- 1Faculty of Biology and Chemistry & Center for Marine Environmental Sciences (MARUM), University of Bremen, Bremen, Germany
- 2Alfred Wegener Institute, Helmholtz Centre for Polar and Marine Research, Bremerhaven, Germany
- 3Max-Planck-Institute for Marine Microbiology, Bremen, Germany
- 4Institute of Biological Sciences, University of Rostock, Rostock, Germany
- 5Julius Kühn-Institute (JKI) – Federal Research Centre for Cultivated Plants, Brunswick, Germany
- 6Institute of Oceanology, Chinese Academy of Sciences, Qingdao, China
The Arctic is seasonally exposed to long periods of low temperatures and complete darkness. Consequently, perennial primary producers have to apply strategies to maximize energy efficiency. Global warming is occurring in the Arctic faster than the rest of the globe. The highest amplitude of temperature rise occurs during Polar Night. To determine the stress resistance of the ecosystem-engineering kelp Laminaria digitata against Arctic winter warming, non-meristematic discs of adult sporophytes from Porsangerfjorden (Finnmark, Norway) were kept in total darkness at 0°C and 5°C over a period of three months. Physiological variables, namely maximum quantum yield of photosynthesis (Fv/Fm) and dry weight, as well as underlying biochemical variables including pigments, storage carbohydrates, total carbon and total nitrogen were monitored throughout the experiment. Although all samples remained in generally good condition with Fv/Fm values above 0.6, L. digitata performed better at 0°C than at 5°C. Depletion of metabolic products resulted in a constant decrease of dry weight over time. A strong decrease in mannitol and laminarin was observed, with greater reductions at 5°C than at 0°C. However, the total carbon content did not change, indicating that the sporophytes were not suffering from “starvation stress” during the long period of darkness. A decline was also observed in the accessory pigments and the pool of xanthophyll cycle pigments, particularly at 5°C. Our results indicate that L. digitata has a more active metabolism, but a lower physiological and biochemical performance at higher temperatures in the Arctic winter. Obviously, L. digitata is well adapted to Arctic Polar Night conditions, regardless of having its distributional center at lower latitudes. Despite a reduced vitality at higher temperatures, a serious decline in Arctic populations of L. digitata due to winter warming is not expected for the near future.
1 Introduction
The Arctic is one of the regions that is changing most rapidly due to climate factors, feedback mechanisms and changes in energy transport towards the poles (Richter-Menge et al., 2017; Previdi et al., 2021). This results in 3.8 times faster warming in the Arctic than the global average (Rantanen et al., 2022), with the strongest temperature rise detected during the winter months (Wang et al., 2017; Maturilli et al., 2019). Generally, organisms populating the Arctic must have the ability to survive extreme abiotic conditions due to the strong seasonality (Zacher et al., 2009). For instance, Arctic kelps have to endure extreme photoperiodic conditions (Gattuso et al., 2020). In winter, they are exposed to very low temperatures and months-long complete darkness (Polar Night). Due to climate change, however, strong fluctuations in sea surface temperature (SST) have occurred in recent decades. For example, in Kongsfjorden, Svalbard, winter SST minima of −1.8°C were measured, while the maximum SST reached ~5°C (Huang et al., 2021; see Supplementary Material; Diehl et al., 2024).
Kelp forests are among the largest biogenic structures of marine benthic habitats and are highly productive ecosystems with structural complexity and phyletic diversity (Steneck et al., 2002; Wernberg et al., 2019). By definition, they are formed by brown algae of the order Laminariales, which dominate shallow rocky shores of temperate and Arctic regions. As sedentary organisms, kelps are particularly affected by changes in their environment and rising temperatures result in shifts in distribution and abundance of many species (Smale et al., 2019). On the one hand, new suitable and ice-free habitats are expected to increasingly appear in the future (Krause-Jensen et al., 2020; Castro de la Guardia et al., 2023). Changes in kelp abundance have already been observed in High Arctic regions (Bartsch et al., 2016; Düsedau et al., 2024). On the other hand, elevated temperatures generally stimulate the metabolic activity of organisms (Pörtner et al., 2005), and may therefore have a negative impact on the dark survival of kelps (Gordillo et al., 2022). Yet, the mechanisms of winter survival are still poorly understood and only a few studies have investigated acclimation of kelps to the Polar Night (Scheschonk et al., 2019; Gordillo et al., 2022; Summers et al., 2023; Diehl et al., 2024).
Kelps have evolved various mechanisms to acclimatize to abiotic variations in the environment (Hurd et al., 2014). Due to the seasonal photoperiods in the Arctic, growth and reproduction of most kelps species is limited to a short time in spring and summer, while in winter they undergo a “starvation mode” due to the lack of light for photosynthesis (Wiencke et al., 2009; Gordillo et al., 2022).
The vitality of kelps can be determined via fluorescence-based measurements of the maximum quantum yield of photosystem II (Fv/Fm), a common parameter for assessing the health and stress level of photosynthetic organisms, including macroalgae (Dring et al., 1996; Dring, 2006). As they are part of the cellular machinery for photosynthesis, pigment contents in some seaweeds decrease during Polar Night, when the metabolism of the organisms slows down to survive this period of total darkness (Wiencke et al., 2009). Moreover, the de-epoxidation state of the xanthophyll cycle pigments (DPS), an intracellular stress response, is affected by light and low temperatures (Fernández-Marín et al., 2011; Li et al., 2020; Monteiro et al., 2021). The carbohydrates mannitol and laminarin play a crucial role in surviving the Polar Night. Mannitol is the primary photosynthesis product and an important short-term storage carbohydrate in brown algae (Yamaguchi et al., 1966). In summer, when photosynthesis rates are high, mannitol is converted into the polysaccharide laminarin, the long-term storage carbohydrate (Johnston et al., 1977). In periods of darkness, laminarin can be reconverted into mannitol to maintain important metabolic functions (Yamaguchi et al., 1966; Johnston et al., 1977; Küppers and Kremer, 1978). We hypothesize that winter warming could accelerate the use of carbon reserves and increase the decomposition of the biomass. Monitoring dry weight and the total carbon content alongside quantifying mannitol and laminarin provides information on the consumption of carbon-containing metabolites as well as the storage carbohydrates.
Laminaria digitata (Hudson) J.V. Lamouroux is a broadly distributed cold–temperate to Arctic North Atlantic kelp growing on hard substrates in the sublittoral zone. In the East Atlantic, it is present from Southern Brittany to Spitsbergen (Lüning, 1990), where it survives up to four months of Polar Night. The species is described to survive and grow at temperatures as low as 0°C, with a temperature optimum at 10°C (Bolton and Lüning, 1982; tom Dieck (Bartsch), 1992). Recent studies showed a distinct decrease in digitate kelps (including L. digitata) in Arctic fjords (Düsedau et al., 2024), potentially in response to changes in the underwater light climate as a consequence of increased meltwater run-off (Schlegel et al., 2024). Liesner et al. (2020) showed that lower temperatures (5 vs. 15°C) led to a higher phenotypic plasticity, as well as higher growth rates of juvenile sporophytes, highlighting the importance of cold seasons for the survival of L. digitata and potential threats of climate change. Exposing L. digitata gametophytes to low temperatures (5 vs. 15°C) also facilitated a positive growth response from subsequent juvenile sporophytes at sub-optimal low (0°C) and warm (20°C) conditions (Gauci et al., 2022).
The aim of this study was to determine the resistance of L. digitata sporophytes to simulated temperature increases during the Polar Night in the High Arctic. Therefore, we kept discs from L. digitata sporophytes at temperatures of 0°C and 5°C in total darkness for three months. Various physiological and biochemical variables were monitored during the experiment. We hypothesized that higher temperatures during Polar Night would lead to an increased metabolism and therefore a reduced survival capacity of L. digitata.
2 Material and methods
2.1 Sampling and experimental design
Twenty adult sporophytes (~75–200 cm) of Laminaria digitata (Hudson) J.V. Lamouroux were collected at Porsangerfjorden (N 70°24’, E 25°32’; N 70°29’, E 25°39’; N 70°30’, E 25°42’), Finnmark in Northern Norway (Figure 1A). Individuals were sampled on July 12, 2022 at depths of 3–5 m, and stored in running seawater until July 20. Between 20 and 50 discs (ø 2.8 cm) per sporophyte were cut 10–40 cm above the meristem and kept moist, cool, and dark during transport. The experiment was conducted at the Alfred Wegener Institute in Bremerhaven, Germany. The samples arrived on July 22. After arrival, the discs were cultivated in a climate chamber at 5°C (± 0.5°C) and constant light (30 µmol photons m−2 s−1, λ ~380–700 nm, ProfiLux 3 with LED Mitras daylight 150, GHL Advanced Technology, Kaiserslautern, Germany) over three days for recovery (Figure 1B). After the recovery period, the subsamples from 12 sporophytes were distributed across the replicates, treatments and time points. To do so, 20 healthy discs (Dring et al., 1996; Fv/Fm >0.6 data not shown) from one individual each were used per replicate and treatment (n = 6), e.g. 0°C Replicate A. First biochemical sampling was conducted on August 01 (week 0 = “w0”). Therefore, five discs per replicate were randomly selected before the acclimation began, shock-frozen in liquid N2 and stored at −80°C until further processing. Then, the samples were maintained in two separate climate chambers (0°C and 5°C; ± 0.5°C) in the dark and the 0°C replicates were stepwise acclimated from 5°C to 0°C. Over a period of three months, three more biochemical samplings were conducted in the same way (September 02: “w4”, September 30: “w8”, October 27: “w12”). During the experiment, each replicate was kept in an aerated 2 L clear plastic bottle containing 1/40 Provasoli-enriched seawater [1/40 PES, 13.7 µmol NO3− L−1; 0.55 µmol PO43− L−1] in total darkness (0 µmol photons m−2 s−1), simulating Polar Night conditions in this High Arctic fjord Kongsfjorden, Svalbard (Bischof et al., 2019). Water was exchanged twice a week.
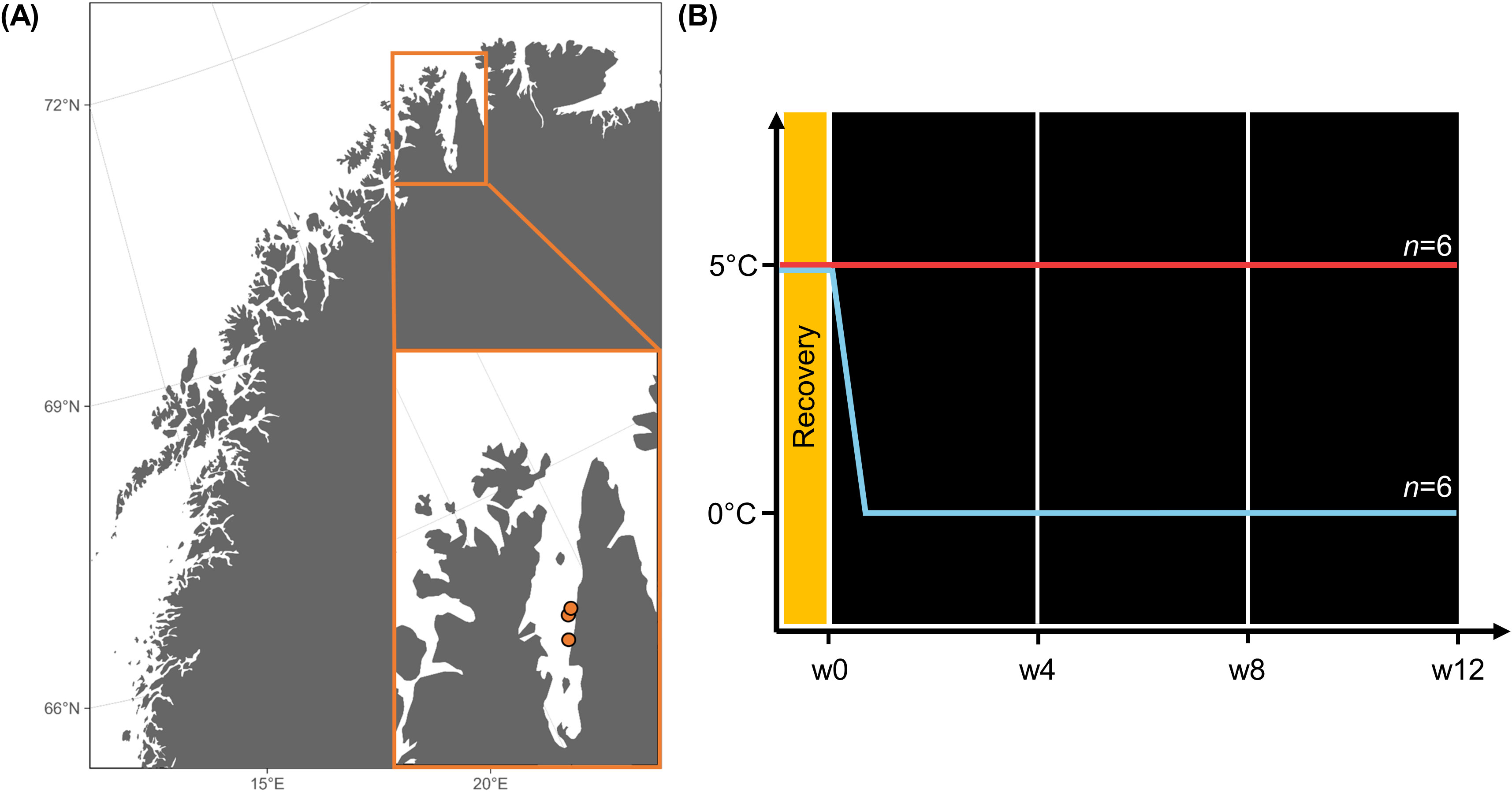
Figure 1. (A) Map of Northern Norway and Porsangerfjorden (Finnmark). The orange dots mark the sampling locations of Laminaria digitata. (B) Experimental setup. Week 0 (w0), w4, w8, and w12: Biochemical sampling during the experiment. Map was created with ggOceanMaps (Vihtakari, 2024).
2.2 Species identification
As discrimination between digitate Laminaria digitata, Hedophyllum nigripes and Laminaria hyperborea is difficult based on morphology alone (Longtin and Saunders, 2015; Dankworth et al., 2020), we genetically identified the collected specimens. Genomic DNA was extracted from subsamples, which were stored in silica gel, using a plant genomic DNA extraction kit (DP305, Tiangen Biotech, China) following the manufacturer’s instructions. Species were identified according to the method of Mauger et al. (2021), which involves amplifying a fragment of the mitochondrial COI gene (COI-5P). In short, two PCR reactions (PCR1 and PCR2) were conducted using a Taq Master Mix kit (Accurate Biology, China) and a T-gradient thermocycler (Biometra, Germany), with the primers and programs outlined in Mauger et al. (2021). PCR products were separated by electrophoresis, and then stained with GelRed and visualized under UV light. The amplified fragment patterns were compared with Mauger et al. (2021) to identify species.
2.3 Physiological response variables
Maximum in vivo chlorophyll-fluorescence of photosystem II (Fv/Fm) was measured weekly, using a pulse-amplitude-modulated fluorometer (Imaging-PAM, Walz GmbH Mess- und Regeltechnik, Effeltrich, Germany) to assess algal vitality. The I-PAM was set up to determine the initial amplitude of the fluorescence signal (Ft) between 0.15 and 0.2 (Int. 4, Gain 4, Damp. 4, SP 8, Width 0.8 s).
Pictures of the samples were taken every two weeks against a white background to exclude potential effects of the discs’ size on dry weight (DW). A ruler was included in the pictures for reference. Areas of the discs (cm2) were determined using ImageJ (Version 1.54d, Java 1.8.0_345, Wayne Rasband, National Institute of Health, USA). For monitoring the DW (w0, w4, w8, w12), samples were freeze-dried (Alpha 1–4 LO plus, Martin Christ Gefriertrocknungsanlagen GmbH, Osterode am Harz, Germany) and then weighed.
2.4 Biochemical response variables
Total carbon (Total C) and nitrogen (Total N) were analyzed following the protocol of Graiff et al. (2015). 2–3 mg of freeze-dried samples were weighed into tin cartridges and incinerated at 950°C in an elemental analyzer (Vario EL III, Elementar). Acetanilide was used as a standard (Verardo et al., 1990).
Mannitol content was determined following the methods of Diehl et al. (2020). In short, 1 mL of 70% ethanol was added to 10–15 mg of freeze-dried samples and incubated at 70°C for three to four hours in a water bath. The samples were then centrifuged for 5 min at 13,000 rpm. 800 μL of the supernatant was transferred and evaporated to dryness. The pellets were re-dissolved in ultrapure water (0.055 µS/cm) by vortexing and ultrasonication. Resuspended samples were then centrifuged for 5 min at 13,000 rpm. Mannitol content in the supernatants was determined following the protocol of Karsten et al. (1991). A High Performance Liquid Chromatography system (HPLC; Agilent Technologies 1200 series, Santa Clara, California, USA) was used with a guard cartridge (Phenomenex, Carbo-Pb2+ 4 x 3.00 mm I.D.) and an analytical Aminex Fast Carbohydrate Analysis Column (100 х 7.8 mm, 9 μm, BioRad, Munich, Germany) using ultrapure water as mobile phase. Calibration standards contained 0.5, 1.0, 2.5, 5.0 and 10.0 mM mannitol. Initial (w0) values were set to 100% and other samples to percentage of the initial.
Laminarin content was quantified following the methods of Becker et al. (2017) and Becker and Hehemann (2018). Laminarin was first extracted from 35–65 mg of freeze-dried material with 50 mM 3-(N-morpholino)propanesulfonic acid (MOPS) buffer (pH 7.0) at 4°C for 5 h (Scheschonk et al., 2019). Three recombinantly expressed laminarinases (FbGH30, FaGH17A and FbGH17A) were used to specifically hydrolyze laminarin to glucose (Becker et al., 2017). The reducing ends of sugars were quantified via the PAHBAH assay (Lever, 1972). The concentration of laminarin in each sample was calculated by comparison to non-hydrolyzed samples and calibration against a standard curve of Laminaria digitata laminarin (Sigma-Aldrich) processed in the same way as the extracts. Calibration concentrations were 7.8125, 15.625, 31.25, 62.5, 125, 250 and 500 µg mL−1. Concentrations were converted to mg of laminarin per mg DW.
Pigments were analyzed following the methods of Koch et al. (2015). 45–55 mg of freeze-dried samples were extracted in 1 mL 90% fridge-cold acetone at 4°C in darkness for 24 h. The filtered supernatant was analyzed by HPLC (LaChromElite® system, L-2200 cooled autosampler, DA-detector L-2450; VWR Hitachi International). Pigments were separated on a Spherisorb® ODS-2 (250 × 4.6 mm, 5 μm; Waters) column following the gradient from Wright et al. (1991). Reference standards were laboratory standard solutions (DHI Lab Products) of chlorophyll a (Chl a), chlorophyll c2 (Chl c2), fucoxanthin (Fuco), violaxanthin (V), antheraxanthin (A) and zeaxanthin (Z). The accessory pigment pool (Acc; Chl c2 + Fuco) as well as the pool size of the xanthophyll cycle pigments (VAZ; V+A+Z) were determined in μg g−1 DW. The ratios of VAZ:Chla and Acc:Chla were calculated to detect modulations in the photosynthetic apparatus. In addition, the de-epoxidation-state of the xanthophyll cycle (DPS), describing the process of converting V via A to Z, which reduces intracellular stress by dissipating excess energy (Wiencke and Bischof, 2012), was calculated after Colombo-Pallotta et al. (2006).
2.4 Statistics
As initial Fv/Fm values (w0) differed significantly between 0°C and 5°C, Fv/Fm and all other variables were displayed as “% of w0”.
“R” version 4.2.2 (R Core Team, 2022) was used for statistical evaluation. First, extreme outliers (Bonferroni, p < 0.05) were excluded. Normal distribution and homogeneity of variances were checked for all datasets using a Shapiro-Wilk test (p > 0.05) and Levene’s test (p > 0.05) respectively (Zuur et al., 2013). Data were also checked visually for normal distribution. If data appeared normally distributed, they were not transformed, regardless of the Shapiro-Wilk test statistic. The F-statistic for small sampling sizes is robust to a moderate deviation from the normal distribution (Blanca et al., 2017). Variation in Fv/Fm and area of all discs were examined using repeated unifactorial ANOVAs followed by post hoc Tukey tests. All other variables measured for the subsampled discs were assessed with two-factorial ANOVAs followed by post hoc Sidak tests, due to multiple pairwise comparison. When interpreting the data, it must be taken into account that the samples were cultivated in two different climate chambers, so that the temperature treatments contain a certain chamber effect. All statistical evaluations of the physiological and biochemical response variables are summarized in Supplementary Table S2.
3 Results
3.1 Species identification
Based on genetic data, the collected specimens were confirmed to be Laminaria digitata (Hudson) J.V. Lamouroux (Supplementary Figure S1).
3.2 Physiological response variables
The physiological vitality of Laminaria digitata, measured as the maximum quantum yield of photosystem II (Fv/Fm as % of w0; Figure 2 was strongly affected by the temperature treatments (0°C > 5°C, p < 0.001). Within each temperature treatment there were no significant changes over time. Fv/Fm was similar at both temperature treatments up to w6. From w8 onwards vitality of samples at 0°C increased slightly, while a trend toward decreasing vitality was observed for samples at 5°C. Throughout the entire experiment, Fv/Fm (raw data as “absolute values”) in both treatments remained above 0.6 (Supplementary Table S1).
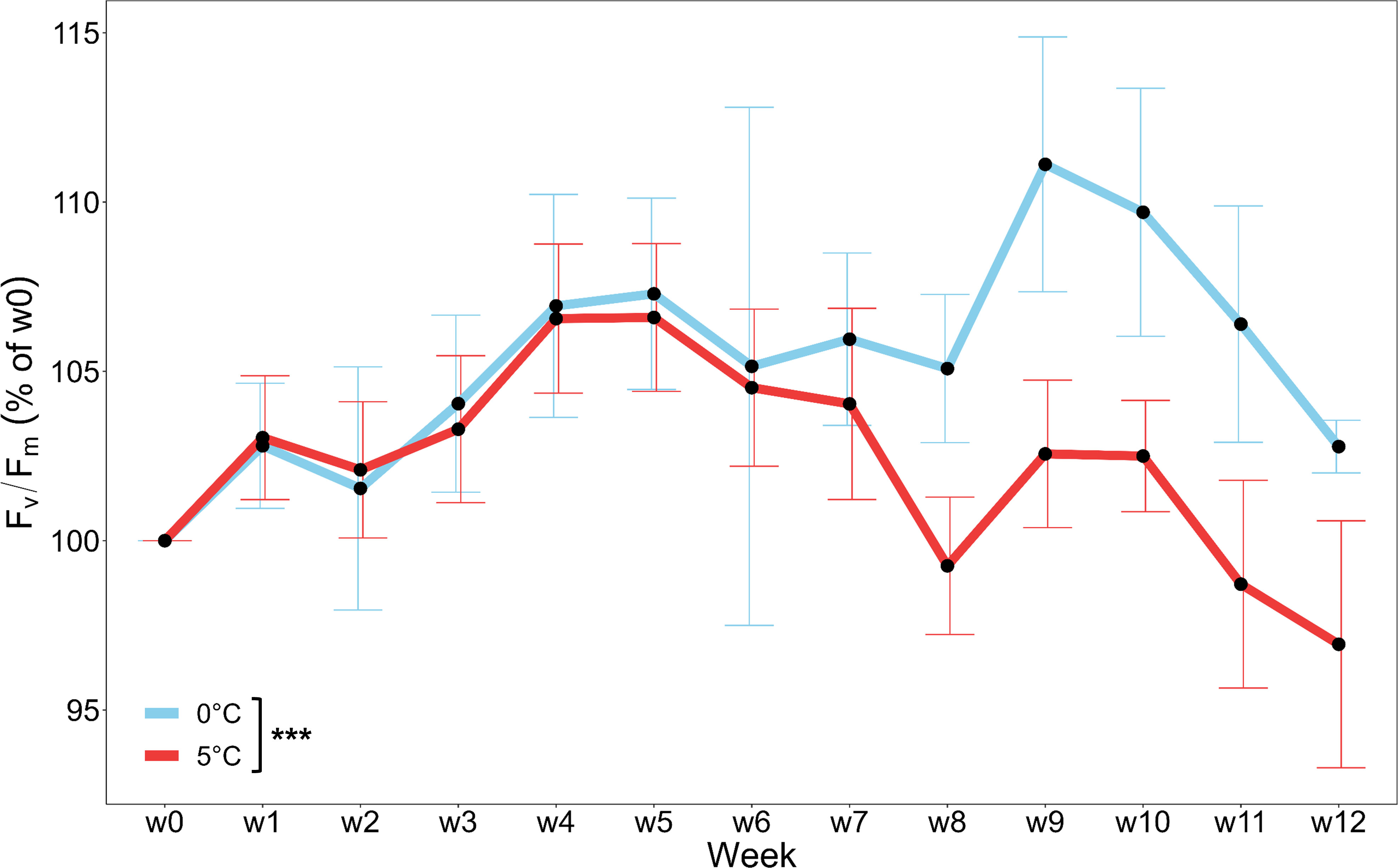
Figure 2. Vitality (maximum quantum yield of photosystem II; Fv/Fm) of Laminaria digitata, monitored weekly over three months under Polar Night conditions at 0°C (blue) and 5°C (red). Values are given as % of week 0 (w0) and means ± SD (n = 6). Significances between temperatures are indicated by black asterisks (p < 0.001***).
Although dry weight (DW as % of w0; Figure 3A) of the freeze-dried samples decreased significantly between w0 and w12 for pooled 0°C and 5°C (p < 0.01), no significant weight loss over time was determined for 0°C and 5°C when considered individually. Time-integrated DW did not differ between the temperature treatments.
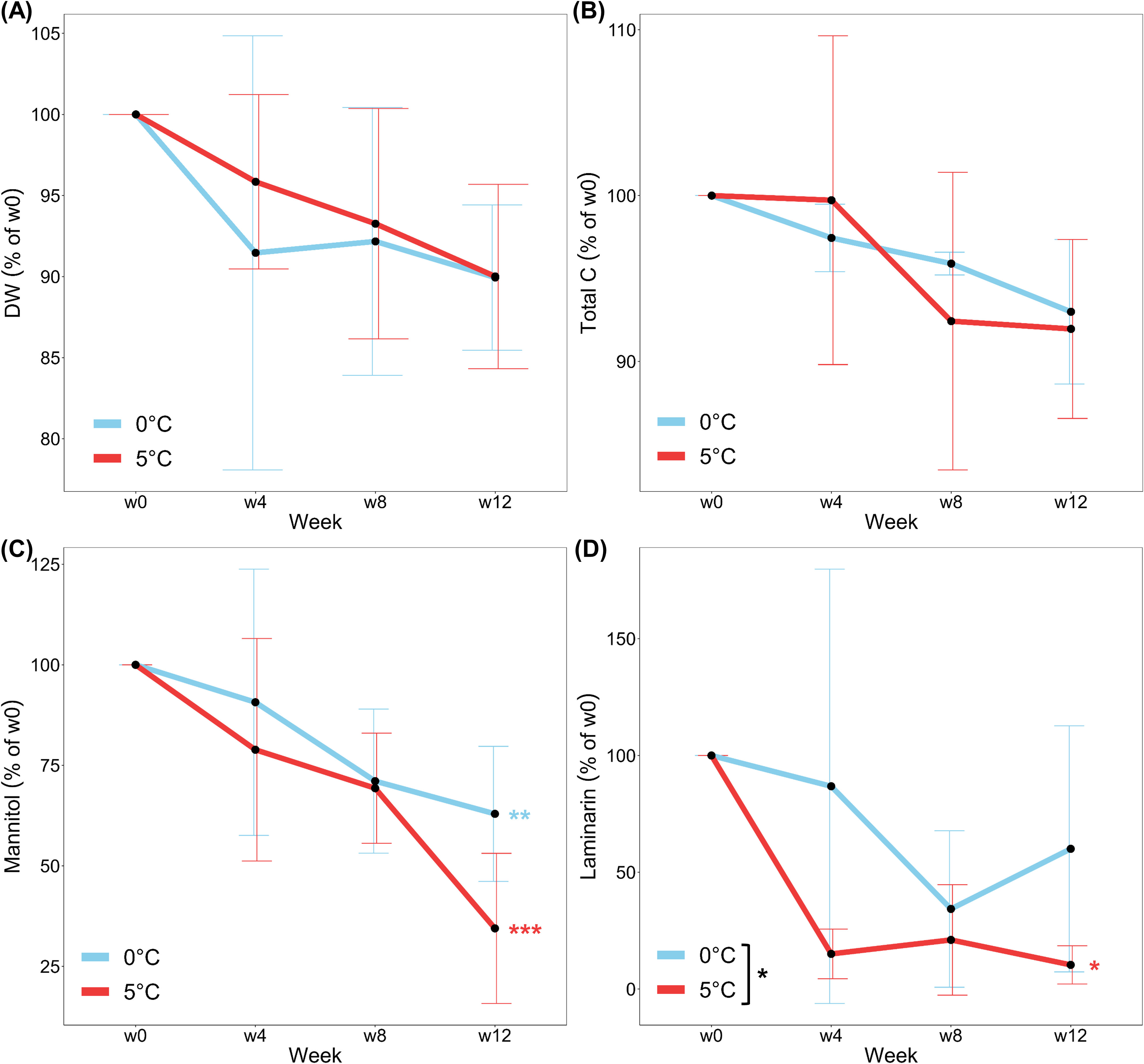
Figure 3. (A) Dry weight (DW) (B) Total carbon (Total C), (C) Mannitol and (D) Laminarin of Laminaria digitata, monitored every four weeks over three months under Polar Night conditions at 0°C (blue) and 5°C (red). Values are given as % of week 0 (w0) and means ± SD (n = 6). Significances between temperatures are indicated by black asterisks (p < 0.05*). Time-integrated significances between w0 and w12 within each temperature are marked by blue and red asterisks (p < 0.01**, p < 0.001***).
3.3 Biochemical response variables
The ratio between carbon and nitrogen (C:N as % of w0; Table 1) in the samples was affected by the temperature treatments (p < 0.05),decreasing significantly from w0 to w12 in samples at 5°C (p < 0.01) but not in samples at 0°C. These changes reflect higher total nitrogen contents (Total N) toward the end of the experiment. Total N (% of w0; Table 1) increased significantly over time at 5°C (p < 0.01), whereas no significant differences were measured for total carbon content (Total C; Figure 3B) of the samples at both temperature treatments. While not statistically significant, trend towards decreasing Total C over time were observed for both temperature treatments. Comparison of the raw data (absolute values) of C:N (Supplementary Table S1) revealed that ratios were slightly above 20 at the beginning of the experiment and declined to 17.9 ± 1.9 (0°C, p = 0.29) and 13.4 ± 0.6 (5°C, p < 0.01) at w12.
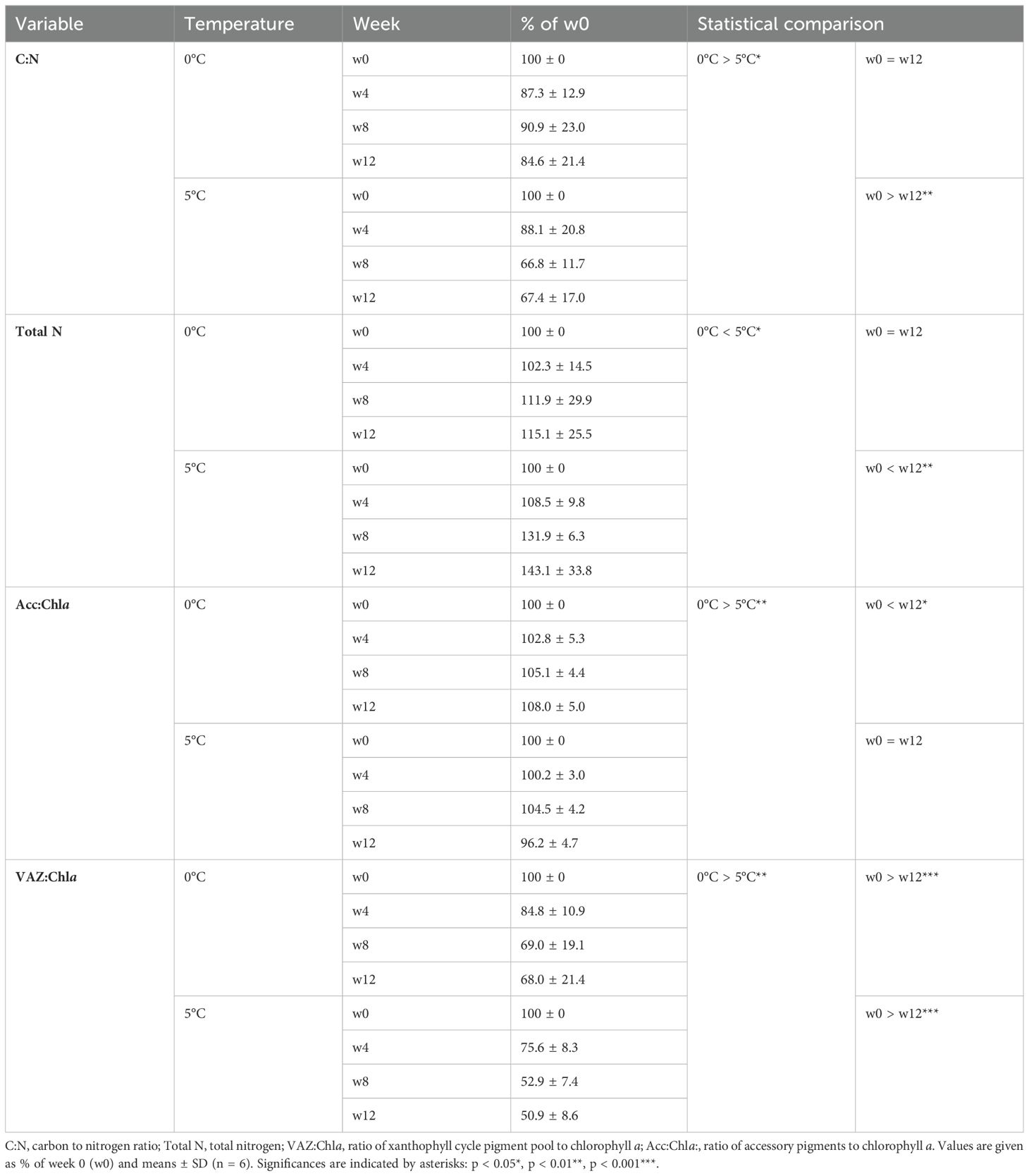
Table 1. Biochemical variables of Laminaria digitata monitored over three months under Polar Night conditions at 0°C and 5°C.
Mannitol content (% of w0; Figure 3C) decreased significantly over time (0°C: p < 0.01; 5°C: p < 0.001) in both treatments. While there was no significant difference between the two temperature treatments, a trend was observed to lower mannitol concentrations at 5°C compared to 0°C. Laminarin content (% of w0; Figure 3D) differed significantly between the temperature treatments (p < 0.05), with lower concentrations measured in samples at 5°C than at 0°C. Significant changes over time were only found for samples at 5°C (w0 > w12; p < 0.05).
Content of Chla, Acc and VAZ (% of w0; Figures 4A–C) in samples decreased over time. Trends to higher concentrations in samples at 0°C than in samples at 5°C were observed for all pigments. Chla, depleted significantly from w0 to w12 of the experiment when treatments were pooled (p < 0.05; Figure 4A). when considering treatments individually there was no significant decrease in Chla over time for either 0°C or 5°C. for a significant reduction in Acc content (% of w0; Figure 4B) was only measured at 5°C (p < 0.05), resulting in significant differences between the temperature treatments (p < 0.05). The greatest effects of sampling time and temperature were detected for VAZ, which was significantly depleted in samples from w0 to w12 at both temperatures (p < 0.001; Figure 4C) and was significantly higher in samples at 0°C than in samples at 5°C (p < 0.01). No significant changes between w0 and w12 were observed in the relative (% of w0; Figure 4D) or absolute (Supplementary Table S1) values of DPS. While temperature had no significant effect on relative DPS, absolute DPS values were significantly higher at 0°C than at 5°C (p < 0.001) at the end of the experiment. Acc:Chla (% of w0; Table 1) increased over time (p < 0.05) at 0°C, while VAZ:Chla (% of w0; Table 1) decreased over time at both temperature treatments (p < 0.001), with lower values at 5°C (p < 0.01).
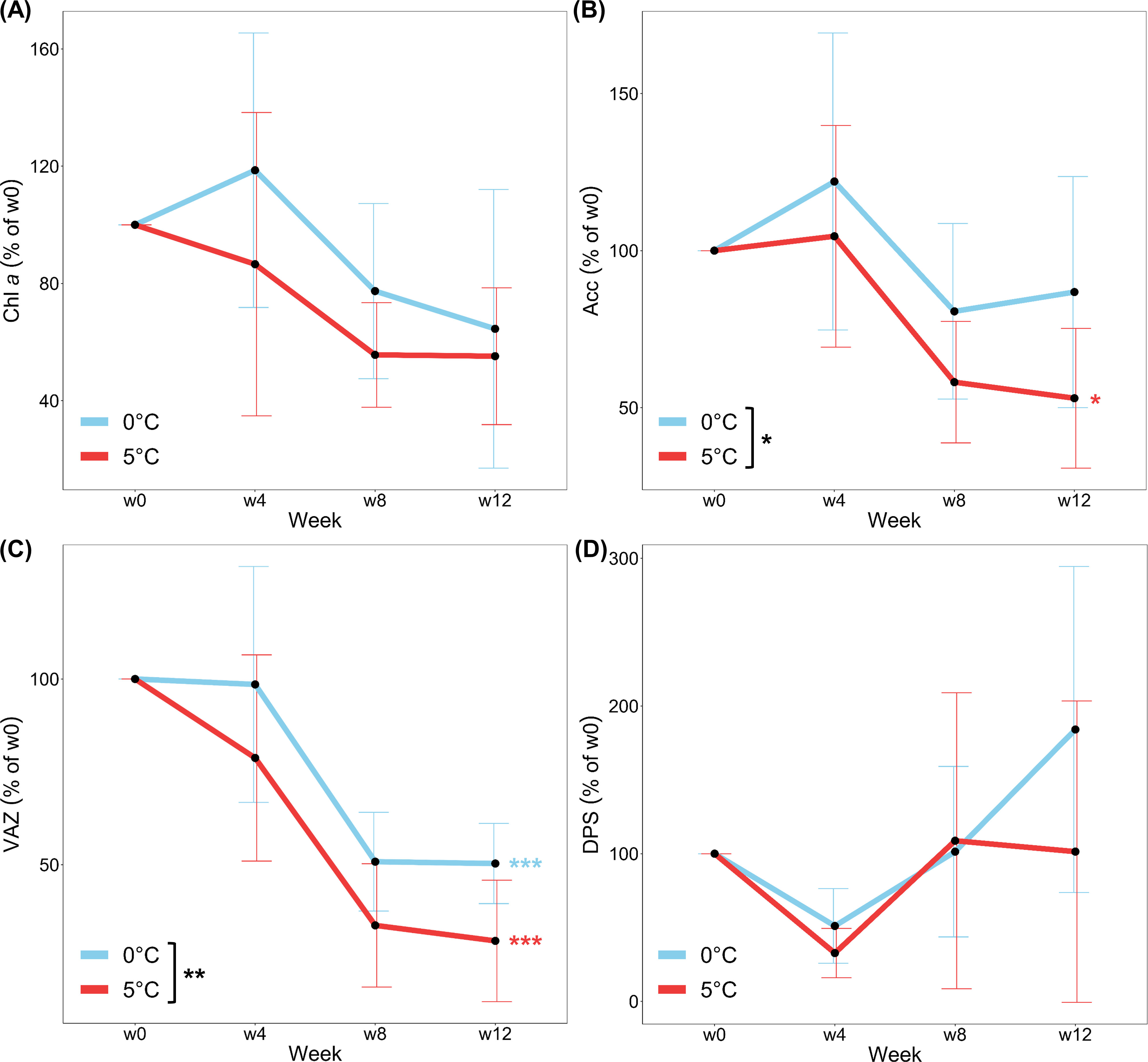
Figure 4. (A) Chlorophyll a (Chl a) (B) Accessory pigments (Acc) (C) Pool of xanthophyll cycle pigments (VAZ) and (D) De-epoxidation state of the xanthophyll cycle pigments (DPS) of Laminaria digitata, monitored every four weeks over three months under Polar Night conditions at 0°C (blue) and 5°C (red). Values are given as % of week 0 (w0) and means ± SD (n = 6). Significances between temperatures are indicated by black asterisks (p < 0.05*, p < 0.01**). Time-integrated significances between w0 and w12 within each temperature are marked by blue and red asterisks (p < 0.05*, p < 0.001***).
4 Discussion
This study determined the impact of Arctic winter warming on Laminaria digitata sporophytes in a simulation of High Arctic Polar Nights. Over a period of three months in darkness, physiological variables and underlying biochemical metabolites were monitored in samples maintained at 0°C and 5°C. The two temperature treatments represented the mean and the maximum winter temperatures that have already been measured in the High Arctic, for example in Kongsfjorden, Svalbard (Huang et al., 2021; see Supplementary Material; Diehl et al., 2024). From our results (Figure 5), it can be deduced that L. digitata sporophytes from Arctic regions are well adapted to prolonged darkness regardless of temperature and do not reach the end of Polar Night with a considerable lack of storage reserves and pigments.
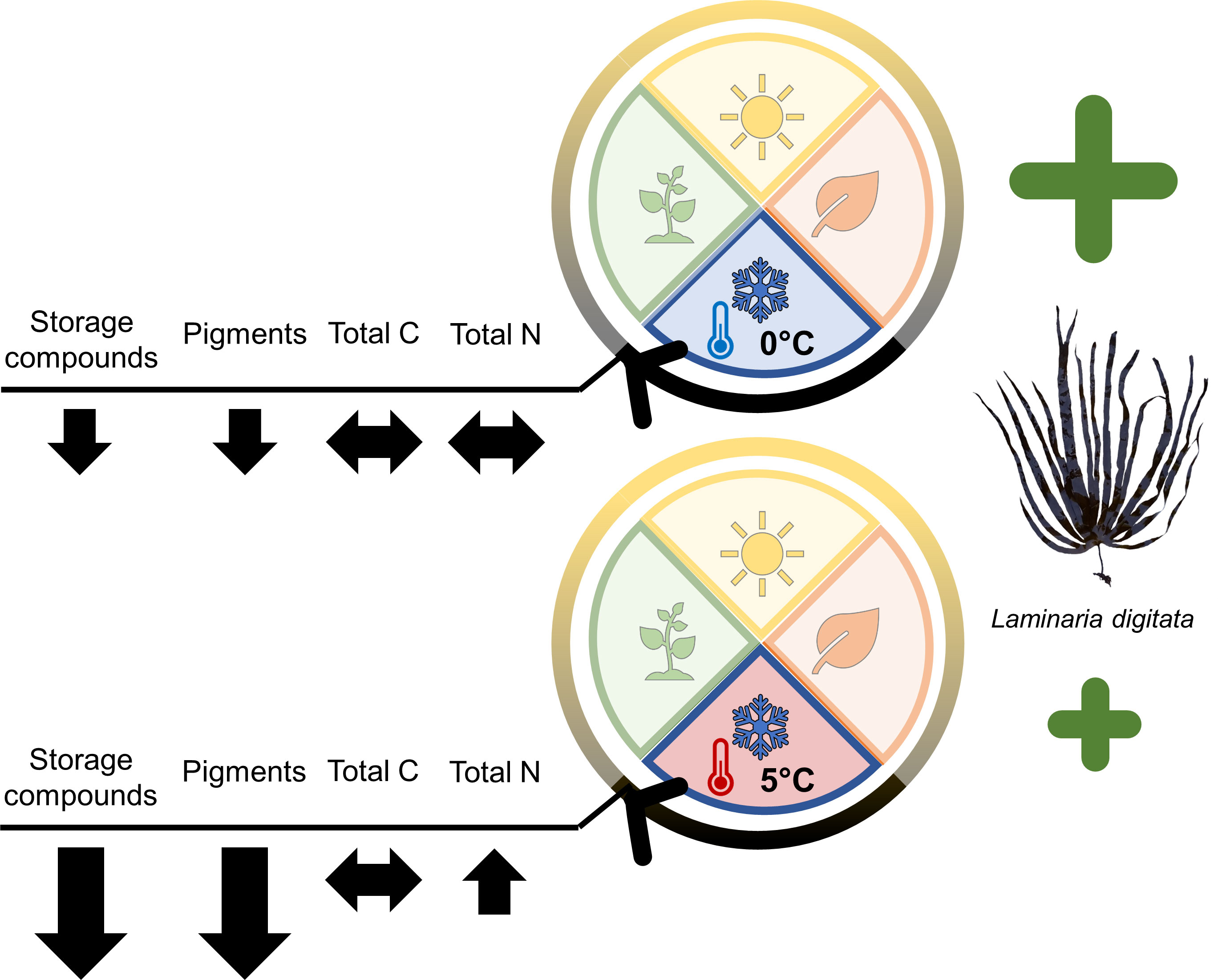
Figure 5. Summary of the biochemical acclimation strategies by Laminaria digitata sporophytes to Arctic winter warming during three months of Polar Night. Picture of L. digitata by D. Liesner.
Relative changes in vitality indicated that L. digitata performed better at 0°C compared to 5°C. Yet, a certain chamber effect in combination with the temperature treatments cannot be ruled out as the samples were maintained in two different climate chambers. Relative Fv/Fm values increased for samples at 0°C and decreased for samples at 5°C from week 8 onwards. However, absolute Fv/Fm values were never below 0.6, so all samples could be considered vital for the duration of the experiment (Dring et al., 1996). Laminariales have been observed to maintain a good vitality after long periods of darkness before (Scheschonk et al., 2019; Gordillo et al., 2022; Summers et al., 2023; Diehl et al., 2024).
The degradation of metabolites during prolonged darkness was reflected in a continuous loss in DW over time, as has also been observed for other kelps (Gordillo et al., 2022). However, contrary to previous data on Saccharina latissima and Alaria esculenta, weight loss in L. digitata did not increase at enhanced temperatures (3°C vs. 8°C; Gordillo et al., 2022). By visually observing the discs and monitoring disc area every two weeks, we were able to exclude any impact of decomposition on the DW (Supplementary Table S1; Supplementary Figure S2).
During polar winter kelps rely on accumulated energy stores, and must therefore be particularly energy efficient (Wiencke et al., 2009). In times of prolonged darkness, kelp derive mannitol from stocks of laminarin that they replenished during summer (Johnston et al., 1977; Küppers and Kremer, 1978). Accordingly, mannitol can be considered the main metabolite for respiration during the Polar Night. It must be taken into account that the storage carbohydrate content varies between the meristem and the distal part of the sporophyte (Scheschonk et al., 2019). By conducting the experiment with subsamples taken from the central part of the phylloid, we aimed to eliminate any impact of these variations. Mannitol content of L. digitata decreased by 37% (0°C) and 65% (5°C) over the three-month experiment, while laminarin decreased by 40% and 90% respectively. When comparing energy stocks in the cold–temperate kelp S. latissima from Kongsfjorden (Svalbard), Scheschonk et al. (2019) measured comparably high declines of mannitol and laminarin between October and February. They concluded that the strong reduction (>90%) of laminarin over the Polar Night maintains the metabolic functions of the kelp in that period. Additionally, lower laminarin concentrations were measured in L. digitata at higher temperatures during an experimental Polar Night treatment, as also observed in S. latissima (Scheschonk et al., 2019). The faster consumption of storage carbohydrates at higher temperatures suggests an increased energy requirement of L. digitata at 5°C. An even stronger rise in winter temperatures in the future could completely deplete L. digitata energy stocks before the end of the Polar Night and thus might lead to starvation or extensive stress. A faster consumption of storage carbohydrates with increasing temperatures during Polar Night was not observed for Laminaria hyperborea (Diehl et al., 2024). The different responses of Laminariales to winter warming will presumably have an impact on seaweed diversity and species abundance in the High Arctic in the future.
Nonetheless, despite the almost complete depletion of laminarin stocks at 5°C, L. digitata was not exposed to a “starvation stress” during three months of total darkness, as seen in stable total carbon content (Total C) over the three-month experiment. Although tendencies towards decreasing Total C content were observed in the samples, the degradation was less than 10% and independent from temperature. Consequently, L. digitata must have developed a strategy to preserve C during the Polar Night period. For S. latissima, also no changes in Total C were measured after four months of darkness (Gordillo et al., 2022). We assume that at complete darkness similar processes take place in L. digitata as have been described for the growth phase, when Laminariales remobilize and utilize their storage carbohydrates for energy generation (Gómez and Huovinen, 2012). Laminarin is first transformed into mannitol. Subsequently, mannitol is degraded stepwise to generate cellular energy, releasing CO2 which can be directly recovered by the enzyme phosphoenolpyruvate carboxykinase (PEP-CK) (Gómez and Huovinen, 2012). Comparable to the mechanism of dark carbon fixation (Wiencke et al., 2009), this allows an efficient recycling of every available C molecule to the kelp. Since we worked with non-meristematic tissues of L. digitata and the total C content was unaffected throughout the experiment, we assume that L. digitata uses the same strategy employed to restore C in times when photosynthesis is not possible. Our samples were taken in July during Polar Day, hence with almost “full carbohydrate stores” (Singh et al., 2024). We conclude that the carbohydrate reserves accumulated over the summer are sufficient to maintain the physiological functions of L. digitata during Polar Nights in future warming scenarios, as reflected in high Fv/Fm values at enhanced temperatures.
This conclusion is further supported by an observed increase in total nitrogen content (Total N) during the experiment. High environmental N availability in winter, which exceeds the N demand for protein and amino acid synthesis, enables and regulates the remobilization of carbon stocks in Laminariales (Gómez and Huovinen, 2012). We kept our samples under realistic Arctic winter nutrient conditions (~14 µmol NO3− L−1; Rokkan Iversen and Seuthe, 2011) and observed an increase in Total N over time, with significant increases at 5°C of about 40%. Potentially, a higher metabolic and enzymatic activity at 5°C enabled enhanced N uptake (Harrison and Hurd, 2001). The stable Total C and increased Total N contents led to C:N ratios below 20 towards the end of the experiment, showing that the samples were not N-limited at both temperatures (Atkinson and Smith, 1983).
The pigment content of seaweeds responds to metabolic processes and light availability (Blain and Shears, 2019), while metabolism and enzyme activity are in turn dependent on temperature (Daniel et al., 2008). Polar red algae are known to strongly adjust their pigments concentrations during the seasonal cycle, degrading pigments during long dark exposure (Wiencke et al., 2009). Pigment degradation during extended darkness is not yet confirmed for kelps, e.g., in Arctic S. latissima populations (Scheschonk et al., 2019; Gordillo et al., 2022). We observed that all pigments in L. digitata tend to deplete over three months in the dark, decreasing more at 5°C than at 0°C. Strongest reductions were measured in the xanthophyll cycle pool (VAZ). Chla remained almost stable and Acc only decreased significantly at 5°C. While Chla is the main photosynthetic pigment and Acc are important antenna pigments, VAZ are not directly involved in the process of photon harvesting, but act as photo-protective pigments (Falkowski and Raven, 2007). Though VAZ content was highly depleted during the experiment, the protective mechanism of the xanthophyll cycle (DPS) was still active and slightly increased over time. DPS increases during stress, such as dehydration or high and low temperatures, and is known to be active in the dark (Fernández-Marín et al., 2019; Li et al., 2020; Monteiro et al., 2021). We assume that the pigments in L. digitata were degraded to save energy or function as an additional energy source to outlast the long period of the Polar Night, while still keeping the photosynthetic machinery and photo-protective mechanisms intact, as was shown by Summers et al. (2023). Pigment reduction was enhanced at 5°C compared to 0°C, which aligns with higher physiological activity in L. digitata at 5°C.
In summary, our study has shown that the cold–temperate to Arctic kelp L. digitata is well adapted to Polar Night conditions in the Arctic. Although it has a lower performance and reveals higher biochemical activity levels at 5°C than at 0°C, our results indicate that Arctic winter warming alone will not result in a serious decline of Arctic L. digitata populations in the near future. Nevertheless, interactions between warming and changing light conditions, e.g. due to terrestrial or glacial run-off, have to be considered in studies on the prospective distribution of L. digitata in High Arctic regions (Niedzwiedz and Bischof, 2023; Düsedau et al., 2024).
Data availability statement
All raw data analyzed for this study can be found in the PANGAEA Database: https://doi.org/10.1594/PANGAEA.972789.
Author contributions
MT: Data curation, Formal analysis, Methodology, Visualization, Writing – original draft, Writing – review & editing. IB: Conceptualization, Methodology, Resources, Supervision, Writing – review & editing. MB: Data curation, Methodology, Writing – review & editing. HB: Data curation, Methodology, Writing – review & editing. JH: Methodology, Resources, Writing – review & editing. SN: Data curation, Methodology, Writing – review & editing. NP: Data curation, Methodology, Writing – review & editing. TS: Data curation, Methodology, Writing – review & editing. KB: Funding acquisition, Project administration, Resources, Supervision, Writing – review & editing. ND: Conceptualization, Data curation, Methodology, Supervision, Visualization, Writing – original draft, Writing – review & editing.
Funding
The author(s) declare financial support was received for the research, authorship, and/or publication of this article. This study was conducted in the frame of the project FACE-IT (The Future of Arctic Coastal Ecosystems – Identifying Transitions in Fjord Systems and Adjacent Coastal Areas). FACE-IT has received funding from the European Union’s Horizon 2020 research and innovation program under grant agreement No. 869154.
Acknowledgments
The authors are grateful to H-K Strand from the Holmfjorden Research Station of the Norwegian Institute for Marine Research for the sampling support and logistics. ND thanks S Jungblut and M Koch for their support in sample preparation and U Karsten for the opportunity to measure mannitol at the University of Rostock. The experiment has been conducted at the Alfred Wegener Institute for Polar and Marine Research (AWI), Bremerhaven. The authors thank A Wagner (AWI) for his support in the set-up of the experiment, and B Iken (University of Bremen) for supporting the pigment and C:N analyses.
Conflict of interest
The authors declare that the research was conducted in the absence of any commercial or financial relationships that could be construed as a potential conflict of interest.
Publisher’s note
All claims expressed in this article are solely those of the authors and do not necessarily represent those of their affiliated organizations, or those of the publisher, the editors and the reviewers. Any product that may be evaluated in this article, or claim that may be made by its manufacturer, is not guaranteed or endorsed by the publisher.
Supplementary material
The Supplementary Material for this article can be found online at: https://www.frontiersin.org/articles/10.3389/fmars.2024.1478238/full#supplementary-material
References
Atkinson M. J., Smith S. V. (1983). C:N:P ratios of benthic marine plants. Limnol. Oceanogr. 28, 568–574. doi: 10.4319/lo.1983.28.3.0568
Bartsch I., Paar M., Fredriksen S., Schwanitz M., Daniel C., Hop H., et al. (2016). Changes in kelp forest biomass and depth distribution in Kongsfjorden, Svalbard, between 1996–1998 and 2012–2014 reflect Arctic warming. Polar Biol. 39, 2021–2036. doi: 10.1007/s00300-015-1870-1
Becker S., Hehemann J.-H. (2018). Laminarin quantification in microalgae with enzymes from marine microbes. Bio-Protocol 8, e2666. doi: 10.21769/bioprotoc.2666
Becker S., Scheffel A., Polz M. F., Hehemann J. H. (2017). Accurate quantification of laminarin in marine organic matter with enzymes from marine microbes. Appl. Environ. Microbiol. 83, 1–14. doi: 10.1128/AEM.03389-16
Bischof K., Buschbaum C., Fredriksen S., Gordillo F. J. L., Heinrich S., Jiménez C., et al. (2019). “Kelps and environmental changes in kongsfjorden: stress perception and responses,” in The Ecosystem of Kongsfjorden. Eds. Hop H., Wiencke C. (Springer, Cham), 373–422. doi: 10.1007/978-3-319-46425-1_10
Blain C. O., Shears N. T. (2019). Seasonal and spatial variation in photosynthetic response of the kelp Ecklonia radiata across a turbidity gradient. Photosynth. Res. 140, 21–38. doi: 10.1007/s11120-019-00636-7
Blanca M. J., Alarcón R., Arnau J., Bono R., Bendayan R. (2017). Datos no normales: ¿es el ANOVA una opción válida? Psicothema 29, 552–557. doi: 10.7334/psicothema2016.383
Bolton J. J., Lüning K. (1982). Optimal growth and maximal survival temperatures of Atlantic Laminaria species (Phaeophyta) in culture. Mar. Biol. 66, 89–94. doi: 10.1007/BF00397259
Castro de la Guardia L., Filbee-Dexter K., Reimer J., MacGregor K. A., Garrido I., Singh R. K., et al. (2023). Increasing depth distribution of Arctic kelp with increasing number of open water days with light. Elem. Sci. Anthr. 11, 51. doi: 10.1525/elementa.2022.00051
Colombo-Pallotta M. F., García-Mendoza E., Ladah L. B. (2006). Photosynthetic performance, light absorption, and pigment composition of Macrocystis pyrifera (Laminariales, Phaeophyceae) blade from different depths. J. Phycol. 42, 1225–1234. doi: 10.1111/j.1529-8817.2006.00287.x
Daniel R. M., Danson M. J., Eisenthal R., Lee C. K., Peterson M. E. (2008). The effect of temperature on enzyme activity: New insights and their implications. Extremophiles 12, 51–59. doi: 10.1007/s00792-007-0089-7
Dankworth M., Heinrich S., Fredriksen S., Bartsch I. (2020). DNA barcoding and mucilage ducts in the stipe reveal the presence of Hedophyllum nigripes (Laminariales, Phaeophyceae) in Kongsfjorden (Spitsbergen). J. Phycol. 56, 1245–1254. doi: 10.1111/jpy.13012
Diehl N., Karsten U., Bischof K. (2020). Impacts of combined temperature and salinity stress on the endemic Arctic brown seaweed Laminaria solidungula J. Agardh. Polar Biol. 43, 647–656. doi: 10.1007/s00300-020-02668-5
Diehl N., Laeseke P., Bartsch I., Bligh M., Buck-Wiese H., Hehemann J.-H., et al. (2024). Photoperiod and temperature interactions drive the latitudinal distribution of kelps under climate change. J. Phycol. 00, 1–19. doi: 10.1111/jpy.13497
Dring M. J. (2006). Stress resistance and disease resistance in seaweeds: the role of reactive oxygen metabolism. Adv. Bot. Res. 43, 175–207. doi: 10.1016/S0065-2296(05)43004-9
Dring M. J., Makarov V., SchosChina E., Lorenz M., Lüning K. (1996). Influence of ultraviolet-radiation on chlorophyll fluorescence and growth in different life-history stages of three species of Laminaria (Phaeophyta). Mar. Biol. 126, 183–191. doi: 10.1007/BF00347443
Düsedau L., Fredriksen S., Brand M., Fischer P., Karsten U., Bischof K., et al. (2024). Kelp forest community structure and demography in Kongsfjorden (Svalbard) across 25 years of Arctic warming. Ecol. Evol. 14, e11606. doi: 10.1002/ece3.11606
Falkowski P. G., Raven J. A. (2007). Aquatic Photosynthesis. 2nd ed (Princeton and Oxford: Princeton University Press).
Fernández-Marín B., Gago J., Clemente-Moreno M. J., Flexas J., Gulías J., García-Plazaola J. I. (2019). Plant pigment cycles in the high-Arctic Spitsbergen. Polar Biol. 42, 675–684. doi: 10.1007/s00300-019-02463-x
Fernández-Marín B., Míguez F., Becerril J. M., García-Plazaola J. I. (2011). Activation of violaxanthin cycle in darkness is a common response to different abiotic stresses: A case study in Pelvetia canaliculata. BMC Plant Biol. 11, 181. doi: 10.1186/1471-2229-11-181
Gattuso J. P., Gentili B., Antoine D., Doxaran D. (2020). Global distribution of photosynthetically available radiation on the seafloor. Earth Syst. Sci. Data 12, 1697–1709. doi: 10.5194/essd-12-1697-2020
Gauci C., Bartsch I., Martins N., Liesner D. (2022). Cold thermal priming of Laminaria digitata (Laminariales, Phaeophyceae) gametophytes enhances gametogenesis and thermal performance of sporophytes. Front. Mar. Sci. 9. doi: 10.3389/fmars.2022.862923
Gómez I., Huovinen P. (2012). “Morpho-functionality of carbon metabolism in seaweeds,” in Seaweed Biology: Novel Insights into Ecophysiology, Ecology and Utilization. Eds. Wiencke C., Bischof K. (Berlin Heidelberg: Springer), 25–46.
Gordillo F. J. L., Carmona R., Jiménez C. (2022). A warmer Arctic compromises winter survival of habitat-forming seaweeds. Front. Mar. Sci. 8. doi: 10.3389/fmars.2021.750209
Graiff A., Bartsch I., Ruth W., Wahl M., Karsten U. (2015). Season exerts differential effects of ocean acidification and warming on growth and carbon metabolism of the seaweed Fucus vesiculosus in the western Baltic Sea. Front. Mar. Sci. 2. doi: 10.3389/fmars.2015.00112
Harrison P. J., Hurd C. L. (2001). Nutrient physiology of seaweeds: Application of concepts to aquaculture. Cah. Biol. Mar. 42, 71–82. doi: 10.1080/00318884.2019.1622920
Huang B., Liu C., Banzon V., Freeman E., Graham G., Hankins B., et al. (2021). Improvements of the daily optimum interpolation sea surface temperature (DOISST) version 2.1. J. Clim. 34, 2923–2939. doi: 10.1175/JCLI-D-20-0166.1
Hurd C. L., Harrison P. J., Bischof K., Lobban C. S. (2014). Seaweed Ecology and Physiology. 2nd ed. (Cambridge: Cambridge University Press).
Johnston C. S., Jones R. G., Hunt R. D. (1977). A seasonal carbon budget for a laminarian population in a Scottish sea-loch. Helgoländer wissenschaftliche Meeresuntersuchungen 30, 527–545. doi: 10.1007/BF02207859
Karsten U., Thomas D. N., Weykam G., Daniel C., Kirst G. O. (1991). A simple and rapid method for extraction and separation of low molecular weight carbohydrates from macroalgae using high-performance liquid chromatography, II. Intracellular inorganic ions and organic compounds. Plant Physiol. Biochem. 29, 373–378. Available online at: https://epic.awi.de/id/eprint/1579/.
Koch K., Thiel M., Tellier F., Hagen W., Graeve M., Tala F., et al. (2015). Species separation within the Lessonia nigrescens complex (Phaeophyceae, Laminariales) is mirrored by ecophysiological traits. Bot. Mar. 58, 81–92. doi: 10.1515/bot-2014-0086
Krause-Jensen D., Archambault P., Assis J., Bartsch I., Bischof K., Filbee-Dexter K., et al. (2020). Imprint of climate change on Pan-Arctic marine vegetation. Front. Mar. Sci. 7. doi: 10.3389/fmars.2020.617324
Küppers U., Kremer B. P. (1978). Longitudinal profiles of carbon dioxide fixation capacities in marine macroalgae. Plant Physiol. 62, 49–53. doi: 10.1104/pp.62.1.49
Lever M. (1972). A new reaction for colorimetric determination of carbohydrates. Anal. Biochem. 47, 273–279. doi: 10.1016/0003-2697(72)90301-6
Li H., Monteiro C., Heinrich S., Bartsch I., Valentin K., Harms L., et al. (2020). Responses of the kelp Saccharina latissima (Phaeophyceae) to the warming Arctic: From physiology to transcriptomics. Physiol. Plant 168, 5–26. doi: 10.1111/ppl.13009
Liesner D., Shama L. N. S., Diehl N., Valentin K., Bartsch I. (2020). Thermal plasticity of the kelp Laminaria digitata (Phaeophyceae) across life cycle stages reveals the importance of cold seasons for marine forests. Front. Mar. Sci. 7. doi: 10.3389/fmars.2020.00456
Longtin C. M., Saunders G. W. (2015). On the utility of mucilage ducts as a taxonomic character in Laminaria and Saccharina (Phaeophyceae) - The conundrum of S. groenlandica. Phycologia 54, 440–450. doi: 10.2216/15-19.1
Lüning K. (1990). Seaweeds - Their environment, biogeography, and ecophysiology. Eds. Yarish C., Kirkman H. (Stuttgart: John Wiley & Sons, Inc).
Maturilli M., Hanssen-Bauer I., Neuber R., Rex M., Edvardsen K. (2019). “The atmosphere above Ny-Ålesund: Climate and global warming, ozone and surface UV radiation,” in The ecosystem of Kongsfjorden, Svalbard. Eds. Hop H., Wiencke C. (Springer Nature Switzerland AG, Cham).
Mauger S., Fouqueau L., Avia K., Reynes L., Serrao E. A., Neiva J., et al. (2021). Development of tools to rapidly identify cryptic species and characterize their genetic diversity in different European kelp species. J. Appl. Phycol. 33, 4169–4186. doi: 10.1007/s10811-021-02613-x
Monteiro C., Li H., Diehl N., Collén J., Heinrich S., Bischof K., et al. (2021). Modulation of physiological performance by temperature and salinity in the sugar kelp Saccharina latissima. Phycol. Res. 69, 48–57. doi: 10.1111/pre.12443
Niedzwiedz S., Bischof K. (2023). Glacial retreat and rising temperatures are limiting the expansion of temperate kelp species in the future Arctic. Limnol. Oceanogr. 68, 816–830. doi: 10.1002/lno.12312
Pörtner H., Lucassen M., Storch D. (2005). Metabolic biochemistry: Its role in thermal tolerance and in the capacities of physiological and ecological gunction. Fish Physiol. 22, 79–154. doi: 10.1016/S1546-5098(04)22003-9
Previdi M., Smith K. L., Polvani L. M. (2021). Arctic amplification of climate change: A review of underlying mechanisms. Environ. Res. Lett. 16, 093003. doi: 10.1088/1748-9326/ac1c29
Rantanen M., Karpechko A. Y., Lipponen A., Nordling K., Hyvärinen O., Ruosteenoja K., et al. (2022). The Arctic has warmed nearly four times faster than the globe since 1979. Commun. Earth Environ. 3, 168. doi: 10.1038/s43247-022-00498-3
R Core Team R. (2022). R: A language and environment for statistical computing. (Vienna, Austria: R Foundation for Statistical Computing).
Richter-Menge J., Overland J. E., Mathis J. T., Osborne E. E. (2017). Arctic report card 2017. Available online at: www.arctic.noaa.gov/Report-Card (Accessed December, 2023).
Rokkan Iversen K., Seuthe L. (2011). Seasonal microbial processes in a high-latitude fjord (Kongsfjorden, Svalbard): I. Heterotrophic bacteria, picoplankton and nanoflagellates. Polar Biol. 34, 731–749. doi: 10.1007/s00300-010-0929-2
Scheschonk L., Becker S., Hehemann J.-H., Diehl N., Karsten U., Bischof K. (2019). Arctic kelp eco-physiology during the polar night in the face of global warming: A crucial role for laminarin. Mar. Ecol. Prog. Ser. 611, 59–74. doi: 10.3354/meps12860
Schlegel R. W., Singh R. K., Gentili B., Bélanger S., Castro de la Guardia L., Krause-Jensen D., et al. (2024). Underwater light environment in Arctic fjords. Earth Syst. Sci. Data 16, 2773–2788. doi: 10.5194/essd-16-2773-2024
Singh A., Pal B., Singh K. S. (2024). Carbohydrate and pigment composition of macroalgae in a kelp-dominated Arctic fjord. Reg. Stud. Mar. Sci. 77, 103644. doi: 10.1016/j.rsma.2024.103644
Smale D. A., Wernberg T., Oliver E. C. J., Thomsen M., Harvey B. P., Straub S. C., et al. (2019). Marine heatwaves threaten global biodiversity and the provision of ecosystem services. Nat. Clim. Change 9, 306–312. doi: 10.1038/s41558-019-0412-1
Steneck R. S., Graham M. H., Bourque B. J., Corbett D., Erlandson J. M., Estes J. A., et al. (2002). Kelp forest ecosystems: Biodiversity, stability, resilience and future. Environ. Conserv. 29, 436–459. doi: 10.1017/S0376892902000322
Summers N., Fragoso G. M., Johnsen G. (2023). Photophysiologically active green, red, and brown macroalgae living in the Arctic Polar Night. Sci. Rep. 13, 17971. doi: 10.1038/s41598-023-44026-5
tom Dieck (Bartsch) I. (1992). North Pacific and North Atlantic digitate Laminaria species (Phaeophyta): hybridization experiments and temperature responses. Phycologia 31, 147–163. doi: 10.2216/i0031-8884-31-2-147.1
Verardo D. J., Froelich P. N., McIntyre A. (1990). Determination of organic carbon and nitrogen in marine sediments using the Carlo Erba NA-1500 Analyzer. Deep. Res. 37, 157–165. doi: 10.1016/0198-0149(90)90034-S
Vihtakari M. (2024). ggOceanMaps: Plot Data on Oceanographic Maps using 'ggplot2'. R package version 2.2.0. Available online at: https://CRAN.R-project.org/package=ggOceanMaps.
Wang Y., Huang F., Fan T. (2017). Spatio-temporal variations of Arctic amplification and their linkage with the Arctic oscillation. Acta Oceanol. Sin. 36, 42–51. doi: 10.1007/s13131-017-1025-z
Wernberg T., Krumhansl K., Filbee-Dexter K., Pedersen M. F. (2019). “Status and trends for the world’s kelp forests,” in World Seas: An environmental evaluation (Cambridge, Massachusetts: Academic Press), 57–78. doi: 10.1016/b978-0-12-805052-1.00003-6
Wiencke C., Bischof K. (2012). Seaweed Biology - Novel insights into ecophysiology, ecology and utilization (Heidelberg New York Dodrecht London: Springer).
Wiencke C., Gómez I., Dunton K. (2009). Phenology and seasonal physiological performance of polar seaweeds. Bot. Mar. 52, 585–592. doi: 10.1515/BOT.2009.078
Wright S. W., Jeffrey S. W., Mantoura R. F. C., Llewelly C. A., Bjørnland T., Repeta D., et al. (1991). Improved HPLC method for the analysis of chlorophylls and carotenoids from marine phytoplankton. Mar. Ecol. Prog. Ser. 77, 183–196. doi: 10.3354/meps077183
Yamaguchi T., Ikawa T., Nisizawa K. (1966). Incorporation of radioactive carbon from H14CO3- into sugar constituents by a brown alga, Eisenia bicyclis, during photosynthesis and its fate in the dark. Plant Cell Physiol. 7, 217–229. doi: 10.1093/oxfordjournals.pcp.a079175
Zacher K., Rautenberger R., Hanelt D., Wulff A., Wiencke C. (2009). The abiotic environment of polar marine benthic algae. Bot. Mar. 52, 483–490. doi: 10.1515/BOT.2009.082
Keywords: Arctic amplification, C:N, Fv/Fm, laminarin, mannitol, pigments, Polar Night
Citation: Trautmann M, Bartsch I, Bligh M, Buck-Wiese H, Hehemann J-H, Niedzwiedz S, Plag N, Shan T, Bischof K and Diehl N (2024) Impact of climate change on the kelp Laminaria digitata – simulated Arctic winter warming. Front. Mar. Sci. 11:1478238. doi: 10.3389/fmars.2024.1478238
Received: 09 August 2024; Accepted: 30 September 2024;
Published: 08 November 2024.
Edited by:
Christopher Edward Cornwall, Victoria University of Wellington, New ZealandCopyright © 2024 Trautmann, Bartsch, Bligh, Buck-Wiese, Hehemann, Niedzwiedz, Plag, Shan, Bischof and Diehl. This is an open-access article distributed under the terms of the Creative Commons Attribution License (CC BY). The use, distribution or reproduction in other forums is permitted, provided the original author(s) and the copyright owner(s) are credited and that the original publication in this journal is cited, in accordance with accepted academic practice. No use, distribution or reproduction is permitted which does not comply with these terms.
*Correspondence: Nora Diehl, bmRpZWhsQHVuaS1icmVtZW4uZGU=