- 1Guangxi Laboratory on the Study of Coral Reefs in the South China Sea, Coral Reef Research Center of China, School of Marine Sciences, Guangxi University, Nanning, China
- 2Southern Marine Science and Engineering Guangdong Laboratory (Guangzhou), Guangzhou, China
Remote seawater has been considered a potential refuge for corals in the face of anthropogenic disturbances. However, these remote areas may receive increased atmospheric N deposition, and the ecological consequences remain unclear. This field survey revealed coral-algal phase shifts in the mid-north of the South China Sea. These shifts were observed in 44%, 13.6%, and 26.5% of the sampled reef sites at depths of 1-4 m, 5-8 m, and 10-15 m, respectively. Over 50% of sections in the deeper depths hosted fewer corals compared to shallower areas, coinciding with a higher abundance of macroalgae in the deeper layers. Furthermore, based on long-term observation of atmospheric N flux, laboratory experiments were conducted to explore the cause of coral declines. The results indicate that N supply efficiently promoted macroalgae growth. The saturation of N absorption by macroalgae occurred within 2 weeks, leading to nutrient accumulation in seawater, especially nitrate, which had a direct impact on corals. While moderate N fluxes appeared to mitigate coral bleaching, high N fluxes, even with a balanced P supply or medium level of nutrients with an imbalanced N/P ratio, can both increase the susceptibility of corals to heat bleaching. This study explains the coral-algal phase shift in remote and relatively deep seawater and improves understanding of the cause-and-effect relationship between atmospheric N deposition and coral reef decline.
1 Introduction
Coral reef ecosystems have extremely high biodiversity (Zhao et al., 2006). However, in recent decades, many of these ecosystems have experienced significant declines due to climate warming and human disturbances (Gardner et al., 2003; Bellwood et al., 2004; Yu, 2012). Remote coral reefs, often considered isolated from coastal human activities, have been thought to potentially serve as refuges for corals (MacDonald et al., 2016). Nevertheless, a considerable amount of land-derived nutrients has entered the open ocean (Safai et al., 2004; Dentener et al., 2006; Kanakidou et al., 2012). Model estimates suggest that atmospheric N (AN) deposition has doubled over the past century and now accounts for one-third of the external N supply for the open ocean (Duce et al., 2008). Previous studies have indicated that coral reefs face pressures from AN. Wet deposition of inorganic nitrogen has led to macroalgae enrichment on remote coral reefs near Green Turtle Cay (Barile and Lapointe, 2005). Overgrowth of macroalgae correlated with AN deposition flux has also been observed in the South China Sea (SCS), and the AN flux could be significant in many coral reefs worldwide (Chen et al., 2019). However, the cause-and-effect relationship between AN deposition and coral reef decline remains unclear.
Negative effects of nutrient enrichment on corals have been demonstrated in many studies (Tomascik and Sander, 1985; Bell, 1992; Wiedenmann et al., 2013; Lapointe et al., 2019). An increase in NO3- concentration, from 0.41 to 5.13 µM, reduced coral growth rate by 53% within 1 month (Renegar and Riegl, 2005). N enrichment (NO3-, 6-7 µM; NH4+, 5-6 µM) reduced symbiotic algae density and gross photosynthesis within 6 weeks at normal temperatures in the Mediterranean (Hadjioannou et al., 2019). In contrast, treatment with dissolved inorganic nitrogen (DIN, 0.36 µM) and dissolved inorganic phosphorus (DIP, 0.14 µM) for 15 months led to a 50% increase in zooxanthellae density compared to that in lower levels of DIN and DIP (both ~ 0.1 µM; Becker et al., 2021). However, the excess zooxanthellae may affect symbiotic stability, potentially reducing the translocation of organic carbon from the symbiont to the coral host (Cunning and Baker, 2013; Ezzat et al., 2015).
The diverse effects of enrichment on corals are likely related to the level, forms, or ratio of nutrient loading. Globally, there are spatial differences in AN deposition flux. More than two-thirds of the world’s coral reefs receive medium to high levels of AN fluxes (400-2100 mg N·m-2·year-1), e.g., in the regions of Asia, Southeast Asia, East and North Asia, and the U.S. Caribbean islands, while others (Australia and Pacific Ocean) are exposed to much lower amounts (43-280 mg N·m-2·year-1; Chen et al., 2019). Rainwater DIN is usually 55.88 µM in the SCS (Cui et al., 2016). Under extreme conditions, such as dust storms and biomass burning, atmospheric deposition flux can increase tens to hundreds of times beyond the usual levels (Sundarambal et al., 2010; Guo et al., 2012). Data from the Acid Deposition Monitoring Network in East Asia (EANET) revealed that rainwater DIN reached 7890 µM in Kototabang in May, 2015, while it was 277 µM in Tanah Rata in June, 2011. These wet deposition fluxes can be estimated to elevate seawater DIN levels by 1.2-30 µM after thorough mixing (precipitation, 30 mm per day; water depth, 7 m). AN has become the primary external N input in the open areas of the SCS (Ren et al., 2017), and the deposition flux has been increasing (Duce et al., 2008).
As a considerable amount of AN continuously enters the ocean, the imbalance of N and P supply tends to be exacerbated, especially in the coral reef regions with weak water exchange, such as lagoons, or shallow semi-closed bays. Laboratory studies have suggested that this imbalance in N and P supply, rather than N enrichment, increases the susceptibility of corals to bleaching. This is because imbalanced nutrients induce P starvation of symbiotic algae, reducing coral tolerance to light and heat stress (Wiedenmann et al., 2013; Rosset et al., 2017). Field studies have also provided evidence that DIN enrichment exacerbates P limitation, leading to increased stress on corals at the Looe Key reef over three decades (Lapointe et al., 2019). In 2019, the N/P ratios of macroalgae were more than two-fold higher than in the 1980s, and P limitation was also observed to stress corals at the Belize Barrier Reef (Lapointe et al., 2021). On the other hand, some areas may receive excessive P supply from sources such as phosphate rock, seabird excrement, or volcanic eruption (Olgun et al., 2013; Shatova et al., 2017). P loading can alleviate P limitation for corals, but the combined effects of N and P have also been documented. For example, NH4+ (8.5 µM) and P (2.4 µM) treatment reduced the coral growth rate by 50% within 4 weeks, while NH4+ (9 µM) treatment only reduced growth by 8~10% (Ferrier-Pagès et al., 2000). Moreover, the effects of enrichment may be associated with different forms of nitrogen, with nitrate found to have greater negative effects on corals than ammonium (Zhao et al., 2021). Further, enrichment not only leads to coral loss but also promotes the growth of coral competitors, such as macroalgae, which can result in coral-algal phase shifts (Lapointe et al., 2004; Lapointe et al., 2005a; Lapointe et al., 2005b). However, the responses of corals or macroalgae to AN and their roles in coral bleaching and coral-algal phase shifts, which are key processes in coral reef ecosystems, are still not well understood.
This study aimed to assess the ecological state of remote coral reefs in the SCS, estimate AN deposition flux into these reefs based on 20 years of observations, and conduct laboratory experiments to investigate how corals and macroalgae respond to different AN deposition scenarios, including variations in AN levels and balanced or imbalanced N and P supplies.
2 Materials and methods
2.1 Study area
The study area (15°07′-17°10′N, 111°-117°50′E) was situated in the mid-northern SCS. The ecological survey was conducted between May and July, 2015, covering nine islands. These islands encompass four within the Yongle Atoll (Panshiyu, Huaguang, Yuzhuo, and Beijiao), four within the Xuande Atoll in the Xisha Islands (Dongdao, Yongxing, Qilianyu, and Langhua), and one in the Zhongsha Islands (Huangyan; Figure 1A). For brevity, the islands are abbreviated as PS, HG, YZ, BJ, DD, YX, QL, LH, and HY, respectively (Figure 1B). Satellite images of the sampling sites were derived from the Geospatial Data Cloud of the Chinese Academy of Sciences in 2015 (http://www.gscloud.cn). The average annual sea surface temperature (SST) in this region is 27.4°C, with variations from 24.7°C (December-February) to 30.0°C (May-September).
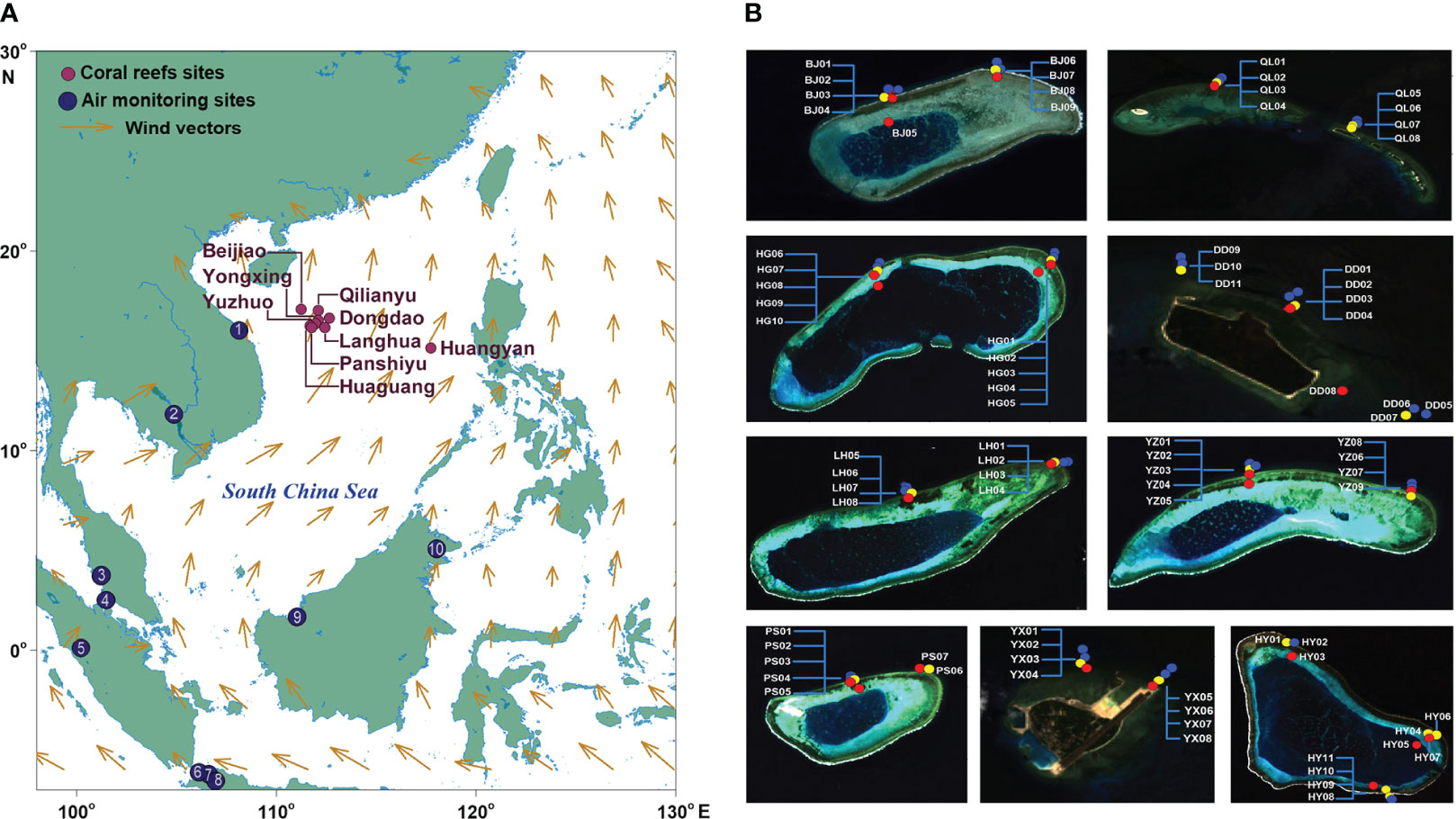
Figure 1 Map of the study area. (A) The study coral reefs in nine islands and monitoring stations of atmospheric N. The numbers 1-10 denote the stations, i.e., 1-Da Nang, 2-PhnomPenh, 3-Tanah Rata, 4-Petaling Jaya, 5-Kototabang, 6-Serpong, 7-Jakarta, 8-Bandung, 9-Kuching, and 10-Danum Valley. (B) Sampling reef sites. The dots in blue, yellow, and red indicate the sites in water depths of 1-4 m, 5-8 m, and 10-15 m, respectively.
2.2 Ecological survey on coral reefs
This survey was conducted at 81 reef sites, spanning four habitats (i.e., lower reef slope, upper reef slope, reef flat, and lagoon) and encompassing water depths of 1-4 m, 5-8 m, and 10-15 m (referred to as shallow, middle, and deep layers; Figure 1B). The survey utilized the line intercept method recommended by the Australian Institute of Marine Science and the Global Coral Reef Monitoring Network. For each station, data on water depth and GPS coordinates were recorded. Scuba divers then deployed a 50 m fiberglass skin gauge along the isobath and employed an Olympus TG‐3 Zoom digital waterproof camera, capturing continuous vertical videos.
Information on benthic categories was extracted from the videos by referencing literature sources, including Corals of the World (Veron, 2000) and the Coral Reef Atlas of Xisha Islands (Huang, 2018). Live coral cover and macroalgae cover were measured by calculating the fraction of the line’s length intercepted by each category, using the formula , where X is the length of live coral or macroalgae, and L is the length of the transect. The ratio of the macroalgae cover to coral cover at each survey site was recorded as the algae-coral ratio. Statistical analyses, one-way ANOVA, and descriptive statistics were performed using SPSS Statistics 22 software for live coral cover, macroalgae cover, and algae-coral ratio. The coral composition data were sourced from Liao (2021) and are listed in Supplementary Table S1.
Following previous conventions (McManus and Polsenberg, 2004; Bruno et al., 2009), we defined macroalgae as the “larger algal forms with canopy heights usually exceeding 10 mm, characterized by greater rigidity and anatomical complexity.” We focused on erect calcareous or fleshy frondose species but did not include microalgae, filamentous algae, or crustose algae.
2.3 Estimation of AN deposition flux
The N deposition data were derived from the EANET (https://monitoring.eanet.asia/document/public/index). EANET has been recording the monthly concentrations of NH4+ and NO3- in rainwater at 31 monitoring stations in East Asia since 2000. Wet deposition samples were collected using wet-only samplers and then stored at 4°C. Ion chromatography was the primary analytical method employed for NH4+ and NO3-, with spectrophotometry as an alternative method.
This study analyzed N deposition data from 10 monitoring stations, i.e., Jakarta, Serpong, Kototabang, Bandung, Petaling Jaya, Tanah Rata, Danum Valley, Kuching, Da Nang, and Phnom Penh. These stations are situated upwind of the study region during the summer monsoon season (April-September), and the AN could be transported by southwest monsoon winds into the study region. The analysis of monsoon winds was based on monthly climatological wind data spanning from 1960 to 2015 (https://www.esrl.noaa.gov).
Temporal variations in AN were analyzed using SPSS Statistics 22 and Origin 2021b software. To calculate the AN deposition flux in SCS, we multiplied the NH4+ and NO3- concentrations in the wet deposition by the rainfall recorded at each monitoring station. It was assumed that there was a 30% loss of AN during transport from the stations to the study region, based on previous literature (Kim et al., 2014).
2.4 Experiment of nitrogen deposition on coral reefs
Five experimental scenarios were designed as below:
(1) The control treatment: no nitrogen input, with the temperature increasing from 26°C to 32°C (1°C per day) and then maintained at 32°C. This treatment aimed to investigate the response of coral reefs to thermal stress in offshore waters without AN deposition. The concentration of DIN and NO3- in seawater was 7.15 µM and 7.03 µM, respectively, the concentration of NH4+ ranged from undetectable to 1.42 µM, and PO43- ranged from undetectable to 1.70 µM. The N/P ratio was 6.7. The highest temperature (32°C) represents the thermal bleaching threshold of corals in the study area, exceeding the mean temperature of the hottest month by 1-2°C.
(2) Ambient N and ambient P treatment (ANAP): this treatment was designed to investigate the response of coral reefs to thermal stress in the open ocean, with a medium level of AN deposition. P supply was sufficient due to better water exchange, maintaining an optimal N/P ratio of 16. DIN in rainwater ranged from 0~8000 µM in this region (EANET dataset), and for this treatment, the medium level of AN was set at 1500 µM. Rainwater in SCS reaches 600 mm per month (Chen et al., 2005), and rainfall was taken as 30 mm per day. It was assumed that AN was carried by rainwater and deposited into coral reefs at a depth of 7 m, with AN loading evenly mixed throughout the water column. Then DIN in seawater increased by 7 µM (2.5 µM NO3- and 4.5 µM NH4+), and PO43- was 0.45 µM. The temperature conditions were the same as those in the control treatment.
(3) High N and high P treatment (HNHP): this treatment aimed to examine the response of coral reefs to thermal stress in the open ocean, under a high level of AN deposition (8000 µM in rainwater). This concentration was derived from the extreme DIN concentration observed in rainwater by ENAET (7890 µM DIN in Kototabang in May, 2015). The treatment retained the same parameters as the ANAP treatment, including rainfall, water depth, N/P ratio, and temperature. But in this scenario, DIN in seawater increased by 30 µM (13 µM NO3- and 17 µM NH4+), with PO43- reaching 1.9 µM.
(4) Ambient N and low P treatment (ANLP): this treatment aimed to investigate coral responses to thermal stress in semi-closed areas such as lagoons, where there is a medium level of AN deposition and an imbalance of N and P supply. The rainfall, water depth, DIN in seawater, and temperature were the same as that in ANAP treatment. However, P supply was limited due to weak water exchange. The PO43- concentration was set as 0.19 µM and the ratio of N/P was 36.
(5) Ambient N and high P treatment (ANHP): this treatment aimed to investigate the coral responses to thermal stress in the relatively closed area. It involved a medium level of AN deposition and an excessive P input (e.g., from seabird droppings or domestic sewage). The PO43- concentration was set at 0.9 µM, and the N/P ratio was 8. Other environmental parameters, including rainfall, water depth, DIN in seawater, and temperature were the same as that in ANAP treatment.
Live corals were collected from the study area and then cultured in the laboratory. The coral species included Porites lutea, Favites pentagona, Acropora crateriformis, Acropora austera, Acropora divaricata, Montipora caliculata, Lithophyllon undulatum, and Oulophyllia bennettae. The first three species were relatively abundant, while the others were rare in the coral community (Liao, 2021; Supplementary Table S1), so they were selected to explore AN impact on corals, as well as the community. They may also reflect the responses of corals in various morphologies, i.e., branching Acropora, foliose Montipora and Lithophyllon, and massive Porites, Favites and Oulophyllia. After 15-day cultivation, the corals were divided into fragments (5 × 5 × 5 cm3), with three coral fragments for each treatment. Then they were transferred to experimental tanks for pre-cultivation for 14 days. Artificial seawater was used in both control and treatment groups, and the artificial seawater was also filled with live rock (mainly calcium carbonate rock formed by the skeletons of dead corals) collected from the SCS to artificially simulate coral reef ecosystems.
The pulse-amplitude modulated fluorometer (Monitoring-PAM, WALZ Germany) was used to measure the photochemical quantum yield of photosystem II (PSII) of coral-algal symbionts at 20:00-21:00 (after-dark treatment for 30 min), and the value was recorded as maximum photosynthetic efficiency (Fv/Fm). Photos of corals in each treatment and macroalgae in three treatments (Control, ANAP, and HNHP) were taken to trace their ecological status. The Symbiodiniaceae (zooxanthellae) were separated from the symbiont by a washer with the high-pressure washing of artificial seawater, and the zooxanthellae density was measured according to the concentration of zooxanthellae and the surface area of the coral skeleton (Li et al., 2011).
The high-purity ammonium chloride (NH4Cl), sodium nitrate (NaNO3), and potassium dihydrogen phosphate (KH2PO4) solids were used to prepare the nutrient solution. The concentrations of NH4+, NO3-, and PO43- in the seawater of each treatment were monitored daily, following the seawater analysis standard of China (GB, 17378.4-2007). These analyses were conducted using Indophenol blue Spectrophotometry (NH4+), Reduction of the cadmium column-Spectrophotometry of N-(1-naphthyl) ethylene diamine hydrochloride (NO3-), and Phosphomolybdenum blue spectrophotometry (PO43-). All nutrient analyses were conducted using an ultraviolet spectrophotometer (Shimadzu, UV-2600i). The minimum detection limits (MDLs) were 0.082 µM NH4+, 0.032 µM NO3-, and 0.2 µM PO43-.
3 Results
3.1 Ecological status of coral reefs
3.1.1 Live coral cover
Horizontally, the Xuande Atoll exhibited the highest average coral cover at 25.2% (± 2.8%), while Yongle Atoll had the lowest at 8.8% ( ± 1.7%). Vertically, the shallow layer (1-4 m) had the lowest average coral cover (13.9%) in contrast to the middle layer (5-8 m) at 17.4% and the deep layer (10-15 m) at 17.5% (Figure 2A). No statistically significant difference was found among the average coral cover values of these three layers (p = 0.852). In 50% of the sampling sections (n = 20), coral cover in the shallow and/or middle layer exceeded that in the deep layer (Figure 3). Coral cover in the deep layer surpassed that in the shallower layer in 20% of sections. In the remaining sections, the coral cover was similar among the three layers. In the deep layer, 44.1% of the sites had coral coverage less than 10%, which was higher than the 36.4% observed in the middle layer. Additionally, 40.9% of sites in the middle layer had coral cover exceeding 20%, surpassing the proportions in the shallow layer (28%) and deep layer (32.4%). This survey revealed a reduced coral cover level in certain parts of this remote region, with no consistent advantage in coral cover for the deeper layers.
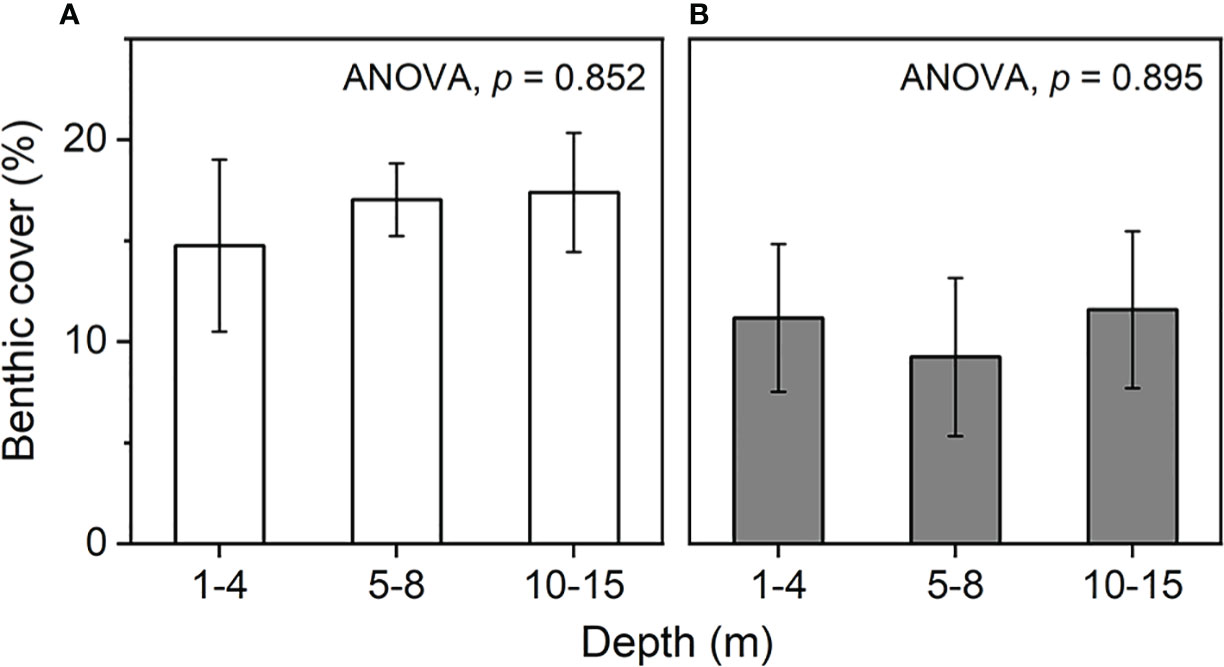
Figure 2 Benthic composition of the South China Sea reefs under various water depths. (A) live coral cover and (B) macroalgae cover (mean ± SE).
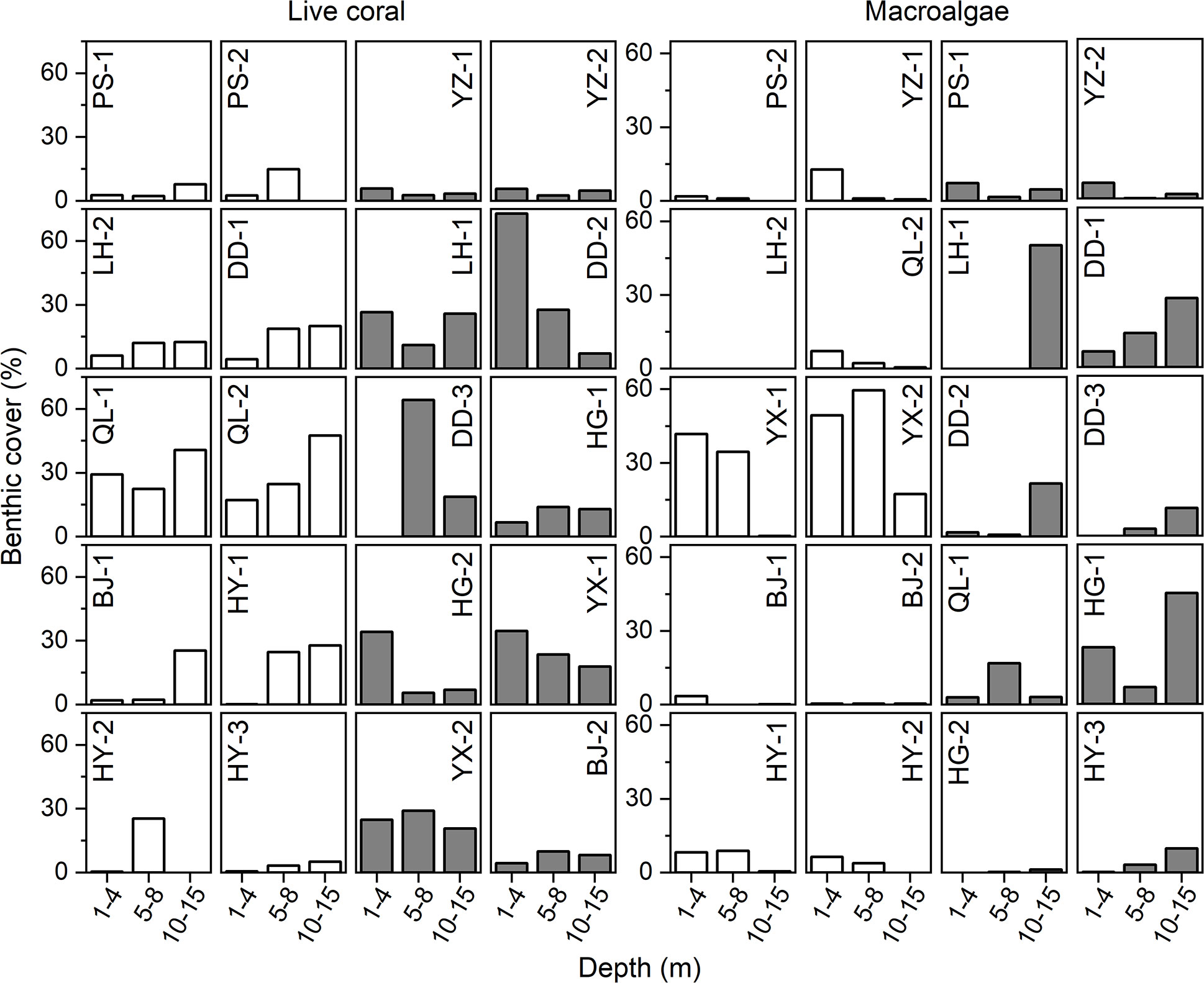
Figure 3 Benthic composition of reef sites under various water depths. Gray histogram bars denote less coral/more macroalgae in deeper waters than in shallower waters; the reverse is shown by the blank bars.
3.1.2 Macroalgae cover
Horizontally, the highest average macroalgae cover (14.5%) was observed in Xuande Atoll, and the lowest (4.9%) was recorded in Huangyan. Across the shallow, middle, and deep layers, the average macroalgae cover was 10.7% (0-49.6%), 7.5% (0-59.5%), and 11.3% (0-57.1%), respectively (Figure 2B). No statistically significant difference was found in the average macroalgae cover among these three layers (p = 0.895). However, at 40% of the sections, the macroalgae cover in the deep layer exceeded that in the shallower layers, while at only 25% of sites, the cover was lower than in the shallower layers (Figure 3). In the deep layer, 26.5% of the sites exhibited macroalgae cover exceeding 10%, which was higher than the proportion observed in the middle layer (18.2%). This survey suggested that macroalgae present a substantial challenge to corals in this remote region, including the relatively deep layers.
3.1.3 Algae-coral ratio
The algae-coral ratio in all the reef sites averaged 0.62 (SE = 2.3%). The average algae-coral ratios in the Yongle Atoll, Xuande Atoll, and Zhongsha Islands were 0.83, 0.58, and 0.40, respectively. In the shallow layer, the ratio averaged 0.8 (0-136), and 44% of the reef sites had a ratio greater than 1, indicating a coral-algae phase shift. The middle layer hosted the lowest algae-coral ratio (0.4), with values ranging from 0 to 2.1. However, 13.6% of the reef sites had a ratio greater than 1. In the deep layer, the algae-coral ratio averaged 0.7 (0-105.7), and 26.5% of reef sites had a ratio greater than 1. The results suggested that corals have a limited advantage over macroalgae in this remote coral reef region.
3.2 AN deposition flux
The monthly concentration of NH4+ in each station ranged from 0 to 7890 µM, and NO3- ranged from 0 to 277.27 µM during the summer monsoon period (April-September; Figures 4A, B). The monthly average concentration of DIN ranged from 256.47 to 2705.11 µM during the summer monsoon season, with NH4+ and NO3- ranging from 119.04 to 2484.29 µM and 89.29 to 251.56 µM, respectively. The monthly DIN concentration increased from 218.48 µM in, 2000 to 436.81 µM in, 2008, peaking at 1568.90 µM in 2015. NH4+ and DIN had similar trends, while NO3- showed no significant trend (Figure 4D). Throughout the year, high levels of NH4+ and NO3- (646.51 and 821.70 µM) were found in April and May. Additionally, March-October constituted the higher value period (418.86-502.24 µM), while November-February constituted the lower value period (237.99-352.30 µM; Figure 4C). During the summer monsoon season, NH4+ accounted for 42-92% of the DIN, and NO3- accounted for 8-58%. Assuming an average annual rainfall of 1500 mm for the Xisha Islands, the deposition of DIN was 15.8 mmol N·m-2·month-2 during the summer monsoon season. If 30% is lost in transit, the deposition of DIN would be 11 mmol N·m-2·month-2.
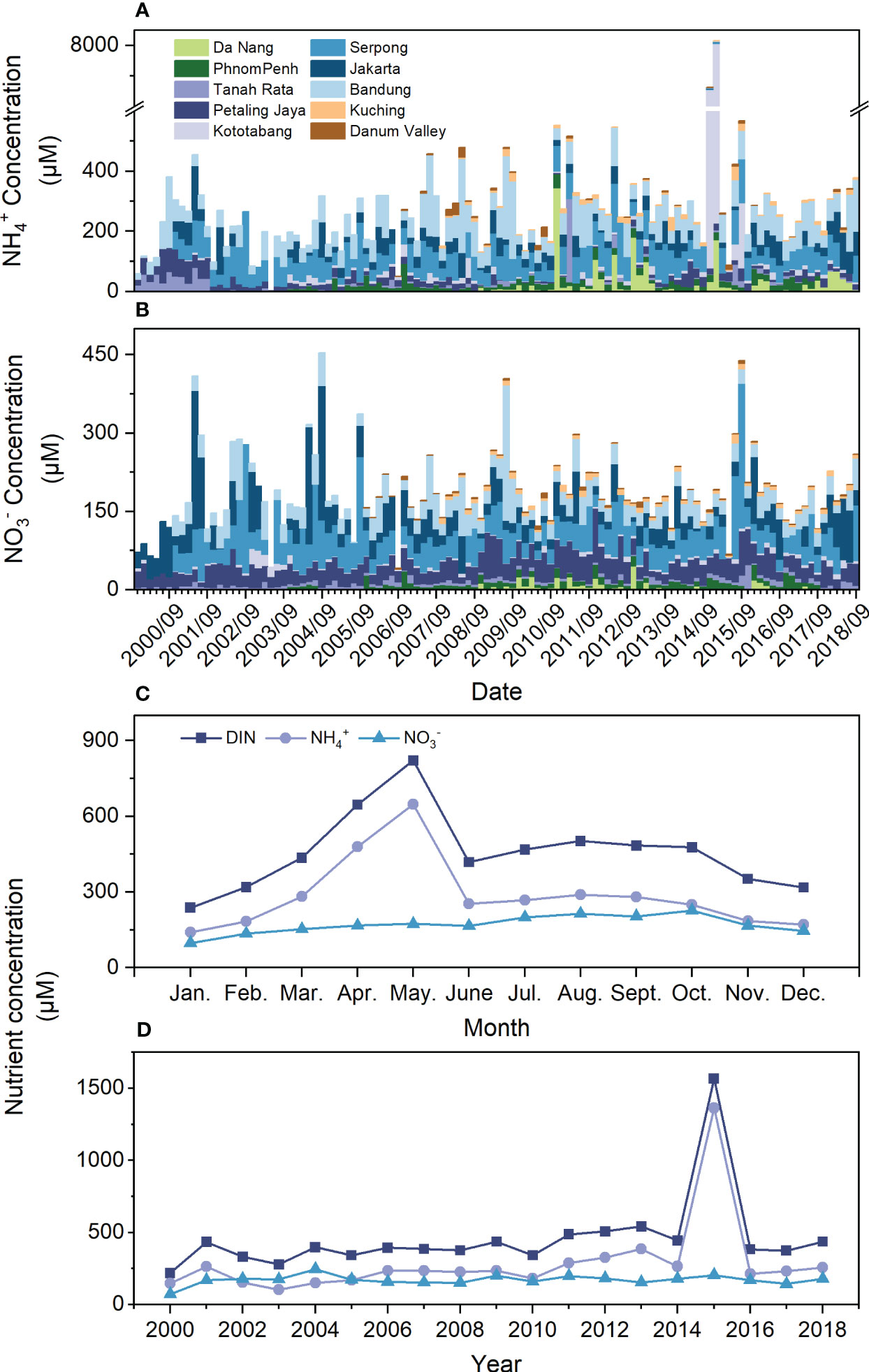
Figure 4 Atmospheric N deposition onto the South China Sea reefs. Monthly (A) NH4+ concentration and (B) NO3- concentration in rainwater at sites upwind of the SCS Reefs in the summer monsoon season (April-September) from 2000 to 2018 (C) seasonal and (D) annual variation of monthly concentration of DIN, NH4+, and NO3-.
3.3 Response of coral reefs to AN deposition
3.3.1 Accumulated nutrients in seawater
In the control treatment, the average concentration of DIN and NO3- in seawater was 7.15 and 7.03 µM, respectively, while the levels of NH4+ ranged from undetectable to 1.42 µM, and PO43- ranged from undetectable to 1.70 µM (Figure 5A). The N/P ratio was calculated as 6.7 based on the available data. In the ANAP treatment, DIN showed fluctuations, ranging from 8.09 to 14.80 µM and peaking at 36.24 µM on day 11 (Figure 5B). NO3- concentrations ranged from 2.56 to 25.63 µM (days 1-17), decreased to 2.24 µM (days 18-33), and then increased to 17.24 µM (days 34-43). However, NH4+ concentration remained relatively low, fluctuating between undetectable values and 10.62 µM, while PO43- ranged from undetectable to 3.12 µM, resulting in an N/P ratio of 14.6. The results suggested that the loading of NH4+ and PO43- was rapidly consumed by algae, while excessive NO3- accumulated in seawater, leading to an increase in the N/P ratio. To explore the potential dynamics of the N/P ratio, multiple imputation methods were employed to replace the missing values (below the MDLs). By integrating the detected and imputed data, the N/P ratio was estimated to be 44 for the control group and 62 for the ANAP group (Supplementary Table S2).
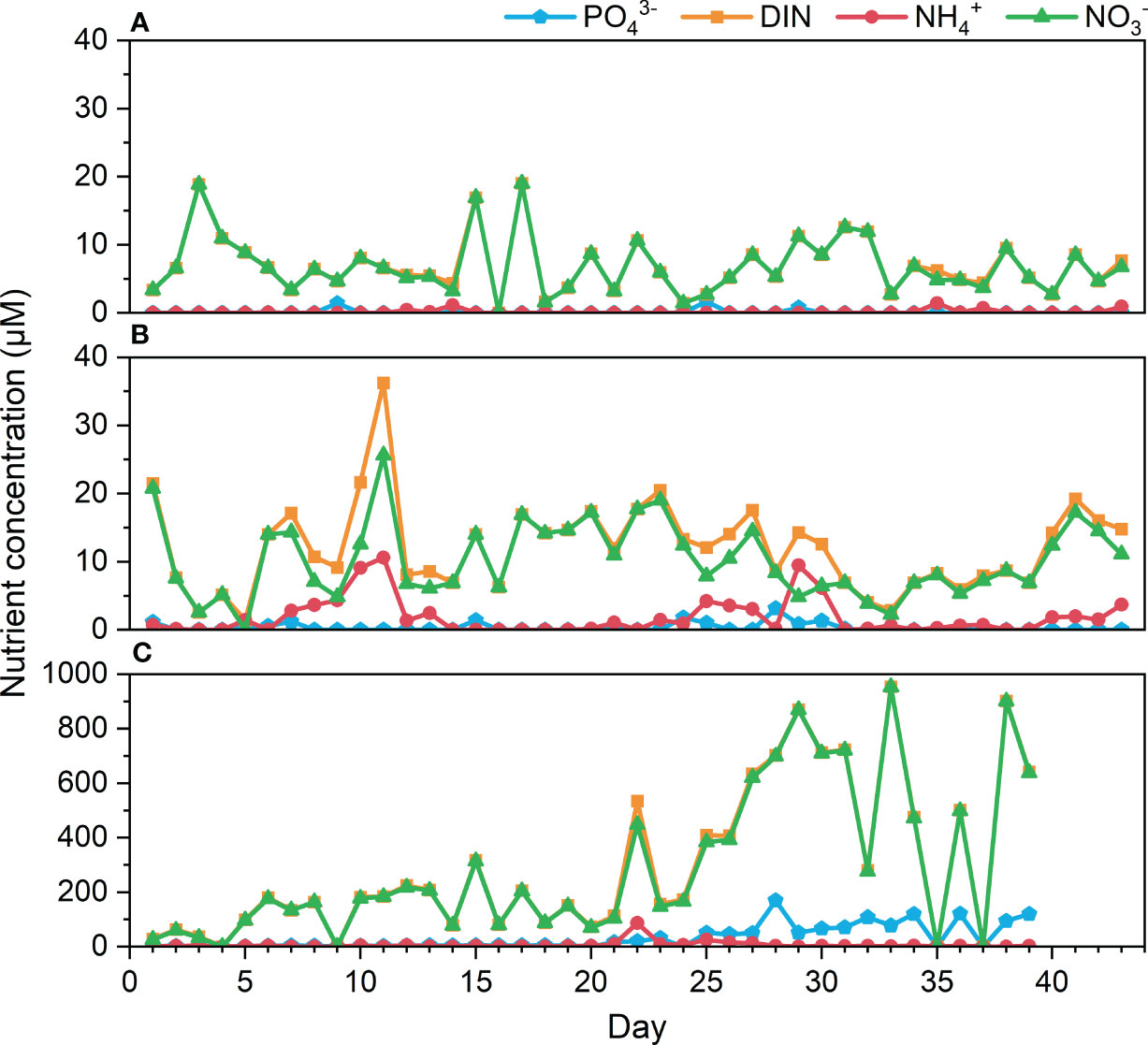
Figure 5 Variations of nutrient concentration in seawater during the experiment. (A) control, (B) ANAP, and (C) HNHP treatment.
In the HNHP treatment, DIN and NO3- rapidly exceeded 100 µM (days 6-20), then increased from 113.64 to 954.23 µM, reaching 641.91 µM by the end (Figure 5C). NH4+ fluctuated slightly from 0.15 to 10 µM, with only two peaks on day 22 (87.16 µM) and day 25 (25.14 µM). PO43- remained at relatively low levels, ranging from 1.62 to 5.64 µM (days 0-20), before experiencing a sharp increase to 169.36 µM on day 21. The N/P ratio in this treatment was 24.5. These results suggested that the loading of DIN and PO43- exceeded the absorption capacity of algae, leading to the accumulation of excessive nutrients at extremely high levels in seawater, which shifted the system from N-limited conditions to P limitation.
3.3.2 Rapidly growing macroalgae
The cover of macroalgae (Caulerpa serrulata, Caulerpa racemosa, Caulerpa racemosa var. turbinata, and Caulerpa sertularioides) increased by 155% in the ANAP treatment (Figures 6A, B). The cover increased from 18.3% to 40% (days 0-14) and peaked at 65% on day 42. In the HNHP treatment, the cover of macroalgae increased by 123%. The cover rose from 21.7% to 68.3% (days 0-14) and remained at 70% (days 15-42). In contrast, macroalgae cover in the control treatment ranged from 0 to 2%. The results indicated that macroalgae can be greatly promoted by AN within a short time frame (2 weeks), suggesting that once nutrient absorption by algae rapidly reaches saturation, excessive loading of AN may stress corals.
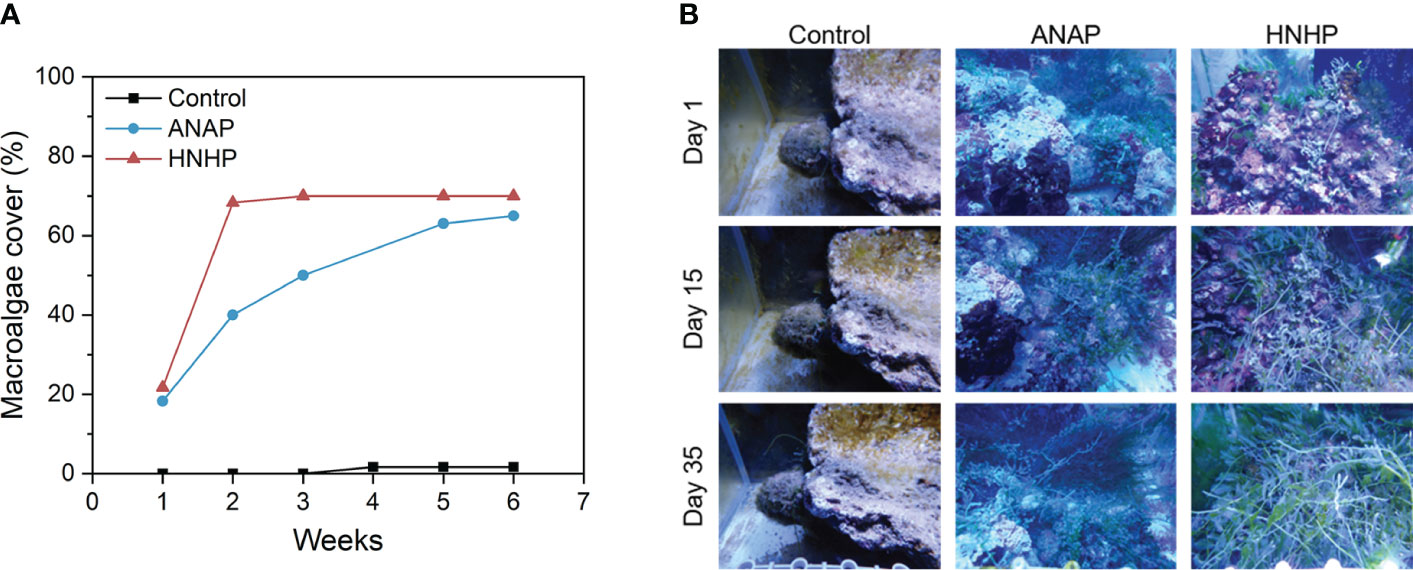
Figure 6 Response of macroalgae to different nutrient supplies. (A) The changes in macroalgae cover over time; (B) macroalgae pattern.
3.3.3 Responses of coral-algal symbionts
(1) Photosynthetic efficiency and survival rate
In the control treatment, when compared to the ANAP and HNHP treatments, most corals declined, and 75% of them died under thermal stress without AN loading (Figure 7). The N/P ratio in this treatment was 6.7 in seawater. Among the A. austera corals, the Fv/Fm values remained above 0.5, but they bleached and died on days 18-26 (Figure 8A). The Fv/Fm values of P. lutea dropped below 0.5 on day 6, and they bleached and died on days 23-25 (Figure 8C). For F. pentagona, the Fv/Fm values decreased below 0.5 on day 38 (Figure 8B), and for L. undulatum, they remained below 0.5 for most of the experiment period (Figure 8D), but no visible bleaching was observed on them during the experiment (Figure 9). Additionally, in the control treatment, when compared to the ANLP and ANHP treatments, all the corals of O. bennettae, A. crateriformis, A. divaricata, and M. caliculata remained in a healthy state, with Fv/Fm values above 0.5 (Figure 10). There were no visible signs of coral bleaching or death observed in the experiment (Figure 9).
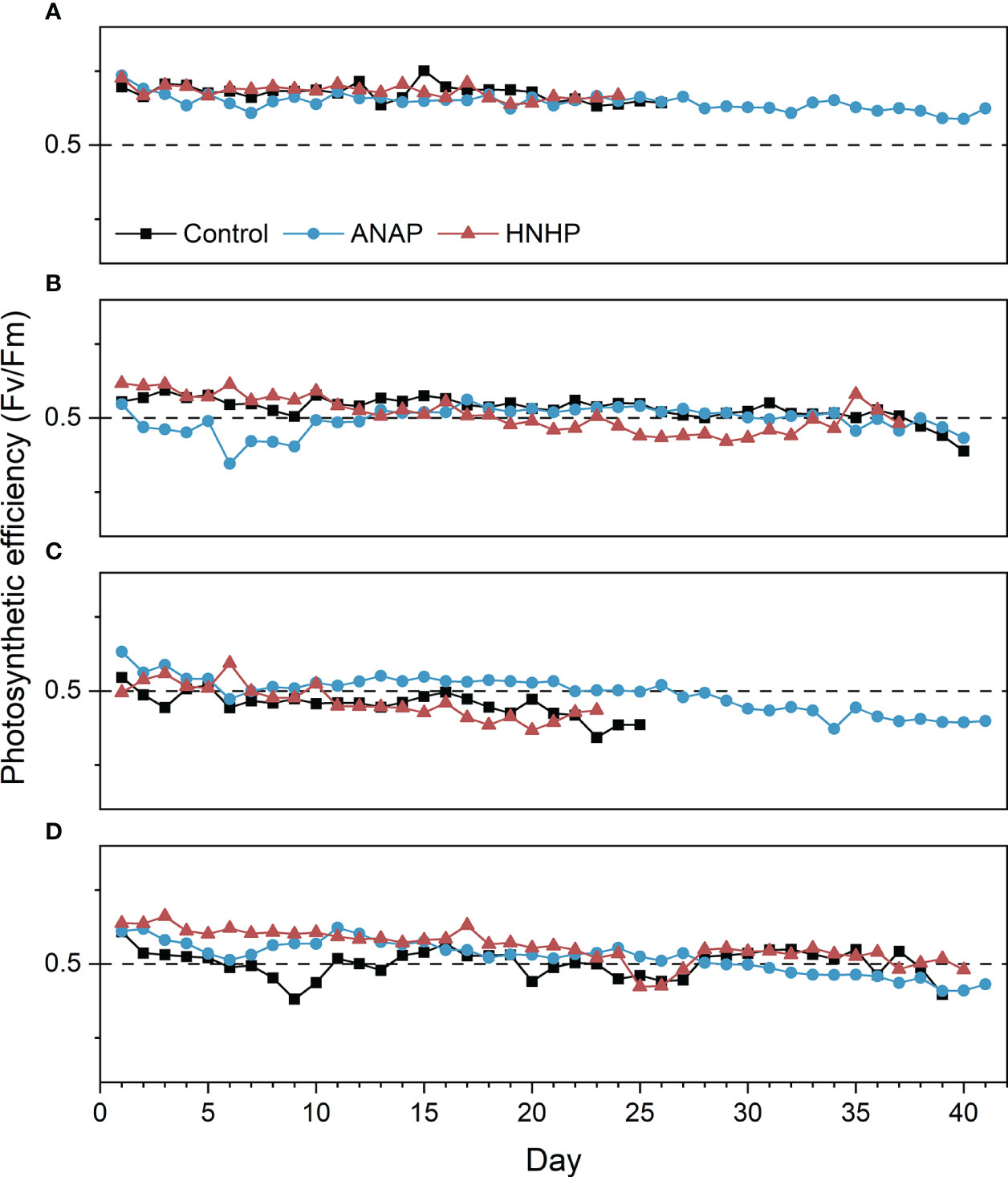
Figure 8 Photosynthetic efficiency of coral colonies exposed to different concentrations of balanced nutrient supplies. (A) A. austera, (B) F. pentagona, (C) P. lutea, (D) L. undulatum. The horizontal dashed line indicates the threshold above which Fv/Fm values are considered to be in a healthy range.
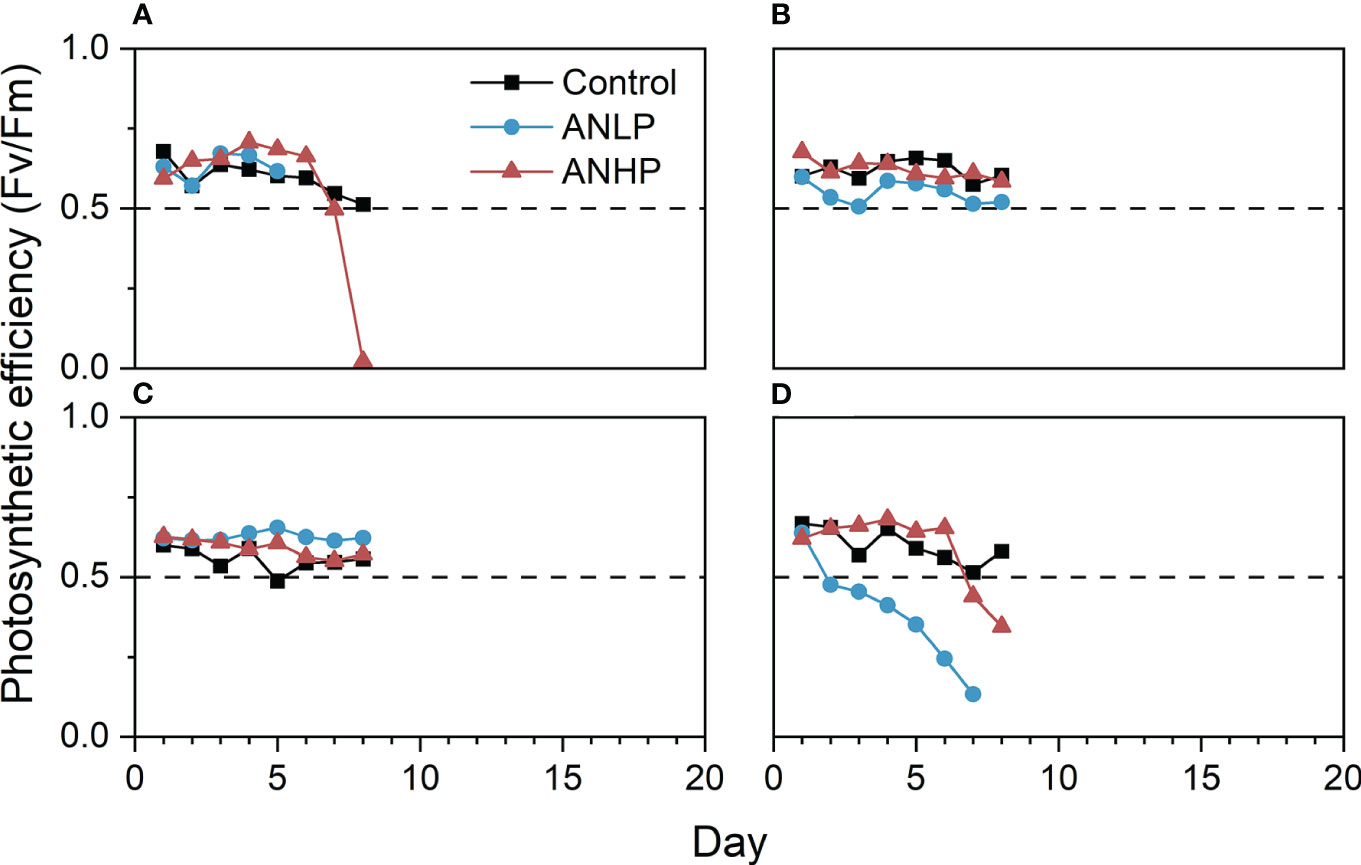
Figure 10 Photosynthetic efficiency of coral colonies exposed to different concentrations of imbalanced nutrient supplies. (A) A. divaricata, (B) A. crateriformis, (C) O. bennettae, (D) M. caliculata. The horizontal dashed line is the same as Figure 8.
In the ANAP treatment, the Fv/Fm values of A. austera remained above 0.5 for 41 days (Figure 8A), and those of F. pentagona decreased below 0.5 on day 35 (Figure 8B), which was similar to what was observed in the control. The Fv/Fm values of P. lutea dropped below 0.5 on day 27 (Figure 8C), and L. undulatum on day 29 (Figure 8D). This is an improvement compared to the control, where Fv/Fm values decreased below 0.5 on day 6 for P. lutea and day 1 for L. undulatum. None of the corals exhibited bleaching, and they survived for 41 days (Figure 9). The survival rate of the corals was significantly higher than that of the control treatment (Figure 7).
In the HNHP treatment, the corals, with the exception of L. undulatum, experienced more severe declines compared to the control. The Fv/Fm values of A. austera remained above 0.5, but they underwent bleaching and died between days 16 and 24 (Figure 8A). The Fv/Fm values of P. lutea and F. pentagona dropped below 0.5 on day 11 and day 19, respectively, resulting in bleaching and mortality between days 21 and 26 and between days 20 and 39 (Figures 8B, C). The survival period was shorter than that observed in the control. However, the Fv/Fm of L. undulatum remained above 0.5 and no visible bleaching was observed in them during the experiment (Figures 8D, 9).
In the ANLP treatment, the Fv/Fm values of A. crateriformis and O. bennettae remained above 0.5 (Figures 10B, C). The Fv/Fm values of A. divaricata stayed above 0.5, while that of M. caliculata decreased below 0.5 on day 2 (28°C; Figures 10A, D). However, the former began to bleach and die on days 4-6 and the latter on days 2-5 (Figures 7, 9). In the ANHP treatment, the Fv/Fm values and survival rates of A. crateriformis and O. bennettae showed similar trends to those in the ANLP treatment (Figures 10B, C). However, the Fv/Fm values of A. divaricata and M. caliculata dropped below 0.5 on day 7 (Figures 10A, D), and the former bleached and died on day 8, while the latter on days 5-8 (Figures 7, 9).
(2) Density of Symbiotic Zooxanthellae
The density of zooxanthellae was analyzed in A. austera in ANAP and HNHP treatments, as well as A. crateriformis and O. bennettae in ANLP and ANHP treatments as these corals had remaining tissue at the end of the experiment (Figure 11). At the beginning of the experiment, the zooxanthellae density for the three corals were 1.03×106, 1.03×106, and 5.33×106 cells/cm-2, respectively.
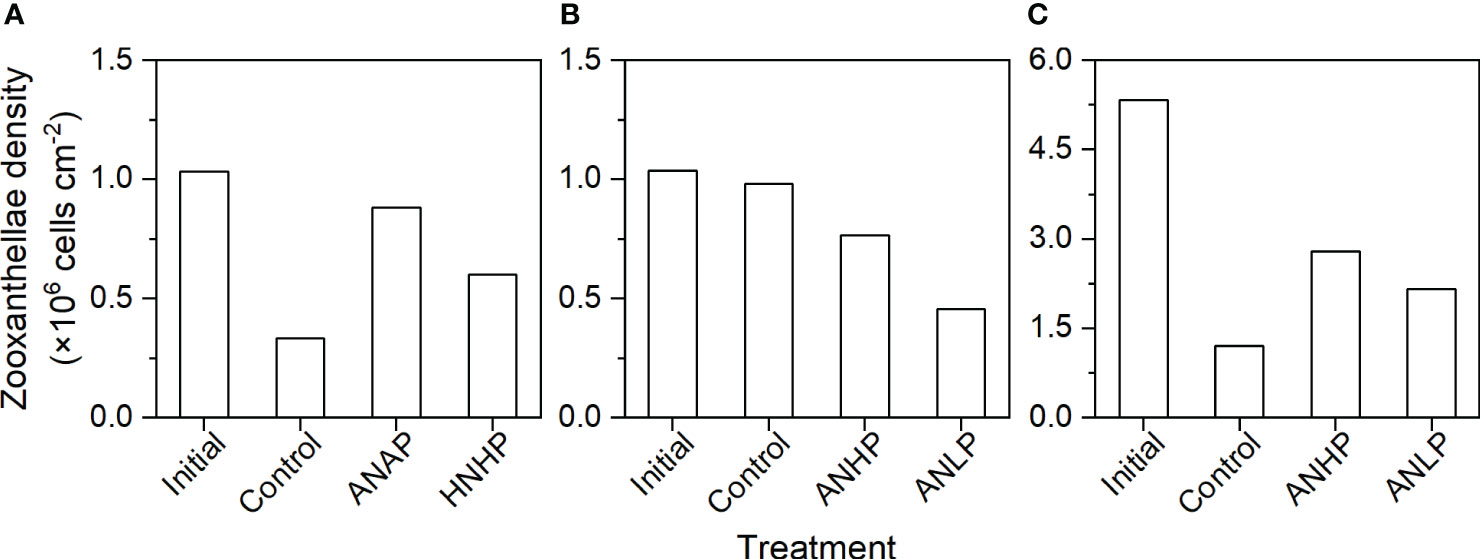
Figure 11 Response of zooxanthellae density of coral colonies to different nutrient supplies. (A) A. austera, (B) A. crateriformis, and (C) O. bennettae.
By the end of the control treatment, the zooxanthellae density for A. austera, A. crateriformis, and O. bennettae decreased to 0.33×106, 0.98×106, and 1.21×106 cells/cm-2, respectively. The heating treatment, without nutrient loading, led to a loss of 5.0~77.0% of the initial zooxanthellae.
The zooxanthellae density of A. austera was 0.88×106 cells/cm-2 in ANAP and 0.60×106 cells cm-2 in HNHP by the end of the experiment. These densities represented a reduction of 15.0% and 42.0% from the initial levels, respectively (Figure 11A). However, the density in ANAP was 2.5 times higher than that in the control. The results suggested that AN deposition may promote the growth of zooxanthellae, potentially alleviating the breakdown of symbiosis between corals and their algal symbionts under thermal stress and thereby mitigating coral bleaching.
The zooxanthellae density of O. bennettae decreased to 2.79×106 cells/cm-2 in ANHP by the end (Figure 11C). This represents a decrease of 47.6% from its initial level and an increase of 130.6% compared to the control treatment. Moreover, in ANLP treatment, the density decreased to 2.16×106 cells/cm-2, which is 59.5% lower than the initial level and 78.3% higher than that in the control. On the other hand, A. crateriformis maintained a similar density of zooxanthellae at both the beginning and the end of the control treatment, indicating that this coral species exhibits relatively strong thermotolerance. However, the zooxanthellae density of A. crateriformis decreased to 0.76×106 cells/cm-2 in ANHP and 0.46×106 cells cm-2 in ANLP, respectively, which is 26.2% and 55.8% lower than the initial level and 22.1% and 53.4% lower than that in the control (Figure 11B). These results, as supplementary evidence, indicate that an imbalanced supply of N and P exacerbates the breakdown of the coral-algal symbionts, with P restriction playing a more significant role in contributing to coral bleaching.
4 Discussion
4.1 Relationship between coral thermotolerance and balanced nutrient supply
The moderate and balanced supply of nutrients (DIN, 7 µM; N/P, 16) enables the survival of the majority (93.8%) of the sampled coral colonies in the ANAP treatment under thermal stress (32°C). The survival period (41 days) of these corals was longer than that of the control (heating without nutrient loading), which began bleaching on day 16. While many studies have indicated that enrichment can inhabit coral growth as they usually favor oligotrophic waters (Lei et al., 2009), an increasing number of studies have found that moderate levels of nutrients have positive effects on corals. Particularly, enrichment may enhance coral thermotolerance when they are exposed to thermal stress. For instance, after a 23-day period of 31.2°C, the survival rate of Acropora millepora in a moderate nutrient environment (3.63 µM NO3-) reached approximately 80%, which is double the survival rate in a low-nutrient environment (NO3-, 2.50 µM; Fabricius et al., 2013). No visible sign of bleaching was observed for Pocillopora damicornis in the treatment of 2.1 µM DIN and 0.78 µM PO43- during 28-day heating, but they bleached without nutrient adding (Mcclanahan et al., 2003). In the control treatment, the N/P ratio (6.7, calculated on detected data) closely resembled that of many coral reef regions, including the central SCS, Great Barrier Reef, and Bonaire islands, which experienced N limitation, with N/P ratios ranging from 4.3 to 12.3 (Ke et al., 2018). This nutrient imbalance makes corals more susceptible to heat-induced bleaching. However, the moderate N loading (7 µM DIN), equivalent to a moderate level of AN deposition in the SCS, may help alleviate seawater nutrient limitation, thereby mitigating coral bleaching under thermal stress.
The improved coral thermotolerance may be attributed to the enhanced photosynthetic efficiency of coral-algal symbionts due to nutrient supply. With nutrient supplementation, the loss of zooxanthellae from the corals (A. austera) under thermal stress was significantly reduced (~ 10%), whereas in the control, the loss was much more severe (~ 68%). Sustained photosynthesis by coral-algal symbionts ensured an energy supply for corals to withstand thermal stress. As a result, A. austera corals survived for an additional 21 days compared to those in the control, and the Fv/Fm values of the former remained at approximately 0.5 on day 27, indicating a relatively healthy status (Wiedenmann et al., 2013). Similar results have been reported in other laboratory studies. With the addition of 3 µM NO3- and 1 µM P, the net photosynthesis of Pocillopora damicornis increased almost two-fold at 25°C (Courtial et al., 2018). Chlorophyll a and total protein content in Stylophora pistillata increased in the presence of 4 µM NH4+ after 6 weeks (Bednarz et al., 2020). Additionally, pigment concentrations decreased by 60% under 30°C without nutrient adding but only decreased by 25% with NH4+ supply (Beraud et al., 2013). Therefore, it is reasonable to conclude that moderate AN supply may mitigate the susceptibility of corals to heat bleaching.
Despite a balanced nutrient input, seawater N/P ratios may fluctuate due to the dynamic uptake by corals (Steven and Atkinson, 2003). As shown in Supplementary Table S2, in the ANAP treatment, NH4+ and PO43- were depleted (undetectable) for 74% and 33% of the experiment period, respectively. It seemed that the nutrients had been consumed, and the corals may have experienced temporary spikes in N/P ratios (imputed as an average of 62). Nevertheless, sustained nutrient input was restored to a relatively balanced condition, e.g., N/P = 17 on day 29. The supplemented nutrients may contribute to sustaining symbiont density and photosynthesis, thereby enhancing coral thermal tolerance (Ezzat et al., 2016). In contrast, the control group had an imputed N/P ratio of 44. The lack of nutrient input extended the periods of nutrient depletion, with proportions reaching 92% (PO43-) and 80% (NH4+). The prolonged nutrient deficiency may reduce coral resilience to heat stress (Rosset et al., 2017).
By contrast, the HNHP treatment showed that a high level of N input weakened coral thermotolerance, even with a balanced supply of nutrients (DIN, 30 µM; N/P, 16; 32°C). Three out of four coral species in the HNHP exhibited shorter survival times than those without nutrient loading. For instance, the survival time of A. austera (16-24 days) was 2 days shorter than in the control. Negative effects of nutrient enrichment on corals have been documented, including inhibition of coral growth and increase in coral thermal susceptibility. The growth rate of Acropora cervicornis decreased by 61% after 1 month with the supply of N and P (NO3-, 10 µM; PO43-, 4 µM; N/P, 2.5; Renegar and Riegl, 2005). Recent studies have shown that coral bleaching was minimal when nutrient levels were high but balanced between N and P. This is true for a relatively modest nutrient enrichment (6.5 µM N, 0.3 µM P; Wiedenmann et al., 2013). However, the treatment in this study, reflecting the response of corals to a wet deposition of 8000 µM DIN into the SCS, suggests the negative effect of high concentrations of nutrients on corals.
The accelerated coral bleaching may be attributed to the loss of zooxanthellae and the reduced energy supply available to corals to withstand thermal stress. The zooxanthellae density of A. austera in the HAHP was only two-thirds of that in the ANAP. It has been reported that high concentrations of nutrients stimulate the division rate of intracellular symbionts, affecting the physiological interaction between corals and zooxanthellae by accelerating the cell division rates of the intracellular symbionts (Szmant, 2002). However, excessive enrichment or an imbalanced N/P ratio can hinder zooxanthellae growth. Enrichment of NO3- (> 20 µM) led to a 20% loss of zooxanthellae density and a 30% decrease in chlorophyll concentration (Lei et al., 2009). Moreover, the continued nutrient loading led to nutrient accumulation in seawater. NH4+ ranged from 0 to 10 µM, while NO3- exceeded 100 µM, peaking at ~ 1 mmol L-1 during the HNHP treatment. This indicates that NH4+ was preferentially absorbed by algae, but NO3- gradually accumulated in the seawater. Compared to NH4+, NO3- enrichment often imposes more stress on coral growth (Fabricius, 2005) and makes corals more sensitive to thermal stress. This is because nitrate needs to be reduced to ammonium by nitrate reductase and nitrite reductase before it can be assimilated by symbiotic algae. The reduction process requires photosynthetic electrons and energy, thus further promoting oxidative stress in coral halobiont under thermal stress (Zhao et al., 2021). Moreover, the N/P ratio increased from 16 to 24.5 during the HNHP treatment. This is likely because the proportion of nutrient uptake by corals is dynamic (Steven and Atkinson, 2003). This N/P ratio (24.5) may represent a mild P limitation, although it exceeds the Redfield ratio, which is considered an ideal reference point for the healthy growth of many marine organisms. However, previous research has indicated that low N and P levels with a similar N/P ratio (N/P = 21.7, 6.5 µM DIN, 0.3 µM P) did not trigger coral bleaching (Wiedenmann et al., 2013). This implies that the primary cause of the severe coral bleaching in the HNHP is likely the high levels of accumulated nutrients in seawater (Figure 5). This study highlights that an extremely high amount of AN, even with a balanced nutrient supply, could increase coral susceptibility to bleaching.
4.2 Imbalance of N/P exacerbated coral bleaching
Our results revealed that corals had a short survival duration (2-8 days) in both ANHP and ANLP treatments. It seemed that an imbalanced nutrient supply posed a greater challenge in meeting the nutrient demand of corals under heat stress compared to a balanced supply. This highlights the potential risks associated with an imbalance of N and P levels in coral ecosystems, which may be the result of continuous AN deposition. In coastal waters off Singapore, for instance, during the haze period, the average wet deposition fluxes of water-soluble NOx- and PO43- were 75.57 ± 7.31 mg·m-2·day-1 and 2.25 ± 1.75 mg·m-2·day-1, respectively (Sundarambal et al., 2010). Conversely, excessive P often comes from seabird emissions or volcanic eruptions (Olgun et al., 2013; Hentati-Sundberg et al., 2020). Guano stock solutions, for instance, have been recorded to contain as much as 1960 µM of P and 4800 µM of N (Shatova et al., 2017). The excessive supply of either N or P can lead to a nutrient imbalance in seawater, especially in regions with weak water exchange.
The corals declined dramatically in response to imbalanced nutrient conditions, primarily due to the breakdown of the symbiotic relationship between the corals and zooxanthellae. In the N-limited treatment (ANHP, N/P = 8), the Fv/Fm values of A. divaricata and M. caliculata on day 7 both dropped below 0.5, constituting a decline of 96.5% and 44.4%, respectively. Additionally, the zooxanthellae density in the N-limited treatment was reduced by 26.2-47.6% compared to the initial levels. In response to P-limited stress, photosynthetic organisms often adapt by substituting phospholipids with sulpholipids. This adaptation allows them to maintain the proper functioning of photosynthetic membranes and the embedded photosystems. The elevated sulpholipids can result in a shift in lipid ratios, presumably altering the normal ionic character of photosynthetic membranes (Wiedenmann et al., 2013). Conversely, under heat stress (30°C), the uptake rates of PO43- by corals can increase compared to ambient conditions (25°C; Ezzat et al., 2016). This increased availability of phosphorus may enhance the deposition of phospholipids in membranes, as well as the biosynthesis and storage of acylated glycerol derivatives and wax esters in vesicles, possibly at the expense of terpene biosynthesis (Fleury et al., 2000). Recent research has highlighted the negative effects of an imbalance of N/P on coral susceptibility (Zhao et al., 2021). The survival times of corals were reduced by over 50% under P-limited conditions (N/P, 43; 33°C). Montipora foliosa lost ~50% of its zooxanthellae, and chlorophyll-a concentration decreased by over 50% after 3 weeks (Wiedenmann et al., 2013). Similarly, the growth rate of Acropora cervicornis decreased by 61% when the N/P ratio was 0.25 after 5 weeks. The decline was even more severe (116%) when corals were subjected to separate N (N/P, 141.86) and P (N/P, 0.18) treatments (Renegar and Riegl, 2005). These independent experiments provide further support for our conclusion that imbalances, both N and P limitations, increase the vulnerability of corals to thermal bleaching.
Our experiments revealed notable interspecific differences in how corals respond to nutrient enrichment and heating. A. austera, A. divaricata, M. caliculata, and F. pentagona exhibited relatively weak tolerance to these stressors, while P. lutea, L. undulatum, A. crateriformis, and O. bennettae had a comparatively stronger tolerance. A review that summarized nutrient thresholds for maintaining healthy coral in laboratory experiments highlighted these variations. For instance, Montipora, Favites, Porities, and Platygyra have health thresholds of 9 µM N and 0.22 µM P, whereas Acropora has a lower threshold (4.6 µM N; 0.13 µM P; Zhao et al., 2021). The Fv/Fm values of Montipora foliosa remained within the normal range under both eutrophic (6.5 µM N; 0.3 µM P) and oligotrophic (0.7 µM N; 0.006 µM P) conditions after 12 weeks (Wiedenmann et al., 2013). In contrast, Acropora tenuis lost 21-61% of zooxanthellae after a 7-day treatment with 5.05 µM DIN, 0.28 µM P, and a temperature of 31°C (Tanaka et al., 2014). Among the coral species in this study, it appeared that relatively abundant species (e.g., F. pentagona) and some rare species (e.g., A. austera, A. divaricata, and M. caliculata) of this coral community were more susceptible to enrichment. Therefore, it can be speculated that AN deposition into coral reefs may not only reduce coral biomass but also alter coral community composition. These changes could involve shifts in dominant species or the loss of rare species.
4.3 Increased coral-algal phase shifts by AN deposition
Macroalgae have a competitive advantage over corals, covering 13.6% to 44% of reef sites across different depths (Figure 3). The phenomenon of coral-algal phase shifts observed in remote coral reefs may be attributed to coral declines and the overgrowth of macroalgae caused by AN deposition. This hypothesis is supported by our experiment. In both the ANAP and HNHP treatments, we observed a significant increase in macroalgae cover. By day 42, the macroalgae cover of C. serrulata, C. racemosa, C. racemosa var. turbinata, and C. sertularioides reached 65% in the ANAP, while in the HNHP, it reached 70% by day 22. The treatments were designed to simulate the response of benthic macroalgae on coral reefs when exposed to AN deposition levels ranging from 450 µM to 2000 µM. These levels of N supply correspond to the wet deposition of AN in the upwind regions of the SCS Reefs in April and May, 2015. For instance, AN concentrations were recorded at 2174.9 µM in locations such as Tanah Rata and Petaling Jaya in Malaysia and Kototabang in Indonesia. Our experimental observations help explain the observed overgrown macroalgae (up to 60%) on the SCS reefs from May to July, 2015. This macroalgae cover far exceeded that found on healthy reefs, which typically have less than 2% macroalgae cover (Hughes et al., 2010). Additionally, we noted that macroalgae cover tended to be greater in deeper layers compared to shallower areas.
The overgrowth of algae, promoted by AN, could potentially be controlled by herbivores such as fish and sea urchins. Research conducted in Main Hawaiian Islands found that gazing functions on turf algae were more effectively maintained by grazers, browsers, or scrapers in the deeper waters (6-30 m) compared to shallower areas (< 6 m; Foo and Asner, 2021). However, the effectiveness of herbivore consumption is often limited, primarily due to overfishing of coral reefs (Mumby et al., 2006). Moreover, dietary preferences of herbivores can affect grazing behavior (Lapointe et al., 2004). Many herbivorous fishes tend to preferentially feed on noncalcareous turf algae while showing a general disinterest in calcareous macroalgae (Littler and Littler, 1984). This dietary preference is also observed in dominant herbivores in the SCS, such as Scarus genera, Acanthurus triostegus, and Chaetodon species. Consequently, despite the higher fish density and relative abundance of herbivores in the Xisha Islands compared to the Luhuitou in northern SCS (Wu et al., 2015; Li et al., 2017), macroalgae cover tends to be greater in the former than the latter (Chen et al., 2019). In many cases, grazers struggle to effectively regulate macroalgae growth. Even slight changes in seawater nutrient levels can trigger macroalgal blooms, as found in Jamaica and southeast Florida, for example, where blooms occurred with as little as 1.0 µM DIN and 0.1 µM PO43- (Lapointe, 1997). It is important to note that the AN flux in this region often exceeds the nutrient thresholds required for macroalgal blooms.
To serve as potential refuges for corals, habitats with fewer disturbances and reduced thermal stress are essential (Kramer et al., 2019). For instance, in cases where shallow-water corals were subjected to elevated temperature or ultraviolet rays and suffered mortality, deep-water corals often survived, turning deep waters (> 10 m) into refuges for corals (Riegl and Piller, 2003). Moreover, research conducted on the Weizhou Island of China revealed that the shallow layer (1-3 m) had lower live coral cover (1.7%) compared to the deeper layer (3-10 m) with higher cover (10.4%; Yu et al., 2019). Our survey results supported this concept as we observed lower water temperatures (29.5-31.6°C) in the deep layer compared to the shallower areas (where temperature ranged from 30.2°C to 32.7°C). However, this cooler environment in the deep layer may also make it suitable for the proliferation of macroalgae due to reduced exposure to solar radiation, ultraviolet radiation, and photoinhibition. As the euphotic layer in the SCS extends down to 80 m (Tang et al., 2007), there is sufficient light penetration for algae photosynthesis even in deeper waters. Importantly, the influx of AN carried by monsoon winds into the SCS (Zhao et al., 2015; Chen et al., 2019) alleviates N limitation in the euphotic layer, thereby stimulating the growth of macroalgae.
Macroalgae are known for their diverse survival strategies, including predation tolerance, opportunism, or stress tolerance, which make them highly competitive in various benthic environments (Littler and Littler, 1984). They often pose a significant competition challenge to corals by inhibiting coral growth, calcification, reproduction, or colonization (Mccook, 1999; Hughes et al., 2007). Our survey of Yongle Atoll showed that live coral cover averaged only 8.8%, falling below the coral cover warning line of 10% (Kennedy et al., 2013). This status is comparable to some coastal coral reefs that experience heavy human disturbances, such as those in Daya Bay (15.3%; Chen et al., 2009), and Weizhou Island (10-15%) in the northern SCS (Wang et al., 2016). Moreover, our findings indicate that over 50% of survey sections had lower coral abundance in deeper waters compared to shallower areas, coinciding with the presence of abundant macroalgae in deeper regions. These results suggest that excessive AN supply may contribute to coral-algal phase shifts, restricting the potential for remote or deep-water areas to serve as refuges for corals in a warming ocean.
Many factors have been investigated as causes of coral reef declines, including climate warming, tropical cyclones, crown-of-thorns starfish outbreaks (Mellin et al., 2019), bottom trawling, and land-sourced pollutants entering the ocean through river runoff (Mallela et al., 2010). However, the influence of AN on coral reefs has received limited attention. AN deposition in our study region, estimated at 66 mmol N·m-2 during the summer monsoon season (April-September; 2000-2020) from the southwest of SCS, surpasses the estimates from all 31 monitoring stations around the SCS (55 mmol N·m-2·yr-1; 2000-2010; Kim et al., 2014). The higher AN flux is related to various factors, including fertilization and biomass burning in Southwest Asia (Latif et al., 2018). It has been noted that approximately two-thirds of the world’s coral reefs are exposed to AN deposition levels similar to those in our study area. Moreover, coral reef regions in Asia, Southeast Asia, East and North Asia, and the U.S. Caribbean experience even higher AN deposition rates. Projections indicate a 50-100% increase in AN deposition into the SCS between, 2000 and, 2030, with a particularly severe increase (> 200%) in the Coral Triangle, as well as the Red Sea, the Gulfs, and the SW Indian Ocean (Chen et al., 2019). The response of coral reefs worldwide to this substantial AN needs further research and attention.
5 Conclusion
This study has identified coral-algae phase shifts in the remote coral reefs of SCS. Interestingly, we observed that the stress imposed by macroalgae on corals was more pronounced in deeper waters. Through laboratory experiments, we explained the cause-and-effect relationship between the AN deposition and coral-algae phase shifts. The findings indicate that AN supply efficiently promotes macroalgae growth. While a moderate amount of AN loading can mitigate coral bleaching, excessive AN, especially when the supply of N and P is imbalanced, significantly increases coral susceptibility to bleaching.
Data availability statement
The raw data supporting the conclusions of this article will be made available by the authors, without undue reservation.
Author contributions
YF: Writing – Original, Formal analysis, Visualization. XC: Conceptualization, Methodology, Writing – Original, Funding acquisition. YCL: Investigation, Data Curation, Writing – Original. YXL: Investigation, Resources, Data curation. KY: Writing – Review & Editing, Funding acquisition. All authors contributed to the article and approved the submitted version.
Funding
The author(s) declare financial support was received for the research, authorship, and/or publication of this article. This work was funded by the National Science Foundation of China (42076157 and, 42030502).
Acknowledgments
The authors wish to acknowledge Prof. Huiwang Gao for his constructive suggestions for the study on atmospheric nitrogen deposition to the ocean.
Conflict of interest
The authors declare that the research was conducted in the absence of any commercial or financial relationships that could be construed as a potential conflict of interest.
Publisher’s note
All claims expressed in this article are solely those of the authors and do not necessarily represent those of their affiliated organizations, or those of the publisher, the editors and the reviewers. Any product that may be evaluated in this article, or claim that may be made by its manufacturer, is not guaranteed or endorsed by the publisher.
Supplementary material
The Supplementary Material for this article can be found online at: https://www.frontiersin.org/articles/10.3389/fmars.2024.1214449/full#supplementary-material
References
Barile P. J., Lapointe B. E. (2005). Atmospheric nitrogen deposition from a remote source enriches macroalgae in coral reef ecosystems near Green Turtle Cay, Abacos, Bahamas. Mar. pollut. Bull. 50, 1262–1272. doi: 10.1016/j.marpolbul.2005.04.031
Becker D. M., Putnam H. M., Burkepile D. E., Adam T. C., Thurber R. V., Silbiger N. J. (2021). Chronic low-level nutrient enrichment benefits coral thermal performance in a fore reef habitat. Coral Reefs 40, 1637–1655. doi: 10.1007/s00338-021-02138-2
Bednarz V. N., Grover R., Ferrier-Pagès C. (2020). Elevated ammonium delays the impairment of the coral-dinoflagellate symbiosis during labile carbon pollution. Aquat. Toxicol. 218, 105360. doi: 10.1016/j.aquatox.2019.105360
Bell P. R. F. (1992). Eutrophication and coral reefs—some examples in the great barrier reef lagoon. Water Res. 36 (5), 553–568. doi: 10.1016/0043-1354(92)90228-V
Bellwood D. R., Hughes T. P., Folke C., Nystrom M. (2004). Confronting the coral reef crisis. Nature 429, 827–833. doi: 10.1038/nature02691
Beraud E., Gevaert F., Rottier C., Ferrier-Pages C. (2013). The response of the scleractinian coral Turbinaria reniformis to thermal stress depends on the nitrogen status of the coral holobiont. J. Exp. Biol. 216, 2665–2674. doi: 10.1242/jeb.085183
Bruno J. F., Sweatman H., Selig E. R., Schutte V. G. W. (2009). Assessing evidence of phase shifts from coral to macroalgal dominance on coral reefs. Ecology 90 (6), 1479–1484. doi: 10.1890/08-1781.1
Chen J., Shi P., Wang D., Du Y. (2005). Spatial distribution and seasonal variability of the rainfall observed from TRMM Precipitation Radar (PR) in the South China Sea Area (SCSA). Advance Earth Sci. 20 (1), 29–35. doi: 10.3321/j.issn:1001-8166.2005.01.007
Chen T., Yu K., Shi Q., Li S., Price G. J., Wang R., et al. (2009). Twenty-five years of change in scleractinian coral communities of Daya Bay (northern South China Sea) and its response to the 2008 AD extreme cold climate event. Chin. Sci. Bull. 54, 2107–2117. doi: 10.1007/s11434-009-0007-8
Chen X., Yu K., Huang X., Wang Y., Liao Z., Zhang R., et al. (2019). Atmospheric nitrogen deposition increases the possibility of macroalgal dominance on remote coral reefs. J. Geophysical Research: Biogeoscience 124 (5), 1355–1369. doi: 10.1029/2019JG005074
Courtial L., Bielsa V. P., Houlbreque F., Ferrier-Pages C. (2018). Effects of ultraviolet radiation and nutrient level on the physiological response and organic matter release of the scleractinian coral Pocillopora damicornis following thermal stress. PloS One 13 (10), e205261. doi: 10.1371/journal.pone.0205261
Cui D., Wang J., Tan L., Dong Z. (2016). Impact of atmospheric wet deposition on phytoplankton community structure in the South China Sea. Estuar. Coast. Shelf Sci. 13, 1–8. doi: 10.1016/j.ecss.2016.02.011
Cunning R., Baker A. (2013). Excess algal symbionts increase the susceptibility of reef corals to bleaching. Nat. Clim. Change 3, 259–262. doi: 10.1038/nclimate1711
Dentener F., Drevet J., Lamarque J. F., Bey I., Eickhout B., Fiore A. M., et al. (2006). Nitrogen and sulfur deposition on regional and global scales: A multimodel evaluation. Glob. Biogeochem. Cycle 20, (4). doi: 10.1029/2005GB002672
Duce R. A., Laroche J., Altieri K., Arrigo K. R., Baker A. R., Capone D. G., et al. (2008). Impacts of atmospheric anthropogenic nitrogen on the open ocean. Science 320 (5878), 893–897. doi: 10.1126/science.1150369
Ezzat L., Maguer J. F., Grover R., Ferrier-Pagès C. (2015). New insights into carbon acquisition and exchanges within the coral-dinoflagellate symbiosis under NH4+ and NO3- supply. Proc. Biol. Sci. 282 (1812), 20150610. doi: 10.1098/rspb.2015.0610
Ezzat L., Maguer J. F., Grover R., Ferrier-Pagès C. (2016). Limited phosphorus availability is the Achilles heel of tropical reef corals in a warming ocean. Sci. Rep. 6, 31768. doi: 10.1038/srep31768
Fabricius K. E. (2005). Effects of terrestrial runoff on the ecology of corals and coral reefs: review and synthesis. Mar. pollut. Bull. 50 (2), 125–146. doi: 10.1016/j.marpolbul.2004.11.028
Fabricius K. E., Cséke S., Humphrey C., De'Ath G. (2013). Does trophic status enhance or reduce the thermal tolerance of scleractinian corals? A review, experiment and conceptual framework. PloS One 8 (1), e54399. doi: 10.1371/journal.pone.0054399
Ferrier-Pagès C., Gattuso J. P., Dallot S., Jaubert J. (2000). Effect of nutrient enrichment on growth and photosynthesis of the zooxanthellate coral Stylophora pistillata. Coral Reefs 19, 103–113. doi: 10.1007/s003380000078
Fleury B. G., Coll J. C., Tentori E., Duquesne S., Figueiredo L. (2000). Effect of nutrient enrichment on the complementary (secondary) metabolite composition of the soft coral Sarcophyton ehrenbergi (Cnidaria: Octocorallia: Alcyonaceae) of the Great Barrier Reef. Mar. Biol. 136, 63–68. doi: 10.1007/s002270050009
Foo S. A., Asner G. P. (2021). Depth-dependent indicators of algal turf herbivory throughout the Main Hawaiian Islands. Coral Reefs 40, 1397–1408. doi: 10.1007/s00338-021-02162-2
Gardner T. A., Cote I. M., Gill J. A., Grant A., Watkinson A. R. (2003). Long-term region-wide declines in Caribbean Corals. Science 301 (5635), 958–960. doi: 10.1126/science.1086050
Guo C., Yu J., Ho T., Wang L., Song S., Kong L., et al. (2012). Dynamics of phytoplankton community structure in the South China Sea in response to the East Asian aerosol input. Biogeosciences 9, 1519–1536. doi: 10.5194/bg-9-1519-2012
Hadjioannou L., Jimenez C., Rottier C., Sfenthourakis S., Ferrier-Pagès C. (2019). Response of the temperate scleractinian coral Cladocora caespitosa to high temperature and long-term nutrient enrichment. Sci. Rep. 9, 14229. doi: 10.1038/s41598-019-50716-w
Hentati-Sundberg J., Raymond C., Sköld M., Svensson O., Gustafsson B., Bonaglia S. (2020). Fueling of a marine-terrestrial ecosystem by a major seabird colony. Sci. Rep. 10, 15455. doi: 10.1038/s41598-020-72238-6
Hughes T. P., Graham N. A. J., Jackson J. B. C., Mumby P. J., Steneck R. S. (2010). Rising to the challenge of sustaining coral reef resilience. Trends Ecol. Evol. 25 (11), 633–642. doi: 10.1016/j.tree.2010.07.011
Hughes T. P., Rodrigues M. J., Bellwood D. R., Ceccarelli D., Hoegh-Guldberg O., Mccook L., et al. (2007). Phase shifts, herbivory, and the resilience of coral reefs to climate change. Curr. Biol. 17 (4), 360–365. doi: 10.1016/j.cub.2006.12.049
Kanakidou M., Duce R. A., Prospero J. M., Baker A. R., Benitez-Nelson C., Dentener F. J., et al. (2012). Atmospheric fluxes of organic N and P to the global ocean. Glob. Biogeochem. Cycle 26, (3). doi: 10.1029/2011GB004277
Ke Z., Tan Y., Huang L., Liu H., Liu J., Jiang X., et al. (2018). Spatial distribution patterns of phytoplankton biomass and primary productivity in six coral atolls in the central South China Sea. Coral reefs 37, (919–927). doi: 10.1007/s00338-018-1717-7
Kennedy E. V., Perry C. T., Halloran P. R., Iglesias-Prieto R., Schoenberg C. H. L., Wisshak M., et al. (2013). Avoiding coral reef functional collapse requires local and global action. Curr. Biol. 23 (10), 912–918. doi: 10.1016/j.cub.2013.04.020
Kim T., Lee K., Duce R., Liss P. (2014). Impact of atmospheric nitrogen deposition on phytoplankton productivity in the South China Sea. Geophys. Res. Lett. 41 (9), 3156–3162. doi: 10.1002/2014GL059665
Kramer N., Eyal G., Tamir R., Loya Y. (2019). Upper mesophotic depths in the coral reefs of Eilat, Red Sea, offer suitable refuge grounds for coral settlement. Sci. Rep. 9, 2263. doi: 10.1038/s41598-019-38795-1
Lapointe B. E. (1997). Nutrient thresholds for bottom-up control of macroalgal blooms on coral reefs in Jamaica and southeast Florida. Limnol. Oceanogr. 42 (5), 1119–1131. doi: 10.4319/lo.1997.42.5_part_2.1119
Lapointe B. E., Barile P. J., Litter M. M., Littler D. S. (2005b). Macroalgal blooms on southeast Florida coral reefs: II. Cross-shelf discrimination of nitrogen sources indicates widespread assimilation of sewage nitrogen. Harmful Algae 4 (6), 1106–1122. doi: 10.1016/j.hal.2005.06.002
Lapointe B. E., Barile P. J., Littler M. M., Littler D. S., Bedford B. J., Gasque C. (2005a). Macroalgal blooms on southeast Florida coral reefs: I. Nutrient stoichiometry of the invasive green alga Codium isthmocladum in the wider Caribbean indicates nutrient enrichment. Harmful Algae 4 (6), 1092–1105. doi: 10.1016/j.hal.2005.06.004
Lapointe B. E., Barile P. J., Yentsch C. S., Littler M. M., Littler D. S., Kakuk B. (2004). The relative importance of nutrient enrichment and herbivory on macroalgal communities near Norman’s Pond Cay, Exumas Cays, Bahamas: a “natural” enrichment experiment. J. Exp. Mar. Biol. Ecol. 298 (2), 275–301. doi: 10.1016/S0022-0981(03)00363-0
Lapointe B. E., Brewton R. A., Herren L. W., Porter J. W., Hu C. (2019). Nitrogen enrichment, altered stoichiometry, and coral reef decline at Looe Key, Florida Keys, USA: a 3-decade study. Mar. Biol. 166, 108. doi: 10.1007/s00227-019-3538-9
Lapointe B. E., Tewfik A., Phillips M. (2021). Macroalgae reveal nitrogen enrichment and elevated N:P ratios on the Belize Barrier Reef. Mar. pollut. Bull. 171, 112686. doi: 10.1016/j.marpolbul.2021.112686
Latif M. T., Othman M., Idris N., Juneng L., Abdullah A. M., Hamzah W. P., et al. (2018). Impact of regional haze towards air quality in Malaysia: A review. Atmos. Environ. 177, 28–44. doi: 10.1016/j.atmosenv.2018.01.002
Lei X., Huang H., Wang H., Li X., Lian J. (2009). Study on the responses of the symbiotic zooxanthellae of hermatypic coral to eutrophication. Mar. Sci. Bull. 28 (1), 43–49. doi: 10.3969/j.issn.1001-6392.2009.01.007
Li S., Yu K., Chen T., Shi Q., Chen T. (2011). Seasonal patterns of densities of symbiotic zooxanthellae in scleractinian corals from Daya Bay, northern South China Sea, and relation to coral bleaching. J. Trop. Oceanography 30 (2), 39–45. doi: 10.3969/j.issn.1009-5470.2011.02.006
Li Y., Wu Z., Chen S., Cai Z., Lan J., Tong Y., et al. (2017). Discussion of the diversity of the coral reef fish in the shallow reefs along the Yongxing and Qilianyu island. Marine Environmental Science 36 (4), 509–516. doi: 10.13634/j.cnki.mes20170405
Liao Z. (2021). Spatial distribution of coral community and benthic algae and their ecological impacts across the South China Sea (Nanning, China: Guangxi University).
Littler M. M., Littler D. S. (1984). Relationships between macroalgal functional form groups and substrata in a subtropical rocky-intertidal stream. J. Exp. Mar. Biol. Ecol. 74 (1), 13–34. doi: 10.1016/0022-0981(84)90035-2
MacDonald C., Bridge T. C. L., Jones G. P. (2016). Depth, bay position and habitat structure as determinants of coral reef fish distributions: Are deep reefs a potential refuge? Mar. Ecol. Prog. Ser. 561, 217–231. doi: 10.3354/meps11953
Mallela J., Parkinson R., Day O. (2010). An assessment of coral reefs in Tobago. Caribb. J. Sci. 46 (1), 83–87. doi: 10.18475/cjos.v46i1.a10
Mcclanahan T. R., Sala E., Stickels P. A., Cokos B. A., Baker A. C., Starger C. J., et al. (2003). Interaction between nutrients and herbivory in controlling algal communities and coral condition on Glover's Reef, Belize. Mar. Ecol. Prog. Ser. 261, 135–147. doi: 10.3354/meps261135
Mccook L. J. (1999). Macroalgae, nutrients and phase shifts on coral reefs: scientific issues and management consequences for the Great Barrier Reef. Coral Reefs 18, 357–367. doi: 10.1007/s003380050213
McManus J. W., Polsenberg J. F. (2004). Coral-algal phase shifts on coral reefs: Ecological and environmental aspects. Prog. Oceanogr. 60 (2-4), 263–279. doi: 10.1016/j.pocean.2004.02.014
Mellin C., Matthews S., Anthony K. R.N., Brown S. C., Caley M. J., Johns K. A., et al. (2019). Spatial resilience of the Great Barrier Reef under cumulative disturbance impacts. Glob Change Biol. 25, 2431–2445. doi: 10.1111/gcb.14625
Mumby P. J., Dahlgren C. P., Harborne A. R., Kappel C. V., Micheli F., Brumbaugh D. R., et al. (2006). Fishing, trophic cascades, and the process of grazing on coral reefs. Science 311 (5757), 98–101. doi: 10.1126/science.1121129
Olgun N., Duggen S., Andronico D., Kutterolf S., Croot P. L., Giammanco S., et al. (2013). Possible impacts of volcanic ash emissions of Mount Etna on the primary productivity in the oligotrophic Mediterranean Sea: Results from nutrient-release experiments in seawater. Mar. Chem. 152, 32–42. doi: 10.1016/j.marchem.2013.04.004
Ren H., Chen Y., Wang X. T., Wong G. T. F., Cohen A. L., Decarlo T. M., et al. (2017). 21st-century rise in anthropogenic nitrogen deposition on a remote coral reef. Science 356 (6339), 749–752. doi: 10.1126/science.aal3869
Renegar D. A., Riegl B. M. (2005). Effect of nutrient enrichment and elevated CO2 partial pressure on growth rate of Atlantic scleractinian coral Acropora cervicornis. Mar. Ecol. Prog. Ser. 293, 69–76. doi: 10.3354/meps293069
Riegl B., Piller W. E. (2003). Possible refugia for reefs in times of environmental stress. Int. J. Earth Sci. 92, 520–531. doi: 10.1007/s00531-003-0328-9
Rosset S., Wiedenmann J., Reed A. J., D'Angelo C. (2017). Phosphate deficiency promotes coral bleaching and is reflected by the ultrastructure of symbiotic dinoflagellates. Mar. pollut. Bull. 118 (1-2), 180–187. doi: 10.1016/j.marpolbul.2017.02.044
Safai P. D., Rao P., Mornin G. A., All K., Chate D. M., Praveen P. S. (2004). Chemical composition of precipitation during 1984-2002 at Pune, India. Atmos. Environ. 38 (12), 1705–1714. doi: 10.1016/j.atmosenv.2003.12.016
Shatova O. A., Wing S. R., Hoffmann L. J., Wing L. C., Gault-Ringold M. (2017). Phytoplankton community structure is influenced by seabird guano enrichment in the Southern Ocean. Estuarine Coast. Shelf Sci. 191, 125–135. doi: 10.1016/j.ecss.2017.04.021
Steven A. D. L., Atkinson M. J. (2003). Nutrient uptake by coral-reef microatolls. Coral Reefs 22, 197–204. doi: 10.1007/s00338-003-0303-8
Sundarambal P., Balasubramanian R., Tkalich P., He J. (2010). Impact of biomass burning on ocean water quality in Southeast Asia through atmospheric deposition: field observations. Atmos. Chem. Phys. 10 (23), 11323–11336. doi: 10.5194/acp-10-11323-2010
Szmant A. M. (2002). Nutrient enrichment on coral reefs: is it a major cause of coral reef decline? Estuaries 25, 743–766. doi: 10.1007/BF02804903
Tanaka Y., Inoue M., Nakamura T., Suzuki A., Sakai K. (2014). Loss of zooxanthellae in a coral under high seawater temperature and nutrient enrichment. J. Exp. Mar. Biol. Ecol. 457, 220–225. doi: 10.1016/j.jembe.2014.04.019
Tang S., Chen C., Zhan H., Xu D., Liu D. (2007). Retrieval of euphotic layer depth of South China Sea by remote sensing. J. Trop. Oceanography 26 (1), 9–15. doi: 10.3969/j.issn.1009-5470.2007.01.002
Tomascik T., Sander F. (1985). Effects of eutrophication on reef-building corals. I. Growth rate of the reef building coral Monstrea annularis. Mar. Biol. 87, 143–155. doi: 10.1007/BF00539422
Veron J. (2000). Corals of the World (3-Volume) (Townsville: Australian Institute of Marine Science).
Wang W., Yu K., Wang Y. (2016). A review on the research of coral reefs in the Weizhou Island, Beibu Gulf. Trop. Geogr. 36 (1), 72–79. doi: 10.13284/j.cnki.rddl.002806
Wiedenmann J., D'Angelo C., Smith E. G., Hunt A. N., Legiret F., Postle A. D., et al. (2013). Nutrient enrichment can increase the susceptibility of reef corals to bleaching. Nat. Clim. Change 3, 160–164. doi: 10.1038/NCLIMATE1661
Wu Z., Cai Z., Chen S., Zhang G., Li X., Wang D., et al. (2015). Species distribution and diversity of the coral reef fishes in the shallow reefs along the east and south coasts of Hainan Island. Journal of Fisheries of China 39 (8), 1203–1217. doi: 10.11964/jfc.20150109688
Yu K. (2012). Coral reefs in the South China Sea: Their response to and records on past environmental changes. Sci. China-Earth Sci. 55, 1217–1229. doi: 10.1007/s11430-012-4449-5
Yu W., Wang W., Yu K., Wang Y., Huang X., Huang R., et al. (2019). Rapid decline of a relatively high latitude coral assemblage at Weizhou Island, northern South China Sea. Biodivers. Conserv. 28, 3925–3949. doi: 10.1007/s10531-019-01858-w
Zhao M., Yu K., Zhang Q. (2006). Review on coral reefs biodiversity and ecological function. Acta Ecologica Sin. 26 (1), 186–194. doi: 10.3321/j.issn:1000-0933.2006.01.025
Zhao H., Yuan M., Strokal M., Wu H. C., Liu X., Murk A., et al. (2021). Impacts of nitrogen pollution on corals in the context of global climate change and potential strategies to conserve coral reefs. Sci. Total Environ. 774, 145017. doi: 10.1016/j.scitotenv.2021.145017
Keywords: atmospheric N, coral reefs, coral-algal symbiont, macroalgae, coral bleaching, South China Sea
Citation: Fu Y, Chen X, Liu Y, Li Y and Yu K (2024) The effects of atmospheric nitrogen deposition in coral-algal phase shifts on remote coral reefs. Front. Mar. Sci. 11:1214449. doi: 10.3389/fmars.2024.1214449
Received: 02 May 2023; Accepted: 23 January 2024;
Published: 15 February 2024.
Edited by:
Hajime Kayanne, The University of Tokyo, JapanReviewed by:
Thamasak Yeemin, Ramkhamhaeng University, ThailandBrian Lapointe, Florida Atlantic University, United States
Copyright © 2024 Fu, Chen, Liu, Li and Yu. This is an open-access article distributed under the terms of the Creative Commons Attribution License (CC BY). The use, distribution or reproduction in other forums is permitted, provided the original author(s) and the copyright owner(s) are credited and that the original publication in this journal is cited, in accordance with accepted academic practice. No use, distribution or reproduction is permitted which does not comply with these terms.
*Correspondence: Xiaoyan Chen, eHljaGVuQGd4dS5lZHUuY24=; Kefu Yu, a2VmdXl1QHNjc2lvLmFjLmNu