- 1Department of Ocean and Earth Sciences, Old Dominion University, Norfolk, VA, United States
- 2Marine Biological Laboratory, The Ecosystems Center, Woods Hole, MA, United States
- 3Marine Science Institute, The University of Texas at Austin, Port Aransas, TX, United States
Marine plastic pollution poses a significant and escalating threat to ocean ecosystems, with microbial colonization on plastics playing a critical role in determining their environmental fate. Despite the recognized importance of microbial biofilms, their composition, dynamics, and functional contributions on buoyant plastics remain poorly understood. In this study, we leveraged a novel integrative approach by simultaneously employing 16S, 18S, and ITS sequencing alongside microscopy, lipidomics, pigment analysis, adenosine triphosphate and biogenic silica quantification. This comprehensive methodology allowed for a thorough characterization of microbial growth and community dynamics on plastic debris in a mid-latitude, tidal sub-estuary. Our analyses consistently identified diatoms, due to their silica shells, as the sole ballast-bearing microorganisms within the biofilm. By examining the accumulation of biogenic silica on plastic surfaces, we evaluated the potential role of microbial biofilms in influencing plastic sinking behavior in the water column. Our findings indicate that the production of microbial ballast material alone is insufficient to significantly alter the buoyancy of most buoyant plastics larger than 15 or 40 μm (depending on shape) under nutrient-rich, undisturbed conditions. These results suggest that additional processes, such as metazoan colonization or sediment accumulation, are likely necessary to transport larger buoyant plastics to deeper waters.
1 Introduction
Plastics are a relatively recent anthropogenic addition to the environment, and understanding their interactions with biological systems is crucial to predicting their environmental fate and impact. Microbial biofilms play a central role in these interactions by colonizing plastic surfaces and influencing their physical, chemical, and biological properties. A biofilm consists of microbial life embedded in a matrix of biogenic secretions, including polysaccharides, proteins, lipids, and DNA, collectively referred to as extracellular polymeric substances (EPS) (Costerton et al., 1999; Hall-Stoodley et al., 2004; López et al., 2010). In physical terms, EPS exist as a spectrum ranging from fully dissolved substances to loose slimes and capsules (Decho, 2000), and in certain conditions can self-aggregate into transparent exopolymer particles (TEP) (Passow, 2000). EPS begin to adsorb onto submerged surfaces almost immediately, providing an essential foundation for microbial colonization (Bhagwat et al., 2021). These substrates offer food and shelter, giving microbes competitive advantages over their pelagic counterparts (Decho, 2000; Jefferson, 2004). Within hours of submersion, bacteria begin to colonize plastic surfaces (Lee et al., 2008), secreting additional EPS which further enhances particle stickiness and facilitates aggregation (Lagarde et al., 2016; Michels et al., 2018). Over time, larger unicellular organisms and multicellular eukaryotes join the biofilm community (Wahl, 1989), with colonization rates and compositions varying depending on season, location, and plastic type (Oberbeckmann et al., 2014). These biofilm communities, collectively referred to as the “plastisphere” (Zettler et al., 2013), are highly diverse and dynamic, with significant implications for the degradation, transport, and ecological impacts of marine plastic debris (MPD). Despite numerous studies on the taxonomic composition of the plastisphere, the functional roles of microbial communities within biofilms on MPD remains poorly characterized.
Biofilm formation plays a pivotal role in shaping the fate of MPD, influencing not only microbial colonization but also the physical properties of plastics, including buoyancy, which is a key factor in determining their distribution and transport in marine environments. A comparison of ocean surface plastic measurements with modeled plastic exports revealed that the surface contains one to two orders of magnitude less plastic than expected, suggesting that plastics are either exported to deeper waters or degraded into forms too small to detect (Cózar et al., 2014; Eriksen et al., 2014). While approximately half of all plastics produced by mass are negatively buoyant and naturally sink, the other half, including polyethylene (PE) and polypropylene (PP), are positively buoyant and are expected to remain at the ocean’s surface (Plastics Europe, 2023; Rabnawaz et al., 2017). Surprisingly, however, buoyant plastics have been discovered in significant quantities at great depths as both smaller microplastics (Abel et al., 2020, 2022; Galgani et al., 2022; Li et al., 2020; Pabortsava and Lampitt, 2020; Tekman et al., 2020) and larger macroplastics (Gündoğdu et al., 2017; Song et al., 2021), prompting investigations into the biological and physical mechanisms driving their vertical transport, which may include natural factors such as biofouling, aggregation with other sinking particles, ocean currents, water turbulence, absorption of water, and incorporation of oxygen during oxidation.
While many studies have looked at the possible impact of organisms on the buoyancy of MPD (Amaral-Zettler et al., 2021; Chen et al., 2019; Fazey and Ryan, 2016; Karkanorachaki et al., 2021; Lobelle and Cunliffe, 2011; Ye and Andrady, 1991), only a few aspects of biological control of buoyancy have been directly examined. Some biofilm-associated organisms produce biogenic minerals such as silica or calcium carbonate, which could contribute to the sinking of plastics (Armstrong et al., 2009; Sanders et al., 2010). In contrast, lipids produced within biofilms may increase buoyancy. Lipids are of interest in this study because of their potential role in buoyancy and their value as a taxonomic classification tool (De Carvalho and Caramujo, 2012; DeForest et al., 2016). Lipids are ubiquitous components of membranes used for lubrication and insulation (Koelmel et al., 2020), and they are also used for buoyancy control among some phytoplankton (Smayda, 1970). The dual role of biofilm components—both enhancing and reducing buoyancy—remains an as of yet unexplored area of research with regards to MPD. Cholesterol is a characteristic component of eukaryotic plasma membranes, while it is absent from prokaryotic ones (Mouritsen and Zuckermann, 2004), allowing for classification of the community through lipidomics. Despite these insights, a significant scientific gap persists regarding the extent to which microbial biofilm communities can increase the density of microplastics to facilitate their sinking as studies lack comprehensive quantitative or statistical analyses to evaluate the net effect of biofilms on plastic buoyancy. Based on theoretical considerations, Benner and Passow (2024) recently argued that microbial biofilm communities are unlikely to contribute to sufficient density increases to make otherwise buoyant plastics sink.
It is thus necessary to understand and quantify the members of this complex community under real world conditions. To achieve this, we conducted in situ incubations in a tidal sub-estuary and in laboratory experiments with estuarine water to examine biofilm development and succession on plastic surfaces. Microbial biomass was quantified using ATP, while photosynthetic pigments provided insight into the relative abundance of primary producers. Biogenic reactive silica was measured to assess the potential for biofilm-mediated ballasting, and metagenetic analyses, pigment composition, and lipidomics were used to characterize community succession.
2 Materials and methods
2.1 Sample incubation setting
The field study was conducted in the Lafayette River (Figure 1a), a mesohaline (5–18 ppt; US EPA, 2015) and eutrophic (Mulholland et al., 2022) tidal tributary of the southern Chesapeake Bay located within Norfolk, Virginia, USA (Figure 1b). The Chesapeake Bay ecosystem contains a diverse assemblage of diatoms throughout the year, as well as chlorophytes, cyanobacteria, cryptophytes, and dinoflagellates (Marshall et al., 2005). In situ incubations were conducted in the Lafayette River (36°52’46” N, 76°16’08” W), and in vitro incubations in our lab using unfiltered Lafayette River water (Figure 1a).
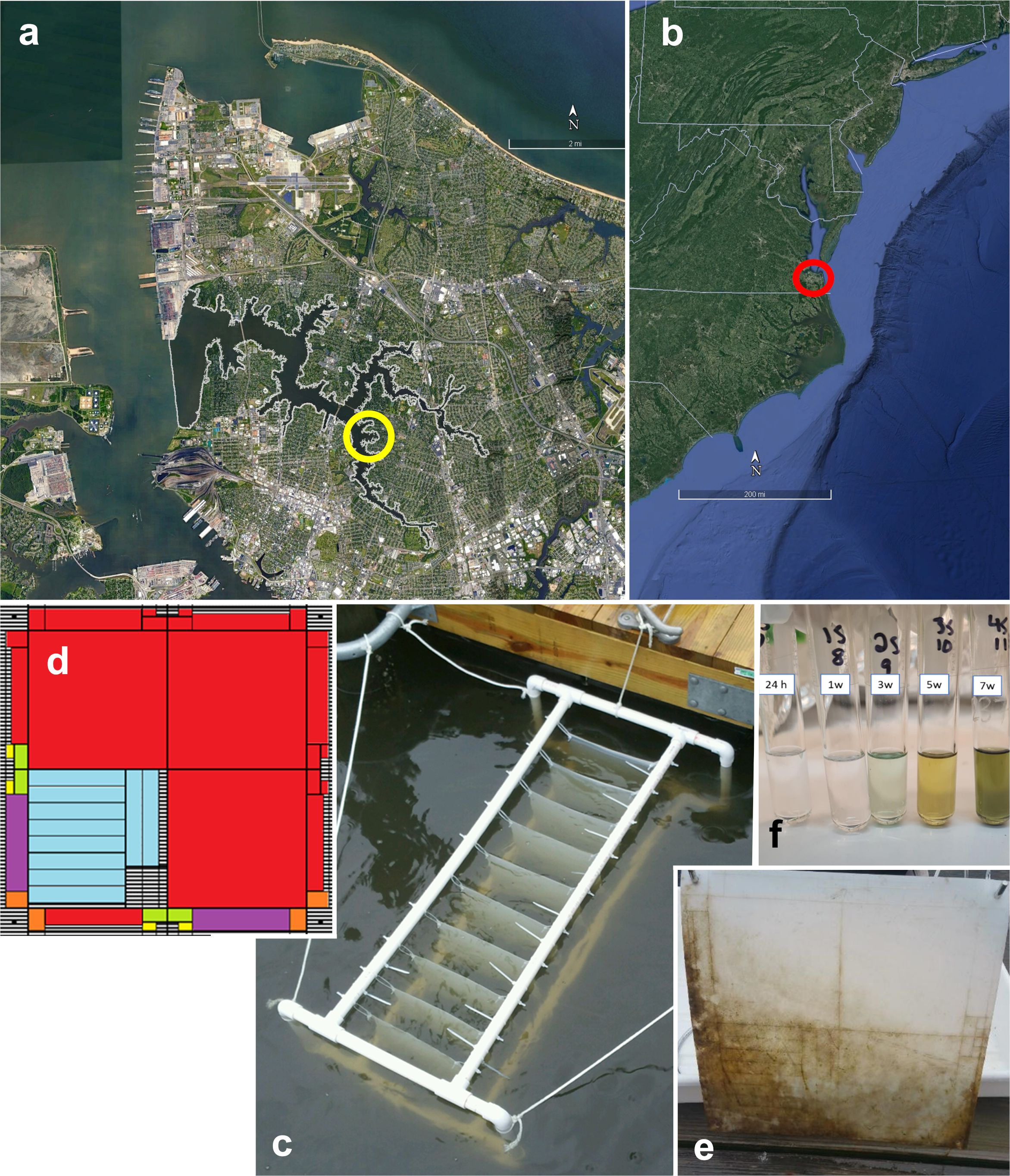
Figure 1. (a) Location of the study within Norfolk, Virginia, USA, indicated by the yellow circle, and (b) the Chesapeake Bay and eastern seaboard of the United States, indicated by the red circle. (c) For field incubations, a PVC rig was used to hold UHMWPE sheets at the surface, with dimensions of 35.6 cm x 35.6 cm x 122 cm for the interior railing. (d) Template used to score plastic sheets (30.5 cm x 30.5 cm). Dashed sections are excess to allow for manipulation without damaging the sample area, yellow sections were used for ATP, green for spectrophotometric pigments, purple for silica, orange for optical microscopy, and blue for lipids, genetics, HPLC pigments, and SEM imaging. Red sections were not used because of the uneven biofouling (e) due to air exposure at extreme low tides or increased shear due to wave action. (f) Lipid extract coloration, with time representing increasing pigmentation (h: hours, w: week).
2.2 In situ incubations and sampling
To study the development of the microbial biofilm in situ, a rectangular rig in the dimensions of 35.6 cm x 35.6 cm x 122 cm was created from polyvinyl chloride (PVC) tubing (Figure 1c). Ten sheets of 30.5 cm x 30.5 cm x 0.15 cm opaque white, ultra-high molecular weight polyethylene (UHMWPE) (McMaster-Carr, product #8752K121) were washed thoroughly with Micro-90 soap and ultra-pure water (UPW) and rinsed with UPW to remove soap residue. Soap was used instead of stronger acids, bases, or solvents because these may affect surface properties of the plastics. UHMWPE was selected as the test material due to the ubiquity of PE in use and the environment, as well as its densities very close to that of seawater (1.02 – 1.03 g/cm3, Supplementary Figure 1). A template was created and printed in a 30.5 cm x 30.5 cm format to define cutting lines for different types of analyses (Figure 1d). Then the plastic sheets were placed over the template, taped in place at their corners, and lines were scored with a hobby knife, deep enough to remain visible during the incubation but not so deep as to cut through or weaken the sheets. Holes were punched in the corners to attach the sheets. Each sheet was then hung in the center of the rig with two zip ties attaching each corner to a rail, and approximately 10 cm between each sheet (Figure 1c). The rig was tied at a depth of 0 - 0.5 m to a floating dock on December 14th, 2020, in order to keep the plastics near the surface where we expect them to float as buoyant plastics and to maximize light exposure for photosynthesis of organisms colonizing the biofilms. The water depth of the site was one meter. During the ensuing 7-week incubation, the study site experienced ranges in water temperature and salinity of 2-13°C and 6-11 (PSS-78), between 9.5 and 10.5 hours of daylight, an average solar irradiance of ~2.3-2.7 kWh/m²/day, no ice cover, and a 0.5–1 m semidiurnal tide. These tides lead to an average residence time of 180 days for the entire estuary. However, the study site’s proximity to the mouth means it has a much shorter residence time of ~40 days (Du and Shen, 2016); therefore, 7 weeks (49 days) approximates the water’s residence time at this location. Due to occasional air exposure of a portion of the sheets at extreme low tides, or from increased shear due to wave action at the surface, the development of the biofilm was uneven with the highest growth towards the bottom. Therefore, some upper sections of the incubated plastic were not used for analysis (Figures 1d, e). The winter incubations afforded an extended period to scrutinize the effects of the microbial biofilm in isolation and without metazoan growth over a period of 49 days. In contrast, subsequent summer incubations (unpublished data) achieved a comparable metazoan colonization level within 4 days due to rapid larval settlement.
Two sheets were randomly selected, then collected and processed at 24 hours, 1 week, 3 weeks, 5 weeks, and 7 weeks, respectively. During sample collection, one sheet was removed from the water at a time and hung from metal hooks to protect the plastic surfaces and left to drip for ~60 seconds without rinsing. Tabs of different sizes were cut along scoring lines with heavy-duty tin snips; where needed, pliers were used to hold the sheet in place by gripping discard areas only (Figure 1d). The tin snips were cleaned between samples by submerging them in river water. Samples were processed with consideration of their stability, with adenosine triphosphate (ATP) and pigments first, followed by lipids, metagenetics, microscopy, and silica. ATP samples were immediately stored in cryovials pre-filled with 1 mL of phosphoric acid–benzalkonium chloride in tricine buffer (P-BAC) extractant and continuously inverted for 30 seconds, then held at room temperature for approximately an hour before they were stored at -80°C (Bochdansky et al., 2021). Samples for pigments, lipids, metagenetics, and scanning electron microscopy (SEM) were placed into 30 mL glass vials, and silica samples were placed into 50 mL centrifuge tubes (Falcon). Optical microscopy samples were wiped on one side with a paper towel and affixed to a petri dish with two-sided tape. Samples were kept in a cooler with ice packs for approximately an hour until they were placed in a -80°C freezer.
2.3 In vitro incubations
Samples were incubated in a controlled laboratory environment to investigate the impact of biogenic ballasting on plastics under optimal conditions, minimizing potential influences from nutrient limitation, grazing, or physical disturbance such as plastic particles chafing against each other or container walls. While it is likely there was some minimal grazing by ciliates or zooplankton, the controlled environment minimized the impact as compared to natural settings. Three aquaria (45.7 cm H x 61 cm W x 122 cm L) each were filled with 12 L of untreated estuarine water and 100 free-floating opaque white high-density polyethylene (HDPE) discs (McMaster-Carr, product #8619K425; 6.2 mm diameter x 0.48 mm thickness) created with a hole punch. UHMWPE was chosen due to its close density to that of seawater, and presumably therefore more sensitive to biofilm-induced buoyancy changes. One aquarium served as a control with no nutrients added, one was enhanced to 1/10 strength f/2+ (Guillard, 1975), and the third with only silica added at 1/10 of f/2+ concentration. Nutrient-enriched aquaria received two additional doses of equal composition and concentration during the incubation (weeks 6 and 12). The nutrient additions served to maximize the growth of biofilm microbes, especially of the ballast-forming diatoms. Aquaria were incubated for six months in a closed system at ~22°C adjacent to a west-facing window receiving sunlight.
2.4 Sample processing
Measurements of ATP followed the protocol of Bochdansky et al. (2021). Briefly, 10 µl of the P-BAC extract from ATP samples (1.27 cm x 0.762 cm) was combined with 3 mL ultra-pure water (UPW) and 50 µl of firefly extract (FFE). Internal standards were made with the addition of 50 µl 16.4 nM ATP, and blanks were created by adding FFE to UPW; all were measured in a scintillation counter (Perkin Elmer Tri-Carb 3110TR) with a single photon counting protocol for 1 minute each.
Photosynthetic pigments were analyzed using high-performance liquid chromatography (HPLC) according to Liu and Xue (2020). Plastic samples (8.89 cm x 1.524 cm) were first cut into smaller pieces and weighed wet, then extracted using 3 mL of acetone within a PP centrifuge tube. This tube was sonicated at room temperature for 15 seconds (Model FS 60, Fisher Scientific), and the resulting acetone extract was filtered through a 0.2‐μm Nylon syringe filter. The plastic sample underwent a second round of extraction following the same procedure, and the two extracts were combined to yield a total volume of 6 mL, which was then stored at -80°C for further analyses. The pigments within the extract were measured with a fluorescence detector attached to the HPLC. This analysis identified six chloropigments: chlorophyll c2 (Chlc2), chlorophyll a (Chl a), chlorophyll b (Chl b), divinyl chlorophyll a (DVChl a), pheophorbide a (Phide), and pheophytin-a (Phytin). Additionally, nine carotenoids, namely peridinin (Peri), 19’-butanoyloxyfucoxanthin (19-but), fucoxanthin (Fuco), prasinoxanthin (Pras), 19’-hexanoyloxyfucoxanthin (19-hex), diadinoxanthin, alloxanthin (Allo), zeaxanthin (Zea), and lutein, were quantified using a photodiode array detector integrated into the same HPLC setup. To determine the composition of the phytoplankton community, the carotenoid data was processed using established algorithms (Letelier et al., 1993; Lambert et al., 1999; Qian et al., 2003; Reyna et al., 2017), based on the principle that each phytoplankton class contains a different set of pigments, such as diatoms being enriched in Fucoxanthin, cyanobacteria in Zeaxanthin, and dinoflagellates in Peri. Duplicate analyses of the same extract agreed within a 20% margin. Pigment concentrations in separately extracted samples (2.286 cm x 1.27 cm) were determined using spectrophotometric absorbance and multiple regression models in accordance with the approach outlined by Parsons et al. (1984).
Biogenic reactive silica was determined following the protocol initially described by Strickland and Parsons (1972), with subsequent enhancements by Fanning and Pilson (1973), and further modifications by Paasche (1980). Biofilm was scraped from each tab (8.89 cm x 2.032 cm) with a spatula into a polycarbonate (PC) petri dish and rinsed into the barrel of a stoppered 10 mL syringe with 0.5% Na2CO3 (5 g/L). The plunger was returned to the syringe, and the syringe was heated at 85°C for 2 hours in a water bath to dissolve biogenic silica (Paasche, 1980). Once cooled to room temperature, samples were filtered through a 25 mm 0.8 µm PC filter and placed into a 50 mL Falcon tube. Two drops of methyl orange were added to each sample, and 0.5 N HCl was added until the turning point of methyl orange (turns pink at pH 3-4). Finally, samples were made up to exactly 25 mL in a volumetric flask with UPW and stored (-80°C) for further analysis. The remaining analysis followed the spectrophotometric protocol for dissolved silica in Strickland and Parsons (1972).
To extract lipids from the biofilm, we employed a modified version of the procedure outlined in Pedrosa-Pàmies et al. (2018, 2019). In summary, the plastic tabs (8.89 cm x 1.52 cm) were first cut into smaller pieces to facilitate their insertion into 16 mm test tubes for the extraction process. Subsequently, each tube received 12 mL of a solvent mixture composed of hexane and methanol in a 2:1 ratio. Prior to the lipid extraction, an internal standard mixture comprising n-C21:0 fatty alcohol, n-C23:0 fatty acid, 5α-cholestane, and n-C36:0 alkane was added to the samples. Lipids were ultrasonically extracted in 2:1 hexane:methanol. The lipid extracts were concentrated to dryness using a rotary evaporator, then resuspended in chloroform, and passed through a short column of combusted, anhydrous sodium sulfate to eliminate any residual water. The purified extract was subsequently transesterified with anhydrous 10% methanolic hydrochloric acid at 55°C for 12 hours, according to the method described by Christie (1982). The transesterified lipids were extracted into hexane and again passed through a short column of sodium sulfate to remove any remaining water. Subsequently, lipid extracts were trimethylsilylated using N,O-Bis(trimethylsilyl)trifluoroacetamide with 1% Trimethylchlorosilane. These transesterified and trimethylsilyl derivatives underwent analysis using an Agilent 7890A gas chromatograph linked to a 5975C mass spectrometer (MS) equipped with a triple-axis MS and a flame ionization detector (FID), and a Varian Colorado Plateau Stable Isotope Laboratory 5CB low bleed MS column (60 m × 0.25 mm diameter × 0.25 µm film thickness) was employed to separate the various lipid compounds. Identification of these compounds was achieved through mass spectra, and quantification was based on their FID response relative to the internal standard.
Diversity analyses of the microbial biofilm community were conducted based on high-throughput Illumina MiSeq (RTL Genomics, Lubbock, Texas, USA). Tabs (8.89 cm x 1.52 cm) were sent on dry ice to RTL Genomics for biofilm extraction and sequencing of the 16S V4–5 region of the 16S gene (bacteria), the 18S V9 region (eukaryotes), and ITS1 region (fungi). Each sample was processed as described in the protocols in the Supplementary section on sequencing (Supplementary Figure 5). Briefly, data analysis included denoising, chimera checking, and FASTQ file generation. Diversity analysis included quality control FASTA Formatted Sequence/Quality File Generation, Operational taxonomic unit (OTU) clustering, and taxonomic identification using the USEARCH global alignment program and the National Center for Biotechnology Information database. Diversity analysis used the OTU/Dereplication table generated from sequence clustering and taxonomic identification output and generated a new OTU table with taxonomic information. The updated OTU table was then output containing both the full and trimmed taxonomic information for each cluster and visualized in Krona plots (National Institutes of Health). There was not enough genetic material for 18S amplicon sequencing for the initial two timepoints (24 hours and one week). Furthermore, large portions of fungal ITS analysis for the final three timepoints returned no hits.
For optical microscopy, a piece of plastic (1.52 cm x 2.03 cm) was subsampled (1 cm x 1 cm), placed on a microscope slide, and two drops of Vectashield with 4′,6-diamidino-2-phenylindole (DAPI) (H-1200, Vector Laboratories) were added to identify live cells in the biofilm due to fluorescence of the DNA-bound dye; no coverslip was added so as to not disturb the biofilm. The biofilm was immediately observed and imaged with a 10x Epifluorescent microscope (EFM) objective lens on a BX50 Olympus microscope. Additionally, a random subset of samples from the aquarium study were removed and viewed under an inverted microscope.
SEM was performed by dehydrating plastic samples (1.52 cm x 2.03 cm) on ice through an ethanol series: 10 min each in 50%, 70%, 85%, and 95%, followed by 3 × 15 min in 100% ethanol. Samples were immediately air-dried overnight after using hexamethyldisilazane (HMDS) (Sigma) (Shively and Miller, 2009), sputter coated with 5 nm of platinum using a Leica EM MED020 (Leica Microsystems, Inc. Buffalo Grove, IL), then visualized and imaged on a Zeiss Supra 40VP SEM (Carl Zeiss Microscopy, Thornwood, NY) with an acceleration voltage of 3.00 kV, and a pressure between 0.1 Pa and 24 Pa. Additionally, the samples in Figure 2f were immersed in 25 mM Ethylenediaminetetraacetic acid (EDTA) solution for 15 min (Cavaliere et al., 2014) before the application of DAPI in order to allow better penetration of the DNA stain, and this seems to have allowed the outer structure to fluoresce lightly.
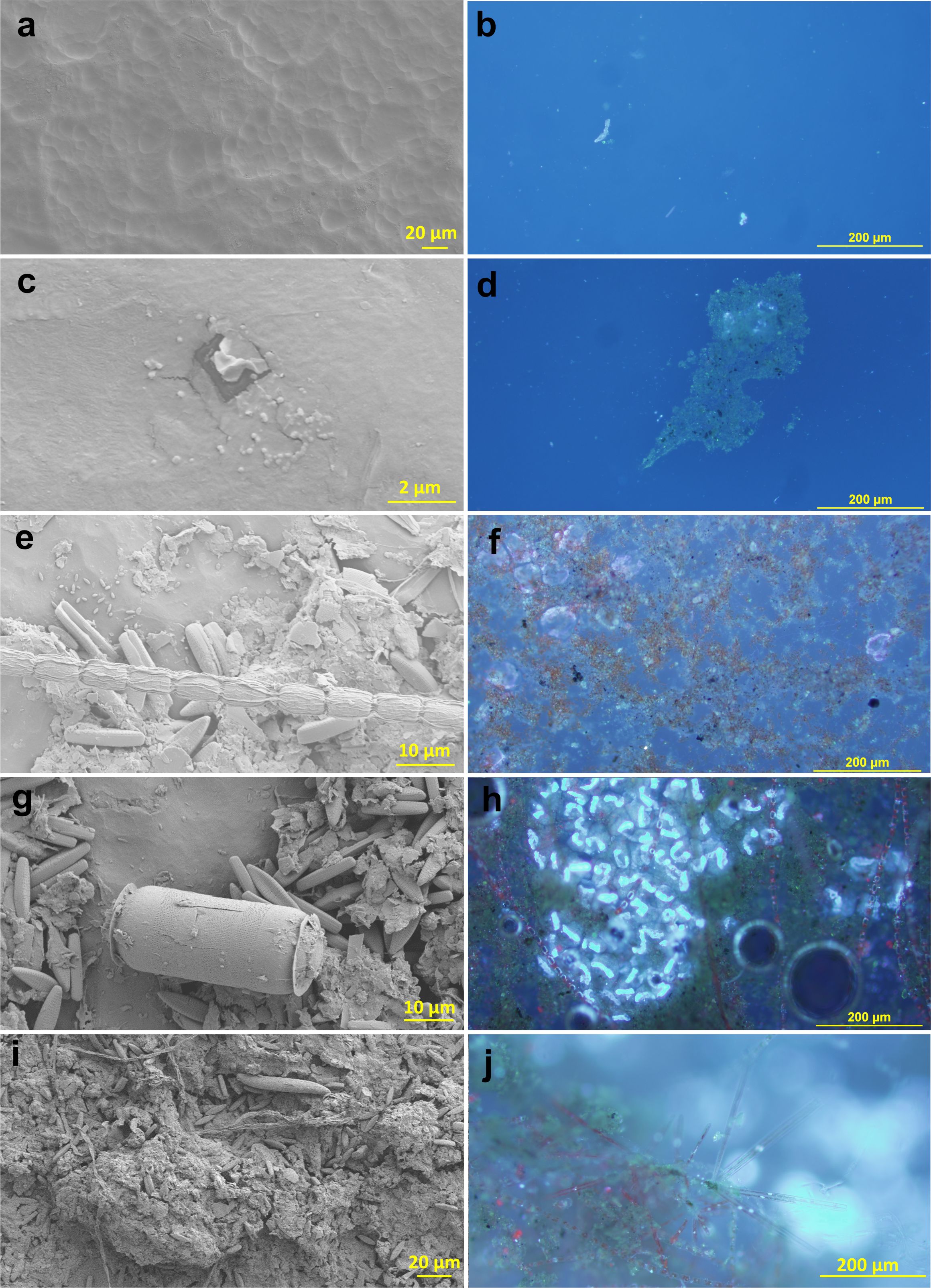
Figure 2. Biofilm on in-situ incubated plastics visualized with scanning electron microscopy (left) and epifluorescence microscopy (DAPI stain) (right) at 24 hours (a, b), 1 week (c, d), 3 weeks (e, f), 5 weeks (g, h), and 7 weeks (i, j).
3 Results
ATP remained below 0.1 µmol/cm2 during the first week, then increased linearly with time up to 28.4 ± 66.4(std. dev.) µmol/cm2 plastic surface, indicating a slow initial growth phase and high variability with time (Figure 3a). Phytoplankton pigments were also at a very low level until after week 1 and plateaued by week 5 (Figure 3b). Spectrophotometric absorbance measurements of Chl a were higher than those seen in the HPLC, reaching a maximum of 1.19 ± 0.25 and 0.5 ± 0.08 µg Chl a/cm2 plastic surface, respectively. Silica remained undetectable for the first week and increased for the remainder of the experiment, achieving a maximum reactive silica concentration of 65.5 ± 28.5 µg/cm2 (Figure 3c). Accessory pigment analysis showed that diatoms were the dominant photosynthesizers, making up to 98% of the phytoplankton community at week 3, reducing to 89% in week 7 (Figure 3d).
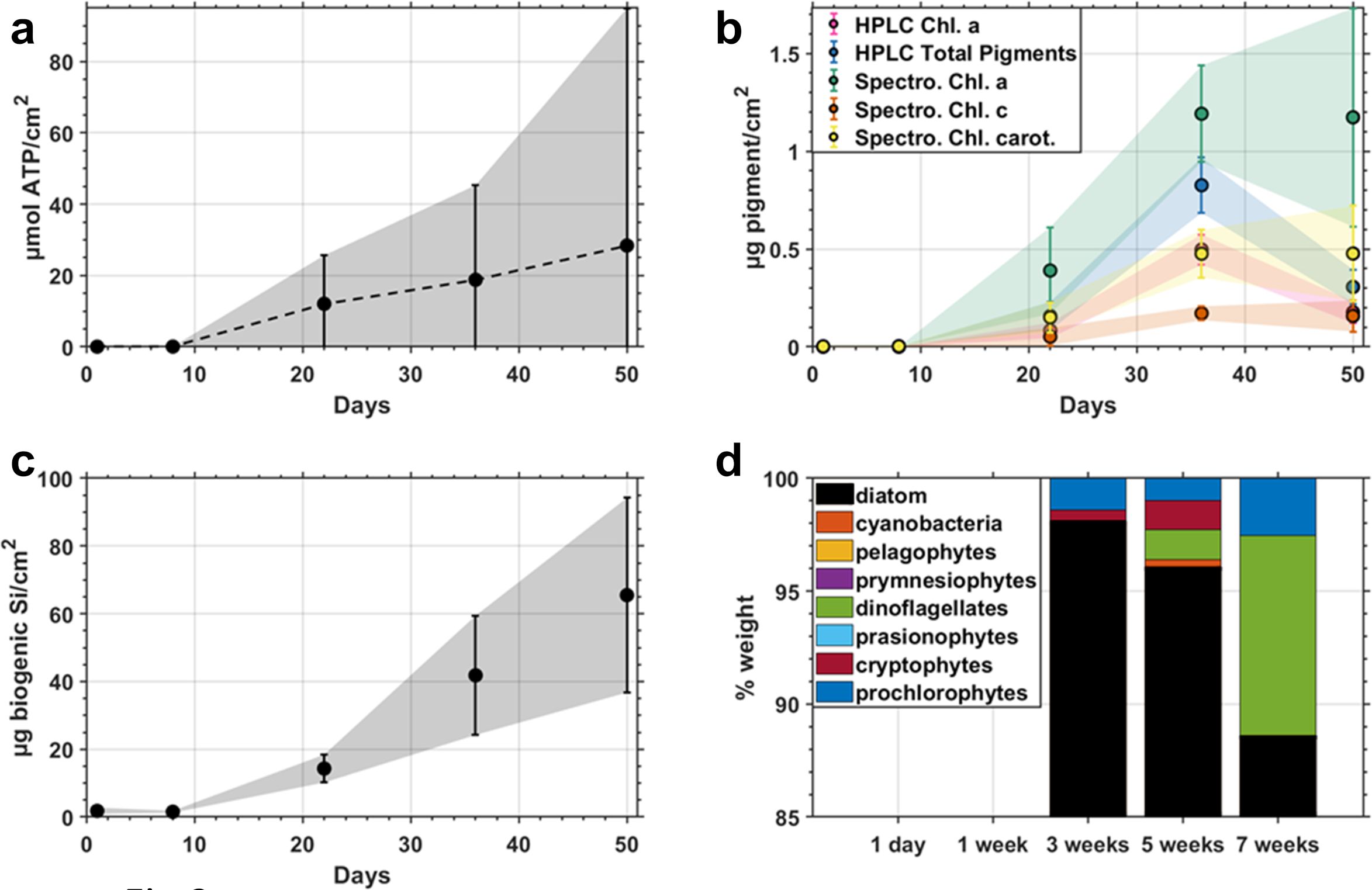
Figure 3. (a) ATP concentrations of field incubated biofilms normalized to the surface area of plastic with standard deviation shaded, (b) Photosynthetic pigments (c) Biogenic silica, and (d) Photoautotrophic community proportions by weight determined by HPLC pigments.
Total extracted lipids during the first 3 weeks remained below 0.2 µg/cm2 and increased to 16.2 µg/cm2 by week 5 (Figure 4a, Supplementary Figure 2). Odd chain fatty acid concentrations accounted for nearly ~20% of total lipids during the initial three weeks, then decreased by an order of magnitude proportionally for the remainder of the experiment (Figure 4b).
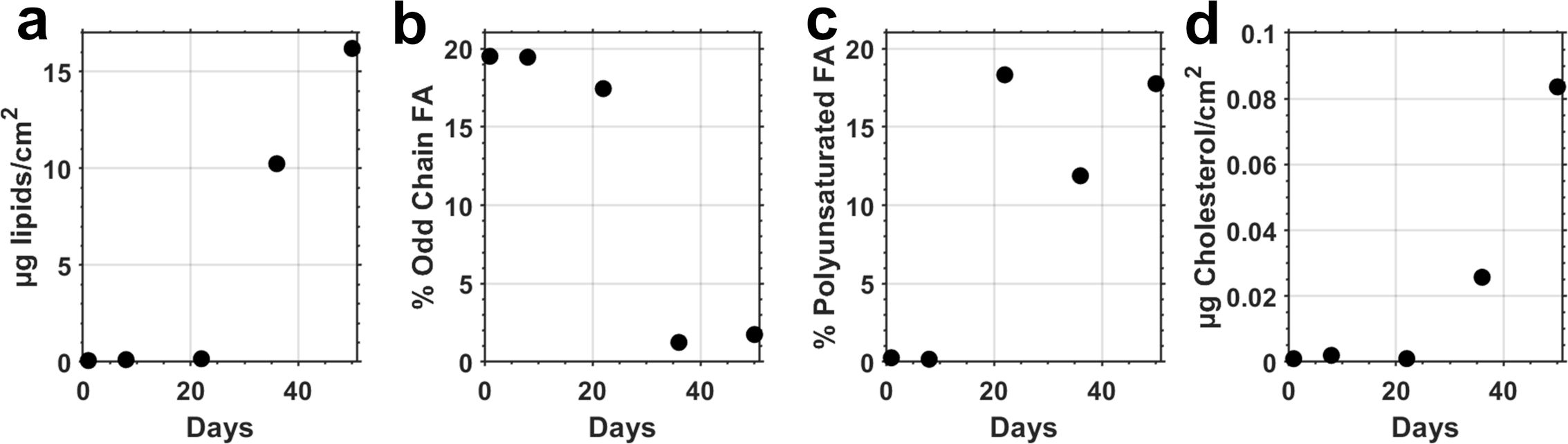
Figure 4. Lipids of field incubated samples: (a) Total lipids (µg/cm²), (b) Odd chain fatty acids/total lipids (%), (c) Polyunsaturated fatty acids/total lipids (%), and (d) Cholesterol (µg/cm²).
In contrast to odd-chain fatty acids, polyunsaturated fatty acid concentrations remained <0.25% of total lipids for the initial week of colonization before increasing to between 12-18% for the remainder (Figure 4c). Cholesterol values show that eukaryotic colonization did not initiate until sometime after week 1, corresponding to our sequencing results (Figure 4d, Table 1). Accessory pigment analysis showed an increase in the relative abundance of dinoflagellates in the final time points (Figure 3d).
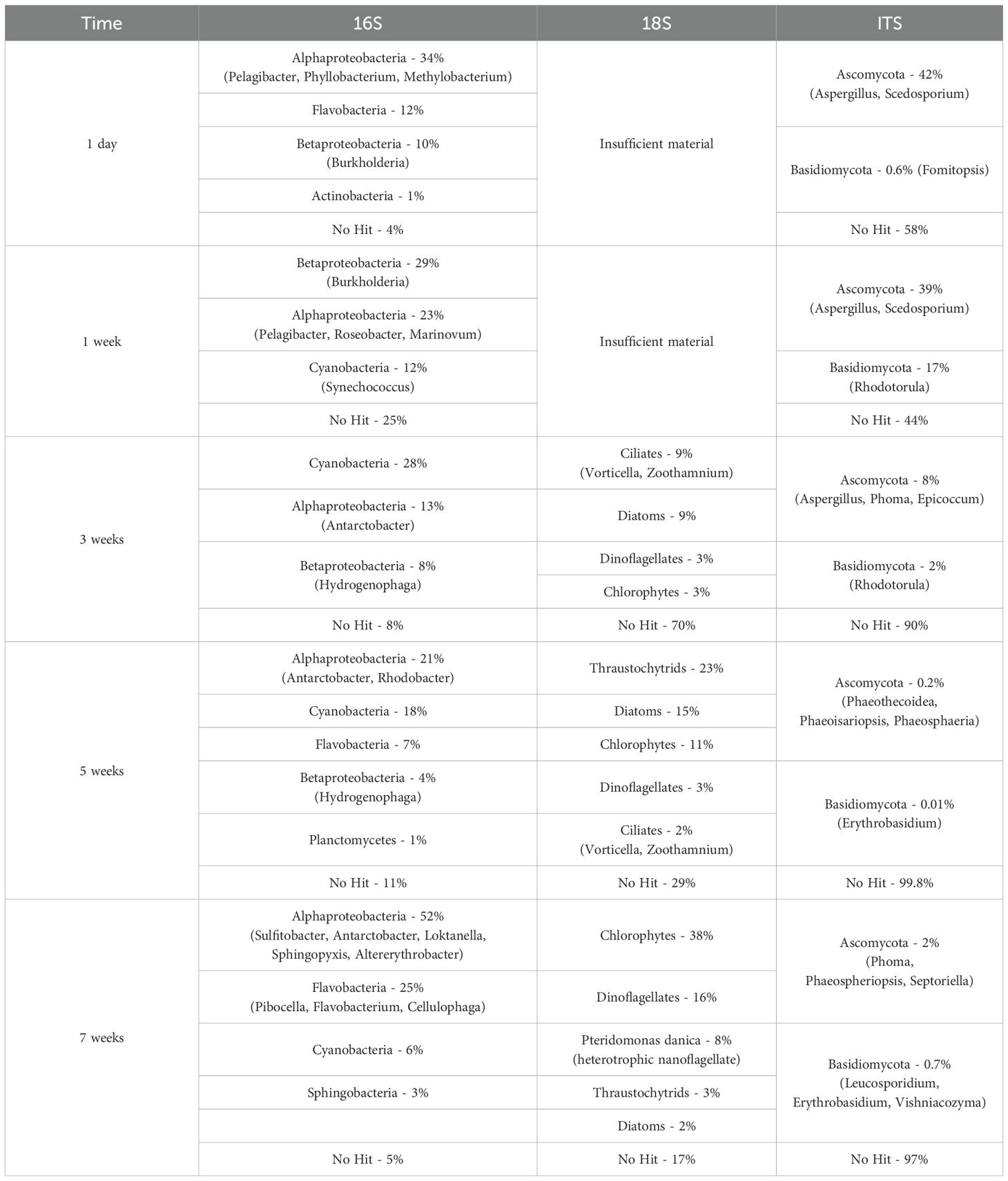
Table 1. Amplicon sequence analysis of microbial communities growing on plastic sheets in the Lafayette River using primers targeting the 16S (bacteria), 18S (eukaryotes), and ITS (fungi) regions. Groups are shown with their relative abundance of recognized sequences with some dominant genera within as examples (in parentheses), with the most abundant groups higher up within a given time period.
Genetic analysis revealed a bacterial community dominated by alphaproteobacteria, betaproteobacteria, and cyanobacteria (Table 1, Supplementary Figure 4). Pelagibacter were detected for the initial week, and Flavobacteria were very abundant, especially in the more mature biofilm. Fungi were mainly composed of Ascomycota, primarily Aspergillus, as well as Basidiomycota (Table 1). Eukaryotic colonization began with ciliates, diatoms, and dinoflagellates before Thraustochytrids, and chlorophytes became more abundant in the fifth and seventh weeks (Table 1). Notably absent from our genetic analysis were metazoan macrofoulers, with the exception of trace amounts of the barnacle Balanus which were detected from week 5 onward (Supplementary Figure 4). Our pigment analysis (Figure 3d) identified key groups such as Diatoms, Cyanobacteria, Dinoflagellates, and Cryptophytes, which corresponded well with the genetic analysis.
SEM and optical microscopy (Figures 2, 5) showed the surface and community development, highlighting not only the surface EPS layer (Figure 2c) but also some of the diversity present (Figure 2e). The distribution of organisms was very patchy with some species dominating locally (Figure 2h). Bacteria can be seen clinging to the surface with mucous threads (Figure 2e). Diatom, cyanobacterial, and fungal strands frequently streak across the surface of larger biofilm structures (Figures 2e, h, i). EFM with DAPI revealed an organism ~50 µm wide with a nucleus that was sparsely detected at 1 week, which then reproduced rapidly (Figures 2f, h) to a maximum at week 5 (Figure 2h) wherein as many as ~20 colonies/cm² were detected with as many as ~100 nuclei per colony. These organisms were largely transparent in visible light, as demonstrated in Figure 2f after the nuclear material (~12 months in -80°C freezer) and DAPI (2–3 hours under EFM) had decayed so the outer structure was illuminated but not lost in the glow. These organisms were notably absent from the final time point at week 7. Due to their size and based on our genetic analysis (Table 1), we concluded that these are very likely to be Zoothamnium or other closely related stalked ciliates.
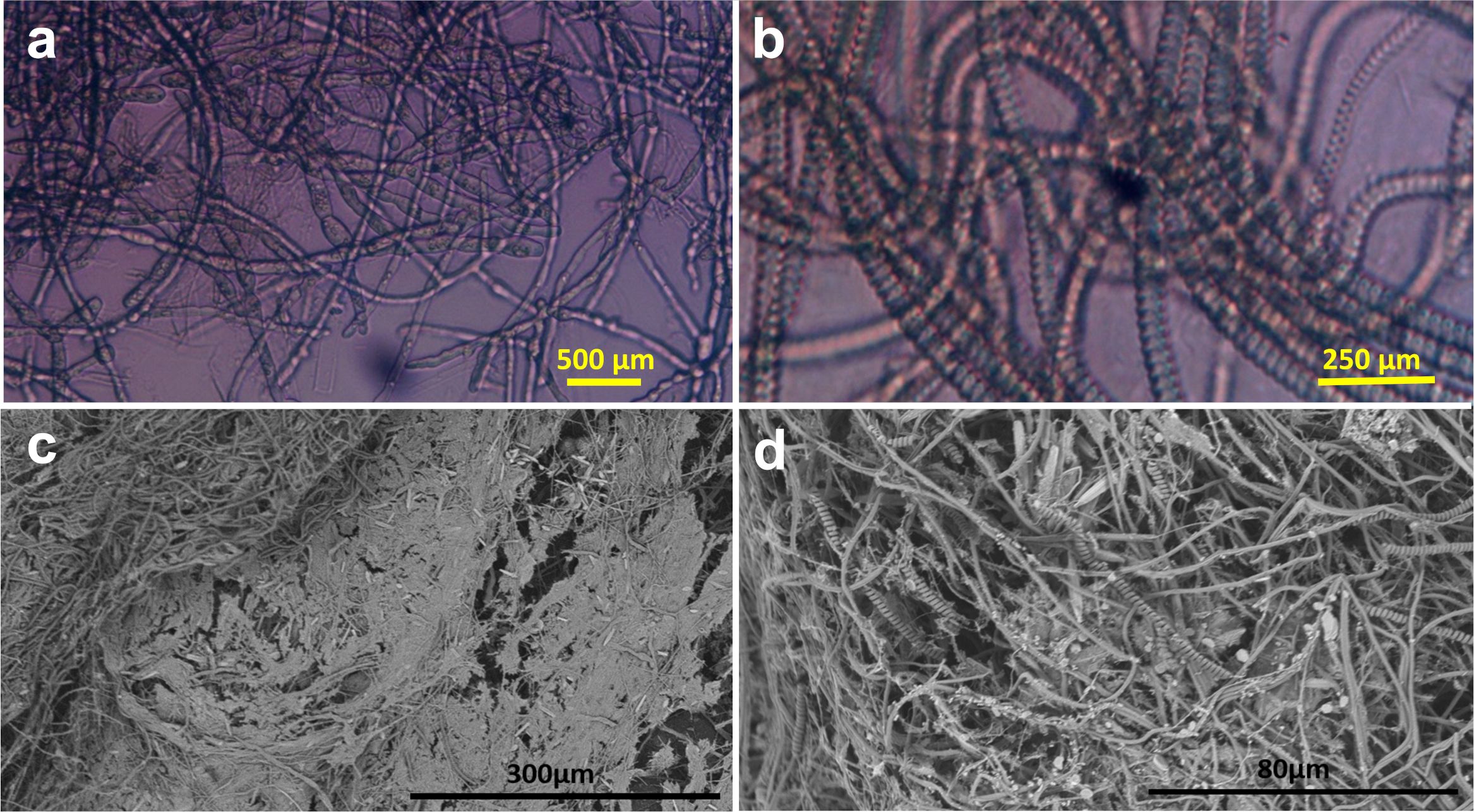
Figure 5. Laboratory incubation experiment with plastic disks. (a) Fungal colonies were found in the unamended estuarine water (inverted microscope). (b) Cyanobacterial chains found in f/2+ enriched water (inverted microscope). (c) Biofilm grown in silica-enriched water (SEM). (d) As in c at higher magnification (SEM).
An unexpected candidate of these frequent and conspicuous structures is the Thraustochytrids, which are single-celled saprotrophic eukaryotes similar to fungi in their ecological niche and have been found worldwide on marine algae and seagrasses (Raghukumar, 2002). They were abundant in our sequence library, especially in week 5 (Table 1). This is surprising as Labyrinthulomycetes (the phylum to which Thraustochytrids belong) are intolerant to salinities below 12 (Raghukumar, 2002), and salinities during sampling ranged from 6 to 11. Zoothamnium on the other hand is a giant stalked sessile colonial ciliate found globally in eutrophic coastal and brackish waters (Schuster and Bright, 2016; Wu et al., 2020). Within each zooid a macronucleus is located, which resembles the curvy nucleus found here (Figure 2h).
In long-term laboratory experiments, the three nutrient treatments selected very different and dense biofilm communities. Unamended estuarine water yielded a predominance of fungal colonies (Figure 5a), whereas f/2+ nutrient additions selected colonial cyanobacteria (Figure 5b), and silica additions alone resulted in more mixed communities of fungi, diatoms, and cyanobacteria, shown in the SEM images (Figures 5c, d). Although the tabs were covered with thick biofilm communities in these in vitro incubations, not a single free-floating plastic disk (6.2 mm diameter x 0.48 mm thickness) sank to the bottom of any of the aquaria during the six-month experiments. This result indicates that even with optimized conditions and very dense microbial biofilm accumulation, the density of plastics did not increase above that of estuarine water.
In all incubations (field and laboratory), we observed a large number of pennate and centric diatoms (Figures 3d; 2g, i, j; 5d) and measured a steep increase in silica on the plastic sheets after week 3 in the field experiment (Figure 3c). Since we were not able to identify any microbial ballasting material other than biogenic silica (such as calcifying organisms), we assume silica was the sole mineral cause of changes in density. We can thus apply the maximum accumulation of silica we measured (69.5 µg/cm2, Figure 3c, Supplementary Figure 3) to various thicknesses of material and generate the curve shown in Figure 6. On one extreme, the substrate is infinitely thin, and the density is that of biogenic silica only (2 g/cm3; Hurd and Theyer, 1977). On the other end, the contribution of the observed biofilm community is negligible and the density approaches that of the plastic (0.97 g/cm3, Figure 6). These calculations suggest that HDPE films would have to be <15 µm in thickness to become negatively buoyant through microbial mineral ballast alone. The same principle can be extended by modeling geometries other than plain sheets (see Discussion section 4.2, Figure 7).
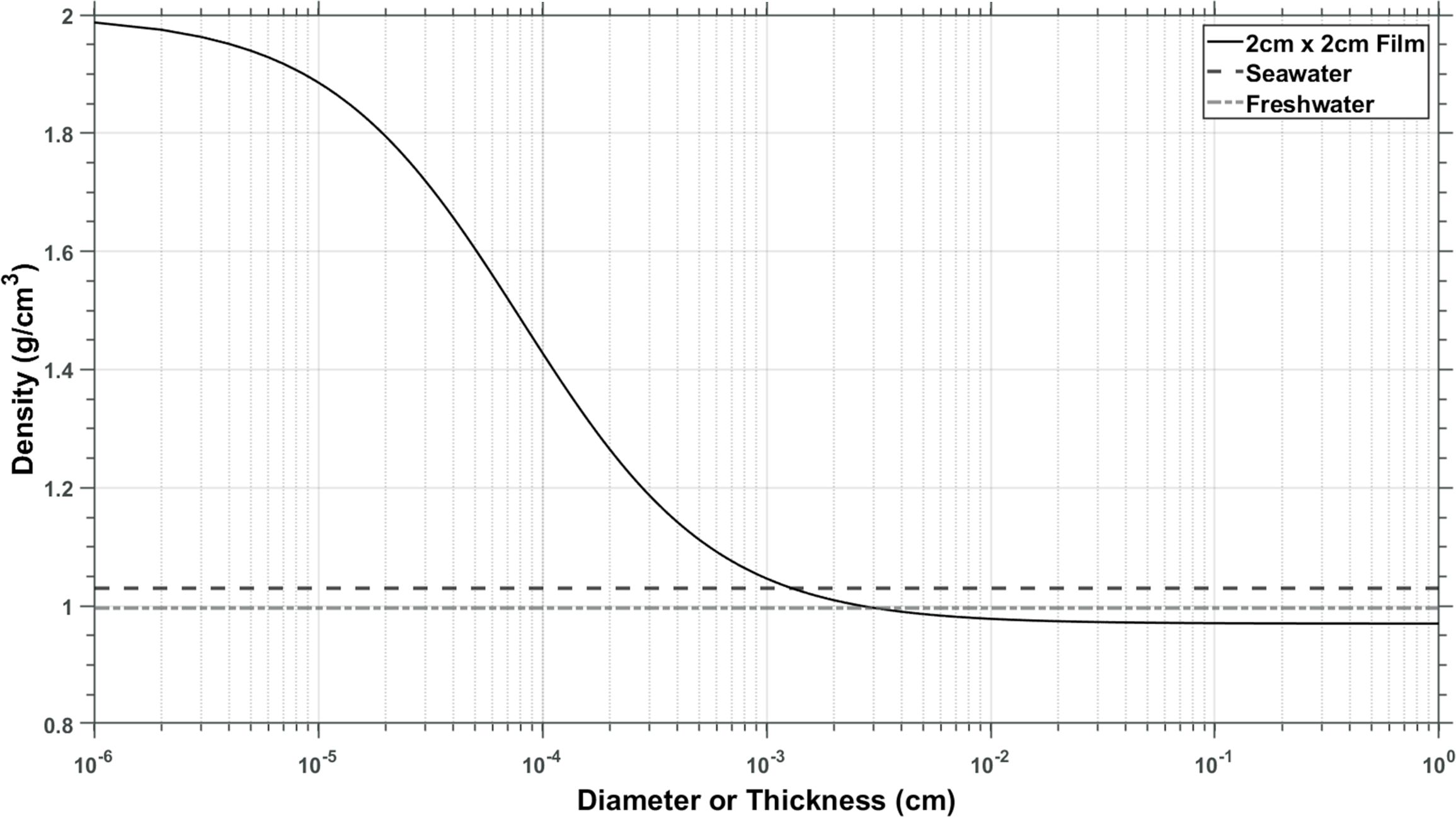
Figure 6. Densities of UHMWDPE plastic at various thicknesses using the maximum observed biogenic silica accumulation during the field incubations (at week 7). If the plastic is very thin, biofilm dominates, and density is close to that of biogenic silica (left side of the figure). The thicker the sheet, the more its density is influenced by the density of the plastic (right side of the graph). Plastic of this density needs to be thinner than plastic wrap to become negatively buoyant in water assuming the existence of the maximum observed biofilm on both sides.
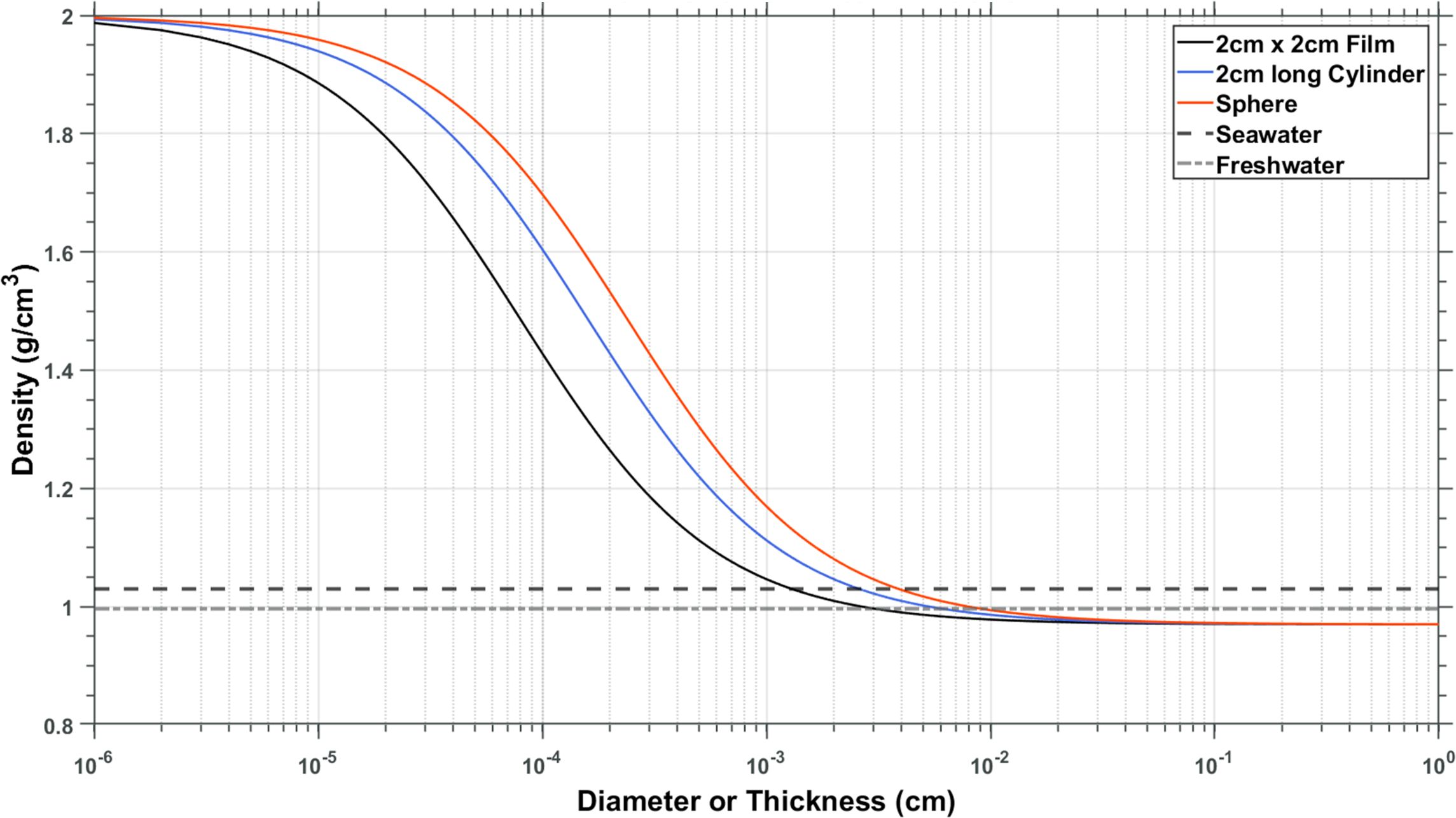
Figure 7. Expanded model considering different shapes of plastic materials of the same density: 2x2 cm sheets (black), 2cm long cylinders (blue), and spheres (red).
4 Discussion
4.1 The succession of in situ microbial communities
The community progressed largely as expected from previous studies of biofouling on plastics within estuaries, with the initial 24 hours characterized by the accumulation of an EPS layer as dissolved and suspended material adhered to the surface (Bhagwat et al., 2021; Rummel et al., 2021) and provided pioneering bacterial communities (Figure 4b, Table 1; Lee et al., 2008; Lobelle and Cunliffe, 2011; Qiang et al., 2021) resources to colonize and grow, primarily including Alphaproteobacteria, Betaproteobacteria, and Cyanobacteria (Figure 4b, Table 1). The relative contribution of Gammaproteobacteria was relatively low in contrast to previous studies (Lee et al., 2008; Qiang et al., 2021).
By the end of the first week, the EPS layer had thickened significantly, as evidenced by SEM images (Figure 2c). During this period, bacterial abundance increased modestly, and initial islands of photosynthetic microorganisms, including diatoms and cyanobacteria, appeared (Figure 2d). This early phase of colonization is crucial as it sets the stage for subsequent microbial succession by altering the surface properties and creating microenvironments suitable for diverse microbial life.
In the third week, the biofilm community became more complex and heterogeneous. Although the surface colonization was still patchy, the presence of eukaryotic organisms such as diatoms and ciliates was notable (Figures 2e, f). Diatoms, in particular, contributed significantly to the silica content of the biofilm, as their frustules are made of biogenic silica, which was reflected in the increase in reactive silica measurements (Figure 3c). This phase marks the transition from bacterial dominance to a more diversified community structure, including significant contributions from eukaryotic photosynthesizers. The strong prevalence of pennate diatoms at the end of the incubation period was similar to that documented previously for an estuarine system in England (Reis and Aldridge, 2024).
Fungi, which have the capability to metabolize complex and recalcitrant compounds found in plastics (Zeghal et al., 2021), were also early colonizers. Their presence was particularly prominent in nutrient-limited environments, as demonstrated in our laboratory experiments (Figure 5a), whereas in the nutrient-amended treatments, cyanobacteria flourished (Figure 5b).
In the fifth week certain eukaryotes became abundant, including Thraustochytrids, dinoflagellates, and stalked ciliates like Zoothamnium (Table 1, Figure 2h). The identification of these organisms through genetic analysis and microscopy provided insights into the complex interactions within the biofilm. Thraustochytrids, although surprising due to their known intolerance to low salinities, were abundant during this period, indicating possible adaptations (Shabala et al., 2009) or microhabitats (Lohan et al., 2020) within the biofilm that supported their growth. Interestingly, the bacteria Planctomycetes appeared transiently in the fifth week, possibly reflecting their preference for nutrient-rich environments coupled with their relatively slow growth rates (Wiegand et al., 2018).
Much of the data’s variability (Figure 3) was due to inherently uneven biofouling (Figure 1e), some of which may be a result of the highly hydrophobic nature of the plastics used, and differences in the microtexture of the plastic surface. Some seeming changes in community structure measured with pigments, metagenetics, and microscopic analyses may thus be the result of subsampling a highly varied and discontinuous environment. For example, although dinoflagellates increased in their relative contribution at the end of the incubation interval, diatoms and ciliates nonetheless still dominated the eukaryotic microbial communities at the end of the incubation interval. Only diatoms with their silica frustules could change the buoyancy of plastic appreciably. In terms of total chlorophyll, the maximum of 1.19 ± 0.25 µg Chl a/cm2 after 7 weeks during the winter was within the observed monthly range in the Bay of Bengal of 0.2-1.5 µg Chl a/cm2 (Sudhakar et al., 2007), and much lower than the highest recorded values in the same region of 800 µg Chl a/cm2 after four months of incubation (Artham et al., 2009) which are the only studies so far to have measured pigments in the plastisphere. Our measured values may be significantly lower than those reported in the previous study, possibly due to differences in incubation depth; their experiment was conducted at three meters depth, whereas ours was performed at the surface. Phytoplankton often compensate for reduced light availability at greater depths by increasing chlorophyll production (Halsey and Jones, 2015).
The observed effect of nutrient additions on the development of cyanobacteria and diatoms in our laboratory experiments underscores the influence of environmental factors on biofilm communities. For instance, the growth of cyanobacteria in dilute f/2+ nutrient conditions and the development of mixed communities with silica addition indicate that nutrient availability can profoundly influence the composition and structure of biofilms. This finding may also have environmental implications, suggesting that seasonal variation in nutrient levels could foster distinct microbial communities in natural settings. Although ecological systems vary widely, it is generally noted that nutrient limitation often occurs during and after spring blooms (Conley, 1999), potentially resulting in less efficient biofilm colonization during that period. Conversely, during nutrient-rich periods such as winter or early spring, biofilm communities may develop more slowly because of the lower temperatures but with greater stability and diversity. Understanding these dynamics can aid in predicting biofilm development on marine plastics across different seasonal and environmental contexts.
There is conspicuous absence of studies performing an integrated analysis using 16S, 18S, and ITS sequencing simultaneously in the study of microbial biofilms on marine plastics (Wright et al., 2020). Such integrated approaches are crucial for achieving a holistic understanding of microbial communities, as they allow for the concurrent examination of bacterial, fungal, and broader eukaryotic taxa.
To date, only a handful of studies have included 18S sequencing (Zettler et al., 2013; Oberbeckmann et al., 2016; Debroas et al., 2017; Kettner et al., 2017, 2019; Kirstein et al., 2018) and even fewer have employed ITS sequencing (De Tender et al., 2017) on marine plastic substrates, which is essential for determining the relative contributions of different organisms to processes influencing the buoyancy of MPD, and only one has employed three primer sets (Davidov et al., 2020). This paucity of data limits our ability to make comprehensive comparisons across different studies and environments. The lack of simultaneous sequencing efforts impedes our understanding of the interactions and successional dynamics within these complex biofilm communities. Microbial communities colonizing marine plastics are shaped by various factors such as environmental conditions, plastic type, geographical location, and interactions like competition and predation. Without concurrent and consistent sequencing across studies, drawing definitive conclusions about these influences and establishing broad ecological or evolutionary trends remains challenging.
4.2 The effect of biofilm on the buoyancy of microplastics
Previous research has shown that both macro- and microfouling can induce buoyancy changes under certain conditions but diverges on whether the buoyancy changes are positive or negative (Amaral-Zettler et al., 2021; Fazey and Ryan, 2016; Kaiser et al., 2017; Karkanorachaki et al., 2021; Wright et al., 2020). Therefore, how and why buoyancy changes due to biofouling needs to be examined in greater detail. There exists a dichotomy between the more rapid accumulation of biofilms on smaller particles because of their larger surface-to-volume ratios, and the greater ability of macrofauna to grow on larger particles and thereby induce negative buoyancy. It has been demonstrated that macrofauna (bryozoans, mussels, tunicates, etc.) and algae can induce negative buoyancy in otherwise positively buoyant plastics (Amaral-Zettler et al., 2021; Fazey and Ryan, 2016; Holmström, 1975; Kaiser et al., 2017; Ye and Andrady, 1991). In this study, however, we were only concerned with microbial biofilms and terminated the experiment as metazoan colonization became apparent.
Models have suggested that microbial biofilms alone can induce negative buoyancy in plastics sufficient to cause them to sink. For instance, Kooi et al. (2017) modeled plastic spheres ≤10 mm and stated that all particles could settle due to microbial biofilms. Similarly, Van Melkebeke et al. (2020) investigated various shapes ≤5 mm and calculated that only spheres smaller than 0.6 mm but all sizes of films could sink due to microbial biofouling. Experimental evidence, however, is limited and conflicting. Lobelle and Cunliffe (2011) incubated PE films (20 cm x 28 cm x unknown thickness) for three weeks in seawater and observed that by the final week of the experiment, the plastic (subsampled to 1 cm x 2 cm) started to float lower in the water than at earlier time points but never became negatively buoyant. Following a series of experiments with diatoms and density measurements of 5mm x 5mm PE sheets of varying thicknesses, Amaral-Zettler et al. (2021) calculated that a 100 µm PE bead could be induced to negative buoyancy with a 10 µm thick biofilm layer if colonized 100% by the diatom Navicula sp.; however, they also noted the low likelihood of 100% colonization by diatoms, and their field-incubated samples sank only after they were settled by Bryozoa (i.e., a metazoan that precipitates calcium carbonate). Chen et al. (2019) incubated PP sheets for 1 month in a lake and harvested the biofilm every three days. Density changes were calculated based on ratios of the ash-free dry-weight and dry-weight. While they found that all of their particles increased in density, no calcareous organisms were found, and inorganic minerals trapped in the biofilm and not the microorganisms themselves were likely responsible for the loss of buoyancy. While the authors calculated that the plastic sheets could become negatively buoyant, this would only have been possible in freshwater, and no particles achieved densities above 1.008 g/cm³.
The only study to show experimentally that positively buoyant particles can be made to sink is by Semcesen and Wells (2021), who incubated PP spheres of various sizes from 125-2000 µm in tanks filled with lake water for 120 days. They sampled the tank floors daily with pipettes for particles that sank and found the smallest plastics (125–250 μm) all sank within 4 weeks, the medium size fraction (325–500 μm) by 9 weeks, and 95% of large microplastics (1000–2000 μm) had settled by the end of the experiment. However, it is not clear what caused these buoyancy changes as there was no description of the community or measurement of other variables that may have affected density.
Biofilms may also increase buoyancy. Karkanorachaki et al. (2021) incubated 30 cm x 2 cm sheets of low-density polyethylene (LDPE), HDPE, PP, PS, and PET, as well as MP pellets of LDPE, HDPE, and PP, with some pellets being artificially aged for 4 months in UV light. Density was measured through immersion in a series of successive deionized water and ethanol mixtures. While density changes were observed, they were never sufficient to change the buoyancy from positive to negative. Some aspects of this experiment are problematic, however, as fixing and drying a biofilm will significantly affect its structure. The addition of ethanol could also decrease the buoyancy of the biofilm due to the dissolution of lipids, which themselves are positively buoyant. By comparison, in our long-term laboratory experiments, we were unable to induce a single particle to become negatively buoyant, despite six months of incubation in a nutrient-enhanced environment with little turbulence, and the formation of a thick biofilm.
Using the maximum amount of biogenic silica we observed accumulating during our experiment, we are able to calculate the effect of this microbial ballasting material on density simply by assuming different thicknesses and geometries of plastics. It is important to keep in mind that live diatoms are not necessarily negatively buoyant (see below), and our calculated buoyancies (Figure 6) represent an extreme scenario towards negative buoyancy as changes are solely based on the observed biogenic silica values and a density of 0.97 g/cm3 for polyethylene. We expanded our calculations of added silica per surface area (Figure 6) to model the effect of different shapes of particles on their ability to become negatively buoyant (Figure 7, Supplementary Figure 3). For comparison, and based on our model, spheres <40 µm could become negatively buoyant through microbial biofilms alone within 7 weeks, however, fibers of 2 cm length would have to be <25 µm in diameter, and 2 cm x 2 cm films would have to be <15 µm in thickness to become negatively buoyant (Figure 7). However, it is important to consider that these shapes are often constrained to maintain their aspect ratios. For instance, a film with either its length or width reduced close to its thickness resembles a fiber, while a fiber shortened to the extent of its diameter resembles a sphere. The closer the density is to that of seawater (e.g., polystyrene; Supplementary Figure 1), the more likely the plastic is to be influenced by ballasting biofilm communities. However, many forms of polystyrene, such as foams, are strongly positively buoyant simply due to air enclosures.
In this study, we were unable to identify any microbial organisms with the capacity to generate calcium carbonate shells or tests, therefore, it is improbable to consider their presence would have exerted a distinct influence on buoyancy. Coccolithophores, the principal autotrophs responsible for calcium carbonate secretion, are predominantly pelagic and not typically associated with biofilm communities. They have been documented embedded in the biofilm only in a single study and even then, their prevalence was considerably less compared to that of diatoms (Reisser et al., 2014). While cyanobacterial-mediated carbonate precipitation has been reported to impact the buoyancy and export of plastics to deeper water (Leiser et al., 2021), it is essential to note that this effect was observed in a eutrophic freshwater reservoir. In the Paleozoic and Mesozoic, calcifying cyanobacteria were common in the marine environment. While modern marine chemistry still allows for thermodynamically favorable conditions for carbonate precipitation near the ocean surface, calcifying cyanobacteria predominantly occur in shallow freshwater streams and lakes over limestone (Leadbeater and Riding, 1986; Merz, 1992; Merz-Preiss, 2000; Riding, 1991). As a result, diatoms are the dominant microorganisms responsible for mineralizing ballast in the marine plastisphere.
Our experimental data thus support the conclusions of Kaiser et al. (2017); Wright et al. (2020); Amaral-Zettler et al. (2021) and most recently, the theoretical considerations by Benner and Passow (2024), who considered that biofilms alone are unlikely to cause most buoyant MPD larger than 15-40 µm to sink. Previous studies highlighting the sinking of buoyant plastics by biology have noted the significant role played by colonization of multicellular lifeforms, especially metazoans containing calcium carbonate exoskeletons and shells such as bryozoans, barnacles, and mussels (Fazey and Ryan, 2016; Kaiser et al., 2017; Ye and Andrady, 1991). While multicellular lifeforms exist as a set within the larger context of biofouling, microbial biofilms alone are unlikely to cause negative buoyancy in MPDs.
In contrast to diatom-derived biogenic silica, many other components of biofilms are neutral, or even positively buoyant as investigations on gels such as transparent exopolymer particles (similar composition to EPS) have concluded (Azetsu-Scott and Passow, 2004). In addition, some phytoplankton that inhabit the microbial plastisphere communities (Carson et al., 2013; Dang and Lovell, 2015; Kaiser et al., 2017; Zettler et al., 2013) have evolved mechanisms such as the storage of lipids, ion exchange, and formation of gas vacuoles to increase buoyancy and prevent their sinking out of the euphotic zone (Fernández-Méndez et al., 2014; Smayda, 1970; Smetacek, 1985; Waite et al., 1992). Any gas bubble formation in biofilms (e.g., oxygen or methane) also increases buoyancy. Furthermore, the consistent formation of biofilm thick enough to achieve the mineralization levels needed to ballast buoyant MPDs is unlikely in open ocean environments, where dynamic conditions can lead to biofilm instability and detachment. The concept of biogenic ballasting used to model the export of plastics from the surface was built on the assumption that microbial biofilms contribute to these buoyancy changes (Kooi et al., 2017; Kvale et al., 2020a; Lobelle et al., 2021; Van Melkebeke et al., 2020), while in contrast, most of the ballasting is more likely due to macrofauna (Amaral-Zettler et al., 2021; Kaiser et al., 2017).
If microbial biofilms are unlikely to cause sinking, how do buoyant MPs make it to the deep sea (Abel et al., 2020, 2022; Choy et al., 2019; Li et al., 2020; Pabortsava and Lampitt, 2020; Tekman et al., 2020)? In estuarine systems with high suspended sediment load, sediments can conceivably be trapped in the EPS in sufficient amounts to induce sinking. Möhlenkamp et al. (2018) incubated buoyant MP beads of unknown polymer types and various sizes from exfoliant skin care products in a roller tank with estuarine riverbed sediments for 72 hours and found that MPs did become entrapped and settled. Sediment entrapment in the EPS is unlikely a major factor in the open ocean, however, given the miniscule amount of sediment in that environment.
Another possibility leading to deep-sea transport of buoyant MPs is the entrapment of small plastic particles in marine snow that subsequently sinks through the water column. However, it would require that marine snow itself is heavily ballasted by dense organic material (e.g., fecal pellets), silica, and calcium carbonate (Bochdansky and Herndl, 1992; Honda and Watanabe, 2010; Ploug et al., 2008). By analogy, Daly et al. (2016) observed during the Deep Horizon spill in the Gulf of Mexico that positively buoyant materials such as oil can be transported to the deep sea via marine snow. Marine snow may thus be an effective vector to inject otherwise buoyant plastic particles into the ocean (Ziervogel et al., 2024).
Long et al. (2015) investigated the impact of aggregates on buoyancy by culturing two pelagic algae, the diatom Chaetoceros neogracile and the cryptophyte Rhodomonas salina, and transferring them to roller tanks. Aggregates of both cultures incorporated PS beads and sank, however, experiments with dense algal cultures producing unrealistically dense algal pellets are not representative of marine snow in the field.
Another vector of plastics transport to the deep ocean is direct ingestion and incorporation into fast-sinking fecal pellets (Katija et al., 2017; Kvale et al., 2020a, b; Shore et al., 2021). This may occur in a variety of crustacean (e.g., copepods, euphausiids) and tunicate (e.g., appendicularians, salps, doliolids) plankton. In particular, non-selective filter feeders such as salps and appendicularians will take up plastics non-selectively and at the same proportion as they exist in the environment. These organisms produce fast-sinking fecal pellets with velocities of 100 m to 2 km d-1 (Bruland and Silver, 1981; Madin, 1982; Ploug et al., 2008). The interactions extend beyond invertebrates to vertebrates, which can consume zooplankton and other organisms that have ingested plastics (López-Martínez et al., 2021). This trophic transfer further encapsulates microplastics within sinking biological material, effectively removing them from the surface waters. These processes highlight the crucial role of the food chain in changing the distribution of plastic debris within marine environments, underscoring the intricate biological pathways that contribute to the deep-sea deposition of plastics. However, subsequent fragmentation and decay of the organic material and the peritrophic membranes of fecal pellets may leave the refractory plastic particles behind.
In addition to biological factors, physical processes such as turbulent mixing and the seasonal mixed-layer pump can also influence the transport of microplastics to deeper waters. Although previous research has primarily demonstrated that these physical processes are responsible for the transport of organic matter (Dall’Olmo et al., 2016; Ezer, 2023; Giering et al., 2016; Lacour et al., 2019), it is plausible that these mechanisms could affect microplastics, although at this stage it is not clear whether the turbulent energy is able to overcome the buoyancy of the plastics and the surface tension of the water.
Certainly, the factors discussed above, including biofilms, invertebrate colonization, and physical processes, work in concert to influence plastic sinking in a marine context. Biofilm organisms, while crucial for initiating changes in the surface characteristics and potentially contributing to mineral deposition, typically exert limited influence on their own. However, when combined with invertebrates such as bryozoans and barnacles, which have substantial ballast effects due to their calcium carbonate structures, the likelihood of achieving negative buoyancy increases significantly. Additionally, physical factors such as ocean currents, turbulence, and sediment loading can further aid in the downward transport of plastics by enhancing biofilm adherence or facilitating sediment entrapment. Understanding the interplay between these biological and physical mechanisms is essential for a more comprehensive view of how plastics transition from buoyant to negatively buoyant states.
5 Conclusion
The combined 16S, 18S, and ITS data fills a critical gap, offering a more integrated view of biofilm composition. This approach facilitates a deeper understanding of microbial interactions and the specific roles different taxa play in biofilm formation and the fate of plastics within the environment. Moving forward, expanding the use of comprehensive sequencing methods is essential for uncovering the complex dynamics of the plastisphere.
In our experiments, microbial growth rapidly spread across the surface of immersed plastics, beginning with the accumulation of EPS before bacteria colonized the surface in approximately one day. Fungi were also early colonizers, possibly due to their role in metabolizing recalcitrant compounds. Within a few weeks, the surface was covered by a variety of other eukaryotes. Our microscopic, lipid, pigment, and metagenetics analyses showed that the only microbes that could have appreciably contributed to an increase in ballast were diatoms with their associated biogenic silica frustules. In contrast, no calcifying organisms were present in the various microbial biofilm communities. Even under the most favorable conditions (i.e., under silica-enhanced nutrient conditions) and many months of biofilm growth under protected conditions, we were unable to induce plastic particles to become negatively buoyant in experiments.
Our data of maximum biogenic silica accumulation from the in-situ experiments applied to various thicknesses of HDPE sheets suggests that microbial biofilms are unlikely to significantly influence the buoyancy of originally positively buoyant HDPE particles unless they are thinner than 15-40 µm depending on the shape. Mechanisms that transport buoyant microplastics into deeper waters and the large amount of buoyant plastics found in deep ocean environments thus remain an enigma.
Data availability statement
The original contributions presented in the study are included in the article/Supplementary Material, further inquiries can be directed to the corresponding author/s.
Author contributions
GJ: Conceptualization, Data curation, Formal Analysis, Funding acquisition, Investigation, Methodology, Project administration, Resources, Validation, Visualization, Writing – original draft, Writing – review & editing. RP-P: Formal Analysis, Funding acquisition, Investigation, Methodology, Resources, Validation, Writing – review & editing. XJ: Formal Analysis, Investigation, Writing – review & editing. ZL: Funding acquisition, Methodology, Resources, Validation, Writing – review & editing. AB: Conceptualization, Funding acquisition, Methodology, Resources, Supervision, Visualization, Writing – review & editing.
Funding
The author(s) declare that financial support was received for the research and/or publication of this article. Financial support for this study came from Old Dominion University graduate student fellowships (GMJ) and the National Science Foundation awards #2319114 (ABB), #2033828(ZL), and #2033860(RP-P).
Acknowledgments
We would like to thank and acknowledge Mauricio González Díaz (ODU) and Jianhong Xue (UT) for assistance with sample collection and analysis, Rodger Harvey (ODU) for access to his private dock for the incubations, Chase Glatz (MBL) for assistance with the SEM, and Rebecca Hopkins (MBL) for assistance with lipid extractions.
Conflict of interest
The authors declare that the research was conducted in the absence of any commercial or financial relationships that could be construed as a potential conflict of interest.
The author(s) declared that they were an editorial board member of Frontiers, at the time of submission. This had no impact on the peer review process and the final decision.
Generative AI statement
The author(s) declare that no Generative AI was used in the creation of this manuscript.
Publisher’s note
All claims expressed in this article are solely those of the authors and do not necessarily represent those of their affiliated organizations, or those of the publisher, the editors and the reviewers. Any product that may be evaluated in this article, or claim that may be made by its manufacturer, is not guaranteed or endorsed by the publisher.
Supplementary material
The Supplementary Material for this article can be found online at: https://www.frontiersin.org/articles/10.3389/fmars.2025.1581727/full#supplementary-material
Supplementary Figure 1 | Plastic polymer types and their associated density and cumulative historical global plastic production percentages. Brown indicates the polymer is naturally denser than seawater, blue is less dense, and grey straddles the density boundary.
Supplementary Figure 2 | Lipid abundance with time, shades indicate lowest value (red) to highest value (green).
Supplementary Figure 3 | Calculations used to create Figures 6 and 7.
Supplementary Figure 4 | Link to KRONA metagenetics plots.
Supplementary Figure 5 | RTL Genomics Supporting Documentation.
References
Abel S., Primpke S., Int-Veen I., Brandt A., and Gerdts G. (2020). Systematic identification of microplastics in abyssal and hadal sediments of the Kuril Kamchatka trench. Environ. Pollution 269, 116095. doi: 10.1016/j.envpol.2020.116095
Abel S. M., Primpke S., Wu F., Brandt A., and Gerdts G. (2022). Human footprints at hadal depths: interlayer and intralayer comparison of sediment cores from the Kuril Kamchatka trench. Sci. Total Environ. 838, 156035. doi: 10.1016/j.scitotenv.2022.156035
Amaral-Zettler L. A., Zettler E. R., Mincer T. J., Klaassen M. A., and Gallager S. M. (2021). Biofouling impacts on polyethylene density and sinking in coastal waters: A macro/micro tipping point? Water Res. 201, 117289. doi: 10.1016/j.watres.2021.117289
Armstrong R. A., Peterson M. L., Lee C., and Wakeham S. G. (2009). Settling velocity spectra and the ballast ratio hypothesis. Deep Sea Res. Part II: Topical Stud. Oceanography 56, 1470–1478. doi: 10.1016/j.dsr2.2008.11.032
Artham T., Sudhakar M., Venkatesan R., Madhavan Nair C., Murty K. V. G. K., and Doble M. (2009). Biofouling and stability of synthetic polymers in sea water. Int. Biodeterioration Biodegradation 63, 884–890. doi: 10.1016/j.ibiod.2009.03.003
Azetsu-Scott K. and Passow U. (2004). Ascending marine particles: Significance of transparent exopolymer particles (TEP) in the upper ocean. Limnology Oceanography 49, 741–748. doi: 10.4319/lo.2004.49.3.0741
Benner I. and Passow U. (2024). Why biofouling cannot contribute to the vertical transport of small microplastic. Micropl.Nanopl. 4, 19. doi: 10.1186/s43591-024-00098-2
Bhagwat G., O’Connor W., Grainge I., and Palanisami T. (2021). Understanding the fundamental basis for biofilm formation on plastic surfaces: role of conditioning films. Front. Microbiol. 12. doi: 10.3389/fmicb.2021.687118
Bochdansky A. and Herndl G. (1992). Ecology of amorphous aggregations (marine snow) in the Northern Adriatic Sea. V. Role of fecal pellets in marine snow. Marine Ecol. Prog. Ser. 89, 297–303. doi: 10.3354/meps089297
Bochdansky A. B., Stouffer A. N., and Washington N. N. (2021). Adenosine triphosphate (ATP) as a metric of microbial biomass in aquatic systems: new simplified protocols, laboratory validation, and a reflection on data from the literature. Limnology Oceanography: Methods 19, 115–131. doi: 10.1002/lom3.10409
Bruland K. W. and Silver M. W. (1981). Sinking rates of fecal pellets from gelatinous zooplankton (Salps, Pteropods, Doliolids). Marine Biol. 63, 295–300. doi: 10.1007/BF00395999
Carson H. S., Nerheim M. S., Carroll K. A., and Eriksen M. (2013). The plastic-associated microorganisms of the North Pacific Gyre. Marine Pollution Bull. 75, 126–132. doi: 10.1016/j.marpolbul.2013.07.054
Cavaliere R., Ball J. L., Turnbull L., and Whitchurch C. B. (2014). The biofilm matrix destabilizers, EDTA and DNaseI, enhance the susceptibility of nontypeable Hemophilus influenzae biofilms to treatment with ampicillin and ciprofloxacin. MicrobiologyOpen 3, 557–567. doi: 10.1002/mbo3.187
Chen X., Xiong X., Jiang X., Shi H., and Wu C. (2019). Sinking of floating plastic debris caused by biofilm development in a freshwater lake. Chemosphere 222, 856–864. doi: 10.1016/j.chemosphere.2019.02.015
Choy C. A., Robison B. H., Gagne T. O., Erwin B., Firl E., Halden R. U., et al. (2019). The vertical distribution and biological transport of marine microplastics across the epipelagic and mesopelagic water column. Sci. Rep. 9, 1–9. doi: 10.1038/s41598-019-44117-2
Christie W. W. (1982). Lipid analysis: isolation, separation, identification, and structural analysis of lipids. 2nd Edn (Oxford UK: Pergamon Press).
Conley D. J. (1999). “Biogeochemical nutrient cycles and nutrient management strategies,” in Man and river systems: the functioning of river systems at the basin scale. Eds. Garnier J. and Mouchel J.-M. (Springer Netherlands, Dordrecht), 87–96. doi: 10.1007/978-94-017-2163-9_10
Costerton J. W., Stewart P. S., and Greenberg E. P. (1999). Bacterial biofilms: A common cause of persistent infections. Science 284, 1318–1322. doi: 10.1126/science.284.5418.1318
Cózar A., Echevarría F., González-Gordillo J. I., Irigoien X., Úbeda B., Hernández-León S., et al. (2014). Plastic debris in the open ocean. Proc. Natl. Acad. Sci. 111, 10239–10244. doi: 10.1073/pnas.1314705111
Dall’Olmo G., Dingle J., Polimene L., Brewin R. J. W., and Claustre H. (2016). Substantial energy input to the mesopelagic ecosystem from the seasonal mixed-layer pump. Nat. Geosci 9, 820–823. doi: 10.1038/ngeo2818
Daly K. L., Passow U., Chanton J., and Hollander D. (2016). Assessing the impacts of oil-associated marine snow formation and sedimentation during and after the Deepwater Horizon oil spill. Anthropocene 13, 18–33. doi: 10.1016/j.ancene.2016.01.006
Dang H. and Lovell C. R. (2015). Microbial surface colonization and biofilm development in marine environments. Microbiol. Mol. Biol. Reviews : MMBR 80, 91–138. doi: 10.1128/MMBR.00037-15
Davidov K., Iankelevich-Kounio E., Yakovenko I., Koucherov Y., Rubin-Blum M., and Oren M. (2020). Identification of plastic-associated species in the Mediterranean Sea using DNA metabarcoding with Nanopore MinION. Sci. Rep. 10, 17533. doi: 10.1038/s41598-020-74180-z
Debroas D., Mone A., and Ter Halle A. (2017). Plastics in the North Atlantic garbage patch: A boat-microbe for hitchhikers and plastic degraders. Sci. Total Environ. 599–600, 1222–1232. doi: 10.1016/j.scitotenv.2017.05.059
De Carvalho C. C. C. R. and Caramujo M. J. (2012). Lipids of prokaryotic origin at the base of marine food webs. Marine Drugs 10, 2698–2714. doi: 10.3390/md10122698
Decho A. W. (2000). Microbial biofilms in intertidal systems: an overview. Continental Shelf Res. 20, 1257–1273. doi: 10.1016/S0278-4343(00)00022-4
DeForest J. L., Drerup S. A., and Vis M. L. (2016). Using fatty acids to fingerprint biofilm communities: a means to quickly and accurately assess stream quality. Environ. Monit Assess. 188, 277. doi: 10.1007/s10661-016-5290-7
De Tender C., Devriese L. I., Haegeman A., Maes S., Vangeyte J., Cattrijsse A., et al. (2017). Temporal dynamics of bacterial and fungal colonization on plastic debris in the north sea. Environ. Sci. Technol. 51, 7350–7360. doi: 10.1021/acs.est.7b00697
Du J. and Shen J. (2016). Water residence time in Chesapeake Bay for 1980–2012. J. Marine Syst. 164, 101–111. doi: 10.1016/j.jmarsys.2016.08.011
Eriksen M., Lebreton L. C. M., Carson H. S., Thiel M., Moore C. J., Borerro J. C., et al. (2014). Plastic Pollution in the World’s Oceans: More than 5 Trillion Plastic Pieces Weighing over 250,000 Tons Afloat at Sea. PloS One 9, e111913. doi: 10.1371/journal.pone.0111913
Ezer T. (2023). Evaluation of the applicability of the Ekman theory for wind-driven ocean currents: a comparison with the Mellor–Yamada turbulent model. Ocean Dynamics 73, 575–591. doi: 10.1007/s10236-023-01570-y
Fanning K. A. and Pilson M. (1973). On the Spectrophotometric determination of dissolved silica in natural waters. Anal. Chem. 45, 136–140. doi: 10.1021/ac60323a021
Fazey F. M. C. and Ryan P. G. (2016). Biofouling on buoyant marine plastics: An experimental study into the effect of size on surface longevity. Environ. Pollution 210, 354–360. doi: 10.1016/j.envpol.2016.01.026
Fernández-Méndez M., Wenzhöfer F., Peeken I., Sørensen H. L., Glud R. N., and Boetius A. (2014). Composition, buoyancy regulation and fate of ice algal aggregates in the central arctic ocean. PloS One 9, e107452. doi: 10.1371/journal.pone.0107452
Galgani L., Goßmann I., Scholz-Böttcher B., Jiang X., Liu Z., Scheidemann L., et al. (2022). Hitchhiking into the Deep: How Microplastic Particles are Exported through the Biological Carbon Pump in the North Atlantic Ocean. Environ. Sci. Technol. 56, 15638–15649. doi: 10.1021/acs.est.2c04712
Giering S. L. C., Sanders R., Martin A. P., Lindemann C., Möller K. O., Daniels C. J., et al. (2016). High export via small particles before the onset of the North Atlantic spring bloom. J. Geophysical Research: Oceans 121, 6929–6945. doi: 10.1002/2016JC012048
Guillard R. R. L. (1975). “Culture of phytoplankton for feeding marine invertebrates,” in Culture of marine invertebrate animals: proceedings — 1st conference on culture of marine invertebrate animals greenport. Eds. Smith W. L. and Chanley M. H. (Springer US, Boston, MA), 29–60. doi: 10.1007/978-1-4615-8714-9_3
Gündoğdu S., Çevik C., and Karaca S. (2017). Fouling assemblage of benthic plastic debris collected from Mersin Bay, NE Levantine coast of Turkey. Marine Pollution Bull. 124, 147–154. doi: 10.1016/j.marpolbul.2017.07.023
Hall-Stoodley L., Costerton J. W., and Stoodley P. (2004). Bacterial biofilms: from the Natural environment to infectious diseases. Nat. Rev. Microbiol. 2, 95–108. doi: 10.1038/nrmicro821
Halsey K. H. and Jones B. M. (2015). Phytoplankton strategies for photosynthetic energy allocation. Annu. Rev. Marine Sci. 7, 265–297. doi: 10.1146/annurev-marine-010814-015813
Holmström A. (1975). Plastic films on the bottom of the Skagerack. Nature 255, 622–623. doi: 10.1038/255622a0
Honda M. C. and Watanabe S. (2010). Importance of biogenic opal as ballast of particulate organic carbon (POC) transport and existence of mineral ballast-associated and residual POC in the Western Pacific Subarctic Gyre. Geophysical Res. Lett. 37. doi: 10.1029/2009GL041521
Hurd D. C. and Theyer F. (1977). Changes in the physical and chemical properties of biogenic silica from the central equatorial Pacific; Part II, Refractive index, density, and water content of acid-cleaned samples. AJS 277, 1168–1202. doi: 10.2475/ajs.277.9.1168
Jefferson K. K. (2004). What drives bacteria to produce a biofilm? FEMS Microbiol. Lett. 236, 163–173. doi: 10.1111/j.1574-6968.2004.tb09643.x
Kaiser D., Kowalski N., and Waniek J. J. (2017). Effects of biofouling on the sinking behavior of microplastics. Environ. Res. Lett. 12, 124003. doi: 10.1088/1748-9326/aa8e8b
Karkanorachaki K., Syranidou E., and Kalogerakis N. (2021). Sinking characteristics of microplastics in the marine environment. Sci. Total Environ. 793, 148526. doi: 10.1016/j.scitotenv.2021.148526
Katija K., Choy C. A., Sherlock R. E., Sherman A. D., and Robison B. H. (2017). From the surface to the seafloor: How giant larvaceans transport microplastics into the deep sea. Sci. Adv. 3, e1700715. doi: 10.1126/sciadv.1700715
Kettner M. T., Oberbeckmann S., Labrenz M., and Grossart H.-P. (2019). The eukaryotic life on microplastics in brackish ecosystems. Front. Microbiol. 10. doi: 10.3389/fmicb.2019.00538
Kettner M. T., Rojas-Jimenez K., Oberbeckmann S., Labrenz M., and Grossart H.-P. (2017). Microplastics alter composition of fungal communities in aquatic ecosystems. Environ. Microbiol. 19, 4447–4459. doi: 10.1111/1462-2920.13891
Kirstein I. V., Wichels A., Krohne G., and Gerdts G. (2018). Mature biofilm communities on synthetic polymers in seawater - Specific or general? Marine Environ. Res. 142, 147–154. doi: 10.1016/j.marenvres.2018.09.028
Koelmel J. P., Napolitano M. P., Ulmer C. Z., Vasiliou V., Garrett T. J., Yost R. A., et al. (2020). Environmental lipidomics: understanding the response of organisms and ecosystems to a changing world. Metabolomics 16, 56. doi: 10.1007/s11306-020-01665-3
Kooi M., Nes E. H., Scheffer M., and Koelmans A. A. (2017). Ups and downs in the ocean: effects of biofouling on vertical transport of microplastics. Environ. Sci. Technol. 51, 7963–7971. doi: 10.1021/acs.est.6b04702
Kvale K., Friederike Prowe A. E., Chien C. T., Landolfi A., and Oschlies A. (2020b). The global biological microplastic particle sink. Sci. Rep. 10, 16670. doi: 10.1038/s41598-020-72898-4
Kvale K. F., Friederike Prowe A. E., and Oschlies A. (2020a). A critical examination of the role of marine snow and zooplankton fecal pellets in removing ocean surface microplastic. Front. Mar. Sci. 6. doi: 10.3389/fmars.2019.00808
Lacour L., Briggs N., Claustre H., Ardyna M., and Dall’Olmo G. (2019). The intraseasonal dynamics of the mixed layer pump in the subpolar north atlantic ocean: A biogeochemical-argo float approach. Global Biogeochemical Cycles 33, 266–281. doi: 10.1029/2018GB005997
Lagarde F., Olivier O., Zanella M., Daniel P., Hiard S., and Caruso A. (2016). Microplastic interactions with freshwater microalgae: Hetero-aggregation and changes in plastic density appear strongly dependent on polymer type. Environ. Pollution 215, 331–339. doi: 10.1016/j.envpol.2016.05.006
Lambert C. D., Bianchi T. S., and Santschi P. H. (1999). Cross-shelf changes in phytoplankton community composition in the Gulf of Mexico (Texas shelf/slope): the use of plant pigments as biomarkers. Continental Shelf Res. 19, 1–21. doi: 10.1016/S0278-4343(98)00075-2
Leadbeater B. S. C. and Riding R. (1986). Biomineralization in lower plants and animals (Oxford Oxfordshire : New York: Oxford University Press).
Lee J.-W., Nam J.-H., Kim Y.-H., Lee K.-H., and Lee D.-H. (2008). Bacterial communities in the initial stage of marine biofilm formation on artificial surfaces. J. Microbiol. 46, 174–182. doi: 10.1007/s12275-008-0032-3
Leiser R., Jongsma R., Bakenhus I., Möckel R., Philipp B., Neu T. R., et al. (2021). Interaction of cyanobacteria with calcium facilitates the sedimentation of microplastics in a eutrophic reservoir. Water Res. 189, 116582. doi: 10.1016/j.watres.2020.116582
Letelier R. M., Bidigare R. R., Hebel D. V., Ondrusek M., Winn C. D., and Karl D. M. (1993). Temporal variability of phytoplankton community structure based on pigment analysis. Limnology Oceanography 38, 1420–1437. doi: 10.4319/lo.1993.38.7.1420
Li D., Liu K., Li C., Peng G., Andrady A. L., Wu T., et al. (2020). Profiling the vertical transport of microplastics in the west pacific ocean and the east Indian ocean with a novel in situ filtration technique. Environ. Sci. Technol. 54, 12979–12988. doi: 10.1021/acs.est.0c02374
Liu Z. and Xue J. (2020). The lability and source of particulate organic matter in the northern gulf of Mexico hypoxic zone. J. Geophys. Res. Biogeosci. 125. doi: 10.1029/2020JG005653
Lobelle D. and Cunliffe M. (2011). Early microbial biofilm formation on marine plastic debris. Marine Pollution Bull. 62, 197–200. doi: 10.1016/j.marpolbul.2010.10.013
Lobelle D., Kooi M., Koelmans A. A., Laufkötter C., Jongedijk C. E., Kehl C., et al. (2021). Global modeled sinking characteristics of biofouled microplastic. J. Geophysical Research: Oceans 126, e2020JC017098. doi: 10.1029/2020JC017098
Lohan K. M. P., DiMaria R., Martin D. L., Ross C., and Ruiz G. M. (2020). Diversity and microhabitat associations of Labyrinthula spp. in the Indian River Lagoon System. Dis. Aquat. Organisms 137, 145–157. doi: 10.3354/dao03431
Long M., Moriceau B., Gallinari M., Lambert C., Huvet A., Raffray J., et al. (2015). Interactions between microplastics and phytoplankton aggregates: Impact on their respective fates. Marine Chem. 175, 39–46. doi: 10.1016/j.marchem.2015.04.003
López D., Vlamakis H., and Kolter R. (2010). Biofilms. Cold Spring Harb Perspect. Biol. 2, a000398. doi: 10.1101/cshperspect.a000398
López-Martínez S., Morales-Caselles C., Kadar J., and Rivas M. L. (2021). Overview of global status of plastic presence in marine vertebrates. Global Change Biol. 27, 728–737. doi: 10.1111/gcb.15416
Madin L. P. (1982). Production, composition and sedimentation of salp fecal pellets in oceanic waters. Mar. Biol. 67, 39–45. doi: 10.1007/BF00397092
Marshall H. G., Burchardt L., and Lacouture R. (2005). A review of phytoplankton composition within Chesapeake Bay and its tidal estuaries. J. Plankton Res. 27, 1083–1102. doi: 10.1093/plankt/fbi079
Merz M. U. E. (1992). The biology of carbonate precipitation by cyanobacteria. Facies 26, 81–101. doi: 10.1007/BF02539795
Merz-Preiss M. (2000). “Calcification in cyanobacteria,” in Microbial sediments. Eds. Riding R. E. and Awramik S. M. (Springer, Berlin, Heidelberg), 50–56. doi: 10.1007/978-3-662-04036-2_7
Michels J., Stippkugel A., Lenz M., Wirtz K., and Engel A. (2018). Rapid aggregation of biofilm-covered microplastics with marine biogenic particles. Proc. R. Soc. B: Biol. Sci. 285, 20181203. doi: 10.1098/rspb.2018.1203
Möhlenkamp P., Purser A., and Thomsen L. (2018). Plastic microbeads from cosmetic products: an experimental study of their hydrodynamic behaviour, vertical transport and resuspension in phytoplankton and sediment aggregates. Elementa: Sci. Anthropocene 6, 61. doi: 10.1525/elementa.317
Mouritsen O. G. and Zuckermann M. J. (2004). What’s so special about cholesterol? Lipids 39, 1101–1113. doi: 10.1007/s11745-004-1336-x
Mulholland M. R., Macías-Tapia A., and Loftis J. D. (2022). “Water quality impacts from tidal flooding in Southern Chesapeake Bay,” in OCEANS 2022 (Hampton Roads: IEEE), 1–5. doi: 10.1109/OCEANS47191.2022.9977117
Oberbeckmann S., Loeder M. G. J., Gerdts G., and Osborn A. M. (2014). Spatial and seasonal variation in diversity and structure of microbial biofilms on marine plastics in Northern European waters. FEMS Microbiol. Ecol. 90, 478–492. doi: 10.1111/1574-6941.12409
Oberbeckmann S., Osborn A. M., and Duhaime M. B. (2016). Microbes on a bottle: substrate, season and geography influence community composition of microbes colonizing marine plastic debris. PloS One 11, e0159289. doi: 10.1371/journal.pone.0159289
Paasche E. (1980). Silicon content of five marine plankton diatom species measured with a rapid filter method. Limnology Oceanography 25, 474–480. doi: 10.4319/lo.1980.25.3.0474
Pabortsava K. and Lampitt R. S. (2020). High concentrations of plastic hidden beneath the surface of the Atlantic Ocean. Nat. Commun. 11, 4073. doi: 10.1038/s41467-020-17932-9
Parsons T. R., Maita Y., and Lalli C. M. (1984). “4.2 - spectrophotometric determination of phaeo-pigments,” in A manual of chemical & Biological methods for seawater analysis. Eds. Parsons T. R., Maita Y., and Lalli C. M. (Pergamon, Amsterdam), 104–107. doi: 10.1016/B978-0-08-030287-4.50033-5
Passow U. (2000). Formation of transparent exopolymer particles, TEP, from dissolved precursor material. Marine Ecol. Prog. Ser. 192, 1–11. doi: 10.3354/meps192001
Pedrosa-Pàmies R., Conte M. H., Weber J. C., and Johnson R. (2018). Carbon cycling in the Sargasso Sea water column: Insights from lipid biomarkers in suspended particles. Prog. Oceanography 168, 248–278. doi: 10.1016/j.pocean.2018.08.005
Pedrosa-Pàmies R., Conte M. H., Weber J. C., and Johnson R. (2019). Hurricanes enhance labile carbon export to the deep ocean. Geophysical Res. Lett. 46, 10484–10494. doi: 10.1029/2019GL083719
Plastics Europe (2023). Plastics - the fast facts 2023 (Brussels, Belgium: Plastics Europe). Available at: https://plasticseurope.org/wp-content/uploads/2023/10/Plasticsthefastfacts2023-1.pdf.
Ploug H., Iversen M. H., and Fischer G. (2008). Ballast, sinking velocity, and apparent diffusivity within marine snow and zooplankton fecal pellets: Implications for substrate turnover by attached bacteria. Limnology Oceanography 53, 1878–1886. doi: 10.4319/lo.2008.53.5.1878
Qian Y., Jochens A. E., Kennicutt M. C. II, and Biggs D. C. (2003). Spatial and temporal variability of phytoplankton biomass and community structure over the continental margin of the northeast Gulf of Mexico based on pigment analysis. Continental Shelf Res. 23, 1–17. doi: 10.1016/S0278-4343(02)00173-5
Qiang L., Cheng J., Mirzoyan S., Kerkhof L. J., and Häggblom M. M. (2021). Characterization of microplastic-associated biofilm development along a freshwater-estuarine gradient. Environ. Sci. Technol. 55, 16402–16412. doi: 10.1021/acs.est.1c04108
Rabnawaz M., Wyman I., Auras R., and Cheng S. (2017). A roadmap towards green packaging: the current status and future outlook for polyesters in the packaging industry. Green Chem. 19, 4737–4753. doi: 10.1039/C7GC02521A
Raghukumar S. (2002). Ecology of the marine protists, the Labyrinthulomycetes (Thraustochytrids and Labyrinthulids). Eur. J. Protistology 38, 127–145. doi: 10.1078/0932-4739-00832
Reis S. N. and Aldridge D. C. (2024). Biofilm development on macroplastics along a freshwater-estuarine gradient with attention to diatom assemblages. ACS EST Water 4, 5522–5530. doi: 10.1021/acsestwater.4c00595
Reisser J., Shaw J., Hallegraeff G., Proietti M., Barnes D. K. A., Thums M., et al. (2014). Millimeter-sized marine plastics: A new pelagic habitat for microorganisms and invertebrates. PloS One 9, e100289. doi: 10.1371/journal.pone.0100289
Reyna N. E., Hardison A. K., and Liu Z. (2017). Influence of major storm events on the quantity and composition of particulate organic matter and the phytoplankton community in a subtropical estuary, texas. Front. Mar. Sci. 4. doi: 10.3389/fmars.2017.00043
Riding R. (1991). “Calcified cyanobacteria,” in Calcareous algae and stromatolites. Ed. Riding R. (Springer, Berlin, Heidelberg), 55–87. doi: 10.1007/978-3-642-52335-9_3
Rummel C. D., Lechtenfeld O. J., Kallies R., Benke A., Herzsprung P., Rynek R., et al. (2021). Conditioning film and early biofilm succession on plastic surfaces. Environ. Sci. Technol. 55, 11006–11018. doi: 10.1021/acs.est.0c07875
Sanders R., Morris P. J., Poulton A. J., Stinchcombe M. C., Charalampopoulou A., Lucas M. I., et al. (2010). Does a ballast effect occur in the surface ocean? Geophysical Res. Lett. 37. doi: 10.1029/2010GL042574
Schuster L. and Bright M. (2016). A Novel Colonial Ciliate Zoothamnium ignavum sp. nov. (Ciliophora, Oligohymenophorea) and Its Ectosymbiont Candidatus Navis piranensis gen. nov., sp. nov. from Shallow-Water Wood Falls. PloS One 11, e0162834. doi: 10.1371/journal.pone.0162834
Semcesen P. O. and Wells M. G. (2021). Biofilm growth on buoyant microplastics leads to changes in settling rates: Implications for microplastic retention in the Great Lakes. Marine Pollution Bull. 170, 112573. doi: 10.1016/j.marpolbul.2021.112573
Shabala L., McMeekin T., and Shabala S. (2009). Osmotic adjustment and requirement for sodium in marine protist thraustochytrid. Environ. Microbiol. 11, 1835–1843. doi: 10.1111/j.1462-2920.2009.01908.x
Shively S. and Miller W. R. (2009). The use of HMDS (hexamethyldisilazane) to replace critical point drying (CPD) in the preparation of tardigrades for SEM (Scanning electron microscope) imaging. tkas 112, 198–200. doi: 10.1660/062.112.0407
Shore E. A., deMayo J. A., and Pespeni M. H. (2021). Microplastics reduce net population growth and fecal pellet sinking rates for the marine copepod, Acartia tonsa. Environ. Pollut. 284, 117379. doi: 10.1016/j.envpol.2021.117379
Smayda T. J. (1970). The suspension and sinking of phytoplankton in the sea. Oceanography Marine Biol. 8, 353–414.
Smetacek V. S. (1985). Role of sinking in diatom life-history cycles: ecological, evolutionary and geological significance. Mar. Biol. 84, 239–251. doi: 10.1007/BF00392493
Song X., Lyu M., Zhang X., Ruthensteiner B., Ahn I.-Y., Pastorino G., et al. (2021). Large plastic debris dumps: new biodiversity hot spots emerging on the deep-Sea floor. Environ. Sci. Technol 8, 148–154. doi: 10.1021/acs.estlett.0c00967
Strickland J. D. H. and Parsons T. R. (1972). A practical handbook of seawater analysis (Ottawa: Fisheries Research Board of Canada).
Sudhakar M., Trishul A., Doble M., Suresh Kumar K., Syed Jahan S., Inbakandan D., et al. (2007). Biofouling and biodegradation of polyolefins in ocean waters. Polymer Degradation Stability 92, 1743–1752. doi: 10.1016/j.polymdegradstab.2007.03.029
Tekman M. B., Wekerle C., Lorenz C., Primpke S., Hasemann C., Gerdts G., et al. (2020). Tying up Loose Ends of Microplastic Pollution in the Arctic: Distribution from the Sea Surface through the Water Column to Deep-Sea Sediments at the HAUSGARTEN Observatory. Environ. Sci. Technol. 54, 4079–4090. doi: 10.1021/acs.est.9b06981
US EPA (2015). Volunteer estuary monitoring: A methods manual. Available online at: https://www.epa.gov/nep/volunteer-estuary-monitoring-methods-manual (Accessed April 3, 2025).
Van Melkebeke M., Janssen C., and De Meester S. (2020). Characteristics and sinking behavior of typical microplastics including the potential effect of biofouling: implications for remediation. Environ. Sci. Technol. 54, 8668–8680. doi: 10.1021/acs.est.9b07378
Wahl M. (1989). Marine epibiosis.1. Fouling and antifouling - some basic aspects. Marine Ecol. Prog. Ser. 58, 175–189. doi: 10.3354/meps058175
Waite A. M., Thompson P. A., and Harrison P. J. (1992). Does energy control the sinking rates of marine diatoms? Limnology Oceanography 37, 468–477. doi: 10.4319/lo.1992.37.3.0468
Wiegand S., Jogler M., and Jogler C. (2018). On the maverick planctomycetes. FEMS Microbiol. Rev. 42, 739–760. doi: 10.1093/femsre/fuy029
Wright R. J., Erni-Cassola G., Zadjelovic V., Latva M., and Christie-Oleza J. A. (2020). Marine plastic debris: A new surface for microbial colonization. Environ. Sci. Technol. 54, 11657–11672. doi: 10.1021/acs.est.0c02305
Wu T., Li Y., Lu B., Shen Z., Song W., and Warren A. (2020). Morphology, taxonomy and molecular phylogeny of three marine peritrich ciliates, including two new species: Zoothamnium apoarbuscula n. sp. and Z. apohentscheli n. sp. (Protozoa, Ciliophora, Peritrichia). Mar Life Sci. Technol. 2, 334–348. doi: 10.1007/s42995-020-00046-y
Ye S. and Andrady A. L. (1991). Fouling of floating plastic debris under Biscayne Bay exposure conditions. Marine Pollution Bull. 22, 608–613. doi: 10.1016/0025-326X(91)90249-R
Zeghal E., Vaksmaa A., Vielfaure H., Boekhout T., and Niemann H. (2021). The potential role of marine fungi in plastic degradation – A review. Front. Mar. Sci. 8, 738877. doi: 10.3389/fmars.2021.738877
Zettler E. R., Mincer T. J., and Amaral-Zettler L. A. (2013). Life in the “Plastisphere”: microbial communities on plastic marine debris. Environ. Sci. Technol. 47, 7137–7146. doi: 10.1021/es401288x
Keywords: biofilm, plastic, buoyancy, ballast, ATP, lipids
Citation: Joern GM, Pedrosa-Pàmies R, Jiang X, Liu Z and Bochdansky AB (2025) Characterization of microbial biofilms on marine plastics: community development and density implications for vertical transport dynamics. Front. Mar. Sci. 12:1581727. doi: 10.3389/fmars.2025.1581727
Received: 23 February 2025; Accepted: 24 April 2025;
Published: 20 May 2025.
Edited by:
Felix Weber, Alfred Wegener Institute Helmholtz Centre for Polar and Marine Research (AWI), GermanyReviewed by:
Youssef Lahbib, Tunis University, TunisiaKatrin Wendt-Potthoff, Helmholtz Association of German Research Centres (HZ), Germany
Copyright © 2025 Joern, Pedrosa-Pàmies, Jiang, Liu and Bochdansky. This is an open-access article distributed under the terms of the Creative Commons Attribution License (CC BY). The use, distribution or reproduction in other forums is permitted, provided the original author(s) and the copyright owner(s) are credited and that the original publication in this journal is cited, in accordance with accepted academic practice. No use, distribution or reproduction is permitted which does not comply with these terms.
*Correspondence: Gregory M. Joern, Z2pvZXIwMDFAb2R1LmVkdQ==