- Univ Lyon, Université Claude Bernard Lyon 1, ISARA Lyon, BioDyMIA (Bioingénierie et Dynamique Microbienne aux Interfaces Alimentaires), Equipe Mixte d’Accueil n°3733, IUT Lyon 1, Technopole Alimentec, Bourg-en-Bresse, France
In recent years, the search for natural plant-based antimicrobial compounds as alternatives to some synthetic food preservatives or biocides has been stimulated by sanitary, environmental, regulatory, and marketing concerns. In this context, besides their established antioxidant activity, the antimicrobial activity of many plant phenolics deserved increased attention. Indeed, industries processing agricultural plants generate considerable quantities of phenolic-rich products and by-products, which could be valuable natural sources of natural antimicrobial molecules. Plant extracts containing volatile (e.g., essential oils) and non-volatile antimicrobial molecules can be distinguished. Plant essential oils are outside the scope of this review. This review will thus provide an overview of current knowledge regarding the promises and the limits of phenolic-rich plant extracts for food preservation and biofilm control on food-contacting surfaces. After a presentation of the major groups of antimicrobial plant phenolics, of their antimicrobial activity spectrum, and of the diversity of their mechanisms of action, their most promising sources will be reviewed. Since antimicrobial activity reduction often observed when comparing in vitro and in situ activities of plant phenolics has often been reported as a limit for their application, the effects of the composition and the microstructure of the matrices in which unwanted microorganisms are present (e.g., food and/or microbial biofilms) on their activity will be discussed. Then, the different strategies of delivery of antimicrobial phenolics to promote their activity in such matrices, such as their encapsulation or their association with edible coatings or food packaging materials are presented. The possibilities offered by encapsulation or association with polymers of packaging materials or coatings to increase the stability and ease of use of plant phenolics before their application, as well as to get systems for their controlled release are presented and discussed. Finally, the necessity to consider phenolic-rich antimicrobial plant extracts in combination with other factors consistently with hurdle technology principles will be discussed. For instance, several authors recently suggested that natural phenolic-rich extracts could not only extend the shelf-life of foods by controlling bacterial contamination, but could also coexist with probiotic lactic acid bacteria in food systems to provide enhanced health benefits to human.
Introduction
Search for natural alternatives to synthetic food preservatives and disinfectants has been the subject of intensive research during the last decade. It has namely been stimulated by increasing concerns regarding their innocuity [e.g., nitrites (EFSA Panel et al., 2017), sulfites (EFSA Panel, 2016), polyhexamethylenebiguanide (PHMB), 5-chloro-2-(2,4-dichlorophenoxy)phenol (triclosan; Müller and Kramer, 2008)] or their environmental impact [e.g., triclosan (Abbott et al., 2020)]. Regulatory changes [e.g., in the European Union (EU)] disinfectants are covered by the European Regulation concerning the marketing and use of biocidal products [Regulation (EU) no 528/2012, 2012] and increasing demand of consumers for organic foods (in which only a limited number of food preservatives are authorized) or for «clean label» food products (without or with a limited number of food additives such as food preservatives) are important drivers of this trend. New natural antimicrobial extracts/molecules are expected (i) to preserve raw foods (e.g., raw fish or meat) or foods minimally processed to better preserve their organoleptic and nutritional properties and (ii) to reduce food waste by extending shelf life of highly perishable foods [one of the objectives of Sustainable Development Goal (SDG) to “End hunger, achieve food security and improved nutrition and promote sustainable agriculture” of United Nations in line with EU objective to reach a 50% food waste reduction by 2030].
In this context, plant extracts are promising sources of antimicrobial molecules. Plant antimicrobial molecules are grouped into different classes based on their chemical structure and properties: essential oils, phenolics, alkaloids, saponins, and peptides (Ferdes, 2018). The present review is focused on phenolics, which are the most numerous among secondary metabolites groups of plants. Plant secondary metabolites (also called phytochemicals) are biosynthesized by plants as a result of biotic (e.g., contamination by phytopathogenic microorganisms) and abiotic factors (e.g., UV light; Harborne, 2001). Some phytochemicals are thus toxic and only edible plants will thus be considered as potential sources of alternative to food preservatives. Another trait of some plant phenols and polyphenols is their biosynthesis in response to seasonal fluctuations in UV light for plant protection (Köhler et al., 2017). This variability of phenolics content of plants as a function of climatic conditions must be kept in mind, when the objective is to produce plant extracts with a standard antimicrobial activity resulting from a given content in antimicrobial phenolics.
As stated by Cheynier et al. (2013), an ever-increasing number of different plant phenolics, representing tens of thousands of different chemical structures, have been identified. Progresses both in analytical techniques, namely mass spectrometry techniques, and in extraction and separation techniques result in a better knowledge of the structural diversity of plant phenolics and of their distribution in plant tissues, as recently reviewed by Piccolella et al. (2019). Essential oil phenolics, which are volatile molecules generally extracted from plants by steam distillation, will be out of the scope of the present review, since their potential use as alternatives to food preservatives (Falleh et al., 2020), as well as their capacity to fight against biofilms (Nuta et al., 2021), have been recently reviewed. Despite their large structural diversity, most antimicrobial plant phenolics belong to six groups: flavonoids, phenolic acids, tannins, stilbenoids, quinones, and coumarins (Bouarab-Chibane et al., 2018a).
The antimicrobial activity of most plant phenolics has been investigated in vitro in microbiological media with a far more simple composition and microstructure than food matrices and against planktonic bacteria. Namely due to interactions of plant phenolics with other food constituents at the expense of their interactions with microorganisms, most antimicrobial plant phenolics have a far lower activity in food than in vitro. A major concern for hygienic safety of food production systems is the presence of microbial biofilms on the surfaces of food production equipment and facilities. Microorganisms in biofilms are embedded in a network of exopolymeric substances. This network of exopolymeric substances limits direct contact of microorganisms with antimicrobial substances, including plant phenolics, exerting thereby a protective effect. Moreover, microorganisms in biofilms are in a particular physiological state, which also modifies their sensitivity to antimicrobial substances. Therefore, after a presentation of the structural diversity of plant phenolics and of their mechanisms of antimicrobial action, a focus on the factors limiting efficiency of antimicrobial plant phenolics in food and biofilms matrices, as well as on their specific mechanisms of action against biofilms, such as quorum sensing inhibition is proposed. Delivery vehicles promoting the antimicrobial activity of plant phenolics in food matrices or against biofilms (e.g., active coatings, films, or particles) are also presented.
Structural Diversity of Antimicrobial Plant Phenolics
Dietary Sources of Phenolics
Phenolics are a class of organic compounds that occurs in all plants as secondary metabolites in varying concentrations. Perez-Jimenez et al. (2010) exploited Phenol-Explorer database to identify the 100 richest dietary sources of polyphenols: they contain from 10 mg per 100 ml (rosé wine) to 15 g per 100 g (cloves). The richest sources are various spices and dried herbs, cocoa products, berries, some seeds, nuts, and some vegetables, including olive and globe artichoke heads. Due to their availability in sufficient amounts for a low cost, some by-products or wastes of phenolic-rich edible plants are promising sources of antimicrobial phenolics. The most studied sources in the 2007–2017 decade for extraction of antimicrobial phenolics were listed by Bouarab-Chibane et al. (2018a) and the potential of application of most of their extracts was recently reviewed by several authors: they include spent coffee (Monente et al., 2015), green tea waste (Siddiqui et al., 2016), olive pomace and olive leaf (Munekata et al., 2020; Difonzo et al., 2021), pomegranate peel (Chen et al., 2020) or aril, grape pomace or seeds (Silva et al., 2021), mango kernel (Mwaurah et al., 2020), myrtle berries seeds (Jabria et al., 2016), dates (Kchaou et al., 2016), walnut green husk (Jahanban-Esfahlan et al., 2019), almond skin (Bolling, 2017), tomato seeds (Taveira et al., 2010; Szabo et al., 2019), buckwheat hull extract (Cabarkapa et al., 2008), pomelo peel (Liu et al., 2017b; Tocmo et al., 2020).
Moreover, for a given plant, phenolics presence and/or concentration greatly varies from a plant tissue to another one: for instance, resveratrol concentration in vine (Vitis vinifera) was reported to vary from less than 1.5 mg/kg of fresh berries (where it is primarily located in skin) to 37 mg/kg in stems (Mikeš et al., 2008). As underlined by Piccolella et al. (2019) in their review, “isolating or detecting phenols and polyphenols necessarily requires a good knowledge of how, when and where these substances are bio-synthesized and stored.”
Antimicrobial Activity of Individual Phenolics
Examples of antimicrobial phenolics belonging to the different structural groups of phenolics (phenolic acids, flavonoids, tannins, stilbenoids, quinones, and coumarins) as well as their minimal inhibitory concentrations (MICs) against various unwanted bacteria and their food sources are presented in Table 1. Examples of phenolic acids belonging to hydroxybenzoic acids and hydroxycinnamic acids are listed, as well as examples of flavonoids belonging to each of its different sub-classes according to their degree of oxidation: flavones, isoflavones, flavanones, flavonols, flavanols, and anthocyanins.
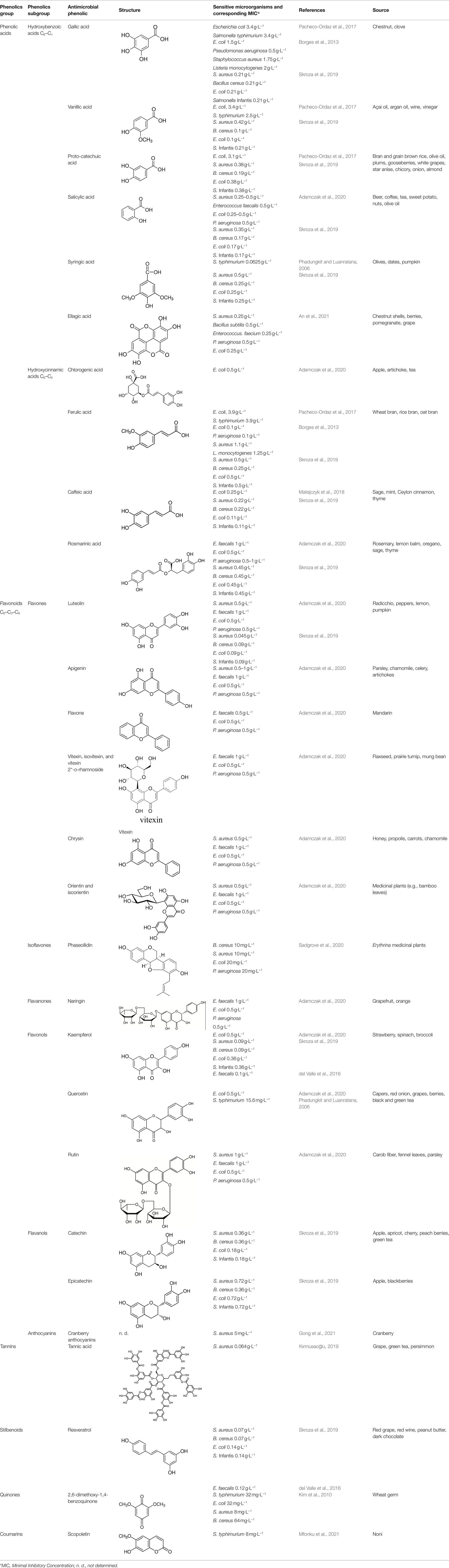
Table 1. Examples of antimicrobial plant phenolics belonging to phenolic acids, flavonoids, tannins, stilbenoids, quinones, and coumarins.
Significantly different MIC values of a given phenolic against the same bacterial species were sometimes reported by different authors. For instance, Pacheco-Ordaz et al. (2017) and Skroza et al. (2019) reported 3.4 g·L−1 and 0.21 g·L−1 MIC values of gallic acid against Escherichia coli, respectively. Such differences are likely due to the differences between the experimental procedures applied for MIC determination: in the absence of the use of a standard MIC determination procedure, differences regarding incubation conditions (initial number of culturable bacteria in the inoculum, temperature …) and bacterial strains can explain such differences. Indeed, MIC values were determined with E. coli O157:H7 ATCC 43890 and ŽMJ 129 (clinical isolate) strains, respectively. A 2.5 106 cfu·ml−1 and a 105–106 cfu·ml−1 inoculum were added in Mueller Hinton broth, which was the incubated for 24 h at 37°C in both studies. Besides possible differences of sensitivity to gallic acid of both E. coli strains, a higher inoculum in the first case results thus in a significantly lower gallic acid to bacteria ratio, which might partly explain the highest MIC value reported by Pacheco-Ordaz et al. Another difference lies in the fact that in the first case MIC was detected by monitoring wells without turbidity, while in the second case absence of viable bacteria was detected following incubation with an indicator of bacterial respiratory activity (iodonitrotetrazolium chloride).
As underlined by Adamczak et al. (2020), differences can also result from the use of different solvents for the dissolution of pure phenolics although dimethylsulfoxide is generally used. However, as can be seen in Table 1, for most phenolics, MIC values determined by different authors against a given bacterial species are similar. The lowest MIC values reported in Table 1 are 5 mg·L−1 for cranberry anthocyanins against Staphylococcus aureus (Gong et al., 2021) and 8 mg·L−1 for scopoletin against Salmonella enteritidis serovar Typhimurium (Mfonku et al., 2021). Since anthocyanin contents of around 50 mg anthocyanins per 100 g of cranberries from different cultivars were reported (Narwojsz et al., 2019), cranberries are a promising source of such antimicrobial phenolics.
As pointed out by Adamczak et al. (2020), compared to the number of studies regarding the antimicrobial activity of phenolic-rich plant extracts, only a few authors investigated the antimicrobial activity of individual pure phenolics (Borges et al., 2013; Pacheco-Ordaz et al., 2017; Bouarab-Chibane et al., 2019; Skroza et al., 2019). Determination of the antimicrobial activity of pure phenolics commonly present in plant extracts opens the possibility to estimate their contribution to the activity of plant extracts, in which they are present: for instance, Phadungkit and Luanratana (2006), determined the MIC against Salmonella of the three phenolics identified in dried fruit extracts of Ardisia elliptica Thunb (syringin, quercetin and a methoxylated derivative of quercetin, isorhamnetin), a fruit used as food, as well as in Thai traditional medicine. Since the antimicrobial activity of plant extracts is generally due to different individual phenolics, antimicrobial activity assays of pure phenolics alone or in combination allows to check whether they have a synergistic activity. Gutiérrez-Fernández et al. (2013) observed thus a synergistic activity against Enterococcus faecalis of gallic acid and octyl gallate, while Mellegard et al. (2009) reported no synergistic activity against S. aureus of the four dominating phenolic compounds in the leaves of Sphagnum papillosum. Comparison of MIC against foodborne pathogenic bacteria of pure phenolics identified with their lower concentrations in the leaves of this plant led these authors to question their potency.
However, these studies with individual pure phenolics are generally performed with synthetic phenolics commercially available. This set of commercial molecules comprises a far lower number of phenolics than the tens of thousands of plant phenolics with different structures, that have been identified. For instance, plant phenolics with high molecular weight, such as tannins, are underrepresented. Moreover, most natural plant phenolics are glycosylated, while only a limited number of commercial phenolics are glycosylated (e.g., vitoxin, orientin, naringin, Table 1). Several authors reported that most aglycones of plant phenolics have a higher antimicrobial activity than their glycosylated forms. This is consistent with Guo et al. (2020) observation of an increase in antibacterial activity of mulberry leaves following their solid state fermentation by edible fungi, which was correlated with an increase in kempferol and quercetin aglycones at the expense of their glycosides.
Identification of antimicrobial phenolics in a plant is necessary to guide their extraction and get extracts with a standardized antimicrobial activity. The most active phenolics of plant extracts launched with a claim for their antimicrobial activity are thus 2,6-dimethoxy-1,4-benzoquinone in moso bamboo extract (Takeguard™, Takexlabo, Osaka, Japan), hydroxytyrosol in olive extract (Hidrox 10X Liquid Concentrate, CreAgri, Inc., Hayward, CA, United States). Nevertheless, it has been reported by many authors that extracts have generally a higher antimicrobial activity than a solution with only the most active phenolic at the same concentration. Serra et al. (2008) reported that a grape extract containing 20 mg·L−1 quercetin totally inhibited the growth of Bacillus cereus unlike a 20 mg·L−1 quercetin solution. Other constituents of grape extract exert thus an additive or synergistic effect with quercetin.
Antimicrobial activity assays of phenolics with different structures also allowed several authors to conduct Structure Activity Relationships (SAR) or Quantitative Structure Activity Relationships (QSAR) studies (Bouarab-Chibane et al., 2019). Since SAR mainly depends on the mechanisms of antimicrobial action on phenolics, the main conclusions of these studies are presented in the next section, after the diversity of mechanisms of actions of plant phenolics has been presented.
Diversity of Antimicrobial Mechanism of Action of Plant Phenolics
Overview of Known Antimicrobial Mechanisms of Action of Plant Phenolics
Different mechanisms of action of plant phenolics active against bacteria, yeasts or fungi have been reported. The reader interested in the mechanisms of action against fungi and more specifically against mycotoxin production can refer to da Cruz Cabral et al. (2013) review. The main mechanisms of antibacterial action of plant phenolics described in this review are summarized in Figure 1. As it can be observed in Figure 1, the most commonly reported mechanisms of action of plant phenolics are at the membrane level. Many authors reported dose-dependent alterations from microbial membranes ranging from reversible membrane permeability perturbations to membrane disruption (inducing leakage of cellular content). However, as stated by Rempe et al. (2017) in their review, while monitoring influx of fluorescent dyes (e.g., propidium iodide can only enter cells with disrupted membrane), efflux of intracellular constituents or direct microscopical observation of microbial cells allow to check, whether their membrane was disrupted following treatment with plant phenolics, this does not give any indication regarding more specific mechanisms of action leading to membrane disruption: did the phenolic compound directly interact with membrane components or alter membrane stability by interfering with intracellular processes?
Nevertheless, flow cytofluorometric analysis of microbial cells stained with different fluorescent dyes allowing to monitor membrane permeability, membrane potential, intracellular pH or intracellular enzymes activity provides information on different functions affected by phenolics at the membrane level (Léonard et al., 2016).
A common proposed mechanism of action is based on the presence of -OH groups in phenolics structure, promoting interaction of phenolics by hydrogen-binding with the microbial cells envelope. Depending on their hydrophobicity, phenolics can accumulate at the surface of the cell envelope, penetrate or even cross their membrane and penetrate in the microbial cells cytoplasm, where they can interact with different cell constituents or alter intracellular pH. Gram-negative bacteria, which possess a hydrophilic cell wall, would be less sensitive to hydrophobic components of polyphenols than Gram-positive ones. Interestingly, Borges et al. (2013) compared the surface (hydrophobicity) and charge (zeta-potential) properties of two Gram-positive (S. aureus and Listeria monocytogenes) and two Gram-negative (E. coli and Pseudomonas aeruginosa) bacterial species following their treatment with either gallic or ferulic acids. Phenolic acids application increased the electron acceptor properties for Gram-positive bacteria and decreased these properties for Gram-negative ones. The surface charge of Gram-negative bacteria was less negative in the presence of the two phenolic acids, while that of Gram-negative bacteria was unchanged. These observations and the differences of susceptibility to phenolic acids of Gram-negative and Gram-positive bacteria support the assumption, that the differences in cell membrane structure and composition play a key role in the susceptibility to plant phenolics.
Undissociated forms of phenolic acids, prevailing at pH values below their pKa values, are uncharged and can thus cross the phospholipid bilayer of bacterial membranes and decrease intracellular pH (Wen et al., 2003; Pernin et al., 2019). Reported consequences of phenolics penetration in the cytoplasm of microorganisms encompass interruption of DNA, RNA, protein synthesis or functions, interference with intermediary metabolism [namely energy (ATP)-generating system], coagulation of cytoplasmic constituents resulting from its acidification. Mora-Pale et al. (2015) observed that resveratrol-trans-dihydromer inhibited DNA gyrase activity in vitro, and concluded from a transcriptomic analysis, that it downregulated ABC transporters, as well as genes involved in cell division and DNA binding proteins. Its bactericidal action against B. cereus, L. monocytogenes, S. aureus, and E. coli results both from membrane potential disruption and from DNA synthesis inhibition.
Phenolics can also interact with membrane proteins involved in different functions: Duggirala et al. (2014) reported that the coumarin, scopoletin, inhibited bacterial cell division protein, FtsZ. They proposed that the increased length of Bacillus subtilis, in the presence of this coumarin, was probably due to the absence of septum formation, resulting from the inhibition of the first step of bacterial cell division. Examples of antimicrobial plant phenolics or phenolic-rich plant extracts with different mechanisms of action are listed in Table 2.
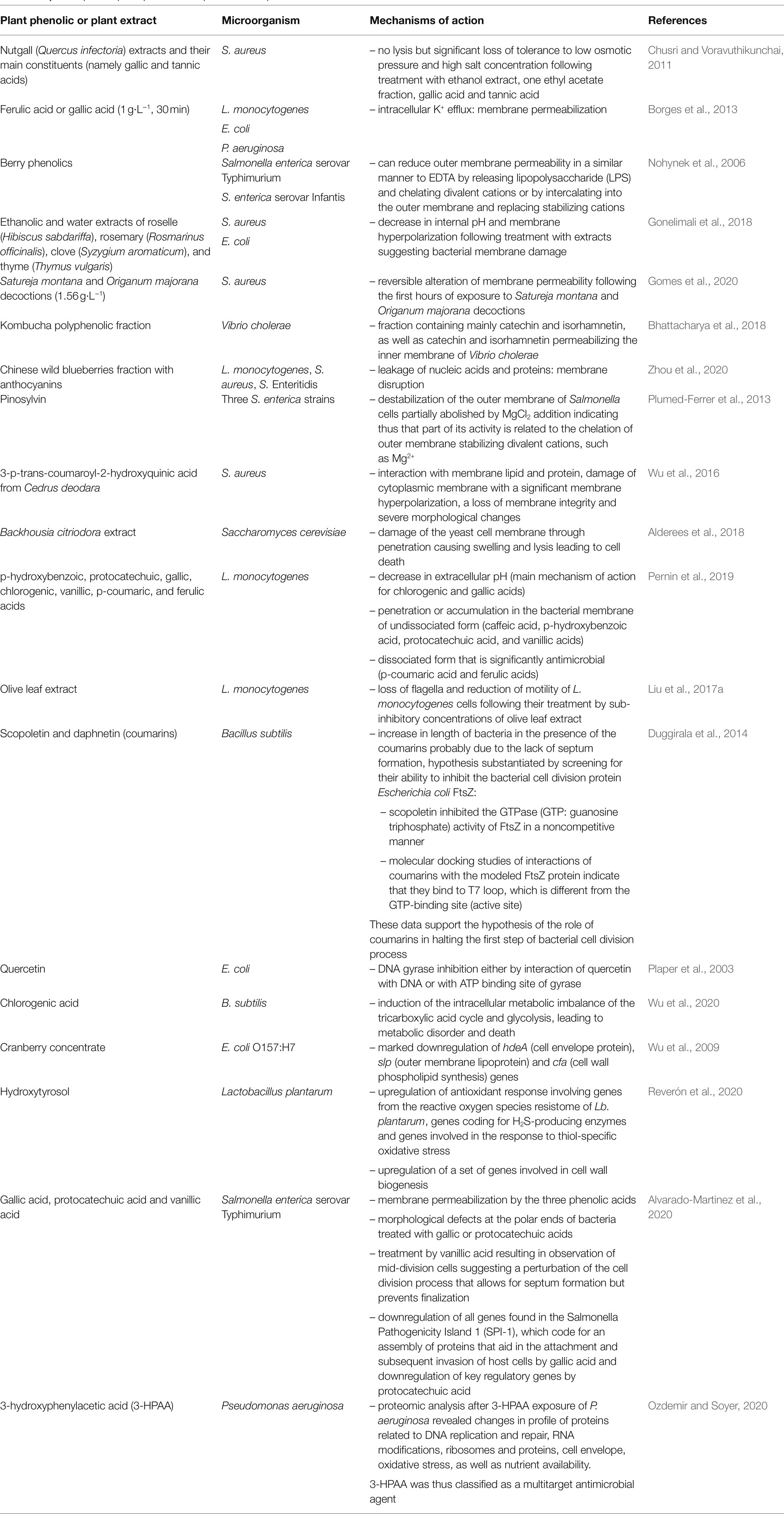
Table 2. Examples of plant phenolics or phenolic-rich plant extracts with different antimicrobial mechanisms of action.
Recent Progress and Future Prospects Regarding Antimicrobial Mechanism of Action of Plant Phenolics
Despite the diversity of mechanisms of action of antimicrobial plant phenolics listed in Table 2, this list is biased by the fact that most investigations were performed using target-directed bioassays [e.g., membrane permeabilization is evaluated by monitoring efflux of intracellular constituents, influx of dyes such as propidium iodide, or 1-N-phenylnaphthylamine (NPN) uptake, DNA gyrase B (gyrB) inhibition assays …], which preclude the discovery of other or even novel mechanisms of antimicrobial action (Rempe et al., 2017).
Nevertheless, multiplication in recent years of studies by -omics approaches opens the possibility to identify new mechanisms of action or to identify several mechanisms of action acting simultaneously (which is generally the case at least for plant extracts and even for some pure plant phenolics). Transcriptomics (Alvarado-Martinez et al., 2020; Linzner et al., 2020; Reverón et al., 2020), proteomics (Ozdemir and Soyer, 2020) and metabolomics (Wu et al., 2020) approaches recently contributed to the identification of targets of antimicrobial action of some plant phenolics or phenolic-rich plant extracts. Transcriptomic (Reverón et al., 2020) and metabolomic (Wu et al., 2020) analyses allow to identify which metabolic pathways are affected by plant phenolics.
Limitations of these -omics approaches are the identification and/or detection thresholds for different technologies, the availability of annotated information for microorganisms tested, and data interpretation difficulties. However, technological evolutions [e.g., more systematic use of high-resolution mass spectrometry (HRMS) for the identification of metabolites] help to solve these limitations. Another bias in past studies lies in the fact, that mechanisms of action were often investigated by treating target microorganisms with plant extracts or phenolics concentration above their MIC or even their Minimal Bactericidal Concentration (MBC). Such conditions often result in observing membrane disruption of target cells, which is the ultimate consequence of the action of some plant phenolics, but rarely the primary cause. It is thus also relevant to investigate the effect of sub-inhibitory concentrations of plant phenolics or extracts on different functions of target microorganisms. -Omics approaches also allow to investigate the effect of phenolics sub-inhibitory concentrations on the physiology of microorganisms.
Fungi are generally more resistant to plant phenolics than bacteria. However, despite their structural diversity, no clear general rule regarding the antimicrobial activity spectrum or the mechanisms of action of a class of plant phenolics could be established to date. Nevertheless, the interested reader can refer to several reviews or articles focused on the antimicrobial activity of each phenolic class: phenolic acids (Wen et al., 2003; Cueva et al., 2010; Borges et al., 2013; Pernin et al., 2019), flavonoids (Cushnie and Lamb, 2005), tannins (Scalbert, 1991), stilbenoids (Plumed-Ferrer et al., 2013), quinones (Linzner et al., 2020), and coumarins (Duggirala et al., 2014). Several authors also presented reviews on phenolic-rich plant extracts or plant phenolics acting on one (L. monocytogenes, Zamuz et al., 2021) or several (Ullah et al., 2020) foodborne pathogenic microorganisms.
Structure-Antimicrobial Activity Relationships of Plant Phenolics
With the increasing number of antimicrobial phenolics with different structures identified, several authors investigated the relationships between phenolics structure and their in vitro antimicrobial activity (Taguri et al., 2006; Bouarab-Chibane et al., 2019). Taguri et al. (2006) compared the antibacterial activity of 22 polyphenols against 26 species of bacteria by determining their MICs in Mueller-Hinton broth. Bouarab-Chibane et al. (2019) investigated the effect of a 1 g·L−1 concentration of 35 polyphenols on the growth at 37°C for 24 h of three Gram-positive bacterial strains (B. subtilis, S. aureus, L. monocytogenes) and three Gram-negative ones (E. coli, P. aeruginosa, and S. Enteritidis). In both studies, the antibacterial activity was dependent on the species of bacteria and no significant difference of susceptibility to polyphenols was observed between Gram-positive and Gram-negative bacteria.
The fact that antibacterial activity of phenolics depends on the species of bacteria motivated some authors to perform QSAR studies focused on one bacterial species. For instance, Fang et al. (2016) built reliable QSAR models for the antibacterial activity against E. coli of 30 flavonoids. Moreover, molecular docking study of interaction with DNA gyrase B (gyrB) of E. coli allowed to establish that half of flavonoids active against E. coli inhibit gyrB by interacting with ATP binding site of this enzyme [the mechanism of antibacterial activity against E. coli of quercetin already proposed by Plaper et al. (2003)]. Pernin et al. (2018) proposed a QSAR model for the antibacterial activity against L. monocytogenes of 21 phenolics and found a good correlation with their octanol–water partition coefficient (log Po/w).
Taguri et al. (2006) observed that antibacterial activity of polyphenols having pyrogallol groups (i.e., with three adjacent hydroxyl groups) was higher than that of polyphenols with catechol and resorcinol rings (i.e., with two adjacent or non-adjacent hydroxyl groups, respectively). This is consistent with Phan et al. (2014) conclusion that “the higher number of hydrophilic side chains (hydroxyl, gallate, galloyl, glucoside), the more interactive the polyphenol was with the membrane” following observation of spatio-temporal real-time membrane dynamics in the presence of different polyphenols. Bouarab-Chibane et al. (2019) were not able to build reliable QSAR models predicting the effect of the set of 35 polyphenols they used on the growth of L. monocytogenes and P. aeruginosa, since they were systematically inhibited or not inhibited by polyphenols, respectively. However, reliable QSAR models, with a few independent physicochemical descriptors linked with lipophilicity and the electronic and charge properties of the polyphenols, were built for each of the four other bacterial strains. These physicochemical descriptors are consistent with the hypothesis that the main antibacterial mechanisms of action of polyphenols depend on their accumulation on the surface of bacteria, which is favored both by hydrogen binding and by their hydrophobicity.
For instance, the reduced susceptibility to polyphenols like epigallocatechin gallate of many lactic acid bacteria compared to other Gram-positive bacteria was proposed to result from exopolysaccharides production, which reduces surface hydrophobicity of lactic acid bacteria (Nakayama et al., 2015). Therefore, Bouarab-Chibane et al. (2019) investigated the surface properties of the six species of test bacteria they used by Microbial Adhesion To Solvents (MATS), following the method described by Bellon-Fontaine et al. (1996). Interestingly, they noted that bacteria, which had a similar affinity for the four solvents they used, had similar QSAR models, while the bacteria which had different affinity for these solvents also had different QSAR models. Since only four bacterial strains had reliable QSAR models, they suggested evaluating, whether this trend would also be observed with a larger number of bacterial strains. Moreover, characterization of the surface properties of bacteria should also integrate other parameters such as their zeta-potential.
Besides investigating the structure-antimicrobial activity relationships of phenolics naturally present in plants, several authors performed SAR or QSAR studies with series of epicatechin gallate groups, from which gallate group was substituted with 3-O-acyl chains of varying lengths (C4–C18; Stapleton et al., 2004), or with series of esters of phenolic acids esterified with various alkyl or aryl substituents (Andrade et al., 2015; Shi et al., 2018; Araujo et al., 2019). This allowed them both to get further insight in the physicochemical characteristics of active antimicrobial phenolics and to design new antimicrobial phenolics.
Antimicrobial Activity of Plant Phenolics in Foods: Interest, Limits and Future Prospects
Examples of Antimicrobial Action of Plant Phenolics in Different Food Matrices
The potential of several phenolic-rich plant extracts or pure plant phenolics to inhibit the growth of unwanted microorganisms in some food matrices has been demonstrated in many studies. Some recent examples are listed in Table 3. As illustrated by these examples, many studies aimed at improving the preservation of meat and meat products, likely since these foods both have a high added-value and are highly perishable. Application of phenolic-rich plant extracts to meat and meat products preservation has been recently reviewed (Efenberger-Szmechtyk et al., 2021). Moreover, addition of herbs and spices in meat and meat products is a common practice to improve their sensory properties. As stated in Table 3 (e.g., Ranucci et al., 2019), plant phenolics and phenolic-rich plant extracts addition in meat and meat products has frequently been reported to delay oxidation reactions altering organoleptic properties of such foods (e.g., color alteration due to myoglobin oxidation into metmyoglobin or taste or aroma alteration due to fat oxidation).
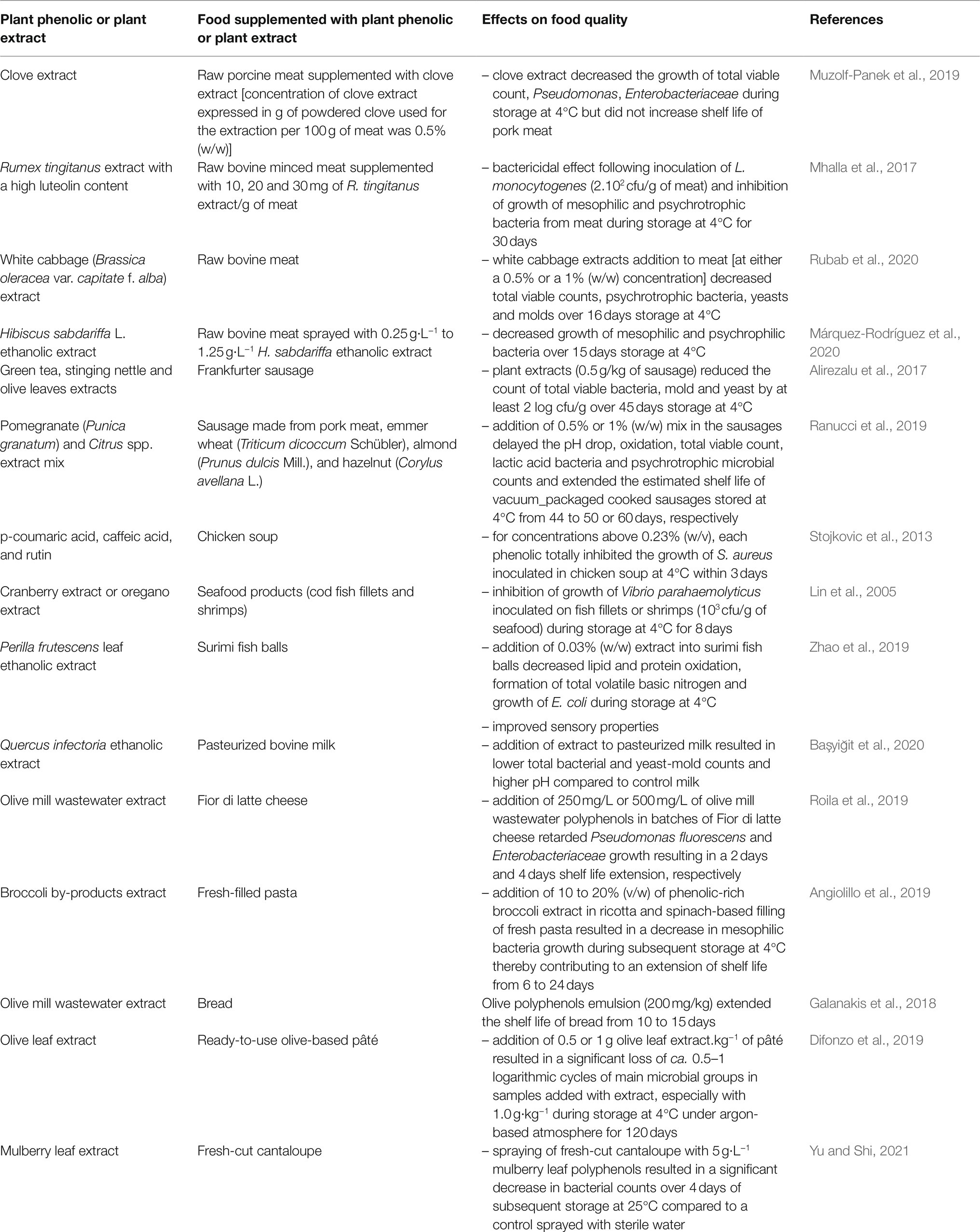
Table 3. Examples of phenolic-rich plant extracts or plant phenolics effectively inhibiting the growth of unwanted microorganisms in food matrices.
However, addition of plant phenolics or phenolic-rich plant extracts to meat and meat products and other food matrices has frequently more positive effects on retardation of oxidation phenomena than on retardation of the growth of unwanted microorganisms. For instance, Bouarab-Chibane et al. (2017) added 1% (w/w) of antimicrobial phenolic-rich plant extracts to raw bovine meat patties, enumerated bacteria and monitored different quality attributes of meat during 12 days storage at 4°C in a high-oxygen modified atmosphere. While pomegranate peel, green tea leaves, grape seed extracts, and Gaillac red wine powder reduced the increase in ThioBarbituric Acid Reactive Substances (TBARS are namely aldehydes generated following the decomposition of lipid peroxidation products) in bovine patties over 12 days by more than 93%, these extracts had no significant effect on total viable and psychrotrophic bacterial counts, despite their in vitro bactericidal activity against several Gram-positive and Gram-negative bacteria at a 0.1% (w/w) concentration. This apparent discrepancy can be due to the absence of susceptibility to these extracts of total viable and psychrotrophic bacterial strains, resulting from the diversity of strains in the meat microbial ecosystem. However, it could also be due to the interactions of antimicrobial plant molecules with proteins and/or fat which are the main constituents of bovine meat: these interactions could limit the quantity of “free” phenolics not interacting with these food constituents.
Nevertheless, despite their generally lower in vitro antimicrobial activity than in microbiological media, several authors reported plant phenolics or phenolic-rich plant extracts effectively inhibiting the growth of foodborne pathogenic micrororganisms [e.g., L. monocytogenes (Mhalla et al., 2017), S. aureus (Stojkovic et al., 2013)] or of food-spoiling microorganisms [e.g., Pseudomonas, Enterobacteriaceae (Muzolf-Panek et al., 2019)] in foods such as meat products. Mhalla et al. (2017) reported that incorporation of a luteolin-rich Rumex tingitanus extract in raw bovine minced meat retarded the growth at 4°C of mesophilic and psyschrophilic bacteria and addition of a pomegranate and Citrus spp. extracts mix in the formulation of pork sausages resulted in a significant extension of their shelf life (Ranucci et al., 2019). The potential of direct addition of plant extracts in other perishable animal origin foods containing proteins and fat than meat was investigated by other authors: addition of Perilla frutescens leaf extract into surimi fish balls decreased growth of E. coli during storage at 4°C (Zhao et al., 2019) and addition of olive mill wastewater polyphenols in batches of Fior di latte cheese retarded Pseudomonas fluorescens and Enterobacteriaceae growth resulting in significant shelf life extension (Roila et al., 2019). Addition of plant extracts at concentrations exceeding 1% (w/w) are generally required to effectively inhibit the growth of unwanted microorganisms in foods. It might even be more promising to add phenolic-rich plant extracts in foods containing lower amounts of food constituents interacting with proteins and fat, such as fruits, vegetables or bread (Galanakis et al., 2018).
Interestingly, non-minced meat pieces or fish fillets can be treated by spraying plant extracts or phenolics solutions on their surface, that is precisely where microbial contaminations and growth occur during storage. For instance, Márquez-Rodríguez et al. (2020) reported that spraying raw bovine meat pieces with a ~ 1 g·L−1 Hibiscus sabdariffa extract solution decreased the growth of mesophilic and psychrophilic bacteria over 15 days subsequent storage at 4°C. Similarly, Lin et al. (2005) voluntarily inoculated seafood with Vibrio parahaemolyticus and observed that spraying cranberry and oregano extracts solutions on their surface inhibited its growth for 8 days storage at 4°C. This strategy is also promising for fresh-cut fruits preservation as recently illustrated by Yu and Shi (2021) who sprayed fresh-cut cantaloupe with 5 g·L−1 mulberry leaf polyphenols to inhibit bacterial growth on their surface.
Limits to the Application of Plant Phenolics for Food Preservation
Effect of Interaction With Food Constituents on Plant Phenolics Antimicrobial Activity
Besides interactions of phenolics with food constituents such as proteins or fat and resulting decrease in their “free” amount available to act on target microorganisms, unwanted microorganisms could also be protected by a “layer” of food constituents limiting the direct contact of antimicrobial phenolics with their cell envelope, the most commonly described mechanism of action of antimicrobial phenolics: da Cruz Cabral et al. (2013) reported that “lipids in food could form a coating around the microorganisms, protecting them from antimicrobial agents.” Boziaris et al. (2011) also reported the absence of effect on viable populations of spoilage or pathogenic bacteria were found between fish flesh or fish roe (tarama salads) treated or not with 10% (v/w) of Filipendula ulmaria liquid extract. For instance, L. monocytogenes Scott A was not affected by 10% (v/w) F. ulmaria extract over 12 days storage at 5°C of seafoods inoculated by this strain, while it was inhibited by the same concentration of this plant extract on solid microbiological medium also incubated at 5°C to better mimic food preservation conditions. They proposed that the loss of susceptibility of L. monocytogenes is due to interactions of F. ulmaria extract active phenolics (e.g., caffeic, p-coumaric and vanillic acids, myricetin …) with proteins and fat (the main constituents of such foods), at the expense of their interactions with unwanted microbial cells.
Indeed most studies regarding the antimicrobial activity of plant phenolics have been performed in vitro in microbiological media with a less complex composition and microstructure than food matrices. Miceli et al. (2014) compared the in vitro activity of borage (Borago officinalis) and Indian mustard (Brassica juncea) aqueous extracts against several foodborne pathogenic bacterial strains in vitro in Brain Heart Infusion broth and in meat, fish and vegetable broths (considered as food models). They observed that a 10-fold higher concentration than the in vitro bactericidal concentration was necessary to get a bacteriostatic effect in food models for both extracts. They suggested that this difference could be due to binding of the active compounds of extracts to food components.
Carbohydrates, lipids and proteins are the main components of food, and interactions between polyphenols and these components have been reported by many authors and reviewed by Jakobek (2015). Most studies regarding interactions between polyphenols and these components aimed at investigating their effect on their bioavailability following their ingestion. Interactions between phenolics and proteins are the most studied for this reason, and because interactions between compounds such as tannic and gallic acids and salivary proteins are involved in their astringency. Xu et al. (2018) compared thus the MICs against different microorganisms of epigallocatechin gallate and grape seed extract in a protein-free chemically defined medium and in the same medium supplemented with up to 0.4 g·L−1 of bovine serum albumin. A 64-fold increase in MIC of epigallocatechin gallate against a Streptococcus mutans strain was observed and a similar trend was observed with grapeseed extract, as well as against other microbial species. Such an increase in MIC was not observed for non-phenolic antimicrobial compounds, which were tested in parallel.
Besides protein content, which is far higher in most perishable foods than in microbiological media, another important difference between in situ antimicrobial activity assays and in vitro ones lies in the physiological state of microorganisms under refrigeration conditions and at optimal temperatures for microbial growth (i.e., between room temperature and 37°C), respectively. Klancnik et al. (2011) compared the activity in buffered peptone water supplemented with 50% (w/v) of meat, vegetable or dairy products to estimate the effect of the components of these foods on the activity against L. monocytogenes and E. coli of rosemary phenolic extracts. They also observed an increase in MIC against these two bacterial species for every food type added in buffered peptone water.
Therefore, Bouarab-Chibane et al. (2018b) compared the antibacterial activity of five bacteriostatic or bactericidal phenolics active against a S. aureus strain for 24 h at 37°C in Mueller-Hinton broth, with their activity in the same medium supplemented with up to 20% (w/w) bovine meat proteins (the protein content of bovine meat) for up to 8 days at 6°C (to mimic refrigeration conditions). While resveratrol and chrysin always lost their bacteriostatic activity in the presence of bovine meat proteins, gallocyanin kept its bactericidal activity at 37°C up to a 5% (w/w) protein content in the medium, but not at 15°C or 6°C, unlike naphthazarin, which was bactericidal at 6°C and 15°C, unlike at 37°C in the presence of bovine meat proteins. Finally, isobutyl-4-hydroxybenzoate kept its bactericidal activity under all the conditions investigated. The partition coefficient at 6°C of each phenolic between a 20% (w/w) bovine meat extract suspension and the same suspension without proteins was determined. Interestingly, the antibacterial activity reduction of phenolics in the presence of bovine meat proteins was correlated with their affinity for bovine meat proteins. Ansari et al. (2015) also reported that antimicrobial activity loss in the presence of casein or gelatin was correlated with their affinity for these proline-rich proteins.
It is thus advisable to perform at an early stage an in vitro screening of antimicrobial activity of phenolic-rich plant extracts in media containing proteins, if an application to foods containing proteins is foreseen (Bouarab-Chibane et al., 2018c), and preferably with the same proteins as in food. Screening at refrigeration temperatures is also preferable, if application to preservation of perishable foods is foreseen. Antimicrobial activity of plant extracts can be greatly influenced by temperature, as underlined by Hayrapetyan et al. (2012). They reported a higher inhibition of L. monocytogenes in meat pâté by pomegranate peel extract at 4°C than at 7°C, or 12°C. More systematic studies on the effect of food ingredients on the antimicrobial activity of phenolic-rich plant extracts or plant phenolics under conditions closer to food preservation conditions than classical in vitro antimicrobial activity screening assays should contribute to anticipate their in situ activity in real foods. While studies regarding the effect of proteins on the antimicrobial activity of plant phenolics exist, studies regarding the effects of carbohydrates or lipids on their activity are scarce and will also be necessary, since these compounds also interact with polyphenols. In the absence of such data, the efficiency of a given plant extract or phenolic to preserve a defined food can hardly be extrapolated to other foods differing in their composition and/or microstructure.
Effect of Plant Phenolics Addition on Foods Organoleptic Properties
Another important limit of many plant extracts is their effect on organoleptic properties of foods: the taste (e.g., astringency of some tannins, bitterness of green tea extract), odor and/or color (e.g., green color of green tea extract) of many plant extracts can alter the organoleptic quality of some foods (Bouarab-Chibane et al., 2017). However, in some cases, plant extracts added in concentrations effectively inhibiting the growth of unwanted microorganisms can also improve the color or the taste of some foods. Swer et al. (2019) added up to 0.2% (w/v) of anthocyanins extracted from Sohiong (Prunus nepalensis L.) in yogurts. Increase in anthocyanins content improved overall acceptability of yogurts by panelists. It is also possible to use plant extracts from which coloring substances have been removed. For instance, Nirmal and Benjakul (2011) added green tea ethanolic extracts without chlorophyll to Pacific white shrimps. One advantage of antimicrobial pure phenolics over plant extracts might also result from their absence of effect on taste of foods at a dose effectively inhibiting unwanted microorganisms, as underlined by Stojkovic et al. (2013) for chicken soup and porcine meat preserved by incorporation of 1.87 g·L−1 or spraying of 1.87 mg.10 cm−2 of phenolics (p-coumaric acid, caffeic acid, or rutin). However, as stated by Albuquerque et al. (2021), only the use of following pure phenolics is authorized in foods: anthocyanins [E 163, authorized as colorant by European Food Safety Authority (EFSA in the European Union) or Food and Drug Administration (FDA in the United States)] and ferulic acid (as antioxidant in Japan).
Prospects for a Broader Use of Plant Phenolics for Food Preservation
Another potential advantage of the addition of some plant extracts or phenolics lies in their health-promoting properties. Since health-promoting properties of edible plant extracts or phenolics are not in the scope of the present review, the interested reader can refer to a recent review on this subject (Samtiya et al., 2021). As stated above, no plant extract will have the capacity to replace synthetic food preservatives, such as potassium sorbate as antifungal agent, in all their applications for the same cost. This is also namely due to the narrower antimicrobial activity spectrum of plant extracts compared to most food preservatives. A solution to have a broader antimicrobial activity spectrum is to use mixtures of plant extracts with different antimicrobial activity spectra. This is likely one of the reasons for the better preservation of raw bovine meat by mixtures of clove, cinnamon and oregano extracts rather than each of these extracts alone, or binary combinations of these extracts reported by Radha Krishnan et al. (2014). The narrow spectrum of antimicrobial activity of phenolic-rich plant extracts can also be positively exploited in some cases. For instance, the fact that lactic acid bacteria are generally less susceptible to the antibacterial plant phenolics compared to most undesirable bacteria (Pacheco-Ordaz et al., 2017; Chan et al., 2018) opens the possibility to add phenolic-rich plant extracts in foods fermented by lactic acid bacteria at sub-inhibitory concentrations of lactic acid bacteria, while effectively inhibiting the growth of unwanted microorganisms.
Another promising strategy to expand the application of phenolic-rich plant extracts is in line with hurdle technology principles application: appropriate combinations of phenolic-rich plant extracts addition, with for instance the addition of organic acids (Apostolidis et al., 2008), inoculation of food with bioprotective microorganisms (Sireswar et al., 2017), modified atmosphere or vacuum (Ranucci et al., 2019) packaging, high hydrostatic pressure (Hygreeva and Pandey, 2016) or UV-A treatment (Cossu et al., 2018) of foods have for instance been used to get a synergistic antimicrobial activity. Sireswar et al. (2017) inoculated seabuckthorn juice supplemented with malt extract with probiotic lactic acid bacteria strains. They demonstrated that growth of probiotic strains was possible thanks to malt extract addition and despite antibacterial phenolics presence in seabuckthorn juice. Moreover, they demonstrated the capacity of this food to rapidly eliminate different enteropathogenic bacteria: they proposed that the rapid elimination of these pathogens results from the synergistic action of metabolites such as lactic acid produced by probiotic bacteria and antimicrobial phenolics from seabuckthorn juice, such as isorhamnetin, myricetin, kaempferol, and quercetin.
Diversity of Mechanisms of Action Against Biofilms of Plant Phenolics
Overview of Known Mechanisms of Action of Plant Phenolics Against Biofilms
Biofilms confer favorable growth environments to pathogens and resistance to antimicrobials and disinfectants (Oulahal et al., 2008). Biofilms formed by undesirable bacteria are a major concern for food microbial safety, due to production of virulence factors, persistence of bacterial pathogens, cross contamination causing many risks for consumers. Mishra et al. (2020) recently reviewed strategies to control biofilm-forming pathogens based on the exploitation of natural anti-biofilm agents, including phytochemicals such as plant antimicrobial phenolics. Guzzo et al. (2020) more specifically reviewed the activity against P. aeruginosa and S. aureus biofilms of plant-derived natural products and Slobodníková et al. (2016) reviewed antibiofilm activity of plant polyphenols. However, these three reviews were focused on their clinical application in the medical and healthcare sectors and not in the food sector. The reader interested in plant phenolics potential against biofilm-forming food-contaminating microorganisms can refer to the review by Takó et al. (2020).
Briefly, increased tolerance to antimicrobial compounds of microorganisms present in biofilms results from different phenomena: microbial cells in biofilms are embedded in self-secreted extracellular polymeric substances (Sivaranjani et al., 2016), that attach these cells. These extracellular polymeric substances are namely polysaccharides, proteins and extracellular DNA. They protect cells from desiccation, pH variations but also from antimicrobial molecules including plant phenolics. They act as a protective layer of the surface of cells, as viscosifying agents slowing down the penetration and the diffusion of antimicrobial molecules in biofilms structure, thereby favoring adaptation of microbial cells, which have more time to become tolerant. Besides this “physical tolerance” favored by extracellular polymeric substances, “physiological tolerance” to antimicrobial molecules of microbial cells embedded in the deepest layers of biofilms has also been reported (Mishra et al., 2020). Indeed, important decreasing gradients of nutrients or oxygen result in the downregulation of the metabolic activity of microbial cells located inside the structure of biofilms. These reversible adaptative stress responses make these cells more tolerant to antimicrobial molecules. That is why these slow-dividing cells are called persister cells. Progresses in -omics (genomics, transcriptomics and metabolomics) allow identification of molecular pathways leading to biofilm formation and will contribute to get information for the rational design of efficient strategies to control biofilm formation.
Knowing the different stages of formation and development of biofilms also guides the design of strategies to prevent biofilms formation or to favor their eradication. Indeed, biofilm formation (i) starts with the attachment of microbial cells to a surface, is followed by (ii) biofilm structure development, (iii) its maturation and finally, (iv) its dispersion. Since attachment involves cytoskeletal elements, such as flagella and lipopolysaccharides, molecules inhibiting their formation are particularly promising to prevent their formation. Many authors recently reported the inhibition of biofilm formation by phenolic-rich plant extracts/plant phenolics by different mechanisms of action. A survey of such studies illustrating the diversity of these mechanisms of action is provided in Table 4.
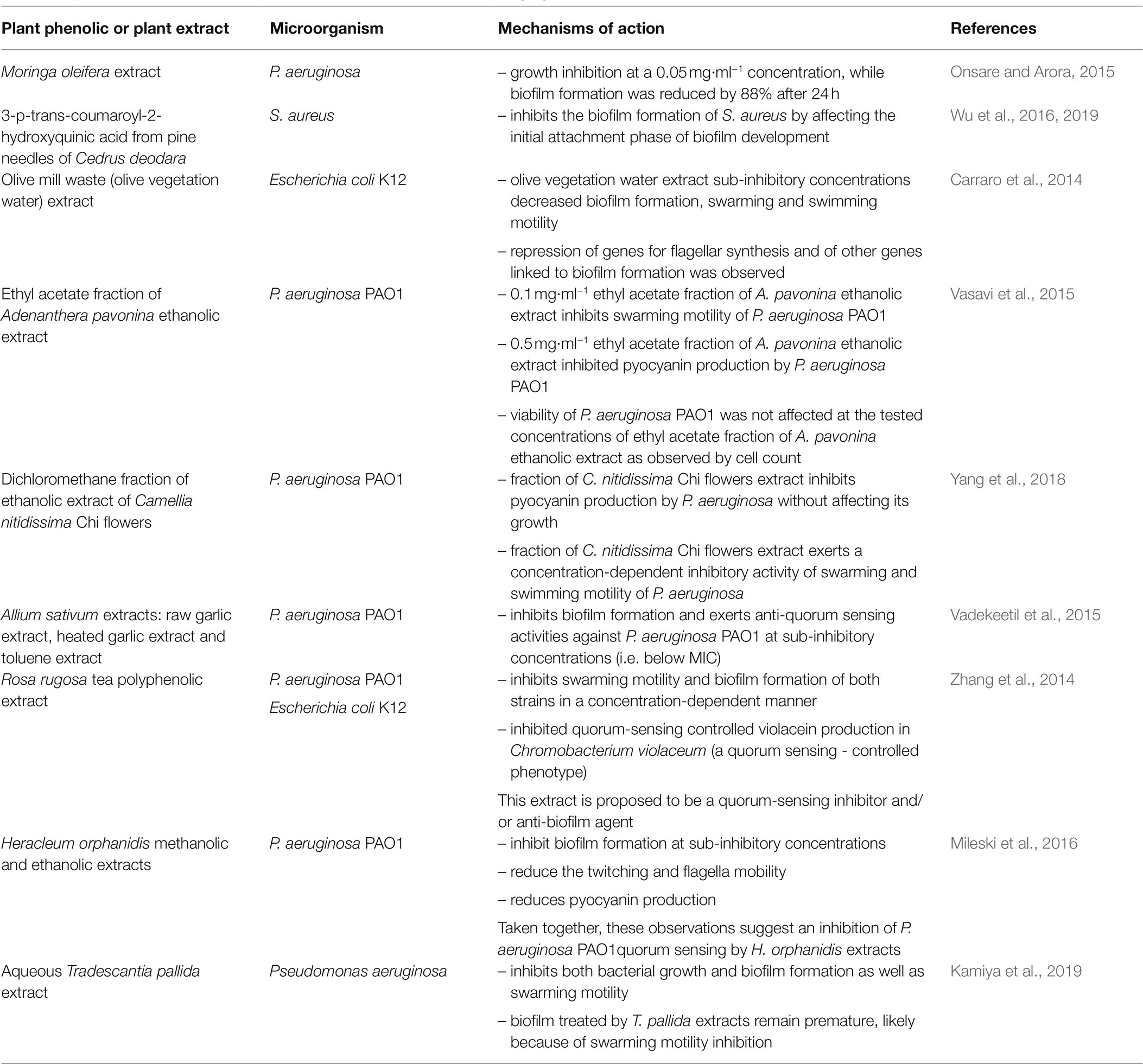
Table 4. Examples of plant phenolics or phenolic-rich plant extracts acting against biofilms by different mechanisms of action.
Recent Progress and Future Prospects Regarding Mechanism of Action Against Biofilms of Plant Phenolics
Staphylococcus aureus biofilms are a major concern for food safety. Interestingly, Wu et al. (2019) observed that coumaroyl-2-hydroxyquinic acid from pine needles of Cedrus deodara, not only inhibits S. aureus biofilm formation, but also the attachment phase, by inhibiting the transmembrane peptidase sortase A, SrtA. This enzyme catalyzes the covalent binding of surface proteins sharing a typical sorting signal, with a conserved C-terminal LPXTG motif, to cell wall peptidoglycan and these cell wall-anchored proteins initiate bacterial adherence by binding host surface. SrtA inhibitors are thus promising to inhibit the initial stage of biofilm formation. The same group (Liu et al., 2021b) performed combined transcriptomic and proteomic analyses in the absence and in the presence of sub-inhibitory concentrations of coumaroyl-2-hydroxyquinic acid, in order to elucidate its molecular mechanism of action against S. aureus. They observed a differential expression of 935 genes and 438 proteins in both situations. Downregulation of surface proteins associated with cell adhesion supports the hypothesis, that this antimicrobial plant phenolic prevents S. aureus biofilm formation by inhibiting adhesion of S. aureus cells. Bioinformatic analysis also demonstrated that coumaroyl-2-hydroxyquinic acid affects different functions of S. aureus, namely at the membrane level.
Recently, when investigating Adiantum philippense extract inhibitory activity of biofilm formation by several foodborne pathogens, Adnan et al. (2020) performed an in silico molecular docking study of the inhibitory activity of S. aureus SrtA by 28 of its identified constitutive phenolics: scutellarin, a glycosyloxyflavone, was found to have the highest affinity for SrtA. Interestingly, this extract also inhibited biofilm formation by other foodborne pathogenic bacteria, and other compounds of the extract had also a high affinity for other adhesins than SrtA. Moreover, this extract significantly inhibited exopolysaccharides production by these bacteria: this is likely another mechanism of biofilm formation inhibition.
Carraro et al. (2014) reported that an olive mill waste extract, inhibiting E. coli K12 biofilms formation, also inhibited swarming and swimming motility of these bacteria. Consistently with this observation, they noticed repression of genes for flagellar synthesis and other genes linked to biofilm formation in the presence of this extract. Indeed, reduction of swarming or swimming motility of bacteria by phenolic-rich plant extracts or plant phenolics inhibiting biofilm formation has been frequently reported (e.g., in Table 4: Carraro et al., 2014; Zhang et al., 2014; Vasavi et al., 2015; Yang et al., 2018; Kamiya et al., 2019).
A promising strategy to prevent biofilm formation is based on the identification of quorum sensing inhibitors. Quorum sensing is a mechanism of regulation of gene expression, as a function of microbial cells population density: this mechanism results in the production of extracellular compounds called autoinducers, which alter gene expression of other bacterial cells, when their concentration exceeds a minimal threshold. Since these autoinducers allow bacteria, not only to communicate within species, but also with different species, this mechanism allows bacteria to induce coordinated responses to their environment, like signaling in higher organisms. The phenotypes regulated by quorum sensing include motility, biofilm formation, and resistance to antibiotics (LaSarre and Federle, 2013; Castillo Rivera et al., 2019). Quorum sensing is also involved in the regulation of phenotypes contributing to virulence of bacteria.
Interestingly, several authors have reported the interaction of plant phenolics with homoserine lactones, which are typical auto-inducers of Gram-negative bacteria (Hossain et al., 2017). They reported that methyl gallate had anti-quorum sensing effect on Chromobacterium violaceum (an aquatic bacterium used to study the inhibition by diverse molecules of acyl homoserine lactone-dependent quorum sensing, since production of violacein pigment is associated with its quorum-sensing regulated gene expression) and on P. aeruginosa, including inhibition of biofilm formation by this bacterium, as well as of its production of exopolysaccharides, which are extracellular polymeric substances in biofilms, and of its swarming motility. Indeed, swarming has been reported to play an important role in the preliminary stage of quorum sensing-regulated bacterial biofilm formation. Since attachment and involvement of quorum sensing are critical steps for the development of biofilms, antimicrobial phenolics acting on these mechanisms are preferred strategies to inhibit biofilm formation.
Baptista et al. (2019) investigated the activity against E. coli biofilms of nine phenolics with a catecholic moiety. Increases in the hydrocarbon side chain and lipophilicity, as well as a contribution of hydroxyl groups, were proposed as structural traits favoring anti-biofilm activity of catecholic molecules following a SAR study. SAR and QSAR studies regarding the activity against biofilms of plant phenolics are still scarce. Multiplication of such SAR and QSAR studies should contribute to get a better insight in structural traits of plant phenolics active against biofilms.
Many plant phenolics [e.g., gallic and ferulic acid (Borges et al., 2012), vanillic, caffeic, cinnamic and ferulic acid (Ugurlu et al., 2016), tannic acid (Dong et al., 2018), morin (Sivaranjani et al., 2016), myricetin (Slobodníková et al., 2016), 4-methylcatechol, 4-tert-butylcatechol, and pyrogallol (Baptista et al., 2019), quercetin (Vazquez-Armenta et al., 2020)] and phenolic-rich plant extracts [e.g., garlic extracts (Vadekeetil et al., 2015), Moringa oleifera extract (Onsare and Arora, 2015), Brassicaceae (radish, radish sprout, red cabbage, kale) extracts (Hu et al., 2019), Capsicum peppers extracts (Castillo Rivera et al., 2019), phenolic-enriched extracts produced by enzyme-assisted extraction from oven-dried and lyophilized black grape, apple and yellow pitahaya (Zambrano et al., 2019), tea and turmeric extracts (Tamfu et al., 2020), anthocyanin-rich aqueous extract from purple highland barley bran (Zhang et al., 2020a), avocado peel extract fractions (Trujillo-Mayol et al., 2021)] have been reported to inhibit formation or to eradicate biofilms with undesirable food spoiling or foodborne pathogenic microorganisms.
Interestingly, many authors reported that biofilm formation inhibition by plant phenolics requires lower concentration than to inhibit the growth of the corresponding microorganisms (Carraro et al., 2014; Zhang et al., 2014; Vasavi et al., 2015; Yang et al., 2018; Kamiya et al., 2019). Since biofilms are major causes of cross-contamination of foods, exploitation of antibiofilm activity of plant phenolics is promising to limit foodborne diseases, as well as food spoilage. Disinfection in food processing factories relies upon biocides, requires large amounts of water and generates huge volumes of sewage with high loads of cleaning agents and biocides. In France, 11,000 tons of biocides are used in the agri/food industry per year (data from the French Association of Detergents and Hygiene Product Industry Professionals). Biodegradability of cleaning agents and toxicity of disinfectants are both issues for public authorities, who have established regulations to protect the environment, including the currently implemented EU no 528/2012 Regulation concerning the making available on the market and use of biocidal products in the European Union. However, among cleaning agents and biocides, the most environmentally friendly products are still not widely used. Use of plant phenolics or phenolic-rich plant extracts for this purpose is still in its infancy.
Besides societal and regulatory evolutions related to environmental concerns and the necessity to limit the emergence of multidrug-resistant microorganisms, a broader use of phenolic-rich plant extracts will require, not only a better understanding of the molecular mechanisms leading to biofilm formation prevention or their eradication, but will also benefit from progresses regarding the formulation of delivery systems improving their efficiency. Ongoing progresses in this field are presented in the next section of this review. A promising strategy presented in the next section relies on functionalization of materials with antimicrobial phenolics (e.g., curcumin as proposed by Dogra et al., 2015), in order to prevent biofilm formation on food-contacting surfaces.
Delivery Modes to Promote the Stability and the Antimicrobial Activity of Plant Phenolics
Antimicrobial plant phenolics direct addition to foods may face the same limits than other antimicrobial compounds, which were listed by Fu et al. (2016) in their review:
− a limited solubility in water, which is a major constituent of perishable foods
− a limited stability, oxidation or heat treatments may affect the activity of some plant phenolics
− uncontrolled release
− alteration of organoleptic properties [alteration of colour or taste (e.g., astrincency of many plant polyphenols)]
Design of food-grade systems to deliver antimicrobial plant phenolics is promising to circumvent these limits. The two main goals of such systems are (i) protection and (ii) sustained release of plant phenolics. Indeed, delivery systems with diverse structures can be prepared with different food-grade components, such as lipids, proteins, carbohydrates, surfactants, and minerals (Zhang et al., 2020b). Controlled release of phenolics from such systems can result from physical entrapment in structures with different geometries (e.g., gels with differing porosity, tortuosity, coated microcapsules …), from weak interactions (hydrophobic or ionic interactions, hydrogen-bonding) between phenolics and delivery systems components or from a mixture of both phenomena. Delivery systems can also be classified according to their dimensions, ranging from molecular inclusion of phenolics in cyclodextrins (Pinho et al., 2015) and the rapidly growing field of nanosystems of delivery [nanoemulsions (Saini et al., 2019; McClements et al., 2021) and nanoparticles (Hu et al., 2017; Hosseini and Jafari, 2020; Vidallon and Teo, 2020; Spizzirri et al., 2021)] to edible coatings or food packagings incorporated with antimicrobial plant phenolics (Mir et al., 2018; Zhu, 2021), and including microemulsions and other microencapsulation systems (Hosseini and Jafari, 2020). Most of systems for controlled delivery of active molecules are issued from the important effort of research for pharmaceutical applications. For controlled delivery of plant phenolics, the goal is often to improve the bioavailability of dietary polyphenols, as reviewed by Hu et al. (2017). Several examples of application to antimicrobial plant phenolics of each of these delivery systems are listed in Table 5.
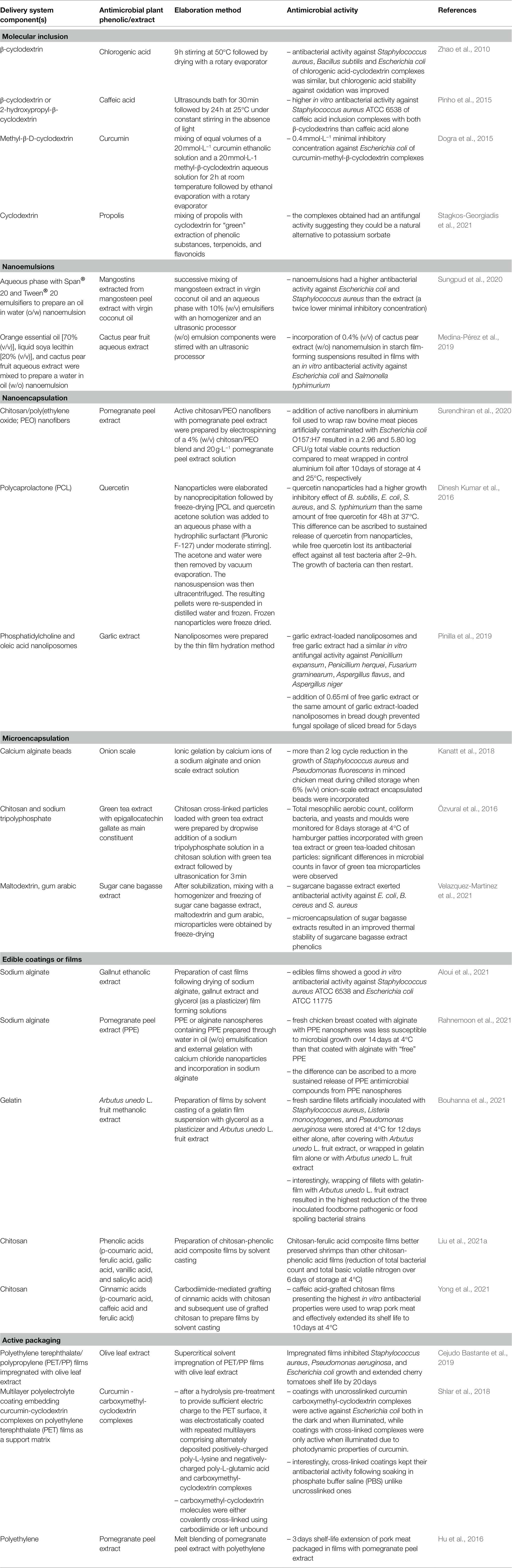
Table 5. Examples illustrating the diversity of systems to deliver antimicrobial plant extracts/phenolics to extend the shelf life or improve the microbial safety of foods or to remove microbial biofilms.
Molecular Inclusion in Cyclodextrins
Molecular inclusion in cyclodextrins of several plant phenolics has increased their solubility in water and their stability before use, while preserving (Zhao et al., 2010) or even in some cases enhancing their antimicrobial activity (Pinho et al., 2015). However, most of antimicrobial activity assays were performed in vitro and efficiency of plant phenolics-cyclodextrin complexes antimicrobial activity in foods has still to be demonstrated. This is likely one of the reasons why, to our knowledge, there is still no commercial application of plant phenolics-cyclodextrin complexes to date unlike for natamycin-cyclodextrin complexes for sliced bread preservation in the United States. Progress is also expected to result from a better understanding of the structural and thermodynamic determinants of interactions between phenolics and cyclodextrins: interestingly, Andreadelis et al. (2021) recently investigated the interactions between two antimicrobial phenolics (caffeic acid and rosmarinic acid) and hydroxypropyl-β-cyclodextrin with either 4, or 10 hydroxypropyl moieties by combining experimental methods, such as isothermal calorimetry and calculatory methods, such as molecular dynamics simulation. Further research in this direction should provide data for the rational design of cyclodextrins with optimized structures for the complexation of a given phenolic.
Nanoemulsion-Based Delivery Systems
Nanoemulsion-based systems to deliver plant antimicrobial compounds in foods have been recently reviewed by McClements et al. (2021). Emulsions are colloidal dispersions of two immiscible fluids. In nanoemulsions, one of them is dispersed in the other one, as small particles with a less than 200 nm diameter. Nanoemulsions can be elaborated with low- or high-energy methods (Saini et al., 2019).
Low-energy methods are based on spontaneous emulsification, phase inversion point, or membrane emulsification. However, they necessitate a high surfactant to oil ratio. Therefore, due to the cost of food-grade surfactants, high-energy methods such as high-pressure homogenization, microfluidization, and sonication are generally preferred for the elaboration of nanoemulsions intended for application in food sector. As illustrated in Table 5, oil-in-water (o/w) nanoemulsions (e.g., antimicrobial phenolics of mangosteen peel soluble in virgin coconut oil; Sungpund et al., 2020) as well as water-in-oil (w/o) nanoemulsions (e.g., water-soluble antimicrobial phenolics of cactus pear; Medina-Pérez et al., 2019) can be prepared and have a protective effect against oxidation of phenolics and/or a positive effect on their antimicrobial activity, by enhancing their dispersion. Although thermodynamically unstable, most of nanoemulsions can be designed to be kinetically stable for sufficient time for commercial applications (McClements et al., 2021), which is advantageous compared to microemulsions. Addition of emulsifiers, which are amphiphilic molecules both facilitating emulsion formation and improving their stability, is necessary. Emulsifiers used can be food ingredients such as proteins, or food additives such as soya lecithin (a phospholipid), modified starch or synthetic food-grade surfactants, such as Tween® 20 [polyoxyethylene (20) sorbitan monolaurate, E 432], which is a non-ionic surfactant.
Besides emulsifiers, the addition of other stabilizers of antimicrobial nanoemulsions, such as thickening agents (e.g., carboxymethyl cellulose, pectin), Ostwald ripening inhibitors (e.g., corn or sunflower oil), weighting agents of the oil phase to inhibit gravitational separation (e.g., dammar gum) is often required to enhance the stability of nano- and micro-emulsions, as briefly reviewed by McClements et al. (2021). As illustrated by Sungpund et al. (2020) in their study on extraction by virgin coconut oil of antimicrobial phenolics from mangosteen peel and subsequent (o/w) nanoemulsion preparation, many other authors explored the possibility to valorize fruits and vegetables wastes through “green” extraction of bioactive compounds, including antimicrobial phenolics and nanoemulsions-based delivery systems, as recently reviewed by Saini et al. (2019). The elaboration of nanoemulsion-based delivery systems is till now better established for essential oils and their components, which are not or poorly soluble in water: essential oil nanoemulsions resulted thus in a better dispersion of their antimicrobial constituents in aqueous systems, often resulting in a higher antimicrobial activity. This approach is more recent for antimicrobial polyphenols and promising for dispersion of poorly soluble in water ones. Future research will not only consider simple (o/w) or (w/o) nanoemulsions of antimicrobial plant phenolics but also the possibilities offered by double (w/o/w) or (o/w/o) nanoemulsions as well as by pickering emulsions to improve their stability and/or their sustained release in food systems. (w1/o/w2) double emulsions make possible the controlled release of water-soluble compounds trapped within the internal aqueous phase. Pickering emulsions are stabilized by solid colloidal particles. The increased stability of pickering emulsions compared to conventional ones is ascribed to the irreversible adsorption of colloidal particles at the interface and the formation of a thicker rigid layer around the dispersed phase (Sabaghi et al., 2021).
Nano- or Micro-Encapsulation
Nano- or micro-encapsulation of ingredients extending the shelf life of foods, including some antimicrobial plant phenolics or phenolic-rich plant extracts, have recently been reviewed by Hosseini and Jafari (2020). Nano- or micro-encapsulation of antimicrobial plant phenolics consists in their incorporation into another(other) compound(s), acting as wall material(s) protecting them from factors affecting their stability and/or activity, such as light or oxygen, before and/or during their use and/or controlling their release once used. Most frequently used wall materials are proteins, polysaccharides or their combinations. Their interactions with antimicrobial phenolics, as well as their intrinsic physicochemical properties condition important parameters, such as encapsulation efficiency or kinetics of release of active phenolics. The choice of wall materials also depends on the technique used to encapsulate antimicrobial plant phenolics. Hosseini and Jafari (2020) classified encapsulation techniques in chemical procedures (e.g., interfacial polymerization), physicochemical procedures (e.g., complex coacervation, entrapment in liposomes, ionic gelation of alginate …), physical procedures (e.g., co-extrusion, freeze- or spray-drying …) and emerging encapsulation procedure (e.g., electrospinning/electrospraying).
Several authors compared the in vitro and the in situ antimicrobial activity of nanoencapsulated antimicrobial plant extracts or phenolics and their free counterparts (Table 5). Interestingly, quercetin-loaded polycaprolactone nanoparticles inhibited for a longer period than free quercetin the in vitro growth of foodborne pathogenic bacteria, likely due to its sustained release from nanoparticles (Dinesh Kumar et al., 2016). However, Pinilla et al. (2019) reported that fungal alteration of sliced bread was not better prevented by the addition of garlic extract-loaded nanoliposomes in dough, than by the addition of the same amount of free garlic extract. More recently, Li et al. (2018) prepared chitosan-based nanoparticles simultaneously loaded with catechin and quercetin by ionic gelation of chitosan by sodium tripolyphosphate followed by crosslinking with genipin. The 180 nm size nanoparticles had a significantly lower MIC against E. coli, S. aureus and B. subtilis than catechin, quercetin and chitosan. These lower MIC values might result both from sustained release of catechin and quercetin and from the electrostatic attraction by the negatively charged surface of bacteria of positively charged chitosan nanoparticles.
Incorporation in Food Contact or Packaging Materials or in Edible Coatings
Incorporation of antimicrobial plant phenolics or extracts in the formulation of food contact materials, deposition of coatings embedding active phenolics or grafting of active phenolics on their surface have been proposed by several authors as a way for controlling microbial growth, cross-contamination, and biofilm formation on food-contacting surfaces (Pei et al., 2014; Dogra et al., 2015; Shlar et al., 2018). Primary food packaging films and trays are also food-contacting surfaces. Moreover, many microbial contaminations of high-added value perishable foods, such as meat pieces or fish fillets, occur in the superficial zone of foods. Therefore, several authors investigated the possibility to functionalize the inner surface of packaging materials with antimicrobial compounds to extend the shelf-life and or improve the microbial safety of perishable foods. Functionalization of food contact materials can rely upon grafting of antimicrobial phenolics resulting from their covalent binding with constitutive polymers or upon their incorporation in the formulation of coatings or packaging materials. In this latter case, coatings or packaging materials act as a reservoir of antimicrobial phenolics, which will reach the surface of food mainly through diffusion. The release of antimicrobial phenolics is controlled by their partition equilibrium between reservoir material and food matrix in direct contact and kinetics of migration of plant phenolics in food contact material and in food, respectively. The release rate of plant phenolics over time should maintain a sufficient concentration to inhibit growth of unwanted microorganisms for a sufficient time to extend the shelf life of food in contact.
In the European Union, the possibility to launch active food packaging systems is authorized since 2009 [Regulation (EC) no 450/2009, 2009]. However, only molecules with a food additive or a food ingredient status might be released from such food packaging systems to foods. This could be limiting for the commercial application of packaging materials releasing phenolic-rich antimicrobial plant extract, since not all molecules transferred from film to food would be characterized. The use of pure phenolics with a food additive [e.g., curcumin (E 100) anthocyanins (E 163) as colorants] or a food ingredient status (e.g., naringin, tannic acid, or hesperetin as food flavourings) would thus be preferred.
Up to now, most of food packaging materials are plastic-based materials made of polyolefins, such as polyethylene (PE), polyethylene terephthalate (PET) or polypropylene (PP) and are elaborated by extrusion-injection for rigid materials such as trays or by extrusion-blowing for films. If antimicrobial compounds are incorporated in their formulation before their elaboration, this requires that antimicrobial activity of phenolics is not lost due to the high temperature conditions (which can exceed 200°C for the extrusion of polypropylene for instance) and the shear stress prevailing during extrusion process. Interestingly, Hu et al. (2016) blended 15 g·kg−1 of pomegranate peel extract with PE by twin-screw extrusion at 160–190°C to elaborate active films and reported a decrease in total volatile basic nitrogen (TVB-N) during refrigerated storage of pork meat packaged in such film and an extension of 3 days of its shelf life. This suggests that pomegranate peel extract kept its antimicrobial activity following extrusion of films. Similarly, Cottaz et al. (2019) incorporated a synthetic antimicrobial phenolic, isobutyl-4-hydroxybenzoate in poly(ethylene-co-vinyl acetate; EVA), linear low density polyethylene (LLDPE), and PP by melt-blending to prepare pellets, which were subsequently used to prepare heat-pressed films: all films had an antibacterial activity, demonstrating thus the preservation of isobutyl-4-hydroxybenzoate antibacterial activity after melt processing. Antimicrobial polyphenols, which are less volatile than antimicrobial essential oil components, and which are not denatured by temperatures exceeding 140–150°C like antimicrobial proteins, such as lysozyme, or inactivated by heat, such as antimicrobial peptides like nisin, are thus promising compounds for incorporation in packaging materials elaborated by extrusion.
However, for regulatory reasons, it might be more interesting to incorporate food-grade phenolic-rich extracts of plants in edible films or coatings: the only condition is that their formulation only contains food ingredients, additives or processing aids. When developing active packaging films or edible films or coatings releasing antimicrobial plant phenolics in the superficial zone of foods (e.g., Zam and Ali, 2018), one must keep in mind that such delivery systems are advantageous, only if a more sustained release of phenolics over time is observed, compared to dipping foods in a plant phenolic solution or spraying a plant phenolic solution on their surface before packaging. Interestingly, Bouhanna et al. (2021) reported that the growth of bacteria contaminating the surface of sardine fillets was better inhibited, when fillets were wrapped with a gelatin film incorporated with Arbutus unedo L. fruit extract, than when they were covered with a layer of A. unedo L. fruit extract. This difference is likely due to the sustained release of A. unedo L. fruit extract antibacterial constituents, resulting from their entrapment in a gelatin-based network and/or to the weak interactions between gelatin and these constituents. Recently, Rahnemoon et al. (2021) compared the preservation of fresh chicken breast meat in alginate coatings with either free pomegranate peel extract or alginate nanospheres loaded with pomegranate peel extract: fresh chicken meat had a longer shelf life when coated with pomegranate peel extract nanospheres. This difference was attributed to the more sustained release of active phenolics from pomegranate peel extract nanospheres. Protein and polysaccharides are the most frequently used biopolymers for the preparation of edible coatings or films. Interestingly, some polysaccharides, such as chitosan, have an intrinsic antimicrobial activity, which can act synergistically with antimicrobial plant phenolics. Yong et al. (2021) recently grafted cinnamic acids with chitosan and observed that films elaborated with caffeic acid-chitosan conjugates had the highest antimicrobial activity. Many other authors recently investigated the effect of the addition of polyphenols to polysaccharides for food packaging applications, as recently reviewed by Zhu (2021).
Further commercial development of antimicrobial plant phenolics delivery systems requires that more studies include their application to a real perishable food to evaluate their potential for shelf life extension. Moreover, there is still a too limited number of studies including an application of such controlled delivery systems to real foods, without including a comparison with the effect of direct addition of the same amount of antimicrobial plant phenolic or extract, which is necessary to estimate the added-value of controlled delivery systems, from which formulation and elaboration represent an additional cost. As suggested by McClements (2018) in his review proposing a “delivery by design” approach for the design of efficacious nanoparticle- and microparticle-based systems to deliver active agents, the development of such systems has to be inspired by quality by design approach used in industrial sector.
Conclusion and Perspectives
Compared to the diversity of antimicrobial plant phenolics and phenolic-rich plant extracts and the current use of biocides for disinfection of food production facilities and of food preservatives, their application is still in its infancy. However, more and more formulations containing phenolic-rich plant extracts with antimicrobial activity are proposed. For instance, the interested reader can refer to Sima et al. (2018), who reported that a commercial formulation with namely citrus, grape seed and oregano extracts in addition to lactic and citric acids effectively reduced in vitro and in vivo pathogenicity of T6SS positive Campylobacter jejuni and Campylobacter coli chicken isolates. T6SS positive highly virulent Campylobacter spp. are positive for the Type VI secretion system (T6SS), which have an increased ability to invade the host gastrointestinal epithelium are highly prevalent in poultry. Therefore, their observations are of particular importance, since preventing Campylobacter spp. infections in humans is considered a public health priority.
Research on antimicrobial activity of edible phenolic-rich plant extracts or plant phenolics expands rapidly, not only benefitting from progress in -omics to better describe their molecular mechanisms of action, but is also being stimulated by (i) the “clean label” marketing trend, which stimulates the search of natural ingredients, as alternatives to synthetic food preservatives, (ii) the necessity to develop more sustainable food systems, favoring the valorization of antimicrobial phenolics-rich food by-products or wastes, by following the principles of circular economy, (iii) the environmental and microbial safety considerations to limit the emergence of multi-drug resistant microorganisms, and (iv) the search for alternatives to plastic food packagings [as illustrated by the “single use plastics” directive in the European Union (EU 2019/904 Directive, 2019)]. However, despite this scientific and societal context favorable to the expansion of the use of antimicrobial phenolic-rich plant extracts or plant phenolics in the food sector, present limitations, such as (i) high cost for extraction of antimicrobial plant phenolics from plants resulting from climate variability and long growth cycle of plants, the requirement of solvents and/or high energy consuming extraction methods or (ii) the necessity to perform more in-depth studies regarding the toxicity of some plant phenolics, which can be pro-oxidant or mutagenic at high dosage and to check that their broad use will not promote the emergence of resistant microbial strains. Some of these challenges were recently reviewed and discussed by Ofosu et al. (2020). The issue of the cost can partly be addressed by applying hurdle technology principles to combine antimicrobial plant phenolics application with other antimicrobial factors (processes, antimicrobial compounds) acting synergistically.
Author Contributions
NO and PD conceptualized, searched initial bibliography, wrote the first manuscript draft, and revised the manuscript. All authors have read and approved the final version of the manuscript.
Funding
The authors gratefully acknowledge the French National Agency for Research (ANR-14-CE20-0005-01 ACTIPHEN) for the financial support of a project on the applicability of antimicrobial plant phenolics for food preservation, which contributed to five the authors more practical insight in the subject of give this review.
Conflict of Interest
The authors declare that the research was conducted in the absence of any commercial or financial relationships that could be construed as a potential conflict of interest.
Publisher’s Note
All claims expressed in this article are solely those of the authors and do not necessarily represent those of their affiliated organizations, or those of the publisher, the editors and the reviewers. Any product that may be evaluated in this article, or claim that may be made by its manufacturer, is not guaranteed or endorsed by the publisher.
References
Abbott, T., Kor-Bicakci, G., Islam, M. S., and Eskicioglu, C. (2020). A review on the fate of legacy and alternative antimicrobials and their metabolites during wastewater and sludge treatment. Int. J. Mol. Sci. 21:9241. doi: 10.3390/ijms21239241
Adamczak, A., Ozarowski, M., and Karpinski, T. M. (2020). Antibacterial activity of some flavonoids and organic acidswidely distributed in plants. J. Clin. Med. 9:109. doi: 10.3390/jcm9010109
Adnan, M., Patel, M., Deshpande, S., Alreshidi, M., Siddiqui, A. J., Reddy, M. N., et al. (2020). Effect of Adiantum philippense extract on biofilm formation, adhesion with its antibacterial activities against foodborne pathogens, and characterization of bioactive metabolites: an in vitro-in silico approach. Front. Microbiol. 11:823. doi: 10.3389/fmicb.2020.00823
Albuquerque, B. R., Heleno, S. A., Oliveira, M. B. P. P., Barros, L., and Ferreira, I. C. F. R. (2021). Phenolic compounds: current industrial applications, limitations and future challenges. Food Funct. 12, 14–29. doi: 10.1039/D0FO02324H
Alderees, F., Mereddy, R., Webber, D., Nirmal, N., and Sultanbawa, Y. (2018). Mechanism of action against food spoilage yeasts and bioactivity of Tasmannia lanceolata, Backhousia citriodora and Syzygium anisatum plant solvent extracts. Foods 7:179. doi: 10.3390/foods7110179
Alirezalu, K., Hesari, J., Eskandari, M. H., Valizadeh, H., and Sirousazar, M. (2017). Effect of green tea, stinging nettle and olive leaves extracts on the quality and shelf life stability of frankfurter type sausage. J. Food Process. Preserv. 41:13100. doi: 10.1111/jfpp.13100
Aloui, H., Deshmukh, A. R., Khomlaem, C., and Kim, B. S. (2021). Novel composite films based on sodium alginate and gallnut extract with enhanced antioxidant, antimicrobial, barrier and mechanical properties. Food Hydrocoll. 113:106508. doi: 10.1016/j.foodhyd.2020.106508
Alvarado-Martinez, Z., Bravo, P., Kennedy, N. F., Krishna, M., Hussain, S., Young, A. C., et al. (2020). Antimicrobial and antivirulence impacts of phenolics on Salmonella enterica serovar Typhimurium. Antibiotics 9:668. doi: 10.3390/antibiotics9100668
An, J. Y., Wang, L. T., Lv, M. J., Wang, J. D., Cai, Z. H., Wang, Y. Q., et al. (2021). An efficiency strategy for extraction and recovery of ellagic acid from waste chestnut shell and its biological activity evaluation. Microchem. J. 160:105616. doi: 10.1016/j.microc.2020.105616
Andrade, M., Benfeito, S., Soares, P., Magalhães e Silva, D., Loureiro, J., Borges, A., et al. (2015). Fine-tuning of the hydrophobicity of caffeic acid: studies on the antimicrobial activity against Staphylococcus aureus and Escherichia coli. RSC Adv. 5:53915. doi: 10.1039/C5RA05840F
Andreadelis, I., Chatziathanasiadou, Μ. V., Ntountaniotis, D., Valsami, G., Papaemmanouil, C., Christodoulou, E., et al. (2021). Charting the structural and thermodynamic determinants in phenolic acid natural product - cyclodextrin encapsulations. J. Biomol. Struct. Dyn. 39, 2642–2658. doi: 10.1080/07391102.2020.1751716
Angiolillo, L., Spinelli, S., Conte, A., and Matteo Del Nobile, M. A. (2019). Extract from broccoli byproducts to increase fresh filled pasta shelf life. Foods 8:621. doi: 10.3390/foods8120621
Ansari, J. A., Naz, S., Tarar, O. M., Siddiqi, R., Haider, M. S., and Jamil, K. (2015). Binding effect of proline-rich-proteins (PRPs) on in vitro antimicrobial activity of the flavonoids. Braz. J. Microbiol. 46, 183–188. doi: 10.1590/S1517-838246120130280
Apostolidis, E., Kwon, Y.-I., and Shetty, K. (2008). Inhibition of Listeria monocytogenes by oregano, cranberry and sodium lactate combination in broth and cooked ground beef systems and likely mode of action through proline metabolism. Int. J. Food Microbiol. 128, 317–324. doi: 10.1016/j.ijfoodmicro.2008.09.012
Araujo, M. O., Freire Pessoa, H. L., Lira, A. B., Castillo, Y. P., and de Sousa, D. P. (2019). Synthesis, antibacterial evaluation, and QSAR of caffeic acid derivatives. J. Chem. 2019:3408315. doi: 10.1155/2019/3408315
Baptista, J., Simões, M., and Borges, A. (2019). Effect of plant-based catecholic molecules on the prevention and eradication of Escherichia coli biofilms: a structure activity relationship study. Int. Biodeterior. Biodegrad. 141:101. doi: 10.1016/j.ibiod.2018.02.004
Başyiğit, B., Sağlam, H., Köroğlu, K., and Karaaslan, M. (2020). Compositional analysis, biological activity, and food protecting ability of ethanolic extract of Quercus infectoria gall. J. Food Process. Preserv. 44:14692. doi: 10.1111/jfpp.14692
Bellon-Fontaine, M. N., Rault, J., and van Oss, C. J. (1996). Microbial adhesion to solvents: a novel method to determine the electron-donor/electron acceptor or Lewis acid-base properties of microbial cells. Colloids Surf. B 7, 47–53. doi: 10.1016/0927-7765(96)01272-6
Bhattacharya, D., Ghosh, D., Bhattacharya, S., Sarkar, S., Karmakar, P., Koley, H., et al. (2018). Antibacterial activity of polyphenolic fraction of Kombucha against Vibrio cholerae: targeting cell membrane. Lett. Appl. Microbiol. 66, 145–152. doi: 10.1111/lam.12829
Bolling, B. W. (2017). Almond polyphenols: methods of analysis, contribution to food quality, and health promotion. Compr. Rev. Food Sci. Food Saf. 16, 346–368. doi: 10.1111/1541-4337.12260
Borges, A., Ferreira, C., Saavedra, M. J., and Simoes, M. (2013). Antibacterial activity and mode of action of ferulic and gallic acids against pathogenic bacteria. Microb. Drug Resist. 19, 256–265. doi: 10.1089/mdr.2012.0244
Borges, A., Saavedra, M. J., and Simoes, M. (2012). The activity of ferulic and gallic acids in biofilm prevention and control of pathogenic bacteria. Biofouling 28, 755–767. doi: 10.1080/08927014.2012.706751
Bouarab-Chibane, L., Degraeve, P., Ferhout, H., Bouajila, J., and Oulahal, N. (2018a). Plant antimicrobial polyphenols as potential natural food preservatives. J. Sci. Food Agric. 99, 1457–1474. doi: 10.1002/jsfa.9357
Bouarab-Chibane, L., Forquet, V., Clément, Y., Lanteri, P., Bordes, C., Bouajila, J., et al. (2018b). Effect of interactions of plant phenolics with bovine meat proteins on their antibacterial activity. Food Control 90, 189–198. doi: 10.1016/j.foodcont.2018.03.006
Bouarab-Chibane, L., Forquet, V., Lantéri, P., Clément, Y., Léonard-Akkari, L., Oulahal, N., et al. (2019). Antibacterial properties of polyphenols: characterization and QSAR (Quantitative Structure–Activity Relationship) models. Front. Microbiol. 10:829. doi: 10.3389/fmicb.2019.00829
Bouarab-Chibane, L., Oulahal, N., Dumas, E., Trinh Thi Thanh, N., Bouajila, J., Souchard, J. P., et al. (2018c). Effect of interaction with food constituents on plant extracts antibacterial activity. Food Sci. Appl. Biotechnol. 1, 77–85. doi: 10.30721/fsab2018.v1.i1.27
Bouarab-Chibane, L., Ouled-Bouhedda, B., Léonard, L., Gemelas, L., Bouajila, J., Ferhout, H., et al. (2017). Preservation of fresh ground beef patties using plant extracts combined with a modified atmosphere packaging. Eur. Food Res. Technol. 243, 1997–2009. doi: 10.1007/s00217-017-2905-3
Bouhanna, I., Boussaa, A., Boumaza, A., Rigano, D., Maisto, M., Basile, A., et al. (2021). Characterization and antibacterial activity of gelatin-based film incorporated with Arbutus unedo L. fruit extract on Sardina pilchardus. J. Food Process. Preserv. 45:e15424. doi: 10.1111/jfpp.15424
Boziaris, I. S., Proestos, C., Kapsokefalou, M., and Komaitis, M. (2011). Antimicrobial effect of Filipendula ulmaria plant extract against selected foodborne pathogenic and spoilage bacteria in laboratory media, fish flesh and fish roe product. Food Technol. Biotechnol. 49:263.
Cabarkapa, I. S., Sedej, I. J., Sakac, M. B., Saric, L. C., and Plavsic, D. V. (2008). Antimicrobial activity of buckwheat (Fagopyrum esculentum Moench) hulls extract. Food Process. Qual. Saf. 35, 159–163.
Carraro, L., Fasolato, L., Montemurro, F., Martino, M. E., Balzan, S., Servili, M., et al. (2014). Polyphenols from olive mill waste affect biofilm formation and motility in Escherichia coli K-12. Microb. Biotechnol. 7, 265–275. doi: 10.1111/1751-7915.12119
Castillo Rivera, M. L., Aymoto Hassimotto, N. M., Vanessa Bueris, V., Palma Sircili, M., Alves de Almeida, F., and Pinto, U. M. (2019). Effect of Capsicum frutescens extract, capsaicin, and luteolin on quorum sensing regulated phenotypes. J. Food Sci. 84, 1477–1486. doi: 10.1111/1750-3841.14648
Cejudo Bastante, C., Casas Cardoso, L., Fernández-Ponce, M. T., Mantell Serrano, C., and Martínez de la Ossa, E. J. (2019). Supercritical impregnation of olive leaf extract to obtain bioactive films effective in cherry tomato preservation. Food Packag. Shelf Life 21:100338. doi: 10.1016/j.fpsl.2019.100338
Chan, C.-L., Gan, R.-Y., Shah, N. P., and Corke, H. (2018). Polyphenols from selected dietary spices and medicinal herbs differentially affect common food-borne pathogenic bacteria and lactic acid bacteria. Food Control 92, 437–443. doi: 10.1016/j.foodcont.2018.05.032
Chen, J., Liao, C., Ouyang, X., Kahramanoglu, I., Gan, Y., and Li, M. (2020). Antimicrobial activity of pomegranate peel and its applications on food preservation. J. Food Qual. 2020:8850339. doi: 10.1155/2020/8850339
Cheynier, V., Comte, G., Davies, K. M., Lattanzio, V., and Martens, S. (2013). Plant phenolics: recent advances on their biosynthesis, genetics, and ecophysiology. Plant Physiol. Biochem. 72, 1–20. doi: 10.1016/j.plaphy.2013.05.009
Chusri, S., and Voravuthikunchai, S. P. (2011). Damage of staphylococcal cytoplasmic membrane by Quercus infectoria G. Olivier and its components. Lett. Appl. Microbiol. 52, 565–572. doi: 10.1111/j.1472-765X.2011.03041.x
Cossu, A., Huang, K., Cossu, M., Rohan, V., Tikekarc, R. V., and Nitin, N. (2018). Fog, phenolic acids and UV-A light irradiation: a new antimicrobial treatment for decontamination of fresh produce. Food Microbiol. 76, 204–208. doi: 10.1016/j.fm.2018.05.013
Cottaz, A., Bouarab, L., De Clercq, J., Oulahal, N., Degraeve, P., and Joly, C. (2019). Potential of incorporation of antimicrobial plant phenolics into polyolefin-based food contact materials to produce active packaging by melt-blending: proof of concept with isobutyl-4-hydroxybenzoate. Front. Chem. 7:148. doi: 10.3389/fchem.2019.00148
Cueva, C., Moreno-Arribas, M. V., Martin-Alvarez, P. J., Bills, G., Vicente, M. F., Basilio, A., et al. (2010). Antimicrobial activity of phenolic acids against commensal, probiotic and pathogenic bacteria. Res. Microbiol. 161, 372–382. doi: 10.1016/j.resmic.2010.04.006
Cushnie, T. P., and Lamb, A. J. (2005). Antimicrobial activity of flavonoids. Int. J. Antimicrob. Agents 26, 343–356. doi: 10.1016/j.ijantimicag.2005.09.002
da Cruz Cabral, L., Fernández Pinto, V., and Patriarca, A. (2013). Application of plant derived compounds to control fungal spoilage and mycotoxin production in foods. Int. J. Food Microbiol. 166, 1–14. doi: 10.1016/j.ijfoodmicro.2013.05.026
del Valle, P., García-Armesto, M. R., de Arriaga, D., Gonzalez-Donquiles, C., Rodríguez-Fernandez, P., and Rúa, J. (2016). Antimicrobial activity of kaempferol and resveratrol in binary combinations with parabens or propyl gallate against Enterococcus faecalis. Food Control 61, 213–220. doi: 10.1016/j.foodcont.2015.10.001
Difonzo, G., Squeo, G., Calasso, M., Pasqualone, A., and Caponio, F. (2019). Physico-chemical, microbiological and sensory evaluation of ready-to-use vegetable pâté added with olive leaf extract. Foods 8:138. doi: 10.3390/foods8040138
Difonzo, G., Squeo, G., Pasqualone, A., Summo, C., Paradiso, V. M., and Caponio, F. (2021). The challenge of exploiting polyphenols from olive leaves: addition to foods to improve their shelf life and nutritional value. J. Sci. Food Agric. 101, 3099–3116. doi: 10.1002/jsfa.10986
Dinesh Kumar, V., Verma, P. R. P., and Singh, S. K. (2016). Morphological and in vitro antibacterial efficacy of quercetin loaded nanoparticles against food-borne microorganisms. LWT Food Sci. Technol. 66, 638–650. doi: 10.1016/j.lwt.2015.11.004
Dogra, N., Choudhary, R., Kohli, P., Haddock, J. D., Makwana, S., Horev, B., et al. (2015). Polydiacetylene nanovesicles as carriers of natural phenylpropanoids for creating antimicrobial food-contact surfaces. J. Agric. Food Chem. 63, 2557–2565. doi: 10.1021/jf505442w
Dong, G., Liu, H., Yu, X., Zhang, X., Lu, H., Zhou, T., et al. (2018). Antimicrobial and anti-biofilm activity of tannic acid against Staphylococcus aureus. Nat. Prod. Res. 32, 2225–2228. doi: 10.1080/14786419.2017.1366485
Duggirala, S., Rakesh, P., Nankar, R. P., Rajendran, S., and Doble, M. (2014). Phytochemicals as inhibitors of bacterial cell division protein FtsZ: coumarins are promising candidates. Appl. Biochem. Biotechnol. 174, 283–296. doi: 10.1007/s12010-014-1056-2
Efenberger-Szmechtyk, M., Nowak, A., and Czyzowska, A. (2021). Plant extracts rich in polyphenols: antibacterial agents and natural preservatives for meat and meat products. Crit. Rev. Food Sci. Nutr. 61, 149–178. doi: 10.1080/10408398.2020.1722060
EFSA Panel (2016). Scientific opinion on the re-evaluation sulfur dioxide (E 220), sodium sulfite (E 221), sodium bisulfite (E 222), sodium metabisulfite (E 223), potassium metabisulfite (E 224), calcium sulfite (E 226), calcium bisulfite (E 227) and potassium bisulfite (E 228) as food additives. EFSA J. 14:4438. doi: 10.2903/j.efsa.2016.4438
EFSA PanelMortensen, A., Aguilar, F., Crebelli, R., Di Domenico, A., Dusemund, B., et al. (2017). Re-evaluation of potassium nitrite (E 249) and sodium nitrite (E 250) as food additives. EFSA J. 15:4786. doi: 10.2903/j.efsa.2017.4786
EU 2019/904 Directive (2019). Directive of the European Parliament and of the Council of 5 June 2019 on the reduction of the impact of certain plastic products on the environment.
Falleh, H., Ben Jemaa, M., Saada, M., and Ksouri, R. (2020). Essential oils: a promising eco-friendly food preservative. Food Chem. 330:127268. doi: 10.1016/j.foodchem.2020.127268
Fang, Y., Lu, Y., Zang, X., Wu, T., Qi, X. J., Pan, S., et al. (2016). 3D-QSAR and docking studies of flavonoids as potent Escherichia coli inhibitors. Sci. Rep. 6:23634. doi: 10.1038/srep23634
Ferdes, M. (2018). “Antimicrobial compounds from plants,” in Fighting Antimicrobial Resistance. ed. A. Budimir (Zagreb, Croatia: IAPC-OBP), 243–271.
Fu, Y., Sarkar, P., Bhunia, A. K., and Yao, Y. (2016). Delivery systems of antimicrobial compounds to food. Trends Food Sci. Technol. 57, 165–177. doi: 10.1016/j.tifs.2016.09.013
Galanakis, C. M., Tsatalas, P., Charalambous, Z., and Galanakis, I. M. (2018). Control of microbial growth in bakery products fortified with polyphenols recovered from olive mill wastewater. Environ. Technol. Innov. 10, 1–15. doi: 10.1016/j.eti.2018.01.006
Gomes, F., Dias, M. I., Lima, A., Barros, L., Rodrigues, M. E., Ferreira, I. C. F. R., et al. (2020). Satureja montana L. and Origanum majorana L. decoctions: antimicrobial activity, mode of action and phenolic characterization. Antibiotics 9:294. doi: 10.3390/antibiotics9060294
Gonelimali, F. D., Lin, J., Miao, W., Xuan, J., Charles, F., Chen, M., et al. (2018). Antimicrobial properties and mechanism of action of some plant extracts against food pathogens and spoilage microorganisms. Front. Microbiol. 9:1639. doi: 10.3389/fmicb.2018.01639
Gong, S., Fei, P., Sun, Q., Guo, L., Jiang, L., Duo, K., et al. (2021). Action mode of cranberry anthocyanin on physiological and morphological properties of Staphylococcus aureus and its application in cooked meat. Food Microbiol. 94:103632. doi: 10.1016/j.fm.2020.103632
Guo, N., Zhu, Y. W., Jiang, Y. W., Li, H. K., Liu, Z. M., Wang, W., et al. (2020). Improvement of flavonoid aglycone and biological activity of mulberry leaves by solid-state fermentation. Ind. Crop. Prod. 148:112287. doi: 10.1016/j.indcrop.2020.112287
Gutiérrez-Fernández, J., García-Armesto, M. R., Álvarez-Alonso, R., del Valle, P., de Arriaga, D., and Rúa, J. (2013). Antimicrobial activity of binary combinations of natural and synthetic phenolic antioxidants against Enterococcus faecalis. J. Dairy Sci. 96, 4912–4920. doi: 10.3168/jds.2013-6643
Guzzo, F., Scognamiglio, M., Fiorentino, A., Buommino, E., and Brigida D’Abrosca, B. (2020). Plant derived natural products against Pseudomonas aeruginosa and Staphylococcus aureus: antibiofilm activity and molecular mechanisms. Molecules 25:5024. doi: 10.3390/molecules25215024
Harborne, J. B. (2001). Twenty-five years of chemical ecology. Nat. Prod. Rep. 18, 361–379. doi: 10.1039/b005311m
Hayrapetyan, H., Hazeleger, W. C., and Beumer, R. R. (2012). Inhibition of Listeria monocytogenes by pomegranate (Punica granatum) peel extract in meat paté at different temperatures. Food Control 23, 66–72. doi: 10.1016/j.foodcont.2011.06.012
Hossain, M. A., Lee, S. J., Park, N. H., Mechesso, A. F., Birhanu, B. T., Kang, J. W., et al. (2017). Impact of phenolic compounds in the acyl homoserine lactonemediated quorum sensing regulatory pathways. Sci. Rep. 7:10618. doi: 10.1038/s41598-017-10997-5
Hosseini, H., and Jafari, S. M. (2020). Introducing nano/microencapsulated bioactive ingredients for extending the shelf-life of food products. Adv. Colloid Interface Sci. 282:102210. doi: 10.1016/j.cis.2020.102210
Hu, B., Liu, X., Zhang, C., and Zeng, X. (2017). Food macromolecule based nanodelivery systems for enhancing the bioavailability of polyphenols. J. Food Drug Anal. 25, 3–15. doi: 10.1016/j.jfda.2016.11.004
Hu, W. S., Nam, D. M., Choi, J. Y., Kim, J. S., and Koo, O. K. (2019). Anti-attachment, anti-biofilm, and antioxidant properties of Brassicaceae extracts on Escherichia coli O157:H7. Food Sci. Biotechnol. 28, 1881–1890. doi: 10.1007/s10068-019-00621-9
Hu, S., Wang, H., Han, W., Ma, Y., Shao, Z., and Li, L. (2016). Development of double-layer active films containing pomegranate peel extract for the application of pork packaging. J. Food Process Eng. 40:12388. doi: 10.1111/jfpe.12388
Hygreeva, D., and Pandey, M. C. (2016). Novel approaches in improving the quality and safety aspects of processed meat products through high pressure processing technology-A review. Trends Food Sci. Technol 54:175. doi: 10.1016/j.tifs.2016.06.002
Jabria, M. A., Rtibib, K., Ben-Saida, A., Aouadhic, C., Hosnid, K., Sakly, M., et al. (2016). Antidiarrhoeal, antimicrobial and antioxidant effects of myrtle berries (Myrtus communis L.) seeds extract. J. Pharm. Pharmacol. 68, 264–274. doi: 10.1111/jphp.12505
Jahanban-Esfahlan, A., Ostadrahimi, A., Tabibiazar, M., and Amarowicz, R. (2019). A comprehensive review on the chemical constituents and functional uses of walnut (Juglans spp.) husk. Int. J. Mol. Sci. 20:3920. doi: 10.3390/ijms20163920
Jakobek, L. (2015). Interactions of polyphenols with carbohydrates, lipids and proteins. Food Chem. 175, 556–567. doi: 10.1016/j.foodchem.2014.12.013
Kamiya, M., Mori, T., Nomura, M., Inagaki, T., Nonogaki, T., Nagatsu, A., et al. (2019). Tradescantia pallida extract inhibits biofilm formation in Pseudomonas aeruginosa. Nagoya J. Med. Sci. 81, 439–452. doi: 10.18999/nagjms.81.3.439
Kanatt, S. R., Tari, S., and Chawla, S. P. (2018). Encapsulation of extract prepared from irradiated onion scales in alginate beads: a potential functional food ingredient. J. Food Meas. Charact. 12, 848–858. doi: 10.1007/s11694-017-9699-7
Kchaou, W., Abbès, F., Ben Mansour, R., Blecker, C., Attia, H., and Besbes, S. (2016). Phenolic profile, antibacterial and cytotoxic properties of second grade date extract from Tunisian cultivars (Phoenix dactylifera L.). Food Chem. 194, 1048–1055. doi: 10.1016/j.foodchem.2015.08.120
Kim, M. H., Jo, S. H., Ha, K. S., Song, J. H., Jang, H. D., and Kwon, Y. I. (2010). Antimicrobial activities of 1,4-benzoquinones and wheat germ extract. J. Microbiol. Biotechnol. 20, 1204–1209. doi: 10.4014/jmb.1004.04037
Kırmusaoğlu, S. (2019). Sensitizing of β-lactam resistance by tannic acid in methicillin-resistant S. aureus. World J. Microbiol. Biotechnol. 35:57. doi: 10.1007/s11274-019-2637-6
Klancnik, A., Piskernik, S., Smole Mozina, S., Gasperlin, L., and Jersek, B. (2011). Investigation of some factors affecting the antibacterial activity of rosemary extracts in food models by a food microdilution method. Int. J. Food Sci. Technol. 46, 413–420. doi: 10.1111/j.1365-2621.2010.02504.x
Köhler, H., Contreras, R. A., Pizarro, M., Cortés-Antíquera, R., and Zúniga, G. E. (2017). Antioxidant responses induced by UVB radiation in Deschampsia antarctica Desv. Front. Plant Sci. 8:921. doi: 10.3389/fpls.2017.00921
LaSarre, B., and Federle, M. J. (2013). Exploiting quorum sensing to confuse bacterial pathogens. Microbiol. Mol. Biol. Rev. 77, 73–111. doi: 10.1128/MMBR.00046-12
Léonard, L., Bouarab Chibane, L., Ouled Bouhedda, B., Degraeve, P., and Oulahal, N. (2016). Recent advances on multi-parameter flow cytometry to characterize antimicrobial treatments. Front. Microbiol. 7:1225. doi: 10.3389/fmicb.2016.01225
Li, F., Jin, H., Xiao, J., Yin, X., Liu, X., Li, D., et al. (2018). The simultaneous loading of catechin and quercetin on chitosan-based nanoparticles as effective antioxidant and antibacterial agent. Food Res. Int. 111, 351–360. doi: 10.1016/j.foodres.2018.05.038
Lin, Y. T., Labbe, R. G., and Shetty, K. (2005). Inhibition of Vibrio parahaemolyticus in seafood systems using oregano and cranberry phytochemical synergies and lactic acid. Innov. Food Sci. Emerg. Technol. 6, 453–458. doi: 10.1016/j.ifset.2005.04.002
Linzner, N., Fritsch, V. N., Busche, T., Tung, Q. N., Loi, V. V., Bernhardt, J., et al. (2020). The plant-derived naphthoquinone lapachol causes an oxidative stress response in Staphylococcus aureus. Free Radic. Biol. Med. 158, 126–136. doi: 10.1016/j.freeradbiomed.2020.07.025
Liu, Y., McKeever, L. C., and Malik, N. S. A. (2017a). Assessment of the antimicrobial activity of olive leaf extract against foodborne bacterial pathogens. Front. Microbiol. 8:113. doi: 10.3389/fmicb.2017.00113
Liu, Z., Pan, Y., Li, X., Jie, J., and Zeng, M. (2017b). Chemical composition, antimicrobial and anti-quorum sensing activities of pummelo peel flavonoid extract. Ind. Crop. Prod. 109, 862–868. doi: 10.1016/J.INDCROP.2017.09.054
Liu, W., Xie, J., Li, L., Xue, B., Li, X., Gan, J., et al. (2021a). Properties of phenolic acid-chitosan composite films and preservative effect on Penaeus vannamei. J. Mol. Struct. 1239:130531. doi: 10.1016/j.molstruc.2021.130531
Liu, X., Yue, Y., Wu, Y., Zhong, K., Bu, Q., and Gao, H. (2021b). Discovering the antibacterial mode of action of 3-p-trans-coumaroyl-2-hydroxyquinic acid, a natural phenolic compound, against Staphylococcus aureus through an integrated transcriptomic and proteomic approach. J. Food Saf. 41:e12861. doi: 10.1111/jfs.12861
Márquez-Rodríguez, A. S., Nevárez-Baca, S., Lerma-Hernández, J. C., Hernández-Ochoa, L. R., Nevárez-Moorillon, G. V., Gutiérrez-Méndez, N., et al. (2020). In vitro antibacterial activity of Hibiscus sabdariffa L. phenolic extract and its in situ application on shelf-life of beef meat. Foods 9:1080. doi: 10.3390/foods9081080
Matejczyk, M., Swisłocko, R., Golonko, A., Lewandowski, W., and Hawrylika, E. (2018). Cytotoxic, genotoxic and antimicrobial activity of caffeic and rosmarinic acids and their lithium, sodium and potassium salts as potential anticancer compounds. Adv. Med. Sci. 63, 14–21. doi: 10.1016/j.advms.2017.07.003
McClements, D. J. (2018). Delivery by Design (DbD): a standardized approach to the development of efficacious nanoparticle- and microparticle-based delivery systems. Compr. Rev. Food Sci. Food Saf. 17, 200–219. doi: 10.1111/1541-4337.12313
McClements, D. J., Das, A. K., Dhar, P., Nanda, P. K., and Chatterjee, N. (2021). Nanoemulsion-based technologies for delivering natural plant-based antimicrobials in foods. Front. Sustain. Food Syst. 5:643208. doi: 10.3389/fsufs.2021.643208
Medina-Pérez, G., Hernández-Uribe, J. P., Fernández-León, D., Prince, L., Fernández-Luqueño, F., and Campos-Montiel, R. G. (2019). Application of nanoemulsions (w/o) with active compounds of cactus pear fruit in starch films to improve antioxidant activity and incorporate antibacterial property. J. Food Process Eng. 42:e13268. doi: 10.1111/jfpe.13268
Mellegard, H., Stalheim, T., Hormazabal, V., Granum, P. E., and Hardy, S. P. (2009). Antibacterial activity of sphagnum acid and other phenolic compounds found in Sphagnum papillosum against foodborne bacteria. Lett. Appl. Microbiol. 49, 85–90. doi: 10.1111/j.1472-765X.2009.02622.x
Mfonku, N. A., Tadjong, A. T., Kamsu, G. T., Kodjio, N., Ren, J., Mbah, J. A., et al. (2021). Isolation and characterization of antisalmonellal anthraquinones and coumarins from Morinda lucida Benth. (Rubiaceae). Chem. Pap. 75, 2067–2073. doi: 10.1007/s11696-020-01460-3
Mhalla, D., Bouaziz, A., Ennouri, K., Chawech, R., Smaoui, S., Jarraya, R., et al. (2017). Antimicrobial activity and bioguided fractionation of Rumex tingitanus extracts for meat preservation. Meat Sci. 125, 22–29. doi: 10.1016/j.meatsci.2016.11.011
Miceli, A., Aleo, A., Corona, O., Sardina, M. T., Mammina, C., and Settanni, L. (2014). Antibacterial activity of Borago officinalis and Brassica juncea aqueous extracts evaluated in vitro and in situ using different food model systems. Food Control 40, 157–164. doi: 10.1016/j.foodcont.2013.12.006
Mikeš, O., Vrchotova, N., Tříska, J., Kyselákova, M., and Šmidrkal, J. (2008). Distribution of major polyphenolic compounds in vine grapes of different cultivars growing in South Moravian vineyards. Czech J. Food Sci. 26, 182–189. doi: 10.17221/1591-CJFS
Mileski, K. S., Ćirić, A. D., Trifunović, S. S., Ristić, M. S., Soković, M. D., Matevski, V. S., et al. (2016). Heracleum orphanidis: chemical characterisation, and comparative evaluation of antioxidant and antimicrobial activities with specific interest in the influence on Pseudomonas aeruginosa PAO1. Food Funct. 7, 4061–4074. doi: 10.1039/C6FO01018K
Mir, S. A., Dar, B. N., Wani, A. A., and Shah, M. A. (2018). Effect of plant extracts on the techno-functional properties of biodegradable packaging films. Trends Food Sci. Technol. 80, 141–154. doi: 10.1016/j.tifs.2018.08.004
Mishra, R., Panda, A. K., De Mandal, S., Shakeel, M., Bisht, S. S., and Khan, J. (2020). Natural anti-biofilm agents: strategies to control biofilm-forming pathogens. Front. Microbiol. 11:566325. doi: 10.3389/fmicb.2020.566325
Monente, C., Bravo, J., Vitas, A. I., Arbillaga, L., Paz de Peña, M., and Cid, C. (2015). Coffee and spent coffee extracts protect against cell mutagens and inhibit growth of food-borne pathogen microorganisms. J. Funct. Foods 12, 365–374. doi: 10.1016/j.jff.2014.12.006
Mora-Pale, M., Bhan, N., Masuko, S., James, P., Wood, J., McCallum, S., et al. (2015). Antimicrobial mechanism of resveratrol-trans dihydrodimer produced from peroxidase-catalyzed oxidation of resveratrol. Biotechnol. Bioeng. 112, 2417–2428. doi: 10.1002/bit.25686
Müller, G., and Kramer, A. (2008). Biocompatibility index of antiseptic agents by parallel assessment of antimicrobial activity and cellular cytotoxicity. J. Antimicrob. Chemother. 61, 1281–1287. doi: 10.1093/jac/dkn125
Munekata, P. E. S., Nieto, G., Pateiro, M., and Lorenzo, J. M. (2020). Phenolic compounds obtained from Olea europaea by-products and their use to improve the quality and shelf life of meat and meat products - a review. Antioxidants 9:1061. doi: 10.3390/antiox9111061
Muzolf-Panek, M., Kaczmareka, A., Tomaszewska-Grasa, J., Cegielska-Radziejewskaa, R., and Majcher, M. (2019). Oxidative and microbiological stability of raw ground pork during chilled storage as affected by plant extracts. Int. J. Food Prop. 22, 111–129. doi: 10.1080/10942912.2019.1579834
Mwaurah, P. W., Kumar, S., Kumar, N., Panghal, A., Attkan, A. K., Singh, V. K., et al. (2020). Physicochemical characteristics, bioactive compounds and industrial applications of mango kernel and its products: a review. Compr. Rev. Food Sci. Food Saf. 19, 2421–2446. doi: 10.1111/1541-4337.12598
Nakayama, M., Tomiyama, D., Shigemune, N., Mitani, A., Xu, W., and Miyamoto, T. (2015). Cell surface hydrophobicity contributes to lactobacillus tolerance to antibacterial actions of catechins. Food Sci. Technol. Res. 21, 583–588. doi: 10.3136/fstr.21.583
Narwojsz, A., Tańska, M., Mazur, B., and Borowska, E. J. (2019). Fruit physical features, phenolic compounds profile and inhibition activities of cranberry cultivars (Vaccinium macrocarpon) compared to wild-grown cranberry (Vaccinium oxycoccus). Plant Foods Hum. Nutr. 74, 300–306. doi: 10.1007/s11130-019-00737-7
Nirmal, N. P., and Benjakul, S. (2011). Use of tea extracts for inhibition of polyphenoloxidase and retardation of quality loss of Pacific white shrimp during iced storage. LWT Food Sci. Technol. 44, 924–932. doi: 10.1016/j.lwt.2010.12.007
Nohynek, L. J., Alakomi, H. L., Kahkonen, M. P., Heinonen, M., Helander, K. M., Oksman-Caldentey, K. M., et al. (2006). Berry phenolics: Antimicrobial properties and mechanisms of action against severe human pathogens. Nutr. Cancer 54:18. doi: 10.1207/s15327914nc5401_4
Nuta, D. C., Limban, C., Chirita, C., Chifiriuc, M. C., Costea, T., Ionita, P., et al. (2021). Contribution of essential oils to the fight against microbial biofilms - a review. PRO 9:537. doi: 10.3390/pr9030537
Ofosu, F. K., Daliri, E.-M., Elahi, F., Chelliah, R., Lee, B.-H., and Oh, D.-H. (2020). New insights on the use of polyphenols as natural preservatives and their emerging safety concerns. Front. Sustain. Food Syst. 4:525810. doi: 10.3389/fsufs.2020.525810
Onsare, J. G., and Arora, D. S. (2015). Antibiofilm potential of flavonoids extracted from Moringa oleifera seed coat against Staphylococcus aureus, Pseudomonas aeruginosa and Candida albicans. J. Appl. Microbiol. 18:313. doi: 10.1111/jam.12701
Oulahal, N., Brice, W., Martial, A., and Degraeve, P. (2008). Quantitative analysis of survival of Staphylococcus aureus or Listeria innocua on two types of surfaces: polypropylene and stainless steel in contact with three different dairy products. Food Control 19, 178–185. doi: 10.1016/j.foodcont.2007.03.006
Ozdemir, O. O., and Soyer, F. (2020). Pseudomonas aeruginosa presents multiple vital changes in its proteome in the presence of 3-hydroxyphenylacetic acid, a promising antimicrobial agent. ACS Omega 5, 19938–19951. doi: 10.1021/acsomega.0c00703
Özvural, E. B., Huang, Q., and Chikindas, M. L. (2016). The comparison of quality and microbiological characteristic of hamburger patties enriched with green tea extract using three techniques: direct addition, edible coating and encapsulation. LWT Food Sci. Technol. 68, 385–390. doi: 10.1016/j.lwt.2015.12.036
Pacheco-Ordaz, R., Wall-Medrano, A., Goni, M. G., Ramos-Clamont-Montfort, G., Ayala-Zavala, J. F., and Gonzalez-Aguilar, G. A. (2017). Effect of phenolic compounds on the growth of selected probiotic and pathogenic bacteria. Lett. Appl. Microbiol. 66, 25–31. doi: 10.1111/lam.12814
Pei, J., Zhang, Y., Zhang, F., Yu, X., and Yan, Y. (2014). Enhancing antimicrobial activity in unbleached kraft pulp using laccase and phenolic compounds. Bioresources 8, 515–529.
Perez-Jimenez, J., Neveu, V., Vos, F., and Scalbert, A. (2010). Identification of the 100 richest dietary sources of polyphenols: an application of the phenol-explorer database. Eur. J. Clin. Nutr. 64, S112–S120. doi: 10.1038/ejcn.2010.221
Pernin, A., Dubois-Brissonnet, F., Roux, S., Masson, M., Bosc, V., and Maillard, M. N. (2018). Phenolic compounds can delay the oxidation of polyunsaturated fatty acids and the growth of Listeria monocytogenes: structure-activity relationships. J. Sci. Food Agric. 98, 5401–5408. doi: 10.1002/jsfa.9082
Pernin, A., Guillier, L., and Dubois-Brissonnet, F. (2019). Inhibitory activity of phenolic acids against Listeria monocytogenes: deciphering the mechanisms of action using three different models. Food Microbiol. 80, 18–24. doi: 10.1016/j.fm.2018.12.010
Phadungkit, M., and Luanratana, O. (2006). Anti-Salmonella activity of constituents of Ardisia elliptica Thunb. Nat. Prod. Res. 20, 693–696. doi: 10.1080/14786410600661849
Phan, H. T. T., Yoda, T., Chahal, B., Morita, M., Takagi, M., and Vestergaard, M. C. (2014). Structure-dependent interactions of polyphenols with a biomimetic membrane system. Biochim. Biophys. Acta 1838, 2670–2677. doi: 10.1016/j.bbamem.2014.07.001
Piccolella, S., Crescente, G., Candela, L., and Pacifico, S. (2019). Nutraceutical polyphenols: new analytical challenges and opportunities. J. Pharm. Biomed. Anal. 175:112774. doi: 10.1016/j.jpba.2019.07.022
Pinho, E., Soares, G., and Henriques, M. (2015). Evaluation of antibacterial activity of caffeic acid encapsulated by β-cyclodextrins. J. Microencapsul. 32, 804–810. doi: 10.3109/02652048.2015.1094531
Pinilla, C. M. B., Thys, R. C. S., and Brandelli, A. (2019). Antifungal properties of phosphatidylcholine oleic acid liposomes encapsulating garlic against environmental fungal in wheat bread. Int. J. Food Microbiol. 293, 72–78. doi: 10.1016/j.ijfoodmicro.2019.01.006
Plaper, A., Golob, M., Hafner, I., Oblak, M., Solmajer, T., and Jerala, R. (2003). Characterization of quercetin binding site on DNA gyrase. Biochem. Biophys. Res. Commun. 306, 530–536. doi: 10.1016/S0006-291X(03)01006-4
Plumed-Ferrer, C., Väkeväinen, K., Komulainen, H., Rautiainen, M., Smeds, A., Raitanen, J. E., et al. (2013). The antimicrobial effects of wood-associated polyphenols on food pathogens and spoilage organisms. Int. J. Food Microbiol. 164, 99–107. doi: 10.1016/j.ijfoodmicro.2013.04.001
Radha Krishnan, K., Babuskin, S., Azhagu, P., Babu, S., Abbas Fayidh, M., Sabina, K., et al. (2014). Bio protection and preservation of raw beef meat using pungent aromatic plant substances. J. Sci. Food Agric. 94, 2456–2463. doi: 10.1002/jsfa.6580
Rahnemoon, P., Sarabi-Jama, M., Bostan, A., and Mansouri, E. (2021). Nano-encapsulation of pomegranate (Punica granatum L.) peel extract and evaluation of its antimicrobial properties on coated chicken meat. Food Biosci. 43:101331. doi: 10.1016/j.fbio.2021.101331
Ranucci, D., Roila, R., Andoni, E., Braconi, P., and Branciari, R. (2019). Punica granatum and Citrus spp. extract mix affects spoilage microorganisms growth rate in vacuum-packaged cooked sausages made from pork meat, emmer wheat (Triticum dicoccum Schübler), almond (Prunus dulcis Mill.) and hazelnut (Corylus avellana L.). Foods 8:664. doi: 10.3390/foods8120664
Regulation (EC) no 450/2009 (2009). Active and intelligent materials and articles intended to come into contact with food.
Regulation (EU) no 528/2012 (2012). Regulation of the European Parliament and of the Council of 22 May 2012 concerning the making available on the market and use of biocidal products.
Rempe, C. S., Burris, K. P., Lenaghan, S. C., and Stewart, C. N. Jr. (2017). The potential of systems biology to discover antibacterial mechanisms of plant phenolics. Front. Microbiol. 8:422. doi: 10.3389/fmicb.2017.00422
Reverón, I., Plaza-Vinuesa, L., Santamaría, L., Oliveros, J. C., de Las Rivas, B., Muñoz, R., et al. (2020). Transcriptomic evidence of molecular mechanisms underlying the response of Lactobacillus plantarum WCFS1 to hydroxytyrosol. Antioxidants 9:442. doi: 10.3390/antiox9050442
Roila, R., Valiani, A., Ranucci, D., Ortenzi, R., Servili, M., Veneziani, G., et al. (2019). Antimicrobial efficacy of a polyphenolic extract from olive oil by-product against “Fior di latte” cheese spoilage bacteria. Int. J. Food Microbiol. 295, 49–53. doi: 10.1016/j.ijfoodmicro.2019.02.013
Rubab, M., Chelliah, R., Saravanakumar, K., Kim, J.-R., Yoo, D., Wang, M.-H., et al. (2020). Phytochemical characterization, and antioxidant and antimicrobial activities of white cabbage extract on the quality and shelf life of raw beef during refrigerated storage. RSC Adv. 10, 41430–41442. doi: 10.1039/D0RA06727J
Sabaghi, M., Hoseyni, S. Z., Tavasoli, S., Mozafari, M. R., and Katouzian, I. (2021). Strategies of confining green tea catechin compounds in nano-biopolymeric matrices: a review. Colloids Surf. B 204:111781. doi: 10.1016/j.colsurfb.2021.111781
Sadgrove, N. J., Oliveira, T. B., Khumalo, G. P., van Vuuren, S. F., and van Wyk, B. E. (2020). Antimicrobial isoflavones and derivatives from Erythrina (Fabaceae): structure activity perspective (SAR & QSAR) on experimental and mined values against Staphylococcus aureus. Antibiotics 9:223. doi: 10.3390/antibiotics9050223
Saini, A., Panesar, P. S., and Bera, M. B. (2019). Valorization of fruits and vegetables waste through green extraction of bioactive compounds and their nanoemulsions-based delivery system. Bioresour. Bioprocess. 6:26. doi: 10.1186/s40643-019-0261-9
Samtiya, M., Aluko, R. E., Dhewa, T., and Moreno-Rojas, J. M. (2021). Potential health benefits of plant food-derived bioactive components: an overview. Foods 10:839. doi: 10.3390/foods10040839
Scalbert, A. (1991). Antimicrobial properties of tannins. Phytochemistry 30, 3875–3883. doi: 10.1016/0031-9422(91)83426-L
Serra, A. T., Matias, A. A., Nunes, A. V., Leitao, M. C., Brito, D., Bronze, R., et al. (2008). In vitro evaluation of olive- and grape-based natural extracts as potential preservatives for food. Innov. Food Sci. Emerg. Technol. 9, 311–319. doi: 10.1016/j.ifset.2007.07.011
Shi, Y., Zhu, Y., Shao, S., Zhang, R., Wu, Y., Zhu, C., et al. (2018). Alkyl ferulate esters as multifunctional food additives: antibacterial activity and mode of action against Escherichia coli in vitro. J. Agric. Food Chem. 66, 12088–12101. doi: 10.1021/acs.jafc.8b04429
Shlar, I., Droby, S., and Rodov, V. (2018). Antimicrobial coatings on polyethylene terephthalate based on curcumin/cyclodextrin complex embedded in a multilayerpolyelectrolyte architecture. Colloids Surf. B 164, 379–387. doi: 10.1016/j.colsurfb.2018.02.008
Siddiqui, M. W., Sharangi, A. B., Singh, J. P., Thakur, P. K., Ayala-Zavala, J. F., Singh, A., et al. (2016). Antimicrobial properties of teas and their extracts in vitro. Crit. Rev. Food Sci. Nutr. 56, 1428–1439. doi: 10.1080/10408398.2013.769932
Silva, A., Silva, V., Igrejas, G., Gaivão, I., Aires, A., Klibi, N., et al. (2021). Valorization of winemaking by-products as a novel source of antibacterial properties: new strategies to fight antibiotic resistance. Molecules 26:2331. doi: 10.3390/molecules26082331
Sima, F., Stratakos, A. C., Ward, P., Linton, M., Kelly, C., Pinkerton, L., et al. (2018). A novel natural antimicrobial can reduce the in vitro and in vivo pathogenicity of T6SS positive Campylobacter jejuni and Campylobacter coli chicken isolates. Front. Microbiol. 9:2139. doi: 10.3389/fmicb.2018.02139
Sireswar, S., Dey, G., Sreesoundarya, T. K., and Sarkar, D. (2017). Design of probiotic-fortified food matrices influence their antipathogenic potential. Food Biosci. 20, 28–35. doi: 10.1016/j.fbio.2017.08.002
Sivaranjani, M., Gowrishankar, S., Kamaladevi, A., Pandian, S. K., Balamurugan, K., and Ravi, A. V. (2016). Morin inhibits biofilm production and reduces the virulence of Listeria monocytogenes - an in vitro and in vivo approach. Int. J. Food Microbiol. 237, 73–82. doi: 10.1016/j.ijfoodmicro.2016.08.021
Skroza, D., Šimat, V., Smole Možina, S., Katalinić, V., Boban, N., and Generalić Mekinic, I. (2019). Interactions of resveratrol with other phenolics and activity against food-borne pathogens. Food Sci. Nutr. 7, 2312–2318. doi: 10.1002/fsn3.1073
Slobodníková, L., Fialová, S., Rendeková, K., Kovác, J., and Mucaji, P. (2016). Antibiofilm activity of plant polyphenols. Molecules 21:1717. doi: 10.3390/molecules21121717
Spizzirri, U. G., Aiello, F., Carullo, G., Facente, A., and Restuccia, D. (2021). Nanotechnologies: an innovative tool to release natural extracts with antimicrobial properties. Pharmaceutics 13:230. doi: 10.3390/pharmaceutics13020230
Stagkos-Georgiadis, A., Masoura, M., Hatzikamari, M., Mourtzinos, I., and Gkatzionis, K. (2021). Synergistic antifungal activity and substitution of sorbate with cyclodextrin-based aqueous extracts of propolis bioactives. J. Food Process. Preserv. 45:e15145. doi: 10.1111/jfpp.15145
Stapleton, P. D., Shah, S., Hamilton-Miller, J. M. T., Hara, Y., Nagaoka, Y., Kumagai, A., et al. (2004). Anti-Staphylococcus aureus activity and oxacillin resistance modulating capacity of 3-O-acylcatechins. Int. J. Antimicrob. Agents 24, 374–380. doi: 10.1016/j.ijantimicag.2004.03.024
Stojkovic, D., Petrovic, J., Sokovic, M., Glamoclija, J., Kukic-Markovic, J., and Petrovic, S. (2013). In situ antioxidant and antimicrobial activities of naturally occurring caffeic acid, p-coumaric acid and rutin, using food systems. J. Sci. Food Agric. 93, 3205–3208. doi: 10.1002/jsfa.6156
Sungpund, C., Panpipat, W., Chaijan, M., and Sae Yoon, A. (2020). Techno-biofunctionality of mangostin extract-loaded virgin coconut oil nanoemulsion and nanoemulgel. PLoS One 15:e0227979. doi: 10.1371/journal.pone.0227979
Surendhiran, D., Li, C., Cui, H., and Lin, L. (2020). Fabrication of high stability active nanofibers encapsulated with pomegranate peel extract using chitosan/PEO for meat preservation. Food Packag. Shelf Life 23:100439. doi: 10.1016/j.fpsl.2019.100439
Swer, T. L., Chauhan, K., Mukhim, C., Bashir, K., and Kumar, A. (2019). Application of anthocyanins extracted from Sohiong (Prunus nepalensis L.) in food processing. LWT Food Sci. Technol. 114:108360. doi: 10.1016/j.lwt.2019.108360
Szabo, K., Dulf, F. V., Diaconeasa, Z., and Vodnar, D. C. (2019). Antimicrobial and antioxidant properties of tomato processing byproducts and their correlation with the biochemical composition. LWT Food Sci. Technol. 116:108558. doi: 10.1016/j.lwt.2019.108558
Taguri, T., Tanaka, T., and Kouno, I. (2006). Antibacterial spectrum of plant polyphenols and extracts depending upon hydroxyphenyl structure. Biol. Pharm. Bull. 29:2226. doi: 10.1248/bpb.29.2226
Takó, M., Kerekes, E. B., Zambrano, C., Kotogán, A., Papp, T., Krisch, J., et al. (2020). Plant phenolics and phenolic-enriched extracts as antimicrobial agents against food-contaminating microorganisms. Antioxidants 9:165. doi: 10.3390/antiox9020165
Tamfu, A. N., Ceylan, O., Kucukaydin, S., and Duru, M. E. (2020). HPLC-DAD phenolic profiles, antibiofilm, anti-quorum sensing and enzyme inhibitory potentials of Camellia sinensis (L.) O. Kuntze and Curcuma longa L. LWT Food Sci. Technol. 133:110150. doi: 10.1016/j.lwt.2020.110150
Taveira, M., Silva, L. R., Vale-Silva, L. A., Pinto, E., Valentaeo, P., and Ferreres, F. (2010). Lycopersicon esculentum seeds: an industrial byproduct as an antimicrobial agent. J. Agric. Food Chem. 58, 9529–9536. doi: 10.1021/jf102215g
Tocmo, R., Pena-Fronteras, J., Calumba, K. F., Mendoza, M., and Johnson, J. J. (2020). Valorization of pomelo (Citrus grandis Osbeck) peel: a review of current utilization, phytochemistry, bioactivities, and mechanisms of action. Compr. Rev. Food Sci. Food Saf. 19, 1969–2012. doi: 10.1111/1541-4337.12561
Trujillo-Mayol, I., Casas-Forero, N., Pastene-Navarrete, E., Lima Silva, F., and Alarcón-Enos, J. (2021). Fractionation and hydrolyzation of avocado peel extract: improvement of antibacterial activity. Antibiotics 10:23. doi: 10.3390/antibiotics10010023
Ugurlu, A., Karahasan Yagci, A., Ulusoy, S., Aksu, B., and Bosgelmez-Tinaz, G. (2016). Phenolic compounds affect production of pyocyanin, swarming motility and biofilm formation of Pseudomonas aeruginosa. Asian Pac. J. Trop. Biomed. 6, 698–701. doi: 10.1016/j.apjtb.2016.06.008
Ullah, F., Ayaz, M., Sadiq, A., Ullah, F., Hussain, I., Shahid, M., et al. (2020). Potential role of plant extracts and phytochemicals against foodborne pathogens. Appl. Sci. 10:4597. doi: 10.3390/app10134597
Vadekeetil, A., Kaur, G., Chhibber, S., and Harjai, K. (2015). Applications of thin-layer chromatography in extraction and characterisation of ajoene from garlic bulbs. Nat. Prod. Res. 29:768. doi: 10.1080/14786419.2014.981815
Vasavi, H. S., Arun, A. B., and Rekha, P. D. (2015). Anti-quorum sensing potential of Adenanthera pavonina. Pharm. Res. 7, 105–109. doi: 10.4103/0974-8490.147220
Vazquez-Armenta, F. J., Hernandez-Oñate, M. A., Martinez-Tellez, M. A., Lopez-Zavala, A. A., Gonzalez-Aguilar, G. A., Gutierrez-Pacheco, M. M., et al. (2020). Quercetin repressed the stress response factor (sigB) and virulence genes (prfA, actA, inlA, and inlC), lower the adhesion, and biofilm development of L. monocytogenes. Food Microbiol. 87:103377. doi: 10.1016/j.fm.2019.103377
Velazquez-Martinez, V., Valles-Rosales, D., Rodriguez-Uribe, L., Holguin, O., Quintero-Quiroz, J., Reyes-Jaquez, D., et al. (2021). Antimicrobial, shelf-life stability, and effect of maltodextrin and gum arabic on the encapsulation efficiency of sugarcane bagasse bioactive compounds. Foods 10:116. doi: 10.3390/foods10010116
Vidallon, M. L. P., and Teo, B. M. (2020). Recent developments in biomolecule-based nanoencapsulation systems for antimicrobial delivery and biofilm disruption. Chem. Commun. 56, 13907–13917. doi: 10.1039/D0CC05880G
Wen, A., Delaquis, P., Stanich, K., and Toivonen, P. (2003). Antilisterial activity of selected phenolic acids. Food Microbiol. 20, 305–311. doi: 10.1016/S0740-0020(02)00135-1
Wu, Y. P., Bai, J. R., Zhong, K., Huang, Y. N., Qi, H., Jiang, Y., et al. (2016). Antibacterial activity and membrane-disruptive mechanism of 3-p-trans-coumaroyl-2-hydroxyquinic acid, a novel phenolic compound from pine needles of Cedrus deodara, against Staphylococcus aureus. Molecules 21:1084. doi: 10.3390/molecules21081084
Wu, Y., Liang, S., Zhang, M., Wang, Z., Wang, Z., and Ren, X. (2020). The effect of chlorogenic acid on Bacillus subtilis based on metabolomics. Molecules 25:4038. doi: 10.3390/molecules25184038
Wu, Y. P., Liu, X. Y., Bai, J. R., Xie, H. C., Ye, S. L., Zhong, K., et al. (2019). Inhibitory effect of a natural phenolic compound, 3-p-trans-coumaroyl-2-hydroxyquinic acid against the attachment phase of biofilm formation of Staphylococcus aureus through targeting sortase A. RSC Adv. 9, 32453–32461. doi: 10.1039/C9RA05883D
Wu, V. C. H., Qiu, X., de los Reyes, B. G., Lin, C. S., and Pan, Y. (2009). Application of cranberry concentrate (Vaccinium macrocarpon) to control Escherichia coli O157:H7 in ground beef and its antimicrobial mechanism related to the downregulated slp, hdeA and cfa. Food Microbiol. 26, 32–38. doi: 10.1016/j.fm.2008.07.014
Xu, X., Ou, Z. M., and Wu, C. D. (2018). Growth media affect assessment of antimicrobial activity of plant-derived polyphenols. Biomed. Res. Int. 2018:8308640. doi: 10.1155/2018/8308640
Yang, R., Guan, Y., Zhou, J., Sun, B., Wang, Z., Chen, H., et al. (2018). Phytochemicals from Camellia nitidissima chi flowers reduce the pyocyanin production and motility of Pseudomonas aeruginosa PAO1. Front. Microbiol. 8:2640. doi: 10.3389/fmicb.2017.02640
Yong, H., Liu, Y., Yun, D., Zong, S., Jin, C., and Liu, J. (2021). Chitosan films functionalized with different hydroxycinnamic acids: preparation, characterization and application for pork preservation. Foods 10:536. doi: 10.3390/foods10030536
Yu, L., and Shi, H. (2021). Effect of two mulberry (Morus alba L.) leaf polyphenols on improving the quality of fresh-cut cantaloupe during storage. Food Control 121:107624. doi: 10.1016/j.foodcont.2020.107624
Zam, W., and Ali, A. (2018). Evaluation of mechanical, antioxidant and antimicrobial properties of edible film containing myrtle berries extract. Nat. Prod. J. 8, 323–330. doi: 10.2174/2210315508666180723155711
Zambrano, C., Kerekesa, E. B., Kotogán, A., Papp, T., Vágvölgyi, C., Krisch, J., et al. (2019). Antimicrobial activity of grape, apple and pitahaya residue extracts after carbohydrase treatment against food-related bacteria. LWT Food Sci. Technol. 100, 416–425. doi: 10.1016/j.lwt.2018.10.044
Zamuz, S., Muneka, P. E. S., Dzuvor, C. K. O., Zhang, W. G., Sant’ana, A. S., and Lorenzo, J. M. (2021). The role of phenolic compounds against Listeria monocytogenes in food. A review. Trends Food Sci. Technol. 110, 385–392. doi: 10.1016/j.tifs.2021.01.068
Zhang, J., Lin, Y., Huang, L., Tekliye, M., Rasheed, H. A., and Dong, M. (2020a). Composition, antioxidant, and anti-biofilm activity of anthocyanin-rich aqueous extract from purple highland barley bran. LWT Food Sci. Technol. 125:109181. doi: 10.1016/j.lwt.2020.109181
Zhang, L., McClements, D. J., Wei, Z., Wang, G., Liu, X., and Liu, F. (2020b). Delivery of synergistic polyphenol combinations using biopolymer-based systems: advances in physicochemical properties, stability and bioavailability. Crit. Rev. Food Sci. Nutr. 60, 2083–2097. doi: 10.1080/10408398.2019.1630358
Zhang, J., Rui, X., Wang, L., Guan, Y., Sun, X., and Dong, M. (2014). Polyphenolic extract from Rosa rugosa tea inhibits bacterial quorum sensing and biofilm formation. Food Control 42, 125–131. doi: 10.1016/j.foodcont.2014.02.001
Zhao, Y., Kong, H., Zhang, X., Hu, X., and Wang, M. (2019). The effect of Perilla (Perilla frutescens) leaf extracts on the quality of surimi fish balls. Food Sci. Nutr. 7, 2083–2090. doi: 10.1002/fsn3.1049
Zhao, M., Wang, H., Yang, B., and Tao, H. (2010). Identification of cyclodextrin inclusion complex of chlorogenic acid and its antimicrobial activity. Food Chem. 120, 1138–1142. doi: 10.1016/j.foodchem.2009.11.044
Zhou, T. T., Wei, C. H., Lan, W. Q., Zhao, Y., Pan, Y. J., Sun, X. H., et al. (2020). The effect of Chinese wild blueberry fractions on the growth and membrane integrity of various foodborne pathogens. J. Food Sci. 85, 1513–1522. doi: 10.1111/1750-3841.15077
Keywords: phenolic-rich plant extracts, antimicrobial activity, food preservation, biocides, biofilms, delivery systems
Citation: Oulahal N and Degraeve P (2022) Phenolic-Rich Plant Extracts With Antimicrobial Activity: An Alternative to Food Preservatives and Biocides? Front. Microbiol. 12:753518. doi: 10.3389/fmicb.2021.753518
Edited by:
Lizziane Kretli Winkelströter, University of Western São Paulo, BrazilReviewed by:
Xiaodong Xia, Dalian Polytechnic University, ChinaDeepansh Sharma, Amity University Jaipur, India
Copyright © 2022 Oulahal and Degraeve. This is an open-access article distributed under the terms of the Creative Commons Attribution License (CC BY). The use, distribution or reproduction in other forums is permitted, provided the original author(s) and the copyright owner(s) are credited and that the original publication in this journal is cited, in accordance with accepted academic practice. No use, distribution or reproduction is permitted which does not comply with these terms.
*Correspondence: Nadia Oulahal, nadia.oulahal@univ-lyon1.fr