- 1The National Key Laboratory of Water Disaster Prevention, Hohai University, Nanjing, China
- 2Key Laboratory of Coastal Zone Environmental Processes and Ecological Remediation, Yantai Institute of Coastal Zone Research, Chinese Academy of Sciences, Yantai, China
- 3Yancheng Wetland and World Natural Heritage Protection Management Center, Yancheng, China
- 4Scripps Institution of Oceanography, UC San Diego, La Jolla, CA, United States
- 5Department of Earth and Environment, Boston University, Boston, MA, United States
- 6Faculty of Science, University of Auckland, Auckland, New Zealand
- 7Jiangsu Key Laboratory of Coastal Ocean Resources Development and Environment Security, Hohai University, Nanjing, China
Introduction: Saltmarsh introduction has been widely implemented to restore ecosystem services and promote sedimentation in tidal mudflats, yet its effects on tidal network dynamics remain hard to predict. The interplay between saltmarsh extent and sediment availability in shaping long-term mudflat morphodynamics is not fully understood.
Methods: We develop a two-dimensional biomorphodynamic model to examine the individual and combined influences of saltmarsh presence and sediment availability on the evolution of tidal-flat channels.
Results and discussion: Our results demonstrate that sediment availability controls the long-term morphological change of mudflats, while the presence of saltmarshes exerts substantial short-term alterations in mudflat evolution. During the initial phase of saltmarsh introduction, vegetation promotes the development of tidal networks, characterized by channel elongation, narrowing and deepening. However, under higher sediment supply, saltmarshes restrict sediment deposition on landward and central mudflats compared to that on unvegetated flats. Furthermore, sediment availability primarily facilitates the extension of pre-existing channels, while saltmarshes play a dual role in both generating new channels and elongating existing ones. This distinction highlights the competing mechanisms driving channel network development.
1 Introduction
Tidal flat-marsh wetlands are one of the most valuable ecosystems globally, serving as a critical transition zone between land and sea. These ecosystems play a crucial role in preventing coastal erosion, supporting nearshore fisheries, and sequestering blue carbon (Murray et al., 2019; Temmink et al., 2022; Kirwan et al., 2023). Tidal flats and saltmarshes are often observed at different zones within the intertidal area, with saltmarshes typically located at the higher elevations, significantly influencing coastal landform evolution (Zhou et al., 2016). In recent years, the intentional introduction of alien saltmarsh has been shown to enhance coastal sedimentation by slowing currents, facilitating sediment deposition, and ultimately contributing to mudflat expansion (Li et al., 2009; Jin et al., 2022). However, sediment accumulation on mudflat depends not only on the presence and distribution of vegetation (Nardin and Edmonds, 2014; Brückner et al., 2019), but also on sediment availability (Mariotti, 2018; Xie et al., 2020). The development and evolution of tidal flat-marsh systems are associated with complex interactions among vegetation growth, water flow, sediment transport, and morphological changes (Coco et al., 2013; Zhou et al., 2022a). Nonetheless, the differential effects of vegetation and sediment availability on wetland morphodynamics need to be further investigated (e.g. Gourgue et al., 2024).
Tidal channel networks are a common coastal landforms, characterized by intricate branching patterns and meandering extensions (Kearney and Fagherazzi, 2016; Xu et al., 2024). These networks serve as effective pathways for water, sediment and nutrients within tidal ecosystems (Zhou et al., 2024). The ontogeny and long-term evolution of tidal channel networks are intrinsically linked to both erosional and depositional processes (D’Alpaos et al., 2005; Stefanon et al., 2010; Belliard et al., 2015). Erosional processes are driven by the concentration of sheet flow into local depressions, where local shear stress surpasses the erosion threshold, leading to the channelization (Fagherazzi and Sun, 2004; Schwarz et al., 2014). Simultaneously, deposition, driven by sediment availability and vegetation presence, influence channel morphology and development (Hood, 2010; Belliard et al., 2015; Schwarz et al., 2022). Tidal channel development typically proceeds in two stages. In the initial phase, tidal channels rapidly incise through intertidal mudflats, forming a basic channel network structure (D’Alpaos et al., 2005; Stefanon et al., 2012; Geng et al., 2020). This is followed by a long-term refinement phase, where the system gradually evolves to morphological equilibrium (Xu et al., 2019; Zhou et al., 2014, 2017).
In recent decades, significant progress has been achieved in understanding the impacts of vegetation on morphodynamic processes, particularly through bio-morphodynamic feedback that consider the nonlinear interactions between biotic and abiotic processes (Möller et al., 2014; Chen et al., 2016; Xu et al., 2022). Previous studies have largely focused on vegetation’s role in promoting vertical accretion and influencing the development of tidal channel systems (Belliard et al., 2015; Geng et al., 2021; Van De Vijsel et al., 2023). Vegetation, through its dense and stiff above-ground shoots, can reduce tidal currents, trap sediment particles, and facilitate local sedimentation (Li and Yang, 2009; Mudd et al., 2010; Chen et al., 2018). Conversely, vegetation also accelerates flow in adjacent unvegetated area, initiating and deepening tidal channels, as the so-called “vegetation-induced channel erosion” (Temmerman et al., 2007; Schwarz et al., 2014). This process leads to a denser network of channels with more efficient drainage systems compared to landscapes without vegetation (Vandenbruwaene et al., 2013; Hautekiet et al., 2024). Moreover, vegetation is also considered as a sustaining factor for stabilizing the banks of existing tidal channels, a process termed “vegetation-stabilized channel inheritance” (Schwarz et al., 2014). The balance between these opposing processes, vegetation-stabilized channel inheritance vs. vegetation-induced channel erosion, depends on the environmental conditions such as sediment availability and vegetation cover (Schwarz et al., 2016). Therefore, understanding how tidal channel dynamics respond to variations in vegetation cover and sediment supply is critical for evaluating the evolution of tidal flats and saltmarshes.
Sediment availability is critical to influence the evolution and development of tidal flat-marsh wetlands, influencing mudflat progradation, degradation and channel dynamics (Maan et al., 2019). Decreasing sediment availability, in combination with SLR and human activities, has a profound impact on morphodynamic change of mudflats, often leading to wetland loss (Ladd et al., 2019; Liu et al., 2021). Higher suspended sediment concentration (SSC) enhances sedimentation rates, promotes lateral expansion of mudflats, and drives the development of channel networks, whereas lower SSC can suppress morphological changes (Xie et al., 2022). However, SSC varies considerably across coastal systems due to local environment conditions (Grandjean et al., 2024; Hou et al., 2024). While most studies rely on site-specific field observation and SSC-specific numerical models (Xie et al., 2018; Gourgue et al., 2024), the long-term impacts of sediment availability on tidal channel network evolution remains poorly understood.
Vegetation introduction, whether through restoration effects or the invasion of alien species, has been observed to significantly influence local ecosystem and influence tidal channel morphodynamics (Schwarz et al., 2018; Xu et al., 2023). For example, Spartina alterniflora (hereafter, S. alterniflora), native to the Atlantic Coast of America, was introduced to China in 1979 to serve as a natural buffer and mitigate erosion. The introduction of S. alterniflora has transformed mudflat ecosystems into saltmarsh dominated landscapes, significantly influencing tidal channel evolution (An et al., 2007). Despite the known impacts of vegetation and sediment availability on wetland morphodynamics, their relative influence on tidal network development remains unclear. To address this knowledge gap, we developed a two-dimensional (2D) bio-morphodynamic model that couples the evolution of tidal channel systems with the dynamics of saltmarsh vegetation. This study aims to (1) analyze the effects of saltmarsh and sediment supply on the formation and evolution of tidal channel networks; (2) explore the long-term impacts of vegetation introduction on tidal channel inheritance; (3) examine the trade-offs between sediment availability and vegetation presence in shaping mudflat morphodynamics.
2 Methods
A 2D bio-morphodynamic model is used to capture the interaction between channel network evolution and vegetation dynamics. This model integrates a hydro-morphodynamic module (Delft3D) to simulate tidal currents, sediment transport and bed evolution, with a dynamic vegetation model implemented in MATLAB (Xie et al., 2022). The two models are seasonally coupled, allowing for a two-way interaction between the physical environment and biological processes. Delft3D calculates local hydroperiods, which are fed into the dynamic vegetation model to determine the establishment, growth and mortality of saltmarshes. The vegetation model, in turn, provides the biotic parameters such as vegetation density, stem diameters and height back to Delft3D to address the effect of vegetation on tidal flow, sediment transport and consequently morphological change. This coupling framework has been successfully applied in previous coastal wetland studies (Xie et al., 2020; Wei et al., 2024).
2.1 Hydro-morphodynamic model
Delft3D computes tidal flow, sediment transport and associated bed level changes, as detailed in previous studies (Lesser et al., 2004). Water level and flow velocity are subsequently utilized to compute suspended sediment transport according to the advection-diffusion equation, resulting in bed level changes calculated by the mass balance equation and then incorporated into the hydrodynamic calculations for the next hydrodynamic time step. Delft3D has been effectively employed to simulate the morphodynamic evolution of tidal channel networks in coastal mudflat environments (Nardin and Edmonds, 2014; Xu et al., 2017; Hanegan et al., 2023). To account for the vegetation effects on water movement and sediment transport, the trachytope approach in Delft3D using the Baptist formula was applied (Baptist et al., 2007). The Baptist formula is based on the concept that vegetation can be modelled as rigid cylinders characterized by vegetation height , density m, stems diameter D and corrected drag coefficient . In this approach, the bed roughness and flow resistance term in both and directions (i.e., ) are incorporated into the momentum equations to represent the effect of vegetation on tidal currents. Both and are calculated based on vegetation characteristics and water depth by Equations 1 and 2:
where is the unvegetated Chézy coefficient, set to 65 ; g = 9.81 m/s2 is the gravity acceleration; = 0.41 is the Von Kármán constant; hv (m) is the vegetation height; CD is the drag coefficient accounting for stems of vegetation. Here we set CD of saltmarsh stems to 1 following previous study (Schwarz et al., 2014). n = mD where m is the number of saltmarsh stems per square meter and D is the diameter of the saltmarsh stems.
2.2 Dynamic vegetation model
The saltmarsh model simulates the processes of establishment, growth, expansion and mortality following the methodology of Schwarz et al. (2014). Net vegetation biomass change is described by Equation 3:
where dP is the derivative of the total stem density of the saltmarsh with respect to the time (stems/m2), with components representing establishment (), growth (), diffusion ( and ), and flow-induced mortality ().
Following previous studies, the establishment (Marani et al., 2013; Wei et al., 2024), growth, and mortality of vegetation are related to local inundation frequency by Equation 4:
Where IR is relative inundation ratio, and a, b and c represents empirical coefficients of the equation, which are set to -28.679, 11.810 and -0.217, respectively, accounting for the field observation of S. alterniflora in the Jiangsu coast, China (Li et al., 2018).
Seeding establishment of saltmarsh plants is modelled as a random process by Equation 5:
where Seed is the probability that a bare cell gets colonized, which is set to 0.01 (Temmerman et al., 2007). P0 is the initial stem density upon establishment (stems/m2), related to the maximum density K1 (Schwarz et al., 2014). P0 = 0.1* K, where K and P0 are set to 600 and 60 based on the field data in Jiangsu coast, China (Li et al., 2018). dt is the time step.
Saltmarsh growth is calculated by Equation 6 (Schwarz et al., 2014).
Where r is intrinsic growth of stem density, which is set to 1 (Schwarz et al., 2014).
The diffusion of saltmarsh is described by Equation 7 and 8 (Schwarz et al., 2014):
Where: D is the plant diffusion coefficient, set to 0.2 (Temmerman et al., 2007). , , , are the stem density in neighboring cells (stems/m2).
Flow-induced mortality is described by Equation 9 (Schwarz et al., 2014):
where is the saltmarsh mortality coefficient related to the bed shear stress (; N/m2), set to 3000, (stems m-2/(N/m2)) (Schwarz et al., 2014). is critical bed shear stress for saltmarsh uproot, set to 0.26 N/m2 (Schwarz et al., 2014).
2.3 Model set up
This study focuses on the evolution of tidal flats in China before and after the introduction of Spartina alterniflora. We create an idealized open coast model domain to represent typical mudflat conditions on the Jiangsu coast, where Spartina was introduced in 1979. The domain is a 6 km by 1.5 km rectangle (Figure 1a). The grid resolution is set to 10 m by 10 m to allow for a relatively refined simulation of tidal channel networks and saltmarsh dynamics (Schwarz et al., 2014; Xie et al., 2023). The mean tidal range is around 3 m, and following a previous study (Xie et al., 2023), the model geometry is composed of an upper gentle-slope (1/2000) region and a lower sharp-slope (50/2000) region (Figure 1b). The initial bed elevation ranges from 2 m at the land boundary, extending 4km offshore. The seaward boundary is set to -50 m to avoid any effect on tidal flat morphodynamics resulting from shallowing at such boundary (Xie et al., 2023). We do not include the effect of wind waves. Initial perturbations are randomly generated on the upper flat to accelerate the morphodynamic evolution (Xu et al., 2017). The initial random perturbations are smoothed with Gaussian distribution and are designed with a root mean square height of 0.1 m.
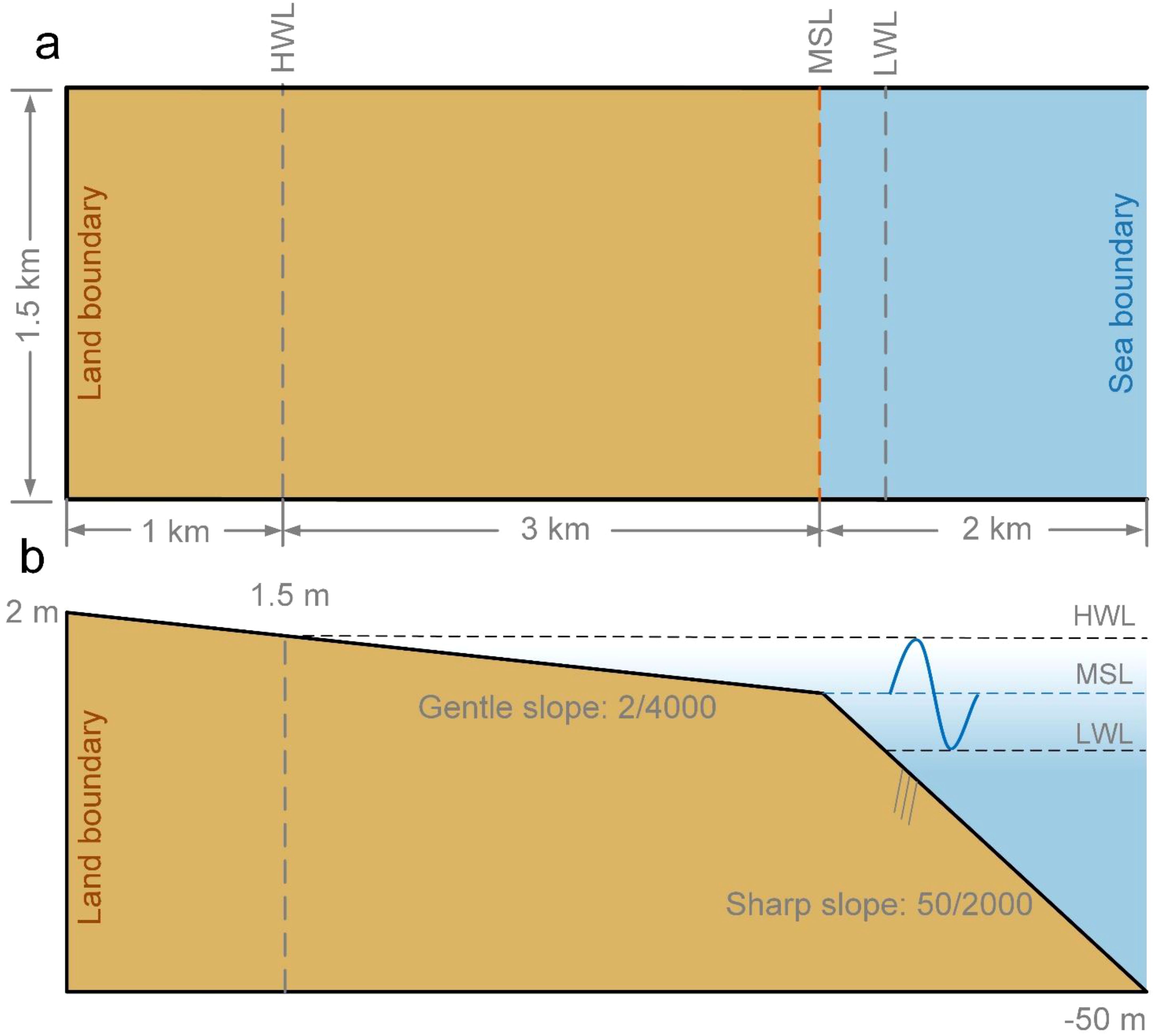
Figure 1. Model layout with initial bathymetry and boundaries. (a) Plan view of model domain; (b) cross-section view along the domain. The characteristic water levels, such as high-water level (HWL), mean sea level (MSL), and low-water level (LWL), are indicated in both model plan view and cross-section view.
The model is characterized by three closed and impermeable boundaries landward and an open water seaward boundary. A semi-diurnal (S2) tidal cycle is applied at the seaward boundary with a 12-hr period and a 3-m tidal range to reproduce the mesotidal characteristics of the Jiangsu coast, China. We only consider mud in this research according to the sediment conditions in the Jiangsu coast (Chen et al., 2024). The critical bed shear stress for mud erosion is set to 0.2 N/m2 (Kuai et al., 2023), and a morphological acceleration factor of 30 is applied to efficiently simulate long-term evolution of tidal flats and saltmarshes (Xie et al., 2022).
We investigate the morphological evolution through a series of simulations with different combinations of sediment supply and vegetation presence. Four constant suspended sediment concentration conditions are imposed at the seaward open boundary: Csed =0, 5, 10, 15 mg/L. Sediment with the prescribed concentration is transported with the inflow current, while during the outflow period, the concentration at the open boundary is determined by the computed values on advection-diffusion equation. It is important to note that this value was chosen to explore the impact of different sediment supply on tidal channel network evolution and does not represent a definitive threshold. To explore the role of saltmarsh in tidal channel network evolution, vegetation is introduced in year 30, after the tidal channel networks have stabilized. Notably, before vegetation was introduced, the models had already undergone 30 years of geomorphic evolution under varying sediment supply conditions.
2.4 Model analysis
In every simulation, channel areas are extracted by comparing the relative elevations of adjacent regions, using the method described by Geng et al. (2018). This method has consistently demonstrated its effectiveness in mapping the creek network structures within intertidal zones. The channel network morphologies from various simulations are compared based on channel dimensions (including mean bed elevation, channel length, channel width, and channel depth) and channel properties such as drainage density, mean unchanneled path length (mUpl) (Schwarz et al., 2022). Total channel length is measured including all channel skeletons. The mean channel width is calculated by dividing the mean tidal channel area by the mean channel length, while mean channel depth reflects the average depth within the channel networks. The drainage density is defined as the ratio between the total channel network length and its watershed area. The mean unchanneled path length (mUpl) represents the average distance a drop of water placed on the vegetated flat would need to travel to reach the nearest channel, providing an indication of the efficiency of tidal channels in draining a watershed (Marani et al., 2003; Schwarz et al., 2022). In simulations with vegetation, to ensure consistency and comparability of the statistical range across different cases, we selected the area with the smallest vegetation coverage for comparison, spanning from x=1 km to 3.5 km and from y=0 km to 1.5 km. This approach ensured a consistent comparison of model results across different scenarios.
3 Results
3.1 Mudflat morphodynamics under varying SSC and vegetation conditions
Through different simulations, the basic structure of tidal channel network, which evolves from the same topography, occurs at the same location where the initial topographic depression exists in the context of different sediment supply (Figures 2a, d, g, j). The initial perturbation facilitates the formation of tidal channels, which takes place within the first few years of the simulation. At the initial stages of tidal channel network development, erosional processes among bed perturbation patches gradually intensify, leading to the formation of curved and branching channels that divert and split tidal flows. Subsequently, multiple tidal channels emerge at the sea boundary and extend landward through headward erosion. This ongoing branching and elongation process contributes to the formation of a dendritic channel network (e.g., Figure 2a).
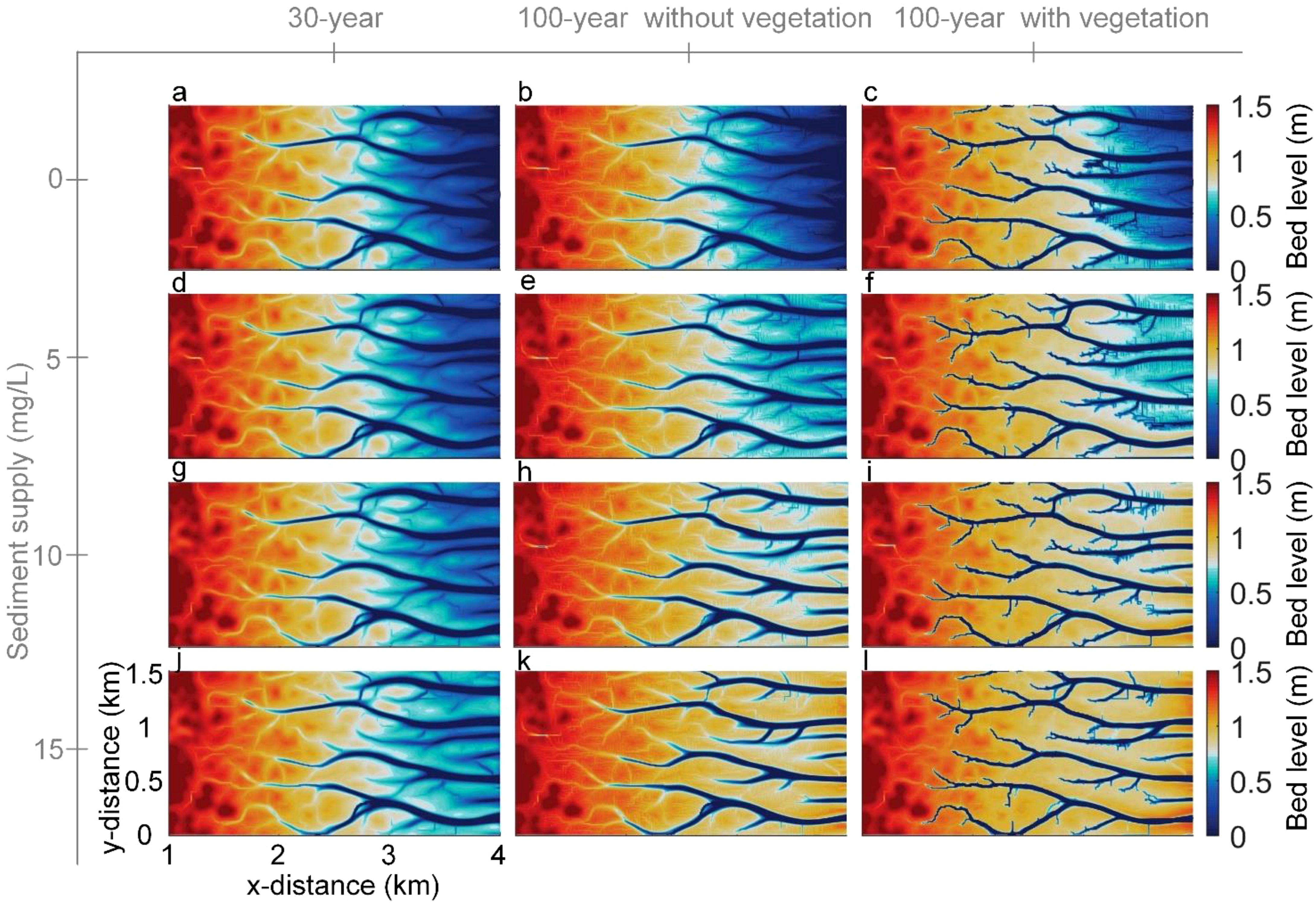
Figure 2. Simulated morphologies under different conditions of sediment supply and vegetation presence. The columns illustrate three scenarios: the 30-year topography in the absence of vegetation (a, d, g, j), the 100-year topography without vegetation (b, e, h, k), the 100-year topography with vegetation (c, f, i, l). The rows show the effects of progressively increasing sediment supply.
Overall, while the tidal channel networks exhibit similar patterns across different simulations, vegetation and sediment supply play a significant role in influencing the morphodynamic evolution of the mudflat. Our results show that increasing SSC promotes mudflat accretion and the development of channel, but does not significantly alter the geometric properties of the tidal networks (Figure 2). Under low SSC conditions, the limited sediment availability results in lower elevations of the tidal channel banks and blurred boundaries of the channels (Figures 2b, e). As SSC increases, more sediment deposits in the lower parts of the tidal flats, where sediment accumulation elevates channel banks and sharpens channel boundary (Figures 2h, k). The presence of vegetation further modifies these dynamics, as it not only promotes mudflat accretion but also significantly alters the geometric properties of the tidal channel networks. Vegetation enhances sediment deposition on the tidal flat surface, leading to higher tidal flats and narrower tidal creeks (Figures 2c, f, i, l). At the same time, vegetation promotes erosion within the landward creeks, which facilitates the upstream extension of the tidal channels (Figures 2c, f, i, l). More branching and tributary channels are observed in the presence of vegetation compared to conditions without vegetation (Figures 2c, f, i, l vs 2b, e, h, k).
3.2 Saltmarsh development across SSC gradients
Initial vegetation distribution in all scenarios is determined by the random establishment of saltmarshes (Equation 5), and is therefore consistent across SSC variations. However, subsequent saltmarsh development is significantly enhanced as SSC increased (Figures 3b, e, h, k). During the early stages of vegetation introduction, around years 30-35, saltmarsh establishment is largely controlled by initial mudflat elevation. Vegetation initially grows rapidly in patches, particularly in the middle and landward areas of the mudflat (Figures 3a, d, g, j). In scenarios with high SSC, enhanced sedimentation allows vegetation patches to expand further seaward compared to lower SSC conditions (e.g., Figures 3g vs. 2j).
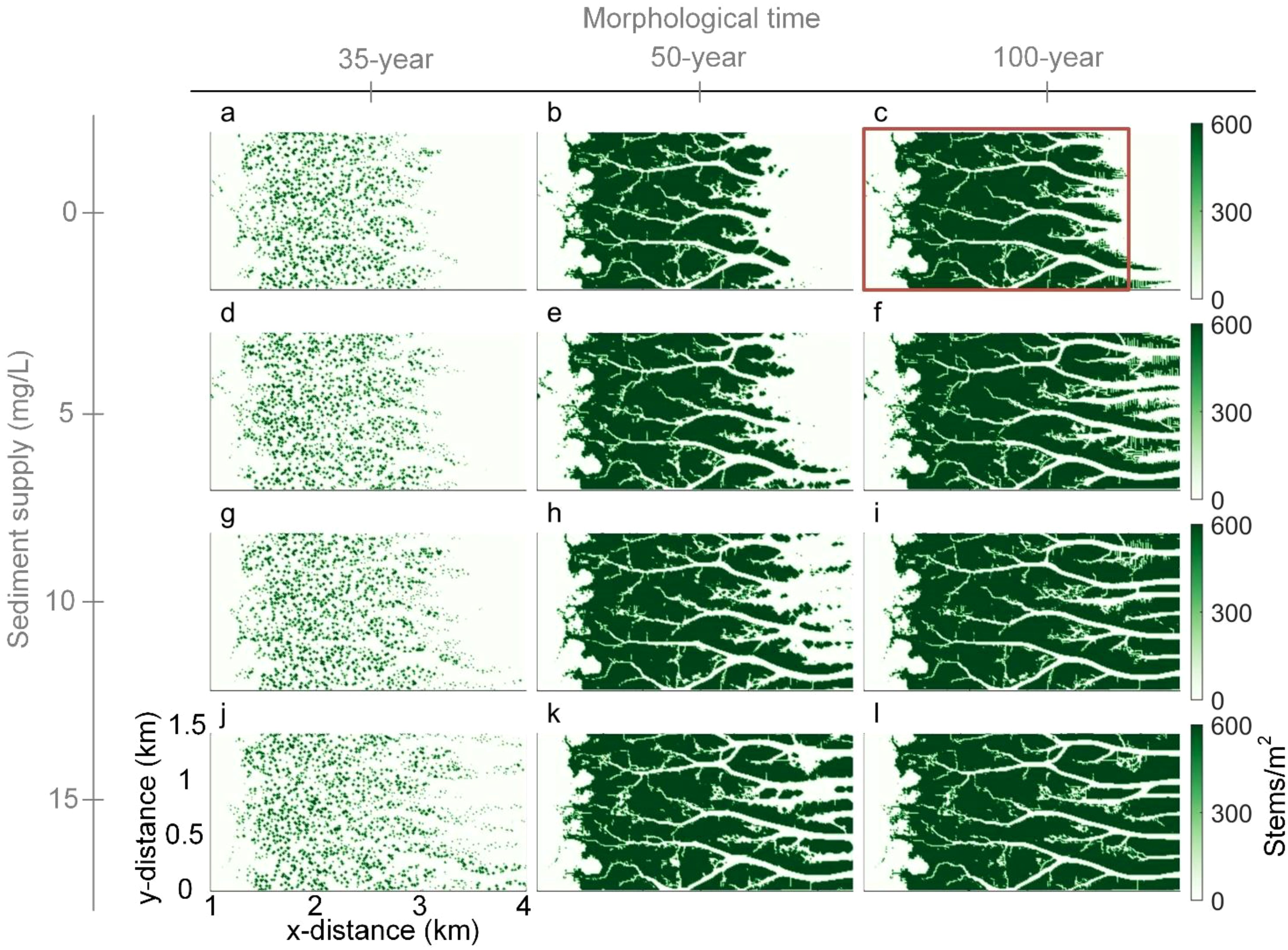
Figure 3. Spatio-temporal distribution of saltmarsh density (stems/m²) under varying sediment supply conditions. The time display on top of the plots shows the total morphological simulation period. Since the saltmarsh is introduced in the 30th morphological year, 35 (a, d, g, j), 50 (b, e, h, k) and 100-year (c, f, i, l) morphological time refer to vegetation encroachment for 5, 20, and 70 years.
As the saltmarshes matures, the expansion rates vary with SSC (Figures 3c, f, i, l). For low SSC conditions, vegetation colonizes only 2-km of tidal flats due to the limited progradation (Figure 2c and Figure 3c). With a moderate increase in SSC, such as 5 mg/L, vegetation spreads more broadly, facilitating both landward and seaward extension of tidal channels (Figure 3f). At higher SSC, both the saltmarshes and channel networks are fully developed, displaying similar patterns across scenarios (Figures 3i, l).
3.3 Spatio-temporal variation in tidal flat and tidal channel elevation
To investigate the evolution and development of tidal networks, we analyzed the temporal and spatial change in mean bed elevation for tidal flats and tidal channels (Figure 4). Overall, saltmarsh introduction leads to higher tidal flats and deeper channels.
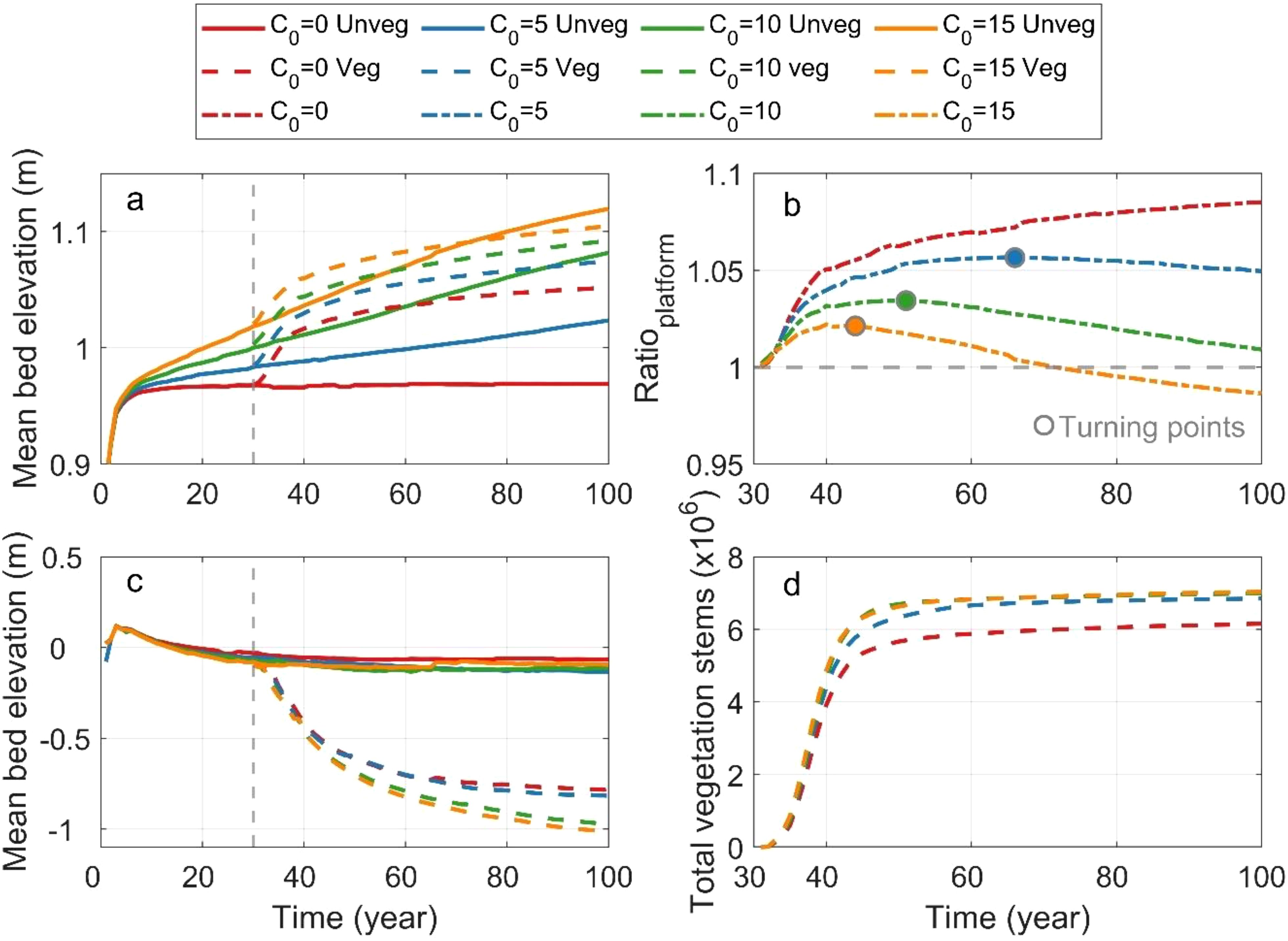
Figure 4. Temporal variations in mean bed elevation in tidal flats (a) and tidal channels (c). (b) Ratio of mean bed elevation with vegetation to mean bed elevation without vegetation. (d) Temporal change of total vegetation stems. The regions used for comparison are evaluated over the red rectangular box in Figure 2c (from x=1 to 3.5 km).
In the absence of vegetation, tidal flat elevation increases with sediment supply, and the accumulation rate accelerates as SSC increases (Figure 4a). In contrast, during the initial stages of vegetation establishment (i.e., the first 5 years), the presence of vegetation can significantly elevate tidal flats. As the vegetation coverage stabilizes and profile reaches equilibrium, the sediment accumulation rate gradually decreases (see dashed lines in Figure 4a). In the scenario without sediment supply, vegetation also promotes the sedimentation in the tidal flat. It is because the eroded sediment from the channels was trapped by vegetation, leading to the deposition in the tidal flat (see the red dashed lines in Figure 4a). Furthermore, with increasing sediment supply, the difference between the average elevations with and without vegetation diminishes over time (see orange, green and blue lines in Figure 4a). Surprisingly, in the scenario with the highest sediment supply (15 mg/L), tidal flat elevation without vegetation eventually exceeds that with vegetation (see orange lines in Figure 4a). This is probably because dense seaward saltmarsh reduces sediment transport into interior mudflats (Xu et al., 2022), while the absence of saltmarsh allows more sediment to be transported further inland.
To further explore the role of saltmarsh on mudflat accretion under different SSC conditions, we calculated the ratio of mean bed elevation with vegetated scenarios to those without vegetation (Figure 4b). Our results show that vegetation provides a stronger contribution on mudflat accretion when SSC is lower (see red lines in Figure 4b). During the initial stages of vegetation establishment, this effect is pronounced but decreases over time as the bed level increases. Furthermore, higher SSC causes this ratio to decline more rapidly (see the turning points in Figure 4b). At the highest SSC condition (i.e., 15 mg/L), the ratio drops below 1 after year 70, indicating that vegetation no longer stimulates sediment accretion. Instead, it starts to constrain sediment accretion in the upper and middle wetland during the later stages of vegetation development.
Different from tidal flats, the mean bed elevation in tidal channels decreases significantly in vegetated scenarios compared to those without saltmarsh, indicating that vegetation enhances tidal channel erosion (Figure 4c). Without vegetation, the mean bed elevation of channels remains consistent regardless of SSC (see solid lines in Figure 4c). However, under the presence of vegetation, channels deepen as SSC increases, demonstrating that higher sediment supply amplifies vegetation-induced erosion along channelized area. In addition, the temporal change of the total vegetation stems showed a positive relationship with sediment supply (Figure 4d). Although higher SSC leads to a higher bed level (yellow dashed line vs. green dashed line in Figure 4a), the number of vegetation stems is similar (yellow dashed line vs. green dashed line in Figure 4d), highlighting that similar vegetation status does not necessarily depend on identical bed level conditions.
Longitudinal variations in bed elevation across tidal flats and channels further illustrates how SSC and vegetation influence tidal flat and tidal channel evolution (Figure 5). During the early stage of vegetation establishment (35 years of morphological time), mean flat elevation increases with SSC, while channel bed elevation remains relatively stable (Figures 5a, b). At this stage, vegetation plays a limited role in the flat sediment accretion and channel deepening. Fifteen years after vegetation introduction, the bed level differences with and without vegetation become more pronounced on both the tidal flats and channels, particularly under low SSC (Figures 5c, d). The largest bed level differences are observed on the seaward side of the tidal flat and in the middle of the tidal channels (Figures 5d, f). Additionally, higher SSC results in a gentler flat slope, allowing the seaward flat to reach elevations similar to those observed inland (orange lines in Figure 5c). Seventy years after saltmarsh introduction, bed level differences between vegetated and unvegetated scenarios continue to increase throughout the system. Under higher sediment supply, the seaward portion of tidal flats are further elevated, surpassing bed levels in nearby landward area (orange, green and blue lines in Figure 5e).
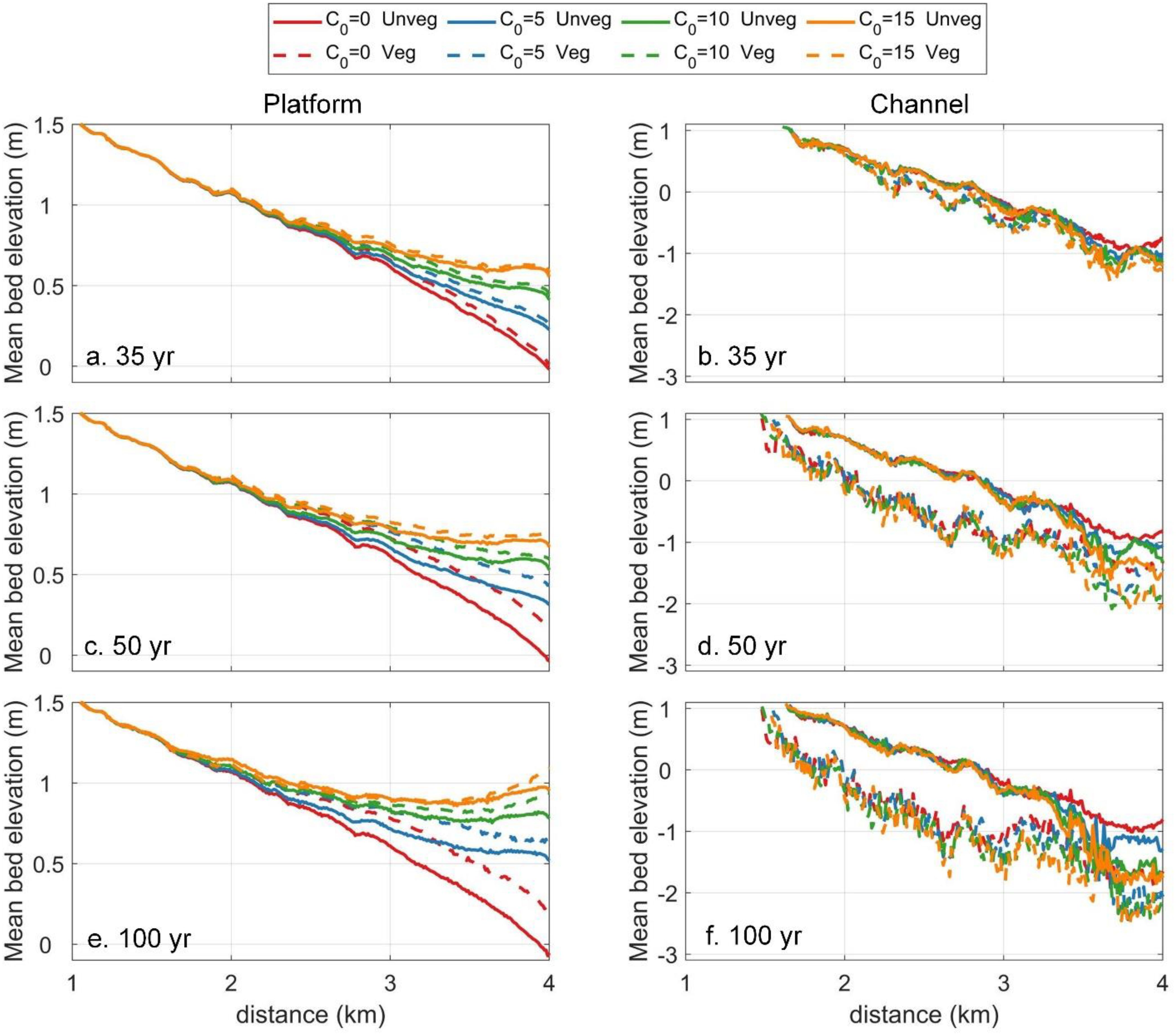
Figure 5. Longitudinal elevation across tidal flats (a, c, e) and tidal channels (b, d, f) at different times: 35 years (a, b); 50 years (c, d); 100 years (e, f). The distributions of saltmarsh at each time step correspond to that in Figure 2.
3.4 Temporal variations of tidal channel dimensions
To investigate the effects of sediment supply and vegetation on the development of tidal channel networks, we compared the temporal variations of key tidal channel parameters, including channel length, width and depth (Figure 6). We also calculated the ratio of these dimensions between vegetated and unvegetated scenarios under different SSC conditions to assess the relative contribution of vegetation and sediment supply on channel development.
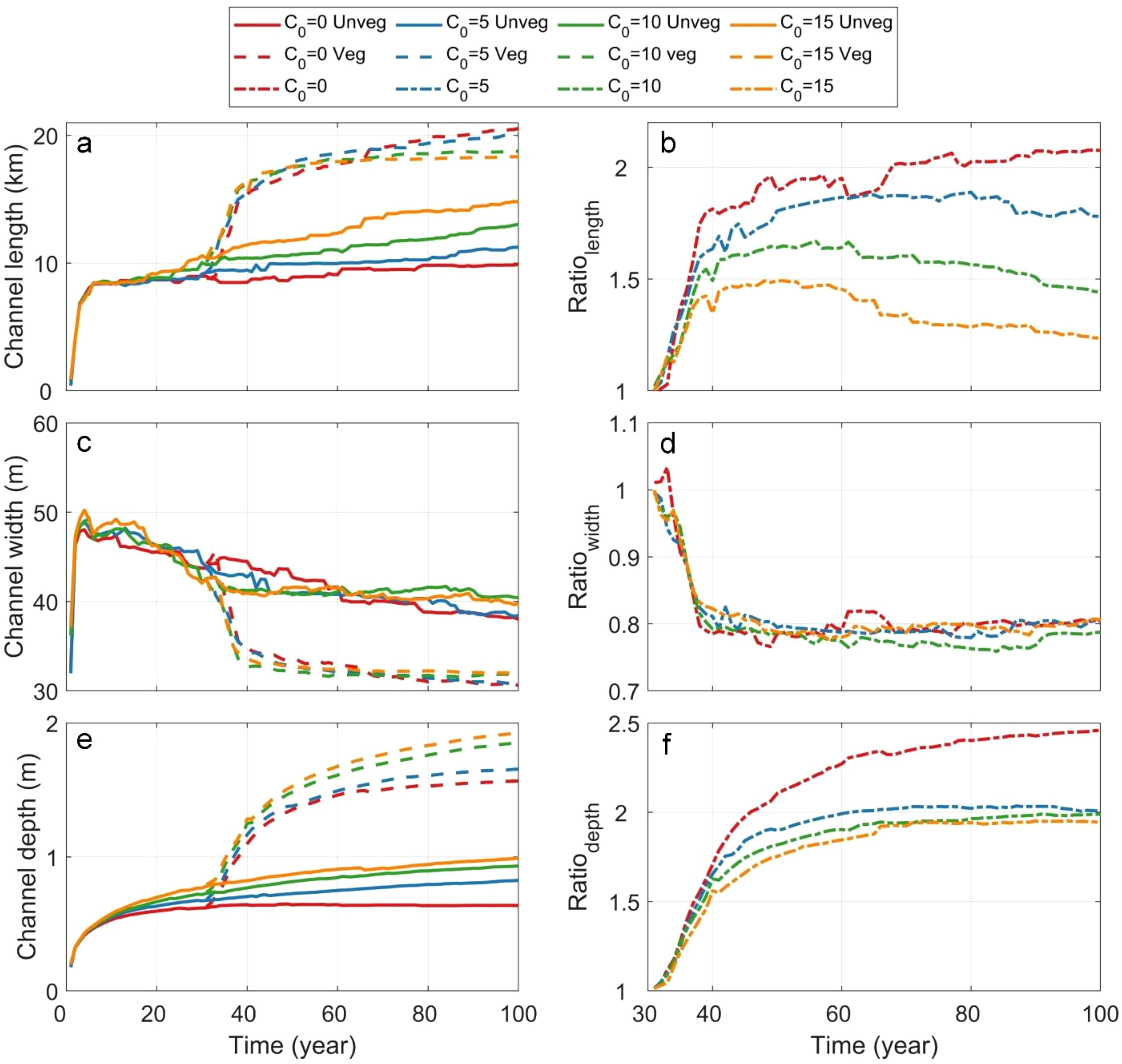
Figure 6. Temporal variations of (a) mean channel length, (c) mean channel width and (e) mean channel depth under different sediment supply scenarios and in the presence of vegetation. Temporal variation of the ratio of (b) channel length, (d) channel width and (f) channel depth between vegetated and unvegetated conditions.
In the absence of vegetation, channel length increases rapidly at the beginning of the evolution, followed by a gradual decrease in elongation rates (Figure 6a). Tidal channels with higher sediment supply maintain a higher elongation rate (orange solid lines in Figure 6a). The introduction of vegetation further enhanced the channel lengthening, particularly during the early stages of development (Dashed lines in Figure 6a and Figure 6b). The temporal variation of the length ratio indicates that sediment supply influences channel lengthening at various stages (Figure 6b). Initially, the ratio steadily increases before reaching a peak value (Figure 6b). The time to reach the peak decreases with increasing sediment supply (orange line vs. red line in Figure 6b). In high SSC scenarios, the ratio gradually decreases over time after reaching its peak, suggesting that the role of vegetation in extending channels diminishes at later stages (e.g., orange line in Figure 6b).
Channel width initially increases rapidly in unvegetated scenarios but subsequently decreases over time (solid lines in Figure 6c). However, the saltmarsh introduction leads to an evident and sustained decrease in channel width, which can also be observed in the width ratio depicted in Figure 6d. In both vegetated and unvegetated scenarios, channel width remains consistent across different SSC levels, indicating that sediment supply has a limited impact on channel width while vegetation plays a more significant role in channel narrowing (Figure 6d).
Mean channel depth increases significantly in the presence of vegetation, demonstrating that vegetation enhanced channel deepening (Figure 6e). The temporal variation in the ratio of tidal channel depth shows significant differences depending on sediment availability, while these differences become minor once sediment supply exceeds 5 mg/L (Figure 6f). This suggests that the role of vegetation in enhancing channel deepening is more pronounced under lower SSC conditions but stabilizes as sediment availability increases (Figure 6f).
4 Discussion
4.1 Development of tidal channel networks
The geometry of tidal channel networks is essential for the exchange of flow, nutrients, sediment and biota (Xu et al., 2024). Drainage density and mUpl are widely-used measures of channel network properties. Although the patterns of the tidal channel system in the study are similar, our model results show that sediment supply and vegetation interact in distinct ways to influence channel network development and complexity, which is meaningful for the modelling and management of coastal environments in the context of climate change and human interventions.
4.1.1 Impact of sediment availability on tidal flats and tidal channel morphodynamics
When vegetation is absent, as the sediment supply grows, the drainage density increased and mUpl decreased, generating more complex channel systems (Figures 7a, b). This finding is consistent with previous studies that demonstrated a positive relationship between sediment availability and channel network maturity (Belliard et al., 2015). The observed increase in channel network complexity is mainly ascribed to the high degree of channel lengthening as the sediment supply increases, rather than the formation of additional low-order channel branches (Figures 2b, e, h, k).
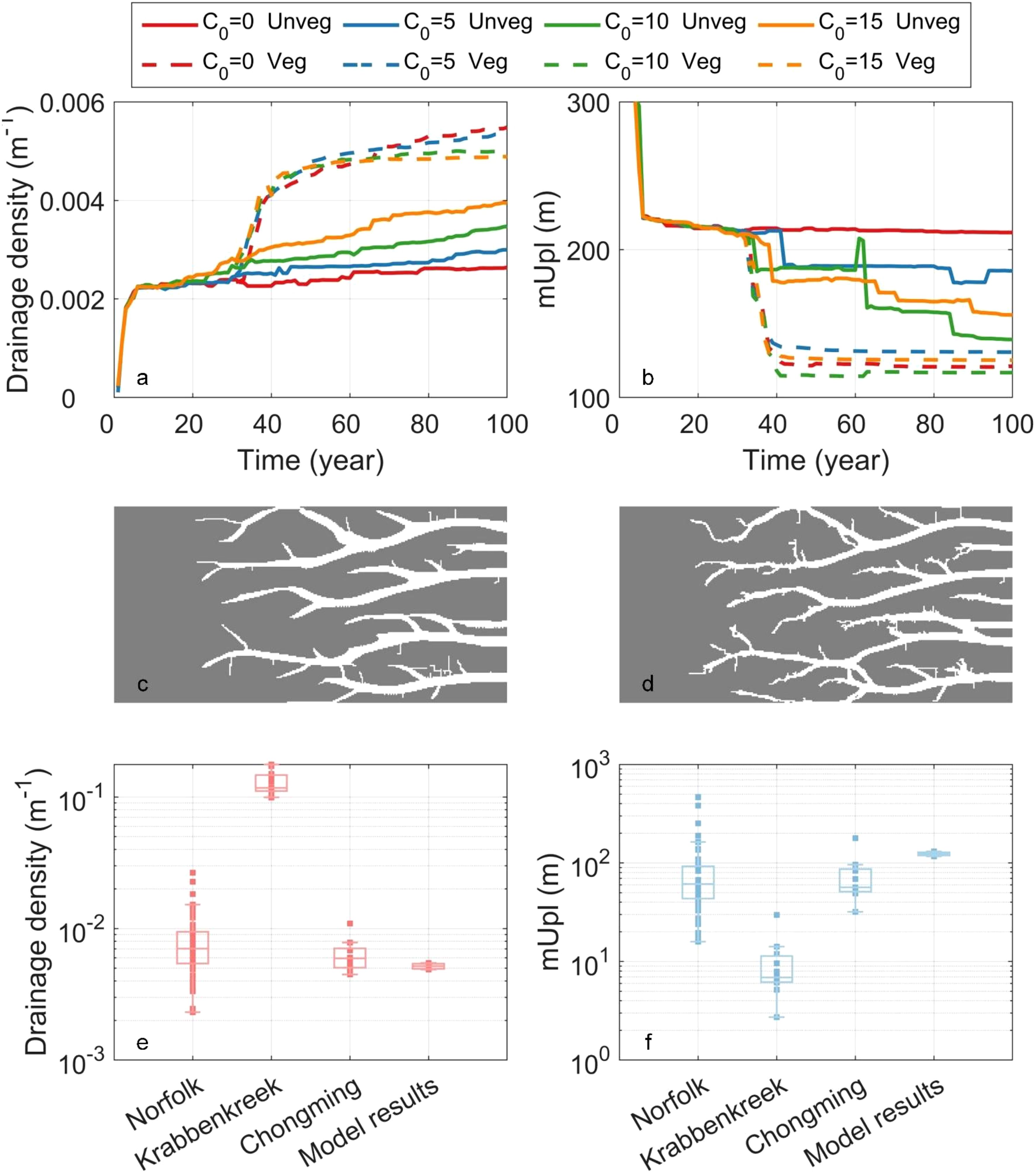
Figure 7. Temporal change of drainage density (a) and unchanneled flow path length (mUpl) (b). (c, d) are the binary results of simulated tidal channels in the absence (c) and presence of vegetation (d) under the highest sediment supply (15 mg/L). (e, f) are the drainage density comparison between model results and real-world networks.
Sediment supply alters the quantity of sediment deposition without changing the pattern and distribution of sedimentation. The increased sediment deposition on the flat limits tidal flow over it, causing the concentration of tidal flow in tidal channels that extend. The increasing growth rates of drainage density (four solid lines in Figure 7a) for higher sediment supply suggests faster elongation and extension of tidal channels. Our results suggest that the role of sediment supply in the development and evolution of tidal channel networks is to promote a long-term, stable, and gradual extension of existing channels, rather than the formation of new ones (Figures 2b, e, h, k).
4.1.2 Impact of vegetation on tidal flats and tidal channel morphodynamics
The impact of saltmarsh introduction further complicates tidal network development. As reported by many other studies (Kearney and Fagherazzi, 2016; Schwarz et al., 2022; Geng et al., 2023), vegetation introduction led to an increase in drainage density and a reduction in the mean unchanneled flow path length (dashed lines in Figures 7a, b). Comparing with the increase of drainage density driven by sediment supply, the density increase under vegetation impacts is more pronounced than that driven by sediment supply. The temporal evolution of drainage density in vegetated scenarios can be divided into two distinct phases, aligned with vegetation development: 1) initial rapid increase and 2) subsequent gradual stabilization.
In the early stages of vegetation development, vegetation rapidly encroaches the tidal flat primarily in the form of patches (Figures 3a, d, g, j), where tidal channels emerge with the “vegetation-induced channel initiation” (Temmerman et al., 2007; Schwarz et al., 2014). Subsequently, once vegetation expanded across the entire flat, its primary role was to stabilize the tidal flats, which can be interpreted as “vegetation-stabilized channel inheritance” (Schwarz et al., 2014). In this phase, the accretion caused by the vegetation facilitates the tidal channels elongation and extension, similarly to the increase in sediment supply.
More small branching channels are observed in the presence of vegetation (Figure 7d), compared to the unvegetated case (Figure 7c). Therefore, vegetation enhances channel network complexity both in terms of elongation and branching. Although the branching expansion occurs over a short period, it plays a significant role in increasing drainage density compared to the later elaboration stage. In addition, the difference in drainage density under different sediment supply scenarios in the presence of vegetation is smaller than that in the absence of vegetation, which indicates that vegetation restricts the role of sediment supply in channel development (Figure 7a).
4.1.3 Trade-offs between vegetation presence and sediment availability
Numerous studies have highlighted the role of vegetation in promoting tidal flat accretion (Li and Yang, 2009; Ma et al., 2014; Chen et al., 2018; Jin et al., 2022). In general, high SSC is positively correlated with accretion processes, which highlights the necessity of sufficient sediment availability to sustain tidal flat morphology and vegetation restoration (Grandjean et al., 2024; Liu et al., 2025). Therefore, the introduction of vegetation is often considered an effective measure to facilitate sedimentation (An et al., 2007; Zhang et al., 2017).
However, our study reveals that the effect of vegetation on tidal flats accretion is highly related to sediment availability (Figure 4a, b). More specifically, under low SSC conditions, vegetation promotes sedimentation for a prolonged duration. As SSC increases, however, the duration of this effect becomes more limited. To further verify this trend, we extended our model simulations to an SSC of 20 mg/L, and the results remained consistent (Supplementary Figure 1). This is likely because high SSC promotes vegetation expansion, which in turn enhances tidal flat accretion. However, the rapid expansion of vegetation also leads to sediment transport limitation to inland tidal flat areas. This bio-morphodynamic feedback intensifies with increasing sediment availability, leading to an earlier restriction of sediment delivery to the upper tidal flat.
This finding aligns with previous studies that suggest vegetation can limit sediment transport under high SSC conditions (Gourgue et al., 2024; Wang et al., 2025). More importantly, it highlights the need for a comprehensive perspective when considering vegetation introduction as a strategy to promote accretion. While vegetation may enhance accretion at a local scale, it can also limit the accretion in the upper and middle tidal flat regions at the landscape scale, potentially altering the overall sediment dynamics of the system.
4.1.4 Model validation and comparison with field observations
To investigate whether the model yields realistic results, we compared modelled and real-world drainage densities in an mesotidal environment (data from Schwarz et al. (2022)), and found same order of magnitude consistency (Figures 7e, f). The drainage density at Norfolk and Chongming are around 0.00703 and 0.0059 m-1 (median value), which is consistent with our model results, around 0.005 m-1 (median value). The drainage density at Krabbenkreek is an order of magnitude higher than other field sites, this is likely due to sediment properties and vegetation traits. The mUpl at three sites is consistent to that of our model results.
In addition, we compared the simulated vegetation growth process with field observational data and found that our simulated saltmarsh development is in agreement with the observed patterns (Temmerman et al., 2007; Schwarz et al., 2014). In the initial stage, vegetation primarily colonizes the tidal flat in a patchy pattern (Figures 3a, d, g, j). During this phase, field data indicates that tidal channels are more likely to form in a patchy pattern due to flow concentration and channel erosion, leading to the formation of branching and tributary channels, which is also observed in our simulation results (Figure 7a). Subsequently, as vegetation gradually grows and expands, the channel morphology stabilizes due to vegetation-stabilized channel inheritance.
At both the landscape scale (from seaward to landward zones) and the local scale (from channel fringes to marsh interiors), the competitive effect of dense vegetation on sediment trapping contributes to the formation of levees (Nardin and Edmonds, 2014; Boechat Albernaz et al., 2020; Xu et al., 2022; Wang et al., 2025). Under high sediment supply scenarios, suspended sediment concentration decreases landward as the flat progressively attains higher elevations seaward (orange and green dashed lines in Figure 5e), leading to intense deposition near the seaward boundary, which was observed in other studies (Belliard et al., 2015; Geng et al., 2023; Wang et al., 2025). The field measurements along the Jiangsu coast in China, with high sediment supply, confirmed the phenomenon. Here, the maximum bed accretion was recorded in the transitional area between bare tidal flats and densely vegetated saltmarshes (Gong et al., 2017). Also, the wetlands in the Yellow River Delta experienced the invasion and rapid expansion of S. alterniflora (Li et al., 2022). Here, the saltmarshes trapped the suspended sediments and raised the elevation of wetland. Meanwhile, field data also indicated that the dense vegetation hindered sediment transport to the landward and central part of the wetlands, resulting in reduced sediment supply to saltmarsh interiors (Ba et al., 2024). The reduced hydrodynamic and sediment supply in the upper and middle wetland may influence inundation of intertidal zones, possibly leading to the degradation of other saltmarsh species (Wang et al., 2022).
4.2 Processes contributing to channel deepening
The channel deepening following saltmarsh introduction can be attributed to two different processes, 1) the increase of tidal flat elevation near channels due to sediment deposition, and 2) the decrease of channel bed elevation due to sediment erosion. To investigate the relative contributions of these processes on the channel depth change, we examine two cross-sectional profiles located at x=2 km and x=3.5 km (Figures 8b, d, respectively). These profiles represent different regions that are influenced by sediment availability and the presence of vegetation. We quantify sediment deposition and erosion by comparing the elevation differences between the 100-year and 30-year time frames (Figure 8).
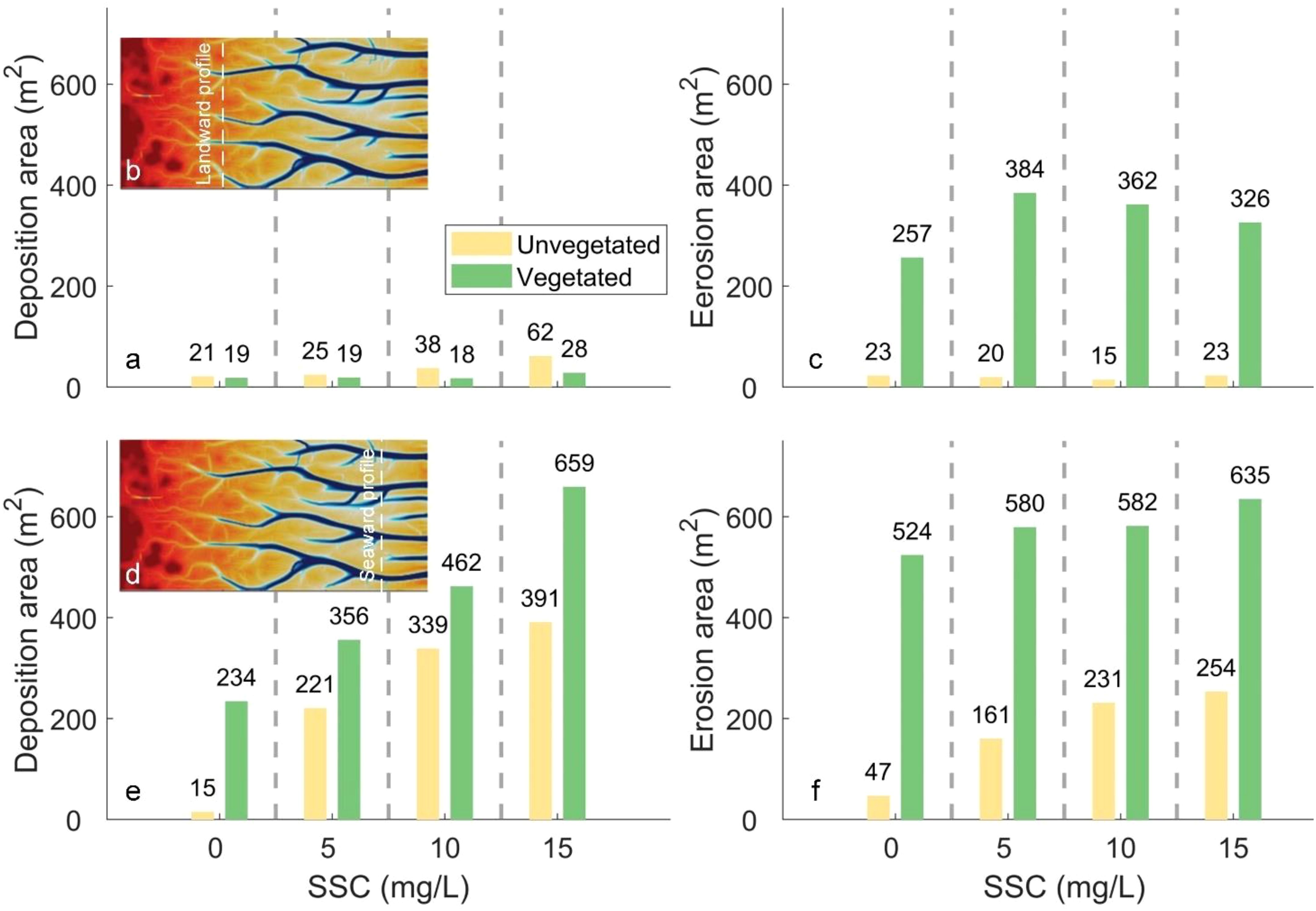
Figure 8. Profile area under different sediment supply and the presence of vegetation due to tidal flat deposition for a landward cross section (a) and seaward cross section (e), and channel bed erosion for a landward cross section (c) and seaward cross section (f). The landward and seaward profiles are indicated by white dashed lines in panels (b, d), respectively.
The model results reveal that the seaward profile is more sensitive to the variation of sediment supply (Figures 8a, c vs 8e, f), suggesting sediment availability plays an important role in the channel development at the seaward side. In contrast, the primary difference of the landward profile is attributed to vegetation-induced erosion (green bars in Figure 8c). Interestingly, the tidal flat deposition in the presence of saltmarshes is smaller than that in the absence of saltmarshes, highlighting how vegetation could hinder sediment transport towards the saltmarsh interior.
Additionally, it is observed that the vegetation-induced erosion area is smaller under higher SSC levels (10 and 15 mg/L) compared to lower SSC levels (5 mg/L). This is likely because, under high SSC, a significant amount of sediment is retained in the channel, and cannot be transported to the flat. Retention promotes sedimentation within the channel and reduces overall erosion, as it was also observed in another study (Gourgue et al., 2024).
On the seaward side, both deposition and erosion areas increase with sediment supply regardless of vegetation (Figures 8e, f). This is because the elevation on the seaward side is generally lower, causing tidal water to be distributed more evenly between the channels and mudflats.
4.3 Model limitations
The present model simulates the co-evolution of tidal channel networks and saltmarshes in an idealized open-coast setting. However, several physical processes have been neglected to make the model more transparent. Firstly, the effects of waves and storms have been excluded, though they may play a significant role in saltmarsh loss and sediment transport, ultimately influencing the development of tidal networks (Leonardi et al., 2016; Zhou et al., 2022b). Field observations suggested that net sediment import or export depends on the modulation of tidal and wave conditions (Green and Coco, 2014; Sun et al., 2024b, a). Waves can lead to sediment resuspension from bare flats, thus promoting sediment transport toward saltmarshes, which is an important source of sediment supply to saltmarshes. Secondly, different saltmarsh species were not included in the modelling works. For example, the dense species Spartina causes stronger flow deviations than the sparse species Salicornia (Bouma et al., 2013). Also, the dense vegetation hinders the sediment transport from tidal channel to flat interiors at the landscape scale (Gourgue et al., 2024). Thirdly, we did not account for the effects of relative sea level rise (RSLR), which is an important factor for long-term evolution of these systems. RSLR can increase the inundation period of saltmarshes and thus threaten the survival of halophytic plants, which can reduce the influence of saltmarshes on tidal channels evolution (Kirwan et al., 2016; Coleman et al., 2022). Overall, despite the aforementioned simplifications, the present model effectively captures the bio-morphodynamic evolution of tidal networks and saltmarshes, generating networks of tidal channels with geometric characteristics that closely resemble those observed in the field.
5 Conclusions
In this study, a bio-morphodynamic model was used to investigate the evolution and development of tidal channels in tidal flats under the presence of vegetation and with varying sediment supply conditions. Firstly, an increase in SSC promotes mudflat accretion and the development of channel networks. As SSC increases, enhanced deposition leads to the expansion of mudflats, which in turn drives the formation of an extensive network of channels across the entire intertidal region.
Secondly, vegetation contributes to more sedimentation on the tidal flat and more erosion within the channels, with the channels lengthening and narrowing. However, over time, vegetation limits landward mudflat accretion, especially under high sediment supply. The impact of vegetation on tidal flat sedimentation is more pronounced at lower SSC levels, but this effect diminishes as sediment supply increases. For example, at the highest sediment supply scenario (15 mg/L), the flat elevation is higher in the absence of vegetation. Vegetation promotes tidal channel erosion, which intensifies when more sediment is available.
Thirdly, increased sediment supply enhances the complexity of the tidal network by increasing drainage density and promoting channel elongation, though it does not significantly initiate new low-order branching channels. In contrast, saltmarsh introduction accelerates the growth of drainage density, particularly in the early stages of development, by promoting small branching channels and stabilizing the tidal flats.
Overall, this research improves our understanding of long-term tidal flat bio-morphodynamic changes resulting from vegetation introduction under different sediment supply conditions, offering valuable insights for future restoration projects.
Data availability statement
The raw data supporting the conclusions of this article will be made available by the authors, without undue reservation.
Author contributions
YG: Conceptualization, Formal Analysis, Methodology, Software, Visualization, Writing – original draft. GH: Writing – review & editing. LG: Writing – review & editing. BX: Writing – review & editing. YZ: Resources, Writing – review & editing. DX: Supervision, Visualization, Writing – review & editing. SF: Writing – review & editing. HZ: Writing – review & editing. GC: Writing – review & editing. ZZ: Funding acquisition, Project administration, Supervision, Writing – review & editing.
Funding
The author(s) declare that financial support was received for the research and/or publication of this article. This study is supported by the National Key R&D Program of China (Grant No. 2024YFE0103100), the National Natural Science Foundation of China (NSFC, Grant Nos. 42361144873, 42376161), the Yellow Sea Wetlands Project (HHSDKT202306), the Jiangsu Provincial Innovation Research Program on Carbon Peaking and Carbon Neutrality (BT2024012), the Fundamental Research Funds for the Central Universities (B230201061), and the fund of National Key Laboratory of Water Disaster Prevention (Grant Nos. 5240152K2, 524015232). SF was partly supported by the USA National Science Foundation Award 2224608 (PIE LTER) and 1832221 (VCR LTER).
Conflict of interest
The authors declare that the research was conducted in the absence of any commercial or financial relationships that could be construed as a potential conflict of interest.
The author(s) declared that they were an editorial board member of Frontiers, at the time of submission. This had no impact on the peer review process and the final decision.
Generative AI statement
The author(s) declare that no Generative AI was used in the creation of this manuscript.
Publisher’s note
All claims expressed in this article are solely those of the authors and do not necessarily represent those of their affiliated organizations, or those of the publisher, the editors and the reviewers. Any product that may be evaluated in this article, or claim that may be made by its manufacturer, is not guaranteed or endorsed by the publisher.
Supplementary material
The Supplementary Material for this article can be found online at: https://www.frontiersin.org/articles/10.3389/fmars.2025.1574276/full#supplementary-material
References
An S. Q., Gu B. H., Zhou C. F., Wang Z. S., Deng Z. F., Zhi Y. B., et al. (2007). Spartina invasion in China: implications for invasive species management and future research. Weed Res. 47, 183–191. doi: 10.1111/j.1365-3180.2007.00559.x
Ba Q., Wang B., Zhu L., Fu Z., Wu X., Wang H., et al. (2024). Rapid change of vegetation cover in the Huanghe (Yellow River) mouth wetland and its biogeomorphological feedbacks. CATENA 238, 107875. doi: 10.1016/j.catena.2024.107875
Baptist M. J., Babovic V., Rodríguez Uthurburu J., Keijzer M., Uittenbogaard R. E., Mynett A., et al. (2007). On inducing equations for vegetation resistance. J. Hydraulic Res. 45, 435–450. doi: 10.1080/00221686.2007.9521778
Belliard J.-P., Toffolon M., Carniello L., D’Alpaos A. (2015). An ecogeomorphic model of tidal channel initiation and elaboration in progressive marsh accretional contexts: TIDAL NETWORK DEVELOPMENT. J. Geophys. Res. Earth Surf. 120, 1040–1064. doi: 10.1002/2015JF003445
Boechat Albernaz M., Roelofs L., Pierik H. J., Kleinhans M. G. (2020). Natural levee evolution in vegetated fluvial-tidal environments. Earth Surf Processes Landf 45, 3824–3841. doi: 10.1002/esp.5003
Bouma T. J., Temmerman S., Van Duren L. A., Martini E., Vandenbruwaene W., Callaghan D. P., et al. (2013). Organism traits determine the strength of scale-dependent bio-geomorphic feedbacks: A flume study on three intertidal plant species. Geomorphology 180–181, 57–65. doi: 10.1016/j.geomorph.2012.09.005
Brückner M. Z. M., Schwarz C., Van Dijk W. M., Van Oorschot M., Douma H., Kleinhans M. G. (2019). Salt marsh establishment and eco-engineering effects in dynamic estuaries determined by species growth and mortality. JGR Earth Surface 124, 2962–2986. doi: 10.1029/2019JF005092
Chen Y., Li Y., Cai T., Thompson C., Li Y. (2016). A comparison of biohydrodynamic interaction within mangrove and saltmarsh boundaries. Earth Surf Processes Landf 41, 1967–1979. doi: 10.1002/esp.3964
Chen L., Moeller I., Zhou Z., Hu Z., Zhang Y., Chu M., et al. (2024). Seasonal biophysical interactions in tidal marsh evolution: insights from a synchronized dataset in Jiangsu, China. Front. Mar. Sci. 11. doi: 10.3389/fmars.2024.1469307
Chen Y., Li Y., Thompson C., Wang X., Cai T., Chang Y. (2018). Differential sediment trapping abilities of mangrove and saltmarsh vegetation in a subtropical estuary. Geomorphology 318, 270–282. doi: 10.1016/j.geomorph.2018.06.018
Coco G., Zhou Z., Van Maanen B., Olabarrieta M., Tinoco R., Townend I. (2013). Morphodynamics of tidal networks: Advances and challenges. Marine Geol. 346, 1–16. doi: 10.1016/j.margeo.2013.08.005
Coleman D. J., Schuerch M., Temmerman S., Guntenspergen G., Smith C. G., Kirwan M. L. (2022). Reconciling models and measurements of marsh vulnerability to sea level rise. Limnol. Oceanogr. Lett. 7, 140–149. doi: 10.1002/lol2.10230
D’Alpaos A., Lanzoni S., Marani M., Fagherazzi S., Rinaldo A. (2005). Tidal network ontogeny: Channel initiation and early development. J. Geophys. Res. 110, 2004JF000182. doi: 10.1029/2004JF000182
Fagherazzi S., Sun T. (2004). A stochastic model for the formation of channel networks in tidal marshes. Geophysical Res. Lett. 31, 2004GL020965. doi: 10.1029/2004GL020965
Geng L., D’Alpaos A., Sgarabotto A., Gong Z., Lanzoni S. (2021). Intertwined eco-morphodynamic evolution of salt marshes and emerging tidal channel networks. Water Resour. Res. 57, e2021WR030840. doi: 10.1029/2021WR030840
Geng L., Gong Z., Lanzoni S., D’Alpaos A. (2018). A new method for automatic definition of tidal creek networks. J. Coastal Res. 85, 156–160. doi: 10.2112/SI85-032.1
Geng L., Gong Z., Zhou Z., Lanzoni S., D’Alpaos A. (2020). Assessing the relative contributions of the flood tide and the ebb tide to tidal channel network dynamics. Earth Surf Processes Landf 45, 237–250. doi: 10.1002/esp.4727
Geng L., Lanzoni S., D’Alpaos A., Sgarabotto A., Gong Z. (2023). The sensitivity of tidal channel systems to initial bed conditions, vegetation, and tidal asymmetry. JGR Earth Surface 128, e2022JF006929. doi: 10.1029/2022JF006929
Gong Z., Jin C., Zhang C., Zhou Z., Zhang Q., Li H. (2017). Temporal and spatial morphological variations along a cross-shore intertidal profile, Jiangsu, China. Continental Shelf Res. 144, 1–9. doi: 10.1016/j.csr.2017.06.009
Gourgue O., Belliard J., Xu Y., Kleinhans M. G., Fagherazzi S., Temmerman S. (2024). Dense vegetation hinders sediment transport toward saltmarsh interiors. Limnol. Oceanogr. Lett. 9, 764–775. doi: 10.1002/lol2.10436
Grandjean T. J., Weenink R., van der Wal D., Addink E. A., Hu Z., Liu S., et al. (2024). Critical turbidity thresholds for maintenance of estuarine tidal flats worldwide. Nat. Geosci. 17, 539–544. doi: 10.1038/s41561-024-01431-3
Green M. O., Coco G. (2014). Review of wave-driven sediment resuspension and transport in estuaries: WAVE-DRIVEN SEDIMENT TRANSPORT. Rev. Geophys. 52, 77–117. doi: 10.1002/2013RG000437
Hanegan K. C., FitzGerald D. M., Georgiou I. Y., Hughes Z. J. (2023). Long-term sea level rise modeling of a basin-tidal inlet system reveals sediment sinks. Nat. Commun. 14, 7117. doi: 10.1038/s41467-023-42895-y
Hautekiet S., Rossius J.-E., Gourgue O., Kleinhans M., Temmerman S. (2024). On the relative role of abiotic and biotic controls in channel network development: insights from scaled tidal flume experiments. Earth Surf. Dynam. 12, 601–619. doi: 10.5194/esurf-12-601-2024
Hood W. G. (2010). Tidal channel meander formation by depositional rather than erosional processes: examples from the prograding Skagit River Delta (Washington, USA). Earth Surf Processes Landf 35, 319–330. doi: 10.1002/esp.1920
Hou X., Xie D., Feng L., Shen F., Nienhuis J. H. (2024). Sustained increase in suspended sediments near global river deltas over the past two decades. Nat. Commun. 15, 3319. doi: 10.1038/s41467-024-47598-6
Jin C., Gong Z., Shi L., Zhao K., Tinoco R. O., San Juan J. E., et al. (2022). Medium-term observations of salt marsh morphodynamics. Front. Mar. Sci. 9. doi: 10.3389/fmars.2022.988240
Kearney W. S., Fagherazzi S. (2016). Salt marsh vegetation promotes efficient tidal channel networks. Nat. Commun. 7, 12287. doi: 10.1038/ncomms12287
Kirwan M. L., Megonigal J. P., Noyce G. L., Smith A. J. (2023). Geomorphic and ecological constraints on the coastal carbon sink. Nat. Rev. Earth Environ. 4, 393–406. doi: 10.1038/s43017-023-00429-6
Kirwan M. L., Temmerman S., Skeehan E. E., Guntenspergen G. R., Fagherazzi S. (2016). Overestimation of marsh vulnerability to sea level rise. Nat. Clim Change 6, 253–260. doi: 10.1038/nclimate2909
Kuai Y., Aarninkhof S., Wang Z. B. (2023). Diagnostic modeling of the shoreline variation along the Jiangsu Coast, China. Geomorphology 425, 108581. doi: 10.1016/j.geomorph.2023.108581
Ladd C. J. T., Duggan-Edwards M. F., Bouma T. J., Pagès J. F., Skov M. W. (2019). Sediment supply explains long-term and large-scale patterns in salt marsh lateral expansion and erosion. Geophysical Res. Lett. 46, 11178–11187. doi: 10.1029/2019GL083315
Leonardi N., Ganju N. K., Fagherazzi S. (2016). A linear relationship between wave power and erosion determines salt-marsh resilience to violent storms and hurricanes. Proc. Natl. Acad. Sci. U.S.A. 113, 64–68. doi: 10.1073/pnas.1510095112
Lesser G. R., Roelvink J. A., Van Kester J. A. T. M., Stelling G. S. (2004). Development and validation of a three-dimensional morphological model. Coastal Eng. 51, 883–915. doi: 10.1016/j.coastaleng.2004.07.014
Li B., Liao C., Zhang X., Chen H., Wang Q., Chen Z., et al. (2009). Spartina alterniflora invasions in the Yangtze River estuary, China: An overview of current status and ecosystem effects. Ecol. Eng. 35, 511–520. doi: 10.1016/j.ecoleng.2008.05.013
Li H., Mao D., Wang Z., Huang X., Li L., Jia M. (2022). Invasion of Spartina alterniflora in the coastal zone of mainland China: Control achievements from 2015 to 2020 towards the Sustainable Development Goals. J. Environ. Manage. 323, 116242. doi: 10.1016/j.jenvman.2022.116242
Li H., Yang S. L. (2009). Trapping effect of tidal marsh vegetation on suspended sediment, Yangtze delta. J. Coastal Res. 254, 915–924. doi: 10.2112/08-1010.1
Li R., Yu Q., Wang Y., Wang Z. B., Gao S., Flemming B. (2018). The relationship between inundation duration and Spartina alterniflora growth along the Jiangsu coast, China. Estuarine Coastal Shelf Sci. 213, 305–313. doi: 10.1016/j.ecss.2018.08.027
Liu Z., Fagherazzi S., Cui B. (2021). Success of coastal wetlands restoration is driven by sediment availability. Commun. Earth Environ. 2, 44. doi: 10.1038/s43247-021-00117-7
Liu S., Hu Z., Grandjean T. J., Wang Z. B., Van Zelst V. T. M., Qi L., et al. (2025). Dynamics and drivers of tidal flat morphology in China. Nat. Commun. 16, 2153. doi: 10.1038/s41467-025-57525-y
Ma Z., Ysebaert T., van der Wal D., De Jong D. J., Li X., Herman P. M. J. (2014). Long-term salt marsh vertical accretion in a tidal bay with reduced sediment supply. Estuarine Coastal Shelf Sci. 146, 14–23. doi: 10.1016/j.ecss.2014.05.001
Maan D. C., Van Prooijen B. C., Wang Z. B. (2019). Progradation speed of tide-dominated tidal flats decreases stronger than linearly with decreasing sediment availability and linearly with sea level rise. Geophysical Res. Lett. 46, 262–271. doi: 10.1029/2018GL079933
Marani M., Belluco E., D’Alpaos A., Defina A., Lanzoni S., Rinaldo A. (2003). On the drainage density of tidal networks. Water Resour. Res. 39, 2001WR001051. doi: 10.1029/2001WR001051
Marani M., Da Lio C., D’Alpaos A. (2013). Vegetation engineers marsh morphology through multiple competing stable states. Proc. Natl. Acad. Sci. U.S.A. 110, 3259–3263. doi: 10.1073/pnas.1218327110
Mariotti G. (2018). Marsh channel morphological response to sea level rise and sediment supply. Estuarine Coastal Shelf Sci. 209, 89–101. doi: 10.1016/j.ecss.2018.05.016
Möller I., Kudella M., Rupprecht F., Spencer T., Paul M., Van Wesenbeeck B. K., et al. (2014). Wave attenuation over coastal salt marshes under storm surge conditions. Nat. Geosci 7, 727–731. doi: 10.1038/ngeo2251
Mudd S. M., D’Alpaos A., Morris J. T. (2010). How does vegetation affect sedimentation on tidal marshes? Investigating particle capture and hydrodynamic controls on biologically mediated sedimentation. J. Geophys. Res. 115, 2009JF001566. doi: 10.1029/2009JF001566
Murray N. J., Phinn S. R., DeWitt M., Ferrari R., Johnston R., Lyons M. B., et al. (2019). The global distribution and trajectory of tidal flats. Nature 565, 222–225. doi: 10.1038/s41586-018-0805-8
Nardin W., Edmonds D. A. (2014). Optimum vegetation height and density for inorganic sedimentation in deltaic marshes. Nat. Geosci 7, 722–726. doi: 10.1038/ngeo2233
Schwarz C., Gourgue O., Van Belzen J., Zhu Z., Bouma T. J., Van De Koppel J., et al. (2018). Self-organization of a biogeomorphic landscape controlled by plant life-history traits. Nat. Geosci 11, 672–677. doi: 10.1038/s41561-018-0180-y
Schwarz C., Van Rees F., Xie D., Kleinhans M. G., Van Maanen B. (2022). Salt marshes create more extensive channel networks than mangroves. Nat. Commun. 13, 2017. doi: 10.1038/s41467-022-29654-1
Schwarz C., Ye Q. H., van der Wal D., Zhang L. Q., Bouma T., Ysebaert T., et al. (2014). Impacts of salt marsh plants on tidal channel initiation and inheritance. JGR Earth Surface 119, 385–400. doi: 10.1002/2013JF002900
Schwarz C., Ysebaert T., Vandenbruwaene W., Temmerman S., Zhang L., Herman P. M. J. (2016). On the potential of plant species invasion influencing bio-geomorphologic landscape formation in salt marshes. Earth Surf Processes Landf 41, 2047–2057. doi: 10.1002/esp.3971
Stefanon L., Carniello L., D’Alpaos A., Lanzoni S. (2010). Experimental analysis of tidal network growth and development. Continental Shelf Res. 30, 950–962. doi: 10.1016/j.csr.2009.08.018
Stefanon L., Carniello L., D’Alpaos A., Rinaldo A. (2012). Signatures of sea level changes on tidal geomorphology: Experiments on network incision and retreat. Geophysical Res. Lett. 39, 2012GL051953. doi: 10.1029/2012GL051953
Sun J., Van Prooijen B., Wang X., Hanssen J., Xie W., Lin J., et al. (2024a). Sources of suspended sediments in salt marsh creeks: Field measurements in China and the Netherlands. Geomorphology 456, 109206. doi: 10.1016/j.geomorph.2024.109206
Sun J., Van Prooijen B., Wang X., Xie W., Xu F., He Q., et al. (2024b). Conditional effects of tides and waves on sediment supply to salt marshes. JGR Earth Surface 129, e2024JF007686. doi: 10.1029/2024JF007686
Temmerman S., Bouma T. J., Van De Koppel J., van der Wal D., De Vries M. B., Herman P. M. J. (2007). Vegetation causes channel erosion in a tidal landscape. Geol 35, 631. doi: 10.1130/G23502A.1
Temmink R. J. M., Lamers L. P. M., Angelini C., Bouma T. J., Fritz C., Van De Koppel J., et al. (2022). Recovering wetland biogeomorphic feedbacks to restore the world’s biotic carbon hotspots. Science 376, eabn1479. doi: 10.1126/science.abn1479
Vandenbruwaene W., Bouma T. J., Meire P., Temmerman S. (2013). Bio-geomorphic effects on tidal channel evolution: impact of vegetation establishment and tidal prism change. Earth Surf Processes Landf 38, 122–132. doi: 10.1002/esp.3265
Van De Vijsel R. C., Van Belzen J., Bouma T. J., van der Wal D., Borsje B. W., Temmerman S., et al. (2023). Vegetation controls on channel network complexity in coastal wetlands. Nat. Commun. 14, 7158. doi: 10.1038/s41467-023-42731-3
Wang D., Gu C., Temmerman S., Belliard J., Gourgue O., Xue L., et al. (2025). Coastal marsh vulnerability to sea-level rise is exacerbated by plant species invasion. Global Change Biol. 31, e70058. doi: 10.1111/gcb.70058
Wang B., Zhang K., Liu Q.-X., He Q., Van De Koppel J., Teng S. N., et al. (2022). Long-distance facilitation of coastal ecosystem structure and resilience. Proc. Natl. Acad. Sci. U.S.A. 119, e2123274119. doi: 10.1073/pnas.2123274119
Wei Y., Van Maanen B., Xie D., Jiang Q., Zhou Z., Schwarz C. (2024). Mangrove-saltmarsh ecotones: are species shifts determining eco-morphodynamic landform configurations? Earth’s Future 12, e2024EF004990. doi: 10.1029/2024EF004990
Xie W., He Q., Wang X., Guo L., Zhang K. (2018). Role of mudflat-creek sediment exchanges in intertidal sedimentary processes. J. Hydrol. 567, 351–360. doi: 10.1016/j.jhydrol.2018.10.027
Xie D., Schwarz C., Brückner M. Z. M., Kleinhans M. G., Urrego D. H., Zhou Z., et al. (2020). Mangrove diversity loss under sea-level rise triggered by bio-morphodynamic feedbacks and anthropogenic pressures. Environ. Res. Lett. 15, 114033. doi: 10.1088/1748-9326/abc122
Xie D., Schwarz C., Kleinhans M. G., Bryan K. R., Coco G., Hunt S., et al. (2023). Mangrove removal exacerbates estuarine infilling through landscape-scale bio-morphodynamic feedbacks. Nat. Commun. 14, 7310. doi: 10.1038/s41467-023-42733-1
Xie D., Schwarz C., Kleinhans M. G., Zhou Z., van Maanen B. (2022). Implications of coastal conditions and sea-level rise on mangrove vulnerability: A bio-morphodynamic modeling study. JGR Earth Surface 127, e2021JF006301. doi: 10.1029/2021JF006301
Xu F., Coco G., Tao J., Zhou Z., Zhang C., Lanzoni S., et al. (2019). On the morphodynamic equilibrium of a short tidal channel. JGR Earth Surface 124, 639–665. doi: 10.1029/2018JF004952
Xu F., Coco G., Zhou Z., Tao J., Zhang C. (2017). A numerical study of equilibrium states in tidal network morphodynamics. Ocean Dynamics 67, 1593–1607. doi: 10.1007/s10236-017-1101-0
Xu Y., Esposito C. R., Beltrán-Burgos M., Nepf H. M. (2022). Competing effects of vegetation density on sedimentation in deltaic marshes. Nat. Commun. 13, 4641. doi: 10.1038/s41467-022-32270-8
Xu C., Silliman B. R., Chen J., Li X., Thomsen M. S., Zhang Q., et al. (2023). Herbivory limits success of vegetation restoration globally. Science 382, 589–594. doi: 10.1126/science.add2814
Xu F., Zhou Z., Fagherazzi S., D’Alpaos A., Townend I., Zhao K., et al. (2024). Anomalous scaling of branching tidal networks in global coastal wetlands and mudflats. Nat. Commun. 15, 9700. doi: 10.1038/s41467-024-54154-9
Zhang D., Hu Y., Liu M., Chang Y., Yan X., Bu R., et al. (2017). Introduction and spread of an exotic plant, spartina alterniflora, along coastal marshes of China. Wetlands 37, 1181–1193. doi: 10.1007/s13157-017-0950-0
Zhou Z., Coco G., Townend I., Olabarrieta M., van der Wegen M., Gong Z., et al. (2017). Is “Morphodynamic equilibrium” an oxymoron? Earth-Science Rev. 165, 257–267. doi: 10.1016/j.earscirev.2016.12.002
Zhou Z., Liang M., Chen L., Xu M., Chen X., Geng L., et al. (2022a). Processes, feedbacks, and morphodynamic evolution of tidal flat–marsh systems: Progress and challenges. Water Sci. Eng. 15, 89–102. doi: 10.1016/j.wse.2021.07.002
Zhou Z., Olabarrieta M., Stefanon L., D’Alpaos A., Carniello L., Coco G. (2014). A comparative study of physical and numerical modeling of tidal network ontogeny. JGR Earth Surface 119, 892–912. doi: 10.1002/2014JF003092
Zhou Z., Wei Y., Geng L., Zhang Y., Gu Y., Finotello A., et al. (2024). Cross-shore parallel tidal channel systems formed by alongshore currents. Nat. Commun. 15, 4732. doi: 10.1038/s41467-024-49176-2
Zhou Z., Wu Y., Fan D., Wu G., Luo F., Yao P., et al. (2022b). Sediment sorting and bedding dynamics of tidal flat wetlands: Modeling the signature of storms. J. Hydrol. 610, 127913. doi: 10.1016/j.jhydrol.2022.127913
Keywords: saltmarshes, morphodynamics, tidal networks, mudflat, sediment availability
Citation: Gu Y, Han G, Geng L, Xie B, Zhang Y, Xie D, Fagherazzi S, Zhang H, Coco G and Zhou Z (2025) Trade-offs between vegetation and sediment availability in shaping long-term tidal network morphodynamics. Front. Mar. Sci. 12:1574276. doi: 10.3389/fmars.2025.1574276
Received: 10 February 2025; Accepted: 08 April 2025;
Published: 14 May 2025.
Edited by:
Haosheng Huang, Louisiana State University, United StatesReviewed by:
Junliang Gao, Jiangsu University of Science and Technology, ChinaQinghua Ye, Deltares, Netherlands
Copyright © 2025 Gu, Han, Geng, Xie, Zhang, Xie, Fagherazzi, Zhang, Coco and Zhou. This is an open-access article distributed under the terms of the Creative Commons Attribution License (CC BY). The use, distribution or reproduction in other forums is permitted, provided the original author(s) and the copyright owner(s) are credited and that the original publication in this journal is cited, in accordance with accepted academic practice. No use, distribution or reproduction is permitted which does not comply with these terms.
*Correspondence: Zeng Zhou, emVuZy56aG91QGhodS5lZHUuY24=; Danghan Xie, ZGFuZ2hhbkB1Y3NkLmVkdQ==