- 1Laboratory for Innovation and Sustainability of Marine Biological Resources (ECOMARE), Centre for Environmental and Marine Studies (CESAM), Department of Chemistry, University of Aveiro, Aveiro, Portugal
- 2ECOMARE, CESAM, Department of Biology, University of Aveiro, Aveiro, Portugal
- 3Associated Laboratory for Green Chemistry of the Network of Chemistry and Technology (LAQV-REQUIMTE), Department of Chemistry, University of Aveiro, Aveiro, Portugal
- 4CESAM, University of Aveiro, Aveiro, Portugal
- 5Aveiro Institute of Materials (CICECO), Department of Materials and Ceramic Engineering, University of Aveiro, Aveiro, Portugal
Some Sacoglossa sea slugs feed on macroalgae and sequester chloroplasts in the cells of their digestive diverticulum. In some species, mostly within the genus Elysia, the stolen chloroplasts – kleptoplasts – remain photosynthetically competent for weeks to months. These sea slugs, like other gastropods, produce a viscous secretion or mucus involved in protection, locomotion, and reproduction. In this study, we profiled the carbohydrate composition of the mucus of the kleptoplast-bearing sea slug Elysia crispata and tracked the incorporation of carbon and nitrogen via stolen chloroplasts. Mucopolysaccharides were mainly composed by uronic acids and galactose with 1→4 glycosidic linkages. Using inorganic 13C-labelled sea water, incorporated carbon was found in the secreted mucus only in the presence of light, mainly in the form of 3-O-methylgalactose, galactose, and fucose. Inorganic 15N in the sea water could be traced in the sea slug tissues and in the mucus in both dark and light, with slightly higher levels found under the latter condition. Results show that inorganic carbon was fixed through photosynthesis in the kleptoplasts of the digestive diverticula, translocated as organic carbon to other animal tissues and incorporated in the secreted mucopolysaccharides. The present study pinpoints the biological relevance of photosynthesis to the metabolism of these remarkable animal-chloroplast symbiotic associations.
1 Introduction
Some Sacoglossa sea slugs feed on algae by piercing the vegetative tissues or thalli, ingesting and digesting the cellular content, while preserving the integrity and functionality of the chloroplasts, a phenomenon known as kleptoplasty (Rumpho et al., 2000). Kleptoplasty in this clade of marine gastropods was first described in Elysia atroviridis in 1965 (Kawaguti and Yamasu, 1965). The stolen chloroplasts – kleptoplasts – can remain functional within the digestive diverticula cells for weeks to months (Hinde and Smith, 1972; Evertsen et al., 2007; Cruz and Cartaxana, 2022). The duration of kleptoplast retention depends on several factors, including sea slug species, origin of the sea slug (wild vs. lab-reared), water temperature, chloroplast algal source, and light conditions (Händeler et al., 2009; Vieira et al., 2009; Pelletreau et al., 2012; Christa et al., 2013; Schmitt et al., 2014). Kleptoplasty plays a vital role in sacoglossan sea slugs, impacting nutrition, reproduction, and survival (Yamamoto et al., 2013; Cartaxana et al., 2017; 2021). Despite its importance, the mechanisms behind the long-term retention of functional kleptoplasts in Sacoglossa sea slugs remain elusive.
Using radioactive carbon (14C), earlier studies indicated that photosynthesis-derived metabolites were translocated from the kleptoplasts in the digestive tubules into kleptoplast-free sea slug tissues (Trench et al., 1969; 1973). More recently, this was confirmed using 13C labelling and Nanoscale Secondary Ion Mass Spectrometry (NanoSIMS), allowing high resolution imaging of carbon translocated from the kleptoplasts (Cruz et al., 2020; Cartaxana et al., 2021). The latter studies showed kleptoplast-mediated light-dependent incorporation of 13C in fatty acids of Elysia viridis and Elysia timida. While the uptake of carbon via kleptoplast photosynthesis is well-established, a possible role of kleptoplasty in nitrogen assimilation remains unclear. Nevertheless, light-dependent incorporation was observed for incubations of Elysia species with 15N-labelled inorganic nitrogen (Teugels et al., 2008; Cruz et al., 2020; Cartaxana et al., 2021), indicating a possible role of the kleptoplasts in the animals’ nitrogen metabolism. Teugels et al. (2008) conducted a series of comparative experiments on the sea slug E. viridis and reported evidence for light-dependent assimilation of ammonium, nitrite, and urea. The assimilated nitrogen was traced into individual hydrolysable amino acids.
Mucus secretions are paramount for the survival of sea slugs and other gastropods, serving several biological functions, such as protection, locomotion, and reproduction (Smith, 2002). The biochemical composition of the mucus varies with species and specific function (Smith and Morin, 2002); however, polysaccharides and proteins are the major components of gastropod mucus (Smith, 2002). Using 14C, earlier studies on kleptoplast-bearing sea slugs have suggested that photosynthesis may provide carbohydrate substrates for the synthesis of mucopolysaccharides (Trench et al., 1969, 1970; 1972).
The sea slug Elysia crispata is a tropical sacoglossan known to feed and incorporate chloroplasts from a wide variety of green macroalgae (Middlebrooks et al., 2014; 2019). In a previous study, we have shown that the E. crispata grown under regular light conditions produced significant higher amount of mucus when compared to quasi-dark reared conspecifics (Lopes et al., 2022). We also found that animals under quasi-dark conditions produced mucus with significant lower total carbohydrate concentrations when compared with animals reared in light. In this study, we present a detailed assessment of the carbohydrate composition of the mucus of E. crispata and track the incorporation of 13C-labelled inorganic carbon and 15N-labelled inorganic nitrogen via kleptoplasts. We aim to contribute for the understanding of the role of photosynthesis in the overall sea slug metabolism and, specifically, in mucopolysaccharide synthesis.
2 Methods
2.1 Animal maintenance
The tropical sea slug Elysia crispata was reared in the laboratory under conditions similar to those described in Cartaxana et al. (2023). Adult animals were maintained in 150 L recirculated life-support systems (LSS) with artificial sea water (ASW) at 35 ppt salinity and 25 °C. ASW was prepared by mixing Red Sea salt (Red Sea Europe, Verneuil d’Avre et d’Iton, France) with reverse osmose water (V2 Pure Advanced Reverse Osmosis System, TMC, Hertfordshire, UK) to achieve the desired salinity. A photoperiod of 12 h light:12 h dark with a photon scalar irradiance of 60–80 µmol photons m−2 s−1 was provided to the sea slugs using T5 fluorescent lamps. Irradiance was measured at the water surface with a Spherical Micro Quantum Sensor and a ULM-500 Universal Light Meter (Heinz Walz GmbH, Effeltrich, Germany). Animals were fed regularly with the alga Bryopsis sp. (strain KU-0990, acquired in 2018 from the Kobe University Macro-Algal Culture Collection, Japan). The alga was grown in 2 L flasks with ASW supplemented with f/2 medium (without silica), at 20°C and with constant aeration. The alga was exposed to an irradiance of 60–80 μmol photons m−2 s−1 provided by LED lamps (Valoya 35 W, spectrum NS12, Helsinki, Finland) and a photoperiod of 12 h light:12 h dark.
2.2 Mucus carbohydrate analysis
2.2.1 Mucus collection and sample preparation
E. crispata adults were randomly sampled from the LSS for mucus collection. Each sea slug was rinsed with clean ASW to remove any debris attached to their bodies. Subsequently, each specimen was carefully placed in a 200 μm sieve and excess water was removed with tissue paper underneath. The sea slugs were then placed in individual 8 cm watch glasses, wetted with 200 μL of ASW and gently swirled for 5 min to induce mucus production. The secreted mucus was collected with a micropipette into previously cooled Eppendorf tubes. Samples were then frozen at –80°C until further processing. Each mucus sample corresponds to mucus secretion collected from seven different sea slugs. Mucus samples underwent an initial dialysis step to remove excess salt. Upon thawing at room temperature, samples were transferred into dialysis sleeves with a 12–14 kDa molecular weight cut-off. The dialysis sleeves were immersed in distilled water at 4°C with stirring, with two daily water changes during 5 days. To prevent microbial contamination, a few drops of toluene and chloroform were added to the dialysis water. Following dialysis, the samples were refrozen, freeze-dried, and stored in a desiccator for subsequent analysis.
2.2.2 Neutral sugars
Mucus samples (1 mg dry weight) from E. crispata were subjected to acid hydrolysis using 1 mL of 2 M trifluoroacetic acid (TFA) for 1 h at 120 °C. After removal of TFA in a speedvac vacuum concentrator, sugars were reduced by adding of 100 μL of 15% (m/v) sodium borohydride (NaBH4) in 3 M ammonia, followed by incubation for 1 h at 30°C. Excess NaBH4 was destroyed in the end by adding 50 μL of acetic acid twice, in a cold-water bath. Acetylation was carried out by adding 450 μL of 1-methylimidazole and 3 mL of acetic anhydride (Blakeney et al., 1983). After vortex mixing, the samples were incubated for 30 min at 30°C. The resulting alditol acetates were then extracted into an organic phase by adding 3 mL of distilled water and 2.5 mL of dichloromethane, followed by vigorous stirring and separation by centrifugation (30 s, 1400 × g). The aqueous phase was subsequently removed by suction with a vacuum. This process (addition, stirring, centrifugation, and removal of the aqueous phase) was repeated two times. The organic phase was further washed twice with 3 mL of distilled water, stirred, and centrifuged, after which the remaining aqueous phase was completely removed. The organic phase was then placed in the speedvac for the evaporation of dichloromethane. Subsequently, 1 mL of anhydrous acetone was added and evaporated twice.
The alditol acetates were dissolved in 30 μL of anhydrous acetone and analyzed by gas chromatography with a quadrupole mass spectrometry detector (GCqMS, GC-2010 Plus, Shimadzu, Kyoto, Japan) using a non-polar column HT5 (30 m length, 0.25 mm internal diameter, and 0.10 μm stationary phase, Trajan, Australia). The sample (1 μL) was injected in split mode with an injector temperature of 350°C. 2-Deoxyglucose was used as an internal standard. The oven temperature program started at 140°C with a temperature increase at a rate of 5°C per min until reaching 180°C and held for 1 min. The temperature was then increased till 250°C by a 5°C per min rate increase, followed by a 100°C rate per min increase to reach the final temperature of 350°C. The carrier gas (He) flow rate was maintained at 0.86 mL min–1. The mass spectrometer was operated in the electron impact mode (EI) at 100 eV scanning the range 50–400 m/z, in a full scan acquisition mode, with an ion source temperature of 250°C and an interface temperature of 325°C. Peaks were identified by comparing all mass spectra with a laboratory made database of alditol acetates and analyzed with Shimadzu GCMSsolution software.
2.2.3 Glycosidic linkages
The composition of glycosidic linkages present in the polysaccharides of the mucus from E. crispata was analyzed through methylation (Passos and Coimbra, 2013). One to two mg of samples were dissolved in 1 mL of anhydrous dimethylsulfoxide, followed by the addition of 40 mg of sodium hydroxide (NaOH) powdered under an argon atmosphere. The samples were methylated by addition of 80 µL of iodomethane and stirring for 20 min for three times. Subsequently, 3 mL of chloroform/methanol (1:1, v/v) was added, and the solution was dialyzed using membranes with a 12–14 kDa cut-off immersed in 1 L of 50% ethanol solution (three times). The dialysate was then evaporated to dryness with a speedvac, and the material was remethylated following the same procedure. The partially methylated material was subjected to hydrolysis with 2 M TFA (500 μL) at 120 °C for 1 h, followed by reduction and acetylation as previously described for neutral sugar analysis (using sodium borodeuteride instead of NaBH4).
The partially methylated alditol acetates were separated and analyzed using the same GCqMS system. The samples (1 μL) were injected of in split mode at an injection temperature of 250°C. The GC oven temperature program started at 80°C, ramped up to 140°C at 7.5°C min–1, held for 5 min, increased to 143.2°C at 0.2°C min–1, further increased to 200°C at 12°C min–1, and finally ramped to 300°C at 50°C min–1, held for 5 min. The carrier gas (He) flow rate was set at 1.84 mL min–1. The mass spectrometer was operated in the electron impact mode (EI) at 100 eV scanning the range 50–700 m/z, in a full scan acquisition mode, with an ion source temperature of 250°C and an interface temperature of 325°C. Chromatogram peaks were identified by comparing all mass spectra with a laboratory-made database of partially methylated alditol acetates.
2.2.4 Uronic acids
Uronic acids were quantified using the m-phenylphenol colorimetric method as outlined by Bastos et al. (2015). Galacturonic acid served as the standard for calibration within the concentration range of 10 to 100 µg mL–1. For the assay, 0.1 mL of hydrolyzed samples (2 M TFA for 1 h at 120°C) were mixed with 1 mL of 200 mM boric acid in 96% (w/w) sulfuric acid and heated to 100°C for 10 min. The samples were then rapidly cooled using ice water bath, followed by the addition of 20 µL of m-phenylphenol. After transferring 300 µL to a microplate, the absorbance was measured at 520 nm.
To identify and quantify the uronic acids, samples were neutralized and passed through a 0.45 µm nylon filter before High-Performance Anion-Exchange Chromatography/Pulsed Amperometric Detection (HPAEC-PAD) analysis. Samples (25 μL) were injected onto the column and the elution followed an 86 min program optimized based on the methods of Corradini et al. (1998) and Zhang et al. (2012), with the following gradient: starting with eluent A:B:C at a ratio of 100:0:0 (v/v/v) from 0 to 39 min, transitioning to 60:25:15 (v/v/v) within 6 min, further transitioning to 55:25:20 (v/v/v) within 8 min, then to 0:75:25 (v/v/v) within 6 min, and finally to 0:100:0 (v/v/v) within 1 min, maintained for 10 min. The eluents returned to the initial ratio within 1 min, and the column was equilibrated for 15 min. The eluents used were as follows: eluent A – MilliQ water; eluent B – 500 mM NaOH; eluent C – 1 M sodium acetate (CH3COONa) in 100 mM NaOH. These solvents were prepared using MilliQ water (resistance of 18 MΩ cm or greater) and degassed with sonication for 30 min. For eluent B, 26.4 mL of 50% NaOH solution (Merck, Darmstadt, Germany) was added per liter. For eluent C, 41.015 g of CH3COONa (Thermo Scientific Dionex AAA-Direct Reagents) was added per liter; the CH3COONa solution was filtered through a 0.2 µm nylon filter after adding to 100 mM NaOH (5.28 mL of 50% NaOH solution per liter). Eluents were blanketed or purged with high-purity nitrogen after transfer to 2 L plastic Thermo Fisher Dionex eluent bottles and were used within one week. The column and detector were maintained at a working temperature of 30°C, with initial equilibration performed using 20% solvent B. The HPAEC-PAD analysis was performed using a Dionex ICS-6000 system (Massachusetts, USA), including a DC chromatography oven, SP pump, and autosampler AS-AP. Carbohydrates were detected electrochemically in integrated amperometry mode with an AgCl reference electrode and a conventional permanent gold electrode. Chromeleon 7.3 software (Thermo Scientific Dionex) was utilized, along with the standard carbohydrate quadruple waveform recommended for CarboPac columns. Compound separation was conducted employing a Dionex CarboPac PA100 guard column (50 mm × 4 mm) and a Dionex CarboPac PA100 analytical column (250 mm × 4 mm), utilizing gradient elution at a flow rate of 1 mL min–1.
To quantify the uronic acids, calibration curves were established for glucuronic acid (1-100 μg/mL), galacturonic acid (1-100 μg/mL), and guluronic acid (1-60 μg/mL).
2.3 Mucus elemental analysis
The elemental composition of mucus samples was analyzed for carbon (C), nitrogen (N), and sulfur (S). One milligram of sample was loaded into a Leco Truspec-Micro CHNS 630-200-200 elemental analyzer, which featured a combustion furnace operating at 1075°C and an afterburner at 850°C. Infrared absorption was used to detect C and S, while N was detected using thermal conductivity.
2.4 Light-dependent incorporation of C and N
2.4.1 Isotopic dual labelling
Isotopic dual labelling experiments were conducted in closed-systems (1 L transparent glass bottles). ASW was prepared as referred in Harrison et al. (1980) but using sodium bicarbonate (NaHCO3) with 13C isotopic abundance of 99% (Sigma-Aldrich) and adding ammonium chloride (NH4Cl) with 15N isotopic abundance of 98% (Sigma-Aldrich) to a final concentration of 2 mM and 20 μM, respectively, for 13C/15N-enriched ASW (here termed “labelled ASW”). Non-labelled ASW contained common NaHCO3 and NH4Cl (Sigma-Aldrich) in the same concentrations as the 13C and 15N isotopically enriched ASW. Sea slugs were not fed for three days before the start of the labelling experiment. Animals were then incubated in labelled and non-labelled ASW at 25°C, in the absence of their food source, under a photon scalar irradiance of 80 μmol photons m–2 s–1 with LED lamps (Valoya 35 W, spectrum NS12), for testing carbon and nitrogen incorporation in the presence of light. In an additionally third treatment, sea slugs were incubated in labelled ASW and in full darkness, for testing light-independent carbon and nitrogen incorporation. The pulse of isotopic dual labelling started 1 h after the onset of the light period. Five independent glass bottles per treatment were used, each containing 7 sea slugs (3 x 5 x 7 = 105 animals).
Following a 12 h incubation period, the animals were washed with clean non-labelled ASW and stimulated to produce mucus as described in 2.2. The duration of the incubation period was chosen based on previous studies (Cruz et al., 2020; Cartaxana et al., 2021) to guarantee relevant carbon and nitrogen assimilation by the sea slugs and in the secreted mucus. Collected mucus samples, corresponding to the mucus secreted by the seven sea slugs of each glass bottle, were frozen in liquid nitrogen and stored at –80°C. After mucus collection, one animal from each glass bottle was rapidly dipped in distilled water, for removal of excessive ASW, immediately frozen in liquid nitrogen, and stored at –80°C for further analysis. In both mucus and sea slugs’ collection, for avoiding potential contamination, control treatment was sampled first. Plastic tools used for sampling labelled materials were discarded and glass material was carefully washed separately from remaining materials. Mucus samples underwent the dialysis preparation step described in 2.2.1. Both mucus and sea slug samples were freeze-dried before isotope ratio mass spectrometry analysis.
2.4.2 Isotopic composition analysis of bulk tissues
Mucus samples were transferred to glass vials and 200.2 ± 3.8 μg was weight (Radwag Model MYA 2.4Y) into tin capsules (universal soft tin containers, vol 157 μL, Thermo Scientific). Sea slug samples were transferred to glass vials and homogenized with a metal spatula before weighting 203.6 ± 2.3 μg into tin capsules. For each treatment, five biological replicates and two technical replicates were analyzed. The ratio (R) of 13C/12C and 15N/14N for each sample were determined using a Flash IRMS (Isotope Ratio Mass Spectrometer) featuring an Elemental Analyzer (EA) IsoLink CNSOH connected to a Delta V Advantage IRMS via a ConFlo IV interface (Thermo Fischer Scientific, Bremen, Germany). Combustion furnace at the EA was operating at 1025°C, CN reactor, carrier and reference gas (He) flow at 180 and 70 mL min-1, respectively, and oxygen flow at 250 mL min-1 with a 3 s injection time. Enrichment in 13C and 15N were determined from the atomic percentage value (At%) of those isotopes (Isodat 3.0 software): At% = R/(1+R) × 100. Samples weight and EA-IRMS analysis always started with non-labelled materials to avoid contamination. Tools were carefully cleaned between each sample weight. Empty caps were run before and after analysis of labelled samples. No carry-over effects were observed.
2.4.3 Compound-specific isotope analysis
Mucus samples underwent the acid hydrolysis steps described in 2.2.3. The CSIA of the neutral sugars was performed by GC-MS/IRMS using a Trace 1310 gas chromatograph connected to a ISQ 7000 mass-selective detector and a Delta V Advantage isotope ratio mass spectrometer via a combustion interface GC-Isolink III (Thermo Fisher Scientific, Bremen, Germany). GC separation of alditol acetates was performed using a T5-MS column (Thermo Fisher Scientific, Bremen, Germany) fused-silica column (30 m × 0.25 mm, 0.25 μm coated with 5% Phenyl Methylpolysiloxane), with injection of 2 μL in split mode at 275°C. The GC oven temperature program started at 140°C, ramped up to 180°C at 5°C min–1, held for 1 min, increased to 230°C at 5°C min–1, held for 2.5 min, further increased to 250°C at 5°C min–1, held for 1 min, ramped up to 325°C at 100°C min–1, held for 1 min, and finally decreased until reach 140°C. The run was completed after 31.95 min. The carrier gas (He) was maintained at a flow rate of 0.86 mL min–1. The mass spectrometer operated in EI at 70 eV, scanning the range 70–420 m/z in a full scan acquisition mode. Chromatogram peaks were identified by comparing mass spectra with a laboratory-made database of alditol acetates. The At% 13C of each identified monosaccharide was calculated from the R 13C/12C as described in 2.4.2. The At% 13C of the minor peak of arabinose was not determined due to partial co-elution with the peak of fucose in the IRMS. A blank determination was performed twice between each sample, ensuring no contamination in the system. Non-labelled samples were always run before 13C- and 15N-enriched samples. Intensive system cleaning was performed after analysis of enriched samples. Technical replicates were run in separated weeks.
2.5 Statistical analysis
Statistically significant differences between different treatments (non-labelled light, labelled dark and labelled light) for sea slug tissue and mucus At% 13C and 15N were tested using one-way ANOVA. Multiple comparisons were performed using Tukey HSD tests. Data complied with the normality assumption (Kolmogorov-Smirnov and Shapiro-Wilk’s tests), but not with the homoscedasticity assumption (Levene’s test). The statistical results of ANOVA are presented as they are fairly robust for heterogeneity of variances when sample sizes are equal (Box, 1954). Furthermore, very similar outputs were obtained with alternatives that do not assume homoscedasticity (Brown-Forsythe and Welch tests). Statistical analyses were carried out using IBM SPSS Statistics 30.
3 Results
The mucus of the sea slug E. crispata was composed of 29.8 ± 1.0% carbon and 5.9 ± 0.2% nitrogen. Relevant concentrations of sulphur (4.9 ± 0.6%) were also observed. Mucus sugar composition was determined in terms of uronic acids and neutral sugars. Uronic acids made up most of the carbohydrate composition with a total concentration of 212.0 ± 4.5 mg g–1. Guluronic acid was the main uronic acid present with a concentration of 85.4 ± 2.2 mg g–1, followed by glucuronic acid (66.8 ± 10.1 mg g–1) and galacturonic acid (59.8 ± 12.4 mg g–1) (Figure 1). Total neutral sugar concentration in the mucus was 40.2 ± 9.4 mg g–1. Galactose (Gal) was the main neutral monosaccharide with a concentration of 28.0 ± 6.0 mg g–1 (Figure 2). Other neutral monosaccharides identified were glucose (Glc, 3.5 ± 1.2 mg g–1), xylose (Xyl, 2.2 ± 1.6 mg g–1), fucose (Fuc, 1.9 ± 1.2 mg g–1), glucosamine (GlcN, 1.6 ± 0.2 mg g–1), ribose (Rib, 1.0 ± 1.0 mg g–1), 3-O-methylgalactose (mGal, 0.9 ± 0.3 mg g–1), galactosamine (GalN, 0.7 ± 0.1 mg g–1), and arabinose (Ara, 0.5 ± 0.4 mg g–1). The most predominant glycosidic linkages observed in the sea slug mucus were (1→4)–Gal (42.2 mol %), followed by (1→3,6)–Gal (9.2 mol %), and terminally linked Gal (t–Gal, 8.7 mol %) (Table 1).
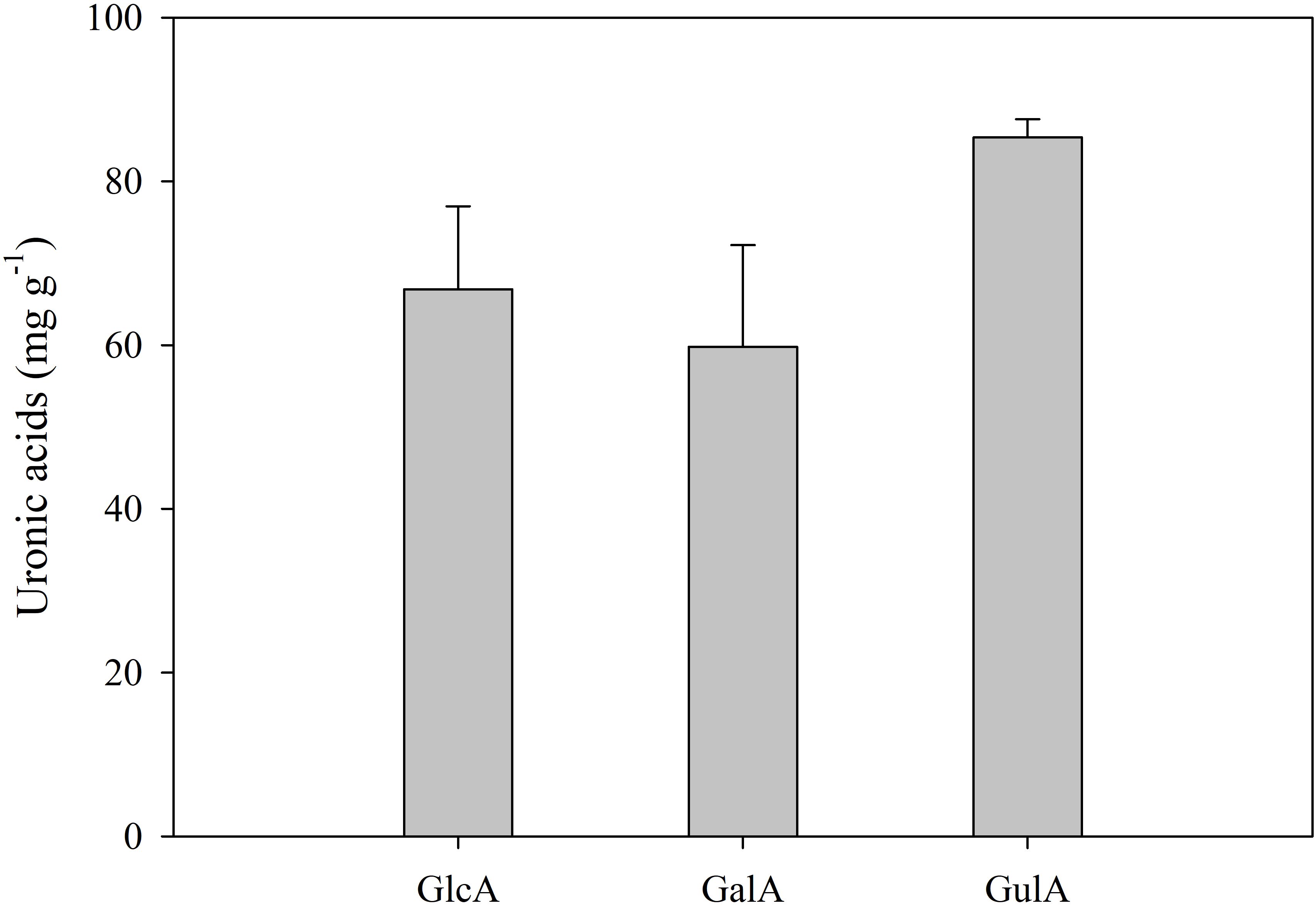
Figure 1. Concentration (mg g–1) of the uronic acids present in the mucus of the sea slug Elysia crispata: GlcA, glucuronic acid; GalA, galacturonic acid; GulA, Guluronic acid. Average ± standard deviation (n=3).
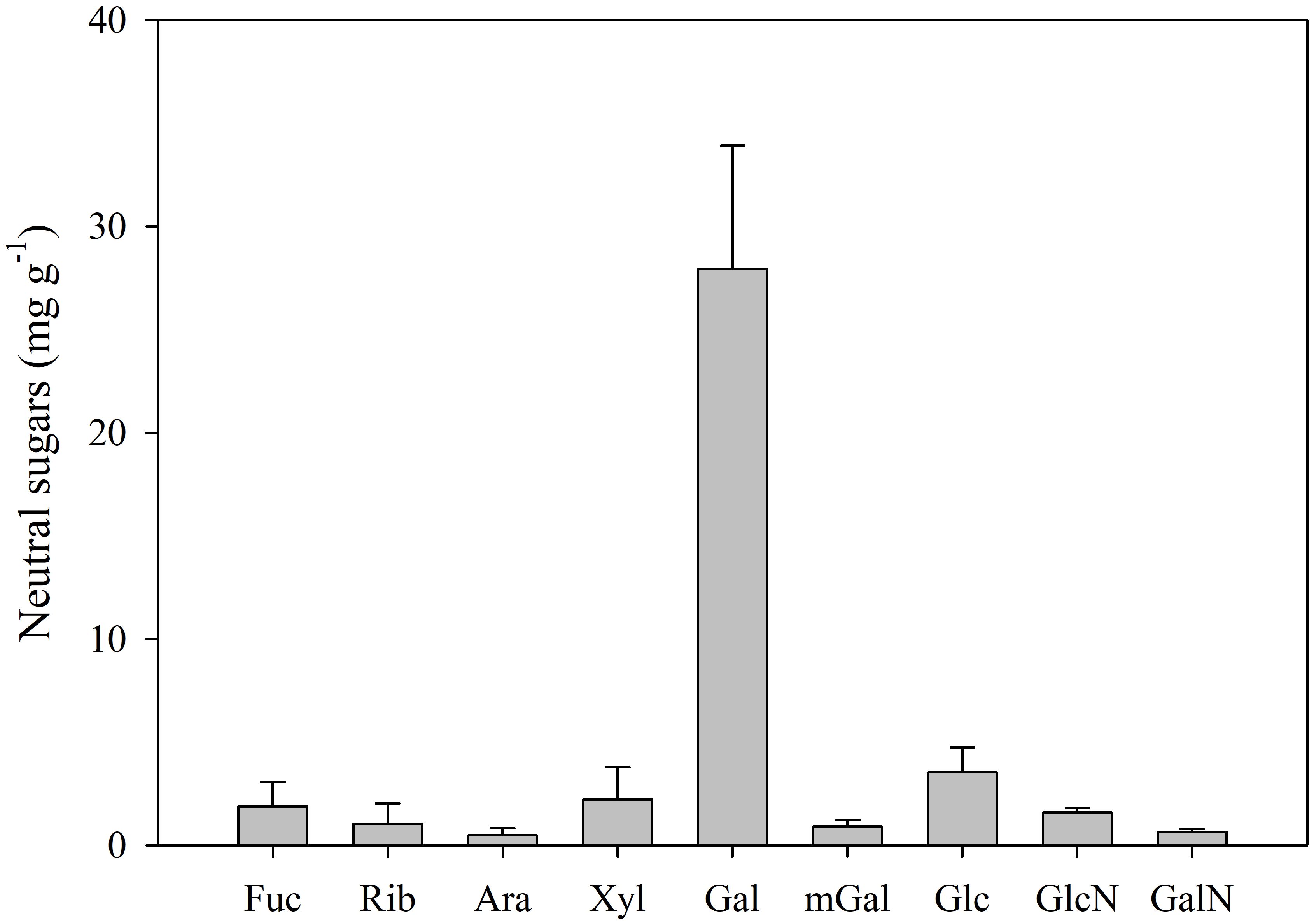
Figure 2. Concentration (mg g–1) of the neutral monosaccharides present in the mucus of the sea slug Elysia crispata: Fuc, fucose; Rib, ribose; Ara, arabinose; Xyl, xylose; Gal, galactose; mGal, 3-O-methylgalactose; Glc, glucose; GlcN, glucosamine; GalN, galactosamine. Average ± standard deviation (n=5).
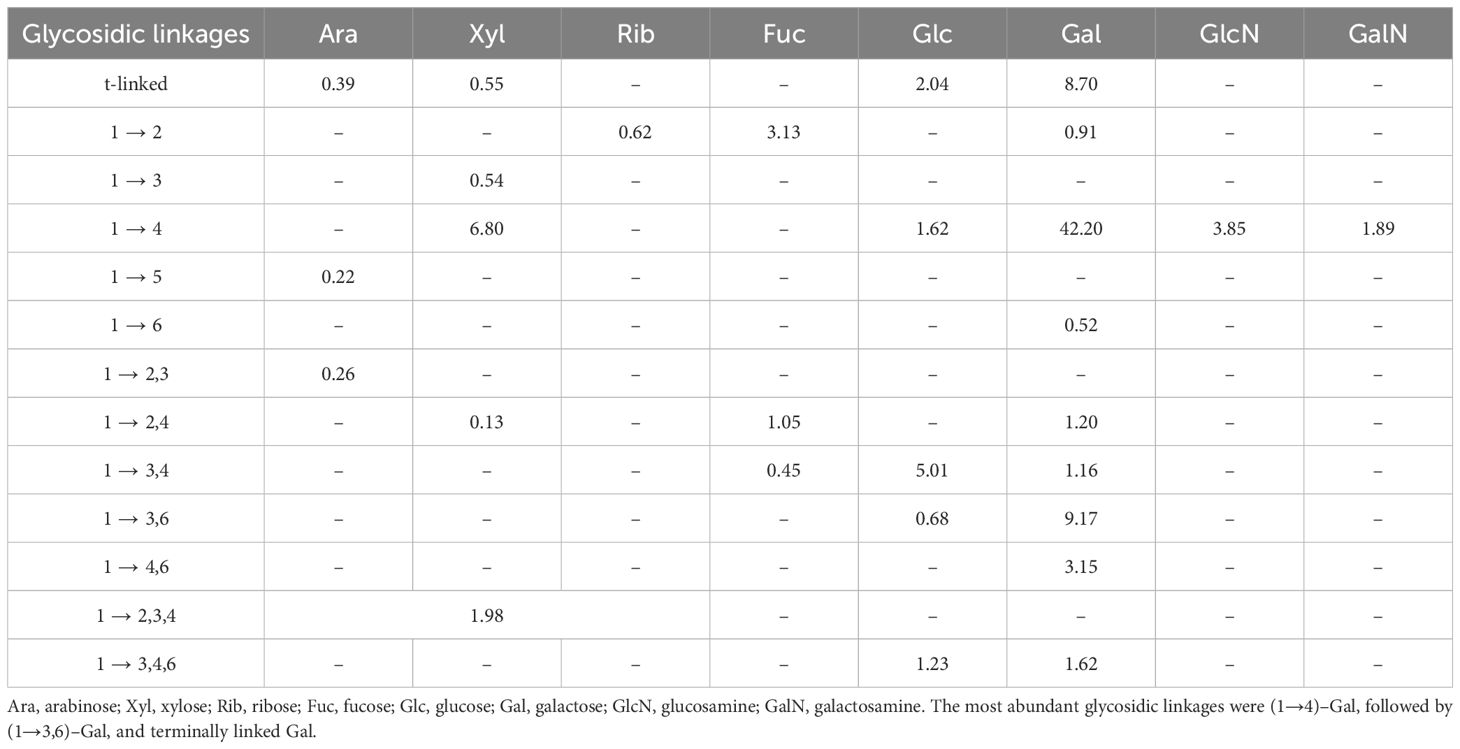
Table 1. Average molar percentage of glycosidic linkages in the carbohydrates of the mucus of the sea slug Elysia crispata.
Incorporation of 13C and 15N in the tissues of the sea slug E. crispata is depicted in Figure 3. There was a significant (F2,12 = 23.375, p< 0.001) effect of the treatment on 13C incorporation in the sea slug tissues. Individuals incubated in 13C-enriched ASW for 12 h showed an increase in At% 13C only when exposed to light. There were no significant (p=0.982) differences between At% 13C in sea slug tissues incubated in labelled dark and in non-labelled light treatments. The At% 13C values for the sea slugs incubated in 13C-enriched ASW under light conditions was significantly higher (p<0.001) than under dark incubation (1.791 ± 0.322% and 1.104 ± 0.005%, respectively). There was also a significant (F2,12 = 22.319, p< 0.001) effect of the treatment on 15N incorporation in the sea slug tissues. Contrary to carbon, significant increase of At% 15N was observed in tissues of animals incubated in 15N-enriched ASW in dark conditions (Labelled dark treatment), when compared to natural abundance values obtained from sea slugs in the non-labelled light treatment. Nevertheless, individuals incubated in 15N-enriched ASW for 12 h in the presence of light, showed a significantly higher (p<0.05) incorporation of 15N than in dark conditions (At% 15N of 0.856 ± 0.194% and 0.659 ± 0.037%, respectively).
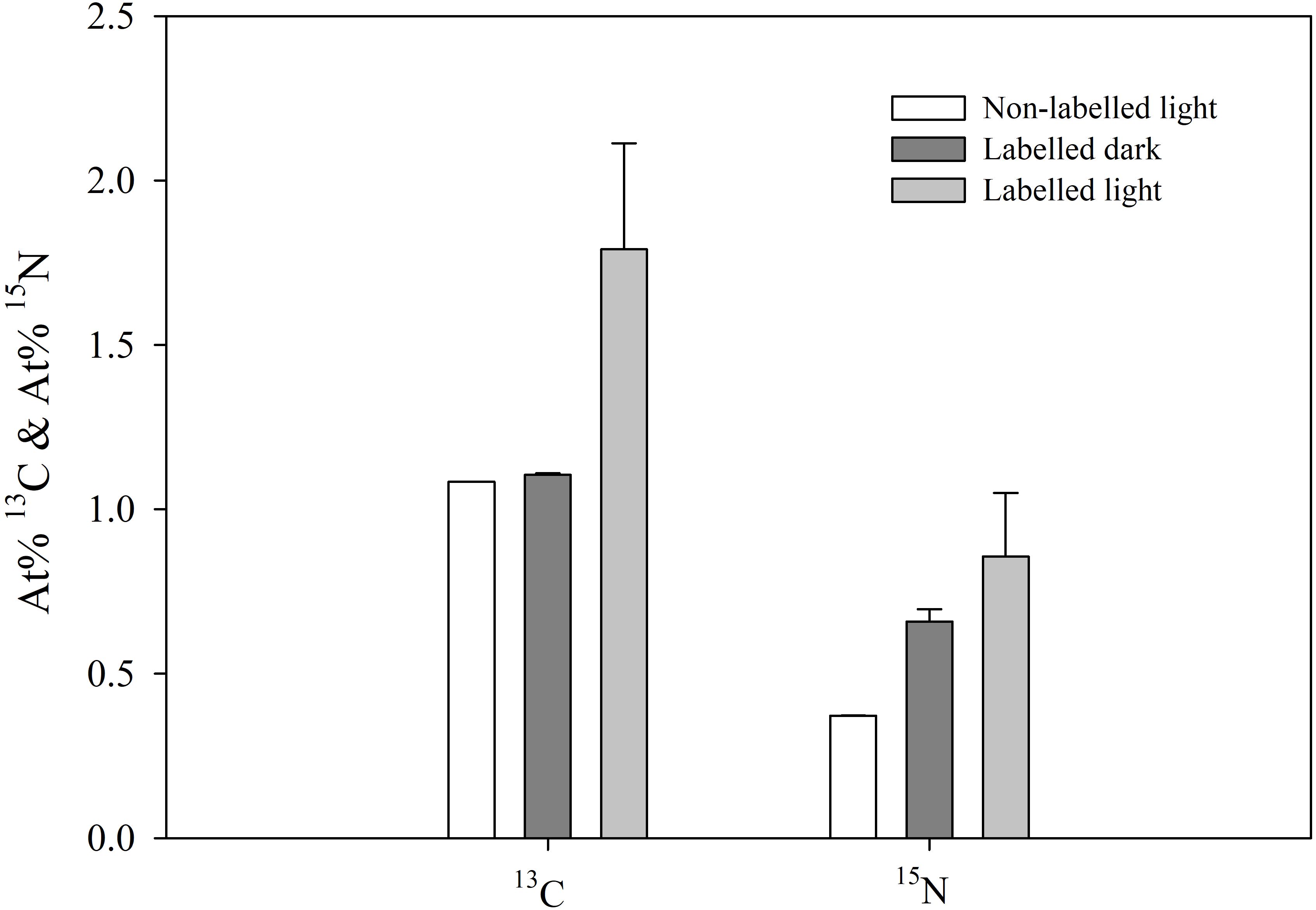
Figure 3. 13C and 15N incorporation in the tissues of the sea slug Elysia crispata. Atomic percentages (At%) of 13C and 15N after 12 h incubation of sea slugs in control (Non-labelled light) and 13C- and 15N-enriched sea water, during dark (Labelled dark) or light (Labelled light) conditions. 13C incorporation in the sea slug tissues occurs exclusively under light conditions. 15N incorporation occurs in both dark and light, with slightly higher levels found under the latter condition. Average ± standard deviation (n=5).
Besides quantifying 13C and 15N incorporation in the sea slugs, integration of these labelled elements in the secreted mucus of E. crispata was determined (Figure 4). There was a significant (F2,12 = 56.255, p< 0.001) effect of the treatment on 13C incorporation in the sea slug mucus. In accordance to results from animal tissue sample, increased values of At% 13C in the secreted mucus was only observed when animals were incubated in the light. There were no significant (p=0.988) differences between At% 13C values in sea slug mucus from labelled dark and non-labelled light treatments. The At% 13C values in the mucus for the labelled treatment under light conditions was significantly higher (p<0.001) than under dark incubation (1.794 ± 0.210% and 1.094 ± 0.001%, respectively). Labelled 15N-nitrogen incorporation in the secreted mucus was independent of the light treatment. No significant differences (p=0.649) were observed between the At% 15N values of the mucus of sea slugs incubated in labelled ASW under light and dark conditions (0.861 ± 0.130% and 0.807 ± 0.098%, respectively).
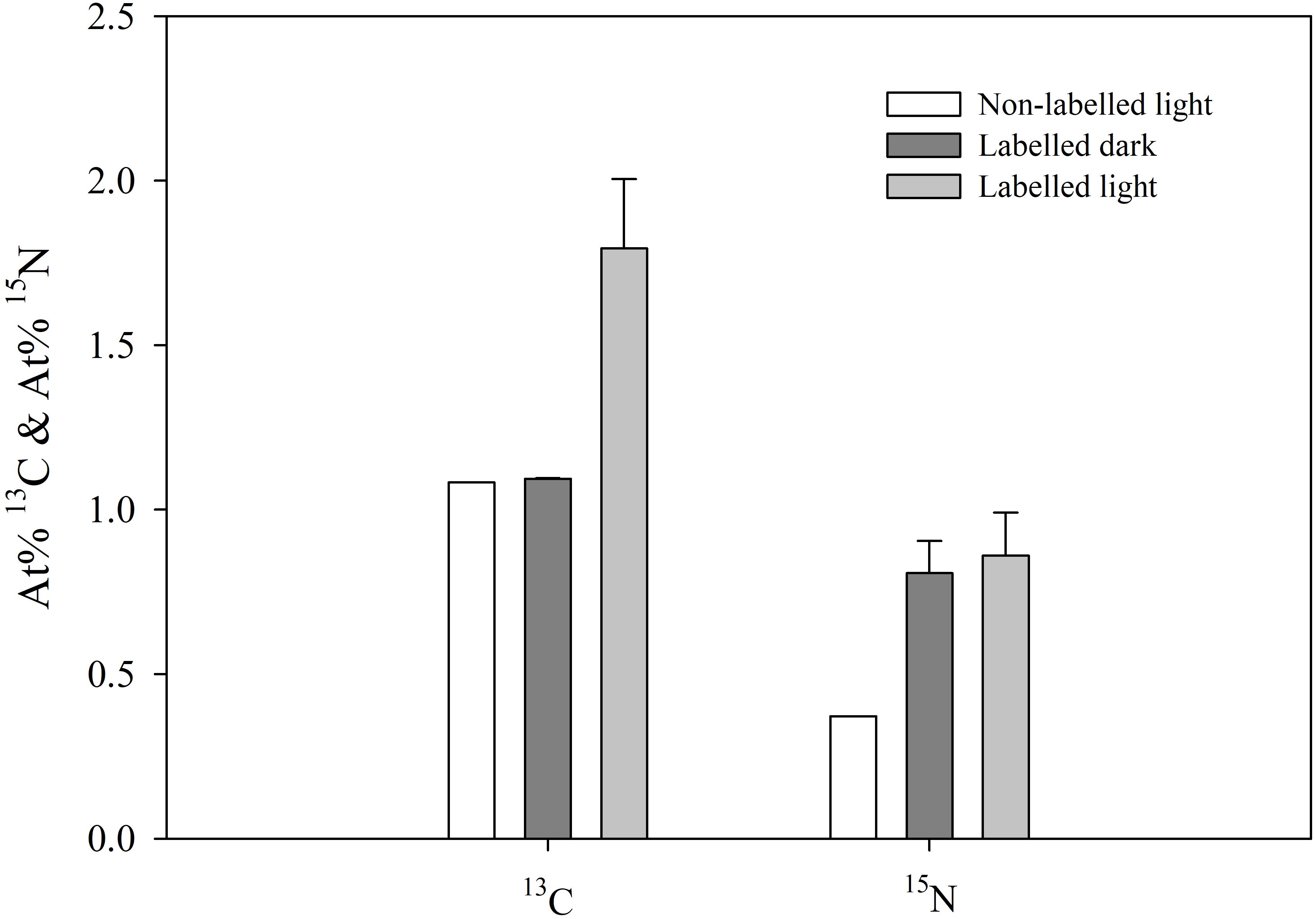
Figure 4. 13C and 15N incorporation in the mucus secreted by the sea slug Elysia crispata. Atomic percentages (At%) of 13C and 15N after 12 h incubation of sea slugs in control (Non-labelled light) and 13C- and 15N-enriched sea water, during dark (Labelled dark) or light (Labelled light) conditions. 13C incorporation in the mucus occurs exclusively under light conditions. 15N incorporation occurs at similar levels under both dark and light. Average ± standard deviation (n=5).
All the identified neutral sugars present in the mucus were shown to be 13C-enriched in the presence of light, although at different levels (Figure 5). In the dark, confirming that inorganic labelled carbon was not incorporated, At% 13C was very stable for all monosaccharides, ranging from 1.056 to 1.071%, corresponding to the overall natural abundance of 13C. In the light, levels of 13C-enrichment were lower for xylose (1.119 ± 0.001%), ribose (1.183 ± 0.018%), glucosamine (1.168 ± 0.025%), and galactosamine (1.172 ± 0.077%), whereas higher incorporation of labelled carbon was observed for glucose (1.551 ± 0.022%), galactose (1.823 ± 0.107%), fucose (1.843 ± 0.136%), and particularly 3-O-methylgalactose (3.135 ± 0.454%).
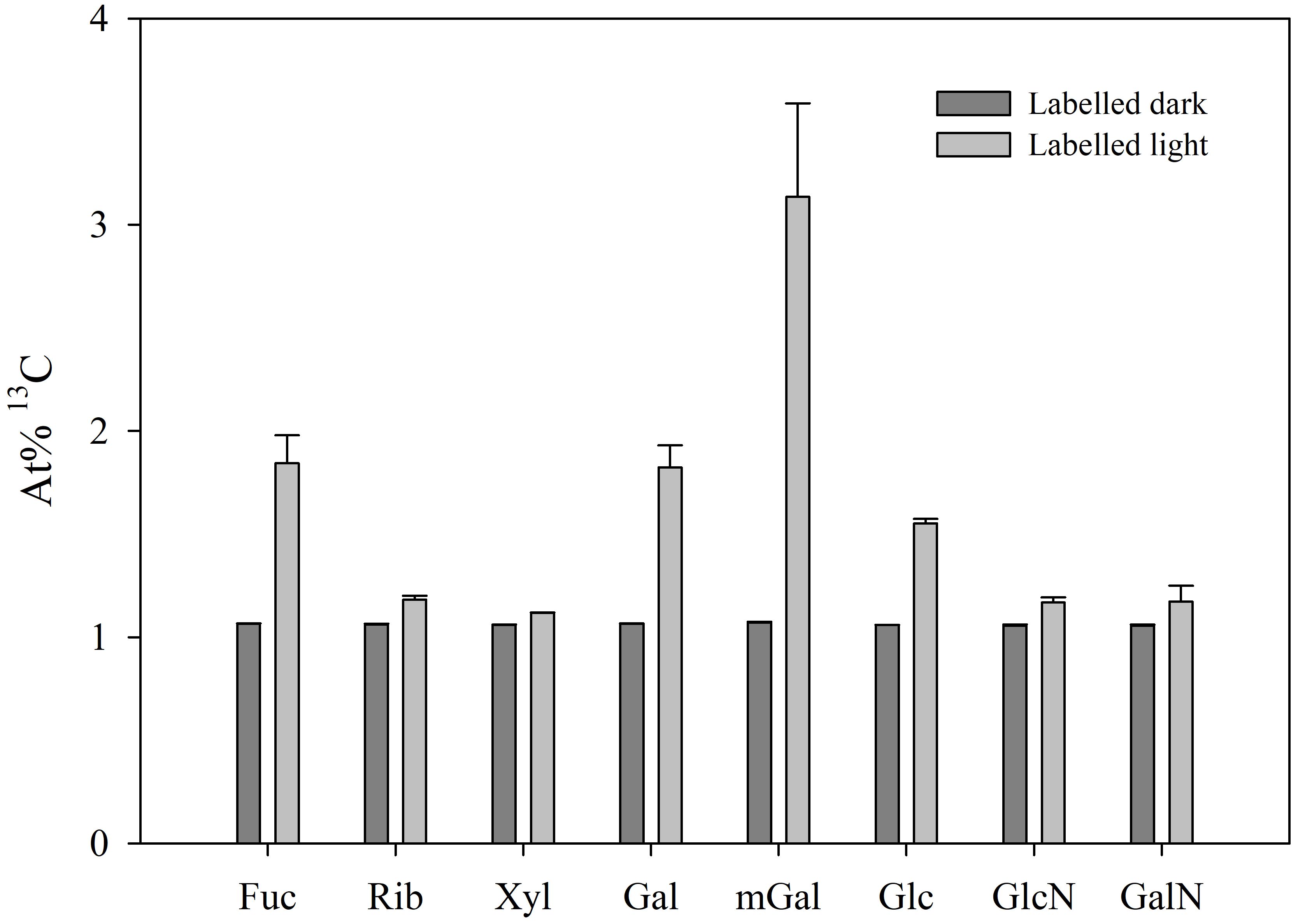
Figure 5. 13C incorporation in neutral sugars of the mucus secreted by the sea slug Elysia crispata. Atomic percentages (At%) of 13C after 12 h incubation of sea slugs in 13C-enriched sea water during dark (Labelled dark) or light (Labelled light) conditions. Fuc, fucose; Rib, ribose; Xyl, xylose; Gal, galactose; mGal, 3-O-methylgalactose; Glc, glucose; GlcN, glucosamine; GalN, galactosamine. 13C incorporation was observed in the light for all neutral sugars present in E. crispata mucopolysaccharides, showing particular high levels in 3-O-methylgalactose. Average ± standard deviation (n=3).
4 Discussion
4.1 Carbohydrate composition
Analysis of hydrolysates of mucus polysaccharides secreted by the tropical E. crispata showed a more diverse composition when compared with previous studies on sacoglossan sea slugs (Trench et al., 1970; 1972), including three uronic acids (guluronic, glucuronic, and galacturonic acids) and nine different neutral sugars (galactose, methyl-galactose, glucose, xylose, fucose, ribose, glucosamine, galactosamine, and arabinose). E. crispata mucus was previously reported to contain glucose, galactose, glucosamine, galactosamine and an unidentified pentose, while hydrolysates of mucus secreted by E. diomedea contained glucose, galactose, possible traces of glucosamine and an unidentified pentose (Trench et al., 1972). The overall carbohydrate composition of the mucus of E. crispata was comparable to that secreted by different scleractinian corals (Wild et al., 2010; Hadaidi et al., 2019).
Galactose stands out as the primary neutral sugar found within the mucus of E. crispata. This prevalence suggests that galactans likely form the bulk of the polysaccharide composition in the mucus of this sea slug. Analysis of the glycosidic linkages reveals that (1→4)–Gal linkages are the predominant type, providing further evidence for the presence of galactans (Delattre et al., 2011). The remaining neutral sugars identified in the mucus of E. crispata likely contribute to the structural diversity of galactans present, influencing their composition and functional activities (Delattre et al., 2011). Examining the cell wall polysaccharides extracted from the macroalga Bryopsis plumosa, in this case the chloroplast donor of E. crispata, Ciancia et al. (2012) identified a sulfated galactan with a linear backbone of galactose units as the major structure. The mucus of E. crispata contained approximately 5% sulfur, a significant finding when considered alongside the monosaccharide data and the sample’s marine origin. Sulfated galactans are exclusively found in marine organisms, functioning as structural components of the extracellular matrix of marine invertebrates (Pomin and Mourão, 2008; Pomin, 2010). These macromolecules are strongly anionic, facilitating electrostatic interactions with other compounds. This unique property enables sulfated galactans to form highly specific complexes with targeted proteins, triggering their biological effects (Pomin, 2010). The relevance of sulfated galactans in the mucus of E. crispata could be related to protection against pathogens, as several studies have identified strong antiviral activities associated to these molecules (Ohta et al., 2009; Wei et al., 2022).
4.2 Incorporation of carbon
The sea slug E. crispata showed significant incorporation of 13C in both the sea slug tissues and in the secreted mucus when light-incubated, while animals incubated in the dark showed no relevant carbon incorporation. Animals that were incubated in labelled ASW were rinsed with non-labelled ASW before mucus collection and dipped in distilled water before sampling (see Methods section), but it is possible that some 13C and 15N remained attached to the sea slug bodies. Although interference of this non-assimilated elements cannot be ruled out, it was not significant. Levels of 13C in dark-incubated labelled sea slugs were very similar to that of light-incubated animals in non-labelled water, but values were slightly higher in the former (average values of 1.10 and 1.08, respectively). This difference could be explained by this potential contamination and not by carbon assimilation in the dark. Light-dependent incorporation of carbon indicates important roles for kleptoplast photosynthesis in both sea slug carbon metabolism and mucus production. A major advantage of the partially photosynthetic lifestyle of these sea slugs is the capacity to survive periods of macroalgal food shortage (Giménez-Casalduero and Muniain, 2008; Yamamoto et al., 2013). Furthermore, kleptoplast-bearing sea slugs may allocate additional resources to reproduction and consequently display higher fecundities (Cartaxana et al., 2021).
Using 14C, Trench et al. (1969) showed carbon incorporation in several tissues of the sea slugs E. crispata and E. diomedea, including the mucus-secreting pedal gland, which functions in secreting a carpet of mucus over which the animal moves. This was the first evidence that kleptoplast photosynthesis could provide substrates for mucus synthesis by the sea slugs. Following on this work, Trench et al. (1970) estimated that 5 to 10% of the carbon fixed by kleptoplast photosynthesis in the sea slug Plakobranchus ocellatus was later detected in the secreted mucus. After acid hydrolysis of the mucus, most of the 14C was found in galactose and glucose (Trench et al., 1970). Later, the products of photosynthetic 14C fixation by kleptoplasts of E. crispata and E. diomedea were compared with those of their chloroplast donor, the siphonous marine alga Caulerpa sertularioides (Trench et al., 1972). Analyzing the polysaccharide fractions, the authors found differences in the monosaccharide ratios of the sea slugs and the alga. These ratio discrepancies indicate differences in the utilization of products of chloroplast photosynthesis by plant and animal tissues. Enzymes for interconversion of hexoses occur in animals as well as plants, so chloroplast products may have been converted by the animal (Trench et al., 1970). In fact, while the major soluble labelled monosaccharide in E. crispata sea slug tissues was galactose, the main labelled component of mucus was glucose, suggesting sugar interconversion before or during mucus synthesis (Trench et al., 1972). In a comprehensive analysis using gel electrophoresis and HPLC-MS/MS, 306 proteins were identified in the mucus secretions of E. crispata (Lopes et al., 2024). Regarding the molecular function, the highest number of proteins identified were associated with carbohydrate-derived metabolic processes, including epimerases catalyzing the reversible conversion of glucose to galactose (Lopes et al., 2024).
In the current study, we present additional data using 13C labelling showing that the incorporation of carbon derived from kleptoplast photosynthesis occurred in all neutral sugars present in E. crispata mucopolysaccharides. Carbon incorporation via stolen chloroplasts was particularly relevant for 3-O-methylgalactose (which possesses an additional carbon from its methyl group), galactose, and fucose. The preferential assimilation of 13C in the form of 3-O-methylgalactose is yet another evidence for the importance of galactans in the composition and function of the mucus.
4.3 Incorporation of nitrogen
The sea slug E. crispata showed significant incorporation of the 15N isotope in both the sea slug tissues and in the secreted mucus when light-incubated. Under dark conditions, contrary to carbon acquisition, animals showed 15N incorporation in the sea slug tissues, despite at a lower rate than in the light exposed conditions. This is accordance with previous studies using high-resolution secondary ion mass spectrometry (NanoSIMS) isotopic imaging of the sea slugs Elysia viridis and Elysia timida to visualize incorporation of 15N in different sea slug tissues (Cruz et al., 2020; Cartaxana et al., 2021). Teugels et al. (2008) investigated 15N incorporation in the tissues of the sea slug E. viridis exposed to different inorganic nitrogen substrates, under both light and dark conditions. The authors reported light-dependent nitrogen assimilation in green specimens with functional kleptoplasts, but not in bleached conspecifics (i.e. with degraded kleptoplasts). Furthermore, using inhibitors for specific potentially involved enzymatic pathways, authors confirmed that at least part of nitrogen assimilation in E. viridis takes place through the kleptoplast glutamine synthetase (GS) - glutamate synthetase (GOGAT) activity. In this pathway, by which inorganic nitrogen is converted into organic nitrogen, GS catalyses the ATP-dependent synthesis of glutamine from ammonium and glutamate, while GOGAT catalyses the conversion of glutamine and 2-oxoglutarate to yield two molecules of glutamate, one of which participates in further ammonium assimilation via GS, while the other is used as a nitrogen donor for the production of nitrogen-containing molecules (Liu et al., 2022). It has been hypothesized that the kleptoplasts of the foraminifera Nonionella stella are used to meet the nitrogen requirements of the host by assimilating inorganic nitrogen (Grzymski et al., 2002). More recently, high levels of GS and GOGAT gene expression were shown for this kleptoplastidic foraminifera, showing that ammonium assimilation occurs likely via the GS-GOGAT pathway (Gomaa et al., 2021). Additional nitrogen incorporation may also take place through the glutamine dehydrogenase (GDH) pathway in the mitochondria (Teugels et al., 2008). Therefore, the enhanced 15N-incorporation into the animal tissue in light conditions, observed in our work, is expected to reflect the contribution of kleptoplasts in the overall sea slugs’ nitrogen assimilation. It is interesting to note that levels of 15N incorporation in the secreted mucus were similar in the mucus of light and dark incubated animals. However, analysis on the contributions of the different enzymatic pathways would require the use of specific inhibitors (Teugels et al., 2008).
Proteins are an important component of the mucus secreted by E. crispata (Lopes et al., 2024), confirmed by the mucus nitrogen content and the levels of 15N incorporation found in this study. De novo protein synthesis has been shown to occur for plastid-encoded membrane proteins in the sea slug Elysia chlorotica, even after several months of starvation (Mujer et al., 1996). This is highly unlikely without an external supply of nitrogen (Teugels et al., 2008). During periods of food shortage, kleptoplasts may not only provide essential energy and carbon skeletons for sacoglossan sea slugs, but could also play a role in nitrogen incorporation and protein synthesis.
5 Conclusion
This study shows that inorganic carbon dissolved in the water is (i) taken up by the kleptoplasts of the sea slug E. crispata, located in the digestive diverticula; (ii) used for carbon fixation; (iii) translocated as organic carbon to kleptoplast-free tissues; and (iv) incorporated in the secreted mucopolysaccharides. While nitrogen was also incorporated in the secreted mucus, levels were similar for light and dark-incubated sea slugs. This study provides evidence supporting the relevant role of photosynthesis to sea slug mucus production. Mucus is crucial for the sea slug, as it serves as both a protective barrier against predators and pathogens and also assists in locomotion by reducing friction. Additionally, it may play a role in reproduction by aiding in egg adhesion or creating a suitable environment for gametes. Further research is necessary to fully assess the role of kleptoplasts in nitrogen incorporation in sacoglossan sea slugs and other kletptoplastidic organisms (dinoflagellates, ciliates, foraminifera, and flatworms).
Data availability statement
The raw data supporting the conclusions of this article will be made available by the authors, without undue reservation.
Ethics statement
Ethical approval was not required for the study involving animals in accordance with the local legislation and institutional requirements because it was conducted with non-cephalopod invertebrates. Animals were initially purchased from Tropical Marine Center (TMC Iberia, Lisbon, Portugal) and reared in the laboratory for several generations. Animals used in this work were maintained in life-support systems in controlled and optimal conditions to ensure maximum fitness. A mild stress treatment was applied for mucus collection that allowed all specimens to recover fully.
Author contributions
DL: Conceptualization, Investigation, Visualization, Writing – original draft. PC: Conceptualization, Formal analysis, Supervision, Validation, Visualization, Writing – review & editing. SF: Investigation, Writing – review & editing. MS: Investigation, Writing – review & editing. MN: Investigation, Methodology, Writing – review & editing. JB: Methodology, Validation, Writing – review & editing. CN: Methodology, Resources, Supervision, Validation, Writing – review & editing. SC: Conceptualization, Funding acquisition, Methodology, Project administration, Resources, Supervision, Validation, Writing – review & editing.
Funding
The author(s) declare that financial support was received for the research and/or publication of this article. This work was supported by the European Research Council (ERC) under the European Union’s Horizon 2020 research and innovation programme, grant agreement no. 949880 to SC (DOI:10.3030/949880), and by Fundação para a Ciência e Tecnologia, grants 2020.08672 BD to DL, 2020.03278.CEECIND to SC (DOI:10.54499/2020.03278.CEECIND/CP1589/CT0012), CEECIND/01434/2018 to PC (DOI:10.54499/CEECIND/01434/2018/CP1559/CT0003), UID + LA/P/0094/2020 to CESAM, UIDB/50006/2020 (DOI:10.54499/UIDB/50006/2020), UIDP/50006/2020 (DOI:10.54499/UIDP/50006/2020), and LA/P/0008/2020 (DOI:10.54499/LA/P/0008/2020) to LAQV/REQUIMTE, and UIDB/50011/2020 (DOI 10.54499/UIDB/50011/2020), UIDP/50011/2020 (DOI 10.54499/UIDP/50011/2020) and LA/P/0006/2020 (DOI 10.54499/LA/P/0006/2020) to CICECO.
Acknowledgments
We thank Ana Resende for help in culturing Bryopsis sp.
Conflict of interest
The authors declare that the research was conducted in the absence of any commercial or financial relationships that could be construed as a potential conflict of interest.
Generative AI statement
The author(s) declare that no Generative AI was used in the creation of this manuscript.
Publisher’s note
All claims expressed in this article are solely those of the authors and do not necessarily represent those of their affiliated organizations, or those of the publisher, the editors and the reviewers. Any product that may be evaluated in this article, or claim that may be made by its manufacturer, is not guaranteed or endorsed by the publisher.
References
Bastos R., Coelho E., Coimbra M. A. (2015). Modifications of Saccharomyces pastorianus cell wall polysaccharides with brewing process. Carbohydr. Polym. 124, 322–330. doi: 10.1016/j.carbpol.2015.02.031
Blakeney A. B., Harris P. J., Henry R. J., Stone B. A. (1983). A simple and rapid preparation of alditol acetates for monosaccharide analysis. Carbohydr. Res. 113, 291–299. doi: 10.1016/0008-6215(83)88244-5
Box G. E. P. (1954). Some theorems on quadratic forms applied in the study of analysis of variance problems, I: Effect of inequality of variance in the one-way classification. Ann. Math Statist 25, 290–302. doi: 10.1214/aoms/1177728786
Cartaxana P., Morelli L., Cassin E., Havurinne V., Cabral M., Cruz S. (2023). Prey species and abundance affect growth and photosynthetic performance of the polyphagous sea slug Elysia crispata. R Soc. Open Sci. 10, 230810. doi: 10.1098/rsos.230810
Cartaxana P., Rey F., LeKieffre C., Lopes D., Hubas C., Spangenberg J. E., et al. (2021). Photosynthesis from stolen chloroplasts can support sea slug reproductive fitness. Proc. R Soc. B: Biol. Sci. 288, 20211779. doi: 10.1098/rspb.2021.1779
Cartaxana P., Trampe E., Kühl M., Cruz S. (2017). Kleptoplast photosynthesis is nutritionally relevant in the sea slug Elysia viridis. Sci. Rep. 7, 7714. doi: 10.1038/s41598-017-08002-0
Christa G., Wescott L., Schäberle T. F., König G. M., Wägele H. (2013). What remains after 2 months of starvation? Analysis of sequestered algae in a photosynthetic slug, Plakobranchus ocellatus (Sacoglossa, Opisthobranchia), by barcoding. Planta 237, 559–572. doi: 10.1007/s00425-012-1788-6
Ciancia M., Alberghina J., Arata P. X., Benavides H., Leliaert F., Verbruggen H., et al. (2012). Characterization of cell wall polysaccharides of the coenocytic green seaweed Bryopsis plumosa (Bryopsidaceae, Chlorophyta) from the Argentine coast. J. Phycol. 48, 326–335. doi: 10.1111/j.1529-8817.2012.01131.x
Corradini C., Canali G., Cavazza A., Delfino D., Teti G. (1998). Compositional analysis of the major capsular polysaccharides of Cryptococcus neoformans by high-performance anion-exchange chromatography with pulsed amperometric detection (HPAEC-PAD). J. Liq. Chromatog. Relat. Technol. 21, 941–951. doi: 10.1080/10826079808005860
Cruz S., Cartaxana P. (2022). Kleptoplasty: getting away with stolen chloroplasts. PloS Biol. 20, e3001857. doi: 10.1371/journal.pbio.3001857
Cruz S., LeKieffre C., Cartaxana P., Hubas C., Thiney N., Jakobsen S., et al. (2020). Functional kleptoplasts intermediate incorporation of carbon and nitrogen in cells of the Sacoglossa sea slug Elysia viridis. Sci. Rep. 10, 10548. doi: 10.1038/s41598-020-66909-7
Delattre C., Andrea F., Michaud P. (2011). Galactans: an overview of their most important sourcing and applications as natural polysaccharides. Braz. Arch. Biol. Techn. 54, 1075–1092. doi: 10.1590/S1516-89132011000600002
Evertsen J., Burghardt I., Johnsen G., Wägele H. (2007). Retention of functional chloroplasts in some sacoglossans from the Indo-Pacific and Mediterranean. Mar. Biol. 151, 2159–2166. doi: 10.1007/s00227-007-0648-6
Giménez-Casalduero F., Muniain C. (2008). The role of kleptoplasts in the survival rates of Elysia timida (Risso 1818): (Sacoglossa: Opisthobranchia) during periods of food shortage. J. Exp. Mar. Biol. Ecol. 357, 181–187. doi: 10.1016/j.jembe.2008.01.020
Gomaa F., Utter D. R., Powers C., Beaudoin D. J., Edgcomb V. P., Filipsson H. L., et al. (2021). Multiple integrated metabolic strategies allow foraminiferan protists to thrive in anoxic marine sediments. Sci. Adv. 7, eabf1586. doi: 10.1126/sciadv.abf1586
Grzymski J., Schofield O. M., Falkowski P. J., Bernhard J. M. (2002). The function of plastids in the deep-sea benthic foraminifer, Nonionella stella. Limnol. Oceanogr. 47, 1569–1580. doi: 10.4319/lo.2002.47.6.1569
Hadaidi G., Gegner H. M., Ziegler M., Voolstra C. R. (2019). Carbohydrate composition of mucus from scleractinian corals from the central Red Sea. Coral Reefs. 38, 21–27. doi: 10.1007/s00338-018-01758-5
Händeler K., Grzymbowski Y. P., Krug P. J., Wägele H. (2009). Functional chloroplasts in metazoan cells – a unique evolutionary strategy in animal life. Front. Zool. 6, 28. doi: 10.1186/1742-9994-6-28
Harrison P. J., Waters R. E., Taylor F. J. R. (1980). A broad spectrum artificial sea water medium for coastal and open ocean phytoplankton. J. Phycol. 16, 28–35. doi: 10.1111/j.0022-3646.1980.00028.x
Hinde R., Smith D. C. (1972). Persistence of functional chloroplasts in Elysia viridis (Opisthobranchia, Sacoglossa). Nat. New Biol. 239, 30–31. doi: 10.1038/newbio239030a0
Kawaguti S., Yamasu (1965). Electron microscopy on the symbiosis between an elysioid gastropod and chloroplasts of a green alga. Biol. J. Okayama Univ 11, 57–65.
Liu X., Hu B., Chu C. (2022). Nitrogen assimilation in plants: current status and future prospects. J. Genet. Genom. 49, 394–404. doi: 10.1016/j.jgg.2021.12.006
Lopes D., Aveiro S. S., Cruz S., Cartaxana P., Domingues P. (2024). Proteomic analysis of the mucus of the photosynthetic sea slug Elysia crispata. J. Proteomics 294, 105087. doi: 10.1016/j.jprot.2024.105087
Lopes D., Cruz S., Martins P., Ferreira S., Nunes C., Domingues P., et al. (2022). Sea slug mucus production is supported by photosynthesis of stolen chloroplasts. Biology 11, 1207. doi: 10.3390/biology11081207
Middlebrooks M. L., Bell S. S., Curtis N. E., Pierce S. K. (2014). Atypical plant–herbivore association of algal food and a kleptoplastic sea slug (Elysia clarki) revealed by DNA barcoding and field surveys. Mar. Biol. 161, 1429–1440. doi: 10.1007/s00227-014-2431-9
Middlebrooks M. L., Curtis N. E., Pierce S. K. (2019). Algal sources of sequestered chloroplasts in the sacoglossan sea slug Elysia crispata vary by location and ecotype. Biol. Bull. 236, 88–96. doi: 10.1086/701732
Mujer C. V., Andrews D. L., Manhart J. R., Pierce S. K., Rumpho M. E. (1996). Chloroplast genes are expressed during intracellular symbiotic association of Vaucheria litorea plastids with the sea slug Elysia chlorotica. Proc. Natl. Acad. Sci. U.S.A. 93, 12333–12338. doi: 10.1073/pnas.93.22.12333
Ohta Y., Lee J. B., Hayashi K., Hayashi T. (2009). Isolation of sulfated galactan from Codium fragile and its antiviral effect. Biol. Pharm. Bull. 32, 892–898. doi: 10.1248/bpb.32.892
Passos C. P., Coimbra M. A. (2013). Microwave superheated water extraction of polysaccharides from spent coffee grounds. Carbohydr. Polym. 94, 626–633. doi: 10.1016/j.carbpol.2013.01.088
Pelletreau K., Worful J., Sarver K., Rumpho M. (2012). Laboratory culturing of Elysia chlorotica reveals a shift from transient to permanent kleptoplasty. Symbiosis 58, 221–232. doi: 10.1007/s13199-012-0192-0
Pomin V. H. (2010). Structural and functional insights into sulfated galactans: a systematic review. Glycoconj. J. 27, 1–12. doi: 10.1007/s10719-009-9251-z
Pomin V. H., Mourão P. A. S. (2008). Structure, biology, evolution, and medical importance of sulfated fucans and galactans. Glycobiology 18, 1016–1027. doi: 10.1093/glycob/cwn085
Rumpho M. E., Summer E. J., Manhart J. R. (2000). Solar-powered sea slugs. Mollusc/algal chloroplast symbiosis. Plant Physiol. 123, 29–38. doi: 10.1104/pp.123.1.29
Schmitt V., Händeler K., Gunkel S., Escande M. L., Menzel D., Gould S. B., et al. (2014). Chloroplast incorporation and long-term photosynthetic performance through the life cycle in laboratory cultures of Elysia timida (Sacoglossa, Heterobranchia). Front. Zool. 11, 5. doi: 10.1186/1742-9994-11-5
Smith A. M. (2002). The structure and function of adhesive gels from invertebrates. Integr. Comp. Biol. 42, 1164–1171. doi: 10.1093/icb/42.6.1164
Smith A. M., Morin M. C. (2002). Biochemical differences between trail mucus and adhesive mucus from marsh periwinkle snails. Biol. Bull. 203, 338–346. doi: 10.2307/1543576
Teugels B., Bouillon S., Veuger B., Middelburg J., Koedam N. (2008). Kleptoplasts mediate nitrogen acquisition in the sea slug Elysia viridis. Aquat. Biol. 4, 15–21. doi: 10.3354/ab00092
Trench R. K., Boyle J. E., Smith D. C. (1973). The association between chloroplasts of Codium fragile and the mollusc Elysia viridis. II. Chloroplast ultrastructure and photosynthetic carbon fixation in E. viridis. Proc. R Soc. Lond B. 184, 63–81. doi: 10.1098/rspb.1973.0031
Trench R. K., Greene R. W., Bystrom B. G. (1969). Chloroplasts as functional organelles in animal tissues. J. Cell Biol. 42, 404–417. doi: 10.1083/jcb.42.2.404
Trench M. E., Trench R. K., Muscatine L. (1970). Utilization of photosynthetic products of symbiotic chloroplasts in mucus synthesis by Placobranchus ianthobaptus (Gould), Opisthobranchia, Sacoglossa. Comp. Biochem. Physiol. 37, 113–117. doi: 10.1016/0010-406X(70)90964-3
Trench R. K., Trench M. E., Muscatine L. (1972). Symbiotic chloroplasts; their photosynthetic products and contribution to mucus synthesis in two marine slugs. Biol. Bull. 142, 335–349. doi: 10.2307/1540236
Vieira S., Calado R., Coelho H., Serôdio J. (2009). Effects of light exposure on the retention of kleptoplastic photosynthetic activity in the sacoglossan mollusc Elysia viridis. Mar. Biol. 156, 1007–1020. doi: 10.1007/s00227-009-1144-y
Wei Q., Fu G., Wang K., Yang Q., Zhao J., Wang Y., et al. (2022). Advances in research on antiviral activities of sulfated polysaccharides from seaweeds. Pharmaceuticals 15, 581. doi: 10.3390/ph15050581
Wild C., Naumann M., Niggl W., Haas A. (2010). Carbohydrate composition of mucus released by scleractinian warm- and cold-water reef corals. Aquat. Biol. 10, 41–45. doi: 10.3354/ab00269
Yamamoto S., Hirano Y. M., Hirano Y. J., Trowbridge C. D., Akimoto A., Sakai A., et al. (2013). Effects of photosynthesis on the survival and weight retention of two kleptoplastic sacoglossan opisthobranchs. J. Mar. Biol. Assoc. U K. 93, 209–215. doi: 10.1017/S0025315412000628
Keywords: carbohydrate, chloroplast, kleptoplasty, isotope ratio mass spectrometry, mucus, Sacoglossa
Citation: Lopes D, Cartaxana P, Ferreira SS, Silva MI, Nunes M, Barata J, Nunes C and Cruz S (2025) Mucopolysaccharides secreted by the sea slug Elysia crispata incorporate carbon via kleptoplast photosynthesis. Front. Mar. Sci. 12:1580478. doi: 10.3389/fmars.2025.1580478
Received: 20 February 2025; Accepted: 28 March 2025;
Published: 25 April 2025.
Edited by:
Vikash Kumar, Central Inland Fisheries Research Institute (ICAR), IndiaReviewed by:
Sofia Priyadarsani Das, National Taiwan Ocean University, TaiwanSoumya Prasad Panda, Central Inland Fisheries Research Institute (ICAR), India
Copyright © 2025 Lopes, Cartaxana, Ferreira, Silva, Nunes, Barata, Nunes and Cruz. This is an open-access article distributed under the terms of the Creative Commons Attribution License (CC BY). The use, distribution or reproduction in other forums is permitted, provided the original author(s) and the copyright owner(s) are credited and that the original publication in this journal is cited, in accordance with accepted academic practice. No use, distribution or reproduction is permitted which does not comply with these terms.
*Correspondence: Sónia Cruz, c29uaWEuY3J1ekB1YS5wdA==