- 1Healthy Ocean Group, Cawthron Institute, Nelson, New Zealand
- 2Environment and Sustainability Group, Lyttelton Port Company, Christchurch, New Zealand
- 3Institute of Life and Earth Sciences, Heriot-Watt University, Edinburgh, United Kingdom
- 4Styles Group Underwater Acoustics, Auckland, New Zealand
Ports in Aotearoa New Zealand have or are currently undergoing extensive infrastructure developments to accommodate the global trend in larger commercial vessels and cruise ships. With no national standards for underwater noise, Lyttelton Port Company is the first Aotearoa New Zealand port to undertake monitoring to assess pile-driving generated noise effects on local Hector’s dolphins, an endemic and nationally vulnerable species. The immediate and shorter-term responses of this species were monitored with autonomous underwater recorders and assessed to understand how dolphins reacted to pile-driving activities. General and site-specific model results indicated that as sound exposure levels from impact pile-driving increased, declines in dolphin detections varied spatially with immediate declines occurring at sites less than 1km for the source. Declines in detections were still apparent up to 2km, but more evident with greater noise exposure levels and in warmer water conditions. Once piling ceased for a day, Hector’s dolphins moved back into inner harbor waters within hours, and acoustic detections returned to within pre-piling levels gradually over a few days. While there is no evidence that dolphins abandoned the port region over the construction period, our findings established a longer-term decline in dolphin detections coinciding with the 2019 construction period that had not returned to pre-construction levels by May 2020. Despite evidence that mitigation measures were successful at protecting against auditory injury impacts, additional measures are warranted for future port infrastructure developments to avoid short and longer term impacts on Hector’s dolphin use of the harbor and while Aotearoa New Zealand develops or adopts national underwater noise guidelines.
1 Introduction
Approximately 80% to 90% of global trade by volume is transported by ocean-going vessels (UNCTAD, 2022). Aotearoa New Zealand, due to its geographic location, strongly relies on an effective port system with close to 99% of its imports and exports passing through them (MOT, 2022). The port system also plays an important part in facilitating cruise tourism across Aotearoa New Zealand, which contribute significant benefits to the country (e.g. Smith, 2024). Continuing increases in the size of commercial ships, particularly cruise ships, has resulted in a need for upgrading of port infrastructures globally (e.g., Son and Cho, 2022; Boorstein, 2024). Over the last decade, 10 of the 13 ports in Aotearoa New Zealand have either completed, or are undergoing, extensive infrastructure upgrades. These upgrades can involve extending existing wharves, reclamation and breakwater installations as well as capital dredging. Such construction activities have been well documented to produce underwater noise and increase ambient sound levels (e.g., Brandt et al., 2011; Todd et al., 2015).
Anthropogenic underwater noise is a widely recognized pollutant that can negatively impact marine wildlife (Slabbekoorn et al., 2010; Duarte et al., 2021) and is a recognized concern for several marine industries and regulatory agencies around the world (e.g., OSPAR, 2009; CEDA, 2011; WODA, 2013; NMFS, 2018, 2024; ACCOBAMS, 2019; DIT, 2023). In shallow coastal waters, one of the loudest sources of underwater radiated noise is pile-driving (Madsen et al., 2006). With high source levels and steep rise-times, impact pile-driving noise can be audible to marine mammals over several kilometers (Madsen et al., 2006). In closer proximity, these impulses can induce acute physiological stress, such as hearing impairment through temporary and permanent auditory threshold shifts (TTS and PTS, respectively) in marine wildlife (Tougaard et al., 2003; Bailey et al., 2010; Slabbekoorn et al., 2010; Brandt et al., 2016; Dähne et al., 2017).
Behavioral disturbance of marine mammals from underwater noise has been well studied, more in regard to large, offshore energy projects (e.g., Edrén et al., 2004; Tougaard et al., 2005; Madsen et al., 2006; Brandt et al., 2016; Dähne et al., 2017; Southall et al., 2021). However, behavioral impacts are context-dependent and therefore, highly variable both within and between species (Southall et al., 2007). For example, behavioral responses vary depending on whether exposed individuals are foraging, milling, travelling or calving (Southall et al., 2019), and seems to have some dependence on the duration of exposure (Tougaard et al., 2003, 2005; Southall et al., 2007; Bailey et al., 2010). Consequently, responses can range from minor (such as changing in swimming direction, speeds or vocalization) to more moderate levels (such as extensive changes to swimming speeds or cessation of vocalizations) to severe (such as complete abandonment or avoidance of primary habitat) (Southall et al., 2007; 2019).
Despite the large number of port infrastructure developments being undertaken in Aotearoa New Zealand, there remains a lack of national or industry standards or guidelines for assessing or mitigating underwater noise generated from these construction activities. The only study to report on impact pile-driving noise levels within Aotearoa New Zealand waters investigated effects potentially associated with Hector’s dolphins (Cephalorhynchus hectori hectori) within Whakaraupō Lyttelton Harbour (hereafter Lyttelton Harbour) (Leunissen, 2017; Leunissen and Dawson, 2018; Leunissen et al., 2019). Lyttelton Port Company Limited (LPC) is the South Island’s largest commercial port, located on the eastern side of Ōtautahi Christchurch within Lyttelton Harbour (Figure 1). The harbor is part of the Banks Peninsula Marine Mammal Sanctuary (BPMMS) and home to the Hector’s dolphin, a nationally and internationally recognized endangered species (Baker et al., 2019; Reeves et al., 2013).
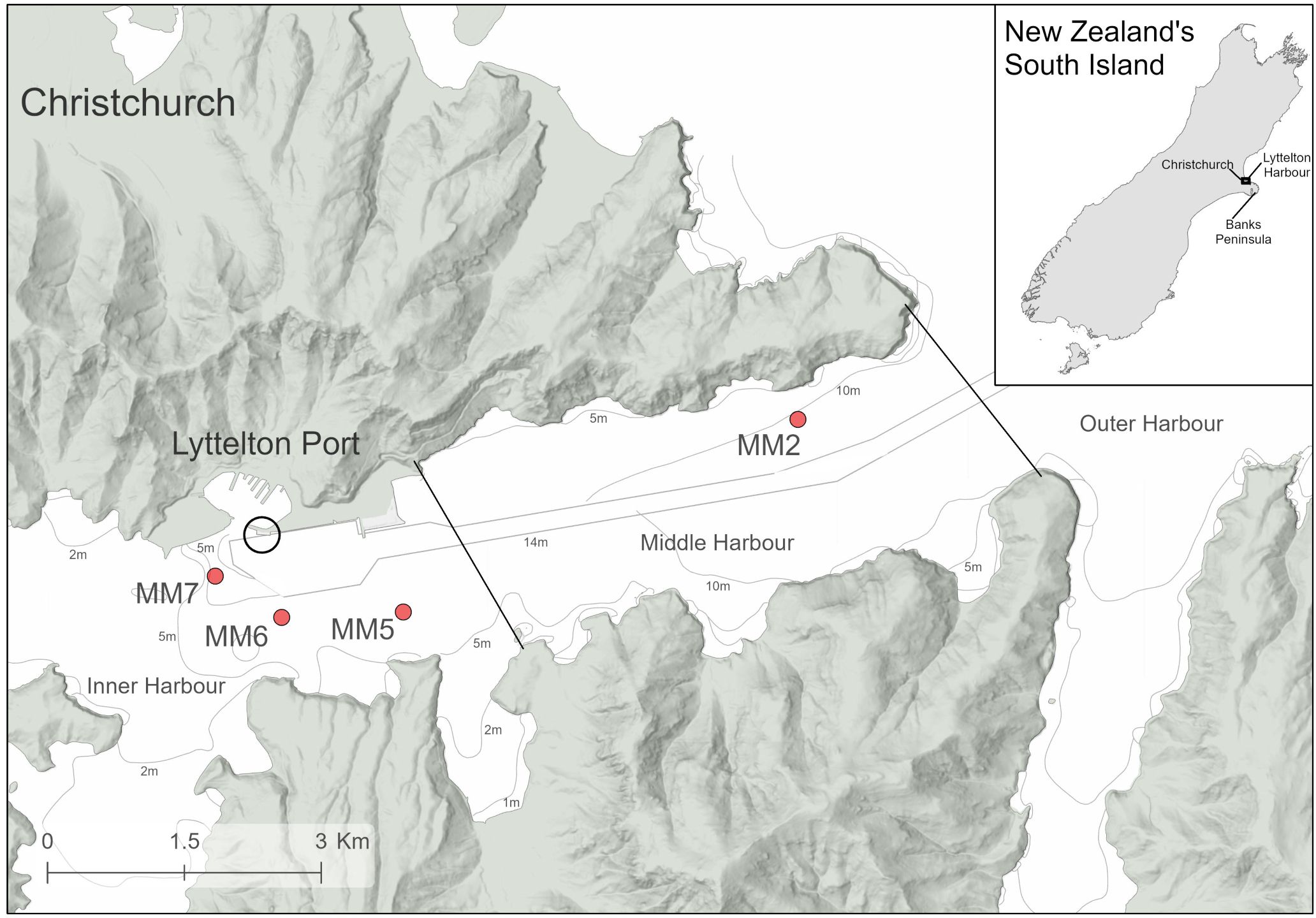
Figure 1. Map of Lyttelton Harbour with the cruise berth construction site (open circle) indicated as well as the divisions of the harbor used in the study and depth contours throughout the harbor. The locations of the underwater acoustic moorings used for Hector’s dolphin monitoring are marked with closed circles and labelled.
As the only dolphin species endemic to Aotearoa New Zealand waters (Baker et al., 2019), Hector’s dolphins occur in three regional sub-populations around the South Island, the main concentration of the east coast sub-population is located around Banks Peninsula (MacKenzie and Clement, 2016). Hector’s dolphins are found within Peninsula waters throughout the year, with individuals having relatively small home ranges (~50 km) and demonstrating high site fidelity (Clement, 2005; Rayment et al., 2009b; Brough et al., 2019). This species is also well-known for its seasonal distribution patterns, moving closer to shore and spreading into most bays and harbors (including Lyttelton Harbour) during the warmer summer and autumn months. Over the colder months, most animals generally move further offshore and generally out of most inner- and middle harbor regions (Clement, 2005; Rayment et al., 2010; MacKenzie and Clement, 2016).
As Hector’s dolphin (and other Cephalorhynchus species) produce narrow-band high frequency echolocation pulses (generally between 80 and 150 kHz; Dawson and Thorpe, 1990) for communication, orientation, and foraging, increasing levels of underwater noise generated from port construction activities is a concern. LPC’s rebuild of their earthquake damaged main wharf in 2014–2015 provided an initial opportunity to examine how impact pile-driving activity might affect local Hector’s dolphins. The subsequent underwater acoustic study by Leunissen and Dawson (2018) suggested Hector’s dolphins near impact piling activities may be at risk of TTS exposure effects while Leunissen et al. (2019) indicated potential movements of dolphins away from inner to middle harbor regions with increasing intensity of impact piling noise. In light of these findings, LPC funded a harbor-wide acoustic monitoring program prior to the commencement of planned large-scale infrastructure developments over 2019 in order to assess the potential underwater noise effects on local Hector’s dolphins. LPC also voluntarily implemented several noise mitigation measures not previously used in Aotearoa New Zealand when undertaking pile-driving, including enforcing shut-down zones using marine mammal observers (MMOs; LPC, 2023).
This paper investigates Hector’s dolphin immediate and short-term reactions to pile-driving activities associated with the 2019 construction of a purpose-built cruise berth wharf to facilitate the return of large cruise vessels to the city of Ōtautahi Christchurch post-earthquake. For this study, Hector’s dolphin behavioral responses to pile-driving noise were inferred from changes in their acoustic detections rates (i.e. echolocation clicks). The overall aims of this study were (1) to investigate how port-generated underwater noise and environmental noise sources compared in their influence on Hectors’ dolphin detections near the port, (2) to understand the spatial scale and extent of dolphins’ behavioral responses to impact pile-driving generated noises, and (3) to evaluate whether and for how long dolphins’ behavioral responses persisted after the cessation of all pile-driving activities.
2 Materials and methods
2.1 Location
Lyttelton Harbour is located on the central east coast of Aotearoa New Zealand’s South Island (Figure 1). It is an approximately 15 km long natural inlet situated on the northwest side of Banks Peninsula. LPC has been operating since 1877 and as the South Island’s largest port and Aotearoa New Zealand’s second largest exporter, it is a regular destination for container vessels and cruise ships. The cruise berth construction area is indicated in Figure 1.
2.2 Construction management and mitigation measures
The construction of the new cruise berth involved the installation of 356 piles through the existing pier (reclaimed land) and 64 piles in the water (sized 914 mm only). The land piles ranged in size from 610, 710, 810, 900 and 914 mm diameter. Piles were installed with a combination of vibratory (ICE Vibro 44B) and impact (maximum 14t hydraulic hammer) driving methods. Only one piling rig was used at any one time, which due to the welding, pile pitching and repositioning of the equipment, limited the actual impact hammering undertaken each day to no more than 6 hours (non-consecutive). At the same time, pile-driving activities were restricted to between Monday to Saturday and during daylight hours only, between 0730 and 1800. The final construction times varied considerably as they were determined by appropriate weather and operational conditions. In-water pile-driving activity took place over 14 months, between December 2018 and February 2020. Additional mitigation measures included pre-start surveys for marine mammals prior to any work commencing each day and after extended breaks, ramping up/soft start procedures over 10 minutes, and the most intensive pile-driving effort was scheduled to take place over colder months (Enviser, 2018).
A designated marine mammal observation zone (MMOZ) with a qualified marine mammal observer was one of the key mitigation actions proposed to minimize the risk of hearing impairment or injury (i.e. temporary or permanent hearing threshold shifts: TTS or PTS) to marine mammals from pile-driving activities (Enviser, 2018). A shut-down monitoring zone of 450 m radius was monitored by an experienced marine mammal observer (MMO) from an elevated, 2.6 m high platform near the piling rig. The MMO focused mainly on the shut-down zone but scanning also took place beyond the zone and up to 1 km radius from the site, when visibility allowed. Monitoring was undertaken during pile-driving activities as well as for at least 30 minutes prior to and following these activities (which took place during daylight hours only). All marine mammal sightings were logged and records kept of all delayed start-up or enforced shut-downs due to presence of marine mammals within the zone.
2.3 Data collection
MMO watches took place intermittently and as required from 7 December 2018 to 5 February 2020, whenever pile-driving (vibratory or impact) activity was underway. All marine mammal sightings were logged with details including date/time, number of animals, their location (distance, bearing), piling activity (type, duration and pile number) and general descriptions of the species and their behavior.
Acoustic data were collected using SoundTrap 300HF recorders (Ocean Instruments NZ) at four monitoring sites throughout Lyttelton Harbour (Figure 1) varying in deployment duration between July 2018 and April 2021 (Table 1). Mooring locations were based on similar site locations used by Leunissen et al. (2019), which followed a gradient sampling design (see Thompson et al., 2010; Brandt et al., 2011). The MM7 and MM2 moorings matched the location of the earlier inner and outer sites, respectively, and our MM6 and MM5 moorings were added to the inner harbor region for the aims of this study. Both the inner and middle harbor divisions are also similar to the earlier study, while the outer harbor boundary was shifted to align with the harbor heads.

Table 1. The deployment dates and locations of the SoundTrap acoustic recorders used to monitor Hector’s dolphins in Lyttelton Harbour from 2018 to 2021.
The SoundTraps were programmed to operate on a 33% duty cycle (5min recordings every 15mins) at a sampling rate of 288 kHz. Each recorder was secured 1m above the seafloor using a subsurface float (3m above the seafloor) and an anchor weight. A grapple line ran along the seafloor to another anchor weight (which spanned twice the depth) and a surface buoy. Batteries and SD cards were replaced monthly followed by immediate re-deployment of the units (or replacement if unit was malfunctioning) on the same mooring. Extraneous noise contamination from the mooring was excluded by securing all moving parts and confirmed by examining spectrograms and through playbacks.
2.4 Data processing
To investigate the effects of pile-driving within Lyttelton Harbour on Hector’s dolphin detection rates (as a proxy for behavioral effects in this study), acoustic recordings were analyzed for ambient sound pressure levels (Lp-1sec), single strike sound exposure levels (LE) from all detected hammer strikes, and the acoustic presence of dolphins as detection positive minutes (DPM).
The broadband Lp-1sec levels were processed for all acoustic data using functions from PAMGuide (Merchant et al., 2015). The output of those functions were CSV files that contained the time-stamped Lp-1sec (10Hz – 48 kHz) of data processed. Those Lp-1sec values were then logarithmically averaged over each hour, providing hourly sound pressure levels (Lp-1-hour) and used in the general model for ambient noise levels.
Underwater noise from pile-driving between December 2018 and February 2020 were analyzed using the official records from the MMOs on site and the piling contractor log. By combing those records, a pile-driving log was compiled that listed all start/end times of piling activity, the drive method (vibratory or impact), the pile number and its GPS position. For the finer-scale models, the acoustic recordings were then split according to the start/end times of known vibratory and impact piling activity and an impulse detector was used to isolate all hammer pulses from the waveform data. The impulse detector used was a modified version of an air-gun pulse detector by Pine et al. (2020) and provided the broadband (10Hz – 48kHz) single-strike sound exposure level (LE,p) for each detected pulse. The LE,p values were defined as:
where is the root-mean-squared (RMS) sound pressure in pascals over the T90 duration (T90). The time integral, T90, used in the above equation was the time (in seconds) over which the cumulative sound energy rises from 5% to 95%, thereby containing 90% of the energy. The 0.458 dB was therefore added to account for the lost energy either side of the 5% and 95% (i.e., 10 × Log10(0.9)). The LE,p for each hammer strike were then averaged over each hour, representing the impact pile-driving noise level for that hour.
2.4.1 Hector’s dolphin detections
To detect the presence of Hector’s dolphin echolocation clicks, the acoustic data were run through an automated detector (see Supplementary Material). The detector used a convoluted neural network (CNN), built using transfer learning of a pretrained ResNet-18 (trained on a subset of the ImageNet database) in MATLAB 2021b. The last learnable layer (fully connected layer) and classification output layers were adapted for our class probabilities, loss values, and predicted labels. This involved replacing the fully connected and classification layers with ones that reflected our classification classes. The classification classes in this study were ‘positives’ (i.e., echolocation clicks were present in the spectrogram) and ‘negatives’ (i.e., no clicks present). The learning rate factors for the weights and biases were set at 10 and the weights of the first four layers were frozen. This prevented the training from recomputing the gradients of those frozen layers, thereby increasing training speed, as well as helping prevent overfitting. Finally, data augmentation was applied to the training datasets.
The training dataset was comprised of known Hector’s dolphin echolocation clicks from a subsample of the data from this study. Using the MMO sighting data, recordings were extracted 1 hour either side of each visual sighting and used to generate 1-min audio samples and spectrograms. The extraction of recordings was done using custom scripts written in MATLAB and were done over a single deployment in each season to capture seasonal variation in the soundscape. Through manual checking of the 1-min snippets, 2-sec spectrograms (50 – 144kHz) of echolocation click trains, burst pulses and buzzes were generated using 512 sample Hanning window size (288 kHz sampling rate) and 50% overlap. The spectrogram’s power spectral density levels were relative levels, set between [-95 -30] dB re µPa2Hz-1. By using relative dB levels, it ensured the detector was trained on the echolocation click’s signal excess relative to any hydrophone sensitivity, thereby maintaining its performance regardless of the specific hydrophone sensitivity.
A total of 9,068 2-sec spectrograms were used as the overall dataset, of which 4,534 spectrograms contained echolocation clicks (positives) and 4,534 contained background noise (negatives). The training dataset comprised of a random 80% of the overall dataset, while the remaining 20% was the validation dataset. A final test dataset that comprised of 13,250 spectrograms (6,625 positives and 6,625 negatives) was used to evaluate the detector’s performance (final precision score 0.995, recall score 0.927, overall accuracy 96% (see Supplementary Material).
The detector was used to quantify dolphin activity as detection positive minutes (DPM). The DPM was defined as any minute that contained at least one dolphin click train, burst pulse or buzz. This unit of measure was used to be commensurate with other studies that use CPODs or TPODs acoustic loggers to assess the impacts of pile-driving on marine mammals (e.g., Brandt et al., 2016; Leunissen et al., 2019). The DPM values could then be averaged over each hour, providing DPM per hour. However, since the SoundTrap recorders were running on a 33% duty cycle, we refer to DPM per hour in this study as a DPM per duty-cycle hour, as the maximum DPM was over 20 minutes (as opposed to 60 minutes if it was a true DPM per hour).
2.5 Data analyses
2.5.1 Effects on dolphin acoustic detection rates
No masking effects of Hector’s dolphin echolocation clicks due to pile-driving noise were found, as the pile-driving noise and echolocation clicks did not spectrally overlap (see Supplementary Material). Furthermore, the substantial differences in the temporal dynamics of echolocation click trains, pulses and buzzes to the impulsive signals from hammer strikes during piling also prevented masking. While the piling noise itself was not masking the SoundTraps receiving dolphin clicks, the MMO visual sighting data were used to test for any differing effects that pile-driving activities might have on the rates that dolphin were clicking. A visual sighting was viewed as definitive evidence of Hector’s dolphin presence in the area. A sub-set of MMO sightings that occurred close enough to the inner moorings (MM6 and MM7) to record an acoustic detection was collated in ArcGIS. Using the initial GPS-position estimates of the sightings, only those sightings that were within 300 m radius of either MM7 or MM6 were selected based on previous collaboration studies (e.g. Tougaard et al., 2005; Rayment et al., 2009a).
The acoustic data (.wav files) were manually inspected for dolphin clicks that were produced from up to 1 hour before the start time of a sighting to up to 1 hour after the end time of each sighting (based on the MMO sighting logs and test dataset). Visual sightings that contained acoustic detections and those without acoustic detections for each of these inner harbor moorings were tabulated. The matched datasets were used to investigate whether the dolphins may react behaviorally to pile-driving activities by reducing clicks or not echolocating at all. Using X2 methods, we tested for potential differences between datasets with and without piling activities underway.
2.5.2 Modelling of noise effects on dolphin detection
Cumulative sound exposure levels were not included in the statistical analyses because the dolphin detection values could not be robustly estimated over the missing recording periods due to the 33% recording duty cycle. To evaluate and separate the potential effects of increased construction noise (i.e. ambient and/or pile-driving noise) on the acoustic detection rates (i.e. DPM) of the dolphins, a general and a fine-scale approach was adopted using random effects models, also known as mixed models (Zuur et al., 2009). Random effects models allow the modelling of correlated data within the context of generalized linear models (GLMM) or generalized additive models (GAMM) as the predictor can be described in terms of both random and fixed effects (Bolker et al., 2009). This class of models is particularly interesting in the current analyses, as DPM per duty-cycle hour is an autocorrelated response variable as we expect that the number of detections in any given hour is similar to the number of detections in adjacent hours within a day of any given month. In the GLMM framework, the random effects were described as an autoregressive structure of order 1 (AR1), which considers correlations between data points to be highest between adjacent hours within a Julian day, and a systematically decreasing correlation with increasing distance between time (Zuur et al., 2009).
Due to the moorings being deployed at different times of the year, the data were first truncated to only include data between September 2018 and May 2020 to allow for seasonal comparison among years. General GLMMs were then fit to the DPM per duty-cycle hour data to investigate for spatio-temporal (site, season and year) variability, and the potential effect of increased ambient sound (Lp-1hr), full pile-driving cycles (including impact and vibration piling together) and environmental variables with potential to affect underwater noise (i.e. wind speed, wind direction, temperature or rainfall accumulation) or with potential ecological effect (i.e. temperature, Table 2).
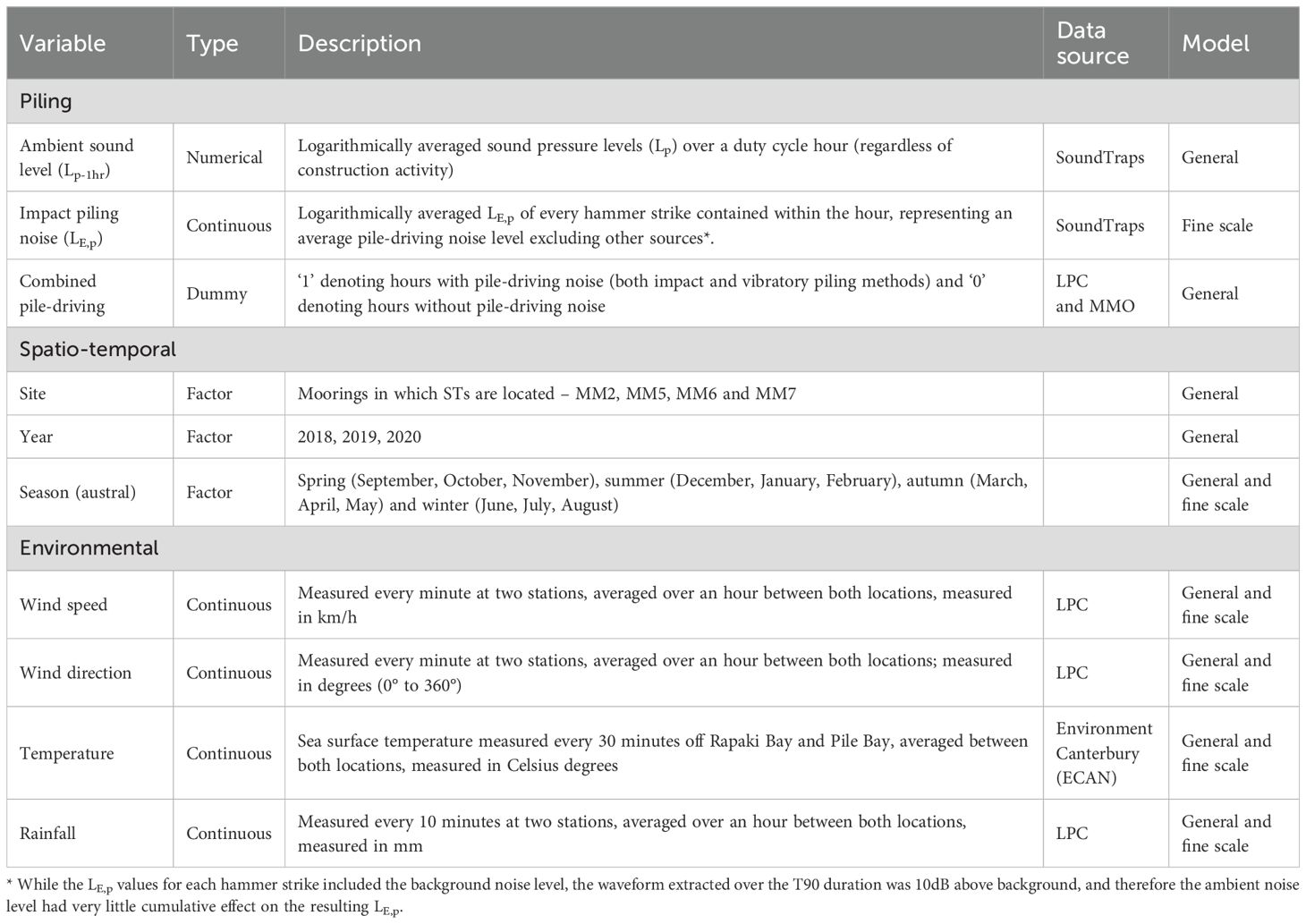
Table 2. Predictor variables included as fixed effects in the general and fine-scale linear mixed models with the potential to explain the observed variability on DPM per duty-cycle hour.
For this project, impact pile-driving methods had the greater potential to exceed marine mammal hearing thresholds and adversely affect Hector’s dolphins. To investigate these potential effects further as well as validate the predictions of Leunissen et al. (2019), finer scale generalized additive models (Wood, 2017) were fit to detection data for those hours in which only impact pile-driving occurred, i.e. from December 2018 to February 2020. Evidence from Leunissen et al. (2019) suggested impact piling may have differing spatial effects on detection rates. As our study collected in situ dolphin detection and impact pile-driving noise data concurrently at each of the mooring locations, we were able to build site-specific models to look at these effects separately for each mooring. For these models, and as noted by Leunissen et al. (2019), we excluded data from mooring site MM2 as impact piling noise (i.e. hammer pulses) was not detectable at this site, located more than 6km from the piling source (see Supplementary Figures).
We used generalized additive mixed models (GAMMs) to account for the hourly nested observations within a particular day of any given month. The GAM/GAMM framework uses smooth functions to estimate the relationship between the response variable and predictor variables, allowing us to investigate covariate effects that are beyond linear, being ideal for a detailed investigation of the acoustic response on piling noise. To evaluate the impact piling noise effect isolated from other sources of noise (e.g. vessel traffic or vibratory pile-driving), the single-strike LE,p of the impact piling pulses was used instead of ambient noise in the fine-scale models. Spatio-temporal and environmental predictor variables, except year, were also considered in the fine-scale models to ensure all other potential effects on DPM per duty-cycle hour were included (Table 3).
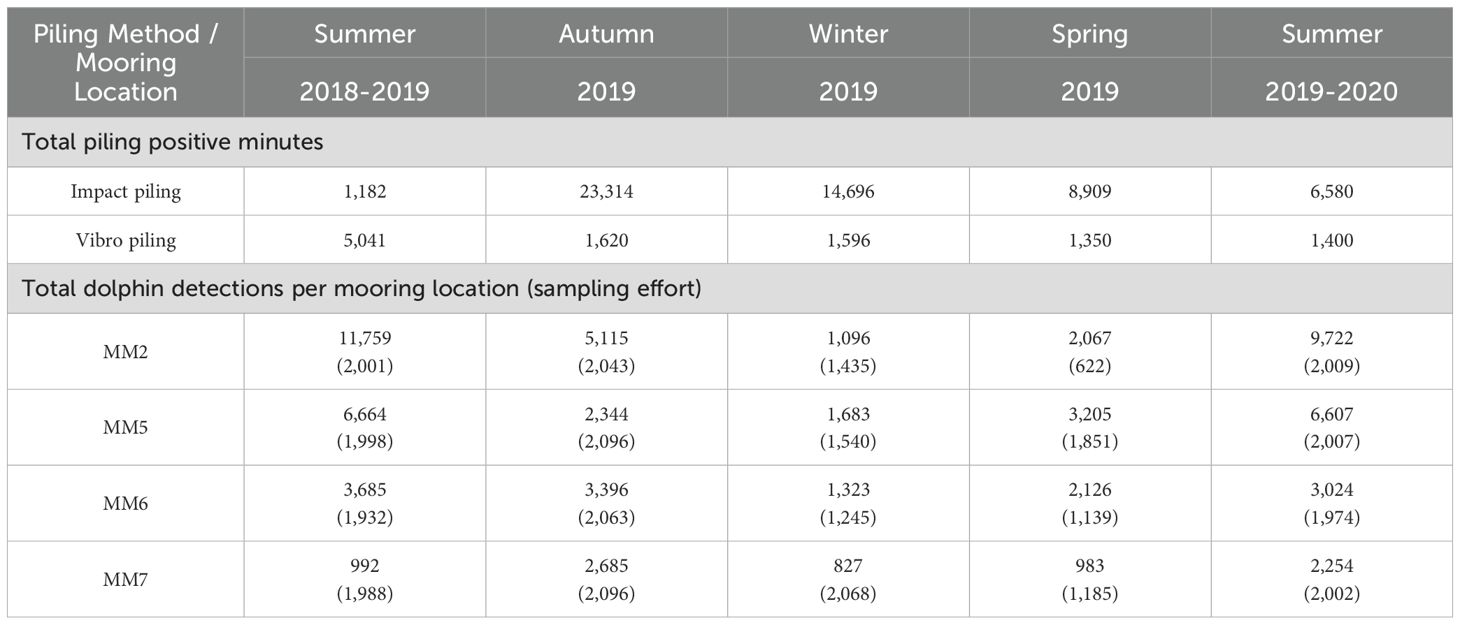
Table 3. Total number of piling positive minutes for impact and vibratory piling methods and total number of dolphin detections at each SoundTrap mooring location (duty hour sampling effort in brackets) summarized by austral seasons over the construction period (December 2018 to February 2020).
For both the general and fine-scale models, the response variable DPM was assumed to follow a binomial distribution (i.e. logistic regression) where the number of trials was given by the number of minutes the SoundTrap was recording per duty cycle (33% duty cycle; i.e. 5 min every 15 min). Before fitting the models, all numerical predictor variables were standardized to avoid convergence issues and to yield comparable effects among them. Collinearity between predictors was also addressed, and if present, correlated variables were not included in the same model. In this case, the predictor variables were chosen based on the Akaike Information Criteria (AIC; Burnham and Anderson, 2002).
Model selection for both approaches was carried out using a full model averaging procedure on the basis of Akaike Information Criteria with small sample size correction (AICc). This is the standard approach available in the package MuMIn available in R (Bartoń, 2023). The procedure calculates a weighted average of model parameter estimates, such that little weight is given to the parameter estimates from models that provide only a limited explanation of the variance in the response variable (Symonds and Moussalli, 2011). Following Burnham et al. (2011), after fitting all possible combinations of predictors (including fixed and random effects), models with ΔAICc > 7 were considered uninformative and therefore, discarded.
2.5.3 Short-term recovery
To assess short-term effects of pile-driving activity on Hector’s dolphins, the recovery rate, or amount of time that it took for dolphin detections to return to within pre-piling rates once all pile-driving (both vibratory and impact) activity ceased, were evaluated. For the purpose of this analysis, pre-piling detection rates do not refer to normal, undisturbed behavior in which the animals have not been exposed to any pile-driving activity. Instead, pre-piling refers to the detection rates of animals that have not been exposed to any piling activity for at least 96 hours (four days). The pile-driving construction period was defined as any specific time intervals in which there was a cessation of all pile-driving activity that lasted for at least 96 hours before a piling session and coincided with no further piling activity (either vibratory or impact) for up to 96 hours after a pile-driving session. As the detection data were not normally distributed, a non-parametric Wilcoxon rank sum test with continuity correction was carried out to evaluate any statistical differences in mean DPM rates between pre- and post-piling periods for each mooring site.
To explore recovery trends further, the pre- and post-piling datasets of the three mooring sites within 2km of piling activity (MM5-MM7) were combined and the mean DPM per duty-cycle hour plotted. The time it took for the combined mean post-piling DPM per duty-cycle hour to increase and peak within the post 96hrs period was assessed.
3 Results
The stages of the cruise berth wharf construction that involved any piling activity began on 7 December 2018 and lasted through 5 February 2020. Pile-driving activity in the form of vibratory, impact or both occurred over 243 days varying widely in duration. Impact pile-driving activity was more concentrated over the austral autumn months, and at slightly lower, but also elevated activity levels over austral winter months of 2019 (Table 3). At the same time, vibratory piling activity was more consistent across the construction period after initial pile setting at the start of the project.
While the overall amount of impact piling was generally lower over austral summer months, it took place over two consecutive Decembers (2018, 2019) and Januarys (2019, 2020), coinciding with the greater peaks in Hector’s dolphin detection rates across the different mooring locations (Table 3). These seasonal changes in Hector’s dolphin’s distribution, as well as the presence of a harbor gradient, were evident in acoustic detections at each individual mooring site (Table 3).
3.1 Effects on dolphin acoustic detection rates
By comparing the matched MMO sighting and acoustic datasets, we found that the acoustic recorders at MM6 and MM7 detected 69% of all visual sightings within their range regardless of piling activity. There was no statistical indication of differences in the proportions of matched acoustic detections between piling and no piling activities (see Supplementary Tables). However, sample sizes were relatively small for both tests (nMM7 = 45, nMM6 = 40).
3.2 Noise effects - general model
As there was evidence that water temperature correlated with season and year (Spearman’s correlation test = -0.51 and 0.37, p< 0.001) when examining predictor variable correlations, these variables were not combined into a single model. Rainfall was excluded as a variable as trial model fits indicated it was acting as ‘pretending’ variable (Sutherland et al., 2023), with point estimates very close to zero. When the global models were fit with either season and year or temperature, and on the basis of AIC, the global model containing season and year was selected (ΔAIC = 593.35). Out of the total 128 models fit, only the global model was considered due to the large difference in ΔAICc to the second-ranked model (Table 4).
Model estimates and respective standard errors are given in the logit scale, and they are given in comparison to a baseline (intercept) that refers to the DPM per duty-cycle hour at MM2, in spring, and during 2018 (Supplementary Tables). The model indicates a strong negative effect on DPM per duty-cycle hour with increases in ambient noise (p< 0.001), any pile-driving (p< 0.001), wind speed (p< 0.001) and wind direction (from northwest to northeast winds; p< 0.001). Based on post-hoc analysis using Tukey’s test, there was also evidence that DPM per duty-cycle hour was greater in summer compared to all other seasons (p< 0.001) while at its lowest in winter (p< 0.001). Evidence also pointed to a general decreasing DPM gradient from the middle harbor region (MM2) to each inner harbor site (all p< 0.001), and from 2018 to both 2019 and 2020 (all p< 0.001).
3.3 Piling effects - fine-scale models
Separate models were fit for two of the three inner harbor mooring sites (MM5 and MM6). Site MM7 was not considered as 86% of the DPM per duty-cycle hour were zero (360/419) and a regression analysis could not be satisfactorily implemented. Site MM2 precluded further investigation with finer-scale modelling based on the lack of any recorded impact pile-driving pulses at this location (see Supplementary Material).
For the two sites together, there was evidence that water temperature correlated with season (Spearman’s correlation test = -0.92, p< 0.001). Therefore, these variables were not combined into a single model and global models were fit with these predictors separately per site. On the basis of AIC, the global model containing temperature was selected for MM5 (ΔAICMM5 = 16.01), and season for MM6 (ΔAICMM6 = 3.54). To make the results more comparable, we considered the predictor temperature in the global models for both sites.
3.3.1 Site MM5
From all possible predictor combinations, only two models were selected as being informative (i.e. ΔAICc< 7, see Supplementary Tables). These models differed by having wind speed as a covariate. The smooth terms in Figure 2 describe the relationship between DPM probability and the numerical predictors. An overall decreasing relationship was estimated between impact piling noise and DPM probability that briefly plateaued between received hourly-averaged noise of 115 to 125 dB before a secondary decline. Increasing water temperatures also resulted in a gentle linear decline in DPM probability. Varying environmental conditions (wind speed and wind directions) had an overall low effect on DPM probability, with a slight increase in DPM mainly in winds from the southeast (between 100° and 175°) between 13–15 knots.
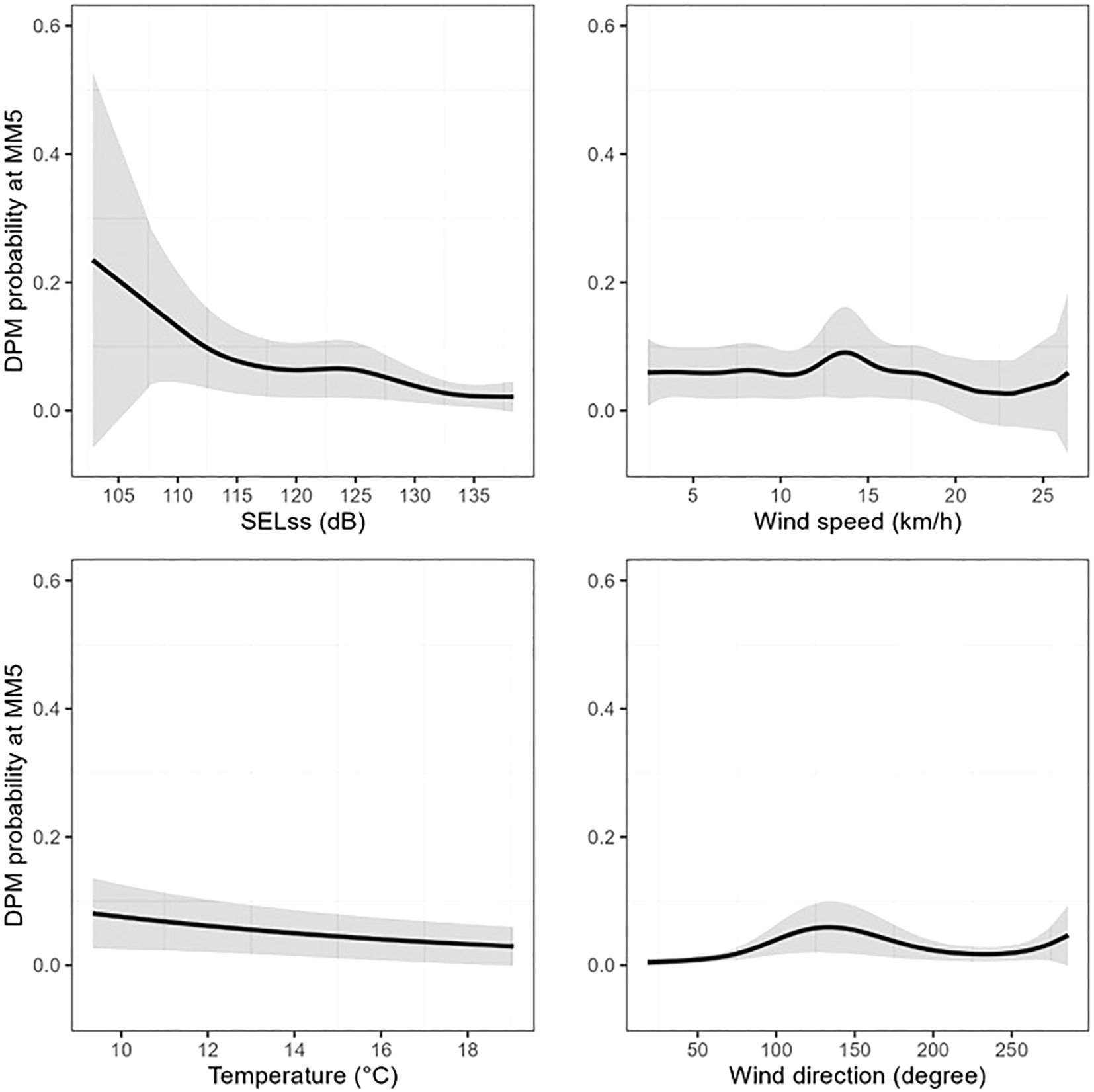
Figure 2. Smooth terms of model averaged estimates for site MM5 including the effects of piling noise (LE,p), wind speed, temperature and wind direction on the probability of DPM per duty-cycle hour. The black lines are the estimates, and the grey areas represent the 95% confidence interval.
3.3.2 Site MM6
From all possible predictor combinations, four models were selected as being informative (i.e. ΔAICc< 7, see Supplementary Tables). From these models, impact piling noise, wind speed and random effect were present in 100%, and temperature and wind direction in 50%. Decreasing relationships were estimated for DPM probability with both increasing impact piling noise and wind speed up to 20 knots (Figure 3). Both temperature and wind direction had little effect.
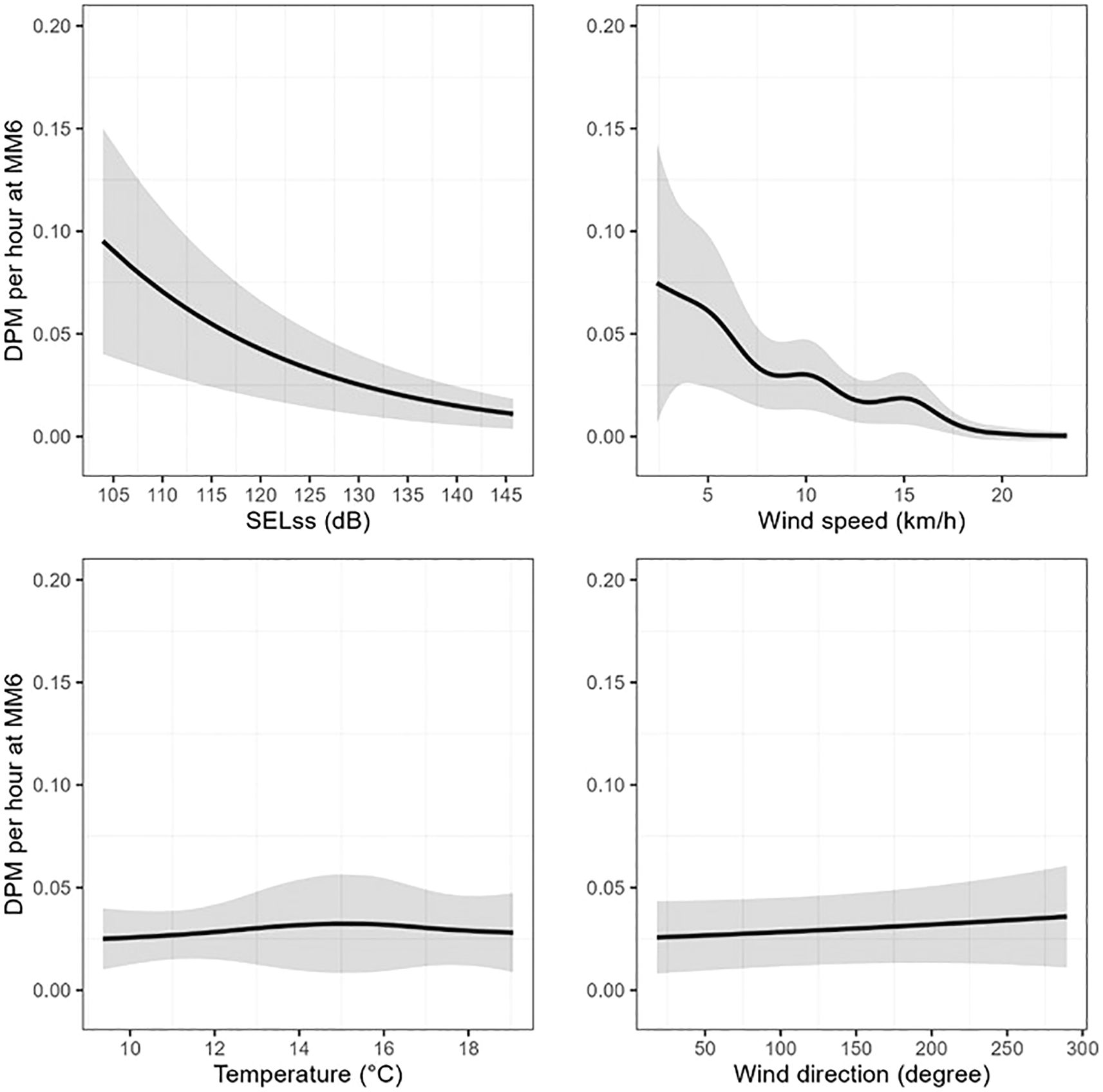
Figure 3. Smooth terms of model averaged estimates for site MM6 including the effects of single-strike LE,p of piling pulses, wind speed, temperature and wind direction on DPM per duty-cycle hour. The black lines are the estimates, and the grey areas represent the 95% confidence interval.
3.4 Short-term recovery
The number of DPM sampling periods (i.e. DPM per duty-cycle hour) in which there was neither vibratory or impact piling activity for the 96 hours immediately prior and after the cessation of a pile-drive session varied slightly between the four mooring sites (1,280 at MM7, 1,013 at MM6, 1,263 at MM5 and 1,136 at MM2). Based on these sampling periods, the Wilcox non-parametric tests suggested that post-piling mean DPMs were significantly lower than the pre-piling means at MM7 and MM2, but there were no statistical differences at either MM5 or MM6 (Table 5).
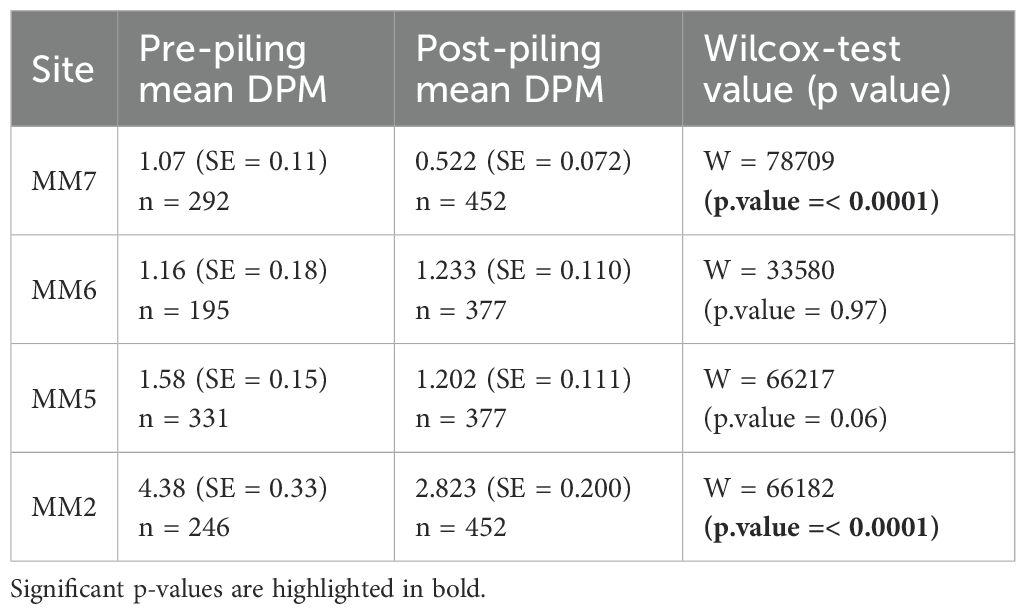
Table 5. Mean DPM per duty-cycle hour at each mooring site (MM7 – MM2) with standard error (SE), and Wilcox non-parametric test outcomes (W statistic and p-value).
When examining the mean DPM per duty-cycle hour at each mooring site before and after piling activity, a notable decline in detections began prior to the start of any piling activity, which was also evident at MM2 where impact piling could not be detected against the ambient background (Figure 4). Following a similar approach by Brandt et al. (2016), Figure 5 excludes pre-piling rates and shows only the post-piling mean DPM per duty-cycle hour for the three inner harbor sites combined. Mean DPM rates began increasing once pile-driving stopped, cycling up and down every 9–12 hrs before reaching a peak DPM rate between 46 and 48 hours post-exposure (Figure 5).
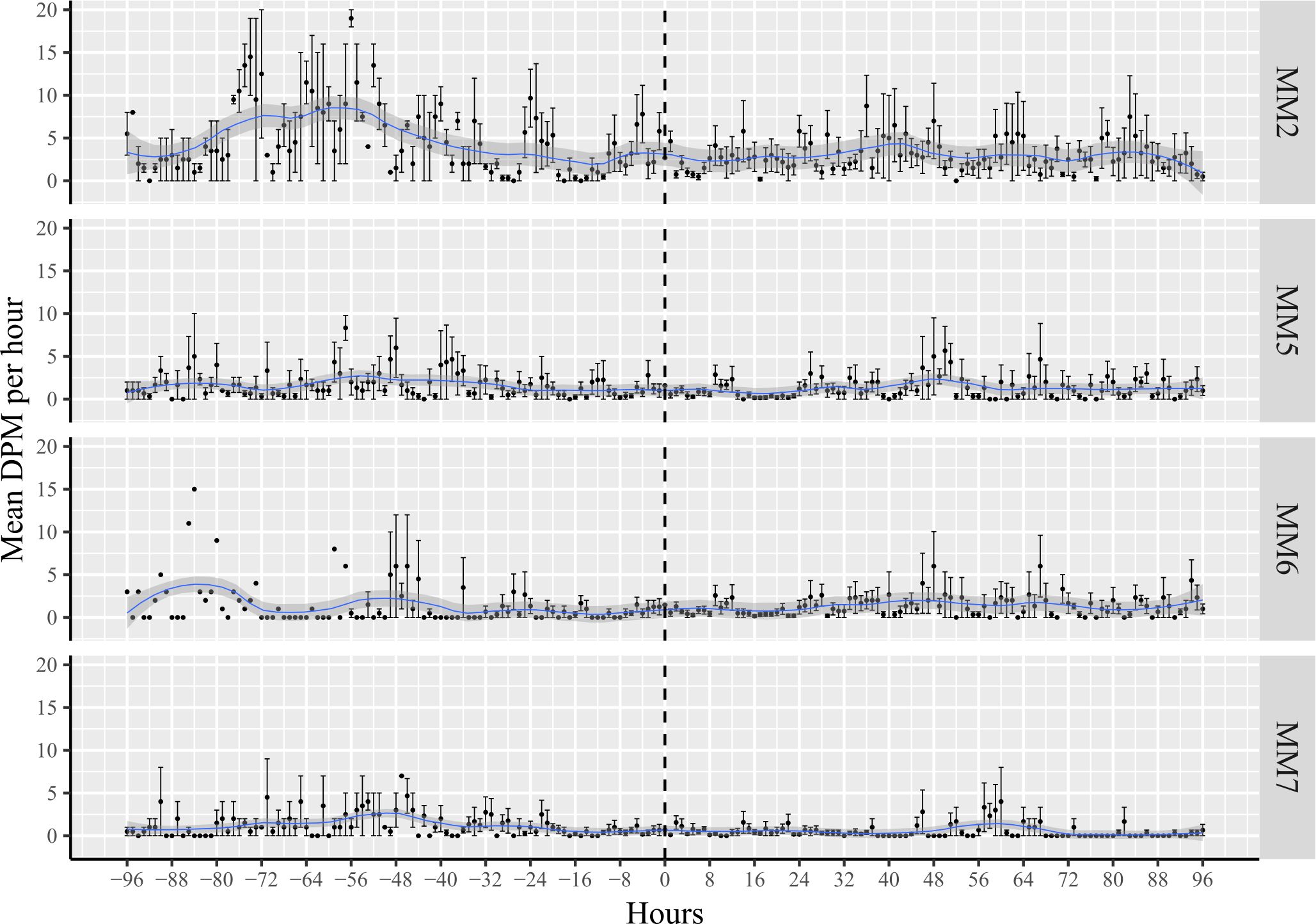
Figure 4. Mean detection positive minutes (DPM) per duty-cycle hour (y-axis) for each of the mooring sites (MM2- top, MM5, MM6, and MM7- bottom) relative to the four day (96 hours) prior and four days (96 hrs) after any piling driving activity occurred (x-axis). The black dots represent mean DPM with black error bars depicting the standard error. A geometric smoother, using LOESS (or Local Polynomial Regression Fitting), was added to the plots to aid in the visual examination of any trends. The solid line is the smoother and the grey areas represent the 95% confidence interval of the smoother function. The span parameter of the smoother function, or degree of smoothing, was set to 0.2.
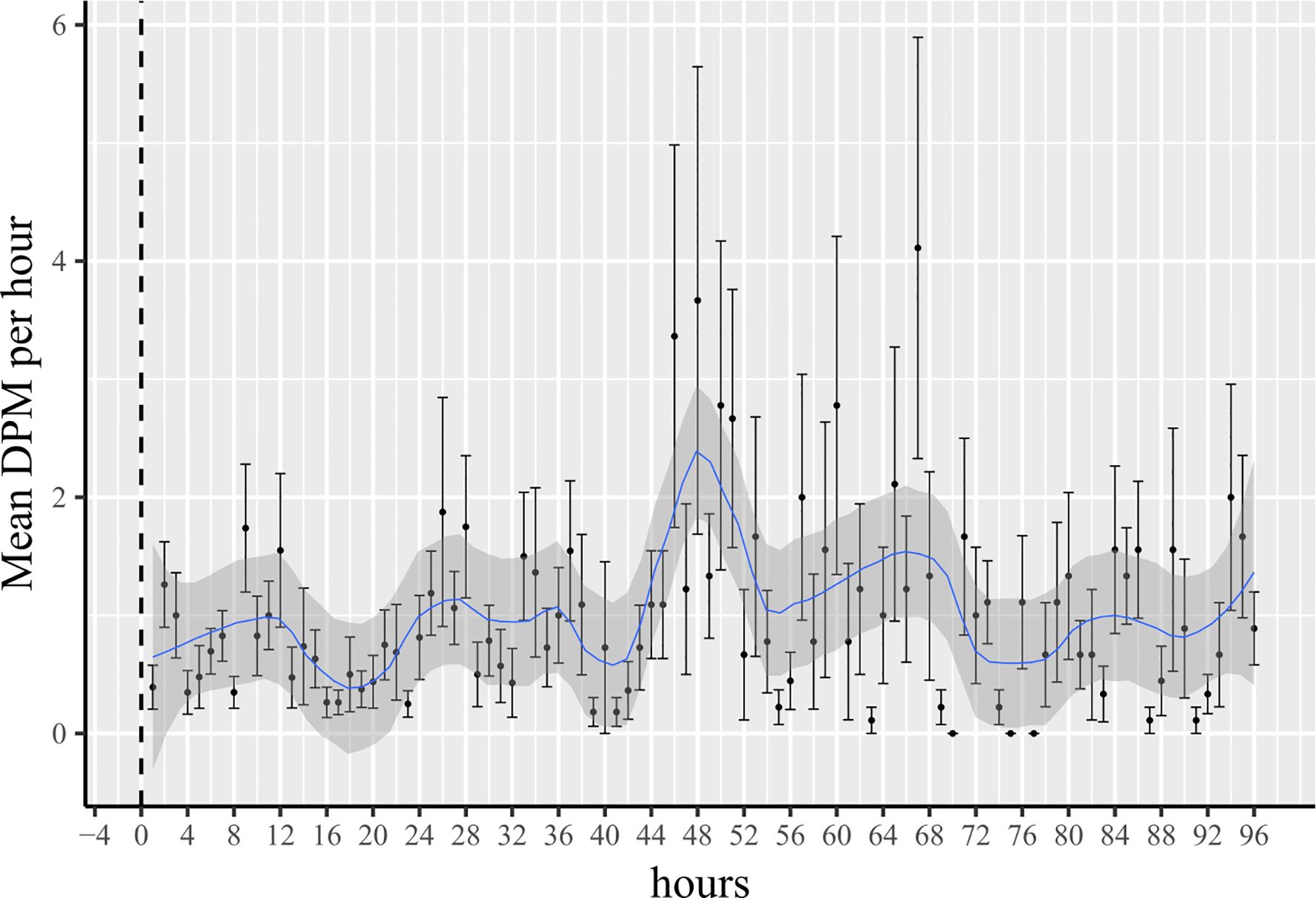
Figure 5. Post-piling mean detection positive minutes (DPM) per duty-cycle hour (y-axis) for the three inner harbor mooring sites (MM7, MM6, and MM5) combined together relative to the four day (96 hours) after piling driving activity ceased (x-axis). The black dots represent mean DPM with black error bars depicting the standard error. A geometric smoother, using LOESS (or Local Polynomial Regression Fitting), was added to the plots to aid in the visual examination of any trends. The solid line is the smoother and the grey areas represent the 95% confidence interval of the smoother function. The span parameter of the smoother function, or degree of smoothing, was set to 0.2.
4 Discussion
Ports can play important roles in helping research to minimize the underwater noise produced during marine infrastructure developments or upgrades. For example, the Vancouver Fraser Port Authority in British Columbia, Canada, developed and led the Enhancing Cetacean Habitat and Observation (ECHO) program to better understand, and subsequently, reduce impacts from the Port of Vancouver’s shipping activity on cetaceans within and around the Salish Sea (e.g., MacGillivray et al., 2019, 2022; Burnham et al., 2021). In Aotearoa New Zealand, LPC is the first port to commit to a long-term acoustic research program in relation to their infrastructure development activities. Building on previous research by Leunissen et al. (2019), this study used autonomous recorders at multiple locations that could record full-spectral data of the entire soundscape providing the first comprehensive dataset on Hector’s dolphin acoustic detections with corresponding noise exposure levels.
Adverse effects on marine fauna associated with increases in anthropogenic underwater noise pollution include behavioral responses (e.g. changes in diving patterns), auditory masking (e.g., interruptions in type or timing of vocalizations) and possible auditory injury (e.g., auditory threshold shifts and stress) (Nowacek et al., 2007; Cato, 2008; Slabbekoorn et al., 2010; Chapman and Price, 2011; Duarte et al., 2021). To avoid any TTS/PTS effects on Hector’s dolphin, LPC put in place measures to help mitigate the pile-driving noise associated with their cruise berth construction project. Measures included implementing MMO protected shut-down zones at 450m and limiting overlap between dolphin presence and piling activities (daily and seasonal piling limits; Enviser, 2018; LPC, 2023). By comparing MMO visual sightings and the acoustic detection data collected, we were able to confirm that dolphins were not significantly reducing their echolocation rates or going acoustically silent while pile-driving was underway. As a result, the immediate and short-term responses of Hector’s dolphins to various levels of pile-driving noise could be assessed.
Out of the main factors considered in our general model, pile-driving (i.e. hours with or without vibratory and impact noise) and ambient noise levels were both associated with greater decreases in dolphin detections at port moorings along with concurrent environmental factors, such as stronger, more northerly winds. Marine mammals have evolved to cope with large variations in ambient sound levels from both geophonic and biophonic sources (Cato, 2008). These natural and noisy sources, such as north-westerly wind that can funnel down from the harbor’s cliffs creating steep chop on Lyttelton’s surface waters, are transient lasting minutes to days. However, numerous studies have demonstrated the prevention or interference of sounds important to marine mammals can occur with sustained and elevated ambient sounds caused by an increase in anthropogenic underwater noise (Richardson, 1995; Nowacek et al., 2007; Cato, 2008; Slabbekoorn et al., 2010; Chapman and Price, 2011; Duarte et al., 2021).
Given that Lyttelton Harbour is the largest commercial port in Aotearoa New Zealand’s South Island, it is not surprising that the port’s more elevated ambient noise levels may affect the dolphins’ overall presence, particularly with increases in construction-related vessels over the same period. This finding might help explain the generally lower presence of Hector’s dolphins within the harbor relative to other regions around Banks Peninsula (Rayment et al., 2010; Brough et al., 2019). Yet, we do not have enough acoustic data on this species in other areas to determine if some noise habituation has occurred in those dolphins that continue to regularly visit the harbor, and/or whether this may have influenced their responses to additional noise pollution.
Our general model results did confirm, that despite any disturbances from construction activities, the persistence of documented seasonal and harbor-wide trends in Hector’s dolphin distribution were maintained (e.g. Table 3). The well-known seasonal movement of Hector’s dolphins (e.g. increased detections over warm months and decreased in cooler months) around Banks Peninsula (e.g., Dawson and Slooten, 1988; Rayment et al., 2010; MacKenzie and Clement, 2016) was apparent within Lyttelton Harbour over the whole monitoring period. At the same time, a clear gradient of decreasing dolphin detections from outer harbor regions to inner waters near the port (e.g., Dawson et al., 2013; Brough et al., 2014; Leunissen et al., 2019) was also evident and remained noticeable across all sampling years and seasons. As a whole, the temporal and spatial gradients in Hector’s dolphin acoustic detections throughout the monitoring program reflect those of pre-construction distributions noted by previous boat-based findings of Hector’s dolphin use within Lyttelton Harbour (Brough et al., 2014; Enviser, 2018) as well as other bays and harbors around Banks Peninsula (e.g., Clement, 2005; Rayment et al., 2010; Brough et al., 2019).
Yet, our findings demonstrated a general decline in DPMs over the 2019 construction period that remained reduced through to May 2020. Hence, finer scale models were used to further investigate this result, focusing on the potential effects of impact pile-driving isolated from other anthropogenic sources of noise (e.g., vibratory piling and vessel traffic) at two of the three inner harbor mooring site separately. These mooring-specific models confirmed the spatial variation in Hector’s dolphin reactions to impact pile-driving noise levels that Leunissen et al. (2019) findings had suggested. Closer to the port, dolphin detections declined more linearly (MM6) with an increase in the hourly-averaged LE,p levels, and when combined with increased wind speeds. Strong winds are known to generate more underwater noise (Cato, 2008). Within this shallower and less protected region of the inner harbor, winds can sweep out from the entrance of the port or over Peninsula cliffs creating steep, choppy waves. Model results at the further away of the inner harbor moorings (MM5) indicated that dolphin presence was affected less by local wind conditions and more by factors that helped the propagation of the impact piling noise. Despite being less than one kilometer from MM6, this location is more protected by hills surrounding the port and the port infrastructure (e.g. buildings, storage towers). Instead, dolphin detections at this location tended to decline, after an initial decrease, once received hourly-averaged noise levels exceeded 125 dB and when warmer water temperatures helped propagate impact piling sound noise faster and further than in cooler water conditions (e.g. NPL, 2025). Sample size was insufficient to examine any trends at MM7, the mooring located furthest up the harbor and closest to the port. However, this result was not unexpected as pre-construction baseline monitoring indicated low use of this area by Hector’s dolphins; a third to half the number of detections reported at MM6, and particularly low over winter months.
Together, these models support mixed responses from Hector’s dolphins to an increase in impact piling noise that varied depending on their distance from the source and the combination of environmental conditions at the time. Along with the MMO sighting data, these spatial results also provide evidence that dolphins are not completely abandoning the port region while piling is underway nor were they being forced into less suitable, inner harbor habitats (e.g. indicated by a lack of increase in detections near or west of MM7) by impact pile-driving noise, a concern considered in the design of the monitoring program (Enviser, 2018).
4.1 Behavioral responses
Leunissen and Dawson (2018) hypothesized that changes in Hector’s dolphin behavior due to impact pile-driving noise (710 mm diameter piles) were possible within 1,120 m of the source, based on captive harbor porpoise data (Kastelein et al., 2013). Harbor porpoise is an acoustically analogous narrow-band, high-frequency species (NBHF) to Hector’s dolphin. Our finer scale models indicated that declines in dolphin acoustic detections occurred at similar distances to those predicted by Leunissen and Dawson (2018), notwithstanding the cruise berth construction involving larger sized piles than the earlier study of Cashin Quay wharf rebuild. Together with the matched dataset findings and along with the short-term recovery analysis, these results indicate that dolphins likely moved away temporarily from the inner harbor regions (or the vicinity of the acoustic recorders) while pile-driving activity was underway, rather than simply reducing their vocalizations. A gradual return of dolphins back into the inner harbor region over several days may explain why the mean post-piling detections at MM7 were still lower than pre-piling rates after four days of recovery while detection rates at the other two inner harbor sites had returned.
An unexpected result was the decline in acoustic detections that occurred et all four mooring sites prior to the commencement of pile-driving activity, similar to the findings of Brandt et al. (2016). The Brandt et al. (2016) study on wind farm locations in the German North Sea found no obvious explanations for their pre-piling detection decreases and suggested that increased shipping activity related to preparatory work combined with low wind conditions may have deterred dolphins. It is possible that Hector’s dolphins may be associating up-coming piling activity with particular vessels or preparatory work and moving away in advance. Alternatively, the short-term recovery results may be influenced by natural, diel movements of the dolphins, as evidenced by similar trends at MM2. Despite this mooring being out of the impact pile-driving noise range, mean pre-piling detections still declined prior to the start of any new piling activity. The combined post-piling recovery rates also showed potentially cyclic patterns in detections roughly every 12 hours. Dawson et al. (2013) found acoustic detection rates of Hector’s dolphins within nearby Akaroa Harbour declined in the late evenings within inner harbor sites while increasing in the outer harbor. It might be that the commencement time of pile-driving activity; for instance, early each morning, corresponded with the dolphins’ daily movements in or out of Lyttelton Harbour.
It is also plausible that both pre- and post-piling detection rates changed over the course of the year as our definition of the pre-piling period was not a true control. Instead, it represented a break in time between successive pile-driving sessions rather than a true lack of any effect. It is possible that over time and with successive piling activity, both pre- and post-piling mean detections might have simultaneously declined over the construction period. With an absence of information on other port or construction preparation activities, and a lack of data around this species diel patterns, we were not able to explore these factors further. However, future investigations are needed to understand the triggers behind this pre-piling decrease and how other factors, such as diel and seasonal patterns, might influence such results.
Despite some evidence as to the success of the mitigation measures employed to reduce the immediate and short-term effects of impact pile-driving noise on Hector’s dolphins, our findings suggest that a longer-term decline in dolphin detections took place starting in 2019 that had not returned to 2018 levels by May 2020. The timing of these declines and our behavioral findings suggest the ongoing disturbance from construction activities (over 14-months) was a potential factor for some animals choosing not to remain within the harbor and/or to venture as far into the harbor as they might have previously (e.g. post-piling declines at the middle harbor site). A review of dredging impacts by Todd et al. (2015) describes a range of similar reactions by other marine mammal species to varying levels of construction activities globally.
Nonetheless, this paper did not examine if declines in dolphin detections were due solely to the construction projects as our models were not exhaustive and did not investigate all possible factors that might influence the dolphins’ presence in the harbor. In particular, we did not consider any effects of larger-scale environmental drivers, such as marine heatwaves affecting the wider Banks Peninsula region and east coast South Island waters (e.g., Perkins-Kirkpatrick et al., 2019; Salinger et al., 2019; Montie, 2023). Marine heatwaves have been associated with decreases in survival, abundance and reproduction in dolphin species in other parts of the world (e.g., Sprogis et al., 2018; Wild et al., 2019; Thorne et al., 2022). Further information and analyses are needed to clarify the main factor(s) associated with the decline and the length of its persistence.
4.2 Temporary threshold shift
The main mitigation measure instigated by LPC was a protective TTS shut down zone based on concerns raised by Leunissen et al. (2019) that Hector’s dolphins may tolerate noise at levels which could induce TTS if there was a sufficient reward (e.g. foraging opportunities). To our knowledge, this was the first instance of a TTS-based mitigation measure for marine mammals to be used in port infrastructure construction projects in Aotearoa New Zealand. The size of this zone was initially based on Leunissen and Dawson (2018) estimate, that was subsequently enlarged for the cruise berth build based on the use of larger piles and the United States’ National Marine Fisheries Services updated underwater acoustic thresholds guidelines (NMFS, 2018). If a Hector’s dolphin (or other marine mammal) was spotted near the boundary of the designated shut down zone, the MMO would notify the pile driver to be on stand-by, ready to shut down piling if the dolphin crossed into the protected zone. Comparisons between MMO visual and acoustic data suggested that dolphins were actively avoiding the MMOZ area when piling was underway, and with the relatively low number of shut-downs (n = 15) over the 14 months of piling, these results highlight the effectiveness of the other management procedures associated with the MMOZ (i.e. ramping up, soft-starts, stand-bys).
5 Conclusions
In Aotearoa New Zealand, any underwater noise management or mitigation measures associated with port infrastructure developments come through resource consent conditions, either offered by the port or enforced through the local regulator. As a result, this process provides considerable inconsistencies and uncertainty for both operators and regulators. Yet, the propagation of underwater noise generated from discrete, high-impact or impulsive noise (such as pile-driving) has been well documented, and with location-specific data (i.e. seafloor sediment composition, depth, stratification and temperature), can be modelled and effects predicted (e.g., NMFS, 2024). Hence, there are several applicable guidelines and thresholds available for these types of activities (e.g. OSPAR, 2009; EC, 2017; ACCOBAMS, 2019; Merchant et al., 2022) and which the ports of Aotearoa New Zealand should look to adopt.
The hearing and physiological effects of such high-impact noises are generally immediate and site-dependent, similar to a point source effect (Hildebrand, 2009; Duarte et al., 2021). As a result, this study found that enforced shut-downs based on TTS standards, requirements for qualified observers, and limits on pile-driving timing (e.g., daily or seasonal) helped minimize, if not avoid, these types of impacts on Hector’s dolphin near the port in Lyttelton Harbour. But appropriate thresholds for behavioral disturbance of marine mammals from high-impact noise sources are more difficult, being both species and context influenced (Southall et al., 2021). Our findings suggest that the mitigation measures used during the cruise berth development may have also helped limit some of the short-term impacts of pile-driving to similar or reduced levels to those of the earlier Cashin Quay rebuild (e.g., Leunissen and Dawson, 2018; Leunissen et al., 2019), despite the larger-scale duration, number and sizes of piles used with the cruise berth.
However, there was also evidence of a longer-term decline in Hector’s dolphin detections in the vicinity of the port lasting at least several months after construction had ceased. Previous studies have noted that the duration of noise disturbances may be an important factor in the extent of behavioral reactions of species and among individual animals (e.g., Southall et al., 2007; Bailey et al., 2010). The construction of the cruise berth spanned two austral summer seasons (December 2018 to February 2019, and December 2019 to February 2020). The decline in Hector’s dolphin detections over the length of the monitoring suggest the ongoing disturbance from construction activities, and in particular pile-driving activities, is a possible factor.
Overall, these results indicate that, while pile-driving noise reduction measures were successful at protecting against TTS/PTS impacts, additional measures are warranted for any future LPC infrastructure development projects to avoid longer term impacts on Hector’s dolphin use of the harbor. The most obvious mitigation measures for ports in Aotearoa New Zealand to consider are noise abatement systems that reduce noise production at the source; e.g. piling method, pile size/type, bubble curtains (Würsig et al., 2000; Bailey et al., 2010; Dähne et al., 2017), limit piling aspects of projects to less than a 12-month duration and to use seasonal restrictions. A combination of both visual and passive acoustic monitoring for marine mammals during construction is also beneficial as real-time impacts can be monitored and the effectiveness of mitigation assessed.
Data availability statement
The raw data supporting the conclusions of this article will be made available by the authors, without undue reservation.
Ethics statement
Ethical approval was not required for the study involving animals in accordance with the local legislation and institutional requirements because the study used passive acoustic (listening) data to study wild population of dolphins.
Author contributions
DC: Conceptualization, Formal Analysis, Investigation, Methodology, Project administration, Resources, Validation, Visualization, Writing – original draft, Writing – review & editing, Funding acquisition. HP: Data curation, Formal Analysis, Methodology, Software, Visualization, Writing – original draft, Writing – review & editing. CL: Conceptualization, Funding acquisition, Project administration, Resources, Writing – original draft, Writing – review & editing. MP: Data curation, Formal Analysis, Investigation, Methodology, Resources, Software, Validation, Visualization, Writing – original draft, Writing – review & editing.
Funding
The author(s) declare that financial support was received for the research and/or publication of this article. Lyttelton Port Company, operating as a commercial entity, funded the monitoring program, analysis, final report and manuscript as part of their local government resource consent requirements to undertake their infrastructure upgrades.
Acknowledgments
The initial sampling framework, mooring locations and implementation of the acoustic moorings (e.g. setting and set-up) was designed by LPC, Styles Group Acoustics and Jared Pettersson (Enviser). Later additions to the monitoring program were implemented under advice from Darran Humpheson (formerly at AECOM-Christchurch), Simon Childerhouse (Blue Planet Marine), Styles Group and Cawthron. Vision Environment (Australia) was responsible for retrieving, deploying and maintaining the acoustic moorings along with LPC staff. Melanie Burns of Environment Canterbury (ECAN) supplied the rainfall, temperature, and wind datasets used in the general models. Jessica Schattschneider, Paula Casanovas and Simon Childerhouse all provided useful advice and help with this manuscript.
Conflict of interest
Author CL was employed by Lyttelton Port Company.
The remaining authors declare that the research was conducted in the absence of any commercial or financial relationships that could be construed as a potential conflict of interest.
The authors declare that this study received funding from Lyttelton Port Company. The funder had the following involvement in the study: study design and logistics, decision to publish and preparation of the manuscript. The funder had no involvement in data collect, analysis or interpretation of results.
Generative AI statement
The author(s) declare that no Generative AI was used in the creation of this manuscript.
Publisher’s note
All claims expressed in this article are solely those of the authors and do not necessarily represent those of their affiliated organizations, or those of the publisher, the editors and the reviewers. Any product that may be evaluated in this article, or claim that may be made by its manufacturer, is not guaranteed or endorsed by the publisher.
Supplementary material
The Supplementary Material for this article can be found online at: https://www.frontiersin.org/articles/10.3389/fmars.2025.1554536/full#supplementary-material
References
ACCOBAMS Agreement on the Conservation of Cetaceans of the Black Sea, Mediterranean Sea and contiguous Atlantic area (2019). Methodological Guide: Guidance on underwater noise mitigation measures (ACCOBAMS-MOP5/2019/Doc 31Rev1). Available online at: https://accobams.org/wp-content/uploads/2019/04/MOP7.Doc31Rev1_Methodological-Guide-Noise.pdf (Accessed December 1, 2024).
Bailey H. B., Senior B., Simmons D., Rusin J., Picken G., and Thompson P. M. (2010). Assessing underwater noise levels during pile-driving at an offshore windfarm and its potential effects on marine mammals. Mar. Pollut. Bull. 60, 888–897. doi: 10.1016/j.marpolbul.2010.01.003
Baker C. S., Boren L., Childerhouse S., Constantine R., van Helden A., Lundquist D., et al. (2019). Conservation status of New Zealand marine mammals 2019. New Zealand Threat Classification Series 29 (Wellington: Department of Conservation). Available online at: https://www.doc.govt.nz/globalassets/documents/science-and-technical/nztcs29entire.pdf (Accessed December 1, 2024).
Bartoń K. (2023). MuMIn: Multi-Model Inference. R package version 1.47.5. Available online at: https://CRAN.R-project.org/package=MuMIn (Accessed October 1, 2024).
Bolker B. M., Brooks M. E., Clark C. J., Geange S. W., Poulsen J. R., Stevens M. H. H., et al. (2009). Generalized linear mixed models: a practical guide for ecology and evolution. Trends Ecol. Evol. 24, 127–135. doi: 10.1016/j.tree.2008.10.008
Boorstein A. (2024). Cargo ships keep getting bigger, and infrastructure is racing to keep up (Smithsonian Magazine). Available online at: https://www.smithsonianmag.com/smart-news/cargo-ships-keep-getting-bigger-and-infrastructure-is-racing-to-keep-up-180984043/ (Accessed December 1, 2024).
Brandt M. J., Diederichs A., Betke K., and Nehls G. (2011). Responses of harbour porpoises to pile driving at the Horns Rev II offshore wind farm in the Danish North Sea. Mar. Ecol. Prog. Ser. 421, 205–216. doi: 10.3354/meps08888
Brandt M. J., Dragon A. C., Diederichs A., Schubert A., Kosarev V., Nehls G., et al. (2016). Effects of offshore pile driving on harbour porpoise abundance in the German Bight. Available online at: http://bioconsult-sh.de/site/assets/files/1573/1573.pdf (Accessed December 1, 2024).
Brough T., Rayment W., Slooten E., and Dawson S. (2019). Fine scale distribution for a population of New Zealand’s only endemic dolphin (Cephalorhynchus hectori) shows long-term stability of coastal hotspots. Mar. Mamm. Sci. 35, 140–163. doi: 10.1111/mms.12528
Brough T., Slooten E., and Dawson S. (2014). Marine mammals and Port Lyttelton development: An environmental impact assessment (Prepared for Lyttelton Port of Christchurch October 2014). Available online at: https://www.lpc.co.nz/wp-content/uploads/2016/06/Appendix-16.pdf (Accessed December 1, 2024).
Burnham K. P. and Anderson D. R. (2002). Model selection and multi-model inference: a practical information-theoretic approach. 2nd edition (New York, NY: Springer).
Burnham K. P., Anderson D. R., and Huyvaert K. P. (2011). AIC model selection and multimodel inference in behavioral ecology: some background, observations, and comparisons. Behav. Ecol. Sociobiol. 65, 23–35. doi: 10.1007/s00265-010-1029-6
Burnham R. E., Vagle S., O’Neill C., and Trounce K. (2021). The efficacy of management measures to reduce vessel noise in critical habitat of southern resident killer whales in the Salish Sea. Front. Mar. Sci. 8. doi: 10.3389/fmars.2021.664691
Cato D. H. (2008). “Ocean ambient noise: its measurement and its significance to marine animals,” in Paper Presented at the Proceedings of the Institute of Acoustics - Underwater Noise Measurement, Impact and Mitigation, (Southampton). 1–9.
CEDA Central Dredging Association (2011). CEDA Position Paper: Underwater sound in relation to dredging (CEDA Environment Commission Working Group). Available online at: https://dredging.org/media/ceda/org/documents/resources/cedaonline/2011-11_ceda_positionpaper_underwatersound_v2.pdf (Accessed December 1, 2024).
Chapman N. R. and Price A. (2011). Low frequency deep ocean ambient noise trend in the Northeast Pacific Ocean. J. Acoust. Soc Am. 129, EL161–EL165. doi: 10.1121/1.3567084
Clement D. M. (2005). Distribution of Hector’s dolphin (Cephalorhynchus hectori) in relation to oceanographic features. PhD thesis (Dunedin, New Zealand: University of Otago).
Dähne M., Tougaard J., Carstensen J., Rose A., and Nabe-Nielsen J. (2017). Bubble curtains attenuate noise from offshore wind farm construction and reduce temporary habitat loss for harbour porpoises. Mar. Ecol. Prog. Ser. 580, 221–237. doi: 10.3354/meps12257
Dawson S., Fletcher D., and Slooten E. (2013). Habitat use and conservation of an endangered dolphin. Endangered Species Res. 21, 45–54. doi: 10.3354/esr00508
Dawson S. M. and Thorpe C. W. (1990). A quantitative-analysis of the sounds of Hector's dolphin. Ethology. 86 (2), 131–145. doi: 10.1111/j.1439-0310.1990.tb00424.x
Dawson S. M. and Slooten E. (1988). “Hector’s dolphin Cephalorhynchus hectori: Distribution and abundance,” in Reports of the International Whaling Commission, Special Issue 9. Biology of the genus Cephalorhynchus, eds. Brownell R. L. and G. P. Donovan G. P. (Cambridge: International Whaling Commission), 315–324.
DIT Department of Infrastructure and Transport (2023). Underwater piling and dredging noise guidelines; EHTM Attachment 7E. Environment and Heritage Technical Manual (Government of South Australia). Available online at: https://www.dit.sa.gov.au/standards?a=955077 (Accessed December 1, 2024).
Duarte C. M., Chapuis L., Collin S. P., Costa D. P., Devassy R. P., Eguiluz V. M., et al. (2021). The soundscape of the Anthropocene Ocean. Science 371, eaba4658. doi: 10.1126/science.aba4658
EC European Commission. Off. J. Eur. Union (2017). Commission Decision (EU) 2017/848 of 17 May 2017 Laying Down Criteria and Methodological Standards on Good Environmental Status of Marine Waters and Specifications and Standardised Methods for Monitoring and Assessment, and Repealing Decision 2010/477/EU. Available online at: https://eur-lex.europa.eu/legal-content/EN/TXT/PDF/?uri=CELEX:32017D0848 (Accessed December 1, 2024).
Edrén S. M. E., Teilmann J., Dietz R., and Carstensen J. (2004). Effect from the construction of Nysted offshore wind farm on seals in Rødsand seal sanctuary based on remote video monitoring (Roskilde: Technical report to Energi E2 A/S. National Environmental Research Institute). Available online at: https://pure.au.dk/portal/en/publications/effect-from-the-construction-of-nysted-offshore-wind-farm-on-seal (Accessed December 1, 2024).
Enviser (2018). Marine mammal monitoring plan – LPC cruise berth project. Prepared for Lyttelton Port Company. Enviser Limited Report Ref: 1005.MMMP. Available online at: https://www.lpc.co.nz/wp-content/uploads/2020/11/MMMP-for-cruise-berth-Nov-2018-Issued.pdf (Accessed December 1, 2024).
Hildebrand J. A. (2009). Anthropogenic and natural sources of ambient noise in the ocean. Mar Ecol. Prog. Ser. 395, 5–20. doi: 10.3354/meps08353
Kastelein R. A., van Heerden D., Gransier R., and Hoek L. (2013). Behavioural responses of a harbor porpoise (Phocoena phocoena) to playbacks of broadband pile driving sounds. Mar. Environ. Res. 92, 206–214. doi: 10.1016/j.marenvres.2013.09.020
Leunissen E. (2017). Underwater noise from pile driving and its impact on Hector’s dolphins in Lyttelton Harbour, New Zealand. Master of Science (Dunedin, New Zealand: University of Otago).
Leunissen E. and Dawson S. (2018). Underwater noise levels of pile-driving in a New Zealand harbour, and the potential impacts on endangered Hector’s dolphins. Mar. Pollut. Bull. 135, 195–204. doi: 10.1016/j.marpolbul.2018.07.024
Leunissen E. M., Rayment W. J., and Dawson S. M. (2019). Impact of pile-driving on Hector’s dolphin in Lyttelton Harbour, New Zealand. Mar. Pollut. Bull. 142, 31–42. doi: 10.1016/j.marpolbul.2019.03.017
LPC Lyttelton Port Company (2023). Upokohue/Hector’s dolphin Science Summary (Produced by LPC). Available online at: https://www.lpc.co.nz/wp-content/uploads/2024/09/LPC_HectorsDolphins_ScienceSummary_oct23_Final_HighRes-1.pdf (Accessed December 1, 2024).
MacGillivray A. O., Ainsworth L. M., Zhao J., Dolman J. N., Hannay D. E., Frouin-Mouy H., et al. (2022). A functional regression analysis of vessel source level measurements from the Enhancing Cetacean Habitat and Observation (ECHO) database. J. Acoust. Soc. America 152, 1547–1563. doi: 10.1121/10.0013747
MacGillivray A. O., Li Z., Hannay D. E., Trounce K. B., and Robinson O. M. (2019). Slowing deep-sea commercial vessels reduces underwater radiated noise. J. Acoust. Soc. America 146, 340–351. doi: 10.1121/1.5116140
MacKenzie D. I. and Clement D. M. (2016). Abundance and distribution of WCSI Hector’s dolphin (New Zealand Aquatic Environment and Biodiversity Report No. 168). Available online at: https://www.mpi.govt.nz/dmsdocument/12129-AEBR-168-Abundance-and-distribution-of-WCSI-Hectors-dolphin (Accessed December 1, 2024).
Madsen P. M., Wahlberg M., Tougaard J., Lucke K., and Tyack P. L. (2006). Wind turbine underwater noise and marine mammals: implications of current knowledge and data needs. Mar. Ecol. Prog. Ser. 309, 279–295. doi: 10.3354/meps309279
Merchant N. D., Fristrup K. M., Johnson M. P., Tyack P. L., Witt M. J., Blondel P., et al. (2015). Measuring acoustic habitats. Methods Ecol. Evol. 6, 257–265. doi: 10.1111/2041-210X.12330
Merchant N. D., Putland R. L., André M., Baudin E., Felli M., Slabbekoorn H., et al. (2022). A decade of underwater noise research in support of the European Marine Strategy Framework Directive. Ocean Coast Manag. , 228. doi: 10.1016/j.ocecoaman.2022.106299
Montie S. (2023). Seasonal trends in marine heatwaves highlight vulnerable coastal ecoregions and historic change points in New Zealand. N. Z. J. Mar. Freshw. Res.. 58 (2), 274–299. doi: 10.1080/00288330.2023.2218102
MOT Ministry of Transport (2022). New Zealand freight and supply chain issues paper: Preparing our freight and supply chain system for the future (New Zealand Ministry of Transport consultation document). Available online at: https://consult.transport.govt.nz/policy/new-zealand-freight-and-supply-chain-issues/ (Accessed December 1, 2024).
NMFS National Marine Fisheries Service (2018). Revisions to technical guidance for assessing the effects of anthropogenic sound on marine mammal hearing (version 2.0). U.S. Dept. of Commer., NOAA (NOAA Technical Memorandum NMFS-OPR-59). Available online at: https://www.fisheries.noaa.gov/s3/2023-05/TECHMEMOGuidance508.pdf (Accessed December 1, 2024).
NMFS National Marine Fisheries Service (2024). Update to: Technical guidance for assessing the effects of anthropogenic sound on marine mammal hearing (version 3.0): Underwater and In-Air Criteria for Onset of Auditory Injury and Temporary Threshold Shifts. U.S. Dept. of Commerce, NOAA (NOAA Technical Memorandum NMFS-OPR-71). Available online at: https://repository.library.noaa.gov/view/noaa/66184 (Accessed December 1, 2024).
Nowacek D. P., Thorne L. H., Johnston D. W., and Tyack P. L. (2007). Responses of cetaceans to anthropogenic noise. Mamm. Rev. 37, 81–115. doi: 10.1111/j.1365-2907.2007.00104.x
NPL National Physical Laboratory (2025). Technical Guides - Speed of sound in sea water (Middlesex, UK: NPL Management Ltd). Available at: http://resource.npl.co.uk/acoustics/techguides/soundseawater/index.html (Accessed December 1, 2024).
OSPAR Convention for the Protection of the Marine Environment of the North-East Atlantic (2009). Assessment of the environmental impact of underwater noise (OSPAR Commission. Publication number 436/2009). Available online at: https://qsr2010.ospar.org/media/assessments/p00436_JAMP_Assessment_Noise.pdf (Accessed December 1, 2024).
Perkins-Kirkpatrick S. E., King A. D., Cougnon E. A., Grosese M. R., Oliver E. C. J., Holbrook N. J., et al. (2019). The role of natural variability and anthropogenic climate change in the 2017/18 Tasman Sea marine heatwave. Bull. Am. Meteorol. Soc. 100 (1), S1–S6. doi: 10.1175/BAMS-D-18-0116.1
Pine M. K., Nikolich K., Martin B., Morris C., and Juanes F. (2020). Assessing auditory masking for management of underwater anthropogenic noise. J. Acoust. Soc Am. 147, 3408–3417. doi: 10.1121/10.0001218
Rayment W., Dawson S., and Slooten E. (2009a). Trialling an automated passive acoustic detection (T-POD) with Hector’s dolphins (Cephalorhynchus hectori). J. Mar. Biol. Assoc. United Kingdom 89, 1015–1022. doi: 10.1017/S0025315409003129
Rayment W., Dawson S., and Slooten E. (2010). Seasonal changes in distribution of Hector’s dolphin at Banks Peninsula, New Zealand: implications for protected area design. Aquat. Conserv.: Mar. Freshwater Ecosyst. 20, 106–116. doi: 10.1002/aqc.v20:1
Rayment W., Dawson S., Slooten E., Bräger S., DuFresne S. D., and Webster T. (2009b). Kernel density estimates of alongshore home range of Hector’s dolphins at Banks Peninsula, New Zealand. Mar. Mamm. Sci. 25, 537–556. doi: 10.1111/j.1748-7692.2008.00271.x
Reeves R. R., Dawson S. M., Jefferson T. A., Karczmarski L., Laidre K., O’Corry-Crowe G., et al. (2013). “Cephalorhynchus hectori,” in The IUCN Red List of Threatened Species 2013:e.T4162A44199757. doi: 10.2305/IUCN.UK.2013-1.RLTS.T4162A44199757.en
Richardson W. J. (1995). “Chapter 1 – Introduction,” in Marine Mammals and Noise. Eds. Richardson W. J., Greene C. R., Malme C. I., and Thomson D. H. (Academic Press, San Diego, CA), 1–13. doi: 10.1016/B978-0-08-057303-8.50004-5
Salinger M. J., Renwick J., Behrens E., Mullan A. B., Diamond H. J., Sirguey P., et al. (2019). The unprecedented coupled ocean-atmosphere summer heatwave in the New Zealand region 2017/18: drivers, mechanisms and impacts. Environ. Res. Lett. 14, p.044023. doi: 10.1088/1748-9326/ab012a
Slabbekoorn N. B., van Opzeeland I., Coers A., Cate C., and Popper A. N. (2010). A noisy spring: the impact of globally rising underwater sound levels on fish. Trends Ecol. Evol. 25, 419–427. doi: 10.1016/j.tree.2010.04.005
Smith S. (2024). Economic impact of cruise tourism in New Zealand 2023-2024 (Blenheim, New Zealand: Prepared for Cruise Lines International Association Australasia and New Zealand Cruise Association). Available at: https://newzealandcruiseassociation.com/wp-content/uploads/2024/11/CLIA-NZ-Cruise-EIA-2023-24-FINAL.pdf (Accessed December 1, 2024).
Son W. J. and Cho I. S. (2022). Analysis of trends in mega-sized container ships using the K-means clustering algorithm. Appl. Sci. 12, 2115. doi: 10.3390/app12042115
Southall B. L., Bowles A. E., Ellison W. T., Finneran J. J., Gentry R. L., Greene C. R. Jr, et al. (2007). Marine mammal noise-exposure criteria: initial scientific recommendations. Bioacoustics 17, 273–275. doi: 10.1080/09524622.2008.9753846
Southall B. L., Finneran J. J., Reichmuth C., Nachtigall P. E., Ketten D. R., Bowles A. E., et al. (2019). Marine mammal noise exposure criteria: updated scientific recommendations for residual hearing effects. Aquat. Mamm. 45, 125–232. doi: 10.1578/AM.45.2.2019.125
Southall B. L., Nowacek D. P., Bowles A. E., Senigaglia V., Bejder L., and Tyack P. L. (2021). Marine mammal noise exposure criteria: assessing the severity of marine mammal behavioral responses to human noise. Aquat. Mamm. 47, 421–464. doi: 10.1578/AM.47.5.2021.421
Sprogis K. R., Christiansen F., Wandres M., and Bejder L. (2018). El Niño Southern Oscillation influences the abundance and movements of a marine top predator in coastal waters. Global Change Biol. 24, 1085–1096. doi: 10.1111/gcb.13892
Sutherland C., Hare D., Johnson P. J., Linden D. W., Montgomery R. A., and Droge E. (2023). Practical advice on variable selection and reporting using Akaike information criterion. Proc. R. Soc. B 290, 20231261. doi: 10.1098/rspb.2023.1261
Symonds M. R. and Moussalli A. (2011). A brief guide to model selection, multi-model inference and model averaging in behavioural ecology using Akaike’s information criterion. Behav. Ecol. Sociobiol. 65, 13–21. doi: 10.1007/s00265-010-1037-6
Thompson P. M., Lusseau D., Barton T., Simmons D., Rusin J., and Bailey H. (2010). Assessing the responses of coastal cetaceans to the construction of offshore wind turbines. Mar. Pollut. Bull. 60, 1200–1208. doi: 10.1016/j.marpolbul.2010.03.030
Thorne L. H., Heywood E. I., and Hirtle N. O. (2022). Rapid restructuring of the odontocete community in an ocean warming hotspot. Global Change Biol. 28, 6524–6540. doi: 10.1111/gcb.16382
Todd V. L., Todd I. B., Gardiner J. C., Morrin E. C., MacPherson N. A., DiMarzio N. A., et al. (2015). A review of impacts of marine dredging activities on marine mammals. ICES J. Mar. Sci./J. du Conseil 72, 328–340. doi: 10.1093/icesjms/fsu187
Tougaard J., Carstensen J., Henriksen O. D., Skov H., and Teilmann J. (2003). Short-term effects of the construction of wind turbines on harbour porpoises at Horns Reef (Hedeselskabet, Roskilde: NERI technical report to Techwise A/S, HME/362–02662). Available online at: https://pure.au.dk/portal/en/publications/short-term-effects-of-the-construction-of-wind-turbines-on-harbou (Accessed December 1, 2024).
Tougaard J., Carstensen J., Teilmann J., Bech N. I., Skov H., and Henriksen O. D. (2005). Effects of the Nysted offshore wind farm on harbour porpoises. Annual Status Report for the T-POD Monitoring Program. Technical report to Energi E2 A/S. Available online at: https://tethys.pnnl.gov/sites/default/files/publications/Effects_of_the_Nysted_Offshore_Wind_Farm_on_Harbour_Porpoises.pdf (Accessed December 1, 2024).
UNCTAD United Nations Conference on Trade and Development (2022). Review of Maritime Transport 2022: Navigating stormy waters (Geneva: UNCTAD/RMT/2022 and Corr.1).
Wild S., Krüzen M., Rankin R. W., Hoppitt W. J. E., Gerber L., and Allen S. J. (2019). Long-term decline in survival and reproduction of dolphins following a marine heatwave. Curr. Biol. 29, 239–240. doi: 10.1016/j.cub.2019.02.047
WODA World Organization of Dredging Associations (2013). WODA Technical guidance on: Underwater sound in relation to dredging. Available online at: https://dredging.org/media/ceda/org/documents/resources/cedaonline/2013-06-woda-technicalguidance-underwatersound_lr.pdf (Accessed December 1, 2024).
Wood S. N. (2017). Generalized additive models: An introduction with R. 2nd edition (New York: Chapman and Hall/CRC).
Würsig B., Greene C. R., and Jefferson T. A. (2000). Development of an air bubble curtain to reduce underwater noise of percussive piling. Mar. Environ. Res. 49, 79–93. doi: 10.1016/S0141-1136(99)00050-1
Keywords: Hector’s dolphin, pile-driving, underwater noise, mitigation, port, construction
Citation: Clement DM, Pavanato H, Lenky C and Pine MK (2025) Immediate and short-term effects of pile-driving on Hector’s dolphin in Lyttelton Harbour, Aotearoa New Zealand. Front. Mar. Sci. 12:1554536. doi: 10.3389/fmars.2025.1554536
Received: 02 January 2025; Accepted: 14 April 2025;
Published: 21 May 2025.
Edited by:
Carlos Gravato, University of Lisbon, PortugalReviewed by:
Francine Kershaw, Natural Resources Defense Council, United StatesJavier Almunia, Loro Parque Foundation, Spain
Copyright © 2025 Clement, Pavanato, Lenky and Pine. This is an open-access article distributed under the terms of the Creative Commons Attribution License (CC BY). The use, distribution or reproduction in other forums is permitted, provided the original author(s) and the copyright owner(s) are credited and that the original publication in this journal is cited, in accordance with accepted academic practice. No use, distribution or reproduction is permitted which does not comply with these terms.
*Correspondence: Deanna M. Clement, ZGVhbm5hLmNsZW1lbnRAY2F3dGhyb24ub3JnLm56