- 1College of Fisheries, Guangdong Ocean University, Zhanjiang, China
- 2Shenzhen Research Institute of Guangdong Ocean University, Shenzhen, China
- 3Zhuhai Longsheng Improved Fingerling Breeding Co., Ltd, Zhuhai, China
- 4Agro-Tech Extension Center of Guangdong Province, Guangzhou, China
- 5Guangdong Provincial Marine Fish Technology Innovation Center, Zhanjiang, China
- 6Guangdong Provincial Key Laboratory of Aquatic Animal Disease Control and Healthy Culture, Zhanjiang, China
Introduction: This study investigates the effects of salinity on the growth, survival, tissue morphology, osmotic regulation, metabolism, and antioxidant responses of juvenile Eleutheronema tetradactylum.
Methods: The experiment was conducted under controlled aquaculture conditions with eight salinity treatments (0, 5, 10, 15, 20, 25, 30, and 35 PSU), each with three replicates (20 fish per replicate) in cylindrical tanks (500 L). Juveniles (mean total length: 16.43 ± 0.87 cm; mean body weight: 35.71 ± 1.067g) were exposed to the treatments for 30 days. Key measurements included plasma osmotic pressure, ion concentrations, and Na+/K+-ATPase (NKA) in the gills, assessed at 0, 1, 10, 20, and 30 days.
Results: Survival rates, growth parameters, and histopathological changes in gill, intestinal, and kidney tissues were also evaluated. Additionally, plasma levels of lactic acid (LD), triglycerides (TG), glucose (GLU), total superoxide dismutase (SOD), glutathione peroxidase (GSH-Px), total antioxidant capacity (T-AOC), and malondialdehyde (MDA) were measured. The results revealed that survival rates were significantly lower in the 0 PSU group compared to all other salinities (P< 0.05). Growth performance, including specific growth rate (SGR), weight gain rate (WGR), and daily weight gain (DWG), was significantly reduced at high salinities (30 and 35 PSU) (P< 0.05). Histopathological alterations were observed in the gills, intestine, and kidneys, particularly in osmoregulatory tissues. Salinity also significantly affected NKA, plasma osmotic pressure, and ion concentrations. The isosmotic point for E. tetradactylum was determined to be approximately 10.88 PSU. Metabolic responses, including LD, TG, and GLU, exhibited a pattern of initial decline followed by an increase with increasing salinity. SOD activity was significantly higher in the 10 PSU group compared to the 30 and 35 PSU groups (P< 0.05), while T-AOC showed a “U”-shaped response to increasing salinity. GSH-Px activity decreased with salinity, especially at 35 PSU (P< 0.05), while MDA levels did not vary significantly (P > 0.05).
Discussion: In conclusion, E. tetradactylum belongs to euryhaline fish species, with optimal growth occurring at lower salinities(5-10PSU). High salinity (30–35 PSU) adversely affects growth and antioxidant defense mechanisms, highlighting the species’ sensitivity to elevated salinity. Beyond identifying species-specific sensitivity, this work provides actionable guidelines for optimizing aquaculture practices, reducing metabolic costs, and mitigating oxidative stress in captive-reared populations.
1 Introduction
The four-finger threadfin (Eleutheronema tetradactylum), also known as Indian salmon or blue threadfin, is a pelagic-neritic fish species belonging to the family Polynemidae in the order Carangaria (Qu et al., 2020). This species is widely distributed in the Indo-West Pacific region, ranging from the Persian Gulf to Papua New Guinea and extending to northern Australia (Yamada et al., 1995; Motomura et al., 2002; Motomura, 2004). Juvenile and sub-adult E. tetradactylum typically inhabit shallow waters near harbor or river mouths, where they forage on crustaceans, mollusks, and cephalopods, utilizing their specialized pectoral fins (Pember, 2006; Wang et al., 2014; Iqbal et al., 2023). E. tetradactylum is known for its remarkable tolerance to a broad range of salinities, making it highly adaptable to various aquatic environments (Motomura and Nations, F. and A. O. of the U, 2004Motomura and Nations, 2004; Ballagh et al., 2012). Due to its rapid growth, short production cycle, and high economic potential, this species has emerged as a promising candidate for mariculture in Southern China (Wang et al., 2014; Huang et al., 2022). However, the effects of varying salinity on its survival, growth, and physiological development remain underexplored, underscoring the need for further research on its adaptation mechanisms to different salinity conditions.
Salinity exerts a significant influence on the growth, development, and reproductive processes of fish species. Japanese eel (A. japonica) larvae at 5 days post-hatch exhibited significant body depth increase after 7-day feeding under 30% and 50% seawater conditions, with the 50% salinity group showing a 2.2-fold higher 2-month cumulative survival rate compared to the 100% salinity group (Okamura et al., 2009). Juvenile fish of Tilapia rendalli at a salinity of 10 show significantly greater growth compared to those in a freshwater environment. For juvenile T. rendalli, the feed conversion ratio (FCR) is lower, the feed conversion efficiency and protein efficiency are higher, and the survival rate is also higher at a salinity of 10 than those in freshwater (An et al., 2008). Fish require different salinity environments at various life stages. For example, the Japanese eel (Anguilla japonica) needs high-salinity waters during spawning and hatching, while in other stages of growth and life, it prefers low-salinity or freshwater environments (Pearse et al., 2014). The sea lamprey (Petromyzon marinus) and the Atlantic salmon (Salmo salar), on the other hand, migrate from the ocean to the upper reaches of rivers for spawning during their life history (O’Malley et al., 2010). The study found that the spawning quantity per unit body weight of female one-year-old Florida red Mozambique tilapia (Oreochromis mossambicus) in each spawning event was significantly higher under freshwater conditions than under high-salinity conditions. This indicates that high salinity has an inhibitory effect on the reproductive performance of the broodstock of red Mozambique tilapia, and the yield of fry decreases as the salinity increases (Watanabe et al., 1989). These species-specific responses to salinity variations across life stages are fundamentally mediated by complex physiological and molecular mechanisms governing osmoregulation. Particularly in teleost fishes, the capacity to maintain internal homeostasis under salinity stress involves precisely coordinated biological processes.
Salinity is a critical environmental factor influencing the growth, survival, and physiological functions of teleost fish. Teleosts, particularly euryhaline species like E. tetradactylum, exhibit a range of physiological responses to cope with salinity fluctuations (Wang et al., 2023). Prolonged exposure to suboptimal salinity can result in stunted growth, reduced feeding, and in extreme cases, mortality (Bøeuf and Payan, 2001). Fish regulate their internal osmotic pressure to maintain homeostasis in response to changes in external salinity, a process often accompanied by structural and functional adaptations in osmoregulatory tissues (Takata et al., 2021). The osmoregulatory capacity of fish, which governs their ability to maintain homeostasis in fluctuating salinity conditions, influences critical physiological processes, including embryonic development, gonadal maturation, feeding and digestion, and overall growth (Zhang et al., 2013). In particular, ions such as Na+ and Cl- play a pivotal role in osmoregulation, with their serum levels often correlating with changes in serum osmotic pressure, particularly in euryhaline species (Zhao et al., 2006). Under both hypo- and hyperosmotic conditions, increased activity of Na+/K+-ATPase (NKA) in gill cells is commonly used as a marker of active osmoregulation (Hwang and Lee, 2007; Lai et al., 2019). Moreover, shifts in salinity affect various physiological processes in teleosts, including energy metabolism, antioxidation, and immune function, which can be assessed by monitoring metabolic and antioxidative indices, such as glucose (GLU), triglycerides (TG), superoxide dismutase (SOD), and glutathione peroxidase (GSH-Px) in tissues to evaluate their adaptation to different salinity conditions (Claireaux and Lagardère, 1999).
Although previous studies on E. tetradactylum have focused on aspects such as resource surveys (Pember, 2006; Ballagh et al., 2012), genetic structure (Zhang et al., 2014; Qu et al., 2020), and artificial breeding (Zamidi et al., 2012; Cheng et al., 2017), limited research has focused on the effects of salinity adaptation on this species, particularly regarding growth, survival, and physiological responses. While acute salinity stress has been investigated (Luo et al., 2015; Niu et al., 2021), a comprehensive understanding of how E. tetradactylum adapts to salinity fluctuations over extended periods is still lacking. This gap is especially relevant in light of the growing demand for sustainable aquaculture in saline-alkaline inland and nearshore brackish environments, driven by the depletion of freshwater resources and the increasing need for aquatic protein sources (Dissanayake, 2019; Durigon et al., 2019; Stiller et al., 2020). The expansion of aquaculture from coastal to inland regions further underscores the importance of studying the salinity tolerance mechanisms in euryhaline species like E. tetradactylum (Zhang et al., 2022).
Optimal salinity adaptation in E. tetradactylum is postulated to involve physiological balance between osmoregulatory costs (gill NKA activity) and antioxidant defense (hepatic SOD/GSH-Px), where growth suppression occurs when combined physiological demands exceed compensatory thresholds. To investigate this mechanism, a 30-day salinity gradient trial (0-35 PSU) was conducted to: (1) determine salinity thresholds affecting growth (SGR, FCR), survival rate, and gill/kidney histology; (2) analyze interactions between osmoregulation (serum osmolality, NKA), energy metabolism (glycogen/lipid content), and oxidative status (SOD, GSH-Px, MDA); (3) establish salinity optimization criteria for aquaculture in variable environments.
2 Materials and methods
2.1 Experimental design, fish stocking and management
Juvenile E. tetradactylum were procured locally and cultured temporarily for two months prior to the experiment. Healthy and robust individuals were selected for the salinity adaptation study. The initial average body length and body weight of the experimental fish were (16.43 ± 0.87) cm and (35.71 ± 1.067) g, respectively. During the acclimation period, the salinity was maintained at 25 PSU, with dissolved oxygen levels above 5 mg/L and a water temperature ranging from 26 to 28°C. The experiment was conducted in a 500 L indoor aquarium system with a continuous water circulation system. Based on prior research on the salinity tolerance of E. tetradactylum conducted in our laboratory, a total of eight salinity levels, ranging from 0 to 35 PSU, were established, including a control group at 25 PSU. Each salinity level was tested in triplicate, with 20 juvenile fish randomly assigned to each replicate group (Cao et al., 2020). Prior to the start of the experiment, the salinity in each aquarium was gradually adjusted to the target level at a rate of 5 PSU/day using dechlorinated freshwater. The transition rate was reduced to 2.5 PSU/day when adjusting from 5 PSU to 0 PSU. Daily measurements (YSI Pro30 salinometer) ensured stability (± 1 ppt).The formal experiment began after a 7-day acclimation period for the fish to adjust to the new environment.
Throughout the experimental period, dissolved oxygen levels were maintained above 5 mg/L by providing continuous aeration. The fish were fed a commercial puffed feed (Guangdong Dongteng Feed Co., China; crude protein ≥43%, crude fat ≥5%, ash ≤15%, moisture ≤10%), at 08:00 am and 16:00 pm, formulated for marine carnivorous fish. The feed contains fishmeal, soybean meal, peanut meal, flour, corn protein meal, and fish oil. The daily feeding amount was set to 3% of the total body weight of the fish. Additionally, 500 L of seawater was replaced with fresh water of the same salinity every two days, with 50% of the water volume replaced during each exchange. Fish survival, movement, and feeding behaviors were carefully monitored and recorded throughout the experiment.
2.2 Sampling and growth evaluation
Fish were systematically sampled at five critical time points (day 0: baseline; day 1: acute phase; days 10/20: adaptive phases; day 30: terminal phase) to capture dynamic physiological responses. For each salinity group, six randomly selected specimens were anesthetized with quick-freeze prior to sampling. Blood was collected via caudal venipuncture using heparinized syringes, and non-hemolyzed serum was obtained through centrifugation (3,000×g, 15 min, 4°C) for subsequent analysis of:
i. Osmotic parameters (osmolarity, Na+, K+, Cl- concentrations).
ii. Metabolic profiles (glucose, triglycerides).
iii. Antioxidant capacity (SOD, CAT, GPx activities).
Gill filaments from the right second arch were immediately flash-frozen in liquid nitrogen and stored at -80°C for Na+/K+-ATPase (NKA) activity quantification. Histological specimens (gill lamellae, intestinal segments, cephalic kidney) were fixed in 4% paraformaldehyde (4°C, 48 hr) for paraffin embedding and H&E staining.
Growth performance was assessed using standardized aquaculture metrics:
Note: W0, Wt: Initial/final body weight (g), L0, Lt: Initial/final body length (cm), t: Experimental duration (days), n: Surviving fish count, F: Cumulative feed intake (g).
2.3 Histological examination of gill, intestinal, and kidney tissues
Tissues preserved in 4% paraformaldehyde were subjected to a standard histological processing procedure. This included dehydration in graded alcohol solutions, clearing in xylene, paraffin embedding, and sectioning. The tissue sections were stained with Hematoxylin and Eosin (H&E) and examined under an inverted fluorescence microscope (Nikon Eclipse Ti-E).
2.4 Measurement of serum osmolarity, ion concentration, and gill NKA activity
Serum osmolarity and aqueous ambient osmolality were measured using a freezing point osmometer (Osmo210PRO, YASN, UK) as described by Cao et al. (2020).
Ion concentrations of K+, Na+, and Cl- in serum and water samples were determined using an electrolyte analyzer (K-Lite5, Meizhou Kangli High-Tech Co., Ltd., Guangzhou, China).
For NKA activity, approximately 0.5 g of gill tissue was homogenized in pre-chilled physiological saline (5 volumes) using an IKA homogenizer (T10B, IKA, Co., Germany) for 1 min on ice. The homogenate was then centrifuged at 4°C at 12,000 rpm for 20 min, and the supernatant was collected for enzyme activity analysis using a commercial assay kit (Nanjing Jiancheng Bioengineering Institute, Nanjing, China).
2.5 Determination of serum metabolic and antioxidant indices
The levels of lactate, triglycerides (TG), and glucose (GLU) in serum were measured using methods outlined by Kavadias et al (Kavadias et al., 2003). The total antioxidant capacity (T-AOC), superoxide dismutase (SOD) activity, glutathione peroxidase (GSH-Px) activity, and malondialdehyde (MDA) content in serum samples were quantified using commercial assay kits (Nanjing Jiancheng Bioengineering Institute, Nanjing, China).
2.6 Statistical analysis
All data were analyzed using SPSS 19.0 statistical software. Growth parameters (e.g., weight gain, specific growth rate) were analyzed using one-way ANOVA followed by Tukey’s post hoc test. Survival rates were compared via chi-square tests. Tissue morphology data (e.g., gill filament length) were assessed using Kruskal-Wallis tests due to non-normal distributions. Calculation of isotonic point by regression analysis. Antioxidant enzyme activities (SOD, CAT) and metabolic indicators (e.g., glucose levels) were evaluated with two-way ANOVA (salinity × time). Data normality and homogeneity of variance were confirmed using Shapiro-Wilk and Levene’s tests, respectively. Statistical significance was set at P< 0.05 for significant differences and P< 0.01 for highly significant differences.
3 Results
3.1 Effects of salinity on survival and growth of E. tetradactylum
At the conclusion of the 30-day salinity acclimation experiment, the survival rates of each group were determined to be 53.33%, 98.33%, 100%, 100%, 100%, 95%, 100%, and 95%, respectively (Figure 1). Notably, there were significant differences observed in the survival rates between the groups cultured in salinity 0 and the other groups (P< 0.05).
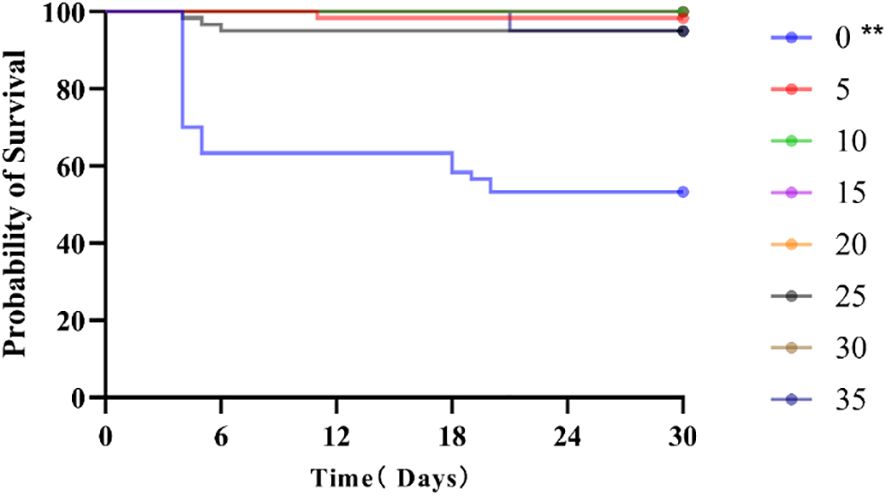
Figure 1. Survival curve of juvenile E. tetradactylum after 30 days of exposure to various salinities. Fish were exposed to the following salinities: 0, 5, 10, 15, 20, 25, 30 and 35 PSU. ** indicates significant differences compared to the control group (p < 0.01).
In terms of growth performance (Table 1), no significant differences were observed among the low salinity groups (0, 5, 10 PSU) (P > 0.05). However, in the high salinity groups (30, 35 PSU), growth indicators such as Growth Rate (SGR), Weight Gain Rate (WGR), and Daily Weight Gain Rate (DWGR) significantly decreased with increasing salinity (P< 0.05). Notably, the Feed Conversion Ratio (FCR) was significantly higher at salinities of 0 and 35 PSU compared to the control group (P< 0.05).
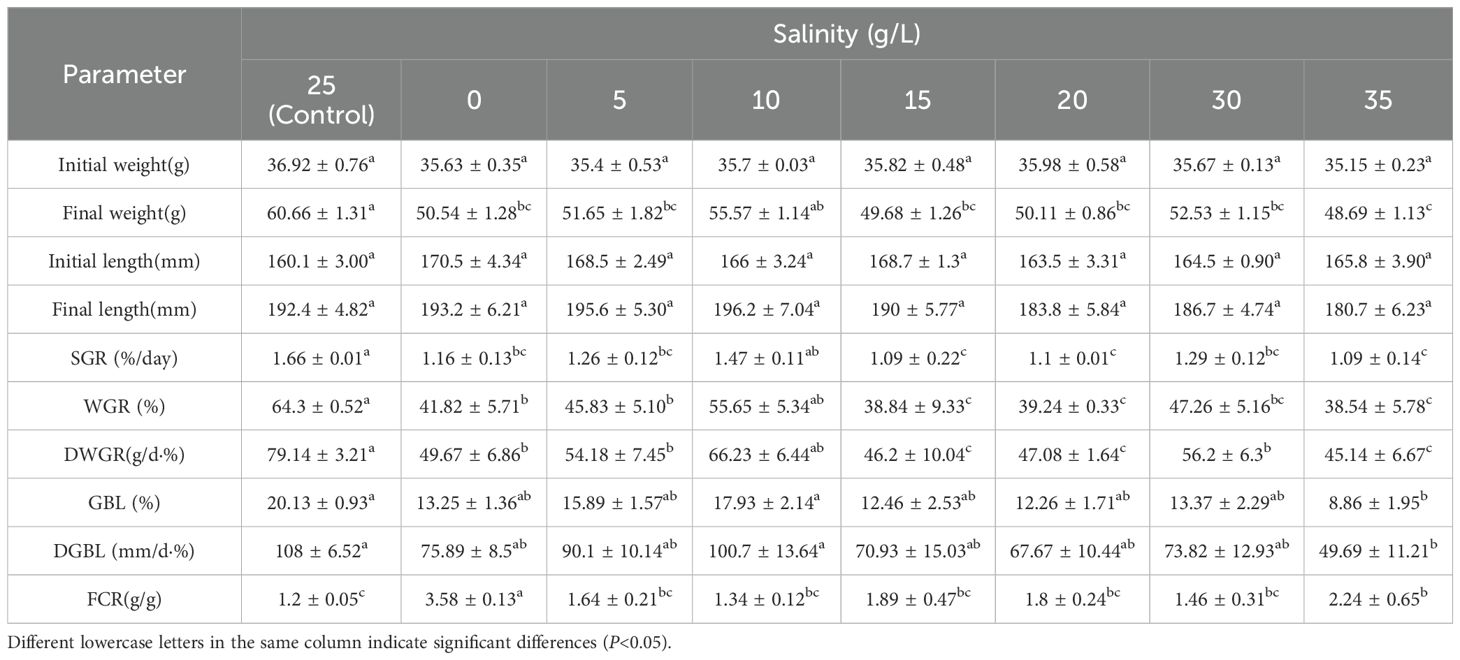
Table 1. Growth and feed conversion rate of juvenile E. tetradactylum after 30 days of exposure to various salinities. Fish were exposed to the following salinities: 0, 5, 10, 15, 20, 25, 30 and 35 PSU.
3.2 Histopathological examination of gill, intestine, and kidney tissues
3.2.1 Gill microstructure
The gill structure of juvenile E. tetradactylum exhibited typical histological characteristics (Figure 2). In the low salinity groups, significant morphological changes were observed after 30 days. The length of the secondary gill lamellae increased significantly (P< 0.05), and the spacing between the lamellae widened (P< 0.05). Furthermore, the gill lamellae cells became more rounded and plumper, and the number of chloride cells on the gill filaments and gill lamellae decreased significantly. In the high salinity groups, the length of the gill lamellae significantly decreased (P< 0.05), while the spacing between the lamellae increased. The number of chloride cells showed only a slight increase, which was not statistically significant (Table 2).
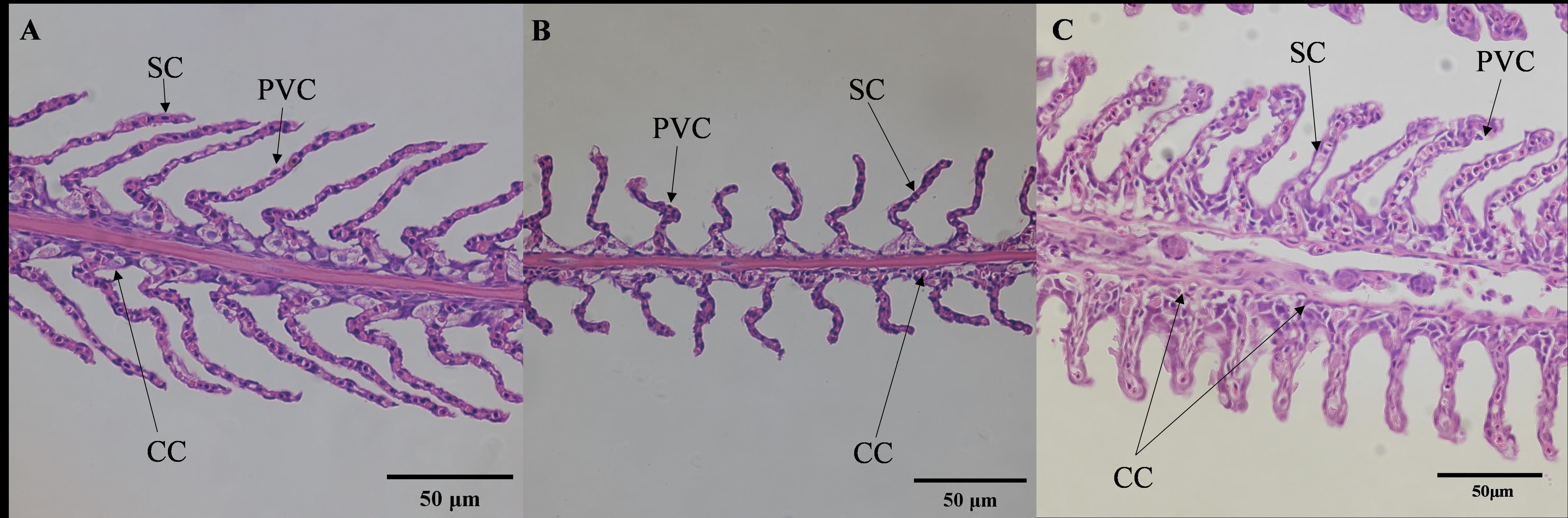
Figure 2. Effects of salinities on microstructure of gill of juvenile E. tetradactylum. (A) salinity 5 PSU(40×); (B) salinity 25 PSU(40×); (C) salinity 35 PSU(40×); PVC, Pavement epithelial cell; SC, Pillar cell; CC, Mucous cell.
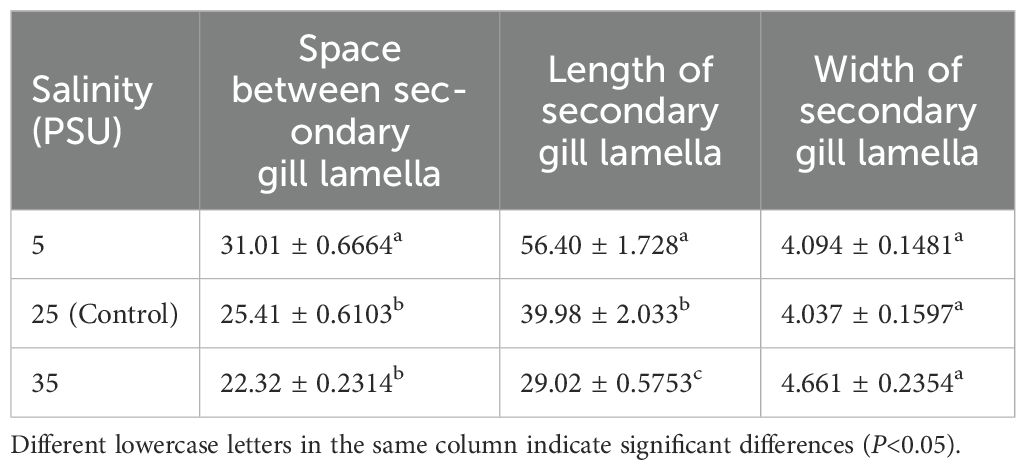
Table 2. Morphological changes in the gill of juvenile E. tetradactylum under different salinity treatments (μm).
3.2.2 Intestinal microstructure
The microscopic examination of the intestines revealed significant structural changes (Figure 3). In the low salinity group, the thickness of the single-layer columnar epithelium on the intestinal villi significantly increased, while the number of goblet cells decreased significantly (P< 0.05), while the size of goblet cell bodies remained unchanged (Figure 3A). Conversely, in the high salinity group, the goblet cells appeared enlarged (Figure 3C), but there was no significant difference in the thickness of the columnar epithelium or the number of goblet cells compared to the control group.
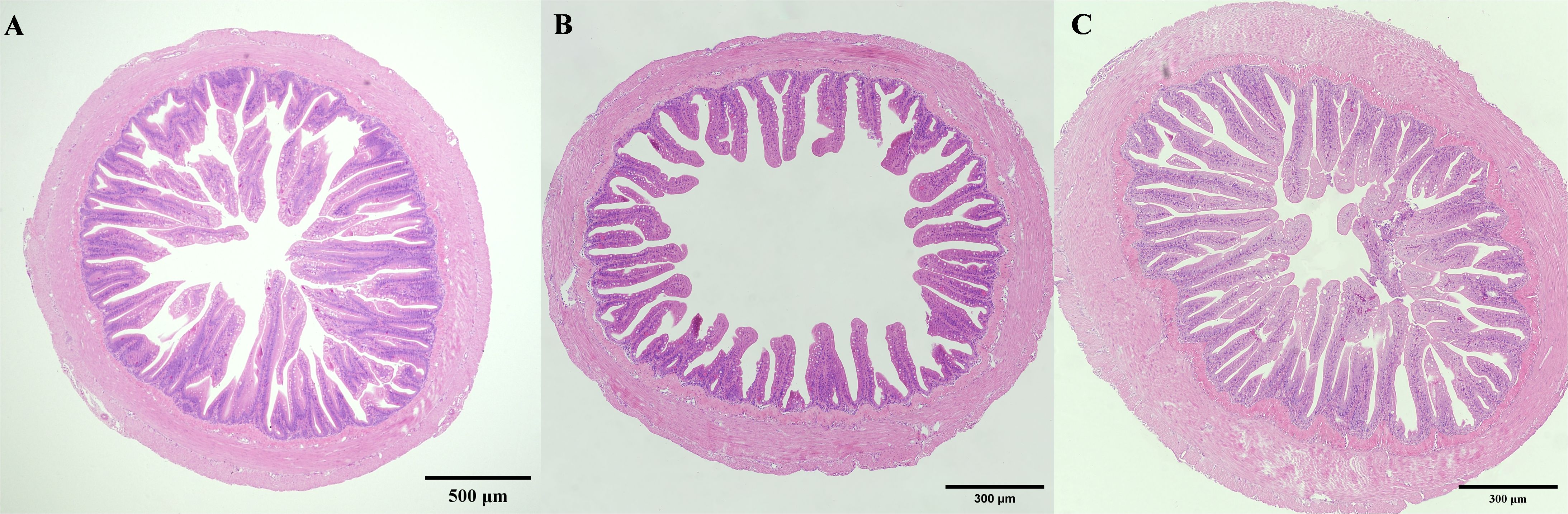
Figure 3. Effects of salinities on microstructure of gill of juvenile E. tetradactylum. (A) salinity 5 PSU(5×); (B) salinity 25 PSU(5×); (C) salinity 35 PSU(5×).
3.2.3 Kidney microstructure
The microscopic structure of the kidneys also exhibited significant alterations under different salinity conditions (Figure 4). In the low salinity group, the renal corpuscles appeared swollen, with small luminal spaces, and the diameter of the renal tubules increased. In the high salinity group, renal corpuscles were atrophied, with larger luminal spaces, and the diameter of the renal tubules slightly decreased.
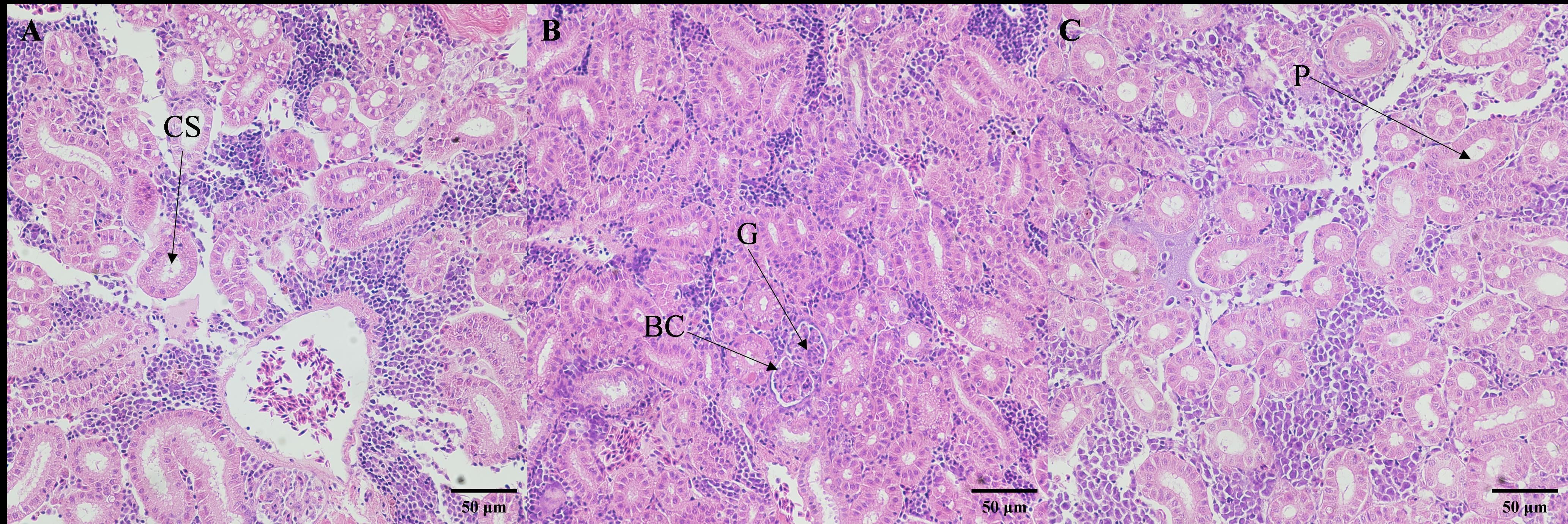
Figure 4. Effects of salinities on microstructure of kidney of juvenile E. tetradactylum. (A) salinity 5 PSU(40×); (B) salinity 25 PSU(40×); (C) salinity 35 PSU(40×); G, Glomerulus; BC, Renal Capsule; P, Renal Tubule; CS, Collecting Duct.
3.3 Osmotic regulation in response to salinity
3.3.1 Gill NKA activity
The activity of NKA in the gills was significantly influenced by salinity (Figure 5). At salinity 25 PSU, NKA activity remained stable throughout the 30-day experiment (3.47 ± 0.68 U/mg protein). In contrast, the maximum activity occurred earlier, on Day 2, in the 10 PSU and 35 PSU groups, after which it gradually decreased and stabilized. The activity for the 0 PSU and 5 PSU groups peaked on Day 20 before stabilizing.
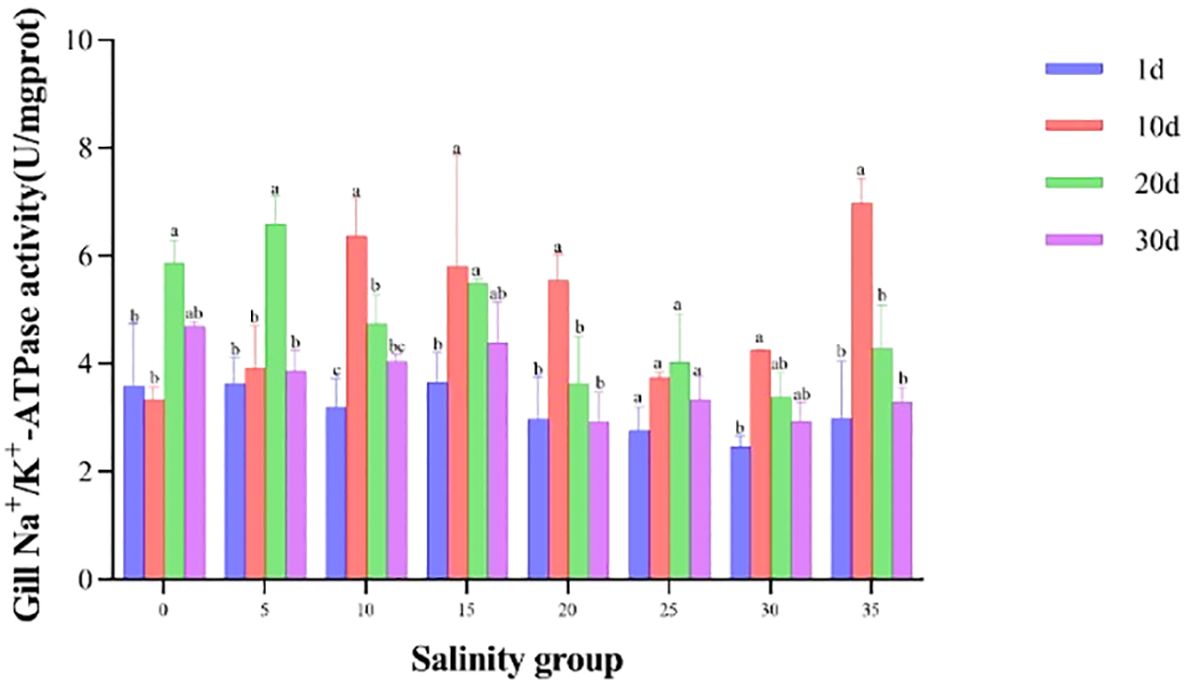
Figure 5. NKA activity in the gill of juvenile E. tetradactylum across different salinities. Fish were exposed to the following salinities: 0, 5, 10, 15, 20, 25, 30 and 35 PSU. Different lowercase letters in the same column indicate significant differences (p<0.05).
3.3.2 Serum osmolality
Salinity had a significant effect on serum osmolality in juvenile E. tetradactylum (Figure 6). In the low salinity groups (0, 5, 10 PSU), serum osmolality sharply decreased on Day 1, followed by a gradual increase and stabilization, remaining lower than that of the control group. Conversely, in the high salinity groups (30, 35 PSU), serum osmolality increased on Day 1, then gradually decreased and stabilized. A significant difference (P< 0.05) was observed in the 35 PSU group on Day 1. Linear regression analysis revealed that the isotonic point salinity for E. tetradactylum juveniles was approximately 10.88 PSU (Figure 7).
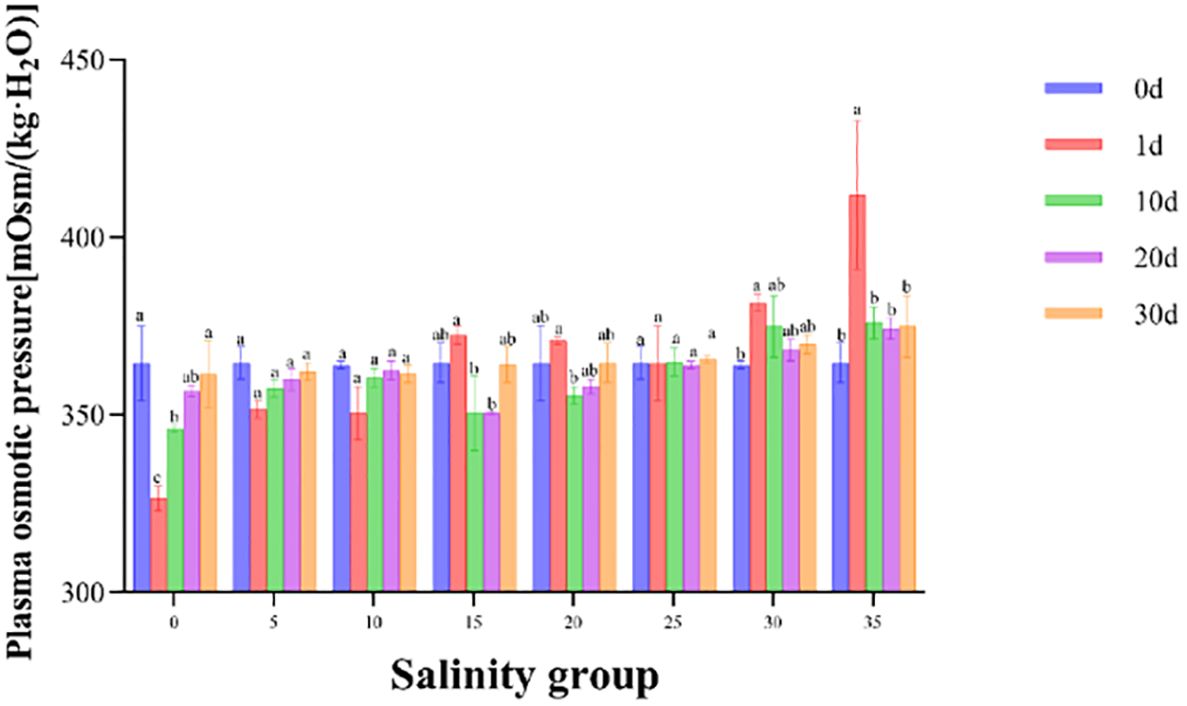
Figure 6. Serum osmolality of juvenile E. tetradactylum in response to different salinities on day 0, 10, 20 and 30. Fish were exposed to the following salinities: 0, 5, 10, 15, 20, 25, 30, and 35 PSU. Different lowercase letters in the same column indicate significant differences (p<0.05).
3.3.3 Serum Na+, K+, and Cl- concentrations
Serum concentrations of Na+, K+, and Cl- were significantly affected by salinity (Figure 8). In the low salinity groups (0, 5, 10 PSU), Na+ and Cl- concentrations were significantly reduced on Day 1, followed by a gradual increase. No significant changes were observed in the 15, 20, and 25 PSU groups during the experiment. In contrast, the concentrations of Na+, K+, and Cl- in the high salinity groups (30, 35 PSU) increased sharply on Day 1 before gradually decreasing.
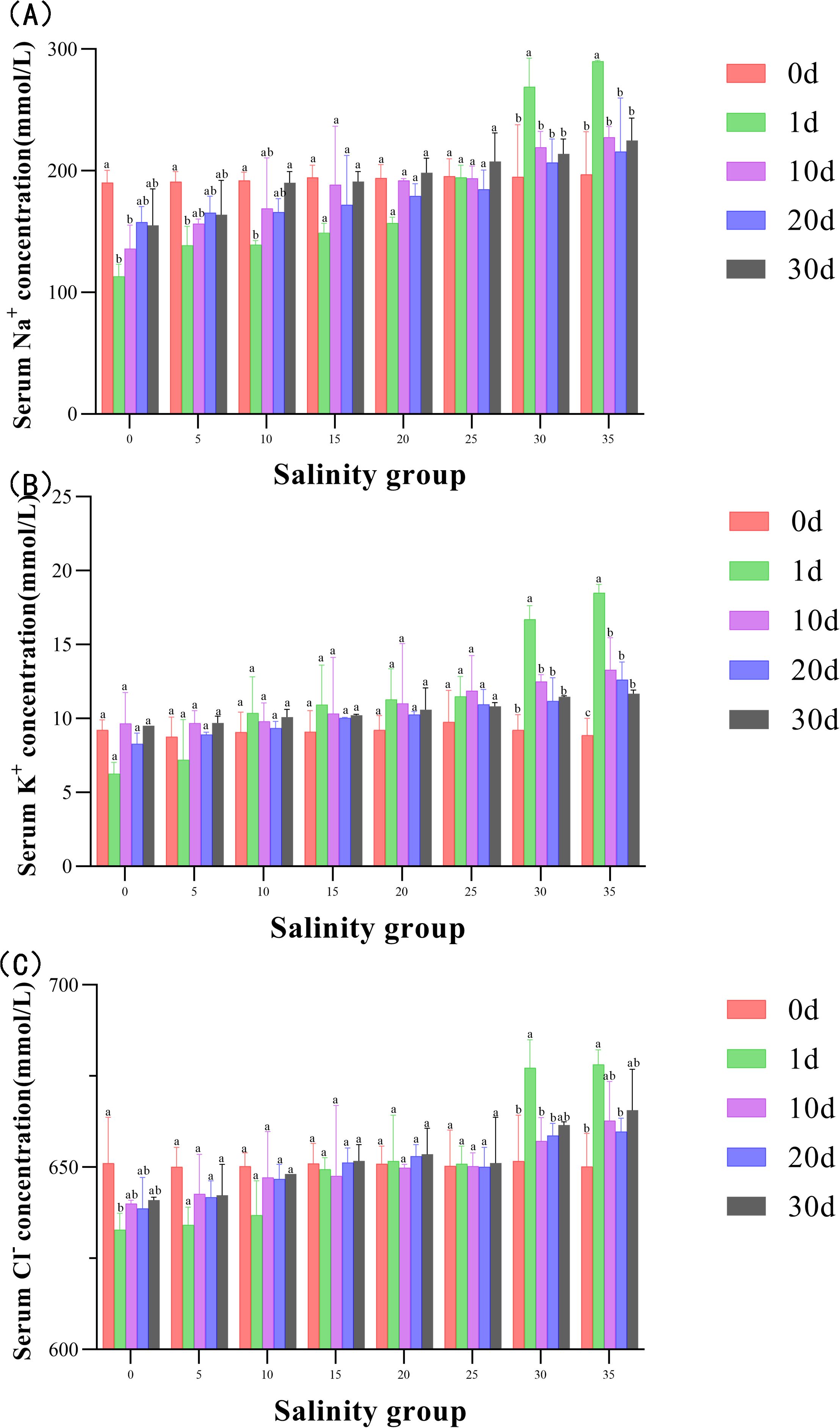
Figure 8. (A–C) Serum ion concentrations of Na+, K+, and Cl- in Juvenile E. tetradactylum at different salinities. Fish were exposed to the following salinities: 0, 5, 10, 15, 20, 25, 30 and 35 PSU. Different lowercase letters in the same column indicate significant differences (p<0.05).
3.4 Metabolic response to salinity
In terms of metabolic indices, glucose (GLU) levels significantly increased in the 35 PSU group compared to the 5-25 PSU groups (P< 0.05). Triglyceride (TG) concentrations were also significantly higher in the 35 PSU group compared to the low salinity groups (0, 5, 10 PSU) and the control group (P< 0.05). The triglycerides (TG) and lactate dehydrogenase (LD) levels exhibited a decreasing-then-increasing trend with increasing salinity. The LD content significantly increased in the 30 and 35 PSU groups compared to the 5-25 PSU groups (P< 0.05) (Figure 9).
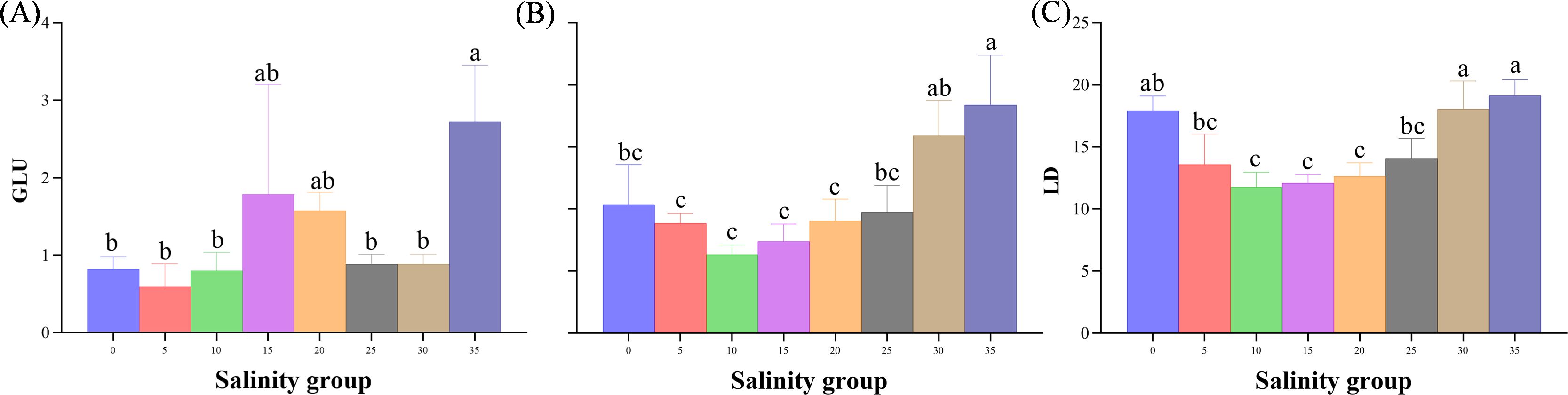
Figure 9. (A–C) Metabolic indices (GLU, TG, LD) in the serum of juveniles E. tetradactylum exposed to different salinities. Fish were exposed to the following salinities: 0, 5, 10, 15, 20, 25, 30, and 35 PSU. Different lowercase letters in the same column indicate significant differences (p<0.05).
3.5 Antioxidant responses to salinity
The antioxidant capacity, measured by superoxide dismutase (SOD) and glutathione peroxidase (GSH-Px), varied with salinity (Figure 10). SOD activity was significantly higher in the 10 PSU group compared to the 30 and 35 PSU groups (P< 0.05). GSH-Px activity showed a decreasing trend with increasing salinity, with the lowest levels observed in the 35 PSU group, significantly lower than in the 5 and 10 PSU groups (P< 0.05).
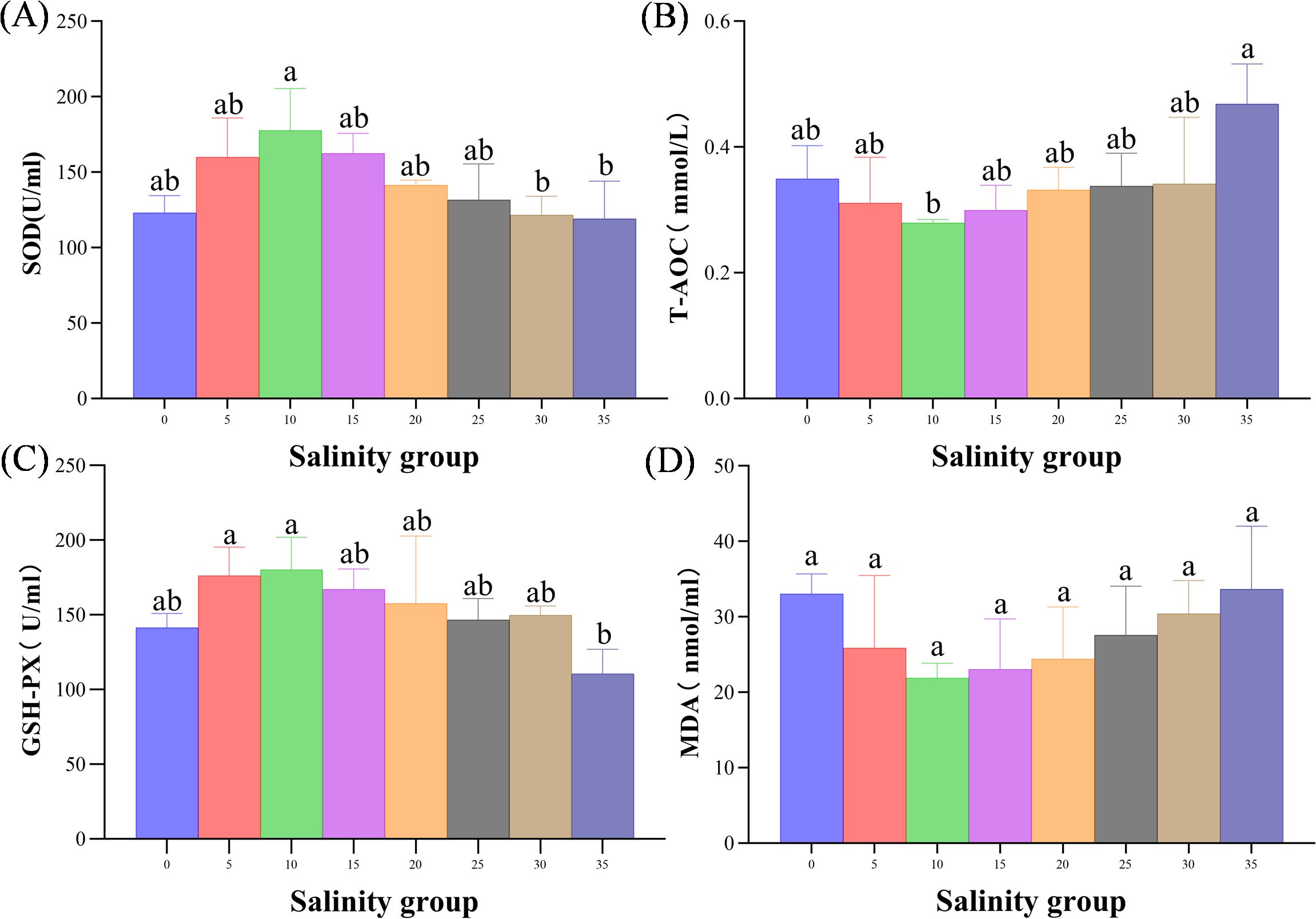
Figure 10. (A–D) Antioxidant responses (SOD and GSH-Px Activity) in the serum of juvenile E. tetradactylum at different salinities. Fish were exposed to the following salinities: 0, 5, 10, 15, 20, 25, 30, and 35 PSU. Different lowercase letters in the same column indicate significant differences (p<0.05).
4 Discussion
4.1 Growth parameters
The present study highlights the significant effect of salinity on the growth and survival of E. tetradactylum juveniles, which inhabit estuarine areas with varying salinity levels (Wang et al., 2014). As a euryhaline species, E. tetradactylum is typically more adapted to higher salinity levels, with previous studies indicating that embryos and larvae thrive in salinities between 28-35 PSU and salinities below 20 PSU significantly impair survival (Xie et al., 2016). However, our results show that juvenile E. tetradactylum have a broader tolerance to salinity fluctuations, with survivability observed even at lower salinities such as 0, 5, and 10 PSU. Notably, although the fish can survive at these lower salinities, growth was negatively impacted at 0 PSU, which aligns with findings from Niu et al. (2021), who reported that E. tetradactylum juveniles showed increased mortality at salinities of 0 and 20 PSU.
In terms of growth, E. tetradactylum juveniles in brackish water (5 and 10 PSU) demonstrated higher growth rates compared to those in high salinity environments (30 and 35 PSU). This is consistent with earlier studies that reported better growth in fish reared at lower salinities (Imsland et al., 2001; Luz et al., 2008; Semra et al., 2013). The observed increase in Feed Conversion Ratio (FCR) at higher salinities suggests that higher energy expenditure for osmoregulation may reduce the efficiency of feed utilization, a phenomenon supported by studies on digestive enzyme activity and intestinal morphology in varying salinity conditions (Usher et al., 1988; Moutou et al., 2004). These findings suggest that brackish water (5 and 10 PSU) may provide the optimal conditions for juvenile E. tetradactylum growth in aquaculture systems. However, further investigations are necessary to determine the effects and economic viability of using these lower salinities for commercial aquaculture operations.
4.2 Osmoregulatory mechanisms and physiological adaptations to salinity adaptation in E. tetradactylum
The salinity adaptation process in fish occurs in two stages: passive adaptation to the external environment and active recovery of osmotic pressure (Li et al., 2022). Fish adapt to varying salinities primarily through the regulation of osmotic pressure and ion concentrations, a process mainly carried out by osmoregulatory organs such as the gills, intestines, and kidneys (Whittamore, 2012; Sun et al., 2016; Dawood et al., 2021; Ali et al., 2024).
Gill filaments of juveniles exposed to 5 PSU became thinner, longer, and more widely spaced, indicating an adaptive response to osmotic pressure changes. This result is consistent with findings by Dawood et al. (2022). However, fish in 35 PSU exhibited gill filaments that were wider, shorter, and more closely spaced, with partial necrosis and enlarged chloride cells, indicating structural damage to the gills and impaired osmoregulatory function.
The observed morphological changes in the intestine and kidney further support these findings. At 5 PSU, the intestinal villi showed increased thickness, while the number of goblet cells decreased, possibly as an adaptive mechanism to optimize nutrient absorption in a low salinity environment. At 35 PSU, although goblet cells became enlarged, there were no significant changes in epithelial thickness or goblet cell number, suggesting limited adaptation. Kidney tissues from fish exposed to 35 PSU showed signs of atrophy, with smaller glomeruli and reduced renal function, which are typical indicators of osmotic stress in hyperosmotic environments (Chourasia et al., 2018). These findings emphasize that extreme salinities, although survivable, result in significant physiological stress and impaired organ function, which could compromise overall fish health and aquaculture productivity.
Osmotic regulation in E. tetradactylum was assessed through NKA, serum osmolality, and ion concentrations. NKA is a key ion transporter in fish that helps maintain osmotic balance by pumping Na+ out and K+ into cells Rodriguez et al., 2002). Our results showed that NKA activity peaked at Day 20 in the 0 and 5 PSU groups, suggesting a passive stress response resulting from a decrease in NKA activity, which reduces cell membrane permeability and decreases Na+ efflux (Parsegian et al., 2000). In contrast, in the 10 and 35 PSU groups, NKA activity peaked earlier at Day 10, followed by a decline, which may reflect acclimatization to these salinity levels (Shaughnessy and McCormick, 2020);. This pattern of NKA activity supports the idea that E. tetradactylum juveniles can effectively regulate their ion balance within a range of 10-35 PSU but experience increased osmoregulatory stress outside of this range (Tomy et al., 2009; Dalziel et al., 2014).
Serum osmolality and ion concentrations followed similar trends, with significant decreases in the low salinity groups (0, 5, 10 PSU) on Day 1, followed by gradual recovery. In contrast, serum osmolality in the high salinity groups (30, 35 PSU) increased on Day 1 and then stabilized. These patterns indicate that E. tetradactylum juveniles can maintain osmotic balance within a specific range of salinities, which is consistent with the isosmotic point of 10.88 PSU identified in this study. Plasma osmotic pressure serves as an early indicator of aquatic stress, reflecting changes in solute concentrations, including inorganic ions (such as Na+, K+, Cl-, and Ca2+), as well as proteins and organic small molecules (Tsui et al., 2012; Kültz, 2015). This study found that Na+ and Cl- concentrations were significantly reduced on Day 1 in the 0, 5, and 10 salinity groups, then gradually increased and eventually stabilized. Na+, K+, and Cl- concentrations remained stable in the 15, 20, and 25 salinity groups, with no significant changes during the experimental period. In contrast, Na+, K+, and Cl- concentrations increased significantly on Day 1 in the 30 and 35 salinity groups, then gradually decreased. The ability of E. tetradactylum to adjust ion concentrations, particularly Na+, K+, and Cl-, under different salinity conditions further illustrates the species’ osmotic adaptability.
4.3 Metabolic response and oxidative enzymes response in serum
Osmoregulation is an energy-intensive process in fish, and metabolic indicators can provide insight into the metabolic status of fish under different salinity conditions (Urbina and Glover, 2015; Xu et al., 2016). Serum glucose (GLU) is a primary energy source during osmotic pressure regulation (Hoseini et al., 2019). In this study, the serum GLU content of E. tetradactylum in the 35 PSU group was significantly higher than that in the low salinity groups (0, 5, 10 PSU) and the control group (25 PSU) (P< 0.05). This increase is attributed to the fact that the salinity in the 35 PSU group was significantly higher than the iso-osmotic point of E. tetradactylum (10.88 PSU), which requires more energy for osmotic pressure regulation. As a result, glycogen in the body of E. tetradactylum is broken down into glucose and transferred to osmoregulatory tissues to assist in osmotic pressure regulation (Zhu et al., 2021), leading to an increase in serum GLU content. Tsui et al. (2012) observed that blood glucose levels in fish exposed to high salinity increased, but returned to normal after 60 minutes, possibly due to the short exposure time.
Triglycerides (TG) also play a critical role as energy substrates in the process of salinity adaptation in fish (Evans and Kültz, 2020). In this study, TG content showed a significant trend of initially decreasing, then increasing, with increasing salinity. The serum TG content in the 35 PSU group was relatively high, likely because under high salinity conditions, fish need to increase energy intake to meet the heightened energy demands for maintaining physiological balance due to water loss and salt absorption (Kim et al., 2021). Similar findings were observed in red-eared sliders (Trachemys scripta elegans), where plasma TG levels increased within two days of exposure to high salinity and gradually decreased thereafter (Hong et al., 2019). Furthermore, some studies have shown that TG may play a role in regulating the immune stress response of aquatic animals (Zhao et al., 2021; Zheng et al., 2022).
Lactic acid (LD) is the end product of anaerobic metabolism, and its content reflects the level of anaerobic metabolic activity (Omlin and Weber, 2010). In aquatic animals’ salinity adaptation, LD content typically increases with environmental salinity (Long et al., 2021). In this study, LD content in the 30 and 35 PSU salinity groups was significantly higher than in the 5 to 25 PSU groups (P< 0.05), with no significant differences observed among the 5 to 25 PSU groups (P > 0.05). Some studies have also found that low salinity can enhance anaerobic metabolism, leading to increased lactic acid production (Rodrigues et al., 2012). This could be due to the higher energy demand in low salinity environments as aquatic animals undergo adaptive regulatory processes.
Antioxidant and immune-related biomarkers are commonly employed to assess the adaptation of aquatic organisms to varying salinity conditions (Mozanzadeh et al., 2021). Under homeostatic conditions, there exists a balance between the generation and elimination of intracellular reactive oxygen species (ROS), including superoxide anions (O2-), hydrogen peroxide (H2O2), and hydroxyl radicals (OH) (Guillou et al., 2010). Disruption of this balance leads to the overproduction of ROS (Kim et al., 2017; Chang et al., 2021), which, if not adequately neutralized, can result in lipid peroxidation and the formation of malondialdehyde (MDA), a marker of oxidative damage (Lushchak, 2011). Elevated MDA levels can impair cellular function and activate antioxidant defenses, including the enzymes superoxide dismutase (SOD), catalase (CAT), and glutathione peroxidase (GPx), which collectively mitigate ROS-induced damage to the organism (Li et al., 2012). SOD catalyzes the conversion of superoxide anions to hydrogen peroxide, which is subsequently broken down into water and oxygen by CAT, thereby preventing lipid peroxidation (Tavares Sánchez et al., 2004). Previous studies have shown a pattern of increasing and then decreasing SOD activity in fish species such as yellowfin seabream (Acanthopagrus latus), Asian seabass (Lates calcarifer), and Rohu (Labeo rohita) across a salinity gradient (0 to 40 PSU) (Mozanzadeh et al., 2021; Patel et al., 2022).
In the present study, salinity acclimation in E. tetradactylum also induced oxidative stress, as evidenced by changes in antioxidant enzyme activity and lipid peroxidation markers. Superoxide dismutase (SOD) activity was significantly higher in the 10 PSU group compared to the 30 and 35 PSU groups, suggesting that moderate salinity promotes antioxidant defense, while higher salinities may overwhelm the antioxidant system, leading to reduced SOD activity (Patel et al., 2022). Similarly, glutathione peroxidase (GPx) activity decreased with increasing salinity, particularly at 35 PSU, further indicating oxidative stress under high salinity conditions.
MDA, a primary byproduct of ROS-induced lipid peroxidation, serves as an indicator of oxidative damage and the extent of cellular injury (Zuo et al., 2013; Long et al., 2017). However, malondialdehyde (MDA) levels did not show significant differences across salinity treatments, possibly because E. tetradactylum is able to adapt to prolonged salinity stress by regulating its oxidative balance (Li et al., 2022).
Antioxidant compounds represent the first line of defense against ROS, while antioxidant enzymes such as CAT, SOD, and GPx form the second line of defense in mitigating oxidative damage (Ighodaro and Akinloye, 2018). The total antioxidant capacity (T-AOC) exhibited a “U”-shaped response to increasing salinity, which suggests that E. tetradactylum can regulate its redox status to maintain cellular function within an optimal salinity range. This finding aligns with studies on black seabream (Acanthopagrus schlegelii) under acute salinity stress, where similar “U”-shaped trends in T-AOC activity were observed (Li et al., 2022). Additionally, Mo et al. (2020) reported a significant increase in T-AOC activity in Scapharca subcrenata prior to a subsequent decline, mirroring the pattern observed in the present study. These results suggest that high salinity conditions (35 PSU) are more likely to induce oxidative stress in E. tetradactylum. Overall, our findings indicate that different salinity levels exert distinct effects on antioxidant enzyme activities, with high salinity potentially disrupting the balance of the antioxidant system and compromising the organism’s physiological health.
5 Conclusions
In summary, this study highlights that juvenile E. tetradactylum exhibits significant physiological flexibility in adapting to varying salinity levels, which is crucial for their survival and growth in aquaculture environments. The results demonstrate that the optimal growth and survival of juveniles occur within the brackish water range of 5-10 PSU, where osmoregulatory processes are most efficient. Importantly, the isotonic point for E. tetradactylum was identified around 10 PSU (10.88 PSU), where the salinity closely matches the internal osmotic pressure, enabling better osmoregulation and metabolic efficiency. In contrast, exposure to extreme salinities, both low (0 PSU) and high (30-35 PSU), leads to severe physiological stress. This is reflected in impaired osmoregulation, growth retardation, and histopathological damage to critical organs such as the gills, intestines, and kidneys. Additionally, alterations in metabolic and antioxidant responses further highlight the detrimental effects of extreme salinities on the overall health of E. tetradactylum. These findings underscore the importance of maintaining stable and optimal salinity conditions in aquaculture practices to enhance the health, growth, and survival of this species. Proper salinity management will be essential for improving the sustainability and efficiency of E. tetradactylum farming, providing a foundation for future research on salinity tolerance mechanisms in marine aquaculture species.
Both of the two authors have made equally significant contributions to the work and share equal responsibility and accountability for it.
Data availability statement
The original contributions presented in the study are included in the article/supplementary material. Further inquiries can be directed to the corresponding author.
Ethics statement
The animal study was approved by This work was approved by the Care and Use of Laboratory Animals in theFisheries College of Guangdong Ocean University (approval number: GDOU-LAE-2023-054). The study was conducted in accordance with the local legislation and institutional requirements.
Author contributions
ZC: Conceptualization, Writing – original draft, Writing – review & editing, Formal Analysis, Methodology, Project administration, Validation, Visualization. WL: Conceptualization, Formal Analysis, Methodology, Project administration, Validation, Visualization, Writing – original draft, Writing – review & editing. ZW: Conceptualization, Formal Analysis, Methodology, Project administration, Resources, Validation, Visualization, Writing – original draft, Writing – review & editing. AZ: Formal Analysis, Investigation, Software, Validation, Writing – review & editing. MJ: Formal Analysis, Investigation, Software, Validation, Writing – review & editing. SH: Conceptualization, Formal Analysis, Investigation, Validation, Writing – review & editing. LZ: Conceptualization, Formal Analysis, Methodology, Validation, Writing – review & editing. ST: Formal Analysis, Methodology, Validation, Writing – review & editing. EM: Formal Analysis, Validation, Writing – review & editing. LW: Investigation, Methodology, Project administration, Supervision, Writing – review & editing. HJZ: Investigation, Methodology, Project administration, Supervision, Writing – review & editing. JL: Investigation, Methodology, Supervision, Validation, Writing – review & editing. HM: Project administration, Software, Validation, Writing – review & editing. BT: Project administration, Software, Validation, Writing – review & editing. HZ: Software, Validation, Writing – review & editing. BW: Formal Analysis, Investigation, Methodology, Project administration, Resources, Software, Validation, Writing – review & editing. JH: Investigation, Methodology, Supervision, Validation, Writing – review & editing.
Funding
The author(s) declare that financial support was received for the research and/or publication of this article. The research was financially supported by the Guangdong Province Ordinary Colleges and Universities Key Field Special Project (Science and Technology Services for Rural Revitalization) (2023ZDZX4011), the Research on breeding technology of candidate species for Guangdong modern marine ranching (2024-MRB-00-001), Guangdong Ocean University Aquaculture Excellent Young Talent Program (2024), the Guangdong Province Ordinary Colleges and Universities Special Innovative Project (2021KTSCX044), the Postgraduate Education Innovation Project of Guangdong Ocean University (202404), and the Guangdong Province Ordinary Colleges and Universities Innovation Team Projects (2021KCXTD026; 2022KCXTD013).
Conflict of interest
Authors SH and ST were employed by the company Zhuhai Longsheng Improved Fingerling Breeding Co., Ltd.
The remaining authors declare that the research was conducted in the absence of any commercial or financial relationships that could be construed as a potential conflict of interest.
Generative AI statement
The author(s) declare that no Generative AI was used in the creation of this manuscript.
Publisher’s note
All claims expressed in this article are solely those of the authors and do not necessarily represent those of their affiliated organizations, or those of the publisher, the editors and the reviewers. Any product that may be evaluated in this article, or claim that may be made by its manufacturer, is not guaranteed or endorsed by the publisher.
References
Ali A., Azom M. G., Sarker B. S., Rani H., Alam M. S., Islam M. S. (2024). Repercussion of salinity on hematological parameters and tissue morphology of gill and kidney at early life of tilapia. Aquac. Fish. 9 (2), 256–264. doi: 10.1016/j.aaf.2022.04.006
An K. W., Kim N. N., Choi C. Y. (2008). Cloning and expression of aquaporin 1 and arginine vasotocin receptor mRNA from the black porgy, Acanthopagrus schlegeli: effect of freshwater acclimation. Fish Physiol. Biochem. 34, 185–194. doi: 10.1007/s10695-007-9175-0
Ballagh A. C., Welch D. J., Newman S. J., Allsop Q., Stapley J. M. (2012). Stock structure of the blue threadfin (Eleutheronema tetradactylum) across northern Australia derived from life-history characteristics. Fisheries Res. 121–122, 63–72. doi: 10.1016/j.fishres.2012.01.011
Bøeuf G., Payan P. (2001). How should salinity influence fish growth? Comp. Biochem. Physiol. Part C: Toxicol. Pharmacol. 130, 411–423.
Cao D. Y., Li J. F., Huang B. S., Zhang J. D., Pan C. H., Huang J. S., et al. (2020). RNA-seq analysis reveals divergent adaptive response to hyper- and hypo-salinity in cobia, Rachycentron canadum. Fish Physiol. Biochem. 46, 1713–1727. doi: 10.1007/s10695-020-00823-7
Chang C. H., Wang Y. C., Lee T. H. (2021). Hypothermal stress-induced salinity-dependent oxidative stress and apoptosis in the livers of euryhaline milkfish, Chanos chanos. Aquaculture 534, 736280. doi: 10.1016/j.aquaculture.2020.736280
Cheng A. C., Liu P. C., Chin T. S., Wang S. B., Liu C. H. (2017). Growth and reproductive biology of the four finger threadfin, Eleutheronema tetradactylum (Shaw), in an aquaculture pond, Pingtung, Taiwan. J. Fisheries Socirty Taiwan 44, 23–35.
Chourasia T. K., D’Cotta H., Baroiller J. F., Slosman T., Cnaani A. (2018). Effects of the acclimation to high salinity on intestinal ion and peptide transporters in two tilapia species that differ in their salinity tolerance. Comp. Biochem. Physiol. Part A: Mol. Integr. Physiol. 218, 16–23. doi: 10.1016/j.cbpa.2018.01.004
Claireaux G., Lagardère J. P. (1999). Influence of temperature, oxygen and salinity on the metabolism of the European sea bass. J. Sea Res. 42, 157–168. doi: 10.1016/S1385-1101(99)00019-2
Dalziel A. C., Bittman J., Mandic M., Ou M., Schulte P. M. (2014). Origins and functional diversification of salinity-responsive Na+, K+ ATPase α1 paralogs in salmonids. Mol. Ecol. 23, 3483–3503. doi: 10.1111/mec.12828
Dawood M. A. O., Gewaily M. S., Sewilam H. (2022). The growth performance, antioxidative capacity, and histological features of intestines, gills, and livers of Nile tilapia reared in different water salinities and fed menthol essential oil. Aquaculture 554, 738122. doi: 10.1016/j.aquaculture.2022.738122
Dawood M. A. O., Noreldin A. E., Sewilam H. (2021). Long term salinity disrupts the hepatic function, intestinal health, and gills antioxidative status in Nile tilapia stressed with hypoxia. Ecotox Environ. Safe 220, 112412. doi: 10.1016/j.ecoenv.2021.112412
Dissanayake D. C. T. (2019). Milkfish Aquaculture in Sri Lanka: Progress, Challenges and Opportunities (Nnw Delhi: South Asian Association for Regional Cooperation).
Durigon E. G., Almeida A. P. G., Jerônimo G. T., Baldisserotto B., Emerenciano M. G. C. (2019). Digestive enzymes and parasitology of Nile tilapia juveniles raised in brackish biofloc water and fed with different digestible protein and digestible energy levels. Aquaculture 506, 35–41. doi: 10.1016/j.aquaculture.2019.03.022
Evans T. G., Kültz D. (2020). The cellular stress response in fish exposed to salinity fluctuations. J. Exp. Zool 333, 421–435. doi: 10.1002/jez.2350
Guillou H., Zadravec D., Martin P. G. P., Jacobsson A. (2010). The key roles of elongases and desaturases in mammalian fatty acid metabolism: Insights from transgenic mice. Prog. Lipid Res. 49, 186–199. doi: 10.1016/j.plipres.2009.12.002
Hong M. L., Li N., Li J. Y., Li W. H., Liang L. Y., Li Q., et al. (2019). Adenosine Monophosphate-Activated Protein Kinase Signaling Regulates Lipid Metabolism in Response to Salinity Stress in the Red-Eared Slider Turtle Trachemys scripta elegans. Front. Physiol. 10. doi: 10.3389/fphys.2019.00962
Hoseini S. M., Gharavi B., Iri Y. (2019). Assessment of vital organ histopathology and plasma oxidative conditions of rainbow trout Oncorhynchus mykiss reared in earthen saltwater pond. RUDN: AA 14, 255–265. doi: 10.22363/2312-797X-2019-14-3-255-265
Huang C. T., Afero F., Lu F. Y., Chen B. Y., Huang P. L., Lan H. Y., et al. (2022). Bioeconomic evaluation of Eleutheronema tetradactylum farming: a case study in Taiwan. Fisheries Sci. 88, 437–447. doi: 10.1007/s12562-022-01591-4
Hwang P. P., Lee T. H. (2007). New insights into fish ion regulation and mitochondrion-rich cells. Comp. Biochem. Physiol. Part A: Mol. Integr. Physiol. 148, 479–497. doi: 10.1016/j.cbpa.2007.06.416
Ighodaro O. M., Akinloye O. A. (2018). First line defence antioxidants-superoxide dismutase (SOD), catalase (CAT) and glutathione peroxidase (GPX): Their fundamental role in the entire antioxidant defence grid. Alexandria J. Med. 54, 287–293. doi: 10.4314/bafm.v54i4
Imsland A. K., Foss A., Gunnarsson S., Berntssen M. H., FitzGerald R., Bonga S. W., et al. (2001). The interaction of temperature and salinity on growth and food conversion in juvenile turbot (Scophthalmus maximus). Aquaculture 198, 353–367. doi: 10.1016/S0044-8486(01)00507-5
Iqbal T. H., Hajisamae S., Lim A., Jantarat S., Wang W. X., Tsim K. W. K. (2023). Feeding habits of four-finger threadfin fish, Eleutheronema tetradactylum, and its diet interaction with co-existing fish species in the coastal waters of Thailand. PeerJ 11, e14688. doi: 10.7717/peerj.14688
Kavadias S., Castritsi Catharios J., Dessypris A. (2003). Annual cycles of growth rate, feeding rate, food conversion, plasma glucose and plasma lipids in a population of European sea bass (Dicentrarchus labrax L.) farmed in floating marine cages. J. Appl. Ichthyology 19, 29–34. doi: 10.1046/j.1439-0426.2003.00346.x
Kim J. H., Jeong E. H., Jeon Y. H., Kim S. K., Hur Y. B. (2021). Salinity-mediated changes in hematological parameters, stress, antioxidant responses, and acetylcholinesterase of juvenile olive flounders (Paralichthys olivaceus). Environ. Toxicol. Phar 83, 103597. doi: 10.1016/j.etap.2021.103597
Kim J. H., Park H. J., Kim K. W., Hwang I. K., Kim D. H., Oh C. W., et al. (2017). Growth performance, oxidative stress, and non-specific immune responses in juvenile sablefish, Anoplopoma fimbria, by changes of water temperature and salinity. Fish Physiol. Biochem. 43, 1421–1431. doi: 10.1007/s10695-017-0382-z
Kültz D. (2015). Physiological mechanisms used by fish to cope with salinity stress. J. Exp. Biol. 218, 1907–1914. doi: 10.1242/jeb.118695
Lai J. C., Kam Y. C., Lin H. C., Wu C. S. (2019). Enhanced salt tolerance of euryhaline tadpoles depends on increased Na+, K+-ATPase expression after salinity acclimation. Comp. Biochem. Physiol. Part A: Mol. Integr. Physiol. 227, 84–91. doi: 10.1016/j.cbpa.2018.09.025
Li Y., Li M., Shi J. Q., Yang X., Wang Z. Y. (2012). Hepatic antioxidative responses to PCDPSs and estimated short-term biotoxicity in freshwater fish. Aquat. Toxicol. 120–121, 90–98. doi: 10.1016/j.aquatox.2012.04.016
Li X. J., Shen Y. D., Bao Y. G., Wu Z. X., Yang B. Q., Jiao L. F., et al. (2022). Physiological responses and adaptive strategies to acute low-salinity environmental stress of the euryhaline marine fish black seabream (Acanthopagrus schlegelii). Aquat. Toxicol. 554, 738117. doi: 10.1016/j.aquaculture.2022.738117
Long J., Cui Y., Wang R., Chen Y., Zhao N., Wang C., et al. (2021). Combined effects of high salinity and ammonia-N exposure on the energy metabolism, immune response, oxidative resistance and ammonia metabolism of the Pacific white shrimp Litopenaeus vannamei. Aquaculture Rep. 20, 100648. doi: 10.1016/j.aqrep.2021.100648
Long X., Wu X., Zhao L., Liu J., Cheng Y. (2017). Effects of dietary supplementation with Haematococcus pluvialis cell powder on coloration, ovarian development and antioxidation capacity of adult female Chinese mitten crab, Eriocheir sinensis. Aquaculture 473, 545–553. doi: 10.1016/j.aquaculture.2017.03.010
Luo H. Z., Li W. Y., Fu R. B., Liu M. H., Xu Y. A., You J. J., et al. (2015). The effects of salinity on the growth of juvenile eleutheronema tetradactylum and na+/K+-ATP enzyme. Prog. Fishery Sci. 36, 94–99. doi: 10.11758/yykxjz.20150212
Lushchak V. I. (2011). Environmentally induced oxidative stress in aquatic animals. Aquat Toxicol. 101, 13–30. doi: 10.1016/j.aquatox.2010.10.006
Luz R. K., Martínez-Álvarez R. M., Pedro N. D., Delgado M. J. (2008). Growth, food intake regulation and metabolic adaptations in goldfish (Carassius auratus) exposed to different salinities. Aquaculture 276, 171–178. doi: 10.1016/j.aquaculture.2008.01.042
Mo Z., Li L., Ying L., Xiaolong G. (2020). Effects of sudden drop in salinity on osmotic pressure regulation and antioxidant defense mechanism of Scapharca subcrenata. Front. Physiol. 11. doi: 10.3389/fphys.2020.00884
Motomura H., Iwatsuki Y., Kimura S., Yoshino T. (2002). Revision of the Indo-West Pacific polynemid fish genus Eleutheronema (Teleostei: Perciformes). Ichthyol Res. 49, 47–61. doi: 10.1007/s102280200005
Motomura H. (2004). Threadfins of the world (Family Polynemidae): An annotated and illustrated catalogue of polynemid species known to date (No. 3) (Food & Agriculture Org).
Moutou K. A., Panagiotaki P., Mamuris Z. (2004). Effects of salinity on digestive protease activity in the euryhaline sparid Sparus aurata L.: a preliminary study. Aquac Res. 35, 912–914. doi: 10.1111/j.1365-2109.2004.01068.x
Mozanzadeh M. T., Safari O., Oosooli R., Mehrjooyan S., Najafabadi M. Z., Hoseini S. J., et al. (2021). The effect of salinity on growth performance, digestive and antioxidant enzymes, humoral immunity and stress indices in two euryhaline fish species: Yellowfin seabream (Acanthopagrus latus) and Asian seabass (Lates calcarifer). Aquaculture 534, 736329. doi: 10.1016/j.aquaculture.2020.736329
Niu Y. Y., Luo Z. P., Ou Y. J., Lan J. N., Wen J. F., Li J. E., et al. (2021). The survival and structural changes in gill of juvenile Eleutheronema tetradactylum under different salinities. J. South. Agric. China 52, 1719–1726.
O’Malley K. G., Ford M. J., Hard J. J. (2010). Clock polymorphism in Pacific salmon: evidence for variable selection along a latitudinal gradient. Proc. R. Soc. B: Biol. Sci. 277, 3703–3714. doi: 10.1098/rspb.2010.0762
Okamura A., Yamada Y., Mikawa N., Horie N., Utoh T., Kaneko T., et al. (2009). Growth and survival of eel leptocephali (Anguilla japonica) in low-salinity water. Aquaculture 296, 367–372. doi: 10.1016/j.aquaculture.2009.08.039
Omlin T., Weber J. M. (2010). Hypoxia stimulates lactate disposal in rainbow trout. J. Exp. Biol. 213, 3802–3809. doi: 10.1242/jeb.048512
Parsegian V. A., Rand R. P., Rau D. C. (2000). Osmotic stress, crowding, preferential hydration, and binding: A comparison of perspectives. Proc. Natl. Acad. Sci. 97, 3987–3992. doi: 10.1073/pnas.97.8.3987
Patel R. K., Verma A. K., Krishnani K. K., Sreedharan K., Chandrakant M. H. (2022). Growth performance, physio-metabolic, and haemato-biochemical status of Labeo rohita (Hamilton 1822) juveniles reared at varying salinity levels using inland saline groundwater. Aquaculture 559, 738408. doi: 10.1016/j.aquaculture.2022.738408
Pearse D. E., Miller M. R., Abadía-Cardoso A., Garza J. C. (2014). Rapid parallel evolution of standing variation in a single, complex, genomic region is associated with life history in steelhead/rainbow trout. P Roy Soc. B-biol Sci. 281, 20140012. doi: 10.1098/rspb.2014.0012
Pember M. B. (2006). Characteristics of fish communites in coastal waters of north-western Australia, including the biology of the threadfin species Eleutheronema tetradactylum and Polydactylus macrochir (Murdoch University).
Qu Z., Nong W. Y., Yu Y. F., Baril T., Yip H. Y., Hayward A., et al. (2020). Genome of the four-finger threadfin Eleutheronema tetradactylum (Perciforms: Polynemidae). BMC Genomics 21, 1–10. doi: 10.1186/s12864-020-07145-1
Rodrigues A. P., Oliveira P. C., Guilhermino L., Guimarães L. (2012). Effects of salinity stress on neurotransmission, energy metabolism, and anti-oxidant biomarkers of Carcinus maenas from two estuaries of the NW Iberian Peninsula. Mar. Biol. 159, 2061–2074. doi: 10.1007/s00227-012-1992-8
Rodriguez A., Gallardo M. A., Gisbert E., Santilari S., Ibarz A., Sanchez J., et al. (2002). Osmoregulation in juvenile Siberian sturgeon (Acipenser baerii). Fish Physiol. Biochem. 26, 345–354.
Semra K., Karul A., Yildirim Ş., Gamsiz K. (2013). Effects of salinity on growth and metabolism in blue tilapia (Oreochromis aureus). Afr. J. Biotechnol. 12 (19).
Shaughnessy C. A., McCormick S. D. (2020). Functional characterization and osmoregulatory role of the Na+-K+-2Cl– cotransporter in the gill of sea lamprey (Petromyzon marinus), a basal vertebrate. Am. J. Physiol-reg I 318, R17–R29. doi: 10.1152/ajpregu.00125.2019
Stiller K. T., Kolarevic J., Lazado C. C., Gerwins J., Good C., Summerfelt S. T., et al. (2020). The effects of ozone on Atlantic salmon post-smolt in brackish water—Establishing welfare indicators and thresholds. Int. J. Mol. Sci. 21, 5109. doi: 10.3390/ijms21145109
Sun M. L., Jiang J. L., Wang L. P., Chen F., Han Y. Z., Jiang Z. Q., et al. (2016). Structural Changes in Gill, Kidney and Intestine of Juvenile Takifugu rubripes under Low Salinity Treatment. J. Guangdong Ocean Univ. China 36, 38–43.
Takata R., Mattioli C. C., Bazzoli N., Júnior J. D. C., Luz R. K. (2021). The effects of salinity on growth, gill tissue and muscle cellularity in Lophiosilurus alexandri juvenile, a Neotropical freshwater catfish. Aquaculture Res. 52, 4064–4075. doi: 10.1111/are.15244
Tavares Sánchez O. L., Gómez Anduro G. A., Felipe Ortega X., Islas Osuna M. A., Sotelo Mundo R. R., Barillas Mury C., et al. (2004). Catalase from the white shrimp Penaeus (Litopenaeus) vannamei: molecular cloning and protein detection. Comp. Biochem. Physiol. Part B: Biochem. Mol. Biol. 138, 331–337. doi: 10.1016/j.cbpc.2004.03.005
Tomy S., Chang Y. M., Chen Y. H., Cao J. C., Wang T. P., Chang C. F. (2009). Salinity effects on the expression of osmoregulatory genes in the euryhaline black porgy Acanthopagrus schlegeli. Gen. Comp. Endocrinol. 161, 123–132. doi: 10.1016/j.ygcen.2008.12.003
Tsui W. C., Chen J. C., Cheng S. Y. (2012). The effects of a sudden salinity change on cortisol, glucose, lactate, and osmolality levels in grouper Epinephelus malabaricus. Fish Physiol. Biochem. 38, 1323–1329. doi: 10.1007/s10695-012-9620-6
Urbina M. A., Glover C. N. (2015). Effect of salinity on osmoregulation, metabolism and nitrogen excretion in the amphidromous fish, inanga (Galaxias maculatus). J. Exp. Mar. Biol. Ecol. 473, 7–15. doi: 10.1016/j.jembe.2015.07.014
Usher M. L., Talbot C., Eddy F. B. (1988). Drinking in Atlantic salmon smolts transferred to seawater and the relationship between drinking and feeding. Aquaculture 73, 237–246. doi: 10.1016/0044-8486(88)90058-0
Wang Z. L., Liu F. B., Huang B. S., Li G. Y., Wang J., Wang B., et al. (2023). Cloning and expression analysis of NHE3 and NKAα1a in cobia (Rachycentron canadum) and targeted regulation of related miRNA. J. fisheries China 47, 41–54. doi: 10.11964/jfc.20211213209
Wang J. J., Sun P., Yin F. (2014). Low mtDNA Cytb diversity and shallow population structure of Eleutheronema tetradactylum in the East China Sea and the South China Sea. Biochem. Syst. Ecol. 55, 268–274. doi: 10.1016/j.bse.2014.03.026
Watanabe W. O., Burnett K. M., Olla B. L., Wicklund R. I. (1989). The effects of salinity on reproductive performance of Florida red tilapia. J. World Aquacult Soc. 20, 223–229. doi: 10.1111/j.1749-7345.1989.tb01006.x
Whittamore J. M. (2012). Osmoregulation and epithelial water transport: lessons from the intestine of marine teleost fish. J. Comp. Physiol. B 182, 1–39. doi: 10.1007/s00360-011-0601-3
Xie M. J., Ou Y. J., Wen J. F., LI J. E., Wang P. F., Zhou H., et al. (2016). Salinity tolerance of fertilized eggs and larva of Eleutheronema tetradactylum. Chin. J. Ecol. 35, 1263–1267. doi: 10.13292/j.1000-4890.201605.007
Xu Z., Li E., Xu C., Gan L., Qin J. G., Chen L. (2016). Response of AMP-activated protein kinase and energy metabolism to acute nitrite exposure in the Nile tilapia Oreochromis niloticus. Aquat. Toxicol. 177, 86–97. doi: 10.1016/j.aquatox.2016.05.020
Yamada U., Shirai S., Irie T., Tokimura M., Deng S., Zheng Y., et al. (1995). Names and illustrations of fishes from the East China Sea and the Yellow Sea: Japanese Chinese Korean (Tokyo: Overseas Fishery Cooperation Foundation).
Zamidi I., Samat A., Zaidi C. C., Mazlan A. G., Gazi M. A., Abul Q., et al. (2012). Fecundity and temporal reproductive cycle of four finger threadfin (Eleutheronema tetradactylum) in Malaysian coastal water. Asian J. Anim. Veterinary Adv. 7, 1100–1109. doi: 10.3923/ajava.2012.1100.1109
Zhang W., Belton B., Edwards P., Henriksson P. J. G., Little D. C., Newton R., et al. (2022). Aquaculture will continue to depend more on land than sea. Nature 603, E2–E4. doi: 10.1038/s41586-021-04331-3
Zhang C. J., Shi Z. H., Wang J. G., Gao Q. X. (2013). On salinity-related effects on osmoregulation mechanism in marine teleost. Mar. Fisheries 35, 108–116. doi: 10.13233/j.cnki.mar.fish.2013.01.015
Zhang B., Sun Y., Shi G. (2014). The complete mitochondrial genome of the fourfinger threadfin Eleutheronema tetradactylum (Perciforms: Polynemidae) and comparison of light strand replication origin within Percoidei. Mitochondrial DNA 25, 411–413. doi: 10.3109/19401736.2013.809433
Zhao H. X., Peng K., Wang G. X., Mo W. Y., Huang Y. H., Cao J. M. (2021). Metabolic changes, antioxidant status, immune response and resistance to ammonia stress in juvenile yellow catfish (Pelteobagrus fulvidraco) fed diet supplemented with sodium butyrate. Aquaculture 536, 736441. doi: 10.1016/j.aquaculture.2021.736441
Zhao F., Zhuang P., Zhang L. Z., Huang X. R., Tian H. J., Zhang T., et al. (2006). The influence of salinity acclimation on activity of Na+/K+-ATPase in branchial epithelium, concentration of ions and osmolarity in serum of Acipenser schrenckii. J. fisheries China 30, 444–449.
Zheng T., Song Z., Tao Y. F., Qiang J., Ma J., Lu S. Q., et al. (2022). Transport stress induces innate immunity responses through TLR and NLR signaling pathways and increases mucus cell number in gills of hybrid yellow catfish (Tachysurus fulvidraco ♀ × Pseudobagrus vachellii ♂). Fish Shellfish Immun. 127, 166–175. doi: 10.1016/j.fsi.2022.06.015
Zhu J. H., Wang X. D., Bu X. Y., Wang C. L., Pan J. Y., Li E. C., et al. (2021). Relationship between myo-inositol synthesis and carbohydrate metabolism changes in Mozambique tilapia (Oreochromis mossambicus) under acute hypersaline stress. Aquaculture 532, 736005. doi: 10.1016/j.aquaculture.2020.736005
Zuo R. T., Ai Q. H., Mai K. S., Xu W. (2013). Effects of conjugated linoleic acid on growth, non-specific immunity, antioxidant capacity, lipid deposition and related gene expression in juvenile large yellow croaker (Larmichthys crocea) fed soyabean oil-based diets. Brit J. Nutr. 110, 1220–1232. doi: 10.1017/S0007114513000378
Keywords: Eleutheronema tetradactylum, salinity, growth, osmoregulation, metabolism
Citation: Chen Z, Liu W, Zheng A, Jin M, Huang S, Zhao L, Tang S, Moses Mkulo E, Wang L, Zhang H, Lu J, Mwemi HM, Tang B, Zhou H, Wang B, Huang J and Wang Z (2025) Effects of salinity on growth, survival, tissue structure, osmoregulation, metabolism, and antioxidant capacity of Eleutheronema tetradactylum (Shaw, 1804). Front. Mar. Sci. 12:1553114. doi: 10.3389/fmars.2025.1553114
Received: 30 December 2024; Accepted: 27 March 2025;
Published: 22 April 2025.
Edited by:
Ivana Veneza, Federal University of Western Pará, BrazilReviewed by:
Yunfei Sun, Shanghai Ocean University, ChinaAmit Ranjan, Tamil Nadu Fisheries University, India
Copyright © 2025 Chen, Liu, Zheng, Jin, Huang, Zhao, Tang, Moses Mkulo, Wang, Zhang, Lu, Mwemi, Tang, Zhou, Wang, Huang and Wang. This is an open-access article distributed under the terms of the Creative Commons Attribution License (CC BY). The use, distribution or reproduction in other forums is permitted, provided the original author(s) and the copyright owner(s) are credited and that the original publication in this journal is cited, in accordance with accepted academic practice. No use, distribution or reproduction is permitted which does not comply with these terms.
*Correspondence: Zhongliang Wang, d2FuZ3psQGdkb3UuZWR1LmNu