- 1Department of Biomedical Engineering, Manipal Institute of Technology, Manipal Academy of Higher Education, Manipal, India
- 2Sunlux Technovations Pvt Ltd, Bangalore, Manipal, India
The rapid development of wearable sensor technology can be attributed to developments in materials, microelectronics, fabrication, communication systems, and Artificial Intelligence (AI). The use of wearable sensors enables continuous acquisition and monitoring of the pathophysiological parameters of a person in real time. The global market for health-related wearables has experienced significant growth, particularly in response to the COVID-19 pandemic. A wearable sensor module is comprised of various components, including a powering unit, sensor(s), acquisition unit, communication unit, and processing unit. The non-fluctuating power source with a long life is of utmost significance to the continuous and real-time operation of a wearable sensor. A wearable device can be powered by a rechargeable battery, such as a lithium-ion battery, which can be charged from a standard power source but requires regular recharging after depletion and has a negative environmental impact. This necessitates using green renewable energy sources like photovoltaic cells, piezoelectric generators, wind energy converters, and thermoelectric generators for powering wearable sensor modules. The photovoltaic cell that converts photonics into electrical energy is deemed a viable green energy source for wearable sensor modules. This article reviews the progress and application of photovoltaic technology in wearable sensor modules.
1 Introduction
Research to develop novel wearable sensors for health monitoring has recently gained momentum, and scientists are constantly exploring new materials and configurations to enhance the sensitivity, selectivity, efficiency, and cost-effectiveness of wearable sensors. A wearable sensor module typically consists of a sensing element, power source, transducer, wireless transmitter, receiving console or computer for display and analysis (Capineri, 2014; Abshirini et al., 2019; Jia et al., 2022; Mukhopadhyay et al., 2022). The basic requirements of the power source for wearable sensor modules are 1) a small footprint, 2) lightweight, 3) good power output and 4) the ability to deliver adequate power for a long operational duration (Chen et al., 2010; Nguyen et al., 2010; Zhang et al., 2014; Mohs et al., 2021; Yin et al., 2021; Yin et al., 2022). The most common power sources are based on electrochemical units, such as rechargeable batteries or supercapacitors. The energy and power density of electrochemical power sources are limited. At the same time, acquiring and transmitting various signals for a longer operational time requires a continuous and sustained supply of electrical energy to the different units of a wearable device. Due to limited energy and power output, electrochemical power sources must be recharged multiple times. To reduce the frequency of these recharging, a sensor that can generate power may be incorporated along with low power-consuming components or units in the wearable sensor module. Even in such cases, the power generated by these sensors may not be sufficient to operate the entire sensor module, and the modules would still depend upon an electrochemical power source. The limitations of electrochemical power sources have impeded the development of next-generation wearable devices that combine materials research and engineering efforts for sustainable energy management. In wearable contexts, various energy sources could be tapped for harvesting energy, such as solar energy, radio waves, movements in the body, thermal gradients and biofuel-based energy harvesters (Hamid and Yuce, 2017; Chong et al., 2019; Khalid et al., 2019).
The advantages and disadvantages of each energy source vary depending on the context(s) in which they are utilised. It is possible to harness energy from body motions using piezoelectric, electromagnetic, or triboelectric generators. Nonetheless, this process requires active movements (such as vibrations) and continuous physical activities from the user (á Nozariasbmarz et al., 2020; Zou et al., 2020). The estimated power density of human motion-derived energy is 45.95 mW and 76.25 mW during walking and jogging, respectively. Thermoelectric generators, with an average power output of µ10 Wcm-2, can harvest thermal gradients between the skin and the surrounding air (Nozariasbmarz et al., 2020). The energy harvesters based on thermal gradients and the presence of biofuels like sweat may not always be uniform and usually require a sizeable thermal gradient or sweating to generate sufficient power to operate a wearable sensor module. Other issues using these energy harvesters are scalability, washability, flexibility and cost, which limits their scope in wearable devices as power sources.
The energy required to power wearables:
Solar energy is widely recognized as a promising alternative renewable energy to conventional non-renewable energy sources, owing to its abundance, affordability, renewability, and notable environmental friendliness (Jokic and Magno, 2017; Kartsch et al., 2018; Páez-Montoro et al., 2022). Photovoltaic (PV) modules or solar cells convert light energy into electrical energy (Singh, 2013). Over 60 years, scientists have developed photovoltaics by addressing challenges related to conversion efficiency, cost, materials and manufacturing methods and other features like flexibility (Lin et al., 2014; Kim S. et al., 2021). These developments in photovoltaics can be categorised into three discernible generations. The primary composition of the first generation of solar cells consists primarily of wafers produced from crystalline silicon (c-Si) (Huld et al., 2011; Kim S. et al., 2021). The subsequent development of thin film solar cells encompasses III-V solar cells, which are composed of several inorganic thin films, including amorphous silicon (a-Si), CdTe, and CuInGaSe2 (CIGS) (Britt and Ferekides, 1993; Ichikawa et al., 2001; Carron et al., 2019), among other options. Further, photovoltaics technology was extended to thin film photovoltaics like organic, dye-sensitised, and perovskite cells, which can be considered the third generation of solar cells (Weerasinghe et al., 2013; Jung et al., 2019; Fukuda et al., 2020).
Crystalline silicon (c-Si) solar cells currently dominate the industry, constituting around 90% of the market share (Baiju and Yarema, 2022). On the other hand, quantum dots (QDs) are primarily utilised for their unique material composition in developing photonic devices (Selopal et al., 2020). In contrast to the energy harvesters from movements, thermal gradient and biofuels, photovoltaic (PV) cells have reported power densities of 10–100 mWcm-2 in ambient indoor light and 100 mWcm-2 in direct sunlight outdoors (Chong et al., 2019). These power densities are independent of user activity. Hence, the photovoltaic converters can be deemed a possible energy source for powering the wearable sensor modules. The energy of solar panels can be defined as below.
The energy of solar panels:
2 Photovoltaic technologies for wearable sensors
The widespread utilisation of personal wearable devices has resulted in a growing need for portable power sources (Seneviratne et al., 2017; Saadatnejad et al., 2019). When incorporating personal electronics into clothing to meet the demands of smart wearable technology, it is crucial to ensure that the garments will not lose essential properties such as stretchability, moisture resistance, and stable operational performance. The power supply systems should not affect the comfort of wearing the sensor modules. Hence, integrating wearable electronics into clothing necessitates characteristics such as stretchability, moisture resistance, and consistent functioning performance. In this regard, traditional silicon-based solar cells lacking stretchability and flexibility is a significant challenge for integrating as a power supply in the wearable sensor module.
The utilisation of flexible solar cells in wearable devices offers multiple benefits. In contrast to conventional solar panels, these cells are lightweight, thin, and very flexible, rendering them appropriate for incorporation into diverse wearable devices, like smartwatches, fitness bands, and even garments. The inherent flexibility of the solar cells enables convenient customisation, facilitating their seamless integration into the overall design allowing unmatched mobility of these devices. Solar cells provide a sustainable alternative to conventional non-renewable energy sources for powering wearable devices (Figure 1).
2.1 Silicon solar cells
Silicon-based are extensively used as photovoltaic cells. In the fabrication process of a solar cell, it is necessary to introduce doping in one or more silicon layers. This involves the addition of impurities to generate n-type or p-type semiconducting layers. The majority carriers of p-type are holes, and n-type layers are electrons, respectively. Photovoltaic cells are prepared by arrangement of a doped p-type layer close to an n-type layer. Electrons and holes are in motion within the layers, creating an internal electric field. When light interacts with a photovoltaic cell, the incident light energy is absorbed, leading to the excitation of electrons and the subsequent generation of electron and hole pairs. The excited electrons in the conduction band move towards the n-type layer and the corresponding hole to the p-type layer, resulting in the current in photovoltaic cells. The typical efficiency of these solar cells is approximately 20%, significantly influenced by the time and intensity of the light exposure (Mulligan et al., 2004; Baca et al., 2010; Bullock et al., 2016; Hwang et al., 2020). Most crystalline silicon (c-Si) solar cells separate photogenerated electrons and holes using doped homojunction.
However, homojunction can lead to issues such as loss in the optics, problems with carrier transport, non-generation of hole and electron due to parasitic absorption, and undesired recombination such as Auger recombination, (Kim J. et al., 2021). The technological obstacles in developing homojunction related to doping, such as elevated processing temperatures, limited contact fractions, removal of dopant glass, and isolation of junctions, can be mitigated by implementing designs incorporating asymmetric carrier-selective heterocontacts (Bullock et al., 2016). Carrier-selective heterocontacts achieve low resistance for a specific charge carrier while impeding the flow of the opposite charge carrier. Several processes can generate carrier-specific heterocontact, including passivating layers on the surface and stacks that introduce asymmetry in the conductivity through creating band offsets, tunnelling effects, or band bending in crystalline silicon (c-Si). The silicon heterojunction cell architecture (SHA) has demonstrated superior efficiency to its homojunction counterpart, achieving the record for crystalline silicon (c-Si) solar cells in 2014 (Bush et al., 2017). Fabrication of asymmetric heterocontacts persistently depends on doped-silicon layers, which necessitate intricate depositions incurring parasitic optical losses. Further developments in the asymmetric carrier-selective heterocontact involve completely substituting doped-silicon layers with alternative materials like amorphous silicon to overcome the inherent limitations and practical challenges associated with doped silicon layers.
The work by (Kabir et al., 2018) proposed a strategy for the large-scale fabrication of foldable and flexible crystalline silicon solar cells by introducing cracks in the wafers. These wafers have a pyramidal structure on the surface to reduce the surface reflectance and increase the optical path length of the incident light. The cracks in the wafers are initiated at the sharp channels located between surface pyramids within the peripheral area of the wafer. The malleability of silicon wafers can be enhanced by reducing the sharpness of the pyramidal structure in the peripheral regions. Incan h et al. (Hwang et al., 2020) employed a random inverted-pyramidal polydimethylsiloxane (RIP-PDMS) film to achieve efficient photon control on thin c-Si solar cells, resulting in an efficiency of 24%. The thin c-Si solar cells, when combined with RIP-PDMS sheets, exhibit a notable 85.4% enhancement in integrated photon flux compared to the unmodified thin c-Si solar cells with a photon flux of 75.0%. The RIP-PDMS films showed light absorption within the wavelength range of 300 to 1,100 nm, leading to a notable increase in efficiency by 17.3% compared to the identical device lacking the film. The efficiency of heterojunction with intrinsic thin layer (HIT) c-Si solar cells with RIP-PDMS sheets is 18.4%. The utilisation of thin c-Si flexible solar cells in conjunction with RIP-PDMS optical films has demonstrated remarkable stability when bending, establishing them as a viable and promising option for developing flexible solar cells with exceptional efficiency. The apparent drawback associated with the decreased lifetimes of minority carriers in the bulk material resulted in diminished sensitivity to longer wavelengths of light and a consequent decrease in short-circuit current. However, this negative effect was counterbalanced by the enhanced voltage. Several drawbacks are associated with silicon-based solar cell technology, including their high cost, substantial weight, limited availability of silicon resources, and production methods that result in significant environmental damage and ensuring cleanliness on the illuminated surface is of utmost importance.
Wearable electronics that use flexible solar cells rely less on traditional charging methods, alleviating users’ need to recharge their devices often. The ability to utilise ambient light as an energy source has enabled users to conveniently recharge their wearable devices while in motion, enhancing their self-sufficiency and diminishing reliance on conventional power sources.
2.2 Quantum dot solar cells
Quantum dot solar cells rely on quantum dots as the primary material for absorbing light. Quantum dots refer to semiconductor particles at the nanoscale that possess distinctive optical and electrical characteristics due to the confinement of the excitons (Aroutiounian et al., 2001; Raffaelle et al., 2002; Kamat, 2008; Kamat, 2013; Kramer and Sargent, 2014). The bandgap of these materials can be adjusted by manipulating their dimensions, enabling light absorption across a broad spectrum of wavelengths (Chuang et al., 2015; Ramiro et al., 2015; Lu et al., 2019; Sharma and Jha, 2019; Mahajan et al., 2020; Nideep et al., 2020). When the dimensions of nanocrystals (NCs) approach or fall below the Bohr radius of the electron-hole bound state, commonly referred to as an exciton, the spatial mobility of electrons and holes becomes limited. Consequently, the energy levels of electrons and holes become discretised. A direct relationship exists between the magnitude of the band gap and the dimensions of quantum dots (QDs), whereby an increase in the band gap corresponds to a decrease in the size of the QDs (Lu et al., 2020a; Sun et al., 2023; Zhao et al., 2023). These materials’ optical and electrical properties are altered due to quantum confinement effects. Using near-infrared (NIR)-absorbing quantum dots (QDs) expands the absorption range in the near-infrared spectrum. Quantum dots (QDs) exhibit a lower photon energy threshold required for generating multiple excitons than perovskites, conventional bulk semiconductors, and organic semiconductors (Chang et al., 2013; Carey et al., 2015; Yang et al., 2020). This characteristic enables quantum dot photovoltaics (QDPVs) to exceed the theoretical efficiency SQ limit. Due to spatial confinement, the Coulomb potential significantly influences the interaction between electron-hole pairs in free-standing quantum dots (QDs). This strong interaction enables the persistence of these pairs as excitons rather than as free carriers. The formation of free carriers can only occur when the excitons dissociate (Hou et al., 2020; Efros and Brus, 2021; Han et al., 2022). Quantum dots (QDs) have the potential to enhance the efficiency of electron-hole pair multiplication mechanisms by effectively utilising excess photon energy to generate additional electron-hole pairs, hence preventing its dissipation as thermal energy (Ikeri et al., 2021; Liu et al., 2022). Numerous electron-hole pairs, or excitons, can be generated from the absorption of a single photon through a phenomenon known as “quantum dot super fluorescence” (Lu et al., 2020b). Meanwhile, the considerable tunability of the bandgap exhibited by quantum dots facilitates effective energy capture throughout the near-to-short-wave infrared region of the solar spectrum.
Nevertheless, the current efficiency of Quantum Dot Photovoltaic (QDPV) in 1 Sun condition is lower when compared to Organic Photovoltaics (OPVs) and perovskite solar cells. This discrepancy primarily arises from the intricate synthesis process of the materials involved and the formation of defect states during the device’s construction. QDPVs generally exhibit a lower power conversion efficiency (PCE) of approximately 13% compared to OPVs and PPVs under standard one Sun conditions. However, QDPVs can attain superior device performance under low light settings owing to their significantly higher bandgap (Mishra et al., 2022). The band gaps of quantum dots (QDs) can be readily adjusted by manipulating their diameters due to the quantum confinement effect, rendering them desirable for solar cell applications. Approximately 50% of the solar irradiance is concentrated within the near-infrared (NIR) region, which remains largely not absorbed by dye molecules and organic semiconductors (Zampetti et al., 2019). The capture of near-infrared (NIR) photons gives a significant opportunity to enhance the efficiency of QD solar cells. PbS and PbSe have garnered considerable interest and have been extensively employed in thin-film solar applications due to their tiny band gap, often ranging from 1.4 to 0.8 eV or even below (Hu L. et al., 2019). Despite their considerable potential, quantum dot solar cells for wearable devices still encounter several hurdles. Considering stability and durability is crucial to guarantee sustained performance across various environmental conditions. Furthermore, the cost-effectiveness of quantum dot synthesis and fabrication would significantly contribute to their widespread utilisation in wearable applications.
It is anticipated that the efficiency and performance of QDSCs will be enhanced through the progress made in quantum dot technology and the implementation of novel fabrication methods. The ongoing development of wearable electronics has sparked interest in the potential of quantum dot solar cells to significantly transform the power supply infrastructure and facilitate the widespread integration of eco-friendly wearable technology. Quantum dot solar cells can be developed using colloidal synthesis, chemical bath deposition, inkjet printing, and vacuum-based techniques like molecular beam epitaxy or sputtering (Giménez et al., 2009; Yang et al., 2017; Das et al., 2019; Liu et al., 2020). The manufacturing process of QD solar cells involves the application of quantum dots onto a substrate, usually accomplished by a step-by-step assembly process and their integration into a device structure alongside a suitable charge transport layer. Quantum dot solar cells have demonstrated significant absorption coefficients, facilitating effective light capture. These solar cells can exceed the Shockley-Queisser limit (Semonin et al., 2012), establishing the upper-efficiency limit for traditional single-junction solar cells. Power conversion efficiency that has surpassed the threshold of 12% is exhibited by Quantum dot solar cells (Hu et al., 2021). Quantum dot solar cells can be used in portable electronics, low-light environments, and building-integrated photovoltaics, including flexible and transparent energy solutions (Huang et al., 2022; Riaz and Park, 2022; Kim et al., 2023). Integration of multiple solar cell technology in tandem can potentially be used to improve performance.
The tunability of the band gap in quantum dots (QDs) enables the optimisation of single-junction solar cells by varying the size of QDs. The highest power conversion efficiency of 31% was predicted theoretically by Shockley and Queisser for the single-junction, non-concentrated solar cells with a semiconductor as an absorber with an ideal band gap of 1.1 eV (Chen et al., 2021; Kamaraki et al., 2021). This band gap restriction imposed by the theoretical model significantly narrows down the range of semiconductors suitable for developing ideal photovoltaics for wearable applications. To mitigate this constraint, low-band-gap quantum dots (QDs) such as PbS and PbSe QDs can be used (Luo et al., 2019; Nishimura et al., 2019). The studies by Beard and others (Gao et al., 2011) found a correlation between the size of quantum dots (QDs) and the photoconversion efficiency of PbS/ZnO solar cells. The precise value band gap did not mention their work. Liayang’s team (Chang et al., 2013) has obtained greater efficiency for PbSQDs with a band gap of 1.33 eV. The band gap value mentioned by Liayang’s team is close to the theoretical values calculated by (Carey et al., 2015). The research conducted by Sargent et al. focused on investigating the photovoltaic performance of p-n heterojunction solar cells with PbS quantum dots (QDs) and titanium dioxide (TiO2) junction. The study involved QDs with three diameters, 3.7nm, 4.3 nm and 5.5nm, corresponding band gaps of 1.3 eV, 1.1 eV, and 0.9 eV. It was observed that the optimal band gap for achieving the highest performance was 1.3 eV, as indicated by the most significant values for open-circuit voltage (VOC), short-circuit current density (ISC), fill factor (FF), and power conversion efficiency (PCE).
The performance of solar cells is also influenced by the architecture of the solar cell devices apart from light absorption. Multiple layers of quantum dots with varying sizes can be used to enhance photon collection efficiency through a broader spectrum of wavelengths. This strategy aims to substantially augment the overall efficiency of the photovoltaic cells for indoor applications (Yang et al., 2020). According to theoretical predictions, the efficiency of tandem (double-junction) and triple-junction cells is expected to surpass the Shockley limit of 31%, reaching 42% and 49%, respectively (Hu Y. et al., 2019; Gan et al., 2021). The commercialisation of these solar cells has been limited due to the expensive manufacturing process and the requirements for close lattice matching between different semiconductor materials in various layers. While the concept of epitaxial compound semiconductor thin films has been successfully demonstrated, its widespread implementation has been impeded. Utilising size-tunable quantum dots (QDs) could potentially facilitate the production of these multijunction cells and the synthesis of photoactive materials (Z et al., 2022). Recent development in tandem QD cells involves the introduction of a graded recombination layer (GRL) positioned between junctions. The GRL layer connects the junctions and improves the flow of charge carriers by reducing the energetic barrier between the front and back cells in tandem multijunction photovoltaics (Wang et al., 2011). The tandem quantum dot (QD) solar cells use a front cell made of a quantum dot layer with an energy bandgap of 1.6 electron volts (eV), while the back cell consists of a quantum dot layer with an energy bandgap of 1.0 eV. The study lays the groundwork for utilising multijunction solar cells in wearable applications.
Huang et al. (Huang et al., 2011) reported a novel strategy to fabricate flexible solar cells utilising CdS/CdSe quantum dots. A photoanode with varied thickness was incorporated with CdS/CdSe quantum dots (QDs) to achieve flexibility. The highest current density achieved was 12.3 J/mAcm-2, with an efficiency rating of 87%. This photovoltaic facilitates easy integration with wearable devices. Quantum dot solar cells are an alternative to conventional solar cells in wearable technology due to tunable characteristics such as enhanced light-capturing abilities, small dimensions, and adaptability (Figure 2). Several materials, such as cadmium selenide (CdSe), lead sulfide (PbS), and perovskite minerals, can make quantum dots. This material variety makes it possible to customise QDSCs to meet the needs of particular applications (Table 1). Many quantum dot materials, like those made of cadmium, are toxic and cause environmental and human health problems during manufacture, use, and disposal. More importantly, due to substantially higher production and application costs, large-scale manufacturing of QDs may not be possible. Their widespread use in real-world applications is therefore constrained. Some QDSCs include hysteresis effects, where the direction of the voltage sweep affects the output current-voltage characteristics. These factors may hamper the design and control of QDSCs.
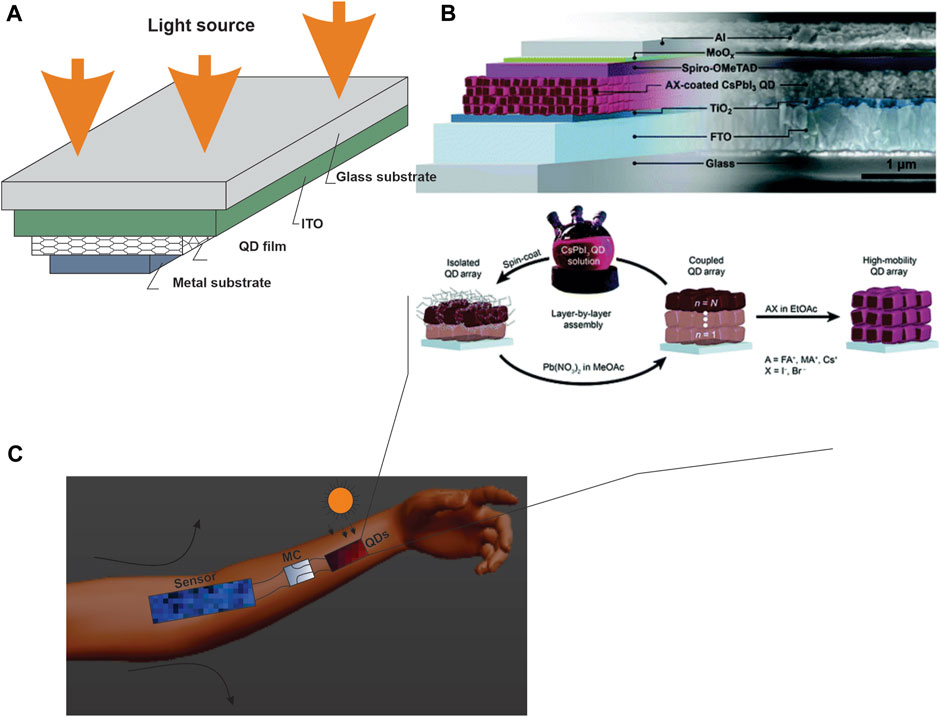
FIGURE 2. (A) working concept of QD solar cell, (B) graphical representation of cross section of solar cell. Reproduced from Sanehira et al. (2017), licensed under CC BY-NC, (C) schematic depiction of Flexible QD based solar cell to wearable sensor.
2.3 Dye-sensitised solar cell
Dye-sensitised solar cells (DSSCs) have garnered considerable interest from both academic and industrial sectors due to their production cost-effectiveness, compact structural design, and efficient power conversion capabilities across varying light intensities. DSSCs utilise organic dyes as photosensitisers and semiconductors to convert light to electrical energy. In 1991, O'Regan and Grätzel (Aslam et al., 2020) reported the development of Ruthenium dye-sensitised solar cells (DSCs) that used wide band gap titania semiconducting film with an efficiency of 7.12%. This advancement marked the commencement of a novel phase in photovoltaic (PV) research, and a remarkable achievement of 13% PCE was obtained under AM1.5 light conditions (Sun and Sariciftci, 2017). Dye-sensitised solar cells (DSCs) exhibit notable attributes such as optical clarity, a wide range of colour options, resistance to variations in lighting conditions, affordability, and straightforward manufacturing processes (Baxter et al., 2009; Bari, 2014; Meddeb et al., 2022).
Furthermore, these solar cells exhibit comparatively more flexibility and low weight, depending upon the specific substrate employed. Due to these distinctive characteristics, dye-sensitised solar cells (DSCs) are well-suited as a power source for wearable sensor modules and other applications such as building-integrated photovoltaics (BIPV), automotive-integrated photovoltaics (AIPV), as well as portable and interior power producers (Ito et al., 2008; Alnoman et al., 2022). The photo-electrode requires flexible substrates with crucial characteristics such as transparency and conductivity. This is because the dyed semiconducting film, such as TiO2, responsible for capturing incident light and facilitating the passage of photoelectrons, is directly affixed to the substrate (Shankar et al., 2009; Friesen, 2013). Besides ruthenium complex dye, porphyrin and organic dyes showed power conversion efficiencies (PCEs) of 13% and 14.7%, respectively (Koteshwar et al., 2022). Nevertheless, using liquid electrolytes (LEs) may lead to leakage and vaporisation, diminishing the overall long-term robustness of dye-sensitised solar cells (DSSCs). Replacing liquid electrolytes (LEs) with solid-state and polymer gel electrolytes has enhanced the long-term durability of dye-sensitised solar cells (DSSCs). However, this substitution has also led to a decrease in their power conversion efficiencies (PCEs). The Internet of Things (IoT) has garnered considerable attention in science and technology due to its applications in several domains, such as smart homes, agriculture, healthcare, transportation, and industry. Dye-sensitised solar cells (DSSCs) are considered highly suitable energy sources for Internet of Things (IoT) devices due to their ability to convert indoor light into electrical energy without the need for further energy input (Chen et al., 2017; Yoo et al., 2020; Kokkonen et al., 2021).
Wearable photovoltaic-powered textiles with good stretchability were fabricated by (Yang et al., 2014), utilising electrically conducting elastic fibers. The fiber electrodes were initially manufactured by wrapping aligned multi-walled carbon nanotube (MWCNT) sheets around rubber fibres with excellent and consistent electrical characteristics when stretching. A Titanium wire that had been modified was utilised as the working electrode and subsequently twisted onto the elastic MWCNT fiber. This was followed by applying photoactive chemicals to create a wire-shaped dye-sensitised solar cell (DSC). The wire-shaped dye-sensitised solar cells (DSCs) were ultimately integrated into the intended stretchable and wearable photovoltaic cloth. The dye-sensitised solar cells (DSCs) used in this study exhibited notable energy conversion efficiencies of 7.13%, which were effectively sustained even when subjected to stretching. Most DSSC substrates are made of plastic and have a low thermal processing limit of about 150 °C. Low-temperature processing reduces semiconductor film adherence to the substrate and results in poor electrical contact between semiconductor particles when plastic substrates are used. Compared to conventional silicon-based solar cells, DSSCs have the potential for cheaper manufacturing costs. The manufacturing techniques and the cost of the raw materials are frequently lower. Due to their low manufacturing costs, simplicity in construction, and customisable optical characteristics, such as colour and transparency, dye-sensitised solar cells have attracted much attention recently.
2.4 Perovskite solar cells
Perovskites have a crystalline structure resembling calcium titanate (CaTiO3) that has shown potential for developing high-performance solar cells with low production costs (Li et al., 2020). Recently developed solid-state solar cells based on inorganic-organic light-absorbing halide perovskites have shown great promise in wearable/portable devices (Kim et al., 2015; Lee et al., 2019). This is due to their economically and practically feasible fabrication processes involving low temperatures and the roll-to-roll technique. The band gap of perovskites can be altered by changing the chemical composition and achieve a broader photon absorption efficiency (Akin et al., 2020). This tunability of the band gap is one of the crucial requirements for selecting the photovoltaic power sources for wearable sensor modules, as the PVs should be able to absorb light from different light sources even during indoor conditions. These solar cells exhibit a high-power conversion efficiency (PCE) exceeding 16%, further highlighting their potential as leading candidates in the wearable technology domain (Chen et al., 2019). Numerous research groups have recently attempted to develop flexible perovskite solar cells by utilising an all-low temperature manufacturing process operating at approximately 1,300 °C. Preliminary findings from these studies indicated that such perovskite solar cells may exhibit superior efficiency compared to flexible organic solar cells. The flexible perovskite solar cells employed two distinct light absorbers, namely, CH3NH3PbI3 and CH3NH3PbI05. The current highest power conversion efficiency (PCE) achieved by flexible perovskite solar cells is 10.2%, in which a layer with a thickness of 300 nm is used as a light absorber (Liu and Kelly, 2014). However, this value remains lower than that of rigid-type perovskite solar cells. This comparatively lower PCE is attributed to series resistance and rapid charge recombination during quick charging.
Nevertheless, these challenges can be addressed by improving the charge collection layer, specifically the TiO2 or ZnO compact layer, on the flexible substrate (Du et al., 2018). Perovskite solar cells are an innovation with excellent power conversion efficiency. These cells can be placed onto flexible substrates like PET or PEN for wearable applications. Flexibility and cost-effectiveness make perovskite-based devices attractive for wearable technologies. There exist three primary impediments that hinder the widespread commercialisation of PSCs. To begin with, it is imperative to address the inherent issue of low stability exhibited by the perovskite material, as this poses a significant obstacle in attaining sustained and reliable power output over an extended period. Additionally, it is necessary to implement measures to regulate and supervise the hazardous Pb2+ waste and contamination resulting from the irreversible degradation of perovskite materials. Furthermore, it is imperative to enhance the performance of PSCs to address the requirements of extensive production and real-world applications effectively. To address these challenges, valence state substitution can be employed to select non-toxic elements that meet the required criteria.
Perovskite solar cells exhibit significant potential in providing energy for wearable devices due to their advantageous characteristics, including higher efficiency, reduced weight, and enhanced flexibility. Perovskite solar cells are anticipated to significantly impact the future of wearable technology since ongoing research addresses issues about stability, toxicity, and manufacturing scalability. The capacity of energy harvesting technologies to offer a durable and adequate power supply for wearable devices has the potential to bring about a transformative impact on how we engage with and utilise such gadgets. This, in turn, could facilitate the development of more sophisticated and ecologically conscious wearable technology. The potential of perovskite solar cells to transform the landscape of wearable technology is evident by their continuous advances and increasing commercialisation (Figure 3).
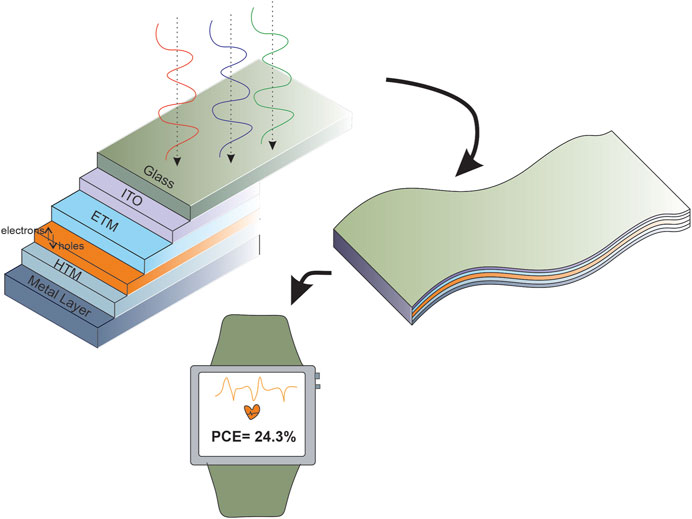
FIGURE 3. Construction of flexible solar cells depicts as using wearable belt for powering smart gadgets.
2.4.1 Perovskite-silicon tandem solar cells
The ongoing development of tandem solar cells incorporating perovskite and silicon materials aims to achieve stability, stretchability and enhanced efficiency. Perovskite-silicon tandem solar cells represent a specific configuration of solar cell architecture wherein a silicon solar cell is integrated with a perovskite solar cell, resulting in enhanced overall efficiency of the photovoltaic system (Sahli et al., 2018; Al-Ashouri et al., 2020; Chen et al., 2022). Perovskite and silicon solar cells are frequently combined in a stacked configuration to form tandem solar cells. The perovskite layer exhibits absorption of high-energy photons, while the silicon layer demonstrates absorption of lower-energy photons (Wali et al., 2018). This enables the enhanced utilisation of the solar spectrum. Tandem solar cells composed of perovskite and silicon have exhibited encouraging performance concerning power conversion efficiency (PCE). Compared to individual solar cells, tandem structures can achieve enhanced efficiency by using the complementary absorption characteristics of silicon and perovskite. Based on a recent study, it has been shown that the efficiency of tandem solar cells has exceeded 25% (Gharibzadeh et al., 2020). Tandem solar cells possess the capacity to revolutionise the solar energy industry through the enhancement of conversion efficiency and reduction of the cost per watt of solar electricity. Integrating the advantages of perovskite and silicon materials, including enhanced efficiency and flexibility, renders them very suitable for wearable applications. It should be mentioned that there are numerous kinds of multijunction (tandem) solar cells, including those based on III-V semiconductors. However, their commercialisation options are constrained by their high manufacturing costs and challenging manufacturing methods. Some researchers showed tin PSCs to be the next-generation of PSCs capable of exceeding 20% efficiency, and procedures for their mass manufacture have been looked into. Since perovskite solar cells can be produced on flexible substrates, they are appropriate for wearable technology and other uses that call for portable, adaptable power sources. The combination of perovskite and silicon materials presents a promising opportunity to develop cost-effective solar cells with enhanced efficiency. Tandem solar cells exhibit versatility in their applicability, encompassing diverse uses such as integrated photovoltaics in architectural structures, solar farms, portable electronic gadgets, and rooftop installations (Miller, 2012).
Metal oxides such as ZnO, SnO2, and TiO2 are frequently employed in conventional Perovskite solar cells (Irfan et al., 2022). When comparing Sno2 to other materials, it is shown that Sno2 exhibits enhanced mobility in the migration from the perovskite layer to the electron transfer layer (ETLs). Additionally, the deposition of charge is also reduced at the interface. J. Qi (Zhang et al., 2018) employed the hydrothermal process to synthesise SnO2 and utilised it as the electron transport layer (ETL) for perovskite solar cells (PSCs). This article reports an efficiency of 17.68% for PCE (Power Conversion Efficiency). Integrating perovskite-silicon tandem solar cells exhibits significant potential as a viable and high-performing energy solution for wearable technology.
2.4.2 Sensitised perovskite solar cells
The development of mesoscopic perovskite solar cells, also known as sensitised PSCs, has been influenced by the advancements in dye-sensitised solar cells (DSSCs). The quick evolution of mesoscopic perovskite solar cells can be linked to the progress achieved in DSSCs. Miyasaka (Kojima et al., 2009) conducted a study in which CH3NH3PbI3 and CH3NH3PbBr3 were utilised as sensitisers on mesoporous TiO2 substrates, replacing traditional dye molecules. When employing an organic liquid electrolyte, the resulting devices exhibited power conversion efficiencies (PCEs) of 3.81% and 3.1%, respectively (Table 1).
The substitution of the component in dye-sensitised solar cells (DSSCs), organic photovoltaic (OPVs), or perovskite solar cells (PSCs) with one-dimensional (1D) engineering nanomaterials has been extensively studies (Wu et al., 2020). This involves the utilisation of nanowires and nanofiber meshes as materials for hole or electron transport within individual solar cell components. In many instances, enhanced performances were attributed to nanoscale characteristics such as high aspect ratio and surface area, low density, or high pore volume. Although the electrospinning technique has demonstrated its versatility in producing nano- or microfibers from various inorganic or polymeric materials, developing organic-inorganic solar cells using this method has not been achieved thus far. Electrospinning technology is widely recognised for its ease of use, capacity for scaling up, and ability to achieve high production rates.
Third-generation solar cells, such as dye-sensitised solar cells (DSSCs) and perovskite solar cells (PSCs), can reduce processing costs compared to first- and second-generation silicon-based PV technologies. Dye-sensitised solar cells (DSSCs) have demonstrated remarkable progress in their power conversion efficiencies (PCEs), indoors reaching a value of 13% (Yang et al., 2011). Furthermore, when subjected to accelerated lifespan testing, these cells have exhibited extraordinary durability and efficiency. The recent PSC technology has achieved a Power Conversion Efficiency (PCE) 22.1%39. The National Renewable Energy Laboratory (NREL) has independently confirmed this achievement. Photovoltaic devices known as large-area perovskite solar cells (PSCs) that possess aperture sizes exceeding 1 cm2 have been demonstrated experimentally and proved to have a verified power conversion efficiency (PCE) of 19.6%, with a maximum PCE reaching 20.5% (Wang et al., 2018).
2.5 Organic-inorganic hybrid solar cells
These solar cells integrate organic and inorganic materials to exploit the respective benefits of each component. The organic components have the advantages of flexibility and cost-effectiveness in processing, whilst inorganic components contribute to enhanced stability and increased efficiency (Sharma et al., 2014). Various fabrication techniques can produce organic and inorganic hybrid solar cells, such as solution processing, vacuum deposition, and spin-coating procedures (Fan et al., 2013). The fabrication entails integrating organic and inorganic elements into a device structure, commonly achieved using layer-by-layer assembly or bulk heterojunction methodologies (Huang et al., 2010). Hybrid solar cells derive advantages from the synergistic characteristics exhibited by organic and inorganic materials. Organic materials show flexibility, lightweight nature, and tunable light absorption (Wright and Uddin, 2012). Conversely, inorganic materials display desirable attributes such as stability, fast charge mobility, and a wider variety of absorption capabilities. The interface between organic and inorganic materials facilitates the effective transfer and separation of charges, enhancing device performance. Several studies have found power conversion efficiencies over 15% in organic and inorganic hybrid solar cells, indicating their significant potential in this field (Wright and Uddin, 2012; Khan et al., 2019; Zhang et al., 2021; Zhao et al., 2021).
Organic photovoltaics (OPVs) are prevalent in wearable technology due to their flexibility and lightweight. OPVs can stretch and conform to the wearer’s movements when made on elastomeric substrates like PDMS or polyurethane. Recent OPV technological improvements have increased efficiency and durability, making them a feasible wearable energy harvesting option. One notable advantage of these solar cells is their ability to demonstrate a broader absorption spectrum than conventional organic or inorganic solar cells, facilitating improved light capture. Hybrid solar cells exhibit versatility in their applicability, including a diverse array of uses such as portable electronic gadgets, solar-powered apparatuses, and integration into the architecture of buildings. Due to their ability to be produced on flexible substrates, organic-inorganic hybrid solar cells are well suited for use in flexible electronics and wearable technologies. With values above 25%, organic-inorganic hybrid solar cells have displayed impressive power conversion efficiency. The distinctive optoelectronic characteristics of perovskite materials are credited with this efficiency. One of the significant perplexing problems seen in perovskite photovoltaics is abnormal hysteresis observed in the current-voltage response of HPSCs. Such hysteresis phenomena could result in inaccurate calculation of the solar cell device’s efficiency, which calls into question its dependability. This poses a significant barrier to advancement from both a research and commercialisation perspective. Flexible and transparent solar cells can be used to integrate into a wide range of substrates and surfaces. Hybrid solar cells exhibit potential for application in promising technologies, including wearable electronics and Internet of Things (IoT) devices.
2.6 Indoor photovoltaic for IoT and other applications
The scientific progress in the field of indoor photovoltaic (IPV) did not experience significant momentum until the year 2010. The present surge in research activity about IPV can largely be attributed to the advancements in IOT and wearable devices. Recently, indoor lighting tends to LED and FL bulbs, deemed more aesthetically pleasing and efficient than incandescent lights (Kim et al., 2022; Ding et al., 2023). Many sensor nodes within the swiftly growing IoT network, which rely on a power source for operation, exist. The main components of an IOT are sensors, actuators, electronics, storage units/memory devices, and communication units (Asemani et al., 2019). Notably, most of these nodes are situated within indoor structures. Due to technical progress, many IoT components’ energy consumption has significantly decreased. However, a significant obstacle to adopting the IoT ecosystem will be providing power to the billions of newly connected IoT devices. Simultaneously, the market for wireless sensors is experiencing growth due to the emergence of novel low-power network protocols incorporating energy-saving capabilities, such as radio frequency (RF) backscatter technology, Zigbee, BLE, Sigfox, and others. The global value of the IPV cell market is projected to reach up to $850 million by the year 2023 (Mathews et al., 2019). Although the current market share of the IPV sector in the global solar module industry is very tiny, estimated at less than 1%, its growth trajectory is expected to be rapid owing to its potential for specialised applications. The market for IPV is projected to experience a significant growth rate of 70% compounded annually, primarily propelled by the surge in the IoT market.
Operating the large PV cells outdoors is vital for supplying energy to the electrical grid and powering household appliances. Despite the notable disparity in light intensity and resulting power generation between indoor and outdoor environments, it is possible to incorporate product-integrated photovoltaics (PIPVs) into various wearable devices. These PIPVs can function at deficient power levels, ranging from 1 mW to 100 mW, and continuously harness the accessible indoor light (Jegadeeswari and Rekha, 2020). A wide range of gadgets, such as wearable devices like smartwatches, computers, remote controls, health monitoring devices, RFID tags, Bluetooth, and other PIPVs in diverse manners. The predominant proportion of (IoT) devices that operate on IPV are characterised by their autonomous configuration, indicating that they are not linked to a centralised power grid. An appealing product alternative is achieved by integrating flexible IPV cells with wearable devices. This capability enhances battery longevity and reduces the expenses associated with battery replacement and upkeep. Due to their ability to facilitate the integration of IPV cells across various applications, they possess a high level of appeal. The crucial elements in this context are lightweight, cost-effective, and exceptionally versatile solution-processable photovoltaic technology. Present-day structures such as residential buildings, commercial establishments, and corporate spaces are eliminated using artificial illumination, a potential photon-capturing source by indoor photovoltaic cells. This practice persists even in instances where natural light, either direct or indirect, may be readily available within interior settings during daylight hours. Different artificial illumination sources include incandescent lighting, halogen bulbs, compact fluorescent lamps (CFL), and light-emitting diode (LED) bulbs (Min et al., 2023).
Furthermore, apart from possessing unique designs and applications, each of these diverse light sources exhibits a distinct emission spectrum. The range of interior lights predominantly encompasses the visible light spectrum, which ranges from 400 to 700 nm. In contrast, the AM 1.5G solar spectrum contains a significantly broader range of wavelengths, extending from 300 to 2,500 nm (Park et al., 2022). Moreover, indoor light sources’ spectral irradiance exhibits significant disparities compared to natural sunlight. Indoor light sources often show intensities 100 to 1,000 times lower than the typical solar radiation of 100 mW cm2 or AM 1.5G (Huang et al., 2021). Illumination refers to the total quantity of light incident upon a particular surface or region. Lighting levels in indoor areas often vary between 100 and 1,000 lux (Kumar and Chen, 2023). In contrast to the external environment, the absence of a standardised approach for assessing the indoor performance of solar cells is evident when the “standard one Sun condition” is considered (Lübke et al., 2021).
The characterisation of indoor performance often involves utilising an illuminance level ranging from 200 to 1,000 lux. An illuminance level of 200 lux typically designates a dim interior environment, while a bright interior environment is denoted by an illuminance level of 1,000 lux (Muhammad et al., 2022). The energy collected by photovoltaic cells from different light sources has fluctuated due to their diverse spectral response, even when subjected to the same illumination (Li et al., 2019; Zarrabi, 2023).
To achieve high efficiency, it is required to utilise photovoltaic materials with a wide bandgap due to the restricted range of the indoor light spectrum, which typically falls within the 400–700 nm wavelength range (Yue et al., 2020). According to Freunek et al., (Freunek et al., 2012), the range of 1.90–2.00 eV is considered the best bandgap for narrow-band artificial light sources, including LEDs and fluorescent tubes. The maximum efficiency of the interior light sources was determined by employing the Shockley-Queisser model for calculation. The highest efficiency obtained for fluorescent tubes, phosphorous white LEDs, sodium discharge lamps, and RGB white light is 67%, 45.7%, 47.70%, and 58.40%, respectively. The preferred bandgap values for fluorescent and sodium discharge lamps are 1.95 eV and 2.10 eV, respectively. The photovoltaic device demonstrates high performance in AM 1.5G conditions and may not exhibit optimal functionality in indoor lighting conditions. The spectrum emission of the two artificial illumination sources was significantly limited in the range above 620 nm, resulting in photon counts that exceeded the maximum power conversion efficiency (PCE) achievable by silicon (Si) photovoltaics under natural sunlight irradiation. To enhance the efficiency of solar cells, it is imperative to develop device designs tailored to the specific features of solar cells when exposed to indoor lighting sources, which are predominantly utilised in indoor environments. To efficiently capture energy from a particular light source, it is necessary to have an absorber layer that possesses semiconductor characteristics that are closely aligned. LEDs are anticipated to become the dominant lighting technology owing to their exceptional color range, great luminosity, extended operational longevity, and superior energy economy. The output power of a PV device depends upon the intensity and spectrum of the indoor light source, size and orientation of the photovoltaic (PV) device, distance from the light source, and transparency. Both artificial lighting sources and diffused sunlight that enters through windows or facades can serve as potential light sources within a building (Venkateswararao et al., 2020). The selection of the most suitable photovoltaic (PV) material should depend upon the prevailing lighting conditions within the building, with the aim of maximising power generation.
3 The utilisation of metal-organic framework (MOF) materials in solar devices
Metal-organic frameworks (MOFs) are coordination compounds characterised by polynuclear metal nodes and organic linkers, forming a well-defined crystalline open framework structure. There has been a growing interest in MOFs for energy-related applications and catalysis in recent years. M. Wei et al. (Abdelhamid et al., 2019) studied the application of MOFs as photoanodes in dye-sensitised solar cells (DSSCs). In this study, a thin coating of a zinc-based zeolitic imidazolate framework, often referred to as ZIF-8, was deposited onto the surface of a titanium dioxide (TiO2), which acted as a photo-anode. This ZIF-8 layer was intended to serve as a blocking layer. Using a ZIF-8 layer improved cell efficiency from 5.11% to 5.34%. A flexible Pt-free dye-sensitised solar cell (DSSC) with enhanced efficiency was developed by Hsu (Hou et al., 2017) with a novel design utilising cobalt sulfide (CoS) nanoparticles as the counter electrode with MOF-ZIF-67 thin layer. The utilisation of this design for the counter electrode resulted in a solar-to-electricity conversion efficiency of 8.1%.
Metal-organic frameworks that incorporate photosensitising organic linkers have the potential to serve as effective sensitisers for the photoanodes in DSSCs. In a study conducted by Garcia et al., in 2011, it was found that a thin film of MOF consisting of Al2 (bdc)3 (where bdc represents p-benzenedicarboxylate) has the potential to serve as the photosensitising material in all-solid-state DSSCs. However, it is essential to note that the reported photocurrent in this study remains at the microampere per square centimeter level.
In another interesting study, an insulating variant of ZIF-8 onto the mesoporous TiO2 (mp-TiO2) layer. The inclusion of ZIF-8 resulted in surface roughening of the mp-TiO2 layer, thereby enhancing the crystallinity of the subsequently grown perovskite layer. Notably, the formation of perovskite crystals is governed by the interplay between primary nucleation and subsequent development, revealing a PCE % of 17.1 (Kaur et al., 2016; Chueh et al., 2019). In conclusion, MOFs’ solution-processability and microporous structure have garnered growing scientific interest in their potential uses in flexible photovoltaic solar cells. Furthermore, because of their chemical and thermal stability, MOFs have demonstrated the ability to enhance the durability of the devices.
4 Challenges and conclusion
However, using photon energy in wearables presents several challenges that must be overcome to make the technology more practical and efficient. One of the main challenges is the limited surface area of photovoltaic cells in wearable devices, which can limit the amount of light that can be absorbed and converted into electrical energy. Flexibility, which enables conformal contact with the human body without significant interference, is another factor to be considered while developing a photovoltaic powering unit. One of the significant challenges is low light intensity, which may result in insufficient output from PV cells when it comes to photovoltaic conversion of ambient light for powering wearable sensors. The fluctuation and interference from other light sources can result in constantly varied output from PV cells.
Researchers have developed flexible photovoltaic cells, which can be integrated into clothing and other wearable materials and can also increase the surface area of the photovoltaic cells. Noteworthy research by Freitag et al. has enhanced the performance of PVs in indoor conditions by co-sensitisation approach and achieved a maximum power density of 88.5 μW/cm2 under 1000-lux LED illumination (Freitag et al., 2017). The above work results in a maximum external quantum efficiency of 90% in the 400–650 nm spectral wavelength region. Other work by Cao (Cao et al., 2018) results in PCE of 31% under the FL lamp. This work includes a DSVP design in which dye-sensitised TiO2 photoanode openly contacted the poly (3,4ethylenedioxythiophene) (PEDOT). These studies focus on the present issues faced by photovoltaic technology in the context of wearable applications.
Author contributions
DN: Conceptualization, Data curation, Formal Analysis, Funding acquisition, Methodology, Project administration, Resources, Supervision, Validation, Visualization, Writing–original draft, Writing–review and editing. JJ: Data curation, Writing–review and editing. BV: Formal Analysis, Writing–review and editing. MP: Supervision, Writing–review and editing, Formal Analysis, Methodology. JM: Formal Analysis, Supervision, Writing–review and editing, Project administration. PK: Conceptualization, Data curation, Formal Analysis, Funding acquisition, Methodology, Project administration, Supervision, Writing–original draft, Writing–review and editing.
Funding
This work supported by the ITR Fellowship (5/3/8/40/ITR-F/2022-ITR) funded by Indian Council of Medical Research, Research Project (SCP/2022/0006264) funded by Science and Engineering Board, Govt. of India and Manipal Academy of Higher Education, Manipal.
Conflict of interest
JJ was employed by Sunlux Technovations Pvt Ltd.
The remaining authors declare that the research was conducted in the absence of any commercial or financial relationships that could be construed as a potential conflict of interest.
Publisher’s note
All claims expressed in this article are solely those of the authors and do not necessarily represent those of their affiliated organizations, or those of the publisher, the editors and the reviewers. Any product that may be evaluated in this article, or claim that may be made by its manufacturer, is not guaranteed or endorsed by the publisher.
References
Abdelhamid, H. N., El-Zohry, A. M., Cong, J., Thersleff, T., Karlsson, M., Kloo, L., et al. (2019). Towards implementing hierarchical porous zeolitic imidazolate frameworks in dye-sensitized solar cells. R. Soc. open Sci. 6 (7), 190723. doi:10.1098/rsos.190723
Abshirini, M., Charara, M., Marashizadeh, P., Saha, M. C., Altan, M. C., and Liu, Y. (2019). Functional nanocomposites for 3D printing of stretchable and wearable sensors. Appl. Nanosci. 9, 2071–2083. doi:10.1007/s13204-019-01032-2
Akin, S., Akman, E., and Sonmezoglu, S. (2020). FAPbI3-based perovskite solar cells employing hexyl-based ionic liquid with an efficiency over 20% and excellent long-term stability. Adv. Funct. Mater. 30 (28), 2002964. doi:10.1002/adfm.202002964
Al-Ashouri, A., Köhnen, E., Li, B., Magomedov, A., Hempel, H., Caprioglio, P., et al. (2020). Monolithic perovskite/silicon tandem solar cell with> 29% efficiency by enhanced hole extraction. Science 370 (6522), 1300–1309. doi:10.1126/science.abd4016
Alnoman, R. B., Nabil, E., Parveen, S., Hagar, M., and Zakaria, M. (2022). UV-selective organic absorbers for the cosensitization of greenhouse-integrated dye-sensitized solar cells: synthesis and computational study. RSC Adv. 12 (18), 11420–11435. doi:10.1039/d2ra01099b
á Nozariasbmarz, A., á Collins, H., á Dsouza, K., á Polash, M. H., á Hosseini, M., á Hyland, M., et al. (2020). Review of wearable thermoelectric energy harvesting: from body temperature to electronic systems. Appl. Energy 258, 114069. doi:10.1016/j.apenergy.2019.114069
Aroutiounian, V. M., Petrosyan, S., Khachatryan, A., and Touryan, K. J. (2001). Quantum dot solar cells. Sol. Switch. Mater. 4458, 2268–2271. doi:10.1063/1.1339210
Asemani, M., Abdollahei, F., and Jabbari, F. (2019). “Understanding IoT platforms: towards a comprehensive definition and main characteristic description,” in 2019 5th international conference on web research (ICWR) (IEEE), 172–177.
Aslam, A., Mehmood, U., Arshad, M. H., Ishfaq, A., Zaheer, J., Khan, A. U. H., et al. (2020). Dye-sensitized solar cells (DSSCs) as a potential photovoltaic technology for the self-powered internet of things (IoTs) applications. Sol. Energy 207, 874–892. doi:10.1016/j.solener.2020.07.029
Baca, A. J., Yu, K. J., Xiao, J., Wang, S., Yoon, J., Ryu, J. H., et al. (2010). Compact monocrystalline silicon solar modules with high voltage outputs and mechanically flexible designs. Energy and Environ. Sci. 3 (2), 208–211. doi:10.1039/b920862c
Baiju, A., and Yarema, M. (2022). Status and challenges of multi-junction solar cell technology. Front. Energy Res. 10, 971918. doi:10.3389/fenrg.2022.971918
Bari, D. (2014). Characterisation and reliability of dye-sensitised solar cells: temperature, illumination, and bias effects.
Baxter, J., Bian, Z., Chen, G., Danielson, D., Dresselhaus, M. S., Fedorov, A. G., et al. (2009). Nanoscale design to enable the revolution in renewable energy. Energy and Environ. Sci. 2 (6), 559–588. doi:10.1039/b821698c
Britt, J., and Ferekides, C. (1993). Thin-film CdS/CdTe solar cell with 15.8% efficiency. Appl. Phys. Lett. 62 (22), 2851–2852. doi:10.1063/1.109629
Bullock, J., Hettick, M., Geissbühler, J., Ong, A. J., Allen, T., Sutter-Fella, C. M., et al. (2016). Efficient silicon solar cells with dopant-free asymmetric heterocontacts. Nat. Energy 1 (3), 15031–15037. doi:10.1038/nenergy.2015.31
Bush, K. A., Palmstrom, A. F., Yu, Z. J., Boccard, M., Cheacharoen, R., Mailoa, J. P., et al. (2017). 23.6%-efficient monolithic perovskite/silicon tandem solar cells with improved stability. Nat. Energy 2 (4), 17009–17017. doi:10.1038/nenergy.2017.9
Cao, Y., Liu, Y., Zakeeruddin, S. M., Hagfeldt, A., and Grätzel, M. (2018). Direct contact of selective charge extraction layers enables high-efficiency molecular photovoltaics. Joule 2, 1108–1117. doi:10.1016/j.joule.2018.03.017
Capineri, L. (2014). Resistive sensors with smart textiles for wearable technology: from fabrication processes to integration with electronics. Procedia Eng. 87, 724–727. doi:10.1016/j.proeng.2014.11.748
Carey, G. H., Abdelhady, A. L., Ning, Z., Thon, S. M., Bakr, O. M., and Sargent, E. H. (2015). Colloidal quantum dot solar cells. Chem. Rev. 115, 12732–12763. doi:10.1021/acs.chemrev.5b00063
Carron, R., Nishiwaki, S., Feurer, T., Hertwig, R., Avancini, E., Löckinger, J., et al. (2019). Advanced alkali treatments for high-efficiency Cu (In, Ga) Se2 solar cells on flexible substrates. Adv. Energy Mater. 9 (24), 1900408. doi:10.1002/aenm.201900408
Chang, L.-Yi, Lunt, R. R., Brown, P. R., Bulović, V., and Bawendi, M. G. (2013). Low-temperature solution-processed solar cells based on PbS colloidal quantum dot/CdS heterojunctions. Nano Lett. 13 (3), 994–999. doi:10.1021/nl3041417
Chen, C., Zheng, S., and Song, H. (2021). Photon management to reduce energy loss in perovskite solar cells. Chem. Soc. Rev. 50 (12), 7250–7329. doi:10.1039/d0cs01488e
Chen, C. Y., Jian, Z. H., Huang, S. H., Lee, K. M., Kao, M. H., Shen, C. H., et al. (2017). Performance characterization of dye-sensitized photovoltaics under indoor lighting. J. Phys. Chem. Lett. 8 (8), 1824–1830. doi:10.1021/acs.jpclett.7b00515
Chen, B., Ren, N., Li, Y., Yan, L., Mazumdar, S., Zhao, Y., et al. (2022). Insights into the development of monolithic perovskite/silicon tandem solar cells. Adv. Energy Mater. 12 (4), 2003628. doi:10.1002/aenm.202003628
Chen, W., Dols, S., Oetomo, S. B., and Feijs, L. (2010). “Monitoring body temperature of newborn infants at neonatal intensive care units using wearable sensors,” in Proceedings of the fifth international conference on body area networks, 188–194.
Chen, Z., Turedi, B., Alsalloum, A. Y., Yang, C., Zheng, X., Gereige, I., et al. (2019). Single-crystal MAPbI3 perovskite solar cells exceeding 21% power conversion efficiency. ACS Energy Lett. 4 (6), 1258–1259. doi:10.1021/acsenergylett.9b00847
Chong, Y. W., Ismail, W., Ko, K., and Lee, C. Y. (2019). Energy harvesting for wearable devices: a review. IEEE Sensors J. 19 (20), 9047–9062. doi:10.1109/jsen.2019.2925638
Chuang, C. H. M., Maurano, A., Brandt, R. E., Hwang, G. W., Jean, J., Buonassisi, T., et al. (2015). Open-circuit voltage deficit, radiative sub-bandgap states, and prospects in quantum dot solar cells. Nano Lett. 15 (5), 3286–3294. doi:10.1021/acs.nanolett.5b00513
Chueh, C. C., Chen, C. I., Su, Y. A., Konnerth, H., Gu, Y. J., Kung, C. W., et al. (2019). Harnessing MOF materials in photovoltaic devices: recent advances, challenges, and perspectives. J. Mater. Chem. A 7 (29), 17079–17095. doi:10.1039/c9ta03595h
Das, S., Alam, I., Raiguru, J., Subramanyam, B. V. R. S., and Mahanandia, P. (2019). A facile method to synthesize CZTS quantum dots for solar cell applications. Phys. E Low-Dimensional Syst. Nanostructures 105, 19–24. doi:10.1016/j.physe.2018.08.020
Ding, Y., Wang, Z., Duan, X., and Liu, R. (2023). Flexible photo-charging power sources for wearable electronics. Materials Today Energy.101276
Du, Y., Cai, H., Bao, X., Xing, Z., Wu, Y., Xu, J., et al. (2018). Flexible perovskite solar cells onto plastic substrate exceeding 13% efficiency owing to the optimization of CH3NH3PbI3–xClx film via H2O additive. ACS Sustain. Chem. Eng. 6 (1), 1083–1090. doi:10.1021/acssuschemeng.7b03382
Efros, A. L., and Brus, L. E. (2021). Nanocrystal quantum dots: from discovery to modern development. ACS Nano 15 (4), 6192–6210. doi:10.1021/acsnano.1c01399
Fan, X., Zhang, M., Wang, X., Yang, F., and Meng, X. (2013). Recent progress in organic–inorganic hybrid solar cells. J. Mater. Chem. A 1 (31), 8694–8709. doi:10.1039/c3ta11200d
Freitag, M., Teuscher, J., Saygili, Y., Zhang, X., Giordano, F., Liska, P., et al. (2017). Dye-sensitized solar cells for efficient power generation under ambient lighting. Nat. Photonics 11, 372–378. doi:10.1038/nphoton.2017.60
Freunek, M., Freunek, M., and Reindl, L. M. (2012). Maximum efficiencies of indoor photovoltaic devices. IEEE J. Photovoltaics 3 (1), 59–64. doi:10.1109/jphotov.2012.2225023
Fukuda, K., Yu, K., and Someya, T. (2020). The future of flexible organic solar cells. Adv. Energy Mater. 10 (25), 2000765. doi:10.1002/aenm.202000765
Gan, J., Yu, M., Hoye, R. L., Musselman, K. P., Li, Y., Liu, X., et al. (2021). Defects, photophysics and passivation in Pb-based colloidal quantum dot photovoltaics. Mater. Today Nano 13, 100101. doi:10.1016/j.mtnano.2020.100101
Gao, J. B., Luther, J. M., Semonin, O. E., Ellingson, R. J., Nozik, A. J., and Beard, M. C. (2011). Quantum dot size dependent J−V characteristics in heterojunction ZnO/PbS quantum dot solar cells. Nano Lett. 11, 1002–1008. doi:10.1021/nl103814g
Gharibzadeh, S., Hossain, I. M., Fassl, P., Nejand, B. A., Abzieher, T., Schultes, M., et al. (2020). 2D/3D heterostructure for semitransparent perovskite solar cells with engineered bandgap enables efficiencies exceeding 25% in four-terminal tandems with silicon and CIGS. Adv. Funct. Mater. 30 (19), 1909919. doi:10.1002/adfm.201909919
Giménez, S., Mora-Seró, I., Macor, L., Guijarro, N., Lana-Villarreal, T., Gómez, R., et al. (2009). Improving the performance of colloidal quantum-dot-sensitized solar cells. Nanotechnology 20 (29), 295204. doi:10.1088/0957-4484/20/29/295204
Hamid, R., and Yuce, M. R. (2017). A wearable energy harvester unit using piezoelectric–electromagnetic hybrid technique. Sensors Actuators A Phys. 257, 198–207. doi:10.1016/j.sna.2017.02.026
Han, M., Karatum, O., and Nizamoglu, S. (2022). Optoelectronic neural interfaces based on quantum dots. ACS Appl. Mater. Interfaces 14 (18), 20468–20490. doi:10.1021/acsami.1c25009
Hou, X., Pan, L., Huang, S., Wei, O. Y., and Chen, X. (2017). Enhanced efficiency and stability of perovskite solar cells using porous hierarchical TiO2 nanostructures of scattered distribution as scaffold. Electrochimica Acta 236, 351–358. doi:10.1016/j.electacta.2017.03.192
Hou, X., Wang, Y., Lee, H. K. H., Datt, R., Miano, N. U., Yan, D., et al. (2020). Indoor application of emerging photovoltaics—progress, challenges and perspectives. J. Mater. Chem. A 8 (41), 21503–21525. doi:10.1039/d0ta06950g
Hu, L., Geng, X., Singh, S., Shi, J., Hu, Y., Li, S., et al. (2019a). Synergistic effect of electron transport layer and colloidal quantum dot solid enable PbSe quantum dot solar cell achieving over 10% efficiency. Nano Energy 64, 103922. doi:10.1016/j.nanoen.2019.103922
Hu, L., Zhao, Q., Huang, S., Zheng, J., Guan, X., Patterson, R., et al. (2021). Flexible and efficient perovskite quantum dot solar cells via hybrid interfacial architecture. Nat. Commun. 12 (1), 466. doi:10.1038/s41467-020-20749-1
Hu, Y., Song, L., Chen, Y., and Huang, W. (2019b). Two-terminal perovskites tandem solar cells: recent advances and perspectives. Sol. RRL 3 (7), 1900080. doi:10.1002/solr.201900080
Huang, J., Ren, Z., Zhang, Y., Liu, K., Zhang, H., Tang, H., et al. (2021). Stretchable ITO-free organic solar cells with intrinsic anti-reflection substrate for high-efficiency outdoor and indoor energy harvesting. Adv. Funct. Mater. 31 (16), 2010172. doi:10.1002/adfm.202010172
Huang, J., Zhou, J., Jungstedt, E., Samanta, A., Linnros, J., Berglund, L. A., et al. (2022). Large-area transparent "quantum dot glass" for building-integrated photovoltaics. ACS Photonics 9 (7), 2499–2509. doi:10.1021/acsphotonics.2c00633
Huang, J. S., Chou, C. Y., and Lin, C. F. (2010). Enhancing performance of organic–inorganic hybrid solar cells using a fullerene interlayer from all-solution processing. Sol. Energy Mater. Sol. Cells 94 (2), 182–186. doi:10.1016/j.solmat.2009.08.019
Huang, X., Huang, S., Zhang, Q., Guo, X., Li, D., Luo, Y., et al. (2011). A flexible photoelectrode for CdS/CdSe quantum dot-sensitized solar cells (QDSSCs). Chem. Commun. 47 (9), 2664–2666. doi:10.1039/c0cc04419a
Huld, T., Friesen, G., Skoczek, A., Kenny, R. P., Sample, T., Field, M., et al. (2011). A power-rating model for crystalline silicon PV modules. Sol. Energy Mater. Sol. Cells 95 (12), 3359–3369. doi:10.1016/j.solmat.2011.07.026
Hwang, I., Jeong, Y., Shiratori, Y., Park, J., Miyajima, S., Yoon, I., et al. (2020). Effective photon management of non-surface-textured flexible thin crystalline silicon solar cells. Cell. Rep. Phys. Sci. 1 (11), 100242. doi:10.1016/j.xcrp.2020.100242
Ichikawa, Y., Yoshida, T., Hama, T., Sakai, H., and Harashima, K. (2001). Production technology for amorphous silicon-based flexible solar cells. Sol. Energy Mater. Sol. Cells 66 (1-4), 107–115. doi:10.1016/s0927-0248(00)00163-x
Ikeri, H. I., Onyia, A. I., and Kalu, F. N. (2021). Hot carrier exploitation strategies and model for efficient solar cell applications. Chalcogenide Lett. 18 (11), 745–757. doi:10.15251/cl.2021.1811.745
Im, J. H., Jang, I. H., Pellet, N., Grätzel, M., and Park, N. G. (2014). Growth of CH3NH3PbI3 cuboids with controlled size for high-efficiency perovskite solar cells. Nat. Nanotechnol. 9 (11), 927–932. doi:10.1038/nnano.2014.181
Irfan, M., Ünlü, F., Lê, K., Fischer, T., Ullah, H., and Mathur, S. (2022). Electrospun networks of ZnO-SnO2 composite nanowires as electron transport materials for perovskite solar cells. J. Nanomater. 2022, 1–13. doi:10.1155/2022/6043406
Ito, S., Murakami, T. N., Comte, P., Liska, P., Grätzel, C., Nazeeruddin, M. K., et al. (2008). Fabrication of thin film dye sensitized solar cells with solar to electric power conversion efficiency over 10%. Thin solid films 516 (14), 4613–4619. doi:10.1016/j.tsf.2007.05.090
Jegadeeswari, M. G., and Rekha, M. K. (2020). Design and implementation of wearable photovoltaic cell. Gt. Any Res. Work is Only through Its Wider Appl. 58. doi:10.47059/CIIR/BP20002/06
Jia, S., Gao, H., Xue, Z., and Meng, X. (2022). Recent advances in multifunctional wearable sensors and systems: design, fabrication, and applications. Biosensors 12 (11), 1057. doi:10.3390/bios12111057
Jokic, P., and Magno, M. (2017). “Powering smart wearable systems with flexible solar energy harvesting,” in 2017 IEEE International symposium on circuits and systems (ISCAS) (IEEE), 1–4.
Jung, H. S., Han, G. S., Park, N. G., and Ko, M. J. (2019). Flexible perovskite solar cells. Joule 3 (8), 1850–1880. doi:10.1016/j.joule.2019.07.023
Kabir, E., Kumar, P., Kumar, S., Adelodun, A. A., and Kim, K. H. (2018). Solar energy: potential and future prospects. Renew. Sustain. Energy Rev. 82, 894–900. doi:10.1016/j.rser.2017.09.094
Kamaraki, C., Klug, M. T., Green, T., Miranda Perez, L., and Case, C. (2021). Perovskite/silicon tandem photovoltaics: technological disruption without business disruption. Appl. Phys. Lett. 119 (7). doi:10.1063/5.0054086
Kamat, P. V. (2008). Quantum dot solar cells. Semiconductor nanocrystals as light harvesters. J. Phys. Chem. C 112 (48), 18737–18753. doi:10.1021/jp806791s
Kamat, P. V. (2013). Quantum dot solar cells. The next big thing in photovoltaics. J. Phys. Chem. Lett. 4 (6), 908–918. doi:10.1021/jz400052e
Kartsch, V., Benatti, S., Mancini, M., Magno, M., and Benini, L. (2018). “Smart wearable wristband for EMG based gesture recognition powered by solar energy harvester,” in 2018 IEEE international symposium on circuits and systems (ISCAS) (IEEE), 1–5.
Kaur, R., Kim, K. H., Paul, A. K., and Deep, A. (2016). Recent advances in the photovoltaic applications of coordination polymers and metal organic frameworks. J. Mater. Chem. A 4 (11), 3991–4002. doi:10.1039/c5ta09668e
Khalid, S., Raouf, I., Khan, A., Kim, N., and Kim, H. S. (2019). A review of human-powered energy harvesting for smart electronics: recent progress and challenges. Int. J. Precis. Eng. Manufacturing-Green Technol. 6, 821–851. doi:10.1007/s40684-019-00144-y
Khan, U., Zhinong, Y., Khan, A. A., Zulfiqar, A., and khan, Q. U. (2019). Organic–inorganic hybrid perovskites based on methylamine lead halide solar cell. Sol. Energy 189, 421–425. doi:10.1016/j.solener.2019.06.061
Kim, B. J., Kim, D. H., Lee, Y. Y., Shin, H. W., Han, G. S., Hong, J. S., et al. (2015). Highly efficient and bending durable perovskite solar cells: toward a wearable power source. Energy and Environ. Sci. 8 (3), 916–921. doi:10.1039/c4ee02441a
Kim, J., Song, S., Kim, Y. H., and Park, S. K. (2021b). Recent progress of quantum dot-based photonic devices and systems: a comprehensive review of materials, devices, and applications. Small Struct. 2 (3), 2000024. doi:10.1002/sstr.202000024
Kim, J. H., Kim, Y. C., Kim, J. Y., Lee, H. S., Han, Y. W., Lee, H. W., et al. (2023). Outdoor organic solar cells with effective photon-harvesting by introducing light-amplification layer with eco-friendly QDs. Small Structures.2300057
Kim, K. A., Bagci, F. S., and Dorsey, K. L. (2022). Design considerations for photovoltaic energy harvesting in wearable devices. Sci. Rep. 12 (1), 18143. doi:10.1038/s41598-022-22232-x
Kim, S., Van Quy, H., and Bark, C. W. (2021a). Photovoltaic technologies for flexible solar cells: beyond silicon. Mater. Today Energy 19, 100583. doi:10.1016/j.mtener.2020.100583
Kojima, A., Teshima, K., Shirai, Y., and Miyasaka, T. (2009). Organometal halide perovskites as visible-light sensitizers for photovoltaic cells. J. Am. Chem. Soc. 131 (17), 6050–6051. doi:10.1021/ja809598r
Kokkonen, M., Talebi, P., Zhou, J., Asgari, S., Soomro, S. A., Elsehrawy, F., et al. (2021). Advanced research trends in dye-sensitized solar cells. J. Mater. Chem. A 9 (17), 10527–10545. doi:10.1039/d1ta00690h
Koteshwar, D., Prasanthkumar, S., Singh, S. P., Chowdhury, T. H., Bedja, I., Islam, A., et al. (2022). Effects of methoxy group(s) on D-π-A porphyrin based DSSCs: efficiency enhanced by co-sensitization. Mater. Chem. Front. 6, 580–592. doi:10.1039/d1qm01450a
Kramer, I. J., and Sargent, E. H. (2014). The architecture of colloidal quantum dot solar cells: materials to devices. Chem. Rev. 114 (1), 863–882. doi:10.1021/cr400299t
Kumar, G., and Chen, F. C. (2023). A review on recent progress in organic photovoltaic devices for indoor applications. J. Phys. D Appl. Phys. 56, 353001. doi:10.1088/1361-6463/acd2e5
Lee, G., Kim, M. C., Choi, Y. W., Ahn, N., Jang, J., Yoon, J., et al. (2019). Ultra-flexible perovskite solar cells with crumpling durability: toward a wearable power source. Energy and Environ. Sci. 12 (10), 3182–3191. doi:10.1039/c9ee01944h
Li, S., Li, X., Yang, J., Jiang, Q., Lai, H., Tan, Y., et al. (2020). Improvement of photovoltaic performance of perovskite solar cells by interface modification with CaTiO3. J. Power Sources 449, 227504. doi:10.1016/j.jpowsour.2019.227504
Li, Y., Sun, Y., and Zhang, Y. (2019). Luminescent solar concentrators performing under different light conditions. Sol. Energy 188, 1248–1255. doi:10.1016/j.solener.2019.07.035
Lin, Q., Huang, H., Jing, Y., Fu, H., Chang, P., Li, D., et al. (2014). Flexible photovoltaic technologies. J. Mater. Chem. C 2 (7), 1233–1247. doi:10.1039/c3tc32197e
Liu, C., Lu, Y., Shen, R., Dai, Y., Yu, X., Liu, K., et al. (2022). Dynamics and physical process of hot carriers in optoelectronic devices. Nano Energy 95, 106977. doi:10.1016/j.nanoen.2022.106977
Liu, D., and Kelly, T. L. (2014). Perovskite solar cells with a planar heterojunction structure prepared using room-temperature solution processing techniques. Nat. photonics 8 (2), 133–138. doi:10.1038/nphoton.2013.342
Liu, D., Liu, J., Liu, J., Liu, S., Wang, C., Ge, Z., et al. (2020). The photovoltaic performance of CdS/CdSe quantum dots co-sensitized solar cells based on zinc titanium mixed metal oxides. Phys. E Low-dimensional Syst. nanostructures 115, 113669. doi:10.1016/j.physe.2019.113669
Lu, H., Carroll, G. M., Neale, N. R., and Beard, M. C. (2019). Infrared quantum dots: progress, challenges, and opportunities. ACS Nano 13 (2), 939–953. doi:10.1021/acsnano.8b09815
Lu, H., Huang, Z., Martinez, M. S., Johnson, J. C., Luther, J. M., and Beard, M. C. (2020a). Transforming energy using quantum dots. Energy and Environ. Sci. 13 (5), 1347–1376. doi:10.1039/c9ee03930a
Lu, H., Huang, Z., Martinez, M. S., Johnson, J. C., Luther, J. M., and Beard, M. C. (2020b). Transforming energy using quantum dots. Energy and Environ. Sci. 13 (5), 1347–1376. doi:10.1039/c9ee03930a
Lübke, D., Hartnagel, P., Angona, J., and Kirchartz, T. (2021). Comparing and quantifying indoor performance of organic solar cells. Adv. Energy Mater. 11 (34), 2101474. doi:10.1002/aenm.202101474
Luo, P., Zhuge, F., Wang, F., Lian, L., Liu, K., Zhang, J., et al. (2019). PbSe quantum dots sensitized high-mobility Bi2O2Se nanosheets for high-performance and broadband photodetection beyond 2 μm. ACS Nano 13 (8), 9028–9037. doi:10.1021/acsnano.9b03124
Mahajan, C., Sharma, A., and Rath, A. K. (2020). Solution-phase hybrid passivation for efficient infrared-band gap quantum dot solar cells. ACS Appl. Mater. Interfaces 12 (44), 49840–49848. doi:10.1021/acsami.0c15703
Mathews, I., Kantareddy, S. N., Buonassisi, T., and Peters, I. M. (2019). Technology and market perspective for indoor photovoltaic cells. Joule 3 (6), 1415–1426. doi:10.1016/j.joule.2019.03.026
Meddeb, H., Götz-Köhler, M., Neugebohrn, N., Banik, U., Osterthun, N., Sergeev, O., et al. (2022). Tunable photovoltaics: adapting solar cell technologies to versatile applications. Adv. Energy Mater. 12 (28), 2200713. doi:10.1002/aenm.202200713
Min, J., Demchyshyn, S., Sempionatto, J. R., Song, Y., Hailegnaw, B., Xu, C., et al. (2023). An autonomous wearable biosensor powered by a perovskite solar cell. Nat. Electron. 6, 630–641. doi:10.1038/s41928-023-00996-y
Mishra, S., Ghosh, S., Boro, B., Kumar, D., Porwal, S., Paul, M., et al. (2022). Solution-processed next generation thin film solar cells for indoor light applications. Energy Adv. 1, 761–792. doi:10.1039/d2ya00075j
Mohsen, S., Zekry, A., Youssef, K., and Abouelatta, M. (2021). A self-powered wearable wireless sensor system powered by a hybrid energy harvester for healthcare applications. Wirel. Personal. Commun. 116 (4), 3143–3164. doi:10.1007/s11277-020-07840-y
Muhammad, B. T., Kar, S., Stephen, M., and Leong, W. L. (2022). Halide perovskite-based indoor photovoltaics: recent development and challenges. Mater. Today Energy 23, 100907. doi:10.1016/j.mtener.2021.100907
Mukhopadhyay, S. C., Suryadevara, N. K., and Nag, A. (2022). Wearable sensors for healthcare: fabrication to application. Sensors 22 (14), 5137. doi:10.3390/s22145137
Mulligan, W. P., Rose, D. H., Cudzinovic, M. J., De Ceuster, D. M., McIntosh, K. R., Smith, D. D., et al. (2004). Manufacture of solar cells with 21% efficiency. Proc. 19th EPVSEC, 387.
Nguyen, K. D., Chen, I. M., Luo, Z., Yeo, S. H., and Duh, H. B. L. (2010). A wearable sensing system for tracking and monitoring of functional arm movement. IEEE/ASME Trans. mechatronics 16 (2), 213–220. doi:10.1109/tmech.2009.2039222
Nideep, T. K., Ramya, M., and Kailasnath, M. (2020). An investigation on the photovoltaic performance of quantum dot solar cells sensitized by CdTe, CdSe and CdS having comparable size. Superlattices Microstruct. 141, 106477. doi:10.1016/j.spmi.2020.106477
Nishimura, N., Allardice, J. R., Xiao, J., Gu, Q., Gray, V., and Rao, A. (2019). Photon upconversion utilizing energy beyond the band gap of crystalline silicon with a hybrid TES-ADT/PbS quantum dots system. Chem. Sci. 10 (18), 4750–4760. doi:10.1039/c9sc00821g
Nozariasbmarz, A., Collins, H., Dsouza, K., Polash, M. H., Hosseini, M., Hyland, M., et al. (2020). Review of wearable thermoelectric energy harvesting: from body temperature to electronic systems. Appl. Energy 258, 114069. doi:10.1016/j.apenergy.2019.114069
Páez-Montoro, A., García-Valderas, M., Olías-Ruíz, E., and López-Ongil, C. (2022). Solar energy harvesting to improve capabilities of wearable devices. Sensors 22 (10), 3950. doi:10.3390/s22103950
Park, S., McCartney, M. R., Smith, D. J., Jeon, J., Kim, Y., and Lee, S. J. (2022). Inverted metamorphic InGaAsP/InGaAs dual-junction solar cells towards full solar spectrum harvesting. J. Mater. Chem. A 10 (24), 13106–13113. doi:10.1039/d2ta01603f
Raffaelle, R. P., Castro, S. L., Hepp, A. F., and Bailey, S. G. (2002). Quantum dot solar cells. Prog. Photovoltaics Res. Appl. 10 (6), 433–439. doi:10.1002/pip.452
Ramiro, I., Villa, J., Lam, P., Hatch, S., Wu, J., Lopez, E., et al. (2015). Wide-bandgap InAs/InGaP quantum-dot intermediate band solar cells. IEEE J. photovoltaics 5 (3), 840–845. doi:10.1109/jphotov.2015.2402439
Riaz, S., and Park, S. J. (2022). O, S-g-C3N4 nanotubes as photovoltaic boosters in quantum dot-sensitized all-weather solar cells: a synergistic approach for enhanced power conversion efficiency in dark-light conditions. Mater. Today Chem. 26, 101125. doi:10.1016/j.mtchem.2022.101125
Saadatnejad, S., Oveisi, M., and Hashemi, M. (2019). LSTM-based ECG classification for continuous monitoring on personal wearable devices. IEEE J. Biomed. health Inf. 24 (2), 515–523. doi:10.1109/jbhi.2019.2911367
Sahli, F., Werner, J., Kamino, B. A., Bräuninger, M., Monnard, R., Paviet-Salomon, B., et al. (2018). Fully textured monolithic perovskite/silicon tandem solar cells with 25.2% power conversion efficiency. Nat. Mater. 17 (9), 820–826. doi:10.1038/s41563-018-0115-4
Sanehira, E. M., Marshall, A. R., Christians, J. A., Harvey, S. P., Ciesielski, P. N., Wheeler, L. M., et al. (2017). Enhanced mobility CsPbI3 quantum dot arrays for record-efficiency, high-voltage photovoltaic cells. Sci. adv. 3 (10), eaao4204.
Selopal, G. S., Zhao, H., Wang, Z. M., and Rosei, F. (2020). Core/shell quantum dots solar cells. Adv. Funct. Mater. 30 (13), 1908762. doi:10.1002/adfm.201908762
Semonin, O. E., Luther, J. M., and Beard, M. C. (2012). Quantum dots for next-generation photovoltaics. Mater. Today 15 (11), 508–515. doi:10.1016/s1369-7021(12)70220-1
Seneviratne, S., Hu, Y., Nguyen, T., Lan, G., Khalifa, S., Thilakarathna, K., et al. (2017). A survey of wearable devices and challenges. IEEE Commun. Surv. Tutorials 19 (4), 2573–2620. doi:10.1109/comst.2017.2731979
Shankar, K., Basham, J. I., Allam, N. K., Varghese, O. K., Mor, G. K., Feng, X., et al. (2009). Recent advances in the use of TiO2 nanotube and nanowire arrays for oxidative photoelectrochemistry. J. Phys. Chem. C 113 (16), 6327–6359. doi:10.1021/jp809385x
Sharma, M., Pudasaini, P. R., Ruiz-Zepeda, F., Elam, D., and Ayon, A. A. (2014). Ultrathin, flexible organic–inorganic hybrid solar cells based on silicon nanowires and PEDOT: PSS. ACS Appl. Mater. interfaces 6 (6), 4356–4363. doi:10.1021/am500063w
Sharma, V., and Jha, P. K. (2019). Enhancement in power conversion efficiency of edge-functionalized graphene quantum dot through adatoms for solar cell applications. Sol. Energy Mater. Sol. Cells 200, 109908. doi:10.1016/j.solmat.2019.04.030
Singh, G. K. (2013). Solar power generation by PV (photovoltaic) technology: a review. Energy 53, 1–13. doi:10.1016/j.energy.2013.02.057
Sun, P., Xing, Z., Li, Z., and Zhou, W. (2023). Recent advances in quantum dots photocatalysts. Chem. Eng. J. 458, 141399. doi:10.1016/j.cej.2023.141399
S. S. Sun, and N. S. Sariciftci (Editors) (2017). Organic photovoltaics: mechanisms, materials, and devices (CRC Press).
Venkateswararao, A., Ho, J. K., So, S. K., Liu, S. W., and Wong, K. T. (2020). Device characteristics and material developments of indoor photovoltaic devices. Mater. Sci. Eng. R Rep. 139, 100517. doi:10.1016/j.mser.2019.100517
Wali, Q., Elumalai, N. K., Iqbal, Y., Uddin, A., and Jose, R. (2018). Tandem perovskite solar cells. Renew. Sustain. Energy Rev. 84, 89–110. doi:10.1016/j.rser.2018.01.005
Wang, F., Cao, Y., Chen, C., Chen, Q., Wu, X., Li, X., et al. (2018). Materials toward the upscaling of perovskite solar cells: progress, challenges, and strategies. Adv. Funct. Mater. 28 (52), 1803753. doi:10.1002/adfm.201803753
Wang, X. H., Koleilat, G. I., Tang, J., Liu, H., Kramer, I. J., Debnath, R., et al. (2011). Tandem colloidal quantum dot solar cells employing a graded recombination layer. Nat. Photonics 5, 480–484. doi:10.1038/nphoton.2011.123
Weerasinghe, H. C., Huang, F., and Cheng, Y. B. (2013). Fabrication of flexible dye sensitized solar cells on plastic substrates. Nano Energy 2 (2), 174–189. doi:10.1016/j.nanoen.2012.10.004
Wright, M., and Uddin, A. (2012). Organic—inorganic hybrid solar cells: a comparative review. Sol. energy Mater. Sol. cells 107, 87–111. doi:10.1016/j.solmat.2012.07.006
Wu, C., Wang, K., Batmunkh, M., Bati, A. S., Yang, D., Jiang, Y., et al. (2020). Multifunctional nanostructured materials for next generation photovoltaics. Nano Energy 70, 104480. doi:10.1016/j.nanoen.2020.104480
Yang, J., Hahm, D., Kim, K., Rhee, S., Lee, M., Kim, S., et al. (2020). High-resolution patterning of colloidal quantum dots via non-destructive, light-driven ligand crosslinking. Nat. Commun. 11 (1), 2874. doi:10.1038/s41467-020-16652-4
Yang, P. C., Chan, I. M., Lin, C. H., and Chang, Y. L. (2011). “Thin film solar cells for indoor use,” in 2011 37th IEEE photovoltaic specialists conference (IEEE), 000696–000698.
Yang, Z., Deng, J., Sun, X., Li, H., and Peng, H. (2014). Stretchable, wearable dye-sensitized solar cells. Adv. Mater. 26 (17), 2643–2647. doi:10.1002/adma.201400152
Yang, Z., Fan, J. Z., Proppe, A. H., Arquer, F. P. G. D., Rossouw, D., Voznyy, O., et al. (2017). Mixed-quantum-dot solar cells. Nat. Commun. 8 (1), 1325. doi:10.1038/s41467-017-01362-1
Yin, L., Kim, K. N., Lv, J., Tehrani, F., Lin, M., Lin, Z., et al. (2021). A self-sustainable wearable multi-modular E-textile bioenergy microgrid system. Nat. Commun. 12 (1), 1542. doi:10.1038/s41467-021-21701-7
Yin, L., Kim, K. N., Trifonov, A., Podhajny, T., and Wang, J. (2022). Designing wearable microgrids: towards autonomous sustainable on-body energy management. Energy and Environ. Sci. 15 (1), 82–101. doi:10.1039/d1ee03113a
Yoo, K., Biswas, S., Lee, Y. J., Shin, S. C., Kim, K. J., Shim, J. W., et al. (2020). Standardizing performance measurement of dye-sensitized solar cells for indoor light harvesting. IEEE Access 8, 114752–114760. doi:10.1109/access.2020.3003242
Yue, Q., Liu, W., and Zhu, X. (2020). n-Type molecular photovoltaic materials: design strategies and device applications. J. Am. Chem. Soc. 142 (27), 11613–11628. doi:10.1021/jacs.0c04084
Zampetti, A., Minotto, A., and Cacialli, F. (2019). Near-infrared (NIR) organic light-emitting diodes (OLEDs): challenges and opportunities. Adv. Funct. Mater. 29 (21), 1807623. doi:10.1002/adfm.201905825
Zarrabi, A. H. (2023). Integrated facade simulator: dynamic Tool to Study the Impact of solar Radiation on human facade interaction (doctoral dissertation. The University of. North Carolina at Charlotte).
Zhang, T., Lu, J., Hu, F., and Hao, Q. (2014). “Bluetooth low energy for wearable sensor-based healthcare systems,” in 2014 IEEE healthcare innovation conference (HIC) (IEEE), 251–254.
Zhang, X., Xiong, H., Qi, J., Hou, C., Li, Y., Zhang, Q., et al. (2018). Antisolvent-derived intermediate phases for low-temperature flexible perovskite solar cells. ACS Appl. Energy Mater. 1 (11), 6477–6486. doi:10.1021/acsaem.8b01413
Zhang, Y., Kirs, A., Ambroz, F., Lin, C. T., Bati, A. S., Parkin, I. P., et al. (2021). Ambient fabrication of organic–inorganic hybrid perovskite solar cells. Small Methods 5 (1), 2000744. doi:10.1002/smtd.202000744
Zhao, C., Zhang, Z., Han, F., Xia, D., Xiao, C., Fang, J., et al. (2021). An organic–inorganic hybrid electrolyte as a cathode interlayer for efficient organic solar cells. Angew. Chem. 133 (15), 8607–8612. doi:10.1002/ange.202100755
Zhao, Q., Wang, S., Kim, Y. H., Mondal, S., Miao, Q., Li, S., et al. (2023). Advantageous properties of halide perovskite quantum dots towards energy-efficient sustainable applications. Green Energy and Environment.
Zhu, J., Chen, H., Zi, Y., Wang, M., and Huang, W. (2022). Size-tunable bismuth quantum dots for self-powered photodetectors under ambient conditions. Nanotechnology 34 (2), 025202. doi:10.1088/1361-6528/ac96f9
Keywords: photovoltaics, wearable devices, clean energy, health monitoring, renewable energy
Citation: Niranjan DB, Jacob J, Vaidehi BR, Peter M, Medikonda J and K. Namboothiri P (2023) Current status and applications of photovoltaic technology in wearable sensors: a review. Front. Nanotechnol. 5:1268931. doi: 10.3389/fnano.2023.1268931
Received: 28 July 2023; Accepted: 23 October 2023;
Published: 09 November 2023.
Edited by:
Adersh Asok, National Institute for Interdisciplinary Science and Technology (CSIR), IndiaReviewed by:
Hani Nasser Abdelhamid, Assiut University, EgyptPreeti Gupta, Leibniz Institute for Solid State and Materials Research Dresden (IFW Dresden), Germany
Copyright © 2023 Niranjan, Jacob, Vaidehi, Peter, Medikonda and K. Namboothiri. This is an open-access article distributed under the terms of the Creative Commons Attribution License (CC BY). The use, distribution or reproduction in other forums is permitted, provided the original author(s) and the copyright owner(s) are credited and that the original publication in this journal is cited, in accordance with accepted academic practice. No use, distribution or reproduction is permitted which does not comply with these terms.
*Correspondence: Pramod K. Namboothiri, cHJhbW9kLmtuQG1hbmlwYWwuZWR1