oxygen concentration (Jones and Yellowlees, 1997). The effect of oxygen on Symbiodiniaceae was explained as hyperoxia inducing the accumulation of ROS (Fagoonee et al., 1999). However, ROS can form during both hypoxia and hyperoxia and can inhibit photosynthesis among other metabolic processes (Ulstrup et al., 2005; Welker et al., 2013; Deleja et al., 2022).
In the ETP only one study reported temporal variation of Symbiodiniaceae density, and found a positive correlation between Symbiodiniaceae density and increased nitrate and phosphate concentrations (Martínez-Castillo et al., 2020). The effect of thermal stress is well documented through experiments and field measurements during El Niño events; temperatures above 30°C resulted in a decrease in symbiont density in Pocillopora capitata, P. verrucosa, P. damicornis, Pocillopora elegans, and Porites lobata (Glynn and D’croz, 1990; Glynn et al., 2001; Hueerkamp et al., 2001; D’Croz and Maté, 2004; Flores-Ramírez and Liñán-Cabello, 2007; Rodríguez-Troncoso et al., 2016). Conversely, low temperatures (19°C) increased the Symbiodiniaceae density in P. verrucosa by 27% (Rodríguez-Troncoso et al., 2014).
Overall, the high density of Symbiodiniaceae in Pocillopora corals during the upwelling season at Gorgona Island could be a result of the combined effects of high nutrient concentrations that enhance algae population growth, low dissolved oxygen levels (but not at hypoxic levels) that reduce the possibility of oxidative stress, and the thermo-tolerance of the symbionts (Cunning et al., 2013; Baker et al., 2017; Palacio-Castro et al., 2022). However, further research is needed to understand the specific contributions of these environmental factors to the population dynamics of Symbiodiniaceae, including the molecular identification of symbionts and the quantification of the translocated fixed carbon from the algae to the host under upwelling conditions (thermal stress and hypoxia).
We also suspect that increases in Symbiodiniaceae density during the upwelling affect the growth rate of corals, because under low temperatures the movement of glycerol might be restricted causing osmotic stress, low temperatures can cause metabolic depression in coral’s cells, and low oxygen in the environment and inside corals tissue (due to increased Symbiodiniaceae number and its night respiration) limits the calcification process (Colombo-Pallotta et al., 2010). In fact Rodríguez-Troncoso et al. (2014) evidenced that during low temperatures Symbiodiniaceae increase but corals lipids (glycerol) decrease.
Additionally, during the upwelling season, Symbiodiniaceae are likely to reduce their photosynthetic capacity due to self-shading resulting from the increased number of algae and less availability of CO2 from the coral metabolism to start up photosynthesis (Hoogenboom et al., 2010). Moreover, if corals host thermo-tolerant Symbiodiniaceae like Durusdinium (formerly known as clade D; LaJeunesse et al., 2018), this might account for reduced growth rates. It is known that symbiosis with this algae reduces coral growth rates but allows higher survivorship under thermal stress (Little et al., 2004; Manzello, 2010a; Cunning et al., 2015; LaJeunesse et al., 2018). Further research is needed to understand the specific contributions of these factors to the growth rates of corals during the upwelling season.
4.3 Critical oxygen tension
Temperature had a significant effect on the Pcrit of Pocillopora corals at Gorgona Island. These parameters were negatively related, as increases in temperature caused a decrease in the Pcrit. Species with a lower Pcrit are likely more tolerant to hypoxic conditions, as they possess a greater capacity to extract O2 from a hypoxic environment and might delay the initiation of anaerobic metabolism (McArley et al., 2019). If an organism is able to do so, it can avoid the accumulation of lactic acid or other by-products of anaerobic metabolism, which cause a decrease in pH within the tissue (Grieshaber et al., 1988). Scleractinian corals activate anaerobic metabolism during hypoxic exposure, but they employ opine dehydrogenases rather than lactate dehydrogenase (Murphy and Richmond, 2016; Linsmayer et al., 2020). However, anaerobic metabolism seems to be inefficient for survival under multifactorial stress conditions or under prolonged periods (Weber et al., 2012).
During the non-upwelling season, it is likely that Pocillopora corals face well-oxygenated conditions, as the Pcrit of coral fragments incubated at 28°C (ambient temperature) was similar to the lower edge of dissolved oxygen (DO) conditions on the reefs of Gorgona Island (Pcrit: 3.7 mg L-1; Environmental DO range: 3.7 – 6.5 mg L-1, mean: 4.9 mg L-1). During the upwelling season, Pocillopora might face hypoxia, as the Pcrit of corals incubated at 24°C falls within the range of DO that the reefs experienced during that season (Pcrit: 4.2 mg L-1; Environmental DO range: 3.0 – 6.6 mg L-1, mean: 4.2 mg L-1). Corals incubated at warm conditions (32°C) had an enhanced ability to extract O2 from the environment (at least up to 6 h, incubation time) and maintain their MO2 over a broad range of DO conditions (Pcrit = 1.9 mg L-1). This suggests that atypical warm episodes during the upwelling season (SM1) could ameliorate the effect of deoxygenation.
To the best of our knowledge, this is the first time that the Pcrit of a tropical scleractinian coral has been tested under different thermal conditions. Only one study assessed the Pcmax (an analogous value to Pcrit) of different coral species, but at their mean ambient temperature (Hughes et al., 2022). In the cold-water coral Lophelia pertusa, the Pcrit was lower at a cooler temperature (6.5°C, Pcrit = 5-6 Kpa) than at normal (9°C) or warm (11°C) conditions (Pcrit = 9-10 Kpa) (Dodds et al., 2007). In Crassostrea gigas, the Pcrit increases with temperature, and this positive relation was assumed to be an effect of the low solubility of oxygen in warm waters (Le Moullac et al., 2007).
As the Pcrit is an effective way to measure tolerance to hypoxic condition, it has been intensively assessed in fish. Within species, high temperatures result in a higher Pcrit (Rogers et al., 2016), but variation in this pattern occurs among species; for example, various Etheostoma species had a low Pcrit at warm temperatures, possibly because they had a mechanism to increase O2 transport at higher temperatures (Ultsch et al., 1978). Because cnidarians lack of ventilation or circulatory systems, we propose that the mechanism that operates in Pocillopora to increase O2 transport at warm conditions is related with the Diffusive Boundary Layer (DBL).
The DBL is the water layer adjacent to the surface of all coral polyps, where diffusion is the main method of transport for oxygen (and other substances) from the environment to the corals’ cells. In this transport process the cilia of polyps aid in generating vortices to move O2 within this layer (Shapiro et al., 2014; Pacherres et al., 2020; Pacherres et al., 2022). We speculate that low temperatures can lead to decreased ciliary activity (Muscatine et al., 1991), and because the viscosity of water increases at cooler temperatures (Bolton and Havenhand, 2005), the O2 diffusion through the DBL is reduced, resulting in a high Pcrit of Pocillopora at 24°C, and in an efficient oxygen transport at warm conditions (28 and 32°C), as result of low water viscosity and normal activity of cilia.
4.4 Metabolic rate
The MO2 is a sensitive indicator of an organism’s response to environmental changes, as it integrates numerous energy-requiring processes, such as growth, reproduction, and tissue repair (Edmunds, 2005). We found that temperature and DO conditions significantly affect the rate of oxygen consumption (MO2), as expected, since enzymes involved in respiration are temperature- and oxygen-dependent in order to successfully produce ATP (Sawall et al., 2022).
Under normoxic conditions, MO2 increases with temperature. This pattern has been observed in all stony corals examined (Coles and Jokiel, 1977; Castillo and Helmuth, 2005; Dodds et al., 2007; Paradis et al., 2019; Gravinese et al., 2022). However, this correlation is only positive within a specific range, known as the optimum temperature range. Beyond this range, the temperature becomes lethal at either low or high critical values (Sokolova and Pörtner, 2003). While the high and low temperatures tested in this study had statistically significant effects on Pocillopora metabolism, the duration of the incubations should not be considered indicative of a critical range. Therefore, further tests are needed to identify long-term thermal tolerance.
An indicator of compensatory acclimation to temperature should display an inverse correlation between MO2 and temperature (Ulbricht, 1973). According to Al-Sofyani and Floos (2013), if acclimation is complete, the MO2 of corals should be identical during the summer and winter, as is the case for P. verrucosa in the Red Sea; this, however, is not the case for Pocillopora at Gorgona Island. Therefore, our corals were not acclimated to the cool stress conditions that occur during the upwelling season, as MO2 at 24°C was statistically different from that at 28°C. However, corals in warm conditions might have been acclimated, as differences in MO2 between 28°C and 32°C were not significant (Table 3). It is important to mention that incubations at 24°C were conducted during the upwelling season, when the corals were naturally subjected to upwelling. Incubations at 28°C and 32°C were conducted during the non-upwelling season.
The low MO2 of Pocillopora at cool temperatures validates the reduced growth rate during the upwelling season reported by this and previous studies, as well as the limited reef development during La Niña events and at upwelling centers (Glynn et al., 2017a). The similar MO2 at normal and warm temperatures supports the idea that moderate El Niño events can ameliorate the negative effect of upwelling, which reduces metabolism and, in particular, skeletal growth (Vargas-Ángel et al., 2001; Jiménez and Cortés, 2003a).
A decrease in MO2 occurs under hypoxic conditions at any temperature, but it is more marked at extreme temperatures (Figure 3, Table 3). In Orbicella faveolata, combined exposure to warm and hypoxic conditions reduces the respiration rate by 62.8%, which is associated with a gradual shift to anaerobic respiration (Gravinese et al., 2022). Testing whether anaerobic metabolism is activated in Pocillopora during extreme upwelling conditions requires further research. However, the fact that corals are less efficient in terms of growth opens up this possibility as a costly way to survive stressful conditions.
5 Conclusion
Our results demonstrated that the environmental variability that occurs during upwelling events at Gorgona Island has negative effects on the physiology of Pocillopora (Figure 4); specifically, cool thermal stress and hypoxia that develop during upwelling result in significant changes in the growth rate, respiration rate, and Symbiodiniaceae density of corals. It is possible that Pocillopora corals on the reefs of Gorgona Island have reached their physiological limits and rely on trade-offs to survive the stressful upwelling conditions, as the aforementioned physiological traits did not appear to contribute to mortality. Therefore, the occurrence of additional stressors or the intensification of upwelling could be detrimental to this island’s primary reef constructors.
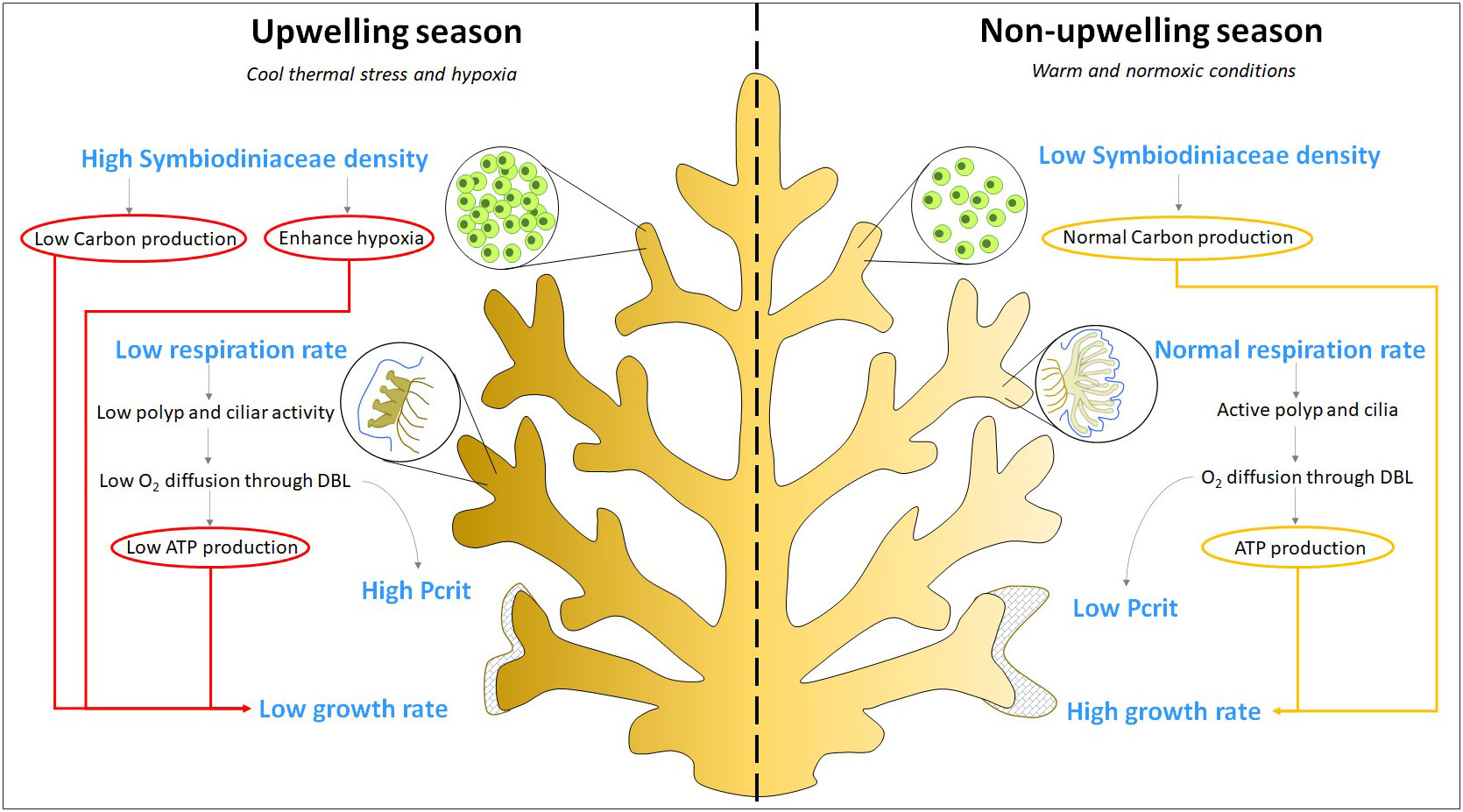
Figure 4 Response of Pocillopora to cool and hypoxic conditions that occur during upwelling events. The observed response of corals during the upwelling and non-upwelling season is presented in blue font. Factors that promote (yellow lines) or depress (red lines) coral growth rate are denoted inside circles. During the upwelling season, the high density of Symbiodiniaceae results in low photosynthesized carbon and enhanced hypoxia within coral tissue. The cool and hypoxic ambient conditions lead to a low respiration rate, resulting in less available energy (ATP) for polyp activity. This reduction in polyp activity, in turn, decreases the diffusion of oxygen (O2) through the Diffusive Boundary Layer (DBL), resulting in a high Pcrit value. A high Pcrit value indicates that corals are less tolerant to hypoxic conditions. Finally, due to low ATP, cool stress, and hypoxia, corals have a reduced growth rate.
In contrast, coral metabolism increased when exposed to elevated temperatures and abundant oxygen. This may explain why Pocillopora in upwelling regions thrive more during El Niño events, as warm conditions appear to alleviate the metabolic constraints that occur during upwelling. However, we do not suggest that El Niño events are advantageous for Pocillopora, as our experiments evaluated coral responses to brief periods of exposure to stressors and not responses to long-term warming. However, the postulated oxygen extraction efficiency under warm conditions could account for the observed tolerance of Pocillopora corals and the resilience of reefs to El Niño events in the ETP.
Data availability statement
The original contributions presented in the study are included in the article/Supplementary Material. Further inquiries can be directed to the corresponding author.
Author contributions
AC-C: conceived, designed, and perform the research; analyzed the results and wrote the manuscript (original and final draft). FZ: coordination of the field work, data analysis, review and editing the manuscript for intellectual content. CW: review and editing the manuscript for intellectual content. All authors contributed to the article and approved the submitted version.
Funding
This research was co-financed by The Intergovernmental Panel on Climate Change and The Cuomo Foundation through the IPCC Scholarship Program (141021_tripartite agreement_6th round), The Rufford Foundation small grant (31547-1), The PADI foundation (68128), and Colfuturo and Minciencias scholarship (202007248), awarded to AC-C. The University of Bremen provided the funds for the article publication. The contents are solely the liability of AC-C and under no circumstances may be considered as a reflection of the position of the funders.
Acknowledgments
We are grateful to Danna Velasco and Juan Fernando Rivera, members of the Coral Reef Ecology Research Group, Universidad del Valle, Cali, Colombia, for the assistance during the fieldwork; the staff of the National Natural Park of Gorgona for their support in coordinating all activities at the island; and to Yubarta foundation, for facilitating the use of their boat for the field work.
Conflict of interest
The authors declare that the research was conducted in the absence of any commercial or financial relationships that could be construed as a potential conflict of interest.
Publisher’s note
All claims expressed in this article are solely those of the authors and do not necessarily represent those of their affiliated organizations, or those of the publisher, the editors and the reviewers. Any product that may be evaluated in this article, or claim that may be made by its manufacturer, is not guaranteed or endorsed by the publisher.
Supplementary material
The Supplementary Material for this article can be found online at: https://www.frontiersin.org/articles/10.3389/fmars.2023.1212717/full#supplementary-material
References
Alderdice R., Perna G., Cárdenas A., Hume B. C. C., Wolf M., Kühl M., et al. (2022). Deoxygenation lowers the thermal threshold of coral bleaching. Sci. Rep. 12, 18273. doi: 10.1038/s41598-022-22604-3
Alderdice R., Pernice M., Cárdenas A., Hughes D. J., Harrison P. L., Boulotte N., et al. (2021). Hypoxia as a physiological cue and pathological stress for coral larvae. Mol. Ecol. 31, 571–587. doi: 10.1111/mec.16259
Alderdice R., Suggett D. J., Cárdenas A., Hughes D. J., Kühl M., Pernice M., et al. (2020). Divergent expression of hypoxia response systems under deoxygenation in reef-forming corals aligns with bleaching susceptibility. Glob Change Biol. 27, 312–326. doi: 10.1111/gcb.15436
Alexander M. A., Seo H., Xie S. P., Scott J. D. (2012). ENSO’s impact on the gap wind regions of the eastern tropical pacific ocean. J. Clim. 25, 3549–3565. doi: 10.1175/JCLI-D-11-00320.1
Al-Sofyani A. A., Floos Y. A. M. (2013). Effect of temperature on two reef-building corals Pocillopora damicornis and P. verrucosa in the Red Sea. Oceanologia 55, 917–935. doi: 10.5697/oc.55-4.917
Altieri A. H., Harrison S. B., Seemann J., Collin R., Diaz R. J., Knowlton N. (2017). Tropical dead zones and mass mortalities on coral reefs. Proc. Natl. Acad. Sci. U. S. A. 114, 3660–3665. doi: 10.1073/pnas.1621517114
Baird A. H., Bhagooli R., Ralph P. J., Takahashi S. (2009). Coral bleaching: the role of the host. Trends Ecol. Evol. 24, 16–20. doi: 10.1016/j.tree.2008.09.005
Baker A. C., Correa A. M. S., Cunning R., Baker A. C., Correa A. M. S., Cunning Á.R., et al. (2017). “Diversity, distribution and stability of symbiodinium in reef corals of the eastern tropical pacific,” in Coral reefs of the eastern tropical pacific. Coral reefs of the world. Eds. Glynn P., Manzello D., Enochs I. (Dordrecht: Springer, Dordrecht), 405–420. doi: 10.1007/978-94-017-7499-4_13
Bakun A., Black B. A., Bograd S. J., García-Reyes M., Miller A. J., Rykaczewski R. R., et al. (2015). Anticipated effects of climate change on coastal upwelling ecosystems. Curr. Clim. Change Rep. 1, 85–93. doi: 10.1007/S40641-015-0008-4/FIGURES/2
Baohua Z., Wang G., Huang B., Tseng C. K. (2004). Effects of temperature, hypoxia, ammonia and nitrate on the bleaching among three coral species. Chin. Sci. Bull. 49, 1923–1928. doi: 10.1007/bf03184283
Blanco J. F. (2009). The hydroclimatology of Gorgona Island: Seasonal and ENSO-Related patterns. Actual. Biol. 31, 111–121. doi: 10.17533/udea.acbi.331494
Bograd S. J., Jacox M. G., Hazen E. L., Lovecchio E., Montes I., Pozo Buil M., et al. (2023). Climate change impacts on eastern boundary upwelling systems. Ann. Rev. Mar. Sci. 15, 303–328. doi: 10.1146/annurev-marine-032122-021945
Bolton T. F., Havenhand J. N. (2005). Physiological acclimation to decreased water temperature and the relative importance of water viscosity in determining the feeding performance of larvae of a serpulid polychaete. J. Plankton Res. 27, 875–879. doi: 10.1093/plankt/fbi060
Castillo K. D., Helmuth B. S. T. (2005). Influence of thermal history on the response of Montastraea annularis to short-term temperature exposure. Mar. Biol. 148, 261–270. doi: 10.1007/s00227-005-0046-x
Castrillón-Cifuentes A. L., Lozano-Cortés D. F., Zapata F. A. (2017). Effect of short-term subaerial exposure on the cauliflower coral, Pocillopora damicornis, during a simulated extreme low-tide event. Coral Reefs 36, 401–414. doi: 10.1007/s00338-017-1552-2
Castrillón-Cifuentes A. L., Zapata F. A., Giraldo A., Wild C. (2023). Spatiotemporal variability of oxygen concentration in coral reefs of Gorgona Island (Eastern Tropical Pacific) and its effect on the coral Pocillopora capitata. PeerJ 11, e14586. doi: 10.7717/peerj.14586
Céspedes-Rodríguez E., Londoño-Cruz E. (2021). Gross calcium carbonate production in Eastern Tropical Pacific coral reefs (Gorgona Island, Colombia). Mar. Ecol. Prog. Ser. 665, 37–46. doi: 10.3354/meps13643
Chollett I., Mumby P. J., Cortés J. (2010). Upwelling areas do not guarantee refuge for coral reefs in a warming Ocean. Mar. Ecol. Prog. Ser. 416, 47–56. doi: 10.3354/meps08775
Clarke T. M., Reygondeau G., Wabnitz C., Robertson R., Ixquiac-Cabrera M., López M., et al. (2021). Climate change impacts on living marine resources in the Eastern Tropical Pacific. Divers. Distrib. 27, 65–81. doi: 10.1111/ddi.13181
Coles S. L., Jokiel P. L. (1977). Effects of temperature on photosynthesis and respiration in hermatypic corals. Mar. Biol. 43, 209–216. doi: 10.1007/BF00402313
Colombo-Pallotta M. F., Rodríguez-Román A., Iglesias-Prieto R. (2010). Calcification in bleached and unbleached Montastraea faveolata: Evaluating the role of oxygen and glycerol. Coral Reefs 29, 899–907. doi: 10.1007/s00338-010-0638-x
Coria-Monter E., Salas de León D. A., Monreal-Gómez M. A., Durán-Campos E. (2019). Satellite observations of the effect of the “Godzilla El Niño” on the Tehuantepec upwelling system in the Mexican Pacific. Helgol. Mar. Res. 73, 1–11. doi: 10.1186/s10152-019-0525-y
Corredor-Acosta A., Cortés-Chong N., Acosta A., Pizarro-Koch M., Vargas A., Medellín-Mora J., et al. (2020). Spatio-temporal variability of chlorophyll-a and environmental variables in the Panama bight. Remote Sens. 12, 2150. doi: 10.3390/rs12132150
Cuellar-Chacon A. (2017). Efecto de la Isla Gorgona, Pacífico Colombiano, sobre el patron de distribucion de las larvas de peces (Cali, Colombia: MSc Thesis. Universidad del Valle, Facultad de Ciencias Naturales y Exactas, Posgrado en Biología).
Cunning R., Gillette P., Capo T., Galvez K., Baker A. C. (2015). Growth tradeoffs associated with thermotolerant symbionts in the coral Pocillopora damicornis are lost in warmer oceans. Coral Reefs 34, 155–160. doi: 10.1007/s00338-014-1216-4
Cunning R., Glynn P. W., Baker A. C. (2013). Flexible associations between Pocillopora corals and Symbiodinium limit utility of symbiosis ecology in defining species. Coral Reefs 32, 795–801. doi: 10.1007/s00338-013-1036-y
Daniel R. M., Peterson M. E., Danson M. J., Price N. C., Kelly S. M., Monk C. R., et al. (2010). The molecular basis of the effect of temperature on enzyme activity. Biochem. J. 425, 353–360. doi: 10.1042/BJ20091254
Davy S. K., Allemand D., Weis V. M. (2012). Cell biology of cnidarian-dinoflagellate symbiosis. Microbiol. Mol. Biol. Rev. 76, 229–261. doi: 10.1128/mmbr.05014-11
D’Croz L., Maté J. L. (2004). Experimental responses to elevated water temperature in genotypes of the reef coral Pocillopora damicornis from upwelling and non-upwelling environments in Panama. Coral Reefs 23, 473–483. doi: 10.1007/s00338-004-0397-7
D’Croz L., O’Dea A. (2007). Variability in upwelling along the Pacific shelf of Panama and implications for the distribution of nutrients and chlorophyll. Estuar. Coast. Shelf Sci. 73, 325–340. doi: 10.1016/j.ecss.2007.01.013
Deleja M., Paula J. R., Repolho T., Franzitta M., Baptista M., Lopes V., et al. (2022). Effects of hypoxia on coral photobiology and oxidative stress. Biol. (Basel). 11, 1068. doi: 10.3390/biology11071068
Dodds L. A., Roberts J. M., Taylor A. C., Marubini F. (2007). Metabolic tolerance of the cold-water coral Lophelia pertusa (Scleractinia) to temperature and dissolved oxygen change. J. Exp. Mar. Bio. Ecol. 349, 205–214. doi: 10.1016/j.jembe.2007.05.013
Edmunds P. J. (2005). Effect of elevated temperature on aerobic respiration of coral recruits. Mar. Biol. 146, 655–663. doi: 10.1007/s00227-004-1485-5
Enochs I. C., Toth L. T., Kirkland A., Manzello D. P., Kolodziej G., Morris J. T., et al. (2021). Upwelling and the persistence of coral-reef frameworks in the eastern tropical Pacific. Ecol. Monogr. 91, 1–16. doi: 10.1002/ecm.1482
Fagoonee I., Wilson H., Hassell M., Turner J. (1999). The dynamics of zooxanthellae population: A long-term study in the field. Science 283, 843–845. doi: 10.1126/science.283.5403.843
Fangue N. A., Richards J. G., Schulte P. M. (2009). Do mitochondrial properties explain intraspecific variation in thermal tolerance? J. Exp. Biol. 212, 514–522. doi: 10.1242/jeb.024034
Fiedler P. C., Lavín M. F. (2017). “Oceanographic conditions of the eastern tropical pacific,” in Coral reefs of the eastern tropical pacific. Coral reefs of the world. Eds. Glynn P. W., Manzello D. P., Enochs I. C. (Dordrecht: Springer, Dordrecht), 59–83. doi: 10.1007/978-94-017-7499-4_3
Fitt W. K., Brown B. E., Warner M. E., Dunner R. P. (2001). Coral bleaching: Interpretation of thermal tolerance limits and thermal thresholds in tropical corals. Coral Reefs 20, 51–65. doi: 10.1007/s003380100146
Flores-Ramírez L. A., Liñán-Cabello M. A. (2007). Relationships among thermal stress, bleaching and oxidative damage in the hermatypic coral, Pocillopora capitata. Comp. Biochem. Physiol. - C Toxicol. Pharmacol. 146, 194–202. doi: 10.1016/j.cbpc.2006.09.008
Gantt S. E., Keister E. F., Manfroy A. A., Merck D. E., Fitt W. K., Muller E. M., et al. (2023). Wild and nursery-raised corals: comparative physiology of two framework coral species. Coral Reefs 42, 299–310. doi: 10.1007/s00338-022-02333-9
García-Reyes M., Sydeman W. J., Schoeman D. S., Rykaczewski R. R., Black B. A., Smit A. J., et al. (2015). Under pressure: Climate change, upwelling, and eastern boundary upwelling ecosystems. Front. Mar. Sci. 2. doi: 10.3389/fmars.2015.00109
Gardella D. J., Edmunds P. J. (1999). The oxygen microenvironment adjacent to the tissue of the scleractinian Dichocoenia stokesii and its effects on symbiont metabolism. Mar. Biol. 135, 289–295. doi: 10.1007/s002270050626
Giraldo A., Rodríguez-Rubio E., Zapata F. (2008). Condiciones oceanográficas en isla Gorgona, Pacífico Oriental Tropical de Colombia. Lat. Am. J. Aquat. Res. 36, 121–128. doi: 10.3856/vol36-issue1-fulltext-12
Giraldo A., Valencia B. (2013). “Monitoreo del ambiente pelágico del PNN Gorgona: marzo 2013,” in Informe Técnico producto No. 8 del proyecto de investigación (Cali: Monitoreo de los valores objeto de conservación priorizados para las áreas protegidas Gorgona y Utría adscritas a la Dirección Terri).
Giraldo A., Valencia B., Acevedo J. D., Rivera M. (2014). Fitoplancton y zooplancton en el área marina protegida de Isla Gorgona, Colombia, y su relación convariables oceanográficas en estaciones lluviosa y seca. Rev. Biol. Trop. 62, 117–132. doi: 10.15517/rbt.v62i0.15982
Glynn P. W., D’croz L. (1990). Experimental evidence for high temperature stress as the cause of El Niño-coincident coral mortality. Coral Reefs 8, 181–191. doi: 10.1007/BF00265009
Glynn P. W., Fong P. (2006). Patterns of reef coral recovery by the regrowth of surviving tissue following the 1997-98 El Niño warming and 2000 , 2001 upwelling cool events in Panamá, eastern Pacific. 10th Int. Coral Reef Symposium, 624–630.
Glynn P. W., Manzello D. P., Enochs I. C. (2017a). Coral reefs of the eastern tropical pacific persistence and loss in a dynamic environment. 8th ed. Eds. Glynn P. W., Manzello D. P., Enochs I. C. (Dordrecht: Springer, Dordrecht). doi: 10.1007/978-94-017-7499-4
Glynn P. W., Maté J. L., Baker A. C., Calderon M. O. (2001). Coral bleaching and mortality in panama and Ecuador during the 1997-998 El Niño-Southern Oscillation Event: Spatial/temporal patterns and comparisons with the 1982-1983 event. Bull. Mar. Sci. 69, 79–109.
Glynn P. W., Mones A. B., Podestá G. P., Colbert A., Colgan M. W. (2017b). “El niño-southern oscillation: effects on eastern pacific coral reefs and associated biota,” in Coral reefs of the eastern tropical pacific. Coral reefs of the world. Eds. Glynn P. W., Manzello D. P., Enochs I. C. (Dordrecht: Springer, Dordrecht), 251–290. doi: 10.1007/978-94-017-7499-4_8
Glynn P. W., Stewart R. H. (1973). Distribution of coral reefs in the pearl islands (Gulf of panama) in relation to thermal conditions. Limnol. Oceanogr. 18, 367–379. doi: 10.4319/lo.1973.18.3.0367
Gnaiger E. (2001). Bioenergetics at low oxygen: dependence of respiration and phosphorylation on oxygen and adenosine diphosphate supply. Respir. Physiol. 128, 277–297. doi: 10.1016/S0034-5687(01)00307-3
Gravinese P. M., Douwes A., Eaton K. R., Muller E. M. (2022). Ephemeral hypoxia reduces oxygen consumption in the Caribbean coral Orbicella faveolata. Coral Reefs 41, 13–18. doi: 10.1007/s00338-021-02197-5
Grieshaber M. K., Kreutzer U., Pörtner H. O. (1988). “Critical PO2 of euryoxic animals,” in Oxygen sensing in tissues. Ed. Acker H. (Berlin, Heidelberg: Springer), 37–48. doi: 10.1007/978-3-642-83444-8_3
Guzman H., Cortés J., Glynn P., Richmond R. (1990). Coral mortality associated with dinoflagellate blooms in the eastern Pacific (Costa Rica and Panama). Mar. Ecol. Prog. Ser. 60, 299–303. doi: 10.3354/meps060299
Haas A. F., Smith J. E., Thompson M., Deheyn D. D. (2014). Effects of reduced dissolved oxygen concentrations on physiology and fluorescence of hermatypic corals and benthic algae. PeerJ 2014, e235. doi: 10.7717/peerj.235
Haydon T. D., Seymour J. R., Raina J.-B., Edmondson J., Siboni N., Matthews J. L., et al. (2021). Rapid shifts in bacterial communities and homogeneity of symbiodiniaceae in colonies of Pocillopora acuta transplanted between reef and mangrove environments. Front. Microbiol. 12. doi: 10.3389/fmicb.2021.756091
Hoogenboom M., Beraud E., Ferrier-Pagès C. (2010). Relationship between symbiont density and photosynthetic carbon acquisition in the temperate coral Cladocora caespitosa. Coral Reefs 29, 21–29. doi: 10.1007/s00338-009-0558-9
Hueerkamp C., Glynn P. W., D’croz L., Maté J. L., Colley S. B. (2001). Bleaching and recovery of five Eastern Pacific corals in an El Niño-related temperature experiment. Bull. Mar. Sci. 69, 215–236.
Hughes D. J., Alderdice R., Cooney C., Kühl M., Pernice M., Voolstra C. R., et al. (2020). Coral reef survival under accelerating ocean deoxygenation. Nat. Clim. Change 10, 296–307. doi: 10.1038/s41558-020-0737-9
Hughes D. J., Alexander J., Cobbs G., Kühl M., Cooney C., Pernice M., et al. (2022). Widespread oxyregulation in tropical corals under hypoxia. Mar. Pollut. Bull. 179, 113722. doi: 10.1016/j.marpolbul.2022.113722
Hughes T. P., Anderson K. D., Connolly S. R., Heron S. F., Kerry J. T., Lough J. M., et al. (2018). Spatial and temporal patterns of mass bleaching of corals in the Anthropocene. Science 359, 80–83. doi: 10.1126/science.aan8048
Hughes T. P., Kerry J. T., Álvarez-Noriega M., Álvarez-Romero J. G., Anderson K. D., Baird A. H., et al. (2017). Global warming and recurrent mass bleaching of corals. Nature 543, 373–377. doi: 10.1038/nature21707
Ishida-Castañeda J., Pizarro V., López-Victoria M., Zapata F. A. (2020). Coral reef restoration in the Eastern Tropical Pacific: feasibility of the coral nursery approach. Restor. Ecol. 28, 22–28. doi: 10.1111/rec.13047
Jiménez C., Cortés J. (2003). Growth of seven species of scleractinian corals in an upwelling environment of the eastern Pacific (Golfo de Papagayo, Costa Rica). Bull. Mar. Sci. 72, 187–198.
Johnson M. D., Scott J. J., Leray M., Lucey N., Bravo L. M. R., Wied W. L., et al. (2021). Rapid ecosystem-scale consequences of acute deoxygenation on a Caribbean coral reef. Nat. Commun. 12, 4522. doi: 10.1038/s41467-021-24777-3
Jones R. J., Yellowlees D. (1997). Regulation and control of intracellular algae (zooxanthellae) in hard corals. Phil. Trans. R. Soc Lond. B 352, 457–468. doi: 10.1098/RSTB.1997.0033
Kemp D. W., Colella M. A., Bartlett L. A., Ruzicka R. R., Porter J. W., Fitt W. K. (2016). Life after cold death: Reef coral and coral reef responses to the 2010 cold water anomaly in the Florida Keys. Ecosphere 7, 1–17. doi: 10.1002/ecs2.1373
Kemp D. W., Oakley C. A., Thornhill D. J., Newcomb L. A., Schmidt G. W., Fitt W. K. (2011). Catastrophic mortality on inshore coral reefs of the Florida Keys due to severe low-temperature stress. Glob. Change Biol. 17, 3468–3477. doi: 10.1111/j.1365-2486.2011.02487.x
Kerswell A. P., Jones R. J. (2003)Effects of hypo-osmosis on the coral Stylophora pistillata: Nature and cause of “low-salinity bleaching.” Mar. Ecol. Prog. Ser. 253, 145–154doi: 10.3354/meps253145
Kongjandtre N., Wattana T., Veerapitipath V., Chinchana A. (2021) Effect of hyposalinity on coral bleaching and survival in a hard (Pocillopora damicornis) and soft coral (Sarcophyton sp) | Naresuan University Journal: Science and Technology (NUJST) Naresuan Univ. J. Sci. Technol. 30, 38–49.
LaJeunesse T. C., Parkinson J. E., Gabrielson P. W., Jeong H. J., Reimer J. D., Voolstra C. R., et al. (2018). Systematic revision of symbiodiniaceae highlights the antiquity and diversity of coral endosymbionts. Curr. Biol. 28, 2570–2580.e6. doi: 10.1016/j.cub.2018.07.008
Ledesma J., Graco M., Tam J., Díaz K., Anculle T., García W., et al. (2022). Efectos de El Niño Costero 2017 sobre la oxigenación, fertilidad y productividad del mar frente a las costas del Perú. Boletin Instituto del Mar. del Perú 36, 409–427. doi: 10.53554/boletin.v36i2.345
Le Moullac G., Quéau I., Le Souchu P., Pouvreau S., Moal J., Le Coz J. R., et al. (2007). Metabolic adjustments in the oyster Crassostrea gigas according to oxygen level and temperature. Mar. Biol. Res. 3, 357–366. doi: 10.1080/17451000701635128
Linsmayer L. B., Deheyn D. D., Tomanek L., Tresguerres M. (2020). Dynamic regulation of coral energy metabolism throughout the diel cycle. Sci. Rep. 10, 19881. doi: 10.1038/s41598-020-76828-2
Little A. F., Van Oppen M. J. H., Willis B. L. (2004). Flexibility in algal endosymbioses shapes growth in reef corals. Science 304, 1492–1494. doi: 10.1126/science.1095733
Lizcano-Sandoval L. D., Londoño-Cruz E., Zapata F. A. (2018). Growth and survival of Pocillopora damicornis (Scleractinia: Pocilloporidae) coral fragments and their potential for coral reef restoration in the Tropical Eastern Pacific. Mar. Biol. Res. 14, 887–897. doi: 10.1080/17451000.2018.1528011
Lucey N. M., Haskett E., Collin R. (2021). Hypoxia from depth shocks shallow tropical reef animals. Clim. Change Ecol. 2, 100010. doi: 10.1016/j.ecochg.2021.100010
Manzello D. P. (2010a). Coral growth with thermal stress and ocean acidification: Lessons from the eastern tropical Pacific. Coral Reefs 29, 749–758. doi: 10.1007/s00338-010-0623-4
Manzello D. P. (2010b). Ocean acidification hot spots: Spatiotemporal dynamics of the seawater CO2 system of eastern Pacific coral reefs. Limnol. Ocean. 55, 239–248. doi: 10.4319/lo.2010.55.1.0239
Manzello D. P., Kleypas J. A., Budd D. A., Eakin C. M., Glynn P. W., Langdon C. (2008). Poorly cemented coral reefs of the eastern tropical Pacific: Possible insights into reef development in a high-CO2 world. Proc. Natl. Acad. Sci. U. S. A. 105, 10450–10455. doi: 10.1073/pnas.0712167105
Martínez-Castillo V., Rodríguez-Troncoso A. P., Santiago-Valentín J. D., Cupul-Magaña A. L. (2020). The influence of urban pressures on coral physiology on marginal coral reefs of the Mexican Pacific. Coral Reefs 39, 625–637. doi: 10.1007/s00338-020-01957-z
Matta J., Trench R. (1991). The enzymatic response of the symbiotic dinoflagellate Symbiodinium microadriaticum (Freudenthal) to growth in vitro under varied oxygen tensions. Symbiosis 11, 31–45.
Mayfield A. B., Gates R. D. (2007). Osmoregulation in anthozoan-dinoflagellate symbiosis. Comp. Biochem. Physiol. - Part A 147, 1–10. doi: 10.1016/j.cbpa.2006.12.042
McArley T. J., Hickey A. J. R., Wallace L., Kunzmann A., Herbert N. A. (2019). Intertidal triplefin fishes have a lower critical oxygen tension (P crit), higher maximal aerobic capacity, and higher tissue glycogen stores than their subtidal counterparts. J. Comp. Physiol. B Biochem. Syst. Environ. Physiol. 189, 399–411. doi: 10.1007/s00360-019-01216-w
McLachlan R. H., Price J. T., Solomon S. L., Grottoli A. G. (2020). Thirty years of coral heat-stress experiments: a review of methods. Coral Reefs 39, 885–902. doi: 10.1007/s00338-020-01931-9
Medellín-Maldonado F., Cabral-Tena R. A., López-Pérez A., Calderón-Aguilera L. E., Norzagaray-López C. O., Chapa-Balcorta C., et al. (2016). Calcification of the main reef-building coral species on the Pacific coast of southern Mexico. Cienc. Mar. 42, 209–225. doi: 10.7773/cm.v42i3.2650
Miller A. W., Richardson L. L. (2015). Emerging coral diseases: A temperature-driven process? Mar. Ecol. 36, 278–291. doi: 10.1111/maec.12142
Mora C., Ospina A. F. (2002). Experimental effect of cold, La Niña temperatures on the survival of reef fishes from Gorgona Island (eastern Pacific Ocean). Mar. Biol. 141, 789–793. doi: 10.1007/s00227-002-0862-1
Muñoz C. G., Jaramillo-González J., Zapata F. A. (2018). Evidence of sexually-produced coral recruitment at Gorgona Island, Eastern Tropical Pacific. Bol. Investig. Mar. y Costeras 47, 97–110. doi: 10.25268/bimc.invemar.2018.47.2.749
Murphy J. W. A., Richmond R. H. (2016). Changes to coral health and metabolic activity under oxygen deprivation. PeerJ 2016, e1956. doi: 10.7717/peerj.1956
Muscatine L., Grossman D., Doino J. (1991). Release of symbiotic algae by tropical sea anemones and corals after cold shock. Mar. Ecol. Prog. Ser. 77, 233–243. doi: 10.3354/meps077233
Negrete B. Jr., Esbaugh A. J. (2019). A methodological evaluation of the determination of critical oxygen threshold in an estuarine teleost. Biol. Open 8, bio045310. doi: 10.1242/bio.045310
Osinga R., Derksen-Hooijberg M., Wijgerde T., Verreth J. A. J. (2017). Interactive effects of oxygen, carbon dioxide and flow on photosynthesis and respiration in the scleractinian coral Galaxea fascicularis. J. Exp. Biol. 220, 2236–2242. doi: 10.1242/jeb.140509
Pacherres C. O., Ahmerkamp S., Koren K., Richter C., Holtappels M. (2022). Ciliary flows in corals ventilate target areas of high photosynthetic oxygen production. Curr. Biol. 32, 4150–4158.e3. doi: 10.1016/j.cub.2022.07.071
Pacherres C. O., Ahmerkamp S., Schmidt-Grieb G. M., Holtappels M., Richter C. (2020). Ciliary vortex flows and oxygen dynamics in the coral boundary layer. Sci. Rep. 10, 1–10. doi: 10.1038/s41598-020-64420-7
Palacio-Castro A. M., Smith T. B., Brandtneris V., Snyder G. A., Van Hooidonk R., Maté J. L., et al. (2022). Increased dominance of heat-tolerant symbionts creates resilient coral reefs in near-term ocean warming. Proc. Natl. Acad. Sci. 120, e2202388120. doi: 10.1073/pnas
Palmer C., Jimenez C., Bassey G., Ruiz E., Cubero T. V., Diaz M. M. C., et al. (2022). Cold water and harmful algal blooms linked to coral reef collapse in the Eastern Tropical Pacific. PeerJ 10, 1–18. doi: 10.7717/peerj.14081
Paradis B. T., Henry R. P., Chadwick N. E. (2019). Compound effects of thermal stress and tissue abrasion on photosynthesis and respiration in the reef-building coral Acropora cervicornis (Lamarc ). J. Exp. Mar. Bio. Ecol. 521, 151222. doi: 10.1016/j.jembe.2019.151222
Paz-García D. A., Hellberg M. E., García-de-León F. J., Balart E. F. (2015). Switch between morphospecies of pocillopora corals. Am. Nat. 186, 434–440. doi: 10.1086/682363
Pinzón J. H., Lajeunesse T. C. (2011). Species delimitation of common reef corals in the genus Pocillopora using nucleotide sequence phylogenies, population genetics and symbiosis ecology. Mol. Ecol. 20, 311–325. doi: 10.1111/j.1365-294X.2010.04939.x
Pinzón J. H., Sampayo E., Cox E., Chauka L. J., Chen C. A., Voolstra C. R., et al. (2013). Blind to morphology: Genetics identifies several widespread ecologically common species and few endemics among Indo-Pacific cauliflower corals (Pocillopora, Scleractinia). J. Biogeogr. 40, 1595–1608. doi: 10.1111/jbi.12110
Poveda G., Waylen P. R., Pulwarty R. S. (2006). Annual and inter-annual variability of the present climate in northern South America and southern Mesoamerica. Palaeogeogr. Palaeoclimatol. Palaeoecol. 234, 3–27. doi: 10.1016/j.palaeo.2005.10.031
Raj K. D., Mathews G., Obura D. O., Laju R. L., Bharath M. S., Kumar P. D., et al. (2020). Low oxygen levels caused by Noctiluca scintillans bloom kills corals in Gulf of Mannar, India. Sci. Rep. 10, 22133. doi: 10.1038/s41598-020-79152-x
Ramírez-Martínez G. A., Giraldo A., Rivera-Gómez M., Aceves-Medina G. (2022). Daily and monthly ichthyoplankton assemblages of La Azufrada coral reef, Gorgona Island, Eastern Tropical Pacific. Reg. Stud. Mar. Sci. 52, 102378. doi: 10.1016/j.rsma.2022.102378
Randall C. J., Toth L. T., Leichter J. J., Maté J. L., Aronson R. B. (2020). Upwelling buffers climate change impacts on coral reefs of the eastern tropical Pacific. Ecology 101, e02918. doi: 10.1002/ecy.2918
Regan M. D., Mandic M., Dhillon R. S., Lau G. Y., Farrell A. P., Schulte P. M., et al. (2019). Don’t throw the fish out with the respirometry water. J. Exp. Biol. 222, 9–10. doi: 10.1242/jeb.200253
Riegl B., Glynn P. W., Banks S., Keith I., Rivera F., Vera-Zambrano M., et al. (2019). Heat attenuation and nutrient delivery by localized upwelling avoided coral bleaching mortality in northern Galapagos during 2015/2016 ENSO. Coral Reefs 38, 773–785. doi: 10.1007/s00338-019-01787-8
Rixen T., Jiménez C., Cortés J. (2012). Impact of upwelling events on the sea water carbonate chemistry and dissolved oxygen concentration in the Gulf of Papagayo (Culebra Bay), Costa Rica: Implications for coral reefs. Rev. Biol. Trop. 60, 187–195. doi: 10.15517/rbt.v60i2.20004
Rodriguez-Ruano V., Toth L. T., Enochs I. C., Randall C. J., Aronson R. B. (2023). Upwelling, climate change, and the shifting geography of coral reef development. Sci. Rep. 13, 1–15. doi: 10.1038/s41598-023-28489-0
Rodriguez-Rubio E., Stuardo J. (2002). Variability of photosynthetic pigments in the Colombian Pacific Ocean and its relationship with the wind field using ADEOS-I data. Proc. Indian Acad. Sci. Earth Planet. Sci. 111, 227–236. doi: 10.1007/BF02701969
Rodríguez-Troncoso A. P., Carpizo-Ituarte E., Cupul-Magaña A. L. (2016). Physiological response to high temperature in the Tropical Eastern Pacific coral Pocillopora verrucosa. Mar. Ecol. 37, 1168–1175. doi: 10.1111/maec.12392
Rodríguez-Troncoso A. P., Carpizo-Ituarte E., Pettay D. T., Warner M. E., Cupul-Magaña A. L. (2014). The effects of an abnormal decrease in temperature on the Eastern Pacific reef-building coral Pocillopora verrucosa. Mar. Biol. 161, 131–139. doi: 10.1007/s00227-013-2322-5
Rogers N. J., Urbina M. A., Reardon E. E., McKenzie D. J., Wilson R. W. (2016). A new analysis of hypoxia tolerance in fishes using a database of critical oxygen level (Pcrit). Conserv. Physiol. 4, 1–19. doi: 10.1093/conphys/cow012
Salin K., Auer S. K., Rey B., Selman C., Metcalfe N. B. (2015). Variation in the link between oxygen consumption and ATP production, and its relevance for animal performance. Proc. R. Soc B Biol. Sci. 282, 20151028. doi: 10.1098/rspb.2015.1028
Sampson L., Giraldo A. (2014). Annual abundance of salps and doliolids (Tunicata) around Gorgona Island (Colombian Pacific), and their importance as potential food for green sea turtles. Rev. Biol. Trop. 62, 149–159. doi: 10.15517/rbt.v62i0.15984
Sawall Y., Al-Sofyani A., Banguera-Hinestroza E., Voolstra C. R. (2014). Spatio-temporal analyses of Symbiodinium physiology of the coral Pocillopora verrucosa along large-scale nutrient and temperature gradients in the Red Sea. PloS One 9, e103179. doi: 10.1371/journal.pone.0103179
Sawall Y., Nicosia A. M., McLaughlin K., Ito M. (2022). Physiological responses and adjustments of corals to strong seasonal temperature variations (20-28°C). J. Exp. Biol. 225, jeb244196. doi: 10.1242/jeb.244196
Schmidt-Roach S., Miller K. J., Lundgren P., Andreakis N. (2014). With eyes wide open: a revision of species within and closely related to the Pocillopora damicornis species complex (Scleractinia; Pocilloporidae) using morphology and genetics. Zool. J. Linn. Soc 170, 1–33. doi: 10.1111/ZOJ.12092
Seibel B. A., Andres A., Birk M. A., Burns A. L., Shaw C. T., Timpe A. W., et al. (2021). Oxygen supply capacity breathes new life into critical oxygen partial pressure (Pcrit). J. Exp. Biol. 224, jeb242210. doi: 10.1242/JEB.242210
Shapiro O. H., Fernandez V. I., Garren M., Guasto J. S., Debaillon-Vesque F. P., Kramarsky-Winter E., et al. (2014). Vortical ciliary flows actively enhance mass transport in reef corals. Proc. Natl. Acad. Sci. U. S. A. 111, 13391–13396. doi: 10.1073/pnas.1323094111
Siebeck U. E., Marshall N. J., Klüter A., Hoegh-Guldberg O. (2006). Monitoring coral bleaching using a colour reference card. Coral Reefs 25, 453–460. doi: 10.1007/s00338-006-0123-8
Smith T. B. (2006). Benthic algal production in an upwelling coral reef environment ( Gulf of Panamá ): evidence for control by fish herbivores. 10th Int. Coral Reef Symposium, 854–861.
Sokolova I. M., Pörtner H. O. (2003). Metabolic plasticity and critical temperatures for aerobic scope in a eurythermal marine invertebrate (Littorina saxatilis, Gastropoda: Littorinidae) from different latitudes. J. Exp. Biol. 206, 195–207. doi: 10.1242/JEB.00054
Spring D. L., Williams G. J. (2023). Influence of upwelling on coral reef benthic communities : a systematic review and meta-analysis. Proc. R. Soc B 290, 20230023. doi: 10.1098/rspb.2023.0023
Stimson J. (1997). The annual cycle of density of zooxanthellae in the tissues of field and laboratory-held Pocillopora damicornis (Linnaeus). J. Exp. Mar. Bio. Ecol. 214, 35–48. doi: 10.1016/S0022-0981(96)02753-0
Stramma L., Johnson G. C., Sprintall J., Mohrholz V. (2008). Expanding oxygen-minimum zones in the tropical oceans. Science 320, 655–658. doi: 10.1126/science.1153847
Stuhldreier I., Sánchez-Noguera C., Rixen T., Cortés J., Morales A., Wild C. (2015). Effects of seasonal upwelling on inorganic and organic matter dynamics in the water column of eastern Pacific coral reefs. PloS One 10, e0142681. doi: 10.1371/journal.pone.0142681
Sydeman W. J., García-Reyes M., Schoeman D. S., Rykaczewski R. R., Thompson S. A., Black B. A., et al. (2014). Climate change and wind intensification in coastal upwelling ecosystems. Science 345, 77–80. doi: 10.1126/science.1251635
Tortolero-Langarica J., de J. A., Rodríguez-Troncoso A. P., Cupul-Magaña A. L. (2017). and carricart-ganivet, J Calcification and growth rate recovery of the reef-building Pocillopora species in the northeast tropical Pacific following an ENSO disturbance. P. PeerJ 5, e3191. doi: 10.7717/peerj.3191
Toth L. T., Aronson R. B., Cheng H., Edwards R. L. (2015). Holocene variability in the intensity of wind-gap upwelling in the tropical eastern Pacific. Paleoceanography 30, 1113–1131. doi: 10.1002/2015PA002794
Toth L. T., Aronson R. B., Vollmer S. V., Hobbs J. W., Urrego D. H., Cheng H., et al. (2012). ENSO drove 2500-year collapse of Eastern Pacific coral reefs. Science 336, 81–84. doi: 10.1126/science.1221168
Toth L. T., Macintyre I. G., Aronson R. B., Toth L. T., Aronson Á.R.B., Macintyre I. G. (2017). “Holocene reef development in the eastern tropical pacific,” in Coral reefs of the eastern tropical pacific. Coral reefs of the world. Eds. Glynn P. W., Manzello D. P., Enochs I. C. (Dordrecht: Springer, Dordrecht), 177–201. doi: 10.1007/978-94-017-7499-4_6
Ulbricht R. J. (1973). Effect of temperature acclimation on the metabolic rate of sea urchins. Mar. Biol. 19, 273–277. doi: 10.1007/BF00348893
Ulstrup K., Hill R., Railp P. (2005). Photosynthetic impact of hypoxia on in hospitezooxanthellae in the scleractinian coralPocillopora damicornis. Mar. Ecol. Prog. Ser. 286, 125–132. doi: 10.3354/meps286125
Ultsch G. R., Boschung H., Ross M. J. (1978). Metabolism , critical oxygen tension , and habitat selection in darters ( Etheostoma ). Ecology 59, 99–107. doi: 10.2307/1936635
Van Oppen M. J. H., Lough J. M. (2009). Coral Bleaching: patterns, processes, causes and consequences. Eds. Van Oppen M. J. H., Lough J. M. (Berlin, Heidelberg: Springer). doi: 10.1017/s1092852900015558
Vaquer-Sunyer R., Duarte C. M. (2008). Thresholds of hypoxia for marine biodiversity. PNAS 105, 15452–15457. doi: 10.1073/pnas.0803833105
Vargas-Angel B. (1996). Distribution and community structure of the reef corals of Ensenada de Utría, Pacitic coast of Colombia. Rev. Biol. Trop. 44, 643–651.
Vargas-Ángel B., Zapata F. A., Hernández H., Jiménez J. M. (2001). Coral and coral reef responses to the 1997-98 El Niño event on the Pacific coast of Colombia. Bull. Mar. Sci. 69, 111–132.
Veron J., Stafford-Smith M., Turak E., DeVantier L. (2022). Corals of the world. Available at: http://www.coralsoftheworld.org/page/citation-guide/ (Accessed August 16, 2022).
Vivas Aguas L. J., Sánchez J., Betancourt J. M., Quintero M., Moreno Y., Cuadrado I., et al. (2014). Diagnóstico y Evaluación de la Calidad de Aguas Marinas y Costeras en el Caribe y Pacífico Colombianos (Santa Marta:Instituto de Investigaciones Marinas y Costeras José Benito Vives de Andréis (Invemar)). Available at: http://www.invemar.org.co/redcostera1/invemar/docs/INFORME_REDCAM_2013_4.pdf.
Wang C., Deser C., Yu J.-Y., Dinezio P., Clement A., Wang C., et al. (2017). “El niño and southern oscillation (ENSO): A review,” in Coral reefs of the eastern tropical pacific. Coral reefs of the world. Eds. Glynn P. W., Manzello D. P., Enochs I. C. (Dordrecht: Springer, Dordrecht), 85–106. doi: 10.1007/978-94-017-7499-4_4
Weber M., De Beer D., Lott C., Polerecky L., Kohls K., Abed R. M. M., et al. (2012). Mechanisms of damage to corals exposed to sedimentation. Proc. Natl. Acad. Sci. U. S. A. 109, E1558–E1567. doi: 10.1073/pnas.1100715109
Welker A. F., Moreira D. C., Campos É.G., Hermes-Lima M. (2013). Role of redox metabolism for adaptation of aquatic animals to drastic changes in oxygen availability. Comp. Biochem. Physiol. - A Mol. Integr. Physiol. 165, 384–404. doi: 10.1016/j.cbpa.2013.04.003
Wijgerde T., Jurriaans S., Hoofd M., Verreth J. A. J., Osinga R. (2012). Oxygen and heterotrophy affect calcification of the scleractinian coral galaxea fascicularis. PloS One 7, e52702. doi: 10.1371/journal.pone.0052702
Wijgerde T., Silva C. I. F., Scherders V., Van Bleijswijk J., Osinga R. (2014). Coral calcification under daily oxygen saturation and pH dynamics reveals the important role of oxygen. Biol. Open 3, 489–493. doi: 10.1242/bio.20147922
Wooldridge S. A. (2014). Assessing coral health and resilience in a warming ocean: Why looks can be deceptive. BioEssays 36, 1041–1049. doi: 10.1002/bies.201400074
Yeager D. P., Ultsch G. R. (1989). Physiological regulation and conformation: A BASIC program for the determination of critical points. Physiol. Zool. 62, 888–907. doi: 10.1086/physzool.62.4.30157935
Zapata F. A. (2001). “Formaciones coralinas de Isla Gorgona,” in Gorgona marina: Con- tribución al conocimiento de una isla única. Eds. Barrios L., López-Victoria M. (Santa Marta: INVEMAR, Serie de Publicaciones Especiales), 27–40.
Keywords: thermal stress, metabolic rate, growth rate, Zooxanthellae density, hypoxic threshold
Citation: Castrillón-Cifuentes AL, Zapata FA and Wild C (2023) Physiological responses of Pocillopora corals to upwelling events in the Eastern Tropical Pacific. Front. Mar. Sci. 10:1212717. doi: 10.3389/fmars.2023.1212717
Received: 26 April 2023; Accepted: 14 July 2023;
Published: 31 July 2023.
Edited by:
Tyler Burton Smith, University of the Virgin Islands, US Virgin IslandsCopyright © 2023 Castrillón-Cifuentes, Zapata and Wild. This is an open-access article distributed under the terms of the Creative Commons Attribution License (CC BY). The use, distribution or reproduction in other forums is permitted, provided the original author(s) and the copyright owner(s) are credited and that the original publication in this journal is cited, in accordance with accepted academic practice. No use, distribution or reproduction is permitted which does not comply with these terms.
*Correspondence: Ana Lucia Castrillón-Cifuentes, YW5hbHVjaWFAdW5pLWJyZW1lbi5kZQ==; Y2FzdHJpbGxvbi5hbmFAY29ycmVvdW5pdmFsbGUuZWR1LmNv
†These authors share senior authorship