- 1Ecotoxicology Research Group, Department of Biological Sciences, Simon Fraser University, Burnaby, BC, Canada
- 2Ocean Pollution Research Unit (OPRU), Institute for the Oceans and Fisheries, University of British Columbia, AERL, Vancouver, BC, Canada
- 3School of Resources and Environmental Management, Simon Fraser University, Burnaby, BC, Canada
- 4Department of Biological Sciences, Simon Fraser University, Burnaby, BC, Canada
Macro- and microplastics and trace metals are significant pollutants in the marine environment and have been reported in all ecosystems around the world. The process of sorption/desorption of trace metals by macro- and microplastics is influenced by various factors, including the morphological characteristics of macro- and microplastics, their adsorption capacity, and environmental conditions. This research provides and discusses laboratory experimental findings on the sorption of trace metals cadmium (Cd), copper (Cu), lead (Pb), and zinc (Zn) by macro- and microplastics of polyethylene terephthalate (PETE) and high-density polyethylene (HDPE) within two contrasting marine intertidal sedimentary environments with high and low organic matter content under conditions of constant temperatures (T=- 4.0°C, T=+ 4.0°C, and T=+18.0°C). Our aim is to determine the effect of temperature on trace metal sorption onto macro- and microplastics. Temperature alters the metals’ sorption by plastic by altering the rate of reaching equilibrium and equilibrium concentration, whereas constant temperature had only a minor influence on the partitioning of trace metals. Sediment organic matter influences sorption dynamics at all three temperatures T=- 4.0°C, T=+ 4.0°C, and T=+18.0°C. This study enhances our understanding of how temperature can effect trace metals-plastic particle interactions in the marine intertidal sedimentary environment providing insight as to conditions that will create the greatest threat to higher trophic levels by providing an additional vector of Cd, Cu, Pb, and Zn exposure into benthic food webs.
1 Introduction
Plastics pollution is one of the fastest growing environmental challenges of our time. Plastic debris has accumulated in increasing amounts with time at a global scale. This is due to the wide-spread consumer use and over-consumption of polymeric materials, their continuing degradation, and high persistence in the environment (Bakir et al., 2020; Baztan et al., 2024; Khalid et al., 2021; Performance Evaluation, 2007; Thompson et al., 2024). The first type of the modern synthetic plastic,”bakelite”, appeared in 1907 (Leo Baekeland invented “bakelite”) and is now considered across disciplines as a key signature of the “plastic age” (William, 2008). The term “plastic” is used to define the class of materials called “polymers” (GESAMP, 2015). Plastics are made from a variety of polymeric materials, with the most common being: polyamides/nylons (PA), polyester (PES), low-density polyethylene (LDPE), high-density polyethylene (HDPE), polyethylene terephthalate (PETE), polyvinyl chloride (PVC), polypropylene (PP), polystyrene (PS), polyurethanes (PU), and acrylic (AC). The mass global production of plastic materials has increased significantly from around 5 million tons per year during the 1950s to over 460 million metric tons produced only in 2019, reaching an estimated cumulative plastic production of 10,000 to 20,000 million metric tons worldwide by 2020 and 2040, respectively (Baztan et al., 2024).
Plastic materials enter the environment through land-based sources such as anthropogenic debris emissions from rivers, littering and waste mismanagement in urban environments and industrial sectors in coastal cities and through ocean-based sources such as littering at sea, fishing, and shipping industries (Jambeck et al., 2015; GESAMP, 2015; Lebreton et al., 2017; Meijer et al., 2021; UNEP, 2021). The environmental factors (e.g., water, sediments, temperature, bacterial community), polymer properties (size, density, composition), and physical, chemical, and microbial processes results in a continual breakdown (e.g., deterioration, fragmentation) of large plastic items into smaller plastic fragments and particles commonly known as macroplastics (>5 mm in size), microplastics (1µm to <5 mm in size), and nanoplastics (0.001 µm to ≤ 1 μm in size) (Andrady, 2011; Arthur et al., 2009; Frias and Nash, 2019; Gigault et al., 2018; Hartmann et al., 2017).
Plastic waste became a concern to the global community in the 1960s (Napper and Thompson, 2020; Ryan, 2015; Thompson et al., 2024). Nowadays, macro- and microplastic particles are ubiquitous in almost all matrices of the surrounding multi-media environment (e.g., ocean bottom, seas, beaches, estuaries, sediments, rivers, lakes, soils, and air) and have been reported in all ecosystems on the planet (Kolda et al., 2024; Thompson et al., 2024; Rochman, 2018). A recent work by Eriksen et al. (2023) estimated that 82–358 trillion plastic particles, equivalent to 1.1–4.9 million tons by weight, float on the surface of the oceans. The COVID-19 pandemic also contributed to the single-use-plastic footprint via the massive use of personal protection equipment (PPE) waste, with an average of 8.4 million tons of COVID-19 pandemic-associated plastic emitted from 193 countries, from which close to 26,000 tons were discharged into the marine environment (Peng et al., 2019; Silva et al., 2021). This eventually lead to plastics being reported as the main fraction of marine and beach litter accounting from 60 to 95% of the total amount of all debris worldwide (Andrady, 2011; Cole et al., 2011; Derraik, 2002; Ryan et al., 2009; Thompson et al., 2024). Thus, plastics pollution in the coastal and marine environments is one of the greatest environmental problems of our time.
Microplastics are ubiquitous and pervasive in the sediments of marine intertidal/coastal area and estuaries (Hidalgo-Ruz et al., 2012; Lusher, 2015; Van Cauwenberghe et al., 2015a, 2015b; Wahyuningsih et al., 2018).The highest concentrations of microplastic detected on shorelines and beaches were 50,000 particles/kg dw on the East Frisian Islands (Liebezeit and Dubaish, 2012) and 285,673 particles/m3 on a beach in South Korea (Kim et al., 2015) with higher number/m2 found to be in surficial sediments (Martin et al., 2017; Moore, 2008). In coastal regions, 60- 90% of land-based macro- and microplastics influenced by hydrodynamic forces such as wind, waves and tidal movements may be washed ashore or deposited on beaches and intertidal zones. After entering the ocean, these plastics can either become buried in seafloor sediments or be transported further inland to the backshore (Ho and Not, 2019). Thus, most of the plastic debris accumulates in the sediment, particularly in the coastal zones (Harris, 2020; Harrison et al., 2014; Kazmiruk et al., 2018, 2024; Peng et al., 2017; Peng et al., 2018; Wahyuningsih et al., 2018). Hence coastal sediments and especially sediments of the intertidal zones can be seen as temporal sinks of macro- and microplastics in the marine environment (Browne et al., 2011; Peng et al., 2019).
A growing concern is that recent studies have reported that synthetic plastic materials both macro- and microparticles can bind to a wide range of pollutants including trace metals, polycyclic aromatic hydrocarbons (PAHs), dichlorodiphenyltrichloroethane (DDT), and polychlorinated biphenyls (PCBs). Due to the small size of macro- and microplastics and their high surface area-volume ratio, contaminants were shown to readily sorb to their surfaces (Boucher et al., 2016; Cole et al., 2011; Holmes et al., 2014; Kazmiruk et al., 2018, 2024; Munier and Bendell, 2018; Rochman et al., 2014; CCME (Canadian Council of Ministers of the Environment), 2007). It is well known that certain trace metals, when present in high enough concentrations, can be toxic to aquatic life. For example, copper (Cu) is highly toxic for fish and other aquatic species in the water column (Porter et al., 2023). Mercury is very toxic and bioaccumulative, and can biomagnify in aquatic food web (Lavoie et al., 2013; UNEP, 2013). In addition, six metals including nickel (Ni), cadmium (Cd), lead (Pb), mercury (Hg), Cu, and zinc (Zn) are listed as priority pollutants by US Environmental Protection Agency (USEPA, 2012). It is well known that plastic materials contain a wide range of chemical compounds and mix of substances (>16,000 chemicals, Wagner et al., 2024), including chemical additives such as organic compounds (Per- and polyfluoroalkyl substances [PFASs], and Polybrominated diphenyl ether (PBDEs) flame retardants), catalysts, solvents, antimicrobial agents, and pigments (Salih et al., 2013; United Nations Environment Programme, Secretariat of the Basel, Rotterdam, and Stockholm Conventions, 2023; Wagner et al., 2024; Wiesinger et al., 2021). These chemicals have been shown to leach from the polymeric materials and at least 4200 of them are of concern as hazardous substances for human and environmental health due their persistence, bioaccumulation capacity, mobile and/or toxic nature (Wagner et al., 2024) that can subsequently accumulate within food webs (Alava et al., 2023; Brander et al., 2024). In addition to the potential physical impacts of ingesting plastic particles, toxicological risks to marine organisms could also arise from the leaching constituent contaminants and plastic additives or organic and inorganic pollutants absorbed to the macro- and microplastics (Holmes et al., 2014; Liu et al., 2021; Rochman et al., 2014).
The use of plastic materials is a reality of our economic culture and, therefore, a better understanding of their behavior and interaction with other pollutants in the surrounding environment is necessary. Recently, the combined pollution of macro- and microplastic particles and trace metals has been brought into scientific and public attention (Cao et al., 2021; Khalid et al., 2021; Vrinda et al., 2023; Weis and Alava, 2023). At present, macro- and microplastic pollution and their interaction with trace metal have gained global concern (Brennecke et al., 2016; Guo and Wang, 2021), but their interaction mechanisms and combined effects are still not well understood. Only a few studies have addressed the ability of microplastics to interact with contaminants such as trace metals, and sorb them from aquatic and sedimentary environments. These studies have indicated that trace metals such as Cd, Cu, Pb, and Zn can sorb onto and accumulate on plastic particles to a high degree due to their hydrophobic potential, small size, and large surface area (Ashton et al., 2010; Barboza et al., 2018; Bouwmeester et al., 2015; Holmes et al., 2014; Kazmiruk et al., 2024; Maršić-Lučić et al., 2018 2018; Rochman et al., 2014). Notably, some contaminants have a greater affinity for plastic matrix (up to one million times higher) than surrounding seawater and sediments (Bouwmeester et al., 2015; Kazmiruk et al., 2024).
Several physicochemical factors that are associated with properties of plastic macro- and microparticles (e. g., type, shape, size, polymeric materials) and characteristics of the marine environmental compartments (e. g., pH, temperature, salinity, hydrodynamic conditions, presence of organic matter, sediment quality parameters) have been shown to affect the sorption/desorption of different contaminants, including trace metals (Cao et al., 2021; Guo and Wang, 2019; Guo and Wang, 2021; Holms et al., 2012; Holms, 2013; Holms et al., 2014; Li et al., 2020; Performance Evaluation, 2007; Rochman et al., 2013, 2014; Wang et al., 2018). However, our understanding on how these factors influence the process of sorption/desorption of different contaminants by plastic macro- and microparticles within marine environmental compartments is still largely limited. Despite plastic pollution being a significant marine environmental concern, the impact of the temperature and temperature cycling (i.e., daily and seasonal temperature changes) on the sorption/desorption of trace metals by polymeric macro- and microparticles remains unknown. Temperature is a crucial parameter in the sorption/desorption process of trace metals to macro- and microplastics in many very important applications (Bakir et al., 2014; Engler, 2012; Holms et al., 2014; Rochman et al., 2014; Turner and Holmes, 2015; Wang et al., 2018). In general, according to the adsorption theory, adsorption decreases with increase in temperature and molecules adsorbed earlier on a surface tend to desorb from the surface at elevated temperatures (Aksu and Kutsal, 1991). It seems to be that the sorption/desorption of trace metals by polymeric macro- and microparticles under condition of different temperatures is an exothermic reaction and is dominated by reversible (physical) mechanisms as per Le Chatelier’s principle (i.e., LCP states that “changes in the temperature, pressure, volume, or concentration of a system will result in predictable and opposing changes in the system in order to achieve a new equilibrium state”) (Wang et al., 2018).
To date the study of the sorption of trace metals by macro- and microplastics have been conducted as field experiments in the aquatic environments, with a little attention given to the temperature as a factor influencing the sorption behavior of the polymeric particles (Holms et al., 2012, 2014; Oz et al., 2019; Rochman et al., 2013, 2014; Wang et al., 2018). Usually the studies on the process of sorption/desorption macro- and microplastics particles to trace metals are conducted as laboratory experiments under temperature conditions of 20°C -25°C (Holms, 2013; Li et al., 2019). Only a few studies have addressed how temperature may affect the sorption characteristics of trace metals by plastic particles and the results of these studies are contradictory. For example, in the study conducted by Li et al. (2019), the process of sorption on polystyrene (PS) microparticles was investigated as a function of temperature, pH, and ionic strength of trace metals (Cu(II) and Zn(II)) concentrations through batch (aqua) incubation experiments. Results show that sorption on PS microparticles was pH-dependent, while temperature and ionic strength are having no significant influence on trace metals sorption. In contrast, temperature-dependent behavior of plastic nanoparticles has been reported for a typical range of groundwater temperatures (4.0°C - 20.0°C) (Alimi et al., 2020).
Liu et al. (2022) investigated the adsorption mechanisms of three different types of microplastics such as PP, PS, and PVC with similar particle sizes, in relation to trace metals of Pb, Cu, Cr, and Cd under different temperature and salinity conditions of the aquatic environments. The results of this study demonstrated that an increase in temperature and decrease in salinity were favorable to improve the affinity of plastic microparticles toward trace metals through the process of adsorption. Some published studies on dependence of trace metals sorption on temperature in the terrestrial environments (soil) have reported an increase in loading capacities of trace elements onto plastic surfaces with increasing temperature (Gomez and Bosecker, 1999). In addition to this, temperature significantly influences all of the physical characteristics of polymeric materials and through this has impact on their sorption/desorption behavior which can be defined as temperature-dependent (He et al., 2023; Alimi et al., 2020; Reis et al, 2013). For example, temperatures can greatly influence the abiotic and biotic aging process of macro- and microplastic particles, thereby controlling the dynamics of various physical processes and chemical reactions (He et al., 2023). It is necessary to note that low temperatures (0°C) make plastic particles more brittle and easier to break (surface breakage) and higher temperatures form large pits and cavities causing damage to the surface and reducing the resistance of the microplastic to the microbial degradation (He et al., 2023; Reis et al, 2013). Thus, environmental transformation of plastic particles (e.g., aging, changes of physical characteristics) under influence of temperature regime has an impact on their sorption/desorption towards trace metals.
To date the partitioning of trace metals (Cd, Cu, Pb, and Zn) between plastic materials in the form of macro- and microparticles and parts of marine intertidal sedimentary environments (e.g., see water, sediments, pore water) and the sorption capacities of macro- and microplastic for different trace elements have not been investigated in the marine intertidal sedimentary environmental conditions. Further, at this time, there are no long-term laboratory studies available on the sorption of trace metals such as Cd, Cu, Pb, and Zn by macro- and microplastic under condition of the intertidal sedimentary environments. Given this lack of research on the role of temperature on influencing the sorption characteristics of metals onto micro- and macroplastic, the objectives of this study were two-fold: 1) determine the role of environmentally realistic temperature conditions on the sorption of trace metals onto plastic macro- and microparticles (PETE and HDPE) under controlled laboratory conditions; and, 2) through such experiments, extrapolate our findings to intertidal sedimentary environments under field conditions. Such extrapolations will provide some insight as to how temperature may affect trace metals -plastic particles interactions under conditions of marine intertidal sedimentary environments. To our knowledge, this is the first study to assess the interactions between trace metals and plastic macro- and microparticles in the intertidal sediments subject to conditions of different constant temperature regime. In addition, these results will help our understanding the impact of environmental factors on sorption/desorption of contaminants to different plastic materials serving as a useful baseline for further studies, especially of their reversible (physical) mechanisms. Our results can be applied for the risk assessment of trace metals and polymeric macro- and microparticles in the sedimentary environments. In addition, because of the bioavailability and toxicity associated with trace metals and plastic particles, their interaction may also be temperature-dependent and have implications for marine environmental fate, transport and ultimately, the ecological risks posed by these contaminants in marine ecosystems.
2 Materials and methods
2.1 Sedimentary environments selected for the study
Surface layers of sediments (∼20.0 cm in depth from the surface) from the Burrard Inlet (British Columbia, Canada) intertidal area were sampled for the long-term laboratory study. Sediments were sampled at the same two sites where the long-term (up to 38 months) field experiments were performed previously (Kazmiruk et al., 2024). Two contrasting sediments with high and low organic matter (OM) content were used for the controlled laboratory-based experiments: A) Horseshoe Bay (HB) (gravel, sand, OM = 5-15%) and B) Maplewood Flats Conservation Area (MWFCA) (sand, mud, OM = 15-50%). Sediments from HB were more coarse, 0.25 to 2.00 mm (average 44.2% of volume) and >2.00 mm (average 35.0% of volume) as compared to sediments collected from MWFCA study site which were finer, 0.063 to 0.25 mm (average 41.4% of volume) and < 0.063 mm (average 36.5% of volume). The average total OM concentration of intertidal sediments taken at HB study site was 2.8% and 15.8% at MWFCA. The detailed description of grain size distribution, OM concentrations, and characteristics of the intertidal sediments is given in the Supplementary Materials (Supplementary Table S1).
2.2 Trace metals selected for the study
In the intertidal area of Burrard Inlet trace metals such as Cd, Cu, Pb, and Zn are of concern because of the high levels and the wide variety of sources from which they are discharged into the inlet (BIAP, 2016). Importantly, the concentrations of Cd, Cu, Pb, and Zn exceeded long-term environmental quality objectives for water and sediments at most of sites in the Burrard Inlet and could have negative effects on aquatic organisms (Bull and Freyman, 2013; Johannessen et al, 2005). Based on the analysis of the level of contamination in the marine water column and sediments at the Burrard Inlet (British Columbia, Canada) intertidal area, trace metals of primary concern such as cadmium (Cd), copper (Cu), lead (Pb), and zinc (Zn) were selected for the controlled long-term laboratory-based study (BIAP, 2011, 2016).
2.3 Selection and preparation of macro- and microplastic particles
Two types of macroplastic polyethylene chips of HDPE (macro-HDPE) (Supplementary Figure S1A) and textile fabric of PETE (macro-PETE) (Supplementary Figure S1B) and 2 types of microplastic (primary) microbeads (micro-HDPE) (Supplementary Figure S1C) and fibers (micro-PETE) (Supplementary Figure S1D) were selected for the laboratory study. The types of polymeric materials and shapes of polymeric particles are the same that were used in the long-term (38 months) field experiments reported by Kazmiruk et al. (2024) with the aim to analyze comparable data obtained from the field and laboratory experiments (Kazmiruk, 2023). An overview (i.e., microscopic images, photos and FTIR spectrums) of the polymeric materials and macro- and microparticles selected for the laboratory-based study is provided in the Supplementary Material (Supplementary Figure S1).
2.4 Laboratory experiments
To increase environmental relevance, the temperature conditions were chosen on the basis of the analysis of temperature amplitude and annual temperature range relevant to the intertidal area of Burrard Inlet (British Columbia, Canada) were sediments were sampled for the long-term laboratory study. To simulate different scenarios for the sorption of trace metals (Cd, Cu, Pb, and Zn) by a particular type of plastic macro- and microparticles under conditions of different temperature, long-term (up to 28 months) controlled laboratory-based experiments with constant temperature of T=- 4.0°C, T=+ 4.0°C, and T=+18.0°C were performed.
All laboratory-based experiments were performed in 6 (3 constant temperatures x 2 types of sediment) containers (plastic boxes 45 cm x 25 cm x 25 cm) with glass-covered sides (Supplementary Figures S2A, B). Containers were filled with sea water and sediments (18-20 cm layer) taken from the surface layer (20 cm) of the intertidal sediments at Horseshoe Bay (HB) and Maplewood Flats Conservation Area (MWFCA) of Burrard Inlet (British Columbia, Canada). At each container, triplicate mesh bags (0.063 µm) (Supplementary Figure S2C), which contained macro- HDPE (Supplementary Figure S2D), macro- PETE (Supplementary Figure S2C), micro- PETE (Supplementary Figure S2E), and micro-HDPE (Supplementary Figure S2F) were buried into the upper 10 cm layer of the intertidal sediment at each experimental unit (Supplementary Figures S2A, B). All sorption experiments were undertaken in three replicates, and the average values are reported here.
2.5 Sampling methodology
Sample collection. The experiment was designed to observe sorption of trace metals by plastic macro- and microparticles under condition of different constant temperatures. Samples of the water, the sediments and the macro- and microplastic were collected at the beginning of the experiment, just after deployment of plastic materials into sediments, and then at the time of residence t = 1, 2, 3, 8, 12, and 21 months respectively. Samples of water, sediments, and plastic macro- and microparticles were collected in triplicate and at the same time in order to receive comparable data.
Water samples. Water samples were collected in triplicated from each experimental unit. Water was sampled from the surface layer using syringe cores (diameter: 2.6 cm). Water samples were filtered immediately after sampling through 0.45 µm Whatman cellulose nitrate filters using a vacuum filter unit and filtrates were frozen (T= -5.0°C) and stored in the dark until further analysis.
Sediment samples. During the laboratory experiments sediment samples were collected in triplicated from each experimental container. Sediments were sampled from the surface layer using syringe cores (diameter: 2.6 cm). Collected sediment samples were placed into plastic zip lock bags and then were frozen (T= -15.0°C) and stored in the dark until further analysis.
Samples of plastic macro- and microparticles. At each experimental container triplicate mesh bags (textile fabric, PETE) with samples of plastic macroparticles (polyethylene chips, HDPE) and microparticles (microbeads, HDPE; fibers PETE) were collected. Samples were taken according to the general methodology of laboratory sampling with some our modifications to the existing protocols (Kazmiruk et al., 2023).
2.6 Laboratory analysis
2.6.1 Sediment quality parameter
Sediment samples were analyzed for general sediment quality parameters including grain size distribution (GS) and OM content (Batley and Simpson, 2016; Kazmiruk et al., 2018, 2024; and Mudroch et al., 1997).
Analysis for grain size distribution. Wet and dry sieving techniques were used to determine GS distribution of sediment samples (Batley and Simpson, 2016; Mudroch et al., 1997; Wentworth, 1922). Sediment particles were separated into four different size factions: gravel (GS >2 mm), coarse sand (2 mm > GS > 0.25 mm), fine sand (0.25 mm > GS > 0.063 mm), and mud or fines (silt, clay) (GS < 0.063 mm). Wet sieving was achieved by using de-ionized water (dH2O) to wash the wet sediment subsamples three times through 2000 µm (2 mm), 250 µm (0.25 mm) and 63 µm (0.063 mm) sieves.
Analysis for organic matter content. The concentration of OM in the sediment samples was determined by “loss-on-ignition” (LOI) method (ASTM, 2000a, 2000, 2000; Kazmiruk et al., 2024; Mudroch et al., 1997; Parker, 1983; Schumacher, 2002). The dried sediment subsamples weighing approximately 1.0 g – 3.0 g were burned at temperature of 400°C – 440°C (to avoid the destruction of any inorganic carbonates in the sediments) for 5-10 hours. The OM content was determined as the difference between the initial and final (ashed) subsample weights.
2.6.2 Analysis of trace metals (Cd, Cu, Pb, and Zn)
Trace metal analysis was carried out by Atomic Absorption Spectroscopy (AAS, PinAAcle 500, Perkin Elmer) for Cd, Cu, Pb, and Zn. Water samples were aspirated directly after methods of ASTM (2000a) and PinAAcle 500 FlameAAS (Perkin Elmer). The determination of the trace metals (Cd, Cu, Pb, and Zn) in the sediment samples and experimental samples of macro- and microplastics were performed on the base of digested subsamples. Sediment subsamples (2.0 g) were digested by Aqua Regia as hydrochloric acid (HCl) and nitric acid (HNO3) mixture (3:1 volume to volume) and solutions analyzed for total trace metal concentrations (Simpson et al., 2005; USEPA 3050B, 1996).
Experimental samples of micro-PETE (fiber), micro-HDPE (microbeads), macro-PETE (textile fabric), and macro-HDPE (polyethylene chips) were dried at room temperature (fume hood). Dried subsamples of macro- and microplastic (0.5 g – 0.7 g) were placed into a 50 mL Falcon tube for acid digestion on the base of Aqua Regia, prepared daily as 375 mL of HCl and 125 mL of HNO3 (Ashton et al., 2010). To each Falcon tube, 10 mL of Aqua Regia was added and subsamples were left overnight (20-28 hours) on a slow shaker to allow for the extraction of the trace metals from macro- and microplastics. A 1-5 mL aliquot was then removed, diluted with ultra-pure water and analyzed for Cu, Cd, Pb, and Zn concentration. These experimental conditions were used throughout all of the analyses.
The main controlling factors of quality samples analysis by AAS were statistical characteristics of measurements: standard deviation (SD), relative standard deviation (RSD <5.0%), and the standard error of replicate runs. Low variability RSD (not greater than 5.0%) in repeated measures indicates a high precision of analysis. Accuracy as indicated by standards was always within 5% and precision as indicated by the residual standard deviations (RSD) of repeated measures was 3%. Before instrumental analysis of subsample extracts, calibration standard was analyzed regularly to check the stability of the instrument response that is the quality control of measurements. Blanks and standards were run with the samples to ensure quality assurance and control.
2.6.3 FTIR analysis
Subsamples of the experimental macro- and microplastic particles from the laboratory experiments were analyzed using a Fourier-Transform Infrared Spectrometer (FTIR) with attenuated total reflectance (ATR) accessory (Perkin Elmer Inc. Spectrum FTIR). The infrared spectra of samples were recorded on a PerkinElmer FTIR spectrometer in the wavenumber range from 4000 cm–1 to 400 cm–1. The FTIR spectroscopy analysis was applied to (1) identification of polymer materials, (2) characterization and identification of microplastic particles, (3) estimation of weathering and aging macro- and microplastics particles. The Perkin-Elmer ATR of Polymers Library and Atlas of Plastics Additives Analysis by Spectrometric Methods (Hummel, 2002) were used for identification of microplastics’ chemical composition and polymeric materials of macro- and microplastics particles.
2.7 Quality assurance/quality control procedures
During the long-term (21 months) laboratory experiments at each time of the sampling a paired background contamination control was used. At each sampling time, prepared clean Petri Dish and three empty glass beakers were placed beside the experimental unit and opened during sample collection (water, sediments, and macro- and microplastic particles) and closed simultaneously with the sample zip lock bags.
For analysis/measurements of trace metals (Cd, Cu, Pb, and Zn) concentration the protocol for QA/QC was maintained by the analysis of reference sediment MESS-3 (National Research Council of Canada) and reagent blanks, as well as sediment sample replicates used as controls. Marine sediment reference materials (MESS-3) were used for measurements of Cd, Cu, Pb, and Zn (Cd = 0.24 ± 0.01 mg/kg; Cu = 33.9 ± 1.6 mg/kg; Pb = 21.1 ± 0.7 mg/kg; Zn = 159 ± 8 mg/kg). The recovery rates for the trace metals in the standard reference material were between 78.9% and 101.0%: Cd (79.8%), Cu (95.2%), Pb (78.9%) and Zn (101.0%). Procedures for QA/QC were maintained by the analysis of reference sediment MESS-3 (National Research Council of Canada) and reagent blanks, as well as sample replicates were used as controls.
2.8 Statistical data analysis and data evaluation
2.8.1 Data analysis
After laboratory sampling and measurements, 120 variables ((4 plastic particle types + sediments) x 4 trace metals x 3 temperatures x 2 sediment types = 120) were created for analyses. For the temporal and absolute comparison of trace metals contents in water, sediments and plastic macro- and microparticles, the concentration of trace metals was calculated as unit µm per g water, sediment, and plastic particles dry weight (µm/g). Basic statistical analysis (means, t-test, ANOVA, correlation, regression) of the data were performed using Statistical Package software “IBM SPSS Statistics” (IBM SPSS Statistics 19.0, IBM, Amonk, NY), R (R Core Team, 2021), and RStudio (RStudio Inc.; MA, USA).
Sediment data (grain size, OM, Cd, Cu, Pb, and Zn) was first tested for normal distribution (test of normality, Shapiro–Wilk test) and equal variances (homoscedasticity test) prior to the application of a two-way ANOVA with sediments/temperature and time as the two factors (Garson, 2012; Shapiro and Wilk, 1965). Macro- and microplastic data (Cd, Cu, Pb, and Zn) was first tested for normal distribution (test of normality, Shapiro–Wilk test) and equal variances (homoscedasticity test) prior to the application of a two-way ANOVA for each of 2 plastic microparticles (HDPE and PETE) and 2 plastic macroparticles (HDPE and PETE) with sediments/temperature and time as the two factors. A further 2-way ANOVA was also applied to determine if within each sediments, sorption of trace metals by plastic macro- and microparticles were dependent on plastic type, temperature, and time. Data were generally normally distributed with equal variances (Shapiro Wilk test), so data was not transformed for statistical analysis. Statistical analysis was performed using Sigma Plot 12 © and significance of all tests was set at p-value <0.05.
Data visualization, tests for normal distribution (Shapiro–Wilk normality test), linear regression analyses, Spearman correlation analyses, and variance analyses (ANOVA) were conducted with the standard R-packages as well. Histograms and boxplots (represent the distribution of the data) were used to estimate the normality of the data distribution. Differences in sorption characteristics (trace metals concentrations, partitioning coefficients) among type of sediments and temperatures versus plastic macro and microparticles and time were tested with linear models (lm) (p < 0.05) using R (R Core Team, 2021). We used linear regression models (lm) with sediments, temperature, and polymeric type as fixed factors to test for temporal variations in concentration of trace metals (Cd, Cu, Pb, and Zn) in sediment samples and experimental samples of plastic macro- and microparticles (HDPE and PETE). Significances were tested on the level of confidence interval 95%. We interpreted the results of statistical analysis to be signficant when p-value < 0.05 (Supplementary Table S2).
2.8.2 Data evaluation
To improve our ability to detect changes over time, data from time 0, 1, and 2 months, 3 and 8 months, and 12 and 21 months were pooled to represent three time periods: “short-term”(0-2 months), “mid-term” (3-8 months) and “long-term” (12-21months). This approach of data analysis was applied in previous field experiments (Kazmiruk et al., 2024) in order to compare data from field and laboratory studies. A linear regression model (lm) (R Core Team, 2021) was applied to test for variations in trace elements concentrations in sediment samples and experimental samples of plastic macro- and microparticles (PETE and HDPE) for three time periods of sampling and measurements (i.e., “short -term”(0-2 months), “mid-term” (3-8 months), and “long-term” (12-21 months)) in each of two types of sediments containing different organic matter (OM) fractions (i.e., OM=2.8% and OM=15.8%) and under conditions of three temperatures (T=- 4.0°C, T=+ 4.0°C, and T=+18.0°C).
To provide an effective analysis of interaction between trace metals (Cd, Cu, Pb, and Zn) and plastic macro- and microparticles in intertidal sediments under temperature conditions of T=- 4.0°C, T=+ 4.0°C, and T=+18.0°C the relative concentration (Cf(t+Δt)/Ci) were applied and calculated as follows:
where Ci = Cf(T=0) (μg/g) is the initial concentration of trace metal at the beginning of the experiments at residence time t = 0; and, Cf(t+Δt) (μg/g) is concentration of trace metal at residence time t = 0, 1, 2,…21 months. By using the relative concentration we can compare the concentration of trace metals at some residence time (i.e., time after deploying of plastic particles in sediments), initial concentration (i.e., concentration at the beginning of the experiments, before deploying of plastic particles in sediments), and equilibrium concentration . By estimating the value of the relative equilibrium concentration as the ratio of equilibrium concentration to the initial concentration (Ci), the trace metals-plastic particles interaction can be defined as follows:
The relative concentration is widely applied in sorption study such as sorption behavior of contaminants on microplastics (Chen et al., 2022).
To assess the potential distribution and re-distribution of trace metals concentration between the intertidal sediments with macro- and microplastics content under temperature conditions of T=-4.0°C, T=+ 4.0°C, and T=+18.0°C the partition (distribution) coefficient (Kd) were used. According to the definition, a partition coefficient is the ratio of the concentration of a substance in one medium or phase (C1) to the concentration in a second phase (C2) when the two concentrations are at equilibrium. In this study the equation for the partition (distribution) coefficient derived from the linear model (Kd) is as follows (Bakir et al., 2014; Wang et al., 2018):
where qe (μg/g) is the amount of contaminant sorbed onto solid phase at equilibrium; and Ce (μg/mL) is the contaminant concentration in the aqueous phase at equilibrium. According to the Equation 4, the sediments-sea water partitioning coefficient (Ksed-sw) is defined as:
where [Me]sed (μg/g) is the concentration of trace metals adsorbed to solid phase (sediments); [Me]sw (μg/mL) is the aqueous (sea water) trace metals concentration. Likewise, the sediments-pore water partitioning coefficient (Ksed-wp) is as follows:
where [Me]sed (μg/g) is the concentration of trace metals adsorbed to solid phase (sediments); [Me]wp (μg/mL) is the aqueous (pore water) trace metals concentration. The plastic materials (macro- and microparticles)-pore water partitioning coefficient (Kpl-wp) is defined as follows:
where [Me]pl (μg/g) is the concentration of trace metals adsorbed to solid phase (plastic materials); [Me]wp (μg/mL) is the aqueous (pore water) trace metals concentration. By dividing sediments-pore water partitioning coefficient (Ksed-wp) (Equation 6) and plastic materials (macro- and microparticles)-pore water (Kpl-wp) partitioning coefficient (Equation 7) we will exclude the concentration of trace metals in pore water of sediments from the final equation:
The ratio of sediments-pore water partitioning coefficient (Ksed-wp) to the plastic materials-pore water partitioning coefficient (Kpl-wp) is as follows:
Linear regression model (lm) was used to test for variations in ratio between partition coefficients [log (Ksed-wp/Kpl-wp)] of trace metals (Cu, Cd, Pb, and Zn) among two type of sediments (OM=2.8% and OM=15.8%) and three temperature conditions (T=- 4.0°C, T=+ 4.0°C, and T=+18.0°C). Significances were tested on the level of confidence interval 95%. We interpreted the results of statistical analysis to be significant when reaching a p-value < 0.05.
3 Results
3.1 Polymer composition and aging of macro- and microplastics in the intertidal sediments under constant temperature conditions (T=- 4.0°C, T=+4.0°C, and T=+18.0°C)
The FTIR spectra of the initial macro- and microparticles of HDPE and PETE exhibit characteristic bands at wavenumbers typical of these plastic material types (Supplementary Figure S1) (Gulmine et al., 2002; Hummel, 2002). For example, as seen in Figure 1A at the beginning of the laboratory experiments (t=0) the FTIR spectrums of initial macro-HDPE samples exhibit characteristics bands at wave number 2914.46 cm−1 (CH2 asymmetric stretching) and 2846.49 cm−1 (CH2 symmetric stretching), 1472.21 cm−1 and 1461.72 cm−1 (bending deformation), 1367.57 cm−1 (wagging deformation), 730.33 cm−1 and 718.79 cm−1 (rocking deformation) that are seen for HDPE (Charles and Ramkumaar, 2009; Gulmine et al., 2002; Hummel, 2002; Pagès, 2008).
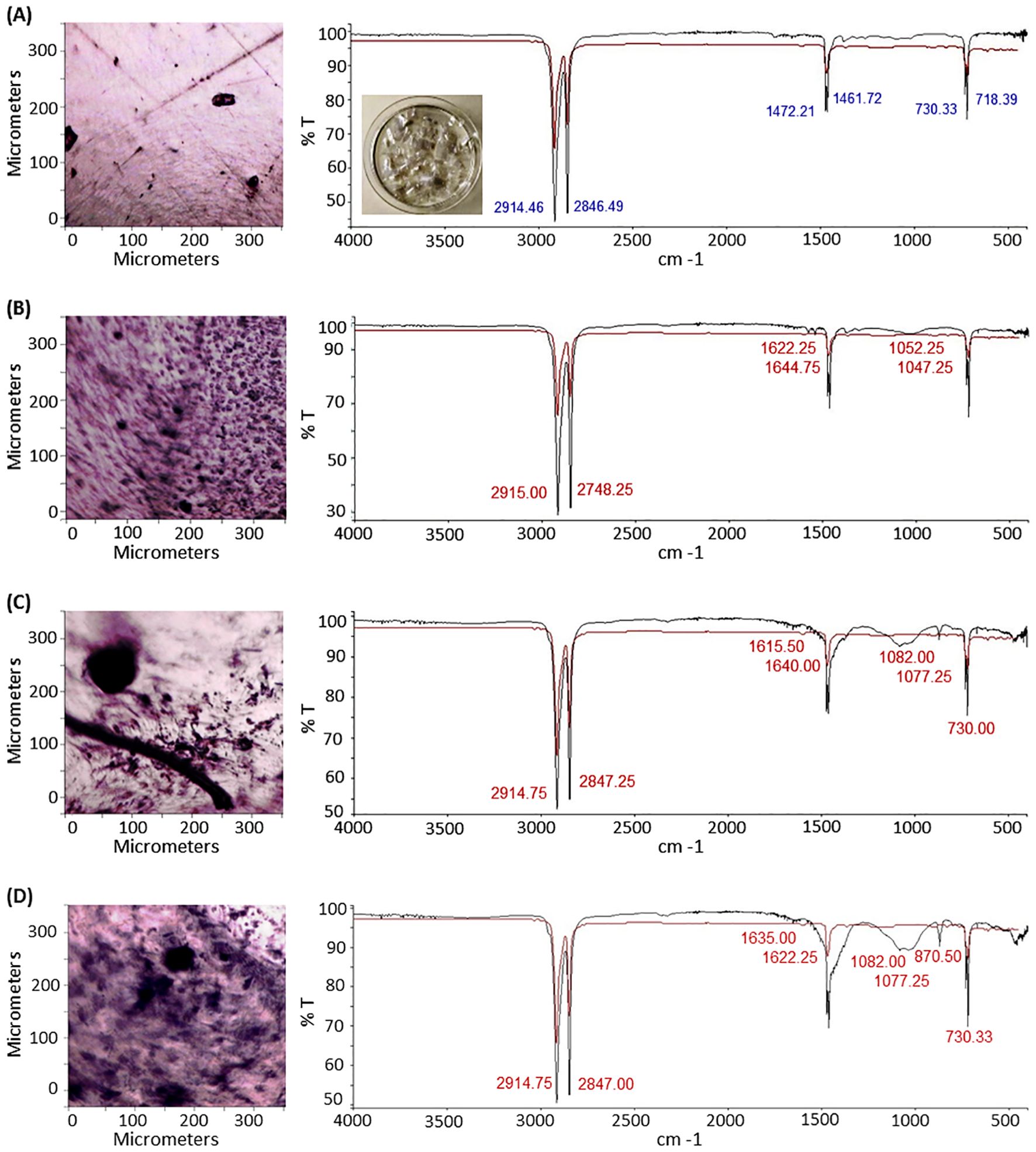
Figure 1. The microscopic images of the surface morphology and FTIR spectrum of macro-HDPE (chips) in the intertidal sediments (OM = 15.8%) under conditions of constant temperature of T=- 4.0°C (B), T= +4.0°C (C), and T= +18.0°C (D) at residence time of t=0 months (A) and t=21 months (B–D). The red spectra are standard spectra of the polymer material (ATR Polymer Introductory Library); blue spectra are spectra of analyzed sample; numbers in red color are values of the spectra (wave number, cm-1) of new functional group. The peak at 1622.25 cm−1 (intensified and shifted to 1615.50 cm−1) and the peak at 1644.75 cm−1 (intensified and shifted to 1640.00 cm−1) are found on the upper blue line of the spectra.
For macro-HDPE (polyethylene chips) after 21 months of deploying in sediments with OM = 15.8% and at constant T=-4.0°C, the FTIR spectrums have occurrence changes as follows: (1) the peak at 2914.46 cm−1 (CH2 asymmetric stretching) is intensified and shifted to 2915.00 cm−1; (2) the peak at 2846.49 cm−1 (CH2 symmetric stretching) is shifted to 2748.25 cm−1; (3) the peak at 1615.25 cm−1 is intensified and shifted to 1622.25 cm−1; (4) the peak at 1646.00 cm−1 is intensified and shifted to 1644.75 cm−1; (5) formation of new functional groups is at 1052.25 cm−1 and 1047.25 cm−1 (Figure 1B).
For macro-HDPE (polyethylene chips) after 21 months of deploying in sediments (OM = 15.8%) at constant T=+4.0°C, the FTIR spectrums have occurrence changes as following: (1) the peak at 2914.46 cm−1(CH2 asymmetric stretching) is intensified and shifted to 2914.75 cm−1; (2) the peak at 2846.49 cm−1 (CH2 symmetric stretching) is shifted to 2847.25 cm−1; (3) the peak at 1615.25 cm−1 is shifted to 1615.50cm−1; (4) the peak at 1646.00 cm−1 is shifted to 1640.00cm−1; (5) formation of new functional groups is at 1082.50 cm−1 and 1077.25 and 864.25 (Figure 1C).
For macro-HDPE (polyethylene chips) after 21 months of deploying in the intertidal sediments (OM = 15.8%) at constant T=+18.0°C, the FTIR spectrums have occurrence changes as following: (1) the peak at 2914.46 cm−1 (CH2 asymmetric stretching) is shifted to 2914.75 cm−1; (2) the peak at 2846.49 cm−1 (CH2 symmetric stretching) is shifted to 2847.00 cm−1; (3) the peak at 1615.25 cm−1 is shifted to 1622.25 cm−1; (4) the peak at 1646.00 cm−1is shifted to 1635.00cm−1; (5) formation of new functional groups is at 1082.50 cm−1 and 1077.25 cm−1 and 870.50 cm−1 revealing peaks that are intensified and shifted (Figure 1D).
In general, the FTIR spectra of HDPE and PETE samples after deployment in the intertidal sediments with low and high OM concentration and under three constant temperature regimes exhibited shifted and intensified peak spectrums along with the formation of new functional groups. The shifting of peaks 2914.46 cm−1 and 2846.49 cm−1 occurs due to degradation of HDPE in the presence of microbial culture (Ojha et al., 2017). The peak at wave number 1628.75 cm−1 corresponds to the carbonyl group (Rajandas et al., 2012) which is due to oxidation of HDPE sample (Coates, 2006; Gulmine et al., 2002). It is important to note that the plastic particles were buried so oxidation by UV light would not occur. Formation and shifting of another peak at 1646.00 cm−1 (Figures 1B–D) occurs and can be interpreting as the formation of the similar functional group as at 1628.75 cm−1. Formation of new functional groups at 1082.50 cm−1 and 1077.25 cm−1 (as seen in Figure 1D) occurs due to C-C stretching (Charles and Ramkumaar, 2009).
Formation of two new functional groups at 1052.25 cm−1 and 1047.25 cm−1 are seen for macroplastic (HDPE, polyethylene chips) deployed at T=-4.0°C (Figure 1B). Formation of three new functional groups is seen at 1082.50 cm−1 and 1077.25 and 864.25 (T=+4.0°C) and at 1082.50 cm−1 and 1077.25 and 870.50 cm−1 (T=+18.0°C) (Figures 1C, D). Formation of new functional groups at 1052.25 cm−1 and 1047.25 cm−1 can be interpreted as the formation of the similar functional group as at 1082.50 cm−1 and 1077.25 cm−1 which occurs due to C-C stretching (Charles and Ramkumaar, 2009; Pagès, 2008). Formation of the peaks at 864.25 cm−1 (T=+ 4.0°C) and 870.50 cm−1 (T=+18.0°C) occurred (see Figures 1C, D), which is consistent with the observed formation of the similar functional group at 864 cm−1 when pre-treated HDPE is subjected to microbial treatment (Sheik et al., 2015).
Importantly, the FTIR spectra for macro- and microplastic samples of HDPE and PETE deployed in sediments under conditions of low temperature (T=-4.0°C) showed less changes in standard characteristics bands at wave’ numbers as compared to those plastic particles deployed in sediments under condition of higher temperature (T= +18.0°C) (Figures 1B, D). Microscopic images of the surface morphology of plastic macro- and microparticles unveiled the roughness, cracks, mechanical and oxidative weathering, indicating their continuous exposure in the sedimentary environment. The FTIR spectrums of macro-HDPE (polyethylene chips) deployed in marine intertidal sediments (OM = 15.8%), under condition of constant temperature, provided the presence of various elements on the macroparticles’ surface (Figure 1). Analyses of the FTIR spectra and microscopic images confirmed the degradation and aging of macro- and microparticles of both HDPE and PETE plastic materials after their deployment in the intertidal sediments with temperatures of T=- 4.0°C, T=+ 4.0°C, and T=+18.0°C. The mechanism of temperature-induced degradation of macro- and microplastic under conditions of intertidal sedimentary environment requires further study. It would be beneficial to investigate the influence of temperature on the degradation/aging of plastic particles and partitioning of trace metals because this is related to their bioavailability.
3.2 Trace metal concentrations
3.2.1 Trace metal concentrations in sediments
Overall, the concentration of Cu, Pb, and Zn were higher in sediments with high OM as compared to that in sediments low in OM content, and only the concentration of Cd was greater in sediments with low OM (2.8%) as compared to that in sediments high in OM content (15.8%) (Table 1).
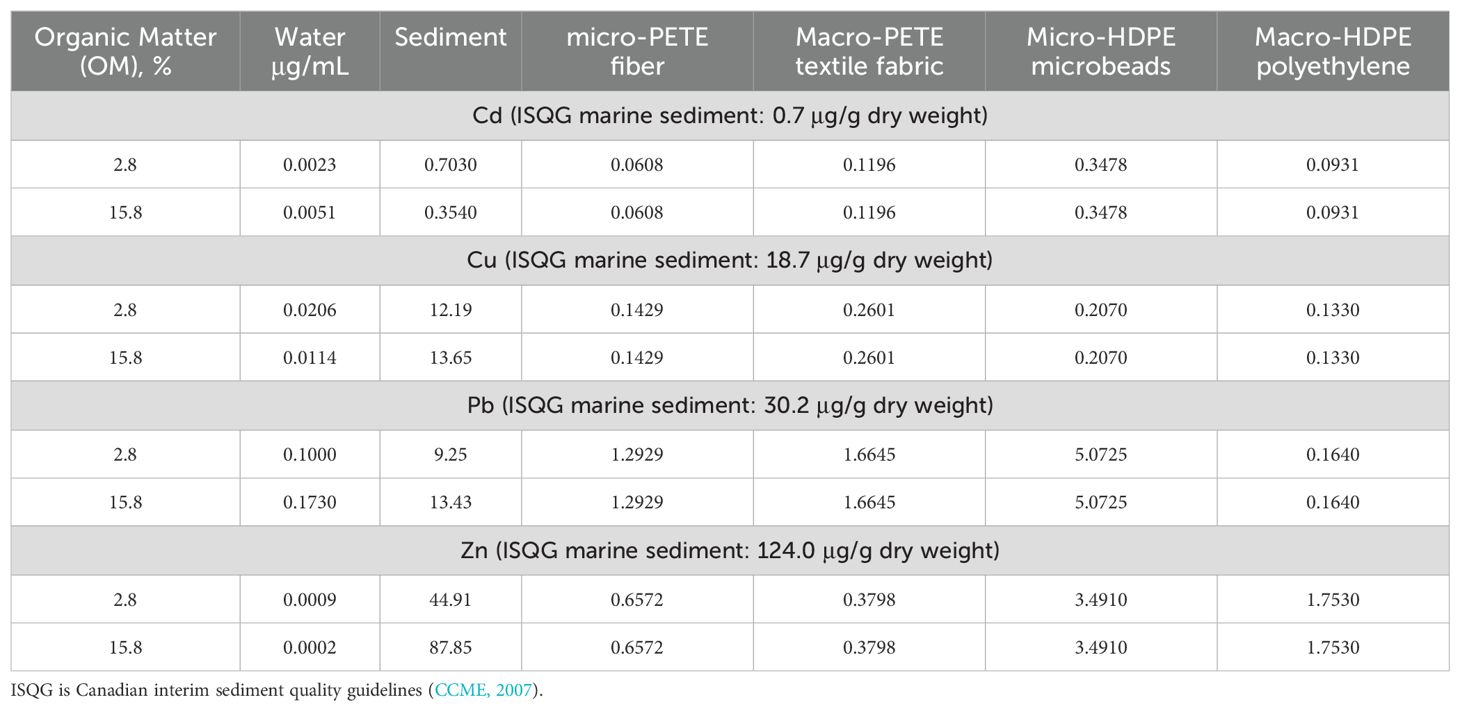
Table 1. Initial concentrations (μg/g dw) of Cd, Cu, Pb, and Zn in sea water, intertidal sediments, and experimental samples of plastic macro- and microparticles.
3.2.2 Trace metal sorption by plastic macro- and microparticles
The initial concentrations of trace metals (Cd, Cu, Pb, and Zn) in the experimental samples of plastic macro- and microparticles (prepared for deployment in sediments) were greater than trace metals concentrations measured in seawater samples, but significantly lower than that concentration measured in sediment samples collected within intertidal area of Burrard Inlet at the HB (OM=2.8%) and MWFCA (OM=15.8%) and used in the laboratory experiments (Table 1). This comparison indicates that macro- and microplastics primarily sorb trace metals from the intertidal sedimentary environment.
In the controlled laboratory-based experiments the concentrations of Cd, Cu, Pb, and Zn increased over time, but with different intensity. The total (initial and adsorbed/loaded) measured concentrations of Cd, Cu, Pb, and Zn recovered from the plastic particles varied as a function of: (a) time of residence in sedimentary environment, (b) type of plastic material (HDPE, PETE) and type of plastic particles (macro-HDPE, polyethylene chips; macro-PETE, textile fabric; micro-HDPE, microbeads; micro-PETE, fibers), (c) characteristics of intertidal sediments (grain size, OM), and (d) temperature conditions (see trends from Figures 2–5).
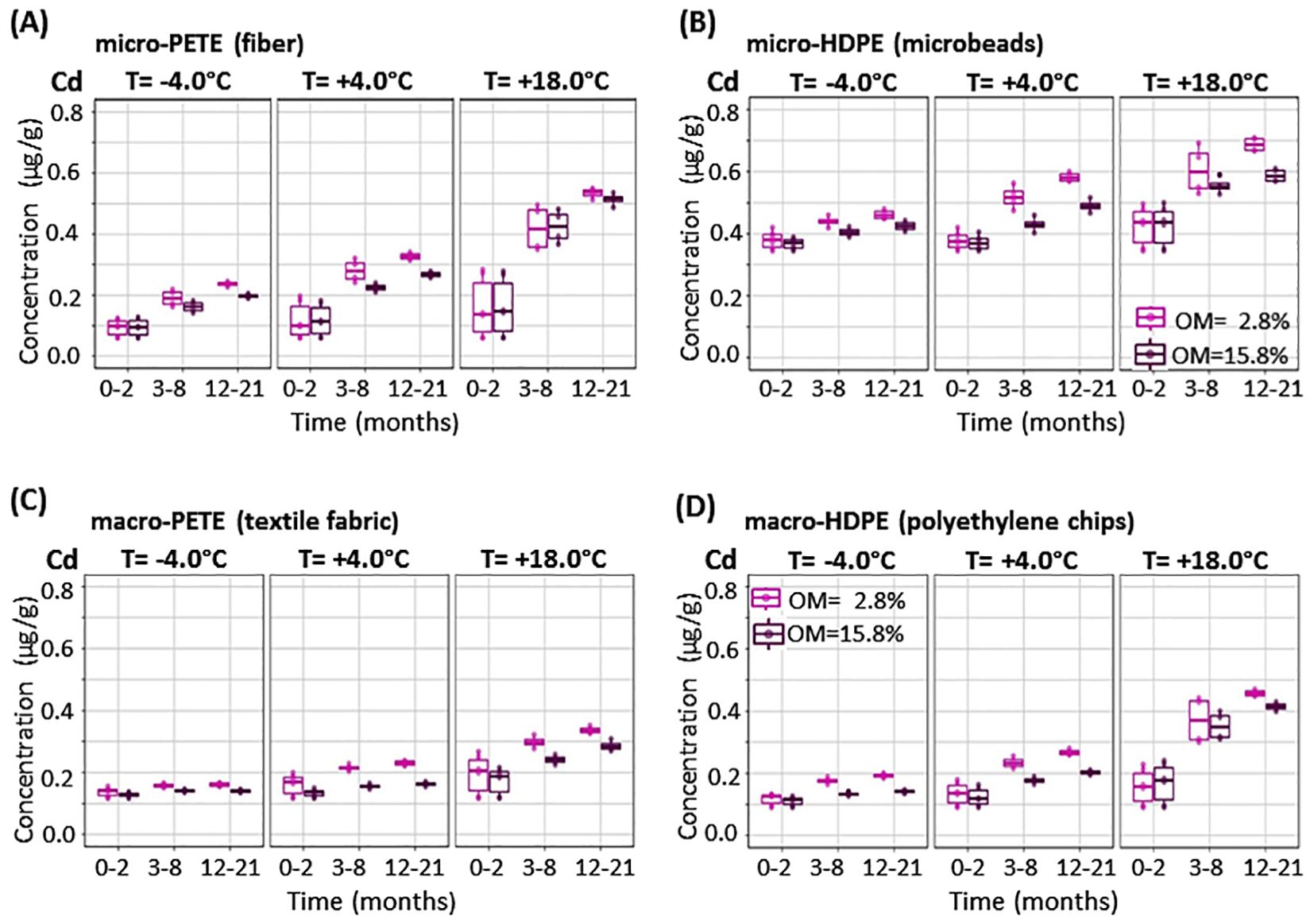
Figure 2. Concentration of Cd over time recovered from micro-PETE (fiber) (A), micro-HDPE (microbeads) (B), macro-PETE (textile fabric) (C), and macro-HDPE(polyethylene chips) (D) deployed in the intertidal sediments. Laboratory experiments were performed with sediments low (OM=2.8%) and high (OM=15.8%) in organic matter content and constant temperature of T=- 4.0°C, T=+ 4.0°C, and T=+18.0°C. Boxplots represent the distribution of observed values, where midline is the median, with the upper and lower limits of the box being 75th and 25th percentiles. Whiskers extend up to the interquartile range and represent the ranges for the bottom 25% and the top 25% of the data values, excluding outliers. Measured values are depicted as points.
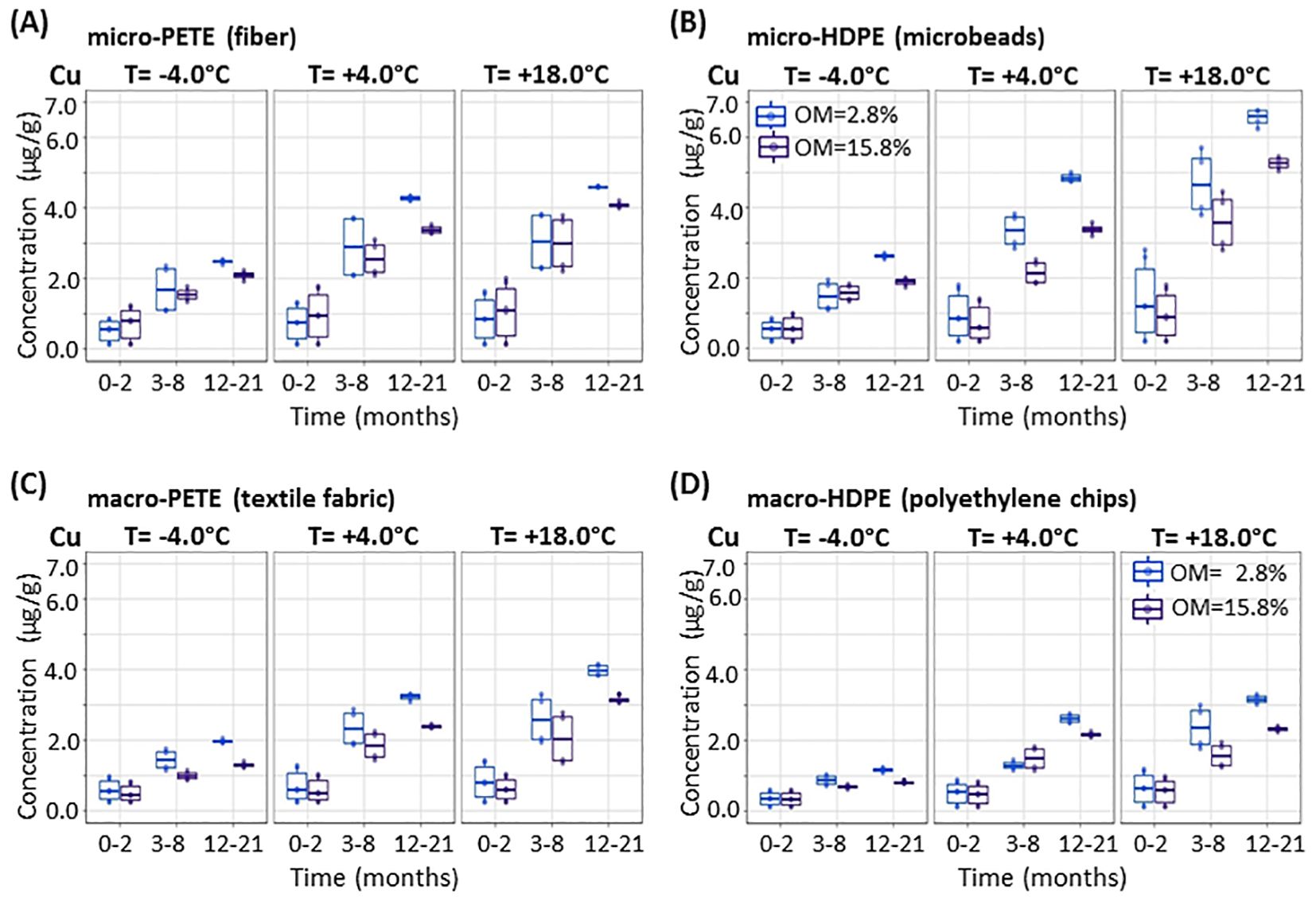
Figure 3. Concentration of Cu over time recovered from micro-PETE (fiber) (A), micro-HDPE (microbeads) (B), macro-PETE (textile fabric) (C), and macro-HDPE(polyethelene chips) (D) deployed in the intertidal sediments. Laboratory experiments were performed with sediments low (OM=2.8%) and high (OM=15.8%) in organic matter content and constant temperature of T=- 4.0°C, T=+ 4.0°C, and T=+18.0°C. Boxplots represent the distribution of observed values, where midline is the median, with the upper and lower limits of the box being 75th and 25th percentiles. Whiskers extend up to the interquartile range and represent the ranges for the bottom 25% and the top 25% of the data values, excluding outliers. Measured values are depicted as points.
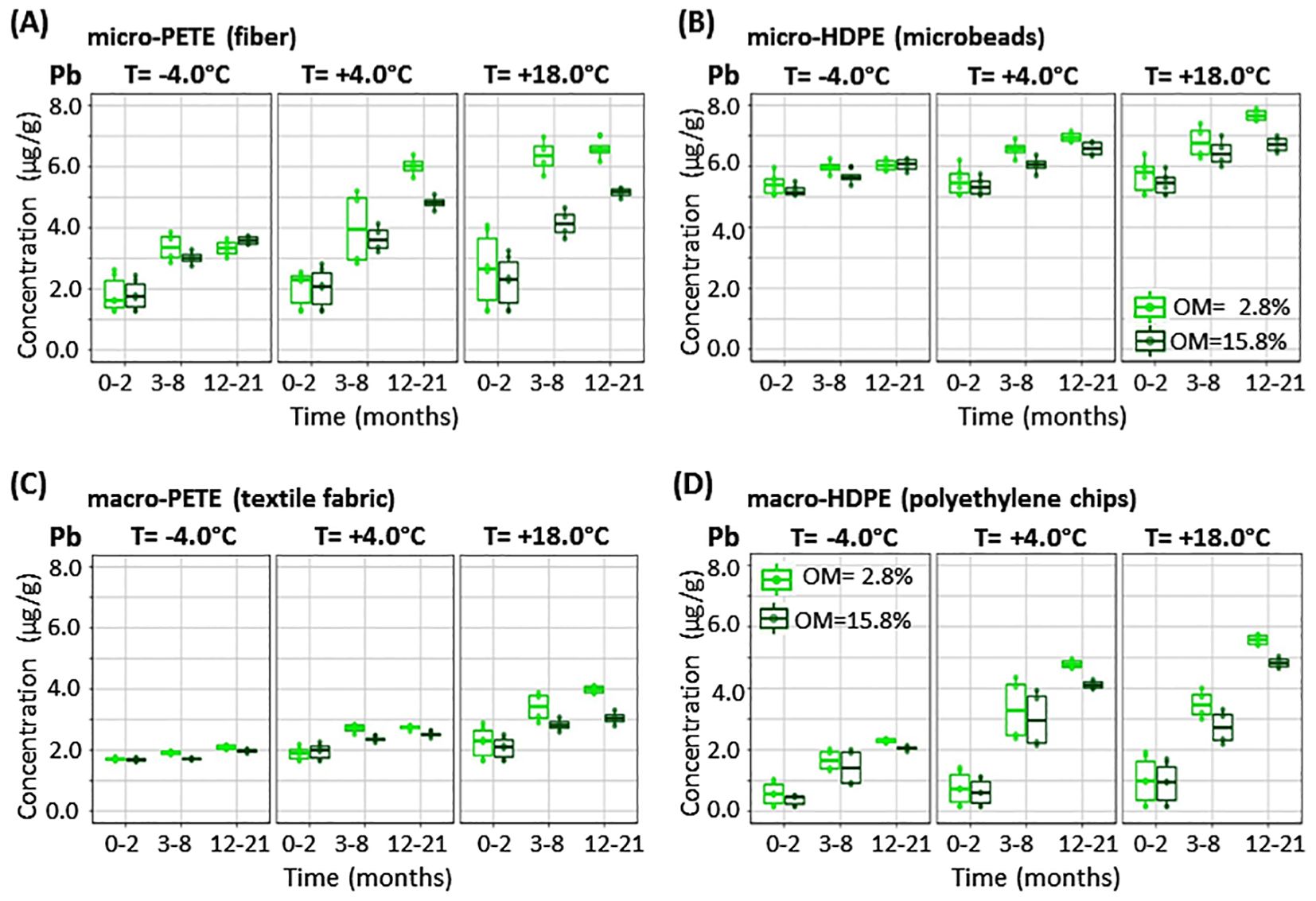
Figure 4. Concentration of Pb over time recovered from micro-PETE (fiber) (A), micro-HDPE (microbeads) (B), macro-PETE (textile fabric) (C), and macro-HDPE(polyethelene chips) (D) deployed in the intertidal sediments. Laboratory experiments were performed with sediments low (OM=2.8%) and high (OM=15.8%) in organic matter content and constant temperature of T=- 4.0°C, T=+ 4.0°C, and T=+18.0°C. Boxplots represent the distribution of observed values, where midline is the median, with the upper and lower limits of the box being 75th and 25th percentiles. Whiskers extend up to the interquartile range and represent the ranges for the bottom 25% and the top 25% of the data values, excluding outliers. Measured values are depicted as points.
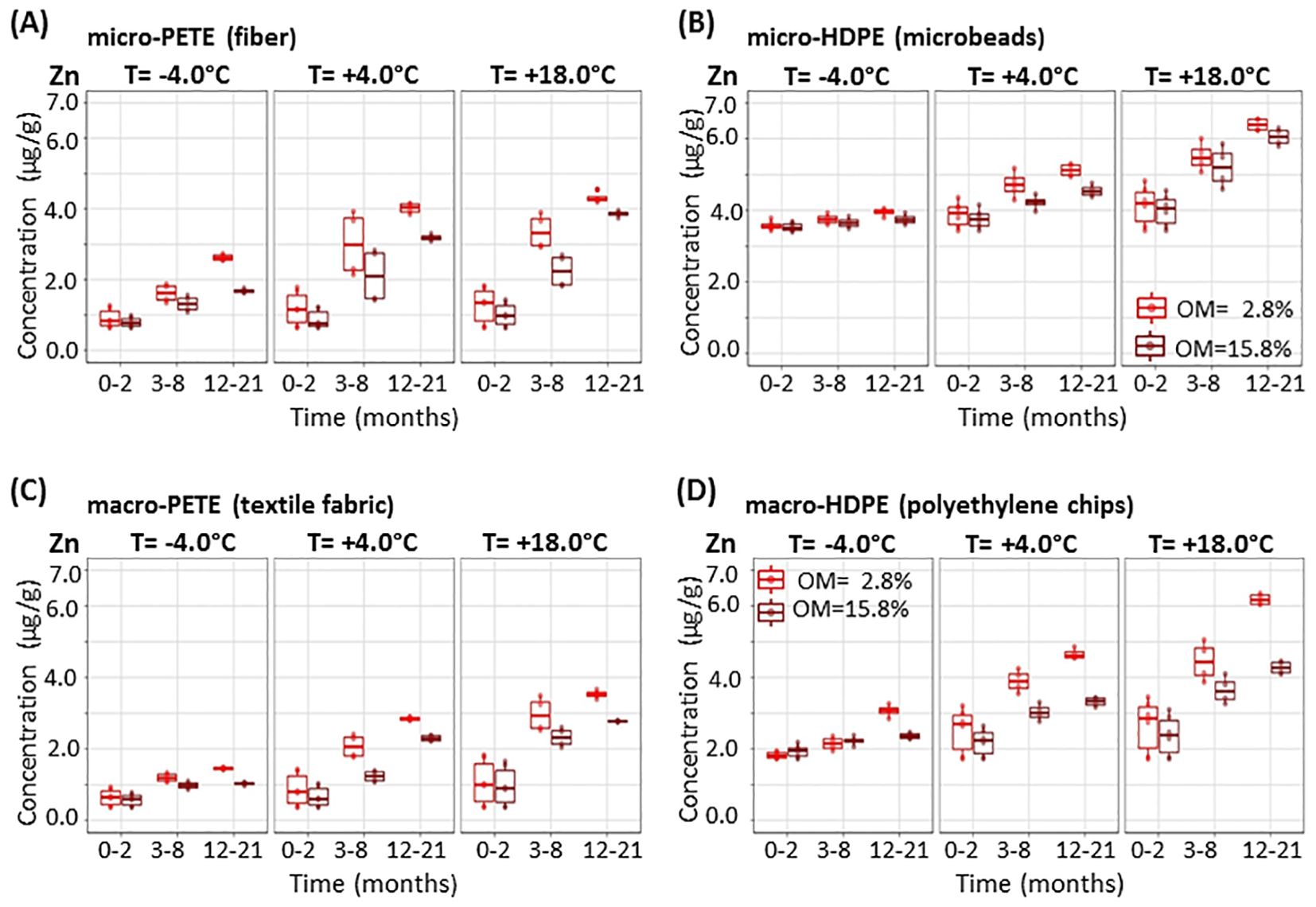
Figure 5. Concentration of Zn over time recovered from micro-PETE (fiber) (A), micro-HDPE (microbeads) (B), macro-PETE (textile fabric) (C), and macro-HDPE(polyethylene chips) (D) deployed in the intertidal sediments. Laboratory experiments were performed with sediments low (OM=2.8%) and high (OM=15.8%) in organic matter content and constant temperature of T=- 4.0°C, T=+ 4.0°C, and T=+18.0°C. Boxplots represent the distribution of observed values, where midline is the median, with the upper and lower limits of the box being 75th and 25th percentiles. Whiskers extend up to the interquartile range and represent the ranges for the bottom 25% and the top 25% of the data values, excluding outliers. Measured values are depicted as points.
4 Discussion
4.1 The interaction between trace metals and macro- and microplastics in the intertidal sediments under constant temperature conditions
Temperature is a crucial parameter in the sorption/desorption process of trace metals by macro- and microplastics in many very important processes and experimental settings (Bakir et al., 2014; Engler, 2012; Holms et al., 2014; Rochman et al., 2014; Turner and Holmes, 2015; Wang et al., 2016, 2018). In general, according to the adsorption theory, adsorption decreases with increase in temperature and molecules adsorbed earlier on a surface tend to desorb from the surface at elevated temperatures (Aksu and Kutsal, 1991). For instance, Gomez and Bosecker (1999) reported that trace metal sorption in terrestrial environments (soil) is temperature-dependent, with an increase in trace metal loading capacities as temperature rises. Notably, temperature plays a crucial role in the aging and degradation of plastic materials (Figure 1). It significantly affects the physical and chemical properties of plastics, influencing their sorption and desorption behavior, which can be classified as temperature-dependent (Alimi et al., 2020; Lohman et al., 2017; Reis et al., 2013).
Our findings suggest that the interaction between trace metals and plastics can be described as a process of time-dependent sorbance. In general, at the beginning of the sorption experiments, during the “short-term” (0-2 months) and “mid-term” (3-8 months) of sampling periods, the concentration of all four trace metals (Cd, Cu, Pb, and Zn) in macro- and microplastics rapidly increased, almost to 1/3-2/3 the value of the total adsorbed/loaded concentration at the end of the experiments (21 months). After the initial period, during the “long-term” (12-21 months) sampling period, the concentration of trace metals (Cd, Cu, Pb, and Zn) increased slowly, reaching equilibrium concentrations (observed stable concentration) after 21 months (Figures 2–5). These findings assume that interaction between trace metals and macro- and microplastics in the intertidal sediments under constant temperatures can be described as process of trace metals accumulation over time that include two time periods a period of rapid adsorption and a period of reaching equilibrium state. This is in accordance with results from the study on trace metals-plastic particles interaction in the aquatic environments (Gao et al., 2019; Holmes et al., 2012; Lindsay, 1979; Rochman et al., 2014).
4.2 The influence of constant temperature
The laboratory-based study demonstrated that macro- and microplastic of HDPE and PETE accumulate Cd, Cu, Pb, and Zn over time but with high significant differences in relation to the type of sediments, temperature conditions, and polymer type and particles shape/size (p<0.05) (Figures 2–5; Supplementary Table S2). To estimate the influence of temperature on trace metals-plastic particles interaction under conditions of three constant temperatures the relative equilibrium concentration (Ce/Ci) as ratio of equilibrium concentration (Ce) to the initial concentration (Ci) was used (Equation 1). The value of the relative equilibrium concentration was calculated for concentrations of Cd, Cu, Pb, and Zn sorbed by macro-PETE (textile fabric), macro-HDPE (polyethylene chips), micro-HDPE (microbeads), and micro-PETE (fibers) deployed in intertidal sediments low (OM=2.8%) and high (OM=15.8%) in OM and under temperature conditions of T=- 4.0°C, T=+ 4.0°C, and T=+18.0°C (Table 2).
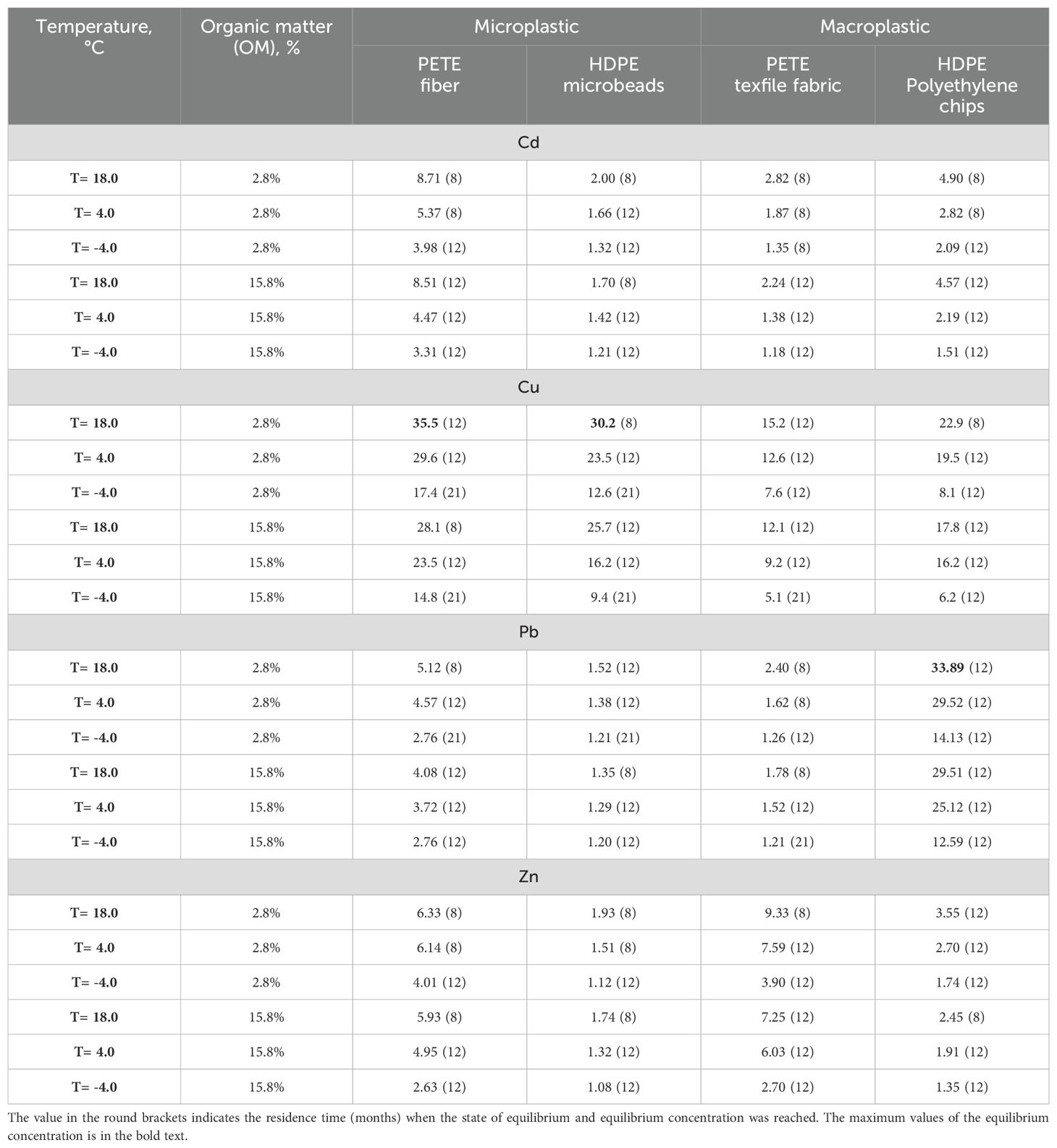
Table 2. The value of the relative equilibrium concentration (Ce/Ci) of trace metals in macro- and microplastics deployed in the intertidal sediments.
The value of the equilibrium concentration of Cu, Zn, Pb, and Cd in macro- and microparticles of HDPE and PETE were significantly dependent (p < 0.05) on temperature (Supplementary Table S2). By comparing the sorption of trace metals under conditions of three constant temperatures (i.e., T=- 4.0°C, T=+ 4.0°C, and T=+18.0°C), it was found that the concentration of Cd, Cu, Pb, and Zn in macro- and microplastics of HDPE and PETE was higher and reached equilibrium state and equilibrium concentration faster under condition of higher temperature (T=+18.0°C) at 8-12 months compared to that at lower temperature (T=-4.0°C) at 12-21 months (Figures 2–5; Table 2). Importantly, the value of the relative equilibrium concentration (Ce/Ci) of all four trace metals (Cu, Zn, Pb, and Cd) in macro- and microplastics of HDPE and PETE were greater under condition of high temperature (T=+18.0°C) compared to that at low temperature (T=-4.0°C). Copper exhibited the highest relative equilibrium concentration in microplastic of both types of polymeric materials, including PETE (fiber) and HDPE (microbeads), and Cu and Pb in macroplastic of HDPE (polyethylene chips). The highest value of relative equilibrium concentration were observed for microplastic of PETE to Cu (Ce/Ci = 35.5) and HDPE to Cu (Ce/Ci =30.2) and macroplastic of HDPE to Pb (Ce/Ci =33.89) (Table 2). It means, for example, that at temperature of T= +18.0°C the equilibrium concentration (Ce) of Cu can be in 35.5 and 30.2 fold greater than the initial concentration (Ci), in microplastics of PETE and HDPE respectively, which is in accordance with Liu et al. (2019).
The value of the relative equilibrium concentration (Ce/Ci) indicated that the sorption behavior of Cd, Cu, Pb, and Zn varied significantly depending on the type of plastic materials and their size/shape (Table 2; Figures 2–5; Supplementary Table S2). By comparing the difference in sorption between two different types of plastic materials, we found that under conditions of all three constant temperatures (T=- 4.0°C, T=+ 4.0°C, and T=+18.0°C) the sorbance of micro-PETE (fiber) to Cd, Cu, Pb, and Zn was higher than micro-HDPE (microbeads) (Figures 2A, B–5A, B). In contrast, the sorbance of macro-PETE (textile fabric) to Cd, Cu, and Pb was lower than macro-HDPE (polyethylene chips) (Figures 2C, D–4C, D), while the sorbance of macro-PETE (textile fabric) to Zn was higher than sorbance of macro-HDPE (polyethylene chips) (Figures 5C, D; Table 2). Such findings may suggest that the same temperature regime had different impact on sorption of trace metals by macro- and microplastics of the same polymeric materials (HDPE and PETE).
At the same time, the relative equilibrium concentration of all trace metals (Cd, Cu, Pb, and Zn) in macro- and microplastics of HDPE and PETE deployed under temperature conditions (i.e., T=- 4.0°C, T=+ 4.0°C, and T=+18.0°C) was higher in sediments with low OM content (2.8%) compared to that in sediments high in OM (15.8%), as shown in Table 2. These findings suggest that the sorption of Cu, Zn, Pb, and Cd by macro- and microplastics is highly competitive with sorption by sediments. It is likely that the characteristics of intertidal sediments (e.g., OM=2.8% and OM=15.8%, grain size distribution) have a greater influence on the sorption of trace metals relative to the influence of constant temperature applied in our simulation experiments (T=- 4.0°C, T=+ 4.0°C, and T=+18.0°C).
The influence of temperature on the sorption of trace metals by macro- and microplastics in the intertidal sediments can be expressed by gradient Δ(log(Ce/Ci))/ΔT) (i.e., relative equilibrium concentration as function of temperature; where Δ implies a change in the quantity). In general, in the range of the constant temperatures of T=- 4.0°C, T=+ 4.0°C, and T=+18.0°C adsorption of Cu, Zn, Pb, and Cd by macro-HDPE (polyethylene chips), macro-PETE (textile fabric), micro-HDPE (microbeads), and micro-PETE (fibers) increased with increasing of temperature, and gradients (Δ(log(Ce/Ci))/ΔT) have positive values. According to the gradient (Δ(log(Ce/Ci))/ΔT), dependence of trace metals sorption by plastic particles on constant temperature are following the order (R2 = 0.78-0.99) (Supplementary Table S2):
a. OM=2.8% (Table 2):
micro-PETE (fiber): Cd (0.0158) > Cu (0.0131) > Pb (0.0125) > Zn (0.0078)
micro-HDPE (microbeads): Cu (0.0162) > Zn (0.0097) > Cd (0.0079) > Pb (0.0044)
b. OM=15.8% (Table 2):
micro-PETE (fiber): Cd (0.0189) > Zn (0.0147) > Cu (0.0119) > Pb (0.0072)
micro-HDPE (microbeads): Cu (0.0194) > Zn (0.0095) > Cd (0.0067) > Pb (0.0022)
By estimating the value of the (Δ(log(Ce/Ci))/ΔT) we can conclude that in relation to polymer type, sorption behavior of micro-PETE towards Cd (Δ(log (Ce/Ci))/ΔT = 0.0189 (Cd, R2 = 0.95)) has a stronger temperature dependence compared to the micro-HDPE (Δ(log(Ce/Ci))/ΔT = 0.0067 (Cd, R2 = 0.85)). In contrast, sorption behavior of macro-PETE towards Pb (Δ(log(Ce/Ci))/ΔT = 0.0079 (Pb, R2 = 0.78)) is less dependent on temperature compared to the macro-HDPE (Δ(log (Ce/Ci))/ΔT = 0.0150 (Pb, R2 = 0.88)). Regarding particle size, sorption behavior of macroplastics towards trace metals (Cd, Cu, Pb, and Zn) (Figures 2C, D–5C, D) is strongly influenced by temperature compared to the microparticles (Figures 2A, B–5A, B).
In relation to the type of trace metals, sorption of Cu by microplastics have stronger dependence on temperature than those observed for Cd, Pb, and Zn. Sorption of trace metals by macroplastics has very similar dependence on temperature, except Zn sorption by macro-HDPE ((Δ(log (Ce/Ci))/ΔT = 0.0084 (Zn)) and Pb by macro-PETE ((Δ(log(Ce/Ci))/ΔT = 0.0079 (Zn)) with lower dependence on temperature in sediments high in OM content (Supplementary Table S2). In general, dependence of trace metals sorption by macro- and microplastics on temperature is slightly different in sediments with low OM (2.8%) and high OM (15.8%) content.
4.3 Partitioning of trace metals in the intertidal sediments with macro- and microplastics content under constant temperature conditions
To compare the distribution of trace metals in two contrasting sedimentary environments with components of plastic particles (PETE and HDPE) and under the three temperature conditions the ratio of intertidal sediments-pore water partitioning coefficients (Ksed-wp) to the polymer materials-pore water partitioning coefficients (Kpl-wp) of trace metals (Cd, Cu, Pb, and Zn) in sediments with low OM (2.8%) and high OM (15.8%) content was calculated as (Ksed-wp)/(Kpl-wp) (Equations 1–9; Figures 6–9).
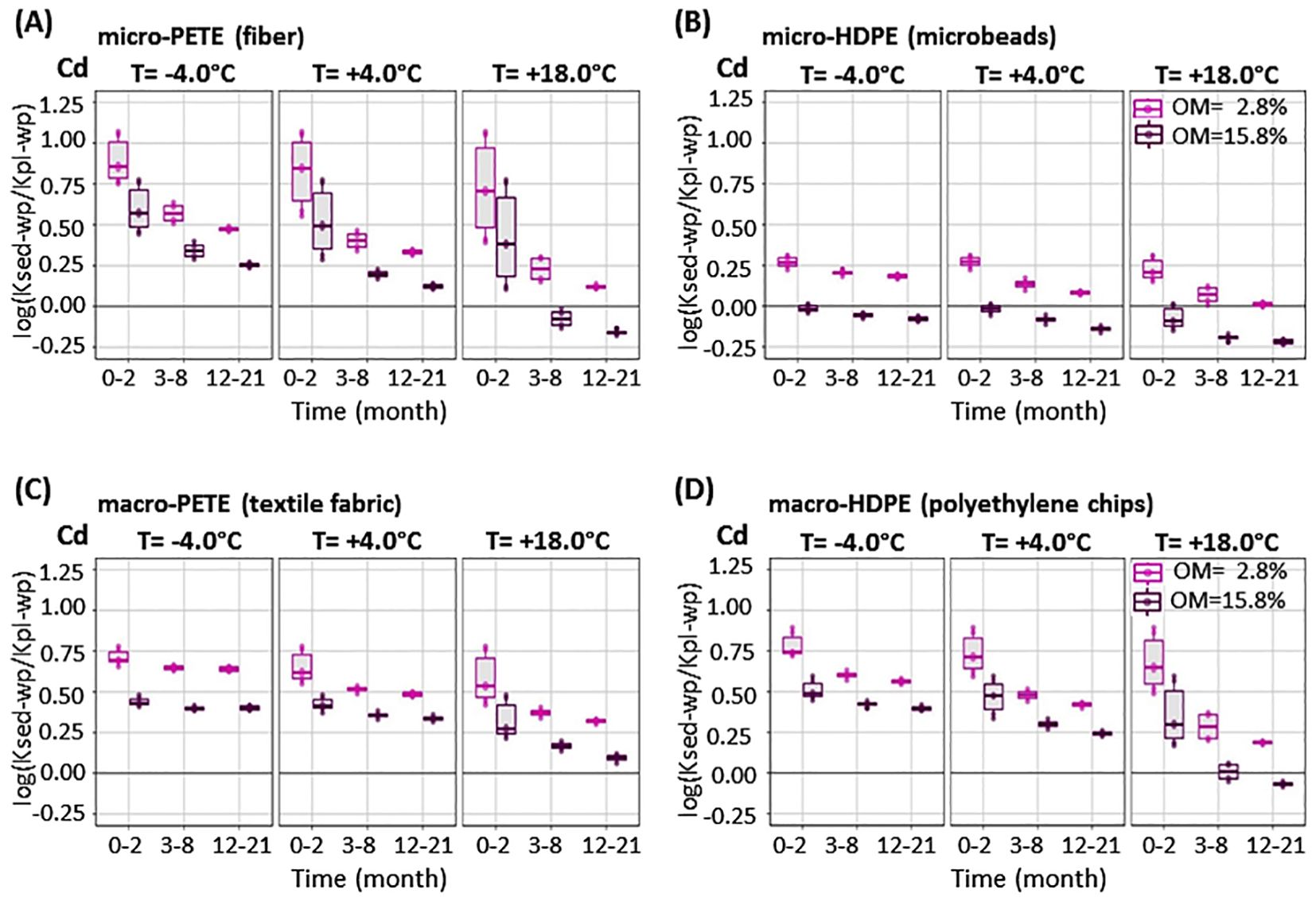
Figure 6. The value of log(Ksed-wp/Kpl-wp) of Cd over time for micro-PETE (fiber) (A),micro-HDPE (microbeads) (B), macro-PETE (textile fabric (C), and macro-HDPE (polyethelene chips) (D) deployed in the intertidal sediments. Laboratory experiments were performed with sediments low (OM=2.8%) and high (OM=15.8%) in organic matter content and constant temperature of T=- 4.0°C, T=+4.0°C, and T=+18.0°C.
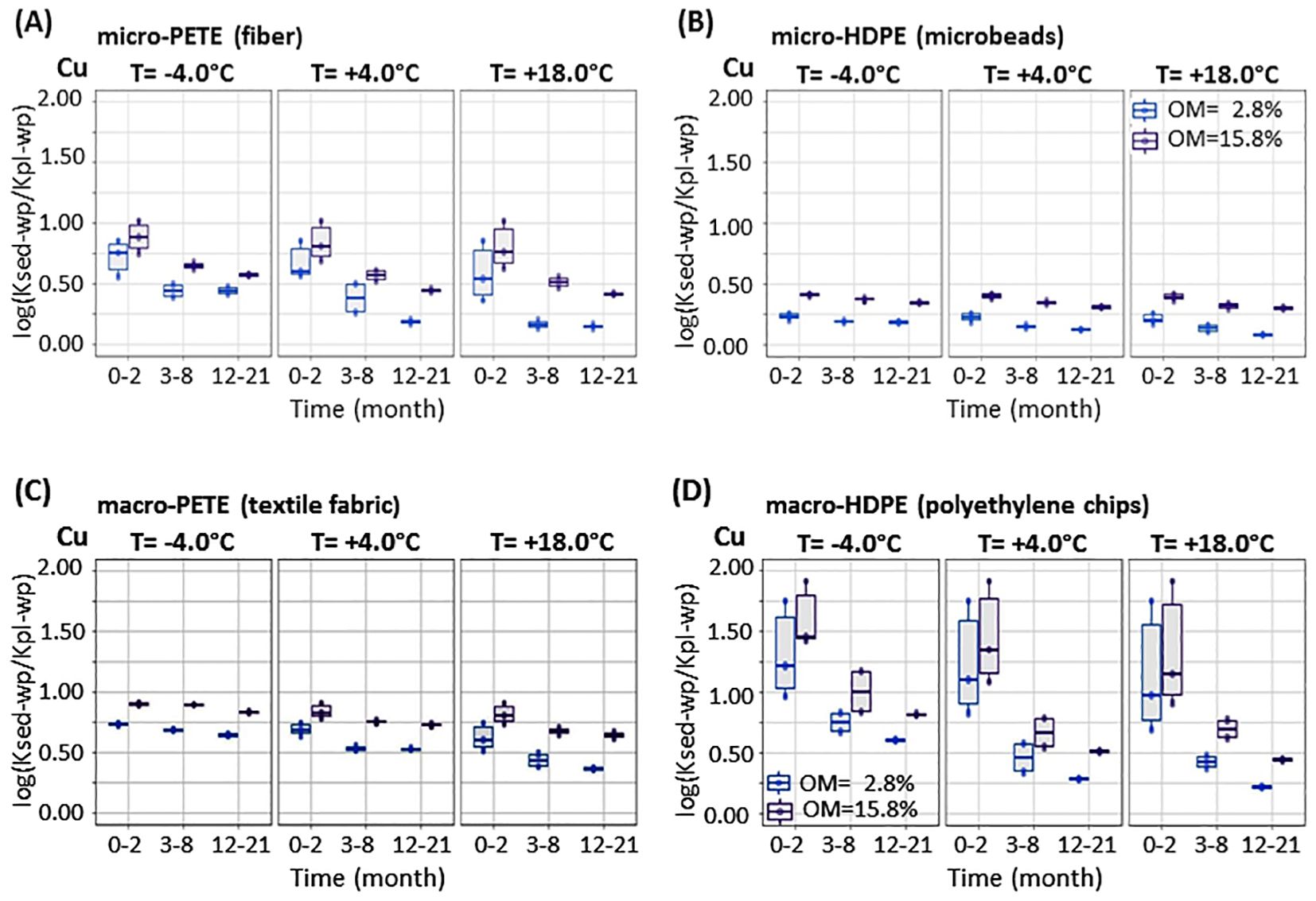
Figure 7. The value of log(Ksed-wp/Kpl-wp) of Cu over time for micro-PETE (fiber) (A), micro-HDPE (microbeads) (B), macro-PETE (textile fabric (C), and macro-HDPE(polyeyhelene chips) (D) deployed in the intertidal sediments. Laboratory experiments were performed with sediments low (OM=2.8%) and high (OM=15.8%) in organic matter content and constant temperature of T=- 4.0°C, T=+4.0°C, and T=+18.0°C.
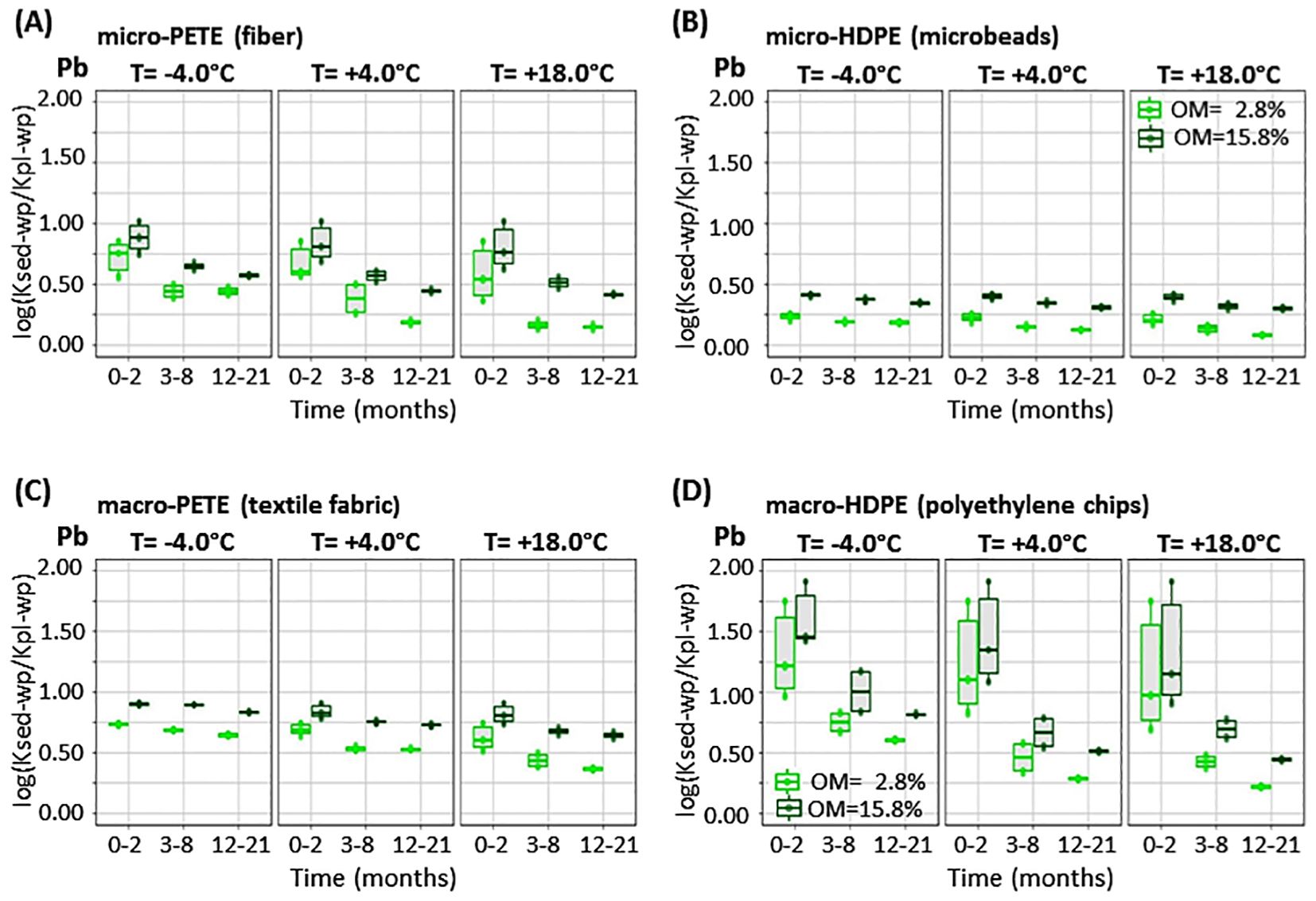
Figure 8. The value of log(Ksed-wp/Kpl-wp) of Pb over time for micro-PETE (fiber) (A), micro-HDPE (microbeads) (B), macro-PETE (textile fabric) (C), and macro-HDPE (polyethelene chips) (D) deployed in the intertidal sediments. Laboratory experiments were performed with sediments low (OM=2.8%) and high (OM=15.8%) in organic matter content and constant temperature of T=- 4.0°C, T=+4.0°C, and T=+18.0°C.
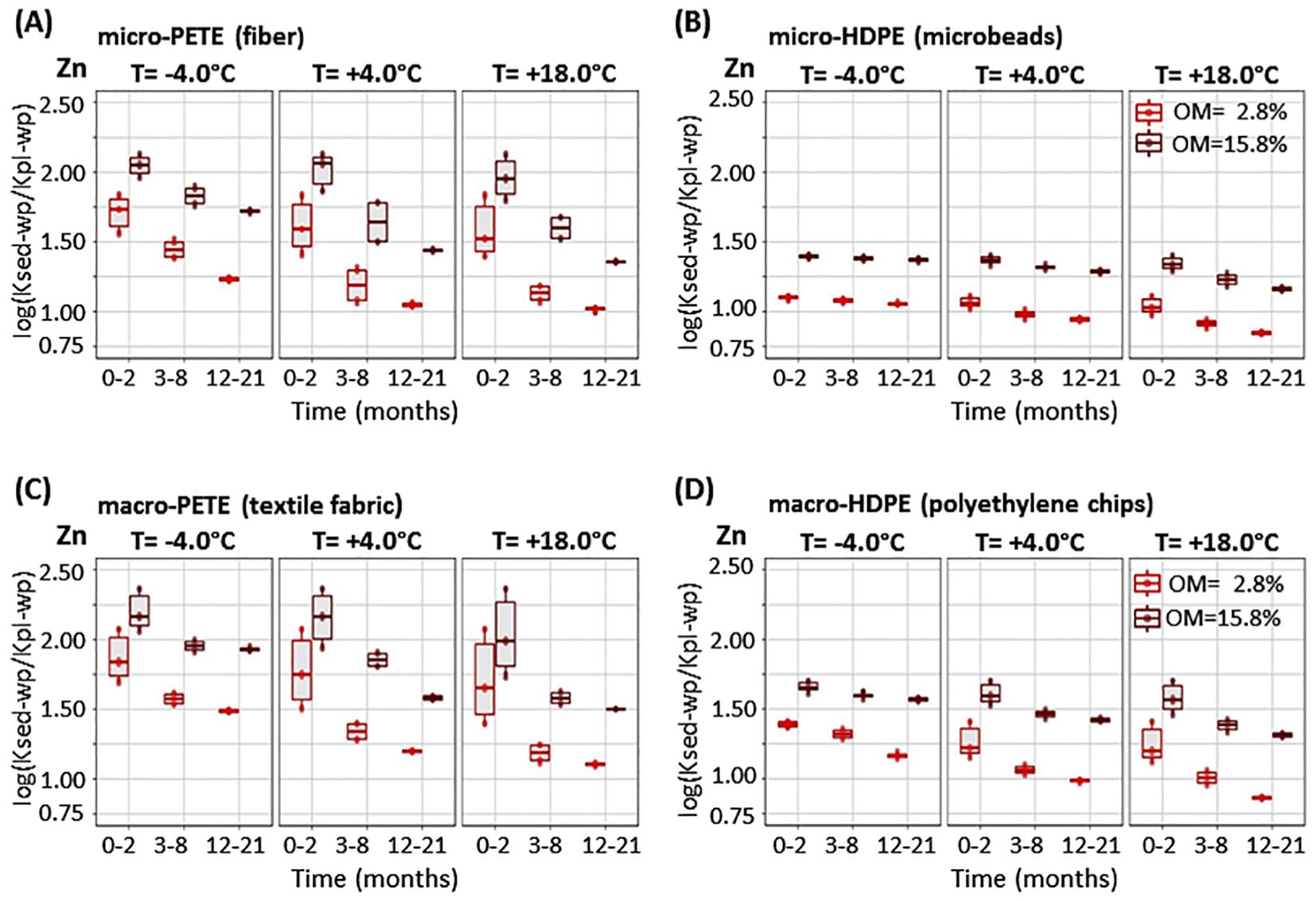
Figure 9. The value of log(Ksed-wp/Kpl-wp) of Zn over time for micro-PETE (fiber) (A),micro-HDPE (microbeads) (B), macro-PETE (textile fabric) (C), and macro-HDPE(polyethylene chips) (D) deployed in the intertidal sediments. Laboratory experiments were performed with sediments low (OM=2.8%) and high (OM=15.8%) in organic matter content and constant temperature of T=- 4.0°C, T=+4.0°C, and T=+18.0°C.
In general, the values of log[(Ksed-wp)/(Kpl-wp)] of Cd, Cu, Pb, and Zn for macro-HDPE (polyethylene chips), macro-PETE (textile fabric), micro-HDPE (microbeads), and micro-PETE (fibers) are significantly time-dependent and strongly decreased over time during all of three periods of sampling (i.e., 0-2 months, “short term”; 3-8 months, “mid-term”; 12-21 months, “long term”) before reaching equilibrium at both sedimentary environments, high and low in OM, and under conditions of all three constant temperature applied in the laboratory experiments: (i.e.,T=- 4.0°C, T=+ 4.0°C, and T=+18.0°C) (Figures 6–9). The intensity of decreasing the value log [(Ksed-wp)/(Kpl-wp)] of Cd, Cu, Pb, and Zn for macro- and microplastics is greater under conditions of high temperature (T=+18°C) compared to that at low temperature (T=- 4.0°C), as observed in Figures 6–9. These findings can be interpreted to suggest the partitioning of trace metals between sediments and macro- and microplastics is greater at higher temperature of T=+18.0°C relative to that at low temperature (T=- 4.0°C).
The value of log[(Ksed-wp)/(Kpl-wp)] of Cu, Pb, and Zn, except of Cd, was greater for macro- and microplastics of HDPE and PETE deployed in sediments high in OM (15.8%) compared to that in sediments with low in OM (2.8%) and under conditions of each constant temperature (see trends from Figures 6–9). These findings can be explained as a phenomenon of competitive adsorption between plastic particles and organic particles in sediments which is in accordance with Gomez et al. (1999). Such findings suggest that temperature has minor influence on the partitioning of Cu, Pb, and Zn, except of Cd, between plastic particles and intertidal sediments comparison to the influence of OM content of 15.8%.
By estimating the logarithmic value of the ratio of (Ksed-wp) to (Kpl-wp) of trace metals, the distribution of Cd, Cu, Pb, and Zn can be defined as following (using Equation 9):
The log[(Ksed-wp)/(Kpl-wp)] may have “positive”, “0”, or “negative” value which indicate that concentration of trace metals in the intertidal sediments is respectively greater, the same, or lower than that in the macro- and microplastics (Equations 10–12). The trace metals - macro- and microplastics interaction study in the intertidal sediments with constant temperature conditions revealed that the log(Ksed-wp/Kpl-wp) have “positive” value for Cu, Zn, and Pb while it yielded “0” and “negative” value for Cd only in sediments high in OM for micro-HDPE (microbeads) at T=- 4.0°C, T=+ 4.0°C, and T=+18.0°C; as well as in micro-PETE (fibers) and macro-HDPE (polyethylene chips) at T=+18.0°C (Figures 6–9). These results illustrate the phenomenon of competitive adsorption between plastic materials and organic particles in sediments. This can be interpreted that under temperature condition of T=+18.0°C only Cd, compared to Cu, Zn, and Pb, can partition to plastic particles more readily than to sediments high in OM content of 15.8%. (Figure 6).
To estimate the degree to which temperature influences the distribution of trace metals between intertidal sediments and macro- and microplastics, the ratio of (Ksed-wp)e to (Kpl-wp)e of Cd, Cu, Pb, and Zn in sediments low (OM = 2.8%) and high (OM = 15.8%) in OM fractions were calculated as [(Ksed-wp)e/(Kpl-wp)e] (Equations 4–9; Table 3). In general, the value of log[(Ksed-wp)e/(Kpl-wp)e] of Cd, Cu, Pb, and Zn for macro- and microplastics of HDPE and PETE decreased with increasing temperature (T=- 4.0°C, T=+ 4.0°C, and T=+18.0°C) in both intertidal sediments with low (OM=2.8%) and high (OM=15.8%) organic matter content.
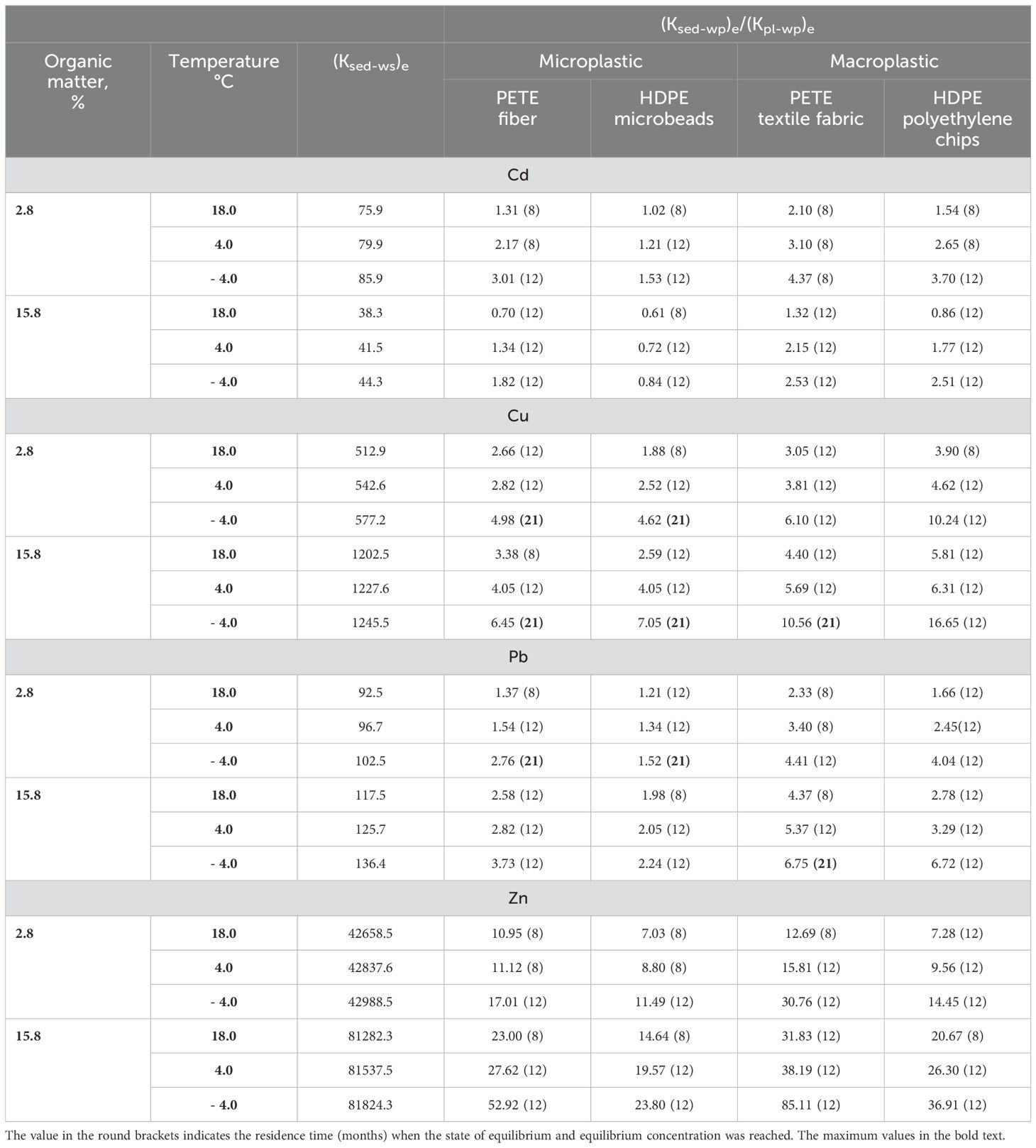
Table 3. The values of the ratio of intertidal sediments-pore water equilibrium partitioning coefficient ((Ksed-wp)e) to the plastic particles-pore water equilibrium partitioning coefficient ((Kpl-wp)e) as [(Ksed-wp)e/(Kpl-wp)e] of Cd, Cu, Pb and Zn.
The value of log[(Ksed-wp)e/(Kpl-wp)e] of Cd, Cu, Pb, and Zn was greater for macro- and microplastics of HDPE and PETE deployed in intertidal sediments high in OM content (OM=15.8%) compared to that in sediments low in OM content (OM = 2.8%) at each constant temperature (Table 3). These finding can be explained that under laboratory controlled temperature condition (i.e., T=- 4.0°C, T=+ 4.0°C, and T=+18.0°C) the constant temperature have minor influence on the partitioning of Cu, Pb, and Zn, except of Cd, between plastic particles and intertidal sediments compare to the influence of organic matter of OM=15.8%.
Dependence of trace metals distribution in the intertidal sediments on temperature can be expressed by gradient (Δ(log[(Ksed-wp)e/(Kpl-wp)e])/ΔT). The value of the gradient Δ(log[(Ksed-wp)e/(Kpl-wp)e])/ΔT of Cd, Cu, Pb, and Zn had very small variation for macro-PETE and macro-HDPE (-0.0165 (Zn, R2 = 0.83); -0.0163 (Pb, R2 = 0.77)) and micro-PETE and micro-HDPE (-0.0077 (Zn, R2 = 0.66); -0.0075 (Cd, R2 = 0.97)) deployed in sediments low in OM content (2.8%). In addition, macroplastics of HDPE and PETE deployed in sediments with low and high OM content showed greater dependence on temperature. Hence, a sediment geochemical characteristic such as OM content has a greater influence on the sorption of trace metals by plastics as compared to temperature.
To increase environmental relevance of our study, the temperature conditions were based of the analysis of temperature amplitude and annual range of temperature (T=- 4.0°C and T=+18.0°C) relevant to the intertidal area of Burrard Inlet (British Columbia, Canada) where the sediments were sampled for the long-term laboratory study. Overall, this study enhances our understanding of trace elements-plastic particles interactions in intertidal sediments. Our goal is working towards a tool that can be used to assess the temperature conditions under which macro- and microplastics pose the greatest threat by providing an additional vector of Cd, Cu, Pb, and Zn exposure into benthic food webs. Our findings unveiled some areas of research that need further consideration. The study on sorption of trace metals by plastic particles within intertidal sedimentary environment with wide range of temperature conditions requires more field-experimental research. It would be beneficial to investigate the influence of temperature on the plastic particles degradation/aging and partitioning of trace metals as this is related to their bioavailability. The long-term field experiments on trace metals – plastic particle interactions are preferable, because they provide valuable information on sorption processes under realistic environmental conditions.
5 Conclusions
This study investigated the effect of constant temperature (T=- 4.0°C, T=+ 4.0°C, and T=+18.0°C) on sorption of trace metals (Cd, Cu, Pb, and Zn) by macro- and microplastics of PETE and HDPE within two contrasting intertidal sedimentary environments which contained low (2.8%) and high (15.8%) levels of OM content.
Key outcomes from this study include the following findings:
1. Temperature influences the sorption and partitioning of Cu, Zn, Pb, and Cd. Concentrations of Cd, Cu, Pb, and Zn in macro- and microplastic of HDPE and PETE were higher and reached equilibrium state and equilibrium concentration faster at higher constant temperature of T=+18.0°C at 8-12 months compared to that at lower constant temperature of T=- 4.0°C at 12-21 months.
2. The interaction between trace metals (Cd, Cu, Pb, and Zn) and macro- and microplastics in the intertidal sediments under laboratory-controlled temperatures conditions of T=- 4.0°C, T=+ 4.0°C, and T=+18.0°C can be characterized as an accumulation process over time, consisting of two distinct phases: an initial phase of rapid adsorption followed by a phase of slowly adsorption towards equilibrium.
3. The adsorbed concentration of Cd, Cu, Pb, and Zn in macro- and microplastics of HDPE and PETE deployed under the three temperature conditions was higher in sediments low in OM content (OM = 2.8%) compare to that in sediments high in OM content.
4. In regards to polymer type, the sorption behavior of micro-PETE towards Cd, Cu, Pb, and Zn has a greater dependence on temperature compared to the micro-HDPE. In contrast, the sorption behavior of macro-PETE towards Cd, Cu, Pb, and Zn is less dependent on temperature compared to the macro-HDPE. In regards to the particle size, sorption behavior of macroplastics towards trace metals (Cu, Zn, Pb, Cd, and Hg) is more dependent on temperature in comparison to the microplastics.
5. In relation to the type of trace metals, sorption of Cu by microplastics exhibits stronger dependence on temperature compared to Cd, Pb, and Zn.
6. The partitioning of trace metals (Cd, Cu, Pb, and Zn) between sediments and macro- and microplastics is higher at high temperature (T=+18.0°C) than at low temperature (T=-4.0°C). In general, the value of log[(Ksed-wp)e/(Kpl-wp)e] of Cd, Cu, Pb, and Zn for macro- and microplastics of HDPE and PETE decreased with increasing temperature (T=- 4.0°C, T=+ 4.0°C, and T=+18.0°C) in intertidal sediments with both low and high OM content (2.8% and 15.8%, respectively).
7. Under laboratory controlled conditions temperature had less influence on the partitioning of Cu, Pb, and Zn, between plastic particles and intertidal sediments compared to the influence of OM=15.8%, except for Cd. It was found that at high temperature (T=+18.0°C) only Cd can partition to plastic particles more readily than to sediments high in OM content (15.8%).
These findings enhances our understanding of how temperature can effect trace metals-plastic particle interactions in the marine intertidal sedimentary environment providing insight as to conditions that will create the greatest threat to higher trophic levels by providing an additional vector of Cd, Cu, Pb, and Zn exposure into benthic food webs. The use of plastic materials is a reality of our economic culture and, therefore, a better understanding of their behavior and interaction with other pollutants in the surrounding environment is necessary.
Data availability statement
The raw data supporting the conclusions of this article will be made available by the authors, without undue reservation.
Author contributions
TK: Conceptualization, Data curation, Formal Analysis, Investigation, Methodology, Software, Validation, Visualization, Writing – original draft, Writing – review & editing. JA: Writing – review & editing. EP: Writing – review & editing. LB: Conceptualization, Funding acquisition, Investigation, Methodology, Supervision, Writing – review & editing.
Funding
The author(s) declare that financial support was received for the research and/or publication of this article. This work partially funded by the Department of Biological Sciences, Simon Fraser University (Canada) and a Discovery NSERC research grant to L.I. Bendell. This work was also supported by the Pacific Wildlife Research Centre Environment and Climate Change Canada (ECCC) and the Nippon Foundation-Ocean Litter Project from the Ocean Pollution Research Unit (OPRU) at the Institute for the Oceans and Fisheries (IOF), University of British Columbia (Canada).
Acknowledgments
The authors would like to thank Wen Zhou (Instrument Technician, Chemistry Department, SFU) for the providing support and performing the FTIR analysis of macro- and microplastic samples. We thank reviewers for the helpful comments on the manuscript.
Conflict of interest
The authors declare that the research was conducted in the absence of any commercial or financial relationships that could be construed as a potential conflict of interest.
The author(s) declared that they were an editorial board member of Frontiers, at the time of submission. This had no impact on the peer review process and the final decision
Generative AI statement
The author(s) declare that no Generative AI was used in the creation of this manuscript.
Publisher’s note
All claims expressed in this article are solely those of the authors and do not necessarily represent those of their affiliated organizations, or those of the publisher, the editors and the reviewers. Any product that may be evaluated in this article, or claim that may be made by its manufacturer, is not guaranteed or endorsed by the publisher.
Supplementary material
The Supplementary Material for this article can be found online at: https://www.frontiersin.org/articles/10.3389/fmars.2025.1570114/full#supplementary-material
References
Aksu Z., Kutsal T. A. (1991). A bioseparation process for removing Pb (II) Ions from wastewater by using C. Vulgaris. J. Chem. Technol. Biotechnol 52, 108–118.
Alava J. J., Jahnke J., Bergmann M., Aguirre-Martínez G. V., Bendell L., Calle P., et al. (2023). A call to include plastics in the global environment in the class of persistent, bioaccumulative, and toxic (PBT) pollutants. Environ. Sci. Technology 57, 8185–8188. doi: 10.1021/acs.est.3c02476
Alimi O. S., Farner J. M., Tufenkji N. (2020). Exposure of nanoplastics to freeze-thaw leads to aggregation and reduced transport in model groundwater environments. Water Res 189, 116533.
Arthur C., Baker J., Bamford H. (Eds.) (2009). Proceedings of the International Research Workshop on the Occurrence, Effects, and Fate of Microplastic Marine Debris, September 9-11, 2008, (University of Washington Tacoma, Tacoma, WA, USA: NOAA Technical Memorandum NOS-OR & R-30).
Ashton K., Holmes L., Turner A. (2010). Association of metals with plastic production pellets in the marine environment. Mar. pollut. Bulletin 60, 2050–2055.
ASTM (2000a). “Standard guide for collection, storage, characterization, and manipulation of sediments for toxicological testing, E 1391-94,” Annual Book of ASTM Standards, Vol. 11.03. (Philadelphia, PA: American Society for Testing and Materials).
ASTM (2000b). “Guide for core-sampling submerged unconsolidated sediments, D 4823-95,” in Annual Book of ASTM Standards, Vol. 11.03. (Philadelphia, PA: American Society for Testing and Materials).
ASTM (2000c). “Standard test methods for moisture, ash, and organic matter of peat and other organic soils,” in Annual Book of ASTM Standards, Vol. 11.03. (Philadelphia, PA: American Society for Testing and Materials).
Bakir A., Desender M., Wilkinson T., Van Hoytema N., Amos R., Airahui S., et al. (2020). Occurrence and abundance of meso and microplastics in sediment, surface waters, and marine biota from the South Pacific region. Mar. pollut. Bulletin 160. doi: 10.1016/j.marpolbul.2020.111572
Bakir A., Rowland S. J., Thompson R. C. (2014). Enhanced desorption of persistent organic pollutants from microplastics under simulated physiological conditions. Environ. Pollution 185, 16–23. doi: 10.1016/j.envpol.2013.10.007
Barboza L. G. A., Vieira L. R., Branco V., Carvalho C., Guilhermino L. (2018). Microplastics increase mercury bioconcentration in gills and bioaccumulation in the liver, and cause oxidative stress and damage in Dicentrarchus labrax juveniles. Sci. Rep 8, 15655. doi: 10.1038/s41598-018-34125-z
Batley G. E., Simpson S. L. (2016). “Sediment sampling, sample preparation and general analysis,” in Sediment quality assessment: A practical guide, 2nd. Eds. Simpson S. L., Batley G. E. (CSIRO Publishing, Clayton South, Vic), 15–45. Available at: http://hdl.handle.net/102.100.100/91038?index=1 (Accessed May 21, 2020).
Baztan J., Jorgensen B., Carney Almroth B., Bergmann M., Farrelly T., Muncke J., et al. (2024). Primary Plastic Polymers: urgently needed upstream reduction. Cambridge Prisms: Plastics 2, 1–2. doi: 10.1017/plc.2024.8
Boucher C., Morin M., Bendell L. I. (2016). The influence of cosmetic microbeads on the sorptive behavior of cadmium and lead within intertidal sediments: a laboratory study. Regional Stud. Mar. Science 3, 1–7.
Bouwmeester H., Hollman P. C. H., Peters R. J. B. (2015). Potential health impact of environmentally released micro- and nanoplastics in the human food production chain: experiences from nanotoxicology. Environ. Sci. Technology 49, 8932–8947.
Brander S. M., Senathirajah K., Fernandez M. O., Weis J. S., Kumar E., Jahnke A., et al. (2024). The time for ambitious action is now: Science-based recommendations for plastic chemicals to inform an effective global plastic treaty. Sci. Total Environment 949, 1–11. doi: 10.1016/j.scitotenv.2024.174881
Brennecke D., Duarte B., Paiva F., Caçador I., Canning-Clode J. (2016). Microplastics as vector for heavy metal contamination from the marine environment. Estuarine Coast. Shelf Science 178, 189–195. doi: 10.1016/j.ecss.2015.12.003
Browne M. A., Crump P., Niven S. J., Teuten E., Tonkin A., Galloway T., et al. (2011). Accumulation of microplastic on shorelines worldwide: sources and sinks. Environ. Sci. Technology 45, 9175–9179.
Bull J., Freyman L. (2013). Status of water quality objectives attainment in burrard inlet and tributaries 1990-2010. (Surrey, BC: Ministry of Environment), 56. Available online at: http://www.env.gov.bc.ca/epd/regions/lower_mainland/water_quality/reports/burrard_inlet/bi_attain.
Burrard Inlet Action Plan: A Tsleil-Waututh Perspective (BIAP) (2016). “A science-based, First Nations-led initiative to improve the health of the Burrard Inlet ecosystem by 2025. Public Review Draft. (January 2016),” in The burrard inlet action plan: A tsleil-waututh perspective (Vancouver, BC, Canada: Kerr Wood Leidal Associates Ltd.), 128. Available at: https://msbernabei.weebly.com/uploads/8/7/7/6/8776151/twn-burrard-inlet-action-plan-summary_1.pdf (Accessed October 20, 2017).
Burrard Inlet Action Program (BIEAP) (2011). “Consolidated environmental management plan for burrard inlet update (CEMP),” in Burrard inlet environmental action program.(November 2011) (Vancouver, BC, Canada: Kerr Wood Leidal Associates Ltd.). Available online at: https://waves-vagues.dfo-mpo.gc.ca/library-bibliotheque/218464_2012.pdf (Accessed September 2, 2017).
Cao Y., Zhao M., Ma X., Song Y., Zuo S., Li H., et al. (2021). A critical review on the interactions of microplastics with heavy metals: Mechanism and their combined effect on organisms and humans. Sci. Total Environ 788. doi: 10.1016/j.scitotenv.2021.147620
CCME (Canadian Council of Ministers of the Environment). (2007). “Canadian sediment quality guidelines for the protection of aquatic life: Summary table. Updated September, 2007,” in Canadian environmental quality guidelines (Winnipeg: Canadian Council of Ministers of the Environment).
Charles J., Ramkumaar G. R. (2009). Qualitative analysis of high density polyethylene using FTIR spectroscopy. Asian J. Chem 21, 4477–4484.
Chen X., Liang J., Bao L., Gu X., Zha S., Chen X. (2022). Competitive and cooperative sorption between triclosan and methyltriclosan on microplastics and soil. Environ. Res 212. doi: 10.1016/j.envres.2022.113548
Coates J. (2006). “Interpretation of infrared spectra, a practical approach,” in Encyclopedia of analytical chemistry. Ed. Meyers R. A. (John Wiley & Sons Ltd, Chichester), 10815–10837. doi: 10.1002/9780470027318.a5606
Cole M., Lindeque P., Halsband C., Galloway T. S. (2011). Microplastics as contaminants in the marine environment: a review. Mar. pollut. Bulletin 62, 2588–2597.
Derraik J. G. B. (2002). The pollution of the marine environment by plastic debris: a review. Mar. pollut. Bulletin 44, 842–852.
Engler R. E. (2012). The complex interaction between marine debris and toxic chemicals in the ocean. Environ. Sci. Technology 46, 12302–12315.
Eriksen M., Cowger W., Erdle L. M., Coffin S., Villarrubia-Gómez P., Moore C. J., et al. (2023). A growing plastic smog, now estimated to be over 170 trillion plastic particles afloat in the world’s oceans—Urgent solutions required. PloS One 18. doi: 10.1371/journal.pone.0281596
Frias J. P., Nash R. (2019). Microplastics: finding a consensus on the definition. Mar. pollut. Bulletin 138, 145–147.
Gao F., Li J., Sun C., Zhang L., Jiang F., Cao W., et al. (2019). Study on the capability and characteristics of heavy metals enriched on microplastics in marine environment. Mar. pollut. Bull 144, 61–67.
Garson G. D. (2012). Testing statistical assumptions (USA: G.David and Statistical Associates Publishing), 54.
GESAMP (2015). “Sources, fate and effects of microplastics in the marine environment: a global assessment. ed. Kershaw P. J.. (IMO/FAO/UNESCO-IOC/UNIDO/WMO/IAEA/UN/UNEP/UNDP Joint Group of Experts on and t. S. A. o. M. E. Protection). Rep. Stud. GESAMP No. 90: 96 pp.
Gigault J., ter Halle A., Baudrimont M., Pascal P., Gauffre F., Phi T., et al. (2018). Current opinion: What is a nanoplastic? Environ. Pollution 235, 1030–1034. doi: 10.1016/j.envpol.2018.01.024
Gomez C., Bosecker K. (1999). Leaching heavy metals from contaminated soil using Thiobacillus ferrooxidans or Thiobacillus thiooxidans. Geomicrobiology J 16, 233–244. doi: 10.1080/014904599270613
Gulmine J. V., Janissek P. R., Heise H. M., Akcelrud L. (2002). Polyethylene characterization by FTIR. Polymer Testing 21, 557–563. doi: 10.1016/S0142-9418(01)00124-6
Guo X., Wang J. L. (2019). The chemical behaviours of microplastics in marine environment: a review. Mar. pollut. Bull 142, 1–14.
Guo X., Wang J. L. (2021). Projecting the sorption capacity of heavy metal ions onto microplastics in global aquatic environments using artificial neural networks. J. Hazardous Materials 402, 123709. doi: 10.1016/j.jhazmat.2020.123709
Harris P. T. (2020). The fate of microplastic in marine sedimentary environments: A review and synthesis. Mar. pollut. Bull. 158, 1–25, 111398.
Harrison J. P., Schratzberger M., Sapp M., Osborn A. M. (2014). Rapid bacterial colonization of low-density polyethylene microplastics in coastal sediment microcosms. BMC Microbiol 14)232. doi: 10.1186/s12866-014-0232-4
Hartmann N. B., Rist S., Bodin J., Jensen L. H., Schmidt S. N., Mayer P., et al. (2017). Microplastics as vectors for environmental contaminants: exploring sorption, desorption, and transfer to biota. Integrated Environ. Assess. Manage 3)13, 488–493.
He W., Liu S., Zhang Z., Yi K., Zhang C., Pang H., et al. (2023). Recent advances on microplastic aging: Identification, mechanism, influence factors, and additives release. Sci. Total Environment 889. doi: 10.1016/j.scitotenv.2023.164035
Hidalgo-Ruz V., Gutow L., Thompson R. C., Thiel M. (2012). Microplastics in the marine environment: A review of the methods used for identification and quantification. Environ. Sci. Technology 46, 3060–3075.
Ho N. H. E., Not C. (2019). Selective accumulation of plastic debris at the breaking wave area of coastal waters. Environ. Pollution 245. doi: 10.1016/j.envpol.2018.11.041
Holmes L. A. (2013). Interactions of trace metals with plastic production pellets in the marine environment. (PhD thesis (UK: University of Plymouth), 199.
Holmes L. A., Turner A., Thompson R. C. (2012). Adsorption of trace metals to plastic resin pellets in the marine environment. Environ. Pollution 160, 42–48.
Holmes L. A., Turner A., Thompson R. C. (2014). Interactions between trace metals and plastic production pellets under estuarine conditions. Mar. Chem 167, 25–32.
Hummel D. O. (2002). Atlas of plastics additives: analysis by spectrometric methods (Berlin Heidelberg: Springer-Verlag).
Jambeck J. R., Geyer R., Wilcox C., Siegler T. R., Perryman M., Andrady A., et al. (2015). Plastic waste inputs from land into the ocean. Science 347, 768–771. doi: 10.1126/science.1260352
Johannessen S. C., Macdonald R. W., Magnus Eek K. (2005). Historical trends in mercury sedimentation and mixing in the Strait of Georgia, Canada. Environ. Sci. Technol 39, 4361–4368.
Kazmiruk T. N. (2023). Sorption of trace elements (Cd, Cu, Hg, Pb, and Zn) by macro- and microplastics within intertidal sediments; A laboratory, field and modeling approach. (PhD Thesis (Burnaby, BC, Canada: Simon Fraser University), 191.
Kazmiruk T. N., Alava J. J., Palsson E., Bendell I. L. (2024). Sorption of trace metals by macro- and microplastics within intertidal sediments: Insights from a long-term field study within Burrard Inlet, British Columbia, Canada. Sci. Total Environment 951. doi: 10.1016/j.scitotenv.2024.175413
Kazmiruk N. T., Kazmiruk V. D., Bendell L. I. (2018). Abundance and distribution of microplastics within surface sediments of a key shellfish growing region of Canada. PloS One 13. doi: 10.1371/journal.pone.0196005
Khalid N., Aqeel M., Noman A., Hashem M., Mostafa Y. S., Alhaithloul H. A. S., et al. (2021). Linking effects of microplastics to ecological impacts in marine environments. Chemosphere 264, 12854.
Kim I.-S., Chae D.-H., Kim S.-K., Choi S., Woo S.-B. (2015). Factors influencing the spatial variation of microplastics on high-tidal coastal beaches in Korea. Arch. Environ. Contamination Toxicology 69, 299–309. doi: 10.1007/s00244-015-0155-6
Kolda A., Mucko M., Rapljenović A., Ljubešić Z., Pikelj K., Kwokal Z., et al. (2024). Beach wracks microbiome and its putative function in plastic polluted Mediterranean marine ecosystem. Mar. Environ. Res 106769. doi: 10.1016/j.marenvres.2024.106769
Lavoie R. A., Jardine T. D., Chumchal M. M., Kidd K. A., Campbell L. M. (2013). Biomagnification of mercury in aquatic food webs: a worldwide meta-analysis. Environ. Sci. Technol 47, 13385–13394. doi: 10.1021/es403103t
Lebreton L. C. M., van der Zwet J., Damsteeg J.-W., Slat B., Andrady A., Reisser J. (2017). River plastic emissions to the world’s oceans. Nat. Commun 8. doi: 10.1038/ncomms15611
Li Y., Li M., Li Z., Yang L., Liu X. (2019). Effects of particle size and solution chemistry on Triclosan sorption on polystyrene microplastic. Chemosphere 231, 308–314. doi: 10.1016/j.chemosphere.2019.05.116
Li W., Lo H. S., Wong H. M., Zhou M., Wong C. Y., Tem N. F. Y., et al. (2020). Heavy metals contamination of sedimentary microplastics in Hong Kong. Mar. pollut. Bulletin 153, 110977.
Liebezeit G., Dubaish F. (2012). Microplastics in beaches of the east frisian islands spiekeroog and kachelotplate. Bull. Environ. Contamination Toxicology. 89 213-217.
Liu S., Shi J., Wang J., Dai Y., Li H., Li J., et al. (2021). Interactions between microplastics and heavy metals in aquatic environments: A review. Front. Microbiol 12. doi: 10.3389/fmicb.2021.652520
Liu Q., Wu H., Chen J., Guo B., Zhao X., Lin H., et al. (2022). Adsorption mechanism of trace heavy metals on microplastics and simulating their effect on microalgae in river. Environ. Res 113777. doi: 10.1016/j.envres.2022.113777
Liu J., Zhang T., Tian L. L., Liu X. L., Qi Z. C., Ma Y. N., et al. (2019). Aging significantly affects mobility and contaminant-mobilizing ability of nanoplastics in saturated loamy sand. Environ. Sci. Technology 53, 5805–5815.
Lohmann R. (2017). Microplastics are not important for the cycling and bioaccumulation of organic pollutants in the oceans-but should microplastics be considered POPs themselves? Integrated Environ. Assess. Manage. (IEAM) 13, 460–465.
Lusher A. L. (2015). “Microplastics in the marine environment: distribution, interactions and effects,” in Marine anthropogenic litter. eds. Bergmann M., Gutow L., Klages K. (Springer), 245–307. doi: 10.1007/978-3-319-16510-3
Maršić-Lučić J., Lušić J., Tutman P., Bojanić V. D., Šiljić J., Pribudić J. (2018). Levels of trace metals on microplastic particles in beach sediments of the island of Vis, Adriatic Sea, Croatia. Mar. pollut. Bull 137, 231–236.
Martin J., Lusher A., Thompson R. C., Morley A. (2017). The deposition and accumulation of microplastics in marine sediments and bottom water from the Irish continental shelf. Sci. Rep 7, 1–9. doi: 10.1038/s41598-017-11079-2
Meijer L. J., van Emmerik T., van der Ent R., Schmidt C., Lebreton L. (2021). More than 1000 rivers account for 80% of global riverine plastic emissions into the ocean. Sci. Adv 7. doi: 10.1126/sciadv.aaz5803
Moore C. J. (2008). Synthetic polymers in the marine environment: a rapidly increasing, long-term threat. Environ. pollut 108, 131–139.
Mudroch A., Azcue J. M., Mudroch P. (1997). Manual of physico-chemical analysis of aquatic sediments (New York, NY: Lewis Publishers).
Munier B., Bendell L. I. (2018). Macro and micro plastics sorb and desorb metals and act as a point source of trace metals to coastal ecosystems. PloS One 13, e0191759. doi: 10.1371/journal.pone.0191759
Napper I. E., Thompson R. C. (2020). Plastic debris in the marine environment: history and future challenges. Global Challenges 4, 1900081. doi: 10.1002/gch2.201900081
Ojha N., Pradhan N., Singh S., Barla A., Shrivastava A., Khatua P. (2017). Evaluation of HDPE and LDPE degradation by fungus, implemented by statistical optimization. Sci. Rep. doi: 10.1038/srep39515
Oz N., Kadizade G., Yurtsever M. (2019). Investigation of heavy metal adsorption on microplastics. Appl. Ecol. Environ. Res 17, 7301–7310. doi: 10.15666/aeer/1704_73017310
Pagès P. (2008). Characterization of polymer materials using FT-IR and DSC techniques. Available online at: https://ruc.udc.es/dspace/bitstream/handle/2183/11499/CC-80%20art%208.pdf (Accessed March 11, 2019).
Parker J. G. (1983). A comparison of methods used for the measurement of organic matter in marine sediment. J. Chem. Ecology 1, 201–209.
Peng L., Fu D., Qi H., Lan C. Q., Yu H., Ge C. (2019). Micro- and nano-plastics in marine environment: source, distribution and threats — a review. Sci. Total Environment 698. doi: 10.1016/j.scitotenv.2019.134254
Peng G., Xu P., Zhu B., Bai M., Li D. (2018). Microplastics in freshwater river sediments in Shanghai, China: a case study of risk assessment in mega-cities. Environ. Pollution 234, 448–456. doi: 10.1016/j.envpol.2017.11.034
Peng G., Zhu B., Yang D., Su L., Shi H., Li D. (2017). Microplastics in sediments of the changjiang estuary, China. Environ. Pollution 225, 283–290. doi: 10.1016/j.envpol.2016.12.064
Performance Evaluation of Environmentally Degradable Plastic Packaging and Disposable Food Service Ware. Final Report June 2007. Produced under contract by: California State University Chico Research Foundation (Integrated Waste Management Board Public Affairs Office, Publications Clearinghouse (MS 6), 75. (432-2008-0001).
Porter D. E., Morris J. M., Trifari M. P., Wooller M. J., Westley P. A., Gorman K. B., et al. (2023). Acute toxicity of copper to three species of Pacific salmon fry in water with low hardness and low dissolved organic carbon. Environ. Toxicol. Chem 42, 2440–2452. doi: 10.1002/etc.5724
Rajandas H., Parimannan S., Sathasivam K., Ravichandran M., Yin L. S. (2012). Analysis method A novel FTIR–ATR spectroscopy based technique for the estimation of low-density polyethylene biodegradation. Polymer Testing 31, 1094–1099. doi: 10.1016/j.polymertesting.2012.07.015
R Core Team (2021). R: A language and environment for statistical computing (Vienna: R Foundation 771 for Statistical Computing). Available at: https://www.R-project.org/ (Accessed March 11, 2022).
Reis J. M. L., Pacheco L. J., da Costa Mattos H. S. (2013). Influence of the temperature and strain rate on the tensile behaviour of post-consumer recycled high-density polyethylene. Polymer Testing 32, 1576–1581.
Rochman C. M. (2018). Microplastics research - from sink to source in freshwater systems. Science 360, 28–29.
Rochman C. M., Hentschel B. T., Teh S. J. (2014). Long-term sorption of metals is similar among plastic types: implications for plastic debris in aquatic environments. PloS One 9, e85433. doi: 10.1371/journal.pone.0085433
Rochman C. M., Hoh E., Hentschel B. T., Kaye S. (2013). Long-term field measurement of sorption of organic contaminants to five types of plastic pellets: implications for plastic marine debris. Environ. Sci. Technology 47, 1646–1654. doi: 10.1021/es303700s
Ryan P. G. (2015). “A brief history of marine litter research,” in Marine anthropogenic litter. eds. Bergmann M., Gutow L., Klages M. (Springer), 1–25. doi: 10.1007/978-3-319-16510-3_1
Ryan P. G., Moore C. J., van Franeker J. A., Moloney C. L. (2009). Monitoring the abundance of plastic debris in the marine environment. Philos. Trans. R. Soc. B: Biol. Sci 364, 1999–2012.
Salih S. E., Hamood A. F., Abdalsalam A. H. (2013). Comparison of the characteristics of LDPE: PP and HDPE: PP polymer blends. Modern Appl. Science 7. doi: 10.5539/mas.v7n3p33
Schumacher B. A. (2002). Methods for the determination of total organic carbon (TOC) in soils and sediments (Washington, DC: nited States Environmental Protection Agency). EPA/600/R-02/069 (NTIS PB2003-100822).
Shapiro S. S., Wilk M. B. (1965). An analysis of variance test for normality (Complete samples). Biometrika 52, 591–611. doi: 10.1093/biomet/52.3-4.591
Sheik S., Chandrashekar K. R. R., Swaroop K., Somashekarappa H. M. M. (2015). Biodegradation of gamma irradiated low density polyethylene and polypropylene by endophytic fungi. Int. Biodeterioration Biodegradation 105, 21–29. doi: 10.1016/j.ibiod.2015.08.006
Silva A. L. P., Prata J. C., Walker T. R., Duarte A. C., Ouyang W., Barcelò D., et al. (2021). Increased plastic pollution due to COVID-19 pandemic: Challenges and recommendations. Chem. Eng. J 405, 126683. doi: 10.1016/j.cej.2020.126683
Simpson S. L., Batley G. E., Chariton A. A., Stauber J. L., King C. K., Chapman J. C., et al. (2005). Handbook for sediment quality assessment (Bangor, NSW: CSIRO), 126. Available at: http://www.clw.csiro.au/cecr/sedimenthandbook (Accessed March 21, 2019).
Thompson C. R., Courtene-Jones W., Boucher J., Pahl S., Raubenheimer K., Koelmans A. (2024). Twenty years of microplastics pollution research – what have we learned? Science. doi: 10.1126/science.adl2746
Turner A., Holmes L. A. (2015). Adsorption of trace metals by microplastic pellets in fresh water. Environ. Chem 12, 600–610.
UNEP (2013). Global mercury assessment 2013: Sources, emissions, releases and environmental transport (Geneva, Switzerland: UNEP: Chemicals Branch).
UNEP (2021). From Pollution to Solution: A global assessment of marine litter and plastic pollution (Nairobi, Kenya: UNEP). Available at: https://www.unep.org/resources/pollutionsolution-global-assessment-marine-litter-and-plastic-pollution (Accessed February 11, 2022).
United Nations Environment Programme, Secretariat of the Basel, Rotterdam, and Stockholm Conventions (2023). Chemicals in plastics: A technical Report (Switzerland, Geneva), 128. Available at: http://www.unep.org/resources/report/chemicals-plastics-technical-report (Accessed February 2, 2024.).
USEPA (2012). Understanding variation in partition coefficient, Kd, values (Washington, DC: United States Environmental Protection Agency). Available at: http://www.epa.gov/radiation/cleanup/402-r-99-004.html (Accessed February 11, 2022).
USEPA 3050B (1996). Acid digestion of sediments, sludges and soils (Washington, DC: United States Environmental Protection Agency).
van Cauwenberghe L., Claessens M., Vandegehuchte M. B., Janssen C. R. (2015a). Microplastics are taken up by mussels (Mytilus edulis) and lugworms (Arenicola marina) living in natural habitats. Environ. pollut 199, 10–17. doi: 10.1016/j.envpol.2015.01.008
van Cauwenberghe L., Devriese L., Galgani F., Robbens J., Janssen C. R. (2015b). Microplastics in sediments: A review of techniques, occurrence and effects. Mar. Environ. Res 111, 5–17. doi: 10.1016/j.marenvres.2015.06.007
Vrinda P. K., Amal R., Abhirami N., Mini D. A., Kumar V. J. R., Devipriya S. P. (2023). Co-exposure of microplastics and heavy metals in the marine environment and remediation techniques: a comprehensive review. Environ. Sci. pollut. Res 30, 114822–114843. doi: 10.1007/s11356-023-30679-2
Wagner M., Monclús L., Arp H. P. H., Groh K. J., Loseth M. E., Muncke J., et al. (2024). State of the science on plastic chemicals. Identifying addressing chemicals polymers concern. doi: 10.5281/zenodo.10701706
Wahyuningsih H., Bangun A. P., Muhtadi A. (2018). The relation of sediment texture to macro- and microplastic abundance in intertidal zone. IOP Conf. Series: Earth Environ. Science 122. doi: 10.1088/1755-1315/122/1/012101
Wang J., Tan Z., Peng J., Qiu Q., Li M. (2016). The behaviours of microplastics in the marine environment: Review. Mar. Environ. Res 113, 7–17.
Wang F., Wong C. S., Chen D., Lu X., Wang F., Zeng E. Y. (2018). Interaction of toxic chemicals with microplastics: A critical review. Water Res 139, 208–219.
Weis J. S., Alava J. J. (2023). (Micro)Plastics are toxic pollutants. Toxics 11, 1–12. doi: 10.3390/toxics11110935
Wentworth C. K. (1922). A scale of grade and class terms for clastic sediments. J. Geology 30, 377–392.
Wiesinger H., Wang Z., Hellweg S. (2021). Deep dive into plastic monomers, additives, and processing aids. Environ. Sci. Technology 55, 9339–9351. doi: 10.1021/acs.est.1c00976
Keywords: polyethylene terephthalate (PETE), high-density polyethylene (HDPE), intertidal sediments, organic matter, temperature, trace metal-plastic particles interaction, partitioning, FTIR spectra
Citation: Kazmiruk TN, Alava JJ, Palsson E and Bendell LI (2025) Temperature affects the sorption of trace metals by macro- and microplastics within marine intertidal sediments: insights from a long-term laboratory-based study. Front. Mar. Sci. 12:1570114. doi: 10.3389/fmars.2025.1570114
Received: 02 February 2025; Accepted: 20 March 2025;
Published: 25 April 2025.
Edited by:
Xiaoshan Zhu, Hainan University, ChinaReviewed by:
Meng Chuan Ong, University of Malaysia Terengganu, MalaysiaMohamed Hamed, Louisiana State University, United States
Copyright © 2025 Kazmiruk, Alava, Palsson and Bendell. This is an open-access article distributed under the terms of the Creative Commons Attribution License (CC BY). The use, distribution or reproduction in other forums is permitted, provided the original author(s) and the copyright owner(s) are credited and that the original publication in this journal is cited, in accordance with accepted academic practice. No use, distribution or reproduction is permitted which does not comply with these terms.
*Correspondence: Tamara N. Kazmiruk, dGthem1pcnVAc2Z1LmNh