- 1Hubei Key Laboratory of Marine Geological Resources, China University of Geosciences, Wuhan, China
- 2Department of Earth, Energy, and Environment, University of Calgary, Calgary, AB, Canada
Instantaneous petroleum charge is uncommon in sedimentary basins, particularly in the Tarim Basin, where petroleum has accumulated from multiple source rocks with multiple episode charge stages. In the present study, an instantaneous petroleum charge was identified within the No.3 fault zone of the Shunbei reservoirs in the Tarim Basin. The oil from Well Shunbei 3 (SHB3 oil) is considered as an end member close to the stratigraphic maturity of source rock at a certain maturity level with evidence from n-alkanes, isoprenoids, steroids, terpenoids, diamondoids and a series of aromatic hydrocarbons. In comparison to oil samples from the No.1 fault zone, SHB3 oil did not receive the early-charged oil whereas it has not undergone petroleum charging during the very late maturation stages. The end member of instantaneous petroleum charge in Shunbei reservoirs provides valuable insights into petroleum generation, expulsion and charge histories of ultra-deep Ordovician reservoirs in the Tarim Basin.
1 Introduction
Petroleum in reservoirs (hereafter referred to as reservoired petroleum) typically contains a complex mixture of varying maturities due to the intricate processes of petroleum generation and accumulation. Petroleum generation, as widely recognized, involves the transformation of solid organic matter within source rocks into liquid or gaseous hydrocarbons through temperature-induced processes (Tissot et al., 1987). According to Huang et al. (2022), the entire petroleum generation process within a suit of source rock might span a few million years or a few tens of millions of years given the geological heating rates of 1–10°C/My for a typical petroleum system. As the maturity of source rocks increases over geological time or burial depth, the expelled petroleum charging into the reservoir is more and more mature provided there is no interruption. However, it is common that petroleum from multiple source rocks could accumulate into the same reservoir with multiple episode charge stages in sedimentary basins. Additionally, secondary alterations, such as biodegradation, thermochemical sulfate reduction (TSR), evaporative fractionation and thermal cracking, can modify the original petroleum compositions (Larter and Aplin, 1995; Horstad and Larter, 1997; Wilhelms and Larter, 2004; Lerch et al., 2016).
Thermal maturity refers to the degree of petroleum generation from source rocks over a geological timescale, a concept often described as “kinetic maturity” (Ungerer and Pelet, 1987). While source rocks and oils are two commonly objects for thermal maturity assessment, they represent distinct maturity concepts. The maturity of source rocks is typically determined by vitrinite reflectance on organic-rich sediments using petrography-based methods. This can be referred to as “stratigraphic maturity” (Huang et al., 2022), which usually records the maximum temperature of the sedimentary basin and the highest maturity level that source rocks have reached (Mukhopadhyay, 1994). In contrast to stratigraphic maturity, the thermal maturity of reservoired petroleum is distinct due to a time-dependent process of petroleum expulsion, migration and secondary alterations. Oil expulsion from source rocks typically occurs continuously over millions of years (Huang et al., 2022). In basins with multiple charging periods, reservoired petroleum often represents a mixture of compounds with a broad range of stratigraphic maturity, rather than the final trapped fractions. As a result, it is challenging to assess the thermal maturity of reservoired petroleum using a single maturity value, as is done for the stratigraphic maturity of source rocks. Under such circumstances, the concept of “mass fraction maturity” was introduced which reflects the cumulative relationship on fractional masses of petroleum generated and expelled from source rocks of varied stratigraphic maturity over geological times, incorporating the effects of charging histories (Wilhelms and Larter, 2004; Huang et al., 2022).
An instantaneous petroleum charge was initially regarded as the petroleum in the source rock, which is effectively at a maturity level equivalent to the stratigraphic maturity of the source rock. In most cases, reservoired oils represent continuous and cumulative petroleum wherein each molecular component exhibits a distinct concentration profile corresponding to the stratigraphic maturity of source rocks (Huang et al., 2022). Biomarkers, such as terpanoids and steroids, are abundant in immature and marginally mature oils but decline sharply during the early oil generation stage, eventually becoming undetectable at high maturity levels (Wilhelms and Larter, 2004). Consequently, maturity estimated by biomarkers is the minimum maturity at the time of expulsion. In contrast, aromatic hydrocarbons, which are more likely retained in the source rocks during petroleum expulsion, are indicative of maximum maturity levels (Huang et al., 2022). However, reservoired petroleum could be considered as an instantaneous petroleum charge when oil expelled from a source rock over a very narrow maturity range, an uncommon phenomenon in sedimentary basins. In this study, an end member of instantaneous petroleum charge is proposed in the Tarim Basin, to provide insights into the geochemical property of individual petroleum charge as well as hydrocarbon accumulation of ultra-deep Ordovician reservoirs.
2 Geological settings
The Tarim Basin is a typical petroliferous basin with multiple charge stages and complex mixing scenarios in northwest China. Since Phanerozoic, the basin has experienced significant tectonic activities. Regional extension occurred in the Tarim Basin from the Early Paleozoic to the Middle Ordovician (Jia, 1997). Large-scale central paleo-uplift and the northern depression were developed at the end of the Middle Ordovician, including the Shuntuoguole low uplift, the Awati depression, and the Manjiaer depression (Qiu et al., 2019). From the Middle to Late Ordovician, the strata witnessed erosion due to uplift in the Tazhong area, whereas it was a platform-slope in the Tabei area (Deng et al., 2022). The Tabei area uplifted and eroded at the end of Ordovician and the Middle Devonian. During the Permian, Tazhong uplift remained relatively stable whereas the Tabei uplift suffered intensive uplift and erosion. Therefore, early-charged petroleum witnessed various secondary alterations and multi-charging periods, resulting in complicated geochemical profiles of reservoired oils (Zhu et al., 2012; Zhang et al., 2014). It remains challenging to figure out an end member of instantaneous petroleum charge that can be correlated to a specific stratigraphic maturity to unravel the geochemical signatures of individual charging episode.
The Shunbei area, crucial ultra-deep petroleum reservoirs in the Tarim Basin, located in the Shuntuoguole low uplift which is surrounded by the Tabei uplift, the Tazhong uplift, the Awati depression, and the Manjiaer depression (Figure 1a). Numerous research has been conducted on liquid petroleum accumulation and preservation within reservoirs of depth below 8,000 m. Currently, petroleum in the Shunbei reservoirs is primarily generated from individual source rocks discontinuously in the Cambrian Yuertusi Formation (Zhang and Huang, 2005; Zhang et al., 2005, 2011) during the Caledonian, Hercynian, Yanshanian and/or Himalayan periods (Wu et al., 2023; Yang et al., 2024). Petroleum has accumulated in the Ordovician Yingshan (O1-2y) and Yijianfang (O2yj) formations where limestone predominates in the sediments. Starting from the latest Middle Ordovician, strike-slip faults of multiphase activities formed as reservoir-scale fault damage zones in the Lower–Middle Ordovician carbonates and cut through the Lower Cambrian source rocks. Muti-phase activities of strike-slip faults in reservoir-scale fault damage zones are well developed in the Shunbei area (Qiu et al., 2019; Wu et al., 2019; Jiang et al., 2024), which play a critical role in facilitating petroleum migration and accumulation (Deng et al., 2022; Liu et al., 2023, 2024). The thick mudstones performed as cap rock in the Upper Ordovician (Figure 1c).
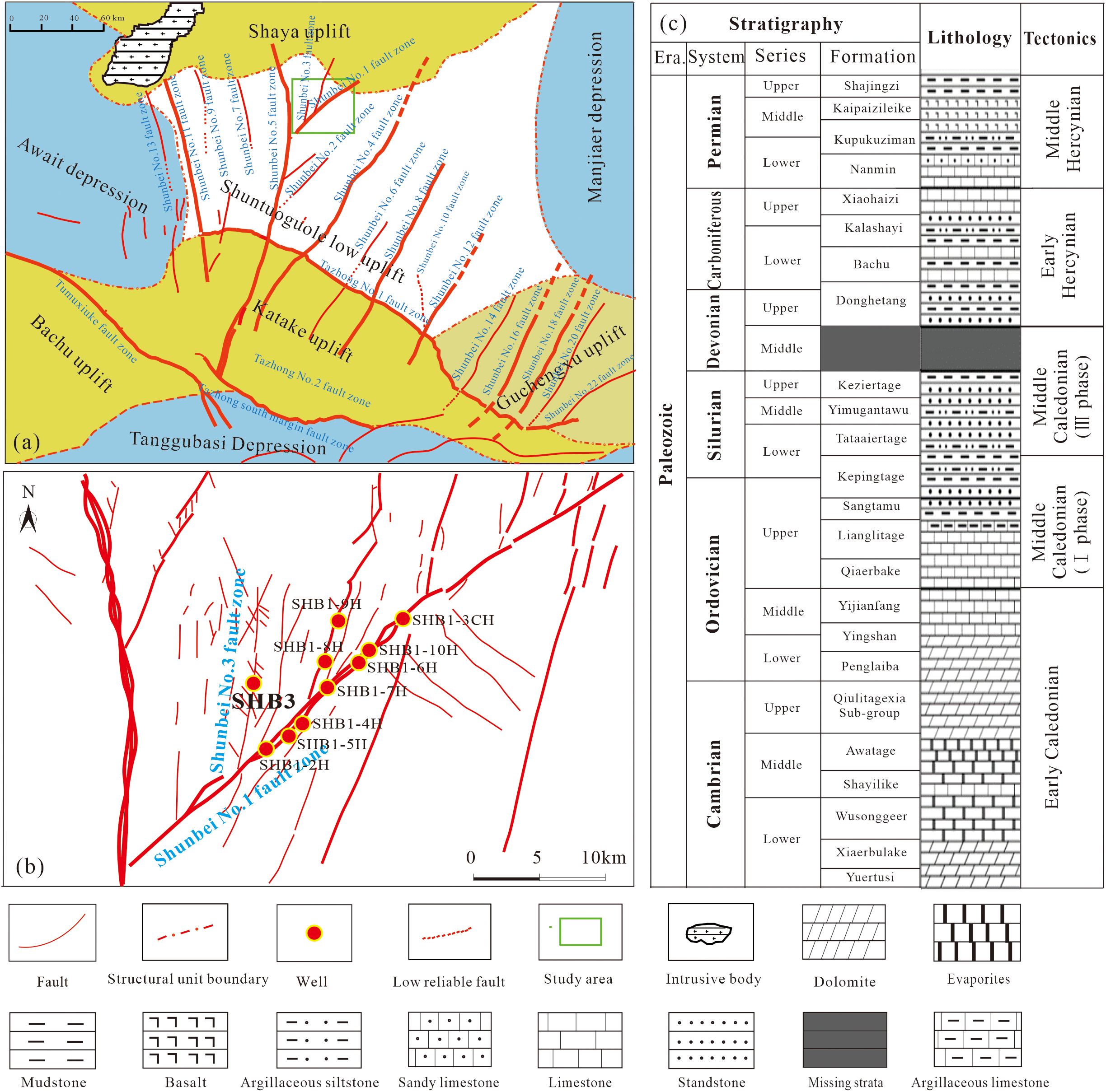
Figure 1. Geological settings. (a) Strike-slip faults in the northern Tarim Basin (modified after Liu, 2020). (b) Sample locations. (c) The general Paleozoic stratigraphy of the Shunbei area, Tarim Basin (modified after Deng et al., 2022).
3 Samples and methods
3.1 Samples
An oil sample from Well Shunbei 3 (SHB3), located in the No.3 fault zone in the Shunbei area, was collected in the Ordovician Yingshan Formation at a depth of 7,518.8–7,891.3 m (Figure 1b). In comparison, ten Ordovician oil samples were collected from the No.1 fault zone in Shunbei reservoirs at a depth of 7,229.5−8,225.4 m (Figure 1b). These samples are typical volatile oils from ultra-deep carbonate reservoirs in the Shunbei area. The oils exhibit high API gravity ranging from 42.27° to 47.84° (Table 1). To avoid light hydrocarbon volatilization, all the samples were sealed in amber airtight vials and stored under refrigeration prior to experimentation.
3.2 Gas chromatography-mass spectrometry analysis
Petroleum composition analysis was conducted in the PRG lab, University of Calgary. First, asphaltenes were removed from the oil samples on a polar Florisil solid phase extraction (SPE) cartridge by hexane and dichloromethane to obtain total hydrocarbon. The total hydrocarbon was then fractionated into the saturated and aromatic hydrocarbon fractions by solvents including pentane, dichloromethane and isopropyl alcohol in a custom-made silica gel column. A suite of internal standards including squalane, cholestane-d4, adamantane-d16, naphthalene-d8, phenanthrene-d10 and 1,1′-binaphthyl, was added in the oil samples to enable quantification of petroleum compositions. Finally, the saturated and aromatic hydrocarbons were performed on an Agilent 7890B gas chromatograph linked to an Agilent 5977A MSD system. Instrument settings and operating procedures followed those described by Wang et al. (2021a).
4 Results
4.1 n-Alkanes and isoprenoids
The oils from both the No.1 and No.3 fault zones contain abundant n-alkanes and isoprenoids (Figure 2). Although biodegradation is common in the Tarim Basin, particularly in Silurian reservoirs in the Tazhong area (Wang et al., 2020), the analyzed samples from the Shunbei area appear to show no signs of biodegradation.
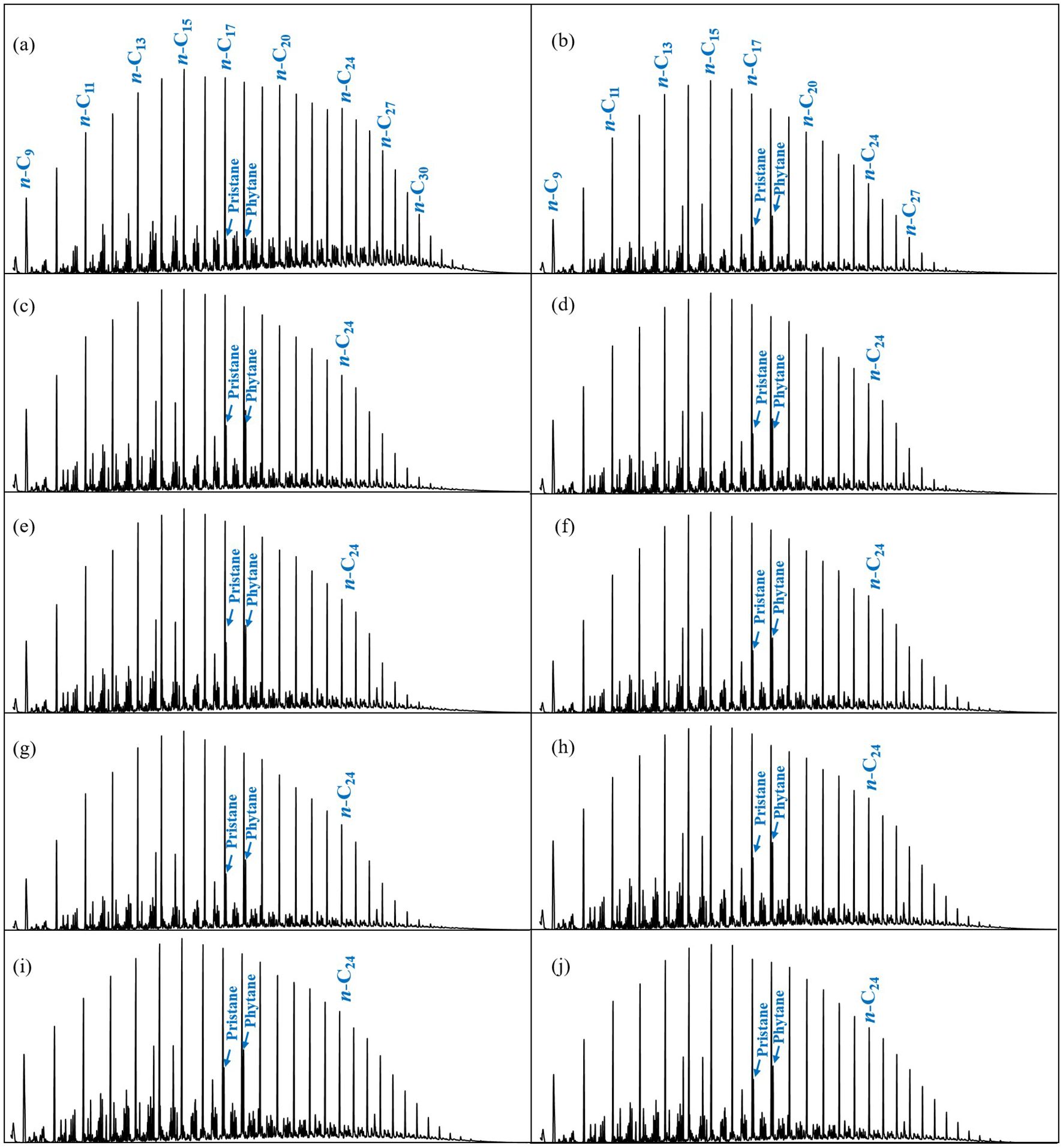
Figure 2. Mass chromatograms of m/z 85 showing occurrences of n-alkanes and isoprenoids. (a) SHB3 oil, O2yj. (b) SHB1-2H oil, O2yj. (c) SHB1-3CH oil, O2yj. (d) SHB1-4H oil, O2yj. (e) SHB1-5H oil, O2yj. (f) SHB1-6H oil, O2yj. (g) SHB1-7H oil, O2yj. (h) SHB1-8H oil, O1-2y. (i) SHB1-9 oil, O1-2y. (g) SHB1-10 oil, O1-2y.
However, noteworthy difference occurred to n-alkane and isoprenoid distribution patterns between oil samples from the No.1 and No.3 fault zones (Figure 2). Oil samples from the No.1 fault zone, exhibit similar n-alkane and isoprenoid distribution patterns, with low carbon n-alkanes dominating over relatively higher carbon n-alkanes (Figure 3). The n-C20-/n-C21+ ratios of oil samples from the No.1 fault zone range from 2.25 to 3.00 (Table 1). The isoprenoids, pristane and phytane, are in obvious lower concentrations compared to the adjacent n-alkanes, with Ph/n-C18 and Pr/n-C17 ratios in the ranges of 0.42–0.48, and 0.29–0.33, respectively (Table 1). In contrast, SHB3 oil has a noteworthy predominance of high-carbon-number n-alkanes (> C19) but less abundant light n-alkanes (< C16) (Figure 3) with a relatively lower n-C20-/n-C21+ ratio (Table 1). Additionally, SHB3 oil is characterized by low concentrations of pristane and phytane, with low Ph/n-C18 and Pr/n-C17 ratios below 0.15 (Table 1).
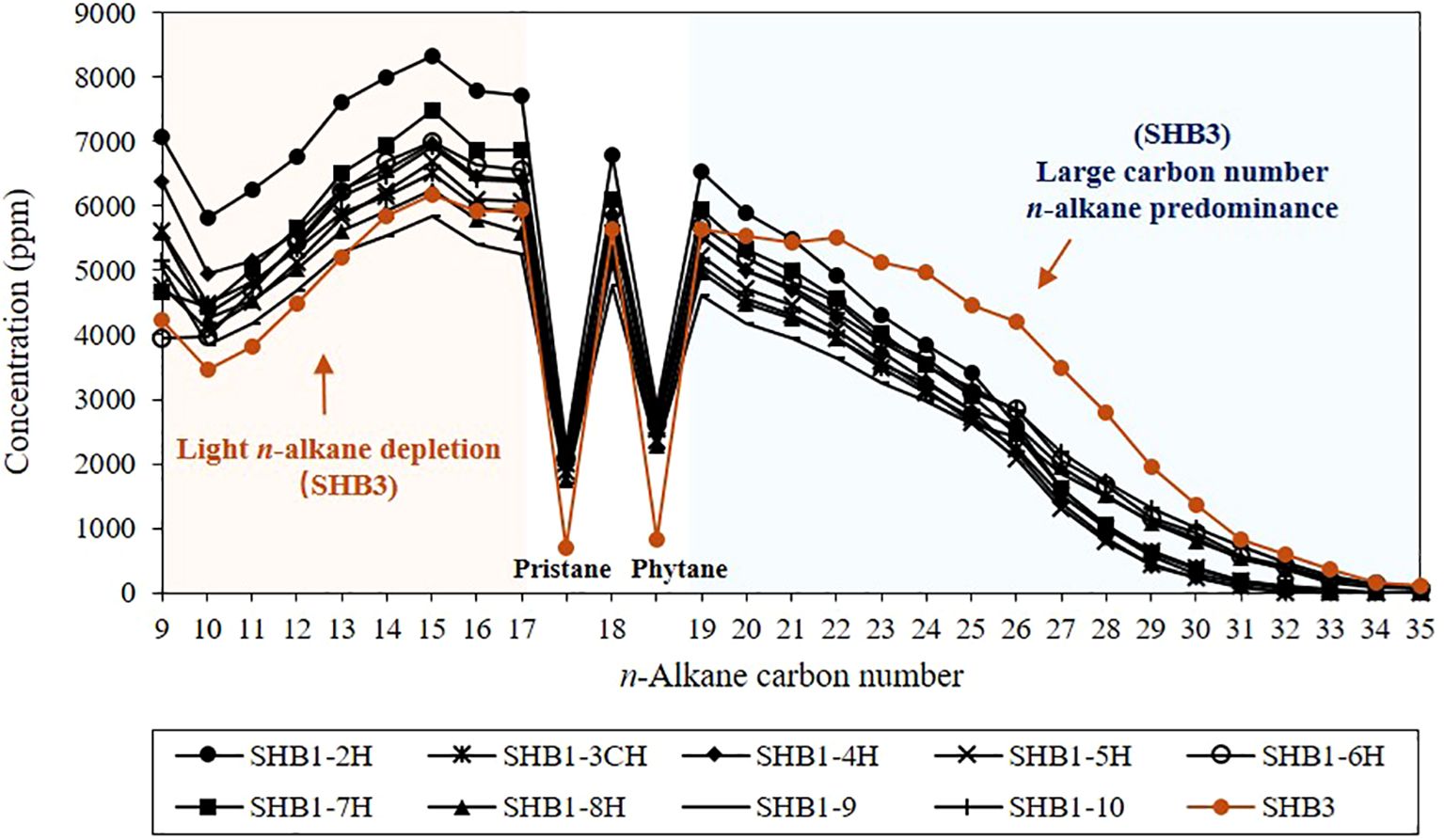
Figure 3. n-Alkane and isoprenoid concentration and distribution of oil samples from the No.1 and No.3 fault zones.
4.2 Steroids and terpenoids
Steroids and terpenoids are common biomarkers in crude oils with compound identification and abbreviation shown in Table 2. However, significant discrepancy occurs in steroid and terpenoid distribution patterns between oils from the No.1 and No.3 fault zones. In the oil samples from the No.1 fault zone, steroids are present but generally in low concentrations (Figure 4a), typically below 10 ppm, with C21 pregnane being relatively higher in a range of 13–17 ppm. The concentrations of regular and rearranged steranes show little variation among these oil samples (Figure 5a). Maturity parameters derived from steroids including C29 20S/(20S + 20R) and C29 ββ/(αα + ββ) fall in narrow ranges, i.e., 0.42–0.50 and 0.45–0.54 (Table 1), respectively, implying similar maturity levels of these oil samples. In contrast, SHB3 oil is absent from steroids (Figure 5a).
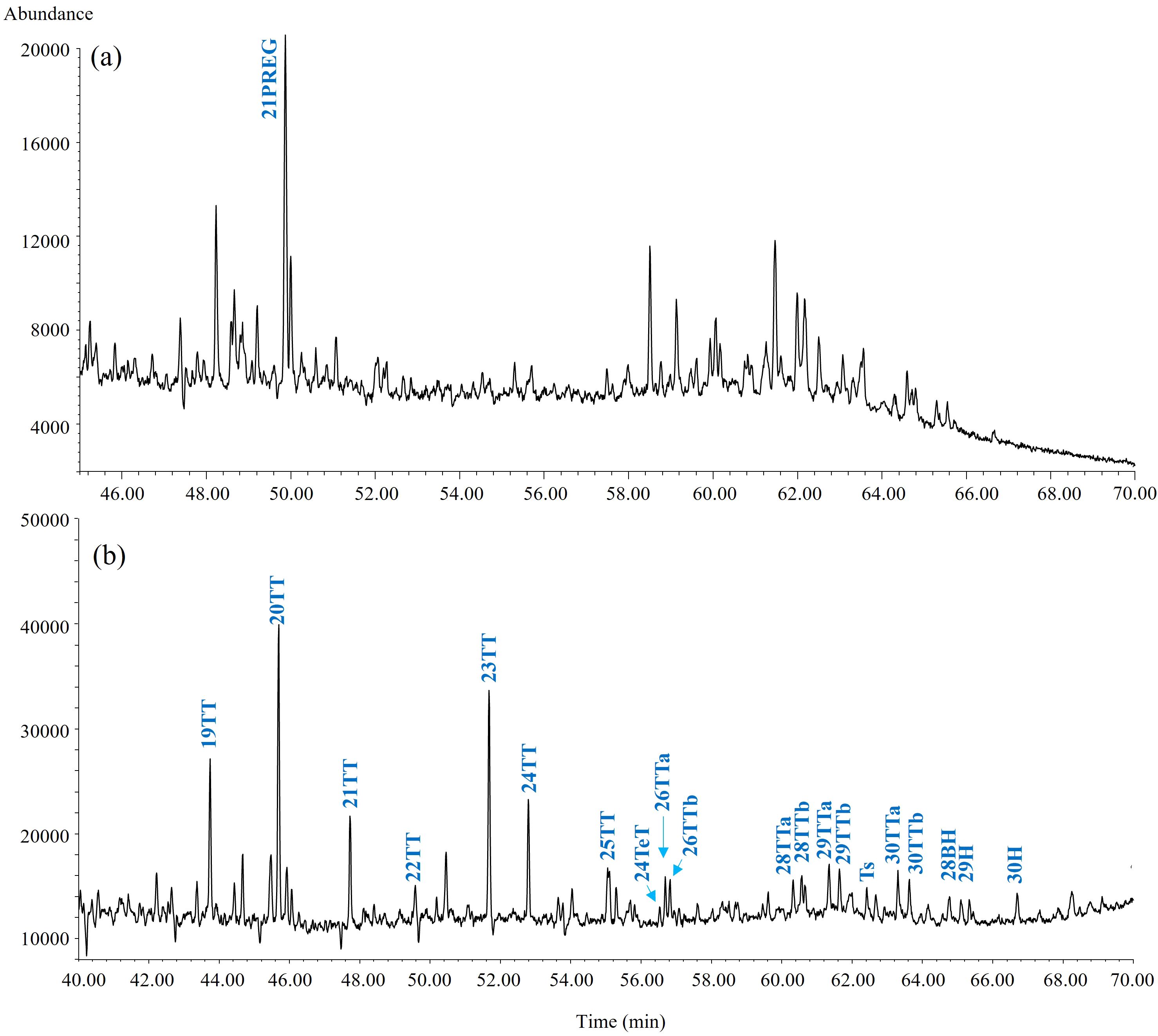
Figure 4. Partial mass chromatograms of m/z 217 and 191 showing typical distribution of steroids and terpenoids of SHB1-7H oil, respectively. (a) Steroids. (b) Terpenoids. PREG, pregnane; TT, tricyclic terpanes; TeT, tetracyclic terpane; PT, pentacyclic terpane; BH, bisnorneohopane; H, hopane.
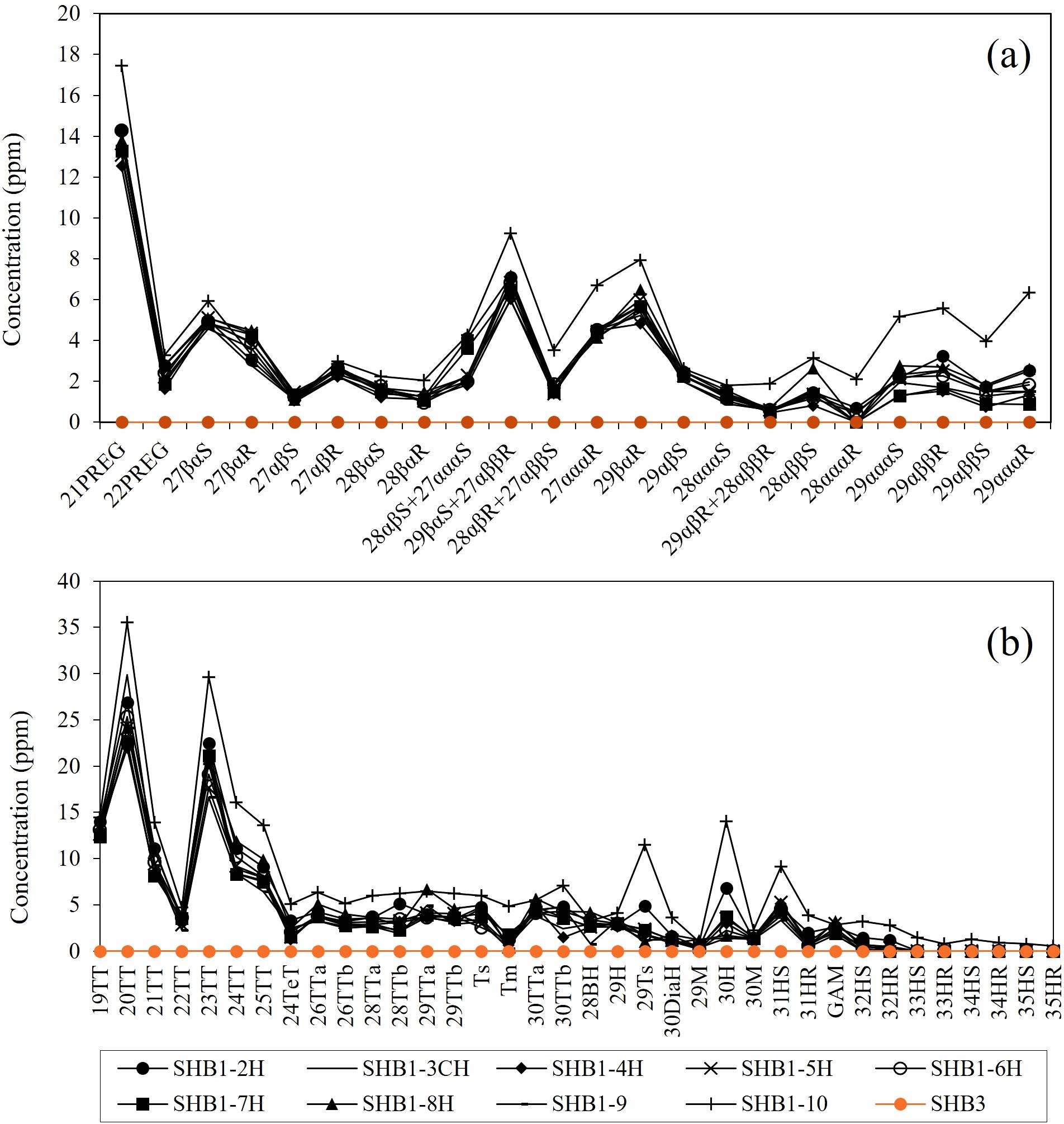
Figure 5. Steroid and terpenoid distribution patterns of oil samples from the No.1 and No.3 fault zones. (a) Steroids. (b) Terpenoids.
Similar to the distribution patterns of steroids, terpenoids including tricyclic terpanes (TTs), tetracyclic terpane (TeT) and pentacyclic terpanes (PTs), have been identified in oil samples from the No.1 fault zone (Figure 4b) with C19–C25 TTs being the most abundant ranging from 20 to 30 ppm in concentration. The Ts/(Ts + Tm) ratio, a maturity parameter derived from terpenoids, varies between 0.55 to 0.92 in these samples (Table 1). However, the C23TT/C30H values exhibit greater variability (Table 1), primarily due to trace amount of C30H in this formula. As a result, caution should be taken when interpreting these ratios due to low concentrations of individual components. In contrast, similar to steroids in SHB3 oil, no individual terpenoid components are detected in these samples (Figure 5b).
4.3 Diamondoid hydrocarbons
Adamantane, diamantane and their homologs are detected in all the oil samples (Figures 6, 7). Generally, SHB3 oil exhibits lower concentrations of diamondoid hydrocarbons compared to samples from the No.1 fault zone (Figure 8). For both sets of oil samples, diamantane species have lower concentrations than their methyl-, dimethyl- and trimethyl-substituted isomers, with a notable decrease in tetramethyldiamantane concentrations (Figure 8). Among the oil samples from the No.1 fault zone, the 1,3-dimethyladamantane (1,3-DMAD) and 1-methyladamantane (1-MAD) are the most abundant species, with concentrations ranging from 192–291 and 314–327 ppm, respectively. Both adamantane and diamantane in the No.1 fault zone samples show similar aggregated concentrations of approximately 50–70 ppm. Maturity-related parameters such as the methyladamantane index [MAI=1-MAD/(1-MAD+2-MAD)] and methyldiamantane index [MDI=4-MD/(1-MD+3-MD+4-MD)] exhibit minor variation, ranging from 2.31–2.69 and 0.43–0.46, respectively, for the No.1 fault zone (Table 3). In contrast, SHB3 oil has significantly lower concentrations of diamondoid hydrocarbons, with individual components generally below 100 ppm. The concentration differences of adamantane and diamantane species are less pronounced in SHB3 oil compared to those from the No.1 fault zone. Methylated diamantanes (15–49 ppm) are notably less abundant than methylated adamantanes in SHB3 oil. SHB3 oil also shows a lower MAI value (MAI=1.65) but a slightly higher MDI value (MDI=0.52) compared to the No.1 fault zone oils (Table 3).
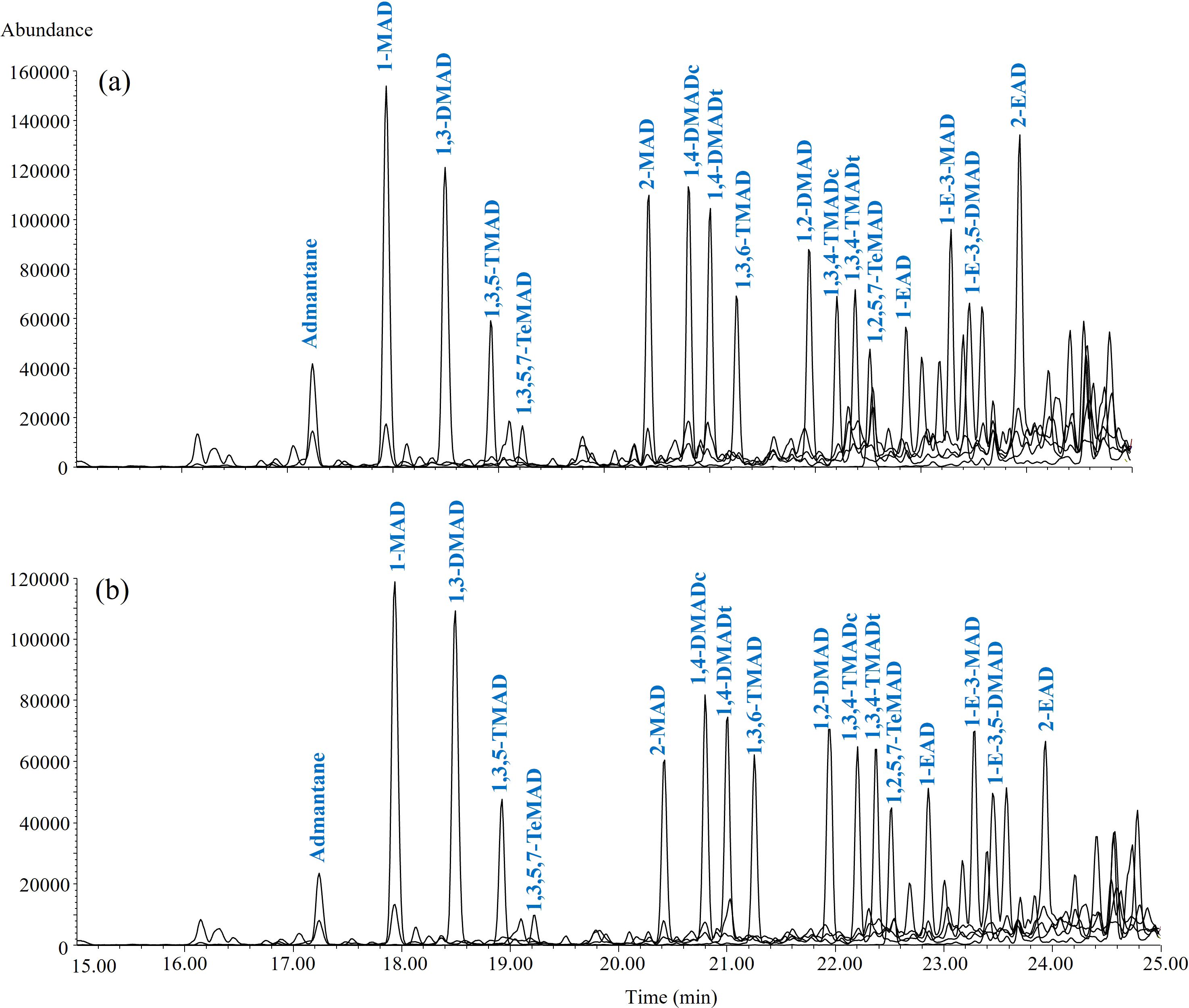
Figure 6. Summed mass chromatograms of m/z 136, 135, 149, 163, and 177 showing typical distribution of alkylated admantanes. (a) SHB3 oil. (b) SHB1-7H oil. MAD, methyladmantane; DMAD, dimethyladmantane; TMAD, trimethyladmantane; TeMAD, tetramethyladmantane; EAD, ethyladmantane.
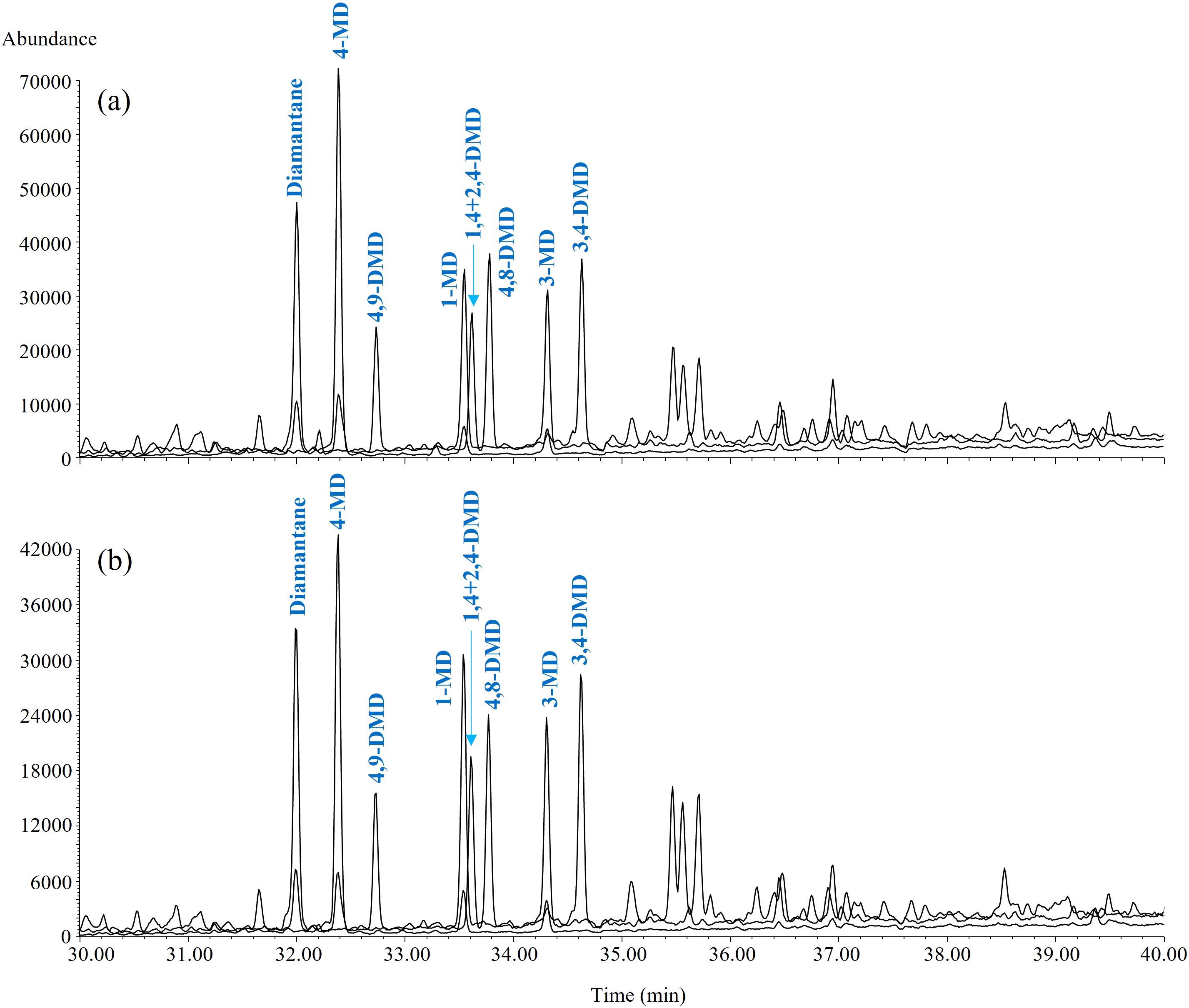
Figure 7. Summed mass chromatograms of m/z 188, 187, and 201 showing typical distribution of alkylated diamantanes. (a) SHB3 oil. (b) SHB1-7H oil. MD, methyladamantane; DMD, dimethyladamantane.
4.4 Aromatic hydrocarbons
The aromatic molecular parameters are more reliable indicators of thermal maturity at relatively high maturity levels. Consistent with this, monoaromatic and triaromatic steroids are absent in all the analyzed oil samples, further supporting their high maturity. Among the aromatic hydrocarbon series, alkylnaphthalenes are the most abundant. The 2-methylnaphthalene (2-MN) exhibits the highest concentration, exceeding 800 ppm, followed by 26,27-dimethylnaphthalene (26,27-DMN), which ranges from 694 to 819 ppm. Among all the studied oils, 2-MN has a pronounced dominance over 1-MN, indicating a higher maturity level. Methylated naphthalenes are detectable up to tetramethylnaphthalenes (TeMNs), while pentamethylnaphthalenes (PMNs) are only in trace amount or even absent in oil samples from the No.1 fault zone. Notably, SHB3 oil shows a relatively higher proportion of TMNs, TeMNs and PMNs (Table 4). Several maturity parameters derived from alkylnaphthalene have been established, including MNR (= 2-MN/1-MN) (Radke et al., 1982a), DNR [= (2,6-DMN + 2,7-DMN)/1,5-DMN] (Radke et al., 1986), TMNr [= 1,3,7-TMN/(1,3,7-TMN + 1,2,5-TMN] (van Aarssen et al., 1999), TeMNr [= 1,3,6,7-TeMN/(1,3,6,7-TeMN + 1,2,5,6-TeMN + 1,2,3,5-TeMN)]) (van Aarssen et al., 1999), and PMNr [= 1,2,4,6,7-PMN/(1,2,4,6,7-PMN + 1,2,3,5,6-PMN)] (van Aarssen et al., 1999). However, parameters such as TeMNr and PMNr, become invalid in highly mature petroleum or sediments with vitrinite reflectance above 1.1% while those derived from methyl- or dimethyl- substituted naphthalenes remain valid (Wang et al., 2022). Consequently, MNR, DNR and TMNr are accepted for assessing thermal maturity in this study. The MNR and DNR values of oil samples from the No.1 fault zone range from 1.95–2.17 and 9.23–11.33, respectively, whereas those of SHB3 oil are relatively lower (Table 3). As the proportion of TMNs increases in SHB3 oil, the discrepancy in TMNr values of oils from the No.1 and No.3 fault zones diminishes.
Dibenzothiophene (DBT) and methyldibenzothiophenes (MDBTs) are also significant components of aromatic hydrocarbons in oil samples from the No.1 fault zone (Figure 9). Concentrations of DBT, 4-MDBT and 2-+3-MDBT exceed 100 ppm, whereas the less stable isomer, 1-MDBT, is below 10 ppm. A stark contrast is observed in SHB3 oil, where concentrations of DBT and MDBTs are below 5 ppm (Figure 9). The methyldibenzothiophene index (MDR = 4-MDBT/1-MDBT) (Radke et al., 1986) ranges from 20.95 to 26.48 for oil samples from the No.1 fault zone, while SHB3 oil shows a slightly lower MDR of 20.67 (Table 3). Phenanthrene (P) concentrations in oil samples from the No.1 fault zone are approximately 100 ppm, compared to 64 ppm in SHB3 oil. Based on previous research, the MPI-3 parameter [= (3‐MP + 2‐MP)/(9‐MP + 1‐MP)] has proven to be a reliable indicator at highly maturity levels (He et al., 2019), whereas MPI‐1 [= 1.5 × (2‐MP + 3‐MP)/(P + 1‐MP + 9‐MP)] (Radke et al., 1982b) and MPI-2 [= 3 × 2‐MP/(P + 1‐MP + 9‐MP)] (Radke et al., 1982b) show poor correlation with thermal maturity due to the faster increase of phenanthrene, which compensates for the thermal stability impact on methylphenanthrene isomers. The MPI-3 values for all studied oils are similar, ranging from 1.04 to 1.24 (Table 3). Maturity parameters derived from biphenyl (BP) are more sensitive to thermal maturation (Alexander et al., 1986; Cumbers and Alexander, 1987; Wang et al., 2019). The methylbiphenyl (MBP) index (MBPI = 4-MBP/2-MBP), is valid in a wide maturity range of 0.4–1.6%Ro (Wang et al., 2019). The MBPI values of oil samples from the No.1 fault zone range from 60.34 to 110.99, whereas SHB3 oil is merely 3.75 (Table 3). The calculated vitrinite reflectance (%Rc) obtained from MBPI, was proposed by Wang et al. (2019), i.e., %Rc= 7.49 × 10–3 MBPI + 0.794 (%R>0.8). The %Rc of oil samples from the No.1 fault zone range from 1.25 to 1.63, whereas SHB3 oil is much lower in maturity with %Rc at 0.82 (Table 3).
5 Discussion
5.1 SHB3 oil as an end member of instantaneous petroleum charge
Thermal maturity exerts significant impact on component concentrations of reservoired petroleum. Regular steranes with biological configuration (e.g., C27-C29 αααR) dominate immature petroleum fractions, whereas steranes with geological configurations (e.g., αααS, αββR and αββS) along with pregnanes and diasteranes, are less abundant in immature petroleum. However, as thermal maturity increases, the relative abundance of steranes with geologically configurations, as well as pregnanes and diasteranes, increase dramatically. The thermal maturity parameter C29 20S/(20S + 20R) was introduced to measure the isomerization of C29 steranes at the C-20 chiral center and the transformation of biological αα and ββ epimers during thermal evolution (Seifert and Moldowan, 1978). Generally, sterane isomerization between biological and geological configurations reaches equilibrium at approximately 0.8%Ro, with a maximum C29 20S/(20S + 20R) value of around 0.65. Notably, steranes become undetectable beyond a maturity threshold of approximately 1.1%Ro, as no steranes are observed at higher levels of thermal maturity.
Oil mixing is a common phenomenon in the Tarim Basin, where the proportions and maturity of the end members dominate the maturity parameters derived from biomarkers. As noted by Huang et al. (2022), the C29 20S/(20S + 20R) ratio is 0.18 when 5% by mass of the very low maturity (Ro=0.5%) that is rich in biomarkers are mixed with 95% of a late generated mature oil (Ro=1.0%). In this scenario, the C29 20S/(20S + 20R) ratio shows a “mature” signature only if the proportion of low maturity oil decreases to less than 1%. If the biomarker concentrations of one end member (Ro=0.65%) decrease while the other end member remains at 1.0%Ro, the C29 20S/(20S + 20R) ratio increases to 0.44 and 0.52 when 50% and 5% by mass of the end member oil at 0.65%Ro is included, respectively. The C29 20S/(20S + 20R) ratios in the oil samples from the No.1 fault zone range from 0.42 to 0.50, indicating contributions from early-charged oil. However, these ratios also reflect dilution caused by subsequent generations of petroleum at higher maturity levels, during which regular steranes and diasteranes were destroyed. In contrast, the absence of steranes in SHB3 oil suggests it did not receive the early-charged oil.
As highlighted in the component concentration profiles by Huang et al. (2022), isoprenoid alkanes constitute a significant proportion of petroleum at low maturity stages (Ro<0.6%) compared to n-alkanes. However, the relative abundance of pristane and phytane decreases dramatically against the adjacent n-alkanes, i.e., Pr/n-C17 and Ph/n-C18, as maturity increases. The Pr/n-C17 and Ph/n-C18 values of SHB3 oil are notably lower than those from the No.1 fault zone, indicating that the reservoir associated with Well SHB3 lacks the early petroleum charging. In contrast, the reservoir within the No.1 fault zone underwent multiple episodes of petroleum charging, with early-charged petroleum characterized by high Pr/n-C17 and Ph/n-C18 values. Although subsequent petroleum charges at the higher maturity levels were accumulated in the reservoir with lower Pr/n-C17 and Ph/n-C18 values, it did not significantly alter the relatively high Pr/n-C17 and Ph/n-C18 values established by the early-charged petroleum.
While the biomarkers such as streranes and terpanes are most abundant in low-maturity petroleum fractions, aromatic hydrocarbons become predominate during later stages of petroleum generation (Wilhelms and Larter, 2004). Aromatic compounds with C–S bonds cyclized in fused aromatic structures, such as DBTs, are typically more thermally stable than those with C–C bonds, e.g., MNs (Wang et al., 2021b). The SHB3 oil exhibits lower concentrations of DBT and MDBTs compared to oils from the No.1 fault zone (Figure 9), indicating that the maturity of later-charged petroleum in SHB3 oil is lower than that in the No.1 fault zone. This is further supported by the lower values of parameters derived from MNs, DMNs and MDBTs, such as MNR, DNR and MDR, in SHB3 oil compared to those from the No.1 fault zone. Another compelling piece of evidence lies in the higher relative proportion on TMNs, TeMNs and PMNs of SHB3 oil. These compounds are preserved because the high degree of alkyl substitution in SHB3 oil has not been thermally cracked, unlike in the more mature oils from the No.1 fault zone.
Additionally, diamondoid hydrocarbons, which are sensitive indicators to multiple hydrocarbon charges and typically generated during later maturation stages (Zhang et al., 2005), are more abundant in oil samples from the No.1 fault zone than in SHB3 oil (Figure 8). This suggests that the reservoir associated with Well SHB3 has not undergone petroleum charging during the very late maturation stages. SHB3 oil spans a very narrow maturity range, which can be regraded as instantaneous petroleum charge (Figure 10). This is evident from the absence of the earliest charged petroleum in the sample trap and the lack of very late mature fractions. Such a scenario likely occurred when petroleum was expelled from source rocks and accumulated in the reservoirs of the No.3 fault zone within a limited oil generation interval. Alternatively, it could result from the opening and closing of strike-slip faults happened over a restricted geological timescale.
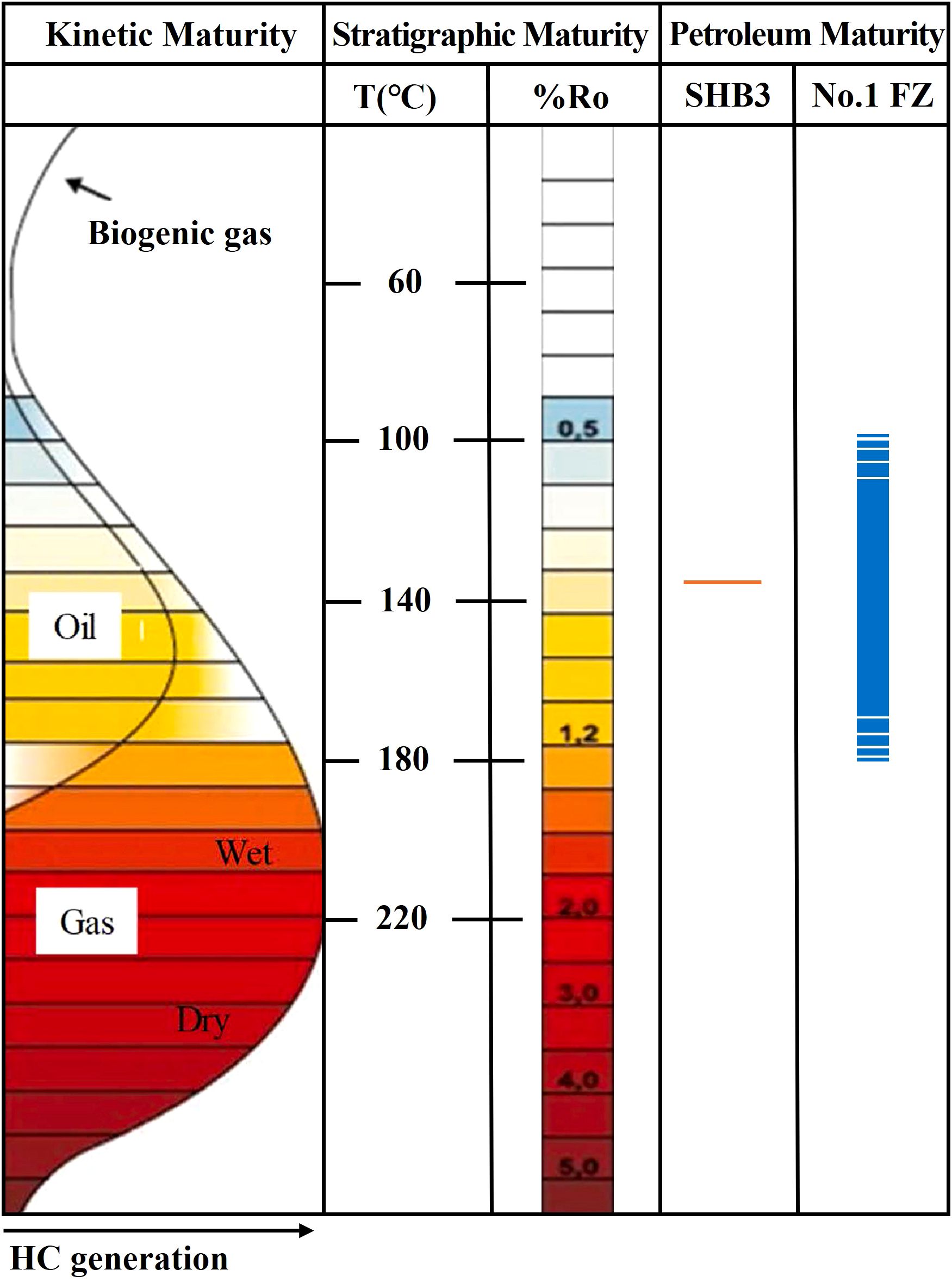
Figure 10. Thermal maturity model of reservoired petroleum in the No.1 and No.3 faults zones (modified after Huang et al., 2022). HC, hydrocarbon; FZ, fault zone.
5.2 Implication for petroleum accumulation in Shunbei reservoirs
The Shunbei reservoirs are predominantly controlled by strike-slip faults (Figure 1a), as most wells with industrial petroleum production were located along these fault zones. Currently, petroleum in the Shunbei reservoirs is primarily sourced from Cambrian Yuertusi Formation, with strike-slip faults serving as pathways from the Cambrian source rocks to Ordovician reservoirs in the Shunbei area (Qi, 2021). The strike-slip faults in the Shunbei area are characterized by vertical stratification and plane segmentation within the fault zones, which is the major controlling factor of hydrocarbon accumulation (Liu, 2020).
These fault zones have recorded multiple stages of tectonic evolution, including the early Caledonian, middle Caledonian, late Caledonian-early Hercynian, late Hercynian, and Indosinian-Himalayan periods, contributing to the heterogeneity of hydrocarbon accumulation. For example, in the No.1 strike-slip fault zone, high-angle strike-slip faults and en échelon normal faults developed during the Paleozoic (Figure 11), while extensional faults were mainly formed during the Mesozoic–Cenozoic. The large-scale, pronounced segmentation and multi-phase activities in the No.1 fault zone facilitated multiple petroleum charging events. In contrast, the No.3 fault zone, characterized by smaller scale and less active tectonics (Figure 11), experienced petroleum charging within a narrower maturity interval.
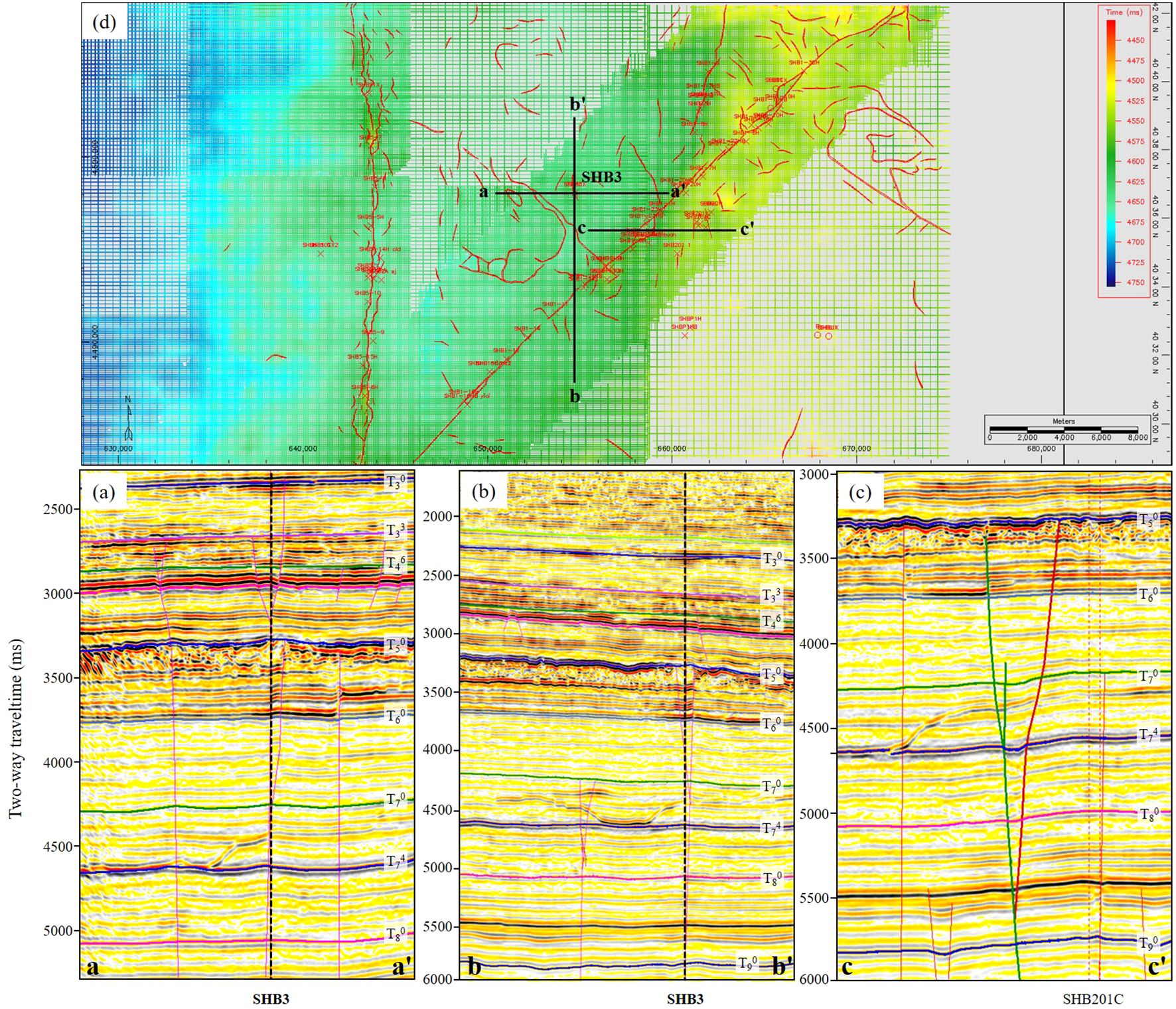
Figure 11. Seismic profiles of the Shunbei areas. (a-c) Fault interpretations of the cross sections with locations shown in Figure 11d. (d) The horizontal slice of T74 interface.
The Tarim Basin exemplifies a region shaped by multi-phase tectonics and multiple petroleum charging periods, resulting in petroleum components that span a broad range of thermal maturities. Petroleum compositions are crucial for reconstructing hydrocarbon accumulation history in terms of petroleum generation, expulsion and charge processes. However, the presence of multiple source rocks and charging stages, coupled with a complex geologic history, poses challenges for deconvoluting source rock properties and expelled petroleum compositions.
The concept of an “instantaneous petroleum charge” offers a critical framework to establish correlations between reservoired oil and source rock maturity, particularly in cases where source rocks are difficult to obtain. The nature of instantaneous petroleum charge is equal to the stratigraphic maturity of source rock at a specific maturity level (Figure 10). Moreover, as petroleum is usually a mixture in the reservoirs, it is difficult to figure out the geochemical signature of individual petroleum charging episode which is the barrier to unravel hydrocarbon accumulation processes. By focusing on fraction maturity, this concept provides a powerful tool for deciphering the intricate petroleum accumulation histories and geological evolution of superimposed basins which could be a reference to identify more end members of instantaneous petroleum charge in reservoirs of worldwide scale.
6 Conclusions
Detailed organic geochemical analysis of oil samples from the No.1 and No.3 fault zones, has been conducted to unravel the petroleum charging history in the Shunbei reservoirs. Significant differences in biomarker and aromatic hydrocarbon distributions were observed between oil samples from these fault zones. SHB3 oil from the No.3 fault zone is characterized by low Ph/n-C18 and Pr/n-C17 ratios, an overwhelming predominance of high-carbon-number n-alkanes (> C19) and an absence of steroid and terpenoid biomarkers. This suggests that SHB3 oil did not receive early-charged oil, in contrast to the oils from the No.1 fault zone. However, oil samples from the No.1 fault zone, exhibit higher concentrations of diamondoid hydrocarbons and more stable aromatic hydrocarbons, such as dibenzothiophene, indicating a higher proportion of mature fractions compared to SHB3 oil. This suggests that the reservoir associated with Well SHB3 has not undergone petroleum charging during the very late maturation stages. SHB3 oil spans a very narrow maturity range, which can be regarded as an end member for instantaneous petroleum charge. The end member of instantaneous petroleum charge in the Shunbei reservoirs offers valuable insights into the properties of hydrocarbons from individual petroleum charging period which sheds light on the complex hydrocarbon accumulation processes involving mixed maturities in superimposed basins.
Data availability statement
The original contributions presented in the study are included in the article/Supplementary Material. Further inquiries can be directed to the corresponding author.
Author contributions
QW: Funding acquisition, Validation, Writing – original draft, Writing – review & editing. HH: Funding acquisition, Investigation, Supervision, Validation, Writing – review & editing. TJ: Supervision, Writing – review & editing.
Funding
The author(s) declare that financial support was received for the research and/or publication of this article. This study was supported by the National Natural Science Foundation of China (Grant No.42473034, No.42406065), the SINOPEC Key Laboratory of Petroleum Accumulation Mechanisms (Grant No.33550007-22-ZC0613-0034), the National Key Research and Development Program of China (No.2024YFC2814702), the China Postdoctoral Science Foundation (Grant No.2023M733302) and the “CUG Scholar” Scientific Research Funds at China University of Geosciences (Wuhan) (Project No.2022096).
Acknowledgments
The author(s) acknowledge Prof. Steve Larter, Prof. Lloyd Snowdon and the other PRG members for their supervision and support of lab analysis. The authors thank Dr. Daqing Tang for his generosity in providing the seismic profiles of the Shunbei reservoirs. We thank the editor and reviewers for their reviews and constructive suggestions to improve the manuscript.
Conflict of interest
The authors declare that the research was conducted in the absence of any commercial or financial relationships that could be constructed as a potential conflict of interest.
Generative AI statement
The author(s) declare that no Generative AI was used in the creation of this manuscript.
Publisher’s note
All claims expressed in this article are solely those of the authors and do not necessarily represent those of their affiliated organizations, or those of the publisher, the editors and the reviewers. Any product that may be evaluated in this article, or claim that may be made by its manufacturer, is not guaranteed or endorsed by the publisher.
References
Alexander R., Cumbers K. M., Kagi R. I. (1986). Alkylbiphenyls in ancient sediments and petroleums. Org. Geochem. 10, 841–845. doi: 10.1016/S0146-6380(86)80021-3
Cumbers K. M., Alexander R. (1987). Methylbiphenyl, ethylbiphenyl and dimethylbiphenyl isomer distributions in some sediments and crude oils. Geochim. Cosmochim. Acta 51, 3105–3111. doi: 10.1016/0016-7037(87)90121-9
Deng S., Zhao R., Kong Q., Li Y., Li B. (2022). Two distinct strike-slip fault networks in the Shunbei area and its surroundings, Tarim Basin: Hydrocarbon accumulation, distribution, and controlling factors. Am. Assoc. Pet. Geol. Bull. 106, 77–102. doi: 10.1306/07202119113
He C., Huang H., Wang Q., Li Z. (2019). Correlation of maturity parameters derived from methylphenanthrenes and methyldibenzothiophenes in the Carboniferous source rocks from Qaidam Basin, NW China. Geofluids, 2019, 5742902. doi: 10.1155/2019/5742902
Horstad I., Larter S.R. (1997). Petroleum migration, alteration, and remigration within Troll Field, Norwegian North Sea. Am. Assoc. Pet. Geol. Bull. 81, 222–248. doi: 10.1306/522B42F3-1727-11D7-8645000102C1865D
Huang H., di Primio R., Pedersen J. H., Silva R., Algeer R., Ma J., et al. (2022). On the determination of oil charge history and the practical application of molecular maturity markers. Mar. Pet. Geol. 139, 105586. doi: 10.1016/j.marpetgeo.2022.105586
Jia C. (1997). Tectonic characteristics and petroleum, Tarim Basin (Beijing, China: Petroleum Industry Press).
Jiang Z., Tang D., Sha X., Shen X., Luo S., Dong K., et al. (2024). Structure and evolution of faults in central and Northern Parts of Tazhong Uplift,Tarim Basin. Bull. Geol. Sci. Tech. 43, 120–132. doi: 10.19509/j.cnki.dzkq.tb20220663
Larter S. R., Aplin A. C. (1995). Reservoir geochemistry: methods, applications and opportunities. Geol. Soc. Spec. Publ. 86, 5–32. doi: 10.1144/GSL.SP.1995.086.01.02
Lerch B., Karlsen D. A., Matapour Z., Seland R., Backer-Owe K. (2016). Organic geochemistry of Barents Sea petroleum: Thermal maturity and alteration and mixing processes in oils and condensates. J. Pet. Geol. 39, 125–148. doi: 10.1111/jpg.12637
Liu B. (2020). Analysis of the main controlling factors of oil and gas differential accumulation in the Shunbei area, Tarim Basin-taking Shunbei No. 1 and No. 5 strike-slip fault zones as examples. China Pet. Explor. 25, 83–95. doi: 10.3969/j.issn.1672-7703.2020.03.008
Liu Y., Suppe J., Cao Y., Hao F., Liu Y., Wang X., et al. (2023). Linkage and formation of strike-slip faults in deep basins and the implications for petroleum accumulation: A case study from the Shunbei area of the Tarim Basin, China. Am. Assoc. Pet. Geol. Bull. 107, 331–355. doi: 10.1306/11142220110
Liu Y., Suppe J., Cao Y., Wu K., Wang J., Du Y., et al. (2024). Strike-slip fault zone architecture and its effect on fluid migration in deep-seated strata: Insights from the Central Tarim Basin. Basin Res. 36, 12868. doi: 10.1111/bre.12868
Mukhopadhyay P. K. (1994). Vitrinite reflectance as maturity parameter: petrographic and molecular characterization and its applications to basin modeling. ACS Symp. Ser. Am. Chem. Soc. 570, 1–24. doi: 10.1021/bk-1994-0570.ch001
Qi L. (2021). Structural characteristics and storage control function of the Shun I fault zone in the Shunbei region, Tarim Basin. J. Pet. Sci. Eng. 203, 108653. doi: 10.1016/j.petrol.2021.108653
Qiu H., Deng S., Cao Z., Yin T., Zhang Z. (2019). The evolution of the complex anticlinal belt with crosscutting strike-slip faults in the central Tarim Basin, NW China. Tectonics 38, 2087–2113. doi: 10.1029/2018TC005229
Radke M., Welte D. H., Willsch H. (1982b). Geochemical study on a well in the Western Canada Basin: relation of the aromatic distribution pattern to maturity of organic matter. Geochim. Cosmochim. Acta 46, 1–10. doi: 10.1016/0016-7037(82)90285-X
Radke M., Welte D. H., Willsch H. (1986). Maturity parameters based on aromatic hydrocarbons: Influence of the organic matter type. Org. Geochem. 10, 51–63. doi: 10.1016/0146-6380(86)90008-2
Radke M., Willsch H., Leythaeuser D., Teichmüller M. (1982a). Aromatic components of coal: relation of distribution pattern to rank. Geochim. Cosmochim. Acta 46, 1831–1848. doi: 10.1016/0016-7037(82)90122-3
Seifert W. K., Moldowan J. M. (1978). Applications of steranes, terpanes and monoaromatics to the maturation, igration and source of crude oils. Geochem. Cosmochim. Acta 42, 77–95. doi: 10.1016/0016-7037(78)90219-3
Tissot B. P., Pelet R., Ungerer P. H. (1987). Thermal history of sedimentary basins, maturation indices, and kinetics of oil and gas generation. Am. Assoc. Pet. Geol. Bull. 71, 1445–1466. doi: 10.1306/703C80E7-1707-11D7-8645000102C1865D
Ungerer P. H., Pelet R. (1987). Extrapolation of the kinetics of oil and gas formation from laboratory experiments to sedimentary basins. Nature 327, 52–54. doi: 10.1038/327052a0
van Aarssen B. G., Bastow T. P., Alexander R., Kagi R. I. (1999). Distributions of methylated naphthalenes in crude oils: Indicators of maturity, biodegradation and mixing. Org. Geochem. 30, 1213–1227. doi: 10.1016/S0146-6380(99)00097-2
Wang Q., Huang H., Chen H., Zhao Y. (2020). Secondary alteration of ancient Shuntuoguole oil reservoirs, Tarim Basin, NW China. Mar. Pet. Geol. 111, 202–218. doi: 10.1016/j.marpetgeo.2019.08.013
Wang Q., Huang H., He C., Li Z., Zheng L. (2022). Methylation and demethylation of naphthalene homologs in highly thermal mature sediments. Org. Geochem. 163, 104343. doi: 10.1016/j.orggeochem.2021.104343
Wang Q., Huang H., Li Z., Li Z. (2019). Novel thermal maturity parameters derived from alkylbiphenyls and alkyldiphenylmethanes. Energ. Fuel 33, 8491–8502. doi: 10.1021/acs.energyfuels.9b02084
Wang Q., Huang H., Li Z., Ma Y., Zeng J., Larter S. (2021a). Geochemical significance of β-carotane in lacustrine oils from the Shahejie Formation of the Dongying Depression, eastern China. Org. Geochem. 156, 104241. doi: 10.1016/j.orggeochem.2021.104241
Wang Q., Huang H., Sun J., Huang J., Jiang T. (2025). Limitations of diamondoids in the quantitative evaluation of petroleum cracking in ultra-deep carbonate reservoirs of the Shunbei area, Tarim Basin. Front. Mar. Sci. 12, 1578161. doi: 10.3389/fmars.2025.1578161
Wang Q., Huang H., Zheng L. (2021b). Thermal maturity parameters derived from tetra-, penta-substituted naphthalenes and organosulfur compounds in highly mature sediments. Fuel 288, 119626. doi: 10.1016/j.fuel.2020.119626
Wilhelms A., Larter S. (2004). Shaken but not always stirred. Impact of petroleum charge mixing on reservoir geochemistry. Geol. Soc. Spec. Publ. 237, 27–35. doi: 10.1144/GSL.SP.2004.237.01.03
Wu W., Chen H., Su A., Wang Y., Zhu Z., He J., et al. (2023). The contribution of petroleum charging episodes to different strike-slip fault zones in the Shunbei area, the Tarim Basin, NW China. Energies 16, 579. doi: 10.3390/en16020579
Wu G., Gao L., Zhang Y., Ning C., Xie E. (2019). Fracture attributes in reservoir-scale carbonate fault damage zones and implications for damage zone width and growth in the deep subsurface. J. Struct. Geol. 118, 181–193. doi: 10.1016/j.jsg.2018.10.008
Yang P., Liu K., Evans N. J., Zhang S., Li Z., Su J., et al. (2024). Petroleum accumulation history of deeply buried carbonate reservoirs in the northern Tarim Basin, northwestern China. Am. Assoc. Pet. Geol. Bull. 108, 1193–1229. doi: 10.1306/06212321210
Zhang S., Huang H. (2005). Geochemistry of Palaeozoic marine petroleum from the Tarim Basin, NW China: Part 1. Oil family classification. Org. Geochem. 36, 1204–1214. doi: 10.1016/j.orggeochem.2005.01.013
Zhang S., Huang H., Su J., Zhu G., Wang X., Larter S. (2014). Geochemistry of Paleozoic marine oils from the Tarim Basin, NW China. Part 4: Paleobiodegradation and oil charge mixing. Org. Geochem. 67, 41–57. doi: 10.1016/j.orggeochem.2013.12.008
Zhang S., Huang H., Xiao Z., Liang D. (2005). Geochemistry of Palaeozoic marine petroleum from the Tarim Basin, NW China. Part 2: maturity assessment. Org. Geochem. 36, 1215–1225. doi: 10.1016/j.orggeochem.2005.01.014
Zhang S., Su J., Wang X., Zhu G., Yang H., Liu K., et al. (2011). Geochemistry of Palaeozoic marine petroleum from the Tarim Basin, NW China: Part 3. Thermal cracking of liquid hydrocarbons and gas washing as the major mechanisms for deep gas condensate accumulations. Org. Geochem. 42, 1394–1410. doi: 10.1016/j.orggeochem.2011.08.013
Keywords: thermal maturity, biomarkers, instantaneous petroleum charge, Shunbei reservoirs, Tarim Basin
Citation: Wang Q, Huang H and Jiang T (2025) Instantaneous petroleum charge in the Shunbei reservoirs, Tarim Basin. Front. Mar. Sci. 12:1553799. doi: 10.3389/fmars.2025.1553799
Received: 31 December 2024; Accepted: 09 April 2025;
Published: 02 May 2025.
Edited by:
Hongjian Zhu, Yanshan University, ChinaReviewed by:
Yazhou Liu, China University of Petroleum, ChinaJin Xiao, China Geological Survey, China
Qin Zhang, Delft University of Technology, Netherlands
Copyright © 2025 Wang, Huang and Jiang. This is an open-access article distributed under the terms of the Creative Commons Attribution License (CC BY). The use, distribution or reproduction in other forums is permitted, provided the original author(s) and the copyright owner(s) are credited and that the original publication in this journal is cited, in accordance with accepted academic practice. No use, distribution or reproduction is permitted which does not comply with these terms.
*Correspondence: Qianru Wang, cWlhbnJ1LndhbmdAY3VnLmVkdS5jbg==