- 1State Environmental Protection Key Laboratory of Biodiversity and Biosafety, Ministry of Ecology and Environment of China, Nanjing, China
- 2Nanjing Institute of Environmental Sciences, Ministry of Ecology and Environment of China, Nanjing, China
- 3Institute of Evolution and Marine Biodiversity, Ocean University of China, Qingdao, China
- 4Department of Ecological Monitoring, Nantong Environmental Monitoring Centre of Jiangsu Province, Nantong, China
Perfluoroalkyl and polyfluoroalkyl substances (PFAS) have been extensively documented as posing significant health risks to human populations. However, there is a lack of research of their impact on endangered species, which significantly affects the effectiveness of conservation efforts and maintenance of these populations. In this study, we examined the levels of PFAS pollution in adults and juveniles of the vulnerable Saunders’s gull (Larus saundersi), along with their various food sources using ultra high-performance liquid chromatography-tandem mass spectrometry and Ecopath model. Long-chain PFAS, predominantly composed of perfluorooctanoic acid (accounting for 51.4% of the total), were identified as the main pollutants in the gull, its food, and the environment. Saunders’s gulls showed significant bioaccumulation and magnification of PFAS, with contamination levels significantly above those recorded in other species. Mean PFAS levels between juveniles (904.26 ng/g wet weight) and adults (407.40 ng/g wet weight) revealed a significant disparity, indicating that PFAS pollution may severely threaten these birds. Among the food sources analyzed, bivalves and polychaetes emerged as the primary contributors to PFAS contamination in Saunders’s gulls, with high transfer efficiency. The fundamental cause of PFAS pollution in benthic organisms and the gulls appears to be baseline environmental pollution, which was highly consistent across all examined pollutant types. Moreover, chemical plants close to breeding areas may cause severe environmental pollution, threatening organisms at various trophic levels through the food web. We suggest enhancing the pollution monitoring of important biological habitats for timely prediction and early warning of chemical risks. Additionally, ecological restoration of key habitats should be strengthened to ensure the effectiveness of biodiversity conservation.
1 Introduction
As portrayed in Rachel Carson’s book Silent Spring, large amounts of chemical pollutants deposited in the environment seriously threaten ecosystem health and human well-being, while precipitating a sharp decline in biodiversity (Jaureguiberry et al., 2022; Sylvester et al., 2023). Some emerging pollutants such as persistent organic pollutants have been detected in many terrestrial or aquatic organisms, including the common dolphins (Delphinus delphi), Cod (Gadus morhua) and white-tailed sea eagles (Haliaeetus albicilla), which have significantly hindered the effectiveness of biodiversity conservation efforts (Kannan et al., 2002; Falandysz et al., 2007). Hence, Target 7 of the Kunming-Montreal Global Biodiversity Framework was established to mitigate pollution risks and minimize the adverse impact of pollution from all sources to levels that are not detrimental to biodiversity and ecosystem functions and services, taking into account their cumulative effects, by 2030 (https://www.cbd.int/doc/c/e6d3/cd1d/daf663719a03902a9b116c34/cop-15-l-25-en.pdf). The Stockholm Convention entered into force in 2004 with the aim of reducing or eliminating persistent organic pollutant emissions and protecting human health and the environment from these pollutants (https://chm.pops.int/TheConvention/ConferenceoftheParties/ReportsandDecisions/tabid/208/ctl/Download/mid/6863/Default.aspx?id=101&ObjID=7671). In spite of these multiple and ambitious international agreements that have been established over several decades, the degradation of ecosystems and the consequent decline in biodiversity persist, with some instances even showing an acceleration.
PFAS are emerging pollutants of considerable relevance with characteristics of biotoxicity, environmental persistence, and bioaccumulation (Evich et al., 2022). PFAS are anthropogenic substances that contain high-energy carbon-fluorine covalent bonds (C-F), which have been produced and widely used in industrial and civilian applications, including the production of clothing, blankets, and electroplating, for over 50 years (Dickman and Aga, 2022). Recent studies and reports have found that PFAS are widespread in drinking water, air, soil, foods, food packaging materials, wild organisms, and has a potential risk of biomagnification in the food chain (Wee and Aris, 2023). The existence of PFAS in organisms is of particular concern given the demonstrated harmful effects to health, including impacts on the reproductive system and immune function (Bach et al., 2015; Stein et al., 2016).In addition to humans, many endangered wildlife species suffer from PFAS contamination. Cui et al. (2019) presented the initial findings regarding the presence of PFAS in the blood and dietary sources of two endangered primate species, the golden snub-nosed monkey (Rhinopithecus roxellana) and Francois’ leaf monkey (Trachypithecus francoisi). Since PFAS are enriched in living organisms through the food chain or food web, species at higher trophic levels will have higher levels of contamination, representing a threat to wildlife survival and population continuation. Most current PFAS exposure research has focused on some special taxa such as seabirds and raptors (Colomer-Vidal et al., 2022; Sun et al., 2022), as important indicator species of environmental change, whereas related studies on shorebirds that are highly dependent on wetland habitats are rather limited (Ma et al., 2022). Given that environmental pollution is one of the most important drivers of the rapid decline of shorebird populations, lack of knowledge of the current status of their PFAS occurrence is not conducive to the conservation of endangered species such as Eurynorhynchus pygmeus, Larus saundersi, and Tringa guttifer (Murray et al., 2018; Ma et al., 2023). For example, concentrations of Perfluorooctane sulfonate (PFOS) in the whole eggs of herring gulls (Larus argentatus) from two coastal areas in northern Norway were found to have increased significantly by a factor of nearly two between 1983 and 2003, which then leveled off in 2003 (Verreault et al., 2007). Charadrius alexandrinus in China are widely exposed to the pollution stress from PFAS, and PFAS concentrations in adult and juvenile birds have reached dangerous levels (Sun et al., 2023). Thus, there is a huge gap concerning the biodiversity conservation and pollution abatement of threatened and vulnerable shorebirds.
Chemical pollution, especially PFAS pollution, has received relatively less attention as a contributing factor to global biodiversity loss compared to other factors (Sonne et al., 2023). China’s chemical industry has been the largest in the world in view of revenue since 2011, especially mainly distributed in some coastal provinces, such as Jiangsu and Shandong (Chen and Reniers, 2020). Due to these sites of chemical industry usually were selected in the undeveloped area with extensive natural habitats, it would formulate a nonnegligible threat on biodiversity and ecosystem health. Not addressing these pollution effects may significantly compromise the effectiveness of biodiversity conservation efforts. Therefore, to fill this gap, we focused on the endangered shorebird species Saunders’s gull (Larus saundersi) in the wetland along the coast of Jiangsu, and analyzed the transfer of PFAS from substrates to foods and to birds to explore the threat of these pollutants from the adult to juvenile stage. The vast wetland along the coast of Jiangsu supports a large flock of migratory, wintering, and breeding birds with abundant food and suitable habitats. Some endangered bird species are highly dependent on this coastal mudflat, including the spoon-billed sandpiper, Nordmann’s greenshank, and the black-faced spoonbill (Yang et al., 2020). Saunders’s gull (Larus saundersi) is classified as “vulnerable” on the International Union for Conservation of Nature (IUCN) Red List (https://www.iuCnredlist.org/species/22694436/132551327), which fed mainly on small fish, crustaceans, and polychaete worms (https://birdsoftheworld.org/bow/species/saugul2/cur/introduction), breeds in eastern mainland China, and sporadically at sites on the south-west coast of South Korea. Saunders’s gull faces endangerment due to habitat loss primarily caused by the diminishing stretches of common seepweed habitat in the study area and adjacent regions (Jiang et al., 2010). This study area thus plays a vital role in the migration, breeding, and conservation of Saunders’s gull.
The results would serve as a theoretical foundation for bird protection and pollution abatement in the biodiversity hotspot. Overall, our work on PFAS pollution in bird habitats can provide not only a scientific basis for the protection of important bird species and data support for the transfer of PFAS in the food chain, but can further offer a reference to facilitate evaluations of the pollution and inform strategies for appropriate habitat management.
2 Material and methods
2.1 Sample collection
In the breeding period (April to August) of 2022, eight fresh carcasses of Saunders’s gull (hereinafter “gull”) were collected on the coast of Xiaoyangkou in Nantong, Jiangsu, China, where there was a chemical plant nearby (Figure 1), including five adult individuals and three juvenile individuals. Highly putrefied carcasses were discarded because of the potential environmental pollution. We dissected these carcasses to obtain chest muscle samples. To determine the content of PFAS in the food of the gull, sediment samples and three types of macrobenthos were collected in these gridded sampling sites in April 2022 (Figure 1), including clamworm (Perinereis nuntia), bivalve (Meretrix meretrix), and shrimp (Acetes chinensis). Macrofauna were collected from 9 sampling sites (Figure 1). In the selected sites, the coastal habitat was quantitatively sampled four times each to the sediment depth of 30 cm using a quadrat (25 cm × 25 cm). A 0.5-mm mesh was utilized to sieve the invertebrate samples in the foraging area, including clamworms, bivalves, shrimps, and sediment samples. All samples underwent lyophilization, homogenization, and storage at −20°C until analysis. Sample collection adhered to national ethical guidelines (Animal management regulations, Order No. 2 of the State Science and Technology Commission, China, 1988), and the collection of carcass samples due to natural causes was given its approval by Jiangsu Wildlife Protection Station (Approval [2017] No. 14).
2.2 Sample pretreatment and analysis
Samples underwent extraction procedures according to the methods outlined by Wang et al. (2021), which are elaborated in detail in the supplementary information (refer to Supplementary Figure S1). The solutions were centrifuged for 3 minutes at 10000 rpm and filtered through a 0.22 μm membrane as a final step before being analyzed using ultra high-performance liquid chromatography (UHPLC)-tandem mass spectrometry (MS/MS). Target PFAS tested encompassed perfluorobutanoic acid (PFBA), perfluoro-n-pentanoic acid (PFPeA), perfluorohexanoic acid (PFHxA), perfluoroheptanoic acid (PFHpA), perfluorooctanoic acid (PFOA), perfluorononanoic acid (PFNA), perluorodecanoic acid (PFDA), perfluoroundecanoic acid (PFUnDA), perfluorododecanoic acid (PFDoDA), perfluorotetradecanoic acid (PFTeDA), perfluoro-1-butane sulfonic acid (PFBS), perfluoro-1-hexane sulfonic acid (PFHxS), perfluorooctanesulfonic acid (PFOS) and perfluoro-1-decanesulfonate (PFDS). The four chemical compounds (PFBA, PFPeA, PFHxA, PFBS) were classified into the short-chain group based on their carbon content, while the remaining compounds belonged to the long-chain category. The UHPLC-ESIMS/MS conditions and instrument parameters are provided in Supplementary Table S1. The native and mass-labeled PFAS standards used in this study were consistent with those utilized in a prior study (Wang et al., 2021). Multi-reaction monitoring mode was used for quantitative analysis, and Supplementary Table S2 lists the ideal MS/MS parameters for the 14 PFAS along with nine surrogate standards. Acquisition and processing of quantitative data from samples and internal calibration standards were made easier by the Analyst software (AB SCIEX, v1.6).
2.3 Quality assurance and control
Prior to use, all laboratory equipment and solvents underwent testing to prevent contamination. To minimize potential laboratory background pollution, two extraction blanks were processed for each batch, yielding undetectable levels of PFAS. The LOQs were assessed as 10 times the standard deviation of the blank samples fortified at 10 ng/g w.w (Wang et al., 2021). The limit of detection (LOD) and the limit of quantification (LOQ) in samples were 0.01-0.05 ng/g and 0.03-0.15 ng/g respectively. Recovery (RE) was calculated for each analyte using the ratio between the actual calculated values of matrix spike sample and spiked values of samples according to the formula: RE % = (actual calculated values/piked values) × 100%. Matrix spike recovery was performed with 3 ng/g contamination levels for five sample matrix from the reference site. Matrix spike recoveries of target compounds ranged from 72.98% to 121.63% for five sample matrix with the relative standard deviation (RSD) values ranged between 0.79% to 19.96% (Supplementary Table S3).
2.4 Data analysis
Statistical analyses were conducted for PFAS compounds with detection frequencies exceeding 50%; in cases where the PFAS concentration was less than the LOQ, the value of LOQ/2 was utilized in the calculations. T-tests were employed to compare concentrations between juvenile and adult gull group, with statistical significance set at p < 0.05. Statistical analyses, including principal components and visualizations of the results were performed using SPSS 22 (IBM Co, Armonk, NY, USA), OriginPro 2023b (Origin Lab Corporation), and R software. According to previous research on Saunders’s gull (Wang et al., 2017), the isotope values from sediment samples, invertebrate, and bird samples were acquired. The biomass data of invertebrates and birds in the study area were obtained from Wang et al. (2019) (Supplementary Table S4). We employed the Ecopath model within Ecopath with Ecosim (EwE model) (Christensen and Walters, 2004) and followed the methodology outlined by Walters and Christensen (2018) to integrate biomass, contaminant concentrations, and production (on a logarithmic scale) as chemical tracers of the food web structure. The EwE model uses a series of linear equations to describe the balance of energy flow or material flow in each functional group of the ecosystem, and makes the whole ecosystem reach equilibrium by establishing a food web, so as to obtain a static equilibrium model of the ecosystem at a given time, and the basic equations used to balance each functional group are:
where: for functional group i, P is its production; B is its biomass (which can be expressed in terms of wet weight, dry weight, energy, nutrient content such as C, N); EE is its Ecotrophic Efficiency (EE), which is usually less than 1; Q is the proportion of food consumed by the predator; and); EE is its ecotrophic efficiency (EE), which is usually less than 1; Q is the proportion of food consumed by the predator; and BAi is the bioaccumulation rate of functional group i; DC is an n x m food matrix (where n is the number of predators and m is the number of baits) describing the predator-prey relationship between functional groups; DCji is the proportion of bait i in the diet of predator j; Yi is the amount of catch; Ei is the net migration rate (emigration minus immigration; in the present model, all functional groups live in the same ecosystem and Ei is 0).
According to the principle of functional group setting in the model, sediment samples, shrimp, clam, bivalve and Saunders’ gull were classified into five ecological functional groups in this study. According to the analysis of structure and energy flow among 20 functional groups in the study area (Wang et al., 2019), we obtained the biomass (B), P/B coefficient, Q/B coefficient of each functional group in this study and other parameters (Supplementary Table S4). The ecological trophic conversion efficiency (EE) refers to the conversion efficiency of the production volume of the functional group, which is a more difficult parameter to obtain, and the model set it as an unknown parameter, which was derived from other parameters by debugging the model. Based on the previous research on the diet of Saunders’ gull on the coast of the Yellow Sea (Wang et al., 2017), the food composition matrix from prey (sediment samples, shrimp, clam, bivalve) to predator (Saunders’s gull) was obtained (Supplementary Table S5). After inputting these model parameters, the fractional trophic level was generated, integrating energy flow and food composition, as well as the ecotrophic efficiency.
3 Results
3.1 Detection rate and concentrations of PFAS
The results of testing 14 PFAS in five samples showed that PFOA, PFBA, PFUnDA, PFDA, PFNA, PFOS, and PFHxS had higher detection rates than the remaining PFAS tested (57.17–100%). The organisms exhibited varying concentrations of PFAS compounds, with PFOA being the most prevalent at 51.4% (mean proportion) of the total PFAS concentration, followed by PFBA (16%), PFOS (15.2%), PFUnDA (5.4%), PFNA (4.6%), PFDA (2.6%), and PFDoDA (1.4%). Lower detection rates (2.22−42%) were found for PEDoDA, PFHpA, PETeDA, PFPeA, PFHxA, PFBS, and PFDS. The average detection frequency of long-chain PFAS (50.35%) was higher than that of short-chain PFAS (32%).
3.2 Differentiation of PFAS between adults and juveniles
All 14 PFAS detected in the juvenile (n = 3) and adult (n = 5) gull chest muscle are presented in Figure 2; Supplementary Table S6; the concentrations of only PFBS and PFDS were below the LOD of the analytical method. In comparison to adult gulls (mean: 407.40 ng/g ww), juvenile gulls exhibited significantly higher concentrations of total PFAS levels in their chest muscles (mean: 904.26 ng/g ww), highlighting a notable disparity. Additionally, the levels of total PFSA in the chest muscles of juvenile gulls (mean: 399.49 ng/g ww) were markedly elevated compared to those in adults (mean: 18.90 ng/g ww; p < 0.05). The differences in the levels of PFUnDA, PFDA, PFDoDA, and PFOS between the two groups were significant (p < 0.05), with concentration ranges of 41.47–134.75, 23.62–82.32, 10.59–31.60, and 187.49–732.05 ng/g ww, respectively, in juvenile gulls, and not detected–6.31, not detected–8.07, not detected–13.18, and 0.62–57.78 ng/g ww, respectively, in adult gulls.
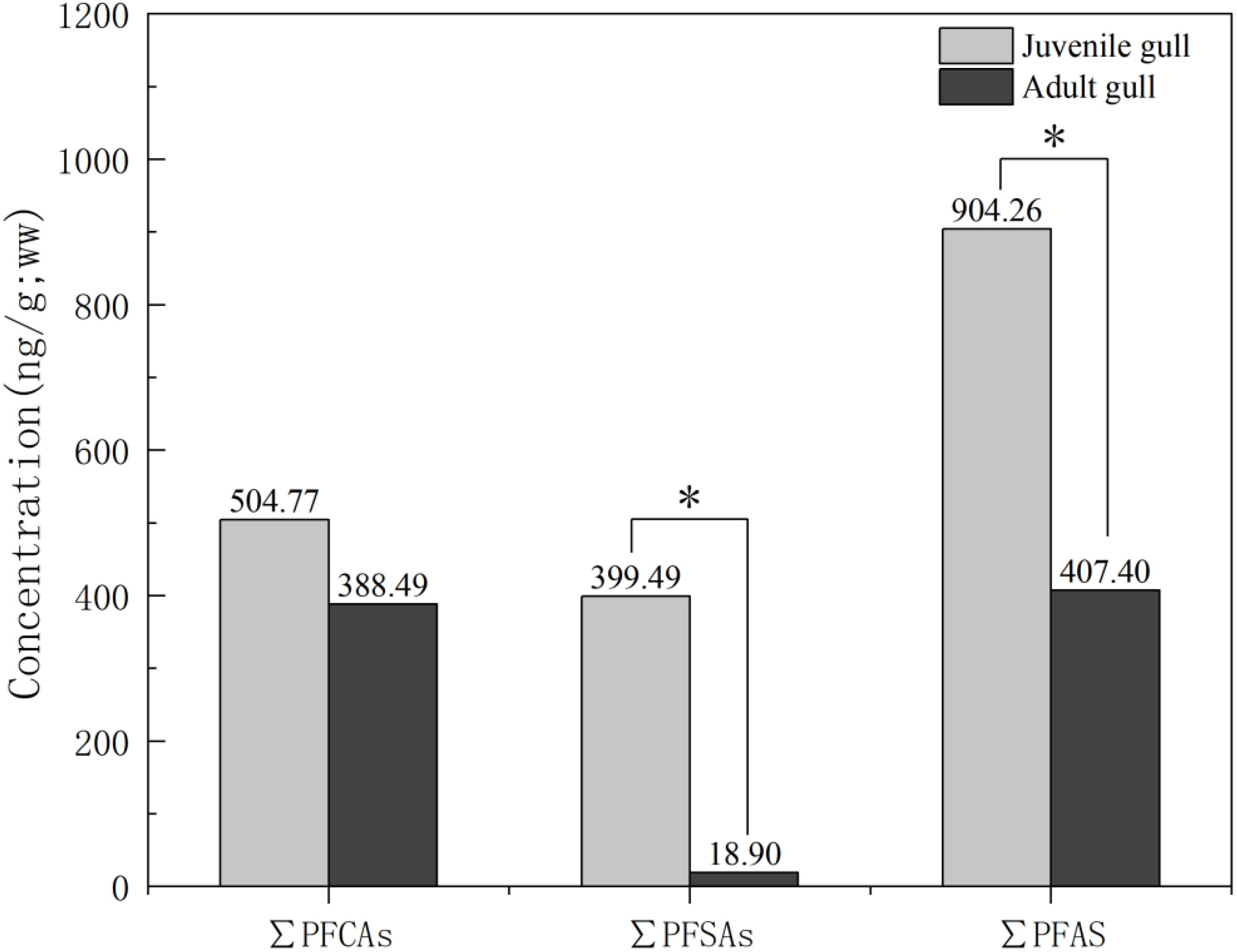
Figure 2. Concentrations of ∑PFCAs, ∑PFSAs and ∑PFAS in chest muscle from juvenile and adult. * indicated p < 0.05. Juvenile gull (n=3) and adult gull (n=5).
3.3 Comparison of PFAS in gulls and their food
PFAS concentrations varied across the organisms collected from the mudflat in the Yellow Sea, ranging from 9.42 ng/g ww (shrimp) to 593.72 ng/g ww (gull chest muscle). Total concentrations of PFAS in the samples followed the sequence gull > clamworm > bivalve > shrimp (refer to Supplementary Table S7; Figure 3), potentially indicative of variations in their bioaccumulative capacities, environmental surroundings, physiology, and dietary preferences. Regardless of taxa, PFOA, PFBA, and PFOS emerged as the predominant substances detected in the organisms. Their respective concentration ranges were 0.47–677.44, not detected–517.70, and 23.5–682 ng/g ww. Notably, the highest levels of PFAS residues were found in the chest muscle of gulls (ranging from 11.82–677.44 ng/g ww). Previous studies with sampling locations distributed in coastal wetlands such as the North Pacific and Arctic Ocean detected a PFOS content in bird muscles in the range of 0.8-65.8 ng/g, which is much lower than the range detected in the present study (Supplementary Table S8).
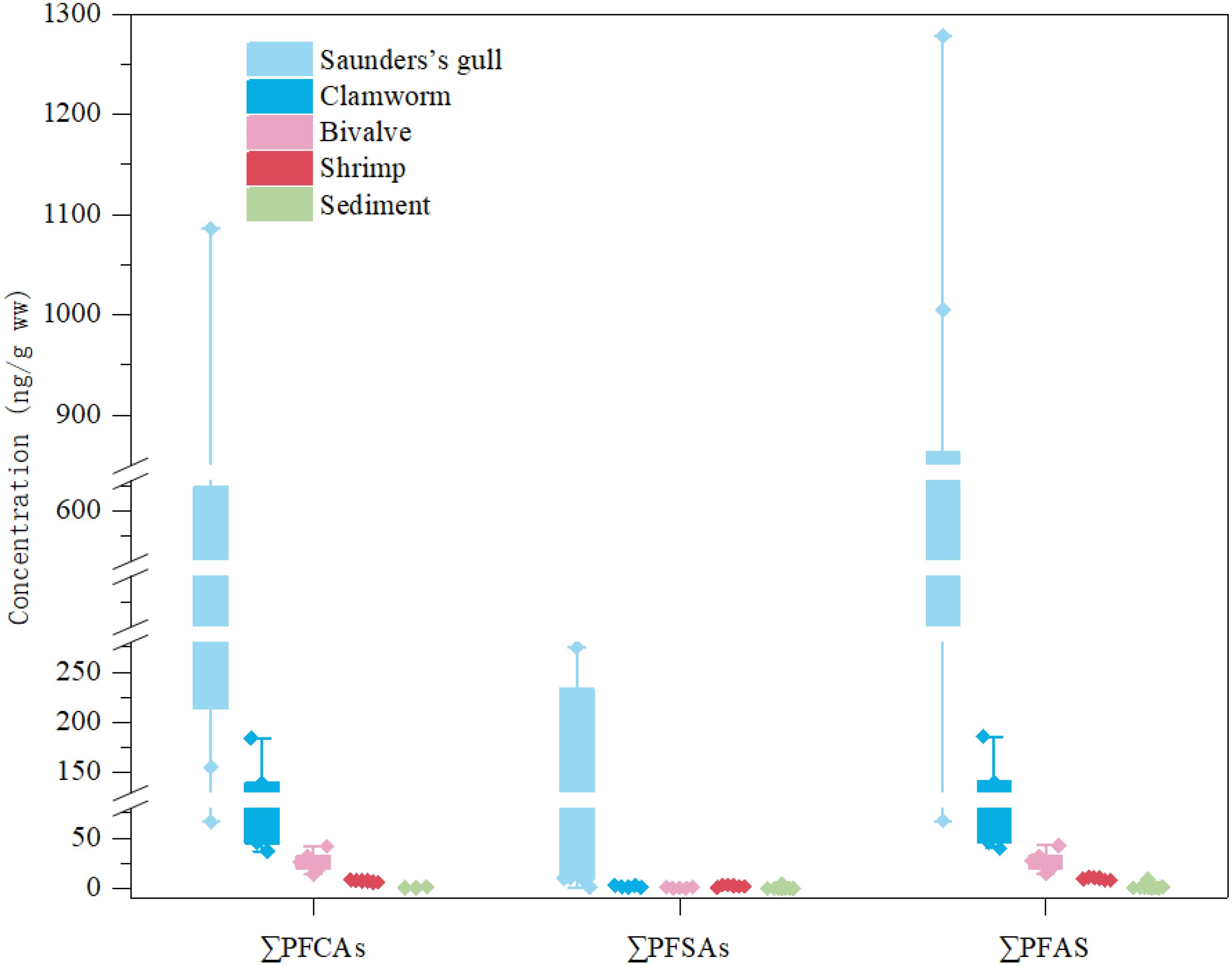
Figure 3. Box plot of concentrations of ∑PFCAs, ∑PFSAs and ∑PFAS in Saunders's gull (n=8), clamworm, bivalve, shrimp and sediment samples.
The sediment samples exhibited total PFAS concentrations ranging from 0.05 to 9.10 ng/g, aligning closely with earlier findings in the Yellow Sea (Zhong et al., 2021), yet notably lower than the levels detected in organisms. Only PFOA, PFBA, and PFOS were above the LOD among the analyzed PFAS at the concentrations of PFOA (0.92 ng/g), PFOS (0.44 ng/g) and PFBA (0.27 ng/g), respectively (Supplementary Table S7).
3.4 Trophic transfer and bioaccumulation of PFAS
The Sankey diagram revealed the correlation among different creatures, the type and characteristic of PFAS (Figure 4A). The types of PAFS in gulls was more abundant than sediment and invertebrates. Most of PFAS existing in gulls and invertebrates were clustered into long-chain group, and only five types of short-chain PFAS were detected. A principal component analysis was used to assess the connections between the 14 PFAS response signals and five different kinds of samples (Figure 4B). The combined contribution rate of the first two principal components (PC1 and PC2) to the total variation in PFAS levels was 63.11% (Supplementary Table S7), which was highly reliable for explaining the composition of PFAS. Meanwhile, PFNA, PFOA, PFDA, PFHpA, PFDoDA, PFUnDA, PFOS had higher contribution for grouping and describing the PFAS in the study area (Supplementary Figure S2; Figure 4B). PFBS and PFDS were negatively correlated with PC1 and PC2, indicating that these two indicators were not detected or had lower detection values in each group. Other 12 indicators were positively correlated with these two components. The bivalve, shrimp, and sediment groups showed good intragroup repeatability and high similarity in the sample data, whereas the gull group was more dispersed and differed from the other clusters, consistent with the results shown in Supplementary Table S8.
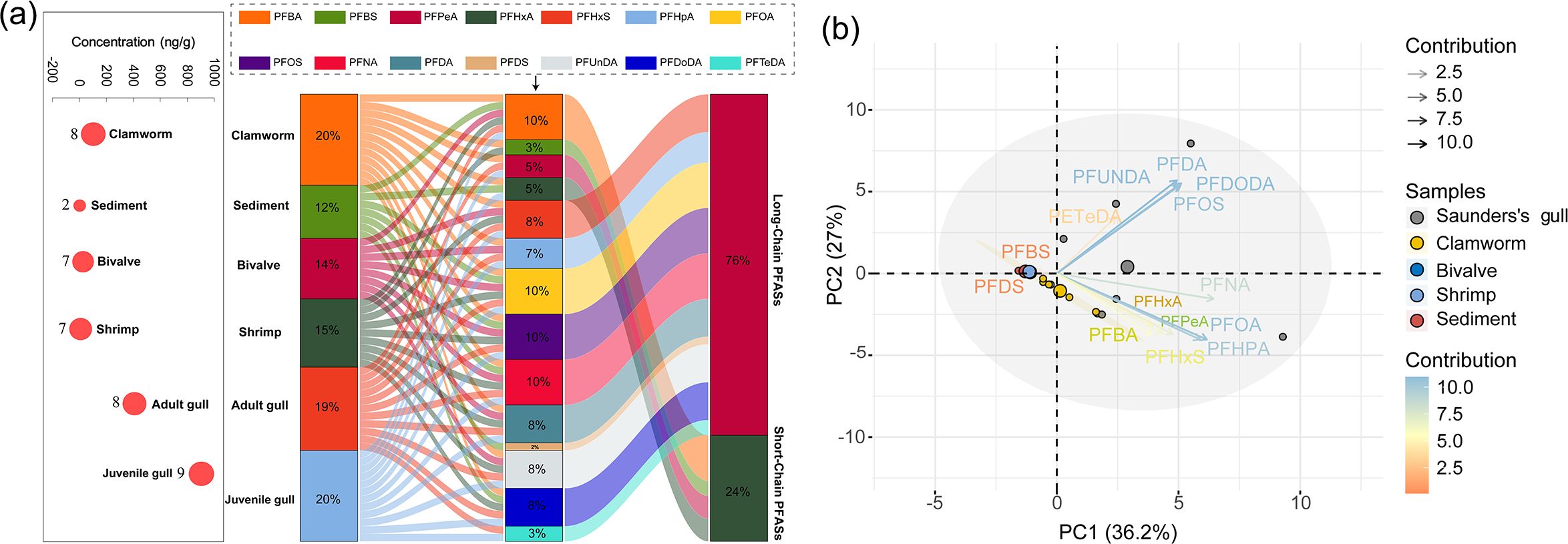
Figure 4. The Sankey diagram of PFAS at different trophic levels (A). The left pink circle represents the concentration of PFAS in different sectors in (A). In the Sanger plot, Color lines and numbers represent the flow between different parts. The size of the histogram of different components indicates the percentage of concentration and the value (%) represents the proportion associated with each measure. Principal component analysis (PCA)-based correlation biplots of pollutant concentrations (14 PFAS) in samples (B). The closer the straight line of arrows in the figure is to the outer circle, the larger the impact of the factor. The bluer and darker the color of the straight line, the greater the contribution of the factor. The size of the circle indicates the relative concentration of PFAS in individuals of that species. Saunder’s gull (n=8), clamworm (n=5), bivalve (n=5), shrimp (n=6) and sediment (n=9).
The predicted trophic and bioaccumulation networks for Saunders’s gulls are shown in Supplementary Table S4 and visually displayed in Figure 5. According to the analysis of the biomass networks, the model grouped the nutrient flows of the system into seven frictional trophic levels (Figure 5A). The energy flow pathways for black-billed gulls were dominated by a grazing food chain, i.e. sediment-shellfish-shrimp-Saunders’s gulls, or sediment-shellfish-Saunders’s gulls (Figure 5A). Mussels and clams are the main food sources for black-billed gulls. Similar to the energy transfer, PFAS showed similar transfer characteristics in the food chain of Saunders’s gulls. The most prevalent groups in terms of fluxes and biomass are those that occupy lower trophic levels. Trophic levels and biomass values showed a negative correlation. In comparison, as trophic levels increased, so did the bioaccumulation values of PFAS. As a result, a biomagnification phenomenon was observed, as the network plot illustrates (Figure 5B). Notably, the estimated PFAS values in clamworm were higher than those in the other food sources for the gull.
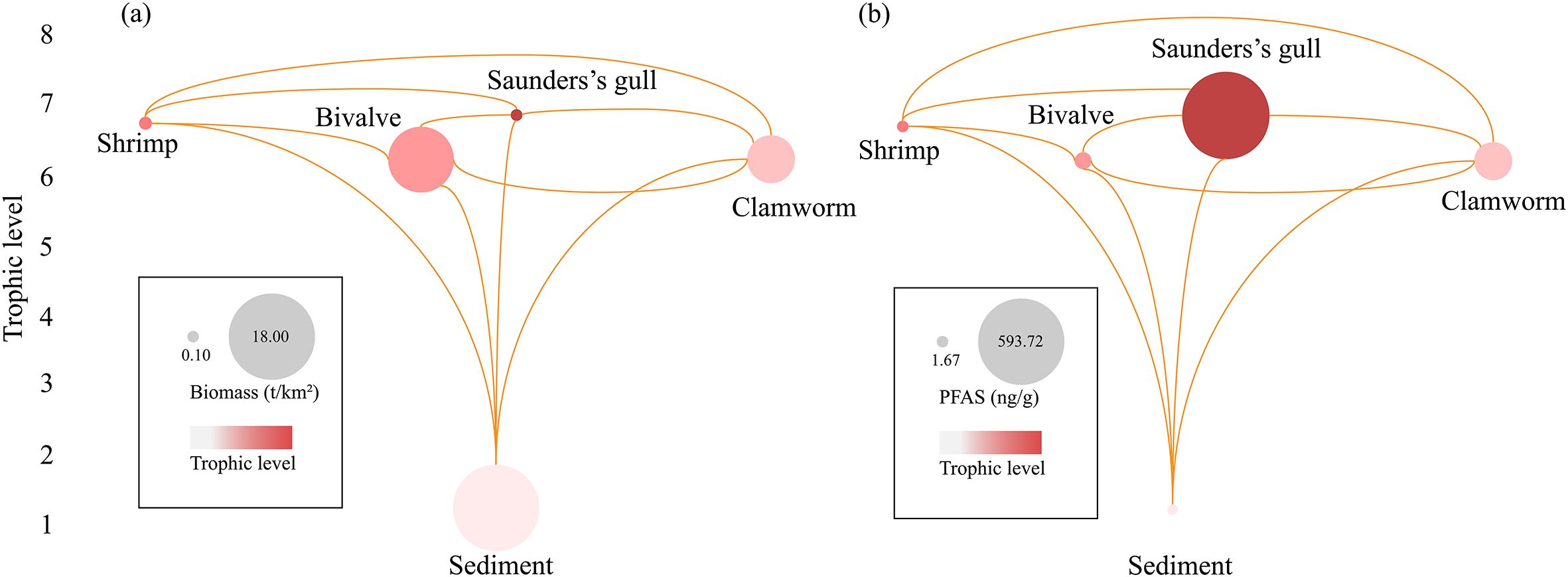
Figure 5. Estimated networks of trophic level and energy flow of Saunders’s gull biomass (A) and PFAS bioaccumulation (B). Nodes represent functional groups with size proportional to the biomass content (t/km2) (A) or PFAS concentration (ng/g) (B). Lines depict feeding connections, illustrating the flow of biomass (A) and contaminants (B) from prey to predator. (A, B) have the same fractional thermodynamic level, indicated by the left number.
4 Discussion
4.1 PFAS concentrations and composition profiles in organisms and the sediment
This results showed that the highest levels of PFAS (11.82–677.44 ng/g) were detected in the gull muscle, which were higher than those previously reported for other seabird species elsewhere (Supplementary Table S9) (Olivero-Verbel et al., 2006; Haukås et al., 2007; Chu et al., 2015; Chen et al., 2018; Robuck et al., 2021). The main detected species of PFAS in endangered gulls in China’s offshore regions were PFOA (Juvenile mean = 234.19 ng/g; Adult mean = 144.11 ng/g) and PFOS (Juvenile mean = 398.03 ng/g; Adult mean = 16.13 ng/g), which is aligning with our research outcomes. Previous reports indicated the lowest visible adverse effect level of PFOS was 100 ng/g of egg weight (Molina et al., 2006). Furthermore, a significant reduction in hatchability was observed in chicken (Gallus domesticus) embryos injected with 0.1 μg of PFOS per gram of egg, a concentration representative of environmentally relevant exposures (Molina et al., 2006).
In addition, the alternative PFBA was also detected at high concentrations, in line with the shifting trend in the production and use of PFAS in the coastal cities of eastern China from the traditional long-chain PFAS to the newer short-chain PFAS (Mahoney et al., 2022). The detection of high concentrations of PFOS, PFOA and PFBA in all samples can be attributed to the persistent nature of these compounds and their elevated environmental concentrations. This underscores the significant biomagnification potential of PFAS. The concentration pattern of PFAS in target species can be influenced by various biological factors such as size/age, feeding ecology, migration, and biotransformation. Accumulation of PFAS in seabirds may stem from compound retention; however, this phenomenon may also arise from the ability of PFAS to biotransform and eliminate other less persistent nature (Haukås et al., 2007).
4.2 Differences in PFAS enrichment levels between juvenile and adult gulls
In this study, it was observed that total PFAS muscle concentrations were significantly higher in juvenile gulls compared to adult gulls, particularly for long-chain PFAS such as c11-PFUnDA, c10-PFDA, c12-PFDoDA, and c8-PFOS. The toxic effects of PFOS and/or PFOA in juveniles may surpass those observed in adults, potentially leading to oxidative stress, disruptions in endocrine and metabolic processes, reduced hatching success or weight gain, and even mortality (Costantini et al., 2019). Some prior investigations have examined potential differences in PFAS concentrations between juvenile and adult animals. For instance, Buytaert et al. (2023) found no discernible difference in PFAS plasma concentrations between juvenile and adult great tits. Conversely, in mallards (Anas platyrhynchos), PFOS concentrations were observed to increase with age (Newsted et al., 2006). Bustnes et al. (2013) reported a positive correlation between PFAS levels and age in goshawks (Accipiter gentilis) and white-tailed eagle (Haliaeetus albicilla) nestlings, with PFOS plasma concentrations showing a marked increase over time. Furthermore, Dauwe et al. found that, given the mobility and wide rang of adult great tits, they may cover a greater region around the source of pollution and may be negatively impacted by local PFOS contamination, as suggested by the high levels of PFOS found in them. Great tit nestlings mostly eat food that has been obtained nearby the nesting site, therefore the PFOS concentrations in the nestlings more closely represent local pollution than the concentrations detected in the adults (Dauwe et al., 2007). Nevertheless, the limited sample size in our study constrains our ability to make definitive conclusions regarding these age-related differences. In addition, Ricolfi et al. (2024) found through meta-analysis that wild birds exhibit maternal to offspring transfer of perfluorinated compounds, and found that the concentration of PFAS in offspring was 41% higher than that in the mother. In this study, the total concentration of PFAS in juvenile birds was more than 50% higher than that in adult birds, indicating the possibility of maternal metastasis.
4.3 Transfer of PFAS in the food chain
Previous research has found that organisms higher in the food chain contain significantly higher concentrations of PFAS than those lower in the food chain, indicating the biomagnification effects of PFAS (Evich et al., 2022). Huang et al. (2022) conducted research to examine the presence and transfer dynamics of PFOS and short-chain PFBA within a typical terrestrial food chain, focusing on the plant-pika-eagle ecosystem in the Nam Co Basin of the Tibetan Plateau. Their findings revealed varying concentrations of PFOS and PFBA across different components of the food chain, showcasing a notable biomagnification trend. Grønnestad et al. (2019) conducted a study to analyze the presence, distribution, and biomagnification tendencies of PFOS in the soil, earthworms (Eisenia fetida), and voles (Myodes glareolus) within a ski resort situated in Wittenham. The results showed that the biomagnification factor of PFOS was greater than 1, but the concentration levels at higher trophic levels (such as top predators) might be much higher (Grønnestad et al., 2019). Ali et al. (2021) conducted a study to explore how PFAS uptake and fate are influenced by both abiotic factors (water, snow, sediment) and biotic factors (zooplankton, benthos, fish, crabs, gulls) in the marine ecosystem of an Arctic fjord near Longyearbyen (Svalbard, Arctic Norway). The concentration of PFAS increased with the trophic level from plankton to polychaetes, crabs, fish liver, and seagull liver (Ali et al., 2021).
Wetlands play a crucial role in ecosystem equilibrium, biodiversity conservation, and the preservation of endangered species. PFAS can have significant impacts on high ecological niche predators through bio-magnification, which may indirectly affect other species through the food chain. Saunders’s gull, as the species of focus selected for this study, is an important component of the wetland ecosystem, and the accumulation of PFAS in its body may have a knock-on effect on the entire ecosystem. For example, as a bio-indicator species, the health and reproductive status of gulls can reflect the overall “health” of the marine environment (Rajpar et al., 2018). Therefore, this pollution may not only affect the gulls themselves but also other species in the ecosystem where they are located.
Our results indicate that the concentrations of PFAS in the pectoral muscles of gulls are much higher than those in their food sources (clamworm, shrimp, and shellfish) and sediment in their habitats. This difference may be related to biological amplification, where the concentration of pollutants in organisms increases with the increase of nutrient levels in the food chain. As a high trophic level organism, the accumulation level of PFAS in gulls is particularly noteworthy. These findings reveal the transmission pathways of PFAS in the food chain and their accumulation characteristics in different ecological environments (Routti et al., 2015). In addition, the physiological characteristics and lifestyle habits of different biological species may also have an impact on the accumulation of PFAS (Ding et al., 2020).
The distribution of PFAS in environmental matrices is also influenced by various factors, including biological species, habitat characteristics, and pollution sources. For example, the distribution of PFAS in wetland ecosystems may be related to local industrial activities and pollution discharge (Zhong et al., 2021). This finding emphasizes the importance of gaining an in-depth understanding of the distribution patterns of PFAS in different environmental matrices, especially in developing effective environmental protection and pollution prevention strategies.
Overall, this study provides an important perspective for understanding the behavior of PFAS in the food chain and different environmental matrices. This is of great significance for evaluating the potential impact of PFAS on biodiversity and developing effective management measures for PFAS pollution. In addition, this work also provides a scientific basis for future environmental monitoring and ecological risk assessment.
4.4 Policy implications and habitat management
Based on this study, we identified several environmental problems in the coastal areas of China, including a high number of chemical enterprise parks along the coast, the lack of effective treatment measures for PFAS, and the low concern for PFAS in organisms (especially endangered species). Therefore, drawing from the findings of this study, we suggest the following recommendations.
First, formulation and improvement of relevant regulations are necessary. It is crucial to strengthen the development and refinement of legal frameworks, particularly those pertaining to environmental protection in specific areas. This includes integrating international conventions such as the Stockholm Convention to establish stricter and more detailed environmental regulations that cater to the unique environmental issues and ecological characteristics of the local region. For instance, specific provisions should be made at the local level for the protection of coastal wetlands, establishment of industrial emission standards, and regulation of pollutants. Moreover, these regulations should be routinely updated to address evolving environmental challenges and emerging contaminants.
Second, there is a need for timely monitoring of factory hazardous waste discharges. Strengthening the surveillance of discharge points at chemical plants is a vital strategy for controlling environmental pollution. This approach should encompass not only traditional chemical monitoring methods but also biological monitoring techniques such as using benthic organisms and birds as bio-indicators to assess environmental health. Biological monitoring offers direct insights into how pollution impacts ecosystems, aiding in the identification and tracking of pollution sources. Additionally, this enhances transparency in the discharge practices of chemical factories and raises public awareness about environmental pollution.
Third, further exploration and implementation of innovative environmental management technologies are required. By combining membrane technology and bioremediation, we can effectively filter and isolate pollutants, thereby naturally reducing the concentration of contaminants in the environment. These technologies are versatile, as they are applicable not only to water purification but also to soil remediation and air purification (Giorno, 2022), playing a crucial role in diminishing toxic substances in the environment. The development of bioremediation techniques is particularly vital for use in areas of critical biodiversity. These methods can minimize secondary pollution during the treatment process and offer significant means for managing pollution in protected areas and heritage sites.
Finally, the management of wetland protected areas and research on new types of pollutants will need to be strengthened. In particular, the scientific and systematic nature of wetland protected area management should be strengthened. This includes comprehensive research on wetland ecosystems and the development and implementation of scientific management plans to balance urbanization and ecological protection. To further our understanding of the effects of these contaminants, more study should be done on novel pollutant classes including PFAS. At the same time, public awareness of environmental protection and biodiversity conservation should be raised through public education and publicity campaigns to promote the participation of the whole society in environmental protection.
5 Conclusion
In this study, we detected the levels of 14 PFAS in Saunders’s gull and food chain samples collected from the Yellow Sea beach wetlands adjacent to the chemical plant. The concentrations of PFAS in gulls were greater than those previously reported for other local shore birds and varied considerably among individuals. The total PFSA level was significantly higher in the muscles of juvenile gulls than in adult gulls, suggesting that PFSA are transferred maternally and re-exposed through food after hatching. Total PFSA concentrations in all biological samples gradually increased when moving up the food chain, confirming PFSA food chain amplification. Long-chain PFOA, PFOS, and short-chain PFBA emerged as the primary substances found across multiple organisms, confirming the environmental persistence of long-chain PFAS and signaling a gradual transition in China’s PFAS production towards short-chain alternatives. It is important to acknowledge that we collected a limited sample size of Saunders’s gull and did not establish a toxicity reference value or lowest visible adverse effect level for the gull muscle, which could potentially constrain the interpretation of individual toxicity or biological/ecological impacts. Consequently, additional studies should be conducted to map these thresholds.
Data availability statement
The raw data supporting the conclusions of this article will be made available by the authors, without undue reservation.
Ethics statement
The animal study was approved by Jiangsu Wildlife Protection Station. The study was conducted in accordance with the local legislation and institutional requirements.
Author contributions
DZ: Data curation, Formal analysis, Writing – original draft. WL: Conceptualization, Data curation, Investigation, Writing – original draft. YX: Data curation, Investigation, Writing – original draft. XL: Supervision, Writing – review & editing. ZZ: Data curation, Formal analysis, Writing – original draft. YL: Supervision, Writing – review & editing.
Funding
The author(s) declare financial support was received for the research, authorship, and/or publication of this article. This work was supported by the Open Research Fund from Biodiversity Investigation, Observation, and Assessment Program of the Ministry of Ecology and Environment of China, State Environmental Protection Key Laboratory of Biodiversity and Biosafety, the National Natural Science Foundation of China (grant number 41961144022), and the Open Research Fund from Key laboratory of Marine Ecosystem Dynamic of the Ministry of Natural Resources of China (grant number CEEES2023010).
Conflict of interest
The authors declare that the research was conducted in the absence of any commercial or financial relationships that could be construed as a potential conflict of interest.
The author(s) declared that they were an editorial board member of Frontiers, at the time of submission. This had no impact on the peer review process and the final decision.
Publisher’s note
All claims expressed in this article are solely those of the authors and do not necessarily represent those of their affiliated organizations, or those of the publisher, the editors and the reviewers. Any product that may be evaluated in this article, or claim that may be made by its manufacturer, is not guaranteed or endorsed by the publisher.
Supplementary material
The Supplementary Material for this article can be found online at: https://www.frontiersin.org/articles/10.3389/fmars.2024.1467022/full#supplementary-material
Abbreviations
PFAS, perfluoroalkyl and polyfluoroalkyl substances; PFCAs, perfluorocarboxylic acids; PFSAs, perfluoroalkanesulfonic acids; PFBA, perfluorobutanoic acid; PFBS, perfluoro-1-butane sulfonic acid; PFDA, perluorodecanoic acid; PFDoDA, perfluorododecanoic acid; PFDS, perfluoro-1-decanesulfonate; PFHpA, perfluoroheptanoic acid; PFHxA, perfluorohexanoic acid; PFHxS, perfluoro-1-hexane sulfonic acid; PFNA, perfluorononanoic acid; PFOS, perfluorooctanesulfonic acid; PFPeA, perfluoro-n-pentanoic acid; PFUnDA, perfluoroundecanoic acid; PFOA, perfluorooctanoic acid; PFTeDA, perfluorotetradecanoic acid.
References
Ali A. M., Langberg H. A., Hale S. E., Kallenborn R., Hartz W. F., Mortensen Å.K., et al. (2021). The fate of poly- and perfluoroalkyl substances in a marine food web influenced by land-based sources in the Norwegian Arctic. Environ. Sci. Process Impacts. 23, 588–604. doi: 10.1039/d0em00510j
Bach C. C., Bech B. H., Brix N., Nohr E. A., Bonde J. P. E., Henriksen T. B. (2015). Perfluoroalkyl and polyfluoroalkyl substances and human fetal growth: a systematic review. Crit. Rev. Toxicol. 45, 53–67. doi: 10.3109/10408444.2014.952400
Bustnes J. O., Bårdsen B. J., Herzke D., Johnsen T. V., Eulaers I., Ballesteros M., et al. (2013). Plasma concentrations of organohalogenated pollutants in predatory bird nestlings: associations to growth rate and dietary tracers. Environ. Toxicol. Chem. 32, 2520–2527. doi: 10.1002/etc.2329
Buytaert J., Eens M., Elgawad H. A., Bervoets L., Beemster G., Groffen T. (2023). Associations between PFAS concentrations and the oxidative status in a free-living songbird (Parus major) near a fluorochemical facility. Environ. pollut. 335, 122304. doi: 10.1016/j.envpol.2023.122304
Chen C., Reniers G. (2020). Chemical industry in China: The current status, safety problems, and pathways for future sustainable development. Saf. Sci. 128, 104741. doi: 10.1016/j.ssci.2020.104741
Chen H., Han J., Cheng J., Sun R., Wang X., Han G., et al. (2018). Distribution, bioaccumulation and trophic transfer of chlorinated polyfluoroalkyl ether sulfonic acids in the marine food web of Bohai, China. Environ. pollut. 241, 504–510. doi: 10.1016/j.envpol.2018.05.087
Christensen V., Walters C. J. (2004). Ecopath with Ecosim: methods, capabilities and limitations. Ecol. Model. 172, 109–139. doi: 10.1016/j.ecolmodel.2003.09.003
Chu S., Wang J., Leong G., Woodward L. A., Letcher R. J., Li Q. X. (2015). Perfluoroalkyl sulfonates and carboxylic acids in liver, muscle and adipose tissues of black-footed albatross (Phoebastria nigripes) from Midway Island, North Pacific Ocean. Chemosphere 138, 60–66. doi: 10.1016/j.chemosphere.2015.05.043
Colomer-Vidal P., Bertolero A., Alcaraz C., Garreta-Lara E., Santos F. J., Lacorte S. (2022). Distribution and ten-year temporal trends, (2009–2018) of perfluoroalkyl substances in gull eggs from Spanish breeding colonies. Environ. pollut. 293, 118555. doi: 10.1016/j.envpol.2021.118555
Costantini D., Blévin P., Herzke D., Moe B., Gabrielsen G. W., Bustnes J. O., et al. (2019). Higher plasma oxidative damage and lower plasma antioxidant defences in an Arctic seabird exposed to longer perfluoroalkyl acids. Environ. Res. 168, 278–285. doi: 10.1016/j.envres.2018.10.003
Cui Q., Shi F., Pan Y., Zhang H., Dai J. (2019). Per- and polyfluoroalkyl substances (PFASs) in the blood of two colobine monkey species from China: Occurrence and exposure pathways. Sci. Total Environ. 674, 524–531. doi: 10.1016/j.scitotenv.2019.04.118
Dauwe T., Van de Vijver K., De Coen W., Eens M. (2007). PFOS levels in the blood and liver of a small insectivorous songbird near a fluorochemical plant. Environ. Int. 33, 357–361. doi: 10.1016/j.envint.2006.11.014
Dickman R. A., Aga D. S. (2022). A review of recent studies on toxicity, sequestration, and degradation of per- and polyfluoroalkyl substances (PFAS). J. Hazard Mater. 436, 129120. doi: 10.1016/j.jhazmat.2022.129120
Ding N., Harlow S. D., Randolph J. F. Jr., Loch-Caruso R., Park S. K. (2020). Perfluoroalkyl and polyfluoroalkyl substances (PFAS) and their effects on the ovary. Hum. Reprod. Update 26, 724–752. doi: 10.1093/humupd/dmaa018
Evich M. G., Davis M. J. B., McCord J. P., Acrey B., Awkerman J. A., Knappe D. R. U., et al. (2022). Per- and polyfluoroalkyl substances in the environment. Science 375, eabg9065. doi: 10.1126/science.abg9065
Falandysz J., Taniyasu S., Yamashita N., Rostkowski P., Zalewski K., Kannan K. (2007). Perfluorinated compounds in some terrestrial and aquatic wildlife species from Poland. J. Environ. Sci. Health A Tox Hazard Subst Environ. Eng. 42, 715–719. doi: 10.1080/10934520701304369
Giorno L. (2022). Membranes that filter and destroy pollutants. Nat. Nanotechnol. 17, 334–335. doi: 10.1038/s41565-021-01064-2
Grønnestad R., Vázquez B. P., Arukwe A., Jaspers V. L. B., Jenssen B. M., Karimi M., et al. (2019). Levels, patterns, and biomagnification potential of perfluoroalkyl substances in a terrestrial food chain in a nordic skiing area. Environ. Sci. Technol. 53, 13390–13397. doi: 10.1021/acs.est.9b02533
Haukås M., Berger U., Hop H., Gulliksen B., Gabrielsen G. W. (2007). Bioaccumulation of per- and polyfluorinated alkyl substances (PFAS) in selected species from the Barents Sea food web. Environ. pollut. 148, 360–371. doi: 10.1016/j.envpol.2006.09.021
Huang K., Li Y., Bu D., Fu J., Wang M., Zhou W., et al. (2022). Trophic magnification of short-chain per- and polyfluoroalkyl substances in a terrestrial food chain from the Tibetan Plateau. Environ. Sci. Technol. Lett. 9, 147–152. doi: 10.1021/acs.estlett.1c01009
Jaureguiberry P., Titeux N., Wiemers M., Bowler D. E., Coscieme L., Golden A. S., et al. (2022). The direct drivers of recent global anthropogenic biodiversity loss. Sci. Adv. 8, eabm9982. doi: 10.1126/sciadv.abm9982
Jiang H. X., Hou Y. Q., Chu G. Z., Qian F. W., Wang H., Zhang G. G., et al. (2010). Breeding population dynamics and habitat transition of Saunders's Gull Larus saundersi in Yancheng National Nature Reserve, China. Bird Conserv. Int. 20, 13–24. doi: 10.1017/S0959270910000017
Kannan K., Corsolini S., Falandysz J., Oehme G., Focardi S., Giesy J. P. (2002). Perfluorooctanesulfonate and related fluorinated hydrocarbons in marine mammals, fishes, and birds from coasts of the Baltic and the Mediterranean Seas. Environ. Sci. Technol. 36, 3210–3216. doi: 10.1021/es020519q
Ma Y., Choi C.-Y., Thomas A., Gibson L. (2022). Review of contaminant levels and effects in shorebirds: knowledge gaps and conservation priorities. Ecotoxicol. Environ. Saf. 242, 113868. doi: 10.1016/j.ecoenv.2022.113868
Ma Z., Choi C. Y., Gan X., Li J., Liu Y., Melville D. S., et al. (2023). Achievements, challenges, and recommendations for waterbird conservation in China's coastal wetlands. Avian Res. 14, 100123. doi: 10.1016/j.avrs.2023.100123
Mahoney H., Xie Y., Brinkmann M., Giesy J. P. (2022). Next generation per- and polyfluoroalkyl substances: status and trends, aquatic toxicity, and risk assessment. EcoEnviron. Health 1, 117–131. doi: 10.1016/j.eehl.2022.05.002
Molina E. D., Balander R., Fitzgerald S. D., Giesy J. P., Kannan K., Mitchell R., et al. (2006). Effects of air cell injection of perfluorooctane sulfonate before incubation on development of the white leghorn chicken (Gallus domesticus) embryo. Environ. Toxicol. Chem. 25, 227–232. doi: 10.1897/04-414r.1
Murray N. J., Marra P. P., Fuller R. A., Clemens R. S., Dhanjal-Adams K., Gosbell K. B., et al. (2018). The large-scale drivers of population declines in a long-distance migratory shorebird. Ecography 41, 867–876. doi: 10.1111/ecog.02957
Newsted J. L., Beach S. A., Gallagher S. P., Giesy J. P. (2006). Pharmacokinetics and acute lethality of perfluorooctanesulfonate (PFOS) to juvenile mallard and northern bobwhite. Arch. Environ. Contam. Toxicol. 50, 411–420. doi: 10.1007/s00244-005-1137-x
Olivero-Verbel J., Tao L., Johnson-Restrepo B., Guette-Fernández J., Baldiris-Avila R., O'byrne-Hoyos I., et al. (2006). Perfluorooctanesulfonate and related fluorochemicals in biological samples from the north coast of Colombia. Environ. pollut. 142, 367–372. doi: 10.1016/j.envpol.2005.09.022
Rajpar M. N., Ozdemir I., Zakaria M., Sheryar S., Rab A. (2018). Seabirds as bioindicators of marine ecosystems. Seabirds. London: Intech. ch. 4. doi: 10.5772/intechopen.75458
Ricolfi L., Taylor M. D., Yang Y., Lagisz M., Nakagawa S. (2024). Maternal transfer of per- and polyfluoroalkyl substances (PFAS) in wild birds: A systematic review and meta-analysis. Chemosphere 361, 142346. doi: 10.1016/j.chemosphere.142346
Robuck A. R., McCord J. P., Strynar M. J., Cantwell M. G., Wiley D. N., Lohmann R. (2021). Tissue-specific distribution of legacy and novel per- and polyfluoroalkyl substances in juvenile seabirds. Environ. Sci. Technol. Lett. 8, 457–462. doi: 10.1021/acs.estlett.1c00222
Routti H., Krafft B. A., Herzke D., Eisert R., Oftedal O. (2015). Perfluoroalkyl substances detected in the world’s southernmost marine mammal, the Weddell seal (Leptonychotes weddellii). Environ. pollut. 197, 62–67. doi: 10.1016/j.envpol.2014.11.026
Sonne C., Desforges J. P., Gustavson K., Bossi R., Bonefeld-Jørgensen E. C., Long M., et al. (2023). Assessment of exposure to perfluorinated industrial substances and risk of immune suppression in Greenland and its global context: a mixed-methods study. Lancet Planet Health 7, 570–579. doi: 10.1016/S2542-5196(23)00106-7
Stein C. R., McGovern K. J., Pajak A. M., Maglione P. J., Wolff M. S. (2016). Perfluoroalkyl and polyfluoroalkyl substances and indicators of immune function in children aged 12–19 y. Pediatr. Res. 79, 348–357. doi: 10.1038/pr.2015.213
Sun J., Fang R., Wang H., Xu D. X., Yang J., Huang X., et al. (2022). A review of environmental metabolism disrupting chemicals and effect biomarkers associating disease risks: Where exposomics meets metabolomics. Environ. Int. 158, 106941. doi: 10.1016/j.envint.2021.106941
Sun J., Xing L., Chu J. (2023). Global ocean contamination of per- and polyfluoroalkyl substances: A review of seabird exposure. Chemosphere. 330, 138721. doi: 10.1016/j.chemosphere.2023.138721
Sylvester F., Weichert F. G., Lozano V. L., Groh K. J., Bálint M., Baumann L., et al. (2023). Better integration of chemical pollution research will further our understanding of biodiversity loss. Nat. Ecol. Evol. 7, 1552–1555. doi: 10.1038/s41559-023-02117-6
Verreault J., Berger U., Gabrielsen G. W. (2007). Trends of perfluorinated alkyl substances in herring gull eggs from two coastal colonies in northern Norway: 1983-2003. Environ. Sci. Technol. 41, 6671–6677. doi: 10.1021/es070723j
Walters W. J., Christensen V. (2018). Ecotracer: analyzing concentration of contaminants and radioisotopes in an aquatic spatial-dynamic food web model. J. Environ. Radioactivity. 181, 118–127. doi: 10.1016/j.jenvrad.2017.11.008
Wang X., Jiang H. X., Zhang Y. N., Chen L. X., Song C. Z., Li Y. X. (2017). Diet composition of Saunders’s Gull (Larus saundersi) determined using stable isotope analysis at the Shuangtaihekou National Nature Reserve, China. Acta Ecol. Sin. 37, 1796–1804. doi: 10.1021/acs.est.9b06379
Wang W., Wang J. J., Zuo P., Li Y., Zou X. Q. (2019). Analysis of structure and energy flow in southwestern Yellow Sea ecosystem based on Ecopath model. J. Appl. Oceanogr. 38, 528–539. doi: 10.1007/s11356-021-16032-5
Wang X. L., Zhang Q. C., Zhao Z. Y., Song W. G., Cheng L., Yang J. H., et al. (2021). A multi-plug filtration (m-PFC) cleanup method based on carboxylic multi-walled carbon nanotubes for the detection of 14 perfluorinated compounds and dietary risk assessment of chicken, beef, and mutton collected from Shanghai markets. Food Control. 130, 108330. doi: 10.1016/j.foodcont.2021.108330
Wee S. Y., Aris A. Z. (2023). Environmental impacts, exposure pathways, and health effects of PFOA and PFOS. Ecotoxicol Environ. Saf. 267, 115663. doi: 10.1016/j.ecoenv.2023.115663
Yang Z., Lagassé B. J., Xiao H., Jackson M. V., Chiang C. Y., Melville D. S., et al. (2020). The southern Jiangsu coast is a critical moulting site for Spoon-billed Sandpiper Calidris pygmaea and Nordmann's Greenshank Tringa guttifer. Bird Conserv. Int. 30, 649–660. doi: 10.1017/S0959270920000210
Zhong H., Zheng M., Liang Y., Wang Y., Gao W., Wang Y., et al. (2021). Legacy and emerging per- and polyfluoroalkyl substances (PFAS) in sediments from the East China Sea and the Yellow Sea: Occurrence, source apportionment and environmental risk assessment. Chemosphere 282, 131042. doi: 10.1016/j.chemosphere.2021.131042
Keywords: shorebirds, food web, PFAS, pollution, threatened species
Citation: Zhang D, Liu W, Xin Y, Liu X, Zhang Z and Liu Y (2024) Trophic transfer of PFAS potentially threatens vulnerable Saunders's gull (Larus saundersi) via the food chain in the coastal wetlands of the Yellow Sea, China. Front. Mar. Sci. 11:1467022. doi: 10.3389/fmars.2024.1467022
Received: 19 July 2024; Accepted: 18 September 2024;
Published: 04 October 2024.
Edited by:
Kun Chen, Jiangsu University, ChinaReviewed by:
Dario Savoca, University of Palermo, ItalyJill Awkerman, United States Environmental Protection Agency (EPA), United States
Copyright © 2024 Zhang, Liu, Xin, Liu, Zhang and Liu. This is an open-access article distributed under the terms of the Creative Commons Attribution License (CC BY). The use, distribution or reproduction in other forums is permitted, provided the original author(s) and the copyright owner(s) are credited and that the original publication in this journal is cited, in accordance with accepted academic practice. No use, distribution or reproduction is permitted which does not comply with these terms.
*Correspondence: Wei Liu, bHdfZWNvbG9neUAxNjMuY29t; Yan Liu, bGl1eWFuQG5pZXMub3Jn
†These authors have contributed equally to this work