- 1Research Department, Flanders Marine Institute, Ostend, Belgium
- 2Marine Biology Research Group, Ghent University, Ghent, Belgium
Pelagic fish species, including Clupea harengus (Atlantic herring), Scomber scombrus (Atlantic mackerel) and Dicentrarchus labrax (European seabass), are integral to the ecological stability of European marine ecosystems. This study employs a mechanistic niche modelling approach to predict the distribution of these key pelagic species in European seas and to assess the impact of predicted changes in climate conditions on their suitable habitat range. By using fuzzy logic principles and mathematical descriptions of species’ niches, we analysed responses to changing temperature and salinity using climate prediction data from six Shared Socioeconomic Pathways (SSP) scenarios, predicting habitat suitability from the present (2010-2019) until 2100. Under the worst-case temperature climate scenario, all three species exhibited a consistent northward shift of suitable habitats by 2100. Specifically, the suitable habitat for C. harengus, S. scombrus and D. labrax is projected to shift approximately 638 km, 799 km and 13 km north, respectively. The independent contributions of temperature and salinity indicate a distinction in habitat suitability between northern European waters and the Mediterranean Sea, with higher suitability scores in the north. For example, by 2100, the habitat suitability index for non-spawning Atlantic herring in the North Atlantic Ocean is projected to be 0.63 ± 0.3 under SSP5-8.5 compared to the current habitat suitability index of 0.49 ± 0.36, while the index is projected to 0.02 ± 0.003 in the Mediterranean Sea-Western Basin with the current index at 0.01 ± 0.03. These findings suggest that northern latitudes, encompassing regions such as the North Sea and the Baltic Sea currently offer more favorable conditions compared to the lower latitudes of the Mediterranean region. The study’s findings should guide policy decisions in environmental and marine resource management, ensuring interventions are based on up-to-date information and account for anticipated climate change impacts.
1 Introduction
Understanding the habitat preferences of commercially important pelagic species is essential for their sustainable management (Fernandez et al., 2022; Schatz, 2020; van Balsfoort et al., 2022). The implementation of effective ocean and coastal management strategies, particularly concerning European Union (EU) fisheries, has become even more crucial in the wake of geopolitical changes, especially Brexit. Brexit significantly impacted European fisheries due to changes in access to United Kingdom (UK) waters and alterations in fishing policies (Fernandez et al., 2022; Phillipson and Symes, 2018; Symes and Phillipson, 2019). Historically, UK waters have been rich fishing grounds for both demersal and pelagic species (Guille et al., 2021). However, the UK’s withdrawal from the European Union (EU) marked the end of the Common Fisheries Policy (CFP) in British waters, leading to reduced access for EU fishing fleets and affecting the livelihoods of European fishermen who relied on these grounds (Fernandez et al., 2022; Phillipson and Symes, 2018; Schatz, 2020). During the transition period, negotiations between the UK and the EU established new arrangements, aiming to mitigate abrupt disruptions (Heath and Cook, 2020). As a result, the EU fishing industry faces challenges in recalibrating strategies, exploring alternative fishing grounds, and managing the economic repercussions of reduced access to UK waters (Phillipson and Symes, 2018; Symes and Phillipson, 2019; van Balsfoort et al., 2022). With Brexit, European fleets may explore alternatives within their fishing grounds, such as pelagic fisheries, which are not currently the focus of Belgian fishing fleets.
The changes to European fisheries management following Brexit emphasize the urgent need for a deeper understanding of pelagic species’ resilience to climate change, as their potential for sustainable fishing practices offers promise for both ecosystem health and the future of European fisheries. High market demand for pelagic species and potentially lower fishing costs contribute to their economic viability (Nielsen et al., 2017; Paoletti et al., 2021). Beyond their economic importance, pelagic fish play crucial ecological roles, maintaining food webs and nutrient cycles within marine ecosystems, which are vital for biodiversity and fisheries productivity (Checkley et al., 2009; Fréon et al., 2005). Additionally, their midwater and surface habitats allow for fishing methods with lower bycatch compared to demersal fisheries, minimizing ecosystem disruptions (Morizur et al., 1996). However, climate change introduces significant challenges by altering both ocean temperatures and salinity. Rising atmospheric temperatures lead to ocean warming, which directly impacts fish metabolism, growth rates, and migration patterns. Climate change also affects ocean salinity through increased precipitation, altered freshwater runoff, and melting ice, which can cause regional shifts in salinity patterns (Helm et al., 2010; Röthig et al., 2023). These salinity changes influence species distribution and behavior, as salinity levels affect osmoregulation, which is vital for fish health and survival (Velotta et al., 2022). Consequently, understanding the combined impacts of temperature and salinity shifts on pelagic fish populations is essential for developing resilient, adaptive fisheries management strategies in a changing environment.
Climate change is profoundly affecting the marine environment, particularly the habitats and spawning grounds of pelagic fish (Asch et al., 2019; IPCC, 2022; Pankhurst and Munday, 2011). Sea surface temperatures are predicted to rise. Warmer waters and heatwaves will increase the metabolic demands of pelagic fish, reducing energy reserves and reproductive success (Muhling et al., 2017; Pankhurst and Munday, 2011; Peck et al., 2013). In addition to temperature changes, the future salinity in European seas will be influenced by climate change, freshwater inputs, and ocean circulation, leading to varying regional impacts (Holt et al., 2010). Coastal areas are likely to experience larger and more variable changes in salinity due to their sensitivity to freshwater inputs from rivers and local factors while offshore regions will generally see more gradual and homogeneous salinity changes. Salinity plays a crucial role in regulating the distribution of marine life, and shifts in salinity can disrupt marine ecosystems, leading to food scarcity and habitat loss for pelagic fish (Hagan and Able, 2003; Peltonen et al., 2007). When salinity shifts beyond optimal ranges, it can create unfavorable conditions for certain plankton species, thereby reducing their availability and affecting the foraging behavior of pelagic fish that depend on them (Asch et al., 2019; Edwards, 2009; Mason and Vincent, 2011; Schickele et al., 2021). Climate change can also disrupt the timing, location, and success of pelagic fish spawning, potentially leading to population declines (Munday et al., 2008; Pankhurst and Munday, 2011). Some studies also indicate a potential population increase under climate change in some pelagic fish species (Perry et al., 2005). Such population growth could influence species distribution in several ways. Expanding populations may intensify competition for limited resources, potentially driving individuals to exploit less favorable habitats or shift their ranges (Rijnsdorp et al., 2009; Schickele et al., 2021). Additionally, increased densities in core habitats might lead to overcrowding, resulting in changes to habitat use patterns and potentially facilitating colonization of new regions (Johnson, 2006; Smith and Anderson, 2016).
Previous research has highlighted the differential impacts of climate change on spawning and non-spawning individuals in marine species, emphasizing the importance of examining these life stages separately. Spawning individuals are particularly sensitive to environmental changes, as temperature and salinity directly affect reproductive processes, including egg viability, spawning success, and larval survival (Cushing, 2001; Haegele and Schweigert, 1985). Studies indicate that spawning fish often require more stable and optimal environmental conditions, whereas non-spawning individuals may exhibit greater tolerance to environmental variability (Petitgas et al., 2013; Schickele et al., 2021). Temperature increases, for example, can accelerate metabolic rates in both spawning and non-spawning individuals, but the physiological demands of reproduction can make spawning individuals more vulnerable to thermal stress (Dahlke et al., 2020; Illing et al., 2020; Singh et al., 2023). In addition, salinity changes can disrupt osmoregulation, with significant consequences for spawning individuals who often inhabit different salinity zones during reproductive phases.
In response to the increasing pressure of climate change on pelagic fish habitats, scientists have turned to habitat suitability models (HSMs) to gain a deeper understanding of these dynamics (Schickele et al., 2021; Townhill et al., 2023). While species distribution models (SDMs) are widely used for predicting species ranges based on correlations between species presence and environmental variables (Schickele et al., 2021), HSMs offer distinct advantages for this study. Specifically, HSMs, particularly mechanistic niche models, provide a more process-based approach by incorporating the physiological and ecological responses of species to environmental factors (Kearney and Porter, 2009). Unlike SDMs, which rely heavily on observed distributions and environmental correlates, mechanistic models explicitly define a species’ environmental niche using mathematical descriptions of key variables like temperature, salinity, and habitat requirements (Baker et al., 2018; Leibold, 1995). This allows for more detailed predictions, especially under changing climate conditions, where the relationship between the environment and species responses may be non-linear or beyond the range of current observations (Briscoe et al., 2022). By incorporating mechanistic links between species and their environment, HSMs offer a more dynamic understanding of how pelagic fish habitats might shift under future climate scenarios, making them particularly suitable for assessing long-term ecological impacts.
In light of the challenges posed by a changing environment, this research seeks to understand how climate change, according to Shared Socioeconomic Pathways (SSPs) scenarios, affects the spawning versus non-spawning habitats of three pelagic species of potential commercial interest in European waters: Atlantic herring (Clupea harengus), Atlantic mackerel (Scomber scombrus), and European seabass (Dicentrarchus labrax). This study developed mechanistic niche models using temperature and salinity data, validated by 655,389 species occurrence records. Our analysis employed habitat suitability index (HSI) values and observed distribution patterns, focusing on how optimal suitability shifted over time, independent of longitudinal variations. We examined the impact of climate change on habitat suitability under six SSP scenarios (SSP1-1.9, SSP1-2.6, SSP2-4.5, SSP3-7.0, SSP4-6.0, SSP5-8.5), representing a range of future socioeconomic and greenhouse gas emission trajectories. By exploring these dynamics, this research sheds light on critical aspects of ecological responses to a changing environment, providing insights crucial for the development of sustainable management strategies for marine ecosystems in the face of climate challenges.
2 Materials and methods
2.1 Study area
Our investigation focused on European marine waters, covering the geographic coordinates between longitudes 20°W and 40°E, and latitudes 30°N and 65°N (Figure 1). The study area covers approximately 9.9 million km² representing about 2.7% of the surface area covered by the global ocean. Regional seas often have distinct characteristics and vary in salinity and temperature. For example, the Baltic Sea, located in Northern Europe, undergoes seasonal fluctuations in temperature, with cold winters and milder summers. It has the lowest salinity in the study area, ranging between 0.7 (parts per thousand) ppt and 7.5ppt (González-Gambau et al., 2022; Lehmann et al., 2022). These low salinity levels are attributed to inputs from freshwater sources like rivers and precipitation (Lehmann et al., 2022; Stigebrandt and Gustafsson, 2003). The Mediterranean Sea is characterized by high air temperatures, and warm summer air conditions exceeding 30°C, and these are accompanied by elevated salinity due to high evaporation rates (Ducrocq, 2016). Therefore, the highest salinity in our study area is found in the Mediterranean Sea with an average of 38.5 ppt (Aydogdu et al., 2023). Seasonal temperature variations in the North Atlantic Shelf are influenced by the North Atlantic Drift, resulting in mild winters and warm summers (Bray, 1982; Ruprich-Robert and Cassou, 2015). These diverse temperature and salinity characteristics contribute to the unique environmental conditions and ecosystems in each of these seas.
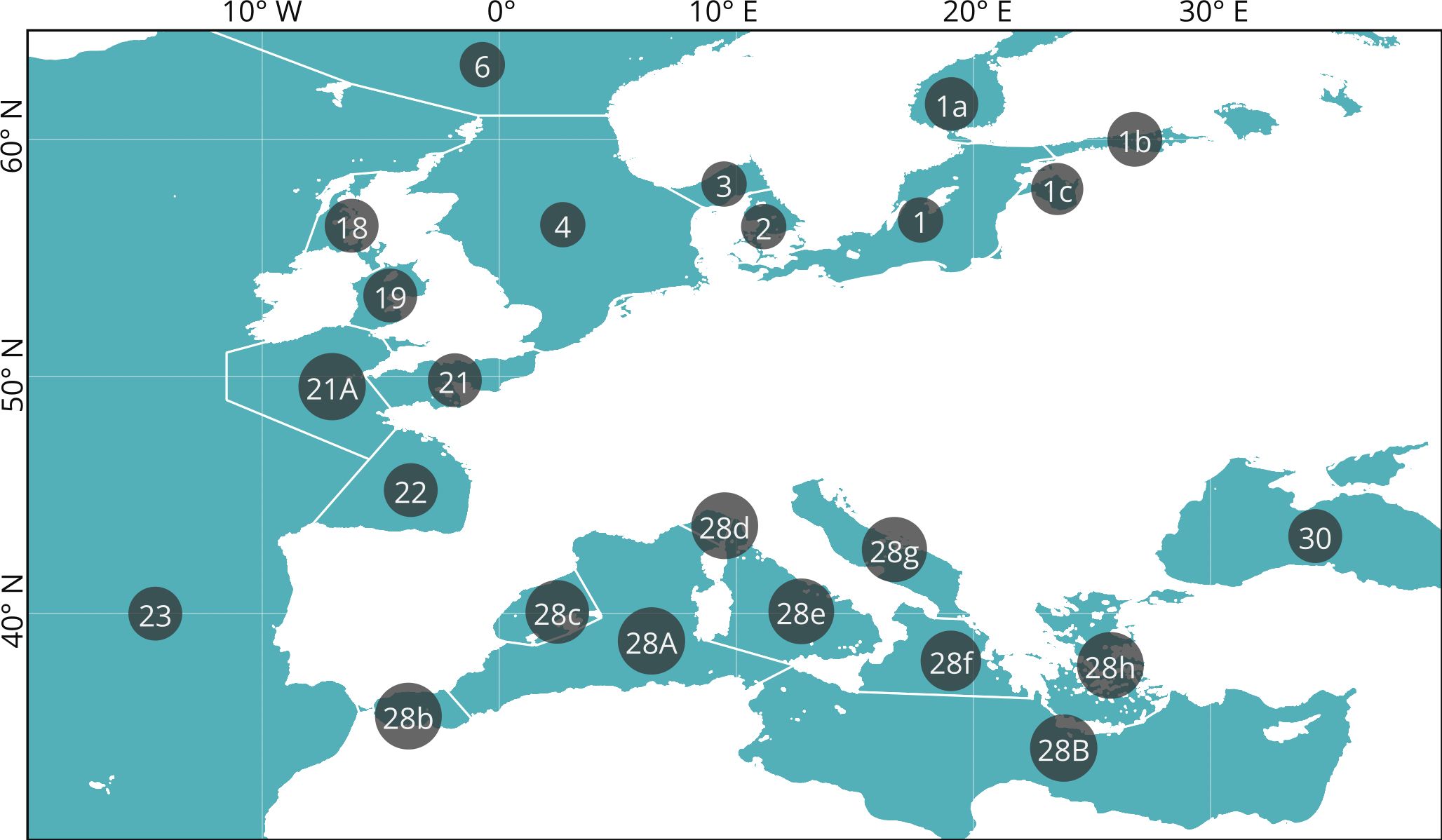
Figure 1. Study area showing the International Hydrographic Office Sea Areas (Flanders Marine Institute, 2025): 1: Baltic Sea, 1a: Gulf of Bothnia, 1b: Gulf of Finland, 1c: Gulf of Riga, 2: Kattegat, 3: Skagerrak, 4: North Sea, 6: Norwegian Sea, 18: Inner Seas off the West Coast of Scotland, 19: Irish Sea and St. George’s Channel, 20: Bristol Channel, 21: English Channel, 21A: Celtic Sea, 22: Bay of Biscay, 23: North Atlantic Ocean, 28a: Strait of Gibraltar, 28A: Mediterranean Sea - Western Basin, 28B: Mediterranean Sea - Eastern Basin, 28b: Alboran Sea, 28c: Balearic (Iberian Sea), 28d: Ligurian Sea, 28e: Tyrrhenian Sea, 28f: Ionian Sea, 28g: Adriatic Sea, 28h: Aegean Sea, 29: Sea of Marmara, 30: Black Sea,.
2.2 Modelling approach
2.2.1 Species
This study investigated the impact of climate-driven changes in sea surface temperature and salinity on the habitat suitability of European seas for three key pelagic species, Clupea harengus (Atlantic herring), Scomber scombrus (Atlantic mackerel), and Dicentrarchus labrax (European seabass), chosen for their ecological importance, economic value, and comprehensive data availability (Froese and Pauly, 2024c, 2024d, 2024a). Each species occupies distinct regions within European waters, enabling an assessment of vulnerabilities across varied marine habitats (Trenkel et al., 2014). Atlantic herring, widely distributed in cooler northern waters, supports marine food webs as a primary prey species and is foundational to ecosystem stability (Munroe, 2002). Atlantic mackerel, known for its extensive migratory behavior and presence in temperate waters, is a crucial link across ecosystems and an important prey species (Jansen et al., 2012; Kvaavik et al., 2019). European seabass, inhabiting coastal and warmer waters, is notable for its adaptability to both temperature and salinity variations, making it valuable for understanding climate resilience (De Pontual et al., 2023). Economically, these species are vital to European fisheries, with herring and mackerel collectively comprising over 50% of the EU pelagic fishery sector’s total economic value supporting industries from artisanal to commercial levels and contributing significantly to food security (European Commission: Joint Research Centre, Scientific, Technical and Economic Committee for Fisheries et al., 2019). Seabass is highly prized in commercial and recreational fisheries, particularly in northern Europe, where it supports local economies (Hyder et al., 2018; Tidbury et al., 2021). The availability of extensive data on these species including physiological characteristics, ecological roles, and historical distributions enables precise modelling to project environmental impacts, providing essential insights for adaptive fisheries management and conservation policies to safeguard ecosystem health and sustain European fisheries.
Information on variables of both spawning and non-spawning aspects of pelagic fish was sourced from a literature review (Table 1). In determining the salinity and temperature ranges for Atlantic herring, Atlantic mackerel, and European seabass, a comprehensive approach was employed, drawing upon a diverse array of scientific literature (Table 1). The chosen ranges (Supplementary Tables S1, S2) represent a synthesis of physiological considerations, reproductive behaviors, and habitat preferences documented in numerous studies. We systematically excluded temperature and salinity ranges that fell outside the physiological tolerances or were irrelevant to the focal species. This process was based on a thorough review of both foundational studies and more recent, updated literature [e.g (Froese and Pauly, 2024b; OBIS, 2024)]. Specifically, ranges identified in earlier research were cross-referenced with updated datasets and observational records to ensure the thresholds reflected the most current understanding of each species’ ecological and physiological limits (Table 1). For instance, salinity ranges that exceeded the osmoregulatory capacities of the species or temperature ranges outside metabolic or reproductive limits were removed. This step-by-step approach ensured that the selected ranges represented ecologically valid conditions while also accommodating variability in life stage requirements.
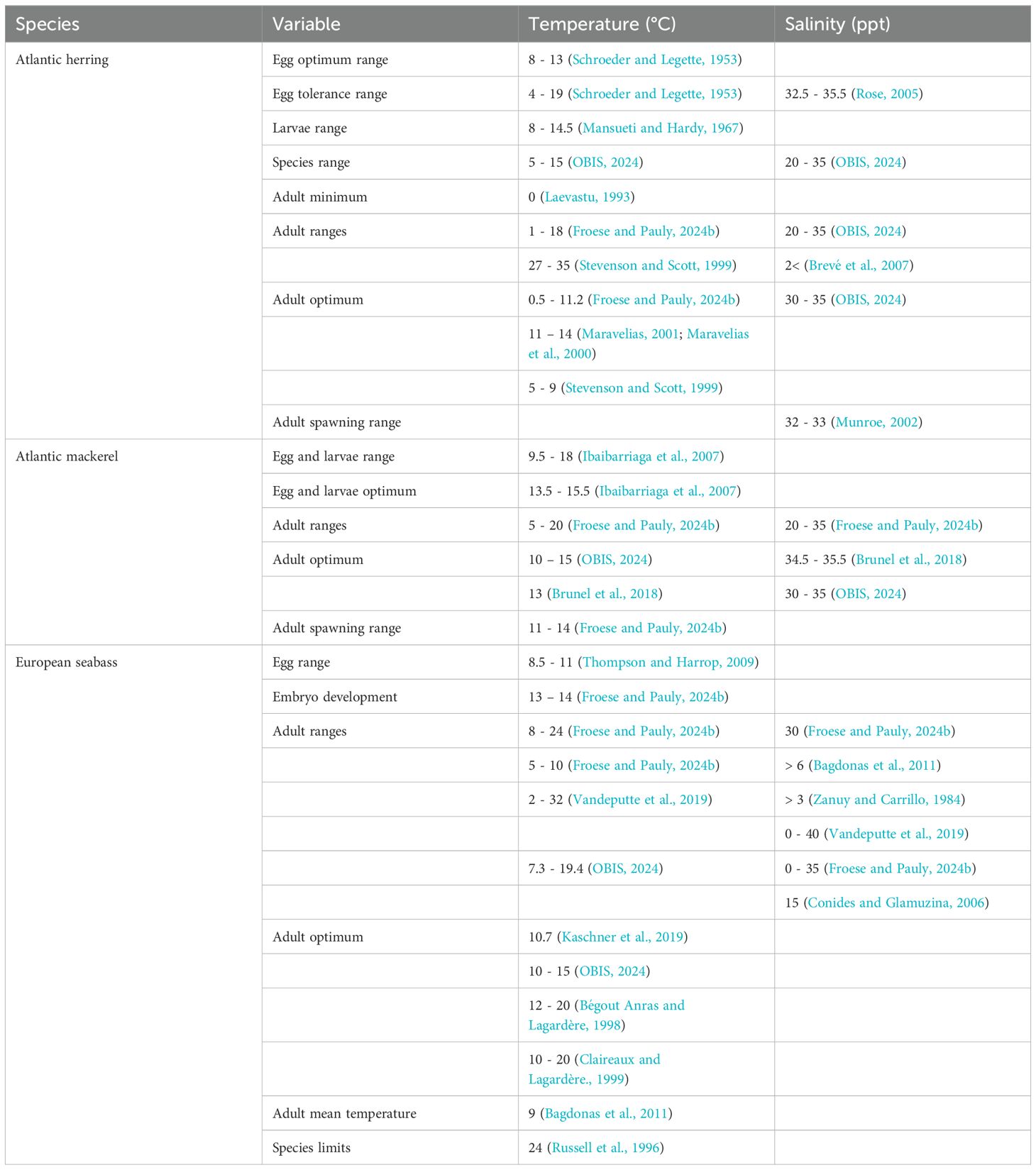
Table 1. Environmental tolerances and optimum ranges for Atlantic herring, Atlantic mackerel, and European seabass.
Atlantic herring shows a preference for coastal and shelf areas where salinity levels typically fall within the range of 2 to 36 ppt (Brevé et al., 2007). Notably, the preference for 30 to 35 ppt aligns with critical conditions for reproductive success, with the adult spawning optimum (OBIS, 2024). Similarly, the temperature range of 8 to 12°C corresponds closely with the species’ known physiological needs and reproductive behavior, with temperatures below 8°C potentially limiting growth and reproductive (Froese and Pauly, 2024b; Mansueti and Hardy, 1967; Schroeder and Legette, 1953). Atlantic mackerel inhabits a wide range of coastal and offshore environments, with a salinity tolerance of 20 to 36 ppt, while salinity values between 30 and 35 ppt reflect optimal conditions for spawning and larval survival (Brunel and Dickey-Collas, 2010; Froese and Pauly, 2024b; OBIS, 2024). The preferred temperature range of 13.5 to 15.5°C aligns with habitat preferences and reproductive behavior, providing optimal conditions for spawning and larval development (Ibaibarriaga et al., 2007). Regarding European seabass, a salinity range of 0.5 to 40ppt is consistent with habitat preferences in coastal and estuarine environments, supported by multiple sources indicating optimal (Froese and Pauly, 2024b; Vandeputte et al., 2019). The broader temperature range of 8 to 24°C reflects the species’ ability to inhabit diverse habitats, with optimal conditions for growth, reproduction, and survival encompassed within this range (Kaschner et al., 2019; OBIS, 2024).
The exclusion of certain ranges from the analysis was guided by factors such as relevance to habitat preferences, consistency across studies, physiological constraints, data quality, and ecological context. Ranges that fell outside of the species’ typical habitat or physiological limits, represented extreme or suboptimal conditions or lacked consistency across studies were omitted to ensure coherence and accuracy in defining habitat preferences. By prioritizing ranges that best reflect the species’ ecological requirements, this approach enables researchers to accurately obtain the species niche.
2.2.2 Climate scenarios
To evaluate the influence of climate change on these three pelagic species habitats, five climate change scenarios from the Shared Socioeconomic Pathways (SSP) were selected as future predictions for the variables of interest, i.e. sea surface temperature (SST) and sea surface salinity (SSS) (Intergovernmental Panel on Climate Change, 2023). Average sea surface temperature and salinity values from six SSP scenarios were selected, including scenarios SSP1-1.9, SSP1-2.6, SSP2-4.5, SSP3-7.0, SSP4-6.0, and SSP5-8.5. Predictions of sea surface temperature and sea surface salinity under these scenarios were obtained from climate prediction data from Bio-ORACLE v3.0 (Assis et al., 2018, 2024; Tyberghein et al., 2012). Shared Socioeconomic Pathways 1-1.9 envisions a future marked by a significant reduction in greenhouse gas emissions, striving for net-zero carbon dioxide emissions around 2050 (Intergovernmental Panel on Climate Change, 2023). The climate model employed in SSP1-1.9, features a resolution of 1/4 degree for oceanic regions (Intergovernmental Panel on Climate Change, 2023). This scenario is unique in meeting the Paris Agreement’s goal of restricting global warming to about 1.5°C above preindustrial levels. Compared to the baseline temperature, the sea surface temperature trajectory projected by Assis et al. (2024) in SSP1-1.9 aligns with this objective, with warming peaking at 0.36°C and then gradually receding and stabilizing around 0.125°C by the century’s end. The worst-case SSP scenario available is SSP5-8.5. According to SSP5-8.5, CO2 emissions are projected to nearly double by 2050, driven by extensive fossil fuel exploitation and energy-intensive lifestyles, resulting from rapid global economic growth (Intergovernmental Panel on Climate Change, 2023). Based on scenario SSP5-8.5, Assis et al. (2024) predict that the average regional sea surface temperature will experience an exponential increase, reaching up to +2.7°C by the year 2100. The salinity data from Assis et al. (2024) associated with the climate change scenario SSP-5.85 indicate a range of values from a minimum of 0.87psu to a maximum of 40psu, with a median salinity of 34.4psu for our study area (Table 2).
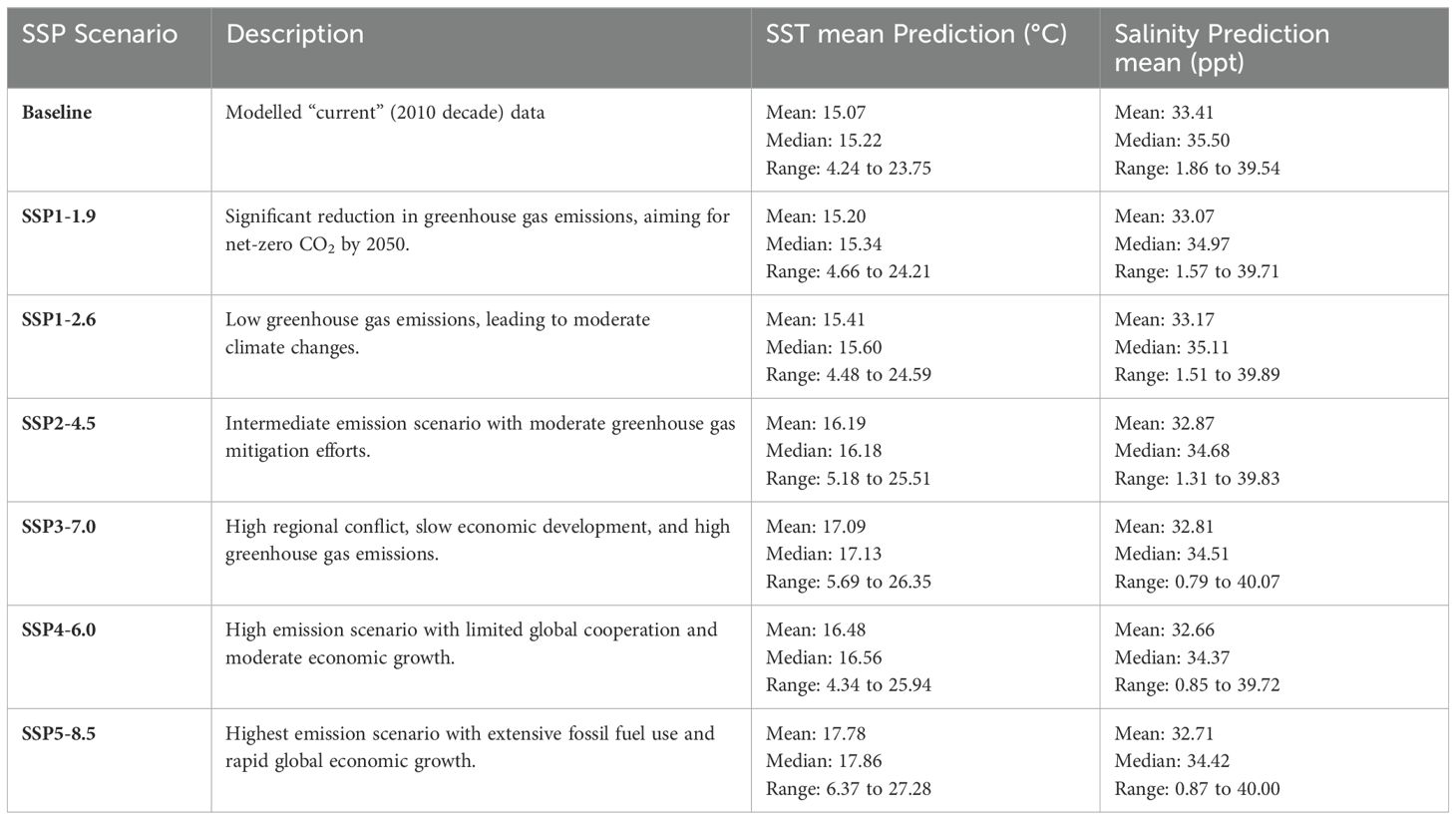
Table 2. Predicted Sea Surface Temperature (SST) and Salinity Means for Different SSP Scenarios Based on Bio-Oracle Data for the year 2100 for the study area.
The SSP scenarios will help to understand how different conditions might impact the species distribution and abundance. Baseline data for the decade of 2010 which is data from 2010 to 2020 formed the foundation for predicting the current habitat suitability for each species, while future projections spanned from 2020 to 2100 (2020 decade to 2090 decade), each prediction covering one decade. Utilizing scenario-driven modelling, we simulated habitat suitability for the selected species from the year 2010 until 2100, delineating potential shifts in their habitats over time. To ensure consistency between the environmental data and the occurrence records, we relied on Bio-Oracle layers derived from surface projections. These layers provide, surface-oriented climate data, which align with the predominantly surface-level occurrence records available for the studied species.
2.2.3 Mechanistic model
A mechanistic modelling approach was used following Westmeijer et al. (2019) whereby the models are based on environmental conditions and specific species responses. The models employed fuzzy logic principles, a mathematical framework that allows for reasoning with uncertainty and enables habitat suitability values to range continuously between 0 and 1. This is particularly useful in ecological contexts where species responses to environmental factors are not strictly defined and can vary in degrees of suitability. In our study, we investigated the temperature and salinity tolerance ranges of the three pelagic fish species through a synthesis of physiological studies and observational data from a comprehensive literature review (Table 1). These ranges were derived as a consensus from multiple sources, ensuring that they account for variability across different regions and life stages. These species-specific responses were then depicted as equations, allowing for a nuanced representation of habitat suitability. It is important to note that adult non-spawning and adult spawning pelagic fish exhibit distinct responses to temperature and salinity; therefore, different equations were created for adult non-spawning and adult spawning phases (Cushing, 2001; Froese and Pauly, 2024b; Haegele and Schweigert, 1985; OBIS, 2024). Equations for temperature and salinity followed a linear equation, where y represents the species’ reaction (suitability), and x represents the corresponding environmental variable (temperature or salinity).
For example, the response of adult non-spawning Atlantic herring to temperature can be modelled as follows. For the lower extinction limit temperatures below 1°C, the response is constant at 0 at Equation 1
For lower temperature tolerance (between 1°C and 8°C, the response follows the linear Equation 2
Between 8°C and 12°C which is the optimum temperature, the response is constant at constant which is Equation 3
For higher tolerance temperatures (12°C to 19°C), the response follows the linear Equation 4
Beyond 19°C which is the upper extinction limit, the values were set to 0.
This piecewise function captures the species-specific response curve, demonstrating how the spawning habitat preferences of Atlantic herring change with varying temperatures.
A mathematical description of the species’ niche was incorporated to predict suitable habitats based on their interactions with environmental factors. These mathematical descriptions were integrated into mechanistic models to predict HSI under varying conditions. R (R Core Team, 2023) was used to process analyse temperature and salinity habitat suitability maps using the packages raster (Hijmans et al., 2023) and rgdal (Bivand et al., 2023). The HSI was then classified as optimal (HSI ≥ 0.75), suboptimal (0.5 < HSI < 0.75) and poor (HSI ≤ 0.5). These rankings followed those of Westmeijer et al. (2019). Maps depicting the habitat suitability for non-spawning and spawning adults were generated for all species, using this methodology in R (R Core Team, 2023) by using the R package raster (Hijmans et al., 2023). To ensure uniformity, all final maps were resampled to a consistent resolution of 0.05° x 0.05° meters and a projection of 4326 yielding a refined habitat suitability index.
2.2.4 Habitat suitability analysis
The study examined the temperature and salinity-driven habitat shifts for Atlantic herring, Atlantic mackerel and European seabass specifically focusing on the decades 2010, 2030, and 2100. Data analysis and visualization was done in R (R Core Team, 2023) using the packages raster (Hijmans et al., 2023) and sf (Bivand et al., 2023), while QGIS (QGIS.org, 2023) aided with spatial processing and map creation. Following the method of Westmeijer et al. (2019) in analyzing habitat suitability, the study employed geospatial analyses to estimate shifts in the minimum latitude of suitable habitats for the HSI under climate change scenarios. Habitat suitability maps were derived from baseline (present-day) and future (SSP585 for 2090) temperature and salinity models. Raster files representing habitat suitability were processed in R using the terra (Hijmans, 2024) and dplyr (Wickham et al., 2023) libraries. The analysis encompassed latitudinal bounds of 30°N to 65°N with a fine resolution of 0.01° and longitudinal bounds from 20W° to 40E° with a step of 2.5°. For each grid cell, mean habitat suitability was calculated. Cells with average suitability >0.5 were identified, and the minimum latitude within this subset was extracted. These suitability thresholds were applied across both baseline and future models.
The spatial extent of suitable habitat was calculated by cropping raster data to defined grid cells and computing mean suitability values within each cell. The latitudinal shift was determined by comparing the minimum latitude of suitability between baseline and future scenarios. The corresponding distance shift was calculated assuming 111 km per degree of latitude. To account for the combined effects of temperature and salinity, known as independent contributions, we developed independent models for each variable as well as an integrated model that combines them. This approach enabled us to assess the individual impacts of temperature and salinity on habitat suitability and their combined influence. The integrated model was created by averaging the habitat suitability indices (HSIs) derived from the individual models for temperature and salinity, using the raster (Hijmans et al., 2023) and rgdal (Bivand et al., 2023) packages in R (R Core Team, 2023).
The integrated habitat suitability index (HSI) which was classified into three categories that were derived from Westmeijer et al. (2019): optimal (HSI ≥ 0.75), suboptimal (0.5 < HSI < 0.75), and poor (HSI ≤ 0.5) inherently accounts for cases where one of the individual indices (temperature or salinity) may be zero. Even if the other parameter yields a high suitability score, the resulting integrated HSI will still be classified as “poor.” This approach ensures that the integrated model reflects the reality that if either temperature or salinity falls outside the physiological tolerance ranges, the overall habitat suitability is significantly reduced, regardless of the suitability of the other parameter. This method assumes an additive interaction between the variables, where both factors independently contribute to habitat suitability. This approach avoids making assumptions about synergistic or antagonistic interactions and allows both factors to independently influence the overall habitat suitability.
2.3 Validation
Fish occurrence data was obtained from the Ocean Biodiversity Information System (OBIS) (OBIS, 2024) for the studied non-spawning fish species within our defined study area, covering the period from 2010 to 2020, consistent with our baseline environmental data from the same timeframe (Supplementary Table S3). The study used 522 051 Atlantic herring occurrence records, 123 913 Atlantic mackerel occurrence records and 9 425 European seabass occurrence records. Validation involved visual comparison of model outputs with actual fish observations, following the approach outlined by Rykiel (1995). To quantify the accuracy of the produced habitat suitability models, the study employed Root Mean Square Error (RMSE) as a measure of predictive performance. The RMSE is a measure used to evaluate the accuracy of a model’s predictions (Hodson, 2022). It is calculated as the square root of the average of the squared differences between the predicted and observed values. A lower RMSE value indicates better model performance, as it means the predictions are closer to the actual observations. In general, an RMSE value close to zero signifies high accuracy, while higher values indicate greater discrepancies between predicted and observed values.
To calculate the RMSE value, the study first imported the habitat suitability maps along with fish occurrence data from OBIS, which included latitude, longitude, and presence information. The study then converted the presence data to observed suitability values (1 for presence). Spatial coordinates from the occurrence data were transformed into Spatial Points objects using the sp package (Pebesma and Bivand, 2005) and then extracted corresponding habitat suitability values using the extract function from the raster package (Hijmans et al., 2023).
After cleaning the data to remove any rows with missing observed or predicted suitability values, RMSE was calculated using Equation 5
where observedi and predictedi are the observed and predicted suitability values at each occurrence point i, and n represents the number of valid data points. The computed RMSE values serve as quantitative metrics of model accuracy, with lower values indicating better agreement between predicted and observed suitability, while higher values suggest greater discrepancies.
3 Results
3.1 Baseline (2010-decade) temperature and salinity-driven habitat suitability
The habitat suitability of pelagic fish was influenced due to the anticipated temperature and salinity changes (Figures 2, 3). For temperature-driven habitat suitability, in the North Atlantic Ocean, European seabass exhibited the highest baseline habitat suitability, with consistently high habitat suitability of 90%, followed by Atlantic herring and Atlantic mackerel showing values of 57% and 45% respectively. All three species also demonstrated optimal habitat suitability in the Norwegian Sea respectively and North Sea throughout the baseline period, indicating favorable environmental conditions for their populations. In contrast, certain areas within the Mediterranean Sea display lower baseline suitability values (e.g., Mediterranean Sea Eastern Basin = 0, Adriatic Sea = 0.04), suggesting less optimal habitat conditions for these pelagic species. The Inner Seas off the West Coast of Scotland consistently showed full suitability (100%) for all species throughout the 2010 decade.
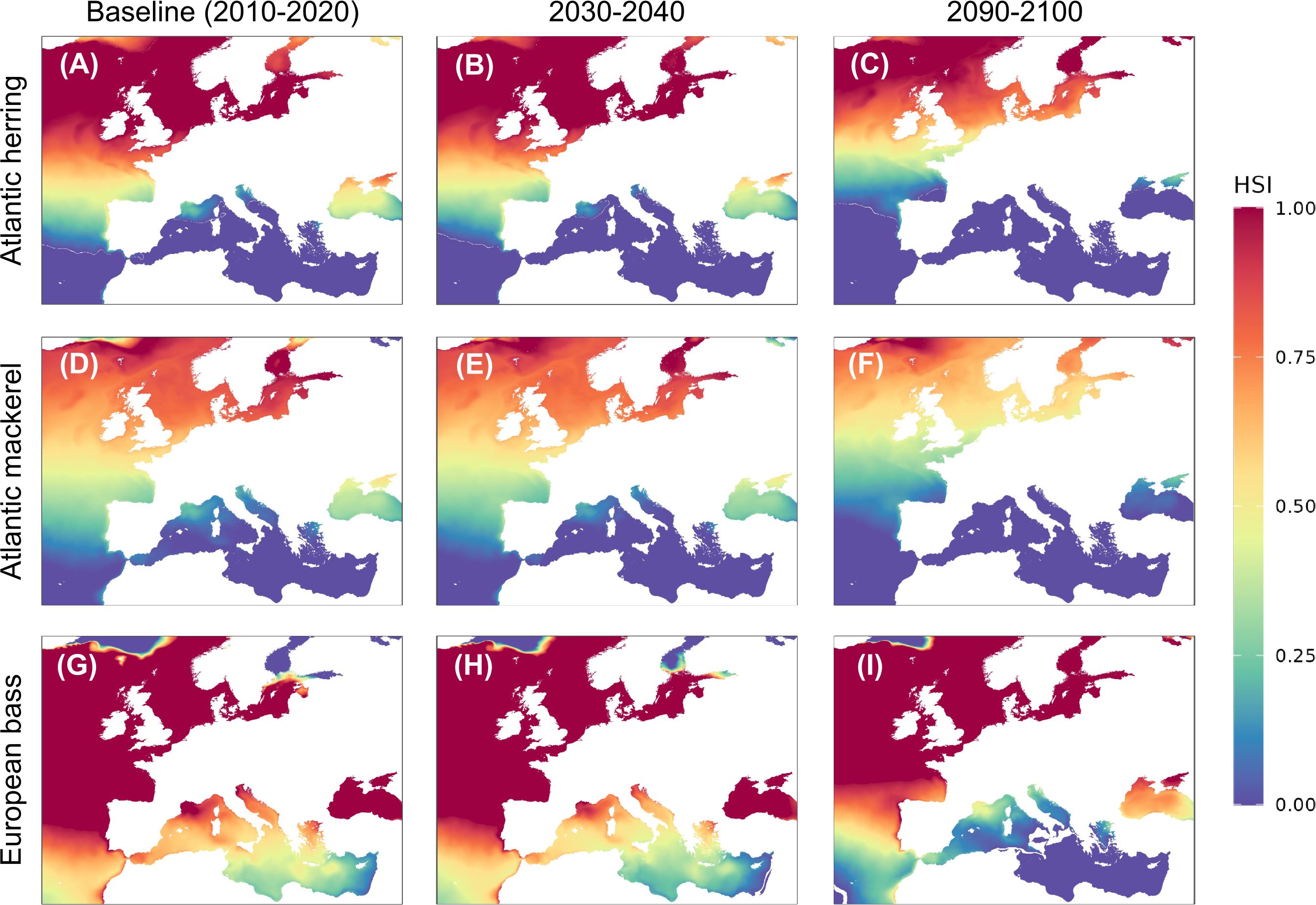
Figure 2. Habitat suitability index (HSI) maps based on temperature for adult non-spawning Atlantic herring [(C. harengus) (A–C)], Atlantic mackerel [(S. scombrus) (D–F)], and European seabass [(D. labrax) (G-I)]. Maps show baseline suitability (A, D, G), SSP5-8.5 scenario for 2030-2040 (B, E, H), and SSP5-8.5 scenario for 2090-2100 (C, F, I).
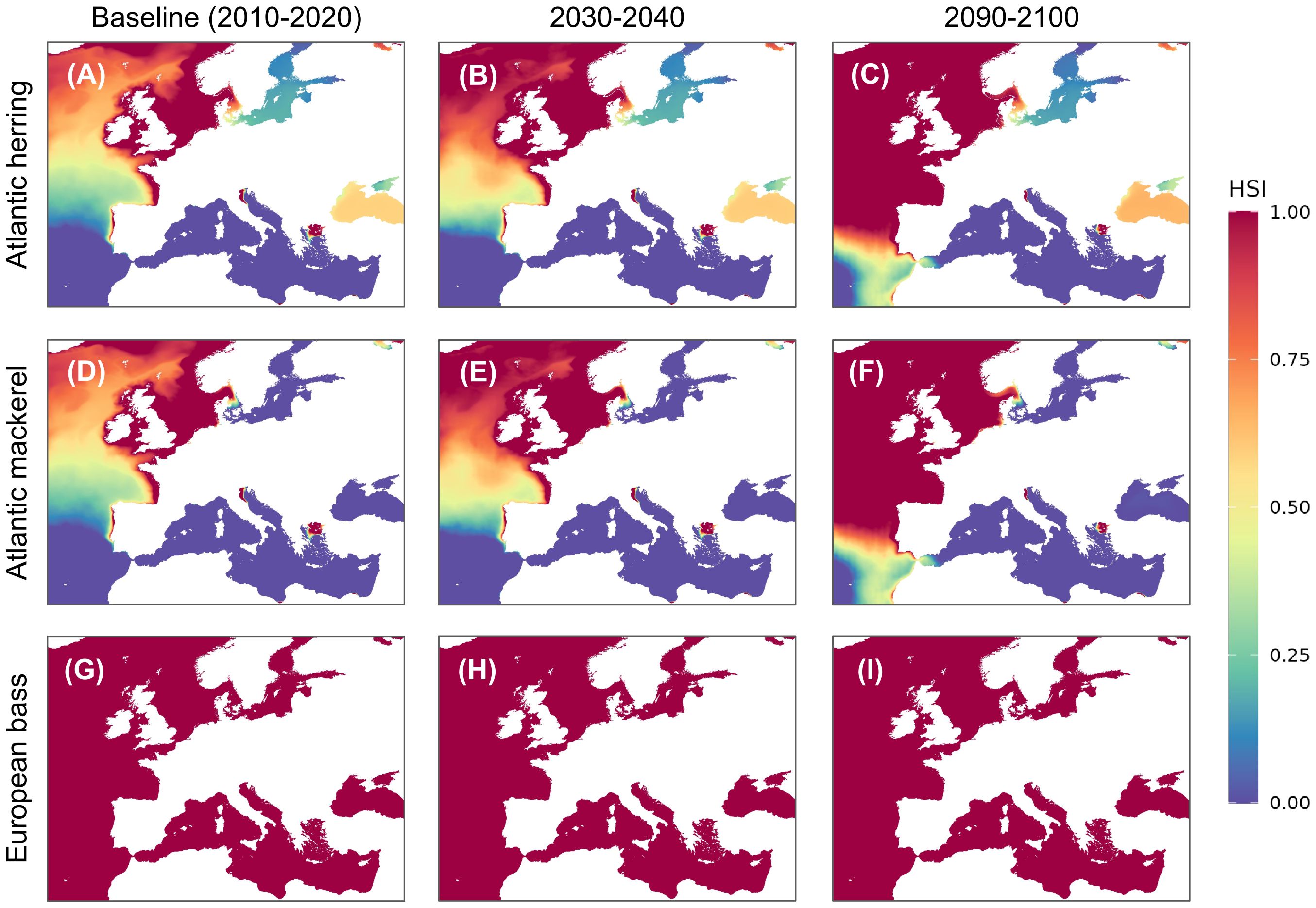
Figure 3. Habitat suitability index (HSI) maps based on salinity for adult non-spawning Atlantic herring [(C. harengus) (A–C)], Atlantic mackerel [(S. scombrus) (D–F)], and European seabass [(D. labrax) (G–I)]. Maps show baseline suitability (A, D, G), SSP5-8.5 scenario for 2030 (B, E, H), and SSP5-8.5 scenario for 2090-2100 (C, F, I).
The baseline distribution of suitable habitats due to salinity for Atlantic herring, Atlantic mackerel, and European seabass revealed distinct geographic patterns (Figure 3). Both Atlantic herring and Atlantic mackerel show optimal suitability in northern seas, with scores of 0.93 and above in the Norwegian Sea, North Sea, and Irish Sea and St George’s Channel. Atlantic herring exhibits optimal suitability in the English Channel (0.95) and suboptimal suitability in the Celtic Sea (0.68). In contrast, their suitability dropped to zero in most of the Mediterranean regions, including the Western Basin, Ligurian Sea, Tyrrhenian Sea, Adriatic Sea, and Ionian Sea, with the only exception being the Eastern Basin, where both species also scored zero. Atlantic herring additionally showed poor suitability in the Aegean Sea (0.17) and suboptimal suitability in the Black Sea (0.58), while Atlantic mackerel shows a lower suitability in these regions, scoring 0.13 and 0, respectively. European seabass, however, exhibited a consistently optimal suitability score of 1 across all assessed regions.
3.2 Salinity and temperature-driven habitat shifts under the SSP5-8.5 climate change scenario
Projected under the SSP5-8.5 high-emission scenario, all three species, Atlantic herring, Atlantic mackerel, and European seabass are expected to undergo northward habitat shifts, with substantial losses in temperature-driven suitability across southern regions and gains in salinity-driven suitability in certain northern areas.
For example, by 2100, Atlantic herring is projected to shift 638 km northward, with its southern boundary moving from 44.2°N to 49.95°N (Figures 2, 3; Supplementary Table S4). In the Gulf of Finland, habitat suitability is predicted to decline sharply due to temperature changes, while salinity adjustments in regions like the Celtic Sea are expected to enhance suitability, creating new optimal zones. Similarly, Atlantic mackerel will lose up to 2.1 million km² of suitable habitat by 2100, with major declines in temperature-driven suitability in the Gulf of Finland and the Western Mediterranean, but salinity changes will improve conditions in the Celtic Sea. In contrast, European seabass shows remarkable stability in temperature-driven suitability, maintaining optimal conditions in most regions, with minimal influence from salinity. In the Gulf of Finland, however, European seabass will see a notable improvement in habitat suitability, transitioning from poor to optimal by 2100.
3.3 Salinity and temperature interaction
3.3.1 Non-Spawning trends
For non-spawning adult Atlantic herring and European seabass, the HSI remains consistently high across all SSP scenarios, particularly in the Northern European regions (Figure 4; Supplementary Table S7). For Atlantic herring, optimal habitats by 2100 are predicted in the Inner Seas off the West Coast of Scotland and Skagerrak, whereas suboptimal habitats are expected in the North Atlantic Ocean and the Gulf of Finland across all SSP scenarios. In the Baltic Sea, under the SSP5-8.5 scenario, habitat suitability is poor, while other scenarios still predict optimal habitats by 2100. Conversely, habitats in the southern waters are poor under all climate change scenarios investigated. The Mediterranean Sea, both Western and Eastern, shows a habitat suitability of zero by 2100 under SSP5-8.5, while the Black Sea’s HSI is expected to decline from suboptimal to poor under the same scenario by 2100.
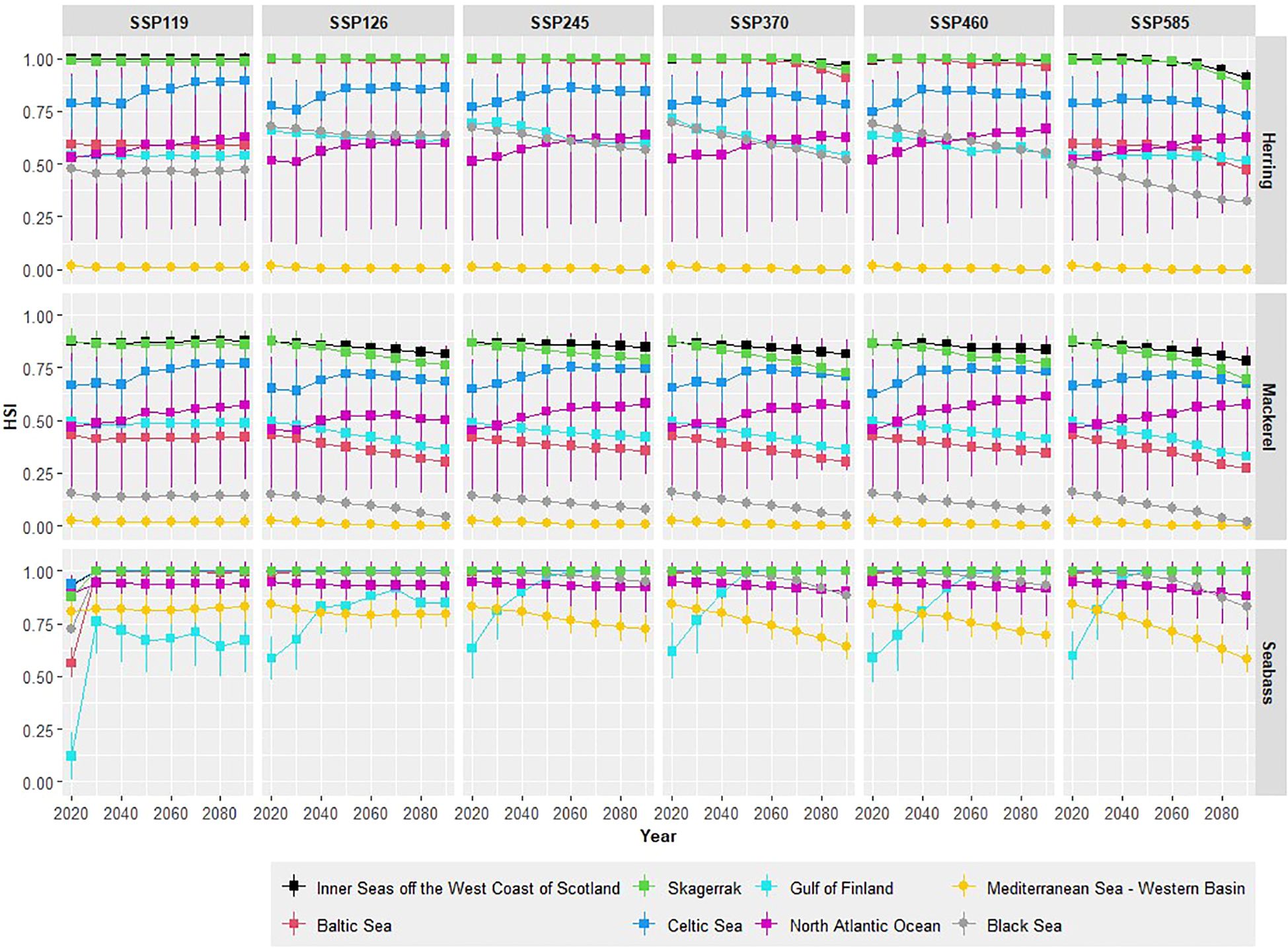
Figure 4. Illustration of the projected habitat suitability index (HSI) for non-spawning three fish species Herring, Mackerel, and Seabass, across different sea regions under six Shared Socioeconomic Pathway (SSP) scenarios: SSP119, SSP126, SSP245, SSP370, SSP460, and SSP585. The areas include the Inner Seas off the West Coast of Scotland, Baltic Sea, Mediterranean Sea (Western Basin), Skagerrak, Gulf of Finland, Celtic Sea, and North Atlantic Ocean. Each subplot shows the predicted changes in HSI over time, from 2020 to 2100. A square denotes Northern seas, while a dot denotes the southern seas.
For Atlantic mackerel, in the northern seas the Inner Seas off the West Coast of Scotland consistently exhibit optimal HSI values (i.e. HSI > 0.75) across all scenarios, though there’s a slight decline in the more extreme scenarios (Figure 4; Supplementary Table S7). The North Atlantic Ocean fluctuates between optimal and suboptimal HSI, with a general trend of slight increase over time (i.e. 1 < HSI > 0.5). The Baltic Sea starts with poor HSI (HSI = 0.44) and declines in most scenarios and the Gulf of Finland shifts from suboptimal (HSI = 0.50) to poor HSI particularly in higher-emission scenarios. In contrast, the southern seas present a different environment the Mediterranean Sea - Western Basin and the Black Sea consistently show poor HSI across all scenarios, with values below 0.25, indicating very low suitability for Atlantic mackerel (Figure 4; Supplementary Table S7).
European seabass displays a range of HSIs from optimal to suboptimal across different regions and SSP scenarios. In the Western Northern regions, HSI remains optimal across all SSPs. In the Northeastern part of the study area, HSI increases as the years progress (Figure 4). In the Southern part, particularly in the Western Mediterranean, habitat suitability decreases under SSP3-7.0, SSP4-6.0, and SSP5-8.5. Under the SSP5-8.5 scenario, habitat suitability in the Black Sea decreases but remains at an optimal level.
3.3.2 Spawning trends
In the northern seas, the suitable habitats for Atlantic herring are projected to increase over time in the Norwegian Sea and North Atlantic Ocean, while Atlantic mackerel is expected to see an increase in the North Atlantic Ocean (Figure 5; Supplementary Table S8). Specifically, in the North Atlantic Ocean, Atlantic herring suitability is expected to rise from 0.53 in 2010 to 0.63 by 2090-2100, while Atlantic mackerel suitability increases from poor 0.53 to 0.62 sub-optimal in the same period. The Norwegian Sea shows consistently high suitability for Atlantic herring across all timeframes (>0.95). In contrast, European seabass, which starts with a high suitability of 0.85 in the North Atlantic Ocean, experiences a significant decline to 0.77 by 2090-2100. In the North Sea, European seabass maintains a high suitability of 1.00 throughout the projected periods, indicating a stable or favorable environment for this species in these northern regions.
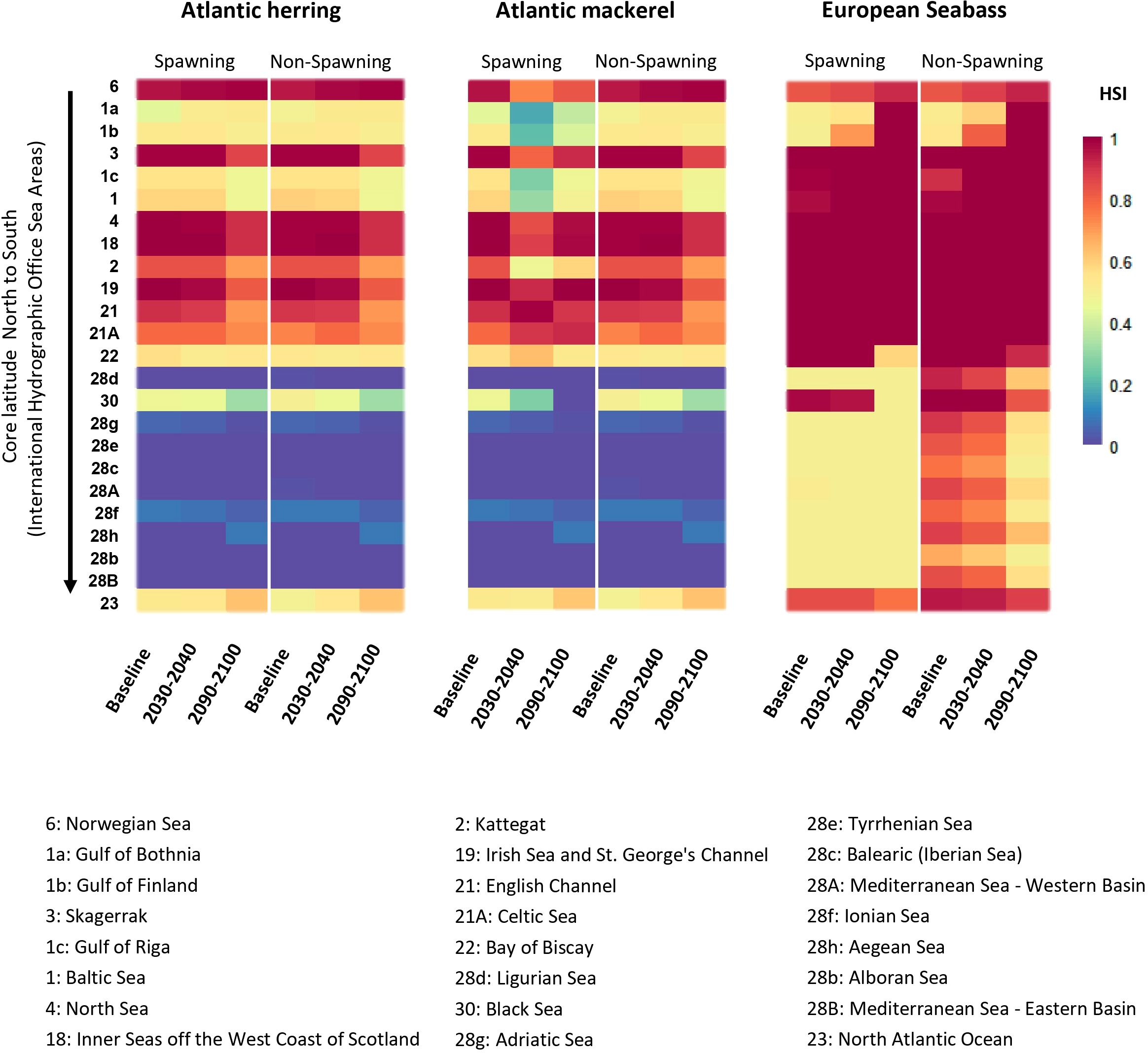
Figure 5. Heatmap of the temperature and salinity interaction projected habitat suitability for SSP5-8.5 for the pelagic fish for both non-spawning and spawning pelagic fish in European waters for the decades 2010, 2030 and 2090. The seas are arranged according to core latitude (North to South).
In Celtic Sea, Atlantic mackerel display notable improvements in habitat suitability. For example, in the Celtic Sea, Atlantic mackerel demonstrates a steady increase, reaching a peak suitability of 0.92 by the final period. However, European seabass suitability remains at 1.00 in the Celtic Sea throughout all time periods. In the Baltic Sea and its surrounding regions, such as the Gulf of Bothnia, Gulf of Riga, and Gulf of Finland, Atlantic herring starts with very low suitability, but the Gulf of Bothnia shows recovery by 2090-2100. At the same time, European seabass generally shows moderate improvements or remains stable in these central regions, particularly in the Gulf of Finland and Gulf of Bothnia, where its suitability increases to 1.00 by 2090-2100.
In the southern part of our study area, including the Mediterranean and its adjacent seas, the impacts of climate change appear more severe (Figure 5). Atlantic herring suitability is projected to remain in worse condition, with suitability close to zero in regions such as the Western Basin and Eastern Basin of the Mediterranean Sea. Similarly, Atlantic mackerel shows a reduction in suitability across most southern seas, with some areas, like the Mediterranean’s Ligurian and Tyrrhenian Seas, experiencing complete unsuitability for spawning. In contrast, European seabass presents a mixed pattern. While some regions like the Alboran Sea and Aegean Sea maintain or even increase their suitability for European seabass over time, others such as the Western Basin of the Mediterranean see a decline in suitability by 2090-2100.
3.4 Model validation
The occurrence points (OBIS, 2024) used for validation of Atlantic herring, Atlantic mackerel, and European seabass align well with areas of high habitat suitability, indicating a strong fit between observed presence and predicted suitable habitats. For Atlantic herring, the RMSE value of 0.29 indicates reasonably accurate predictions, particularly matching high suitability areas along the coasts of Norway and the North Sea. However, while herring occurrences in the southern Baltic Sea are higher, the predicted HSI values in this region are lower (Supplementary Figure S1). Similarly, Atlantic mackerel occurrence points closely correspond to highly suitable regions along the western coasts of Europe and the central North Atlantic, supported by an RMSE value of 0.4549. There are however occurrence points in the Mediterranean region where the study predicted low HSI (Supplementary Figure S1). For European seabass, with an RMSE value of 0.0184, presence is well-aligned with high suitability zones, despite the sparse occurrence points that do not fully reflect the geographical coverage of the species (Supplementary Figure S1). It is acknowledged that uneven data distribution across space and time can impact validation, necessitating efforts to match the spatio-temporal coverage of validation data to environmental data for more robust assessments.
4 Discussion
4.1 Predicted acclimatization patterns of non-spawning pelagic fish in European seas
The most critical finding of this study is the projected northward shift of over 500 kilometers for all studied fish species by 2100 under the SSP5-8.5 climate change scenario, driven primarily by temperature changes (Figure 2), along with the compounded impacts of both salinity and temperature variations across different European seas (Figures 4, 5; Supplementary Table S7, S8). The predicted latitudinal shift aligns with previous observations of marine species moving poleward due to rising temperatures (Chaudhary et al., 2021; Dulvy et al., 2009; Pinsky et al., 2020). Dulvy et al. (2009) reported historical latitudinal shifts of demersal fish in the North Sea, while Gordó-Vilaseca et al. (2023) observed a significant northward shift in the biomass of marine fish communities, with 20% of species in the Barents Sea and 25% in the North Sea displaying similar northward shifts. Furthermore, predictions of latitudinal shifts extend to other marine species such as algae (Westmeijer et al., 2019). These northward movements are also linked to reduced suitable habitats in southern regions, potentially leading to significant ecosystem changes. Due to each species’ unique ecological niche, these adaptation patterns can affect ecosystems variably, including shifts in predator-prey relationships, alterations in competition dynamics, and changes in community composition (Cheung and Frölicher, 2020).
The projected shifts in the HSI for Atlantic herring, Atlantic mackerel, and European seabass by 2100 have significant implications for marine ecosystems, biodiversity, and the economy, particularly in fisheries-dependent regions. These findings align with current research suggesting that climate change will drive substantial changes in species distributions and community structures (Doney et al., 2012; IPCC, 2022). For instance, the increased HSI for Atlantic mackerel in northern waters and the stable HSI for European seabass could lead to shifts in species dominance, consistent with studies predicting that warmer waters will favor certain species over others (Laevastu, 1993; Pinsky et al., 2020).
In southern regions, particularly the Mediterranean Seas and Black Sea, deteriorating habitat suitability for Atlantic mackerel suggests a range contraction and possible local extinctions. In the Mediterranean Sea, reduced habitat suitability for Atlantic mackerel and the persistence of European seabass may impact ecosystem resilience. Similar outcomes have been observed in other regions where altered predator-prey dynamics led to decreased biodiversity, such as the study by Doney et al. (2012) in the Southern California Current System (SCCS), where long-term studies have shown that functional complementarity among species is a key mechanism for reducing community variability and enhancing ecosystem stability despite significant environmental fluctuations.
These shifts could significantly impact fisheries, with short-term gains for Atlantic mackerel in northern regions but potential economic losses for Atlantic herring in areas such as the Baltic Sea, where population collapses have previously been observed (Dickey-Collas et al., 2010). In southern regions, the consistent decline in habitat suitability for economically important species like Atlantic mackerel could result in reduced fishery yields, threatening livelihoods and leading to economic instability in coastal communities. While the potential for European seabass to maintain or expand its range might offset some economic losses, this will depend on market demand and the species’ ability to support sustainable fisheries.
Overall, the projected changes in HSI for these key species stresses the need for adaptive management strategies that consider both ecological and economic impacts, ensuring the sustainability of fisheries-dependent communities.
4.2 Conservation and fisheries management strategies in a changing environment
Our study has revealed that climate-driven changes in habitat suitability for pelagic fish in European seas create a dynamic and challenging environment, particularly in the post-Brexit landscape. As warming waters push species like Atlantic herring, Atlantic mackerel, and European seabass northward, these shifts potentially encroach upon defined national boundaries, necessitating collaborative efforts to establish fair and sustainable fishing agreements across Exclusive Economic Zones (EEZs). The current lack of robust data sharing further complicates these efforts, affecting existing Marine Protected Areas (MPAs), which may require adjustments within national boundaries. This also stresses the need for renewed international cooperation for shared-water conservation, potentially revising existing agreements and creating new transnational MPAs. Our study will aid in addressing this challenge by providing information to inform future policy decisions. For example, our habitat suitability models can help identify critical areas for conservation.
Our findings on the changes in species spawning grounds to projected environmental changes illustrate the need for region-specific management and conservation strategies. For Atlantic herring and Atlantic mackerel, adaptive management that considers shifting spawning habitats and environmental conditions will be crucial (Petitgas et al., 2013). For European seabass, maintaining the health of key spawning grounds, particularly in northern and temperate regions, will be essential. These findings highlight the need for continuous monitoring and flexible management to sustain fisheries amid climate change. Changes in fish spawning habitats could affect the structure and function of marine ecosystems, as well as the livelihoods of communities dependent on these fisheries. Effective management strategies should incorporate climate models and ecological forecasts to anticipate and mitigate the impacts of environmental changes (Millar et al., 2007). Additionally, international cooperation will be vital in managing migratory species like Atlantic mackerel and Atlantic herring, ensuring that conservation efforts are harmonized across borders (ICES, 2018). The importance of such cooperation is underscored by recent research by Goossens et al. (2024) on European seabass in the North Sea, which revealed complex migration patterns and fine-scale population structuring. This study shows the need for cross-border fisheries management strategies that consider the specific ecological behaviors of species like seabass, whose movements may not align with current seasonal fisheries closures.
Post-Brexit, the UK’s departure from the CFP will require new bilateral agreements to address shifting stocks, complicating cooperative efforts due to differing priorities. The UK Fisheries Act 2020 aims to maintain sustainable fisheries and scientific evidence-based management but requires continuous negotiation with the EU and other coastal states, adding layers of complexity to managing shared resources effectively. The Trade and Cooperation Agreement (TCA) framework for cooperation will be essential in facilitating data sharing, access to waters, and dispute resolution, which are critical for adapting to climate-driven habitat changes. The TCA must be robust and flexible to respond to the dynamic nature of fish populations and ensure fair access and sustainable practices across borders. Our study provides critical scientific evidence to inform policy development and implementation under all these policies. By demonstrating the direct impacts of climate change on pelagic fish distribution and habitat, our research highlights the need for dynamic and adaptive quota-setting mechanisms within the CFP. Furthermore, the study emphasizes the importance of robust data sharing and scientific cooperation as outlined in the TCA, facilitating evidence-based decision-making and promoting sustainable fisheries management across borders. Our findings show the need to incorporate ecosystem-based approaches into policies to safeguard pelagic fish stocks.
Recognizing the adaptability of European seabass in our study, presents information for fisheries management to develop dynamic and location-specific strategies that can effectively safeguard marine biodiversity and support the livelihoods of communities dependent on fisheries resources. European seabass demonstrates a remarkable ability to thrive in diverse thermal and saline environments, which can serve as a model for resilient species management (Chatelier et al., 2005; Islam et al., 2020). By understanding and leveraging the adaptive capacity of species like European seabass, fisheries management can implement flexible approaches that account for changing environmental conditions and uncertainties associated with data limitations and modelling. This adaptive management framework allows for the adjustment of conservation and harvesting strategies in response to emerging scientific knowledge and real-time ecological feedback. Incorporating these uncertainties into decision-making processes enhances the resilience of marine ecosystems and ensures the long-term sustainability of fisheries resources for present and future generations. This approach not only promotes ecosystem health but also supports the socioeconomic well-being of coastal communities reliant on thriving fisheries industries.
The implications of these findings for Brexit and fisheries management in Europe are multifaceted. As the UK and the EU navigate separate regulatory frameworks, understanding the projected shifts in fish distribution and habitat suitability due to climate change becomes imperative (Bentley et al., 2017). With species like Atlantic mackerel facing potential habitat loss in areas such as the Baltic Sea, there may be heightened competition among nations for access to remaining fish stocks. The adaptability of other species, such as European seabass, stresses the importance of cooperative management frameworks post-Brexit.
In conclusion, climate change, fisheries management, and evolving legal frameworks in Europe present complex challenges. Effective management strategies must be adaptive, collaborative, and grounded in robust scientific evidence to ensure the long-term sustainability of marine ecosystems and the communities that depend on them. The integration of our study in policies such as the CFP, the UK Fisheries Act 2020, the TCA, and the MSFD within a cohesive and responsive framework will be essential in addressing the dynamic environmental changes and securing a sustainable future for Europe’s fisheries.
4.3 Limitations of the study
This study acknowledges limitations in solely using temperature and salinity as proxies for climate change. Temperature and salinity changes can ripple down the food chain, impacting plankton populations and fish species that rely on them (Free et al., 2019; Young et al., 2018). While acknowledging the crucial role of climate change impacts on other biotic communities and their influence on predator-prey relationships within pelagic fish species, this study focused solely on abiotic data for two key reasons. Firstly, comprehensive modelled climate-biological data were unavailable across the European seas at the time of publishing, limiting our ability to incorporate such complex interactions. Secondly, our understanding of the focal pelagic fish species and their key abiotic drivers suggests a strong primary influence of environmental factors on their habitat distributions. Previous research, including Montero-Serra et al. (2015), has emphasized temperature as a critical variable shaping the distribution of European pelagic fish. Recognizing the importance of abiotic factors, this study lays the groundwork for future research by establishing a robust understanding of abiotic drivers and their impact on habitat suitability under changing climate conditions. By focusing on readily available and reliable abiotic data, this initial assessment provides a clear and generalizable foundation for further studies that can integrate species-specific biotic interactions as data and modelling techniques improve.
While temperature and salinity significantly influence fish distribution, other abiotic variables like oxygen levels and CO2 concentrations can also play a crucial role. The limitation concerning other abiotic variables was the challenge in the acquisition of precise and extensive data concerning the physiological tolerances and ecological intricacies of diverse pelagic fish species across European marine environments. Species diversity introduces uncertainties in model parameterization, but temperature and salinity were chosen based on stronger evidence from the literature. To further validate this, our response curves were validated using occurrence data.
The study’s reliance on mechanistic modelling does not include the intricate web of biological interactions among different fish species and their broader ecosystem contexts. Furthermore, the computational demands associated with simulating these complex ecological processes at a regional scale may impose practical constraints, necessitating careful consideration of data availability and computational resources. These limitations underscore the importance of judiciously navigating the trade-offs between model complexity and computational feasibility. It is important to remember that modelling results are predictions, and the intricate dynamics of the ecosystem may lead to unforeseen outcomes. Ultimately, the model’s predictions should be viewed in the context of each species’ known physiological tolerances to temperature and salinity to ensure a more comprehensive understanding of climate change impacts.
While the study focused on surface temperature and salinity, we recognize that vertical distribution is a critical aspect of habitat suitability for pelagic species (Afonso et al., 2014; Hrabik et al., 2006). Many pelagic fish species exhibit diel vertical migration, moving between surface and deeper waters depending on the time of day, prey availability, and predator avoidance (Mehner and Kasprzak, 2011; Baker et al., 2023). By not incorporating vertical stratification into our model, we may have overlooked key habitat features that could either constrain or expand the potential distributions of these species. Specifically, we acknowledge that our model may overestimate habitat suitability for species like Atlantic mackerel, which are highly mobile and capable of extensive vertical migration. Atlantic mackerel can access deeper, cooler waters to mitigate unfavorable surface conditions, suggesting that our surface-focused model could overestimate habitat loss under warming scenarios (Edwards, 2009; Bernal, 2011). Conversely, for species like European seabass, which are generally associated with surface and nearshore environments, our model may underestimate sensitivity to environmental changes (Rodríguez-García et al., 2024). Additionally, while we used surface and benthic conditions from Bio-Oracle, derived from aggregated CMIP6 projections, we recognize that incorporating depth-specific CMIP6 layers in future studies would enhance model accuracy by capturing vertical dynamics more effectively. This approach, driven by the nature of the available occurrence data being predominantly surface-oriented, was necessary for consistency but limits the scope of our current model.
The observed alignment between general occurrence points and areas of optimal habitat suitability for Atlantic herring, Atlantic mackerel, and European seabass suggests that our habitat suitability index (HSI) models are generally reliable in predicting suitable habitats based on key environmental factors. The observed presence of Atlantic herring in optimal suitability areas, such as the coasts of Norway and the North Sea, underscores the model’s effectiveness in capturing the species’ habitat preferences, which are influenced by temperatures and optimal salinity levels. However, it is important to consider potential biases in the data due to over-sampling (Altmann, 1974). Atlantic herring and Atlantic mackerel have been extensively studied and sampled, particularly in commercially important regions, leading to a disproportionate representation of occurrence points in these areas as seen in this study. There are also discrepancies between our models and occurrences. One notable discrepancy observed in our model is the higher occurrences of herring in the southern Baltic Sea, which contrast with the lower predicted habitat suitability index (HSI) values in this region. This discrepancy may arise from a combination of factors not fully captured in the model. While the model relies primarily on abiotic variables such as temperature and salinity, it does not account for the full range of biotic and ecological processes that influence species distribution, such as prey availability, zooplankton dynamics, and predator interactions. These factors, which are likely more favorable in the southern Baltic Sea, may explain the observed higher occurrences of herring despite the lower HSI values. Additionally, the southern Baltic Sea is known for its complex hydrographic conditions, including seasonal variations in temperature and salinity, which could influence herring behavior and distribution in ways not fully represented by the abiotic predictors used in our model.
The HSI model for European seabass identifies suitability zones, but sparse occurrence data suggests incomplete geographical coverage. The fewer data points for European seabass may be attributed to the species being relatively novel in some of these areas, possibly due to recent shifts in distribution patterns influenced by environmental changes (Dambrine et al., 2020; De Pontual et al., 2023). This novelty could result in underreporting or gaps in data collection, illustrating the need for more comprehensive surveys and improved data collection methods. The limited occurrence points may also reflect the species’ broader tolerance to environmental variations, which allows them to inhabit a wide range of suitable habitats not fully represented in the dataset (Pickett and Pawson, 1994).
The performance of the HSI models for Atlantic herring and Atlantic mackerel illustrates the critical need for comprehensive and accurate occurrence data in validating predictive models. For European seabass, addressing data deficiencies would significantly enhance validation reliability, better capture the species’ environmental preferences, and provide a more accurate representation of habitat suitability. Additionally, the limited observation data in regions with a high HSI index indicates insufficient sampling efforts, potentially overlooking critical habitats or fishing grounds. Addressing these biases is essential. Future research should prioritize standardized and systematic sampling methods to reduce over-representation, ensure balanced data, and improve the robustness of habitat suitability models for broader ecological and management applications.
5 Conclusion
From our study, climate change-induced changes are projected to have significant impacts on the distribution of suitable habitats for Atlantic herring, Atlantic mackerel, and European seabass in European seas as early as 2030, with changes extending in magnitude until the predicted conditions for 2090. Responding to increasing water temperatures, Atlantic herring and Atlantic mackerel shifted 638.25 km and 799.2 km respectively northwards, and European seabass about 13.32 km northwards at SSP5-8.5 by 2090. In conclusion, this study emphasizes the need for adaptive management strategies to address the effects of climate change on pelagic fish distributions in a post-Brexit climate. To support these strategies, there is a need for international cooperation and data sharing. The knowledge gained here will contribute to more holistic fisheries management considering climate-induced habitat shifts.
Data availability statement
The original contributions presented in the study are included in the article/Supplementary Material. Further inquiries can be directed to the corresponding author.
Author contributions
RM: Conceptualization, Data curation, Formal analysis, Investigation, Methodology, Resources, Software, Validation, Visualization, Writing – original draft, Writing – review & editing. WS: Formal analysis, Investigation, Methodology, Writing – review & editing. MS: Conceptualization, Visualization, Writing – review & editing. SB: Methodology, Visualization, Writing – review & editing. CM: Project administration, Visualization, Writing – review & editing. ED: Funding acquisition, Project administration, Visualization, Writing – review & editing. SP: Conceptualization, Methodology, Supervision, Validation, Visualization, Writing – review & editing. GE: Conceptualization, Data curation, Funding acquisition, Methodology, Project administration, Supervision, Writing – review & editing.
Funding
The author(s) declare that financial support was received for the research, authorship, and/or publication of this article. This work was supported by internal funds from the Flanders Marine Institute (VLIZ), Belgium; European Commission’s Brexit Adjustment Reserve (BAR) – Veerkracht voor de kustvloot en klein vlootsegment post-Brexit (project No 1654).
Acknowledgments
We gratefully acknowledge Willem Boone for creating an interactive viewer of the model.
Conflict of interest
The authors declare that the research was conducted in the absence of any commercial or financial relationships that could be construed as a potential conflict of interest.
Generative AI statement
The author(s) declare that no Generative AI was used in the creation of this manuscript.
Publisher’s note
All claims expressed in this article are solely those of the authors and do not necessarily represent those of their affiliated organizations, or those of the publisher, the editors and the reviewers. Any product that may be evaluated in this article, or claim that may be made by its manufacturer, is not guaranteed or endorsed by the publisher.
Supplementary material
The Supplementary Material for this article can be found online at: https://www.frontiersin.org/articles/10.3389/fmars.2025.1501751/full#supplementary-material
Supplementary Figure 1 | Validation maps with habitat suitability index (HSI) maps based on both temperature and salinity for Atlantic herring (C. harengus) (A), Atlantic mackerel (S. scombrus) (B), and European seabass (D. labrax) (C). White dots show occurrence data retrieved from EurOBIS, aggregated to a hexagonal grid of 2 degrees. Point size shows the number of occurrences.
Supplementary Figure 2 | Habitat suitability index (HSI) difference maps based on temperature for adult non-spawning Atlantic herring [(C. harengus) (A, B)], Atlantic mackerel [(S. scombrus) (C, D)], and European seabass [(D. labrax) (E, F)]. Maps show differences from baseline suitability to SSP5-8.5 scenario for 2030-2040 (A, C, E) and SSP5-8.5 scenario for 2090-2100 (B, D, F).
Supplementary Figure 3 | Habitat suitability index (HSI) difference maps based on salinity for adult non-spawning Atlantic herring [(C. harengus) (A, B)], Atlantic mackerel [(S. scombrus) (C, D)], and European seabass [(D. labrax) (E, F)]. Maps show differences from baseline suitability to SSP5-8.5 scenario for 2030-2040 (A, C, E) and SSP5-8.5 scenario for 2090-2100 (B, D, F).
References
Afonso P., McGinty N., Graça G., Fontes J., Inácio M., Totland A., et al. (2014). Vertical migrations of a deep-sea fish and its prey. PloS One 9. doi: 10.1371/journal.pone.0097884
Altmann J. (1974). Observational study of behavior: sampling methods. Behaviour 49, 227–267. doi: 10.1163/156853974X00534
Asch R. G., Stock C. A., Sarmiento J. L. (2019). Climate change impacts on mismatches between phytoplankton blooms and fish spawning phenology. Global Change Biol. 25, 2544–2559. doi: 10.1111/gcb.14650
Assis J., Bejarano S. J. F., Salazar V. W., Schepers L., Gouvêa L., Fragkopoulou E., et al. (2024). Bio-ORACLE v3.0. Pushing marine data layers to the CMIP6 Earth System Models of climate change research. Global Ecol. Biogeogr. 33. doi: 10.1111/geb.13813
Assis J., Tyberghein L., Bosch S., Verbruggen H., Serrão E. A., De Clerck O. (2018). Bio-ORACLE v2.0: Extending marine data layers for bioclimatic modelling. Global Ecol. Biogeogr. 27, 277–284. doi: 10.1111/geb.12693
Aydogdu A., Miraglio P., Escudier R., Clementi E., Masina S. (2023). The dynamical role of upper layer salinity in the Mediterranean Sea. State Planet 1, 1–9. doi: 10.5194/sp-2022-11
Bagdonas K., Nika N., Bristow G., Jankauskiene R., Salyte A., Kontautas A. (2011). First record of Dicentrarchus labrax (Linnaeus 1758) from the southeastern Baltic Sea (Lithuania). J. Appl. Ichthyol. 27, 1390–1391. doi: 10.1111/j.1439-0426.2011.01817.x
Baker R. E., Peña J. M., Jayamohan J., Jérusalem A. (2018). Mechanistic models versus machine learning, a fight worth fighting for the biological community? Biol. Lett. 14, 1–4. doi: 10.1098/rsbl.2017.0660
Baker M., Smeltz T., Williams K., Greufe C., Ewing M., Chapman J., et al. (2023). Diel vertical migration in Pacific sand lance (Ammodytes personatus)—a pelagic forage fish associated with benthic substrates. ICES J. Mar. Sci. 80 (6), 1758–1772. doi: 10.1093/icesjms/fsad106
Bégout Anras M. L., Lagardère J. P. (1998). Variabilité météorologique et hydrologique. Conséquences sur l’activité natatoire d´un poisson marin. Biologie Animale CR Acad. Sci. Pris. Sci. la Vie 321, 641–648. doi: 10.1016/S0764-4469(98)80003-6
Bentley J. W., Serpetti N., Heymans J. J. (2017). Investigating the potential impacts of ocean warming on the Norwegian and Barents Seas ecosystem using a time-dynamic food-web model. Ecol. Model. 360, 94–107. doi: 10.1016/j.ecolmodel.2017.07.002
Bernal D. (2011). PELAGIC FISHES | An introduction to the biology of pelagic fishes 1887–1902. doi: 10.1016/B978-0-12-374553-8.00112-X
Bivand R., Keitt T., Rowlingson B. (2023). rgdal: bindings for the “Geospatial. Data Abstraction Library. Available at: http://rgdal.r-forge.r-project.org (Accessed July 22, 2024).
Bray N. A. (1982). Seasonal variability in the intermediate waters of the eastern North Atlantic. J. Phys. Oceanogr. 12, 972–983. doi: 10.1175/1520-0485(1982)012<0972:SVITIW>2.0.CO;2
Brevé N. P. W., van Emmerik W. A. M., van Beek G. C. W. (2007). Kennisdocument Atlantische haring. Sportvisserij Nederland.
Briscoe N., Morris S., Mathewson P., Buckley L., Jusup M., Levy O., et al. (2022). Mechanistic forecasts of species responses to climate change: The promise of biophysical ecology. Global Change Biol. 29, 1451–1470. doi: 10.1111/gcb.16557
Brunel T., van Damme C. J. G., Samson M., Dickey-Collas M. (2018). Quantifying the influence of geography and environment on the northeast Atlantic mackerel spawning distribution. Fish. Oceanogr. 27 (2), 159–173. doi: 10.1111/fog.12242
Brunel T., Dickey-Collas M. (2010). Effects of temperature and population density on von Bertalanffy growth parameters in Atlantic herring: A macro-ecological analysis. Mar. Ecol. Prog. Ser. 405, 15–28. doi: 10.3354/meps08491
Chatelier A., McKenzie D. J., Claireaux G. (2005). Effects of changes in water salinity upon exercise and cardiac performance in the European seabass (Dicentrarchus labrax). Mar. Biol. 147, 855–862. doi: 10.1007/s00227-005-1624-7
Chaudhary C., Richardson A. J., Schoeman D. S., Costello M. J. (2021). Global warming is causing a more pronounced dip in marine species richness around the equator. Proc. Natl. Acad. Sci. United States America 118, 1–6. doi: 10.1073/pnas.2015094118
Checkley D., Alheit J., Oozeki Y., Roy C. (2009). Climate Change and Small Pelagic Fish (Cambridge, United Kingdom: Cambridge University Press).
Cheung W. W. L., Frölicher T. L. (2020). Marine heatwaves exacerbate climate change impacts for fisheries in the northeast Pacific. Sci. Rep. 10. doi: 10.1038/s41598-020-63650-z
Claireaux G., Lagardère. J. P. (1999). Influence of temperature, oxygen and salinity on the metabolism of the European sea bass. J. Sea Res. 42, 157–168. doi: 10.1016/S1385-1101(99)00019-2
Conides A. J., Glamuzina B. (2006). Laboratory simulation of the effects of environmental salinity on acclimation, feeding and growth of wild-caught juveniles of European sea bass Dicentrarchus labrax and gilthead sea bream, Sparus aurata. Aquaculture 256, 235–245. doi: 10.1016/j.aquaculture.2006.02.029
Cushing D. H. (2001). Pelagic fishes. Encyclopedia Ocean Sci. 4, 364–369. doi: 10.1016/B978-012374473-9.00011-4
Dahlke F. T., Wohlrab S., Butzin M., Pörtner H. O. (2020). Thermal bottlenecks in the life cycle define climate vulnerability of fish. Science 369, 65–70. doi: 10.1126/science.aaz3658
Dambrine C., Huret M., Woillez M., Pecquerie L., Allal F., Servili A., et al. (2020). Contribution of a bioenergetics model to investigate the growth and survival of European seabass in the Bay of Biscay–English Channel area. Ecol. Model. 423, 109007. doi: 10.1016/j.ecolmodel.2020.109007
De Pontual H., Heerah K., Goossens J., Garren F., Martin S., Le Ru L., et al. (2023). Seasonal migration, site fidelity, and population structure of European seabass (Dicentrarchus labrax). ICES J. Mar. Sci. 80, 1606–1618. doi: 10.1093/icesjms/fsad087
Dickey-Collas M., Nash R. D. M., Brunel T., van Damme C. J. G., Marshall C. T., Payne M. R., et al. (2010). Lessons learned from stock collapse and recovery of North Sea herring: A review. ICES J. Mar. Sci. 67, 1875–1886. doi: 10.1093/icesjms/fsq033
Doney S. C., Ruckelshaus M., Emmett Duffy J., Barry J. P., Chan F., English C. A., et al. (2012). Climate change impacts on marine ecosystems. Annu. Rev. Mar. Sci. 4, 11–37. doi: 10.1146/annurev-marine-041911-111611
Ducrocq V. (2016). “Climate change in the Mediterranean region,” in The Mediterranean Region under Climate Change, a Scientific Update Allenvi (French National Alliance for Environmental Research), 71–104.
Dulvy N. K., Chassot E., Hyemans J., Hyde K., Pauly D., Platt T., et al. (2009). “Climate change, ecosystem variability and fisheries productivity,” in Remote Sensing in Fisheries and Aquaculture: The Societal Benefits, IOCCG Report, vol. 8, 11–28.
Edwards M. (2009). Sea life (Pelagic and planktonic ecosystems) as an indicator of climate and global change. In Climate Change, 233–251. doi: 10.1016/B978-0-444-53301-2.00012-9
European Commission: Joint Research Centre, Scientific, Technical and Economic Committee for Fisheries, Keatinge M., Guillen J., Carvalho N. (2019). The 2019 annual economic report on the EU fishing fleet (STECF 19-06). Eds. Keatinge M., Guillen J., Carvalho N. (Luxembourg: Publications Office).
Fernandez M., Sillero N., Yesson C. (2022). To be or not to be: the role of absences in niche modelling for highly mobile species in dynamic marine environments. Ecol. Model. 471, 110040. doi: 10.1016/j.ecolmodel.2022.110040
Flanders Marine Institute. (2025). MarineRegions.org. Available online at: www.marineregions.org (Accessed February 18, 2025).
Free C. M., Thorson J. T., Pinsky M. L., Oken K. L., Wiedenmann J., Jensen O. P. (2019). Impacts of historical warming on marine fisheries production. Science 365, 979–983. doi: 10.1126/science.aax5721
Fréon P., Cury P., Shannon L., Roy C. (2005). Sustainable exploitation of small pelagic fish stocks challenged by environmental and ecosystem changes: A review. Bull. Mar. Sci. 76, 385–462.
Froese R., Pauly D. (2024a). Dicentrarchus labrax (Linnaeus 1758) (World Register of Marine). Available at: https://www.marinespecies.org/aphia.php?p=taxdetails&id=126975 (Accessed August 13, 2024).
Froese R., Pauly D. (2024b). FishBase (World Wide Web Electronic Publication). Available at: www.fishbase.org (Accessed August 13, 2024).
Froese R., Pauly D. (2024c). Clupea harengus (Linnaeus 1758) (World Register of Marine). Available at: https://www.marinespecies.org/aphia.php?p=taxdetails&id=126417 (Accessed August 13, 2024).
Froese R., Pauly D. (2024d). Scomber scombrus (Linnaeus 1758) (World Register of Marine Species). Available at: https://www.marinespecies.org/aphia.php?p=taxdetails&id=127023 (Accessed August 13, 2024).
González-Gambau V., Olmedo E., Turiel A., González-Haro C., García-Espriu A., Martínez J., et al. (2022). First SMOS Sea Surface Salinity dedicated products over the Baltic Sea. Earth Syst. Sci. Data 14, 2343–2368. doi: 10.5194/essd-14-2343-2022
Goossens J., Woillez M., Wright S., Edwards J. E., De Putter G., Torreele E., et al. (2024). Elucidating the migrations of European seabass from the southern north sea using mark-recapture data, acoustic telemetry and data storage tags. Sci. Rep. 14, 1–15. doi: 10.1038/s41598-024-63347-7
Gordó-Vilaseca C., Pecuchet L., Coll M., Reiss H., Jüterbock A., Costello M. J. (2023). Over 20% of marine fishes shifting in the North and Barents Seas, but not in the Norwegian Sea. PeerJ 11, 1–23. doi: 10.7717/peerj.15801
Guille H., Gilmour C., Willsteed E. (2021). UK Fisheries Audit. Report produced by Macalister Elliott and Partners Ltd. for Oceana. Available online at: https://europe.oceana.org/en/uk-fisheries-audit-2021 (Accessed January 4, 2021).
Haegele C. W., Schweigert F. (1985). Distribution and characteristics of herring spawning grounds and description of spawning behavior. Can. J. Fish. Aquat. Sci. 42 (S1), s39–s55. doi: 10.1139/f85-261
Hagan S. M., Able K. W. (2003). Seasonal changes of the pelagic fish assemblage in a temperate estuary. Estuar. Coast. Shelf Sci. 56, 15–29. doi: 10.1016/S0272-7714(02)00116-6
Heath M., Cook R. (2020). Risks to North Sea fish stocks and wildlife if post-Brexit fishery negotiations fail to reach agreement on quotas and access to UK waters: Extended technical report. Glasgow: Worldwide Fund for Nature Scotland. doi: 10.17868/71708
Helm K. P., Bindoff N. L., Church J. A. (2010). Changes in the global hydrological-cycle inferred from ocean salinity. Geophys. Res. Lett. 37, 2–6. doi: 10.1029/2010GL044222
Hijmans R. J. (2024). terra: Spatial Data Analysis (R package version 1.8-2). Available online at: https://github.com/rspatial/terra (Accessed November 20, 2024).
Hijmans R. J., Phillips S., Leathwick J., Elith J. (2023). dismo: Species Distribution Modeling. Available online at: https://CRAN.R-project.org/package=dismo (Accessed November 29, 2023).
Hodson T. O. (2022). Root-mean-square error (RMSE) or mean absolute error (MAE): when to use them or not. Geoscientific Model. Dev. 15, 5481–5487. doi: 10.5194/gmd-15-5481-2022
Holt J., Wakelin S., Lowe J., Tinker J. (2010). Progress in Oceanography The potential impacts of climate change on the hydrography of the northwest European continental shelf. Prog. Oceanogr. 86, 361–379. doi: 10.1016/j.pocean.2010.05.003
Hrabik T. R., Jensen O. P., Martell S. J. D., Walters C. J., Kitchell J. F. (2006). Diel vertical migration in the Lake Superior pelagic community. I. Changes in vertical migration of coregonids in response to varying predation risk. Can. J. Fish. Aquat. Sci. 63, 2286–2295. doi: 10.1139/F06-124
Hyder K., Weltersbach M. S., Armstrong M., Ferter K., Townhill B., Ahvonen A., et al. (2018). Recreational sea fishing in Europe in a global context—participation rates, fishing effort, expenditure, and implications for monitoring and assessment. Fish Fish. 19, 225–243. doi: 10.1111/faf.12251
Ibaibarriaga L., Irigoien X., Santos M., Motos L., Fives J. M., Franco C., et al. (2007). Egg and larval distributions of seven fish species in north-east Atlantic waters. Fish. Oceanogr. 16, 284–293. doi: 10.1111/j.1365-2419.2007.00430.x
ICES (2018). Report of the Benchmark Workshop on Pelagic Stocks (WKPELA 2018). 12–16 February 2018 ICES HQ, Copenhagen, Denmark (ICES ADVISORY COMMITTEE), 509p. Available at: https://archimer.ifremer.fr/doc/00444/55562/. Ref. ICES CM 2018/ACOM:32.
Illing B., Downie A. T., Beghin M., Rummer J. L. (2020). Critical thermal maxima of early life stages of three tropical fishes: Effects of rearing temperature and experimental heating rate. J. Thermal Biol. 90, 102582. doi: 10.1016/j.jtherbio.2020.102582
Intergovernmental Panel on Climate Change (2023). “Climate change 2021 – the physical science basis,” in Climate Change 2021 – The Physical Science Basis. (Cambridge, United Kingdom, and New York, NY, USA: Cambridge University Press). doi: 10.1017/9781009157896
IPCC (2022). “Climate change 2022: impacts, adaptation, and vulnerability,” in Contribution of Working Group II to the Sixth Assessment Report of the Intergovernmental Panel on Climate Change. Eds. Pörtner H.-O., Roberts D. C., Tignor M., Poloczanska E. S., Mintenbeck K., Alegría A., Craig M., Langsdorf S., Löschke S., Möller V., Okem A., Rama B., Roberts D. C., Tignor M., Poloczanska E. S., Mintenbeck K., Ale A. (Cambridge University Press, Cambridge, UK), 3056. doi: 10.1017/9781009325844
Islam M. J., Slater M. J., Kunzmann A. (2020). What metabolic, osmotic and molecular stress responses tell us about extreme ambient heatwave impacts in fish at low salinities: The case of European seabass, Dicentrarchus labrax. Sci. Total Environ. 749, 141458. doi: 10.1016/j.scitotenv.2020.141458
Jansen T., Campbell A., Kelly C., Hátún H., Payne M. R. (2012). Migration and fisheries of North East Atlantic mackerel (Scomber scombrus) in autumn and winter. PloS One 7, e51541. doi: 10.1371/journal.pone.0051541
Johnson D. (2006). Predation, habitat complexity, and variation in density-dependent mortality of temperate reef fishes. Ecology 87 (5), 1179–1188. doi: 10.1890/0012-9658(2006)87[1179:phcavi]2.0.co;2
Kaschner K., Kesner-Reyes K., Garilao C., Segschneider J., Rius-Barile J., Rees T., et al. (2019). AquaMaps: Predicted range maps for aquatic species. Available online at: https://www.aquamaps.org (Accessed April 23, 2024).
Kearney M., Porter W. (2009). Mechanistic niche modelling has emerged as a valuable tool for assessing the environmental conditions that influence the distribution and abundance of diverse populations. Ecol. Lett. 12, 334–350. doi: 10.1111/j.1461-0248.2008.01277.x
Kvaavik C., Óskarsson G. J., Daníelsdóttir A. K., Marteinsdottir G. (2019). Diet and feeding strategy of Northeast Atlantic mackerel (Scombrus scomber) in Icelandic waters. PloS One 14, e0225552. doi: 10.1371/journal.pone.0225552
Laevastu T. (1993). Marine climate, weather and fisheries: the effects of weather and climatic changes on fisheries and ocean resources. Oxford, United Kingdom: Fishing News Books.
Lehmann A., Myrberg K., Post P., Chubarenko I., Dailidiene I., Hinrichsen H. H., et al. (2022). Salinity dynamics of the Baltic Sea. Earth Syst. Dynamics 13, 373–392. doi: 10.5194/esd-13-373-2022
Leibold M. A. (1995). The niche concept revisited: mechanistic models and community context. Ecology 76, 1371–1382. doi: 10.2307/1938141
Maravelias C. D. (2001). Habitat associations of Atlantic herring in the Shetland area: Influence of spatial scale and geographic segmentation. Fish. Oceanogr. 10, 259–267. doi: 10.1046/j.1365-2419.2001.00172.x
Maravelias C. D., Reid D. G., Swartzman G. (2000). Modelling spatio-temporal effects of environment on Atlantic herring, Clupea harengus. Environ. Biol. Fishes 58, 157–172. doi: 10.1023/A:1007693732571
Mason D., Vincent P. E. (2011). Transactions of the American fisheries society A model for the space – time dependence of feeding for pelagic fish populations. Trans. Am. Fish. Soc. 122, 884–891. doi: 10.1577/1548-8659(1993)122<0884:AMFTST>2.3.CO;2
Mehner T., Kasprzak P. (2011). Partial diel vertical migrations in pelagic fish. J. Anim. Ecol. 80 4, 761–770. doi: 10.1111/j.1365-2656.2011.01823.x
Millar C. I., Stephenson N. L., Stephens S. L. (2007). Climate change and forests of the future: Managing in the face of uncertainty. Ecol. Appl. 17, 2145–2151. doi: 10.1890/06-1715.1
Montero-Serra I., Edwards M., Genner M. J. (2015). Warming shelf seas drive the subtropicalization of European pelagic fish communities. Global Change Biol. 21, 144–153. doi: 10.1111/gcb.12747
Morizur Y., Tregenza N., Heessen H., Berrow S., Pouvreau S. (1996). By-catch and Discarding in Pelagic Trawl Fisheries. Available online at: http://archimer.ifremer.fr/doc/00000/1236/ (Accessed January 16, 2024).
Muhling B., Lindegren M., Clausen L. W., Hobday A., Lehodey P. (2017). Impacts of climate change on pelagic fish and fisheries. Climate Change Impacts Fish. Aquac. II, 771–814. doi: 10.1002/9781119154051.ch23
Munday P. L., Jones G. P., Pratchett M. S., Williams A. J. (2008). Climate change and the future for coral reef fishes. Fish Fish. 9, 261–285. doi: 10.1111/j.1467-2979.2008.00281.x
Munroe T. A. (2002). “Herrings. Family clupeidae,” in Bigelow and Schroeder’s fishes of the Gulf of Maine (3rd ed.). (Washington DC: Smithsonian Institution Press), 122–160.
Nielsen M., Andersen P., Ravensbeck L., Laugesen F., Kristófersson D. M., Ellefsen H. (2017). Fisheries management and the value chain: The Northeast Atlantic pelagic fisheries case. Fish. Res. 186, 36–47. doi: 10.1016/j.fishres.2016.08.004
OBIS (2024). Ocean Biodiversity Information System (Intergovernmental Oceanographic Commission of UNESCO). Available at: www.obis.org (Accessed February 19, 2024).
Pankhurst N. W., Munday P. L. (2011). Effects of climate change on fish reproduction and early life history stages. Mar. Freshw. Res. 62, 1015–1026. doi: 10.1071/MF10269
Paoletti S., Nielsen J. R., Sparrevohn C. R., Bastardie F., Vastenhoud B. M. J. (2021). Potential for mesopelagic fishery compared to economy and fisheries dynamics in current large scale Danish pelagic fishery. Front. Mar. Sci. 8. doi: 10.3389/fmars.2021.720897
Pebesma E., Bivand R. (2005). Classes and methods for spatial data in R. R News 5, 9–13. Available at: https://CRAN.R-project.org/doc/Rnews/ (Accessed November 22, 2024).
Peck M. A., Reglero P., Takahashi M., Catalán I. A. (2013). Life cycle ecophysiology of small pelagic fish and climate-driven changes in populations. Prog. Oceanogr. 116, 220–245. doi: 10.1016/j.pocean.2013.05.012
Peltonen H., Luoto M., Pääkkönen J. P., Karjalainen M., Tuomaala A., Pönni J., et al. (2007). Pelagic fish abundance in relation to regional environmental variation in the Gulf of Finland, northern Baltic Sea. ICES J. Mar. Sci. 64, 487–495. doi: 10.1093/icesjms/fsl044
Perry A. L., Low P. J., Ellis J. R., Reynolds J. D. (2005). Climate change and distribution shifts in marine fishes. Science. Science 308, 1912–1915. doi: 10.1126/science.1111322
Petitgas P., Rijnsdorp A. D., Dickey-Collas M., Engelhard G. H., Peck M. A., Pinnegar J. K., et al. (2013). Impacts of climate change on the complex life cycles of fish. Fish. Oceanogr. 22, 121–139. doi: 10.1111/fog.12010
Phillipson J., Symes D. (2018). A sea of troubles”: Brexit and the fisheries question. Mar. Policy 90, 168–173. doi: 10.1016/j.marpol.2017.12.016
Pickett G. D., Pawson M. G. (1994). Sea bass: biology, exploitation and conservation (Lowestoft, Suffolk, United Kingdom: Springer Science & Business Media).
Pinsky M. L., Selden R. L., Kitchel Z. J. (2020). Climate-driven shifts in marine species ranges: scaling from organisms to communities. Annu. Rev. Mar. Sci. 12, 153–179. doi: 10.1146/annurev-marine-010419-010916
R Core Team (2023). R: A Language and Environment for Statistical Computing (R Foundation for Statistical Computing). Available at: https://www.R-project.org/ (Accessed November 29, 2023).
Rijnsdorp A. D., Peck M. A., Engelhard G. H., Möllmann C., Pinnegar J. K. (2009). Resolving the effect of climate change on fish populations. ICES J. Mar. Sci. 66, 1570–1583. doi: 10.1093/icesjms/fsp056
Rodríguez-García C., Toro-Podadera A., Sarmiento-Carbajal J., Cabrera-Castro R. (2024). Coexisting in the Surf Zone: Age and Feeding Habits of the Spotted Seabass (Dicentrarchus punctatus) and European Seabass (Dicentrarchus labrax) on the Gulf of Cádiz Beaches (Southwest Iberian Peninsula). Fishes. 9 (5), 173. doi: 10.3390/fishes9050173
Rose G. A. (2005). On distributional responses of North Atlantic fish to climate change. ICES J. Mar. Sci. 62, 1360–1374. doi: 10.1016/j.icesjms.2005.05.007
Röthig T., Trevathan-Tackett S. M., Voolstra C. R., Ross C., Chaffron S., Durack P. J., et al. (2023). Human-induced salinity changes impact marine organisms and ecosystems. Global Change Biol. 29, 4731–4749. doi: 10.1111/gcb.16859
Ruprich-Robert Y., Cassou C. (2015). Combined influences of seasonal East Atlantic Pattern and North Atlantic Oscillation to excite Atlantic multidecadal variability in a climate model. Climate Dynamics 44, 229–253. doi: 10.1007/s00382-014-2176-7
Russell N. R., Fish J. D., Wootton R. J. (1996). Feeding and growth of juvenile sea bass: the effect of ration and temperature on growth rate and efficiency. J. Fish Biol. 49, 206–220. doi: 10.1111/j.1095-8649.1996.tb00017.x
Rykiel E. J. J. (1995). Testing ecological models: the meaning of validation. Ecol. Model. 90, 229–244. doi: 10.1111/j.1467-971X.1990.tb00262.x
Schatz V. J. (2020). The International legal framework for post-Brexit EEZ fisheries access between the United Kingdom and the European Union. Int. J. Mar. Coast. Law 35, 133–162. doi: 10.1163/15718085-23343067
Schickele A., Goberville E., Leroy B., Beaugrand G., Hattab T., Francour P., et al. (2021). European small pelagic fish distribution under global change scenarios. Fish Fish. 22, 212–225. doi: 10.1111/faf.12515
Schroeder W. A., Legette J. (1953). A study of the quantitative dinitrophenylation of amino acids and peptides. J. Am. Chem. Soc. 75, 4612–4615. doi: 10.1021/ja01114a531
Singh N. R., Love B., Murray C. S., Sobocinski K. L., Cooper W. J. (2023). The combined effects of acidification and acute warming on the embryos of Pacific herring (Clupea pallasii). Front. Mar. Sci. 10, 1307617. doi: 10.3389/fmars.2023.1307617
Smith A. N. H., Anderson M. J. (2016). Marine reserves indirectly affect fine-scale habitat associations, but not overall densities, of small benthic fishes. Ecol. Evol. 6, 6648–6661. doi: 10.1002/ece3.2406
Stevenson D., Scott M. (1999). Essential fish habitat source document: Atlantic herring, Clupea harengus, life history and habitat characteristics (NOAA Technical). Available at: https://repository.library.noaa.gov/view/noaa/3101.
Stigebrandt A., Gustafsson B. G. (2003). Response of the Baltic Sea to climate change - Theory and observations. J. Sea Res. 49, 243–256. doi: 10.1016/S1385-1101(03)00021-2
Symes D., Phillipson J. (2019). [amp]]lsquo;A sea of troubles’ (2): Brexit and the UK seafood supply chain. Mar. Policy 102, 5–9. doi: 10.1016/j.marpol.2019.01.015
Thompson B. M., Harrop R. T. (2009). The distribution and abundance of bass (Dicentrarchus labrax) eggs and larvae in the English Channel and Southern North Sea. J. Mar. Biol. Assoc. United Kingdom 67, 263–274. doi: 10.1017/S0025315400026588
Tidbury H. J., Muench A., Lamb P. D., Hyder K. (2021). Balancing biological and economic goals in commercial and recreational fisheries: systems modelling of sea bass fisheries. ICES J. Mar. Sci. 78, 1793–1803. doi: 10.1093/icesjms/fsab087
Townhill B. L., Couce E., Tinker J., Kay S., Pinnegar J. K. (2023). Climate change projections of commercial fish distribution and suitable habitat around north western Europe. Fish Fish. 24, 848–862. doi: 10.1111/faf.12773
Trenkel V. M., Huse G., MacKenzie B. R., Alvarez P., Arrizabalaga H., Castonguay M., et al. (2014). Comparative ecology of widely distributed pelagic fish species in the North Atlantic: implications for modelling climate and fisheries impacts. Prog. Oceanogr. 129, 219–243. doi: 10.1016/j.pocean.2014.04.030
Tyberghein L., Verbruggen H., Pauly K., Troupin C., Mineur F., De Clerck O. (2012). Bio-ORACLE: A global environmental dataset for marine species distribution modelling. Global Ecol. Biogeogr. 21, 272–281. doi: 10.1111/j.1466-8238.2011.00656.x
van Balsfoort G., Barnard C., Rosello M., Sánchez M. N., Barnes R., Bourke E., et al. (2022). A synoptic overview of expert opinion on fisheries in a post-brexit world. Fish. Law Europe: Regul. After Brexit, 122–149. doi: 10.4324/9781003252481-9
Vandeputte M., Gagnaire P. A., Allal F. (2019). The European sea bass: a key marine fish model in the wild and in aquaculture. Anim. Genet. 50, 195–206. doi: 10.1111/age.12779
Velotta J. P., Mccormick S. D., Whitehead A., Durso C. S., Schultz E. T. (2022). Repeated genetic targets of natural selection underlying adaptation of fishes to changing salinity. Integr. Comp. Biol. 62, 357–375. doi: 10.1093/icb/icac072
Westmeijer G., Everaert G., Pirlet H., De Clerck O., Vandegehuchte M. B. (2019). Mechanistic niche modelling to identify favorable growth sites of temperate macroalgae. Algal Res. 41, 101529. doi: 10.1016/J.ALGAL.2019.101529
Wickham H., François R., Henry L., Müller K., Vaughan D. (2023). dplyr: A Grammar of Data Manipulation (R package version 1.1.4). Available online at: https://dplyr.tidyverse.org (Accessed November 20, 2024).
Young E. F., Tysklind N., Meredith M. P., de Bruyn M., Belchier M., Murphy E. J., et al. (2018). Stepping stones to isolation: Impacts of a changing climate on the connectivity of fragmented fish populations. Evolutionary Appl. 11, 978–994. doi: 10.1111/eva.12613
Keywords: climate change, mechanistic niche modelling, pelagic fish, habitat suitability index, species-specific response curves
Citation: Musimwa R, Standaert W, Stevens M, Fernández Bejarano SJ, Muñiz C, Debusschere E, Pint S and Everaert G (2025) Climate-induced habitat suitability modelling for pelagic fish in European seas. Front. Mar. Sci. 12:1501751. doi: 10.3389/fmars.2025.1501751
Received: 25 September 2024; Accepted: 10 February 2025;
Published: 27 February 2025.
Edited by:
Shin-ichi Ito, The University of Tokyo, JapanCopyright © 2025 Musimwa, Standaert, Stevens, Fernández Bejarano, Muñiz, Debusschere, Pint and Everaert. This is an open-access article distributed under the terms of the Creative Commons Attribution License (CC BY). The use, distribution or reproduction in other forums is permitted, provided the original author(s) and the copyright owner(s) are credited and that the original publication in this journal is cited, in accordance with accepted academic practice. No use, distribution or reproduction is permitted which does not comply with these terms.
*Correspondence: Rutendo Musimwa, cnV0ZW5kby5tdXNpbXdhQHZsaXouYmU=