- 1Department of Biochemistry, Faculty of Mathematics and Natural Sciences, Bogor Agricultural University, Bogor, Indonesia
- 2Laboratory of Cell Biochemistry, Department of Biochemistry and Molecular Biology, Faculty of Medicine, University of Debrecen, Debrecen, Hungary
- 3Universitas Muhammadiyah Bangka Belitung, Pangkalpinang, Indonesia
- 4Department of Medical Chemistry, Faculty of Medicine, University of Debrecen, Debrecen, Hungary
Post-transcriptional RNA modifications have recently emerged as critical regulators of gene expression programs. Understanding normal tissue development and disease susceptibility requires knowledge of the various cellular mechanisms which control gene expression in multicellular organisms. Research into how different RNA modifications such as in N6-methyladenosine (m6A), inosine (I), 5-methylcytosine (m5C), pseudouridine (Ψ), 5-hydroxymethylcytosine (hm5C), N1-methyladenosine (m1A), N6,2′-O-dimethyladenosine (m6Am), 2′-O-methylation (Nm), N7-methylguanosine (m7G) etc. affect the expression of genes could be valuable. This review highlights the current understanding of RNA modification, methods used to study RNA modification, types of RNA modification, and molecular mechanisms underlying RNA modification. The role of RNA modification in modulating gene expression in both physiological and diseased states is discussed. The potential applications of RNA modification in therapeutic development are elucidated.
1 Introduction
Gene expression ─ a cellular process by which the information encoded in a gene is converted into a functional gene product ─ is tightly controlled at multiple layers to ensure production of appropriate level of each gene product, such as a protein (Lackner et al., 2007). The vast majority (up to 90%) of eukaryotic genomes is pervasively transcribed (Kaikkonen et al., 2011). It is interesting to note, however, that only about 1.5% of the human genome represents protein-coding genes which are transcribed into messenger RNA (mRNA), while the rest, about 98.5%, consists of non-protein-coding DNA sequences, which are transcribed into non-coding RNA (ncRNA) molecules. Compared to that of mRNAs, the transcription levels of most of ncRNAs are significantly lower indicating that ncRNAs primarily serve regulatory functions (Kaikkonen et al., 2011; Richard Boland, 2017). The ncRNAs can be further classified into infrastructural ncRNAs and regulatory ncRNAs. The infrastructural ncRNAs include ribosomal RNA (rRNA), transfer RNA (tRNA), small nuclear RNA (snRNA), and small nucleolar RNA (snoRNA). The regulatory ncRNAs consist of microRNAs (miRNAs), Piwi-interacting RNAs (piRNAs), small interfering RNAs (siRNAs), and long non-coding RNAs (lncRNAs) (Kaikkonen et al., 2011). Circular RNA (circRNA) is a novel type of ncRNA ubiquitously expressed in eukaryotic cells during posttranscriptional processes. This type of RNA forms covalent-closed continuous loops without 5′ to 3′ polarities and poly (A) tails. With the aid of high-throughput sequencing methods, numerous circRNAs have been discovered in humans, animals, and plants (Wang et al., 2017). Other classes of RNA molecule, promoter-associated RNAs (PARs) and enhancer RNAs (eRNAs), have been identified through high-throughput sequencing of RNA molecules (Kaikkonen et al., 2011).
Both coding and ncRNA can undergo biochemical modification co- or post-transcriptionally which diversify RNA molecules and affect their cellular function. Apart from the well-known 5′ capping and 3′ polyadenylation, numerous internal nucleoside modifications also occur in RNA transcripts which exhibit a profound impact on their biochemical characteristics (Roundtree et al., 2017; McCown et al., 2020). When post-transcriptional RNA modification changes the nucleotide sequence in the coding region of a primary transcript which may change the amino acid sequence of the encoded protein, the alteration is classed as RNA editing. Thus, RNA editing is part of RNA modification and can be defined as posttranscriptional alterations of RNA molecules through insertion, deletion, or modification of nucleotides (except RNA processing events such as splicing, capping, or polyadenylation) which bring about differences between the actual genomic sequence and the corresponding RNA sequence (Xu and Zhang, 2014). RNA editing includes base modifications such as deamination of adenosine (A) to inosine (I) and deamination of cytidine (C) to uridine (U). These base alterations are catalyzed by deaminases which act as editors. The A-to-I conversion is the most prevalent type of RNA editing in animal cells. In humans, more than 4.6 million A-to-I modification sites have been identified (Christofi and Zaravinos, 2019; Lo Giudice et al., 2020). The majority of RNA editing sites are located in non-coding regions and only a small proportion occurs in the coding sequences of RNA, thus altering the amino acid sequence and the function of their encoded proteins (Zinshteyn and Nishikura, 2009). RNA editing has been linked to various human diseases such as autoimmune and inflammatory pathologies, neurodegenerative and psychiatric disorders, and cancer (Lo Giudice et al., 2020).
RNA modifications have been found to take place in all living cells (Zhao et al., 2017) as well as in both DNA and RNA viruses (Baquero-Perez et al., 2021). Notably, tRNAs have been found to be the most heavily modified, each of which has on average 13 modifications. Similarly, rRNAs are also frequently modified although, to a lesser extent than tRNAs (Arzumanian et al., 2022). Chemical modifications found in human rRNA include 2′-O-methylation, pseudouridines (Ψs), and base methylations. The biogenesis of rRNA is prevented in the absence of internal Ψs and 2′-O-methylated sugars, indicating the crucial roles of rRNA chemical modifications (Roundtree et al., 2017). Currently, more than 170 different types of posttranscriptional RNA modification have been identified (Wiener and Schwartz, 2021; Cappannini et al., 2024; Xuan et al., 2024). Of these, the N6-methyladenosine (m6A), I, 5-methylcytosine (m5C), Ψ, 5-hydroxymethylcytosine (hm5C), N1-methyladenosine (m1A), N6,2′-O-dimethyladenosine (m6Am), 2′-O-methylation (Nm), and N7-methylguanosine (m7G) (Figure 1) are among the most common RNA modifications (Song and Yi, 2017; Roundtree et al., 2017; Cai et al., 2023). The m6A RNA methylation is the most prevalent RNA modification (He and He, 2021). The collection of RNA modifications presents in a living organism or a virus is termed epitranscriptome, and the field that studies RNA modifications is referred to as epitranscriptomics (Song and Yi, 2017; Xiong et al., 2017). This newly emerged field is progressing rapidly along with the advancement of both experimental and computational methods for deciphering RNA modifications (Primac et al., 2022). Mutations in genes encoding enzymes for RNA modifications have been linked to different types of human diseases (Jonkhout et al., 2017).
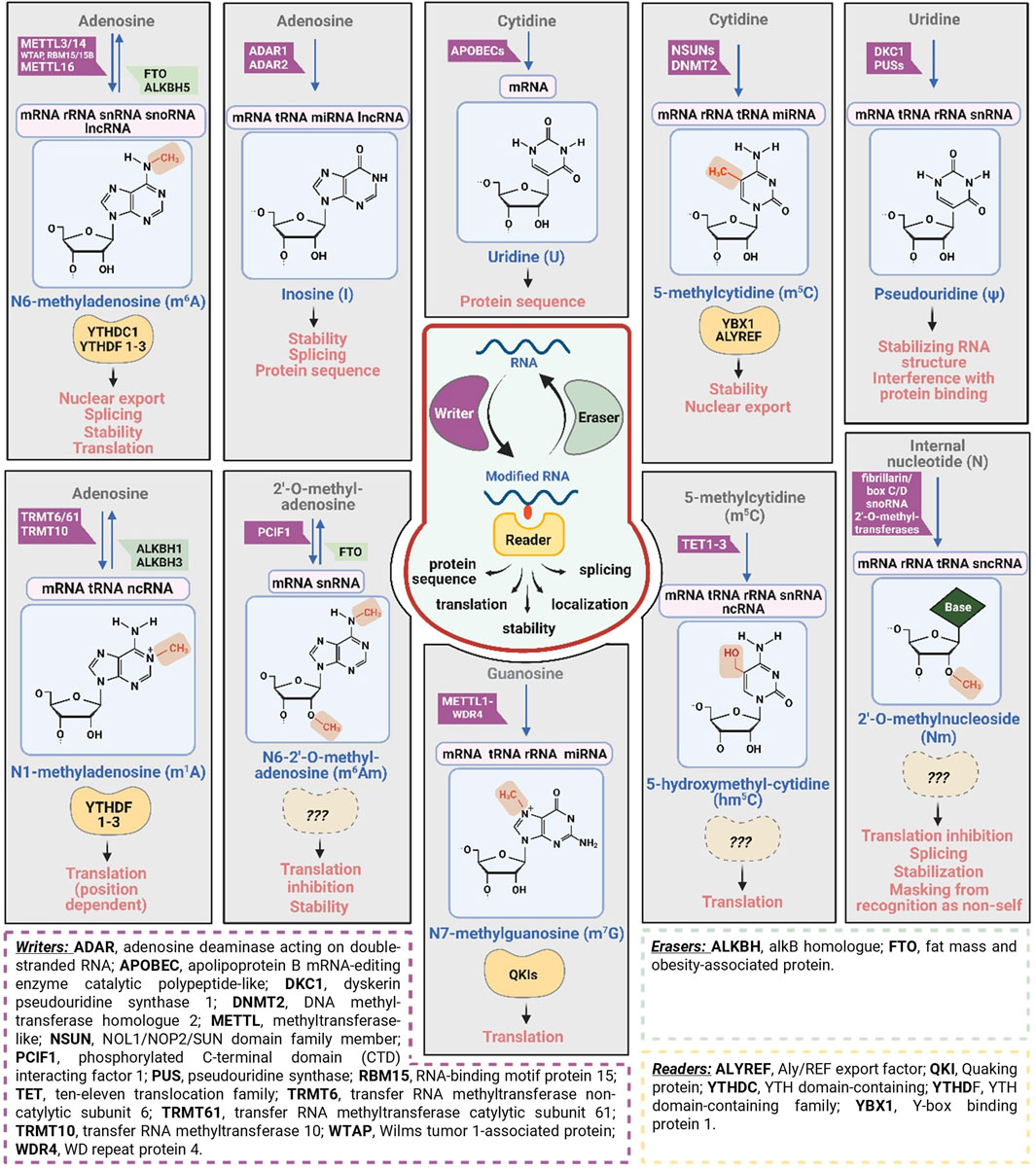
Figure 1. The RNA modifications discussed in this article. The main writer (purple boxes) and eraser (green boxes) enzymes and the known reader proteins (yellow shapes) are shown together with the modifications’ recognized effect and their distribution in different RNA types. Created in BioRender. Demeny (2025) https://BioRender.com/n91u871.
Several databases related to RNA modifications have been developed (Table 1). These include MODOMICs (Cappannini et al., 2024), RMBase (Xuan et al., 2024), RMDisease V2.0 (Song B. et al., 2023), and RNAMDB (Cantara et al., 2011). MODOMICS is a comprehensive database on the chemical structures of modified RNA nucleosides, their biosynthetic pathways, the position of modified residues in RNA sequences, and enzymes responsible for RNA modifications (Cappannini et al., 2024). RMBase provides various resources and tools useful for studying RNA modifications. This database enables integrated analysis of diverse RNA modification profiles and makes possible exploration of transcriptome-wide landscape, biogenesis, molecular interactions, and functions of RNA modifications (Xuan et al., 2024). RMDisease V2.0 is an updated database of genetic variants which affect RNA modifications with disease implications. This database is intended to unmask the link between disease-related genetic variants and their epitranscriptome alterations (Song B. et al., 2023). The RNAMDB database provides information on different aspects of naturally occurring RNA modifications such as chemical structure, common name and symbol, elemental composition, and mass (Cantara et al., 2011). In addition, a comprehensive database of RNA modifying enzymes has also been developed. This database, called RNAME, lists more than 21,000 RNA modification enzymes from 456 species and is aimed to facilitate studies on RNA modifications (Nie et al., 2022). A knowledgebase for m6A epitranscriptome, m6A-Atlas v2.0, has also been created (Liang et al., 2024). Considering the critical roles of RNA modification throughout development, and the current intense research on RNA modification, this review highlights the recent studies and progress related to dynamics of RNA modification. Current knowledge of RNA modification and their important roles on regulation of gene expression in both physiological and diseased states are addressed.
2 Methods to study RNA modification
Improved methodologies have stimulated research and led to better understanding of RNA modification. Different techniques with diverse strategies have been employed to detect, map, quantify, analyze, and illuminate cellular function of RNA modifications (McMahon, 2021). These include microarray (Hiley et al., 2005), restriction fragment length polymorphism (RFLP) (Wulff et al., 2017), mass spectrometry (You and Yuan, 2021; Zhang et al., 2021), nuclear magnetic resonance (NMR) (Gato et al., 2021), polymerase chain reaction (PCR) (Elliott and Holley, 2021; Olazagoitia-Garmendia and Castellanos-Rubio, 2021), Northern blot (Cirzi and Tuorto, 2021), enzymatic (Czekay et al., 2021), next-generation sequencing (NGS) (Marchand et al., 2021; Cullen and Tsai, 2021), nanopore direct RNA sequencing (Liu H. et al., 2021; Jain et al., 2021), and CRISPR-Cas9 (Liu and Qian, 2021) methods. In addition, bioinformatics tools have been applied to analyze RNA modification data (Manfredonia and Incarnato, 2021; Liu Q. et al., 2021).
The microarray technique can be used to differentiate RNA molecules, both with and without modification based on the binding of the RNA molecules to the probes on the array. Using this method, Hiley and coworkers could detect at least five different RNA modifications (Hiley et al., 2005). A protocol based on differential enzymatic digestions coupled with liquid chromatography-tandem mass spectrometry (LC-ESI-MS/MS) has been developed and applied to identify internal m7G in mRNA of different types of human cell. This protocol is also applicable for detection and quantification of m7G at the 5′ cap of mRNA (You and Yuan, 2021). A method based on general LC-MS has also been developed for direct and de novo sequencing of purified RNAs, containing both canonical and modified nucleotides such as Ψ and m5C (Zhang et al., 2021). Furthermore, in order to study tRNA maturation, a method using NMR has been developed to directly monitor the introduction of biochemical modifications in the process of tRNA maturation (Gato et al., 2021).
Detecting 2′-O-methylation (Nm) on specific mRNA transcripts is technically challenging because mRNAs are much less abundant compared with rRNA. A strategy based on quantitative PCR in conjunction with reverse transcription at a low level of dNTPs has been developed and was demonstrated to be sensitive to detect changes to Nm modification of mRNA (Elliott and Holley, 2021). Similarly, site-specific detection and quantification of m6A is technically difficult. A simple reverse transcription-qPCR-based assay has been developed which can be implemented for the relative quantification of candidate m6A regions. This strategy takes advantage of the reduced capacity of BstI enzyme to retrotranscribe m6A residues (Olazagoitia-Garmendia and Castellanos-Rubio, 2021). A reverse transcription polymerase chain reaction (RT-PCR) and gel electrophoresis-based method has been developed to detect and quantify Ψ RNA modification. This simple technique was found to be helpful in validating Ψ sites identified by high throughput sequencing, quantifying Ψ levels in mRNA and lncRNA, and effectively elucidate the mechanisms and function of the Ψ modification (Zhang and Pan, 2022).
A protocol using N-acryloyl-3-aminophenylboronic acid (APB) during Northern blot has been developed for fast and reliable detection of queuosine (Q) tRNA modification. This assay allows separation of Q-modified tRNA from unmodified tRNA and quantification can be carried out using Northern blot analysis (Cirzi and Tuorto, 2021). The Northern blot technique has also been applied for detection of RNA modifications by using antibodies against modified nucleosides. The development of this immuno-Northern blotting approach was intended to facilitate studies on RNA modifications and metabolism (Mishima et al., 2015).
Besides the classical approaches, NGS-based methods have been applied to study RNA modifications. A method called AlkAniline-Seq was developed and found to be fast and efficient for simultaneously mapping two different RNA modifications, the m7G and m3C (Marchand et al., 2021). The high-throughput NGS can also be employed to identify antibody-bound modified transcripts. Based on this principle, a method termed “photoactivatable ribonucleoside-enhanced cross-linking and immunoprecipitation” (PAR-CLIP) has been developed for mapping various RNA modifications for which specific antibodies against the RNA modifications are available (Cullen and Tsai, 2021). The advent of NGS technologies has accelerated research on RNA editing. Recently, a computational method for profiling the editome (the entire RNA editing in a genome) from single-cell RNA sequencing (scRNA-seq) data has been developed. This tool is useful for detecting RNA editing events in functionally heterogeneous cell populations (Wu et al., 2023a).
Due to its abundance and critical cellular roles of m6A RNA modification, a method which enables identification of m6A sites in the whole transcriptome of single cells is required to study m6A contribution to normal cellular function and disease pathogenesis. A method called deamination adjacent to RNA modification targets sequencing (DART-seq) was found to be applicable for transcriptome-wide profiling of m6A sites to reveal different m6A signatures and mRNA methylation heterogeneity in single cells (Tegowski et al., 2022). In addition, to improve resolution and allow quantitative detection of m6A, a method named “evolved TadA-assisted N6-methyladenosine sequencing” (eTAM-seq) has been created. It is an enzyme-assisted sequencing platform which detects and quantifies m6A by global adenosine deamination (Xiao et al., 2023).
The high-throughput sequencing techniques either based on antibodies, enzymes, or novel chemistry have been employed to study m6A and Ψ RNA modification (Zhang et al., 2023a). Recently, a chemical assisted-method called “glyoxal and nitrite-mediated deamination of unmethylated adenosines” (GLORI) has been developed and used for absolute quantification of single-base m6A methylation in the mammalian transcriptome (Liu C. et al., 2023). Similarly, a sensitive and convenient chemical assisted-method termed PRAISE was developed to measure transcriptome-wide Ψ (Zhang et al., 2023b). Methods for the precise mapping of individual RNA modifications throughout the transcriptome are critical in studying roles of a specific transcriptome. A method called hydrazine-aniline cleavage sequencing (HAC-seq) has been developed and applied to specifically map m3C throughout a transcriptome. This novel method can be used to reveal the m3C methylome in various cells and tissues (Cui et al., 2021). Recently, a specific and sensitive technique called “m6Am-seq” has been introduced to investigate the prevalence, topology, and dynamics of m6Am in the human transcriptome. This technique is based on a selective demethylation reaction to achieve specific and sensitive detection of m6Am (Sun et al., 2021).
The advancement of the nanopore direct RNA sequencing (dRNA-seq) technique has further improved the methodology for identification of posttranscriptional RNA modification. This technique enables direct sequencing of full-length native RNA molecules without the need of a reverse-transcription or amplification step and can provide a comprehensive picture of individual RNA molecules as their existence in cells. More importantly, this emerging method allows detection of different nucleotide modifications present in the native RNA molecules on single-read level data using a portable device (Leger et al., 2021; Zhao et al., 2022; Jain et al., 2022). An algorithm, called Epi Nano has been developed to detect RNA base modifications, such as m6A, from data generated using nanopore dRNA-seq (Liu H. et al., 2021). A protocol for sequencing canonical and modified nucleotides of human rRNA using nanopore dRNA-seq has been established (Jain et al., 2021). Recently, a study comparing the use of dRNA-seq and methylated RNA immunoprecipitation and sequencing (MeRIP-seq) in detecting m6A modification in ncRNAs of glioblastoma suggested that MeRIP-seq is preferable for a preliminary m6A screening study, as it exhibits a higher lncRNA coverage, while the dRNA-seq is more useful for in depth analysis of m6A quantity and exact location. Of note, MeRIP-seq is the most common method for m6A detection (Krusnauskas et al., 2023).
Analysis of data generated from high-throughput sequencing techniques has been the main bottleneck in experiments using these assays. Systematic identification of different types of RNA-modification sites remains a major challenge. More than 20 computational methods have been developed to map RNA-modification sites (Chen et al., 2020). A generalized toolkit for the analysis of NGS-based RNA posttranscriptional modification mapping experiments has been generated (Manfredonia and Incarnato, 2021). A protocol for identification and annotation of individual RNA modifications throughout the transcriptome has also been created to promote research on the roles of the epitranscriptome in the control of gene expression and other cellular processes (Liu Q. et al., 2021). The availability of large datasets of transcriptomics has led to the increase of application of machine learning approaches to identify RNA modifications (El Allali et al., 2021). Considering the pivotal roles of m6Am RNA modification, a Catboost-based model, using machine learning algorithms was developed for predicting the m6Am sites on mRNA (Liu Z. et al., 2023). Machine learning has also been used to predict genes linked to RNA methylation pathways (Tsagkogeorga et al., 2022). An effective computational method, iRNA5hmC, which is complementary to the high-throughput sequencing technologies, has been introduced for identification of RNA hm5C sites using machine learning (Liu Y. et al., 2020). A predictor named iRNA5hmC-HOC based on a high-order correlation information method has been proposed for identification of hm5C sites (Zou, 2022).
3 Types of RNA modification
RNA modifications are dynamic processes, catalyzed by a series of specific modifying enzymes or proteins, which are based on their functions can be grouped into the so-called writer, eraser, and reader categories (Figure 1). Writers are enzymes which play roles in installing chemical modifications into RNA molecules, while those functions in removing the chemical modifications are termed erasers. Proteins which recognize the chemical marks are called readers. These play a critical role in transducing signal for downstream functions (Ontiveros et al., 2019; Nie et al., 2022; Qiu et al., 2023). Currently, our detailed understanding of RNA modifying enzymes and their mechanisms of action is limited because only a small number of experimentally validated RNA modification enzymes are documented (Nie et al., 2022). The majority of internal RNA modifications occur post-transcriptionally. Notably, co-transcriptional modifications have been documented for m6A and Ψ (Gilbert and Nachtergaele, 2023). The types of chemical modification that decorate RNA molecules are diverse which include methylation, deamination, isomerization, thiolation, glycosylation, transglycosylation, attachment of amino acid, addition of sugar, etc. (Jackman and Alfonzo, 2013; Ontiveros et al., 2019). These modifications may affect folding, Watson-Crick base pairing, 3D structure, molecular flexibility, molecular interaction with other molecules, molecular stability, and biological function of the modified RNAs (Ontiveros et al., 2019; Adamopoulos et al., 2023).
3.1 N6-methyladenosine (m6A)
So far, the molecular mechanism underlying the m6A RNA modification system is the most well studied and hence well understood among hundreds known types of RNA modification (Nie et al., 2022). The m6A modifications are found on mRNA, tRNA, rRNA, snRNA, and ncRNAs such as lncRNAs, miRNAs, and circRNAs (Zhou et al., 2020; Bedi et al., 2023). It is enriched near stop codons and 3′-untranslated terminal regions (UTRs) (Zhou et al., 2020). The m6A modification results from a methylation reaction at the N6-position of adenosine in the RNA molecule catalyzed by a complex writer-protein comprised of methyltransferase-like (METTL) 3, METTL5, METTL14, METTL16 and their cofactors such as Wilms tumor 1-associated protein (WTAP), RNA-binding motif protein 15 (RBM15/15B), Cbl proto-oncogene-like 1 (CBLL1; also named HAKAI), zinc finger CCCH-type containing 13 (ZC3H13), and Vir-like m6A methyltransferase-associated (VIRMA; also termed KIAA1429) (Zhou et al., 2020). METTL3 and METTL14 form a heterodimeric complex forming the core methyltransferase that catalyzes the m6A modification. METTL3 is the catalytic subunit of the complex responsible for binding the co-substrate S-adenosyl methionine (SAM), while METTL14 functions as structural support for METTL3 and is involved in mRNA binding (Zhou et al., 2020; Jiang et al., 2021; Bedi et al., 2023). SUMOylation of METTL3 reduces its m6A methytransferase activity, hence decreasing m6A levels in mRNAs (Du et al., 2018). The precise function of METTL16 is still being explored particularly with respect to its roles in mRNA and snRNA methylation (Satterwhite and Mansfield, 2022). In addition, it has been shown to significantly affect various cellular processes (Talic et al., 2023). WTAP stabilizes the core complex and promotes METTL3-METTL14 heterodimer to the nuclear speckles. The RBM15/15B is essential in assisting binding of METTL3 and WTAP, directing the two proteins to their target sites. VIRMA directs the methyltransferase components to specific RNA regions. Other proteins, such as ZC3H13 and CBLL1, together with additional cofactors, including WTAP, regulate nuclear m6A methylation (Zhou et al., 2020; Jiang et al., 2021).
The m6A mRNA modifications are installed to nascent pre-mRNA molecules and chemical modification is finished by the release of mRNA into nucleoplasm. Moreover, quantitative m6A analysis suggested that little of the methylation reaction actually takes place in the cytoplasm (Ke et al., 2017). The m6A modification affects multiple stages of the mRNA life cycle such as splicing, nuclear export, translation, and degradation (Bedi et al., 2023) and altered m6A levels disturb gene expression and other essential cellular processes (Zhou et al., 2020). Regarding the role of m6A modification in mRNA splicing, it is important to note that a study suggested that m6A mRNA modifications are not essential for most splicing events (Ke et al., 2017). The m6A RNA modification is a reversible reaction. The m6A can be removed by RNA demethylases. At present, two RNA demethylases are known, fat mass and obesity-associated protein (FTO) and alkylation protein AlkB homolog 5 (ALKBH5) (Shen et al., 2022). The FTO was first discovered to exhibit demethylase activity to m6A in 2011 (Jia et al., 2011). The ALKBH5 was first identified in 2013 (Zheng et al., 2013). Both demethylases and methyltransferases collectively contribute to the modulation of m6A levels in eukaryotic organisms (Shen et al., 2022). Of note, the role of FTO as a demethylase for m6A and m6Am or for m6Am only, remains ambiguous (Nabeel-Shah et al., 2024).
3.2 N6,2-O-dimethyladenosine (m6Am)
Beside modification to form m6A, the adenine base of RNA molecule can undergo alteration to generate m6Am, m1A, and can be edited to inosine (A-to-I) (Adamopoulos et al., 2023; Wu et al., 2023b). The m6Am is resulted from adenosine N6-methylation of 2′-O-methyladenosine (Am) (Mauer et al., 2019). In the case that the first nucleotide after the m7G cap is adenosine, it will be methylated at the N6-position to form m6Am catalyzed by an enzyme called phosphorylated C-terminal domain (CTD) interacting factor 1 (PCIF1) (Wei et al., 1975; Akichika et al., 2019; Sendinc et al., 2019). The majority of PCIF1 is found in the nucleus, playing a role in generating the m6Am modification on new transcripts. Currently, PCIF1 is the only mammalian methyltransferase of m6Am known (Sendinc et al., 2019; Wu et al., 2023b). Similar with m6A RNA modification, the m6Am modification is also a reversible reaction. It is dynamically modulated by PCIF1 and FTO (Sun et al., 2021). The FTO RNA demethylase functions as an eraser which removes the methyl group from the N6-position (Cesaro et al., 2023).
3.3 N1-methyladenosine (m1A)
The m1A modifications have been found in tRNA, rRNA, mRNA, and mitochondrial tRNA (Jin et al., 2022; Adamopoulos et al., 2023). The m1A RNA modification is catalyzed by TRMT10 and the TRMT6/TRMT61 complex. The protein subunits of this complex are members of tRNA methyltransferase (TRMT) protein family. Similarly to the m6A RNA modification, the m1A RNA modification is reversible in nature. The m1A chemical modification can be removed by ALKBH1 and ALKBH3, the key enzymes functioning as erasers for this type of RNA modification (Adamopoulos et al., 2023). Readers for m1A include YTHDC1, YTHDF2, and YTHDF3 (Adamopoulos et al., 2023).
3.4 Adenosine-to-inosine (A-to-I)
A-to-I RNA editing is one of the most common posttranscriptional RNA modifications in metazoans and in humans (Yang Y. et al., 2021; Adamopoulos et al., 2023). This base conversion reaction is catalyzed by enzymes termed adenosine deaminases acting on RNA (ADARs). These enzymes (ADAR1 and ADAR2) are present throughout the body but are most abundant in the central nervous system (Slotkin and Nishikura, 2013). The A-to-I editing has been found in both coding and ncRNA transcripts (Yang Y. et al., 2021). As the translation machinery generally interprets inosine as guanosine, A-to-I editing within the coding sequence can cause amino acid substitution and diversify the proteome (Gabay et al., 2022). Different from other RNA modifications such as RNA methylation, the A-to-I editing process is totally regulated by ADARs without the involvement of other readers or erasers (Li et al., 2021).
3.5 5-Methylcytidine (m5C)
In addition to adenine, the cytosine of RNA molecule can also undergo posttranscription modifications. Cytosine can be modified to generate m5C and 3-methylcytosine (m3C). The cytosine base can also be edited to form uridine (C-to-U RNA editing) (Adamopoulos et al., 2023). The m5C RNA modification is catalyzed by RNA m5C methyltransferases (RCMTs), which consist of the NOL1/NOP2/SUN domain (NSUN) family of proteins and DNA methyltransferase (DNMT) homologue DNMT2 (Gao and Fang, 2021; Li M. et al., 2022). The m5C RNA modification is a reversible reaction. The removal of m5C is catalyzed by enzymes termed the “ten-eleven translocation” (TET) family proteins which oxidize m5C in RNA into cytosine-5-hydroxymethylation (hm5C) (Gao and Fang, 2021). Two proteins have been identified as readers for m5C, YBX1 and ALYREF (Gao and Fang, 2021).
3.6 5-Hydroxymethyl cytidine (hm5C)
In mammals, m5C can undergo oxidative processing generating hm5C and 5-formylcytidine (f5C) (Huber et al., 2015). The hm5C has been identified in all three domains of life, and is mainly present in mRNA (Huber et al., 2015; Huang et al., 2016). The TET enzymes which catalyze oxidative demethylation of m5C in DNA molecule forming hm5C, were also found to catalyze formation of hm5C in human cells in vitro (Fu et al., 2014). A study using Drosophila melanogaster demonstrated that hm5C is deposited by TET methyldioxygenases. Furthermore, TET and hydroxymethylated RNA were found to be the most abundant in the Drosophila brain. Of note, hm5C also occurs, and is well documented, in DNA (Delatte et al., 2016). The hm5C RNA modification was also found in mouse brain but at a lower level than for the hm5C DNA modification (Miao et al., 2016).
3.7 3-Methylcytidine (m3C)
The m3C RNA modification has been identified in both tRNA and mRNA (Xu et al., 2017; Chen et al., 2021). It was reported that in eukaryotic cells the m3C RNA modification is widely distributed at position C32 of tRNAThr and tRNASer molecules (Mao et al., 2021). The cellular formation of m3C is catalyzed by the writer enzymes RNA methyltransferases. Notably, RNA methyltransferases constitute a diverse family of enzymes that transfer a methyl group from SAM to a variety of positions in RNA. There are currently 4 METTL enzymes (METTL2A, METTL2B, METTL6, and METTL8) found in mammals (Mao et al., 2021; Lentini et al., 2022). The METTL8 is responsible for catalyzing the m3C addition in human mitochondrial tRNAs (Lentini et al., 2022). Of note, only two methyltransferases (Trm140 and Trm141) were identified in fission yeast and only one (Trm140) was present in budding yeast (Mao et al., 2021). The m3C RNA modification is reversible. There are two demethylases (erasers), ALKBH1 and ALKBH3 which have been identified in human cells. ALKBH1 removes methyl (CH3) groups in human mRNA (Ma et al., 2019) while ALKBH3 demethylates human tRNA. It should be noted that ALKBH3 is also a m1A demethylase of tRNA (Chen Z. et al., 2019).
3.8 Cytidine to uridine (C-to-U)
The C-to-U RNA editing has been found in both mammals and plants. The molecular mechanism of C-to-U RNA editing involves the hydrolytic deamination of a cytosine to a uracil base which is catalyzed by multiple cytosine deaminases, which belong to a family of mammalian enzymes known as the “activation-induced cytidine deaminase/apolipoprotein B mRNA-editing enzyme catalytic polypeptide-like” (AID/APOBEC) protein family. The first member of this family is APOBEC1 (Pecori et al., 2022). The activity of RNA-specific cytidine deaminases requires several complementation factors (Vu and Tsukahara, 2017). The C-to-U RNA editing is subject to induction by relevant environmental factors such as hypoxia (Baysal et al., 2013). This editing may alter the characteristics of the encoded proteins. For example, the C-to-U editing in the nuclear transcript encoding intestinal apolipoprotein B (apoB) resulted in a truncated apoB protein. This editing reaction is catalyzed by APOBEC1 cytidine deaminase which changes a CAA to a UAA stop codon (Blanc and Davidson, 2003; Baysal et al., 2013). Similarly, the C-to-U RNA editing which changes an arginine (CGA) to a UGA translational stop codon, in the neurofibromatosis type 1 (NF1) mRNA in mammals, is predicted to generate a truncated neurofibromin protein. Of note, neurofibromin is a large and multifunctional protein encoded by the tumor suppressor gene NF1 (Mukhopadhyay et al., 2002; Baysal et al., 2013). Overexpression of exogenous APOBEC3A was reported to induce C-to-U RNA editing of thousands of genes (Sharma et al., 2017).
3.9 Pseudouridine (Ψ)
Ψ is a derivative of uridine (U) formed via base-specific isomerization reactions catalyzed by pseudouridine synthases (PUSs). There are 13 PUSs found in humans (Borchardt et al., 2020). Ψ is found in both ncRNA and mRNA and is conserved across species (Zhao and He, 2015). There are two independent molecular mechanisms underlying the formation of Ψ. The first mechanism involves single protein enzymes (PUSs), which recognize the substrate and catalyze the isomerization of uridine to Ψ (RNA-independent pseudouridylation). In contrast, the second mechanism is an RNA-dependent mechanism involving unique RNA and four common core proteins. The RNA component functions as a guide which base pairs with the substrate RNA and directs an enzyme (Cbf5), which is part of the core proteins, to carry out the pseudouridylation reaction at a specific site (De Zoysa and Yu, 2017). Unlike m6A, m6Am, and m1A, which are all reversible, the conversion from U to Ψ is irreversible (Zhao and He, 2015). Notably, compared to uridine, Ψ has an extra hydrogen-bond donor at its non-Watson-Crick edge. Therefore, when incorporated into RNA, it can change the chemical and physical properties of RNA and hence its cellular function (Zhao and He, 2015).
3.10 2′-O-methylation (Nm)
RNA 2′-O methylation (Nm, where N stands for any nucleotide) is a common RNA modification found in different types of RNA such as rRNA, tRNA, mRNA, and sncRNAs (miRNAs and siRNAs). The Nm modification is generated by addition of a methyl group to 2′ hydroxyl (–OH) of the ribose component of nucleotide either co- or post-transcriptionally (Dimitrova et al., 2019). It is catalyzed by either stand-alone methyltransferases or by the enzyme fibrillarin which is guided by snoRNAs. It has been suggested that Nm RNA modification may cause structural bias which leads to a more stable RNAs and alter cellular activities of the RNA molecules (Abou Assi et al., 2020). It was found that inflammation promotes secretion of snoRNA out of the nucleus, and RNA-Seq data indicate that extracellular vesicles released from cells harbor snoRNAs. These suggest the extended role of snoRNA in cell-cell communication (Rimer et al., 2018). Most mammalian mRNAs have 2′-O methylation at nucleotide 1 (cap 1 mRNA) (Bélanger et al., 2010).
3.11 N7-methylguanosine (m7G)
M7G is a common RNA modification which occurs at the 5′ terminal (m7G-cap) or within RNA molecules. The m7G has been found in tRNA, rRNA, mRNA, and miRNA (Chu et al., 2018; Luo et al., 2022; Cai et al., 2023). Different m7G methyltransferases (writers) have been identified. In mammals, METTL1, which binds to its cofactor WD repeat domain 4 (WDR4), catalyzes m7G modifications in tRNA, miRNA, and mRNA. Internal m7G is recognized by Quaking proteins (QKIs) which also bind to the stress granule (SG) core protein G3BP1 thereby recruiting internal m7G-modified transcripts into SGs presumably to regulate their stability and translation (Zhao et al., 2023).
4 Cap modification
4.1 Canonical RNA capping
The account of RNA modifications would be incomplete without briefly addressing the modifications of the RNA ends (Figure 2). Except for circRNA, cellular RNA molecules are linear polymers with 5′ and 3′ ends. These ends are potentially vulnerable to degradation by exonucleases or recognition by innate immune sensors like RIG-I, MDA5, or IFITs mediating defense against intracellular bacteria and viruses, whence they must be protected (Leung and Amarasinghe, 2016). Long before the internal modifications, it was discovered that most eukaryotic cellular mRNAs carry a 5’ “cap,” m7GpppN that protects the mRNA against attack by phosphatases and nucleases. 5′-mRNA capping occurs shortly after and in concert with transcription initiation. The 5′-capping enzymes, RNA guanylyltransferase (RNGTT) (harboring both 5′-triphosphatase and guanylyltransferase activities) and RNMT, are targeted to the pre-mRNA through binding to the phosphorylated carboxy-terminal domain of RNA polymerase II (RNAPII) (Figure 2). In higher eukaryotes, the m7GpppN structure (cap 0) can be methylated also at the ribose’s 2′-O position within the second (cap 1) and third (cap 2) nucleotides by the cap methyltransferases 1 and 2 (CMTR1 and 2) (Bélanger et al., 2010; Werner et al., 2011). A subset of RNAP II-transcribed cellular RNAs, including snRNA, snoRNA, and telomerase RNA, are further methylated at the N2 of the guanosine to create an trimethylguanosine (m2,2,7G)-capped RNA (Monecke et al., 2009). Besides stabilizing the RNA, the cap has been shown to facilitate splicing, nuclear export, and translation initiation by recruiting protein complexes involved in RNA processing (Shatkin, 1976; Rottman et al., 1974; Hamm and Mattaj, 1990; Izaurralde et al., 1994). The splicing and nuclear export-related effects of RNA capping can be ascribed to the cap-binding complex, CBC. The RNA-binding subunit CBP20 forms CBC with its partner, CBP80. CBC also mediates RNA quality control in the nonsense-mediated decay pathway (Schoenberg and Maquat, 2012). The primary reader for the m7G cap modification during translation is eukaryotic translation initiation factor 4E (EIF4E).
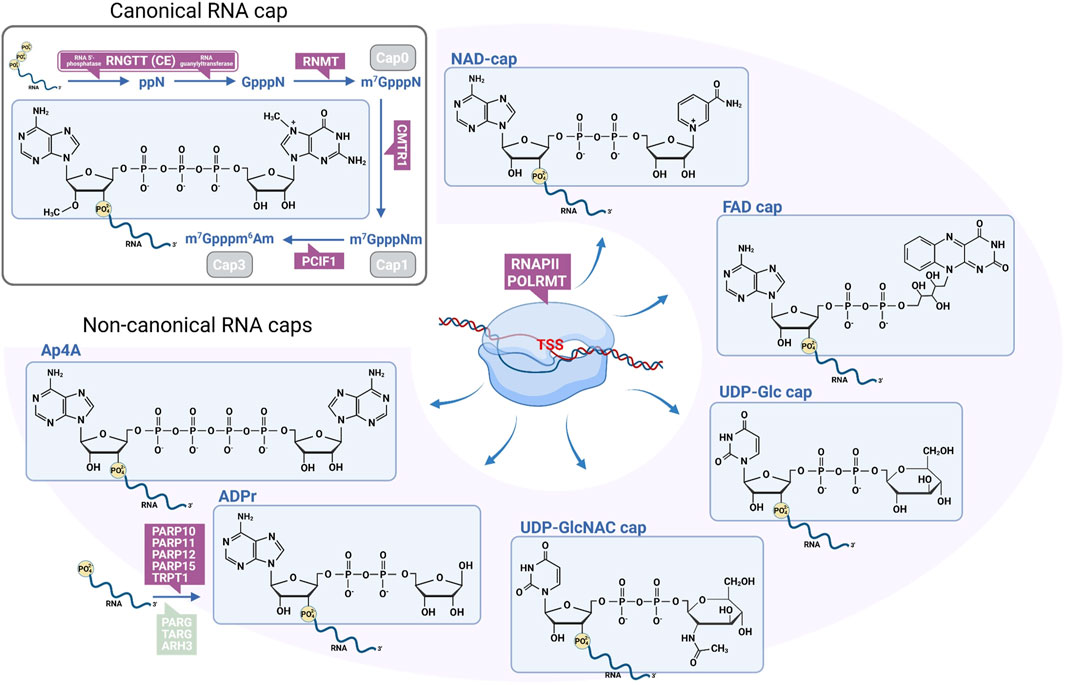
Figure 2. Canonical and non-canonical RNA 5′ cap structures. The canonical pathway requires the RNA 5′-phosphatase activity of RNGTT to cleave the γ-phosphate from the nascent mRNA. Next, the guanylyltransferase domain of RNGTT transfers a GMP through its 5′ phosphate to the 5′ diphospho-RNA. RNMT then methylates the N7 position of the terminal guanine from SAM. The m7GpppN structure (Cap 0) can be further methylated by CMTR1 to form m7GpppNm (Cap 1) and by CMTR2 to form m7GpppNmNm (Cap 2, not shown) structures. When the penultimate nucleoside is adenosine, PCIF1 methylates the N6 position of the adenosine to form m7Gpppm6Am (Cap 3). The non-canonical caps are generated by RNAPII or POLRMT when they initiate transcription with an adenine or uridine nucleotide-containing metabolite instead of ATP or UTP. ADPr-caps can also be generated by several PARPs or TRPT1 through direct ADP-ribosylation of the 5′-phoshate end of an RNA. While the 7mG-cap stabilizes the mRNA and is essential for efficient translation, non-canonical capped RNAs are not transcribed and have varying effect on stability. However, the RNAs capped in this way are protected from innate immune recognition within the cell. ADPr, ADP-ribose; Ap4A, diadenosine tetraphosphate; ARH, ADP-ribosylserine hydrolase; CE, capping enzyme; CMTR, cap (nucleoside-2′-O)-methyltransferase; FAD, flavine adenine dinucleotide; NAD, nicotinamide adenine dinucleotide; PARP, poly(ADP-ribose)-polymerase; PARG, poly(ADP-ribose) glycohydrolase; PCIF1, phosphorylated CTD interacting factor 1; POLRMT, mitochondrial RNA polymerase; RNAPII, RNA polymerase II; RNGTT, RNA guanilyltransferase; RNMT, RNA (guanine N7)-methyltransferase; RTPase, RNA triphosphatase; SAM, S-adenosyl-L-methionine; TARG, O-acyl-ADP-ribose deacylase; TRPT1, tRNA phosphotransferase 1; TSS, transcription start site; UDP-Glc, uridine diphosphate glucose; UDP-GlcNAM, uridine diphosphate N-acetylglucoseamine. Created in BioRender. Demeny (2025) https://BioRender.com/l07c373.
4.2 Non-canonical RNA capping
During the past decade, novel metabolite-derived (NAD, FAD, ADPr, dpCoA, UDP-Glc, and UDP-GlcNAC) terminal cap structures have been discovered, the biosynthesis of which differs from that of the m7G-cap (Pelletier et al., 2021; Wiedermannová et al., 2021) (Figure 2). Metabolite caps are incorporated into the RNA when an RNAP initiates transcription with an adenine nucleotide-derived cofactor or an UDP-sugar instead of an ATP or UTP at transcription start sites featuring A or U in the +1 position (Julius and Yuzenkova, 2017). These non-canonical initiating nucleotide (NCIN) caps have been found in mRNAs of nuclear and mitochondrial origin, snRNAs, and snoRNAs. In mammals, the frequency of metabolite caps is 0.1%–5% and up to 15% in mitochondrial RNA, determined by an interplay between the metabolites’ availability, the RNA polymerases’ affinity, and transcript-specific promoter sequences (Wang et al., 2019). Dinucleoside polyphosphates (NpnN), also called alarmones, are stress-related molecules in bacteria and eukaryotes, although their function is not precisely understood. Diadenosine tetraphosphate (Ap4A), the most abundant NpnN in humans, can also be incorporated into RNA by RNAPII as an NCIN (František Potužník et al., 2024). Interestingly, for this modification, the amount of modified RNAs appears to be independent of the abundance of Ap4A in the cell.
4.2.1 RNA regulation by non-canonical caps
NCIN-capped mRNAs are generally not translated in human cells, but the various caps have been shown to confer different stability. In eukaryotes, the NAD-cap was found to promote RNA decay by the decapping exoribonuclease (DXO) and the Nudix hydrolases, Nudt12 and Nudt16, whereas Ap4A-capped RNA is as stable as a canonical m7G-RNA (Jiao et al., 2017; František Potužník et al., 2024). Various forms of cellular stress have been shown to increase the abundance of NAD-capped RNAs, establishing a link between the cell’s metabolic state, redox homeostasis, and post-transcriptional RNA regulation (Grudzien-Nogalska et al., 2019). Regulation of the level of decapping enzymes under these conditions suggests the further possibility that once the stress has subsided, the cell may revert from NCIN caps to canonical caps through cap-removal and recapping (Grudzien-Nogalska et al., 2019). Cytoplasmic addition of an m7GpppN cap to uncapped RNA is mediated by RNGTT, which has been shown to translocate from the nucleus to the cytoplasm and to form a complex there with RNMT, the latter’s regulatory subunit RAM, and an, as yet, unidentified RNA 5′-monophosphate kinase (Otsuka et al., 2009; Trotman et al., 2017; Gonatopoulos-Pournatzis et al., 2011). Nck-1, a scaffold protein that binds to the proline-rich C-terminus of RNGTT is also a component of the cytoplasmic capping enzyme complex, and it is known to help restore translation following stress by directly interacting with eIF2 and blocking its phosphorylation (Mukherjee et al., 2014). The recently reported association of the cytoplasmic capping enzyme with SGs – membrane-less organelles regarded as translation regulation hubs during stress – equally supports the idea that the cap status of non-canonically capped translationally suppressed transcripts may be restored (Gayen et al., 2024; Baymiller and Moon, 2023).
5 Role of RNA modification in gene expression
Regulation of gene expression is critical for a wide variety of key biological processes, such as organismal development, cell differentiation, cellular stress responses, tissue homeostasis, and immunity (Pope and Medzhitov, 2018). RNA modifications serve as critical posttranscriptional regulators of gene expression programs and their correct deposition is essential for normal development (Frye et al., 2018). Accumulating evidence reveals that the dynamics of internal RNA modifications play critical roles in multiple RNA-processing events including splicing, transport, translation, and degradation all of which in turn regulate gene expression (Zhao et al., 2017; Dominissini and Rechavi, 2018). The expression patterns of RNA modifications and also of their regulators have the potential to be used as biomarkers for diseases or absorption of disease-causing hazard (Chen et al., 2022; Chen et al., 2023; Takahashi et al., 2023). There is mounting evidence that RNA modifications are associated with diverse biological processes including human diseases. Mutations of the genes encoding RNA modifying enzymes have been linked to basic cellular functions such as cell differentiation, sex determination, stress responses, and various human diseases including cancer, cardiovascular diseases, genetic birth defects, metabolic diseases, neurological disorders, and mitochondrial-related defects (Jonkhout et al., 2017). Due to the recent intensive research, a large amount of relevant published reports has appeared. Only selected articles are included in the following discussion on the role of RNA modification in gene expression.
The m6A is known to affect various fundamental cellular processes by regulating target gene expression (Liu and Pan, 2016). In modulating gene expression, m6A controls mRNA stability (Wang et al., 2014), translation efficiency (Wang et al., 2015), and RNA-protein interactions (Liu et al., 2015). The m6A RNA modifications have been linked to various diseases and deterioration of physiological functions such as pancreatic carcinoma (Cao et al., 2023), hepatocellular carcinoma (HCC) (Liu H. et al., 2023), ovarian cancer (Gan et al., 2023), glioma (Wu Z. et al., 2023), osteoarthritis (Liu Y. et al., 2023), Alzheimer’s disease (Ni et al., 2023), pulpitis (Xu et al., 2023), metabolic disorder (Liu K. et al., 2023), impaired immunity (Zhang Y. et al., 2023), hearing loss (Feng et al., 2023), hypoxia (Li S. et al., 2023), aging (Huang et al., 2023), male infertility (Li H. et al., 2023), viral infection (Vaid et al., 2023), etc. The m6A mRNA methylase, WTAP, has been demonstrated to promote progression of diffuse large B-cell lymphoma (DLBCL) by inducing the expression of its target gene hexokinase 2 (HK2), hence increasing the HK2 m6A level. Of note, DLBCL is one of the most common subtypes of lymphoid malignancy (Han et al., 2021). The m6A regulators may serve as a prognostic signature for esophageal squamous cell carcinoma (ESCC). As many as six m6A regulators, METTL3, WTAP, IGF2BP3, YTHDF1, HNRNPA2B1, and HNRNPC, showed increased expression in patients with ESCC. Similarly, increased expression of programmed cell death ligand 1 (PD-L1) was also observed. It was suggested that the m6A methylation regulators play a key role as a mediator for PD-L1 expression (Guo et al., 2021). YTHDF1 has also been reported to promote ovarian cancer progression by augmenting translation of EIF3C, a subunit of eukaryotic initiation factor 3 (EIF3), a complex translation initiation factor in mammalian cells (Liu T. et al., 2020).
Overexpression of a newly discovered m6A reader, named IGF2BP2, was shown to promote lymphatic metastasis and epithelial mesenchymal transition of head and neck squamous carcinoma (HNSCC) through stabilization of mRNA in an m6A-dependent fashion. Notably, epithelial mesenchymal transition is a process by which epithelial cells gain migratory and invasive properties. Overexpression of IGF2BP2 was demonstrated to be associated with a poor overall survival probability of patients with HNSCC (Yu et al., 2022). Following their discovery, more and more studies have been conducted to elucidate the physiological functions of m6A erasers, FTO and ALKBH5, and their roles in disease development (Shen et al., 2022). The association between the demethylase FTO and obesity is currently well documented (Tóth et al., 2020; Al-Jawadi et al., 2021; Vámos et al., 2023), while ALKBH5 has been indicated to play a role in human malignancies (Qu et al., 2022). Recently, it was reported that m6A mediates expression of Frizzled 10 (FZD10) in liver cancer stem cells (CSCs), which in turn stimulates FZD10 self-renewal, tumorigenicity, and metastasis of liver CSCs. The METTL3-dependent m6A modification of FZD10 mRNA also leads to lenvatinib resistance of the CSCs (Wang et al., 2023a). In addition, a study showed that m6A modification of eRNA leads to its activation and promotes transcription and gene activation (Lee et al., 2021).
It has been suggested that m6Am exhibits a significant impact on gene expression regulation. The specific methyltransferase of m6Am, PCIF1, has been indicated to affect mRNA stability, transcription, and translation. Moreover, PCIF1 has been associated with tumor, viral, and endocrine diseases (Wu et al., 2023b). The m6Am modification has unequivocally demonstrated to increase mRNA stability, translation efficiency, and protein levels which may play a dynamic role in obesity-related translation regulation (Ben-Haim et al., 2021). The m6Am RNA modification was suggested to have a negative impact on the translation of mRNAs with m6Am at the 5′ end (Sendinc et al., 2019). However, a study has recently demonstrated that the PCIF1-mediated installment of 5′-cap m6Am increases susceptibility to severe acute respiratory syndrome coronavirus 2 (SARS-CoV-2) infection by stabilizing mRNA which leads to sustained transcription and translation of genes encoding the coronavirus receptors angiotensin-converting enzyme 2 (ACE2) and transmembrane serine protease 2 (TMPRSS2) (Wang L. et al., 2023).
A-to-I RNA editing is important to prevent undesired immune activation (Mann et al., 2023). In addition, dysregulation of A-to-I RNA editing has also been associated with neurological or neurodegenerative diseases such as amyotrophic lateral sclerosis, epilepsy, depression, encephalopathy, suicidal behavior associated with schizophrenia, astrocytoma, bipolar disorder, and episodic ataxia type 1 (Yang Y. et al., 2021). In addition, aberrance in ADAR activity has been linked to human diseases such as cancer, metabolic diseases, viral infections, and autoimmune disorders (Slotkin and Nishikura, 2013). Increased A-to-I RNA editing was observed in relapsed tumor samples from patients with melanoma during targeted therapy. This may be due to increased expression of ADAR enzymes because RNA editing indexes showed positive correlation with the expression levels of genes coding for ADAR enzymes (Amweg et al., 2022). Similarly, A-to-I RNA editing was shown to play a critical role in the development of liver cancer. It was found that in tumor samples, expression of the gene encoding the enzyme ADAR was elevated and A-to-I RNA editing was enhanced. In addition, it was indicated that ADAR regulates its own expression by self-editing, and also affects the global transcription and translation products of cancer-related genes by editing and changing their expression profiles (Li et al., 2021). Recently, a study reported increased A-to-I RNA editing in patients with atherosclerosis, cardiomyopathies, and heart failure. The insulin-like growth factor binding protein 7 (IGFBP7) was identified as the main editing site. Of note, IGFBP7 is a protein which functions to regulate the availability of insulin-like growth factors and their binding to their receptors (Mann et al., 2023). The A-to-I RNA editing events were suggested to be involved in Parkinson’s disease through their effects on gene expression. The editing events were found to occur mainly in protein-coding genes and Arthrobacter luteus (Alu) repeats. Lower overall editing frequency, and hence, decreased editing levels were observed in patients with Parkinson’s disease. It was proposed that A-to-I RNA editing regulates gene expression by changing the miRNA binding sites of the host gene (Wu S. et al., 2023). A study using Caenorhabditis elegans demonstrated that A-to-I RNA editing stimulates developmental stage–specific genes and the expression of lncRNA. As competition between RNA editing mechanisms and RNA interference (RNAi) had previously been indicated, it was hypothesized that A-to-I RNA editing is essential for normal growth and development by regulating the process of silencing gene expression through RNAi (Goldstein et al., 2017).
The m5C has been identified in mRNA, rRNA, and tRNA in organisms from all species and plays a critical role in diverse biological processes such as the modulation of transcription, RNA stability, and protein synthesis (Song et al., 2022). The m5C reader protein, YBX1 is essential for mediating mRNA stability (Chen X. et al., 2019) and the reader, ALYREF plays a role in facilitating mRNA nuclear export (Dominissini and Rechavi, 2017; Yang et al., 2017). The m5C methyltransferase, NSUN6 was indicated to suppress pancreatic cancer development by controlling cell proliferation. Significantly reduced expression of NSUN6 was observed in pancreatic cancer tissues compared to normal controls (Yang R. et al., 2021). Overexpression of m5C methyltransferase, NSUN2 has been found to cause resistance of small-cell lung cancer to the epidermal growth factor receptor (EGFR) inhibitor, gefitinib. The mechanism was suggested to involve increased methylation of the quiescin sulfhydryl oxidase 1 (QSOX1) coding sequence region which leads to enhanced QSOX1 translation through m5C reader Y-box binding protein 1 (YBX1) (Wang Y. et al., 2023). The m5C RNA modification was also found to play a role in regulating the innate immune response to virus infection by modulating type I interferons. Depletion of m5C methyltransferase, NSUN2, was demonstrated to reduce m5C methylation and inhibit replication and gene expression of different viruses, although the m5C methylation of viral RNA was unaffected (Zhang et al., 2022). The m5C has been found to activate cancer metastasis by promoting mitochondrial protein translation. In mitochondria, the biosynthesis of the mitochondrially encoded subunits of the oxidative phosphorylation complexes is dependent on formation of m5C at position 34 in the mitochondrial methionine tRNA. Notably, the mitochondrial oxidative phosphorylation system plays a critical role in the efficient generation of cellular energy in the form of ATP. A metabolic switch from glycolysis to oxidative phosphorylation was found to facilitate tumorigenesis (Delaunay et al., 2022).
The hm5C RNA modification has been indicated to play an important regulatory role inside the cells (Huber et al., 2015). In mammals, TET2 was reported to stimulate pathogen infection-induced myelopoiesis, a common host immune response in acute and chronic infections (Shen et al., 2018). A study employing mouse embryonic stem cells, suggested that hm5C plays an important role in the regulation of the embryonic stem cell self-renewal network. In this study, Tet mediated RNA hydroxymethylation was found to reduce the stability of pluripotency promoting transcripts. A reduced level of hm5C was observed during cell differentiation. It was hypothesized that hm5C is a mark of transcriptome flexibility which is important for controlling the balance between pluripotency and lineage commitment (Lan et al., 2020). In Drosophila melanogaster, it was discovered that RNA hydroxymethylation promotes RNA translation. As previously mentioned, Tet and hm5C were prevalent in Drosophila brain. Fruit flies lacking Tet suffer from decreased RNA hydroxymethylation and impaired brain development (Delatte et al., 2016).
The detailed biological function of m3C RNA modification has yet to be fully elucidated. Considering that it is mainly present in the anticodon loop of tRNAs, it is hypothesized that m3C affects precise pairing between codon and anti-codon (Mao et al., 2021). It has also been indicated that m3C is important for tRNA structure and folding (Lentini et al., 2022). The m3C RNA modification has been suggested to be essential for ensuring proper architecture of tRNAs which is critical for translation fidelity. The lack of tRNA m3C modifications may cause impaired translation process (Bohnsack et al., 2022).
Dysregulation of C-to-U miRNA editing may contribute to pathogenesis of Huntington’s disease (Guo et al., 2022). A study suggested that APOBEC3-mediated C-to-U RNA editing is positively associated with elevated immune activity and improved survival of patients with breast cancer (Asaoka et al., 2019). Mutations in the APOBEC1 cofactors, RBM47, have been linked to breast cancer progression and increased metastatic potential (Lerner et al., 2019). C-to-U RNA editing has been indicated to accelerate the evolution of RNA viruses such as SARS-CoV-2. Comparative genomic analysis of world-wide SARS-CoV-2 strains showed that C-to-U RNA editing is the main source of SARS-CoV-2 mutation (Wang et al., 2023b).
RNA pseudouridylation has been suggested to affect RNA metabolism and gene expression (Borchardt et al., 2020). In humans, co-transcriptional pseudouridylation of pre-mRNA was found to be essential for pre-mRNA processing. Three PUSs, PUS1, PUS7, and RNA PUS D4 (RPUSD4), were suggested to be involved in pseudouridylation process (Martinez et al., 2022). Ψ has been indicated to increase transcript stability (Schwartz et al., 2014) and therefore it can alter efficiency of translation initiation and other cellular processes (Carlile et al., 2014). Similarly, a study found that in yeast, pseudouridylation of tRNA and mRNA by PUS6 is essential for promoting translation. The mechanism involves increased binding of yeast methionine aminoacyl tRNAMet synthetase (MetRS), which functions as a reader, to both pseudouridylated tRNA and pseudouridylated mRNA which results in an enhanced translation process (Levi and Arava, 2021). A previous study has also demonstrated that when uridine molecules in the mRNA are replaced with Ψ, the translation level is improved. The mechanism involves decreased activation of RNA-dependent protein kinase (PKR), a mammalian enzyme which regulates translation during stress conditions (Anderson et al., 2010). In humans, mutations in PUS3 protein were shown to reduce PUS3-dependent Ψ levels which cause intellectual disability (Lin et al., 2022). Similarly, mutations in human PUS7 were found to cause intellectual disability and microcephaly due to impaired pseudouridylation (Shaheen et al., 2019). Ψ is also installed to RNA molecules by the H/ACA small ribonucleoprotein (snoRNP) complex which shares four core proteins, dyskerin (DKC1), NOP10, NHP2, and GAR1. It was reported that mutations in DKC1 and NOP10 genes cause nephrotic syndrome with cataracts, hearing impairment, and enterocolitis (Balogh et al., 2020). Recently, DKC1 was indicated to play a role in regulating translation via mRNA pseudouridylation (Pederiva et al., 2023). The translation process was also shown to be regulated by rRNA pseudouridylation (Zhao et al., 2023). Furthermore, alterations of rRNA pseudouridylation levels at specific sites have been linked to human breast cancer (Barozzi et al., 2023).
In human cells, the snoRNA-guided Nm modifications of mRNA have been suggested to play an important role in modulating gene expression by altering mRNA levels and controlling protein biosynthesis. Nm RNA modifications were found to increase peroxidasin mRNA expression but inhibit its translation (Elliott et al., 2019). Similarly, in yeast, Nm RNA modifications were also suggested to play a role in translation regulation. Aberrant rRNA Nm patterns or hypo-2′-O-methylated ribosomes were revealed to cause drastic defects in translation fidelity (Khoshnevis et al., 2022). The expression of the Nm factors which mediate RNA 2′-O-methylation was shown to be associated with malignant melanoma formation. Upregulation of Nm factors such as fibrillarin, nucleolar protein (NOP) 56, NOP58, or SNU13 was found to be correlated with this disease and has a negative impact on overall survival of patients with melanoma (Jasinski-Bergner et al., 2021). Nm within bacterial RNA was suggested to suppress activation of human innate immune response by inhibiting Toll-like receptor (TLR) 7-mediated-IFN-α production. It is important to note that the innate immune system plays a critical role in the early sensing and clearance of infecting pathogens and differences in posttranscriptional RNA modification profiles are used by the immune system to discriminate between the host and pathogenic nucleic acids. This principle is likely exploited by certain bacteria to evade the host immune responses (Rimbach et al., 2015). Increased Nm was observed in polyadenylated RNA in virus infected-macrophages. Fibrillarin and its mediated Nm RNA modifications may promote viral infection (Li P. et al., 2022). The internal Nm RNA modifications on the human immunodeficiency virus type-1 (HIV-1) genome are employed by the virus to limit the host immune sensing and interferon production. However, the Nm marks are observed to impair HIV-1 reverse transcriptase activity and hence inhibit viral replication (Decombe et al., 2024). The human mRNA Cap 2′-O-Methyltransferase 1 (CMTR1) was found to regulate the expression of certain interferon-stimulated genes which are essential for restricting viral infection. CMTR1 was shown to mediate the protein expression of IFN-stimulated genes by preventing interferon-induced protein with tetratricopeptide repeats 1 (IFIT1) from inhibiting the translation of mRNAs lacking cap 2′-O-methylation. Therefore, CMTR1 stimulates the IFN-mediated antiviral response (Williams et al., 2020).
The m7G modification is associated with the biological processes and regulation of various diseases. It has been indicated that m7G is tightly linked to tumor prognosis, development, and the immune response. A number of m7G regulatory genes have been proposed as risk signatures of HCC considering their significant effects on prognosis, progression, and antitumor immune response of HCC (Zhou et al., 2022). A study has indicated that m7G-related lncRNAs are associated with the tumor immune landscape and the prognosis of HCC. Moreover, as many as 32 m7G-related lncRNAs were confirmed to be prognostic lncRNAs and can be applied as independent prognostic markers of HCC (Li Y. et al., 2023). Similarly, expression of several m7G methylation-related regulator genes, such as EIF4E3, LARP1, NCBP3, and IFIT5 have been shown as good prognostic predictors for melanoma (Deng et al., 2022). Recently, a study revealed the involvement of m7G in the development of drug resistance in acute myeloid leukemia (AML), a type of blood cancer characterized by uncontrolled proliferation of myeloid cells. It was observed that lncRNA m7G methylations are more abundant in drug-resistant AML cells compared to that in drug-sensitive AML cells (Han et al., 2023). Similarly, an association between m7G modifications in circRNAs and drug-resistant AML has been suggested. A significant difference in m7G level between AML cells and drug-resistant AML cells was found which indicates a potential role of m7G in circRNAs in drug-resistant AML development. It was hypothesized that the m7G methylation affects co-expression of circRNA, miRNA, and mRNA which may further affect the modulation of resistance-associated genes in AML (Fu et al., 2023). Recently, it has been reported that internal m7G when located within a GANGAN (N = A/C/U/G) motif is selectively recognized by QKIs (Zhao et al., 2023). QKIs transport internal m7G-modified RNAs into SGs presumably modulating the modified mRNA’s half-life and expression. QKI7, e.g., attenuates the translation efficiency of essential genes in the Hippo signaling pathway sensitizing cancer cells to chemotherapy.
6 Therapeutic developments
To this end, it is now appreciated that numerous cellular processes are finely regulated by RNA modifications, such as RNA localization, stability, degradation, binding to other molecules, and protein biosynthesis. RNA modifications and the set of proteins involved in their installment, removal, and interpretation have been evidenced to associate with multiple types of human diseases including cancer development. Therefore, the RNA modification pathway has been considered as an ideal novel therapeutic target for treatment of various human diseases. The roles of RNA modification and related techniques in therapeutic development have been reviewed (Nombela et al., 2021; Berdasco and Esteller, 2022; Wang C. et al., 2023; Warminski et al., 2023). In this review, the current strategies and progress of epitranscriptomic-based therapeutic development are briefly highlighted (Figure 3).
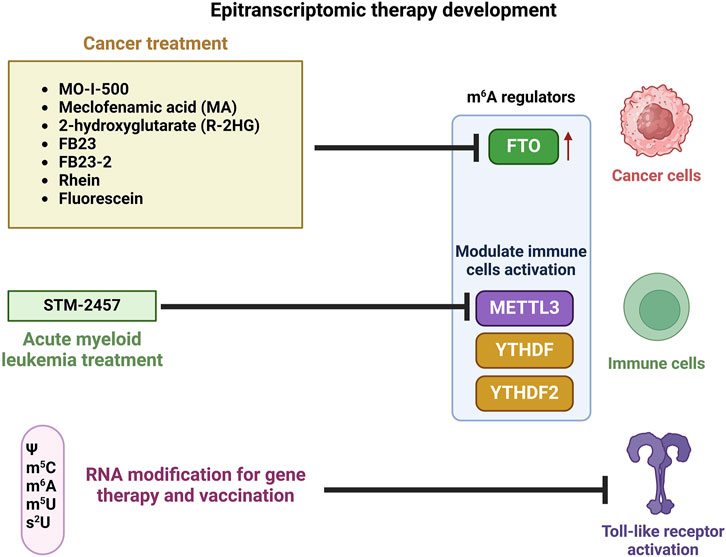
Figure 3. Epitranscriptomic-based therapy development for diseases. FTO (eraser), METTL3 (writer), YTHDF and YTHDF2 (readers) are m6A regulators. Created in BioRender. Demeny (2025) https://BioRender.com/v73i340.
As mentioned previously, m6A is the most prevalent internal modification in mRNA of eukaryotic species including mammals. The m6A modification is reversible and its dynamics are of functional importance. It has been suggested that aberrant levels of m6A and dysregulation of expression of its regulators (writers, erasers, and readers) are often linked to various types of cancer. Therefore, m6A regulators have been targeted in cancer therapies. A number of FTO inhibitors such as MO-I-500, meclofenamic acid (MA), 2-hydroxylglutarate (R-2HG), FB23, FB23-2, rhein, and fluorescein have been developed for cancer treatment (Huang et al., 2020; Li X. et al., 2022). In addition, the m6A regulators, METTL3, YT521-B homology domain family 1 (YTHDF1), and YTHDF2, have been indicated to modulate immune cell activation and infiltration into the tumor microenvironment and hence can influence the efficacy of immunotherapy. To develop effective strategies in targeting these regulatory proteins, a more detailed understanding on their modes of action is required (Li X. et al., 2022). A METTL3 inhibitor, STM-2457 has been reported to exhibit promising results in preclinical studies on a mouse model for AML (Berdasco and Esteller, 2022). The FDA-approved DNA methylation inhibitor, 5-azacytidine may also inhibit RNA methylation as the vast majority of 5-azacytidine is incorporated into the RNA molecule (Berdasco and Esteller, 2022). Recently, it was shown that a strategy involving inactivation of the host YTHDF2 gene has the potential to be used to improve recombinant therapeutic protein production (Lao and Barron, 2023).
The natural form of in vitro-transcribed mRNAs of physiologically important proteins was considered unsuitable for clinical application because of instability. In addition, the native mRNAs activate cells of the innate immune system by stimulating TLRs. Importantly, RNA modifications through incorporation of natural nucleosides such as Ψ, m5C, m6A, 5-methyluridine (m5U), or 2-thiouridine (s2U) was demonstrated to diminish the TLRs activation (Karikó et al., 2005; Karikó et al., 2008). Moreover, in mammalian cells, mRNAs containing Ψs were found to have a higher translational capacity compared to the unmodified mRNAs, making the mRNAs harboring Ψs promising tools for gene therapy and vaccination (Karikó et al., 2008). The development of mRNA vaccines against the coronavirus disease of 2019 (COVID-19) caused by the SARS-CoV-2 was regarded as the fastest and most efficient vaccine development in human history. It should be noted that a key aspect of COVID-19 mRNA vaccines is the application of the modified nucleobase N1-methylpseudouridine (m1Ψ) to improve their effectiveness. Every uridine residue in the mRNA was replaced with m1Ψ. The m1Ψ nucleobase was used to enhance immune evasion and promote protein biosynthesis (Nance and Meier, 2021).
In principle, the development of mRNA therapeutics is based on the delivery of a synthetic transcript which is followed by biosynthesis of the encoded pharmacologically active protein by the cellular translational machinery (Liu and Wang, 2022). The majority of mRNA drugs is generated by in vitro transcription from a DNA template and can then be enzymatically modified through incorporation of modified nucleotides (Liu and Wang, 2022). Bacteriophage T7 RNA polymerase (T7 RNAP) is widely used to synthesize RNA molecules with synthetic modifications and unnatural base pairs for therapeutic purposes. It has recently been revealed that the T7 RNAP recognizes the unnatural substrates at the pre-insertion state in a different manner compared to natural substrates. This information may be useful in the generation of unnatural base pairs which are valuable for therapeutic applications (Oh et al., 2023). In addition, the use of N2 modified dinucleotide cap analogs as components of mRNA transcripts was demonstrated to enhance mRNA translation both in vitro and in human cells (Grzela et al., 2023). A programmable RNA base editor named RESTART has been developed for replacing uridine with Ψ in stop codons to suppress premature termination codons. This RNA-editing tool is expected to be useful in research and development of RNA-based therapeutics (Song J. et al., 2023).
7 Conclusion
The advancement of robust methods for detection of RNA modifications has stimulated intense research and revolutionized our understanding of multiple fundamental aspects of RNA modifications. These emerging techniques enable precise and reliable detection of the numerous modified nucleotides in RNA molecules and together with the advent of computational tools have driven the rise of the field epitranscriptomics. Improved knowledge on molecular mechanisms underlying the association of RNA modifications with various critical biological processes, including disease and its development has facilitated the construction of effective strategies for disease control and improvement of human life. Future studies should be directed towards development of more sensitive and accurate methods for detection of biochemical modifications on RNA molecules which relatively of low abundance. Detailed elucidation of molecular mechanism of each chemical modification on cellular RNA and the illumination of its biological functions remain a huge future challenge. Better understanding on distribution and signature of RNA modifications and their functional consequences is required. In addition, more accurate and reliable bioinformatics tools for data analysis need to be established. Research and development on epitranscriptomics-based therapeutics need to be strengthened in order to accelerate vaccine development and drug discovery in response to the global health issues on both communicable and noncommunicable diseases.
Author contributions
IMA: Conceptualization, Data curation, Supervision, Writing – original draft. RA: Visualization, Writing – original draft. MÁD: Funding acquisition, Visualization, Writing – review and editing. EK: Funding acquisition, Supervision, Writing – review and editing.
Funding
The author(s) declare that financial support was received for the research and/or publication of this article. EK, MÁD, and RA were funded by the National Research, Development and Innovation Office (NKFIH- FK145866, K147482, and PD146202, respectively) of Hungary and the University of Debrecen Program for Scientific Publication.
Acknowledgments
The authors thank John Acton for his assistance at the manuscript preparation stage.
Conflict of interest
The authors declare that the research was conducted in the absence of any commercial or financial relationships that could be construed as a potential conflict of interest.
Generative AI statement
The author(s) declare that no Gen AI was used in the creation of this manuscript.
Publisher’s note
All claims expressed in this article are solely those of the authors and do not necessarily represent those of their affiliated organizations, or those of the publisher, the editors and the reviewers. Any product that may be evaluated in this article, or claim that may be made by its manufacturer, is not guaranteed or endorsed by the publisher.
References
Adamopoulos, P. G., Athanasopoulou, K., Daneva, G. N., and Scorilas, A. (2023). The repertoire of RNA modifications orchestrates a plethora of cellular responses. Int. J. Mol. Sci. 24, 2387. doi:10.3390/ijms24032387
Akichika, S., Hirano, S., Shichino, Y., Suzuki, T., Nishimasu, H., Ishitani, R., et al. (2019). Cap-specific terminal N 6-methylation of RNA by an RNA polymerase II-associated methyltransferase. Science 363, eaav0080. doi:10.1126/science.aav0080
Al-Jawadi, A. A., Priliani, L., Oktavianthi, S., Febinia, C. A., Daya, M., Artika, I. M., et al. (2021). Association of FTO rs1421085 single nucleotide polymorphism with fat and fatty acid intake in Indonesian adults. BMC Res. Notes 14, 411. doi:10.1186/s13104-021-05823-1
Amweg, A., Tusup, M., Cheng, P., Picardi, E., Dummer, R., Levesque, M. P., et al. (2022). The A to I editing landscape in melanoma and its relation to clinical outcome. RNA Biol. 19, 996–1006. doi:10.1080/15476286.2022.2110390
Anderson, B. R., Muramatsu, H., Nallagatla, S. R., Bevilacqua, P. C., Sansing, L. H., Weissman, D., et al. (2010). Incorporation of pseudouridine into mRNA enhances translation by diminishing PKR activation. Nucleic Acids Res. 38, 5884–5892. doi:10.1093/nar/gkq347
Abou Assi, H., Rangadurai, A. K., Shi, H., Liu, B., Clay, M. C., Erharter, K., et al. (2020). 2'-O-Methylation can increase the abundance and lifetime of alternative RNA conformational states. Nucleic Acids Res. 48, 12365–12379. doi:10.1093/nar/gkaa928
Arzumanian, V. A., Dolgalev, G. V., Kurbatov, I. Y., Kiseleva, O. I., and Poverennaya, E. V. (2022). Epitranscriptome: review of top 25 most-studied RNA modifications. Int. J. Mol. Sci. 23, 13851. doi:10.3390/ijms232213851
Asaoka, M., Ishikawa, T., Takabe, K., and Patnaik, S. K. (2019). APOBEC3-mediated RNA editing in breast cancer is associated with heightened immune activity and improved survival. Int. J. Mol. Sci. 20, 5621. doi:10.3390/ijms20225621
Balogh, E., Chandler, J. C., Varga, M., Tahoun, M., Menyhárd, D. K., Schay, G., et al. (2020). Pseudouridylation defect due to DKC1 and NOP10 mutations causes nephrotic syndrome with cataracts, hearing impairment, and enterocolitis. Proc. Natl. Acad. Sci. U. S. A. 117, 15137–15147. doi:10.1073/pnas.2002328117
Baquero-Perez, B., Geers, D., and Díez, J. (2021). From A to m6A: the emerging viral epitranscriptome. Viruses 13, 1049. doi:10.3390/v13061049
Barozzi, C., Zacchini, F., Corradini, A. G., Morara, M., Serra, M., De Sanctis, V., et al. (2023). Alterations of ribosomal RNA pseudouridylation in human breast cancer. Nar. Cancer 5, zcad026. doi:10.1093/narcan/zcad026
Baymiller, M., and Moon, S. L. (2023). Stress granules as causes and consequences of translation suppression. Antioxid. Redox Signal 39, 390–409. doi:10.1089/ars.2022.0164
Baysal, B. E., De Jong, K., Liu, B., Wang, J., Patnaik, S. K., Wallace, P. K., et al. (2013). Hypoxia-inducible C-to-U coding RNA editing downregulates SDHB in monocytes. PeerJ 1, e152. doi:10.7717/peerj.152
Bedi, R. K., Huang, D., Li, Y., and Caflisch, A. (2023). Structure-based design of inhibitors of the m6A-RNA writer enzyme METTL3. ACS Bio Med. Chem. Au 3, 359–370. doi:10.1021/acsbiomedchemau.3c00023
Bélanger, F., Stepinski, J., Darzynkiewicz, E., and Pelletier, J. (2010). Characterization of hMTr1, a human Cap1 2'-O-ribose methyltransferase. J. Biol. Chem. 285 (43), 33037–33044. doi:10.1074/jbc.M110.155283
Ben-Haim, M. S., Pinto, Y., Moshitch-Moshkovitz, S., Hershkovitz, V., Kol, N., Diamant-Levi, T., et al. (2021). Dynamic regulation of N6,2'-O-dimethyladenosine (m6Am) in obesity. Nat. Commun. 12, 7185. doi:10.1038/s41467-021-27421-2
Berdasco, M., and Esteller, M. (2022). Towards a druggable epitranscriptome: compounds that target RNA modifications in cancer. Br. J. Pharmacol. 179, 2868–2889. doi:10.1111/bph.15604
Blanc, V., and Davidson, N. O. (2003). C-to-U RNA editing: mechanisms leading to genetic diversity. J. Biol. Chem. 278, 1395–1398. doi:10.1074/jbc.R200024200
Bohnsack, K. E., Kleiber, N., Lemus-Diaz, N., and Bohnsack, M. T. (2022). Roles and dynamics of 3-methylcytidine in cellular RNAs. Trends Biochem. Sci. 47, 596–608. doi:10.1016/j.tibs.2022.03.004
Borchardt, E. K., Martinez, N. M., and Gilbert, W. V. (2020). Regulation and function of RNA pseudouridylation in human cells. Annu. Rev. Genet. 54, 309–336. doi:10.1146/annurev-genet-112618-043830
Cai, M., Yang, C., and Wang, Z. (2023). N7-methylguanosine modification: from regulatory roles to therapeutic implications in cancer. Am. J. Cancer Res. 13, 1640–1655.
Cantara, W. A., Crain, P. F., Rozenski, J., McCloskey, J. A., Harris, K. A., Zhang, X., et al. (2011). The RNA modification database, RNAMDB: 2011 update. Nucleic Acids Res. 39 (Database issue), D195–D201. doi:10.1093/nar/gkq1028
Cao, P., Wu, Y., Sun, D., Zhang, W., Qiu, J., Tang, Z., et al. (2023). IGF2BP2 promotes pancreatic carcinoma progression by enhancing the stability of B3GNT6 mRNA via m6A methylation. Cancer Med. 12, 4405–4420. doi:10.1002/cam4.5096
Cappannini, A., Ray, A., Purta, E., Mukherjee, S., Boccaletto, P., Moafinejad, S. N., et al. (2024). MODOMICS: a database of RNA modifications and related information. 2023 update. Nucleic Acids Res. 52, D239–D244. doi:10.1093/nar/gkad1083
Carlile, T. M., Rojas-Duran, M. F., Zinshteyn, B., Shin, H., Bartoli, K. M., and Gilbert, W. V. (2014). Pseudouridine profiling reveals regulated mRNA pseudouridylation in yeast and human cells. Nature 515, 143–146. doi:10.1038/nature13802
Cesaro, B., Tarullo, M., and Fatica, A. (2023). Regulation of gene expression by m6Am RNA modification. Int. J. Mol. Sci. 24, 2277. doi:10.3390/ijms24032277
Chen, H., Zhao, X., Yang, W., Zhang, Q., Hao, R., Jiang, S., et al. (2023). RNA N6-methyladenosine modification-based biomarkers for absorbed ionizing radiation dose estimation. Nat. Commun. 14, 6912. doi:10.1038/s41467-023-42665-w
Chen, R., Zhou, J., Liu, L., Mao, X.-L., Zhou, X., and Xie, W. (2021). Crystal structure of human METTL6, the m3C methyltransferase. Commun. Biol. 4, 1361. doi:10.1038/s42003-021-02890-9
Chen, X., Li, A., Sun, B.-F., Yang, Y., Han, Y.-N., Yuan, X., et al. (2019). 5-methylcytosine promotes pathogenesis of bladder cancer through stabilizing mRNAs. Nat. Cell Biol. 21, 978–990. doi:10.1038/s41556-019-0361-y
Chen, Z., Qi, M., Shen, B., Luo, G., Wu, Y., Li, J., et al. (2019). Transfer RNA demethylase ALKBH3 promotes cancer progression via induction of tRNA-derived small RNAs. Nucleic Acids Res. 47, 2533–2545. doi:10.1093/nar/gky1250
Chen, Z., Zhang, Z., Ding, W., Zhang, J.-H., Tan, Z.-L., Mei, Y.-R., et al. (2022). Expression and potential biomarkers of regulators for M7G RNA modification in gliomas. Front. Neurol. 13, 886246. doi:10.3389/fneur.2022.886246
Chen, Z., Zhao, P., Li, F., Wang, Y., Smith, A. I., Webb, G. I., et al. (2020). Comprehensive review and assessment of computational methods for predicting RNA post-transcriptional modification sites from RNA sequences. Brief. Bioinform 21, 1676–1696. doi:10.1093/bib/bbz112
Christofi, T., and Zaravinos, A. (2019). RNA editing in the forefront of epitranscriptomics and human health. J. Transl. Med. 17, 319. doi:10.1186/s12967-019-2071-4
Chu, J. M., Ye, T. T., Ma, C. J., Lan, M. D., Liu, T., Yuan, B. F., et al. (2018). Existence of internal N7-methylguanosine modification in mRNA determined by differential enzyme treatment coupled with mass spectrometry analysis. ACS Chem. Biol. 13, 3243–3250. doi:10.1021/acschembio.7b00906
Cirzi, C., and Tuorto, F. (2021). Analysis of queuosine tRNA modification using APB Northern blot assay. Methods Mol. Biol. 2298, 217–230. doi:10.1007/978-1-0716-1374-0_14
Cui, J., Liu, Q., Sendinc, E., Shi, Y., and Gregory, R. I. (2021). Nucleotide resolution profiling of m3C RNA modification by HAC-seq. Nucleic Acids Res. 49, e27. doi:10.1093/nar/gkaa1186
Cullen, B. R., and Tsai, K. (2021). Mapping RNA modifications using photo-crosslinking-assisted modification sequencing. Methods Mol. Biol. 2298, 123–134. doi:10.1007/978-1-0716-1374-0_8
Czekay, D. P., Schultz, S. K., and Kothe, U. (2021). Assaying the molecular determinants and kinetics of RNA pseudouridylation by H/ACA snoRNPs and stand-alone pseudouridine synthases. Methods Mol. Biol. 2298, 357–378. doi:10.1007/978-1-0716-1374-0_21
Decombe, A., Peersen, O., Sutto-Ortiz, P., Chamontin, C., Piorkowski, G., Canard, B., et al. (2024). Internal RNA 2'-O-methylation on the HIV-1 genome impairs reverse transcription. Nucleic Acids Res. 52, 1359–1373. doi:10.1093/nar/gkad1134
Delatte, B., Wang, F., Ngoc, L. V., Collignon, E., Bonvin, E., Deplus, R., et al. (2016). RNA biochemistry. Transcriptome-wide distribution and function of RNA hydroxymethylcytosine. Science 351, 282–285. doi:10.1126/science.aac5253
Delaunay, S., Pascual, G., Feng, B., Klann, K., Behm, M., Hotz-Wagenblatt, A., et al. (2022). Mitochondrial RNA modifications shape metabolic plasticity in metastasis. Nature 607, 593–603. doi:10.1038/s41586-022-04898-5
Deng, J., Lin, J., Liu, C., Li, J., Cai, J., Zhou, X., et al. (2022). N7-methylguanosine methylation-related regulator genes as biological markers in predicting prognosis for melanoma. Sci. Rep. 12, 21082. doi:10.1038/s41598-022-25698-x
De Zoysa, M. D., and Yu, Y.-T. (2017). Posttranscriptional RNA pseudouridylation. Enzymes. 41, 151–167. doi:10.1016/bs.enz.2017.02.001
Dimitrova, D. G., Teysset, L., and Carré, C. (2019). RNA 2'-O-methylation (Nm) modification in human diseases. Genes (Basel). 10, 117. doi:10.3390/genes10020117
Dominissini, D., and Rechavi, G. (2017). 5-methylcytosine mediates nuclear export of mRNA. Cell Res. 27, 717–719. doi:10.1038/cr.2017.73
Dominissini, D., and Rechavi, G. (2018). Epitranscriptome regulation. Nat. Struct. Mol. Biol. (Poster). doi:10.1038/s41594-018-0140-7
Du, Y., Hou, G., Zhang, H., Dou, J., He, J., Guo, Y., et al. (2018). SUMOylation of the m6A-RNA methyltransferase METTL3 modulates its function. Nucleic Acids Res. 46, 5195–5208. doi:10.1093/nar/gky156
El Allali, A., Elhamraoui, Z., and Daoud, R. (2021). Machine learning applications in RNA modification sites prediction. Comput. Struct. Biotechnol. J. 19, 5510–5524. doi:10.1016/j.csbj.2021.09.025
Elliott, B. A., Ho, H.-T., Ranganathan, S. V., Vangaveti, S., Ilkayeva, O., Abou Assi, H., et al. (2019). Modification of messenger RNA by 2'-O-methylation regulates gene expression in vivo. Nat. Commun. 10, 3401. doi:10.1038/s41467-019-11375-7
Elliott, B. A., and Holley, C. L. (2021). Assessing 2'-O-methylation of mRNA using quantitative PCR. Methods Mol. Biol. 2298, 171–184. doi:10.1007/978-1-0716-1374-0_11
Feng, M., Zhou, X., Hu, Y., Zhang, J., Yang, T., Chen, Z., et al. (2023). Comprehensive transcriptomic profiling of m6A modification in age-related hearing loss. Biomolecules 13, 1537. doi:10.3390/biom13101537
František Potužník, J., Nešuta, O., Škríba, A., Voleníková, B., Mititelu, M. B., Mancini, F., et al. (2024). Diadenosine tetraphosphate (Ap4 A) serves as a 5' RNA cap in mammalian cells. Angew. Chem. Int. Ed. Engl. 63, e202314951. doi:10.1002/anie.202314951
Frye, M., Harada, B. T., Behm, M., and He, C. (2018). RNA modifications modulate gene expression during development. Science 361, 1346–1349. doi:10.1126/science.aau1646
Fu, J., Si, L., Zhou, Y., Li, D., and Wang, R. (2023). Distinct N7-methylguanosine profiles of circular RNAs in drug-resistant acute myeloid leukemia. Sci. Rep. 13, 14704. doi:10.1038/s41598-023-41974-w
Fu, L., Guerrero, C. R., Zhong, N., Amato, N. J., Liu, Y., Liu, S., et al. (2014). Tet-mediated formation of 5-hydroxymethylcytosine in RNA. J. Am. Chem. Soc. 136, 11582–11585. doi:10.1021/ja505305z
Gabay, O., Shoshan, Y., Kopel, E., Ben-Zvi, U., Mann, T. D., Bressler, N., et al. (2022). Landscape of adenosine-to-inosine RNA recoding across human tissues. Nat. Commun. 13, 1184. doi:10.1038/s41467-022-28841-4
Gan, L., Zhao, S., Gao, Y., Qi, Y., Su, M., Wang, A., et al. (2023). N6-methyladenosine methyltransferase KIAA1429 promoted ovarian cancer aerobic glycolysis and progression through enhancing ENO1 expression. Biol. Direct 18, 64. doi:10.1186/s13062-023-00420-7
Gao, Y., and Fang, J. (2021). RNA 5-methylcytosine modification and its emerging role as an epitranscriptomic mark. RNA Biol. 18 (Suppl. 1), 117–127. doi:10.1080/15476286.2021.1950993
Gato, A., Catala, M., Tisné, C., and Barraud, P. (2021). A method to monitor the introduction of posttranscriptional modifications in tRNAs with NMR spectroscopy. Methods Mol. Biol. 2298, 307–323. doi:10.1007/978-1-0716-1374-0_19
Gayen, A., Mukherjee, A., Kumar, K., Majumder, S., Chakrabarti, S., and Mukherjee, C. (2024). The mRNA-capping enzyme localizes to stress granules in the cytoplasm and maintains cap homeostasis of target mRNAs. J. Cell Sci. 137, jcs261578. doi:10.1242/jcs.261578
Gilbert, W. V., and Nachtergaele, S. (2023). mRNA regulation by RNA modifications. Annu. Rev. Biochem. 92, 175–198. doi:10.1146/annurev-biochem-052521-035949
Goldstein, B., Agranat-Tamir, L., Light, D., Ben-Naim Zgayer, O., Fishman, A., and Lamm, A. T. (2017). A-to-I RNA editing promotes developmental stage-specific gene and lncRNA expression. Genome Res. 27, 462–470. doi:10.1101/gr.211169.116
Gonatopoulos-Pournatzis, T., Dunn, S., Bounds, R., and Cowling, V. H. (2011). RAM/Fam103a1 is required for mRNA cap methylation. Mol. Cell 44, 585–596. doi:10.1016/j.molcel.2011.08.041
Grudzien-Nogalska, E., Wu, Y., Jiao, X., Cui, H., Mateyak, M. K., Hart, R. P., et al. (2019). Structural and mechanistic basis of mammalian Nudt12 RNA deNADding. Nat. Chem. Biol. 15, 575–582. doi:10.1038/s41589-019-0293-7
Grzela, R., Piecyk, K., Stankiewicz-Drogon, A., Pietrow, P., Lukaszewicz, M., Kurpiejewski, K., et al. (2023). N2 modified dinucleotide cap analogs as a potent tool for mRNA engineering. RNA 29, 200–216. doi:10.1261/rna.079460.122
Guo, S., Yang, J., Jiang, B., Zhou, N., Ding, H., Zhou, G., et al. (2022). MicroRNA editing patterns in Huntington's disease. Sci. Rep. 12, 3173. doi:10.1038/s41598-022-06970-6
Guo, W., Tan, F., Huai, Q., Wang, Z., Shao, F., Zhang, G., et al. (2021). Comprehensive analysis of PD-L1 expression, immune infiltrates, and m6A RNA methylation regulators in esophageal squamous cell carcinoma. Front. Immunol. 12, 669750. doi:10.3389/fimmu.2021.669750
Hamm, J., and Mattaj, I. W. (1990). Monomethylated cap structures facilitate RNA export from the nucleus. Cell 63, 109–118. doi:10.1016/0092-8674(90)90292-m
Han, H., Fan, G., Song, S., Jiang, Y., Qian, C., Zhang, W., et al. (2021). piRNA-30473 contributes to tumorigenesis and poor prognosis by regulating m6A RNA methylation in DLBCL. Blood 137, 1603–1614. doi:10.1182/blood.2019003764
Han, J., Liu, Q., Zhou, Y., Li, D., and Wang, R. (2023). Landscape of internal N7-methylguanosine of long non-coding RNA modifications in resistant acute myeloid leukemia. BMC Genomics 24, 425. doi:10.1186/s12864-023-09526-8
He, P. C., and He, C. (2021). m6A RNA methylation: from mechanisms to therapeutic potential. EMBO J. 40, e105977. doi:10.15252/embj.2020105977
Hiley, S. L., Jackman, J., Babak, T., Trochesset, M., Morris, Q. D., Phizicky, E., et al. (2005). Detection and discovery of RNA modifications using microarrays. Nucleic Acids Res. 33, e2. doi:10.1093/nar/gni002
Huang, H., Song, R., Wong, J. J.-L., Anggono, V., and Widagdo, J. (2023). The N6-methyladenosine RNA landscape in the aged mouse hippocampus. Aging Cell 22, e13755. doi:10.1111/acel.13755
Huang, H., Weng, H., and Chen, J. (2020). m6A modification in coding and non-coding RNAs: roles and therapeutic implications in cancer. Cancer Cell 37, 270–288. doi:10.1016/j.ccell.2020.02.004
Huang, W., Lan, M.-D., Qi, C.-B., Zheng, S.-J., Wei, S.-Z., Yuan, B.-F., et al. (2016). Formation and determination of the oxidation products of 5-methylcytosine in RNA. Chem. Sci. 7, 5495–5502. doi:10.1039/c6sc01589a
Huber, S. M., van Delft, P., Mendil, L., Bachman, M., Smollett, K., Werner, F., et al. (2015). Formation and abundance of 5-hydroxymethylcytosine in RNA. Chembiochem. 16, 752–755. doi:10.1002/cbic.201500013
Izaurralde, E., Lewis, J., McGuigan, C., Jankowska, M., Darzynkiewicz, E., and Mattaj, I. W. (1994). A nuclear cap binding protein complex involved in pre-mRNA splicing. Cell. 78, 657–668. doi:10.1016/0092-8674(94)90530-4
Jackman, J. E., and Alfonzo, J. D. (2013). Transfer RNA modifications: nature's combinatorial chemistry playground. Wiley Interdiscip. Rev. RNA 4, 35–48. doi:10.1002/wrna.1144
Jain, M., Abu-Shumays, R., Olsen, H. E., and Akeson, M. (2022). Advances in nanopore direct RNA sequencing. Nat. Methods 19, 1160–1164. doi:10.1038/s41592-022-01633-w
Jain, M., Olsen, H. E., Akeson, M., and Abu-Shumays, R. (2021). Adaptation of human ribosomal RNA for nanopore sequencing of canonical and modified nucleotides. Methods Mol. Biol. 2298, 53–74. doi:10.1007/978-1-0716-1374-0_4
Jasinski-Bergner, S., Blümke, J., Wickenhauser, C., and Seliger, B. (2021). Relevance of 2'-O-methylation and pseudouridylation for the malignant melanoma. Cancers (Basel). 13, 1167. doi:10.3390/cancers13051167
Jia, G., Fu, Y., Zhao, X., Dai, Q., Zheng, G., Yang, Y., et al. (2011). N6-methyladenosine in nuclear RNA is a major substrate of the obesity-associated FTO. Nat. Chem. Biol. 7, 885–887. doi:10.1038/nchembio.687
Jiang, X., Liu, B., Nie, Z., Duan, L., Xiong, Q., Jin, Z., et al. (2021). The role of m6A modification in the biological functions and diseases. Signal Transduct. Target Ther. 6 (1), 74. doi:10.1038/s41392-020-00450-x
Jiao, X., Doamekpor, S. K., Bird, J. G., Nickels, B. E., Tong, L., Hart, R. P., et al. (2017). 5' end nicotinamide adenine dinucleotide cap in human cells promotes RNA decay through DXO-mediated deNADding. Cell. 168, 1015–1027. doi:10.1016/j.cell.2017.02.019
Jin, H., Huo, C., Zhou, T., and Xie, S. (2022). m1A RNA modification in gene expression regulation. Genes (Basel) 13, 910. doi:10.3390/genes13050910
Jonkhout, N., Tran, J., Smith, M. A., Schonrock, N., Mattick, J. S., and Novoa, E. M. (2017). The RNA modification landscape in human disease. RNA 23, 1754–1769. doi:10.1261/rna.063503.117
Julius, C., and Yuzenkova, Y. (2017). Bacterial RNA polymerase caps RNA with various cofactors and cell wall precursors. Nucleic Acids Res. 45, 8282–8290. doi:10.1093/nar/gkx452
Kaikkonen, M. U., Lam, M. T. Y., and Glass, C. K. (2011). Non-coding RNAs as regulators of gene expression and epigenetics. Cardiovasc Res. 90, 430–440. doi:10.1093/cvr/cvr097
Karikó, K., Buckstein, M., Ni, H., and Weissman, D. (2005). Suppression of RNA recognition by Toll-like receptors: the impact of nucleoside modification and the evolutionary origin of RNA. Immunity 23, 165–175. doi:10.1016/j.immuni.2005.06.008
Karikó, K., Muramatsu, H., Welsh, F. A., Ludwig, J., Kato, H., Akira, S., et al. (2008). Incorporation of pseudouridine into mRNA yields superior nonimmunogenic vector with increased translational capacity and biological stability. Mol. Ther. 16, 1833–1840. doi:10.1038/mt.2008.200
Ke, S., Pandya-Jones, A., Saito, Y., Fak, J. J., Vågbø, C. B., Geula, S., et al. (2017). m6A mRNA modifications are deposited in nascent pre-mRNA and are not required for splicing but do specify cytoplasmic turnover. Genes Dev. 31, 990–1006. doi:10.1101/gad.301036.117
Khoshnevis, S., Dreggors-Walker, R. E., Marchand, V., Motorin, Y., and Ghalei, H. (2022). Ribosomal RNA 2'- O-methylations regulate translation by impacting ribosome dynamics. Proc. Natl. Acad. Sci. U. S. A. 119, e2117334119. doi:10.1073/pnas.2117334119
Krusnauskas, R., Stakaitis, R., Steponaitis, G., Almstrup, K., and Vaitkiene, P. (2023). Identification and comparison of m6A modifications in glioblastoma non-coding RNAs with MeRIP-seq and Nanopore dRNA-seq. Epigenetics 18, 2163365. doi:10.1080/15592294.2022.2163365
Lackner, D. H., Beilharz, T. H., Marguerat, S., Mata, J., Watt, S., Schubert, F., et al. (2007). A network of multiple regulatory layers shapes gene expression in fission yeast. Mol. Cell. 26, 145–155. doi:10.1016/j.molcel.2007.03.002
Lan, J., Rajan, N., Bizet, M., Penning, A., Singh, N. K., Guallar, D., et al. (2020). Functional role of Tet-mediated RNA hydroxymethylcytosine in mouse ES cells and during differentiation. Nat. Commun. 11, 4956. doi:10.1038/s41467-020-18729-6
Lao, N., and Barron, N. (2023). Enhancing recombinant protein and viral vector production in mammalian cells by targeting the YTHDF readers of N6-methyladenosine in mRNA. Biotechnol. J. 18, e2200451. doi:10.1002/biot.202200451
Lee, J.-H., Wang, R., Xiong, F., Krakowiak, J., Liao, Z., Nguyen, P. T., et al. (2021). Enhancer RNA m6A methylation facilitates transcriptional condensate formation and gene activation. Mol. Cell 81, 3368–3385.e9. doi:10.1016/j.molcel.2021.07.024
Leger, A., Amaral, P. P., Pandolfini, L., Capitanchik, C., Capraro, F., Miano, V., et al. (2021). RNA modifications detection by comparative Nanopore direct RNA sequencing. Nat. Commun. 12, 7198. doi:10.1038/s41467-021-27393-3
Lentini, J. M., Bargabos, R., Chen, C., and Fu, D. (2022). Methyltransferase METTL8 is required for 3-methylcytosine modification in human mitochondrial tRNAs. J. Biol. Chem. 298, 101788. doi:10.1016/j.jbc.2022.101788
Lerner, T., Papavasiliou, F. N., and Pecori, R. (2019). RNA editors, cofactors, and mRNA targets: an overview of the C-to-U RNA editing machinery and its implication in human disease. Genes (Basel) 10, 13. doi:10.3390/genes10010013
Leung, D. W., and Amarasinghe, G. K. (2016). When your cap matters: structural insights into self vs non-self recognition of 5' RNA by immunomodulatory host proteins. Curr. Opin. Struct. Biol. 36, 133–141. doi:10.1016/j.sbi.2016.02.001
Levi, O., and Arava, Y. S. (2021). Pseudouridine-mediated translation control of mRNA by methionine aminoacyl tRNA synthetase. Nucleic Acids Res. 49, 432–443. doi:10.1093/nar/gkaa1178
Li, H., Zhao, J., Deng, H., Zhong, Y., Chen, M., Chi, L., et al. (2023). N6-methyladenosine modification of PLOD2 causes spermatocyte damage in rats with varicocele. Cell Mol. Biol. Lett. 28, 72. doi:10.1186/s11658-023-00475-4
Li, J., Li, Q., Yu, C.-P., Chang, S., Xie, L.-L., and Wang, S. (2021). Genome-wide expression changes mediated by A-to-I RNA editing correlate with hepatic oncogenesis. Transl. Cancer Res. 10, 2725–2737. doi:10.21037/tcr-21-236
Li, M., Tao, Z., Zhao, Y., Li, L., Zheng, J., Li, Z., et al. (2022). 5-methylcytosine RNA methyltransferases and their potential roles in cancer. J. Transl. Med. 20 (1), 214. doi:10.1186/s12967-022-03427-2
Li, P., Liu, Y., Song, R., Zhao, L., Yang, J., Lu, F., et al. (2022). RNA 2 '-O-methyltransferase fibrillarin facilitates virus entry into macrophages through inhibiting type I interferon response. Front. Immunol. 13, 793582. doi:10.3389/fimmu.2022.793582
Li, S., Hu, W., Gong, S., Zhang, P., Cheng, J., Wang, S., et al. (2023). The role of PRRC2B in cerebral vascular remodeling under acute hypoxia in mice. Adv. Sci. (Weinh) 10, e2300892. doi:10.1002/advs.202300892
Li, X., Ma, S., Deng, Y., Yi, P., and Yu, J. (2022). Targeting the RNA m6A modification for cancer immunotherapy. Mol. Cancer 21, 76. doi:10.1186/s12943-022-01558-0
Li, Y., Zhao, K., Wu, R., Wang, J., Wang, Q., Xiong, Q., et al. (2023). Identification of N7-methylguanosine-related lncRNAs for the risk stratification of hepatocellular carcinoma. J. Gastrointest. Oncol. 14, 1392–1411. doi:10.21037/jgo-23-227
Liang, Z., Ye, H., Ma, J., Wei, Z., Wang, Y., Zhang, Y., et al. (2024). m6A-Atlas v2.0: updated resources for unraveling the N6-methyladenosine (m6A) epitranscriptome among multiple species. Nucleic Acids Res. 52, D194–D202. doi:10.1093/nar/gkad691
Lin, T. Y., Smigiel, R., Kuzniewska, B., Chmielewska, J. J., Kosińska, J., Biela, M., et al. (2022). Destabilization of mutated human PUS3 protein causes intellectual disability. Hum. Mutat. 43, 2063–2078. doi:10.1002/humu.24471
Liu, A., and Wang, X. (2022). The pivotal role of chemical modifications in mRNA therapeutics. Front. Cell Dev. Biol. 10, 901510. doi:10.3389/fcell.2022.901510
Liu, C., Sun, H., Yi, Y., Shen, W., Li, K., Xiao, Y., et al. (2023). Absolute quantification of single-base m6A methylation in the mammalian transcriptome using GLORI. Nat. Biotechnol. 41, 355–366. doi:10.1038/s41587-022-01487-9
Liu, H., Begik, O., and Novoa, E. M. (2021). EpiNano: detection of m6A RNA modifications using Oxford nanopore direct RNA sequencing. Methods Mol. Biol. 2298, 31–52. doi:10.1007/978-1-0716-1374-0_3
Liu, H., Jiang, Y., Lu, J., Peng, C., Ling, Z., Chen, Y., et al. (2023). m6A-modification regulated circ-CCT3 acts as the sponge of miR-378a-3p to promote hepatocellular carcinoma progression. Epigenetics 18, 2204772. doi:10.1080/15592294.2023.2204772
Liu, K., He, X., Huang, J., Yu, S., Cui, M., Gao, M., et al. (2023). Short-chain fatty acid-butyric acid ameliorates granulosa cells inflammation through regulating METTL3-mediated N6-methyladenosine modification of FOSL2 in polycystic ovarian syndrome. Clin. Epigenetics 15, 86. doi:10.1186/s13148-023-01487-9
Liu, N., Dai, Q., Zheng, G., He, C., Parisien, M., and Pan, T. (2015). N(6)-methyladenosine-dependent RNA structural switches regulate RNA-protein interactions. Nature. 518, 560–564. doi:10.1038/nature14234
Liu, N., and Pan, T. (2016). N6-methyladenosine–encoded epitranscriptomics. Nat. Struct. Mol. Biol. 23, 98–102. doi:10.1038/nsmb.3162
Liu, Q., Lang, X., and Gregory, R. I. (2021). An informatics pipeline for profiling and annotating RNA modifications. Methods Mol. Biol. 2298, 15–27. doi:10.1007/978-1-0716-1374-0_2
Liu, T., Wei, Q., Jin, J., Luo, Q., Liu, Y., Yang, Y., et al. (2020). The m6A reader YTHDF1 promotes ovarian cancer progression via augmenting EIF3C translation. Nucleic Acids Res. 48, 3816–3831. doi:10.1093/nar/gkaa048
Liu, X.-M., and Qian, S.-B. (2021). Targeted RNA m6A editing using engineered CRISPR-Cas9 conjugates. Methods Mol. Biol. 2298, 399–414. doi:10.1007/978-1-0716-1374-0_23
Liu, Y., Chen, D., Su, R., Chen, W., and Wei, L. (2020). iRNA5hmC: the first predictor to identify RNA 5-hydroxymethylcytosine modifications using machine learning. Front. Bioeng. Biotechnol. 8, 227. doi:10.3389/fbioe.2020.00227
Liu, Y., Yang, Y., Lin, Y., Wei, B., Hu, X., Xu, L., et al. (2023). N6 -methyladenosine-modified circRNA RERE modulates osteoarthritis by regulating β-catenin ubiquitination and degradation. Cell Prolif. 56, e13297. doi:10.1111/cpr.13297
Liu, Z., Lan, P., Liu, T., Liu, X., and Liu, T. (2023). m6Aminer: predicting the m6Am sites on mRNA by fusing multiple sequence-derived features into a CatBoost-based classifier. Int. J. Mol. Sci. 24, 7878. doi:10.3390/ijms24097878
Lo Giudice, C., Tangaro, M. A., Pesole, G., and Picardi, E. (2020). Investigating RNA editing in deep transcriptome datasets with REDItools and REDIportal. Nat. Protoc. 15, 1098–1131. doi:10.1038/s41596-019-0279-7
Luo, Y., Yao, Y., Wu, P., Zi, X., Sun, N., and He, J. (2022). The potential role of N7-methylguanosine (m7G) in cancer. J. Hematol. Oncol. 15, 63. doi:10.1186/s13045-022-01285-5
Ma, C.-J., Ding, J.-H., Ye, T.-T., Yuan, B.-F., and Feng, Y.-Q. (2019). AlkB homologue 1 demethylates N3-methylcytidine in mRNA of mammals. ACS Chem. Biol. 14, 1418–1425. doi:10.1021/acschembio.8b01001
Manfredonia, I., and Incarnato, D. (2021). RNA post-transcriptional modification mapping data analysis using RNA framework. Methods Mol. Biol. 2298, 3–13. doi:10.1007/978-1-0716-1374-0_1
Mann, T. D., Kopel, E., Eisenberg, E., and Levanon, E. Y. (2023). Increased A-to-I RNA editing in atherosclerosis and cardiomyopathies. PLoS Comput. Biol. 19, e1010923. doi:10.1371/journal.pcbi.1010923
Mao, X.-L., Li, Z.-H., Huang, M.-H., Wang, J.-T., Zhou, J.-B., Li, Q.-R., et al. (2021). Mutually exclusive substrate selection strategy by human m3C RNA transferases METTL2A and METTL6. Nucleic Acids Res. 49, 8309–8323. doi:10.1093/nar/gkab603
Marchand, V., Ayadi, L., Bourguignon-Igel, V., Helm, M., and Motorin, Y. (2021). AlkAniline-Seq: a highly sensitive and specific method for simultaneous mapping of 7-methyl-guanosine (m7G) and 3-methyl-cytosine (m3C) in RNAs by high-throughput sequencing. Methods Mol. Biol. 2298, 77–95. doi:10.1007/978-1-0716-1374-0_5
Martinez, N. M., Su, A., Burns, M. C., Nussbacher, J. K., Schaening, C., Sathe, S., et al. (2022). Pseudouridine synthases modify human pre-mRNA co-transcriptionally and affect pre-mRNA processing. Mol. Cell. 82, 645–659.e9. doi:10.1016/j.molcel.2021.12.023
Mauer, J., Sindelar, M., Despic, V., Guez, T., Hawley, B. R., Vasseur, J.-J., et al. (2019). FTO controls reversible m6Am RNA methylation during snRNA biogenesis. Nat. Chem. Biol. 15, 340–347. doi:10.1038/s41589-019-0231-8
McCown, P. J., Ruszkowska, A., Kunkler, C. N., Breger, K., Hulewicz, J. P., Wang, M. C., et al. (2020). Naturally occurring modified ribonucleosides. Wiley Interdiscip. Rev. RNA 11, e1595. doi:10.1002/wrna.1595
M. McMahon (2021). RNA modifications: methods and protocols, methods in molecular biology (Springer Nature), 2298. doi:10.1007/978-1-0716-1374-0
Miao, Z., Xin, N., Wei, B., Hua, X., Zhang, G., Leng, C., et al. (2016). 5-hydroxymethylcytosine is detected in RNA from mouse brain tissues. Brain Res. 1642, 546–552. doi:10.1016/j.brainres.2016.04.055
Mishima, E., Jinno, D., Akiyama, Y., Itoh, K., Nankumo, S., Shima, H., et al. (2015). Immuno-Northern blotting: detection of RNA modifications by using antibodies against modified nucleosides. PLoS One 10, e0143756. doi:10.1371/journal.pone.0143756
Monecke, T., Dickmanns, A., and Ficner, R. (2009). Structural basis for m7G-cap hypermethylation of small nuclear, small nucleolar and telomerase RNA by the dimethyltransferase TGS1. Nucleic Acids Res. 37, 3865–3877. doi:10.1093/nar/gkp249
Mukherjee, C., Bakthavachalu, B., and Schoenberg, D. R. (2014). The cytoplasmic capping complex assembles on adapter protein nck1 bound to proline-rich C-terminus of Mammalian capping enzyme. PloS Biol. 12, e1001933. doi:10.1371/journal.pbio.1001933
Mukhopadhyay, D., Anant, S., Lee, R. M., Kennedy, S., Viskochil, D., and Davidson, N. O. (2002). C→U editing of neurofibromatosis 1 mRNA occurs in tumors that express both the type II transcript and apobec-1, the catalytic subunit of the apolipoprotein B mRNA-editing enzyme. Am. J. Hum. Genet. 70, 38–50. doi:10.1086/337952
Nabeel-Shah, S., Pu, S., Burke, G. L., Ahmed, N., Braunschweig, U., Farhangmehr, S., et al. (2024). Recruitment of the m6A/m6Am demethylase FTO to target RNAs by the telomeric zinc finger protein ZBTB48. Genome Biol. 25, 246. doi:10.1186/s13059-024-03392-7
Nance, K. D., and Meier, J. L. (2021). Modifications in an Emergency: the role of N1-methylpseudouridine in COVID-19 vaccines. ACS Cent. Sci. 7, 748–756. doi:10.1021/acscentsci.1c00197
Ni, P., Pan, K., and Zhao, B. (2023). Influence of N6-methyladenosine (m6A) modification on cell phenotype in Alzheimer's disease. PLoS One 18, e0289068. doi:10.1371/journal.pone.0289068
Nie, F., Tang, Q., Liu, Y., Qin, H., Liu, S., Wu, M., et al. (2022). RNAME: a comprehensive database of RNA modification enzymes. Comput. Struct. Biotechnol. J. 20, 6244–6249. doi:10.1016/j.csbj.2022.11.022
Nombela, P., Miguel-López, B., and Blanco, S. (2021). The role of m6A, m5C and Ψ RNA modifications in cancer: novel therapeutic opportunities. Mol. Cancer 20, 18. doi:10.1186/s12943-020-01263-w
Oh, J., Kimoto, M., Xu, H., Chong, J., Hirao, I., and Wang, D. (2023). Structural basis of transcription recognition of a hydrophobic unnatural base pair by T7 RNA polymerase. Nat. Commun. 14, 195. doi:10.1038/s41467-022-35755-8
Olazagoitia-Garmendia, A., and Castellanos-Rubio, A. (2021). Relative quantification of residue-Specific m6A RNA methylation using m6A-RT-QPCR. Methods Mol. Biol. 2298, 185–195. doi:10.1007/978-1-0716-1374-0_12
Ontiveros, R. J., Stoute, J., and Liu, K. F. (2019). The chemical diversity of RNA modifications. Biochem. J. 476, 1227–1245. doi:10.1042/BCJ20180445
Otsuka, Y., Kedersha, N. L., and Schoenberg, D. R. (2009). Identification of a cytoplasmic complex that adds a cap onto 5'-monophosphate RNA. Mol. Cell Biol. 29, 2155–2167. doi:10.1128/MCB.01325-08
Pecori, R., Di Giorgio, S., Paulo Lorenzo, J., and Papavasiliou, F. N. (2022). Functions and consequences of AID/APOBEC-mediated DNA and RNA deamination. Nat. Rev. Genet. 23, 505–518. doi:10.1038/s41576-022-00459-8
Pederiva, C., Trevisan, D. M., Peirasmaki, D., Chen, S., Savage, S. A., Larsson, O., et al. (2023). Control of protein synthesis through mRNA pseudouridylation by dyskerin. Sci. Adv. 9, eadg1805. doi:10.1126/sciadv.adg1805
Pelletier, J., Schmeing, T. M., and Sonenberg, N. (2021). The multifaceted eukaryotic cap structure. Wiley Interdiscip. Rev. RNA 12 (2), e1636. doi:10.1002/wrna.1636
Pope, S. D., and Medzhitov, R. (2018). Emerging principles of gene expression programs and their regulation. Mol. Cell. 71, 389–397. doi:10.1016/j.molcel.2018.07.017
Primac, I., Penning, A., and Fuks, F. (2022). Cancer epitranscriptomics in a nutshell. Curr. Opin. Genet. Dev. 75, 101924. doi:10.1016/j.gde.2022.101924
Qiu, L., Jing, Q., Li, Y., and Han, J. (2023). RNA modification: mechanisms and therapeutic targets. Mol. Biomed. 4, 25. doi:10.1186/s43556-023-00139-x
Qu, J., Yan, H., Hou, Y., Cao, W., Liu, Y., Zhang, E., et al. (2022). RNA demethylase ALKBH5 in cancer: from mechanisms to therapeutic potential. J. Hematol. Oncol. 5, 8. doi:10.1186/s13045-022-01224-4
Richard Boland, C. (2017). Non-coding RNA: it's not junk. Dig. Dis. Sci. 62, 1107–1109. doi:10.1007/s10620-017-4506-1
Rimbach, K., Kaiser, S., Helm, M., Dalpke, A. H., and Eigenbrod, T. (2015). 2'-O-Methylation within bacterial RNA acts as suppressor of TLR7/TLR8 activation in human innate immune cells. J. Innate Immun. 7, 482–493. doi:10.1159/000375460
Rimer, J. M., Lee, J., Holley, C. L., Crowder, R. J., Chen, D. L., Hanson, P. I., et al. (2018). Long-range function of secreted small nucleolar RNAs that direct 2'- O-methylation. J. Biol. Chem. 293, 13284–13296. doi:10.1074/jbc.RA118.003410
Rottman, F., Shatkin, A. J., and Perry, R. P. (1974). Sequences containing methylated nucleotides at the 5' termini of messenger RNAs: possible implications for processing. Cell 3, 197–199. doi:10.1016/0092-8674(74)90131-7
Roundtree, I. A., Evans, M. E., Pan, T., and He, C. (2017). Dynamic RNA modifications in gene expression regulation. Cell 169, 1187–1200. doi:10.1016/j.cell.2017.05.045
Satterwhite, E. R., and Mansfield, K. D. (2022). RNA methyltransferase METTL16: targets and function. Wiley Interdiscip. Rev. RNA 13, e1681. doi:10.1002/wrna.1681
Schoenberg, D. R., and Maquat, L. E. (2012). Regulation of cytoplasmic mRNA decay. Nat. Rev. Genet. 13, 246–259. doi:10.1038/nrg3160
Schwartz, S., Bernstein, D. A., Mumbach, M. R., Jovanovic, M., Herbst, R. H., León-Ricardo, B. X., et al. (2014). Transcriptome-wide mapping reveals widespread dynamic-regulated pseudouridylation of ncRNA and mRNA. Cell 159, 148–162. doi:10.1016/j.cell.2014.08.028
Sendinc, E., Valle-Garcia, D., Dhall, A., Chen, H., Henriques, T., Navarrete-Perea, J., et al. (2019). PCIF1 catalyzes m6Am mRNA methylation to regulate gene expression. Mol. Cell. 75, 620–630. doi:10.1016/j.molcel.2019.05.030
Shaheen, R., Tasak, M., Maddirevula, S., Abdel-Salam, G. M. H., Sayed, I. S. M., Alazami, A. M., et al. (2019). PUS7 mutations impair pseudouridylation in humans and cause intellectual disability and microcephaly. Hum. Genet. 138, 231–239. doi:10.1007/s00439-019-01980-3
Sharma, S., Patnaik, S. K., Kemer, Z., and Baysal, B. E. (2017). Transient overexpression of exogenous APOBEC3A causes C-to-U RNA editing of thousands of genes. RNA Biol. 14, 603–610. doi:10.1080/15476286.2016.1184387
Shatkin, A. J. (1976). Capping of eucaryotic mRNAs. Cell 9, 645–653. doi:10.1016/0092-8674(76)90128-8
Shen, D., Wang, B., Gao, Y., Zhao, L., Bi, Y., Zhang, J., et al. (2022). Detailed resume of RNA m6A demethylases. Acta Pharm. Sin. B 12, 2193–2205. doi:10.1016/j.apsb.2022.01.003
Shen, Q., Zhang, Q., Shi, Y., Shi, Q., Jiang, Y., Gu, Y., et al. (2018). Tet2 promotes pathogen infection-induced myelopoiesis through mRNA oxidation. Nature 554, 123–127. doi:10.1038/nature25434
Slotkin, W., and Nishikura, K. (2013). Adenosine-to-inosine RNA editing and human disease. Genome Med. 5, 105. doi:10.1186/gm508
Song, B., Wang, X., Liang, Z., Ma, J., Huang, D., Wang, Y., et al. (2023). RMDisease V2.0: an updated database of genetic variants that affect RNA modifications with disease and trait implication. Nucleic Acids Res. 51, D1388–D1396. doi:10.1093/nar/gkac750
Song, H., Zhang, J., Liu, B., Xu, J., Cai, B., Yang, H., et al. (2022). Biological roles of RNA m5C modification and its implications in cancer immunotherapy. Biomark. Res. 10, 15. doi:10.1186/s40364-022-00362-8
Song, J., Dong, L., Sun, H., Luo, N., Huang, Q., Li, K., et al. (2023). CRISPR-free, programmable RNA pseudouridylation to suppress premature termination codons. Mol. Cell 83, 139–155.e9. doi:10.1016/j.molcel.2022.11.011
Song, J., and Yi, C. (2017). Chemical modifications to RNA: a new layer of gene expression regulation. ACS Chem. Biol. 12, 316–325. doi:10.1021/acschembio.6b00960
Sun, H., Li, K., Zhang, X., Liu, J., Zhang, M., Meng, H., et al. (2021). m6Am-seq reveals the dynamic m6Am methylation in the human transcriptome. Nat. Commun. 12, 4778. doi:10.1038/s41467-021-25105-5
Takahashi, K., Shigeyasu, K., Kondo, Y., Gotoh, K., Yano, S., Umeda, Y., et al. (2023). RNA editing is a valuable biomarker for predicting carcinogenesis in ulcerative colitis. J. Crohns Colitis 17, 754–766. doi:10.1093/ecco-jcc/jjac186
Talic, E. S., Wooten, A., Zeczycki, T. N., and Mansfield, K. D. (2023). RNA Methyltransferase METTL16's protein domains have differential functional effects on cell processes. Curr. Issues Mol. Biol. 45, 5460–5480. doi:10.3390/cimb45070346
Tegowski, M., Flamand, M. N., and Meyer, K. D. (2022). scDART-seq reveals distinct m6A signatures and mRNA methylation heterogeneity in single cells. Mol. Cell. 82, 868–878.e10. doi:10.1016/j.molcel.2021.12.038
Tóth, B. B., Arianti, R., Shaw, A., Vámos, A., Veréb, Z., Póliska, S., et al. (2020). FTO intronic SNP strongly influences human neck adipocyte browning determined by tissue and PPARγ specific regulation: a transcriptome analysis. Cells 9, 987. doi:10.3390/cells9040987
Trotman, J. B., Giltmier, A. J., Mukherjee, C., and Schoenberg, D. R. (2017). RNA guanine-7 methyltransferase catalyzes the methylation of cytoplasmically recapped RNAs. Nucleic Acid. Res. 45, 10726–10739. doi:10.1093/nar/gkx801
Tsagkogeorga, G., Santos-Rosa, H., Alendar, A., Leggate, D., Rausch, O., Kouzarides, T., et al. (2022). Predicting genes associated with RNA methylation pathways using machine learning. Commun. Biol. 5, 868. doi:10.1038/s42003-022-03821-y
Vaid, R., Mendez, A., Thombare, K., Burgos-Panadero, R., Robinot, R., Fonseca, B. F., et al. (2023). Global loss of cellular m6A RNA methylation following infection with different SARS-CoV-2 variants. Genome Res. 33, 299–313. doi:10.1101/gr.276407.121
Vámos, A., Arianti, R., Vinnai, B. Á., Alrifai, R., Shaw, A., Póliska, S., et al. (2023). Human abdominal subcutaneous-derived active beige adipocytes carrying FTO rs1421085 obesity-risk alleles exert lower thermogenic capacity. Front. Cell Dev. Biol. 11, 1155673. doi:10.3389/fcell.2023.1155673
Vu, L. T., and Tsukahara, T. (2017). C-to-U editing and site-directed RNA editing for the correction of genetic mutations. Biosci. Trends 11, 243–253. doi:10.5582/bst.2017.01049
Wang, C., Hou, X., Guan, Q., Zhou, H., Zhou, L., Liu, L., et al. (2023). RNA modification in cardiovascular disease: implications for therapeutic interventions. Signal Transduct. Target Ther. 8, 412. doi:10.1038/s41392-023-01638-7
Wang, J., Alvin Chew, B. L., Lai, Y., Dong, H., Xu, L., Balamkundu, S., et al. (2019). Quantifying the RNA cap epitranscriptome reveals novel caps in cellular and viral RNA. Nucleic Acids Res. 47 (20), e130. doi:10.1093/nar/gkz751
Wang, J., Wu, L., Pu, X., Liu, B., and Cao, M. (2023b). Evidence supporting that C-to-U RNA editing is the major force that drives SARS-CoV-2 evolution. J. Mol. Evol. 91, 214–224. doi:10.1007/s00239-023-10097-1
Wang, J., Yu, H., Dong, W., Zhang, C., Hu, M., Ma, W., et al. (2023a). N6-Methyladenosine-Mediated Up-Regulation of FZD10 Regulates Liver Cancer Stem Cells' Properties and Lenvatinib Resistance Through WNT/β-Catenin and Hippo Signaling Pathways. Gastroenterology 164, 990–1005. doi:10.1053/j.gastro.2023.01.041
Wang, L., Wang, S., Wu, L., Li, W., Bray, W., Clark, A. E., et al. (2023). PCIF1-mediated deposition of 5'-cap N6,2'- O-dimethyladenosine in ACE2 and TMPRSS2 mRNA regulates susceptibility to SARS-CoV-2 infection. Proc. Natl. Acad. Sci. U. S. A. 120, e2210361120. doi:10.1073/pnas.2210361120
Wang, M., Yu, F., Wu, W., Zhang, Y., Chang, W., Ponnusamy, M., et al. (2017). Circular RNAs: a novel type of non-coding RNA and their potential implications in antiviral immunity. Int. J. Biol. Sci. 13, 1497–1506. doi:10.7150/ijbs.22531
Wang, X., Lu, Z., Gomez, A., Hon, G. C., Yue, Y., Han, D., et al. (2014). N6-methyladenosine-dependent regulation of messenger RNA stability. Nature 505, 117–120. doi:10.1038/nature12730
Wang, X., Zhao, B. S., Roundtree, I. A., Lu, Z., Han, D., Ma, H., et al. (2015). N(6)-methyladenosine modulates messenger RNA translation efficiency. Cell 161, 1388–1399. doi:10.1016/j.cell.2015.05.014
Wang, Y., Wei, J., Feng, L., Li, O., Huang, L., Zhou, S., et al. (2023). Aberrant m5C hypermethylation mediates intrinsic resistance to gefitinib through NSUN2/YBX1/QSOX1 axis in EGFR-mutant non-small-cell lung cancer. Mol. Cancer 22, 81. doi:10.1186/s12943-023-01780-4
Warminski, M., Mamot, A., Depaix, A., Kowalska, J., and Jemielity, J. (2023). Chemical modifications of mRNA ends for therapeutic applications. Acc. Chem. Res. 56, 2814–2826. doi:10.1021/acs.accounts.3c00442
Wei, C., Gershowitz, A., and Moss, B. (1975). N6, O2'-dimethyladenosine a novel methylated ribonucleoside next to the 5' terminal of animal cell and virus mRNAs. Nature 257, 251–253. doi:10.1038/257251a0
Werner, M., Purta, E., Kaminska, K. H., Cymerman, I. A., Campbell, D. A., Mittra, B., et al. (2011). 2'-O-ribose methylation of cap2 in human: function and evolution in a horizontally mobile family. Nucleic Acids Res. 39 (11), 4756–4768. doi:10.1093/nar/gkr038
Wiedermannová, J., Julius, C., and Yuzenkova, Y. (2021). The expanding field of non-canonical RNA capping: new enzymes and mechanisms. R. Soc. Open Sci. 8 (5), 201979. doi:10.1098/rsos.201979
Wiener, D., and Schwartz, S. (2021). The epitranscriptome beyond m6A. Nat. Rev. Genet. 22 (2), 119–131. doi:10.1038/s41576-020-00295-8
Williams, G. D., Gokhale, N. S., Snider, D. L., and Horner, S. M. (2020). The mRNA cap 2'- O-methyltransferase CMTR1 regulates the expression of certain interferon-stimulated genes. mSphere 5, e00202-20–e00220. doi:10.1128/mSphere.00202-20
Wu, S., Xue, Q., Qin, X., Wu, X., Kim, P., Chyr, J., et al. (2023). The potential regulation of A-to-I RNA editing on genes in Parkinson's disease. Genes (Basel) 14, 919. doi:10.3390/genes14040919
Wu, Y., Hao, S., Xu, X., Dong, G., Ouyang, W., Liu, C., et al. (2023a). A novel computational method enables RNA editome profiling during human hematopoiesis from scRNA-seq data. Sci. Rep. 13, 10335. doi:10.1038/s41598-023-37325-4
Wu, Y., Pu, X., Wu, S., Zhang, Y., Fu, S., Tang, H., et al. (2023b). PCIF1, the only methyltransferase of N6,2-O-dimethyladenosine. Cancer Cell Int. 23, 226. doi:10.1186/s12935-023-03066-7
Wu, Z., Lin, Y., and Wei, N. (2023). N6-methyladenosine-modified HOTAIRM1 promotes vasculogenic mimicry formation in glioma. Cancer Sci. 114, 129–141. doi:10.1111/cas.15578
Wulff, T. F., Argüello, R. J., Jordàn, M. M., Frigolé, H. R., Hauquier, G., Filonava, L., et al. (2017). Detection of a subset of posttranscriptional transfer RNA modifications in vivo with a restriction fragment length polymorphism-based method. Biochemistry 56, 4029–4038. doi:10.1021/acs.biochem.7b00324
Xiao, Y.-L., Liu, S., Ge, R., Wu, Y., He, C., Chen, M., et al. (2023). Transcriptome-wide profiling and quantification of N6-methyladenosine by enzyme-assisted adenosine deamination. Nat. Biotechnol. 41, 993–1003. doi:10.1038/s41587-022-01587-6
Xiong, X., Yi, C., and Peng, J. (2017). Epitranscriptomics: toward a better understanding of RNA modifications. Genomics Proteomics Bioinforma. 15, 147–153. doi:10.1016/j.gpb.2017.03.003
Xu, G., and Zhang, J. (2014). Human coding RNA editing is generally nonadaptive. Proc. Natl. Acad. Sci. U. S. A. 111, 3769–3774. doi:10.1073/pnas.1321745111
Xu, H., Chen, G., Zhou, J., Zhou, X., Wang, P., Chen, C., et al. (2023). Identification and validation of m6A RNA regulatory network in pulpitis. BMC Oral Health 23, 878. doi:10.1186/s12903-023-03578-8
Xu, L., Liu, X., Sheng, N., Oo, K. S., Liang, J., Chionh, Y. H., et al. (2017). Three distinct 3-methylcytidine (m3C) methyltransferases modify tRNA and mRNA in mice and humans. J. Biol. Chem. 292, 14695–14703. doi:10.1074/jbc.M117.798298
Xuan, J., Chen, L., Chen, Z., Pang, J., Huang, J., Lin, J., et al. (2024). RMBase v3.0: decode the landscape, mechanisms and functions of RNA modifications. Nucleic Acids Res. 52, D273–D284. doi:10.1093/nar/gkad1070
Yang, R., Liang, X., Wang, H., Guo, M., Shen, H., Shi, Y., et al. (2021). The RNA methyltransferase NSUN6 suppresses pancreatic cancer development by regulating cell proliferation. EBioMedicine 63, 103195. doi:10.1016/j.ebiom.2020.103195
Yang, X., Yang, Y., Sun, B.-F., Chen, Y.-S., Xu, J.-W., Lai, W.-Y., et al. (2017). 5-methylcytosine promotes mRNA export - NSUN2 as the methyltransferase and ALYREF as an m5C reader. Cell Res. 27, 606–625. doi:10.1038/cr.2017.55
Yang, Y., Okada, S., and Sakurai, M. (2021). Adenosine-to-inosine RNA editing in neurological development and disease. RNA Biol. 18, 999–1013. doi:10.1080/15476286.2020.1867797
You, X.-J., and Yuan, B.-F. (2021). Detecting Internal N7-methylguanosine mRNA modifications by differential enzymatic digestion coupled with mass spectrometry analysis. Methods Mol. Biol. 2298, 247–259. doi:10.1007/978-1-0716-1374-0_16
Yu, D., Pan, M., Li, Y., Lu, T., Wang, Z., Liu, C., et al. (2022). RNA N6-methyladenosine reader IGF2BP2 promotes lymphatic metastasis and epithelial-mesenchymal transition of head and neck squamous carcinoma cells via stabilizing slug mRNA in an m6A-dependent manner. J. Exp. Clin. Cancer Res. 41, 6. doi:10.1186/s13046-021-02212-1
Zhang, M., Jiang, Z., Ma, Y., Liu, W., Zhuang, Y., Lu, B., et al. (2023b). Quantitative profiling of pseudouridylation landscape in the human transcriptome. Nat. Chem. Biol. 19, 1185–1195. doi:10.1038/s41589-023-01304-7
Zhang, M., Xiao, Y., Jiang, Z., and Yi, C. (2023a). Quantifying m6A and Ψ modifications in the transcriptome via chemical-assisted approaches. Acc. Chem. Res. 56, 2980–2991. doi:10.1021/acs.accounts.3c00436
Zhang, N., Shi, S., Yuan, X., Ni, W., Wang, X., Yoo, B., et al. (2021). A general LC-MS-based method for direct and de novo sequencing of RNA mixtures containing both canonical and modified nucleotides. Methods Mol. Biol. 2298, 261–277. doi:10.1007/978-1-0716-1374-0_17
Zhang, W., and Pan, T. (2022). Pseudouridine RNA modification detection and quantification by RT-PCR. Methods 203, 1–4. doi:10.1016/j.ymeth.2021.05.010
Zhang, Y., Zhang, L.-S., Dai, Q., Chen, P., Lu, M., Kairis, E. L., et al. (2022). 5-methylcytosine (m5C) RNA modification controls the innate immune response to virus infection by regulating type I interferons. Proc. Natl. Acad. Sci. U. S. A. 119, e2123338119. doi:10.1073/pnas.2123338119
Zhang, Y., Zhang, W., Zhao, J., Ito, T., Jin, J., Aparicio, A. O., et al. (2023). m6A RNA modification regulates innate lymphoid cell responses in a lineage-specific manner. Nat. Immunol. 24, 1256–1264. doi:10.1038/s41590-023-01548-4
Zhao, B. S., and He, C. (2015). Pseudouridine in a new era of RNA modifications. Cell Res. 25, 153–154. doi:10.1038/cr.2014.143
Zhao, B. S., Roundtree, I. A., and He, C. (2017). Post-transcriptional gene regulation by mRNA modifications. Nat. Rev. Mol. Cell Biol. 18, 31–42. doi:10.1038/nrm.2016.132
Zhao, X., Zhang, Y., Hang, D., Meng, J., and Wei, Z. (2022). Detecting RNA modification using direct RNA sequencing: a systematic review. Comput. Struct. Biotechnol. J. 20, 5740–5749. doi:10.1016/j.csbj.2022.10.023
Zhao, Z., Qing, Y., Dong, L., Han, L., Wu, D., Li, Y., et al. (2023). QKI shuttles internal m7G-modified transcripts into stress granules and modulates mRNA metabolism. Cell 186, 3208–3226.e27. doi:10.1016/j.cell.2023.05.047
Zheng, G., Dahl, J. A., Niu, Y., Fedorcsak, P., Huang, C.-M., Li, C. J., et al. (2013). ALKBH5 is a mammalian RNA demethylase that impacts RNA metabolism and mouse fertility. Mol. Cell. 49, 18–29. doi:10.1016/j.molcel.2012.10.015
Zhou, K., Yang, J., Li, X., Xiong, W., Zhang, P., and Zhang, X. (2022). N7-methylguanosine regulatory genes profoundly affect the prognosis, progression, and antitumor immune response of hepatocellular carcinoma. Front. Surg. 9, 893977. doi:10.3389/fsurg.2022.893977
Zhou, Z., Lv, J., Yu, H., Han, J., Yang, X., Feng, D., et al. (2020). Mechanism of RNA modification N6-methyladenosine in human cancer. Mol. Cancer 19, 104. doi:10.1186/s12943-020-01216-3
Zinshteyn, B., and Nishikura, K. (2009). Adenosine-to-inosine RNA editing. Wiley Interdiscip. Rev. Syst. Biol. Med. 1, 202–209. doi:10.1002/wsbm.10
Keywords: RNA, RNA modification, epitranscriptomics, gene expression, RNA editing, therapeutic development
Citation: Artika IM, Arianti R, Demény MÁ and Kristóf E (2025) RNA modifications and their role in gene expression. Front. Mol. Biosci. 12:1537861. doi: 10.3389/fmolb.2025.1537861
Received: 01 December 2024; Accepted: 02 April 2025;
Published: 25 April 2025.
Edited by:
Jiao Li, Sichuan University, ChinaReviewed by:
Daniel L. Kiss, Houston Methodist Research Institute, United StatesMassimo Bogliolo, Autonomous University of Barcelona, Spain
Copyright © 2025 Artika, Arianti, Demény and Kristóf. This is an open-access article distributed under the terms of the Creative Commons Attribution License (CC BY). The use, distribution or reproduction in other forums is permitted, provided the original author(s) and the copyright owner(s) are credited and that the original publication in this journal is cited, in accordance with accepted academic practice. No use, distribution or reproduction is permitted which does not comply with these terms.
*Correspondence: I. Made Artika, aW1hcnRAYXBwcy5pcGIuYWMuaWQ=; Endre Kristóf, a3Jpc3RvZi5lbmRyZUBtZWQudW5pZGViLmh1
†These authors have contributed equally to this work and share last authorship