- School of Marine and Atmospheric Sciences, Stony Brook University, Southampton, NY, United States
Bivalves are economically and ecologically important species and the estuarine systems they inhabit can experience diel fluctuations in dissolved oxygen (DO) as a result of the shifting balance between photosynthesis and respiration. During warmer summer months, these fluctuations are often intensified, potentially exposing local bivalve populations to repeated episodes of hypoxia. For this study, in situ flow-through experiments tested the effects of naturally-occurring nocturnal hypoxia on early life stage bivalves. Juvenile bivalves (hard clams, M. mercenaria; Eastern oyster, C. virginica; and bay scallop, A. irradians) were subjected to either natural estuarine cycles of DO and pH or amended, static normoxic but still acidified conditions during the peak cycling season (summer). Growth and survival rates of bivalves were quantified. During nine experiments across three summers, nocturnal hypoxia in unamend controls was moderate, with, on average, 3 hours per night of DO below 3 mg L-1, 1 hour per night of DO below 1 mg L-1, and a mean nocturnal DO concentration of 4.7 mg L-1. Still, amelioration of this nocturnal hypoxia during experiments yielded a mean increase in bivalve growth of 20% (range: 0 - 70%). Hard clams were more resilient to bouts of nocturnal hypoxia than scallops and oysters. The percent increases in growth rates of the hypoxia-ameliorated, aerated treatments were significantly correlated with hours of hypoxia during experiments (p<0.0001) and were significantly and inversely correlated with average nocturnal DO concentrations in control treatments (p<0.001). Application of these relationships to DO patterns at 19 sites across NY during summer indicated nocturnal hypoxia at these locales may have repressed bivalve growth rates by 10 – 240%. Given the enhanced predation pressure experienced by smaller bivalves, the slowed growth of unamended controls demonstrates that even small bouts of nocturnal hypoxia are a threat to estuarine bivalve populations.
Introduction
Bivalves are an economically and ecologically important group of organisms. They are ecosystem engineers that create habitat structure, modify phytoplankton communities, and can increase species richness (Borthagaray and Carranza, 2007; Gutiérrez et al., 2003; Peterson and Black, 1987), while their shellfisheries are worth $2B to the US economy (NOAA, 2019). Reductions in bivalve populations can have wide ranging effects through in ecosystem functionality (Gutiérrez et al., 2003; Wijsman et al., 2019). Bivalves can be negatively impacted by multiple anthropogenic stressors such as hypoxia, acidification, or harmful algal blooms (Breitburg et al., 2018; Diaz and Rosenberg, 2008; Tomasetti and Gobler, 2020; Shumway, 1990).
Eutrophication is the progressive overload of organic matter to a coastal ecosystem, often driven by excessive nutrient loading that increases organic matter production by algae (Nixon, 1995). Microbial respiration of excessive organic matter can lead to the formation of low oxygen, hypoxic zones (Diaz and Rosenberg, 2008; Breitburg et al., 2018). Additionally, many of the primary producers that respond to increased nutrient and organic matter loading may be mixotrophic and, therefore, may display strong respiration and exacerbate nocturnal hypoxia (Burkholder, 1998; Burkholder et al., 2008; Heisler et al., 2008). Hypoxia affects coastal systems around the world and has intensified with time (Diaz and Rosenberg, 2008; Steckbauer et al., 2011). The severity and length of hypoxic conditions can vary tidally, on day-night cycles, and seasonally and is driven by multiple biological, physical, and chemical conditions (Baumann et al., 2015; Tyler et al., 2009; Wallace and Gobler, 2021).
Dissolved oxygen (DO) can display extreme diel cycles in eutrophic coastal waters with hyperoxic conditions found during the day and hypoxia occurring at night (Baumann and Smith, 2018; Gobler and Baumann, 2016; Tyler et al., 2009; Wallace et al., 2021). Diel cycling of DO is generally most severe during mid/late summer when temperatures and photosynthetically active radiation are maximal (Breitburg, 1990; Caffrey, 2004; Wallace et al., 2014, 2021). Physical processes such as tidally flushing may moderate or intensify conditions in estuaries; more intensive tidal flushing can raise the DO via increased mixing of non-hypoxic, ocean end-member water, while reduced tidal flow may maximize the influence of localized respiration rates on DO levels (Baumann et al., 2015; Tyler et al., 2009).
As concentrations of DO decline, organisms may transition to anaerobic respiration and experience impaired physiological performance (Miller et al., 2016; Pörtner, 2012; Stevens and Gobler, 2018). Hypoxic conditions generally occur as a vertical gradient with more severe conditions in bottom waters, although in shallow systems, hypoxic conditions can appear throughout the water column (Beck and Bruland, 2000; Tyler et al., 2009; Wallace et al., 2021). As sessile or semi-sessile benthic organisms, bivalves are often more vulnerable to hypoxia than mobile animals (Diaz and Rosenberg, 2008; Vaquer-Sunyer and Duarte, 2008; Wang and Widdows, 1993).
While previous research has provided important information on the impacts of static hypoxic on shellfish (Steckbauer et al., 2015; Tomasetti et al., 2018; Vaquer-Sunyer and Duarte, 2008; Wang and Widdows, 1993), such experiments are not necessarily representative of dynamic estuarine ecosystems. Recent studies have explored diel cycling of hypoxia and have shown that brief periods of low DO can reduce the growth and/or survival of several species of early life stage shellfish (Clark and Gobler, 2016; Keppel et al., 2016; Miller et al., 2016; Tomasetti et al., 2021). Few studies, however, have considered how naturally occurring diel cycles of hypoxia in estuaries may impact juvenile bivalves.
The objective of this study was to determine how juvenile bivalves are affected by natural, diel cycling of hypoxia in an estuarine setting. Nine experiments were performed over three years with three species of North Atlantic bivalves: Argopecten irradians (bay scallops), Crassostrea virginica (Eastern oysters), and Mercenaria mercenaria (hard clams). Juvenile bivalves were grown in estuarine waters experiencing nocturnal hypoxia or in the same source water with hypoxia experimentally ameliorated. Differences in growth and survival rates were assessed and trends were considered in light of the intensity and duration of nocturnal hypoxia. We hypothesized nocturnal hypoxia would reduce the growth and survival of each bivalve species.
Methods
Organism care and maintenance
The effects of diel cycling dissolved oxygen were examined through laboratory flow-through experiments exposing juvenile bivalves to naturally occurring diel cycles of DO and pH and ameliorated oxygen conditions. Experimental organisms used were three important species from North America, namely bay scallops (Argopecten irradians), Eastern oysters (Crassostrea virginica), and hard clams (Mercenaria mercenaria). All experiments were run between July 2021 and September 2023 with a new cohort each year. Juvenile scallops were obtained from the Cornell Cooperative Hatchery in Southold, NY, USA, oysters from the East Hampton Shellfish Hatchery in East Hampton, NY, USA, and clams were spawned and raised in the Marine Sciences Center at the Stony Brook Southampton campus. All juveniles were reared in flow-through systems at their respective hatcheries until being moved to the Marine Sciences Center where they were kept on a low flow-through system filtered through a 100 µm sock. Bivalves fed on naturally occurring algae from Old Fort Pond, Shinnecock Bay, NY, USA when on flow-through and were fed cultured live algae (Tetraselmis spp., P. lutheri, C. muelleri) when flow-through was turned off due to HABs.
For each experiment, 20 individuals per replicate were tagged for individual survival and growth measurements with queen bee tags (Opalith), which were fixed to the shell using gel superglue (Gorilla Glue). Oysters were measured using digital pictures taken with a Cannon Rebel T6i and analyzed using imageJ software, and scallops and clams were measured using calipers (Sylvac). Survival and growth were quantified weekly. The two clam experiments used juveniles with lengths of 9.3 ± 1.0 mm and 9.6 ± 0.9 mm (n=120), respectively. The three oyster experiments used juveniles with shell heights of 15.5 ± 1.6 mm, 13.6 ± 1.4 mm, and 12.1 ± 1.4 mm (n=120), respectively. The four scallop experiments used juveniles with heights of 10.3 ± 1.7 mm, 13.0 ± 0.8 mm, 13.3 ± 0.6 mm, and 12.3 ± 0.8 mm (n=120), respectively. Average daily growth rates for each individual and then each replicate were determined, and the percent change in growth was determined by subtracting the average aeration treatment growth rate by the average control growth and dividing by the control growth (Tomasetti et al., 2021).
Experimental design
In these experiments, bivalves were subjected to natural cycling conditions and DO amended flow-through water for two to three weeks in late summer/early fall. Each of the two treatments supplied three 18L HDPE replicates (Leakmaster buckets) with seawater at 4500 Lh-1 using magnetic drive pumps (Danner, pondmaster 12b utility), which flushed each replicate about every 45 seconds. In order to ensure unaltered natural cycling, water was pumped from Old Fort Pond in Shinnecock Bay into the Marine Sciences Center in airtight lines and then pumped directly into unamended control replicates. The aeration treatment used the same water from Old Fort Pond but bubbled the water in a maze prior to entering the experimental replicate. In the maze, water was sprayed into a 6-inch drainage pvc pipe, containing bonded silica and wooden aerators, creating unidirectional flow into a sea table. This sea table was sectioned off by cinderblocks to restrict flow allowing all water to pass another wooden aerator and increase residence time around additional silica bonded air stones (Pentair). After the water made its way to the final section of the maze it was further aerated by silica bonded air stones and pumped into the aerated treatment replicates. This removed the extreme DO cycling in the aeration treatment, but pH remained similar to values in the control water from Old Fort Pond, which was likely due to short residence time within the maze. Input water was plumbed to the lower half of the replicate and wastewater flowed out the top of the replicate so that the bivalves continuously received new water. Organisms and replicates were cleaned every three to four days to reduce the amount of sedimentation in the replicates. Bivalves fed on natural algae from the flow-through water during the course of the experiment.
Chemistry
The chemistry for the control treatment was the result of natural processes in the source water of Old Fort Pond, Shinnecock Bay, NY, USA, which was slightly different from the source water due to further respiration processes as water was pumped through the Marine Sciences Center. In the aeration treatment, flow-through water was aerated to remove DO cycling but not pH. In one of the replicates from each treatment a YSI EXO 3 sonde continuously measured parameters every 5 seconds. Parameters recorded were dissolved oxygen (mg L-1), pH (NBS), temperature (°C), salinity (psu) and chlorophyll a (RFU). In parallel, samples were collected and processed for extracted chlorophyll a following the methods of Welschmeyer (1994) and the chlorophyll a data from the sonde was converted to µg L-1 using a linear regression of the measurements (r2 = 0.94). For several of the experiments one of the sondes malfunctioned resulting in short periods of no chemistry data for that treatment between some water changes. The range of conditions for all experiments were 0.06 – 14.02 mg L-1 for DO, 6.67 – 8.49 for pH, 13.32 – 30.50°C for temperature, 4.34 – 55.5 µg L-1 for chlorophyll a and 22.4 – 32.14 for salinity; the conditions present during for each experiment are presented in Table 1. The continuous and experimental mean chlorophyll a values appear in Supplementary Figures 1, 2. Average daily duration of hypoxia was determined by the length of time any of the treatments had DO values below 3 mg L-1 each day and averaged over the length of the experiment. The hypoxic threshold is not well established for many marine taxa nor coastal ecosystems as a whole, therefore 3 mg L-1 was used for this study in line with New York State DEC regulations (Steckbauer et al., 2011; Vaquer-Sunyer and Duarte, 2008; Wallace et al., 2021). Average nocturnal DO concentration was determined by taking the average DO during the night phase throughout the experiment. The night phase was considered to be half an hour before sunrise and half an hour after sunset on the central date of the experiment.
Analysis, statistics, and ecosystem comparison
All results are presented as mean ± standard deviation. Chemistry values were rounded to two decimal places with error representing day-to-day temporal variation across replicates. Length and growth measurements were rounded to two significant digits with error representing replicate vessels. Growth rates were compared between the two treatments using a t-test run with R-studio (r version 2023.12.1 + 402) to test for significance. All growth and survival data were tested for normality and homoscedasticity using Shapiro and Bartletts tests; if data failed these tests, it was either arcsin squareroot (survival data) or log transformed. In addition to this, the percent increase in growth rates of bivalves exposed to aeration compared to the control treatment was compared to the average daily duration of hypoxia, the average nocturnal DO value, and the chlorophyll a concentrations present during the experiments. These data were analyzed via a linear regression model in R-studio. Regressions were performed with and without clam data to determine the impact of that bivalve on the correlations due to their hardiness relative to the other bivalves.
Finally, to obtain a broader understanding of the implications of the experimental results to ecosystem settings, DO levels were monitored every 10 minutes for the final two weeks of July, 2024, at 18 sites across NY (Supplementary Figure 3; Supplementary Table 1). This represents the time of year when recently spawned bivalves of the species studied here are within the range of sizes used during experiments (Kennedy et al., 1996; Kraeuter and Castagna, 2001) and the time of year when nocturnal hypoxia is often maximal in NY (Wallace et al., 2014, 2021). DO levels were measured YSI EXO3 multi-parameter sondes and Onset HOBO dissolved oxygen data loggers (U26-001). Sondes and loggers were calibrated prior to deployment according to manufacturer’s instructions and were cleaned and recalibrated after one week (Wallace and Gobler, 2021). After two weeks, sensors were retrieved and the hours of nocturnal hypoxia and mean nocturnal DO was calculated for each site as described above. In addition, the linear relationships between increases in bivalve growth rates in aeration treatments and hours of nocturnal hypoxia and mean nocturnal DO concentration described above were applied to each of the 19 study sites to assess the potential growth enhancement via the application of aeration. The mean and standard error of the percent enhancement in growth rate was calculated for all sites based on each of the two (mean nocturnal DO and mean daily hours of hypoxia) regressions.
Results
Mercenaria mercenaria
Two experiments with hard clams lasted for three weeks each in July and August 2023. The mean shell height for the July and August cohorts of clams were 9.3 ± 1.0 and 9.6 ± 0.9 mm (n=120), respectively. Mean temperatures for these experiments were 26.80 ± 0.98 and 24.69 ± 1.18°C, average chlorophyll a levels were 40.4 ± 32.1 µg L-1 and 22.9 ± 15.2 µg L-1 while average nocturnal pH was 7.80 ± 0.22 and 7.66 ± 0.15, respectively (Table 1). Mean nocturnal dissolved oxygen (DO) values for the two experiments were 5.42 ± 1.72 and 4.52 ± 1.48 mg L-1 in the unamended control and 6.42 ± 0.28 and 6.54 ± 0.30 mg L-1 in the aeration treatment, respectively (Table 1; Figure 1). In the first clam experiment, the aeration treatment had a growth rate of 0.11 ± 0.0036 mm d-1 while the control had 0.12 ± 0.013 mm d-1 (n=3), with the aeration treatment growing 7% slower (p>0.05; Figure 1A). In the second experiment, the aeration treatment had a growth rate of 0.11 ± 0.0075 mm d-1 (n=3), growing 10% faster than the control (0.10 ± 0.0067 mm d-1 (n=3); p>0.05; Figure 1B). Unamended control and experimental treatment growth rates were not significantly different in either experiment.
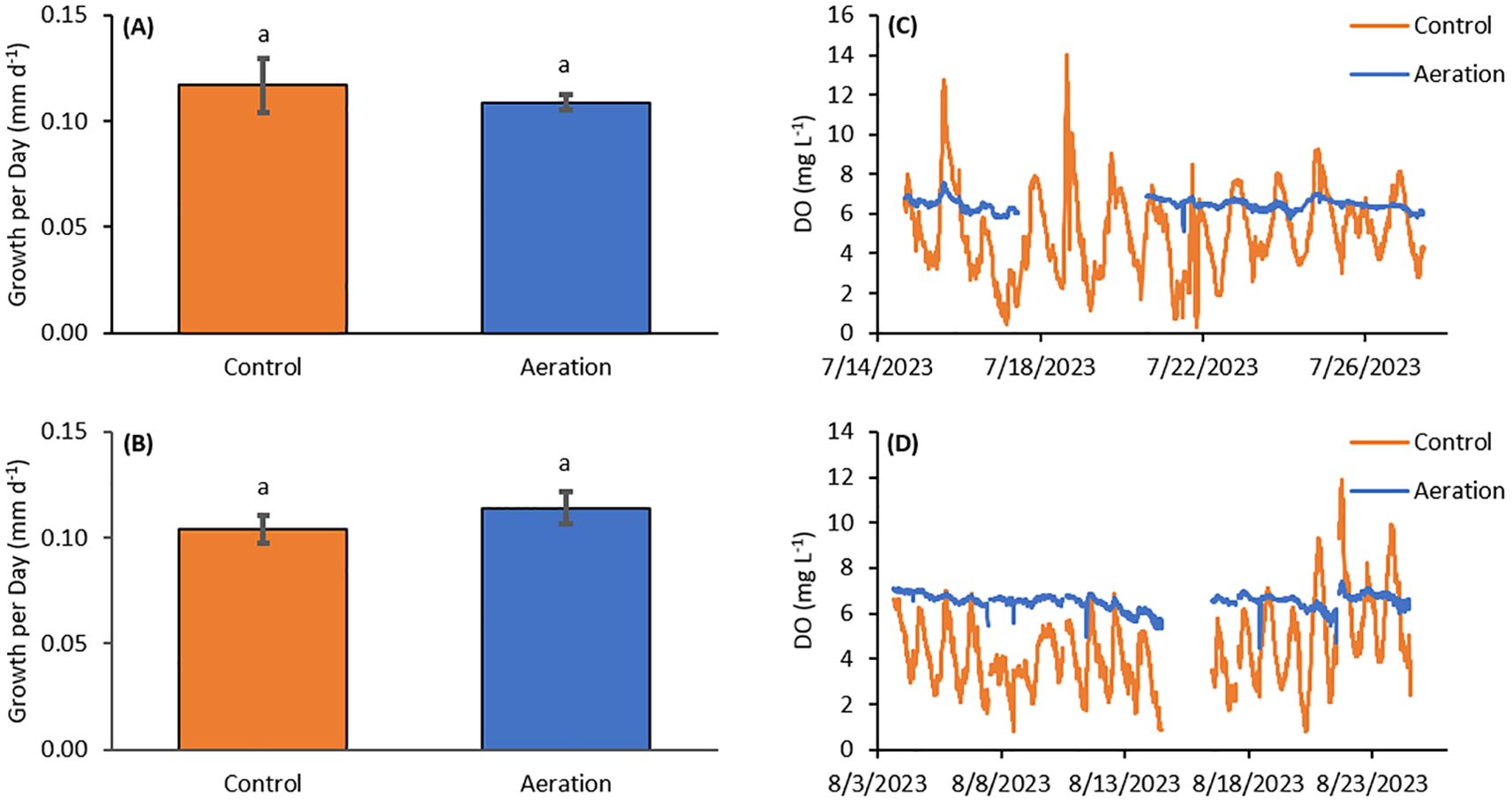
Figure 1. (A) Average daily growth over three weeks for the first hard clam (M. mercenaria) experiment. There was no significant difference in growth rates between the two treatments, however the Aerated treatment grew 7% slower. (B) Average daily growth over three weeks for the second hard clam experiment. There was no significant difference in growth rates between the two treatments, however the Aerated treatment grew 10% faster. (C) DO timeseries from the first experiment with missing data from the Aeration treatment was between 7/17 and 7/21. (D) DO timeseries from the second experiment with missing data from both treatments was between 8/14 and 8/16. Letter groupings represent statistical similarities and differences.
Crassostrea virginica
Three experiments were completed with Eastern oysters in August of 2022, July 2023, and September 2023. The first two experiments persisted for two weeks with average starting shell heights of 15.5 ± 1.6 and 13.6 ± 1.4 mm (n=120) while the third experiment ran for three weeks with average starting height of oysters being 12.1 ± 1.4 mm (n=120). Mean temperatures for the experiments were 27.60 ± 1.14, 25.66 ± 1.39, and 24.19 ± 2.69°C. Average chlorophyll a levels were 29.2 ± 17.6 µg L-1, 34.9 ± 21.8 µg L-1, and 30.1 ± 39.1 µg L-1, respectively (Table 1). Mean nocturnal pH values were 7.61 ± 0.15, 7.72 ± 0.14, and 7.73 ± 0.18, respectively and mean nocturnal DO values during exposures were 3.82 ± 1.61, 4.85 ± 1.30, and 5.46 ± 1.55 mg L-1 in the unamended control and 5.43 ± 0.73, 6.71 ± 0.31, and 6.67 ± 0.64 in the aeration treatment, respectively (Figure 2). The first oyster experiment experienced on average 8.83 ± 4.87 hours of hypoxia (< 3 mg L-1) per day whereas there was 0.25 ± 0.53 h and 1.25 ± 2.12 h in the second and third experiments, respectively (Table 1). The first oyster experiment had growth rates of 0.667 ± 0.103 mm d-1 (n=3) in the aeration treatment, 67% and significantly higher than the unamended control which had a growth rate of 0.40 ± 0.042 mm d-1 (n=3) (p<0.05; Figure 2A). In the second experiment, the aeration treatment had a growth rate of 0.51 ± 0.019 mm d-1 (n=3) and the control grew 14% slower at 0.45 ± 0.064 mm d-1 (n=3) (p>0.05; Figure 2B). The third experiment had growth rates of 0.41 ± 0.045 mm d-1 in the aeration treatment with controls rates being 0.38 ± 0.023 mm d-1, with the aeration treatment growing 9% faster (p>0.05; Figure 2C).
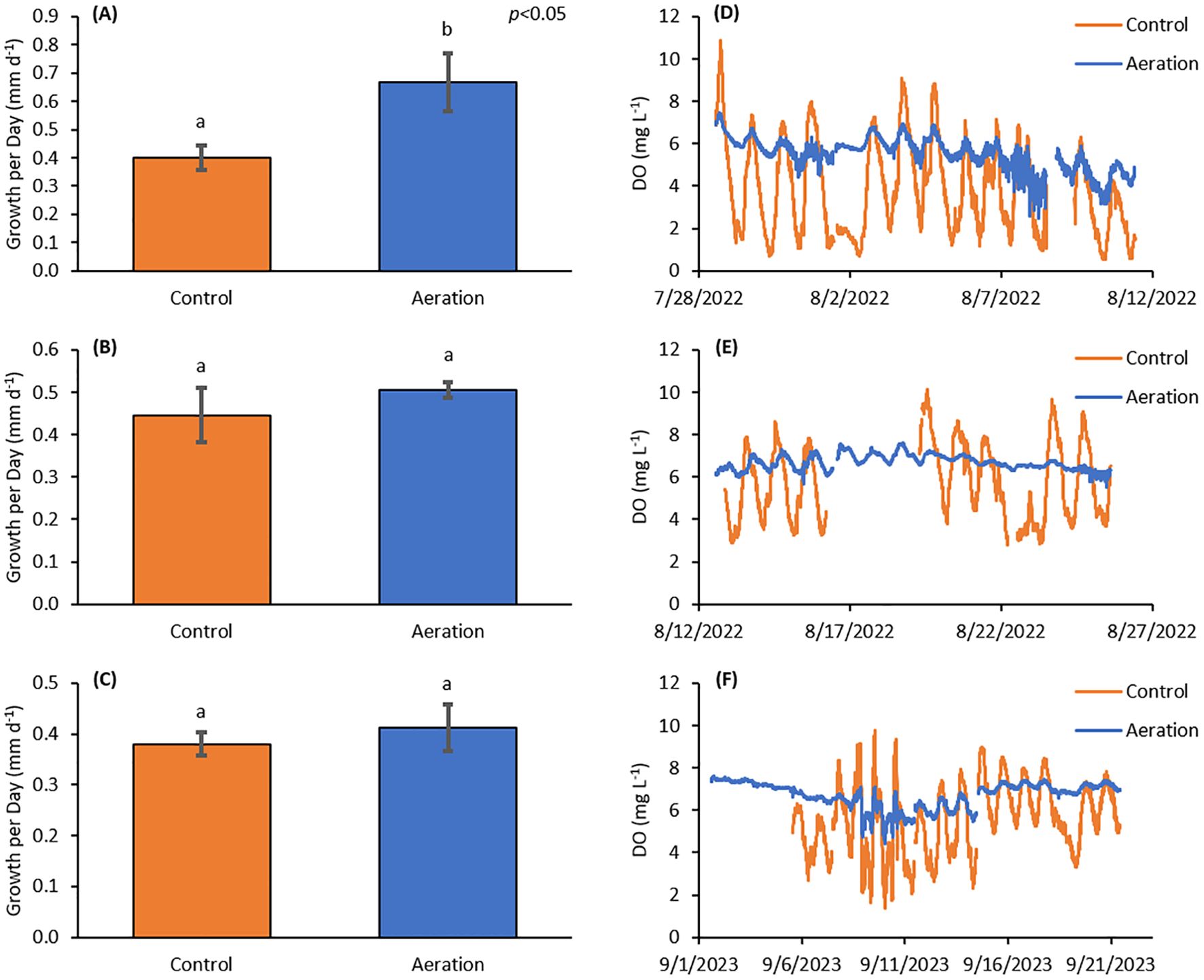
Figure 2. (A) Average daily growth over two weeks for the first oyster (C. virginica) experiment. The Aerated treatment had significantly higher growth rates (70%) than the control treatment (p<0.05). (B) Average daily growth over two weeks for the second oyster experiment. There was no significant difference in growth rates between the two treatments, however the Aerated treatment grew 14% faster. (C) Average daily growth over three weeks for the third oyster experiment. There was no significant difference in growth rates between the two treatments, however the Aerated treatment grew 9% faster. (D) DO timeseries from the first experiment. (E) DO timeseries for the second experiment with missing data from the control treatment was between 8/16 and 8/19. (F) DO timeseries from the third oyster experiment with missing data from the control treatment was between 9/1 and 9/5. Letter groupings represent statistical similarities and differences.
Argopecten irradians
Four bay scallop experiments were completed in September 2021, August and September 2022, and August 2023. Experiments one and four ran for three weeks with average starting heights of 10.3 ± 1.7 and 12.3 ± 0.8 mm (n=120), respectively, while experiments two and three ran for two weeks with average starting heights of 13.0 ± 0.8 and 13.3 ± 0.6 mm (n=120), respectively. Mean temperatures for the experiments were 23.54 ± 0.82, 26.29 ± 1.70, 17.21 ± 2.33, and 24.69 ± 1.18°C and nocturnal pH values of 7.78 ± 0.22, 7.72 ± 0.12, 7.65 ± 0.12, and 7.67 ± 0.12, in experimental order. Average chlorophyll a levels were 55.5 ± 71.9 µg L-1, 26.1 ± 20.4 µg L-1, 4.34 ± 7.63 µg L-1, and 22.9 ± 15.2 µg L-1, respectively (Table 1). Mean nocturnal DO values for these experiments were 5.00 ± 2.65, 4.87 ± 1.44, 6.13 ± 1.05, and 4.52 ± 1.48 in the unamended controls and 6.61 ± 0.98, 6.52 ± 0.30, 7.81 ± 0.34, and 6.54 ± 0.30 in the aeration treatment, in experimental order (Figure 3). The scallop experiments experienced an average 6.90 h, 5.65 h, 0.00 h, and 4.82 h of nocturnal hypoxia in the respectively (Table 1). The first bay scallop experiment had growth rates of 0.17 ± 0.0088 mm d-1 (n=3) in the aeration treatment, 35% and significantly faster than the unamended control (0.12 ± 0.0042 mm d-1 (n=3); p<0.01; Figure 3A). In the second experiment, the aeration treatment had a growth rate of 0.42 ± 0.027 mm d-1 and the control had 0.29 ± 0.0060 mm d-1 (n=3), with the aeration treatment growing significantly faster by 41% (p<0.005; Figure 3B). During the third experiment, scallops had growth rates of 0.046 ± 0.011 mm d-1 in the aeration treatment growing 5% slower than the control at 0.049 ± 0.0042 mm d-1 (n=3) (Figure 3C). Finally, in the fourth experiment, scallops had growth rates of 0.34 ± 0.0082 mm d-1 in the aeration treatment and 0.29 ± 0.0040 mm d-1 (n=3) in the control, with the aeration treatment growing 19% and significantly faster (p<0.0005; Figure 3D).
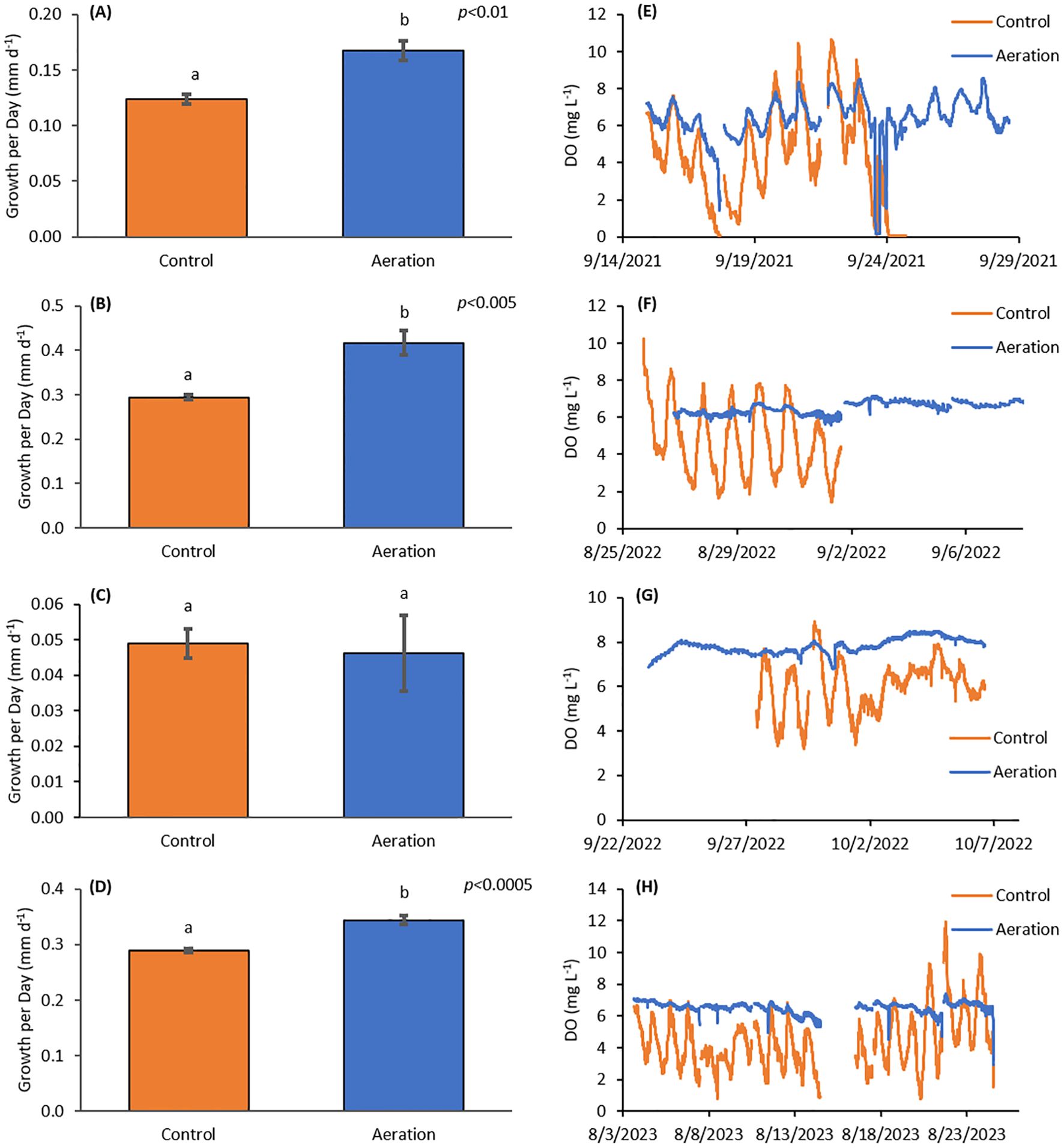
Figure 3. (A) Average daily growth over three weeks for the first bay scallop (A. irradians) experiment. The Aerated treatment had significantly higher growth rates (35%) than the control treatment (p<0.01). (B) Average daily growth over two weeks for the second bay scallop experiment. The Aerated treatment had significantly higher growth rates (42%) than the control treatment (p<0.05). (C) Average daily growth over three weeks for the third experiment. This was the only experiment to have no hypoxia in the Control treatment. There was no significant difference in growth rates between the two treatments, however the Aerated treatment grew 5.48% slower. (D) Average daily growth over three weeks for the fourth bay scallop experiment. The Aerated treatment had significantly higher growth rates (20%) than the Control treatment (p<0.0005). (E) DO timeseries for the first bay scallop experiment. This was the only experiment to have hypoxia in the Aerated treatment due to experimental design, and missing data was between 9/24 and 9/28. (F) DO timeseries for the second experiment with missing data from the control treatment was between 9/1 and 9/8. (G) DO timeseries for the third experiment with missing data from the Control treatment was between 9/23 and 9/27. (H) DO timeseries for the last bay scallop experiment. This experiment was run concurrently with the second hard clam experiment. Missing data from both treatments was between 8/14 and 8/16. Letter groupings represent statistical similarities and differences.
Meta-analysis
To resolve the role of differences in DO across experiments, regressions analyses were performed, examining how improvements in growth rates achieved within aeration treatments were related to the severity of hypoxia and mean nocturnal DO in unamended controls. Across all experiments, scallops and oysters most frequently experienced improvement in growth via aeration displaying significantly improved growth in three of four and one of three experiments, respectively (Figures 1-3). In contrast, amelioration of low DO never improved hard clam growth rates (two experiments; Figures 1, 2). Across all experiments, the percent increase of bay scallop and Eastern oyster’s growth rates as a result of aeration were significantly correlated with the mean hours of nocturnal hypoxia during the experiment (p<0.00001; Figure 4A). Similarly, the percent improvement of their growth rates was inversely correlated with mean nocturnal DO during the experiments (p<0.00001; Figure 4B). Improvement in growth rates of bivalves were also significantly correlated with mean hours of experimental hypoxia and nocturnal DO when clams were included in the regression, albeit at a slightly lower level of significance (p<0.0001; Figures 4A, B). In contrast, improvements in growth rates of bivalves in the aeration treatments were not correlated with the mean levels of chlorophyll a present during each experiment (Supplementary Figure 4).
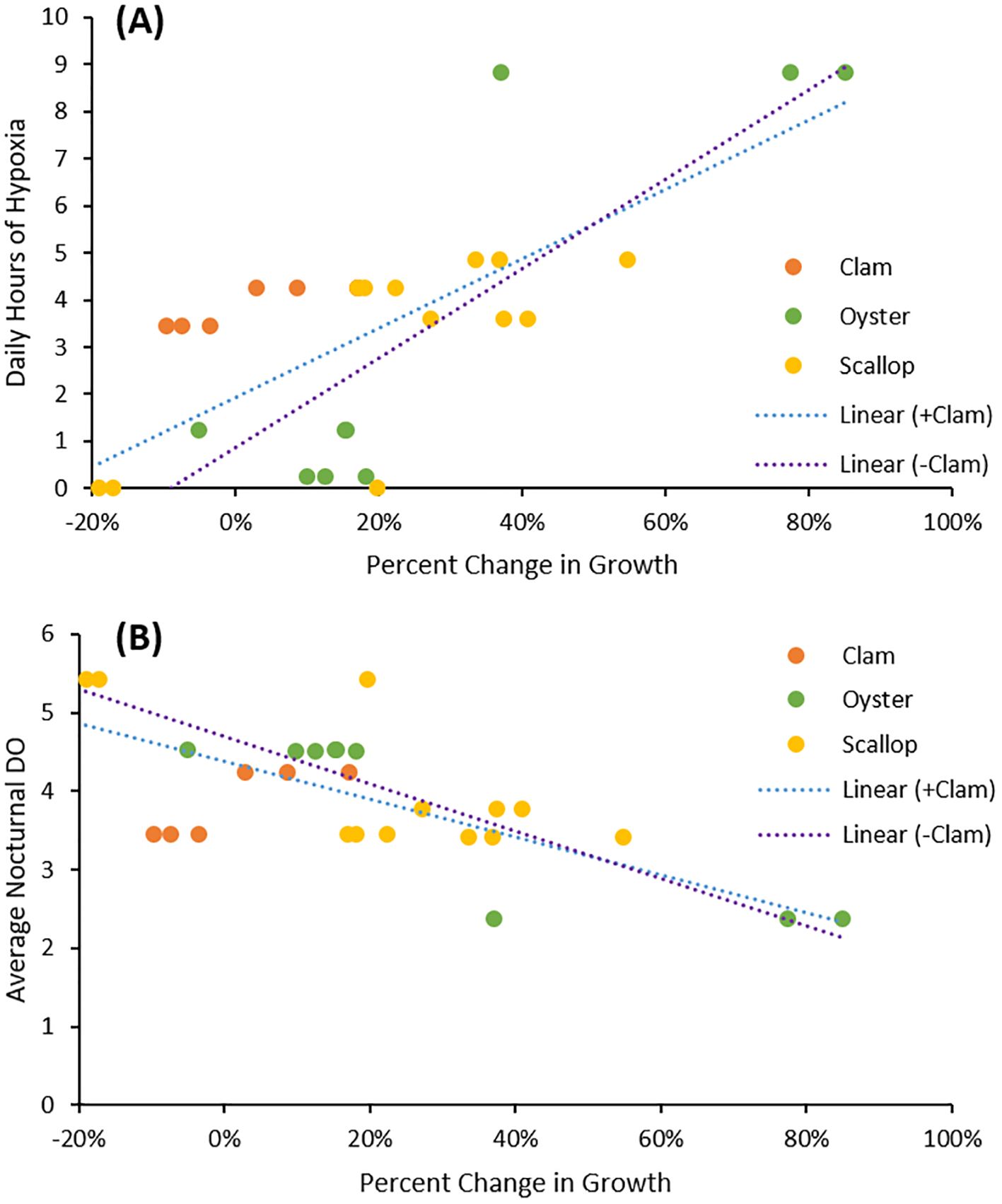
Figure 4. (A) Linear regression with a significant correlation between change in growth rates and daily hours of hypoxia (p<0.0001 with clam included; p<0.00001 with clams excluded). The total hours of hypoxia observed were normalized by the duration of the experiment to yield daily hours of hypoxia. (B) Linear regression with a significant negative correlation between change in growth rates and average nocturnal DO (p<0.0001 with clam included; p<0.00001 with clams excluded). Nocturnal DO values were averaged from 30 minutes after sunset to 30 minutes before sunrise.
Ecosystem comparison
Across the 19 study sites assessed in NY during July of 2024, mean nocturnal DO levels ranged from 0.11 to 3.9 mg L-1 and the mean hours of nocturnal hypoxia ranged from 1 to 23 hours (Supplementary Table 1). Using the regressions described above in the meta-analysis section, the potential percent increase in bivalve growth rates from applying aeration ranged from 10% for Mount Sinai Harbor which experienced an average of one hour of nocturnal hypoxia in late July to 240% for the Peconic River that experienced 23 hours of nocturnal hypoxia (Figure 5). The average across all sites was a 90% increase (Figure 5). About half of sites (n=10) had 10 or fewer hours of nocturnal hypoxia and potential growth enhancements generally within the range found in the experiments (up to 90%; Figure 5). The other half of the sites (n=9) had longer periods of hypoxia than those observed in experiments (10–23 hours) that projected to a potential growth rate of increase of 100 – 240% from aeration (Figure 5).
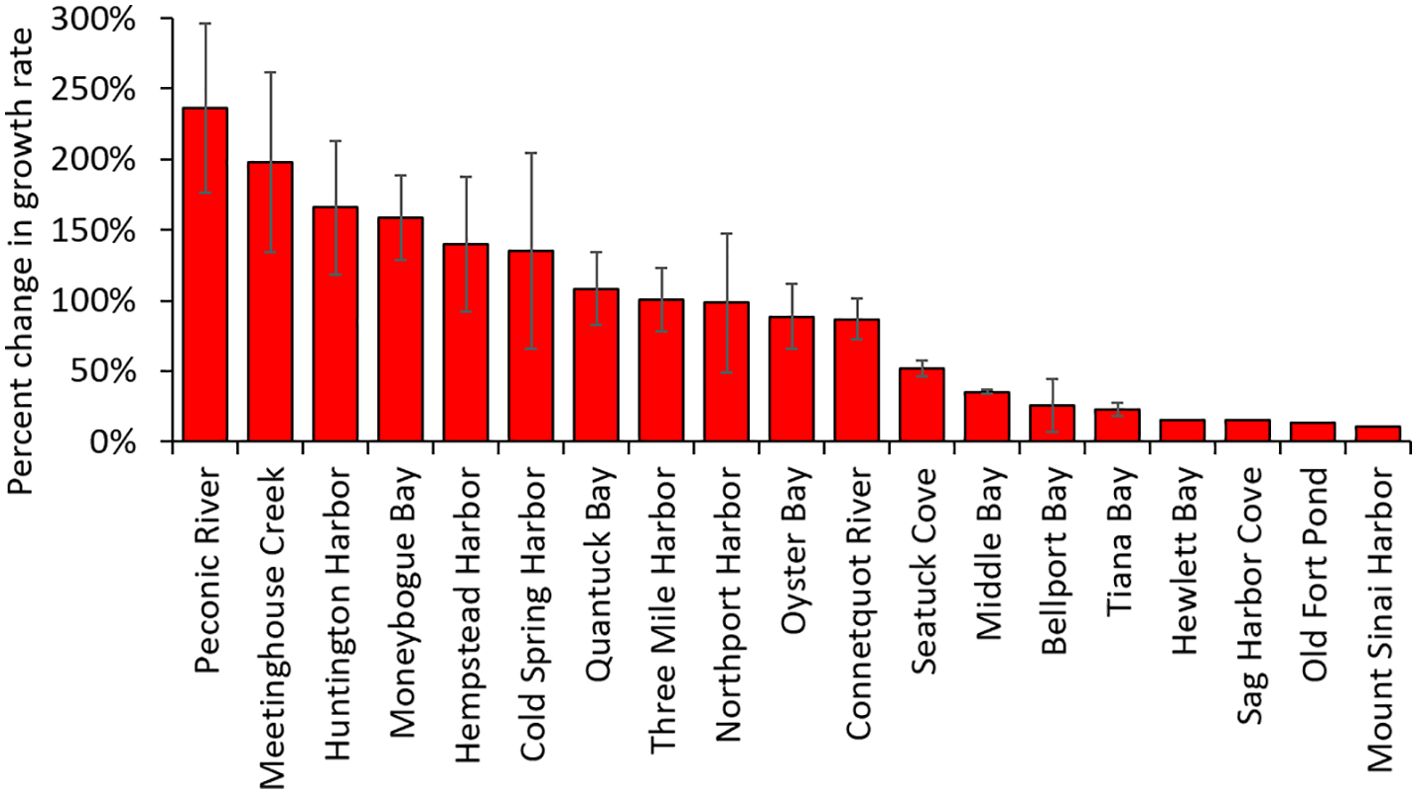
Figure 5. Potential increase in bivalve growth rate with aeration at 18 locations using regressions from Figure 4. Bars represent the mean of the two projected changes based on the regressions in Figures 4A, B and the error bars are standard error (SE). Details of the locations and DO data appear in Supplementary Table 1; Supplementary Figure 3.
Discussion
Hypoxia and acidification are well-known co-stressors that can have additive and synergistic negative effects on marine life (Alter et al., 2015; Gobler and Baumann, 2016; Waldbusser and Salisbury, 2014) and studies have shown that diel cycles of low pH and low DO negatively impact survival and growth of early life stage bivalves (Clark and Gobler, 2016; Gobler et al., 2017; Wallace et al., 2021). Here, experiments ameliorated nocturnal hypoxia allowing for the effects of this stressor, but not acidification, to be resolved. Oysters and scallops were found to be more responsive to the amelioration of nocturnal hypoxia compared to hard clams that, in contrast, appeared more resilient to this stressor. Importantly, the intensity of hypoxia during this study was relatively mild, with DO levels below 2 mg L-1 rarely occurring, suggesting that extended exposure to even mild hypoxia can have significantly negative effects on bivalves. Collectively, these findings bring new insight toward understanding how nocturnal hypoxia may affect bivalve populations in coastal ecosystems.
In these experiments, DO cycling was ameliorated by vigorous aeration with microbubbles coupled with an extended residence time of seawater via a maze. In contrast, pH was unaffected. pH is influenced by multiple chemical species (CO2, H2CO3, HCO3-, CO32-, HSO4, etc.) (Sarmiento and Gruber, 2006) that react to changes in carbonate chemistry and ultimately change pH (Sarmiento and Gruber, 2006; Tomasetti et al., 2023a; Wallace et al., 2021). In contrast, oxygen, which in an aqueous environment is in the form of a dissolved gas (O2(aq)) was directly and rapidly influenced by forced aeration. Regardless, the amelioration of nocturnal hypoxia alone allowed for experiments to isolate the effects of dissolved oxygen on bivalves.
Hard clams (Mercenaria mercenaria) are known to be less sensitive to low oxygen conditions than other temperate bivalves such as oysters and scallops (Levin et al., 2009; Morrison, 1971; Stevens and Gobler, 2018). As infaunal organisms, hard clams live within sediments that can be anoxic (Nitsche et al., 2007; Ripley, 1998). These bivalves may sometimes even benefit from hypoxia on a population level due to reduced predation pressure (Altieri, 2008; Galligan et al., 2022). A lower sensitivity to hypoxia would account for the small and non-significant changes in growth rates seen in these experiments. On the other hand, hard clam juvenile growth and development has been shown to be significantly impacted by high CO2 conditions (Talmage and Gobler, 2010, 2011; Waldbusser et al., 2010). Had pH cycling been ameliorated, there may have been a larger difference in growth rates compared to the clams exposed to lower nocturnal pH values.
In contrast to hard clams, oysters and scallops significantly benefited from the amelioration of nocturnal hypoxia. Their growth rates significantly increased in the majority of experiments, with aeration-induced increases in growth significantly correlated with the duration of nocturnal hypoxia, and average nocturnal DO concentrations. This is congruent with other studies that have found juvenile oysters and scallops experience decreased growth rates when exposed to static or cycling hypoxia (Gobler et al., 2017, 2014; Johnson et al., 2009; Levin et al., 2009; Stevens and Gobler, 2018; Tomasetti et al., 2023b). Reduced growth rates of bivalves occurred even at hypoxia thresholds of > 3 mg L-1, which is higher than hypoxia thresholds set by most US states (2 mg L-1) (Tomasetti and Gobler, 2020). Extrapolation of results to other locations across NY demonstrated hypoxia can be significantly more sever than this (Supplementary Table 1) and thus the benefits of aeration can be larger (up to 240% increase in bivalve growth rates). To provide managers with a better understanding of the impacts of diel cycles of hypoxia and acidification, more studies need to be conducted on other ecologically and economically important species and across more locations.
Prior research has demonstrated that stressors such as ocean acidification, warming, and hypoxia can be overcome in some bivalves if there is adequate food, providing the excess energy needed upregulate physiological pathways to mitigate stress (Thomsen et al., 2013; Mackenzie et al., 2014; Schwaner et al., 2023). During this study, however, the levels of chlorophyll a were, on average, ~20 µg per L or higher for 8 of the 9 experiments, a level known to be sufficient for the maximal performance of the bivalves studied here (Coutteau et al., 1994; Weiss et al., 2007; Wall et al., 2013). There was a single experiment (scallop experiment 3) when chlorophyll a levels averaged < 5 µg per L, a level known to potentially restrict the growth of bivalves (Coutteau et al., 1994; Weiss et al., 2007; Wall et al., 2013). However, this was also the only scallop experiment where the control outgrew the treatment and there was no nocturnal hypoxia. This experiment also had the highest control DO levels of any of the four scallop experiments, 5.92 ± 1.19 mg L-1, a level not known to impair bay scallop performance (Gurr et al., 2018; Tomasetti et al., 2023b). Moreover, a regression analyses of the mean chlorophyll a level present during each experiment and the increase in growth rate as a consequence of aeration depicted no relationship between these parameters (Supplementary Figure 3). While chlorophyll a may not be a perfect proxy for food availability, it was been a useful predictor in NY estuaries in the past (Weiss et al., 2007; Wall et al., 2013). Regardless, while food supply can be important for ameliorating stress in coastal bivalves, it appears food levels as estimated by chlorophyll a were adequate for nearly all experiments performed here and were unrelated to improved growth rates bivalves experienced as a consequence of higher DO levels.
As temperatures continue to increase across marine habitats, so will oxygen demand, which could lead to oxygen and capacity-limited thermal tolerance (OCLT) (Pörtner and Knust, 2007). Every species has a specific thermal tolerance range; just outside of this optimal range is the pejus range, where conditions will become disadvantageous to the organism. Higher average temperatures and heat waves could have an impact on bivalve populations by limiting their aerobic scope due to higher metabolism rates (Pörtner and Peck, 2010). Most of the experiments performed here were during maximal water annual temperatures in NY, typically around 25°C. When coupled with nocturnal hypoxia, increased temperatures may force organisms into of their respective pejus range on a daily basis (Pörtner and Peck, 2010). At lower DO concentrations, the pejus temperatures are increased, decreasing optimal temperature ranges. The faster growth rates observed within the aerated treatment suggest that more energy was available for processes other than maintaining homeostasis translating to increased growth (Pörtner and Peck, 2010). Due to low DO and lack of excess energy, some proteins may not be produced in the necessary amounts to combat environmental stresses such as heat-shock (Pörtner and Knust, 2007). Over time reductions in an organism’s fitness due to low oxygen supply and high demand coupled with an increased likelihood of experiencing conditions inside of the pejus range will eventually cause reduced population abundances (Pörtner and Peck, 2010).
The results of this study suggest that diel cycling of hypoxia may have significant impacts on fisheries and ecological services in eutrophic coastal regions (Diaz and Rosenberg, 2008; Vaquer-Sunyer and Duarte, 2008). Reductions in juvenile bivalve growth rates due to nocturnal hypoxia would increase the increase risk of predation for individuals prior to reaching predation refuge sizes (Juanes, 1992; Summerson and Peterson, 1984; Wong et al., 2010) increasing population level stressors (Johnson and Smee, 2012). Detrimental nocturnal hypoxia will become more severe as climate change and eutrophication increase (Nixon, 2009; Sunda and Cai, 2012; Tomasetti and Gobler, 2020; Wootton et al., 2008). Rising temperatures could extend the season of severe diel cycling earlier and create more extreme daily maxima and nightly minima due to higher metabolic rates and lower oxygen saturation concentrations (Baumann and Smith, 2018; Sunda and Cai, 2012; Tyler et al., 2009). Increased nutrient loading can promote algal blooms that can also intensify nocturnal hypoxia and the magnitude of diel cycles (Nixon, 2009; Oelsner and Stets, 2019; Paerl et al., 2006; Steel, 1991). Reductions in populations of coastal bivalves due to extreme diel DO cycles would have impacts on important fisheries by reducing harvest and ecosystems services (Newell, 2004).
Current dissolved oxygen regulations are not representative of lethal/sub-lethal oxygen minimum thresholds for many taxa (Vaquer-Sunyer and Duarte, 2008). It has been shown that 61% and 43% of taxa tested experienced mortality and sub-lethal effects, respectively, above a 2 mg L-1 threshold commonly cited in literature (Tomasetti and Gobler, 2020; Vaquer-Sunyer and Duarte, 2008). It has been suggested that a new threshold of 4.6 mg L-1 should be adopted to be representative of the 90th percentile of mean lethal concentrations (Steckbauer et al., 2011; Vaquer-Sunyer and Duarte, 2008). Based on the regression analyses used in this study (Figure 4), however, even at a threshold of 4.6 mg L-1, Eastern oysters and bay scallops could experience a decrease in growth rates of 39%. Further research is needed on other coastal taxa to determine their susceptibility to repeated, short bouts of hypoxia to establish similar representative thresholds. Nocturnal extremes are often not considered within managerial frameworks and, therefore, may allow detrimental conditions to persist without regulatory action (Tomasetti and Gobler, 2020). For example, historically, most dissolved oxygen monitoring has occurred during the day, and during this study hypoxia was generally absent after 0800, even when DO minimums of < 2 mg L-1 occurred only an hour earlier. Advancements in continuous remote monitoring have refined our understanding of nocturnal hypoxia and may, therefore, facilitate future management reform.
Restoration of bivalve populations has received significant attention recently (Duarte et al., 2020; Gobler et al., 2022), with a focus on ecosystem services such as improved water clarity, increased habitat, and reduced algal biomass (Duarte et al., 2020; Kreeger et al., 2018). Two of the main roadblocks to restoring bivalve populations are water quality issues and low natural abundance (Duarte et al., 2020). Diel cycling of hypoxia and acidification affect many of these habitats and may make restoration efforts more difficult due to lower larval survival and slower juvenile growth rates as seen in this study (Wallace et al., 2021). Aquaculture of bivalves may serve as an intermediate step to population and habitat recovery by allowing for more control over conditions and improving water quality (Kreeger et al., 2018). Gobler et al. (2022) described the recovery of a hard clam population in a coastal lagoon in NY using spawner sanctuaries. Given other bivalves species (oysters and scallops) are less tolerant of nocturnal hypoxia compared to M. mercenaria, improved water quality might be needed for such species to experience similar restoration recoveries.
Aquaculture is one of the fastest growing food production sectors in the world with lower trophic levels, including shellfish, constituting about half of all production (Subasinghe et al., 2009; van der Schatte Olivier et al., 2020), and many studies focused on the means to increase aquaculture output (Creswell and McNevin, 2008). Given that oxygenation in flow through systems significantly increased growth rates of Eastern oysters and bay scallops in this study similar results might be achieved in larger but localized aquaculture settings. For example, the inclusion of aeration using floating upweller systems (FLUPSY; (RaLonde, 1999) or similar environmental aeration in semi-enclosed bodies of water could be incorporated to ameliorate nocturnal hypoxia. In the experiments presented here, bivalve densities were low at 20 individuals per replicate (~50 individuals m-2) and high survival was found in both treatments. Given that substantially higher densities (>250 individuals m-2) are common in hatchery and farm settings (Tobi and Ward, 2019; Widman and Rhodes, 1991), it is possible that increased survival could also be achieved via such aeration.
Recent studies have explored co-culturing macroalgae to improve water quality on commercial bivalve farms (Fernández et al., 2019; Young and Gobler, 2018; Young et al., 2022). Young et al. (2022) found that co-culture with sugar kelp (Saccharina latissimi) increased seawater pH and the growth rates of Eastern oysters whereas Young and Gobler (2018) demonstrated that sea lettuce (Ulva spp.) could increase calcium carbonate saturation states and bivalve growth rates. Such studies, however, have not fully investigated the changes in diel cycles of DO nor the manner in which higher DO may benefit bivalves. The introduction of additional photosynthetic organisms could improve oxygen conditions during the day, but respiration could also cause greater declines in nocturnal concentrations. A better understanding of diel cycles during macroalgae co-culture could be achieved via experiments that investigate all water chemistry parameters including DO on a continuous basis. Artificially perturbing diel cycles in the presence of macroalgal co-cultures, by aerating for example, may create an excellent opportunity to investigate this interaction.
Conclusion
Climate change and eutrophication fueled diel cycles of hypoxia and acidification result in organisms being repeatedly subjected to environmental extremes. The experiments presented here demonstrated that amelioration of these conditions improves juvenile bivalve growth compared to individuals experiencing diel DO cycles. Given that experiments were performed with water that displayed only mild nocturnal hypoxia (mean nocturnal DO across all experiments was 4.7 mg L-1), the growth and potentially survival improvement via aeration in more severe hypoxic zones would likely be greater than observed here. Scallop and oyster growth rates were improved on average by 23% and 30%, respectively, and by up to 70% relative to the controls, demonstrating the impact of nocturnal hypoxia on these species. Extrapolation of results to 19 other locations across NY suggested substantially larger benefits at some location with increases in bivalve growth exceeding 100% for half of the locations. While mitigating nocturnal hypoxia via aeration was effective on a small scale, reducing coastal eutrophication to improve DO levels will be needed for sustainable, large-scale improvement.
Data availability statement
The original contributions presented in the study are included in the article/Supplementary Material. Further inquiries can be directed to the corresponding author.
Author contributions
JK: Conceptualization, Data curation, Formal Analysis, Investigation, Validation, Visualization, Writing – original draft, Writing – review & editing. CJG: Conceptualization, Funding acquisition, Methodology, Project administration, Resources, Validation, Visualization, Writing – original draft, Writing – review & editing.
Funding
The author(s) declare that financial support was received for the research and/or publication of this article. This work was support by NOAA’s HAB OA program award NA22NOS4780167 to CJG and a New York Sea Grant award R-FMB-38 to CJG.
Acknowledgments
This work was support by NOAA’s HAB OA program award NA22NOS4780167 to CG and a New York Sea Grant award R-FMB-38 to CG. We thank the Stony Brook – Southampton Marine Science Center Staff for logistical support of experiments and Jennifer Goleski and Andrew Lundstrom for organizing dissolved oxygen data.
Conflict of interest
The authors declare that the research was conducted in the absence of any commercial or financial relationships that could be construed as a potential conflict of interest.
Generative AI statement
The author(s) declare that Generative AI was used in the creation of this manuscript as a tool for gathering general information. All of the text in the manuscript was conceived by and written by the human authors.
Publisher’s note
All claims expressed in this article are solely those of the authors and do not necessarily represent those of their affiliated organizations, or those of the publisher, the editors and the reviewers. Any product that may be evaluated in this article, or claim that may be made by its manufacturer, is not guaranteed or endorsed by the publisher.
Supplementary material
The Supplementary Material for this article can be found online at: https://www.frontiersin.org/articles/10.3389/fmars.2025.1535142/full#supplementary-material
References
Alter K., Paschke K., Gebauer P., Cumillaf J.-P., Pörtner H.-O. (2015). Differential physiological responses to oxygen availability in early life stages of decapods developing in distinct environments. Marine. Biol. 162, 1111–1124. doi: 10.1007/s00227-015-2654-4
Altieri A. H. (2008). Dead zones enhance key fisheries species by providing predation refuge. Ecology 89, 2808–2818. doi: 10.1890/07-0994.1
Baumann H., Smith E. M. (2018). Quantifying Metabolically Driven pH and Oxygen Fluctuations in US Nearshore Habitats at Diel to Interannual Time Scales. Estuaries. Coasts. 41, 1102–1117. doi: 10.1007/s12237-017-0321-3
Baumann H., Wallace R. B., Tagliaferri T., Gobler C. J. (2015). Large natural pH, CO2 and O2 fluctuations in a temperate tidal salt marsh on diel, seasonal, and interannual time scales. Estuaries. Coasts. 38, 220–231. doi: 10.1007/s12237-014-9800-y
Beck N. G., Bruland K. W. (2000). Diel biogeochemical cycling in a hyperventilating shallow estuarine environment. Estuaries 23, 177–187. doi: 10.2307/1352825
Borthagaray A. I., Carranza A. (2007). Mussels as ecosystem engineers: Their contribution to species richness in a rocky littoral community. Acta Oecol. 31, 243–250. doi: 10.1016/j.actao.2006.10.008
Breitburg D. L. (1990). Near-shore hypoxia in the Chesapeake Bay: Patterns and relationships among physical factors. Estuarine. Coastal. Shelf. Sci. 30, 593–609. doi: 10.1016/0272-7714(90)90095-9
Breitburg D., Levin L. A., Oschlies A., Grégoire M., Chavez F. P., Conley D. J., et al. (2018). Declining oxygen in the global ocean and coastal waters. Science 359, eaam7240. doi: 10.1126/science.aam7240
Burkholder J. M. (1998). Implications of harmful microalgae and heterotrophic dinoflagellates in management of sustainable marine fisheries. Ecol. Appl. 8, S37–S62. doi: 10.1890/1051-0761(1998)8[S37:IOHMAH]2.0.CO;2
Burkholder J. M., Glibert P. M., Skelton H. M. (2008). Mixotrophy, a major mode of nutrition for harmful algal species in eutrophic waters. Harmful. Algae. 8, 77–93. doi: 10.1016/j.hal.2008.08.010
Caffrey J. M. (2004). Factors controlling net ecosystem metabolism in U.S. estuaries. Estuaries 27, 90–101. doi: 10.1007/bf02803563
Clark H., Gobler C. (2016). Diurnal fluctuations in CO2 and dissolved oxygen concentrations do not provide a refuge from hypoxia and acidification for early-life-stage bivalves. Marine. Ecol. Prog. Ser. 558, 1–14. doi: 10.3354/meps11852
Coutteau P., Hadley N. H., Manzi J. J., Sorgeloos P. (1994). Effect of algal ration and substitution of algae by manipulated yeast diets on the growth of juvenile Mercenaria mercenaria. Aquaculture 120, 135–150. doi: 10.1016/0044-8486(94)90229-1
Creswell R. L., McNevin A. A. (2008). “Better management practices for bivalve molluscan aquaculture,” in Environmental best management practices for aquaculture, eds. Tucker C. S., Hargreaves J. A. (Hoboken, New Jersey: John Wiley & Sons, Inc), 427–486. doi: 10.1002/9780813818672
Diaz R. J., Rosenberg R. (2008). Spreading dead zones and consequences for marine ecosystems. Science 321, 926–929. doi: 10.1126/science.1156401
Duarte C. M., Agusti S., Barbier E., Britten G. L., Castilla J. C., Gattuso J.-P., et al. (2020). Rebuilding marine life. Nature 580, 39–51. doi: 10.1038/s41586-020-2146-7
Fernández P. A., Leal P. P., Henríquez L. A. (2019). Co-culture in marine farms: macroalgae can act as chemical refuge for shell-forming molluscs under an ocean acidification scenario. Phycologia 58, 542–551. doi: 10.1080/00318884.2019.1628576
Galligan B. P., Stuart Y. E., McManus M. C., Stoffel H. E. (2022). Hypoxia-induced predation refuge for northern quahogs (Mercenaria mercenaria) in a temperate estuary. Estuarine. Coastal. Shelf. Sci. 265, 107732. doi: 10.1016/j.ecss.2021.107732
Gobler C. J., Baumann H. (2016). Hypoxia and acidification in ocean ecosystems: coupled dynamics and effects on marine life. Biol. Lett. 12, 20150976. doi: 10.1098/rsbl.2015.0976
Gobler C. J., Clark H. R., Griffith A. W., Lusty M. W. (2017). Diurnal Fluctuations in Acidification and Hypoxia Reduce Growth and Survival of Larval and Juvenile Bay Scallops (Argopecten irradians) and Hard Clams (Mercenaria mercenaria). Front. Marine. Sci. 3. doi: 10.3389/fmars.2016.00282
Gobler C. J., Depasquale E. L., Griffith A. W., Baumann H. (2014). Hypoxia and acidification have additive and synergistic negative effects on the growth, survival, and metamorphosis of early life stage bivalves. PloS One 9, e83648. doi: 10.1371/journal.pone.0083648
Gobler C. J., Doall M. H., Peterson B. J., Young C. S., DeLaney F., Wallace R. B., et al. (2022). Rebuilding A collapsed bivalve population, restoring seagrass meadows, and eradicating harmful algal blooms in A temperate lagoon using spawner sanctuaries. Front. Marine. Sci. 9. doi: 10.3389/fmars.2022.911731
Gurr S. J., Goleski J., Lima F. P., Seabra R., Gobler C. J., Volkenborn N. (2018). Cardiac responses of the bay scallop Argopecten irradians to diel-cycling hypoxia. J. Exp. Marine. Biol. Ecol. 500, 18–29. doi: 10.1016/j.jembe.2017.12.011
Gutiérrez J. L., Jones C. G., Strayer D. L., Iribarne O. O. (2003). Mollusks as ecosystem engineers: the role of shell production in aquatic habitats. Oikos 101, 79–90. doi: 10.1034/j.1600-0706.2003.12322.x
Heisler J., Glibert P. M., Burkholder J. M., Anderson D. M., Cochlan W., Dennison W. C., et al. (2008). Eutrophication and harmful algal blooms: A scientific consensus. Harmful. Algae. 8, 3–13. doi: 10.1016/j.hal.2008.08.006
Johnson M. W., Powers S. P., Senne J., Park K. (2009). Assessing in situ tolerances of eastern oysters (Crassostrea virginica) under moderate hypoxic regimes: implications for restoration. J. Shellfish. Res. 28, 185–192. doi: 10.2983/035.028.0202
Johnson K. D., Smee D. L. (2012). Size matters for risk assessment and resource allocation in bivalves. Marine. Ecol. Prog. Ser. 462, 103–110. doi: 10.3354/meps09804
Juanes F. (1992). Why do decapod crustaceans prefer small-sized molluscan prey? Marine. Ecology-Prog. Ser. 87, 239–239. doi: 10.3354/meps087239
Kennedy V. S., Newell R. I., Eble A. F., Leffler M., Harpe S. R. D. (1996). The eastern oyster: Crassostrea virginica (College Park, MD: University of Maryland Sea Grant College).
Keppel A. G., Breitburg D. L., Burrell R. B. (2016). Effects of co-varying diel-cycling hypoxia and pH on growth in the juvenile eastern oyster, Crassostrea virginica. PloS One 11, e0161088. doi: 10.1371/journal.pone.0161088
Kraeuter J. N., Castagna M. (Eds.) (2001). Biology of the hard clam Vol. 31 (Amsterdam, Netherlands: Elsevier).
Kreeger D. A., Gatenby C. M., Bergstrom P. W. (2018). Restoration potential of several native species of bivalve molluscs for water quality improvement in mid-Atlantic watersheds. J. Shellfish. Res. 37, 1121–1157. doi: 10.2983/035.037.0524
Levin L. A., Ekau W., Gooday A. J., Jorissen F., Middelburg J. J., Naqvi S. W. A., et al. (2009). Effects of natural and human-induced hypoxia on coastal benthos. Biogeosciences 6, 2063–2098. doi: 10.5194/bg-6-2063-2009
Mackenzie C. L., Ormondroyd G. A., Curling S. F., Ball R. J., Whiteley N. M., Malham S. K. (2014). Ocean warming, more than acidification, reduces shell strength in a commercial shellfish species during food limitation. PloS One 9, e86764. doi: 10.1371/journal.pone.0086764
Miller S., Breitburg D., Burrell R., Keppel A. (2016). Acidification increases sensitivity to hypoxia in important forage fishes. Marine. Ecol. Prog. Ser. 549, 1–8. doi: 10.3354/meps11695
Morrison G. (1971). Dissolved oxygen requirements for embryonic and larval development of the hardshell clam, Mercenaria mercenaria. J. Fish. Board. Canada. 28, 379–381. doi: 10.1139/f71-050
Newell R. I. (2004). Ecosystem influences of natural and cultivated populations of suspension-feeding bivalve molluscs: a review. J. Shellfish Res. 23 (1), 51–62.
Nitsche F. O., Ryan W. B. F., Carbotte S. M., Bell R. E., Slagle A., Bertinado C., et al. (2007). Regional patterns and local variations of sediment distribution in the Hudson River Estuary. Estuarine. Coastal. Shelf. Sci. 71, 259–277. doi: 10.1016/j.ecss.2006.07.021
Nixon S. W. (1995). Coastal marine eutrophication: a definition, social causes, and future concerns. Ophelia 41, 199–219. doi: 10.1080/00785236.1995.10422044
Nixon S. W. (2009). “Eutrophication and the macroscope,” in Eutrophication in Coastal Ecosystems (Springer Netherlands), 5–19. doi: 10.1007/978-90-481-3385-7_2
NOAA (2019). NOAA Fisheries Commercial Landings Data Query. Available online at: https://www.fisheries.noaa.gov/foss/f?p=215:200:::::: (Accessed May 5, 2025).
Oelsner G. P., Stets E. G. (2019). Recent trends in nutrient and sediment loading to coastal areas of the conterminous US: Insights and global context. Sci. Total. Environ. 654, 1225–1240. doi: 10.1016/j.scitotenv.2018.10.437
Paerl H. W., Valdes L. M., Peierls B. L., Adolf J. E., Harding L. J. W. (2006). Anthropogenic and climatic influences on the eutrophication of large estuarine ecosystems. Limnol. Oceanogr. 51, 448–462. doi: 10.4319/lo.2006.51.1_part_2.0448
Peterson C. H., Black R. (1987). Resource depletion by active suspension feeders on tidal fiats: Influence of local density and tidal elevation1. Limnol. Oceanogr. 32, 143–166. doi: 10.4319/lo.1987.32.1.0143
Pörtner H. O., Knust R. (2007). Climate change affects marine fishes through the oxygen limitation of thermal tolerance. Science 315, 95–97. doi: 10.1126/science.1135471
Pörtner H. O. (2012). Integrating climate-related stressor effects on marine organisms: unifying principles linking molecule to ecosystem-level changes. Marine. Ecol. Prog. Ser. 470, 273–290. doi: 10.3354/meps10123
Pörtner H. O., Peck M. A. (2010). Climate change effects on fishes and fisheries: towards a cause-and-effect understanding. J. Fish. Biol. 77, 1745–1779. doi: 10.1111/j.1095-8649.2010.02783.x
RaLonde R. (1999). Final Report of the Kachemak Bay Shellfish Nursery Culture Project 1997-98. Alaska Dep. Fish and Game. (Juneau, Alaska). 53 pp.
Ripley B. J. (1998). Life history traits and population processes in marine bivalve molluscs (Cambridge, MA: Massachusetts Institute of Technology, Dept. of Biology). 181 pages.
Sarmiento J. L., Gruber N. (2006). Ocean Biogeochemical Dynamics (Princeton, NJ: Princeton University Press). doi: 10.2307/j.ctt3fgxqx
Schwaner C., Barbosa M., Schwemmer T. G., Pales Espinosa E., Allam B. (2023). Increased food resources help eastern oyster mitigate the negative impacts of coastal acidification. Animals 13, 1161. doi: 10.3390/ani13071161
Shumway S. E. (1990). A review of the effects of algal blooms on shellfish and aquaculture. J. World Aquacult. Soc. 21, 65–104. doi: 10.1111/j.1749-7345.1990.tb00529.x
Steckbauer A., Duarte C. M., Carstensen J., Vaquer-Sunyer R., Conley D. J. (2011). Ecosystem impacts of hypoxia: thresholds of hypoxia and pathways to recovery. Environ. Res. Lett. 6, 25003. doi: 10.1088/1748-9326/6/2/025003
Steckbauer A., Ramajo L., Hendriks I. E., Fernandez M., Lagos N. A., Prado L., et al. (2015). Synergistic effects of hypoxia and increasing CO2 on benthic invertebrates of the central Chilean coast. Front. Marine. Sci. 2. doi: 10.3389/fmars.2015.00049
Steel J. (1991). Status and trends report of the Albemarle-Pamlico estuarine study (Raleigh: NC DEHNR and US EPA National Estuarine Program).
Stevens A., Gobler C. (2018). Interactive effects of acidification, hypoxia, and thermal stress on growth, respiration, and survival of four North Atlantic bivalves. Marine. Ecol. Prog. Ser. 604, 143–161. doi: 10.3354/meps12725
Subasinghe R., Soto D., Jia J. (2009). Global aquaculture and its role in sustainable development. Rev. Aquacult. 1, 2–9. doi: 10.1111/j.1753-5131.2008.01002.x
Summerson H. C., Peterson C. H. (1984). Role of predation in organizing benthic communities of a temperate-zone seagrass bed. Marine. Ecol. Prog. Ser. 15, 63–77. doi: 10.3354/meps015063
Sunda W. G., Cai W.-J. (2012). Eutrophication induced CO2-acidification of subsurface coastal waters: interactive effects of temperature, salinity, and atmospheric p CO2. Environ. Sci. Technol. 46, 10651–10659. doi: 10.1021/es300626f
Talmage S. C., Gobler C. J. (2010). Effects of past, present, and future ocean carbon dioxide concentrations on the growth and survival of larval shellfish. Proc. Natl. Acad. Sci. 107, 17246–17251. doi: 10.1073/pnas.0913804107
Talmage S. C., Gobler C. J. (2011). Effects of Elevated Temperature and carbon dioxide on the growth and survival of larvae and juveniles of three species of northwest atlantic bivalves. PloS One 6, e26941. doi: 10.1371/journal.pone.0026941
Thomsen J., Casties I., Pansch C., Körtzinger A., Melzner F. (2013). Food availability outweighs ocean acidification effects in juvenile M ytilus edulis: laboratory and field experiments. Global Change Biol. 19, 1017–1027. doi: 10.1111/gcb.2013.19.issue-4
Tobi H., Ward D. (2019). Nursery and Grow‐Out Strategy Optimization in Bay Scallop Argopecten irradians Aquaculture. N. Am. J. Aquac. 81 (2), 130–139.
Tomasetti S. J., Doall M. H., Hallinan B. D., Kraemer J. R. Jr., Gobler C. J. (2023a). Oyster reefs’ control of carbonate chemistry—Implications for oyster reef restoration in estuaries subject to coastal ocean acidification. Global Change Biol. 29, 6572–6590. doi: 10.1111/gcb.16960
Tomasetti S. J., Gobler C. J. (2020). Dissolved oxygen and pH criteria leave fisheries at risk. Science 368, 372–373. doi: 10.1126/science.aba4896
Tomasetti S. J., Hallinan B. D., Tettelbach S. T., Volkenborn N., Doherty O. W., Allam B., et al. (2023b). Warming and hypoxia reduce the performance and survival of northern bay scallops (Argopecten irradians irradians) amid a fishery collapse. Global Change Biol. 29, 2092–2107. doi: 10.1111/gcb.16575
Tomasetti S. J., Kraemer J. R., Gobler C. J. (2021). Brief episodes of nocturnal hypoxia and acidification reduce survival of economically important blue crab (Callinectes sapidus) larvae. Front. Marine. Sci. 8. doi: 10.3389/fmars.2021.720175
Tomasetti S. J., Morrell B. K., Merlo L. R., Gobler C. J. (2018). Individual and combined effects of low dissolved oxygen and low pH on survival of early stage larval blue crabs, Callinectes sapidus. PloS One 13, e0208629. doi: 10.1371/journal.pone.0208629
Tyler R. M., Brady D. C., Targett T. E. (2009). Temporal and spatial dynamics of diel-cycling hypoxia in estuarine tributaries. Estuaries. Coasts. 32, 123–145. doi: 10.1007/s12237-008-9108-x
van der Schatte Olivier A., Jones L., Vay L. L., Christie M., Wilson J., Malham S. K. (2020). A global review of the ecosystem services provided by bivalve aquaculture. Rev. Aquacult. 12, 3–25. doi: 10.1111/raq.12301
Vaquer-Sunyer R., Duarte C. M. (2008). Thresholds of hypoxia for marine biodiversity. Proc. Natl. Acad. Sci. 105, 15452–15457. doi: 10.1073/pnas.0803833105
Waldbusser G. G., Bergschneider H., Green M. A. (2010). Size-dependent pH effect on calcification in post-larval hard clam Mercenaria spp. Marine. Ecol. Prog. Ser. 417, 171–182. doi: 10.3354/meps08809
Waldbusser G. G., Salisbury J. E. (2014). Ocean acidification in the coastal zone from an organism’s perspective: multiple system parameters, frequency domains, and habitats. Annu. Rev. Marine Sci. 6, 221–247. doi: 10.1146/annurev-marine-121211-172238
Wall C. C., Gobler C. J., Peterson B. J., Ward J. E. (2013). Contrasting Growth Patterns of Suspension-Feeding Molluscs (Mercenaria mercenaria, Crassostrea virginica, Argopecten irradians, and Crepidula fornicata) Across a Eutrophication Gradient in the Peconic Estuary, NY, USA. Estuaries. Coasts. 36, 1274–1291. doi: 10.1007/s12237-013-9632-1
Wallace R. B., Baumann H., Grear J. S., Aller R. C., Gobler C. J. (2014). Coastal ocean acidification: The other eutrophication problem. Estuarine. Coastal. Shelf. Sci. 148, 1–13. doi: 10.1016/j.ecss.2014.05.027
Wallace R. B., Gobler C. J. (2021). The role of algal blooms and community respiration in controlling the temporal and spatial dynamics of hypoxia and acidification in eutrophic estuaries. Marine. Pollut. Bull. 172, 112908. doi: 10.1016/j.marpolbul.2021.112908
Wallace R. B., Peterson B. J., Gobler C. J. (2021). Ecosystem metabolism modulates the dynamics of hypoxia and acidification across temperate coastal habitat types. Front. Marine. Sci. 8, 611781. doi: 10.3389/fmars.2021.611781
Wang W. X., Widdows J. (1993). Metabolic responses of the common mussel Mytilus edulis to hypoxia and anoxia. Marine. Ecology-Prog. Ser. 95, 205–205. doi: 10.3354/meps095205
Weiss M. B., Curran P. B., Peterson B. J., Gobler C. J. (2007). The influence of plankton composition and water quality on hard clam (Mercenaria mercenaria L.) populations across Long Island’s south shore lagoon estuaries (New York, USA). J. Exp. Marine. Biol. Ecol. 345, 12–25. doi: 10.1016/j.jembe.2006.12.025
Welschmeyer N. A. (1994). Fluorometric analysis of chlorophyll a in the presence of chlorophyll b and pheopigments. Limnol. Oceanogr 39, 1985–1992. doi: 10.4319/lo.1994.39.8.1985
Widman J. C., Rhodes E. W. (1991). Nursery culture of the bay scallop, Argopecten irradians irradians, in suspended mesh nets. Aquaculture. 99 (3-4), 257–267.
Wijsman J., Troost K., Fang J., Roncarati A. (2019). “Global production of marine bivalves. Trends and challenges.” in Goods and Services of Marine Bivalves, eds. A. C. Smaal, J. G. Ferreira, JonGrant, J. K. Petersen, Ø. Strand., 7–26. doi: 10.1007/978-3-319-96776-9
Wong M. C., Peterson C. H., Kay J. (2010). Prey size selection and bottom type influence multiple predator effects in a crab–bivalve system. Marine. Ecol. Prog. Ser. 409, 143–156. doi: 10.3354/meps08621
Wootton J. T., Pfister C. A., Forester J. D. (2008). Dynamic patterns and ecological impacts of declining ocean pH in a high-resolution multi-year dataset. Proc. Natl. Acad. Sci. 105, 18848–18853. doi: 10.1073/pnas.0810079105
Young C. S., Gobler C. J. (2018). The ability of macroalgae to mitigate the negative effects of ocean acidification on four species of North Atlantic bivalve. Biogeosciences 15, 6167–6183. doi: 10.5194/bg-15-6167-2018
Young C. S., Sylvers L. H., Tomasetti S. J., Lundstrom A., Schenone C., Doall M. H., et al. (2022). Kelp (Saccharina latissima) mitigates coastal ocean acidification and increases the growth of north atlantic bivalves in lab experiments and on an oyster farm. Front. Marine. Sci. 9. doi: 10.3389/fmars.2022.881254
Keywords: hypoxia, Mercenaria mercenaria, Crassostrea virginica, Argopecten irradians, diel cycling hypoxia
Citation: Kraemer J and Gobler CJ (2025) Natural estuarine cycles of nocturnal hypoxia significantly reduce growth rates of North Atlantic bivalves. Front. Mar. Sci. 12:1535142. doi: 10.3389/fmars.2025.1535142
Received: 26 November 2024; Accepted: 14 April 2025;
Published: 14 May 2025.
Edited by:
Elva G. Escobar-Briones, National Autonomous University of Mexico, MexicoReviewed by:
Raul Llera-Herrera, National Autonomous University of Mexico, MexicoChiara Pennesi, Anton Dohrn Zoological Station Naples, Italy
Copyright © 2025 Kraemer and Gobler. This is an open-access article distributed under the terms of the Creative Commons Attribution License (CC BY). The use, distribution or reproduction in other forums is permitted, provided the original author(s) and the copyright owner(s) are credited and that the original publication in this journal is cited, in accordance with accepted academic practice. No use, distribution or reproduction is permitted which does not comply with these terms.
*Correspondence: Christopher J. Gobler, Y2hyaXN0b3BoZXIuZ29ibGVyQHN0b255YnJvb2suZWR1