- São Carlos Institute of Physics, University of São Paulo, São Carlos, Brazil
Perovskite structures have attracted scientific interest as a promising alternative for water treatment due to their unique structural, high oxidation activity, electronic stability, and optical properties. In addition, the photocatalytic activity of perovskite structures is higher than that of many transition metal compounds. A critical property that determines the high-performance photocatalytic and optical properties is the band gap, lifetime of carrier charge, and band edges relative to the redox potential. Thus, the synthesis/processing and study of the effect on the band gap, lifetime of carrier charge, and band edges relative to the redox potential in the development of high-performance photocatalysts for water treatment are critical. This review presents the basic physical principles of optical band gaps, their band gap tunability, potentials, and limitations in the applications for the water treatment. Furthermore, it reports recent advances in the synthesis process and comparatively examines the band gap effect in the photocatalytic response. In addition to the synthesis, the physical mechanisms associated with the change in the band gap have been discussed. Finally, the conclusions of this review, along with the current challenges of perovskites for photocatalysis, are presented.
1 Introduction
The development of human society today diverges from preserving life’s most essential natural resource, water. Water pollution is generated by different sources of industrialization, conventional fossil fuel use, and population growth (Yousif and Gurjar, 2018), which leads to an increase in demand for clean water supply. The global challenge is achieving environmental sustainability since only less than 1% of fresh water on the earth is readily accessible (Reid et al., 2019). In addition to the increase in both the volumes and the concentrations of polluted water, a decrease in biodegradability of the pollutants was observed. Such problems have created a strong incentive for the development of new sustainable and high-efficiency materials, when compared to those already existing (Patel et al., 2019; Schanze et al., 2020), aiming at the abatement of pollutants. In this context, the solar energy source (as future sustainability) is very attractive in photocatalytic materials due to their remarkable effectiveness for water treatment. Solar energy harvesting is also enjoyed in other fields as photothermal and photovoltaic. Photocatalytic materials are a rapidly growing field for potential application with clean and renewable energy sources (Belver et al., 2019; Odling and Robertson, 2019; Xia et al., 2021). The photocatalytic materials absorb sunlight to produce electron (
Recent advances in nanostructured materials have opened the way for new types of semiconductor catalysts and improving TiO2 or ZnO for the destruction of polluting materials (Roy and Chakraborty, 2021; Chen et al., 2020a). Among the various classes of materials explored, perovskite (ABX3) has been given much interest due to its framework’s easiness and versatility, excellent photostability, and photocatalytic nature (Zhang et al., 2016; Hwang et al., 2017), and its structural integrity representation is shown in Figure 1. The A-site ions are generally rare or alkaline earth elements (often occupied by a larger-size cation), B-site ions are usually transitioned metal elements (occupied by a smaller cation), and X is often a chalcogen or halogen. The advantage of perovskite structures is that they can incorporate a wide variety of ions of various sizes and charges showing great flexibility of composition; besides, their crystal structure can remain unchanged (Tasleem and Tahir, 2020). However, most perovskites are usually distorted. The partial substitution of these cations, both A-site and B-site ions, by other foreign ions leads to changes in the oxidation states of metal ions. The B-site substitution can result in the double perovskite formula (A2BB′X6).
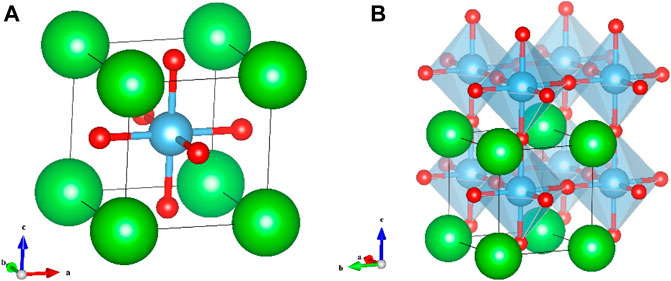
FIGURE 1. (A) Unit cell of ABX3 perovskite. (B) Crystal structure of a simple perovskite compound. Blue color (A-site), green color (B-site), and red color (X-site).
Furthermore, the substitutions are accompanied by the formation of intrinsic defects due to the mechanism of charge compensation (Liu et al., 2020a). Kurbakov (2010) stated that replacing an A3+ with A2+ results in lattice distortion and the formation of oxygen vacancies. Golsdschmidt (1962) proposed that the formation of a perovskite structure can be governed by a tolerance factor, which predicts the stability and distortion of the perovskite structure, as follows:
where
The formation of single and double perovskite materials has exceptional properties for a variety of applications such as ferroelectricity (Cohen, 1992), ferromagnetism (Clabel H. et al., 2016), multiferroic (Clabel H. et al., 2014), electrocatalysis (Hwang et al., 2017), optical (Clabel H. et al., 2017), and photocatalytic (Grabowska, 2016), among others (Kovalenko et al., 2017; Correa-Baena et al., 2017). Due to its application potential, researchers have made extensive efforts on perovskite material as a photocatalyst. As shown in Figure 2, an upward trend of the number of publications based on perovskite photocatalysts was observed from 2010 to 2021. Although there is growing research into perovskites for photocatalytic applications, there are still inherent limitations associated with the following: 1) the wide band gap (
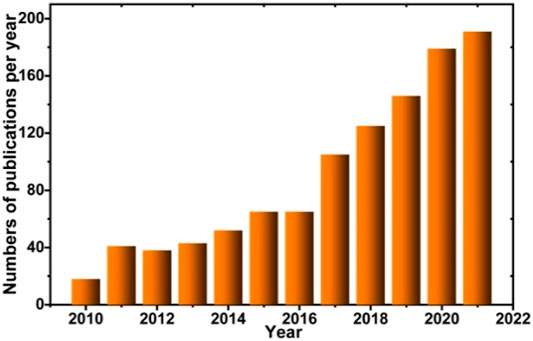
FIGURE 2. Number of publications per year and the cumulative number of publications on perovskites and photocatalysis from 2010 to 2021 from Scopus with work: perovskite and photocatalysis.
In this review, many single perovskite compounds have been reported to be photocatalysts for water treatment. We discuss the perovskite structures that have been considered, their properties, and current synthesis methods to form oxide and halide perovskites. Following this, we have summarized the recent reports addressing the influence of band gap and lifetime of charge carrier of perovskite structure in photocatalytic activity efficiency for water treatment. Factors associated with the tunable band gap will be discussed. Finally, a summary of the recent advances in perovskite photocatalysts will be given.
2 Synthesis Methods of the Perovskite Particles
Among the strategies adopted in optimizing photocatalysts, the synthesis methods greatly impact their physical properties. Several strategies were developed to adjust the particle size, surface area, shape, and composition to optimize the photocatalytic process. The photocatalytic activity of perovskite structure dramatically depends upon the specific surface area and band gap as well. The high surface area provides enough reaction sites and improves the adsorption properties of the photocatalysts; furthermore, an appropriate band gap allows for tuning the absorption of a particular wavelength region of light. Thus, the relevance in the synthesis methods is significant in the photocatalytic performance for water treatment.
2.1 Solid-State Reaction
Synthesis by solid-state reactions (SSRs) results in a mixture of nitrates, carbonates, acetate, and oxides, with desired stoichiometric ratios. Perovskites are frequently synthetized by SSRs, including mixing, milling, and calcination at high temperatures to form the perovskite phase. During this process, surface chemical defect and particle size effects affect the process of recombination of photoinduced charge carriers (
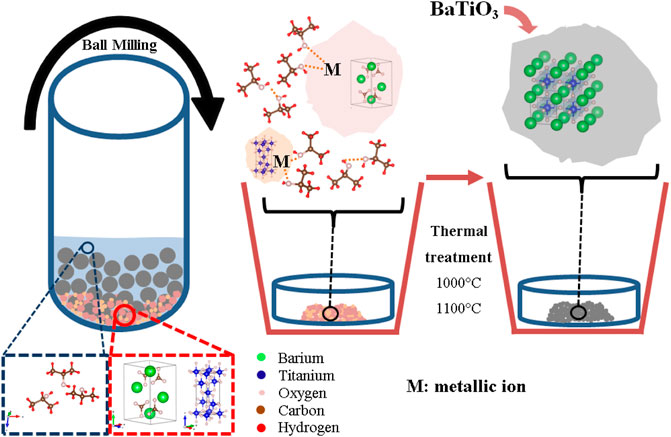
FIGURE 3. Schematic views of the synthesis process by solid-state reaction to prepare nanopowders of BaTiO3 (Clabel H et al., 2020).
2.2 Sol-Gel
Perovskite structure synthesized by sol-gel method involves the formation of a sol by dissolving the metal alkoxide, metal-organic, or metal-inorganic salt precursors in a suitable solvent, which is subsequently condensed to form a gel, followed by pre-calcination (removal of organic material) and calcination at much lower temperatures than those of the solid-state reaction for the formation of perovskite structure. Some frequently used gels include ethanol, ammonia, polyvinyl alcohol, and citric acid, among others. Furthermore, used precursors, the pH value, and the heat treatment schedule play an essential role in affecting the properties of the perovskite structure (Brutchey and Morse, 2006; Ismael and Wark, 2019).
2.3 Hydrothermal and Solvothermal
Hydrothermal and solvothermal methods require heating an aqueous suspension of insoluble salts, that is, reaction mixture, in an autoclave at high temperature and pressure conditions where the crystallization of the desired phase is taking place. The product generates perovskite powders with high crystallinity and purity, low concentration of defects, and controllable size distribution and morphologies. High photocatalytic activity in KNbO3 materials for H2 evolution from aqueous CH3OH solutions was reported for high surface area and crystallinity (Ding et al., 2008). In perovskites of Cr-doped SrTiO3, high surface area (19.3–65.4 m2 g−1), good crystallinity, and small crystalline size (20–30 nm) were obtained using a sol-gel hydrothermal method (Yu et al., 2011). Solvothermal synthesis of BaTiO3, PbTiO3, SrTiO3, and BaZrO3 leads to particles with different morphologies (Caruntu et al., 2015). The characteristic of perovskite powders with unique optical properties makes the method advantageous in optimizing photocatalysts.
2.4 Co-Precipitation
In the co-precipitation method, the starting materials used have been taken in stoichiometric proportions in molar solutions. These are denominated precursors such as metal acetate, sulfates, chloride, or nitrate. Afterward, the solutions have been poured into a precipitant solution (e.g., NaOH or Na2CO3) under constant stirring. In this step, the nucleation process forms a large number of small particles, and then the post nucleation process and Ostwald ripening and aggregation dramatically affect the product size, morphology, and properties. During co-precipitation, the pH value of the solution has been controlled. Precipitation takes place in the solution at a determined temperature (>70°C), in which the supersaturation conditions help to induce precipitation as the result of a chemical reaction (Chang Chien et al., 2022). The precipitates are collected and washed several times and then dried in a hot air oven at a temperature >70°C. The advantages of this method are the high yield, high product purity, the lack of necessity to use organic solvents, easy reproducibility, and low cost (Letifi et al., 2021). However, the properties of the obtained particles, such as size, shape, and composition, are highly dependent on the reaction parameters such as temperature, pH, and ionic strength (Haron et al., 2018). Furthermore, co-precipitation is also a more straightforward method; however, it is not stable and hence is stabilized by using a surfactant.
2.5 In Situ Anion Exchange Reaction
This method was demonstrated to be an effective strategy to synthesize nanocrystals with engineered compositions and structures (Fenton et al., 2018). Also, it has attracted increasing attention in the fabrication of heterostructure photocatalysts to improve photocatalytic performance. The ion-exchange reaction is normally performed in molten salt or solvothermal conditions. Heating to a high temperature (e.g., 300°C or above) is needed in the former case, often leading to decomposition of the material or the formation of a more stable structure. Using ion-exchange reactions under mild solvothermal conditions would be preferable in many cases, wherein the reaction is performed at the lowest possible temperature (below 200°C) sufficient to accelerate exchange kinetics (Park et al., 2017). Different perovskites by anion exchange reaction (see Figure 4) were mentioned in the literature showing enhanced photocatalytic properties, like CsPbX3 (X = Cl, Br, I) (Akkerman et al., 2015; Loiudice et al., 2019), Cs2AgBiX6, (X = Cl or Br) (Creutz et al., 2018), and Cs2AgBiX6 (X = Cl, Cl0.5Br0.5, Br, Br0.5I0.5, I) (Wu et al., 2021).
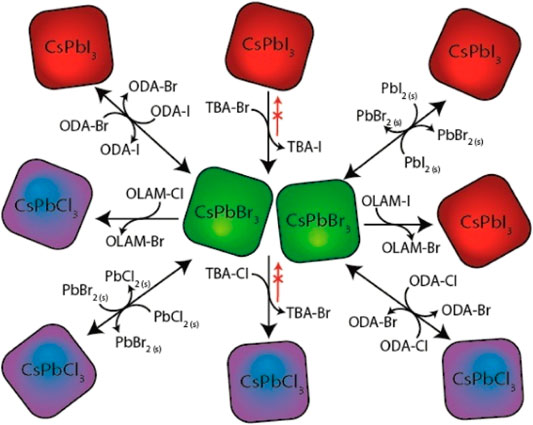
FIGURE 4. Schematic views of the different routes and precursors for the anion exchange reactions on CsPbX3 (X = Cl, Br, I) nanocrystals (Akkerman et al., 2015).
3 Perovskite Structure in Photocatalysis
Human activity and industrialization growth lead to environmental pollution, especially clean water reduction. Thus, perovskite structures based on the crystal structure, physical properties, and their strategies help degrade pollutants, which are highly active in the presence of ultraviolet (UV) energy radiations but weakly active in visible (vis) and near-visible (NIR) light. On the other hand, in addition to the new tolerance factor
The
4 Photocatalytic Mechanism
Understanding the source of solar energy irradiation is the first step when enhancing the optical properties and, consequently, photocatalytic activity. Photocatalytic activity from solar energy has become an essential topic in the scientific community to solve the polluted water problem and eliminate pollutants in natural aquatic systems. In this sense, the solar energy irradiation contributed with different ultraviolet (UV), visible (VIS), and near-infrared (NIR) bands. The solar energy irradiation is lower in the UV band, at 3.4%. The solar energy irradiation in the VIS band understands a fraction at 42%. The most considerable portion of total solar energy irradiation occurs in the NIR band, at 54.6%. Solar-driven photocatalyst involves the generation of active oxidizing species under solar irradiation, in the presence of a catalyst, as a perovskite structure. A suitable band gap and high photoinduced charge separation efficiency are critical factors for ideal photocatalysts. A severe limitation of most photocatalysts is that they can only absorb photons with light wavelengths <400 nm (UV band), which accounts for 3.4% of the total solar energy irradiation. As described before, solar energy irradiation in the VIS band is high, so the development and optimization of photocatalysts with high efficiency and chemical stability in the VIS band will favor the photocatalytic reaction processes.
Different pathways characterize the photocatalytic process, and they are described as follows:
(i) photons with higher energy (
(ii) subsequently, the electrons (
(iii) the production electron (
The photocatalytic activity depends on the generation and the separation of
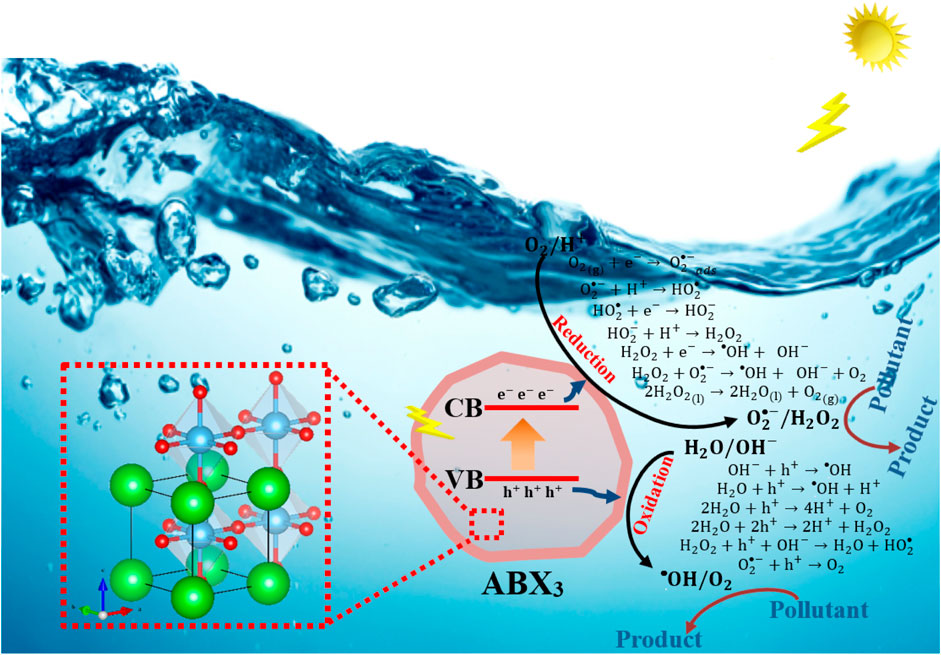
FIGURE 5. Schematic representation of the band structure and mechanism of photogenerated charge carrier transfer to photocatalytic reaction on perovskites.
In addition to the limitation mentioned above for photocatalyst application, other factors can influence photocatalytic activity, as described in the following section. An efficient photocatalyst should contain the following: 1) a band gap ≥1.2 eV to provide energetic electrons and a band gap ≤3.0 eV to allow effective absorption of overlap with the solar spectrum and 2) a high surface area to optimize the redox reaction and minimize the recombination process.
4.1 Band Gaps in Perovskite Materials
In condensed matter physics, the band gap energy is an energy region where the electronic states cannot exist. It separates the upper part of the valence band from the lower part of the conduction band and characterizes the electronic properties of the solids depending on the magnitude of this separation (measured in eV). One way to calculate band gaps from experimental data for nanostructured semiconductor materials, such as perovskites, is through the model proposed by Tauc (Tauc and Menth, 1972), who performed the analysis of the incident photon energy hν and the absorption coefficient α in the following expression:
where coefficient A is a proportionality constant that depends on the electronic transition probability, Ei is the edge of the optical band, and r is a real number, which depends on the type of band–band transition, as seen in Table 2, which indicates whether the transition is allowed or forbidden.
For direct transitions, the values of r are 1/2 and 3/2 for the allowed and forbidden processes, respectively, while for indirect transitions, the values of r are 2 and 3 for the allowed and forbidden processes, respectively (Mott, 1968).
So, materials with allowed band–band transitions can classify these transitions into two types: 1) direct transition, where the minimum energy state of the conduction band and the maximum energy state of the valence band are aligned with each other, that is, they have the same value of momentum k in the Brillouin zone, and 2) indirect transition, where the respective minimum and maximum energy states of the conduction and valence bands are misaligned, for example, due to the contribution of the phonons of the lattice (Zanatta, 2019), as can be seen in Figure 6.
Perovskites have unique optical properties related to a particle size such as high quantum yield, ionic conductivity, tunable composition-dependent luminescence throughout the visible region, multiphoton absorption and corresponding stimulated emission spectra, and good quantum confinement, which are essential in the field of light-active photocatalyst design because many materials are only active in the UV region (4%), so the use of nanostructured perovskites is essential because visible light occupies 45% of the spectrum of sunlight due to its wide band gap. Photocatalysts with a suitable band gap absorb sunlight, which produces an electronic transition from the conduction band to the valence band, leaving a hole in the first band; then, the photoinduced charge carriers separate and transfer reaction sites for, finally, the oxidation and reduction reactions to occur at the reaction sites. To increase photocatalytic efficiency, wide band gaps with adequate energy levels must be obtained (Liang et al., 2020; Xiao et al., 2020). The scaling influences the band gap value of nanostructured perovskite photocatalysts due to quantum confinement (Ou et al., 2019); other factors that also alter the perovskite band gap, in addition to size, are shape, defects, and doping, which we will discuss next.
4.1.1 Size Particle Effect on Band Gap
It is known that the nanostructuring of materials influences the physical properties of solids, and this is not alien to perovskites. The catalytic properties of perovskites depend on the size; this phenomenon is called the size effect and is fundamental in photocatalytic reactions (Hoshina, 2013), although other properties are also affected (Liu et al., 2017; Clabel H. et al., 2019; Clabel H. et al., 2021b). The band gap is a parameter key in photocatalytic reactions influenced by the particle size. The size-dependent band gap has been carried by many experimental and theoretical researchers (Schilling et al., 2018; Liu et al., 2019). Among the different characteristics in the perovskite structure is the band gap variation (Eg) with the particle size change. Size-dependent band gap of particles with perovskite structure is a well-known and studied quantum confinement effect (Efros, 1982).
Physical–chemical variables can affect the particle size, such as 1) synthesis method, 2) thermal treatment, 3) phases ratio, 4) mechanical property, 5) type bonding utilized, 6) milling time, and 7) concentration of dopant (Li et al., 2019; Liu et al., 2021). In nanoscale materials, the bands are formed by almost discrete energy levels; this is due to the confinement of a smaller size of the particle that also groups together a small number of atoms, thus decreasing the superposition of energy levels, so there is an increase in the separation between bands. Then, the confinement of the electrons within the atoms means the limitation of their movement to a space with dimensions of the de Broglie wavelength, and then they will present a lower electrical conductivity (Pellegrini et al., 2005; Li and Li, 2006).
Liu et al. (2021) reported an analysis of the relationship between band gap energy and the Ba:Sr relationship in the Ba1-xSrxTiO3 synthesized using the sol-gel method. Table 3 shows that the band gap increases as the grain size decreases; the lowest band gap energy is 3.027 eV, corresponding to the 7:3 ratio, which has more effective use of sunlight due to the lowest band gap of nanoparticles and absorption of visible light at wavelengths less than 409.6 nm, which promotes a higher photocatalytic hydrogen production rate. On the other hand, Ramakanth and James Raju (2014) calculated the band gap using the Tauc plot of BaTiO3 nanoparticles synthesized using the sol-gel method at different calcination temperatures, as shown in Table 3.
Furthermore, other types of morphology are also dependent on size, as revealed by Oksenberg et al. (2020), and they show the lattice distortions and size-dependent band gap in CsPbBr3 perovskite nanowires. They will identify that the contribution to the band gap modulation is height-dependent on the nanowire. In the excitation configuration, the emission spectra blue-shift with decreasing height (thickness) of the nanowires, and such shift reflects the ability of the nanowires to absorb light of different wavelengths. Thus, thinner nanowires have higher band gap energies than thicker nanowires. With decreasing nanowire heights from ∼400 to ∼100 nm, a significant emission blue-shift from ∼525 to ∼490 nm (∼2.36–∼2.53 eV) was observed. On the other hand, Pei-Lun et al. (Hsieh et al., 2019) explored the effects of the particle size distribution and faces on the charge carrier dynamics and the photocatalytic activity in SrTiO3 cubes. The cube’s size affects the band gap, showing more blue-shifted light absorption than the other particles exposing significant {110} faces. However, lattice distortion in the corners of the {100}-truncated rhombic dodecahedra are far more efficient than cubes at photodegradation of methylene blue and photocatalyzed hydrogen evolution from water in the presence of methanol, as shown in Figure 7G. Therefore, the photocatalytic reaction depends on particle size and faces of SrTiO3, suggesting surface facet control as a strategy for enhancing photocatalyzed hydrogen production. Clabel H et al. (2020) showed that the milling time and calcination temperature modified the band gap; in addition, a lattice strain was observed, caused both by milling time and calcination. In this sense, light absorption and slight shift enhancement can be associated with strain, particle size, and chemical defects in their surface (Clabel H et al., 2020). Also, the particle strain is most likely caused by lattice defects. These lattice defects can act as traps for photoexcited electrons and holes, leading to a decrease in the efficiency of the photocatalyst. Therefore, the small values of strains suggest the presence of fewer lattice defects, which might lead to a decrease in the recombination of photogenerated charge carriers and thereby an increase in photocatalytic activity.
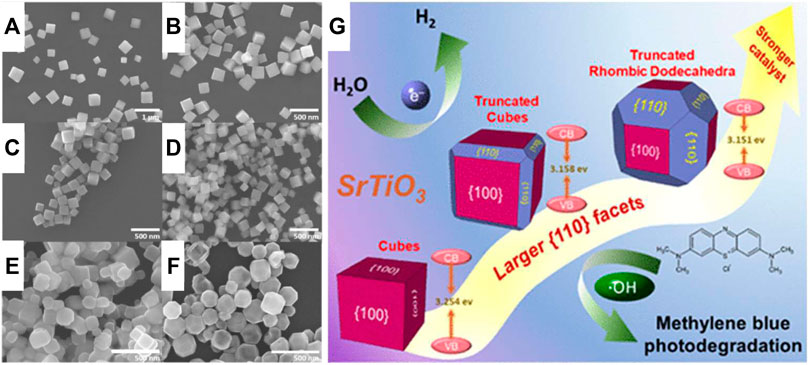
FIGURE 7. SEM images of the synthesized SrTiO3 cubes with tunable edge lengths of (A) 290 nm, (B) 250 nm, (C) 200 nm, and (D) 160 nm, (E) edge-truncated cubes, (F) {100}-truncated rhombic dodecahedra, and (G) The cubes display slight size-related optical band shifts, and they show clearly more blue-shifted light absorption than the other particles exposing significant {110} faces. These factors lead to a high photocatalytic activity (Hsieh et al., 2019).
Several authors show the relationship between particle size and surface area (Parida et al., 2010). In general, smaller particles in a sample lead to a higher surface area, as reported in the literature (Parida et al., 2010; see Table 3). Also, nanomaterials’ small size and large surface area are preferred to facilitate the charge transfer between the catalyst and the redox molecules, thus achieving a high catalyst activity (Hsieh et al., 2019).
4.1.2 Shape Effect on Band Gap
The band gap energy depends, in addition to the size, on the nanomaterials’ shape, and this is based on the fact that the cohesive energy of nanostructured materials is different from that of bulky materials (Sapra and Sarma, 2004). The surface/volume ratio of nanomaterials plays an important role in characterizing their properties; therefore, it is essential to analyze them. The energy due to the contributions of the interior and surface atoms is defined as the cohesive energy, which we can express in relation to the energies for nano and bulky materials as follows:
where
where
where D and
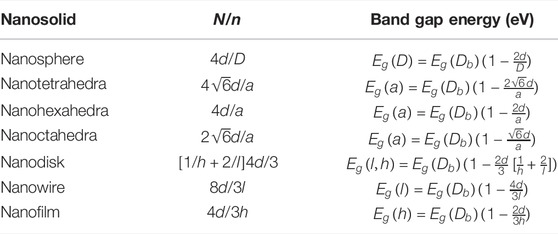
TABLE 4. N/n ratio for different nanosolids (Qi and Wang, 2004; Qi, 2005).
The band gap of perovskites can be broadly tuned with a light emission that varies from the wavelength of ultraviolet (UV) to near infrared (NIR). Quingdong et al. (Ou et al., 2019) reported that this was due to dimensionality. In the case specific to hexahedric metal halide perovskites, due to quantum confinement, band gap energy increases in the counterpart of its bulky counterpart continuously by gradually reducing the size of FaPbI3 perovskites (Li et al., 2018; see Table 5).
4.1.3 Effect of Defect on Band Gap
Particles formed after the synthesis process and thermal treatment contains surface defect owing to the reaction process. In this sense, it is essential to understand the nature and influence of surface chemical states on the photocatalytic activity of perovskites for further optimization of this photocatalytic system. Some analysis techniques such as X-ray photoelectron spectroscopy (XPS) allowed a quantitative and qualitative analysis of these defects and chemical states of the surface composition (Patil et al., 2021). This review will discuss the consequences of surface defects in the band gap and their consequence on photocatalytic activity, and the nature of these superficial states will not be analyzed. Under these circumstances, the nanostructured synthesis of perovskite structure has become an effective tool for diminishing surface states’ contribution toward the optical properties because they have a large surface-to-volume ratio. Although the band gap variation is dependent on particle size, it also might be due to a surface effect (Oksenberg et al., 2020).
Defects are known to manifest themselves in the band structure of a material as an energy level within the band gap that depends on the properties of the defect. When the defect is created (product by the example of calcination or doping), such as oxygen vacancy from the crystal lattice of perovskite structure of SrTiO3, BaTiO3, SrTiO3, and SrSnO3, among others, two electrons are released into the conduction band as follows:
Theoretical study using first principles showed that the substitution of 3D cations induces an occupied energy level either in band valence or in band gap on account of the t2g level of substituent (Fang et al., 2019; Zhu et al., 2019). Fang et al. (2019) showed that the substitution of metal cation in the BixSr1-xTiO3 structure gives rise to energy levels that appeared within the forbidden band and caused a narrowing of the band gap. The Materials Project initiative (Jain et al., 2013) presents a compilation of band gap energies from different perovskite materials from DFT results (see Table 6). Other parameters, that is, variation in crystallite size, structural deformation, and surface area/volume ratio, might have a specific effect on the optical energy band gap. Although many methods, such as modulating band gap, employment of composite semiconductors, metallic or non-metallic doping, regulating nanoscale morphology and structure, and introducing defects in the lattice, have been utilized to enhance the photocatalytic activity, effective carrier separation is still far from improving its photocatalytic efficiency.
4.1.4 Effect of Doping on Band Gap
Another parameter to band gap modulation is doping. The implication of the doping, with cations of different sizes, for band gaps was observed in the different perovskite types. Several studies showed a significant band gap tuning in doped-perovskite oxide as a function of the dopant element and the synthesis process. To LaNiO3 doped with ions of Gd, Fe, and Co with different ratios, a significant decline in the Eg values from 2.90 to 2.43 eV was shown. The band gap decrease might be due to the deformation induced in Fe–O octahedral or rearranging of the molecular orbitals (Ghafoor et al., 2021). However, the substitution generates certain impurities within the forbidden band, which facilitates the narrowing of the band gap. Yang et al. (2019) calculated the Eg for BiFeO3 and BiFeO3:Gd3+ being 2.28 and 2.17 eV, respectively. Such results indicate that BiFeO3:Gd3+ is an excellent photocatalyst and has potential applications in photocatalytic hydrogen production. They indicate that the doping Gd3+ induces the formation levels in the forbidden band, leading to a decrease in Eg; however, increased oxygen vacancies also exist, which could contribute to the decrease of Eg. On the other hand, it is clear that the doping is escorted by the generation of defects that are likely to behave as recombination centers of
In halide perovskites, different physical properties can be modified by substitution in the A-site, B-site, or X-site. The A-site substitution can improve the overall stability by tuning the tolerance factor. B-site substitution can reduce the harmful Pb content and improve phase stability by altering the B–X bond length. X-site substitution can tune the band gap by changing the ratio of mixed-halide ions (Lu et al., 2020). The variation in the band gaps in halide perovskites changes with cell volume (Borriello et al., 2008), as follows:
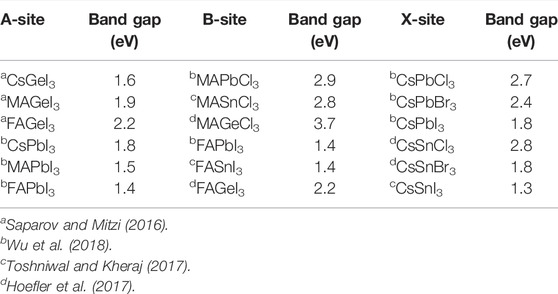
TABLE 7. Band gap values following the substitution in the A-site, B-site, and X-site in halide perovskites.
4.2 Lifetime of the Charge Carriers in Perovskites
For a photocatalyst, beyond the surface area, chemical defect, and band gap, the effective generation, separation, and recombination of
Analysis of BiFeO3:Gd3+ nanoparticles from photocurrent curves and electrochemical impedance spectra show a higher photocurrent intensity than BiFeO3, which suggests the higher efficient generation and separation of
In halide perovskites, the lifetime was determined by employing time-resolved photoluminescence spectroscopy. The result indicates that the substitution in the X-site decreases the lifetime values of 4.38, 0.73, and 0.86 ns for Cs2AgBiCl6, Cs2AgBi(Br0.5I0.5)6, and Cs2AgBiI6 nanocrystals, respectively (Wu et al., 2021). Much longer lifetimes have been found in Cs2AgBiBr6, around 660 ns (Slavney et al., 2016). The longer lifetime helps charge carriers in motion to reach active sites on the surface, consequently promoting the surface redox reactions. On the other hand, perovskite heterojunctions are emerging as a promising material for high-performance photocatalytic applications. The heterojunctions present two independent band gaps, which overlapped, and hence,
The importance of the lifetime of the charge carriers lies in the possibility of determining the efficiency of the photocatalytic performance, as longer-lived carriers will have a higher probability of reaching the interface, that is, recombination will be limited (or will not lead to recombination losses) by the time the electrons take to diffuse through the perovskite layer. Thus, in an efficient photocatalytic process, the charge carriers combine radiatively, and techniques such as time-resolved photoluminescence spectroscopy become a powerful tool to monitor the charge carrier lifetime and further the understanding of the perovskite photocatalytic process.
4.3 Band Edges Relative to the Redox Potential of Water Splitting
The band gap of the perovskite structures is essential for improving light absorption and charge carrier generation and depend on valence band and conduction band positions. These characteristics lead to high performance for CO2 reduction, N2 reduction, and water splitting. However, another important parameter is the position of valence- and conduction-band edges relative to the water redox potential levels, which are also critical factors governing photocatalytic water splitting activity, where the band edges should be close to the required redox potentials to enable the photocatalytic process. Such parameters will determine the hydrogen and oxygen evolution reactions under light. Thus, in the process of water splitting the charge transfer between the interface and optical transitions are depending strongly on the nature of the band edge. Hydrogen evolution reactions (HERs) occur when the conduction-band edges are more negative than the hydrogen evolution potential level, H+/H2. Oxygen evolution reactions (OERs) occur when the valence-band edges are more positive than the oxygen evolution potential level, H2O/O2. A representation is shown in Figure 8.
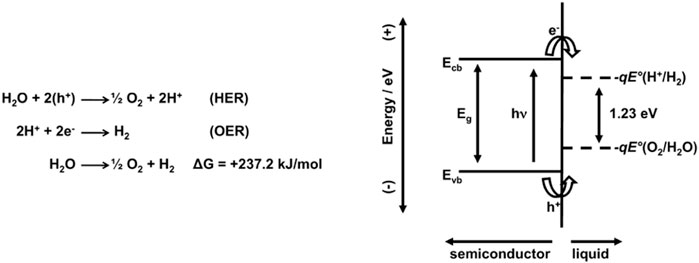
FIGURE 8. Oxygen evolution reaction (HER) and hydrogen evolution reaction (OER) for overall water splitting (under acidic conditions); ideal semiconductor material for splitting water at its surface under illumination with absolute energy scale represented [left vertical axis (−) and (+)] for Ecb and Evb and the electrochemical potentials given by −qE°, where E° is the reduction potential for both (H+/H2) and (O2/H2O) redox couples (Walter et al., 2010).
Although an appropriate band gap of 1.23 eV is necessary for the potential for the water splitting process, and its band edges do not straddle the HER and OERs. The band edge positions relative to the water redox potential levels are significantly influenced by the material, crystal structure, surface characteristics, and operating environment of a photocatalyst (Park and Kolpak, 2019). In perovskite structure MLnTa2O7 (M = Cs, Rb, Na, and H; Ln = La, Pr, Nd, and Sm), the photocatalytic activity is sensitive to Ln and M. The highest activity was obtained for Ln = Nd, where the activity decreased in the sequence of Rb > Cs > Na > H (Machida et al., 2003). The band gap energy is strongly dependent on Ln, but negligibly affected by M. The effect of Ln can be explained by the shift of the Ln 4f levels from the conduction-band edge to the valence-band edge with increasing numbers of Ln 4f electrons, suggesting that lanthanide 4f shell affects the positions of not only the valence-band edge but also the conduction-band edge and played an important role in photocatalytic reaction. The band edge alignments relative to the water redox potential levels at the interface directly influence light absorption, the energies of photogenerated charge carriers, and photocatalytic reactions at the surfaces. Burnside et al. (1999) argued that the nanometer-sized SrTiO3 particles induced a quantum confinement effect and resulted in changes in the band structure. The nanosheets had a 0.16-eV wider band gap and a 0.47-eV raised CB edge, as compared to a commercial WO3. Xie et al., on the other hand, assumed that the band structure of WO3 can be engineered by exploiting the crystal facet–dependent electronic structure effect. The authors showed that WO3 nanosheets with exposed {002} crystal facets reduced CO2 to CH4, whereas WO3 cubes with equal amounts of {200}, {020}, and {002} facets were inactive. The authors proposed that {002} crystal facets have a higher CB edge than the bulk WO3 due to the different atomic structures.
In halide perovskite CsPbX3 (X = Cl, Br, I) the modification of site-X significantly contributes to the valence-band edge with excitonic transition probability decreasing from X = Cl to Br to I, without changing the size of the nanocrystals (Ravi et al., 2016; Ravi et al., 2020). When compared, ZnS and CsPbBr3 both have similar valence-band edges, while the conduction-band edge of ZnS > that of CsPbBr3. However, the formation of CsPbBr3/ZnS (core/shell) nanocrystals enables the alignment at the core/shell interface where the hole is delocalized over both the core and shell and the electron is localized inside the core. In order to optimize the determination of band edge, studies using first-principles density functional theory calculations help to predict the environment-dependent band edge positions in vacuum and water (Park and Kolpak, 2019).
Some successful strategies of band structure include modification of the surface composition, quantum confinement, crystal facet–dependent electronic effect, and chemical modification. On the other hand, light-harvesting can be improved by modification with either organic or inorganic components: metal oxides and sulfides, carbon allotropes, dyes and other optically active molecules, and plasmonic nanoparticles. However, the inclusion of new strategies also gives rise to common problems, such as quenching of photoluminescence, multiple nanocrystalline cores inside a single shell, and an insulating shell that prevents the charge transport. Thus, an ideal photocatalyst to the CO2 conversion should possess 1) possibly a large surface area with favorable chemistry and energetics, 2) well-developed suitable porosity with good mass transfer properties, 3) a narrow band gap and good light-harvesting properties, 4) valence-band and conduction-band edges positioned properly to drive both CO2 reduction and H2O oxidation, 5) high purity and crystallinity, 6) proper morphology with a short bulk-to-surface distance, and 7) an efficient spatial charge separation (Marszewski et al., 2015).
Different physical and chemical parameters must be considered in perovskite material to make them photocatalyzed with high performance. Among them, the contributions of band gap, lifetime of the charge carriers, and band edges relative to the redox potential in perovskites play a vital role in photocatalytic activity. In this sense, different strategies were adopted to maximize the performance of the photocatalysts. As previously shown, particle size, doping, and chemical defect influence the band gap and lifetime of the charge carriers. Other factors are associated as shape, composition, substitution in the A-site, B-site, or X-site facility, and the light absorption owing to the narrowing of Eg; this has the objective of taking advantage of the dominant solar irradiation. In addition, fabricate photocatalytic material with a longer lifetime of charger carriers to suppress the process of
5 Applications of Halide and Oxide Perovskites to Water Treatment
Owing to its unique optical properties and versatility in halide and oxide perovskites, these materials become a compelling platform well suited to emerging applications. In this section, some photocatalysis-based applications of halide and oxide perovskites, including water splitting, photocatalytic CO2 reduction, and photocatalytic N2 reduction, are discussed briefly, in order to motivate new applications in water treatment.
5.1 Perovskite Materials to Water Splitting
Perovskite-based compounds are photoactive materials that have been widely investigated for photocatalytic water splitting (Wang et al., 2017b). This process originates from the conversion from the absorption of sunlight to produce an electron-hole pair for photocatalytic water splitting in oxygen and hydrogen, that is, water molecules are oxidized by the holes to protons and O2, and the released protons are reduced by the electrons to H2. The chemical reaction is 2H2O → 2H2 + O2. However, due to the loss of the charges through e−-h+ recombination processes, which decreases the number of electron−hole pairs via proton regeneration or light emission; the water-splitting efficiencies reported so far are limited. Other, no less important, factors making the high-efficiency photocatalytic materials difficult include inferior durability, slow kinetics, poor sunlight absorption, and poor charge carrier separation capability of photocatalysts. In addition, theoretical studies show that the energetic reaction required for the separation of water into H2 and O2 corresponds to 1.23 eV (1,100 nm) (Wang et al., 2017b). However, some perovskites (halide and oxides) do not have suitable band structures that are favorable, that is, 1.23 eV, impeding their use as highly efficient photocatalysts. Likewise, the lower level the conduction band should be more negative than the redox potential of H+/H2 (0.0 V vs. normal hydrogen electrode (NHE) at pH = 0), while the level tops the valence band should be more positive than the redox potential of O2/H2O (1.23 V vs. NHE at pH = 0) (Maeda and Domen, 2007). Halide and oxides perovskites the band gaps and band edges with respect to the redox potential is shown in Figures 9A,B. Among other advantages, perovskites offer flexibility in the composition, either in site A or B, which allows you to adjust the band structure, as mentioned in the previous section. The latter allows optimizing the water splitting and its functionalities.
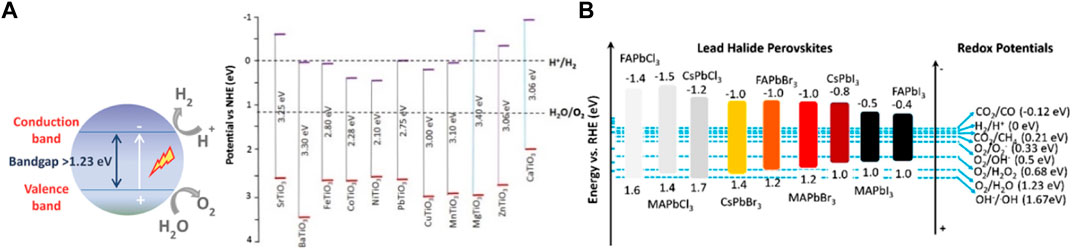
FIGURE 9. Band gaps and band edges with respect to the redox potential in (A) schematic of water splitting using photocatalyst of oxide perovskites (Bin Adnan et al., 2018) and (B) halide perovskites (Romani and Malavasi, 2020).
Oxide-based perovskite materials are photoactive materials, and the structure type ATiO3 (A = Ba, Sr, Ca, Mg, and Mn) has been reported as stable and suitable materials as water splitting, due to its functionality, and is likely to be the most cost-effective approach to achieve water splitting (Osterloh, 2008; Kudo and Miseki, 2009). Oxide perovskites reveal several advantages such as enhanced charge separation, excellent thermal stability, high chemical stability, large optical absorption coefficients, and extended electronic carrier diffusion lengths. The majority of oxide perovskite band gaps are above 3 eV and do not meet the criteria for photocatalytic water splitting. However, there are some oxide perovskites that have a narrow band gap such as LaFeO3, BiFeO3, and BaTiO3 (Parida et al., 2010; Yang et al., 2019; Clabel H. et al., 2021a). When oxide perovskites are doped, their structure can be tuned and holds great promise for water splitting. In doped perovskites, the band gap of the compound material is decreased and it apparently becomes a UV-visible-light response photocatalyst. In order to maximize the functionality of water splitting, the perovskite structure was doped, and the most common materials for water splitting under UV and visible-light are Mn-, Ta-, and Nb-based perovskite oxides. Yang et al. studied the effects of Sr-doped LaMnO3 perovskites, targeted for thermochemical dissociation of water splitting (Yang et al., 2014). Studies for visible light photocatalytic hydrogen production from water splitting in pristine and chalcogen-doped BaTiO3 were realized by first-principles DFT (Huang et al., 2019). The doped chalcogens can significantly decrease the band gap of BaTiO3, and this is especially true for the larger concentration of the dopant, leading to significant reduction from 3.13 to 1.01 eV, satisfying the conditions of water splitting. Luo et al. studied the effect of B and Fe co-doping SrTiO3 in the band gap (Humayun et al., 2018). The co-doping leads to the band gap of SrTiO3 being significantly reduced from 3.4 to 1.9 eV. The band gap of B and Fe co-doping SrTiO3 is significantly reduced and exhibits absorption up to a wavelength of 650 nm. This confirmed the enhanced charge carrier separation and improved photocatalytic activities of the photocatalysts for CO2 conversion and water splitting.
Also, halide perovskites are used as an efficient compound for water splitting. Mainly, halide perovskite CH3NH3PbI3 exhibits clear ambipolar nature of charge carrier transport, a behavior where both electrons and holes exhibit very good mobility. Because of this unique property, CH3NH3PbI3 can be used as both photoanode for water oxidation and photocathode for water reduction reaction (Singh et al., 2020). However, due to Pb2+ toxicity, B-sites in the perovskite structure have been explored. Also, in another work, using lead-free and phase-stable Sn(II) halide perovskite CH3NH3SnI3 has been prepared and tested (Yang et al., 2015; Volonakis and Giustino, 2018). This Sn(II) perovskite has a band gap of 1.3 eV, with charge carrier mobility of ≈1.6 cm2 V−1 s−1, and demonstrated photocatalytic H2 evolution making them functional for water splitting. Other alternatives of potentially promising materials of lead-free halide perovskites are Cs2BiAgCl6, Cs2BiAgBr6, Cs2SbAgCl6, and Cs2InAgCl6 (Volonakis and Giustino, 2018). The electronic properties and ionization potential were studied from first principles examining the different possible surface terminations and the thermodynamic stability. It was found that the band levels of Cs2BiAgCl6 and Cs2BiAgBr6 are matching the redox potential of water. Cs2SbAgCl6 and Cs2InAgCl6 could also be suitable for hydrogen evolution or water evolution, and such factors are favorable to water splitting.
5.2 Perovskite Materials to Photocatalytic CO2 Reduction
As a result of human activity and industrial development, concentrations of carbon dioxide have increased in recent decades. In this scenario, a possible way is the use of highly active photocatalytic systems for photocatalytic reduction of CO2, leading to hydrocarbons in the gas phase and oxygenates in the liquid phase (Hong et al., 2013), with the aid of solar energy. Several mechanisms are crucial for improving the photoactivity: 1) surface area, 2) surface energetics, 3) mass transfer phenomena, 4) light absorption, 5) excitation (bulk and surface recombination), and 6) charge separation (Marszewski et al., 2015). These important aspects will impact the performance of a photocatalyst. The CO2 adsorption by physisorption and chemisorption, where the molecule is susceptible to reduction by photogenerated electrons, these electrons are generated through light absorption, excitation, and charge separation. The CO2 adsorption is promoted by several factors physical and chemical as the increase of catalyst surface area, surface defects, basic sites, and Cu and/or Pt co-catalyst nanoparticles surfaces. Oxide and halide perovskites due to their high reactivity surface and tuning of physical and chemical properties can lead to a significant improvement of photoelectrochemical activities (Shi and Zou, 2012; Xu et al., 2017).
Perovskite oxide type NaNbO3 and NaTaO3 prepared by solid-state reactions were applied for the photocatalytic reduction of CO2 (Fresno et al., 2017). Due to extrinsic and intrinsic properties, such as surface area, structural modification, e−-h+ recombination, and the reducing potential of conduction band electrons, a competition of water protons for photogenerated electrons leads to greater performance in CO2 reduction. The main products obtained in the photocatalytic reduction of CO2 over NaNbO3 and NaTaO3 is shown in Figure 10A. Hemminger reported the role of SrTiO3 perovskites for photocatalytic reduction of CO2 to CH4 production (Hemminger et al., 1978). Co-catalysts nanostructured perovskite oxides also exhibit a band edge tuning, long charge diffusion lengths, longer charge carrier lifetimes (not in all perovskite oxides), and structural good stability (Shi et al., 2017). Au/Rh nanoparticle addition in SrTiO3 showed good photocatalytic performance for CO2 reduction and water splitting in the visible light spectra, and the degree concentration of the Au/Rh ratio leads to variation in the CO2 reduction (Li et al., 2016). The difference in the transfer of interband-transition photoelectron from SrTiO3 to Rh and Rh/Au@SrTiO3 was evidenced by photoluminescence spectra, in this last in low photoluminescence evidence that recombination between the photoelectron and the positive-charged Au is suppressed. In addition, Rh as co-catalyst has a function as accumulated photoelectrons. Thus, the photoelectrons induced by interband transition and plasmon resonance in Au indeed transfer from SrTiO3 to the Rh co-catalyst during visible light irradiation, leading to both suppressing the CH4 production and significantly enhancing the photoactivity. The improved performance of photocatalytic reaction can also be enhanced by other transition metal co-catalysts which extend visible light absorption through localized surface plasmon resonance of metal nanostructures, such as Ag, Pt, Ir, and Cu (Wood et al., 2001; Kamat, 2012). In addition, the interface between the metal and semiconductor type perovskite, a Schottky barrier, is formed, where the work function difference gives rise to a potential offset. Such Schottky barriers can reduce the kinetics of electron flux from the perovskite conduction band into the metal, that is, transfer of photogenerated electrons across the interface. This accumulation of photogenerated electrons on the metal enhanced the performance in the CO2 reduction (Wood et al., 2001).
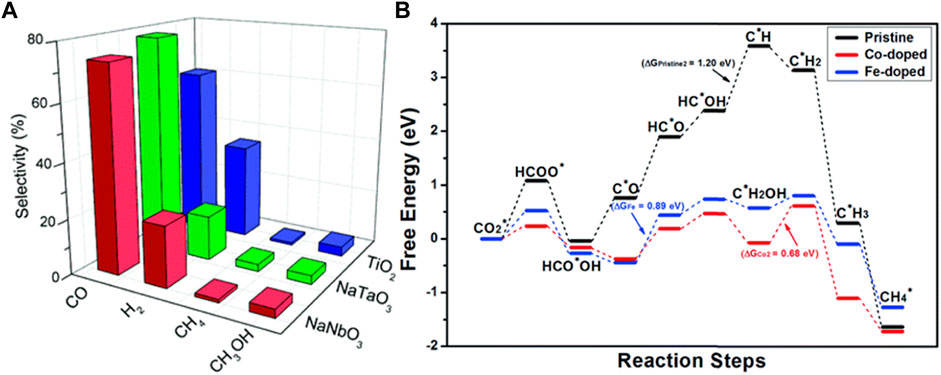
FIGURE 10. (A) Selectivities toward the main products obtained in the photocatalytic reduction of CO2 over NaNbO3, NaTaO3, and TiO2, (B) The free energy diagrams of the most favored paths of CO2 reduction on pristine, Co-doped, and Fe-doped CsPbBr3 perovskite cases (Fresno et al., 2017; Tang et al., 2019).
Light-emitting lead halide perovskite nanocrystals CsPbBr3 were explored for catalytic CO2 reduction (Shyamal et al., 2020). Shyamal et al. (2020) showed the surface facet-dependent catalytic activities in cube and faceted non-cube shapes, where the adsorption/desorption and the suppression of carrier recombination are essential in the CO2 reduction. Surfaced isotropic polyhedron-shaped poor emitter noncubes were observed to be the superior catalyst. Moreover, the DFT study will reveal that the adsorption/desorption of CO2 and reduction have an efficient activity along with the {112} and {102} facets compared to the dominant {110} and {002} facets of orthorhombic cube-shaped CsPbBr3 nanostructures. A size-dependent enhancement in photocatalytic degradation has been previously established for Au-TiO2 composites. Using density functional theory (DFT), the enhancement of the photocatalytic capability was shown in pristine CsPbBr3 perovskites and doped CsPbBr3 perovskites, which transform CO2 into methane (Tang et al., 2019). Doped CsPbBr3 with Co, Fe, Ni, Cu, Ag, Mg, Mn, and Bi shows a better catalytic performance than pristine CsPbBr3. Particularly, Co- and Fe-doped CsPbBr3 perovskites are promising candidates due to the better adsorption, promoting the CO2 reduction process. The CO2 reduction process is mainly reduced to HCOO* in the hydrogenation, through successive proton absorptions on the O and C atom generating C*H and C*H2 for the pristine and Co- and Fe-doped CsPbBr3 perovskites, respectively, releasing H2O and then hydrogenating to CH4 (see Figure 10B). The free energy barrier is 1.20, 0.68, and 0.23 eV for the pristine, Co-doped, and Fe-doped CsPbBr3 perovskites, respectively, thus showing the catalytic efficiency in Co and Fe doped CsPbBr3 perovskites. Such results are consistent with the previous experiment (Xu et al., 2017).
6 Conclusion and Outlook
Investigations into the use of photocatalytic perovskites for the elimination of emerging contaminants are of great current interest. A suitable band gap taking advantage of dominant solar irradiation and high photoinduced charge separation efficiency are key factors for ideal photocatalysts and are under constant research. A wide range of synthesis methods allows the creation of unique structures that can enhance photocatalytic activity, as evidenced by the other research group in this area. The present review focuses on three parameters essential in photocatalytic activity: the role of band gap, band edges, and the lifetime of charger carriers. Additionally, the electronic properties as the band gap can be affected in many different ways for all types of perovskite structures. In pure perovskites and doped perovskites with single-phase structures, factors of calcination temperature, doping, the concentration of doping, composition, and density of defect can affect the band gap. Synthesis methods also determine the band gap characteristics where the shape and size drive the formation of different facets, surface area, and porosity. Different mechanisms for reducing the band gap (<3 eV) in order to improve the narrow light-response to the ultraviolet spectrum (∼4% of sunlight) and inhibit the recombination process were considered, thus improving photocatalytic efficiency. The fundamental understanding of such mechanisms helps provide much broad insight into the role of band gap and lifetime in advanced perovskites applied to photocatalysis. An attempt is realized in this review to differentiate the contribution between both band gap and lifetime of charger carriers.
The narrow band gap is mainly considered to play an important role in light absorption capability, though the modulation of band gap is dependent on both extrinsic and intrinsic parameters. Changes in the defect density at the surface can impact the redox reaction at the surface and band gap. The particle size influences the band gap due to the confinement of the electrons; likewise, the small size and large surface area of nanomaterials are preferred to facilitate the charge transfer between the catalyst and the redox molecules. Doping has been shown to alter the optical properties of perovskite structures and improve their stability. Meanwhile, the lifetime of charger carriers is considered necessary because it facilitates the photoexcited charge carriers to reach the active sites to complete the surface redox reactions. In addition, it promotes the increase in photogenerated
Although the mechanisms of doping are effective in optimization, they merit a more thorough investigation. In this sense, a challenge is using the doping type within the host matrix, optimized substitution in the A-, B-, or X-site, and doping concentration. The complementation with current techniques that help identify and clarify the reaction mechanisms between the dopant and the matrix will contribute enormously to this challenge. Quantifying the defect density and identifying the type of defects present on the surface would help understand and interpret perovskite structures lifetime and decay kinetics. Although techniques such as transient absorption, time-resolved photoluminescence, and confocal microscopy explore the lifetime and energy transfer process in perovskite structures, these techniques only contribute to certain types of perovskites. Incorporating knowledge focused on surface science would be helpful in understanding the sites active in the surface affect redox reaction and at what level according to the type of defect. Additionally, theoretical calculations are also useful in helping to predict the observed effects, from the surface chemical defect, particle size, surface area, and porosity. Finally, the different types of perovskite structures are promising; however, we still have a broad horizon to explore to make it more efficient.
Author Contributions
JH: conceptualization, methodology, investigation, visualization, and writing—original draft. JC-R: review investigation and writing. EJ: conceptualization, review and editing, and supervision.
Funding
This research was supported by the São Paulo Research Foundation (FAPESP) through the Centro de Pesquisas em Óptica e Fotônica (CePOF) under the grant 2013/07276-1 and Conselho Nacional de Desenvolvimento Científico e Tecnológico (CNPq) grant 310925/2017-7.
ACKNOWLEDGMENTS
This research was supported by the São Paulo Research Foundation (FAPESP) through the Centro de Pesquisas em Óptica e Fotônica (CePOF) under the grant 2013/07276-1 and Conselho Nacional de Desenvolvimento Científico e Tecnológico (CNPq) grant 310925/2017-7.
Conflict of Interest
The authors declare that the research was conducted in the absence of any commercial or financial relationships that could be construed as a potential conflict of interest.
The handling editor declared a past co-authorship with one of the authors JLCH.
Publisher’s Note
All claims expressed in this article are solely those of the authors and do not necessarily represent those of their affiliated organizations, or those of the publisher, the editors, and the reviewers. Any product that may be evaluated in this article, or claim that may be made by its manufacturer, is not guaranteed or endorsed by the publisher.
References
Akkerman, Q. A., D’Innocenzo, V., Accornero, S., Scarpellini, A., Petrozza, A., Prato, M., et al. (2015). Tuning the Optical Properties of Cesium lead Halide Perovskite Nanocrystals by Anion Exchange Reactions. J. Am. Chem. Soc. 137, 10276–10281. doi:10.1021/jacs.5b05602
Alammar, T., Hamm, I., Grasmik, V., Wark, M., and Mudring, A.-V. (2017). Microwave-Assisted Synthesis of Perovskite SrSnO3 Nanocrystals in Ionic Liquids for Photocatalytic Applications. Inorg. Chem. 56, 6920–6932. doi:10.1021/acs.inorgchem.7b00279
Bartel, C. J., Sutton, C., Goldsmith, B. R., Ouyang, R., Musgrave, C. B., Ghiringhelli, L. M., et al. (2019). New Tolerance Factor to Predict the Stability of Perovskite Oxides and Halides. Sci. Adv. 5, 1–10. doi:10.1126/sciadv.aav0693
Belver, C., Bedia, J., Gómez-Avilés, A., Peñas-Garzón, M., and Rodriguez, J. J. (2019). Semiconductor Photocatalysis for Water Purification, 581–651. doi:10.1016/B978-0-12-813926-4.00028-8
Bin Adnan, M. A., Arifin, K., Minggu, L. J., and Kassim, M. B. (2018). Titanate-Based Perovskites for Photochemical and Photoelectrochemical Water Splitting Applications: A Review. Int. J. Hydrogen Energ. 43, 23209–23220. doi:10.1016/j.ijhydene.2018.10.173
Borriello, I., Cantele, G., and Ninno, D. (2008). Ab Initioinvestigation of Hybrid Organic-Inorganic Perovskites Based on Tin Halides. Phys. Rev. B 77, 1–9. doi:10.1103/PhysRevB.77.235214
Brutchey, R. L., and Morse, D. E. (2006). Template-Free, Low-Temperature Synthesis of Crystalline Barium Titanate Nanoparticles under Bio-Inspired Conditions. Angew. Chem. Int. Ed. 45, 6564–6566. doi:10.1002/anie.200602571
Burnside, S., Moser, J.-E., Brooks, K., Grätzel, M., and Cahen, D. (1999). Nanocrystalline Mesoporous Strontium Titanate as Photoelectrode Material for Photosensitized Solar Devices: Increasing Photovoltage Through Flatband Potential Engineering. J. Phys. Chem. B 103, 9328–9332. doi:10.1021/jp9913867
Caruntu, D., Rostamzadeh, T., Costanzo, T., Salemizadeh Parizi, S., and Caruntu, G. (2015). Solvothermal Synthesis and Controlled Self-Assembly of Monodisperse Titanium-Based Perovskite Colloidal Nanocrystals. Nanoscale 7, 12955–12969. doi:10.1039/c5nr00737b
Chang Chien, S.-W., Ng, D.-Q., Kumar, D., Lam, S.-M., and Jaffari, Z. H. (2022). Investigating the Effects of Various Synthesis Routes on Morphological, Optical, Photoelectrochemical and Photocatalytic Properties of Single-Phase Perovskite BiFeO3. J. Phys. Chem. Sol. 160, 110342. doi:10.1016/j.jpcs.2021.110342
Chen, D., Cheng, Y., Zhou, N., Chen, P., Wang, Y., Li, K., et al. (2020). Photocatalytic Degradation of Organic Pollutants Using TiO2-Based Photocatalysts: A Review. J. Clean. Prod. 268, 121725. doi:10.1016/j.jclepro.2020.121725
Chen, D., Niu, F., Qin, L., Wang, S., Zhang, N., and Huang, Y. (2017). Defective BiFeO3 with Surface Oxygen Vacancies: Facile Synthesis and Mechanism Insight into Photocatalytic Performance. Solar Energ. Mater. Solar Cell 171, 24–32. doi:10.1016/j.solmat.2017.06.021
Chen, D., and Ye, J. (2007). SrSnO3 Nanostructures: Synthesis, Characterization, and Photocatalytic Properties. Chem. Mater. 19, 4585–4591. doi:10.1021/cm071321d
Chen, P., Ong, W. J., Shi, Z., Zhao, X., and Li, N. (2020). Pb‐Based Halide Perovskites: Recent Advances in Photo(electro)catalytic Applications and Looking Beyond. Adv. Funct. Mater. 30, 1–27. doi:10.1002/adfm.201909667
Cheng, C., Gao, S., Zhu, J., Wang, G., Wang, L., and Xia, X. (2020). Enhanced Performance of LaFeO3 Perovskite for Peroxymonosulfate Activation Through Strontium Doping Towards 2,4-D Degradation. Chem. Eng. J. 384, 123377. doi:10.1016/j.cej.2019.123377
Clabel, H., J. L., Awan, I. T., Pinto, A. H., Nogueira, I. C., Bezzon, V. D. N., Leite, E. R., et al. (2020). Insights on the Mechanism of Solid State Reaction Between TiO2 and BaCO3 to Produce BaTiO3 Powders: The Role of Calcination, Milling, and Mixing Solvent. Ceramics Int. 46, 2987–3001. doi:10.1016/j.ceramint.2019.09.296
Clabel H., J. L., Ferri, F. A., Zabotto, F. L., Rivera, V. A. G., Nogueira, I. C., Garcia, D., et al. (2016). Grain Size and Interfacial Interdiffusion Influence on the Magnetic and Dielectric Properties of Magnetoelectric La0.7Ba0.3MnO3-BaTiO3 Composites. J. Magnetism Magn. Mater. 407, 160–166. doi:10.1016/j.jmmm.2016.01.082
Clabel H., J. L., Awan, I. T., Rivera, V. A. G., Nogueira, I. C., Pereira-da-Silva, M. A., Li, M. S., et al. (2019). Growth Process and Grain Boundary Defects in Er Doped BaTiO3 Processed by EB-PVD: A Study by XRD, FTIR, SEM and AFM. Appl. Surf. Sci. 493, 982–993. doi:10.1016/j.apsusc.2019.07.003
Clabel H., J. L., Nazrin, S. N., Lozano C., G., Pereira da Silva, M., Siu Li, M., and Marega Jr., E. (2021). Activation Energy and its Fluctuations at Grain Boundaries of Er3+:BaTiO3 Perovskite Thin Films: Effect of Doping Concentration and Annealing Temperature. Vacuum 194, 110562. doi:10.1016/j.vacuum.2021.110562
Clabel H., J. L., Nicolodelli, G., Lozano C., G., G. Rivera, V. A., Ferreira, S. O., Pinto, A. H., et al. (2021). The Extrinsic Nature of Double Broadband Photoluminescence from the BaTiO3 Perovskite: Generation of White Light Emitters. Phys. Chem. Chem. Phys. 23, 18694–18706. doi:10.1039/d1cp01765a
Clabel H., J. L., Rivera, V. A. G., Nogueira, I. C., Leite, E. R., Pereira-da-Silva, M. A., Li, M. S., et al. (2017). Effects of Defects, Grain Size, and Thickness on the Optical Properties of BaTiO3 Thin Films. J. Lumin. 192, 969–974. doi:10.1016/j.jlumin.2017.08.043
Clabel H., J. L., Zabotto, F. L., Nogueira, I. C., Schio, P., Garcia, D., De Lima, O. F., et al. (2014). Magnetoelectric Properties of Laminated La0.7Ba0.3MnO3-BaTiO3 Ceramic Composites. J. Magnetism Magn. Mater. 364, 18–23. doi:10.1016/j.jmmm.2014.04.014
Cohen, R. E. (1992). Origin of Ferroelectricity in Perovskite Oxides. Nature 358, 136–138. doi:10.1038/358136a0
Correa-Baena, J.-P., Saliba, M., Buonassisi, T., Grätzel, M., Abate, A., Tress, W., et al. (2017). Promises and Challenges of Perovskite Solar Cells. Science 358, 739–744. doi:10.1126/science.aam6323
Creutz, S. E., Crites, E. N., De Siena, M. C., and Gamelin, D. R. (2018). Colloidal Nanocrystals of Lead-Free Double-Perovskite (Elpasolite) Semiconductors: Synthesis and Anion Exchange to Access New Materials. Nano Lett. 18, 1118–1123. doi:10.1021/acs.nanolett.7b04659
Da Silva, L. F., Avansi, W., Andrés, J., Ribeiro, C., Moreira, M. L., Longo, E., et al. (2013). Long-Range and Short-Range Structures of Cube-Like Shape SrTiO3 Powders: Microwave-Assisted Hydrothermal Synthesis and Photocatalytic Activity. Phys. Chem. Chem. Phys. 15, 12386–12393. doi:10.1039/c3cp50643f
Ding, Q.-P., Yuan, Y.-P., Xiong, X., Li, R.-P., Huang, H.-B., Li, Z.-S., et al. (2008). Enhanced Photocatalytic Water Splitting Properties of KNbO3 Nanowires Synthesized Through Hydrothermal Method. J. Phys. Chem. C 112, 18846–18848. doi:10.1021/jp8042768
Efros, A. (1982). Interband Light Absorption in Semiconductor Spheres. Sov. Phys. Semicond. 16, 772–775. Available at: https://www.researchgate.net/publication/279890805.
Fang, Y., Kong, X., Wang, D., Liu, J., and Cui, S. (2019). First Principle Calculations of Electronic, Band Structural, and Optical Properties of BixSr1-xTiO3 Perovskite. J. Phys. Chem. Sol. 127, 107–114. doi:10.1016/j.jpcs.2018.11.023
Fenton, J. L., Steimle, B. C., and Schaak, R. E. (2018). Tunable Intraparticle Frameworks for Creating Complex Heterostructured Nanoparticle Libraries. Science 360, 513–517. doi:10.1126/science.aar5597
Fresno, F., Jana, P., Reñones, P., Coronado, J. M., Serrano, D. P., and de la Peña O'Shea, V. A. (2017). CO2 Reduction over NaNbO3 and NaTaO3 Perovskite Photocatalysts. Photochem. Photobiol. Sci. 16, 17–23. doi:10.1039/c6pp00235h
Ghafoor, A., Bibi, I., Ata, S., Majid, F., Kamal, S., Iqbal, M., et al. (2021). Energy Band Gap Tuning of LaNiO3 by Gd, Fe and Co Ions Doping to Enhance Solar Light Absorption for Efficient Photocatalytic Degradation of RhB Dye: A Mechanistic Approach. J. Mol. Liquids 343, 117581. doi:10.1016/j.molliq.2021.117581
Ghiasi, M., and Malekzadeh, A. (2014). Solar Photocatalytic Degradation of Methyl Orange Over La0.7Sr0.3MnO3 Nano-Perovskite. Sep. Purif. Tech. 134, 12–19. doi:10.1016/j.seppur.2014.07.022
Golsdschmidt, V. V. M. (1962). Die gesetze der krystallochemie. Naturwissenschaften, Naturwissenschaften. 14, 477–485. doi:10.1177/089686089501500104
Gomathi Devi, L., and Krishnamurthy, G. (2009). TiO2/BaTiO3-Assisted Photocatalytic Mineralization of Diclofop-Methyl on UV-Light Irradiation in the Presence of Oxidizing Agents. J. Hazard. Mater. 162, 899–905. doi:10.1016/j.jhazmat.2008.05.116
Gómez-Solís, C., Oliva, J., Diaz-Torres, L. A., Bernal-Alvarado, J., Reyes-Zamudio, V., Abidov, A., et al. (2019). Efficient Photocatalytic Activity of MSnO3 (M: Ca, Ba, Sr) Stannates for Photoreduction of 4-nitrophenol and Hydrogen Production Under UV Light Irradiation. J. Photochem. Photobiol. A: Chem. 371, 365–373. doi:10.1016/j.jphotochem.2018.11.039
Grabowska, E. (2016). Selected Perovskite Oxides: Characterization, Preparation and Photocatalytic Properties-A Review. Appl. Catal. B: Environ. 186, 97–126. doi:10.1016/j.apcatb.2015.12.035
Guleria, A., Sharma, R., Singh, A., Upadhyay, N. K., and Shandilya, P. (2021). Direct Dual-Z-Scheme PANI/Ag2O/Cu2O Heterojunction with Broad Absorption Range for Photocatalytic Degradation of Methylene Blue. J. Water Process Eng. 43, 102305. doi:10.1016/j.jwpe.2021.102305
Haron, W., Wisitsoraat, A., Sirimahachai, U., and Wongnawa, S. (2018). A Simple Synthesis and Characterization of LaMo3 (M=Al, Co, Fe, Gd) Perovskites via Chemical Co-Precipitation Method. Songklanakarin J. Sci. Technol. 40, 484–491. doi:10.14456/sjst-psu.2018.64
Hemminger, J. C., Carr, R., and Somorjai, G. A. (1978). The Photoassisted Reaction of Gaseous Water and Carbon Dioxide Adsorbed on the SrTiO3 (111) Crystal Face to Form Methane. Chem. Phys. Lett. 57, 100–104. doi:10.1016/0009-2614(78)80359-5
Hoefler, S. F., Trimmel, G., and Rath, T. (2017). Progress on Lead-Free Metal Halide Perovskites for Photovoltaic Applications: A Review. Monatsh Chem. 148, 795–826. doi:10.1007/s00706-017-1933-9
Hoffmann, M. R., Martin, S. T., Choi, W., and Bahnemann, D. W. (1995). Environmental Applications of Semiconductor Photocatalysis. Chem. Rev. 95, 69–96. doi:10.1021/cr00033a004
Hong, J., Zhang, W., Ren, J., and Xu, R. (2013). Photocatalytic Reduction of CO2: A Brief Review on Product Analysis and Systematic Methods. Anal. Methods 5, 1086–1097. doi:10.1039/c2ay26270c
Hoshina, T. (2013). Size Effect of Barium Titanate: Fine Particles and Ceramics. J. Ceram. Soc. Jpn. 121, 156–161. doi:10.2109/jcersj2.121.156
Hsieh, P.-L., Naresh, G., Huang, Y.-S., Tsao, C.-W., Hsu, Y.-J., Chen, L.-J., et al. (2019). Shape-Tunable SrTiO3 Crystals Revealing Facet-Dependent Optical and Photocatalytic Properties. J. Phys. Chem. C 123, 13664–13671. doi:10.1021/acs.jpcc.9b02081
Huang, H.-C., Yang, C.-L., Wang, M.-S., and Ma, X.-G. (2019). Chalcogens Doped BaTiO3 for Visible Light Photocatalytic Hydrogen Production from Water Splitting. Spectrochimica Acta A: Mol. Biomol. Spectrosc. 208, 65–72. doi:10.1016/j.saa.2018.09.048
Humayun, M., Xu, L., Zhou, L., Zheng, Z., Fu, Q., and Luo, W. (2018). Exceptional Co-Catalyst Free Photocatalytic Activities of B and Fe Co-Doped SrTiO3 for CO2 Conversion and H2 Evolution. Nano Res. 11, 6391–6404. doi:10.1007/s12274-018-2164-z
Hwang, J., Rao, R. R., Giordano, L., Katayama, Y., Yu, Y., and Shao-Horn, Y. (2017). Perovskites in Catalysis and Electrocatalysis. Science 358, 751–756. doi:10.1126/science.aam7092
Ibarra-Rodriguez, L. I., Huerta-Flores, A. M., Mora-Hernandez, J. M., and Torres-Martínez, L. M. (2020). Photocatalytic Evolution of H2 Over Visible-Light Active LaMO3 (M: Co, Mn, Fe) Perovskite Materials: Roles of Oxygenated Species in Catalytic Performance. J. Phys. Chem. Sol. 136, 109189. doi:10.1016/j.jpcs.2019.109189
Ismael, M., and Wark, M. (2019). Perovskite-Type LaFeO3: Photoelectrochemical Properties and Photocatalytic Degradation of Organic Pollutants Under Visible Light Irradiation. Catalysts 9, 342. doi:10.3390/catal9040342
Jain, A., Ong, S. P., Hautier, G., Chen, W., Richards, W. D., Dacek, S., et al. (2013). Commentary: The Materials Project: A Materials Genome Approach to Accelerating Materials Innovation. APL Mater. 1, 011002. doi:10.1063/1.4812323
Kamat, P. V. (2012). Manipulation of Charge Transfer Across Semiconductor Interface. A Criterion that Cannot Be Ignored in Photocatalyst Design. J. Phys. Chem. Lett. 3, 663–672. doi:10.1021/jz201629p
Kanhere, P., Zheng, J., and Chen, Z. (2012). Visible Light Driven Photocatalytic Hydrogen Evolution and Photophysical Properties of Bi3+ Doped NaTaO3. Int. J. Hydrogen Energ. 37, 4889–4896. doi:10.1016/j.ijhydene.2011.12.056
Karthikeyan, C., Arunachalam, P., Ramachandran, K., Al-Mayouf, A. M., and Karuppuchamy, S. (2020). Recent Advances in Semiconductor Metal Oxides with Enhanced Methods for Solar Photocatalytic Applications. J. Alloys Comp. 828, 154281. doi:10.1016/j.jallcom.2020.154281
Kirchartz, T., Márquez, J. A., Stolterfoht, M., and Unold, T. (2020). Photoluminescence‐Based Characterization of Halide Perovskites for Photovoltaics. Adv. Energ. Mater. 10, 1904134. doi:10.1002/aenm.201904134
Kovalenko, M. V., Protesescu, L., and Bodnarchuk, M. I. (2017). Properties and Potential Optoelectronic Applications of Lead Halide Perovskite Nanocrystals. Science 358, 745–750. doi:10.1126/science.aam7093
Krückemeier, L., Krogmeier, B., Liu, Z., Rau, U., and Kirchartz, T. (2021). Understanding Transient Photoluminescence in Halide Perovskite Layer Stacks and Solar Cells. Adv. Energ. Mater. 11, 2003489. doi:10.1002/aenm.202003489
Kudo, A., and Miseki, Y. (2009). Heterogeneous Photocatalyst Materials for Water Splitting. Chem. Soc. Rev. 38, 253–278. doi:10.1039/b800489g
Kumar, A., Kumar, A., and Krishnan, V. (2020). Perovskite Oxide Based Materials for Energy and Environment-Oriented Photocatalysis. ACS Catal. 10, 10253–10315. doi:10.1021/acscatal.0c02947
Kumar, S., Sharma, M., Powar, S., Kabachkov, E. N., and Vaish, R. (2019). Impact of Remnant Surface Polarization on Photocatalytic and Antibacterial Performance of BaTiO3. J. Eur. Ceram. Soc. 39, 2915–2922. doi:10.1016/j.jeurceramsoc.2019.03.029
Kurbakov, A. I. (2010). Electronic, Structural and Magnetic Phase Diagram of Sm1-xSrxMnO3 Manganites. J. Magnetism Magn. Mater. 322, 967–972. doi:10.1016/j.jmmm.2009.11.034
Letifi, H., Dridi, D., Litaiem, Y., Ammar, S., Dimassi, W., and Chtourou, R. (2021). High Efficient and Cost Effective Titanium Doped Tin Dioxide Based Photocatalysts Synthesized via Co-Precipitation Approach. Catalysts 11, 803. doi:10.3390/catal11070803
Li, C., Lu, X., Ding, W., Feng, L., Gao, Y., and Guo, Z. (2008). Formability of ABX3 (X = F, Cl, Br, I) Halide Perovskites. Acta Crystallogr. Sect. B 64, 702–707. doi:10.1107/S0108768108032734
Li, D., Ouyang, S., Xu, H., Lu, D., Zhao, M., Zhang, X., et al. (2016). Synergistic Effect of Au and Rh on SrTiO3 in Significantly Promoting Visible-Light-Driven Syngas Production from CO2 and H2O. Chem. Commun. 52, 5989–5992. doi:10.1039/c6cc00836d
Li, M., Begum, R., Fu, J., Xu, Q., Koh, T. M., Veldhuis, S. A., et al. (2018). Low Threshold and Efficient Multiple Exciton Generation in Halide Perovskite Nanocrystals. Nat. Commun. 919, 1–7. doi:10.1038/s41467-018-06596-1
Li, M., and Li, J. C. (2006). Size Effects on the Band-Gap of Semiconductor Compounds. Mater. Lett. 60, 2526–2529. doi:10.1016/j.matlet.2006.01.032
Li, N., Tao, S., Chen, Y., Niu, X., Onwudinanti, C. K., Hu, C., et al. (2019). Cation and Anion Immobilization Through Chemical Bonding Enhancement with Fluorides for Stable Halide Perovskite Solar Cells. Nat. Energ. 4, 408–415. doi:10.1038/s41560-019-0382-6
Liang, J., Chen, D., Yao, X., Zhang, K., Qu, F., Qin, L., et al. (2020). Recent Progress and Development in Inorganic Halide Perovskite Quantum Dots for Photoelectrochemical Applications. Small 16, 1903398. doi:10.1002/SMLL.201903398
Liu, B., and Zhao, X. (2010). A Kinetic Model for Evaluating the Dependence of the Quantum Yield of Nano-TiO2 Based Photocatalysis on Light Intensity, Grain Size, Carrier Lifetime, and Minority Carrier Diffusion Coefficient: Indirect Interfacial Charge Transfer. Electrochimica Acta 55, 4062–4070. doi:10.1016/j.electacta.2010.01.087
Liu, J., Liu, L., Zhang, J., Jin, L., Wang, D., Wei, J., et al. (2020). Charge Effects in Donor‐Doped Perovskite Ferroelectrics. J. Am. Ceram. Soc. 103, 5392–5399. doi:10.1111/jace.17270
Liu, K., Mi, L., Wang, H., Xiong, X., Zhang, K., and Wang, B. (2021). Preparation of Ba1-xSrxTiO3 by the Sol-Gel Assisted Solid Phase Method: Study on its Formation Mechanism and Photocatalytic Hydrogen Production Performance. Ceramics Int. 47, 22055–22064. doi:10.1016/j.ceramint.2021.04.226
Liu, L., Zhao, R., Xiao, C., Zhang, F., Pevere, F., Shi, K., et al. (2019). Size-Dependent Phase Transition in Perovskite Nanocrystals. J. Phys. Chem. Lett. 10, 5451–5457. doi:10.1021/acs.jpclett.9b02058
Liu, S., Wang, J., Shen, B., Zhai, J., Hao, H., and Zhao, L. (2017). Poly(vinylidene Fluoride) Nanocomposites with a Small Loading of Core-Shell Structured BaTiO3@Al2O3 Nanofibers Exhibiting High Discharged Energy Density and Efficiency. J. Alloys Comp. 696, 136–142. doi:10.1016/j.jallcom.2016.11.186
Liu, X., Xiao, L., Zhang, Y., and Sun, H. (2020). Significantly Enhanced Piezo-Photocatalytic Capability in BaTiO3 Nanowires for Degrading Organic Dye. J. Materiomics 6, 256–262. doi:10.1016/j.jmat.2020.03.004
Loiudice, A., Strach, M., Saris, S., Chernyshov, D., and Buonsanti, R. (2019). Universal Oxide Shell Growth Enables In Situ Structural Studies of Perovskite Nanocrystals During the Anion Exchange Reaction. J. Am. Chem. Soc. 141, 8254–8263. doi:10.1021/jacs.9b02061
Lu, C.-H., Biesold-Mcgee, G. V., Liu, Y., Kang, Z., and Lin, Z. (2020). Doping and Ion Substitution in Colloidal Metal Halide Perovskite Nanocrystals. Chem. Soc. Rev. 49, 4953–5007. doi:10.1039/c9cs00790c
Lu, S., Wang, G., Chen, S., Yu, H., Ye, F., and Quan, X. (2018). Heterogeneous Activation of Peroxymonosulfate by LaCo1-xCuxO3 Perovskites for Degradation of Organic Pollutants. J. Hazard. Mater. 353, 401–409. doi:10.1016/j.jhazmat.2018.04.021
Machida, M., Miyazaki, K., Matsushima, S., and Arai, M. (2003). Photocatalytic Properties of Layered Perovskite Tantalates, MLnTa2O7 (M = Cs, Rb, Na, and H; Ln = La, Pr, Nd, and Sm). J. Mater. Chem. 13, 1433–1437. doi:10.1039/b301938c
Maeda, K., and Domen, K. (2007). New Non-Oxide Photocatalysts Designed for Overall Water Splitting Under Visible Light. J. Phys. Chem. C 111, 7851–7861. doi:10.1016/S1380-7323(06)80014-210.1021/jp070911w
Marszewski, M., Cao, S., Yu, J., and Jaroniec, M. (2015). Semiconductor-Based Photocatalytic CO2 Conversion. Mater. Horiz. 2, 261–278. doi:10.1039/c4mh00176a
Meng, J., Lan, Z., Lin, Q., Chen, T., Chen, X., Wei, X., et al. (2019). Cubic-Like BaZrO3 Nanocrystals with Exposed {001}/{011} Facets and Tuned Electronic Band Structure for Enhanced Photocatalytic Hydrogen Production. J. Mater. Sci. 54, 1967–1976. doi:10.1007/s10853-018-2995-8
Miao, J., Duan, X., Li, J., Dai, J., Liu, B., Wang, S., et al. (2019). Boosting Performance of Lanthanide Magnetism Perovskite for Advanced Oxidation Through Lattice Doping with Catalytically Inert Element. Chem. Eng. J. 355, 721–730. doi:10.1016/j.cej.2018.08.192
Miao, J., Sunarso, J., Su, C., Zhou, W., Wang, S., and Shao, Z. (2017). SrCo1−xTixO3−δ Perovskites as Excellent Catalysts for Fast Degradation of Water Contaminants in Neutral and Alkaline Solutions. Sci. Rep. 7, 1–10. doi:10.1038/srep44215
Modak, B., Srinivasu, K., and Ghosh, S. K. (2014). Photocatalytic Activity of NaTaO3 Doped with N, Mo, and (N,Mo): A Hybrid Density Functional Study. J. Phys. Chem. C 118, 10711–10719. doi:10.1021/jp410995g
Mott, N. F. (1968). Conduction in Glasses Containing Transition Metal Ions. J. Non-Crystalline Sol. 1, 1–17. doi:10.1016/0022-3093(68)90002-1
Odling, G., and Robertson, N. (2019). Bridging the Gap Between Laboratory and Application in Photocatalytic Water Purification. Catal. Sci. Technol. 9, 533–545. doi:10.1039/c8cy02438c
Oksenberg, E., Merdasa, A., Houben, L., Kaplan-Ashiri, I., Rothman, A., Scheblykin, I. G., et al. (2020). Large Lattice Distortions and Size-Dependent Bandgap Modulation in Epitaxial Halide Perovskite Nanowires. Nat. Commun. 11, 1–11. doi:10.1038/s41467-020-14365-2
Osterloh, F. E. (2008). Inorganic Materials as Catalysts for Photochemical Splitting of Water. Chem. Mater. 20, 35–54. doi:10.1021/cm7024203
Ou, Q., Bao, X., Zhang, Y., Shao, H., Xing, G., Li, X., et al. (2019). Band Structure Engineering in Metal Halide Perovskite Nanostructures for Optoelectronic Applications. Nano Mater. Sci. 1, 268–287. doi:10.1016/j.nanoms.2019.10.004
Palstra, T. T. M., Batlogg, B., Van Dover, R. B., Schneemeyer, L. F., and Waszczak, J. V. (1990). Dissipative Flux Motion in High-Temperature Superconductors. Phys. Rev. B 41, 6621–6632. doi:10.1103/PhysRevB.41.6621
Parida, K. M., Reddy, K. H., Martha, S., Das, D. P., and Biswal, N. (2010). Fabrication of Nanocrystalline LaFeO3: An Efficient Sol-Gel Auto-Combustion Assisted Visible Light Responsive Photocatalyst for Water Decomposition. Int. J. Hydrogen. Energ. 35, 12161–12168. doi:10.1016/j.ijhydene.2010.08.029
Park, K.-W., and Kolpak, A. M. (2019). Optimal Methodology for Explicit Solvation Prediction of Band Edges of Transition Metal Oxide Photocatalysts. Commun. Chem. 2, 79. doi:10.1038/s42004-019-0179-3
Park, Y.-U., Bai, J., Wang, L., Yoon, G., Zhang, W., Kim, H., et al. (2017). In Situ Tracking Kinetic Pathways of Li+/Na+ Substitution During Ion-Exchange Synthesis of LixNa1.5-xVOPO4F0.5. J. Am. Chem. Soc. 139, 12504–12516. doi:10.1021/jacs.7b05302
Patel, M., Kumar, R., Kishor, K., Mlsna, T., Pittman, C. U., and Mohan, D. (2019). Pharmaceuticals of Emerging Concern in Aquatic Systems: Chemistry, Occurrence, Effects, and Removal Methods. Chem. Rev. 119, 3510–3673. doi:10.1021/acs.chemrev.8b00299
Patil, M. S., Kitchamsetti, N., Mulani, S. R., Rondiya, S. R., Deshpande, N. G., Patil, R. A., et al. (2021). Photocatalytic Behavior of Ba(Sb/Ta)2O6 Perovskite for Reduction of Organic Pollutants: Experimental and DFT Correlation. J. Taiwan Inst. Chem. Eng. 122, 201–209. doi:10.1016/j.jtice.2021.04.032
Pellegrini, G., Mattei, G., and Mazzoldi, P. (2005). Finite Depth Square Well Model: Applicability and Limitations. J. Appl. Phys. 97, 073706. doi:10.1063/1.1868875
Peoples, J., Li, X., Lv, Y., Qiu, J., Huang, Z., and Ruan, X. (2019). A Strategy of Hierarchical Particle Sizes in Nanoparticle Composite for Enhancing Solar Reflection. Int. J. Heat Mass Transfer 131, 487–494. doi:10.1016/j.ijheatmasstransfer.2018.11.059
Protesescu, L., Yakunin, S., Nazarenko, O., Dirin, D. N., and Kovalenko, M. V. (2018). Low-Cost Synthesis of Highly Luminescent Colloidal Lead Halide Perovskite Nanocrystals by Wet Ball Milling. ACS Appl. Nano Mater. 1, 1300–1308. doi:10.1021/acsanm.8b00038
Qi, W. H. (2005). Size Effect on Melting Temperature of Nanosolids. Physica B: Condensed Matter 368, 46–50. doi:10.1016/j.physb.2005.06.035
Qi, W. H., and Wang, M. P. (2004). Size and Shape Dependent Melting Temperature of Metallic Nanoparticles. Mater. Chem. Phys. 88, 280–284. doi:10.1016/j.matchemphys.2004.04.026
Ramakanth, S., and James Raju, K. C. (2014). Band Gap Narrowing in BaTiO3 Nanoparticles Facilitated by Multiple Mechanisms. J. Appl. Phys. 115, 173507–7. doi:10.1063/1.4871776
Rao, M. P., Nandhini, V. P., Wu, J. J., Syed, A., Ameen, F., and Anandan, S. (2018). Synthesis of N-Doped Potassium Tantalate Perovskite Material for Environmental Applications. J. Solid State. Chem. 258, 647–655. doi:10.1016/j.jssc.2017.11.031
Ravi, V. K., Markad, G. B., and Nag, A. (2016). Band Edge Energies and Excitonic Transition Probabilities of Colloidal CsPbX3 (X = Cl, Br, I) Perovskite Nanocrystals. ACS Energ. Lett. 1, 665–671. doi:10.1021/acsenergylett.6b00337
Ravi, V. K., Saikia, S., Yadav, S., Nawale, V. V., and Nag, A. (2020). CsPbBr3/ZnS Core/Shell Type Nanocrystals for Enhancing Luminescence Lifetime and Water Stability. ACS Energ. Lett. 5, 1794–1796. doi:10.1021/acsenergylett.0c00858
Reid, A. J., Carlson, A. K., Creed, I. F., Eliason, E. J., Gell, P. A., Johnson, P. T. J., et al. (2019). Emerging Threats and Persistent Conservation Challenges for Freshwater Biodiversity. Biol. Rev. 94, 849–873. doi:10.1111/brv.12480
Rekavandi, N., Malekzadeh, A., and Ghiasi, E. (2019). Methyl Orange Degradation Using Nano-LaMnO3 as a Green Catalyst Under the Mild Conditions. Nanochem. Res. 4, 1–10. doi:10.22036/ncr.2019.01.001
Romani, L., and Malavasi, L. (2020). Solar-Driven Hydrogen Generation by Metal Halide Perovskites: Materials, Approaches, and Mechanistic View. ACS Omega 5, 25511–25519. doi:10.1021/acsomega.0c03829
Rose, J. H., Ferrante, J., and Smith, J. R. (1981). Universal Binding Energy Curves for Metals and Bimetallic Interfaces. Phys. Rev. Lett. 47, 675–678. doi:10.1103/PhysRevLett.47.675
Roy, N., and Chakraborty, S. (2021). ZnO as Photocatalyst: An Approach to Waste Water Treatment. Mater. Today Proc. 46, 6399–6403. doi:10.1016/j.matpr.2020.06.264
Saparov, B., and Mitzi, D. B. (2016). Organic-Inorganic Perovskites: Structural Versatility for Functional Materials Design. Chem. Rev. 116, 4558–4596. doi:10.1021/acs.chemrev.5b00715
Sapra, S., and Sarma, D. D. (2004). Evolution of the Electronic Structure with Size in II-VI Semiconductor Nanocrystals. Phys. Rev. B 69, 1–7. doi:10.1103/PhysRevB.69.125304
Schanze, K. S., Kamat, P. V., Yang, P., and Bisquert, J. (2020). Progress in Perovskite Photocatalysis. ACS Energ. Lett. 5, 2602–2604. doi:10.1021/acsenergylett.0c01480
Schilling, C., Ganduglia-Pirovano, M. V., and Hess, C. (2018). Experimental and Theoretical Study on the Nature of Adsorbed Oxygen Species on Shaped Ceria Nanoparticles. J. Phys. Chem. Lett. 9, 6593–6598. doi:10.1021/acs.jpclett.8b02728
Serpone, N., Lawless, D., Khairutdinov, R., and Pelizzetti, E. (1995). Subnanosecond Relaxation Dynamics in TiO2 Colloidal Sols (Particle Sizes Rp = 1.0-13.4 Nm). Relevance to Heterogeneous Photocatalysis. J. Phys. Chem. 99, 16655–16661. doi:10.1021/j100045a027
Sheng, J., He, Y., Li, J., Yuan, C., Huang, H., Wang, S., et al. (2020). Identification of Halogen-Associated Active Sites on Bismuth-Based Perovskite Quantum Dots for Efficient and Selective CO2-to-CO Photoreduction. ACS Nano 14, 13103–13114. doi:10.1021/acsnano.0c04659
Shi, H., and Zou, Z. (2012). Photophysical and Photocatalytic Properties of ANbO3 (A=Na, K) Photocatalysts. J. Phys. Chem. Sol. 73, 788–792. doi:10.1016/j.jpcs.2012.01.026
Shi, R., Waterhouse, G. I. N., and Zhang, T. (2017). Recent Progress in Photocatalytic CO2 Reduction over Perovskite Oxides. Sol. RRL 1, 1700126. doi:10.1002/solr.201700126
Shyamal, S., Dutta, S. K., Das, T., Sen, S., Chakraborty, S., and Pradhan, N. (2020). Facets and Defects in Perovskite Nanocrystals for Photocatalytic CO2 Reduction. J. Phys. Chem. Lett. 11, 3608–3614. doi:10.1021/acs.jpclett.0c01088
Sieland, F., Schneider, J., and Bahnemann, D. W. (2018). Photocatalytic Activity and Charge Carrier Dynamics of TiO2 Powders with a Binary Particle Size Distribution. Phys. Chem. Chem. Phys. 20, 8119–8132. doi:10.1039/c8cp00398j
Singh, S., Chen, H., Shahrokhi, S., Wang, L. P., Lin, C.-H., Hu, L., et al. (2020). Hybrid Organic-Inorganic Materials and Composites for Photoelectrochemical Water Splitting. ACS Energ. Lett. 5, 1487–1497. doi:10.1021/acsenergylett.0c00327
Slavney, A. H., Hu, T., Lindenberg, A. M., and Karunadasa, H. I. (2016). A Bismuth-Halide Double Perovskite with Long Carrier Recombination Lifetime for Photovoltaic Applications. J. Am. Chem. Soc. 138, 2138–2141. doi:10.1021/jacs.5b13294
Soltani, T., Tayyebi, A., and Lee, B.-K. (2018). Quick and Enhanced Degradation of Bisphenol A by Activation of Potassium Peroxymonosulfate to SO4− with Mn-Doped BiFeO3 Nanoparticles as a Heterogeneous Fenton-Like Catalyst. Appl. Surf. Sci. 441, 853–861. doi:10.1016/j.apsusc.2018.02.063
Takasugi, S., Tomita, K., Iwaoka, M., Kato, H., and Kakihana, M. (2015). The Hydrothermal and Solvothermal Synthesis of LiTaO3 Photocatalyst: Suppressing the Deterioration of the Water Splitting Activity Without Using a Cocatalyst. Int. J. Hydrogen Energ. 40, 5638–5643. doi:10.1016/j.ijhydene.2015.02.121
Tang, C., Chen, C., Xu, W., and Xu, L. (2019). Design of Doped Cesium Lead Halide Perovskite as a Photo-Catalytic CO2 Reduction Catalyst. J. Mater. Chem. A. 7, 6911–6919. doi:10.1039/c9ta00550a
Tang, J., Durrant, J. R., and Klug, D. R. (2008). Mechanism of Photocatalytic Water Splitting in TiO2. Reaction of Water with Photoholes, Importance of Charge Carrier Dynamics, and Evidence for Four-Hole Chemistry. J. Am. Chem. Soc. 130, 13885–13891. doi:10.1021/ja8034637
Tasleem, S., and Tahir, M. (2020). Recent Progress in Structural Development and Band Engineering of Perovskites Materials for Photocatalytic Solar Hydrogen Production: A Review. Int. J. Hydrogen. Energ. 45, 19078–19111. doi:10.1016/j.ijhydene.2020.05.090
Tauc, J., and Menth, A. (1972). States in the Gap. J. Non-Crystalline Sol. 8-10, 569–585. doi:10.1016/0022-3093(72)90194-9
Toshniwal, A., and Kheraj, V. (2017). Development of Organic-Inorganic Tin Halide Perovskites: A Review. Solar Energy 149, 54–59. doi:10.1016/j.solener.2017.03.077
Volonakis, G., and Giustino, F. (2018). Surface Properties of Lead-Free Halide Double Perovskites: Possible Visible-Light Photo-Catalysts for Water Splitting. Appl. Phys. Lett. 112, 243901. doi:10.1063/1.5035274
Walter, M. G., Warren, E. L., McKone, J. R., Boettcher, S. W., Mi, Q., Santori, E. A., et al. (2010). Solar Water Splitting Cells. Chem. Rev. 110, 6446–6473. doi:10.1021/cr1002326
Wang, M., Fang, M., Min, X., Huang, Z., Tang, C., Liu, Y. g., et al. (2017). Molten Salt Synthesis of NaNbxTa1−xO3 Perovskites with Enhanced Photocatalytic Activity. Chem. Phys. Lett. 686, 18–25. doi:10.1016/j.cplett.2017.08.029
Wang, R., Zhu, Y., Qiu, Y., Leung, C.-F., He, J., Liu, G., et al. (2013). Synthesis of Nitrogen-Doped KNbO3 Nanocubes with High Photocatalytic Activity for Water Splitting and Degradation of Organic Pollutants Under Visible Light. Chem. Eng. J. 226, 123–130. doi:10.1016/j.cej.2013.04.049
Wang, W., Xu, X., Zhou, W., and Shao, Z. (2017). Recent Progress in Metal‐Organic Frameworks for Applications in Electrocatalytic and Photocatalytic Water Splitting. Adv. Sci. 4, 1600371. doi:10.1002/advs.201600371
Wang, Y., Wang, L., Liu, R., and Li, X. (2019). Casein Templated Synthesis of Porous Perovskite and its Application in Visible-Light Photocatalytic Degradation of Methylene Blue. Mater. Sci. Semiconductor Process. 103, 104597. doi:10.1016/j.mssp.2019.104597
Wood, A., Giersig, M., and Mulvaney, P. (2001). Fermi Level Equilibration in Quantum Dot−Metal Nanojunctions. J. Phys. Chem. B 105, 8810–8815. doi:10.1021/jp011576t
Wu, D., Zhao, X., Huang, Y., Lai, J., Li, H., Yang, J., et al. (2021). Lead-Free Perovskite Cs2AgBiX6 Nanocrystals with a Band Gap Funnel Structure for Photocatalytic CO2 Reduction Under Visible Light. Chem. Mater. 33, 4971–4976. doi:10.1021/acs.chemmater.1c00753
Wu, X., Yin, S., Dong, Q., and Sato, T. (2013). Preparation and Visible Light Induced Photocatalytic Activity of C-NaTaO3 and C-NaTaO3-Cl-TiO2 Composite. Phys. Chem. Chem. Phys. 15, 20633–20640. doi:10.1039/c3cp53437e
Wu, Y., Chen, W., Chen, G., Liu, L., He, Z., and Liu, R. (2018). The Impact of Hybrid Compositional Film/Structure on Organic-Inorganic Perovskite Solar Cells. Nanomaterials 8, 1–27. doi:10.3390/nano8060356
Xia, Y., Zhang, L., Hu, B., Yu, J., Al-Ghamdi, A. A., and Wageh, S. (2021). Design of Highly-Active Photocatalytic Materials for Solar Fuel Production. Chem. Eng. J. 421, 127732. doi:10.1016/j.cej.2020.127732
Xiao, H., Liu, P., Wang, W., Ran, R., Zhou, W., and Shao, Z. (2020). Ruddlesden−Popper Perovskite Oxides for Photocatalysis-Based Water Splitting and Wastewater Treatment. Energy Fuels. 34, 9208–9221. doi:10.1021/acs.energyfuels.0c02301
Xu, Y.-F., Yang, M.-Z., Chen, B.-X., Wang, X.-D., Chen, H.-Y., Kuang, D.-B., et al. (2017). A CsPbBr3 Perovskite Quantum Dot/Graphene Oxide Composite for Photocatalytic CO2 Reduction. J. Am. Chem. Soc. 139, 5660–5663. doi:10.1021/jacs.7b00489
Yan, Y., Yang, H., Zhao, X., Li, R., and Wang, X. (2018). Enhanced Photocatalytic Activity of Surface Disorder-Engineered CaTiO3. Mater. Res. Bull. 105, 286–290. doi:10.1016/j.materresbull.2018.05.008
Yang, C.-K., Yamazaki, Y., Aydin, A., and Haile, S. M. (2014). Thermodynamic and Kinetic Assessments of Strontium-Doped Lanthanum Manganite Perovskites for Two-Step Thermochemical Water Splitting. J. Mater. Chem. A. 2, 13612–13623. doi:10.1039/c4ta02694b
Yang, W. S., Noh, J. H., Jeon, N. J., Kim, Y. C., Ryu, S., Seo, J., et al. (2015). High-Performance Photovoltaic Perovskite Layers Fabricated Through Intramolecular Exchange. Science 348, 1234–1237. doi:10.1126/science.aaa9272
Yang, Y., Kang, L., and Li, H. (2019). Enhancement of Photocatalytic Hydrogen Production of BiFeO3 by Gd3+ Doping. Ceramics Int. 45, 8017–8022. doi:10.1016/j.ceramint.2018.12.150
Yousif, N., and Gurjar, B. R. (2018). “Water Pollution, Human Health and Remediation,” in Water Remediat. Energy, Environ. Sustain., 1–50. doi:10.1016/j.neuropsychologia.2015.07.010%0A
Yu, H., Ouyang, S., Yan, S., Li, Z., Yu, T., and Zou, Z. (2011). Sol-Gel Hydrothermal Synthesis of Visible-Light-Driven Cr-Doped SrTiO3 for Efficient Hydrogen Production. J. Mater. Chem. 21, 11347–11351. doi:10.1039/c1jm11385b
Zanatta, A. R. (2019). Revisiting the Optical Bandgap of Semiconductors and the Proposal of a Unified Methodology to its Determination. Sci. Rep. 9, 11225. doi:10.1038/s41598-019-47670-y
Zhang, G., Liu, G., Wang, L., and Irvine, J. T. S. (2016). Inorganic Perovskite Photocatalysts for Solar Energy Utilization. Chem. Soc. Rev. 45, 5951–5984. doi:10.1039/c5cs00769k
Zhang, L., Nie, Y., Hu, C., and Qu, J. (2012). Enhanced Fenton Degradation of Rhodamine B Over Nanoscaled Cu-Doped LaTiO3 Perovskite. Appl. Catal. B: Environ. 125, 418–424. doi:10.1016/j.apcatb.2012.06.015
Keywords: photocatalysis, band gap, lifetime, water pollution, perovskite
Citation: Clabel H. JL, Chacaliaza-Ricaldi J and Marega Jr E (2022) Potential Application of Perovskite Structure for Water Treatment: Effects of Band Gap, Band Edges, and Lifetime of Charge Carrier for Photocatalysis. Front. Nanotechnol. 4:827925. doi: 10.3389/fnano.2022.827925
Received: 02 December 2021; Accepted: 14 March 2022;
Published: 09 June 2022.
Edited by:
Alexandre Pinto, Manhattan College, United StatesReviewed by:
Kesong Yang, University of California, San Diego, United StatesJun Ke, Wuhan Institute of Technology, China
Copyright © 2022 Clabel H., Chacaliaza-Ricaldi and Marega Jr. This is an open-access article distributed under the terms of the Creative Commons Attribution License (CC BY). The use, distribution or reproduction in other forums is permitted, provided the original author(s) and the copyright owner(s) are credited and that the original publication in this journal is cited, in accordance with accepted academic practice. No use, distribution or reproduction is permitted which does not comply with these terms.
*Correspondence: J. L. Clabel H., amNsYWJlbEBpZnNjLnVzcC5icg==