- 1Department of Life Sciences and Systems Biology, University of Turin, Turin, Italy
- 2Institute for the Study of Anthropogenic Impacts and Sustainability in the Marine Environment, National Research Council of Italy, Campobello di Mazara, Italy
- 3Department of Ecoscience, Aarhus University, Roskilde, Denmark
- 4Brookfield Zoo Chicago’s Sarasota Dolphin Research Program, Mote Marine Laboratory, Sarasota, FL, United States
- 5Department of Biology, Syracuse University, Syracuse, NY, United States
- 6Biology Department, Woods Hole Oceanographic Institution, Woods Hole, MA, United States
- 7Department for the Ecology of Animal Societies, Max Planck Institute of Animal Behavior, Konstanz, Germany
Understanding cetacean echolocation behavior is important for effective population monitoring and conservation. Using passive acoustic monitoring (PAM), researchers can listen for the biosonar clicks produced by echolocating animals to estimate both diurnal and seasonal variations in their presence and activity. Furthermore, if species-specific click rates are known, cue counting techniques can be used to provide an estimate of population density. This study investigated the click rates of wild bottlenose dolphins tagged with sound and movement recording DTAG3s during health assessments over the West Florida Shelf in the Gulf of Mexico to quantify individual variability and explore factors influencing click production. We observed modest but significant differences in click rates across individuals, and higher click rates during dives compared to inter-dive surface intervals. Within dives, dive depth was the most important in shaping click rates, reflecting that dolphins adjust their echolocation behavior to tailor their acoustic field of view based on both predator-prey distance and their proximity to other large reflectors such as the ocean bottom. Click rates also showed subtle diurnal peaks at dawn and dusk, aligning with increased foraging efforts. The findings lay the groundwork for bottlenose dolphin density estimation using the cue counting technique and underscore the importance of incorporating region-specific information on foraging ecology and diving behavior into models of click rates. Our study provides the first estimate of bottlenose dolphin click rates but calls for further research to refine these click rate estimates to facilitate acoustic monitoring of delphinids.
1 Introduction
Effective wildlife management is important for safeguarding vulnerable marine species from increasing anthropogenic pressures. To support conservation and management efforts, accurately estimating population sizes and tracking population trends are fundamental goals. Traditional methods for assessing wildlife abundance, such as visual surveys and physical mark-capture techniques, are often challenging to implement in marine environments, particularly for elusive, nocturnal, or deep-dwelling species. In recent years, passive acoustic monitoring (PAM) has become a key tool for studying cetacean populations in a non-invasive manner, enabling population estimates for effective conservation and management (Van Parijs et al., 2009).
PAM offers several advantages, including the ability to detect vocalizing animals over long distances, operate continuously regardless of light or weather conditions, and facilitate automated data collection. This makes PAM especially suited for monitoring remote or difficult-to-access environments. By recording and analyzing the acoustic signals produced by cetaceans, PAM provides insights into their presence, behaviors, and habitat use across various regions and seasons. Compared to traditional boat surveys, PAM is often more cost-effective and may offer higher detection rates, particularly for species that spend limited time at the surface (Marques et al., 2013).
PAM can be employed to estimate population abundance or density, and to evaluate trends over time (Marques et al., 2013). One method applied for cetacean density estimation is cue counting, where the rate of detected acoustic signals from a given species is used to estimate animal population density under the assumption that the rate of cue production is constant (Marques et al., 2009). Cue counting has been applied to estimate population density of a few echolocating beaked whales (Hildebrand et al., 2015; Küsel et al., 2011; Marques et al., 2009), sperm whales (Li et al., 2021) and harbor porpoises (Amundin et al., 2022), and for manatees based on communication signals (Rycyk et al., 2022). Converting cue counts to population density requires accounting for the probability that not all cues are detected, correction factors to account for classification mistakes, and a multiplication factor based on the average cue rate for the signal of interest, assuming a constant cue rate that is independent of density (Marques et al., 2009).
However, natural variations in cue rates exist and can affect the estimate of population density. These variations have been investigated for echolocating sperm whales (Physeter macrocephalus, Barkley et al., 2024; Marques et al., 2023), narwhals (Monodon monoceros, Marques et al., 2024), and beaked whales (Mesoplodon densirostris and Ziphius cavirostris, Warren et al., 2017), and for communication signals of bowhead whales (Balaena mysticetus, Blackwell et al., 2021), right whales (Eubalaena japonica, Marques et al., 2011), minke whales (Balaenoptera acutorostrata, Martin et al., 2013), and gray whales (Eschrichtius robustus, Guazzo et al., 2019). While cue counting for echolocating animals assumes a constant click rate, click rates of these echolocating predators are intrinsically dynamic. Click rates and interclick intervals depend on factors including species and body size (Jensen et al., 2018), depth and bathymetry (Jensen et al., 2020; Madsen et al., 2013), prey distance (Au and Benoit-Bird, 2003; Jensen et al., 2009; Ladegaard et al., 2019), environment (Ladegaard and Madsen, 2019) and even prior knowledge of resource distribution (Fais et al., 2015). Variations in echolocation behavior and cue rates across populations highlight the need for population-specific cue rate estimates (Marques et al., 2024) and emphasize that factors such as prey availability, behavioral state of the group (Barkley et al., 2024) and individual variability in response to tagging should also be considered.
Bottlenose dolphins (Tursiops truncatus) are a widely distributed species and, arguably, the most studied cetacean, with extensive research on biosonar parameters and biosonar behavior both in human care (Au, 1993), in the wild (Jensen et al., 2009) and across field sites (Wahlberg et al., 2011). Despite this, we have little information on their typical click rates and the mechanistic factors or behavioral drivers underlying variation in individual click rates. This gap highlights the need for focused research to better understand delphinid click rates and their implications for effective population monitoring. Therefore, this study aims to estimate bottlenose dolphin click rates from tag data and to investigate the intra- and inter-individual variability in echolocation activity.
2 Materials and methods
2.1 Field tagging
Bottlenose dolphins were temporarily caught over the West Florida Shelf in the Gulf of Mexico, approximately 43–77 km offshore of Sarasota, FL, USA, in water depths of 24–37 m, for a dolphin health assessment and tagging program (Wells, 2024). During catch-and–release operations, dolphins were caught using a hoop-net while bow-riding and briefly restrained on a floating mat for a health assessment, sampling, and attachment of a long-term, dorsal-fin-mounted satellite-linked location and dive data recording SPLASH tag (Wildlife Computers, Redmond, WA, USA), under continuous veterinary supervision (Fahlman et al., 2018). Dolphins were also equipped with high-resolution audio and movement recording DTAG3s (Johnson and Tyack, 2003; Johnson et al., 2009). After carefully scrubbing the skin of the animal, the tag was attached through four sterilized suction cups, approximately halfway between the blowhole and the dorsal fin as also done for inshore dolphin health assessments (Kragh et al., 2019).
The DTAG3 continuously recorded sound on two hydrophones (16-bit, sample rate 240 kHz, flat frequency response within ± 2 dB between 0.5 and 80 kHz). A pressure sensor, along with a tri-axial accelerometer and magnetometer, was sampled at a rate of either 200 or 250 Hz. Before sampling, the signals were processed through an analog low-pass filter with a −3 dB cut-off frequency set to one-third of the sampling rate. Tags were programmed to release after approximately 24 hours, but some detached before this time (Table 1). Once the tags detached, they floated to the surface, where they were recovered by tracking their VHF beacons from a boat using a 216–220 MHz 3-element handheld Yagi antenna (Advanced Telemetry Systems, Isanti, MN, USA) and an R1000 or R5000 VHF receiver (Communications Specialists, Inc., Orange, CA, USA).
2.2 Permitting
Fieldwork was conducted under National Marine Fisheries Service Scientific Research Permit #20455–02 issued to R.S.W., and with IACUC approval from Mote Marine Laboratory, reviewed annually, and Syracuse University (#22-001).
2.3 Processing of tag recordings
All tag recordings were audited in MATLAB R2024a (The MathWorks, Inc., Natick, MA, USA) using DTAG-toolbox codes (https://www.soundtags.org/). Each recording was manually reviewed in 10-second intervals. Acoustic data were downsampled to 60 kHz, and both amplitude envelopes and spectrograms (Hamming window, FFT size of 512, 50% overlap, 80 dB dynamic range) were generated. These visualizations were displayed alongside a synchronized dive profile for comprehensive analysis. For this study, click series (animals echolocating for food or for navigating their environment) and foraging buzzes (a rapid series of echolocation clicks emitted at high repetition rates, typically associated with prey capture attempts and close-range targeting, Wisniewska et al., 2014) were labeled manually. Focal dolphin’s click series and buzzes (emitted by the tagged animal) were differentiated from non-focal click series by the increased low-frequency energy associated with recording at an extreme off-axis angle and from low-frequency sounds coupling through the tissues (Johnson et al., 2009). To improve subsequent post-processing of biosonar clicks, click series that overlapped with significant high-frequency noise or conspecific clicks were labeled for additional quality checking of click extraction.
An automated click detector was implemented to extract echolocation clicks from each labeled click series. The audio signal was first filtered with a 6-pole Butterworth high-pass filter with -3 dB cutoff frequency of 10–80 kHz. A dynamic threshold was used whereby the instantaneous signal envelope was calculated, and a default threshold was estimated based on the distribution of the envelope values, with a lower limit of -66 dB and an upper threshold limit of -40 dB. The time of the peak of each click exceeding this threshold was extracted. A blanking time of 10 ms was used to exclude subsequent clicks within this time frame, most often reflections of the outgoing sonar click. The click detector was tested on several animals with an interactive display to verify that clicks were correctly detected with these settings. All click series labeled for a subsequent quality check (because they overlapped with conspecific clicks or other noise that might interfere with a fully automated detection algorithm) were visualized in an interactive graphical user interface, that displayed the signal envelope as well as the time and intensity, angle-of-arrival (in degrees), and inter-click interval of all detected clicks.
2.4 Dive behavior and movement context
Custom-made MATLAB R2024a codes, together with the freely available DTAG-toolbox scripts (https://www.soundtags.org/), were used to process data from each tag. First, the depth time series for each animal was cropped to the time from when the animal was released to when the tag fell off, and the minimum depth set to -0.1 to remove a few surface artifacts. We then pooled data across tags to define dive events based on a statistically determined breakpoint depth. First, dives were identified using the finddives function from the DTAG toolbox, using a 2-meter depth threshold to exclude surface noise. The maximum depth of dives from all tags was then pooled and analyzed with a kernel density estimation (KDE) method with a 2 m Gaussian kernel. This KDE plot showed a bimodal distribution with the two modes separated around 8 m, so we chose an 8 m threshold to define a dive.
For each tag, dives deeper than 8 m were identified using the finddives function, and periods between the end of one and the start of the next dive were considered as a surfacing period. For each dive, we extracted the start and end time, the maximum dive depth, as well as the number of foraging buzzes contained within the dive. Movement data (accelerometer and magnetometer) were downsampled to 25 Hz for analysis and calibrated using the DTAG toolbox. We computed the animal’s pitch, roll, and compass heading from calibrated data by estimating the animal’s expected orientation at the surface. (Johnson, 2011) and updated following any tag slides along the dolphin’s body during the deployment. We used the pitch, roll and heading of the animal together with a Kalman-filtered estimate of speed from the kalmanspeedest function from the DTAG toolbox to calculate the dead-reckoned track across the deployment, with the ptrack function. Finally, we used the horizontal x-y coordinates of the track bounded at the start and end of the dive to compute the track tortuosity, reflecting how circuitous the dead-reckoned track was (Benhamou, 2004). Tortuosity represents the extent of deviation from a straight line, with higher tortuosity (closer to 1) indicating a more complex, indirect path, and low tortuosity indicating a more direct path.
2.5 Data analysis
2.5.1 Biosonar click rates
To reduce the effects of catch-and-release effort on echolocation activity, each dataset was constrained to the period from the onset of clicking and until the tag fell off the animal (tag-off). For each tag, the click rate (clicks/second) was calculated as the total number of detected clicks divided by the time between the onset of clicking and tag-off. The time spent echolocating was calculated as the total duration of the labeled click series divided by the time between the onset of clicking and tag-off. Since buzzes were emitted at much lower amplitude and would not be as suitable for PAM, buzz clicks were not included as clicks in the estimated click rates. Hourly click rates were then calculated in one-hour non-overlapping blocks from the onset of clicking until tag-off, with the last (partial) block ignored. Inter-individual differences in hourly click rates were analyzed with the Kruskal-Wallis test (significance set at p < 0.05) and Dunn’s post hoc test (significance set at p < 0.001) in R (http://www.R-project.org/).
2.5.2 Diurnal variation in click rate
To assess diurnal variation in click rates, a General Additive Mixed Model (GAMM) was applied using the gam function of the mgcv package in R (Wood and Wood, 2015). All statistical models were selected based on the Akaike Information Criterion (Akaike, 2011). The GAMM used a Gaussian distribution with an identity link function. The click rate was set as the response variable, while local time was used as a predictor. To account for individual variability, deployment ID was included as a random effect.
2.5.3 Click rate as a function of behavioral context
Click rates were calculated for each dive and surface period as the number of clicks divided by dive duration or surface period duration. We performed a Mann-Whitney U test with the default wilcox.test function in R to investigate the relationship between click rates and context (dive vs surface).
To investigate the relationship between click rate and behavior during dives, we fitted a GAMM (gam function, mgcv package) with a Gaussian family and identity link function, with a response variable of click rate and predictor variables including maximum dive depth, mean tortuosity and buzz rate (number of buzzes in a dive divided by dive duration). We included dive depth as it may be associated with prey availability, with deeper dives potentially leading to different foraging dynamics. Tortuosity was incorporated to capture the complexity of the dolphins’ movement patterns, as more tortuous paths may require adjustments in click rates to ensonify a more rapidly changing field of view. Buzz rate serves as an indicator of foraging behavior. To account for individual variability, we included deployment ID as a random effect in our models.
3 Results
3.1 DTAG deployments
In total, 5 bottlenose dolphins were temporarily caught during 2022–2023 health assessments over the West Florida Shelf. Two animals were caught in September 2022, two in May 2023, and one in September 2023. Of these, only one was a female (tt22_263a). She had a tag attachment duration of only 0.67 hours and was excluded from further analysis. The remaining animals were males, with tag attachment durations of 5.4 hours to 25 hours (Table 1). The dolphins conducted dives deeper than 8m within 4.45 - 11.90 s of being released, began echolocating within 0.4 seconds - 49 min of being released, and emitted their first foraging buzzes within 7–125 min after release.
3.2 Echolocation behavior and click rates
During tag deployments, animals exhibited extensive diving activity (8 dives/hr on average per animal), with foraging behavior marked by frequent buzzing sounds (n = 3085 buzzes, range 216–1804 buzzes per tag, Figure 1). Almost half of the total tag time was characterized by echolocation activity (41.09%, range 17.75% – 60.62%).
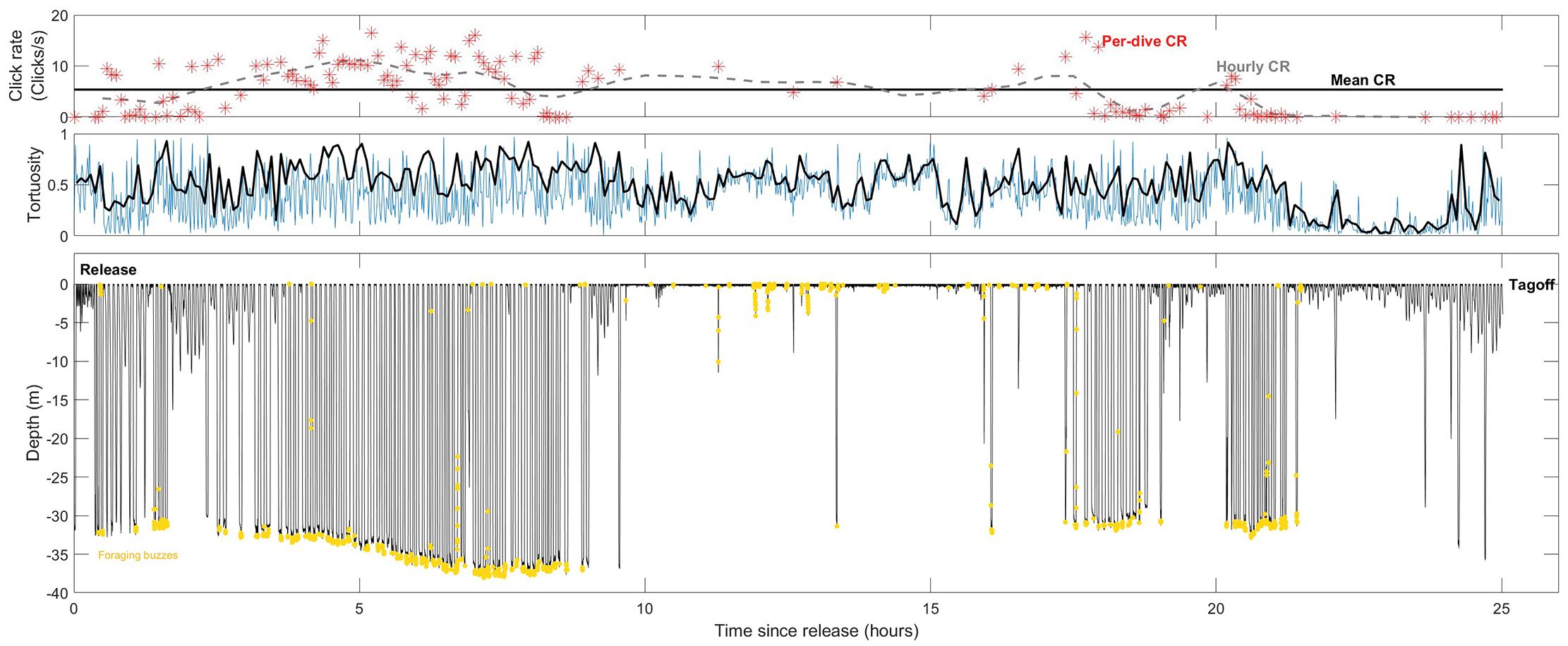
Figure 1. Diving behavior and click rates for a single offshore bottlenose dolphin. Top: Click rates measured in three different ways: Mean click rate across the entire tag deployment (black solid line); hourly click rates (gray dashed line) and per-dive click rates for dives exceeding 8 m (red star). Middle: Dead-reckoned track tortuosity, indicating how straight the horizontal component of the track is (lower values) measured in 1-min (blue) and 5-min (black) segments. Bottom: Depth profile (black) and foraging buzzes (yellow) over time.
Click rates varied widely across individual animals (2.87 – 7.68 clicks/s, Table 2) but averaged 4.94 clicks per second across the 4 analyzed tag deployments. While click rates fluctuated throughout the deployment period, no consistent pattern with time since tagging was observed (Figure 2). Individual tags showed differences in both the average hourly click rates and click rates during surface and dive phases, with dive-related click rates consistently higher than those at the surface.
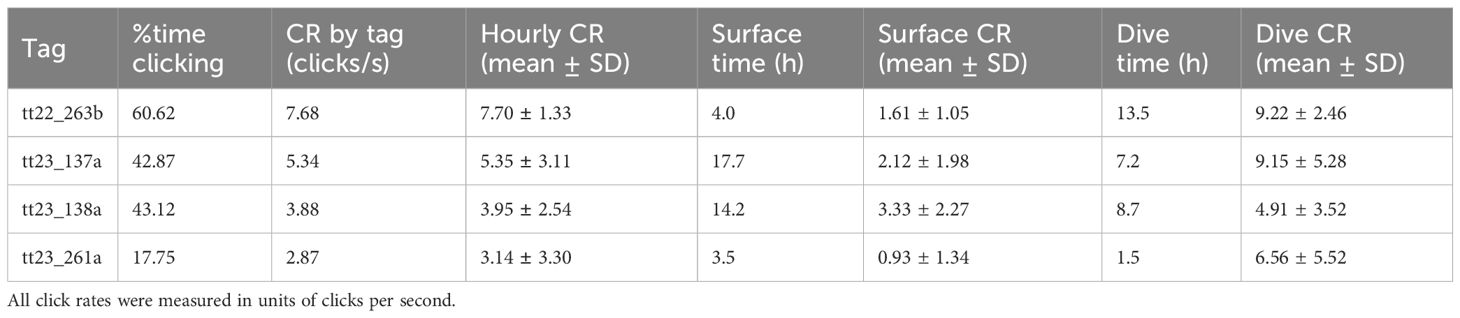
Table 2. Variation in echolocation behavior and click rates: Percentage of time spent echolocating, click rate (CR) by tag, by hour into tag, during dives, and during surface (inter-dive) periods.
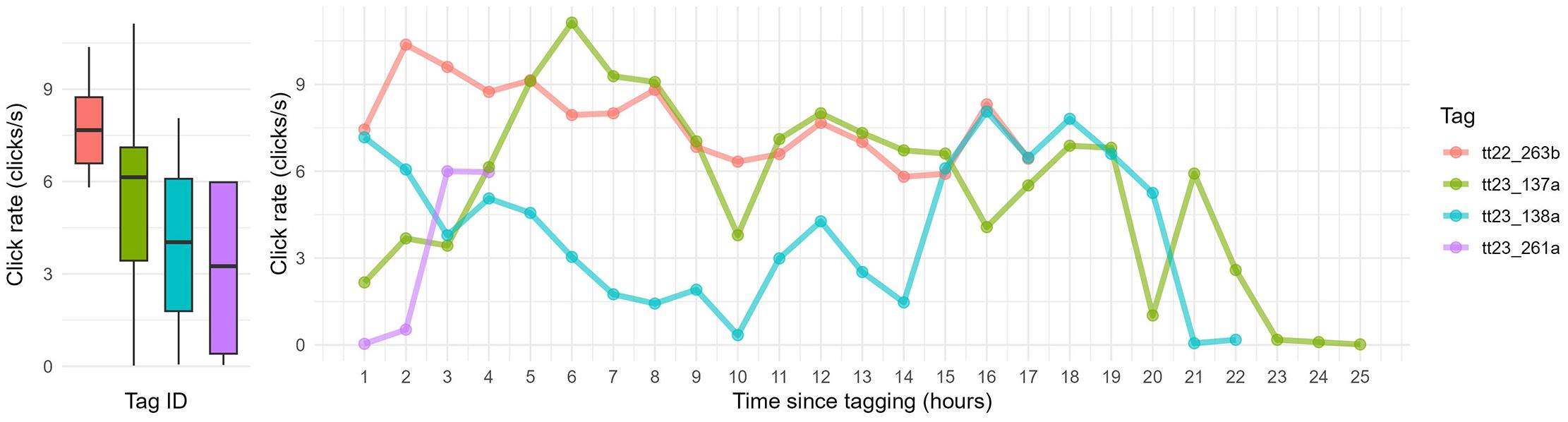
Figure 2. Average click rates and variation. The boxplots display the median, interquartile range, and outliers of hourly click rates extracted for each tag. The right-hand plot shows hourly click rates as a function of time since release from the health assessment.
There were significant differences in hourly CRs across individuals (Kruskal-Wallis: chi-squared = 19.026, df = 3, p < 0.001). Using a Dunn’s post hoc test for pairwise comparisons between tags, significant differences were observed between `tt22_263b` and `tt23_138a` (Z = 4.03, p < 0.001, adjusted p = 0.0003), as well as between `tt22_263b` and `tt23_261a` (Z = 2.89, p = 0.0038, adjusted p = 0.0228).
3.3 Click rates as a function of time-of-day
The diurnal variation model accounted for 25.3% of the deviance in click rates (R2 = 0.243). Time of day (local) exhibited a significant non-linear effect (edf = 5.43, F = 3.82, p = 0.00072), with lower click rates observed around midday and higher rates about one hour before dusk (7:30 - 8:15 pm) and dawn (6:30 - 7:30 am) (Figure 3). The intercept was estimated at 7.65 clicks/s (SE = 0.64). The random effect of tags was significant (edf = 2.70, F = 45.69, p < 0.001), indicating considerable variability in click rates between tag deployments.
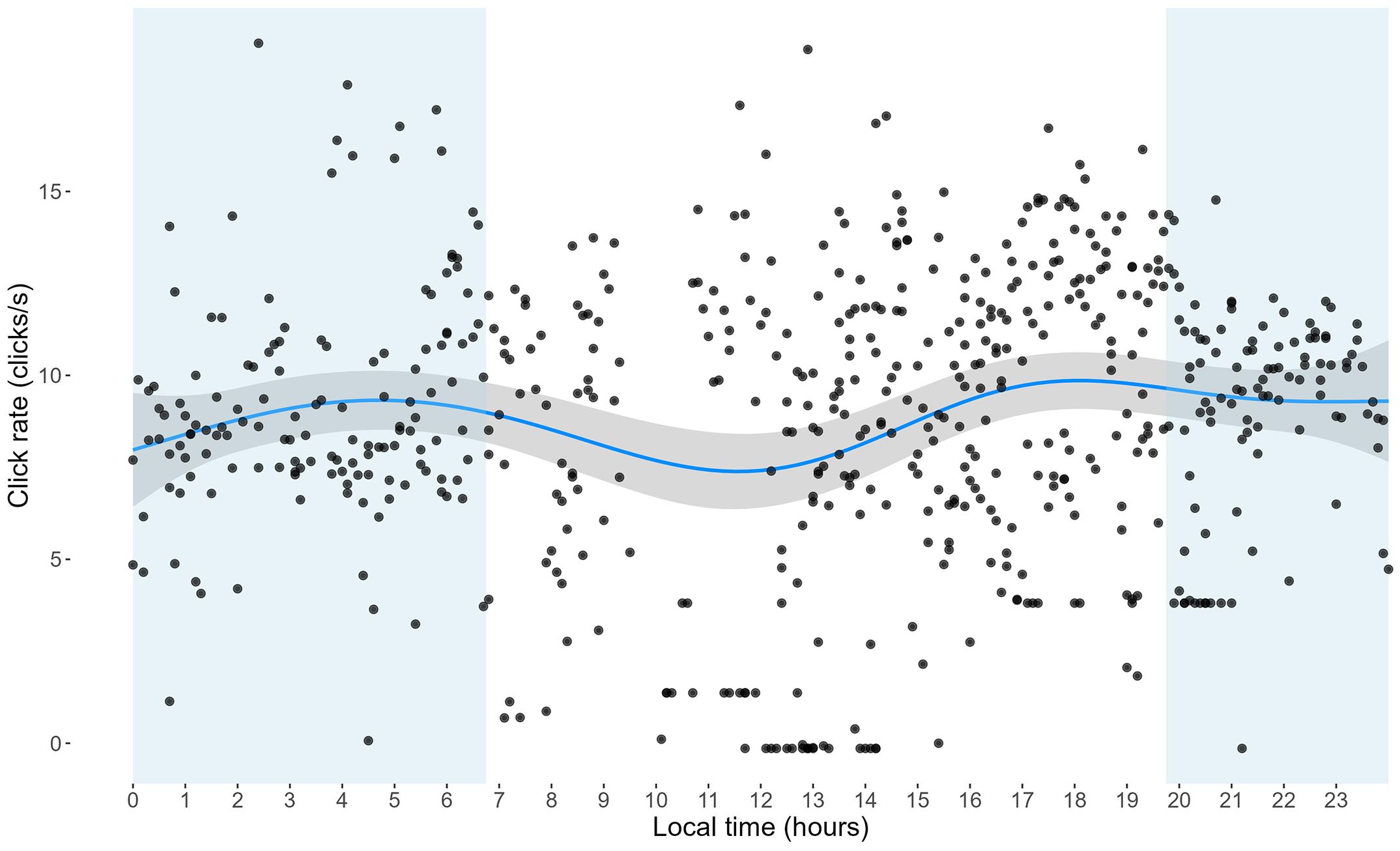
Figure 3. Variation in click rates as a function of time of day. The plot highlights variations across different times of the day estimated from the GAMM. The grey shaded area represents the 95% confidence interval. The blue shaded areas represent nighttime hours.
3.4 Click rate variability with context
The diving context had a significant influence on click rates (Mann-Whitney U test: W = 303595, p < 0.001), with higher clicking rates during dives than during periods near the surface (Figure 4).
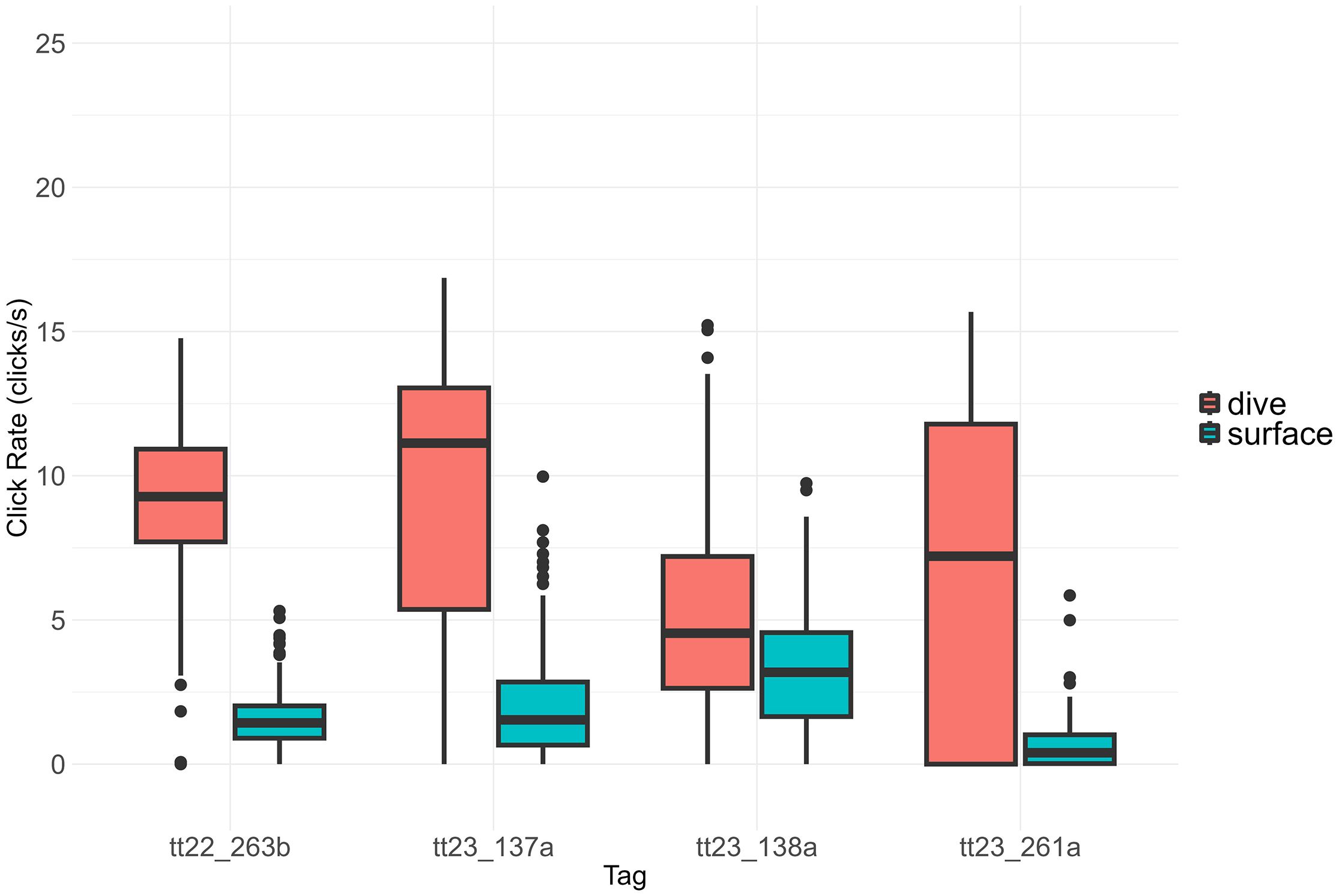
Figure 4. Click rate variation as a function of diving behavior. The median click rate is represented by the bold line within each box, while the whiskers indicate the interquartile range. Significant differences in click rates were observed between the two contexts (Mann-Whitney U test, p < 0.001).
Behavior within each dive significantly influenced click rate variation (Figure 5). The GAMM revealed a significant non-linear effect of maximum dive depth (edf = 8.707, F = 22.057, p < 0.001) and buzz rate (edf = 6.121, F = 2.402, p = 0.0195) but no significant effect of mean tortuosity (edf = 1.000, F = 0.398, p = 0.528). The model had an adjusted R2 of 0.516 and explained 53.3% of the deviance. The intercept was estimated at 9.255 clicks/s (SE = 1.847). The random effect of individual tags was significant (edf = 2.926, F = 17. 244, p < 0.001), indicating considerable variability in click rates among individuals.
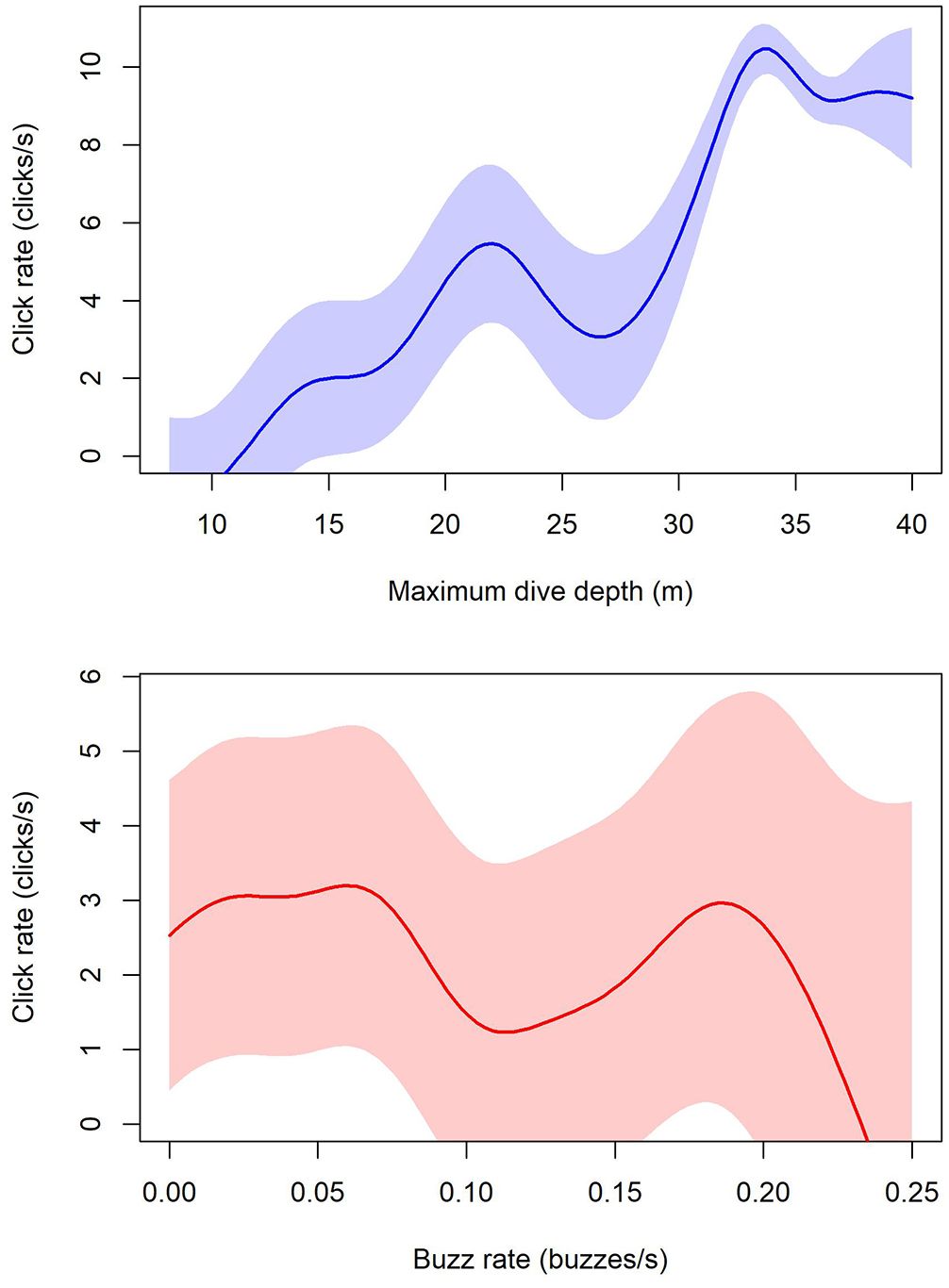
Figure 5. Effect of dive depth and buzz rate on click rates. The plot illustrates the significant non-linear relationship estimated from the GAMM, between click rates and maximum dive depth in meters (above), and buzz rate (below), analyzed for each dive deeper than 8m. The black line represents the mean smooth term, and the shaded area represents the 95% confidence interval of the smooth term.
4 Discussion
4.1 Click rates of offshore dolphins
The present study aimed to increase our understanding of bottlenose dolphin echolocation behavior in the wild to improve acoustic population monitoring efforts. our findings provide the first estimate of echolocation click rates for offshore bottlenose dolphins on a continental shelf, an essential step for density estimation based on cue-counting methods. Besides the conservation applications, our findings also help us to understand habitat use of bottlenose dolphins in this continental shelf ecosystem, which is important to mitigate potential threats for these populations (Wells and Fahlman, 2023).
Acoustic cue counting has been increasingly used to estimate the population density of echolocating toothed whales, largely due to their reliance on biosonar for locating and capturing prey (Amundin et al., 2022; Hildebrand et al., 2015; Küsel et al., 2011; Li et al., 2021; Marques et al., 2009). These studies have demonstrated that passive acoustic monitoring (PAM) can generate reliable abundance estimates using cue counting techniques. However, to ensure accurate density estimates, the cue rate must represent a long-term average throughout the survey period. Extended silent periods or other temporal fluctuations in cue production rates reduce the likelihood of detecting passing animals on acoustic recorders. Consequently, achieving reliable density estimates with PAM requires not only an understanding of a species’ biosonar characteristics for designing detection and classification algorithms, but also knowledge of its biosonar behavior and foraging ecology.
Despite extensive monitoring efforts, the cue counting method has not yet been applied for population density estimation of bottlenose dolphins or other smaller delphinids. The preliminary cue rate estimates presented here for offshore bottlenose dolphins on the West Florida Shelf lay the groundwork for local density estimation using acoustic monitoring techniques. In addition, these findings may serve as a stepping stone to extend cue-based density estimation to other populations in similar habitats, thereby strengthening the utility of PAM for marine mammal population assessment and ecosystem monitoring.
4.2 Low temporal variation in echolocation click rates
We found a relatively stable biosonar click rate of 4.94 clicks per second across tags, with some variation both between individuals (ranging from 2.87 clicks/s to 7.68 clicks/s) and within individuals over time. Temporal fluctuations did not seem to be associated with the catch-and-release event. Most individuals quickly resumed diving behavior (within few seconds), clicking to find prey (within less than one hour), and foraging (with first foraging buzz within 7–125 min), indicating a rapid return to baseline behavior following health assessments.
The individual with the highest click rate, both overall and per hour, also spent more time diving than the others. Click rates were significantly higher during dives than during near-surface periods, likely reflecting increased echolocation activity as the dolphins actively foraged for prey, in addition to gradual adjustments of interclick intervals as the maximum search ranges change. In contrast, lower click rates at the surface may correspond to periods of rest, social interaction, or a reduced need for continuous echolocation, though occasional surface buzzes suggest some behavioral plasticity in feeding strategies across the water column.
The link between click rate and diving behavior might suggest that large-scale diurnal activity shifts would impact click rates. We observed slight diel fluctuations in click rates, with a peak at 5 AM and at 6 PM, and a marked decrease around 12 AM. However, while significant, these diurnal changes in click rates were relatively small and unlikely to affect cue counting methods where detections are accumulated across much longer time periods. It is also important to note that only two dolphins contributed data for a full diel cycle, making it challenging to generalize too much to population-level diurnal activity changes without additional data.
4.3 Foraging context shapes biosonar click rates
Foraging context significantly influenced biosonar click rates. Our findings revealed a distinct difference between surface intervals and dives, even though the click rates analyzed are based solely on periods of regular clicks and exclude the rapid sequences characteristic of buzzes. Therefore, this change is a consequence of decreasing search click intervals and click duty cycle rather than an artefact of changes to click rates during terminal prey captures.
The impact of buzz rates on overall click rates was relatively minor, suggesting that this change is less associated with changes in prey density (Madsen et al., 2013) or gradual adjustments to decreasing range to target (i.e. automatic gain control) (Au and Benoit-Bird, 2003; Jensen et al., 2009, 2020). Click rate was also not influenced by increased turning rates (i.e., tortuosity) associated with tracking and capturing more mobile or highly patchy prey such as seen for Blainville’s beaked whales (Madsen et al., 2013).
Most dives in this area reach the seafloor depth. Depth seems to have a significant effect on click rates even after accounting for gross differences between dives and near-surface behaviors. This likely reflects gradual adjustments of biosonar field-of-view, in particular biosonar search range, as delphinids approach major obstacles such as the ocean bottom and features on the bottom (Verfuss et al., 2005). This change in overall click rates matches the decreasing interclick intervals as a function of depth shown for Gulf of Mexico bottlenose dolphins using acoustic array recordings (Simard et al., 2010) and shown using acoustic tags on other species such as Risso’s dolphins (Jensen et al., 2020) and sperm whales (Isojunno and Miller, 2018; Isojunno et al., 2021). A recent study of click rates in narwhals also demonstrated that depth is a significant factor in influencing both probability of clicking as well as click rates, and therefore should be accounted for in cue counting methods for density estimation when possible (Marques et al., 2024).
4.4 How much does habitat matter?
Our findings underscore that echolocation click rates are strongly influenced by diving behavior and local foraging conditions – factors that may vary substantially among different regions. While there are no studies examining geographic differences in click rates of bottlenose dolphins, biosonar interclick intervals vary across populations (Wahlberg et al., 2011) suggesting that average click rates likely do as well. As a result, click rates estimated in one area may not be generalizable across habitats and it is important to estimate local click rates whenever possible. Admittedly, acquiring site-specific click rates by acoustically tagging small delphinids remains challenging. An alternative approach might be to use time-depth recording tags to understand local diving behavior and foraging ecology and then model click rates for subsequent density estimation based on in-situ diving behavior. Emerging technologies such as the pole-mounted Tag Attachment Device (TADpole, Moore et al., 2024) might facilitate such approaches in the future by making it easier to tag and instrument free-swimming, bow-riding delphinids with dorsal fin pin-mounted satellite-linked tags.
4.5 Conclusions
In conclusion, our study provided the first estimate of biosonar click rates for bottlenose dolphins on the West Florida Shelf, providing critical information that can enable acoustic density estimation of bottlenose dolphins using the cue counting method. As in previous studies, we found significant variability in click rates among animals, suggesting that additional data for refining cue rate estimates would further improve the accuracy of PAM-based population assessments.
Although click rate fluctuations due to diurnal rhythms and individual variability were relatively modest, our findings highlight the importance of incorporating local foraging ecology and dive-related changes in biosonar behavior when applying cue-counting methods for density estimation. Crucially, these results suggest that click rates derived in one habitat may not be readily transferred to other regions, underscoring the need for site-specific data collection efforts.
By identifying key drivers of echolocation behavior—particularly diving activity and foraging context—this work lays the groundwork for future acoustic population monitoring initiatives. Developing practical ways to estimate local click rates, such as via time-depth-location recording satellite-linked tags, will help refine density estimates for bottlenose dolphins and other delphinids. Ultimately, such improvements in passive acoustic methodologies will contribute to more robust population assessments and promote a better understanding of delphinid behavior and ecology in rapidly changing marine ecosystems.
Data availability statement
The raw data supporting the conclusions of this article will be made available by the authors, without undue reservation.
Ethics statement
The animal study was approved by Mote Marine Laboratory and Syracuse University IACUC committees and conducted under National Marine Fisheries Service Scientific Research Permit #20455-02 issued to R.S.W. The study was conducted in accordance with the local legislation and institutional requirements.
Author contributions
MB: Conceptualization, Formal Analysis, Investigation, Visualization, Writing – original draft, Writing – review & editing. EP: Funding acquisition, Methodology, Supervision, Writing – review & editing. JS: Data curation, Investigation, Resources, Writing – review & editing. RW: Funding acquisition, Investigation, Project administration, Resources, Writing – review & editing. FJ: Conceptualization, Funding acquisition, Investigation, Methodology, Project administration, Software, Supervision, Writing – original draft, Writing – review & editing.
Funding
The author(s) declare that financial support was received for the research, authorship, and/or publication of this article. MB was supported by PON Green REACT-EU DM 1061 doctoral funding. Field efforts to deploy tags were supported primarily by a grant to RSW from the Florida RESTORE Act Centers of Excellence Program, administered through Mote Marine Laboratory, and with additional support from Dolphin Quest via their General Science and Conservation Fund. The funders were not involved in the study design, collection, analysis, interpretation of data, the writing of this article, or the decision to submit it for publication.
Conflict of interest
The authors declare that the research was conducted in the absence of any commercial or financial relationships that could be construed as a potential conflict of interest.
Generative AI statement
The author(s) declare that no Generative AI was used in the creation of this manuscript.
Publisher’s note
All claims expressed in this article are solely those of the authors and do not necessarily represent those of their affiliated organizations, or those of the publisher, the editors and the reviewers. Any product that may be evaluated in this article, or claim that may be made by its manufacturer, is not guaranteed or endorsed by the publisher.
References
Akaike H. (2011). “Akaike’s information criterion,” in International encyclopedia of statistical science, ed. M. Lovric. (Berlin, Heidelberg: Springer), 25. doi: 10.1007/978-3-642-04898-2_110
Amundin M., Carlström J., Thomas L., Carlén I., Koblitz J., Teilmann J., et al. (2022). Estimating the abundance of the critically endangered Baltic Proper harbour porpoise (Phocoena phocoena) population using passive acoustic monitoring. Ecol. Evol. 12, e8554. doi: 10.1002/ece3.8554
Au W. W., Benoit-Bird K. J. (2003). Automatic gain control in the echolocation system of dolphins. Nature 423, 861–863. doi: 10.1038/nature01727
Barkley Y. M., Merkens K. P. B., Wood M., Oleson E. M., Marques T. A. (2024). Click detection rate variability of central North Pacific sperm whales from passive acoustic towed arrays. J. Acoust. Soc. America 155, 2627–2635. doi: 10.1121/10.0025540
Benhamou S. (2004). How to reliably estimate the tortuosity of an animal’s path: straightness, sinuosity, or fractal dimension? J. Theor. Biol. 229, 209–220. doi: 10.1016/j.jtbi.2004.03.016
Blackwell S. B., Thode A. M., Conrad A. S., Ferguson M. C., Berchok C. L., Stafford K. M., et al. (2021). Estimating acoustic cue rates in bowhead whales, Balaena mysticetus, during their fall migration through the Alaskan Beaufort Sea. J. Acoust. Soc. America 149, 3611–3625. doi: 10.1121/10.0005043
Fahlman A., McHugh K., Allen J., Barleycorn A., Allen A., Sweeney J., et al. (2018). Resting metabolic rate and lung function in wild offshore common bottlenose dolphins, Tursiops truncatus, near Bermuda. Front. Physiol. 9. doi: 10.3389/fphys.2018.00886
Fais A., Aguilar Soto N., Johnson M., Pérez-González C., Miller P. J. O., Madsen P. T. (2015). Sperm whale echolocation behaviour reveals a directed, prior-based search strategy informed by prey distribution. Behav. Ecol. Sociobiol. 69, 663–674. doi: 10.1007/s00265-015-1877-1
Guazzo R. A., Weller D. W., Europe H. M., Durban J. W., D’Spain G. L., Hildebrand J. (2019). Migrating eastern North Pacific gray whale call and blow rates estimated from acoustic recordings, infrared camera video, and visual sightings. Sci. Rep. 9, 12617. doi: 10.1038/s41598-019-49115-y
Hildebrand J. A., Baumann-Pickering S., Frasier K. E., Trickey J. S., Merkens K. P., Wiggins S. M., et al. (2015). Passive acoustic monitoring of beaked whale densities in the Gulf of Mexico. Sci. Rep. 5, 16343. doi: 10.1038/srep16343
Isojunno S., Miller P. J. (2018). Movement and biosonar behavior during prey encounters indicate that male sperm whales switch foraging strategy with depth. Front. Ecol. Evol. 6, 200. doi: 10.3389/fevo.2018.00200
Isojunno S., von-Benda-Beckmann A. M., Wensveen P. J., Kvadsheim P. H., Lam F. P. A., Gkikopoulou K. C., et al. (2021). Sperm whales exhibit variation in echolocation tactics with depth and sea state but not naval sonar exposures. Marine Mamm. Sci. 38, 682–704. doi: 10.1111/mms.12890
Jensen F. H., Bejder L., Wahlberg M., Madsen P. T. (2009). Biosonar adjustments to target range of echolocating bottlenose dolphins (Tursiops sp.) in the wild. J. Exp. Biol. 212, 1078–1086. doi: 10.1242/jeb.025619
Jensen F. H., Johnson M., Ladegaard M., Wisniewska D. M., Madsen P. T. (2018). Narrow acoustic field of view drives frequency scaling in toothed whale biosonar. Curr. Biol. 28, 3878–3885. doi: 10.1016/j.cub.2018.10.037
Jensen F. H., Keller O. A., Tyack P. L., Visser F. (2020). Dynamic biosonar adjustment strategies in deep-diving Risso’s dolphins driven partly by prey evasion. J. Exp. Biol. 223, jeb216283. doi: 10.1242/jeb.216283
Johnson M. (2011). "Measuring the orientation and movement of marine animals using inertial and magnetic sensors - a tutorial" in Fine-scale animal movement workshop., 1–22.
Johnson M., de S. A., Madsen P. T. (2009). Studying the behaviour and sensory ecology of marine mammals using acoustic recording tags: a review. Marine Ecol. Prog. Ser. 395, 55–73. doi: 10.3354/meps08255
Johnson M. P., Tyack P. L. (2003). A digital acoustic recording tag for measuring the response of wild marine mammals to sound. IEEE J. ocean. Eng. 28, 3–12. doi: 10.1109/JOE.2002.808212
Kragh I. M., McHugh K., Wells R. S., Sayigh L. S., Janik V. M., Tyack P. L., et al. (2019). Signal-specific amplitude adjustment to noise in common bottlenose dolphins (Tursiops truncatus). J. Exp. Biol. 222, jeb216606. doi: 10.1242/jeb.216606
Küsel E. T., Mellinger D. K., Thomas L., Marques T. A., Moretti D., Ward J. (2011). Cetacean population density estimation from single fixed sensors using passive acoustics. J. Acoust. Soc. America 129, 3610–3622. doi: 10.1121/1.3583504
Ladegaard M., Madsen P. T. (2019). Context-dependent biosonar adjustments during active target approaches in echolocating harbour porpoises. J. Exp. Biol. 222, jeb206169. doi: 10.1242/jeb.206169
Ladegaard M., Mulsow J., Houser D. S., Jensen F. H., Johnson M., Madsen P. T., et al. (2019). Dolphin echolocation behaviour during active long-range target approaches. J. Exp. Biol. 222, jeb189217. doi: 10.1242/jeb.189217
Li K., Sidorovskaia N. A., Guilment T., Tang T., Tiemann C. O. (2021). Decadal assessment of sperm whale site-specific abundance trends in the northern Gulf of Mexico using passive acoustic data. J. Marine Sci. Eng. 9 (5), 454. doi: 10.3390/jmse9050454
Madsen P. T., de Soto N. A., Arranz P., Johnson M. (2013). Echolocation in Blainville’s beaked whales (Mesoplodon densirostris). J. Comp. Physiol. A 199, 451–469. doi: 10.1007/s00359-013-0824-8
Marques C. S., Marques D. A., Blackwell S. B., Heide-Jørgensen M. P., Malinka C. E., Marques T. A. (2024). Narwhal (Monodon monoceros) echolocation click rates to support cue counting passive acoustic density estimation. J. Acoust. Soc. America 155, 891–900. doi: 10.1121/10.0024723
Marques T. A., Marques C. S., Gkikopoulou K. C. (2023). A sperm whale cautionary tale about estimating acoustic cue rates for deep divers. J. Acoust. Soc. America 154, 1577–1584. doi: 10.1121/10.0020910
Marques T. A., Munger L., Thomas L., Wiggins S., Hildebrand J. A. (2011). Estimating North Pacific right whale Eubalaena japonica density using passive acoustic cue counting. Endanger. Spec. Res. 13, 163–172. doi: 10.3354/esr00325
Marques T. A., Thomas L., Martin S. W., Mellinger D. K., Ward J. A., Moretti D. J., et al. (2013). Estimating animal population density using passive acoustics. Biol. Rev. 88, 287–309. doi: 10.1111/brv.12001
Marques T. A., Thomas L., Ward J., DiMarzio N., Tyack P. L. (2009). Estimating cetacean population density using fixed passive acoustic sensors: An example with Blainville’s beaked whales. J. Acoust. Soc. America 125, 1982–1994. doi: 10.1121/1.3089590
Martin S. W., Marques T. A., Thomas L., Morrissey R. P., Jarvis S., DiMarzio N., et al. (2013). Estimating minke whale (Balaenoptera acutorostrata) boing sound density using passive acoustic sensors. Marine Mamm. Sci. 29, 142–158. doi: 10.1111/j.1748-7692.2011.00561.x
Moore M. J., Lanagan T. M., Wells R. S., Kapit J., Barleycorn A. A., Allen J. B., et al. (2024). Development of single-pin, un-barbed, pole-tagging of free-swimming dolphins and sharks with satellite-linked transmitters. Animal Biotelemetry 12 (1), 6.
Rycyk A. M., Berchem C., Marques T. A. (2022). Estimating Florida manatee (Trichechus manatus latirostris) abundance using passive acoustic methods. JASA Expr. Lett. 2, 051202. doi: 10.1121/10.0010495
Simard P., Hibbard A. L., McCallister K. A., Frankel A. S., Zeddies D. G., Sisson G. M., et al. (2010). Depth dependent variation of the echolocation pulse rate of bottlenose dolphins (Tursiops truncatus). J. Acoust. Soc. America 127, 568–578. doi: 10.1121/1.3257202
Van Parijs S. M., Clark C. W., Sousa-Lima R. S., Parks S. E., Rankin S., Risch D., et al. (2009). Management and research applications of real-time and archival passive acoustic sensors over varying temporal and spatial scales. Marine Ecol. Prog. Ser. 395, 21–36. doi: 10.3354/meps08123
Verfuss U. K., Miller L. A., Schnitzler H. U. (2005). Spatial orientation in echolocating harbour porpoises (Phocoena phocoena). J. Exp. Biol. 208, 3385–3394. doi: 10.1242/jeb.01786
Wahlberg M., Jensen F. H., Aguilar Soto N., Beedholm K., Bejder L., Oliveira C., et al. (2011). Source parameters of echolocation clicks from wild bottlenose dolphins (Tursiops aduncus and Tursiops truncatus). J. Acoust. Soc. America 130, 2263–2274. doi: 10.1121/1.3624822
Warren V. E., Marques T. A., Harris D., Thomas L., Tyack P. L., Aguilar de Soto N., et al. (2017). Spatio-temporal variation in click production rates of beaked whales: Implications for passive acoustic density estimation. J. Acoust. Soc. America 141, 1962–1974. doi: 10.1121/1.4978439
Wells R. S. (2024). “Health and movements of Florida’s Gulf dolphins,” in Results and Impacts of the First Decade of the Florida RESTORE Act Centers of Excellence Program. Ed. Raineault N. A. (Florida Institute of Oceanography), 34–35. doi: 10.5281/zenodo.10735729
Wells R. S., Fahlman A. (2023). “Human impacts on dolphins: Physiological effects and Conservation,” in The Physiology of Dolphins. Eds. Fahlman A., Hooker S. (Zenodo: Elsevier), 267–284.
Wisniewska D. M., Johnson M., Nachtigall P. E., Madsen P. T. (2014). Buzzing during biosonar-based interception of prey in the delphinids Tursiops truncatus and Pseudorca crassidens. J. Exp. Biol. 217, 4279–4282. doi: 10.1242/jeb.113415
Keywords: click rates, bottlenose dolphin, Tursiops truncatus, echolocation, biosonar, density estimation, abundance
Citation: Baldachini M, Papale E, Shearer JM, Wells RS and Jensen FH (2025) Drivers of biosonar click rates in bottlenose dolphins (Tursiops truncatus) over the West Florida Shelf. Front. Mar. Sci. 12:1558655. doi: 10.3389/fmars.2025.1558655
Received: 10 January 2025; Accepted: 07 April 2025;
Published: 29 April 2025.
Edited by:
James Scott Maki, Marquette University, United StatesReviewed by:
David Moretti, Retired, Newport, RI, United StatesZhongchang Song, Xiamen University, China
Copyright © 2025 Baldachini, Papale, Shearer, Wells and Jensen. This is an open-access article distributed under the terms of the Creative Commons Attribution License (CC BY). The use, distribution or reproduction in other forums is permitted, provided the original author(s) and the copyright owner(s) are credited and that the original publication in this journal is cited, in accordance with accepted academic practice. No use, distribution or reproduction is permitted which does not comply with these terms.
*Correspondence: Frants H. Jensen, ZmplbnNlbkBlY29zLmF1LmRr