- 1Department of Physical Education, Xidian University, Xi’an, Shaanxi, China
- 2School of Marxism, Xi’an Jiaotong University, Xi’an, Shaanxi, China
The intricate interplay between physical training and non-coding RNAs, specifically microRNAs (miRNAs) and long non-coding RNAs (lncRNAs), has attracted considerable attention in understanding physiological adaptations and pathological conditions. Both miRNAs and lncRNAs are essential modulators of gene expression, influencing various cellular processes, including those related to muscle metabolism, inflammation, and recovery from injury. This review investigates the bifunctional role of miRNAs and lncRNAs in response to physical training, highlighting their involvement in muscle hypertrophy, endurance adaptations, and the modulation of inflammatory pathways. Additionally, we examine how pathological conditions, such as cardiovascular disease, heart failure, can alter the expression profiles of miRNAs and lncRNAs, potentially disrupting the beneficial effects of physical training. The crosstalk between these non-coding RNAs under physiological and pathological states underscores their potential as biomarkers for assessing training responses and therapeutic targets for enhancing recovery and performance. Understanding these interactions may pave the way for novel interventions to optimize health outcomes through tailored physical training programs.
1 Introduction
It is widely acknowledged that physical activity has numerous beneficial impacts on health, particularly in preventing and managing long-term conditions such as cancer, metabolic disorders, neurological diseases, musculoskeletal disorders, and cardiovascular disease (Dhuli et al., 2022). This is because physical activity is essential for overall wellbeing. Muscle regeneration depends on satellite cells, diminutive adult stem cells proficient in re-entering the cell cycle after injury or growth stimuli. These cells migrate and proliferate extensively for muscle restoration. The destiny of satellite cells is governed by both intrinsic and extrinsic factors, which may be compromised by ageing, genetic myopathy, and significant muscle atrophy. Surgery is frequently employed in clinical practice; however, its substantial expense and significant dissatisfaction may deter certain patients. Tissue engineering (TE) is currently regarded as an innovative approach for the management of myopathies and the enhancement of regeneration (Ganassi et al., 2022). In addition to causing significant disruptions in various organs and tissues, physical training also causes disturbances in the homeostasis of the body as a whole. The contraction of myofibers is the first step in the movement process, which leads to metabolic and morphological changes in skeletal muscle. Enhanced fuel and oxygen are supplied to the body by the cardiovascular, respiratory, neural, and endocrine systems responsible for supporting activity (Plaza-Diaz et al., 2022; Qiu et al., 2023; Chen et al., 2022).
Postnatal development of skeletal muscle in humans primarily results from the hypertrophy of preexisting muscle fibers, rather than from cellular proliferation. Historical evidence of muscle fiber division or bifurcation originates from the 19th century, and contemporary research corroborates this with findings from regenerating and stress-induced muscle fibres. Nonetheless, there exists circumstantial evidence of hypertrophy and hyperplasia induced by exercise, primarily derived from subsequent observations of variations in fibre size or quantity. Researchers have created three animal models to replicate exercise-induced muscle hypertrophy in humans (Snijders et al., 2015). The ageing population poses a global challenge to governments, as age-associated ailments such as sarcopenia become more widespread. Sarcopenia is a clinical syndrome marked by the age-related decline in skeletal muscle mass, strength, and function, frequently observed in elderly patients with chronic illnesses. Alterations in lean mass are pivotal factors influencing the progression of cardiovascular disease. Sarcopenia may lead to impaired physical function and diminished cardiopulmonary function in elderly patients with cardiovascular diseases via shared pathogenic mechanisms. Furthermore, cardiac modifications in the left ventricle, especially among elderly individuals with skeletal muscle sarcopenia, have been thoroughly investigated for decades (He et al., 2021). There is an increasing interest in comprehending the myocardial alterations that accompany these changes, resulting in a revitalised emphasis on the cardiac-skeletal muscle axis. Interestingly, sarcopenia, a condition marked by age-associated muscle deterioration, is also linked to Type 2 Diabetes Mellitus (T2DM) and dementia. It elevates Insulin resistance and the susceptibility to type 2 diabetes mellitus, resulting in cognitive deterioration and an increased risk of dementia. Sarcopenia may serve as a mediator between these conditions, intensifying cognitive decline in elderly individuals. Recent studies have highlighted the correlation between sarcopenia and dementia, rendering it a critical area of research. Comprehending these associations can facilitate the formulation of strategies to promote healthy ageing and avert dementia in elderly individuals with T2DM. Further investigation is necessary to improve understanding of these complex relationships (Liu et al., 2024).
It has been established that non-coding RNAs, also known as ncRNAs, have the potential to act as mediators in the physiological processes that are associated with exercise adaptation. In addition to small nucleolar RNA, ribosomal RNA, circular RNA, transfer RNA, long non-coding RNA, and microRNA, the comprehensive catalog of non-coding RNAs also includes many other types of RNA (Wadley et al., 1985; Piergentili and Sechi, 2024). Throughout the 1950s, the first noncoding RNAs were found. lncRNAs, miRNAs, small interfering RNAs (siRNAs), and PIWI-interacting RNAs (piRNAs) were added to the repertoire of recognized non-coding RNAs as a result of this research. Additionally, the research identified multiple RNA categories, including snoRNAs that are involved in the processing and splicing of rRNA (Burgos et al., 2021; Chen and Kim, 2024). In light of the rapidly growing amount of data, this study investigates the synthesis and function of microRNAs (miRNAs) and long non-coding RNAs (lncRNAs) in skeletal muscle. Moreover, Physiological enhancements resulting from exercise appear to influence the expression of diverse miRNAs, encompassing myo-miRNAs, c-miRNAs, and lncRNAs. Consequently, the examination of exercise-induced modifications in miRNAs and lncRNAs expression in cardiovascular disease and heart failure may yield novel insights into the epigenetic modifications prompted by exercise. However, MiRNAs and lncRNAs may contribute to health-related enhancements; however, their molecular mechanisms are inadequately comprehended in individuals with cardiovascular disease and heart failure. Prior studies have concentrated on identifying myo-c-miRNAs or lncRNAs that exhibit differential expression in reaction to both acute and chronic physical exercise in human. Furthermore, investigations concentrated on enhancing health outcomes for patients, especially those impacted by cardiovascular disease and heart failure (Afzal et al., 2024). This review aims to examine the impact of exercise on the expression of myo- or c-miRNAs and lncRNAs in cardiovascular disease and heart failure, highlighting potential molecular pathways for improved health outcomes (Figure 1).
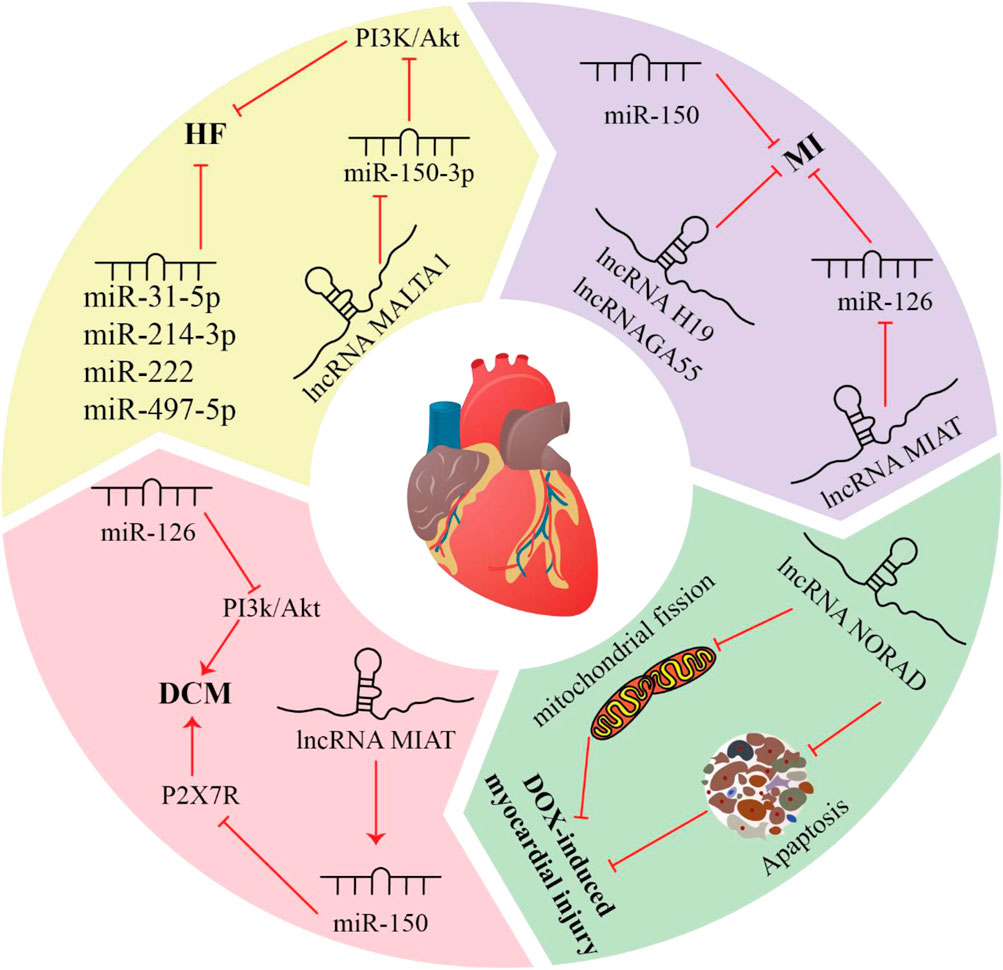
Figure 1. Exercise-induced miRNAs and lncRNAs are crucial in the progression of various cardiovascular diseases, including hypertension, dilated cardiomyopathy, atherosclerotic vascular diseases, myocardial ischemia-reperfusion injury, myocardial infarction, heart failure, and doxorubicin-induced cardiomyopathy.
2 Biogenesis and mechanism of action ncRNAs
MicroRNAs, small and plentiful molecules with seed sequences, facilitate target recognition, improving interaction with miRNA processing machinery for varied identification and prediction (Desvignes et al., 2015). MicroRNAs are essential in modulating adaptive processes, including muscle atrophy, cardiovascular disease, and aging (O'Brien et al., 2018; Seo et al., 2024). Recent studies indicate that lncRNAs are critical to regulating locus-specific gene expression by functioning as cofactors, competitors, or decoys for RNA-binding proteins and microRNAs (Zhang et al., 2019).
Other ncRNAs subtypes include cirRNA, siRNA, tiRNA, and piRNA.Circular RNAs, which are non-coding RNAs, form closed loops in mammals and regulate gene expression via miRNA sponges or RNA-binding proteins, thus influencing gene transcription (Greene et al., 2017). Small interfering RNAs, encompassing natural antisense, trans-acting, and heterochromatic siRNAs, impede protein synthesis by cleaving target mRNA at a designated site within the siRNA-RISC complex (Deng et al., 2018). Transfer RNA-derived small RNAs (tsRNAs), a novel class of regulatory non-coding RNAs, range from 15 to 42 nucleotides in length and fulfill diverse biological functions through protein interactions, translation inhibition, and gene expression regulation (Zong et al., 2021). PiRNAs, found in germline DNA, inhibit transposable elements. PIWI proteins, integrated into Argonaute proteins, cleave target mRNAs while maintaining the integrity of germline DNA (Iwasaki et al., 2015) (Figure 2).
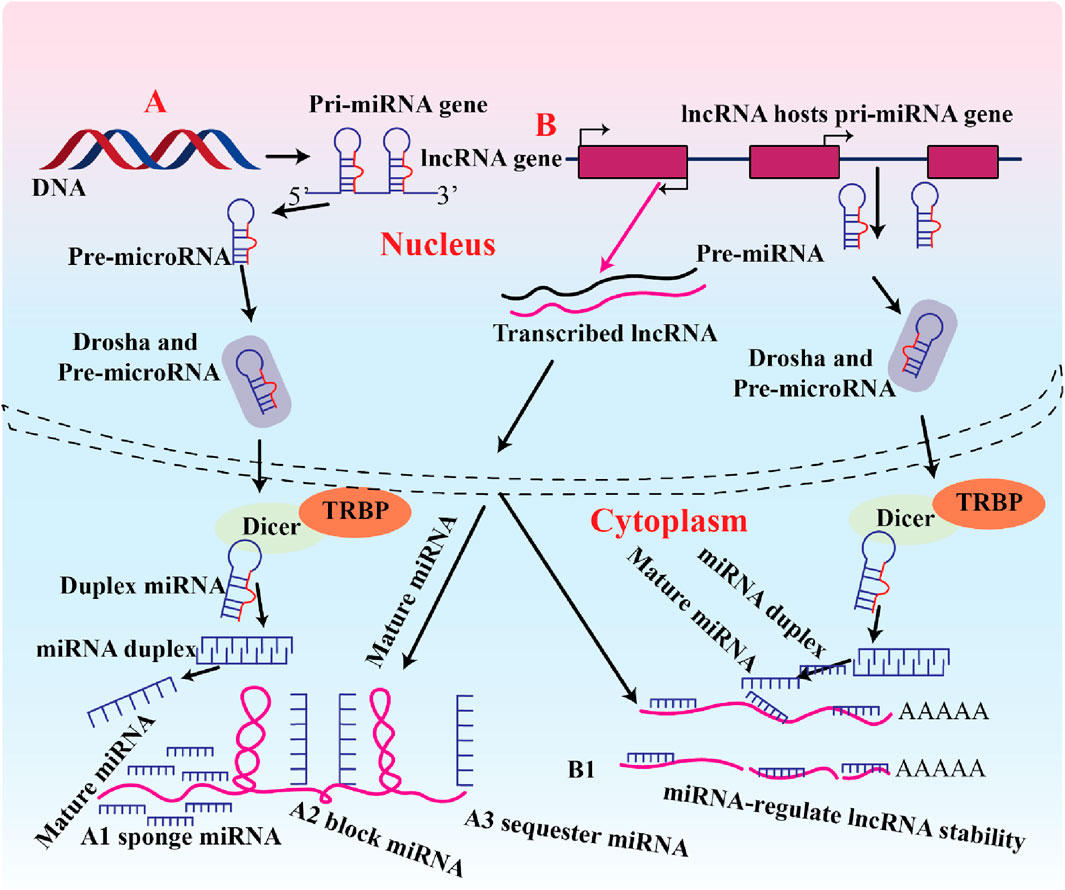
Figure 2. Depicts the biogenesis of lncRNA-miRNA interactions, encompassing binding, blocking, sequestering, and processing of lncRNA genes, wherein miRNAs destabilise lncRNAs and modulate exercise.
3 The role of microRNAs and lncRNAs in the exercise and in skeletal muscle adaptation to exercise
MicroRNAs in skeletal muscle, cardiac tissue, and vasculature have been the subject of recent research that has shed light on their role in exercise adaptations and brought to light potential therapeutic targets (Güller and Russell, 2010). The regulatory roles that microRNAs play in physical fitness will be investigated in this review, which will focus on these domains (Wang et al., 2018). Despite the limited characterization that has been done, functional lncRNAs fulfill the role of essential regulatory molecules in cells. They have piqued people’s interest in their possible role in both health and disease through their discovery. Another putative is responsible for encoding myoregulin (MLN), a micropeptide that is specific to skeletal muscle and was recently discovered. Integration of MLN into the SR membrane, colocalization of MLN with SERCA1, and modulation of Ca2+ management through inhibition of SERCA pump activity were all observed. The exercise performance of MLN-KO mice was significantly improved, as was their ability to handle calcium in muscle tissue (Moore and Uchida, 2020; Anderson et al., 2015). Although the study discovered a correlation between lncRNAs and skeletal muscle during physical training, Further research is necessary to fully understand the role of lncRNAs in the exercise process. A recent study indicates that lncRNAs may inhibit osteoporosis and demonstrates that exercise modulates the expression levels of particular lncRNAs in osteoporotic mice. Within the context of this process, it is conceivable that specific lncRNAs, such as lncRNA H19 and LOC102637959, are involved.
The adaptation of skeletal muscle to exercise is affected by the structure of contractile proteins, mitochondrial functionality, metabolic regulation, intracellular signaling, and transcriptional responses such as MEF2, HDACs, and NRFs (Smith et al., 2023). Some physiological changes take place in myofibers, which are the primary components of muscle tissue, after exercise to ensure that they continue to function at their highest potential. There have been experimental studies that have demonstrated fitness-related changes in microRNAs. These microRNAs are thought to influence muscle myogenesis, muscle mass, and metabolism in skeletal muscle by modulating specific genes that are involved in myogenic regulatory signaling (Ultimo et al., 2018). The dysregulation of microRNAs in skeletal muscle following physical training brings to light the connection between exercise-induced physiological changes and changes in muscle function. Comprehending miRNA biogenesis in skeletal muscle following exercise could enhance the efficacy of therapies. MicroRNAs are essential in muscle function during physical training (Zacharewicz et al., 2013) (Table 1).
Skeletal muscle can undergo metabolic and functional adaptations as a result of the activation of signaling pathways from physical exercise. In this response, PGC-1α plays a crucial role as a mediator, contributing to the regulation of angiogenesis, inflammation, mitochondrial metabolism, and β-oxidation, respectively (Olesen et al., 2010). In male C57Bl/6J mice, endurance exercise resulted in a significant increase in the expression of miR-107, -181, and -1 (Safdar et al., 2009). The research indicates that microRNAs significantly regulate mitochondrial biogenesis in skeletal muscle following physical activity, suggesting that post-transcriptional regulation of PGC-1α by miR-23 could play a role in adaptation in skeletal muscle, highlighting the importance of microRNAs in skeletal muscle function. Specifically, the study reveals that exercise has a significant effect on miR-696, which targets PGC-1α. In murine myoblast C2C12 cells, the study discovered that the expression of miR-494 decreased during the process of myogenic differentiation (Aoi et al., 2010). It was observed that there was an increase in mitochondrial DNA as well as predicted target genes such as mitochondrial transcription factors A and Foxj3, which are essential for the construction of mitochondria (Kang et al., 2018). Following endurance exercise, the expression of miR-494 in the skeletal muscle of C57BL/6J mice decreased, indicating that it plays an important role in mitochondrial biogenesis through the mechanisms of mtTFA and Foxj3. Analysis was performed on the regulation of mRNA components in the miRNA biogenesis pathway (Drosha, Dicer, and Exportin-5), as well as muscle-specific miRNAs (miR-206, -133a, -1, and -133b), and many miRNAs that are altered in muscle myopathies (miR-181, -29, -9, -23, and -31) (Yamamoto et al., 2012; Kirby et al., 2015). After undergoing an acute short-term exercise, it was observed that the miRNA biogenesis pathway, along with miR-133a, -181a -1, and -133b, exhibited an increase. On the other hand, miR-31, -23a -9, and-23b, exhibited a decrease. Following twelve weeks of endurance training, the expression of myomiRs, specifically miR-206, -133b, -1, and -133a, was found to decrease in human muscle biopsies (Russell et al., 2013; Ultimo et al., 2018). MyomiR levels returned to their baseline level fourteen days after regular training had been completed. There is still a lack of knowledge regarding the precise mechanisms that myomiRs use to influence human physiology in response to exercising for long periods. Through the regulation of processes such as gene transcription and mitochondrial biogenesis, exercise-induced microRNA changes facilitate muscle adaptation to exercise. These alterations regulate extremely important processes, including the regeneration of muscle tissue, the transcription of genes, and the biogenesis of mitochondria (Nielsen et al., 2010). Myogenesis and muscle function are governed by diverse signalling pathways, modulated by an array of miRNAs, encompassing both ubiquitous and tissue-specific variants, throughout muscle differentiation and activity. MiR-133a, a constituent of the myomiRs family, is essential for myogenic differentiation, muscle fibre type specification, and mitochondrial biosynthesis in skeletal muscle (Wang, 2013). Dey’s 2012 study (Dey et al., 2012) demonstrated that miR-26a inhibits the expression of Smad1 and Smad4, thereby affirming the role of miRNAs in myogenic differentiation. In addition, MiR-206 and miR-29 can attenuate the inhibitory effects of TGFβ signaling on myogenic differentiation by suppressing Smad3 expression, which subsequently downregulates HDAC4 expression. The myomiRs miR-1 and miR-206 modulate Pax7 expression. An antimiR suppresses the activity of miR-1 and miR-206, thereby enhancing Pax7 expression and cellular proliferation. Pax7 regulates miR-486, a member of the myomiR family that is upregulated during differentiation (Chen et al., 2010). Myostatin, a growth factor-8, modulates skeletal muscle mass by inhibiting the IGF-1/Akt/mTOR pathway, thereby influencing muscle protein synthesis, although the precise mechanism is not fully understood (Rodriguez et al., 2014). The research identified miR-486, a crucial regulator of the IGF-1/Akt pathway, as a target of myostatin signaling. In myostatin knockout mice, miR-486 expression was elevated, and myostatin signaling suppressed its activity. The overexpression of miR-486 induced myotube hypertrophy and maintained skeletal muscle mass (Hitachi et al., 2014). Suppression of MiR-486 diminished Akt activity in C2C12 myotubes, whereas MiR-145 safeguarded cardiomyocytes from reactive oxygen species-induced damage by inhibiting mitochondrial apoptosis, averting Ca2+ overload, and obstructing cardiomyocyte hypertrophy. Reduced miR-145 levels may facilitate the expression of genes associated with muscle atrophy (Cha et al., 2013).
Changes in skeletal muscle associated with aging impact mobility and overall quality of life. The capacity for physical activity is essential for longevity. Older males exhibit elevated levels of pri-miR-1 and -133a, whereas younger males demonstrate heightened miR-1 expression in skeletal muscle (Ultimo et al., 2018; Distefano and Goodpaster, 2018). This suggests that younger males have a different age-related muscular response to fitness than older men. The miR-126-IGF-1 axis is responsible for regulating exercise-induced adaptations in skeletal muscle, which indicates that dysregulation occurs with aging. Certain microRNAs may play a role in the progression of sarcopenia and have the potential to be used as therapeutics for the aging process. Resistance training can stimulate Akt-mTOR signaling, which is decreased in conditions associated with age-related muscle atrophy (Rivas et al., 2014). Analysis of 26 microRNAs that target the Akt-mTOR pathway revealed that dysregulation occurs as a result of both aging and physical activity. The microRNA family known as miR-99/100 is accountable for the regulation of Akt-mTOR signaling and muscle protein synthesis in individuals of all ages, including those who are young and those who are elderly (Gao et al., 2023). According to this, it appears that the modifications of miRNA that are induced by exercise may be able to help mitigate the effects of age-related muscle deterioration and muscle disorders (Table 2).
MicroRNAs possess the capacity to modulate genes associated with mitochondrial processes, including ATP synthesis, oxidative stress, and overall mitochondrial functionality. MiR-4485 is associated with the regulation of mitochondrial respiratory complex I activity, signifying its role in mitochondrial metabolism. Exercise has been also shown to modulate miR-696, which is associated with PGC-1α, an essential regulator of mitochondrial biogenesis (Sripada et al., 2017). Another study showed the critical function of miR-133a in mitochondrial biogenesis within skeletal muscle, exercise endurance, and adaptation to exercise training (Nie Y. et al., 2016). In addition, Research indicates that miRNAs may indirectly affect the activity of the TOM complex during exercise by modulating genes associated with TOM complex functionality and mitochondrial protein import. For example, miR-494 indirectly affects TOM complex activity by contributing to mitochondrial biogenesis and exercise adaptation (Yamamoto et al., 2012). miR-106b has been identified as a regulator of genes associated with mitochondrial biogenesis and dynamics, potentially influencing the functionality of the TOM complex (Zhang et al., 2013). miR-124a is essential for mitochondrial function, impacting mitophagy and mitochondrial dynamics, which may indirectly influence the TOM complex (Saikia et al., 2023).
The results have improved our comprehension of exercise’s influence on lncRNA expression and offered evidence for the underlying mechanism of this regulation (Chen et al., 2021). The evidence should facilitate the development of novel therapeutic interventions for the prevention and treatment of osteoporosis. Another study demonstrated that the lncRNA CRNDE was highly expressed in exosomes that were derived from long-term exercise. While inhibiting the progression of myocardial infarction, CRNDE knockdown led to an increase in apoptosis and oxidative stress in cardiomyocytes. CRNDE acted as a sponge for miR-489-3p, which is responsible for affecting the expression of Nrf2. The inhibition of miR-489-3p was able to effectively reverse the effects of CRNDE depletion on cardiomyocytes that were working under hypoxia. These discoveries presented a potentially useful therapeutic option for the treatment of myocardial infarction (Han et al., 2024). The research conducted by Wohlwend et al. revealed that the long noncoding RNA CYTOR, which is upregulated in human, rat, and mouse muscle post-exercise, enhances myogenic differentiation and facilitates the formation of type II muscle fibers in vitro, underscoring the substantial influence of exercise and aging on skeletal muscle. Manipulation of cytor expression can modify skeletal muscle mass, strength, and performance in both mice and humans. A single-nucleotide polymorphism associated with elevated CYTOR expression in human skeletal muscle and enhanced distance in the Helsinki Birth Cohort Study may facilitate future interventions to improve myogenesis (Wohlwend et al., 2021).
4 The role of microRNAs and lncRNAs in the cardiovascular adaptation to exercise and in cardiovascular disease and heart failure
Ischemia-reperfusion injury and myocardial infarction are both protected against by exercise, which also induces physiological cardiac hypertrophy in athletes’ hearts. Exercise also promotes cardiac hypertrophy in athletes, which protects against these injuries. Cardiovascular hypertrophy that is induced by exercise is considered to be protective and has the potential to occasionally improve cardiac function. This is in contrast to pathological hypertrophy, which can lead to a poor prognosis and heart failure (Calvert et al., 2011). As a result of their physiological adaptation, microRNAs have garnered attention in the field of cardiovascular health and physical activity. This is because of their ability to increase heart size, cardiac output, injury resistance, and improve vascularization characteristics. MiRNAs are becoming more widely recognized for their physiological adaptations, which include increased heart size, cardiac output, injury resistance, and improved vascularization (Liu et al., 2017; Nappi et al., 2023). These adaptations contribute to the potential role that miRNAs could play in the realm of physical activity and cardiovascular health.
Studies indicate that miR-1 and -133 are downregulated in rat hearts due to treadmill training and in transgenic mice exhibiting cardiac overexpression of Akt1. MiR-222, -34a, and -210 are downregulated in physiological hypertrophy and upregulated in dominant-negative PI3K transgenic hearts. In rats undergoing 8 weeks of swim training, the cardiac expression of miR-21, -144, and -145 was elevated, whereas miR-124 was diminished. In exercised hearts, PTEN and TSC2 levels were diminished, whereas PI3K(110α) levels were elevated. The data indicate that certain miRNAs modified by exercise influence PI3K/AKT/mTOR signaling, a crucial regulator of the cardiac response to exercise (Fathi et al., 2020). Soci and colleagues identified 87 differentially expressed cardiac miRNAs following 10 weeks of exercise in comparison to sedentary controls. These miRNAs, comprising 48 upregulated and 39 downregulated species, were implicated in pathological and stress-induced cardiac hypertrophy (Soci et al., 2011). Nonetheless, miR-1, -133a, and -133b diminished following exercise training, whereas miR-29c was elevated in the hearts of exercised subjects. In exercised rats, miR-27a and -27b levels increased, whereas miR-143 levels decreased, indicating that specific miRNAs targeting genes of the renin-angiotensin system are dynamically regulated by exercise training and may influence the development of cardiac hypertrophy (Liu et al., 2017). Martinelli et al. (Martinelli et al., 2014) identified 35 differentially expressed miRNAs following 1 week of exercise and 25 differentially expressed after 5 weeks of training, illustrating the temporal regulation of cardiac miRNAs during exercise training.
A study investigating miRNAs in the hearts of mice subjected to rigorous swimming or voluntary wheel running identified 124 differentially expressed miRNAs in the hearts of wheel-running mice and 55 differentially expressed miRNAs in the hearts of swimming mice, relative to sedentary controls (Inukai et al., 2012). The research indicates that forced swim training may elicit a stress response absent in voluntary wheel running. Sixteen miRNAs, including miR-222, were independently confirmed to be consistently regulated in both models (Liu et al., 2015). Ramasamy et al. (2015) conducted a comparable study revealing that more than 80% of the 201 identified miRNAs exhibited differential regulation. The research indicates that these miRNAs are linked to cardiac hypertrophy and apoptosis. Cardiac development and physiological stimuli or pathological stress induce hypertrophic growth, increasing in cardiomyocyte size. Numerous lncRNAs, such as cardiac hypertrophy-associated transcript (Chast) and cardiac hypertrophy-associated epigenetic regulator (Chaer), participate in this process and exhibit differential regulation in reaction to pathological stress. These transcripts are upregulated in mice after transverse aortic constriction (TAC) and downregulated following TAC (Mably and Wang, 2024). Research indicates that cardiac lncRNAs, including H198 and NRON, may function as therapeutic targets in pressure overload induced by TAC. These lncRNAs can confer cardioprotection in mouse models of TAC, either by targeted knockdown or deletion of specific lncRNAs or through overexpression (Busscher et al., 2022). LncRNAs are integral to pathological cardiac hypertrophy, yet their involvement in physiological growth processes remains inadequately understood. Physiological hypertrophy induced by exercise safeguards against cardiac injury and heart failure, and is associated with cardiomyogenesis. The sole lncRNA essential for exercise-induced physiological cardiac hypertrophy in mice is cardiac physiological hypertrophy-associated regulator (CPhar), as evidenced by a forced swim training model. Research indicates that lncExACT1 exhibits specific mechanisms of action, underscoring the distinctive characteristics of lncRNAs (Gao et al., 2021). It is located in the nuclei and cytoplasm of cardiomyocytes, exhibiting a 2.5-fold enrichment in the nucleus. In the cytoplasm, it suppresses the cardiac-expressed miRNA miR-222, which is essential for physiological cardiac growth and exercise-induced cardiomyogenesis. In the nucleus, it associates with the promoter region of the adjacent gene DCHS2 and enhances its transcription. Nuclear lncRNAs modulate gene expression by interacting with the promoter region of the adjacent DCHS2 gene. They can be categorised as locally modified chromatin structures or distally executed functions. lncExACT1 functions locally by binding to the promoter region of the DCHS2 gene, but exogenous expression of the lncExACT1 transcript can mediate this effect. DCHS2 is positively modulated by lncExACT1; overexpression of lncExACT1 in mice leads to the upregulation of DCHS2, while the inhibition of lncExACT1 is associated with the downregulation of DCHS2(102). The parallel expression patterns are consistent in patients with human heart failure, where both lncExACT1 and DCHS2 are upregulated. DCHS2 is essential and adequate for the effects of lncExACT1 on cardiomyocyte growth, indicating it serves as a downstream effector of lncExACT1 activity (Li et al., 2022).
DCHS2 regulates cell proliferation via the Hippo/Yap1 signaling pathway, a conserved mechanism present in various organ systems, including the heart. Exercise and the inhibition of lncExACT1 or DCHS2 augmented the nuclear fraction of Yap1, leading to the expression of downstream Yap1 targets. The knockdown of DCHS2 diminished the cytoplasmic levels of phosphorylated MST1/2, a fundamental regulatory protein in the Hippo pathway. The data indicate that lncExACT1 and DCHS2 are new regulators of cardiac Yap1, playing a role in exercise-induced cardiac hypertrophy and cardiomyogenesis. Nevertheless, previous research indicates that postnatal activation of cardiac Yap1 does not enhance cardiomyocyte size, implying that Yap1 activation alone is inadequate to stimulate physiological growth. Consequently, the effects noted on cardiac hypertrophy may be facilitated by Yap1-independent mechanisms downstream of lncExACT1 and DCHS2 (Li et al., 2022).
A recent analysis of microRNAs indicated that the expression of miR-222, a protein vital to cardiac function, fluctuates in response to physical training, with its therapeutic cardioprotective properties upregulated in two exercise models and heightened in patients with heart failure (Liu et al., 2015). These properties were demonstrated in mice that overexpressed MiR-222, which prevented exercise-induced cardiac hypertrophy. There is a correlation between elevated levels of the cardiac renin-angiotensin system (RAS), which includes angiotensinogen, angiotensin-converting enzyme (ACE), angiotensin II, and left ventricular hypertrophy. Through the regulation of the equilibrium between these substances, ACE2, a newly discovered component of the RAS, contributes to the preservation of cardiovascular homeostasis. The levels of ACE and Ang II in the heart can be decreased through swimming exercises, while the levels of ACE2 and Ang can be increased (Iwai et al., 1995; Adamcova et al., 2021). Through aerobic exercise, miR-27a and -27b can be increased, which targets atrial fibrillation (ACE), and miR-143 can be reduced, which causes left ventricular hypertrophy. In rats, exercise-induced cardiac hypertrophy leads to a reduction in the expression of miR-29, along with downregulation of miR-133b, -1, and -133a, and upregulation of miR-29a. The expression of miR-29a is correlated with a lower concentration of hydroxyproline and collagen in the left ventricle (LV), which suggests that the LV is more compliant and has beneficial effects on the heart (Rodriguez et al., 2024). The expression of miR-145, -21, -144, is found to be upregulated in response to swimming exercise training-induced left ventricular remodeling, whereas the expression of miR-124 is found to be downregulated. It appears from this that activation of PI3K/AKT/mTOR is essential for the development of cardiac hypertrophy. It has been discovered that particular microRNAs are capable of targeting the PI3K/AKT/mTOR signaling pathway as well as its negative regulators (Da Silva et al., 2012). A rat model of cardiac hypertrophy induced by prolonged swimming was used to investigate the relationship between microRNAs and the PI3K/AKT/mTOR pathway. The results of this study showed that miR-19b, -133b, -30e, and -208a exhibited upregulation, whereas miR-191a, -99b, -22, -100, and -181a exhibited downregulation (Ramasamy et al., 2015). It has been established that the majority of microRNAs, such as miR-19, -208, -99, -100, and, -181, are associated with cardiac hypertrophy and apoptosis, primarily through the MAPK and the PI3K/Akt/mTOR signaling pathways. By gaining an understanding of the microRNAs and cellular pathways that regulate exercise-induced cardiac hypertrophy, it may be possible to accelerate the development of treatments for cardiovascular disease (Zhang et al., 2020). MiRNA-195 is upregulated during cardiac hypertrophy, resulting in pathological hypertrophy and heart failure in mice. It is integral to hypertrophic growth and cardiac remodeling resulting from pathological signaling (van Rooij et al., 2006). MiR-208, a cardiac-specific microRNA, is essential for hypertrophy, fibrosis, and the upregulation of βMHC in cardiomyocytes under stress conditions. MiR-133 and miR-1 are downregulated in models of cardiac hypertrophy, and their overexpression mitigates hypertrophy. Inhibition of MiR-133 results in considerable cardiac hypertrophy and dysfunction, indicating its cardioprotective role in hypertrophic conditions by upregulating the targets Rho1, Cdc42, and Nelf-A/WHSC2. MicroRNAs such as miR-1, miR-23a, and miR-34 can influence cardiac hypertrophy, either providing protection or exacerbating the condition, underscoring their significance as prospective therapeutic targets in the treatment of heart disease (Callis et al., 2009; Carè et al., 2007). In a study that investigated the expression of microRNAs in exercise-induced left ventricular hypertrophy, researchers discovered that after 35 days, the levels of miR-150 increased, while the levels of miR-143, -26b, and-27a, decreased after 7 days. To confirm the targets of these microRNAs and to gain a better understanding of their roles in the adaptation of the heart to physical training, additional research is required (Sanchis-Gomar et al., 2021).
MiRNA-541-5p may function as a biomarker for myocardial ischemia/reperfusion injury, modulating oxidative stress and ferroptosis through the inhibition of its expression (Zhao et al., 2025). The research indicates that miRNA-27a may intensify ferroptosis in brain tissue during ischemic stroke by potentially suppressing Nrf2. In vitro investigations demonstrated that miR-182-5p and miR-378a-3p promote ferroptosis in cells by modulating the expression of GPX4 and SLC7A11. In vivo studies demonstrated that silencing these genes mitigated ischemia/reperfusion-induced renal injury in rats (Zhang et al., 2022). MiR-126, Spred-1, and PI3KR2 inhibit angiogenesis in a rat swimming model by decreasing heart rate and blood pressure, while enhancing the expression of anti-angiogenic microRNA in the soleus muscle (Improta Caria et al., 2018). Physical activity promotes angiogenic factors in hypertension, inhibits apoptotic signaling, and modulates vascular disease. MicroRNAs induced by exercise mitigate hypertension-related microvascular abnormalities by preserving the equilibrium between angiogenic and apoptotic factors (Fernandes et al., 2012). Recent studies demonstrate that miR-221 can attenuate VEGF receptor signaling through the regulation of PI3K regulatory subunits. The MiR-221/222 family, a target gene of c-Kit and let-7f, facilitates angiogenesis via thrombospondin 1, as evidenced by deep sequencing in zebrafish embryos. Suppression of MiR-221 impairs embryonic vascular development, resulting in anomalies in angiogenesis and lymphatic vasculature, whereas overexpression promotes proliferation and migration in apical cell activity (Nicoli et al., 2012). The regulation of phosphoinositide-3-kinase by MiR-126 not only amplifies the effects of Ang-1 on vessel stabilization and maturation but also modulates VEGF signaling. It has been showed that miR-200b modulates angiogenic signals in endothelial cells by targeting VEGF signaling receptors, including VEGFR2, Flt1, KDR, and GATA binding protein 2. Another study indicated that miR-329 can suppress angiogenesis and arteriogenesis by targeting the co-receptor for VEGFR2, CD136, and MEF2a (Fish et al., 2008).
Research indicates that pathogenic cardiac stressors enhance miR-574 expression in humans and mice, resulting in cardiac dysfunction in knockout mice, whereas nanoparticle-mediated delivery alleviates this effect. Transcriptomic analysis of miR-574-deficient hearts revealed FAM210A as a common target mRNA of miR-574-5p and miR-574-3p. Modulating miR-574 deficiency or FAM210A expression influences the protein levels of mitochondrial-encoded electron transport chain genes, potentially impacting cardiac remodeling in heart failure (Wu et al., 2021). MicroRNA-574, particularly the 5p arm, is integral to mitochondrial biogenesis, modulating the expression of genes such as PGC-1α and SIRT1, which are vital for mitochondrial function (Wu et al., 2021). MicroRNA-21, a vital microRNA in the cardiovascular system, is indispensable for the proliferation of vascular smooth muscle cells, apoptosis, cardiac cell growth, and fibroblast activity. MiR-21, a gene associated with the pathogenesis of cardiovascular diseases, may represent a potential therapeutic target for Programmed Cell Death 4, PTEN, SPRY1, and SPRY2 (Cheng and Zhang, 2010).
Frank et al. have shown that long non-coding RNAs (lncRNAs) are essential in the advancement of cardiac hypertrophy (Frank et al., 2016). Researchers identified that CHAST, a transcript associated with cardiac hypertrophy, is upregulated in mice subjected to pressure overload, a condition capable of inducing cardiac hypertrophy. LncRNAs can engage with chromatin remodeling factors, modifying chromatin structure and establishing a feedback loop that governs lncRNA expression. The lncRNA Mhrt and chromatin feedback loop are essential for cardiac function and the prevention of pathological hypertrophy, whereas the restoration of Mhrt expression safeguards against heart failure (Viereck et al., 2016; Goh et al., 2019). A study examines the correlation between MALAT1 and miR-320a and the improvement of endothelial dysfunction in obese children and adolescents, emphasizing the potential protective benefits of exercise (Montero et al., 2012). Exercise training diminishes levels of VCAM-1, ICAM-1, and E-selectin in obese children and adolescents, suppresses MALAT1 expression, and elevates miR-320a expression, indicating that physical activity may safeguard against endothelial dysfunction (Zhao et al., 2021). The MALAT1/miR-320a axis may be associated with the beneficial effects of exercise on endothelial function in obese children and adolescents, as evidenced by their correlation with markers of endothelial dysfunction.
5 The diagnostic and clinical efficacy of microRNAs and lncRNAs in the cardiovascular diseases and heart failure
Recent studies indicate that microRNAs, functioning as biomarkers for health and disease, mature within the circulatory system and associate with RNA-binding proteins such as Argonaute2 or HDL/LDL (Gayosso-Gómez and Ortiz-Quintero, 2021). Extracellular vesicles are also responsible for this release. The contents of vesicles are being transported to target cells by ci-miRNAs. ci-miRNAs, which can be extracted from bodily fluids, demonstrate stability after freezing, thawing, and temperature fluctuations, which suggests that they could serve as biomarkers for monitoring these conditions. Exercise affects the expression of miRNA in tissue and circulation (Xu et al., 2022). A profile of specific ci-miRNAs associated with angiogenesis (miR-222,-328, -, -20a, -221, and-210), inflammation (miR-21 and -146a), skeletal and cardiac muscle contractility (miR-133a and miR-21), and hypoxia/ischemia adaptation (miR--146a,- 210, and-21) has been examined in healthy competitive athletes before, during, and after acute exhaustive exercise testing (Kilian et al., 2016). The goal of this study is to identify exercise-induced changes in the expression of small interfering microRNAs in humans. It has been demonstrated that after 90 days of aerobic exercise training, there is an increase in the levels of microRNAs miR-221, -222, , -20a, -146a, and --21, and in that individual’s plasma (Sullivan et al., 2022). They discovered that peak levels of miR-146a and miR-20a exhibited a positive correlation with peak oxygen consumption (VO2 max), which suggests that there is a viable approach to utilizing ci-miRNAs as biomarkers for physical fitness. After that, the involvement of muscle-specific microRNAs (miR-1, -499, --208a, -208b, -133b, and 133a) as well as muscle-related microRNAs (miR—214, and-181) in physical activity was investigated. After exercise, particularly after downhill training, the plasma concentrations of microRNAs miR-1, miR-133a, and miR-133b significantly increased (Baggish et al., 2011). This was especially true after the exercise. Short-term increases in miR-181b and miR-214 expression were observed. To evaluate muscle-enriched ci-miRNA alterations, additional research is required. Plasma concentrations of miR-133 b, -1, and -133a, significantly increased after exercise, particularly after downhill training. This was especially the case. During this brief period, miR-181b and -214 were enhanced. Assessment of muscle-enriched ci-miRNA alterations requires additional research to be conducted (Sieland et al., 2021). A subsequent study discovered that an increase in miR-149 levels occurred during physical exercise, while miR-146a and -221 showed significant reductions. The initial study discovered that a decrease in c-miR-486 can lead to metabolic changes during physical exercise. These changes may be influenced by the type of exercise, the duration of the exercise, and the intensity of the exercise, according to the findings of the study, which found that muscle-specific miRNA concentrations did not change after acute resistance exercise (Sawada et al., 2013). MiR-126, a biomarker for endothelial cell damage, was utilized in the study to analyze plasma samples obtained from healthy subjects following endurance exercise evaluations. These evaluations included maximal symptom-limited tests, cycling, marathon running, and resistance training. Endothelial damage is indicated by elevated levels of microRNAs miR-126 and -133, which are produced as a result of endurance exercise protocols (Uhlemann et al., 2014). Increasing the levels of miR-133 is another benefit of eccentric resistance training. As indicated by elevated biomarkers, the completion of a marathon causes significant damage to the skeletal muscles, stressful conditions for the cardiac muscles, and inflammation throughout the body. During the process of dynamic regulation of ci-miRNAs, a group of miRNAs participates in the process both before and after the completion of extended, submaximal aerobic training (for example, marathon running) (Siracusa et al., 2018). Ci-miRNAs associated with vascular endothelium (miR-126) and inflammatory miRNAs (miR-146a) are present at relatively elevated levels in plasma during resting conditions before marathon running. On the other hand, cardiac and muscle-enriched ci-miRNAs (miR-499–5p, -1, and -133a) exhibit significantly low expression in plasma during these conditions (Baggish et al., 1985). All candidate ci-miRNAs displayed a significant increase immediately after the marathon, and then within twenty-four hours after the race, they returned to the levels they had been at before the race or even decreased altogether. There was a significant increase in the levels of miR-499, -133a, -1, -208b, and -206 in humans before, immediately after, and 24 h after the marathon, according to a report that was published more recently on heart- and muscle-specific microRNAs (Ramos et al., 2018). While the levels of miR-499 and -208b returned to their initial levels, the levels of the other microRNAs remained elevated twenty-four hours later. According to the findings of the study, there were significant correlations between aerobic performance capacity, VO2 max, running speed, miR-206, -1, and -133a. On the other hand, the expression of miR-21 and miR-155, which are related to fibrosis and inflammation, was not affected by exercise (Mooren et al., 2014). Following acute endurance exercise and a 12-week endurance training regimen, a comprehensive ci-miRNA analysis was performed on young, healthy males. The results showed that the levels of ci-miRNA increased, particularly for miR-139-5p, −1, -338-3p, -330-3p, −223, and 143. On the other hand, eight ci-miRNAs, including let-7i, miR-221, -151-3p, -146a, -106a, -30b, -652, and -151-5p, showed a reduction after the exercise (Wang H. et al., 2017). In addition, chronic modifications of ci-miRNAs have been evaluated after undertaking an endurance training regimen for 12 weeks. Let-7d, miR-21, -148a, -25, -342-3p, miR-766, and miR-185, were among the seven ci-miRNAs that showed a significant decrease after the training period (Xu et al., 2015). On the other hand, miR-103 and -107 were among the two ci-miRNAs that showed a significant increase after the training period. One study investigated the global response of inflammation-related microRNAs (c-inflammamiRs) to various exercise doses. Moderate aerobic exercise has been linked to powerful anti-inflammatory mechanisms, and the study was conducted to investigate this relationship (de Gonzalo-Calvo et al., 1985). An increase in miR-150-5p was found immediately after the 10-kilometer race, while the levels of 12 c-inflammamiRs rose immediately after the marathon (miR-148a-3p, -143-3p, -424-5p, -223-5p, -125b-5p, -132-3p, -223-3p, -29a-3p, -34a-5p, -424-3p, let-7d-3p, and let-7f-2-3p). These findings were discovered from the profiles of c-inflammamiRs before, immediately after, and 24 h after participation in marathon and 10-kilometer races (Domańska-Senderowska et al., 2019; Abd El-Kader et al., 2013). According to the findings of the study, the levels of microRNA named miR-193b and -192 in humans with prediabetes and mice that are unable to tolerate glucose have the potential to act as a novel biomarker for prediabetes and a key indicator for therapeutic exercise intervention. According to the findings of the study, circulating microRNAs have the potential to act as a real-time biomarker that is non-invasive and can be used to investigate exercise-induced tissue adaptation (Ma et al., 2022).
Research is underway on cardiovascular non-coding RNA therapies for conditions such as cardiovascular hypertrophy, disrupted excitation–contraction coupling, cellular apoptosis, interstitial fibrosis, and microvascular rarefaction. A common approach to inhibit miRNAs implicated in cardiac hypertrophy involves the utilization of 2′-OMe-modified antagomiRs or locked nucleic acids (LNAs) (Jalink et al., 2024). Numerous miRNAs that function in either cardiomyocytes or fibroblasts have been shown to inhibit hypertrophy and remodeling during experimental heart failure in mice. Alternative cell-type-specific approaches may also prove effective, including the inhibition of leucocyte-expressed miR-155, angiogenesis, osteopontin, endothelial miR-24, and the targeting of lncRNA Chast (Topkara and Mann, 2010). Multiple ncRNA therapeutics are being developed to treat hyperlipidaemia, owing to the liver’s accessibility as a target. Lipoprotein A (LPA) is a crucial target that remains unaddressed by small-molecule therapies, yet it serves as a primary carrier of oxidised phospholipids in human plasma and is a causal risk factor for atherosclerotic cardiovascular disease and aortic stenosis. Pelacarsen, an ASO-targeting LPA mRNA, reduces Lp(a) levels by as much as 80% and is generally well tolerated, except for injection-site reactions (Thau et al., 2024). An antisense oligonucleotide directed at LPA mRNA is conjugated with triantennary N-acetylgalactosamine to facilitate the therapy. Olpasiran, a GalNAc-conjugated siRNA that reduces Lp(a), is presently undergoing Phase 2 trials (NCT04270760), while SLN360, another GalNAc-conjugated siRNA, is in Phase 1 trials (NCT04606602). Angiopoietin-like 3 (ANGPTL3) represents a compelling target for hyperlipidaemia, as studies indicate that loss-of-function variants are associated with markedly reduced LDL-cholesterol and triglyceride levels, along with a diminished risk of coronary heart disease (CHD) (Sosnowska et al., 2022). The circulating inhibitor of lipoprotein lipase and endothelial lipase, ANGPTL3, predominantly synthesized in the liver, regulates the absorption of muscle-free fatty acids, lipogenesis in adipose tissue, and the hepatic uptake of LDL and residual cholesterol. Inhibition of miR-92a using antisense oligonucleotides enhances wound healing, accelerates re-endothelialization, and prevents endothelial dysfunction and atherosclerosis in murine and porcine models following myocardial infarction and hind limb ischemia (Sylvers-Davie and Davies, 2021). In swine, the delivery of an anti-miR-92a LNA ASO through catheter markedly reduced infarct size and improved cardiac function. A LNA ASO aimed at miR-132-3p for heart failure patients has presented initial human data (Gallant-Behm et al., 2018).
6 Conclusion and future prospects
Consistent physical activity directly influences skeletal muscle performance, nutrient metabolism, and muscular strength, while diminishing the risk of cardiovascular disease, type 2 diabetes, and specific cancers. MicroRNAs have facilitated a deeper comprehension of the molecular mechanisms regulating exercise-induced adaptations in muscles, cardiac tissue, and vascular systems. According to the findings of a large number of studies conducted on both animal models and human subjects, microRNAs are subject to dynamic regulation in response to physical activity. In contrast to the comprehensive research that has been conducted on miRNAs in the field of fitness, there is a lack of knowledge concerning lncRNAs in the field of exercise training. to gain a better understanding of the role that lncRNAs play in exercise adaptation in skeletal muscle, the heart, and the circulatory system, additional research and development are required. The identification of the exercise-induced signals that regulate microRNAs and lncRNAs will be essential for the development of therapy, and it will also determine whether or not physical activity is the most effective intervention in the prevention of disease development such as cardiovascular disease and heart failure. Interestingly, microRNAs and lncRNAs are integral to the advantageous effects of exercise on cardiac health and the progression of certain cardiovascular diseases. Investigating their response to exercise may yield novel pharmacological treatments for cardiovascular diseases or therapeutic approaches. Nonetheless, disparities between human and murine species must be acknowledged before the implementation of therapeutic strategies from the laboratory to clinical practice. Muscle-specific c-miRNAs are examined more thoroughly, whereas non-muscle-specific variants are investigated less frequently. Investigating exercise-responsive, non-muscle-specific circulating miRNAs is essential for comprehending their roles and potential application as biomarkers for physical performance. The investigation of circulating microRNAs in blood as prospective biomarkers is highly significant. C-miRNAs can be released into the bloodstream via association with lipid vesicles or proteins, facilitating intercellular communication. Lipid vesicles derived from various cells and tissues display distinct secretion properties and carrier selectivity upon release into the bloodstream. c-miRNAs can be taken up by remote tissue cells, modulating metabolism and intercellular communication. The degree to which c-miRNAs can be released from particular tissues and perform paracrine functions is yet to be explored. Additional investigation is required to comprehend their function in intercellular communication. On the other hand, recent results have proved that exercise causes significant reductions in blood pressure, as well as a reduced risk of subsequent cardiovascular incidents and mortality caused by cardiovascular disease. As of right now, a great number of lncRNAs that have been associated with cardiovascular diseases and regular exercise have been discovered through the use of RNA sequencing (RNA-seq) and bioinformatics analyses. In the context of cardiovascular diseases, the clinical relevance of lncRNAs is substantial, and they present a variety of opportunities for diagnosis and treatment opportunities. Furthermore, lncRNAs exhibit distinct expression patterns that are specific to a variety of tissues and cell types. This makes it easier to classify them into distinct subclasses of cardiovascular diseases and potentially predict how patients will react to treatment. On the other hand, our current understanding of the impact that lncRNAs have on cardiovascular diseases is probably limited. On account of this, it is of the utmost importance to carry out investigations that are more comprehensive to enhance our understanding of the mechanisms by which lncRNAs influence cardiovascular diseases and contribute to the development of novel therapeutic approaches.
Exercise training is a non-pharmacological intervention employed to prevent and manage CVD and heart failure. Nonetheless, evidence connecting epigenetic modifications to alterations in the heart and blood vessels induced by exercise is scarce. Current evidence indicates that ET’s protective effects entail alterations in the expression patterns of specific miRNAs and lncRNAs that positively influence cardiovascular remodeling. MicroRNAs (miRNAs) and lncRNAs are essential in regulating numerous physiological processes in mammals, such as cell proliferation, differentiation, migration, angiogenesis, apoptosis, tissue development, and remodelling. Further research is required to elucidate the pathways and mechanisms through which ET influences miRNAs in correlation to lncRNAs, and to expand the scope of ET’s application in rectifying pathological processes via miRNA and lncRNAs therapy. This may aid in the prevention of cardiovascular and heart damage, enhancing patient survival and quality of life.
Author contributions
GY: Conceptualization, Investigation, Methodology, Supervision, Validation, Visualization, Writing – original draft, Writing – review and editing. WY: Conceptualization, Investigation, Methodology, Validation, Visualization, Writing – original draft, Writing – review and editing.
Funding
The author(s) declare that financial support was received for the research and/or publication of this article. The project is supported by the central university basic scientific research operating expenses (Project No. RW170121). Regular Projects of Shaanxi Provincial Sports Bureau (Project No. 2023012).
Conflict of interest
The authors declare that the research was conducted in the absence of any commercial or financial relationships that could be construed as a potential conflict of interest.
Generative AI statement
The author(s) declare that no Generative AI was used in the creation of this manuscript.
Publisher’s note
All claims expressed in this article are solely those of the authors and do not necessarily represent those of their affiliated organizations, or those of the publisher, the editors and the reviewers. Any product that may be evaluated in this article, or claim that may be made by its manufacturer, is not guaranteed or endorsed by the publisher.
References
Abd El-Kader, S., Gari, A., and Salah El-Den, A. (2013). Impact of moderate versus mild aerobic exercise training on inflammatory cytokines in obese type 2 diabetic patients: a randomized clinical trial. Afr. Health Sci. 13 (4), 857–863. doi:10.4314/ahs.v13i4.1
Adamcova, M., Kawano, I., and Simko, F. (2021). The impact of microRNAs in renin–angiotensin-system-induced cardiac Remodelling. Int. J. Mol. Sci. 22 (9), 4762. doi:10.3390/ijms22094762
Afzal, M., Greco, F., Quinzi, F., Scionti, F., Maurotti, S., Montalcini, T., et al. (2024). The effect of physical activity/exercise on miRNA expression and function in non-Communicable diseases—a systematic review. Int. J. Mol. Sci. 25 (13), 6813. doi:10.3390/ijms25136813
Alexander, M., Kawahara, G., Motohashi, N., Casar, J., Eisenberg, I., Myers, J., et al. (2013). MicroRNA-199a is induced in dystrophic muscle and affects WNT signaling, cell proliferation, and myogenic differentiation. Cell Death and Differ. 20 (9), 1194–1208. doi:10.1038/cdd.2013.62
Alexander, M. S., Casar, J. C., Motohashi, N., Myers, J. A., Eisenberg, I., Gonzalez, R. T., et al. (2011). Regulation of DMD pathology by an ankyrin-encoded miRNA. Skelet. muscle 1, 27–17. doi:10.1186/2044-5040-1-27
Anderson, D. M., Anderson, K. M., Chang, C. L., Makarewich, C. A., Nelson, B. R., McAnally, J. R., et al. (2015). A micropeptide encoded by a putative long noncoding RNA regulates muscle performance. Cell 160 (4), 595–606. doi:10.1016/j.cell.2015.01.009
Aoi, W., Naito, Y., Mizushima, K., Takanami, Y., Kawai, Y., Ichikawa, H., et al. (2010). The microRNA miR-696 regulates PGC-1{alpha} in mouse skeletal muscle in response to physical activity. Am. J. Physiol. Endocrinol. Metab. 298 (4), E799–E806. doi:10.1152/ajpendo.00448.2009
Badi, I., Burba, I., Ruggeri, C., Zeni, F., Bertolotti, M., Scopece, A., et al. (2015). MicroRNA-34a induces vascular smooth muscle cells senescence by SIRT1 downregulation and promotes the expression of age-associated pro-inflammatory secretory factors. J. Gerontol. A Biol. Sci. Med. Sci. 70 (11), 1304–1311. doi:10.1093/gerona/glu180
Baggish, A. L., Hale, A., Weiner, R. B., Lewis, G. D., Systrom, D., Wang, F., et al. (2011). Dynamic regulation of circulating microRNA during acute exhaustive exercise and sustained aerobic exercise training. J. Physiol. 589 (Pt 16), 3983–3994. doi:10.1113/jphysiol.2011.213363
Baggish, A. L., Park, J., Min, P. K., Isaacs, S., Parker, B. A., Thompson, P. D., et al. (1985). Rapid upregulation and clearance of distinct circulating microRNAs after prolonged aerobic exercise. J. Appl. Physiol. 116 (5), 522–531. doi:10.1152/japplphysiol.01141.2013
Bahrami, F., Fathi, M., Ahmadvand, H., and Pajohi, N. (2023). Endurance training changes the expression of miR-1 and miR-133 and predicted genes in slow and fast twitch muscles. Arch. Gerontol. Geriatr. 108, 104929. doi:10.1016/j.archger.2023.104929
Balchin, C., Tan, A. L., Wilson, O. J., McKenna, J., and Stavropoulos-Kalinoglou, A. (2023). The role of microRNAs in regulating inflammation and exercise-induced adaptations in rheumatoid arthritis. Rheumatology Adv. Pract. 7 (1), rkac110. doi:10.1093/rap/rkac110
Borja-Gonzalez, M., Casas-Martinez, J. C., McDonagh, B., and Goljanek-Whysall, K. (2020). Aging Science Talks: the role of miR-181a in age-related loss of muscle mass and function. Transl. Med. Aging 4, 81–85. doi:10.1016/j.tma.2020.07.001
Brzeszczyńska, J., Brzeszczyński, F., Hamilton, D. F., McGregor, R., and Simpson, A. (2020). Role of microRNA in muscle regeneration and diseases related to muscle dysfunction in atrophy, cachexia, osteoporosis, and osteoarthritis. Bone Jt. Res. 9 (11), 798–807. doi:10.1302/2046-3758.911.bjr-2020-0178.r1
Bulaklak, K., Xiao, B., Qiao, C., Li, J., Patel, T., Jin, Q., et al. (2018). MicroRNA-206 downregulation improves therapeutic gene expression and motor function in mdx mice. Mol. Ther. Nucleic Acids 12, 283–293. doi:10.1016/j.omtn.2018.05.011
Burgos, M., Hurtado, A., Jiménez, R., and Barrionuevo, F. J. (2021). Non-Coding RNAs: lncRNAs, miRNAs, and piRNAs in Sexual Development. Sex. Dev. 15 (5-6), 335–350. doi:10.1159/000519237
Busscher, D., Boon, R. A., and Juni, R. P. (2022). The multifaceted actions of the lncRNA H19 in cardiovascular biology and diseases. Clin. Sci. (Lond). 136 (15), 1157–1178. doi:10.1042/CS20210994
Callis, T. E., Pandya, K., Seok, H. Y., Tang, R. H., Tatsuguchi, M., Huang, Z. P., et al. (2009). MicroRNA-208a is a regulator of cardiac hypertrophy and conduction in mice. J. Clin. Invest 119 (9), 2772–2786. doi:10.1172/JCI36154
Calvert, J. W., Condit, M. E., Aragón, J. P., Nicholson, C. K., Moody, B. F., Hood, R. L., et al. (2011). Exercise protects against myocardial ischemia-reperfusion injury via stimulation of β(3)-adrenergic receptors and increased nitric oxide signaling: role of nitrite and nitrosothiols. Circ. Res. 108 (12), 1448–1458. doi:10.1161/CIRCRESAHA.111.241117
Carè, A., Catalucci, D., Felicetti, F., Bonci, D., Addario, A., Gallo, P., et al. (2007). MicroRNA-133 controls cardiac hypertrophy. Nat. Med. 13 (5), 613–618. doi:10.1038/nm1582
Cha, M. J., Jang, J. K., Ham, O., Song, B. W., Lee, S. Y., Lee, C. Y., et al. (2013). MicroRNA-145 suppresses ROS-induced Ca2+ overload of cardiomyocytes by targeting CaMKIIδ. Biochem. Biophys. Res. Commun. 435 (4), 720–726. doi:10.1016/j.bbrc.2013.05.050
Chen, J., Zhou, R., Feng, Y., and Cheng, L. (2022). Molecular mechanisms of exercise contributing to tissue regeneration. Signal Transduct. Target. Ther. 7 (1), 383. doi:10.1038/s41392-022-01233-2
Chen, J. F., Tao, Y., Li, J., Deng, Z., Yan, Z., Xiao, X., et al. (2010). microRNA-1 and microRNA-206 regulate skeletal muscle satellite cell proliferation and differentiation by repressing Pax7. J. Cell Biol. 190 (5), 867–879. doi:10.1083/jcb.200911036
Chen, L., Bai, J., and Li, Y. (2020). miR-29 mediates exercise-induced skeletal muscle angiogenesis by targeting VEGFA, COL4A1 and COL4A2 via the PI3K/Akt signaling pathway. Mol. Med. Rep. 22 (2), 661–670. doi:10.3892/mmr.2020.11164
Chen, L.-L., and Kim, V. N. (2024). Small and long non-coding RNAs: past, present, and future. Cell. 187 (23), 6451–6485. doi:10.1016/j.cell.2024.10.024
Chen, S., Liu, D., Zhou, Z., and Qin, S. (2021). Role of long non-coding RNA H19 in the development of osteoporosis. Mol. Med. 27 (1), 122. doi:10.1186/s10020-021-00386-0
Cheng, Y., and Zhang, C. (2010). MicroRNA-21 in cardiovascular disease. J. Cardiovasc Transl. Res. 3 (3), 251–255. doi:10.1007/s12265-010-9169-7
Da Silva, N. D., Fernandes, T., Soci, U. P. R., Monteiro, A. W. A., Phillips, M. I., and De Oliveira, E. M. (2012). Swimming training in rats increases cardiac MicroRNA-126 expression and angiogenesis. Med. Sci. Sports Exerc 44 (8), 1453–1462. doi:10.1249/MSS.0b013e31824e8a36
de Gonzalo-Calvo, D., Dávalos, A., Montero, A., García-González, Á., Tyshkovska, I., González-Medina, A., et al. (1985). Circulating inflammatory miRNA signature in response to different doses of aerobic exercise. J. Appl. Physiol. 119 (2), 124–134. doi:10.1152/japplphysiol.00077.2015
Deng, P., Muhammad, S., Cao, M., and Wu, L. (2018). Biogenesis and regulatory hierarchy of phased small interfering RNAs in plants. Plant Biotechnol. J. 16 (5), 965–975. doi:10.1111/pbi.12882
Desvignes, T., Batzel, P., Berezikov, E., Eilbeck, K., Eppig, J. T., McAndrews, M. S., et al. (2015). miRNA nomenclature: a view incorporating genetic origins, biosynthetic pathways, and sequence variants. Trends Genet. 31 (11), 613–626. doi:10.1016/j.tig.2015.09.002
Dey, B. K., Gagan, J., Yan, Z., and Dutta, A. (2012). miR-26a is required for skeletal muscle differentiation and regeneration in mice. Genes Dev. 26 (19), 2180–2191. doi:10.1101/gad.198085.112
Dhuli, K., Naureen, Z., Medori, M. C., Fioretti, F., Caruso, P., Perrone, M. A., et al. (2022). Physical activity for health. J. Prev. Med. Hyg. 63 (2 Suppl. 3), E150–E159. doi:10.15167/2421-4248/jpmh2022.63.2S3.2756
Distefano, G., and Goodpaster, B. H. (2018). Effects of exercise and aging on skeletal muscle. Cold Spring Harb. Perspect. Med. 8 (3), a029785. doi:10.1101/cshperspect.a029785
Domańska-Senderowska, D., Laguette, M. N., Jegier, A., Cięszczyk, P., September, A. V., and Brzeziańska-Lasota, E. (2019). MicroRNA profile and adaptive response to exercise training: a review. Int. J. Sports Med. 40 (4), 227–235. doi:10.1055/a-0824-4813
Fathi, M., Gharakhanlou, R., and Rezaei, R. (2020). The changes of heart miR-1 and miR-133 expressions following physiological hypertrophy due to endurance training. Cell J. 22 (Suppl. 1), 133–140. doi:10.22074/cellj.2020.7014
Fernandes, T., Magalhães, F. C., Roque, F. R., Phillips, M. I., and Oliveira, E. M. (2012). Exercise training prevents the microvascular rarefaction in hypertension balancing angiogenic and apoptotic factors: role of microRNAs-16, -21, and -126. Hypertension 59 (2), 513–520. doi:10.1161/HYPERTENSIONAHA.111.185801
Fish, J. E., Santoro, M. M., Morton, S. U., Yu, S., Yeh, R. F., Wythe, J. D., et al. (2008). miR-126 regulates angiogenic signaling and vascular integrity. Dev. Cell 15 (2), 272–284. doi:10.1016/j.devcel.2008.07.008
Frank, S., Aguirre, A., Hescheler, J., and Kurian, L. (2016). A lncRNA perspective into (Re)building the heart. Front. Cell Dev. Biol. 4, 4–2016. doi:10.3389/fcell.2016.00128
Gallant-Behm, C. L., Piper, J., Dickinson, B. A., Dalby, C. M., Pestano, L. A., and Jackson, A. L. (2018). A synthetic microRNA-92a inhibitor (MRG-110) accelerates angiogenesis and wound healing in diabetic and nondiabetic wounds. Wound repair Regen. 26 (4), 311–323. doi:10.1111/wrr.12660
Ganassi, M., Muntoni, F., and Zammit, P. S. (2022). Defining and identifying satellite cell-opathies within muscular dystrophies and myopathies. Exp. Cell Res. 411 (1), 112906. doi:10.1016/j.yexcr.2021.112906
Gao, J., Song, J., Yan, Y., Gokulnath, P., Vulugundam, G., Li, G., et al. (2023). Exercise training-induced MicroRNA alterations with protective effects in cardiovascular diseases. Rev. Cardiovasc Med. 24 (9), 251. doi:10.31083/j.rcm2409251
Gao, R., Wang, L., Bei, Y., Wu, X., Wang, J., Zhou, Q., et al. (2021). Long noncoding RNA cardiac physiological hypertrophy-associated regulator induces cardiac physiological hypertrophy and promotes functional recovery after myocardial ischemia-reperfusion injury. Circulation 144 (4), 303–317. doi:10.1161/CIRCULATIONAHA.120.050446
Gayosso-Gómez, L. V., and Ortiz-Quintero, B. (2021). Circulating MicroRNAs in blood and other body fluids as biomarkers for diagnosis, prognosis, and therapy response in lung cancer. Diagnostics 11 (3), 421. doi:10.3390/diagnostics11030421
Gidaro, T., Negroni, E., Perié, S., Mirabella, M., Lainé, J., Lacau St Guily, J., et al. (2013). Atrophy, fibrosis, and increased PAX7-positive cells in pharyngeal muscles of oculopharyngeal muscular dystrophy patients. J. Neuropathol. Exp. Neurol. 72 (3), 234–243. doi:10.1097/NEN.0b013e3182854c07
Goh, K. Y., He, L., Song, J., Jinno, M., Rogers, A. J., Sethu, P., et al. (2019). Mitoquinone ameliorates pressure overload-induced cardiac fibrosis and left ventricular dysfunction in mice. Redox Biol. 21, 101100. doi:10.1016/j.redox.2019.101100
Greene, J., Baird, A. M., Brady, L., Lim, M., Gray, S. G., McDermott, R., et al. (2017). Circular RNAs: biogenesis, function and role in human diseases. Front. Mol. Biosci. 4, 38. doi:10.3389/fmolb.2017.00038
Gu, C., Mo, W., Wang, K., Gao, M., Chen, J., Zhang, F., et al. (2023). Exosomal miR-370-3p increases the permeability of blood-brain barrier in ischemia/reperfusion stroke of brain by targeting MPK1. Aging (Albany NY) 15 (6), 1931–1943. doi:10.18632/aging.204573
Güller, I., and Russell, A. P. (2010). MicroRNAs in skeletal muscle: their role and regulation in development, disease and function. J. Physiol. 588 (Pt 21), 4075–4087. doi:10.1113/jphysiol.2010.194175
Han, C., Zhai, C., Li, A., Ma, Y., and Hallajzadeh, J. (2024). Exercise mediates myocardial infarction via non-coding RNAs. Front. Cardiovasc Med. 11, 1432468. doi:10.3389/fcvm.2024.1432468
He, N., Zhang, Y., Zhang, L., Zhang, S., and Ye, H. (2021). Relationship between sarcopenia and cardiovascular diseases in the elderly: an overview. Front. Cardiovasc Med. 8, 743710. doi:10.3389/fcvm.2021.743710
He, W. A., Calore, F., Londhe, P., Canella, A., Guttridge, D. C., and Croce, C. M. (2014). Microvesicles containing miRNAs promote muscle cell death in cancer cachexia via TLR7. Proc. Natl. Acad. Sci. U. S. A. 111 (12), 4525–4529. doi:10.1073/pnas.1402714111
Hitachi, K., Nakatani, M., and Tsuchida, K. (2014). Myostatin signaling regulates Akt activity via the regulation of miR-486 expression. Int. J. Biochem. Cell Biol. 47, 93–103. doi:10.1016/j.biocel.2013.12.003
Huang, Z., Chen, X., Yu, B., He, J., and Chen, D. (2012). MicroRNA-27a promotes myoblast proliferation by targeting myostatin. Biochem. Biophys. Res. Commun. 423 (2), 265–269. doi:10.1016/j.bbrc.2012.05.106
Improta Caria, A. C., Nonaka, C. K. V., Pereira, C. S., Soares, M. B. P., Macambira, S. G., and Souza, B. S. F. (2018). Exercise training-induced changes in MicroRNAs: beneficial regulatory effects in hypertension, type 2 diabetes, and obesity. Int. J. Mol. Sci. 19 (11), 3608. doi:10.3390/ijms19113608
Inukai, S., de Lencastre, A., Turner, M., and Slack, F. (2012). Novel microRNAs differentially expressed during aging in the mouse brain. PLoS One 7 (7), e40028. doi:10.1371/journal.pone.0040028
Iwai, N., Shimoike, H., and Kinoshita, M. (1995). Cardiac renin-angiotensin system in the hypertrophied heart. Circulation 92 (9), 2690–2696. doi:10.1161/01.cir.92.9.2690
Iwasaki, Y. W., Siomi, M. C., and Siomi, H. (2015). PIWI-interacting RNA: its biogenesis and functions. Annu. Rev. Biochem. 84, 405–433. doi:10.1146/annurev-biochem-060614-034258
Jalink, E. A., Schonk, A. W., Boon, R. A., and Juni, R. P. (2024). Non-coding RNAs in the pathophysiology of heart failure with preserved ejection fraction. Front. Cardiovasc. Med. 10, 10–2023. doi:10.3389/fcvm.2023.1300375
Joris, V., Gomez, E. L., Menchi, L., Lobysheva, I., Di Mauro, V., Esfahani, H., et al. (2018). MicroRNA-199a-3p and MicroRNA-199a-5p take part to a redundant Network of regulation of the NOS (NO synthase)/NO pathway in the endothelium. Arterioscler. Thromb. Vasc. Biol. 38 (10), 2345–2357. doi:10.1161/ATVBAHA.118.311145
Kang, I., Chu, C. T., and Kaufman, B. A. (2018). The mitochondrial transcription factor TFAM in neurodegeneration: emerging evidence and mechanisms. FEBS Lett. 592 (5), 793–811. doi:10.1002/1873-3468.12989
Kiełbowski, K., Bakinowska, E., Procyk, G., Ziętara, M., and Pawlik, A. (2024). The role of microRNA in the pathogenesis of Duchenne muscular dystrophy. Int. J. Mol. Sci. 25 (11), 6108. doi:10.3390/ijms25116108
Kilian, Y., Wehmeier, U. F., Wahl, P., Mester, J., Hilberg, T., and Sperlich, B. (2016). Acute response of circulating vascular regulating MicroRNAs during and after high-intensity and high-volume cycling in children. Front. Physiology 7, 92. doi:10.3389/fphys.2016.00092
Kirby, T. J., Chaillou, T., and McCarthy, J. J. (2015). The role of microRNAs in skeletal muscle health and disease. Front. Biosci. Landmark Ed. 20 (1), 37–77. doi:10.2741/4298
Lee, K. P., Shin, Y. J., Panda, A. C., Abdelmohsen, K., Kim, J. Y., Lee, S. M., et al. (2015). miR-431 promotes differentiation and regeneration of old skeletal muscle by targeting Smad4. Genes Dev. 29 (15), 1605–1617. doi:10.1101/gad.263574.115
Li, H., Trager, L. E., Liu, X., Hastings, M. H., Xiao, C., Guerra, J., et al. (2022). lncExACT1 and DCHS2 regulate physiological and pathological cardiac growth. Circulation 145 (16), 1218–1233. doi:10.1161/CIRCULATIONAHA.121.056850
Li, J., Chan, M. C., Yu, Y., Bei, Y., Chen, P., Zhou, Q., et al. (2017). miR-29b contributes to multiple types of muscle atrophy. Nat. Commun. 8, 15201. doi:10.1038/ncomms15201
Liu, X., Platt, C., and Rosenzweig, A. (2017). The role of MicroRNAs in the cardiac response to exercise. Cold Spring Harb. Perspect. Med. 7 (12), a029850. doi:10.1101/cshperspect.a029850
Liu, X., Xiao, J., Zhu, H., Wei, X., Platt, C., Damilano, F., et al. (2015). miR-222 is necessary for exercise-induced cardiac growth and protects against pathological cardiac remodeling. Cell Metab. 21 (4), 584–595. doi:10.1016/j.cmet.2015.02.014
Liu, Z., Guo, Y., and Zheng, C. (2024). Type 2 diabetes mellitus related sarcopenia: a type of muscle loss distinct from sarcopenia and disuse muscle atrophy. Front. Endocrinol. 15, 15–2024. doi:10.3389/fendo.2024.1375610
Lopes, L. O., Cury, S. S., de Moraes, D., Oliveira, J. S., de Oliveira, G., Cabral-Marques, O., et al. (2024). The impact of miR-155-5p on myotube differentiation: elucidating molecular targets in skeletal muscle disorders. Int. J. Mol. Sci. 25 (3), 1777. doi:10.3390/ijms25031777
Ma, G., Wang, Y., Li, Y., Cui, L., Zhao, Y., Zhao, B., et al. (2015). MiR-206, a key modulator of skeletal muscle development and disease. Int. J. Biol. Sci. 11, 345–352. doi:10.7150/ijbs.10921
Ma, L., Gilani, A., Yi, Q., and Tang, L. (2022). MicroRNAs as mediators of adipose thermogenesis and potential therapeutic targets for obesity. Biol. (Basel). 11 (11), 1657. doi:10.3390/biology11111657
Mably, J. D., and Wang, D. Z. (2024). Long non-coding RNAs in cardiac hypertrophy and heart failure: functions, mechanisms and clinical prospects. Nat. Rev. Cardiol. 21 (5), 326–345. doi:10.1038/s41569-023-00952-5
Martinelli, N. C., Cohen, C. R., Santos, K. G., Castro, M. A., Biolo, A., Frick, L., et al. (2014). An analysis of the global expression of microRNAs in an experimental model of physiological left ventricular hypertrophy. PLoS One 9 (4), e93271. doi:10.1371/journal.pone.0093271
McCormack, N. M., Calabrese, K. A., Sun, C. M., Tully, C. B., Heier, C. R., and Fiorillo, A. A. (2023). Deletion of miR-146a enhances therapeutic protein restoration in model of dystrophin exon skipping. bioRxiv. doi:10.1101/2023.05.09.540042
Montero, D., Walther, G., Perez-Martin, A., Roche, E., and Vinet, A. (2012). Endothelial dysfunction, inflammation, and oxidative stress in obese children and adolescents: markers and effect of lifestyle intervention. Obes. Rev. 13 (5), 441–455. doi:10.1111/j.1467-789X.2011.00956.x
Moore, J. B., and Uchida, S. (2020). Functional characterization of long noncoding RNAs. Curr. Opin. Cardiol. 35 (3), 199–206. doi:10.1097/hco.0000000000000725
Mooren, F. C., Viereck, J., Krüger, K., and Thum, T. (2014). Circulating microRNAs as potential biomarkers of aerobic exercise capacity. Am. J. Physiol. Heart Circ. Physiol. 306 (4), H557–H563. doi:10.1152/ajpheart.00711.2013
Muñoz-Braceras, S., Pinal-Fernandez, I., Casal-Dominguez, M., Pak, K., Milisenda, J. C., Lu, S., et al. (2023). Identification of unique microRNA profiles in different types of idiopathic inflammatory myopathy. Cells 12 (17), 2198. doi:10.3390/cells12172198
Nappi, F., Avtaar Singh, S. S., Jitendra, V., Alzamil, A., and Schoell, T. (2023). The roles of microRNAs in the cardiovascular system. Int. J. Mol. Sci. 24 (18), 14277. doi:10.3390/ijms241814277
Nicoli, S., Knyphausen, C.-P., Zhu, L. J., Lakshmanan, A., and Lawson, N. D. (2012). miR-221 is required for endothelial tip cell behaviors during vascular development. Dev. cell 22 (2), 418–429. doi:10.1016/j.devcel.2012.01.008
Nie, M., Liu, J., Yang, Q., Seok, H. Y., Hu, X., Deng, Z. L., et al. (2016b). MicroRNA-155 facilitates skeletal muscle regeneration by balancing pro- and anti-inflammatory macrophages. Cell Death Dis. 7 (6), e2261. doi:10.1038/cddis.2016.165
Nie, Y., Sato, Y., Wang, C., Yue, F., Kuang, S., and Gavin, T. P. (2016a). Impaired exercise tolerance, mitochondrial biogenesis, and muscle fiber maintenance in miR-133a–deficient mice. FASEB J. 30 (11), 3745–3758. doi:10.1096/fj.201600529R
Nielsen, S., Scheele, C., Yfanti, C., Akerström, T., Nielsen, A. R., Pedersen, B. K., et al. (2010). Muscle specific microRNAs are regulated by endurance exercise in human skeletal muscle. J. Physiol. 588 (Pt 20), 4029–4037. doi:10.1113/jphysiol.2010.189860
O'Brien, J., Hayder, H., Zayed, Y., and Peng, C. (2018). Overview of MicroRNA biogenesis, mechanisms of actions, and circulation. Front. Endocrinol. (Lausanne) 9, 402. doi:10.3389/fendo.2018.00402
Olesen, J., Kiilerich, K., and Pilegaard, H. (2010). PGC-1alpha-mediated adaptations in skeletal muscle. Pflugers Arch. 460 (1), 153–162. doi:10.1007/s00424-010-0834-0
Parkes, J. E., Thoma, A., Lightfoot, A. P., Day, P. J., Chinoy, H., and Lamb, J. A. (2020). MicroRNA and mRNA profiling in the idiopathic inflammatory myopathies. BMC Rheumatol. 4, 25. doi:10.1186/s41927-020-00125-8
Piergentili, R., and Sechi, S. (2024). Non-coding RNAs of mitochondrial origin: roles in cell division and implications in cancer. Int. J. Mol. Sci. 25 (13), 7498. doi:10.3390/ijms25137498
Plaza-Diaz, J., Izquierdo, D., Torres-Martos, Á., Baig, A. T., Aguilera, C. M., and Ruiz-Ojeda, F. J. (2022). Impact of physical activity and exercise on the epigenome in skeletal muscle and effects on systemic metabolism. Biomedicines 10 (1), 126. doi:10.3390/biomedicines10010126
Podkalicka, P., Mucha, O., Kaziród, K., Szade, K., Stępniewski, J., Ivanishchuk, L., et al. (2022). miR-378 affects metabolic disturbances in the mdx model of Duchenne muscular dystrophy. Sci. Rep. 12 (1), 3945. doi:10.1038/s41598-022-07868-z
Qiu, Y., Fernández-García, B., Lehmann, H. I., Li, G., Kroemer, G., López-Otín, C., et al. (2023). Exercise sustains the hallmarks of health. J. Sport Health Sci. 12 (1), 8–35. doi:10.1016/j.jshs.2022.10.003
Ramasamy, S., Velmurugan, G., Shanmugha Rajan, K., Ramprasath, T., and Kalpana, K. (2015). MiRNAs with apoptosis regulating potential are differentially expressed in chronic exercise-induced physiologically hypertrophied hearts. PLoS One 10 (3), e0121401. doi:10.1371/journal.pone.0121401
Ramos, A. E., Lo, C., Estephan, L. E., Tai, Y. Y., Tang, Y., Zhao, J., et al. (2018). Specific circulating microRNAs display dose-dependent responses to variable intensity and duration of endurance exercise. Am. J. Physiol. Heart Circ. Physiol. 315 (2), H273–H283. doi:10.1152/ajpheart.00741.2017
Rapps, K., Marco, A., Pe'er-Nissan, H., Kisliouk, T., Stemp, G., Yadid, G., et al. (2024). Exercise rescues obesogenic-related genes in the female hypothalamic arcuate nucleus: a potential role of miR-211 modulation. Int. J. Mol. Sci. 25 (13), 7188. doi:10.3390/ijms25137188
Rivas, D. A., Lessard, S. J., Rice, N. P., Lustgarten, M. S., So, K., Goodyear, L. J., et al. (2014). Diminished skeletal muscle microRNA expression with aging is associated with attenuated muscle plasticity and inhibition of IGF-1 signaling. Faseb J. 28 (9), 4133–4147. doi:10.1096/fj.14-254490
Rodriguez, J., Vernus, B., Chelh, I., Cassar-Malek, I., Gabillard, J. C., Hadj Sassi, A., et al. (2014). Myostatin and the skeletal muscle atrophy and hypertrophy signaling pathways. Cell Mol. Life Sci. 71 (22), 4361–4371. doi:10.1007/s00018-014-1689-x
Rodriguez, N. M., Loren, P., Paez, I., Martínez, C., Chaparro, A., and Salazar, L. A. (2024). MicroRNAs: the missing link between hypertension and periodontitis? Int. J. Mol. Sci. 25 (4), 1992. doi:10.3390/ijms25041992
Russell, A. P., Lamon, S., Boon, H., Wada, S., Güller, I., Brown, E. L., et al. (2013). Regulation of miRNAs in human skeletal muscle following acute endurance exercise and short-term endurance training. J. Physiol. 591 (18), 4637–4653. doi:10.1113/jphysiol.2013.255695
Safdar, A., Abadi, A., Akhtar, M., Hettinga, B. P., and Tarnopolsky, M. A. (2009). miRNA in the regulation of skeletal muscle adaptation to acute endurance exercise in C57Bl/6J male mice. PLoS One 4 (5), e5610. doi:10.1371/journal.pone.0005610
Saikia, B. J., Bhardwaj, J., Paul, S., Sharma, S., Neog, A., Paul, S. R., et al. (2023). Understanding the roles and regulation of mitochondrial microRNAs (MitomiRs) in neurodegenerative diseases: current status and advances. Mech. Ageing Dev. 213, 111838. doi:10.1016/j.mad.2023.111838
Sanchis-Gomar, F., Arnau-Moyano, M., Daimiel, L., Lippi, G., Leischik, R., Vallecillo, N., et al. (2021). Circulating microRNAs fluctuations in exercise-induced cardiac remodeling: a systematic review. Am. J. Transl. Res. 13 (12), 13298–13309.
Sawada, S., Kon, M., Wada, S., Ushida, T., Suzuki, K., and Akimoto, T. (2013). Profiling of circulating microRNAs after a bout of acute resistance exercise in humans. PLoS One 8 (7), e70823. doi:10.1371/journal.pone.0070823
Seo, Y., Rhim, J., and Kim, J. H. (2024). RNA-binding proteins and exoribonucleases modulating miRNA in cancer: the enemy within. Exp. and Mol. Med. 56 (5), 1080–1106. doi:10.1038/s12276-024-01224-z
Sieland, J., Niederer, D., Engeroff, T., Vogt, L., Troidl, C., Schmitz-Rixen, T., et al. (2021). Effects of single bouts of different endurance exercises with different intensities on microRNA biomarkers with and without blood flow restriction: a three-arm, randomized crossover trial. Eur. J. Appl. Physiol. 121 (11), 3243–3255. doi:10.1007/s00421-021-04786-2
Siracusa, J., Koulmann, N., and Banzet, S. (2018). Circulating myomiRs: a new class of biomarkers to monitor skeletal muscle in physiology and medicine. J. Cachexia Sarcopenia Muscle 9 (1), 20–27. doi:10.1002/jcsm.12227
Smith, J. A. B., Murach, K. A., Dyar, K. A., and Zierath, J. R. (2023). Exercise metabolism and adaptation in skeletal muscle. Nat. Rev. Mol. Cell Biol. 24 (9), 607–632. doi:10.1038/s41580-023-00606-x
Snijders, T., Nederveen, J. P., McKay, B. R., Joanisse, S., Verdijk, L. B., van Loon, L. J. C., et al. (2015). Satellite cells in human skeletal muscle plasticity. Front. Physiology 6, 6–2015. doi:10.3389/fphys.2015.00283
Soci, U. P. R., Fernandes, T., Hashimoto, N. Y., Mota, G. F., Amadeu, M. A., Rosa, K. T., et al. (2011). MicroRNAs 29 are involved in the improvement of ventricular compliance promoted by aerobic exercise training in rats. Physiol. genomics 43 (11), 665–673. doi:10.1152/physiolgenomics.00145.2010
Sosnowska, B., Surma, S., and Banach, M. (2022). Targeted treatment against lipoprotein (a): the coming Breakthrough in lipid lowering therapy. Pharm. (Basel) 15 (12), 1573. doi:10.3390/ph15121573
Sripada, L., Singh, K., Lipatova, A. V., Singh, A., Prajapati, P., Tomar, D., et al. (2017). hsa-miR-4485 regulates mitochondrial functions and inhibits the tumorigenicity of breast cancer cells. J. Mol. Med. Berl. 95 (6), 641–651. doi:10.1007/s00109-017-1517-5
Sullivan, B. P., Nie, Y., Evans, S., Kargl, C. K., Hettinger, Z. R., Garner, R. T., et al. (2022). Obesity and exercise training alter inflammatory pathway skeletal muscle small extracellular vesicle microRNAs. Exp. Physiol. 107 (5), 462–475. doi:10.1113/EP090062
Sylvers-Davie, K. L., and Davies, B. S. J. (2021). Regulation of lipoprotein metabolism by ANGPTL3, ANGPTL4, and ANGPTL8. Am. J. Physiol. Endocrinol. Metab. 321 (4), E493–e508. doi:10.1152/ajpendo.00195.2021
Thau, H., Neuber, S., Emmert, M. Y., and Nazari-Shafti, T. Z. (2024). Targeting lipoprotein(a): can RNA therapeutics provide the next step in the prevention of cardiovascular disease? Cardiol. Ther. 13 (1), 39–67. doi:10.1007/s40119-024-00353-w
Topkara, V. K., and Mann, D. L. (2010). Clinical applications of miRNAs in cardiac remodeling and heart failure. Per Med. 7 (5), 531–548. doi:10.2217/pme.10.44
Uhlemann, M., Möbius-Winkler, S., Fikenzer, S., Adam, J., Redlich, M., Möhlenkamp, S., et al. (2014). Circulating microRNA-126 increases after different forms of endurance exercise in healthy adults. Eur. J. Prev. Cardiol. 21 (4), 484–491. doi:10.1177/2047487312467902
Ultimo, S., Zauli, G., Martelli, A. M., Vitale, M., McCubrey, J. A., Capitani, S., et al. (2018). Influence of physical exercise on microRNAs in skeletal muscle regeneration, aging and diseases. Oncotarget 9 (24), 17220–17237. doi:10.18632/oncotarget.24991
van Rooij, E., Sutherland, L. B., Liu, N., Williams, A. H., McAnally, J., Gerard, R. D., et al. (2006). A signature pattern of stress-responsive microRNAs that can evoke cardiac hypertrophy and heart failure. Proc. Natl. Acad. Sci. U. S. A. 103 (48), 18255–18260. doi:10.1073/pnas.0608791103
Viereck, J., Kumarswamy, R., Foinquinos, A., Xiao, K., Avramopoulos, P., Kunz, M., et al. (2016). Long noncoding RNA Chast promotes cardiac remodeling. Sci. Transl. Med. 8 (326), 326ra22–ra22. doi:10.1126/scitranslmed.aaf1475
Wadley, G. D., Lamon, S., Alexander, S. E., McMullen, J. R., and Bernardo, B. C. (1985). Noncoding RNAs regulating cardiac muscle mass. J. Appl. Physiol. 127 (2), 633–644. doi:10.1152/japplphysiol.00904.2018
Wang, B., Zhang, A., Wang, H., Klein, J. D., Tan, L., Wang, Z. M., et al. (2019a). miR-26a limits muscle wasting and cardiac fibrosis through exosome-mediated microRNA transfer in chronic kidney disease. Theranostics 9 (7), 1864–1877. doi:10.7150/thno.29579
Wang, B., Zhang, C., Zhang, A., Cai, H., Price, S. R., and Wang, X. H. (2017a). MicroRNA-23a and MicroRNA-27a mimic exercise by ameliorating CKD-induced muscle atrophy. J. Am. Soc. Nephrol. 28 (9), 2631–2640. doi:10.1681/ASN.2016111213
Wang, F., Liang, R., Tandon, N., Matthews, E. R., Shrestha, S., Yang, J., et al. (2019b). H19X-encoded miR-424(322)/-503 cluster: emerging roles in cell differentiation, proliferation, plasticity and metabolism. Cell Mol. Life Sci. 76 (5), 903–920. doi:10.1007/s00018-018-2971-0
Wang, H., Liang, Y., and Li, Y. (2017b). Non-coding RNAs in exercise. Non-coding RNA Investig. 1, 10. doi:10.21037/ncri.2017.09.01
Wang, L., Lv, Y., Li, G., and Xiao, J. (2018). MicroRNAs in heart and circulation during physical exercise. J. Sport Health Sci. 7 (4), 433–441. doi:10.1016/j.jshs.2018.09.008
Wang, X., Chen, S., Gao, Y., Yu, C., Nie, Z., Lu, R., et al. (2021). MicroRNA-125b inhibits the proliferation of vascular smooth muscle cells induced by platelet-derived growth factor BB. Exp. Ther. Med. 22 (2), 791. doi:10.3892/etm.2021.10223
Wang, X. H. (2013). MicroRNA in myogenesis and muscle atrophy. Curr. Opin. Clin. Nutr. Metab. Care 16 (3), 258–266. doi:10.1097/MCO.0b013e32835f81b9
Wohlwend, M., Laurila, P. P., Williams, K., Romani, M., Lima, T., Pattawaran, P., et al. (2021). The exercise-induced long noncoding RNA CYTOR promotes fast-twitch myogenesis in aging. Sci. Transl. Med. 13 (623), eabc7367. doi:10.1126/scitranslmed.abc7367
Wu, J., Venkata Subbaiah, K. C., Jiang, F., Hedaya, O., Mohan, A., Yang, T., et al. (2021). MicroRNA-574 regulates FAM210A expression and influences pathological cardiac remodeling. EMBO Mol. Med. 13 (2), e12710. doi:10.15252/emmm.202012710
Xu, D., Di, K., Fan, B., Wu, J., Gu, X., Sun, Y., et al. (2022). MicroRNAs in extracellular vesicles: sorting mechanisms, diagnostic value, isolation, and detection technology. Front. Bioeng. Biotechnol. 10, 948959. doi:10.3389/fbioe.2022.948959
Xu, T., Liu, Q., Yao, J., Dai, Y., Wang, H., and Xiao, J. (2015). Circulating microRNAs in response to exercise. Scand. J. Med. Sci. Sports 25 (2), e149–e154. doi:10.1111/sms.12421
Yamamoto, H., Morino, K., Nishio, Y., Ugi, S., Yoshizaki, T., Kashiwagi, A., et al. (2012). MicroRNA-494 regulates mitochondrial biogenesis in skeletal muscle through mitochondrial transcription factor A and Forkhead box j3. Am. J. Physiol. Endocrinol. Metab. 303 (12), E1419–E1427. doi:10.1152/ajpendo.00097.2012
Zacharewicz, E., Lamon, S., and Russell, A. P. (2013). MicroRNAs in skeletal muscle and their regulation with exercise, ageing, and disease. Front. Physiology 4, 266. doi:10.3389/fphys.2013.00266
Zhang, H., Wang, F., Pang, X., Zhou, Y., Li, S., Li, W., et al. (2023). Decreased expression of H19/miR-675 ameliorates muscle atrophy by regulating the IGF1R/Akt/FoxO signaling pathway. Mol. Med. 29 (1), 78. doi:10.1186/s10020-023-00683-w
Zhang, J., Sun, H., Zhu, L., Du, L., Ma, Y., Ma, Y., et al. (2022). Micro ribonucleic acid 27a aggravates ferroptosis during early ischemic stroke of rats through nuclear factor erythroid-2-related factor 2. Neuroscience 504, 10–20. doi:10.1016/j.neuroscience.2022.09.014
Zhang, S., Lin, S., Tang, Q., and Yan, Z. (2021). Knockdown of miR-205-5p alleviates the inflammatory response in allergic rhinitis by targeting B-cell lymphoma 6. Mol. Med. Rep. 24 (5), 818. doi:10.3892/mmr.2021.12458
Zhang, X., Wang, W., Zhu, W., Dong, J., Cheng, Y., Yin, Z., et al. (2019). Mechanisms and functions of long non-coding RNAs at multiple regulatory levels. Int. J. Mol. Sci. 20 (22), 5573. doi:10.3390/ijms20225573
Zhang, Y., He, N., Feng, B., and Ye, H. (2020). Exercise mediates heart protection via non-coding RNAs. Front. Cell Dev. Biol. 8, 182. doi:10.3389/fcell.2020.00182
Zhang, Y., Yang, L., Gao, Y. F., Fan, Z. M., Cai, X. Y., Liu, M. Y., et al. (2013). MicroRNA-106b induces mitochondrial dysfunction and insulin resistance in C2C12 myotubes by targeting mitofusin-2. Mol. Cell Endocrinol. 381 (1-2), 230–240. doi:10.1016/j.mce.2013.08.004
Zhao, W., Yin, Y., Cao, H., and Wang, Y. (2021). Exercise improves endothelial function via the lncRNA MALAT1/miR-320a Axis in obese children and adolescents. Cardiol. Res. Pract. 2021, 8840698. doi:10.1155/2021/8840698
Zhao, Z., Li, B., Cheng, D., and Leng, Y. (2025). miRNA-541-5p regulates myocardial ischemia-reperfusion injury by targeting ferroptosis. J. Cardiothorac. Surg. 20 (1), 63. doi:10.1186/s13019-024-03260-2
Zhou, Q., Shi, C., Lv, Y., Zhao, C., Jiao, Z., and Wang, T. (2020). Circulating microRNAs in response to exercise training in healthy adults. Front. Genet. 11, 256. doi:10.3389/fgene.2020.00256
Zhuang, X., Gao, F., Shi, L., Liu, W., Wang, W., He, X., et al. (2021). MicroRNA-146b-3p regulates the dysfunction of vascular smooth muscle cells via repressing phosphoinositide-3 kinase catalytic subunit gamma. Bioengineered 12 (1), 2627–2638. doi:10.1080/21655979.2021.1937904
Keywords: exercise, microRNAs, long non-coding RNAs, cardiovascular diseases, heart failure
Citation: Yang G and Yang W (2025) Regulating the expression of exercise-induced micro-RNAs and long non-coding RNAs: implications for controlling cardiovascular diseases and heart failure. Front. Mol. Biosci. 12:1587124. doi: 10.3389/fmolb.2025.1587124
Received: 04 March 2025; Accepted: 01 May 2025;
Published: 20 May 2025.
Edited by:
Lynda Bourebaba, Wroclaw University of Environmental and Life Sciences, PolandReviewed by:
Bhola Shankar Pradhan, Łukasiewicz Research Network – PORT Polish Center for Technology Development, PolandYuquan Tong, The Scripps Research Institute, United States
Feng Jiang, Stanford University, United States
Copyright © 2025 Yang and Yang. This is an open-access article distributed under the terms of the Creative Commons Attribution License (CC BY). The use, distribution or reproduction in other forums is permitted, provided the original author(s) and the copyright owner(s) are credited and that the original publication in this journal is cited, in accordance with accepted academic practice. No use, distribution or reproduction is permitted which does not comply with these terms.
*Correspondence: Guobiao Yang, Z2J5YW5nQHhpZGlhbi5lZHUuY24=