- 1Engineering Research Center of Eco-environment in Three Gorges Reservoir Region, Ministry of Education, China Three Gorges University, Yichang, China
- 2School of Energy and Power Engineering, Xihua University, Chengdu, China
- 3School of Agriculture and Biology, Shanghai Jiao Tong University, Shanghai, China
- 4State Key Laboratory of Hydraulics and Mountain River Engineering, Sichuan University, Chengdu, China
- 5School of Environmental Science and Engineering, Southwest Jiaotong University, Chengdu, Sichuan, China
Total dissolved gas supersaturation (TDGS) due to flood or hydropower station discharge adversely affects the swimming performance of migratory fish, thereby reducing passage efficiency. This study assessed the swimming performance of bighead carp in an experimental vertical slot fishway under varied slot flow velocities of 0.2, 0.25, and 0.3 m/s after 2 h of exposure to different levels of TDGS water. The results demonstrated that increased TDGS levels and flow velocities significantly reduced the fish passage efficiency. Specifically, passage success rates reached 61%, 48%, 37%, and 37% at TDGS levels of 100%, 110%, 120%, and 130% respectively, at a flow velocity of 0.2 m/s. At flow velocities of 0.2, 0.25, and 0.3 m/s with 100% TDGS water, success rates were 61%, 53%, and 47%, respectively. Moreover, increased TDGS levels and increased flow velocities notably extended the passage time in the fishway. Both TDGS levels and fishway flow velocities significantly influenced the swimming trajectories of the fish. Preferences for flow velocities were notably affected by the TDGS levels, whereas preferences for turbulent kinetic energy were affected by both the TDGS levels and the velocities of the fishway flow. In this study, an evaluation method was developed to assess the adverse effects of TDGS on fish passage efficiency based on the following critical parameters: passage success rate, time, trajectory, and preferred hydraulic factor. This study offers valuable insights for optimizing operations and fishway management to enhance fish protection.
1 Introduction
The construction and operation of hydropower stations have altered the natural hydraulic characteristics of rivers, causing a series of environmental issues. These include reduced temperature variability (Wang et al., 2020b), marked reductions in sediment content (Yang et al., 2014), habitat fragmentation (Zheng et al., 2024), and total dissolved gas supersaturation (TDGS) (Wang et al., 2020a). During floods or hydropower station discharges, gas becomes entrained in the high-velocity flow, resulting in a gas-water mixture. This mixture, under high pressure in the stilling basin, leads to the formation of TDGS water (Li et al., 2022). Owing to its slow gas release rate, such water can extend along considerable stretches of the river, posing significant risks to aquatic life. Fish are particularly susceptible to gas bubble trauma (GBT) caused by TDGS, which may result in abnormal behavior, organ damage, and mortality (Yuan et al., 2021). Elevated TDGS levels in high dams in the upper Yangtze River represent a substantial threat to fish populations (Qu et al., 2011). Moreover, the cascading hydropower stations increase the extent of river regulation, thereby prolonging the impact of the TDGS during the flood season. Consequently, the Yangtze River has become one of the three key areas for global TDGS studies (Pleizier et al., 2020).
The operation of cascading power stations along the Yangtze River has introduced another environmental challenge: reduced river connectivity (Zheng et al., 2024). This cascade of dams has resulted in the loss of vital habitats for 46 endemic species, disrupted the populations of 134 fish species, and obstructed the migratory routes of 35 potamodromous fish species (Cheng et al., 2015). Migratory fish, which rely on movement between diverse habitats for spawning, nursing, and foraging, are particularly affected (Pan et al., 2024). Therefore, the Yangtze River has become a focus for the development of fish passage facilities, as highlighted by the considerable conservation value of local fish species that encounter various barriers. To date, 28 fishways have been constructed to mitigate these disruptions, including seven large-scale projects (Liu et al., 2019). Among these, the vertical slot fishway (VSF) design has been widely adopted and is essential for enabling the passage of fish over substantial artificial barriers, such as dams, weirs, and sluices (Puertas et al., 2012). Given the diverse and rich potamodromous fish population in the Yangtze River Basin, conducting efficient assessments of passage efficiency through these fishways is crucial.
The efficacy of fishways depends critically on the swimming performance of the target fish, which is influenced by various factors (Zhang et al., 2024). A significant yet overlooked environmental issue for migratory fish in the Yangtze River during the flood season is the impact of the TDGS. As a result of flood or hydropower station discharge, TDGS water extends along stretches below the dams, forcing fish to experience prolonged TDGS exposure before reaching the fishway entrances. Research indicates that TDGS can impair critical and burst swimming abilities in species like the Chinese sucker, rock carp, and bighead carp, including the Chinese sucker Schizothorax, rock carp, and bighead carp (Li et al., 2019; Wang et al., 2017; Yuan et al., 2023; Zhang et al., 2023). These findings suggest that TDGS could potentially compromise the passage performance of fish. However, most studies on the effects of TDGS on fish swimming performance have relied on enclosed swim chamber methods to assess critical and burst swimming abilities, making it difficult to directly apply these findings to assess the impact of TDGS on fish passage efficiency.
Recent studies have increasingly focused on the volitional swimming behavior of fish in open channels, which provides richer insights, including swimming motivation and behavioral responses, compared to forced swimming tests conducted in swim chambers (Bayse et al., 2019; Tan et al., 2019). Fish swimming ability is not the sole determinant of overcoming the flow barriers presented by fishways. Rather, the motivation of the fish and active attempts to navigate these passages are equally critical, as they are influenced by various factors such as physiological conditions, olfactory signals, and their responses to water currents (Castro-Santos et al., 2013; Elings et al., 2024; Tan et al., 2019). For example, the optimal upstream migration efficiency of Scaphirhynchus platorynchus was noted in fishways with flow velocities between 0.6 and 0.9 m/s, with flow velocities below 0.6 m/s being insufficiently attractive and those above 0.9 m/s obstructing fish migration (White and Mefford, 2002). Furthermore, TDGS levels can influence fish behavior under diverse flow conditions (Pleizier et al., 2023; Yuan et al., 2023). Thus, current swim chamber research on the impact of TDGS on swimming capabilities is insufficient to provide a comprehensive evaluation method for assessing the effectiveness of fishways under the influence of TDGS. Currently, it is essential to assess the impact of TDGS on fishway performance, particularly for fish that migrate during the flood season.
Four native Chinese carp species (grass carp (Ctenopharyngodon idella), black carp (Mylopharyngodon piceus), silver carp (Hypophthalmichthys molitrix), and bighead carp (Hypophthalmichthys nobilis)) represent China’s most commercially significant Asian carp species, contributing to 63% of China’s total natural fishery production (Wu et al., 1992). However, by 2005, the abundance of these species had declined to a mere 4.2% of historical averages, largely because of extensive hydraulic project construction (Duan et al., 2010). This study specifically focused on the bighead carp, which predominantly inhabits the surface area of water. Previous research has suggested that adequate depth compensation can mitigate GBT with a recommended 10% TDGS compensation per meter of depth (Fickeisen and Montgomery, 1978). Consequently, fish residing in the surface waters are more vulnerable to TDGS than those residing in deeper waters.
Therefore, this study investigated the swimming performance of bighead carp in an experimental VSF after TDGS exposure. This study aimed to develop an evaluation method for assessing the impact of TDGS on fish passage efficiency, focusing on several key aspects, including passage success rate, passage time, characteristic routes, and preferred hydrodynamic factors. Additionally, this study examined whether changes in flow velocities could mitigate the negative effects of TDGS on fishway passage efficiency. These findings are expected to provide scientific support for fish protection related to TDGS issues and offer theoretical insights for improving the efficiency of fishways during hydropower station discharge.
2 Materials and methods
2.1 Experimental apparatus and design of TDGS conditions
To effectively replicate the conditions encountered by bighead carp during floods or hydropower station discharges, an experiment was designed to replicate the scenario in which fish, after being exposed to TDGS conditions, reached the entrance of a fishway and began their upstream migration. Prior to assessing swimming performance in the VSF, bighead carp were exposed to different levels of TDGS water. The exposure lasted for 2 h, reflecting a realistic scenario based on field observations. Notably, during the Yangtze River flood season, the maximum recorded TDGS level was approximately 130%. To investigate the threshold effects of TDGS on fish swimming performance, TDGS exposure conditions were set as 100%, 110%, 120%, and 130%.
The generation and exposure systems for the TDGS water were set up at the Key Laboratory of Fluid Machinery and Engineering of Xihua University. This system was described in detail by Li et al. (2019). The TDGS water generation system was operated by pumping freshwater from a reservoir into a high-pressure autoclave, into which air was simultaneously injected using an air pump. Under these high-pressure conditions, the mixture of water and gas yielded a high level of TDGS. The exposure system consisted of several acrylic overflow tanks equipped with two pipes. One pipe, fitted with a valve, transported highly supersaturated water from the TDGS generation system, whereas the second pipe introduced equilibrated water from the reservoir. The TDGS level within the exposure tanks was meticulously controlled by adjusting the valves in both pipes, thereby regulating the mixing of the two types of water to achieve the required TDGS levels.
During TDGS exposure, critical parameters, including TDGS levels, temperature, and pH, were continuously monitored and controlled. TDGS levels were quantified using a TDGS pressure meter (PT4 TRACKER, Point Four Systems Inc., Canada), which recorded levels at 102% ± 1%, 108% ± 2%, 121% ± 1%, and 132% ± 2%. Temperature was consistently tracked with a portable water quality monitor (AZ8351, Hengxin Inc., China), maintaining an average of 24 ± 0.6°C. The pH was measured with a multiparameter water quality monitor (YSI6600V2, NCL of Wisconsin, Inc., USA), which showed a stable pH of 7.6 ± 0.1. After 2 h of TDGS exposure, there were a few instances of mortality (Table 1). Among the deceased juveniles, symptoms of GBT, such as hemorrhage and popped eyes, were observed, indicating a severe impact of elevated TDGS levels on the fish.
2.2 Experimental VSF
The experimental VSF facility was constructed at the Key Laboratory of Fluid Machinery and Engineering, Xihua University. The facility was comprised of an acrylic structure, a large reservoir, and a pump system that facilitates water circulation. This structure was externally supported by a steel frame measuring 17 m in length, 0.5 m in width, and 0.4 m in height. For enhanced visibility of the fish during the experiments, the bottom of the structure was lined with white waterproof paper (Figure 1A). Water was pumped from the reservoir to the upstream end of the fishway using a steel pipe. The middle section of the fishway featured 11 pools constructed using alternating long and short acrylic baffles; detailed dimensions and descriptions are provided in Figure 1B. Downstream, two screens spaced 1 m apart created zones for fish acclimation. Flow was regulated using a manual valve at the upstream end for flow adjustment and a tail gate at the downstream end to control the water level. To record and analyze fish behavior, fluorite cameras (C6C, 1080p, 25 FPS) were installed above pools #1, #6, and #11 (Figure 1A). These cameras captured real-time video footage, which was monitored and managed using the Ezviz Studio software. Specifically, cameras positioned above pools #1 and #11 focused on recording the passage success of the bighead carp, whereas cameras above pool #6 tracked the passage time and trajectories of fish through the central pool, as illustrated in Figure 1C.
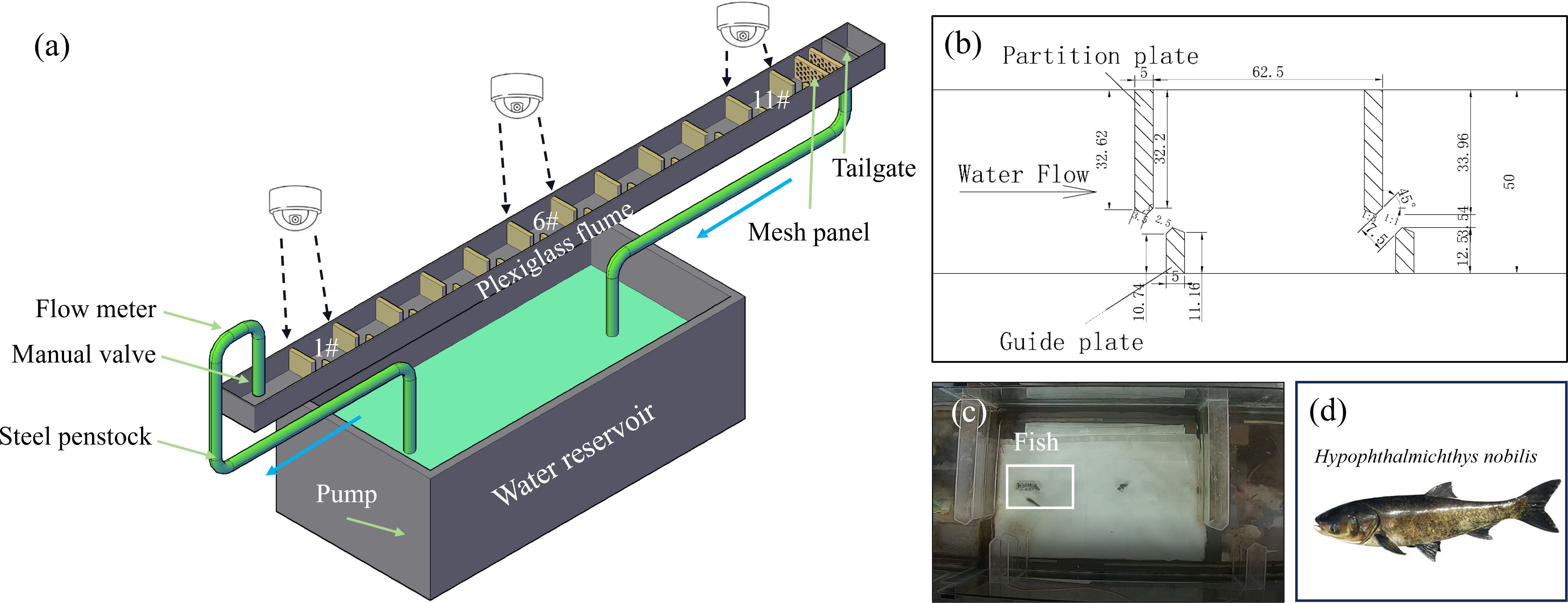
Figure 1. Experimental apparatus and target fish: (A) overall view of the VSF, (B) specific dimensions of a single pool, (C) camera footage of the pool, and (D) target fish.
2.3 Fish species husbandry and trials
Juvenile bighead carp, supplied by the Sichuan Province Academy of Agricultural Sciences Institute of Fisheries (Figure 1D), were transported to the Key Laboratory of Fluid Machinery and Engineering at Xihua University for experimentation. Upon arrival, the fish were acclimatized in a glass pool measuring 4 m in length, 1 m in width, and 1 m in depth. The pool was filled with pre-aerated tap water to facilitate acclimation to the new environment. To ensure optimal conditions, the fish were continuously oxygenated for 48 h. During this period, the monitoring of environmental parameters was rigorous, with dissolved oxygen (DO) levels maintained at 8.1 ± 0.2 mg/L, pH levels at 7.2 ± 0.3, and temperatures at 23 ± 0.6°C. To minimize stress and maintain consistent experimental conditions, fish were not fed during the acclimatization and experimental phases.
Juvenile fish were exposed to varying TDGS levels (100%, 110%, 120%, and 130%) for 2 h. Following this exposure, the surviving fish were transferred to an experimental VSF, where they were observed to move upstream under three different slot flow velocity scenarios. These scenarios were specifically designed based on the swimming capabilities of the bighead carp to negotiate the vertical slot located between the downstream long and short baffles in the fishway.
The detailed conditions of these scenarios, including the flow velocity, inflow rate, inflow velocity, inflow height, and outflow height at the critical vertical slot are listed in Table 2. Preliminary experiments indicated that 2h exposure to 130% TDGS allowed for sufficient analysis while maintaining fish survival rates, as it allowed a sufficient number of fish to successfully pass through the fishway, facilitating effective analysis. Consequently, the flow velocities at the slot were designed at 0.2, 0.25, and 0.3 m/s, resulting in a total of 12 (3 × 4) conditions (Table 1).

Table 2. Details of hydraulic parameters for three flow velocity scenarios in an experimental vertical slot fishway.
After TDGS exposure, the bighead carp were transferred to an acclimation zone situated downstream of the experimental fishway, defined by two nets spaced one meter apart. This setup facilitated a 20-min acclimation period designed to alleviate stress from transportation and adapt to the experimental environment. Following acclimation, the upstream net was removed, allowing the fish to navigate upstream within the fishway for 30 min. Each fish participated in the test once to evaluate their upstream movement performance. To reflect natural group behaviors and enhance the reliability of the results, seven to nine fish were simultaneously released in each trial and each condition included five to eight trials. The specifics of the fish used in these trials, including body length and weight, for each scenario tested are detailed in Table 1.
2.4 Hydraulic measurements and numerical simulation
2.4.1 Particle image velocimetry system and measurement process
The experimental VSF consisted of 11 pools. Given the pronounced effects of the upstream and downstream boundary conditions on the first and last pools, flow field measurements were conducted in the middle pool, designated as pool #6. The measured water depth was set to 0.5 H0, where H0 (0.2 m) represents the total water depth. The arrangement of the flow-velocity measurement points within the pool is illustrated in Figure 2A. These points were systematically spaced at x = y = 5 cm. Particle image velocimetry (PIV) technology was employed to measure the velocity distribution in pool #6. PIV, a transient, multipoint, noncontact fluid dynamics velocimetry method, enables the comprehensive analysis of fluid flow in experimental settings. This system provides detailed measurements of the velocity components (u and v) and associated vorticity and velocity gradients. The calculated hydrodynamic characteristics were scrutinized for accuracy with corrections made to erroneous velocity vectors, thereby establishing a precise flow field.
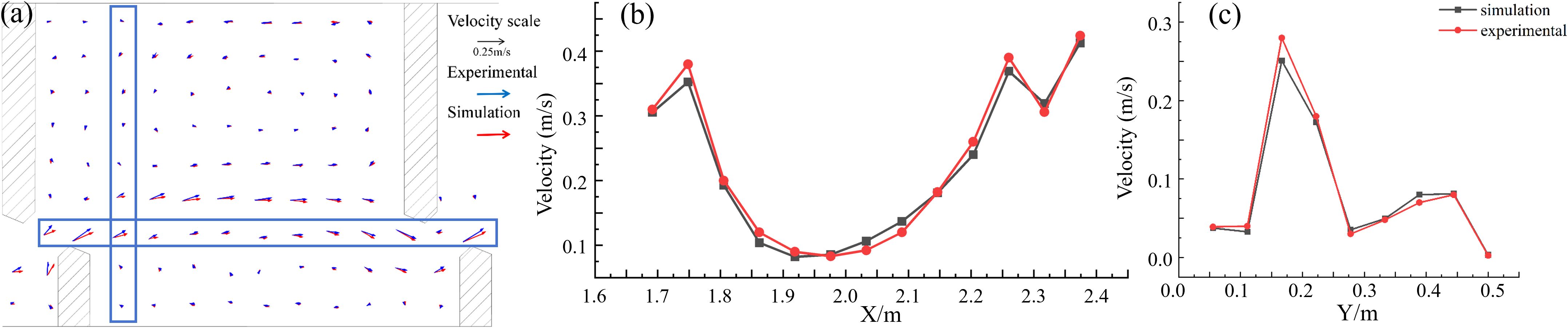
Figure 2. (A) Comparison of flow regime between numerical simulation results and PIV measured results. The comparison between the simulated velocity results of the (B) third row in the x-direction and (C) third column in the y-direction with the PIV measured velocity results.
2.4.2 Numerical simulation
A numerical simulation of the experimental VSF structure was developed, including 11 pools with a 1-m section for both the inflow and outflow. The advanced three-dimensional computational fluid dynamics solver FLUENT was employed to simulate the flow field distribution within the fishway, focusing particularly on the hydraulic distribution across the x plane. The RNG k-ϵ turbulence model was selected for calculating the turbulence continuity, momentum continuity, and RNG k-ϵ equations, as outlined by Rastogi (Rastogi and Rodi, 1978). The constants for the RNG k-ϵ model adhered to the guidelines suggested by Orszag for computational fluid dynamics applications (Yakhot and Orszag, 1986). The computational domain was defined meticulously using a structured grid comprising 2,119,010 cells. The boundary conditions at the fishway inlet were defined using the measured velocity and height, while the outlet information utilized the measured height, as specified in Table 2. Standard wall boundary conditions were uniformly applied across all fishway surfaces and baffles. The model executed over 13,000 time steps at intervals of 0.01 s, ultimately achieving a stable, converged solution.
2.4.3 Comparison of measured and numerical simulation results
A comparative analysis of the numerical simulations and PIV measurements of the water flow field at a depth of 0.5H0 in pool #6 revealed that both flow fields exhibited similar distribution trends (Figure 2A). At the designated measurement points, 13 in the x-direction on the 3rd row (Figure 2B) and 9 in the y-direction on the 3rd column of the 6th chamber (Figure 2C), the velocities recorded by PIV closely approximated those predicted by numerical simulations, both horizontally and vertically. Consequently, this agreement validates the reliability of the numerical VSF used in this study, affirming that further analyses employing a simulated flow field and hydraulic parameters are valid.
2.5 Statistical analysis
2.5.1 Swimming performance analysis
Passage success rate was defined as the percentage of fish that successfully migrated from downstream to upstream in the experimental VSF within 30 min. Passage time refers to the period required for fish to traverse pool #6. Passage route analysis involved the trajectories chosen by the fish in pool #6. To facilitate this, a camera mounted above the pool captured video footage, which was processed at a rate of 24 fps (Figure 1A). These images were subsequently analyzed using Tracker, a complementary video analysis and modeling tool based on the open-source physics (OSP) Java framework. This tool determined the positions of the fish, which were then categorized within 2.5 × 2.5 m quadrats. The frequency of fish occurrence within these quadrats was quantified and visualized as a heat map. This heatmap illustrates the positional frequency of each fish along the passage route. The data from the heatmap were used to plot three distinct passage routes: Route 1 comprised locations with frequency counts between 15 and 30; Route 2 included counts from 7 to 15; and Route 3 encompassed counts from 0 to 7.
The analysis was conducted in pool #6, which was divided into 2 cm grids. Here, the trajectory points were reorganized into grid nodes. For trajectory points sharing the same x value, all corresponding y values were arranged in ascending order. The y-values at the 25%, 50%, and 75% percentiles were then identified. Trajectory lines representing these percentiles were connected to form distributions at frequencies of 25%, 50%, and 75%, denoted as trajectories T25, T50, and T75, respectively. To assess the impact of water dynamics on migratory behavior, these trajectories were superimposed on plots of flow velocity and turbulent kinetic energy (TKE). This overlay provides insight into how variations in water flow and speed influence the migratory paths of fish.
Furthermore, we enhanced our analysis by overlaying the occurrence heatmap on the flow velocity and TKE plots to assess the frequency distribution of these factors along the fish trajectories. This approach facilitated a detailed examination of the influence of TDGS and flow velocity on the distribution of TKE across these pathways. Flow velocity and TKE were specifically chosen as the key hydraulic factors for this study, based on prior research indicating their significant impact on fish passage routes. Notably, a study by Tan et al. (2019) on bighead carp in VSF identified velocity (V), TKE, turbulent dissipation rate (TDR), and strain rate (SR) as the most influential factors affecting passage behaviors.
2.5.2 Data analysis
To analyze the data, a multiple linear regression model was constructed using the “lm” function within the R programming environment. This model was employed to evaluate the significance and contributions of the TDGS levels and flow velocities to various parameters, including passage success rate, passage time, preferred velocity, and preferred TKE.
where h(t) is the dependent variable; xi is the influencing factors; and bi is their influence coefficients corresponding to those factors. This study initially assessed the data distribution and subsequently employed multiple linear regression models utilizing the minimum Akaike information criterion (AIC) to determine the optimal model. Statistical analyses were conducted using SPSS 10.0. Results are reported as mean ± standard deviation (SD). Differences between means were evaluated using one-way analysis of variance (ANOVA), followed by Tukey’s post hoc test for multiple comparisons. A significance threshold of P < 0.05 was established for all statistical tests.
3 Results
3.1 Fish passage success rate
The analysis revealed a negative correlation between TDGS levels and passage success rates across different flow velocities. Specifically, as the TDGS levels increased, the passage success rate decreased at flow velocities of 0.2, 0.25, and 0.3 m/s. As the flow velocity increased, the upstream passage success decreased across various TDGS levels (Figure 3). For the control group, the baseline passage success was 61%. However, at TDGS levels of 110%, 120%, and 130%, passage success rates dropped to 48%, 37%, and 37% respectively at 0.2 m/s. At a flow velocity of 0.25 m/s, passage success for the control group was 53%, which decreased to 38%, 27%, and 23% at TDGS levels of 110%, 120%, and 130%, respectively. Similarly, at 0.3 m/s, passage success decreased from 47% in the control group to 37%, 27%, and 20% at the same TDGS levels. The analysis showed that TDGS levels alone significantly influenced passage success rates, while flow velocity did not. However, when both the flow velocities and TDGS levels were considered together, the combined factors significantly influenced the passage success rate, as detailed in Table 3.
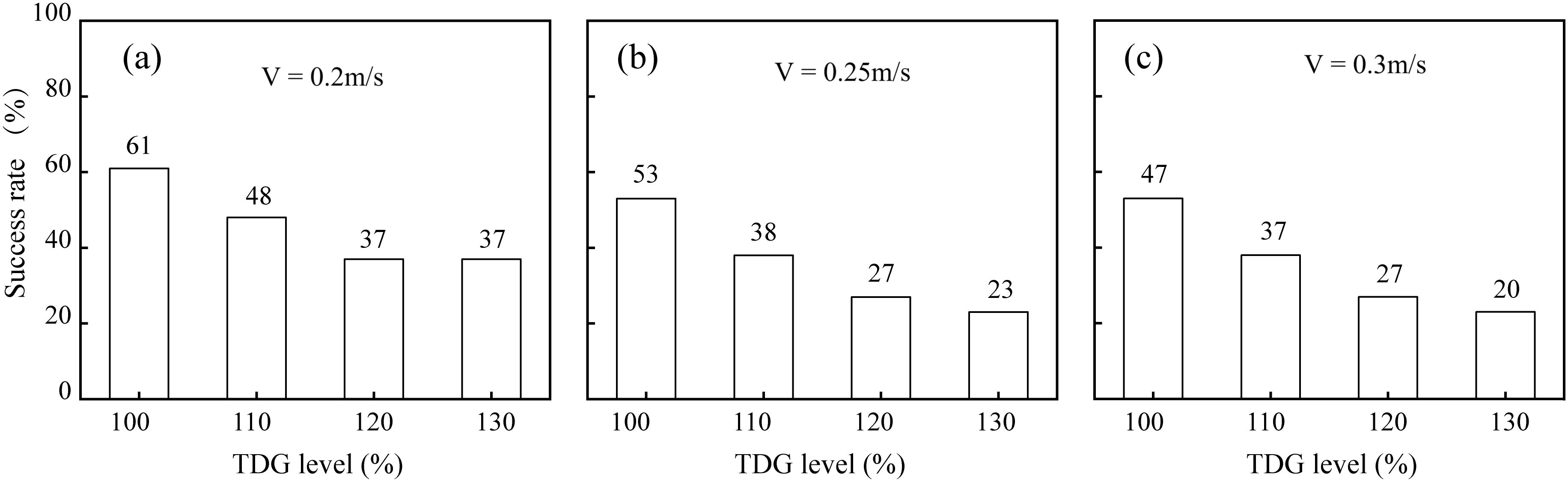
Figure 3. Passage success rate for juvenile bighead carp tested at various TDGS levels under (A) 0.2, (B) 0.25, and (C) 0.3 m/s.
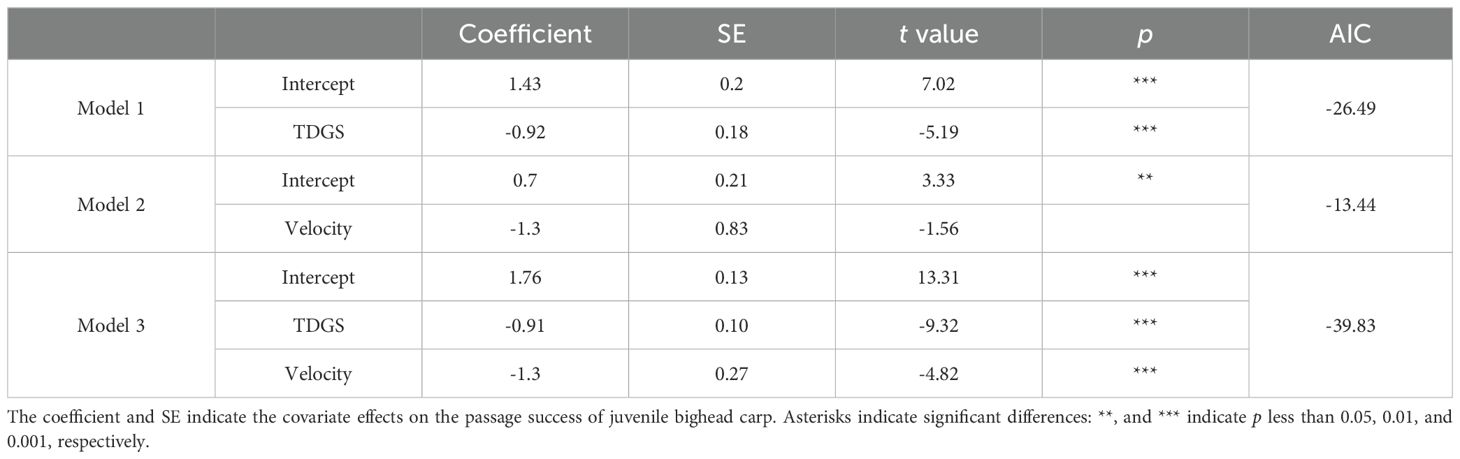
Table 3. Comparison of linear models of passage success rate for juvenile bighead carp tested under different TDGS levels and flow velocities.
Analysis of the passage success rate was supported by four selected models, as detailed in Table 3. Among these, Model 3, which incorporates both TDGS levels and flow velocities, offers the best fit to the data, evidenced by its AIC of -39.83. The relationship between the passage success rate, TDGS level, and flow velocity was quantitatively assessed using a multiple linear regression model:
3.2 Passage time in the central pool (#6) of the fishway
The study found that as TDGS levels increased, passage time also increased, and individual passage times showed greater dispersion across flow velocities of 0.2, 0.25, and 0.3 m/s (Figure 4). Specifically, at a flow velocity of 0.2 m/s, the average passage times were 7.2, 12.4, 25.3, and 38.4 s. At 0.25 m/s, the average time increased to 9.2, 13.3, 18.0, and 36.3 s. Further, at 0.3 m/s, the times were longer at 11.4, 18.6, 31.0, and 56.6 s. Analysis of the impact of TDGS levels alone revealed a significant increase over time. In contrast, the flow velocity alone did not significantly affect the passage time. However, when considering the combined effects of both the TDGS levels and flow velocities, the analysis indicated that these factors significantly influenced the passage time, as detailed in Table 4.
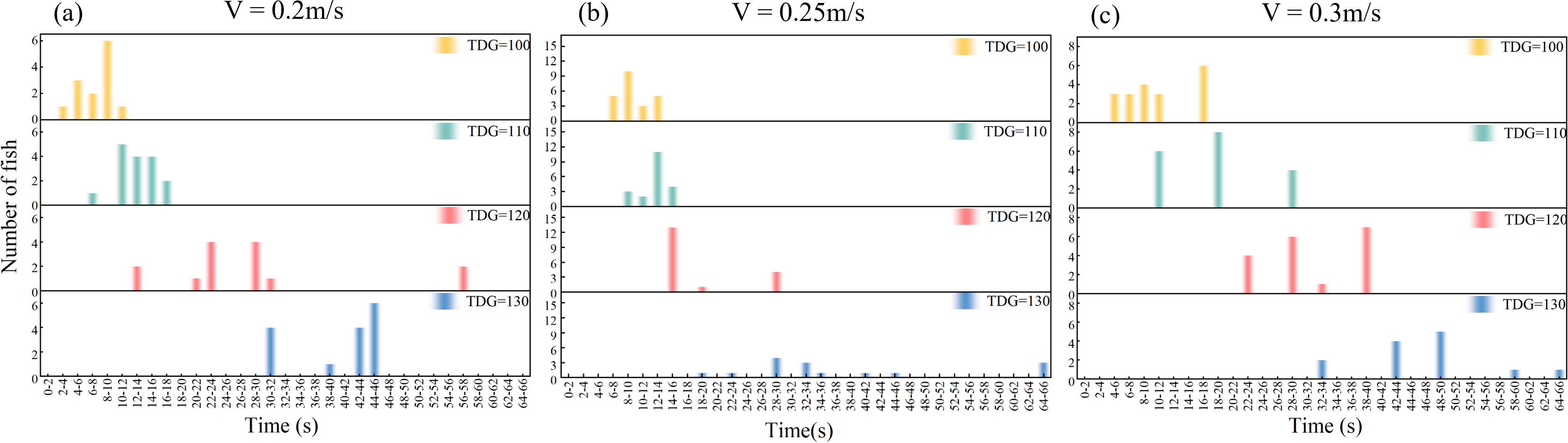
Figure 4. Distribution of the passage times of successful migration fish at various TDGS levels under (A) 0.20, (B) 0.25, and (C) 0.3 m/s.
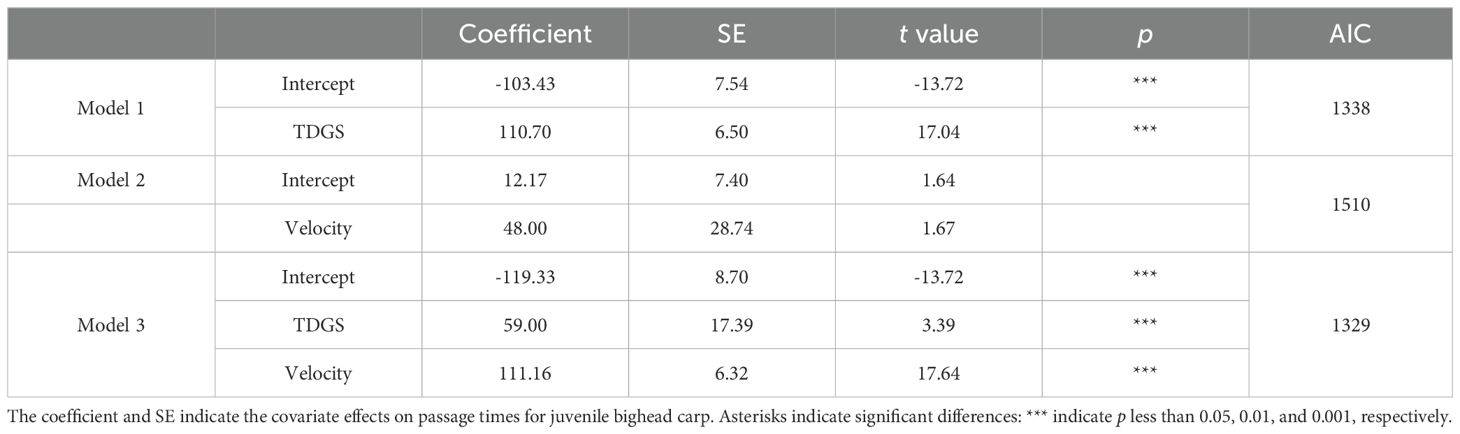
Table 4. Comparison of linear models of passage times for juvenile bighead carp experiencing different TDGS levels and flow velocities.
Three models were selected to analyze the factors influencing passage time, as shown in Table 4. Among them, Model 3, which incorporated both the TDGS levels and flow velocities, demonstrated the best fit with the data, with an AIC of 1329. The relationships between passage time, TDGS level, and flow velocity were quantitatively assessed using a multiple linear regression model.
3.3 Analysis of the fish swimming trajectory
In the mainstream area, flow velocities were observed to range from 0.16 to 0.40 m/s, showing a decrease from the center towards the sides and from upstream to downstream. A prominent counter-clockwise circulation area was observed on the left, influencing fish trajectories, whereas a smaller clockwise circulation area developed on the right, as illustrated in Figures 5A–C, respectively. With an increase in the flow velocity, the velocities in the main stream area intensified and the circulation areas on both the left and right sides became more pronounced. The highest velocities were recorded in the mainstream, followed by the left and right pool walls. Additionally, as the velocity increased, the extent of the high-velocity zones expanded, as depicted in Figures 5D–F. Turbulence was predominantly observed downstream of the short baffle at a flow rate of 0.2 m/s. At higher flow velocities of 0.25 and 0.3 m/s, significant turbulence appeared near the downstream short baffle within the right small circulation area, as shown in Figures 5G–I.
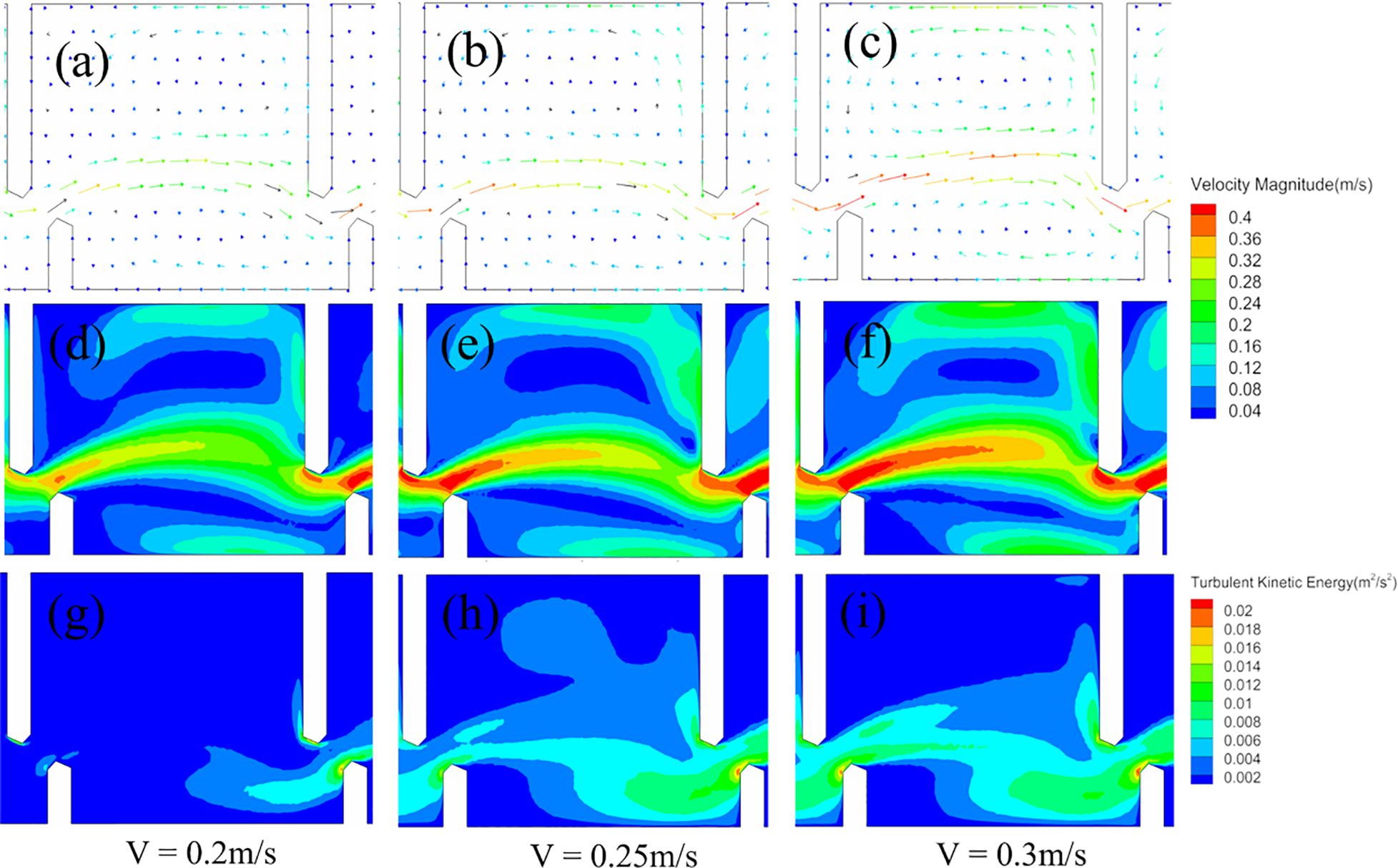
Figure 5. (A–C) Flow field vector distribution, (D–F) velocity distribution, and (G–I) turbulence distribution of three different flow velocities in the middle pool (#6). Profiles are shown at a single pool depth of 0.5 H0 (where H0 is the total water depth).
Heat maps illustrating the frequency of bighead carp occurrence revealed a distinct preference for pool walls adjacent to short baffles (Figure 6). As the TDGS levels increased, the dispersion of fish trajectories became more obvious at flow velocities of 0.2 and 0.25 m/s. However, at a higher flow velocity (0.3 m/s), the degree of trajectory dispersion exhibited no significant changes across different TDGS levels.
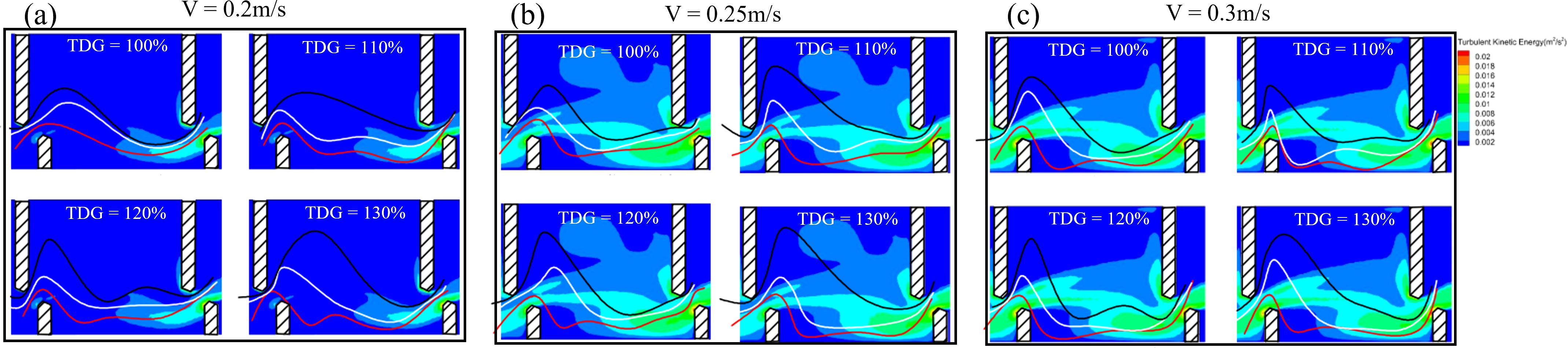
Figure 6. Heatmaps of the frequency of fish occurrence and typical passage routes with respect to various TDGS levels (100%, 110%, 120%, and 130%) for bighead carp passing through the vertical slot fishway, with quadrat dimensions of 2.5 × 2.5 cm. The black curve represents Route 1, with the frequency of bighead carp occurrence ranging from 15 to 30; red curve represents Route 2, where the frequency of bighead carp occurrence ranges from 7 to 15; and white curve represents Route 3, with the frequency of bighead carp occurrence of 0–7. The legend bar of the frequency of fish occurrence ranges from 0 to 30. The color grassy green represents that there are 0–2 instances of fish passage and red denotes that there are more than 30 instances of fish passage. The color legend indicates the frequency of fish passage, with darker colors representing higher frequencies of fish presence.
As detailed in Table 5, although fish swimming trajectories are random in nature, routes 1, 2, and 3 did not align strictly with decreasing frequency. Typically, Route 1 is indicative of the path with the highest probability of occurrence. Notably, the probability associated with route 1 increased as the flow velocity increased, and decreased with higher TDGS levels.
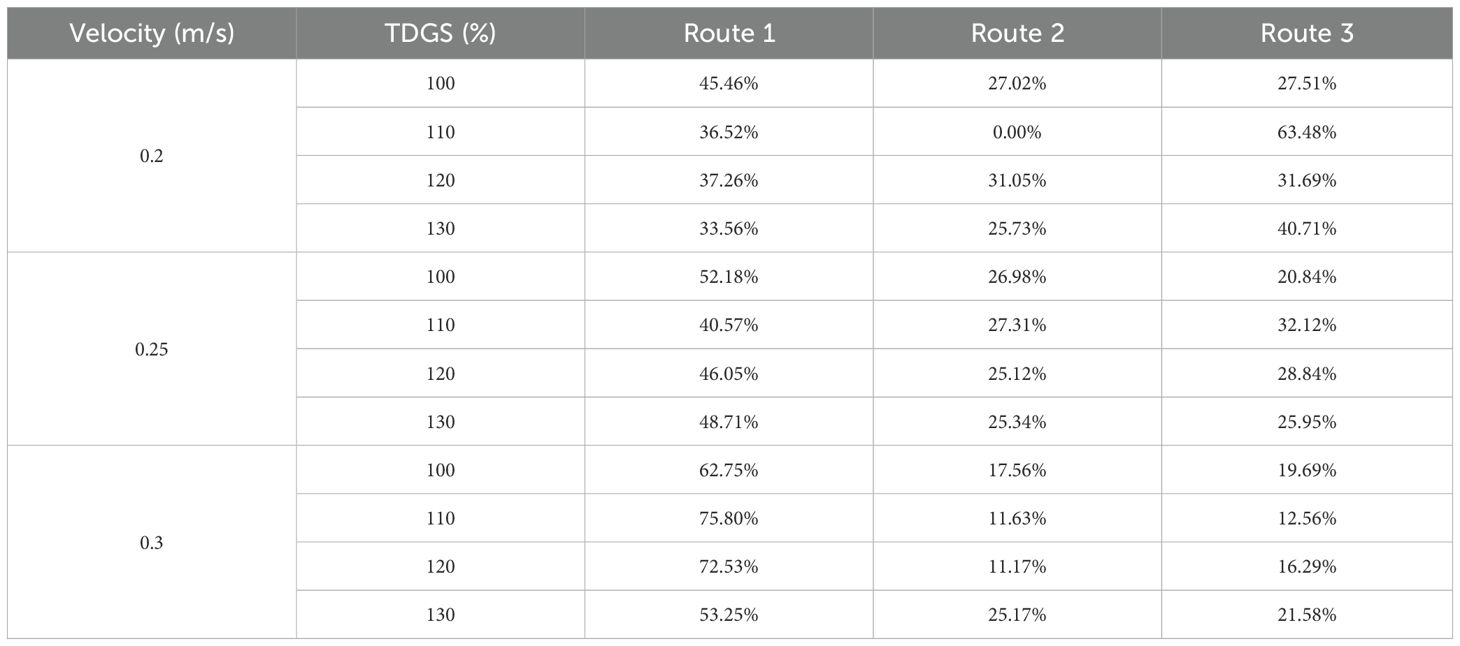
Table 5. Proportions of three main passage routes at various TDGS levels (100, 110, 120, and 130%) at 0.2, 0.25, and 0.3 m/s.
3.4 Analysis of the preference for hydrodynamic factors
3.4.1 Preference for flow velocity under different conditions
The fish’s 75%, 50%, and 25% probability characteristic curves were superimposed onto the flow field diagram, as shown in Figure 7. These curves approximate the proximity to the long baffle, near the mainstream, and close to the short baffle. At flow velocities of 0.2 and 0.25 m/s, the 75% probability characteristic curve indicated that fish tended to swim closer to the long baffle and pool wall as TDGS levels increased. This resulted in the initial trajectory of fish occurring frequently in areas with lower flow velocity. The 50% probability characteristic curve showed no significant changes with varying TDGS levels. Conversely, the 25% probability curve positioned the fish trajectory closer to the short baffle and pool wall, thereby frequently exposing them to higher flow velocity zones, as depicted in Figures 7A, B. At a flow velocity of 0.3 m/s, the fish trajectories did not exhibit a clear trend across the different TDGS levels, as illustrated in Figure 7C.
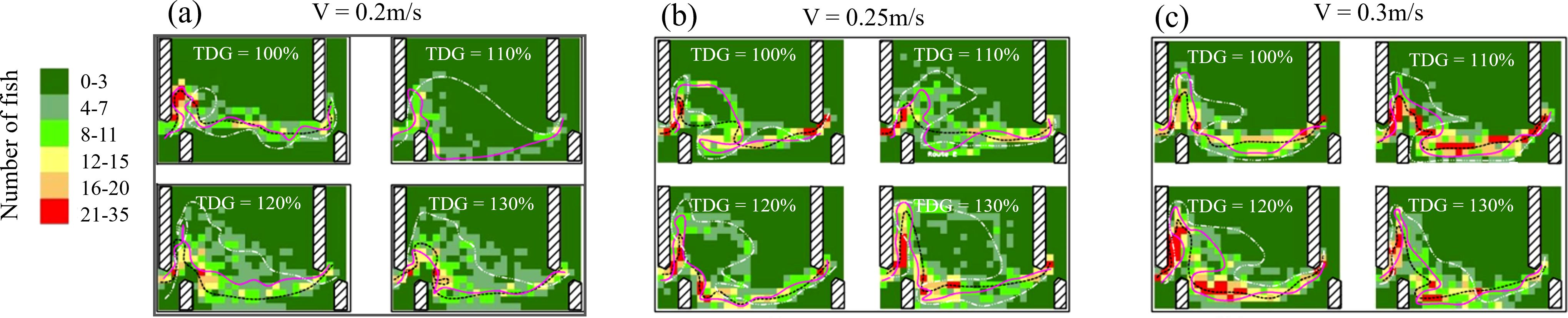
Figure 7. 75%, 50%, and 25% probability typical passage routes superimposed onto the flow field diagram for the conditions at 0.2, 0.25, and 0.3 m/s. The black curve represents the 75% probability passage route, white curve represents the 50% probability passage route, and red curve represents the 25% probability passage route.
This study revealed distinct preferences for fish flow velocities under different TDGS conditions, as illustrated in Figure 8. At a flow velocity of 0.2 m/s, fish showed the highest preference for the slowest flow velocity range of 0–0.05 m/s, with preference values of 26.53%, 29.92%, 39.40%, and 32.95% for TDGS levels of 100%, 110%, 120%, and 130%, respectively (Figure 8A). When flow velocities increased to 0.25 m/s, the highest frequency preference shifted to the 0.05–0.1 m/s range, displaying frequencies of 40.05%, 43.26%, 54.02%, and 51.96% across the same respective TDGS levels (Figure 8B). At a flow velocity of 0.3 m/s, the preferred flow velocity remained at 0.05–0.1 m/s, with frequencies of 34.85%, 39.14%, 42.13%, and 38.54% for TDGS levels from 100% to 130% (Figure 8C). The preference for low-velocity ranges under different flow velocity conditions increased with the TDGS level, indicating that fish exposed to TDGS stress exhibited a stronger preference for low-velocity environments. Furthermore, according to the analysis presented in Table 6, the TDGS level was identified as the only significant factor influencing flow velocity preferences.
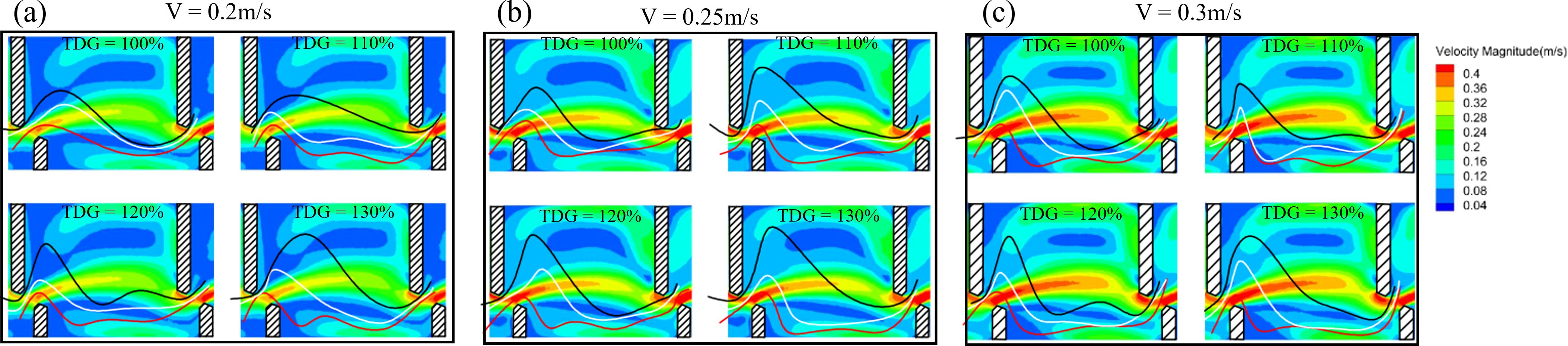
Figure 8. Distribution frequency of flow velocity values at the fish’s occurrence locations in the middle pool of the fishway. The locations and frequencies of fish occurrences were based on the passage routes experienced by the fish at various TDGS levels under conditions of (A) 0.2, (B) 0.25, and (C) 0.3 m/s.
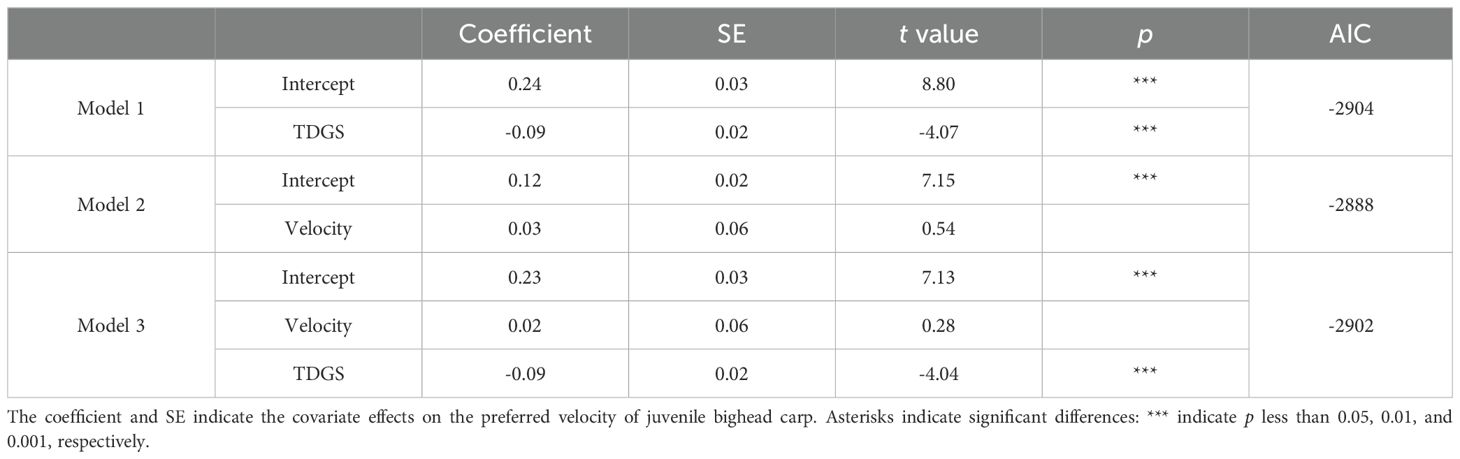
Table 6. Comparison of linear models of preferred velocity for juvenile bighead carp experiencing different TDGS levels and flow velocities.
Three models were selected to analyze the preferred velocity of the fish, as detailed in Table 6. Model 1, which included only the TDGS level as a variable, demonstrated the best fit to the data, with an AIC of -2904. The relationship between the preferred velocity and TDGS level was quantitatively assessed using a multiple linear regression model:
3.4.2 Preference for TKE under different conditions
The 75%, 50%, and 25% probability characteristic curves of the fish were superimposed onto the TKE distribution map (Figure 9). At a flow velocity of 0.2 m/s, high turbulence was observed exclusively in the region between the long and short baffles downstream. As flow velocities increased to 0.25 and 0.3 m/s, areas of high turbulence predominantly appeared near the short baffle and along the pool walls. Notably, the 50% and 75% probability characteristic routes of the fish primarily traversed these areas of elevated turbulence.
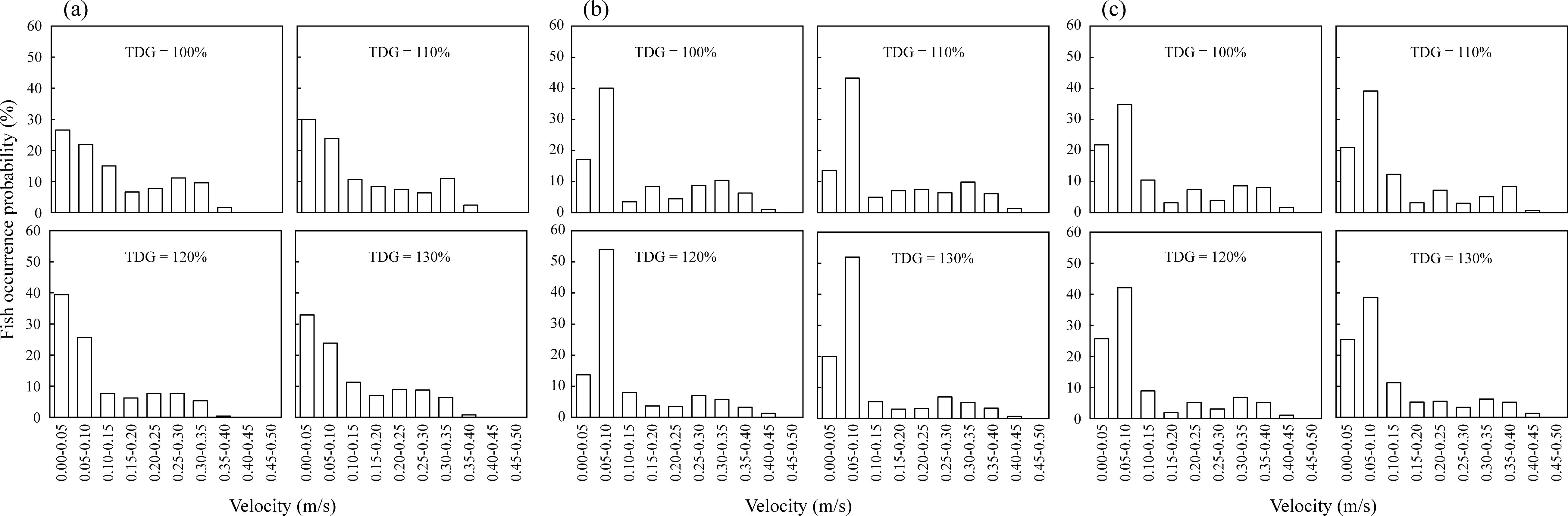
Figure 9. 75%, 50%, and 25% probability typical passage routes were projected onto the TKE distribution maps for the conditions at 0.2, 0.25, and 0.3 m/s. The black curve represents the 75% probability passage route, white curve represents the 50% probability passage route, and red curve represents the 25% probability passage route.
This study details the preferences of fish for specific TKE values under varying conditions. At a flow velocity of 0.2 m/s, the fish exhibited the highest frequency preference for TKE values ranging from 0 to 0.002, with occurrence frequencies of 45.86%, 53.93%, 57.60%, and 57.52% at TDGS levels of 100%, 110%, 120%, and 130%, respectively (Figure 10A). As the flow velocity increased to 0.25 m/s, the preferred TKE range shifted to 0.006–0.008 with frequencies of 37.01%, 37.14%, 24.65%, and 25.59%, corresponding to the same TDGS levels (Figure 10B). At a higher flow velocity of 0.3 m/s, this preference for TKE values of 0.006–0.008 persisted, with frequencies of 34.05%, 34.33%, 33.61%, and 29.97% at TDGS levels of 100%, 110%, 120%, and 130% (Figure 10C). Compared with the preferred flow velocities, the preference for specific ranges of the TKE did not show a clear trend of increased selection for lower turbulence levels as the TDGS concentration increased. The analysis confirmed that both TDGS levels and flow velocities significantly influenced the preferred TKE, as shown in Table 7.
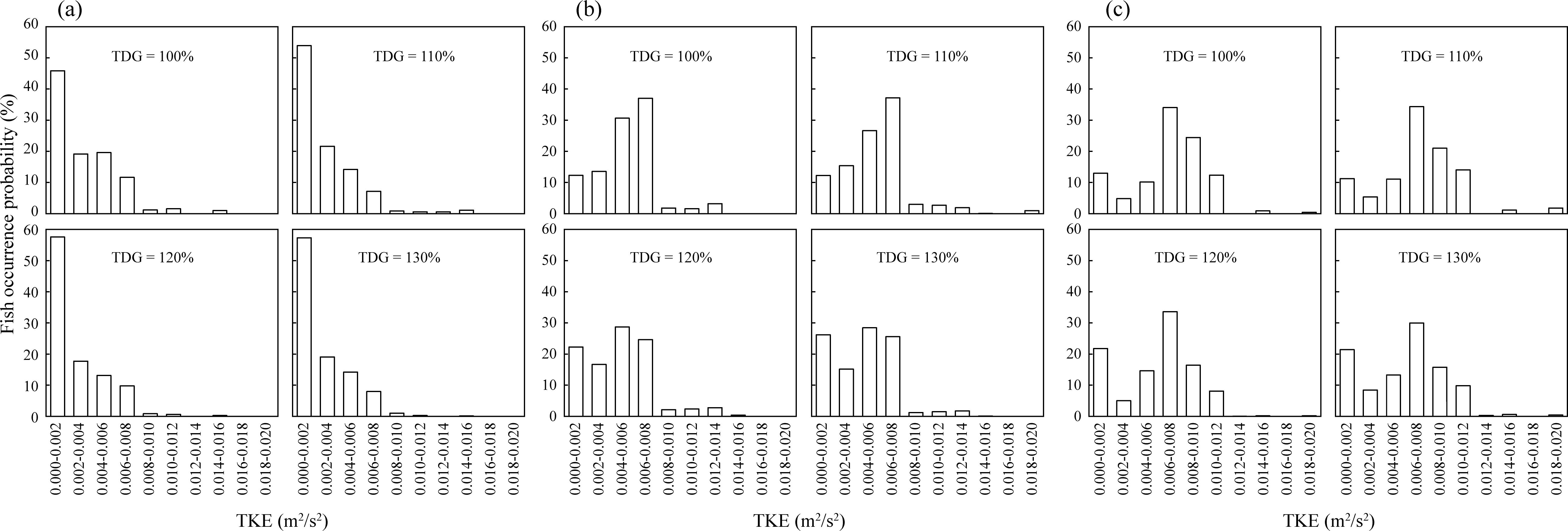
Figure 10. Distribution frequency of TKE values at the fish’s occurrence locations in the middle pool of the fishway was analyzed. The locations and frequencies of fish occurrences were based on the passage routes experienced by the fish at various TDGS levels under conditions of (A) 0.2 m/s, (B) 0.25 m/s, and (C) 0.3 m/s.
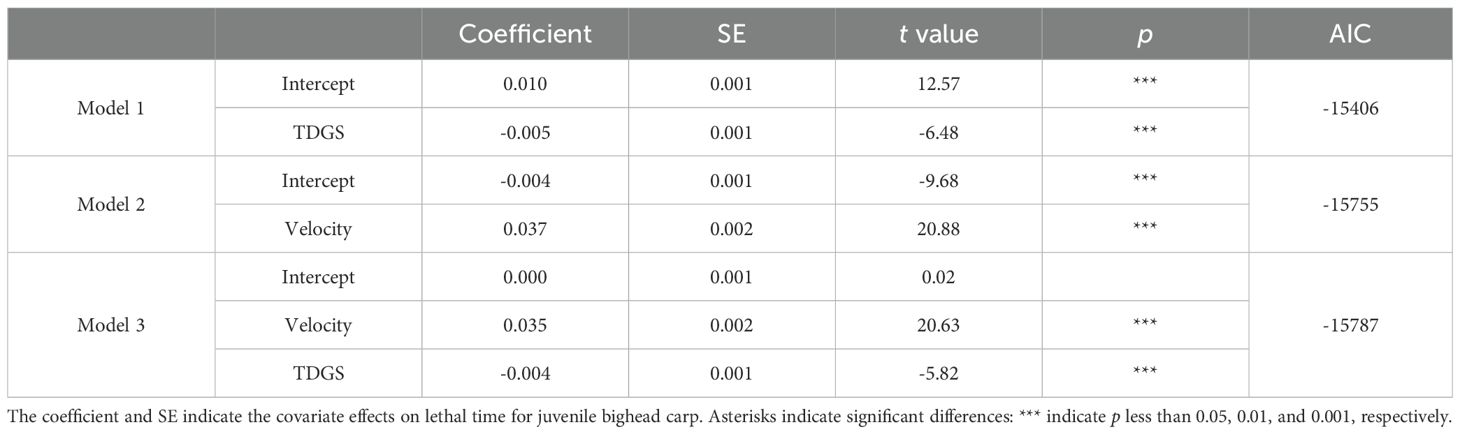
Table 7. Comparison of linear models of preferred TKE for juvenile bighead carp experiencing different TDGS levels and flow velocities.
The analysis of the preferred TKE was supported by three models, as detailed in Table 7. Among these, Model 3, which incorporated both TDGS levels and flow velocities, demonstrated the most robust fit to the data, with an AIC score of -15787. The relationship between the preferred TKE, TDGS level, and flow velocity was rigorously assessed using the following multiple linear regression model:
4 Discussion
4.1 Effect of TDGS on fish passage efficiency
This study demonstrated that exposure to elevated TDGS levels significantly prolonged the passage time of bighead carp, predominantly due to a decline in their swimming ability. Consistent with findings from previous studies, TDGS has been shown to impair critical and burst swimming capabilities in various species (Li et al., 2019; Wang et al., 2017; Yuan et al., 2023; Zhang et al., 2023). Adverse effects of TDGS include damage to fish gills and the formation of tiny air bubbles in the bloodstream, which impair the respiratory and circulatory systems (Chen et al., 2023; Pleizier et al., 2023). Such physiological effects hinder energy supply and metabolic rates, leading to reduced swimming ability and increased passage time.
We attributed the significant increase in passage time to a decline in both critical and burst swimming abilities. Specifically, because bighead carp rely predominantly on critical swimming speeds to navigate fishway pools powered by aerobic respiration, any decrease in aerobic metabolism significantly extends the time required to complete the passage. Burst swimming speed is an anaerobic movement mode that fish use when passing through vertical slots with a high flow velocity. TDGS stress may weaken the fish’s burst swimming ability, leading to repeated attempts at the vertical slot in the fishway, thus increasing passage time.
Exposure to elevated TDGS levels may also induce muscle oxidative stress in bighead carp (Liu et al., 2015), thereby diverting considerable energy to counteract this stress. This diversion may impair the capacity of the muscles to rapidly generate energy to overcome flow barriers. After exposure to TDGS stress, metabolic processes were weakened and muscle tension increased, ultimately leading to a decrease in fish passage success and prolonged passage time.
The relationship between the fishway flow regions and fish passage efficiency is complex. Although higher flow velocities within a certain range may attract more fish, suggesting a potential increase in passage success, excessively high velocities can reduce the number of successful passages (Noonan et al., 2012; White and Mefford, 2002). An increase in fishway flow velocity generally results in greater energy expenditure by fish, which could extend the passage time. The results of this study revealed that when considering only the fishway flow velocity, its variations did not significantly affect passage success or time. This observation implies that the swimming ability of bighead carp was sufficient to overcome the challenges posed by the range of flow velocities tested in this study. However, after being exposed to TDGS and responding to the flow field in the fishway, the passage success rate and passage time showed significant variations compared to the unstressed free condition.
4.2 Effect of TDGS on the specific selection of fish swimming trajectory
At a flow velocity of 0.2 m/s, juvenile bighead carp not exposed to TDGS swam against the main current. However, as the flow velocities increased to 0.25 m/s and 0.3 m/s, bighead carp tended to avoid flow field with high flow speed, preferring to select the area near the mainstream edge (Figure 7). Conversely, at a flow velocity of 0.2 m/s, there was carp tended to move along the long baffle to avoid a high flow speed (Figure 7). At higher flow velocities and TDGS levels, carp exhibited similar movement patterns. The routes chosen by fish are affected by a combination of biological traits and environmental factors (Rahel and McLaughlin, 2018; Tan et al., 2019). In bighead carp, TDGS significantly influences route selection by potentially damaging organs critical for swimming performance and sensory functions. The key organs affected include the gills, muscles, and heart, all of which undergo functional decline under TDGS.
As the primary respiratory organs of fish, the gills are crucial for maintaining vitality and stamina. In TDGS environments, microbubbles have been observed in the gills of many species, leading to significant physiological stress (Chen et al., 2023). TDGS disrupts the antioxidant balance within the gills, causing cellular damage, inflammation, and pathological changes, such as cell proliferation (Chen et al., 2023). Additionally, alterations in gene expression and metabolism in the gills compromise the integrity of cell membranes and disrupt energy metabolism (Chen et al., 2023). These changes can significantly affect respiratory capacity and frequency, thereby influencing the response of fish to flow fields and, ultimately, their route selection within fishways. As the primary driver of locomotion, the muscle state directly affects a fish’s swimming speed and endurance. Exposure to TDGS can increase oxidative stress within muscle tissues (Liu et al., 2015), which may divert energy from swimming to mitigate this stress. This diversion can alter fish trajectory and speed in flow currents, thereby influencing their ability to navigate fishways effectively. The heart is essential for blood circulation and energy supply throughout the fish body. Healthy hearts enable fish to sustain high-intensity activities and choose challenging routes through the fishways. However, TDGS exposure can lead to the formation of gas bubbles in the bloodstream, impairing heart function, and interfering with fish’s free selection of trajectories (Pleizier et al., 2021).
Flow information plays a crucial role in guiding fish through aquatic environments (White and Mefford, 2002). However, TDGS can significantly impair the sensory organs of fish such as the eyes, lateral line, and skin, thereby diminishing their ability to perceive the direction of water flow (Pleizier et al., 2020). This sensory impairment affects fish navigation and their ability to choose optimal routes through fishways. We observed varying degrees of pop-eye symptoms in some bighead carps, indicative of visual impairment. Such damage likely hinders their ability to discern and navigate complex current patterns, thereby affecting route selection. The fish lateral line system is a specialized sensory organ capable of detecting subtle vibrations and flow directions in water (McHenry and Liao, 2014). This system is vital for navigation, particularly in conditions where vision is compromised or other sensory systems are impaired. However, TDGS exposure may cause blockages in the lateral line systems, depending on the size of the lateral pores in different species (McGrath et al., 2006). In bighead carp, such blockages can reduce their ability to sense changes in water flow, further increasing their navigational challenges. Fish skin hosts various sensory cells that sense tactile and pressure changes, thereby influencing navigational decisions in complex currents (Horodysky et al., 2022). We observed that the yellow epidermis of bighead carp darkened under TDGS. Possible mechanisms include TDGS-induced stress hormone production, such as cortisol, which may alter pigment cell distribution and pigment synthesis, as suggested by Sugimoto (Sugimoto, 2002). Alternatively, TDGS could disrupt normal metabolic processes in bighead carp, affecting enzyme activity and hormone levels associated with pigment production. These changes imply that skin functionality may be compromised, potentially diminishing the sensitivity to hydraulic stimuli.
In summary, compared with experimental fish not exposed to TDGS, those exposed to TDGS exhibited varying degrees of impairment in their gill and muscle conditions, cardiopulmonary function, vision, lateral line system, and metabolic processes. These physiological factors can significantly affect fish swimming performance and the perception of environmental information, ultimately constraining their ability to volitionally select their swimming trajectories.
4.3 Effect of TDGS on hydrodynamic factor preferences
The flow velocity and TKE are crucial hydraulic parameters that influence the movement trajectory of bighead carp within fishway flow fields (Tan et al., 2019). Our findings indicate that the preference of bighead carp for flow velocities is primarily affected by TDGS levels rather than by variations in fishway flow velocities. These results indicated that as the TDGS level increased, fish exhibited a stronger preference for lower flow velocity ranges. However, carp preference for TKE was significantly modulated by both TDGS levels and flow velocity. The preference for specific ranges of TKE did not show a clear trend of increased selection for lower turbulence levels as the TDGS level increased. Within the fishway, fish movement trajectories typically avoided high-speed zones, opting for paths through lower velocities and turbulence to ascend the pool. Fish exposed to TDGS tended to choose paths with lower flow velocities and turbulence, highlighting potential adverse effects of TDGS on swimming performance. Notably, the preference for TKE increased with higher flow velocities, which were in the range of 0 to 0.02 m2/s2, below the 0.02 to 0.043 m2/s2 range observed in a study on bighead carp in VSFs (Tan et al., 2019). This variance could be attributed to the smaller body length of the bighead carp used in this study (8 cm) compared to the 11 cm fish used in Tan et al. (2019). We hypothesized that the TDGS level could be a key factor altering bighead carp preferences for specific hydraulic characteristics, such as flow velocity and TKE, consequently affecting their path selection within complex flow environments.
An increased preference for the TKE among bighead carp with increasing fishway flow velocity. If fish possess mechanisms capable of adapting to specific hydrodynamic conditions, they are generally attracted to turbulence (Calluaud et al., 2014). This attraction could be attributed to the ability of fish to use turbulent energy to minimize their energy expenditure under certain conditions. For instance, Standen et al. (2004) observed that the swimming speed of sockeye salmon (Oncorhynchus nerka) in turbulent flow within a flume varied from 1.4 to 76 times greater than in steady flow. However, greater turbulent energy may compromise the swimming ability of the fish by affecting their swimming posture. The necessity for fish to frequently adjust their posture, such as utilizing pectoral fins to swing their bodies and maintaining balance in highly turbulent conditions, leads to an increase in total energy expenditure due to the additional energy required to manage the intensified turbulence (Enders et al., 2005).
4.4 Application and future studies
The Yangtze River Basin, which has abundant water resources and geographical advantages, has developed into a crucial hydropower energy base through the construction of numerous high dams (Penghao et al., 2019). However, this development has exposed the river’s aquatic ecosystem to significant environmental challenges, including high TDGS levels and habitat fragmentation. Designed to enhance habitat connectivity, the efficiency of fish-passage facilities has often fallen below expectations. Specifically, the effectiveness of upstream fish passages was recorded at 41.7%, significantly lower than the 68.5% efficiency of downstream passages. Furthermore, non-salmonid fish demonstrate a notably lower success rate in these upstream facilities (21.1%) compared to salmonids (61.7%) (Noonan et al., 2012). The effectiveness of these fish passages is highly dependent on the swimming performance of the species, which is influenced by their inherent traits and environmental conditions, such as temperature, sediment load, and TDGS (Ji et al., 2022; Li et al., 2019). The potential adverse impacts of TDGS on non-salmonid fish in the upper reaches of the Yangtze River Basin raise concerns about the reduced effectiveness of these ecological corridors.
The emphasis on fish passage facilities has evolved from prioritizing the sustainable yield of commercial species to adopting biodiversity conservation approaches that focus on the swimming abilities and behaviors of entire fish communities (Oldani et al., 2007). In scenarios where multiple species utilize a single passage, the water velocity must be maintained within the swimming performance range of all species involved. In the Yangtze River Basin, fish passages primarily target four domestic Chinese carp species. Habitats of species such as silver carp and bighead carp are typically located in the upper water layers. Because of the compensatory water depth effect of supersaturation, fish residing in surface waters face a heightened risk of adverse effects from TDGS compared with those in deeper waters. Additionally, given that bighead carp exhibit lower activity levels than silver carp, this study specifically examined the effect of TDGS on the passage performance of bighead carp.
The efficacy of fish passages depends crucially on the relationship between the upstream swimming behavior of fish and the hydraulics within the passages (Calluaud et al., 2014). Furthermore, factors such as trajectory selection and flow velocity preferences within passages are critical determinants of passage effectiveness. This study employed several evaluation criteria, including passage success, passage time, preferred routes, and preferred hydraulic factor to develop a methodology for assessing the impact of TDGS. This study explored how adjustments to fish passage flow can mitigate reduced passage success attributed to TDGS. The findings indicated that high TDGS levels were associated with decreased passage success, extended passage times, altered swimming routes, and shifted hydraulic preferences. Additionally, TDGS exposure had both direct and indirect lethal effects on fish, contributing to mortality (Table 1). Fish exposed to TDGS also exhibited increased swimming fatigue, characterized by depleted energy reserves, elevated lactate accumulation, and prolonged recovery periods after continuous anaerobic swimming during upstream migration. This fatigue can delay passage times and potentially increase mortality rates during migration. Consequently, implementing ecological scheduling to control TDGS levels during flood periods is crucial. Moreover, adopting comprehensive strategies such as adjusting fish passage flows in conjunction with managing TDGS levels may enhance the overall effectiveness of these ecological corridors.
This study’s findings were based on experimental fish passage results and thus may not fully reflect the conditions in riverine fish passage facilities. Given these limitations, future research should be conducted during flood periods to investigate additional mitigation measures. Such studies should aim to alleviate the adverse effects of TDGS exposure on the effectiveness of fish passages, thereby enhancing our understanding of and improving management strategies for aquatic ecosystems in natural river settings.
Data availability statement
The original contributions presented in the study are included in the article/supplementary material. Further inquiries can be directed to the corresponding author/s.
Ethics statement
Animal experiments were approved by the Animal Experiment Ethics Committee of Sichuan University. All experimental procedures were performed in accordance with the Experimental Animal Management Regulations endorsed by the State Council of the People’s Republic of China. The study was conducted in accordance with the local legislation and institutional requirements.
Author contributions
ML: Conceptualization, Data curation, Formal analysis, Funding acquisition, Investigation, Methodology, Writing – review & editing. YO: Project administration, Resources, Software, Writing – original draft. JL: Software, Supervision, Validation, Writing – original draft. HS: Investigation, Methodology, Project administration, Writing – original draft. BZ: Investigation, Methodology, Project administration, Writing – original draft. XL: Conceptualization, Data curation, Software, Writing – original draft. QY: Conceptualization, Data curation, Funding acquisition, Investigation, Writing – original draft, Writing – review & editing. XY: Formal analysis, Funding acquisition, Investigation, Writing – original draft.
Funding
The author(s) declare that financial support was received for the research and/or publication of this article. This work was supported by the National Natural Science Foundation of China (grant number: 52209100), Open Fund Research at the Engineering Research Center of Eco-environment in Three Gorges Reservoir Region, Ministry of Education, China (grant number: KF2023-14), and the Sichuan Science and Technology Program (2024NSFSC0855).
Acknowledgments
Thanks to all the authors of this paper for their efforts in experimental design, conducting experiments, and data analysis. We also thank Xihua University for providing the experimental platform.
Conflict of interest
The authors declare that the research was conducted in the absence of any commercial or financial relationships that could be construed as a potential conflict of interest.
Generative AI statement
The author(s) declare that no Generative AI was used in the creation of this manuscript.
Publisher’s note
All claims expressed in this article are solely those of the authors and do not necessarily represent those of their affiliated organizations, or those of the publisher, the editors and the reviewers. Any product that may be evaluated in this article, or claim that may be made by its manufacturer, is not guaranteed or endorsed by the publisher.
References
Bayse S. M., McCormick S. D., Castro-Santos T. (2019). How lipid content and temperature affect American shad (Alosa sapidissima) attempt rate and sprint swimming: implications for overcoming migration barriers. Can. J. fisheries Aquat. Sci. 76, 2235–2244. doi: 10.1139/cjfas-2018-0406
Calluaud D., Cornu V., Baran P., David L. (2014). “Relationship between fish behaviour, turbulence and unsteady flow in experimental vertical slot fishways.” in 10th International Symposium on Ecohydraulics. 1–4.
Castro-Santos T., Sanz-Ronda F. J., Ruiz-Legazpi J. (2013). Breaking the speed limit—comparative sprinting performance of brook trout (Salvelinus fontinalis) and brown trout (Salmo trutta). Can. J. Fisheries Aquat. Sci. 70, 280–293. doi: 10.1139/cjfas-2012-0186
Chen Y., Wu X., Lai J., Yan B., Gong Q. (2023). Molecular mechanisms of physiological change under acute total dissolved gas supersaturation stress in yellow catfish (Pelteobagrus fulvidraco). Environ. Sci. pollut. Res. 30, 97911–97924. doi: 10.1007/s11356-023-29157-6
Cheng F., Li W., Castello L., Murphy B. R., Xie S. (2015). Potential effects of dam cascade on fish: lessons from the Yangtze River. Rev. Fish Biol. Fisheries 25, 569–585. doi: 10.1007/s11160-015-9395-9
Duan X., Liu S., Huang M., Qiu S., Li Z., Wang K., et al. (2010). Changes in abundance of larvae of the four domestic Chinese carps in the middle reach of the Yangtze River, China, before and after closing of the Three Gorges Dam. Environmental Biol. Fishes 86 (1), 13.
Elings J., Bruneel S., Pauwels I. S., Schneider M., Kopecki I., Coeck J., et al. (2024). Finding navigation cues near fishways. Biol. Rev. 99, 313–327. doi: 10.1111/brv.13023
Enders E. C., Boisclair D., Roy A. G. (2005). A model of total swimming costs in turbulent flow for juvenile Atlantic salmon (Salmo salar). Can. J. Fisheries Aquat. Sci. 62, 1079–1089. doi: 10.1139/f05-007
Fickeisen D., Montgomery J. (1978). Tolerances of fishes to dissolved gas supersaturation in deep tank bioassays. Trans. Am. Fisheries Soc. 107, 376–381. doi: 10.1577/1548-8659(1978)107<376:TOFTDG>2.0.CO;2
Horodysky A. Z., Schweitzer C. C., Brill R. W. (2022). “Applied sensory physiology and behavior”. In Fish physiology. (Academic Press) 39, 33–90.
Ji Q., Li K., Wang Y., Liang R., Feng J., Li R., et al. (2022). Swimming performance of a pelagic species in the Yangtze River under different exposure modes of the total dissolved gas supersaturation. Conserv. Physiol. 10, coac047. doi: 10.1093/conphys/coac047
Li N., Fu C., Zhang J., Liu X., Shi X., Yang Y., et al. (2019). Hatching rate of Chinese sucker (Myxocyprinus asiaticus Bleeker) eggs exposed to total dissolved gas (TDG) supersaturation and the tolerance of juveniles to the interaction of TDG supersaturation and suspended sediment. Aquaculture Res. 50, 1876–1884. doi: 10.1111/are.2019.50.issue-7
Li P., Zhu D. Z., Li R., Wang Y., Crossman J. A., Kuhn W. L. (2022). Production of total dissolved gas supersaturation at hydropower facilities and its transport: A review. Water Res. 223, 119012. doi: 10.1016/j.watres.2022.119012
Liu J., Kattel G., Wang Z., Xu M. (2019). Artificial fishways and their performances in China’s regulated river systems: A historical synthesis. J. Ecohydraulics 4, 158–171. doi: 10.1080/24705357.2019.1644977
Liu X. Q., Li K. F., Jiang W., Wu S. (2015). Biochemical responses and survival of rock carp (Procypris rabaudi) to total dissolved gas supersaturated water. Ichthyological Res. 62, 171–176. doi: 10.1007/s10228-014-0420-0
McGrath K. E., Dawley E., Geist D. R. (2006). Total dissolved gas effects on fishes of the lower Columbia River (Richland, WA (United States: Pacific Northwest National Lab.(PNNL).
Noonan M. J., Grant J. W., Jackson C. D. (2012). A quantitative assessment of fish passage efficiency. Fish Fisheries 13, 450–464. doi: 10.1111/j.1467-2979.2011.00445.x
Oldani N. O., Baigún C. R. M., Nestler J. M., Goodwin R. A. (2007). Is fish passage technology saving fish resources in the lower La Plata River basin? Neotropical Ichthyology 5, 89–102. doi: 10.1590/S1679-62252007000200002
Pan X., Ye Z., Wo J., Xing Q., Yang J., Chen Y., et al. (2024). Interannual variability in otolith biogeochemical signatures of Japanese Spanish mackerel in the Yellow Sea. J. Mar. Syst. 242, 103933. doi: 10.1016/j.jmarsys.2023.103933
Penghao C., Pingkuo L., Hua P. (2019). Prospects of hydropower industry in the Yangtze River Basin: China’s green energy choice. Renewable Energy 131, 1168–1185. doi: 10.1016/j.renene.2018.08.072
Pleizier N. K., Algera D., Cooke S. J., Brauner C. J. (2020). A meta-analysis of gas bubble trauma in fish. Fish Fisheries 21, 1175–1194. doi: 10.1111/faf.12496
Pleizier N. K., Cooke S. J., Brauner C. J. (2023). Does swimming activity influence gas bubble trauma in fish? River Res. Appl. 39, 65–72. doi: 10.1002/rra.4069
Pleizier N. K., Rost-Komiya B., Cooke S. J., Brauner C. J. (2021). The lack of avoidance of total dissolved gas supersaturation in juvenile rainbow trout. Hydrobiologia 848, 4837–4850. doi: 10.1007/s10750-021-04676-w
Puertas J., Cea L., Bermúdez M., Pena L., Rodríguez Á., Rabuñal J. R., et al. (2012). Computer application for the analysis and design of vertical slot fishways in accordance with the requirements of the target species. Ecol. Eng. 48, 51–60. doi: 10.1016/j.ecoleng.2011.05.009
Qu L., Li R., Li J., Li K., Deng Y. (2011). Field observation of total dissolved gas supersaturation of high-dams. Sci. China Technological Sci. 54, 156–162. doi: 10.1007/s11431-010-4217-8
Rahel F. J., McLaughlin R. L. (2018). Selective fragmentation and the management of fish movement across anthropogenic barriers. Ecol. Appl. 28, 2066–2081. doi: 10.1002/eap.2018.28.issue-8
Rastogi A., Rodi W. (1979). Predictions of heat and mass transferin open channels. J Hydraulic Engineering. 104, 397–420.
Standen E. M., Hinch S. G., Rand P. S. (2004). Influence of river speed on path selection by migrating adult sockeye salmon (Oncorhynchus nerka). Can. J. Fisheries Aquat. Sci. 61, 905–912. doi: 10.1139/f04-035
Sugimoto M. (2002). Morphological color changes in fish: regulation of pigment cell density and morphology. Microscopy Res. technique 58, 496–503. doi: 10.1002/jemt.10168
Tan J., Gao Z., Dai H., Yang Z., Shi X. (2019). Effects of turbulence and velocity on the movement behaviour of bighead carp (Hypophthalmichthys nobilis) in an experimental vertical slot fishway. Ecol. Eng. 127, 363–374. doi: 10.1016/j.ecoleng.2018.12.002
Wang Y., An R., Li Y., Li K. (2017). Swimming performance of rock carp Procypris rabaudi and Prenant’s schizothoracin Schizothorax prenanti acclimated to total dissolved gas supersaturated water. North Am. J. Fisheries Manage. 37, 1183–1190. doi: 10.1080/02755947.2017.1353558
Wang Y., Liang R., Li K., Li R. (2020a). Tolerance and avoidance mechanisms of the rare and endemic fish of the upper Yangtze River to total dissolved gas supersaturation by hydropower stations. River Res. Appl. 36, 993–1003. doi: 10.1002/rra.v36.7
Wang Y., Zhang N., Wang D., Wu J. (2020b). Impacts of cascade reservoirs on Yangtze River water temperature: Assessment and ecological implications. J. Hydrology 590, 125240. doi: 10.1016/j.jhydrol.2020.125240
White R. G., Mefford B. (2002). Assessment of behavior and swimming ability of Yellowstone River sturgeon for design of fish passage devices. Citeseer. doi: 10.1177/00145246111220070608
Wu X., Rao J., He B. (1992). The history of the Chinese freshwater fisheries. Cultivation Chin. Freshw. fishes 3, 5–29.
Yakhot V., Orszag S. A. (1986). Renormalization group analysis of turbulence. I. Basic theory. J. Sci. computing 1, 3–51. doi: 10.1103/PhysRevLett.57.1722
Yang S., Milliman J., Xu K., Deng B., Zhang X., Luo X. (2014). Downstream sedimentary and geomorphic impacts of the Three Gorges Dam on the Yangtze River. Earth-Science Rev. 138, 469–486. doi: 10.1016/j.earscirev.2014.07.006
Yuan Q., Du J., Li K., Wang Y., Liang R. (2023). Effect of total dissolved gas supersaturation and flow velocity on survival and swimming ability of juvenile Schizothorax prenanti. Conserv. Physiol. 11, coad091. doi: 10.1093/conphys/coad091
Yuan Y., Wei Q., Yuan Q., Wang Y., Liang R., Li K., et al. (2021). Impact of TDG supersaturation on native fish species under different hydropower flood discharge programs. Aquat. Toxicol. 237, 105898. doi: 10.1016/j.aquatox.2021.105898
Zhang D., Xu Y., Deng J., Shi X., Liu Y. (2024). Relationships among the fish passage efficiency, fish swimming behavior, and hydraulic properties in a vertical-slot fishway. Fisheries Manage. Ecol. 3 (31), e12681. doi: 10.1111/fme.12681
Zhang Q., Liu X., Shi H., Yang Y. (2023). Interaction of total dissolved gas supersaturation and suspended sediment on swimming performance of bighead carp (Hypopthalmichthys nobilis). J. Fish Biol. 103, 1289–1299. doi: 10.1111/jfb.v103.6
Keywords: TDGS, swimming performance, passage efficiency, vertical slot fishway, bighead carp
Citation: Li M, Ou Y, Liao J, Shi H, Zhu B, Li X, Yuan Q and Yang X (2025) Assessment of total dissolved gas supersaturation stress on passage performance of bighead carp (Hypophthalmichthys nobilis) in vertical slot fishway. Front. Mar. Sci. 12:1549115. doi: 10.3389/fmars.2025.1549115
Received: 20 December 2024; Accepted: 29 January 2025;
Published: 20 March 2025.
Edited by:
Shulian Wang, Hubei University of Technology, ChinaReviewed by:
Jianghui Bao, Chinese Academy of Sciences (CAS), ChinaYuanming Wang, Sichuan University, China
Copyright © 2025 Li, Ou, Liao, Shi, Zhu, Li, Yuan and Yang. This is an open-access article distributed under the terms of the Creative Commons Attribution License (CC BY). The use, distribution or reproduction in other forums is permitted, provided the original author(s) and the copyright owner(s) are credited and that the original publication in this journal is cited, in accordance with accepted academic practice. No use, distribution or reproduction is permitted which does not comply with these terms.
*Correspondence: Quan Yuan, eXVhbnExOTkyQDEyNi5jb20=