- Zhejiang Provincial Center for Disease Control and Prevention, Department of Occupational Health and Radiological Protection, Hangzhou, Zhejiang, China
Introduction: Tritium exists in the environment primarily in the form of tritiated water (HTO). There is a significant correlation between the radioactive levels of tritium in seawater and the safety of seafood. By accurately and rapidly detecting the tritium activity concentration in seawater, it is possible to effectively assess its potential impact on the seafood supply chain. At present, the detection of tritium in environmental water samples is often by liquid scintillation counting (LSC). To enable the measure of tritium by LSC, sample purification is necessary, which is commonly performed by distillation. The sample purification by distillation method is somewhat time consuming, especially when processing many samples or large volumes.
Methods: To improve the analytical efficiency in tritium determination, a method for the purification of tritium in seawater using ion exchange resin was developed in this work, which is highly suitable for large-scale environmental monitoring in emergency situations as it significantly shortens the sample processing time (A sample can be purified in 10 min, and multiple samples can be processed simultaneously, which is at least 50 min shorter than the distillation method). The influence of the 90Sr-90Y on the detection of tritium in seawater treated with ion-exchange resin was also discussed. The removal of metal ions from the seawater was achieved using magnetic agitation and filtration by mixing the sample with ion exchange resins.
Results: The results show that the ion exchange resin can effectively remove metal ions from seawater, and reduce the conductivity of seawater to< 1 μS/cm, and effectively reduce the influence of the 90Sr-90Y on tritium detection. The method was validated using spiked samples and compared with other methods.
Discussion: The recovery of tritium for standard solutions treated by ion exchange method ranged from 85.1% to 98.45%, indicating satisfactory efficiency in sample purification for tritium determination in seawater. 10 seawater samples were collected and used to verify the applicability of the method.
1 Introduction
Tritium (3H, t½=12.3 y) is a radioisotope of hydrogen that is a pure β-decay particle. Tritium is produced both naturally and by human nuclear activities (Kaizer et al., 2023; Oms et al., 2019; Wallova et al., 2020). During the operation of nuclear power plants, waste water containing radioactive substances is inevitably discharged into the environment, among which tritium is one of the primary radionuclides (Adam-Guillermin et al., 2010; Guo et al., 2020; Köllö et al., 2010; Liang et al., 2022). The Japanese government has begun dumping contaminated radioactive wastewater from the Fukushima Daiichi Nuclear Power Plant (FDNPP) into the ocean on Aug. 23, 2023, which contains a large amount of tritium (Subban and Gadgil, 2019). After the discharge of nuclear wastewater or sewage into the sea (Dallas et al., 2016), tritium can be rapidly incorporated into marine organisms either by uptake from seawater or by food ingestion (Alava and Gobas, 2016; Madigan et al., 2012; Yankovich et al., 2011), thereby enters the human body through the food chain. Tritium can pose a health risk if ingested through drinking water or food, inhaled in the respiratory tract, or absorbed through the skin (Butler and Leroy, 1965; Matsumoto et al., 2021). Although tritium belongs to the low radiotoxicity group (Buesseler, 2020; Iryna, 2017; Men, 2021), once entering into human body, tritium may lead to long-term radiation-induced oxidative damage to cells (Gagnaire et al., 2020; Li et al., 2021; Rozhko et al., 2016), genotoxic and reprotoxic effects (Adam-Guillermin et al., 2012; Wakeford, 2014; Wanigaratne et al., 2013), and damages of DNA (Hatano et al., 2022).
There is a close connection between the radioactivity of seawater and seafood safety, as tritium produced by nuclear power plants can affect the safety of seafood through the food chain. Marine organisms absorb and accumulate tritium from their environment during their growth, and this accumulation effect can lead to tritium concentrations in seafood that are higher than those in the surrounding water. Monitoring tritium activity concentration in seawater can help identify and assess potential sources of contamination in a timely manner, allowing for appropriate measures to reduce or eliminate the impact of tritium on the environment and human health. This is essential for ensuring that the public can safely utilize seawater and consume seafood from the ocean. Therefore, method establishment for effective determination of tritium in seawater is important for radioanalytical experiment involved in nuclear emergency preparedness and environmental monitoring.
The liquid scintillation counting (LSC) is the most used method for its detection. Chemical and color quenching are typical challenges encountered when using LSC for sample measurement. In general, removing impurities from environmental water samples is necessary to reduce the chemical quenching for a highly precise tritium analysis using the liquid scintillation counting method. Therefore, to ensure accurate detection of tritium by LSC, effective impurities removal becomes extremely important. Standard procedures for the purification of tritium in water samples are usually performed by distillation (Varlam et al., 2009). However, the distillation process is time-consuming (Sakuma et al., 2012). In addition, the distillation process may result in isotopic fractionation between tritium and hydrogen during the evaporation process, introducing bias in tritium measurement (Atkinson et al., 2014).
With regards to water purification, several methods have been reported including reverse osmosis, filtration, ion exchange and distillation etc. Among which, ion exchange is widely used for water softening, pure water production (Iourtchouk and Bergmann, 2012; Lee et al., 2020), wastewater purification and seawater desalination (He et al., 2020), owning some attractive advantages, such as fast reaction kinetics, low cost, and high reliability (Ma et al., 2019; Subban and Gadgil, 2019). However, there are only some reports on the purification of tritium from seawater by ion exchange resin, and the results obtained are different according to the types of resin used, and there is a certain gap in the recovery rate of tritium standard solution (Nakasone et al., 2021; Tarancón et al., 2010).
For environmental-level seawater samples, even after purification, the low concentrations of HTO and significant dilution of regular water still make the direct measurement of tritium by LSC difficult (Janković et al., 2012). For such cases, further concentration of tritium with e.g., electrolytic enrichment, is needed (Garbarino et al., 2009; Groning et al., 2009; Kumar et al., 2016), while high purity of water sample is also required before the concentration treatment.
This study aims to develop an improved and rapid method for the determination of tritium in seawater samples by LSC after purification using ion exchange resin. In this study, several ion exchange resins were screened and performances for seawater purification were investigated. The accuracy of the method was validated using spiked samples and the results were compared with the traditional atmospheric distillation method. The method performance was evaluated using parameters of recovery, precision, and trueness.
2 Experimental
2.1 Selection of resin
The selected ion exchange resin for seawater adsorption should possess appropriate selectivity, rapid exchange rate, high exchange capacity, and resistance to physical rupture. Additionally, it should be easy to regenerate and economically affordable. In this work, 5 commercially available and inexpensive resins were selected for purification of seawater. Listed in Table 1 of 5 kinds of typical characteristics of ion exchange resin, and the water purification tests. As electrical conductivity is a straightforward indicator for the purity of a liquid solution, thus we utilized the changes of electrical conductivity before and after sample purification to evaluate the efficiency of matrix elements removal from seawater. The electrical conductivity of seawater collected from the South China Sea, the waters near the Republic of Kiribati, the sea near the Mariana Trench, the western Pacific Ocean and the Southwestern Indian Ocean ranges between 3×104 - 6×104 μS/cm (Zheng et al., 2018).
In order to select the appropriate resin, to 20 mL of seawater, 5, 10, 11, 12, 13, 14, 15 or 20 g of MB-20, MB-106UP, MB-450, T-42 or CH-99 resin was added and mixed in a beaker for 5 min with stirring. The seawater sample was filtered through a 0.22 µm hydrophilic filter (Polytetrafluoroethylene, diameter of 13.00 mm, Shanghai Anpu Experimental Technology Co., Ltd.) and its conductivity (Seven Excellence, METTLER TOLEDO) was measured. The resin that can significantly reduce the conductivity of seawater was selected for subsequent experiments.
2.2 ICP-OES measurement
Inductively coupled plasma optical emission spectrometry (ICP-OES, ICAP PRO, Thermo Scientific) was mainly used to determine the content of metal ions in solutions. To compare the changes in the types and contents of metal ions before and after purification of seawater, ICP-OES was used to measure metal ions in seawater treated with MB-20 resin, MB-106UP resin, MB-450 resin, and atmospheric distillation after dilution for 100-fold.
The measurement of 27 metal ions such as Ca, K, Na, Mg, Cu, Mn, Fe and Zn in seawater were performed under the following operating conditions: air/acetylene flow of 13.50/2.00 L min-1; burner height and still width of 13.5 and 0.2 - 1.0 mm; lamp current of 4-10 mA. The wavelengths and detection limits of 31 metal ions are shown in Table 2. Moreover, calibration curves were prepared from standard solutions of each metal with linear correlation of determinations above 0.999.
2.3 EDS, SEM and XPS
Energy dispersive spectrometer (EDS, X-max 50, OXFORD), scanning electron microscopy (SEM, Sigma 300, ZEISS) and X-ray photoelectron spectroscopy (XPS, K-Alpha, Thermo Scientific) were performed for the resin material before and after the purification process. To prepare the processed resin material, 15 g of MB-20, MB-106UP or MB-450 resin was mixed with 20 mL of seawater in a beaker and stirred with a on a magnetic stirrer for 30 minutes. After filtering the mixture through a 0.22 µm filter, the resin was dried in an oven at 60°C for 5 h. 5g of dried ion-exchange resin and unused resin were ground into uniform powder samples, respectively, and then used for EDS, SEM, and XPA detection.
2.4 Conditions for screening
1. 5, 6, 7, 8, 9, 10, 11, 12, 13, 14, and 15 g of MB-20 resin and 20 mL of seawater were magnetically stirred for 5 min. The conductivity was measured and recorded, and the experiment was repeated 3 times.
2. The mixed samples of 9, 10, and 11 g of resin with 20 mL of seawater were magnetically stirred, and the electrical conductivity was measured at 1, 2, 3, 4, 5, 10, 15, 20, and 30 min, respectively.
2.5 Method verification
2.5.1 Comparison of recovery rate
In this study, 3 spiked samples with tritium concentrations of 5.1, 51 and 102 Bq/L were prepared by spiking different amount (0.25, 2.5 and 5 mL) of a tritium standard solution (1022 Bq/g, the expanded uncertainty is 3.5%, k = 2, Chinese Academy of Metrology, No. DLhd2021-13,611) into a 500 mL volumetric bottle and seawater was added to the constant volume.
To 20 mL of spiked sample, 11 g of MB-20 resin was added and then magnetic stirring for 10 min. After filtering the sample through a 0.22 µm filter, the sample was measured for tritium by LSC. To ensure the reliability of the results, the experiment was repeated four times for each group of spiked samples.
In addition, 3 groups of spiked samples were processed by atmospheric pressure distillation in the experiment. The specific operation is as follows: add 30 mL spiked sample, appropriate amount of zeolite and 0.05 g potassium permanganate to the distillation flask for distillation, and collect the middle distillate. To ensure the reliability of the results, four repeated experiments were carried out for each group of spiked samples.
2.5.2 Effects of 90Sr-90Y
0.5 mL tritium standard solution with a concentration of 10 Bq/g and 4 mL 90Sr-90Y standard solution (9.87 Bq/g, the expanded uncertainty is 3.0%, k = 2, Chinese Academy of Metrology, RYSR90180726-01) with a concentration of 0.28 Bq/g were respectively taken into 100 mL volumetric bottles, and water was added for constant volume. The activity concentrations of tritium and 90Sr-90Y in the mixed solution were 50 Bq/L and 11.2 Bq/L, respectively. The solution was evenly divided into 3 parts, two of which were treated with atmospheric distillation and ion exchange in accordance with section 2.5.1.
2.6 Application of the method
To test the applicability of the developed method, 10 seawater samples were collected around Hangzhou Bay, Zhejiang Province, China on August 27, 2023. To compare the performance, each seawater sample was purified by both ion exchange resin and distillation method, followed by electrolysis enrichment as detailed below.
2.6.1 Ion exchange method
Seawater was mixed with the optimum resin and the ratio in a beaker and stir continuously with a magnetic stirrer for 10 min. The solution was separated from the resin with 0.22 μm filter membrane. 500 mL of filtered sample was taken and placed it into the electrolytic enrichment device, and electrolyzed for 60 h to enrich tritium. After electrolysis was complete, the obtained solution was transferred into a glass bottle and sealed for LSC measurement.
2.6.2 Atmospheric distillation
700 mL of seawater, zeolite and 0.2 g potassium permanganate were added to the distillation flask, and distillation process was repeated for several times until the conductivity of obtained sample was < 5 μS/cm (a single distillation process required about 12 h). 500 mL of condensed sample solution was taken and proceed with electrolysis as described above to enrich tritium.
2.6.3 Electrolysis enrichment factor
50 μL tritium standard solution (10.2 Bq/g) was diluted with background water a final volume of 500 mL, and proceed with electrolysis as described above to enrich tritium. The enriched tritium solution was measured by LSC to determine electrolysis enrichment factor.
2.7 Measurement of tritium by LSC
8 mL of enriched tritium fraction after electrolysis or background water or samples of standard solutions containing tritium was placed in a 20 mL polyethylene scintillation vial, and mixed with 12 mL of Ultima Gold LLT scintillation cocktail (PerkinElmer). The tritium activity concentration in the sample was determined by low-level LSC (Quantulus GCT 6220/Quantulus 1220, PerkinElmer), with energy range between 0 and 5 keV, and counting time of 1000 min. The radiochemical procedure is illustrated in Figure 1.
2.8 Tritium activity concentration
The tritium activity concentration in the enriched sample after electrolysis was calculated according to the following equation:
Where is the tritium activity concentration in the enriched sample after electrolysis (Bq/L), is the count rate of the enriched sample (cpm), is the count rate of the backgrand (cpm), is the weight of the sample contained in the counting vial, is the counting efficiency of LSC for tritium, and is the electrolysis enrichment factor.
The electrolytic enrichment factor was calculated according to the following equation:
Where , and are the activity concentration (Bq/L), activity (Bq) and volume (mL) of the working solution before the electrolysis enrichment, respectively. , and are the activity concentration (Bq/L), activity (Bq) and volume (mL) of the working solution after the electrolysis enrichment, respectively.
2.9 Statistics
The activity concentration is calculated as shown in Equations 1 and 2. The data was processed using Excel 2019.
3 Results and discussion
3.1 Conditional screening
3.1.1 Resin selection
The tritium measurement using LSC method requires the sample solution to be salt-free, transparent and colorless to ensure high counting efficiency (Pourimani and Aghamohammadi, 2014; Tjahaja and Sukmabuana, 2012). Therefore, removing impurities is an essential step before the LSC measurement. Electrical conductivity is a straightforward indicator for water salinity monitoring (Subaer et al., 2021; Thorslund and van Vliet, 2020; Wilson et al., 2011). The results for the 5 resins used in the screening are shown in Figure 2. Among the 5 resins, MB-106UP, MB-20 and MB-450 resins showed the ability to reduce the conductivity of seawater. Referring to the characteristics of the 5 resins in Table 1, it shows that the strong acidic cation and strong basic anion mixed-bed exchange resin is more effective to remove ions from seawater. In the case of a certain amount of seawater and conductivity, the least amount of resin: was required for MB-20 resin, followed by MB-450.
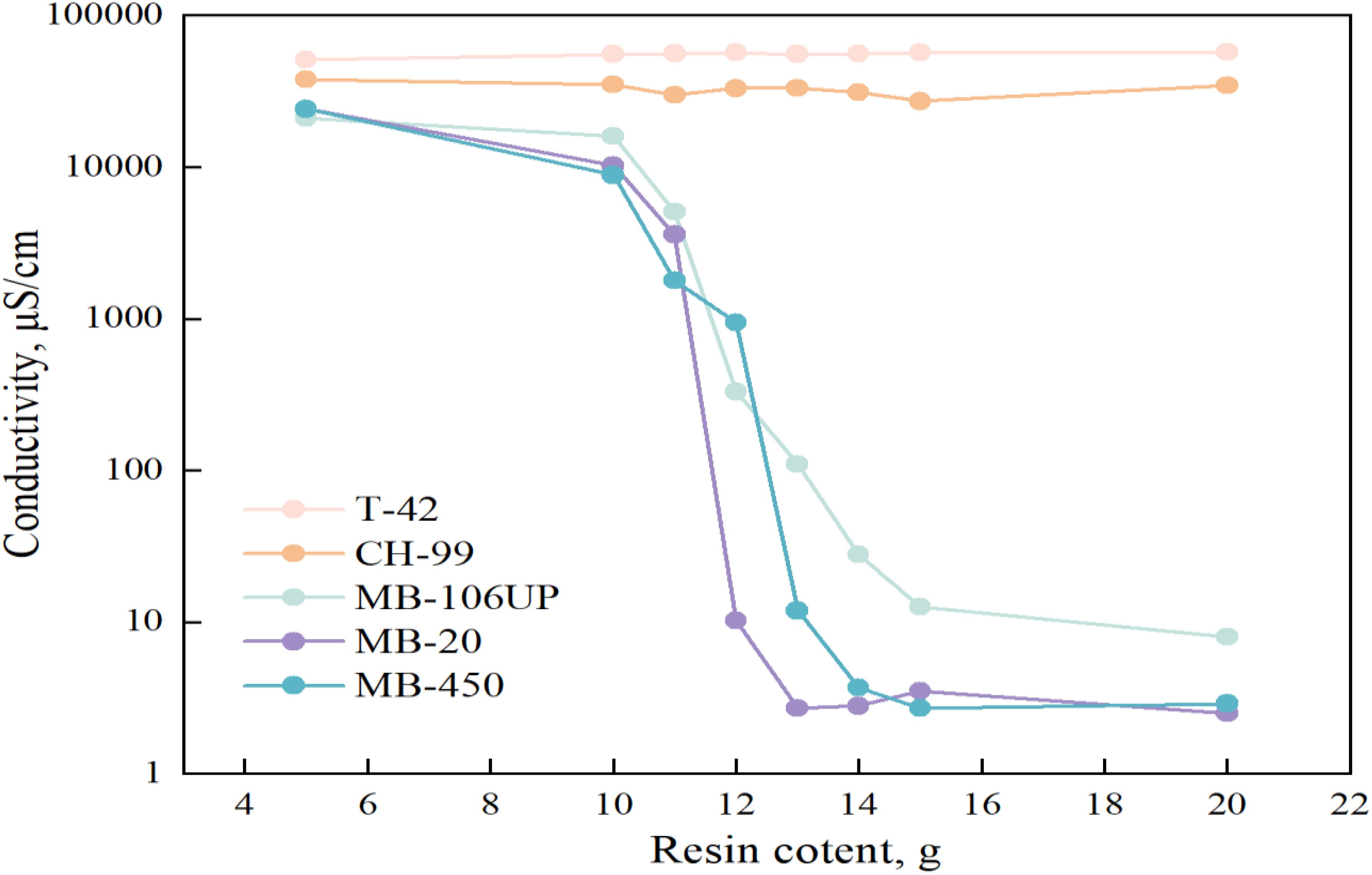
Figure 2. Effect of type and dosage of ion exchange resin on the removal of impurities from seawater.
3.1.2 ICP-OES
Seawater typically contains 96.5% of water, 2.5% of salt and small amounts of other substances, with the major metal ions including sodium, potassium, magnesium, and calcium (Mackenzie, 2024). The salinity of seawater was too high to be measured directly by ICP-OES, consequently, 100-fold dilution was performed for each sample prior to the measurement. Table 3 shows the composition of seawater determined by ICP-OES, before and after passing over an ion exchange resin (the figure shows only the detected metal ions, and the metal ions below the lower detection limit are not shown). The main metal ions detected in seawater were sodium, potassium and magnesium, and their contents were in the order of sodium > magnesium > potassium. All the 3 cation-anion mix-bed resins (MB-106UP, MB-20 and MB-450 resins) and atmospheric distillation process could remove magnesium, sodium and potassium ions in seawater, comparatively, the impurity removal rate is significantly higher by ion exchanges resins than the atmospheric distillation, for the aluminum.
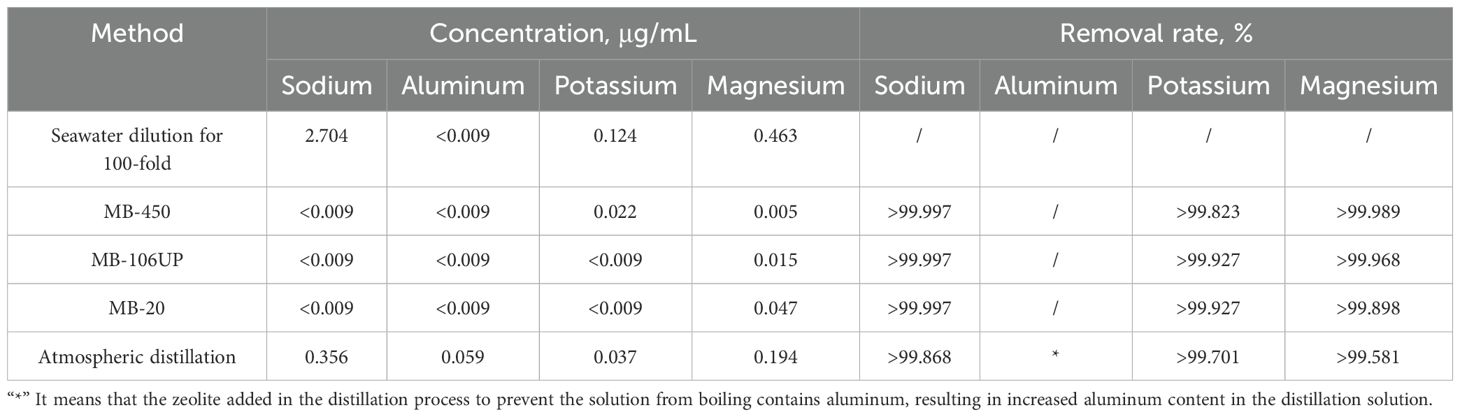
Table 3. Concentration of metal ions in seawater before and after treatment with ion exchange resin or atmospheric distillation.
3.2 Microscopic characterization
3.2.1 SEM-EDS
To further study the effect of ion exchange resin treatment on ions in seawater, SEM was used to characterize the surface morphology of resin before and after seawater treatment, while EDS was used to analyze the changes of element content in resin before and after seawater treatment. The results are shown in Figure 3 and Supplementary Figures S1-S2 in Supplementary Material. The SEM scanning results show that the surface of the 3 kinds of resins has no obvious change before and after treatment of seawater, and it is smooth and uniform. It may indicate that the resin can be reused after elution treatment.
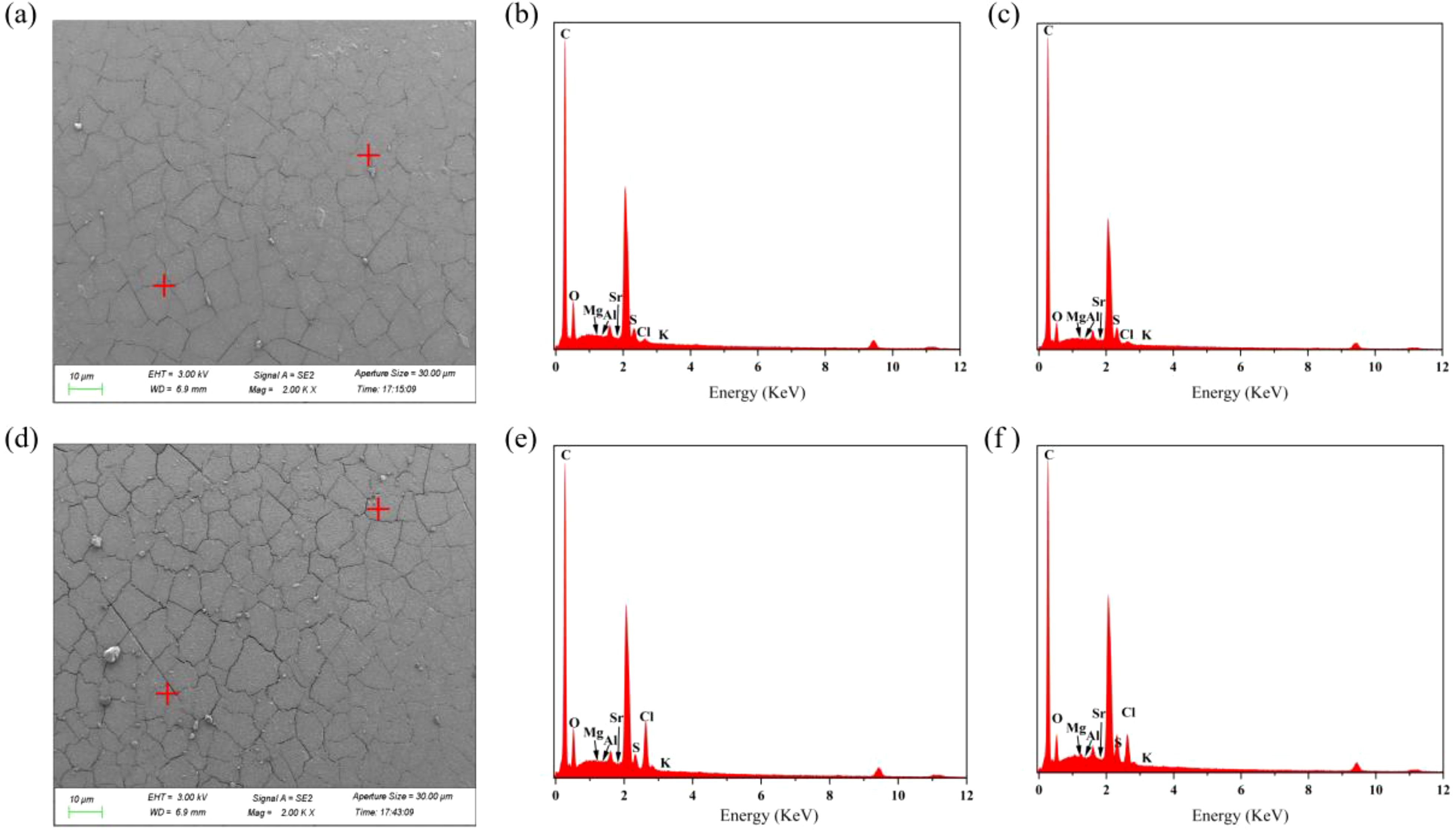
Figure 3. SEM images and EDS spectra of MB-20 resin before and after seawater treatment. (a) is the SEM image of MB-20 resin before seawater treatment, and Figures (b) and (c) are the EDS spectra of MB-20 resin before seawater treatment. (d) is the SEM image of MB-20 resin after seawater treatment, and Figures (e) and (f) are the EDS spectra of MB-20 resin after seawater treatment.
As shown in Figure 3 and Supplementary Figure S1, the contents of sulfur, chlorine, and carbon ions in the MB-20 and MB-106UP resins remained relatively stable after seawater treatment, with no significant changes observed. However, the atomic percentages of sodium, potassium, and magnesium increased in both resins, specifically by 1.84%, 0.07%, and 0.25% in MB-20 resin, and by 0.08%, 0.02%, and 0.13% in MB-106 resin, respectively. As can be seen from Supplementary Figure S2, after treating seawater with the MB-450 resin, the levels of sulfur, chlorine, and sodium increased by 2.4%, 0.32%, and 0.03%, respectively, while other ions showed no significant changes.
3.2.2 XPS analysis
XPS was used to detect the elemental content in the resin before and after seawater treatment, and the results are shown in Figure 4 and Supplementary Figures S3-S4 in Supplementary Material. Through the modification of the external spectra of the three kinds of resin before and after the specific absorption of metal ions, the infrared spectra of the three types of resin before and after metal ion adsorption remain largely unchanged, and the position and shape of the main characteristic peaks change little, indicating that the skeletal structure of the resin does not undergo chemical reactions.
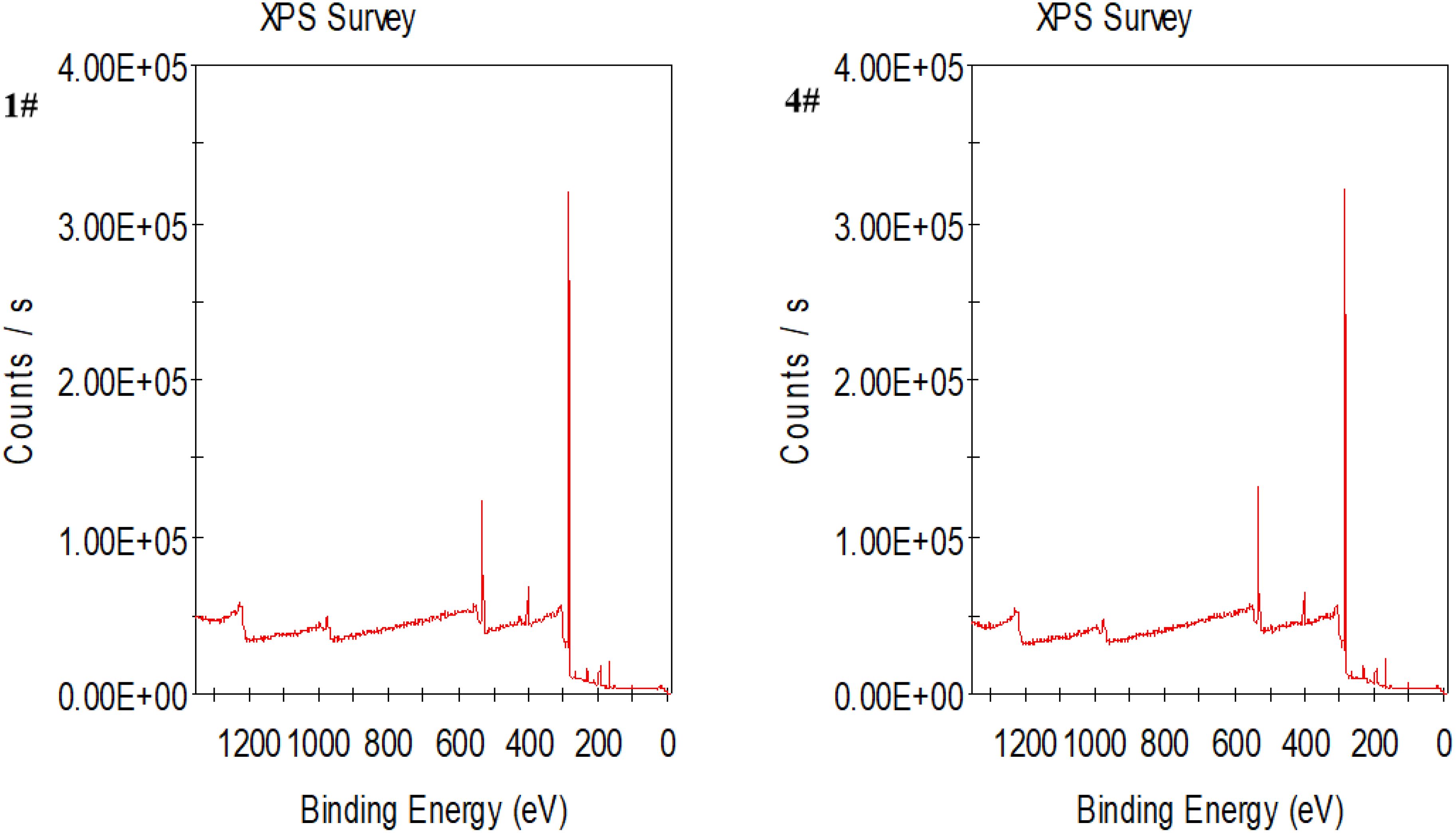
Figure 4. XPS wide-scan spectra and spectra for different elements in MB-20 resin before and after sample treatment (1# is before use, 4# is after use).
Comparisons were made regarding the exchange capacity and conductivity reduction ability of the three resins, and MB-106UP resin could not reduce the conductivity to below 5 μS/cm. Under fixed seawater volume and conductivity conditions, the amount of MB-20 resin is the least. EDS results showed that MB-20 resin had stronger ability to adsorb metal ions and anions in seawater. After comprehensive evaluation of various relevant factors, MB-20 resin was selected as the best choice for seawater purification process in this study.
3.3 Condition optimization
3.3.1 Ratio of resin to sample
With the consideration of account, the loss of the sample during resin purification and to meet the measurement requirements of LSC, the seawater sample volume was set at 20 mL. The relationship between the seawater conductivity and the amount of resin added was discussed when the sample size was 20 mL, and the optimal resin dosage was determined. As shown in the Figure 5, under the condition that the seawater sample volume is set to 20 mL and the reaction time is set to 10 min, when the resin dosage was within the range of 5 to 10 g, it was observed that the seawater conductivity gradually decreases with the increase of the resin addition amount. However, when the resin dosage is 11 g, the seawater conductivity drops below 1.5 μS/cm, and with further increases in resin dosage, no significant change in seawater conductivity was observed.
3.3.2 Relation between electrical conductivity and stirring time
To investigate the effect of different magnetic stirring durations on seawater conductivity, mixed samples containing 9, 10, and 11 g of resin and 20 mL of seawater were prepared. As shown in Figure 6, when 9 g of resin was added, the conductivity of seawater gradually decreased with the extension of stirring duration and stabilized at 1.6 μS/cm after 20 min. When 10 g of resin was added, the seawater conductivity decreased gradually within 15 min of stirring, after which it remained constant at 0.9 μS/cm. With 11 g of resin, the seawater conductivity decreased within 10 min, and after that, it stabilized at approximately 0.9 μS/cm.
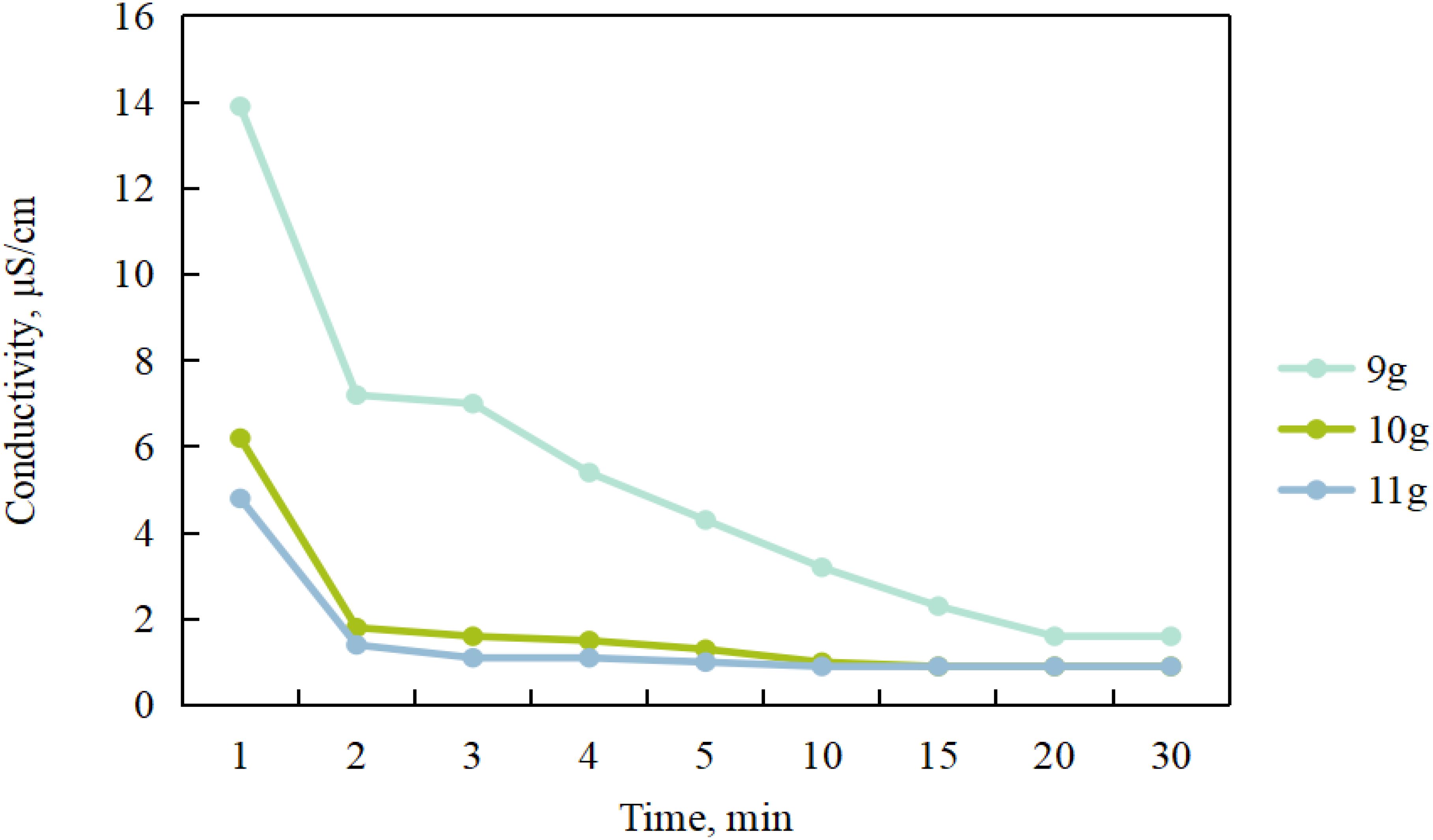
Figure 6. The relationship between the amount of resin added, stirring time and electrical conductivity.
To optimize efficiency and cost-effectiveness, the dosage of resin and mixing time were comprehensively analyzed and compared. The results indicate that optimal reaction efficiency and economic benefits can be achieved when the amount of seawater is 20 g, the resin dosage is 11 g, and the stirring time is 10 min. There is a big difference in purification time between ion exchange and atmospheric distillation, and the results are shown in Table 4. Atmospheric distillation took a long time to purify the samples (about 24 h), and the sample recovery was 75.71 - 86.89%. The ion exchange purification process was simple, taking about 10 min, and the sample recovery was 85.71%.
3.4 Method verification
To verify the accuracy of the method, spiked samples with three different tritium activity concentrations (5.1, 51 and 102 Bq/L) were purified and measured. The recoveries were calculated according to the ratio of the tritium activity concentration of the treated seawater to that of the standard solution prepared before the treatment. As shown in Table 5, the recoveries of tritium treated by MB-20 resins were all above 85%, within the ranges of 85.12 - 98.45%. Keisuke Soga et al. used distillation method to treat tritium as tissue free water in food, and the recovery was higher than 75% (Soga et al., 2016). Shunya Nakasone, et al. used ion-exchange resin to treat tritium in river water, and the recovery ranged from 77% to 111% (Nakasone et al., 2021). Our results are comparable to those reported earlier, demonstrating the accuracy of the developed method.
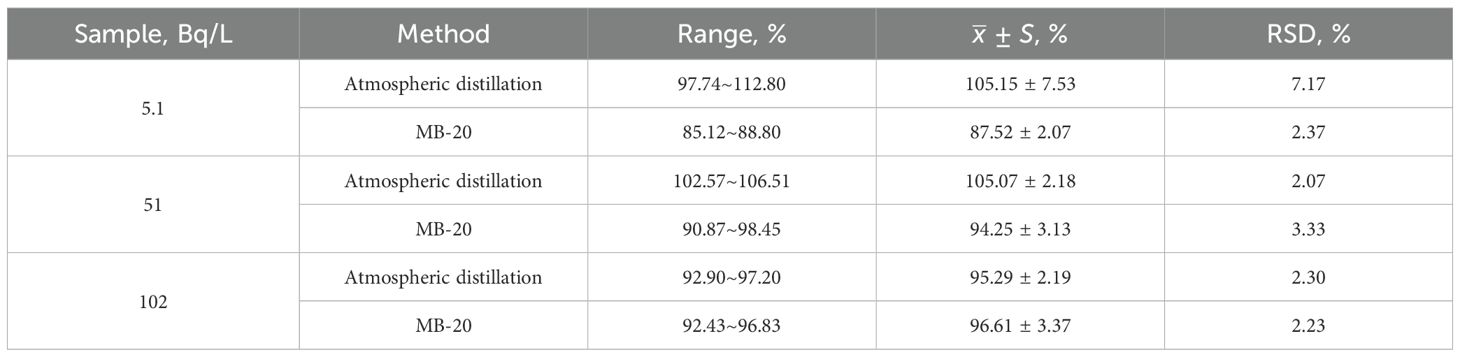
Table 5. Comparison of tritium recovery for spike sample prepared with ion exchange and distillation.
To consider the influence of 90Sr-90Y on the experiment, the mixed solution samples containing tritium and 90Sr-90Y with a certain concentration were prepared in this study and tested by LSC. The tritium recovery of the three samples within low energy wide channel were shown in Table 6. 90Sr-90Y contributed little to the tritium detection count rate in the low energy wide channel, and there was little difference in the tritium recovery between the samples before treatment and the samples after ion exchange or atmospheric distillation. The spectrogram of LSC of tritium is shown in Figure 7. Before the measurement wide channel of 200, there is no significant difference between the sample spectra before treatment, ion exchange treatment and atmospheric distillation treatment. When the measurement width was 300 - 900, the peak of the samples before treatment was significantly higher than that after MB-20 resin and atmospheric distillation, indicating that both atmospheric distillation and MB-20 resin could remove 90Sr-90Y from water.
3.5 Application of the method
For environmental-level seawater sample analysis, due to its low tritium concentration, direct measurement using LSC is still challenging (Muranaka et al., 2011). The International Atomic Energy Agency recommends the addition of an electrolytic concentration pretreatment, which can significantly reduce the detection limit of the liquid scintillation to accommodate measurement of tritium in the environment (Plastino et al., 2007; Saito, 2008; Satake and Takeuchi, 1991). To further verify the feasibility of this method, 10 seawater samples were collected and treated with MB-20 resin and atmospheric distillation respectively. Table 7 shows the activity concentration and standard deviation of tritium in different seawater. The tritium activity concentrations in seawater treated with ion-exchange resin and atmospheric distillation were respectively 1.30 ± 0.35 Bq/L and 1.58 ± 0.32 Bq/L. Calculating the uncertainty of each test result increases the reliability of the results. The results are shown in Table 7. The two methods have good consistency in the detection of tritium in seawater. For the purification of tritium activity concentration in each seawater sample, the ion exchange resin method took a total of 10 min, and the atmospheric distillation method took a total of >24 h (two or more distillation treatments). In summary, the detection results of tritium in seawater treated by ion exchange resin are comparable to those by distillation method, and the sample treatment time is 1/144.
When measuring low-level environmental samples, electrolysis enrichment would be necessary prior to LSC measurement, therefore, large volume (200 - 500 mL) of seawater should be purified, and accordingly the amount of resin required also increases. To ensure cost-effective, green and sustainable development, the team will consider re-using ion exchange resins in the future.
4 Conclusions
With the rapid development of nuclear technology, it is necessary to monitor tritium levels discharged into the sea from nuclear power plants. In this study, a rapid method was developed using MB-20 ion exchange resin followed by LSC measurement for determining tritium in seawater. The accuracy of the developed method was confirmed by analyzing spiked samples. The distinct advantage of the developed method is its significantly reduced analytical time compared to the traditional method. Particularly, it is suitable for environmental monitoring, especially under emergency situations. The results show that MB-20 resins have good adsorption effect on metal elements in seawater, and can rapidly reduce the content of sodium and potassium in seawater, and then reduce the conductivity of seawater (less than 1 μS/cm). The recoveries of the tritium standard liquid treated by ion exchange method were 85.12 - 98.45%, and those treated by atmospheric distillation method were 92.90 - 112.80%. In the field of radionuclide detection, especially in the detection process of tritium in complex samples such as seawater, environmental samples or nuclear facility emissions, it is particularly critical to effectively reduce the influence of other radionuclides on tritium detection. In this study, ion-exchange resin and atmospheric distillation were used to treat the mixed solution containing a certain concentration of tritium and 90Sr-90Y, and the spectra and recovery of the mixed solution were compared. The results show that the ion-exchange resin can remove 90Sr-90Y from seawater very well, and the peak of the spectrum can be observed to decrease obviously, and the tritium recovery was 97.13%. The sample processing time of ion exchange method is short, only 10 min to complete the sample purification treatment, which significantly improve the analytical efficiency. Based on the above experiments, the purification of seawater by ion exchange resin has high recovery rate of tritium and short sample processing time. It is an efficient, rapid, simple and environmentally friendly sample processing method for tritium analysis in seawater, which can meet the existing needs.
In nuclear energy production, monitoring tritium - a radioactive isotope - is crucial for environmental and public health safety. Rapid and accurate measurement of tritium is vital for assessing environmental impacts and responding to emergencies at nuclear plants. This study’s proposed sample treatment method enables swift tritium activity measurements in seawater, with the simplicity and ease for field application. Implementing this method will enhance emergency response efficiency and ensure timely monitoring of environmental radioactivity, thus safeguarding marine environments and human health from potential radioactive threats.
Data availability statement
The original contributions presented in the study are included in the article/Supplementary Material. Further inquiries can be directed to the corresponding author.
Author contributions
HR: Investigation, Writing – original draft, Writing – review & editing, Methodology, Funding acquisition. MX: Investigation, Writing – original draft, Writing – review & editing. PW: Funding acquisition, Methodology, Writing – review & editing. YS: Investigation, Writing – original draft. HZ: Investigation, Writing – original draft. YC: Funding acquisition, Writing – original draft, Writing – review & editing.
Funding
The author(s) declare that financial support was received for the research and/or publication of this article. This work was funded by the Key Research and Development Plan of Zhejiang Province (2024C03229), Zhejiang Health Science and Technology Plan (No. 2022RC120, 2022KY130, 2022KY132, 2024KY903, 2024KY892).
Conflict of interest
The authors declare that the research was conducted in the absence of any commercial or financial relationships that could be construed as a potential conflict of interest.
Generative AI statement
The author(s) declare that no Generative AI was used in the creation of this manuscript.
Publisher’s note
All claims expressed in this article are solely those of the authors and do not necessarily represent those of their affiliated organizations, or those of the publisher, the editors and the reviewers. Any product that may be evaluated in this article, or claim that may be made by its manufacturer, is not guaranteed or endorsed by the publisher.
Supplementary material
The Supplementary Material for this article can be found online at: https://www.frontiersin.org/articles/10.3389/fmars.2025.1518506/full#supplementary-material
References
Adam-Guillermin C., Bailly Du Bois P., Beaugelin-Sellier, Boyer P., Fiévet B., Garnier-Laplace J., et al. (2010). Le tritium dans l’environnement, synthèse des connaissances. 44–110 (ASN). Available at: https://www.researchgate.net/publication/295694727
Adam-Guillermin C., Pereira S., Della-Vedova C., Hinton T., Garnier-Laplace J. (2012). Genotoxic and reprotoxic effects of tritium and external gamma irradiation on aquatic animals. Rev. Environ. Contam Toxicol. 220, 67–103. doi: 10.1007/978-1-4614-3414-6_3
Alava J. J., Gobas F. A. (2016). Modeling (137)Cs bioaccumulation in the salmon-resident killer whale food web of the Northeastern Pacific following the Fukushima Nuclear Accident. Sci. Total Environ. 544, 56–67. doi: 10.1016/j.scitotenv.2015.11.097
Atkinson R., Eddy T., Kuhne W., Jannik T., Brandl A. (2014). Measurement of the tritium concentration in the fractionated distillate from environmental water samples. J. Environ. Radioact 135, 113–119. doi: 10.1016/j.jenvrad.2014.04.012
Buesseler K. (2020). Buesseler Opening Floodgates at Fukushima Science Perspective Aug 2020. AAAS. Available at: https://www.researchgate.net/publication/344036480
Butler H. L., Leroy J. H. (1965). Observation of biological half-life of tritium. Health Phys. 11, 283–285. doi: 10.1097/00004032-196504000-00005
Dallas L. J., Devos A., Fievet B., Turner A., Lyons B. P., Jha A. N. (2016). Radiation dose estimation for marine mussels following exposure to tritium: Best practice for use of the ERICA tool in ecotoxicological studies. J. Environ. Radioact 155-156, 1–6. doi: 10.1016/j.jenvrad.2016.01.019
Gagnaire B., Arcanjo C., Cavalié I., Camilleri V., Simon O., Floriani M., et al. (2020). Tritiated water exposure in zebrafish (Danio rerio): effects on the early-life stages. Environ. Toxicol. Chem. 39, 648–658. doi: 10.1002/etc.4650
Garbarino G., Magnoni M., Bertino S., Losana M. C. (2009). Development of an electrolysis system for tritium enrichment in superficial water samples. Radiat Prot. Dosim 137, 329–331. doi: 10.1093/rpd/ncp244
Groning M., Auer R., Brummer D., Jaklitsch M., Sambandam C., Tanweer A., et al. (2009). Increasing the performance of tritium analysis by electrolytic enrichment. Isot Environ. Health Stud. 45, 118–125. doi: 10.1080/10256010902872042
Guo F., Wu W., Feng Y., Shen H. (2020). Distribution of tritium concentration in the 0–25 cm surface soil of cultivated and uncultivated soil around the Qinshan nuclear power plant in China. Appl. Radiat Isot 164, 109311. doi: 10.1016/j.apradiso.2020.109311
Hatano Y., Nakamura H., Fujiwara S., Saito S., Kenmotsu T. (2022). Damages of DNA in tritiated water. Enzymes 51, 131–152. doi: 10.1016/bs.enz.2022.08.009
He S., Zhang X., Xia X., Wang C., Xiang S. (2020). Low energy consumption electrically regenerated ion-exchange for water desalination. Water Sci. Technol. 82, 1710–1719. doi: 10.2166/wst.2020.442
Iourtchouk T., Bergmann H. (2012). Electrodeionization – possibilities and limitations. 63th ISE Annual MeetingAt: Prague
Iryna P. (2017). Data from: toxicity of radionuclides in determining harmful effects on humans and environment. J. Environ. Sci. Public Health 1 (2), 115–119. doi: 10.26502/jesph.96120011
Janković M. M., Janković B. J., Todorović D. J., Ignjatović L. M. (2012). Tritium concentration analysis in atmospheric precipitation in Serbia. J. Environ. Sci. Health Part A-Toxic/Hazard Subst. Environ. Eng 47, 669–674. doi: 10.1080/10934529.2012.660039
Kaizer J., Kontuľ I., Povinec P. P. (2023). Impact of the fukushima accident on (3)H and (14)C environmental levels: A review of ten years of investigation. Molecules 28 (6), 2548. doi: 10.3390/molecules28062548
Köllö Z., Palcsu L., Major Z., Papp L., Molnár M., Ranga T., et al. (2010). Experimental investigation and modelling of tritium washout by precipitation in the area of the nuclear power plant of Paks, Hungary. J. Environ. Radioact 102, 53–59. doi: 10.1016/j.jenvrad.2010.09.002
Kumar B., Han L. F., Wassenaar L. I., Klaus P. M., Kainz G. G., Hillegonds D., et al. (2016). A compact tritium enrichment unit for large sample volumes with automated re-filling and higher enrichment factor. Appl. Radiat Isot 118, 80–86. doi: 10.1016/j.apradiso.2016.07.018
Lee J. J., Park J., Kim B., Lee S. (2020). Ultra-low concentration of total organic carbon in ultrapure water using ion-exchange resin embedding silanized magnetic nanoparticles. J. Environ. Sci. 92, 11–17. doi: 10.1016/j.jes.2019.11.023
Li H., Yin Y., Liu J., Lu B., Wan H., Yang L., et al. (2021). Hydrogen-rich water attenuates the radiotoxicity induced by tritium exposure in vitro and in vivo. J. Radiat Res. 62, 34–45. doi: 10.1093/jrr/rraa104
Liang J., Cheng W., Xue X., Li J., Zhang M., Wei F., et al. (2022). Annual variation of different forms of tritium in the soil around Qinshan Nuclear Power Plant. J. Environ. Radioact, 251–252, 106957. doi: 10.1016/j.jenvrad.2022.106957
Ma A., Abushaikha A., Allen S. J., McKay G. (2019). Ion exchange homogeneous surface diffusion modelling by binary site resin for the removal of nickel ions from wastewater in fixed beds. Chem. Eng J. 358, 1–10. doi: 10.1016/j.cej.2018.09.135
Mackenzie F. T, Duxbury A. C., Byrne R. H. (2024). “seawater”. Encyclopedia Britannica, 214–217. Available at: https:www.britannica.com/.cience/seawater (Accessed July 11, 2024).
Madigan D. J., Baumann Z., Fisher N. S. (2012). Pacific bluefin tuna transport Fukushima-derived radionuclides from Japan to California. Proc. Natl. Acad. Sci. U S A 109, 9483–9486. doi: 10.1073/pnas.1204859109
Matsumoto H., Shimada Y., Nakamura A. J., Usami N., Ojima M., Kakinuma S., et al. (2021). Health effects triggered by tritium: how do we get public understanding based on scientifically supported evidence? J. Radiat Res. 62, 557–563. doi: 10.1093/jrr/rrab029
Men W. (2021). Discharge of contaminated water from the Fukushima Daiichi Nuclear Power Plant Accident into the Northwest Pacific: What is known and what needs to be known. Mar. pollut. Bull. 173, 112984. doi: 10.1016/j.marpolbul.2021.112984
Muranaka T., Yamashita J., Shima N. (2011). Variation of tritium concentration in coastal seawater collected along the pacific coast in aomori prefecture. Fusion Sci. Technol. 60, 1264–1267. doi: 10.13182/FST11-A12660
Nakasone S., Yokoyama S., Takahashi T., Ota M., Kakiuchi H., Sugihara S., et al. (2021). Simple pretreatment method for tritium measurement in environmental water samples using a liquid scintillation counter. Plasma Fusion Res. 16, 2405035. doi: 10.1585/pfr.16.2405035
Oms P. E., Bailly D. B. P., Dumas F., Lazure P., Morillon M., Voiseux C., et al. (2019). Inventory and distribution of tritium in the oceans in 2016. Sci. Total Environ. 656, 1289–1303. doi: 10.1016/j.scitotenv.2018.11.448
Plastino W., Chereji I., Cuna S., Kaihola L., De Felice P., Lupsa N., et al. (2007). Tritium in water electrolytic enrichment and liquid scintillation counting. Radiat Meas 42, 68–73. doi: 10.1016/j.radmeas.2006.07.010
Pourimani R., Aghamohammadi M. (2014). Determination of tritium concentration in heavy water. World J. Nucl. Sci. Technol. 4, 170–176. doi: 10.4236/wjnst.2014.43022
Rozhko T. V., Badun G. A., Razzhivina I. A., Guseynov O. A., Guseynova V. E., Kudryasheva N. S. (2016). On the mechanism of biological activation by tritium. J. Environ. Radioact 157, 131–135. doi: 10.1016/j.jenvrad.2016.03.017
Saito M. (2008). Enrichment reliability of solid polymer electrolysis for tritium water analysis. J. Radioanalytical Nucl. Chem. 275, 407–410. doi: 10.1007/s10967-007-6894-8
Sakuma Y., Yamanishi H., Koganezawa T., Iida T., Ogata Y., Tsuji N., et al (2012). Development of a Simplified Method for Tritium Measurement in the Environmental Water Samples. Environmental Science. Available at: https://www.researchgate.net/publication/48931099.
Satake H., Takeuchi S. (1991). Electrolytic enrichment of tritium with Fe-Ni and Ni-Ni electrodes and estimation of tritium enrichment factor using Fe-Ni electrodes. Geochem J. 25, 429–435. doi: 10.2343/geochemj.25.429
Soga K., Kamei T., Hachisuka A., Nishimaki-Mogami T. (2016). An analytical method to measure free-water tritium in foods using azeotropic distillation. Food Hyg. Saf Sci. 57, 81–88. doi: 10.3358/shokueishi.57.81
Subaer S., Fansuri H., Haris A., Misdayanti, Irfanita R., Ramadhan I., et al. (2021). Pervaporation membranes for seawater desalination based on geo-rGO-tiO(2) nanocomposites. Part 1: microstructure properties. Membranes 11 (12), 966. doi: 10.3390/membranes11120966
Subban C. V., Gadgil A. J. (2019). Electrically regenerated ion-exchange technology for desalination of low-salinity water sources. Desalination 465, 38–43. doi: 10.1016/j.desal.2019.04.019
Tarancón A., Bagán H., Rauret G., García J. F. (2010). Comparative study of pre-treatment procedures for (3)H monitoring in water samples from environmental protection programs. Sci. Total Environ. 408, 2233–2238. doi: 10.1016/j.scitotenv.2010.01.021
Thorslund J., van Vliet M. T. H. (2020). A global dataset of surface water and groundwater salinity measurements from 1980–2019. Sci. Data 7, 231. doi: 10.1038/s41597-020-0562-z
Tjahaja P., Sukmabuana P. (2012). The Separation of Tritium Radionuclide from Environmental Samples by Distillation Technique. doi: 10.5772/38169
Varlam C., Stefanescu I., Duliu O. G., Faurescu I., Popescu I. (2009). Applying direct liquid scintillation counting to low level tritium measurement. Appl. Radiat Isot 67, 812–816. doi: 10.1016/j.apradiso.2009.01.023
Wakeford R. (2014). The risk of leukaemia in young children from exposure to tritium and carbon-14 in the discharges of German nuclear power stations and in the fallout from atmospheric nuclear weapons testing. Radiat Environ. Biophys. 53, 365–379. doi: 10.1007/s00411-014-0516-y
Wallova G., Meresova J., Zvachova S., Petranova I., Sykora I. (2020). New electrolytic enrichment system for tritium determination in water research institute in Bratislava and IT’S first results of tritium activity in precipitation. J. Environ. Radioact 216, 106177. doi: 10.1016/j.jenvrad.2020.106177
Wanigaratne S., Holowaty E., Jiang H., Norwood T. A., Pietrusiak M. A., Brown P. (2013). Estimating cancer risk in relation to tritium exposure from routine operation of a nuclear-generating station in Pickering, Ontario. Chronic Dis. Inj Can. 33, 247–256. doi: 10.24095/hpcdp.33.4.06
Wilson B., Gandhi J., Zhang C. C. (2011). Analysis of inorganic nitrogen and related anions in high salinity water using ion chromatography with tandem UV and conductivity detectors. J. Chromatogr. Sci. 49, 596–602. doi: 10.1093/chrsci/49.8.596
Yankovich T. L., Kim S. B., Baumgärtner F., Galeriu D., Melintescu A., Miyamoto K., et al. (2011). Measured and modelled tritium concentrations in freshwater Barnes mussels (Elliptio complanata) exposed to an abrupt increase in ambient tritium levels. J. Environ. Radioact 102, 26–34. doi: 10.1016/j.jenvrad.2010.06.014
Keywords: ion exchange resin, purification, seawater, determination, tritium
Citation: Ren H, Ma X, Wang P, Yu S, Zou H and Cao Y (2025) Rapid sample purification approach for 3H determination in seawater using ion exchange resin. Front. Mar. Sci. 12:1518506. doi: 10.3389/fmars.2025.1518506
Received: 28 October 2024; Accepted: 12 March 2025;
Published: 01 April 2025.
Edited by:
Christian Joshua Sanders, Southern Cross University, AustraliaReviewed by:
Intae Kim, Korea Institute of Ocean Science and Technology (KIOST), Republic of KoreaPriyanka Reddy, Bhabha Atomic Research Centre (BARC), India
Copyright © 2025 Ren, Ma, Wang, Yu, Zou and Cao. This is an open-access article distributed under the terms of the Creative Commons Attribution License (CC BY). The use, distribution or reproduction in other forums is permitted, provided the original author(s) and the copyright owner(s) are credited and that the original publication in this journal is cited, in accordance with accepted academic practice. No use, distribution or reproduction is permitted which does not comply with these terms.
*Correspondence: Yiyao Cao, eXljYW9AY2RjLnpqLmNu
†These authors have contributed equally to this work