- Chemical and Petroleum Engineering Department, UAE University, Al Ain, United Arab Emirates
Recent research has increasingly focused on finding low-cost, eco-friendly sources to produce high-quality materials. Since its discovery, graphene has garnered significant attention due to its exceptional properties. However, large-scale production of graphene remains challenging due to the expensive and harmful reagents typically used in its synthesis. Agricultural waste, a rich carbon source, offers a potential solution, with date palm trees generating substantial amounts of waste annually. This review is the first comparative study that systematically explores graphene materials derived from date palm trees, date seeds, and date syrup, emphasising their environmental applications. The paper begins by explaining the basics of graphene and various synthesis approaches from solid waste and different derivatives. It covers the properties of graphene and how various parameters can alter these characteristics. The primary focus is on the synthesis, characterization, and properties of graphene derived from date palm waste. A comparative analysis of graphene derived from date palm versus oil palm is also provided. The environmental applications of graphene produced from date palm waste are discussed in detail. Additionally, the paper outlines the prospects and future opportunities for producing graphene from date palm waste. In summary, graphene materials derived from date palm waste showed superior properties and exceptional adsorption capacities in pollutant removal. Although research in this area is still in its early stages, the pressing need for sustainable graphene production methods is expected to drive significant advancements in this field and this pioneering review can provide a roadmap for researchers.
1 Introduction
The high cost and the lack of sustainable graphene limit its use in applications that require large-scale manufacturing and supplies. Recently, biomass has drawn a lot of interest because it can be converted into useful carbon-based compounds (Idris et al., 2023; Joshi et al., 2023). Biomass is a plentiful and natural source of carbon. A high carbon content ranging between 45 and 55 wt. % is found in a variety of biomass forms, such as municipal solid wastes, plant leaves, grass and agricultural and food residues (Urzúa et al., 2024). Different types of carbonaceous materials can be prepared from different types of biomass such as carbon quantum dots (Hao et al., 2023), graphene quantum dots (GQDs) (Abbas et al., 2023), carbon nanofibers (Gao et al., 2023), carbon nanotubes (Yang et al., 2024) and graphene (Safian et al., 2023).
Depending on the biomass source, biomass materials have different compositions. Nonetheless, the composition is mostly composed of cellulose, hemicellulose, and lignin. The characteristics of the resultant carbonaceous materials based on biomass are influenced by process conditions, including pressure, heating rate, residence time, temperature, and kind of feedstock. Different combinations of process conditions result in different properties, including porosity, surface area, and surface functional groups (Idris et al., 2023). Carbon-based nanomaterials, such as graphene and its derivatives, can be produced from agricultural waste that contains a lot of carbonaceous cellulose (Li L. et al., 2024). Many agricultural wastes have been studied as raw materials for the production of graphene and graphene-based materials such as paddy straw (Chaturvedi et al., 2022), coconut shell and coconut coir (Tamilselvi et al., 2020), wheat straw (Dawood Saleh et al., 2023), corncob (Tinh et al., 2023), sugar beet bagasse (Faghiri and Ghorbani, 2020), sugarcane dry leaves (Thangaraj et al., 2024) and oil palm empty fruit bunch (Mahalingam et al., 2022).
One of the first plants that people ever cultivated is the date palm (Phoenix dactylifera). It is a member of the Palmae (Arecaceae) family and is a tropical and subtropical tree (Saleem et al., 2023). Each date palm tree generates roughly 40 kg of waste from leaves, surface fibres surrounding the trunk and fruit-bearing bunches per year (Galiwango et al., 2017). Therefore, in countries where there is a large number of date palm trees, this waste causes environmental problems. According to Scopus, there is a total of 1,654 research documents in the area of graphene and biomass (between 2009 and 2023), and 225 research documents with keywords of graphene and agricultural waste (between 2013 and 2023). While there are about 174 research documents with keywords of graphene and oil palm (between 2013 and 2023), there are only 11 in the area of graphene and date palm (between 2017 and 2023). Statistics of research documents and trends are shown in Figure 1. These statistics clearly indicate that studies in the area of producing graphene from date palm waste are not well investigated yet.
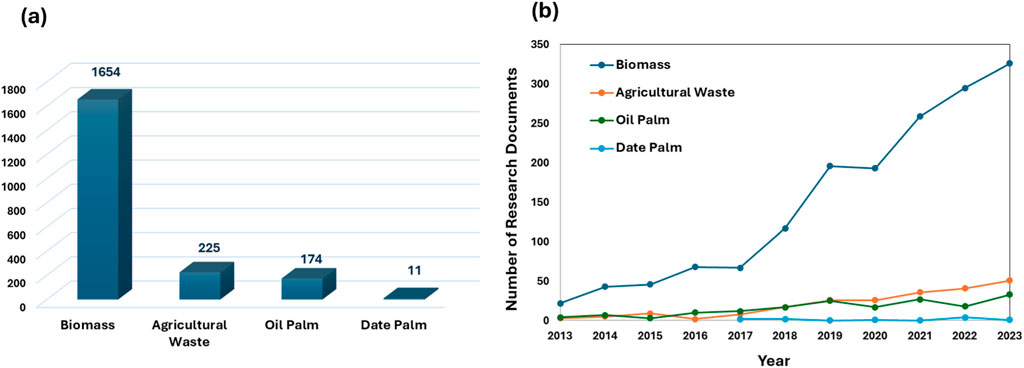
Figure 1. (a) Total number of publications and (b) trend in publications between 2013 and 2023 using the keywords “graphene”, “biomass”, “agricultural waste”, “oil palm” and “date palm”.
Some reviews have been published in the area of biomass-derived graphene and graphene-like materials (Ouyang et al., 2021), using agricultural waste to produce graphene and its derivatives (Li L. et al., 2024), graphene synthesis from lignocellulosic biomass (Ahmad Farid and Andou, 2022) and the synthesis of carbon nanoparticles from materials derived from oil palm byproducts (Zakaria et al., 2022). However, to date, there is no review paper focusing on the synthesis and reduction of graphene materials using date palms. The objective of this review paper is to provide a comprehensive analysis of the recent studies in synthesizing and reducing graphene materials using different date palm parts. The paper detailly investigates the different preparation methods used in producing graphene materials from date palm, the results of characterization tests used to study the surface of the produced date palm-derived graphene as well as its performance in environmental applications. Challenges and opportunities in the synthesis and reduction of graphene materials using date palm waste are also discussed. This novel review sheds light on the opportunity of utilizing this abundant type of agricultural waste and open the doors for more research in the area of converting date palms into valuable materials for different applications.
2 Overview of date palm and graphene
2.1 Date palm overview
In dry and semi-arid regions, especially in the Arab world, date palm is a significant crop (Saleem et al., 2023). Frequently, the plant grows from a single root system as a clump of one or more trunks, although it can also grow as a single tree. It is around 15–25 m tall tree and the leaves are 3–5 m long. It has 150 leaflets that are roughly 30 cm long and 2 cm wide (Alkhalaf et al., 2023). In addition to being an essential source of food for humans and animals, other date palm components are used in chemistry, medicine, pharmacognosy, and building industries (Kwaasi, 2003). The chemical analysis of different parts of the date palm tree is presented in Table 1. It shows a high percentage of carbon and oxygen. The date seed consists of 44.1 wt. % carbon and 48.3 wt. % oxygen. While the date palm leaf contains a higher carbon percentage of 50.4 wt. %, the stem contains a lower carbon percentage of only 38.1 wt. % (Hussain et al., 2014). The date palm tree has an extremely hard body that allows it to endure dry and harsh conditions and even grow again after fire damage (Tahir et al., 2020).

Table 1. Chemical analysis of different date palm parts (Hussain et al., 2014).
Globally, one million hectares are used for date palm tree farming. Date palms are believed to occupy approximately 3% of the world’s cultivable land (Tahir et al., 2020; Younis et al., 2023).
Date fruit is sold as a high-value product all over the world, and in most arid regions, it is considered a crop of significant importance (Chandrasekaran and Bahkali, 2013). It is reported that there are between 100–120 million date palm trees in the world and 70%–90% of them are present in the Middle East and North Africa region (Makkawi et al., 2019). An estimated 25–30 kg of waste is produced by each date palm tree from leaves and surface fibres surrounding the trunk. Moreover, there is another 10–15 kg from the fruit-bearing bunches. Every year, about 4 million tons of date palm waste is produced worldwide (Figure 2) (Galiwango et al., 2017). Furthermore, the Middle East and North Africa (MENA) region produces more than 2.7 million tons of waste yearly. After harvesting of palm trees, a lot of residues build up in agricultural areas every year. Leaves, midrib base or petiole, leaflets, spadix stems, mesh, pits, and rachis are examples of these residues (Manai et al., 2024).
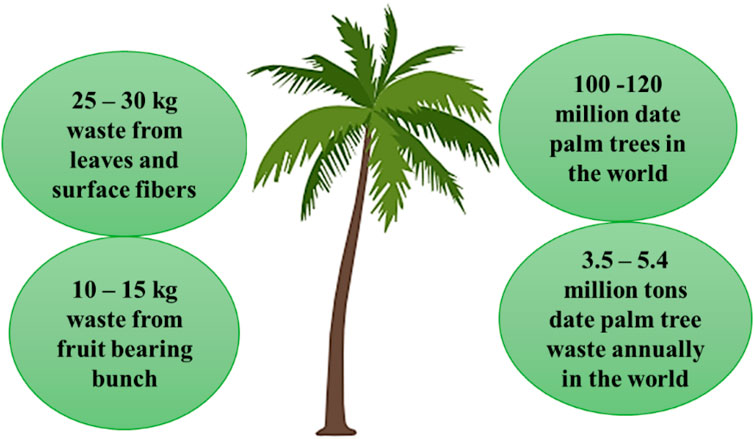
Figure 2. Total approximate number of date palm trees and the approximate global annual date palm waste.
Date palm wood, leaves, and stems are considered agricultural waste with a lot of potential for use as polymeric matrix reinforcement (Bezazi et al., 2024). The fibers from date palms exhibit remarkable qualities, such as low density and excellent inherent mechanical qualities. It is also cheap, plentiful, biodegradable, and renewable (Chkala et al., 2024). Natural fibers are becoming more and more popular due to the growing demand for more renewable and green resources. Natural fibers such as flax, sisal, kenaf, pineapple, oil palm fiber, and date palm have become more well-known as possible reinforcement for composite materials. The basic building blocks of a natural fiber are stiff, crystalline cellulose microfibrils covered in an amorphous matrix composed of hemicellulose and lignin (Al-Awa et al., 2023; Sarmin et al., 2023). Cellulosic polysaccharides, which have good binding properties, make up around 44% of the date palm tree’s fibers, followed by hemicellulose (26%), lignin (11.5%), and wax, fat, pectin, and inorganic substance lignin (18.5%) (Supian et al., 2021). Natural fiber composites find use in the furniture, automotive, building, and packaging industries (Abdellah et al., 2023). Utilizing date palm fibers and leftover cardboard, a unique thermal and acoustic insulating material was developed that can reduce energy use, enhance thermal comfort in buildings, and minimize greenhouse gas emissions (Benallel et al., 2024). Furthermore, the reinforcing material for the bumper beam was selected to be date palm fibers. The most crucial part for passenger safety in cars is the bumper beam. Using the hand lay-up approach, date palm reinforced epoxy composites and Kevlar were combined to make specimens with different weight percentages. Better boost in tensile strength and tensile modulus was demonstrated by the manufactured specimen (Muthalagu et al., 2021). An analysis of the mechanical properties of several date palm fiber kinds found in the Middle East revealed that the average tensile strength of date palm fiber is 135 ± 44 MPa, and ranges from 97 to 196 MPa. On average, Young’s modulus is 4.6 ± 0.6 GPa, however, it can vary from 2.5 to 5.4 GPa. These average qualities are far superior to typical building materials which highlight the possible use of date palm fiber in composites (El-Shekeil et al., 2024).
2.2 Overview of graphene and its derivatives
A single-layer, two-dimensional carbon-based nanomaterial is called graphene. It is made up of carbon atoms that have undergone sp2 hybridization and are structured like a honeycomb. (Joshi et al., 2023; Kumar et al., 2023; Manikandan and Lee, 2023). Graphene’s sp2-hybridized carbon atoms leave a free pz orbital that is perpendicular to the plane. Three sp2-bonded carbon atoms make up the honeycomb lattice of graphene (Figure 3a) (Tucek et al., 2018). Two equivalent sub-lattices of carbon atoms make up each unit cell in graphene. These sub-lattices are connected by σ bonds (Balasubramanian and Chowdhury, 2015). Covalent bonds between all atoms are formed by in-plane σ-bonds (sp2 orbitals) and π-bonds (2p orbitals) that are oriented perpendicularly to the basal plane (Figure 3b) (Joshi et al., 2023).
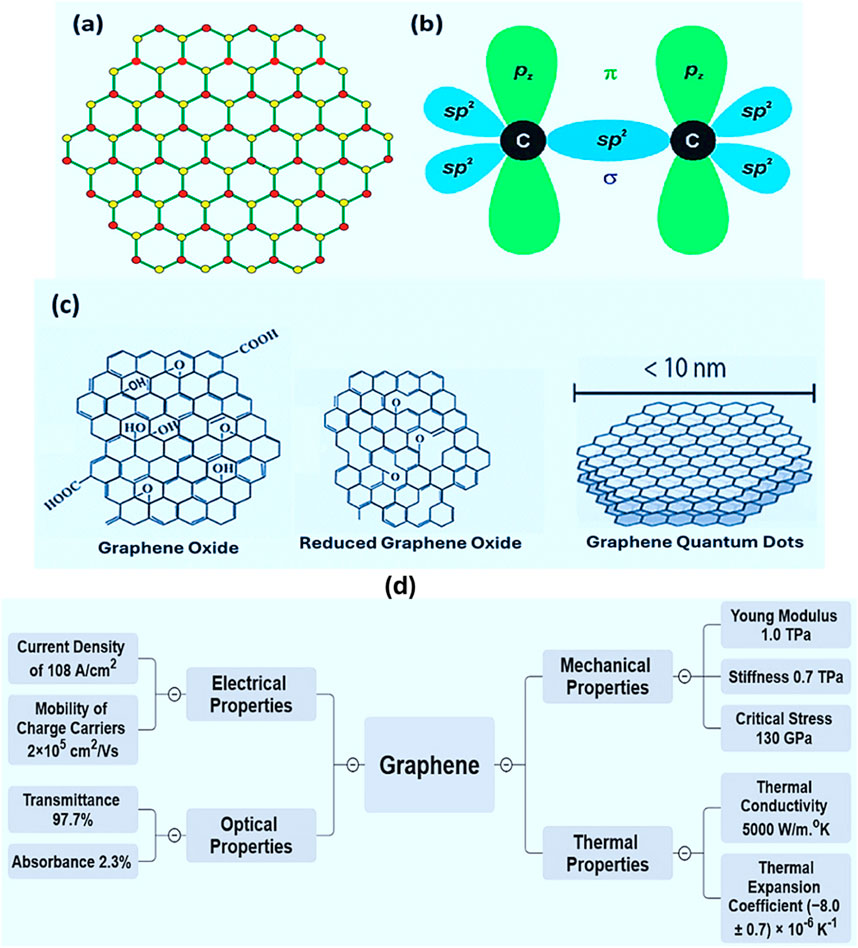
Figure 3. (a) Representation of the honeycomb lattice of graphene (Reproduced with permission from Balasubramanian and Chowdhury, 2015 ©Royal Society of Chemistry), (b) sp2 hybridization in graphene (Reproduced with permission from Tucek et al., 2018 © Royal Society of Chemistry), (c) structure of different graphene derivatives (Reproduced with permission from Chung et al., 2021 ©Wiley; Balqis et al., 2023), and (d) values of different graphene’s mechanical, thermal, electrical, and optical properties.
Graphene was discovered in 2004 by Novoselov et al. The groundbreaking discoveries of compounds of hybridized carbon with sp2 such as graphene, carbon nanotubes, and C60 opened completely new areas of study. Graphene appears to have the most potential within the carbon nanomaterial family because of its remarkable electronic, optical, magnetic, mechanical, and thermal characteristics. Because of its multilayered three-dimensional (3D) structure and two-dimensional (2D) lattice, graphene is believed to be the most layered and stable substance ever known. In the last several years, there has been an accelerated number of studies devoted to different aspects of graphene (Joshi et al., 2023; Kumar et al., 2023; Manikandan and Lee, 2023). Carbon-based nanomaterials, including graphene, fullerenes, and carbon nanotubes, have garnered a lot of attention due to their numerous applications. These materials find application in numerous fields, including chemical engineering, photochemistry, optical, sensors, mechanical, electrical, biocompatible materials, adsorption technologies, photocatalysis, energy storage, thermal management, and biomedical applications (Kumar et al., 2023).
Even though pristine graphene has many special qualities, employing it in its native form can be difficult because of its low solubility and quick aggregation in the solution because of van der Waals interactions. Consequently, to preserve the special qualities of pure graphene and to benefit from alterations such as the surface having a functionalized oxygen group in the case of graphene oxide (GO), graphene derivatives were created (Joshi et al., 2023). GO is a system of carbon rings with a two-dimensional structure with a variety of surface functional groups, including carboxyls, hydroxyls, epoxy, and carbonyls. It has several unique features such as high surface area, augmented oxygen-containing groups and hydrophilicity (Mahor et al., 2021; Kumar et al., 2023). Due to its excellent dispersion in aqueous solutions and high oxygen content, graphene oxide, which is a single sheet of graphite oxide crystal is widely used as chemical precursor for a range of graphene materials in diverse applications (Chiang et al., 2023). For GO nanoparticles to be potentially used in medical applications, especially in drug delivery systems, their small size (less than 100 nm) is essential (Casallas Caicedo et al., 2020). The Staudenmaier method and the Hummers approach are two extensively used methods for producing graphene oxide. Graphite is treated with different oxidizing agents in both methods. The Hummers' procedure uses sulfuric acid and potassium manganate (VII) solution as oxidizing agents. Conversely, the Staudenmaier process uses nitric acid and potassium (Chiang et al., 2023).
A novel kind of layered carbon nanomaterial with excellent chemical stability, elasticity, conductivity, and desirable thermal properties is called reduced graphene oxide (rGO). It is a good candidate for application as a photocatalyst due to its high absorbance, excellent chemical stability, and simplicity of production (Manikandan and Lee, 2023). GO is converted to rGO via thermochemical, electrochemical, or photoreaction processes. The number of functional groups containing oxygen is significantly reduced as a result of these approaches. When rGO is compared to GO, it has a higher specific area due to a lower concentration of functional groups. GO has hydrophilic inclinations, whereas rGO has hydrophobic qualities due to variations in the functional groups on the surface (Thangaraj et al., 2024). Many applications, including those in health, chemistry, nuclear physics, the environment, and engineering, have profited from this diversity of properties, which are determined by the functional groups that reside on the surface of GO and rGO (Torrisi et al., 2022).
Another graphene material that has an anisotropic lattice structure is graphene quantum dots (Li L. et al., 2024). A few nanometers make up the lateral dimension of GQDs. The majority of synthesized GQDs are elliptical or circular, while they can also be triangular, quadrate, or hexagonal. They are smaller and have a higher crystallinity than carbon dots. Different defects and functional groups are added to the GQDs depending on the synthetic methods, which may drastically change the structural and physicochemical characteristics (Zheng et al., 2015). GQDs are considered zero-dimensional materials and in addition to having the fascinating characteristics of graphene, they also show remarkable characteristics like non-zero band gaps, edge effects, and quantum confinement (Chaturvedi et al., 2022). Because of its unique physicochemical characteristics and potential for economic uses, there has been a lot of interest in graphene quantum dots. They have great solubility, tunable photoluminescence, low toxicity, strong biocompatibility, and simplicity of functionalization. These characteristics make them excellent alternatives to conventional semiconductor quantum dots (Abbas et al., 2023). Carbonic precursors are oxidatively cut to create GQDs under extreme conditions with potent oxidizing agents such as nitric acid and concentrated sulfuric acid (Mahalingam et al., 2022). The structure of different graphene derivatives is shown in Figure 3c.
2.3 Graphene properties
Graphene has special mechanical, thermal, electrical and optical characteristics due to the highly organized and firmly packed monolayer of carbon atoms (Khoei and Khorrami, 2016; Chung et al., 2021). Its surface area is 2,630 m2/g, its strength is 1.0 TPa, and its stiffness is 0.7 TPa. In addition to its optical transmittance of 97.7% and electrical conductivity of 108 A/cm2, graphene can handle electrical current densities one million times higher than copper (Kumar et al., 2019). It has a specific capacity of 744 mA h/g and a specific capacitance of 550 F/g. Its high electrical conductivity which is equal to 106 S/cm is more than copper by a factor of six and 60 times greater than single-walled carbon nanotubes (Chakraborty and Hashmi, 2018). Graphene’s amazing features are caused by its 2p orbitals, which form the π state bands that delocalise along its sheet of carbons (Papageorgiou et al., 2017). Figure 3d shows some values of the exceptional graphene properties, and a comparison of different graphene materials properties is provided in Table 2.
2.3.1 Mechanical properties
Graphene has distinguished mechanical properties that make it a good reinforcing agent in composites. Graphene’s mechanical characteristics are attributed to the stable sp2 bonds that form the hexagonal lattice. High-quality monolayer graphene with no defects is thought to be the strongest material yet tested. Its intrinsic strength was reported as 130 GPa and the Young modulus of elasticity was found to have a value of approximately 1 TPa (Papageorgiou et al., 2017). Single-layer graphene is the best nanofiller ever tested (Kumar et al., 2019). Among graphene’s most important mechanical properties is fracture toughness since it is a feature that is crucial for engineering applications. The energy release rate under critical strain was determined to be 15.9 J/m2, and the critical stress intensity factor of 4.0 ± 0.6 MPa indicated the fracture toughness of graphene. These values suggest that the toughness of graphene is mostly related to the membrane’s weakest point, or the initial point of failure (Papageorgiou et al., 2017).
Graphene oxide is mechanically less resistant than graphene and reduced graphene oxide is more mechanically resistant than graphene oxide. On the other hand, graphene oxide has the benefits of easy exfoliation, bulk availability, and low cost in the commercial domain (Torrisi et al., 2022). For graphene oxide, it was demonstrated that adding more oxide agents causes the graphene sheet’s Young’s modulus and ultimate tensile stress to decrease. Moreover, the elastic constants drop as the amount of oxide agents in the graphene sheet increases, with the armchair direction seeing a greater loss of elastic characteristics than the zigzag direction. Furthermore, with an increase of oxide agents (-O/-OH), it was found that the graphene sheet’s failure behaviour shifts from brittle to ductile (Khoei and Khorrami, 2016).
2.3.2 Thermal properties
Graphene is considered a good thermal conductor (Khoei and Khorrami, 2016). A trench was covered with graphene, and by applying a laser to the material and measuring the temperature increase in the center using Raman-scattered light, the thermal conductivity of the material was ascertained (Freitag, 2010). The thermal conductivity of graphene is much greater than that of graphite and diamond and similar to carbon nanotubes (Chakraborty and Hashmi, 2018). According to published data, graphene has a heat conductivity of roughly 5000 W/m. °K. High increases in effective thermal conductivity can be obtained by adding an optimum blend of multilayer graphene to composites with varying matrix components. For a range of matrix materials, the increased heat conductivity brought about by the addition of graphene to the composites has been documented (Shahil and Balandin, 2012; Korkmaz and Kariper, 2020).
Apart from thermal conductivity, graphene has other good thermal properties such as thermal expansion coefficient. This coefficient is important in the thermal management of nano and microelectromechanical systems. Using temperature-dependent Raman spectroscopy, the thermal expansion coefficient was calculated in the 200–400°K temperature range. It is shown to be negative across the whole temperature range and temperature-dependent, with a value of (−8.0 ± 0.7) × 10–6 °K−1 at room temperature (Yoon et al., 2011; Kumar et al., 2019).
2.3.3 Electrical properties
Although graphene’s atoms are all exposed to the environment, the substance is inert and does not chemically react with other atoms. However, it can absorb other molecules and atoms. This significantly alters graphene’s electrical characteristics, making it suitable for use in sensor applications. Graphene has an intrinsic charge mobility higher than silicon and a current density higher than copper (Pandey et al., 2024). A graphene sheet’s electrical properties are significantly influenced by the quantity of graphene layers within it. The possible use of graphene in transistors is attributed to the electron-hole dependence. However, this reliance is exclusive to graphene sheets with a single layer. The reliance decreases with an increase in the number of layers (Phiri et al., 2017).
Due to its high electron mobility, graphene exhibits an electrical conductivity of 6500 S/m. It possesses a zero-band gap, which prevents its sheet from dispersing in the aqueous solutions (Joshi et al., 2023). The zero-energy bandgap makes graphene the most effective conductor (Pandey et al., 2024). This property is associated with infinite-dimensional, defect-free graphene. When the material’s physical dimensions are reduced to finite values and defects are added to the lattice, the electronic structure of graphene alters so that non-zero values of energy band gaps can be identified. Reducing the size of the graphene fragment can raise the graphene’s bandgap. This programmable electronic structure finds uses in electronics, energy harvesting, and medicine (Chung et al., 2021). Graphene oxide’s structure is greatly disorganized and numerous oxygen functional groups exist on the basal plane and at the edges. Low electrical conductivity is caused by the charge carriers being dispersed by this chaotic structure (M K and Jaiswal, 2016).
2.3.4 Optical properties
The optical characteristics of the graphene generated through each synthesis method are unique because of the structural flaws. For example, in addition to line defects at the grain boundaries, chemical vapour deposition graphene is characterized by tiny densities of sp3 defects and a certain quantity of amorphous carbon and metal catalyst impurities. The presence of these contaminants can affect the optical response, even though their effects on the electrical properties are often negligible (M K and Jaiswal, 2016). Applications of graphene in the optical and optoelectronic domains have garnered increasing attention since 2008, due to advancements in graphene production processes and electronics understanding. For instance, indium tin oxide, which is costly, fragile, and based on the scarce element indium, may be replaced by graphene, a promising transparent conductive electrode. Touch screen prototypes have been developed using transparent 30-inch graphene electrodes. High-frequency photodetectors, photovoltaics, and biosensors are three areas where graphene shows great promise (Chang and Wu, 2012).
As the number of graphene layers increases, graphene’s optical transmittance falls. Single-layer graphene has a transparency of about 97.7%. In other words, it can absorb 2.3% of white light. However, bilayer graphene absorbance of white light increases to 4.6% (Phiri et al., 2017; Joshi et al., 2023). The optical absorption of graphene is due to the interband and intraband transitions. This small absorption percentage needs to be viewed in the context of the absorbent layer’s single-atom thickness. When this thickness is taken into account, it can be seen that the light-matter interaction in graphene is extraordinarily large (M K and Jaiswal, 2016).
2.4 Biomass-derived graphene synthesis
Currently, a growing body of research indicates that the most effective method for producing high-quality, reasonably priced graphene materials is by using agricultural waste as the precursor raw material (Li L. et al., 2024). Despite the great purity of graphene obtained from biomass, its structural integrity can be affected by a variety of source materials and processing techniques. Therefore, optimizing biomass materials and synthesis techniques is necessary (Zhou et al., 2022). Numerous agricultural wastes have been the subject of in-depth research as raw materials for the synthesis of graphene and products derived from it. Graphene oxide was produced using wheat straw (Dawood Saleh et al., 2023), corncob (Tinh et al., 2023), and sugar beet bagasse (Faghiri and Ghorbani, 2020) whereas graphene quantum dots were produced using paddy straw (Chaturvedi et al., 2022) and oil palm empty fruit bunch (Mahalingam et al., 2022). Reduced graphene oxide was produced from coconut shells and coconut coir (Tamilselvi et al., 2020) and dry leaves of sugarcane (Thangaraj et al., 2024).
2.4.1 Conventional synthesis approaches
Graphene synthesis methods usually include the usage of large amounts of expensive and toxic chemicals. Therefore, researchers have focused on creating more practical and reliable processes for synthesizing graphene from biomass precursor materials (Li L. et al., 2024). Carbon-based materials are conventionally prepared from biomass by simple procedures such as pyrolysis, carbonization and activation and hydrothermal (Figure 4a). In most cases, no harmful chemicals are used (Urzúa et al., 2024). The synthesis procedures and a summary of various agricultural wastes utilized to make graphene, and its derivatives are given in Table 3. Carbonization and activation are the main two steps in the production of graphene from biomass. Firstly, biomass gets dehydrated and then crystallized at high temperatures. The microstructure of obtained carbon depends on the carbonization temperature. The activation can either be physical using steam and/or CO2 or chemical using NaCl, KCl, KOH, ZnCl2 or H3PO4 (Sawant et al., 2022; Zhou et al., 2022). Although it has been demonstrated that chemical activation can create a broad pore size distribution with large channels for quick ion transfer and effective storage, their severe toxicity, thermal instability, and corrosivity preclude their widespread use in clean and sustainable energy storage (Li J. et al., 2024).
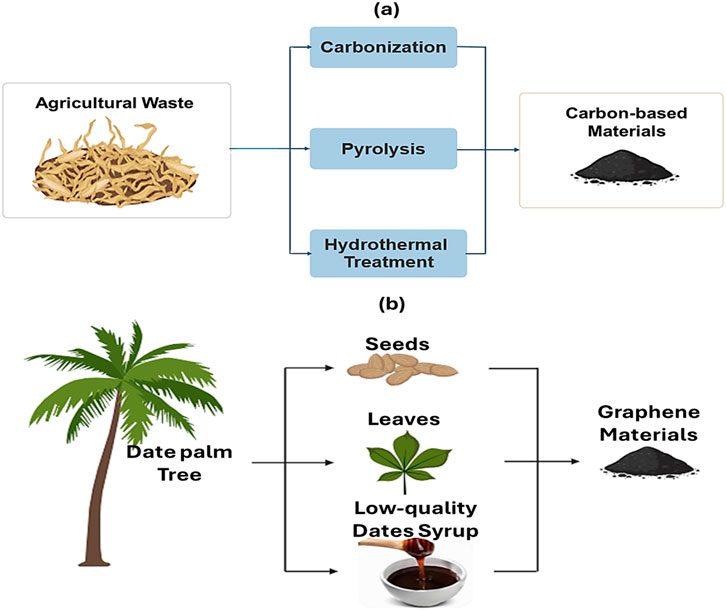
Figure 4. (a) Conventional synthesis methods of carbon-based materials from agricultural waste, and (b) types of date palm tree wastes used to produce graphene materials.
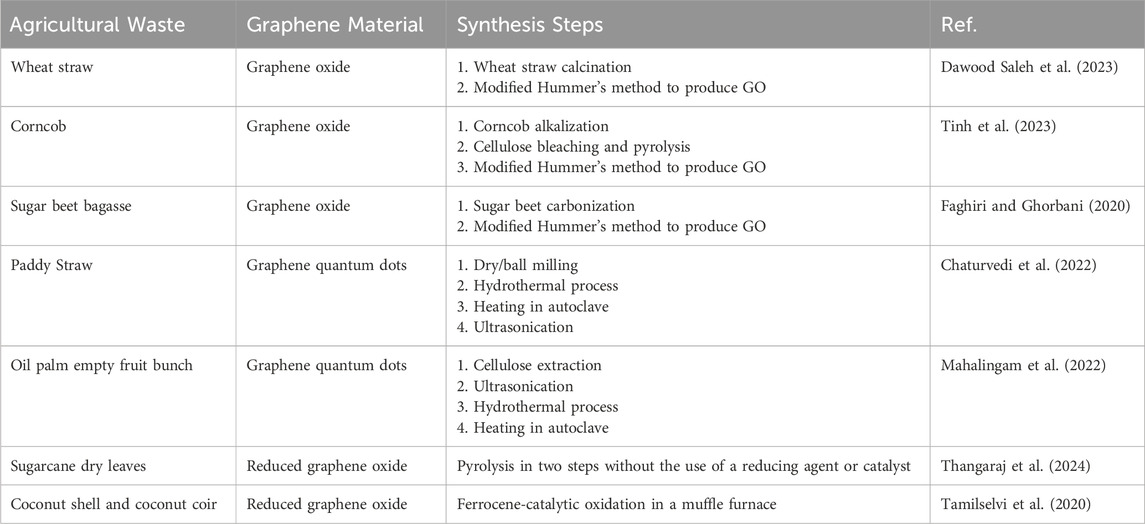
Table 3. Examples of some agricultural wastes and the synthesis methods used to produce graphene and its derivatives.
One process for turning biomass into graphene is pyrolysis. It is the process of oligomers and polymer precursors being thermally deposited onto silicon carbide surfaces while a catalyst is present, and the atmosphere is inert. Over the whole silicon carbide substrate, it continually creates high-purity functionalized graphene. Carbon nanostructures build up because of thermal disintegration at excessive temperatures during graphene production without oxidation processes, which lowers the total specific surface area of the generated graphene materials (Kumari and Samadder, 2022). Pyrolysis has a number of benefits, although often being carried out at a higher temperature. For instance, pyrolysis eliminates the requirement for water resources, saving energy on wastewater treatment and solid-liquid separation. In addition, pyrolysis can increase the material’s porosity and surface area. Temperature, reaction duration, and additives can also be used to adjust the characteristics of carbon compounds produced by pyrolysis (Yu et al., 2022). Another common strategy is hydrothermal treatment for converting biodegradable waste to carbon-based materials which has various structures and compositions. It turns biomass precursors into structured carbon materials. Hydrothermal treatment is often conducted at a temperature, typically between 180 and 250°C and at pressures more than 1 atm. The purpose of pre-treatment is to increase biomass solubility, biomass-solvent interaction, and the chemical reaction (Sawant et al., 2022; Dong et al., 2023). In hydrothermal processes, water acts as a catalyst, reactant, and solvent. A range of feedstocks can be converted into a number of desirable products thanks to the hot compressed water (such as subcritical or supercritical water). Hydrothermal processing is a method of accelerating natural transformation pathways to create desired products by processing organic matter at high pressures and temperatures (Zhang et al., 2023). The capacity to function at low temperatures, the ability to process any kind of wet biomass without pre-drying, and the high surface functionality of the resulting materials are only a few benefits of the hydrothermal process. The process does, however, have many drawbacks, such as the requirement for costly, sophisticated, and pressured equipment, the extra expense of separating the solid and liquid products, and the significant process water discharge (Ercan et al., 2023).
2.4.2 Non-conventional synthesis approaches
One promising technique for the effective and versatile conversion of biomass is molten salt technology. Molten salts are used in thermochemical processes as solvents, catalysts, and heat carriers due to their exceptional thermal conductivity, solubility, and catalytic activity. Some of the characteristics of molten salt technology include high thermal efficiency, even temperature distribution, good heat storage, increased reaction speeds, and a regulated reaction environment. Molten salts are very successful at increasing the conversion efficiency and product quality of materials derived from biomass because of their multifunctionality (Wang et al., 2025). To create carbon with significant capacitance, molten carbonates with mild characteristics and good stability have been successfully used in tobacco stems, peanut shells, bamboo shells, and other biomass. However, it was discovered that molten carbonates encourage the release of nitrogen from carbon in the form of NH3, which lowers the amount of pyrrole-N and pyridine-N in the carbon. These factors have been demonstrated to have a major impact on capacity of capacitance. Therefore, while utilizing molten carbonates as activators to further increase the capacitive performance, it is crucial to retain more nitrogen in carbon and control it to the required functional group (Li J. et al., 2024). Nevertheless, among the most promising techniques for converting biomass is molten salt-assisted pyrolysis. This is due to its benefits, which include easier equipment and operation, better product quality, higher pyrolysis efficiency, and faster mass and heat transfer (Atakoohi et al., 2024).
An effective conversion technique that can convert biomass quickly, distribute heating evenly, and have a smaller environmental impact is microwave-assisted conversion. The produced material has better qualities than other conversion processes, such as a higher concentration of carbon, a large reactive surface area, a larger volume of microporosity, and rich carboxylic and hydroxyl surface groups (Allende et al., 2024b). Random external elements such as plasma generation, metal discharge, thermal hotspot formation, etc., can also disrupt the chemistry of microwave heating because of instantaneous non-uniform heating and varying electric fields. Due to its dielectric properties, which alter microwave permittivity, biomass, on the other hand, is a poor microwave absorber. This results in the need for the installation of microwave absorbers, which raises energy expenses. For the design and development of microwave reactors with specific temperatures and frequencies to be successful, the dielectric characteristics need to be well specified by additional research. Due to our poor understanding of the electromagnetic field dispersion in a microwave environment and the interaction between biomass and microwaves, large-scale applications are difficult (Divyabharathi et al., 2024).
Another non-conventional synthesis approach is flash Joule heating. This is an efficient and cost-effective method for the synthesis of 2D materials. It produces an immediate high temperature in less than a second using the sample’s electrical resistance exotherm. Low-defect graphene can be synthesized in less than a second with very high yields using biomass. The flash Joule heating process involves mixing biomass feedstock with carbon black to improve its electrical conductivity, then placing it between two electrodes in a quartz or ceramic tube. The carbon source quickly reaches high temperatures (above 2,700°C) in extremely short time (less than 100 ms) under the high-voltage discharge resulting in the transformation of amorphous carbon into layered graphene material. This technique does not require using any harmful or dangerous chemicals. Therefore, neither traditional furnaces nor the use of solvents or reactive agents are necessary for the synthesis process and there is no need for further purification steps (Dong et al., 2023; Li Q. et al., 2024). However, specialized equipment that can handle the harsh process conditions—such as high voltage, temperature, and pressure—is needed for flash Joule heating. This equipment can have a significant upfront cost, and it may be difficult to maintain overtime (Hosny et al., 2025). A summary of the advantages and limitations of the different synthesis approaches is provided in Table 4.
3 Date palm-derived graphene synthesis and characterization
Worldwide, date palm trees are estimated to number between 100 and 120 million and each tree produces about 40 kg of waste per year. This means that the annual quantity of date palm waste is about 4 million tons. Currently, the majority of date palm waste is burned to provide heat or composted, even though it contains a high lignin, cellulose, and hemicellulose content suitable for conversion into higher-value goods (Galiwango et al., 2017; Makkawi et al., 2019). The efforts made to create graphene materials and to reduce graphene oxide using date palms are discussed in the section that follows. The characterization results of the produced graphene materials are discussed and compared to graphene produced from oil palm waste as both oil palm and date palm are closely related species and belong to the same family which is the (Arecaceae) Palmae family (Bourgis et al., 2011). The coming section is divided into four categories according to the part of the date palm tree which was used as a raw material (Figure 4b).
3.1 Graphene from date palm seeds
Seeds make up 8–15 wt. % of the fruit on date palms (Jonoobi et al., 2019). Several research looked into the production of biodiesel from date palm seeds (Demirbas, 2017), others employed it as an adsorbent for several pollutants such as boron, lithium, and molybdenum (Da’na et al., 2023). As a carbon source, date seeds or pits were used to produce graphene oxide (Al-Zahrani et al., 2022). First, the date seeds were processed to make a lignin. Then, the lignin was converted to graphite which was finally used to prepare the graphene oxide through modified Hummer’s method. The powdered date seed was mixed with 98% sulfuric acid to make lignin. After several steps of diluting, heating, washing, drying, and chilling, the acid-insoluble lignin was obtained. Iron (III) nitrate served as the catalyst source to prepare graphite from lignin. An iron nitrate solution was prepared and added to the lignin solution at 70°C. The iron-lignin mixture was stirred, dried, and heated which led to the formation of a solid iron-promoted lignin precursor. This precursor was then added to a quartz tube reactor where N2 and CO2 gases were passed through the reactor while heating to 900°C. After cooling, a few layers of graphite were taken out of the quartz tubular reactor and used to prepare graphene oxide. Figure 5 depicts a schematic of the process of synthesizing graphene oxide from date seeds.
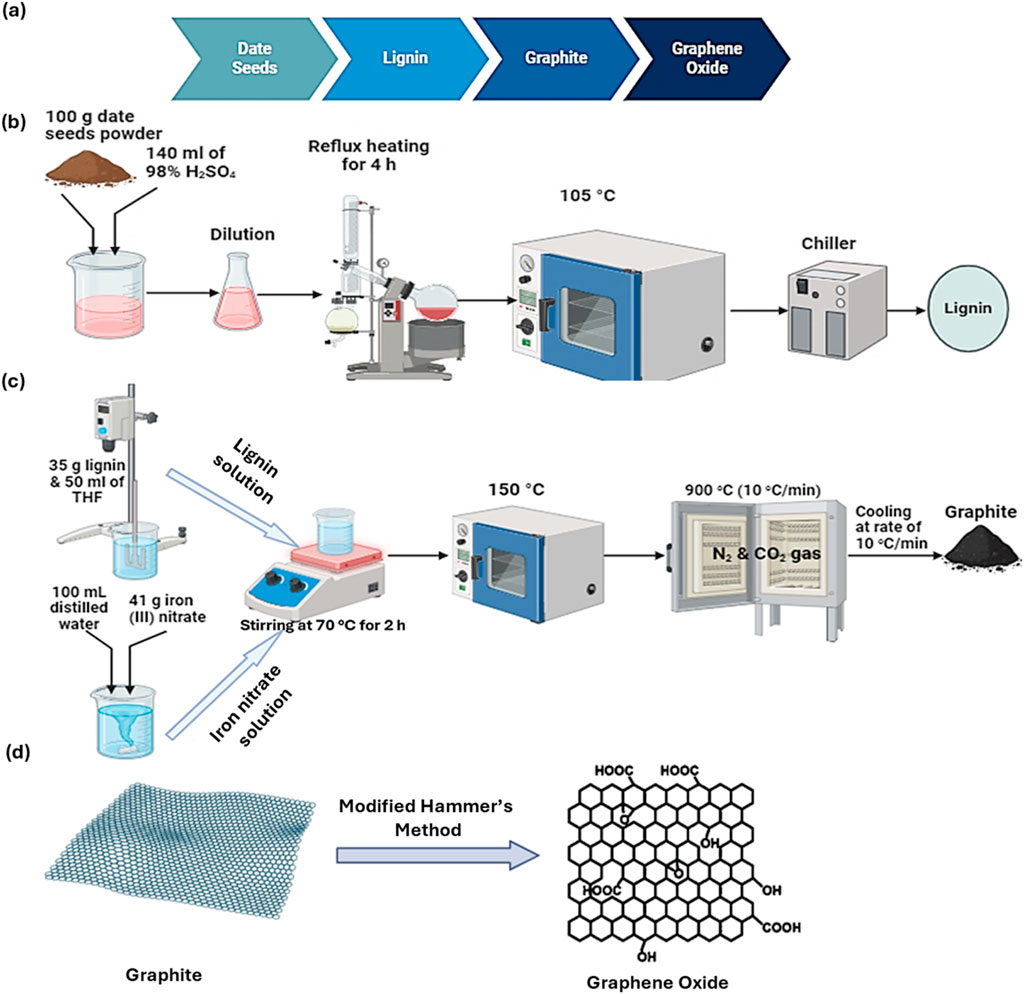
Figure 5. Schematic representation of the synthesis of graphene oxide from date seeds (a) the overall process, (b) extraction of lignin from date seeds, (c) synthesis of graphite from lignin and (d) production of graphene oxide from graphite.
Yaqoob et al. (2021) also created a graphene oxide substance and used it in microbiological fuel cells to enhance electron transportation. However, the raw material was the lignin of oil palm. Both oil palm and date palm are closely related species and belong to the same family which is the (Arecaceae) Palmae family (Bourgis et al., 2011). The lignin powder was heated in a furnace with argon gas to 1,100°C at a rate of 20°C per minute and the produced lignin carbon was used to synthesize graphene oxide through the Hummers’ method. In another research, (Mao et al., 2025), synthesized graphene via flash Joule heating technique within milliseconds utilizing lignin. To prevent excessively large particle size or excessive moisture content from reducing flash Joule heating efficiency, the lignin was processed in a blast drying oven at 45°C for 24 h before being combined with a superconducting carbon black for the reaction. Lignin’s substantial carbon content and stable aromatic ring structure made it a promising precursor to produce flash graphene. Following flash Joule heating, sp3 C-C bond composition dropped from 59.8% to 24.0%, whilst sp2 C-C bond content rose from 0% to 38.8%.
Characterization of synthesized materials is a crucial part of each study because it provides information regarding defects, layers, surface chemistry, morphology, texture, and other physicochemical characteristics (Kumari and Samadder, 2022). Several characterization methods were employed to examine the graphene oxide produced from both date palms and oil palms. According to scanning electron microscopy (SEM) the morphology of the manufactured graphene oxide and raw date seeds differed; date seeds had a smooth surface with no pores, while the graphene oxide showed some porosity as displayed in Figure 6a (Al-Zahrani et al., 2022). On the other hand, the SEM image of graphene oxide prepared from oil palm demonstrated an irregular, thin-layered and wrinkled structure (Figure 6b) (Yaqoob et al., 2021). Both graphene oxide materials' SEM showed some degree of an uneven and irregular structure, indicating a transformation in their morphological structure. Nevertheless, the SEM image of the date palm-derived graphene revealed certain portions of a smooth structure, which led to a lower surface area when compared to the oil palm-derived graphene oxide.
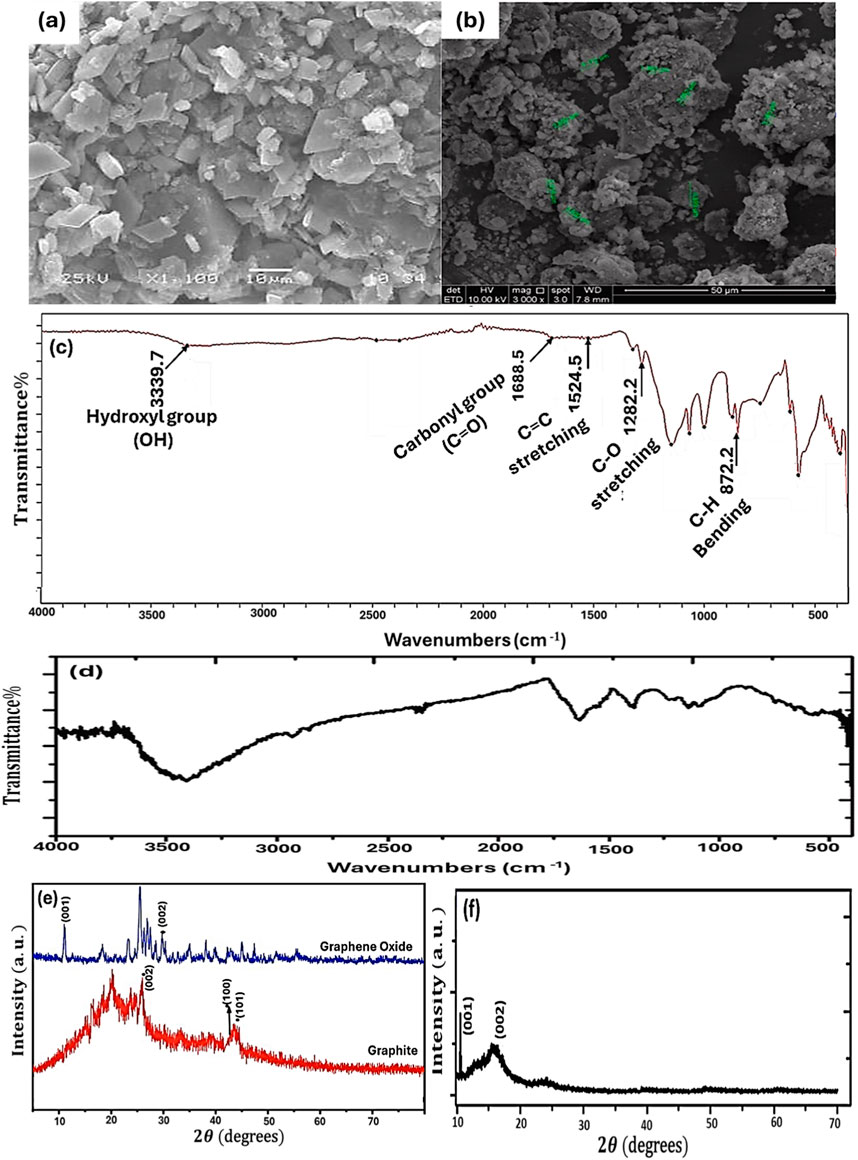
Figure 6. (a, c, e) SEM images, FTIR and XRD of graphene oxide prepared from date palm, respectively (Al-Zahrani et al., 2022). (b, d, f) SEM images, FTIR and XRD of graphene oxide prepared from date palm oil palm, respectively (Reproduced with permission from Yaqoob et al., 2021 ©Elsevier).
The surface area of the graphene oxide prepared from oil palm is 280.122 m2/g which is almost 10 times the surface area of graphene oxide prepared from date palm. This indicates a smaller particle size and higher porosity of oil palm-derived graphene oxide. While both graphene oxide materials were developed through Hammar’s method using graphite derived from lignin, date palm lignin underwent an additional co-precipitation process using iron (III) nitrate as a catalyst source before being carbonized. Moreover, the method employed in extracting lignin may also contribute to dissimilar characteristics of the extracted lignin. These disparities in graphite preparation can result in variations in the surface area of the produced graphene oxide.
A high-resolution, quick and non-destructive method for analyzing the electrical, optical, and phonon characteristics of carbon materials as well as their lattice structure is Raman spectroscopy (Tajik et al., 2020). Graphene has distinct vibration bands due to the resonantly enhanced Raman scattering of carbon materials, which has led to the widespread use of Raman spectroscopy to study the molecular structures of carbon compounds (Lee et al., 2021). The D band, G band, and 2D band are the strongest and most extensively researched Raman bands for sp2 carbon materials and their composites. The first order G band is about 1,580 cm-1, while the second order bands D and 2D are between 1,300 ∼ 1,400 cm-1 and 2,600 ∼ 2,700 cm-1, respectively (Li Z. et al., 2023). The D peak can be considered as a carbon atom’s sp3 hybrid structure or sp2 bond hybrid defect. Fully structured graphite crystals do not have the D peak. The crystalline structure of the material is represented by the stretching movement of the sp2 carbon atom, which produces the G peak (Wu and Yu, 2020). Both date palm graphene oxide and oil palm graphene oxide’s D and G bands in Raman spectroscopy showed close values, which indicates similarity in sp2 hybridization disorder and lattice vibration, respectively (Mbayachi et al., 2021).
Fourier transform infrared analysis (FTIR) is one of the characterization techniques that provide information about the functional groups present on the outer layer of the fabricated nanomaterials and their vibrational characteristics (Vasudevan et al., 2023). Due to the presence of cellulose and hemicellulose in date seeds, graphene oxide prepared from them had a high hydroxyl group at 3,339.9 cm-1 (Figure 6c), which could be the result of water molecules. This attribution can also apply to the graphene oxide prepared from oil palm, which also showed a close peak at 3,450 cm-1 (Figure 6d). C = C stretching was shown at 1,524.5 and 1,635 cm-1 peaks for date-palm graphene oxide and oil-palm graphene oxide, respectively. The adsorption band at 1,688.5 cm-1 in date palm graphene oxide was attributed to the carbonyl group (C=O), which suggested the presence of oxygen.
Regarding XRD, graphite prepared from date seeds (Figure 6e) showed a peak (002) at 26.5° and an inter-planar distance (d 002) of 0.334 nm. This implied the carbonaceous nature of graphite as well as the high orientation degree. Yao et al. (2018) used in situ XRD to study the evolution of graphitic carbon structures from loblolly pine wood. The intensity of the disordered peak, which was situated at about 24°, diminished with temperature as the temperature rises from 800°C to 1,300°C. There was an intermediary step in the process where no solid crystalline graphitic structure forms, rather than the graphitic structure developing constantly from the disordered carbon structure. According to the XRD pattern, at 1,400°C, the disordered carbon had all vanished. A tiny, modest peak that is characteristic of graphite (002) emerged at 26.67° after the temperature reached 1,500°C. These findings implied that the final graphite structure was formed by reorganizing the intermediate turbostratic carbon structure. Conversely, the date seeds graphene oxide XRD pattern changed from 26.5° to 11.66°, meaning that there was a 0.80 nm inter-planar gap. An increase in graphene oxide’s inter-planar distance was attributed to the presence of oxygen functional groups (Al-Zahrani et al., 2022). On the other hand, oil palm graphene oxide showed an identical XRD peak at 2
Heat treatment of date seeds at different temperatures was studied by (Bin Sharfan et al., 2024) and resulted in the production of a graphene-like material. One pristine date seeds sample (S0) was prepared to be used as a reference along with the other three carbonized samples. The three samples S1, S2 and S3 have undergone pyrolysis at different temperatures 600°C, 800°C and 1,000°C. Characterization results revealed that the pristine date seeds sample has a non-porous structure (Figure 7a) while the produced graphene-like materials have a porous structure. The porous structure of the carbonized samples (S1, S2 and S3) can be noticed in the SEM images (Figures 7b–d). It can be observed that as the annealing temperature increases, the porosity also increases.
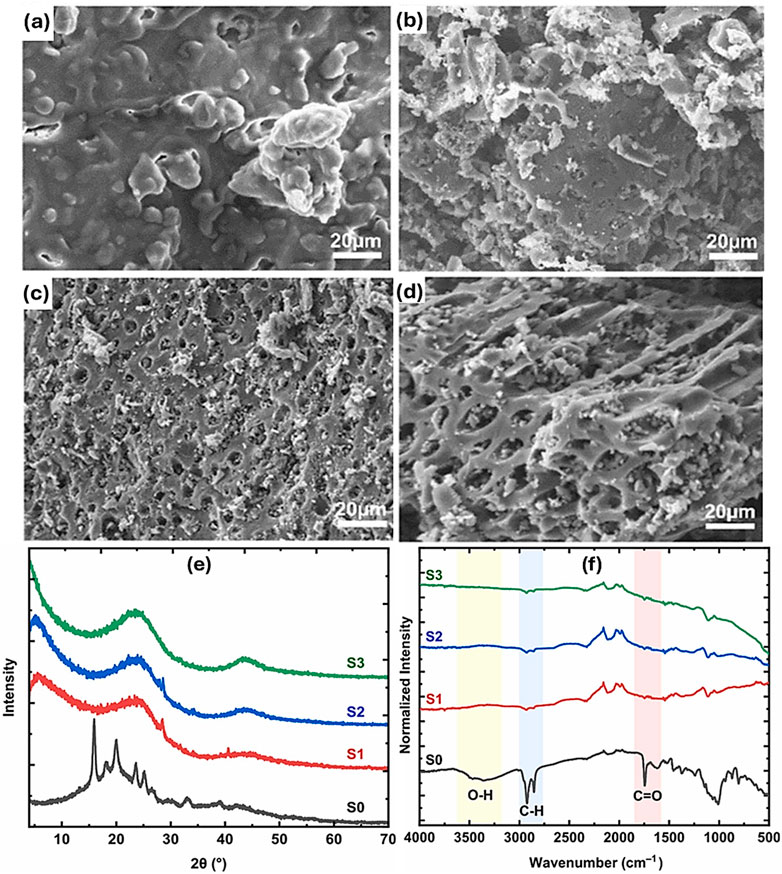
Figure 7. SEM images of (a) pristine date seeds powder S0, and pyrolyzed date seeds powder at different temperatures; (b) S1 at 600, (c) S2 at 800 and (d) S3 at 1000, (e) WXRD and (f) FTIR of pristine date seeds powder S0 and pyrolyzed date seeds powder at different temperatures (Reproduced with permission from Bin Sharfan et al., 2024 ©Elsevier).
Because cellulose, lignin, and hemicellulose were present, the wide-angle X-ray diffraction (WXRD) spectra of S0 (Figure 7e) revealed notable peaks of 15.9, 18.1, 20, 23.6, 25.1, and 32.8°. The presence of these peaks suggests that the atomic structure of the pristine date seeds sample is semi-crystalline. Following thermal annealing of date seed samples, the majority of the peaks observed in the X-ray diffraction pattern disappeared, resulting in the emergence of two distinct broad peaks at 2θ angles of 23.5° and 43.50° . These findings suggest the presence of carbonaceous material and indicate a morphological transition from a semi-crystalline to an amorphous state. This shift was also attributed to the fact that d-spacing between neighbouring planes has increased, which may enhance the porosity. Furthermore, in samples S2 and S3, raising the annealing temperature produced an amorphous morphology resembling that of S1. However, as the temperature rose, the peak at 43.5° became more intense, which suggested a rise in microporosity.
However, FTIR readings of the powdered date seeds at 150°C (before pyrolysis) (Figure 7f) displayed a broad absorption band for–OH stretching vibration between 3,200 and 3,400 cm-1. This is a sign that water, carboxylic acids, alcohols, and phenols are present. Moreover, results demonstrated peaks between 2,800 and 2,900 cm-1, and they were attributed to the stretching vibration of alkyl groups (–CH) resulting from the presence of lignin, cellulose, and hemicelluloses. The FTIR results of the pyrolyzed date seeds powder at 600°C, 800°C and 1,000°C showed the disappearance of functional groups such as–OH and–C––O, which indicated the conversion to carbonaceous materials. Brunauer–Emmett–Teller (BET) surface area of this graphene-like material produced through the thermal treatment of date seeds was 117 ± 13 m2/g. It was noticed that the pyrolyzed date seeds at 600°C have more porosity than the pristine sample which has only a BET surface area of 50 m2/g which indicated that carbonization at higher temperatures led to the production of a material with a large surface area and a porous structure. In another study, 3D porous graphene-like was created by carbonizing coffee and tea waste at 550°C and then thermochemically activating the mixture in KOH at 850°C. Coffee and tea waste’s microstructure demonstrated the formation of micro- and mesopores during carbonization, and additional chemical activation creates hierarchically extremely porous carbon that resembles graphene in three dimensions. According to the BET method, graphene-like derived from coffee waste had a greater specific surface area of 3,486 m2/g than graphene-like derived from tea waste, which had a specific surface area of 2,407 m2/g (Beissenov et al., 2024). These high reported specific surface areas, which even exceed the theoretical value of commercial graphene’s surface area, highlight the potential of graphene-like materials derived from biomass waste in applications that require high surface area, such as adsorption. It can be observed that the surface areas of graphene-like materials derived from tea and coffee waste are significantly higher than those derived from date palm seeds. This can be attributed to the difference in synthesis procedures, as graphene-like materials derived from date palm seeds did not undergo the KOH activation process, which can enhance the specific surface area and create a well-developed microporous structure.
3.2 Graphene from date palm leaves
The most residues that date palm trees produce each year are leaves (Jonoobi et al., 2019). About 30 kg of leaf waste is produced annually from each date palm tree (Galiwango et al., 2017). About 50.4% of the date palm leaves' overall makeup is carbon, making them carbon-rich (Hussain et al., 2014). Graphene quantum dots were synthesized utilizing two processes using date palm leaves (Saleem et al., 2023). Hydrothermal technique for 12 h at 200°C in water was used in both procedures. No reducing, passivizing agents or organic solvents were used in the first procedure. However, in the second one, absolute ethanol was used. In the first synthesis method, 5 g of leaf powder (obtained through ball milling) was boiled in 200 mL of deionized water at 80°C for 1 h. The extract underwent several steps of centrifugation, filtration, sonication, and hydrothermal treatment for 12 h at 200°C and finally, after drying the precipitate at 70°C for 4 h, GQDs powder was obtained. On the other hand, in the second method, leaf pieces were soaked in pure ethanol and the solution was stirred, centrifuged, filtered, and concentrated in a rotary evaporator. After that, the slurry was thoroughly dissolved in 100% ethanol to ensure that the GQDs were evenly dispersed and finally the dispersion was filtered out and dried to obtain GQDs. The results showed that the yields for GQD-1 and GQD-2, which were produced using methods 1 and 2, respectively, were 52% and 49.2%. Thus, procedure 1 was deemed to be more advantageous than the other one due to its larger yield. A schematic representation of the preparation of GQDs from date palm leaves using the two methods is shown in Figures 8a,b. Similar hydrothermal process was followed in a recent study where GQDs were prepared over 8–12 h from spent tea leaves at 200°C to create turbostratic carbon, which then was transformed into aromatic carbon and generated bright blue, fluorescent GQDs (Abbas et al., 2023).
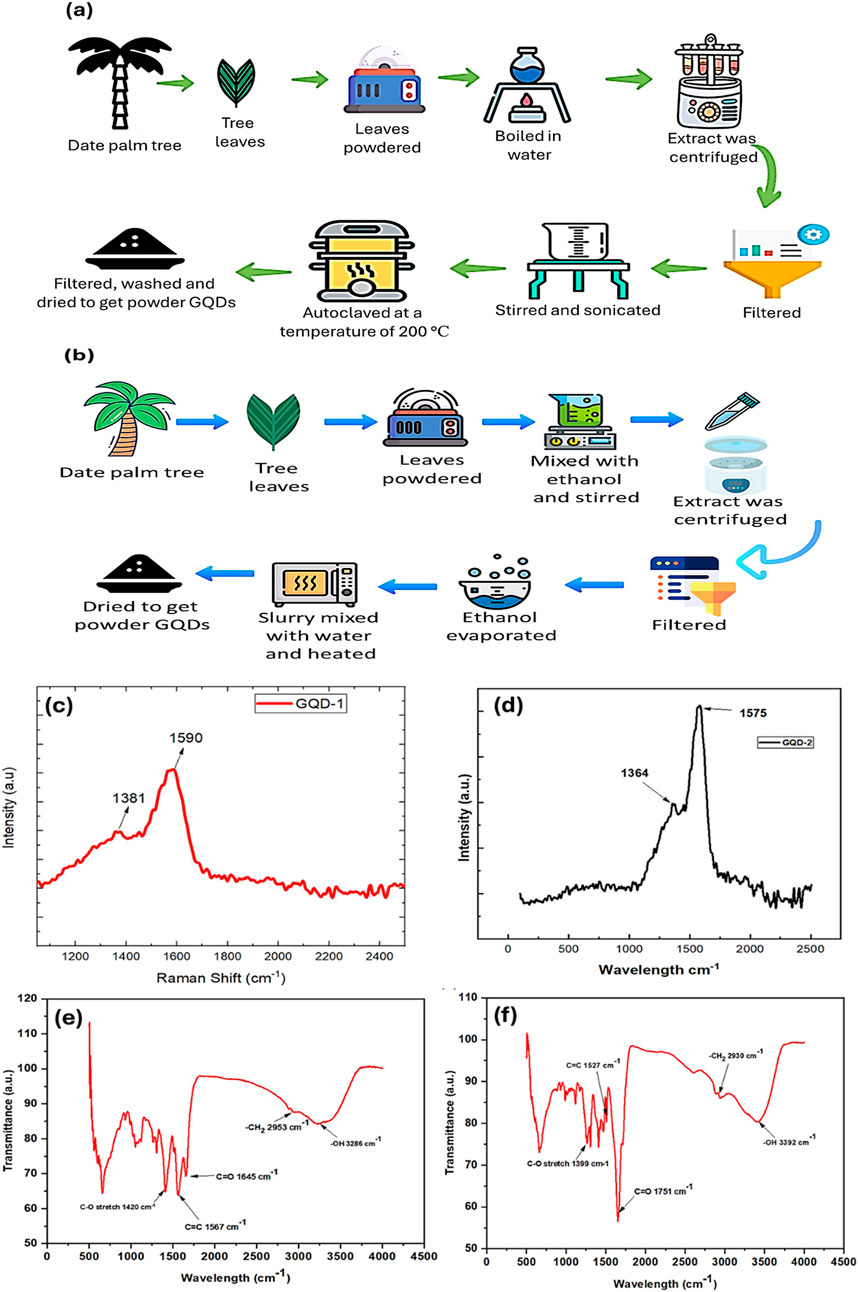
Figure 8. Schematic representation of the preparation of GQDs from date palm leaves using (a) method 1 (distilled water) and (b) method 2 (ethanol), Raman spectroscopy results of date palm graphene quantum dots prepared following (c) method 1 and (d) method 2, FTIR results of date palm graphene quantum dots prepared following (e) method 1 and (f) method 2 (Saleem et al., 2023).
The structure of GQD was studied using Raman spectroscopy (Figures 8c,d). The presence of D and G bands in GQD-1 (prepared from date palm leaves without using any chemicals) and GQD-2 (prepared from date palm leaves using only pure ethanol) was confirmed through Raman spectroscopy characterization. The D band is linked to sp3 hybridized carbon atoms that originate from defects and oxygen functional groups, and it is correlated with the crystalline quality. The G band is associated with the nature of crystalline, and it arises from sp2 hybridized carbon atoms (Chua et al., 2015; Saleem et al., 2023). For GQD-1 and GQD-2, the D band was detected at 1,381 cm-1 and 1,364 cm-1, respectively. Conversely, for GQD-1 and GQD-2, the G band was detected at 1,590 cm-1 and 1,575 cm-1, respectively (Saleem et al., 2023). These outcomes closely resemble those of graphene quantum dots synthesized from fullerene C60 (Chua et al., 2015). The results presented in Table 5 demonstrate the highly similar crystalline nature and quality of the three materials, as evidenced by the nearly identical values of the D and G bands for the GQD synthesized from date palm leaves and GQD synthesized from fullerene.
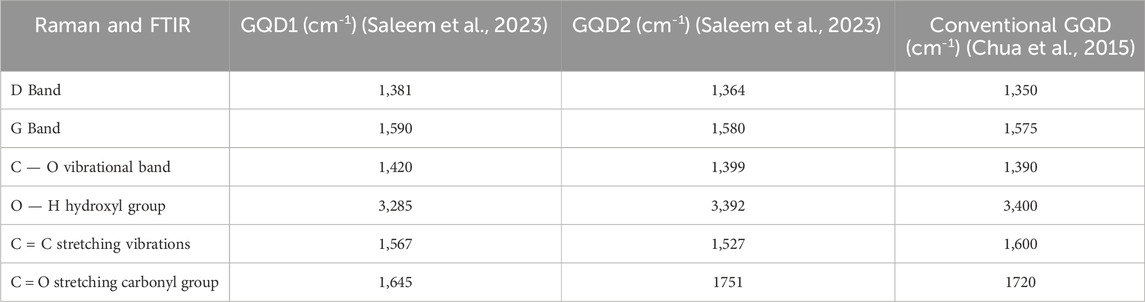
Table 5. Summary of Raman and FTIR results of conventional graphene quantum dots and date-palm graphene quantum dots.
On the other hand, transmission electron microscopy (TEM) results revealed that the size of GQD-1 ranges from 3.5 to 8 nm, while the size of GQD-2 ranges from 3.5 to 7 nm. Although GQD-1 and GQD-2 had approximately similar size, their size is higher than that of conventional graphene quantum dots synthesized from fullerene which comes in diameters between 2 and 3 nm. Kalita et al. (2016) used rice grains as a carbon source to create GQDs with diameters ranging from 2 to 6.5 nm. The quantum confinement effect caused a red shift in the photoluminescence emission spectra as the size of the GQDs increased from 2 to 6.5 nm. Reactant concentration, temperature, and reaction time all affect the size of GQDs, which in turn affects their characteristics. In the top-down technique in particular, the GQDs typically show a wide range of sizes. It is possible to regulate the size and shape of GQDs by altering the synthesis conditions (Zhao et al., 2024). Nevertheless, their size is < 20 nm which is the typical size of GQDs indicating their robust luminous characteristics that may be adjusted and high photoluminescence emissions (Gozali Balkanloo et al., 2023).
On the other hand, the FTIR spectroscopy data revealed the stretching vibrations of the hydroxyl group, C=C, carbonyl group, and C-O for both GQD-1 and GQD-2 prepared from date palm leaves (Figures 8e,f). Results confirm that carbonization has occurred during the hydrothermal reaction. The same peaks of the carbonyl group, C=C, hydroxyl group and C-O were detected in the FTIR analysis of the conventional GQD which indicates that both date palm GQDs and the conventional one has similar surface characteristics. A summary of FTIR results of conventional GQDs and date-palm GQDs is provided in Table 5.
3.3 Graphene from low-quality dates
Another kind of date palm waste that can be used to make worthwhile items is spoilt and low-quality dates. Utilizing low-grade dates and sand, graphene-coated sand was produced. The dates pulp was boiled for 20 min, filtered and the date juice mixture was centrifuged at 7,000 rpm for 30 min. The extract was concentrated under vacuum at 70°C and then mixed with the sand. Following a thorough mixing, the mixture was heated to 90°C in a hot air oven until the date liquid solidified on the surfaces of the sand. After drying, the product was pyrolyzed for 3 hours in an N2 carbonized furnace at 750°C. When the melting point of hardened date juice (185°C) was reached, the colour of the sample changed to dark brown and then transformed into carbon. After graphitization, ashes from the surface were removed through activation by strong H2SO4 and the final graphene-coated sand was produced (Abd Ali, 2019).
Similar to the above pyrolysis method used to produce graphene-coated sand, (Khan et al., 2019), generated another hybrid material made of graphene and sand (graphene sand hybrid). Nevertheless, the H2SO4 activation’s final step was not used. Sucrose and fructose molecules in the date syrup completely exfoliated to generate graphene nanosheets on the sand surface. The full exfoliation of the sucrose and fructose molecules in the date syrup produced graphene nanosheets on the sand surface. The date syrup and sand were mixed in a ratio of 2:5. After stirring for 1 h and drying at 80°C, the produced solid was burned at 750°C in a furnace under N2 flow. The mixture was first heated to 100°C at a rate of 2.5°C/min; after that, it was raised to 200°C at a rate of 3.3°C/min, until the dates reached their fusion point. Lastly, the temperature was raised to 750°C at a rate of 9.1°C/min, indicating the date juice’s sucrose and fructose molecules' transformation into carbon material. In a recent study, the impact of using date syrup-based graphene-coated sand hybrid (D-GSH) as a partial sand substitution and its potential to improve both fresh and hardened properties were investigated in 3D-printed cement composites. By reducing the fluctuation in double layer thickness from 38% to 28%, the addition of 0.5% D-GSH considerably enhanced the printing quality when compared to the control mix. The rheological characteristics improved flowability by demonstrating decreased viscosity and shear stress against shear rates (Ali et al., 2024).
The Energy-dispersive X-ray spectroscopy (EDS) results of sand and graphene-coated sand material prepared from low-quality dates demonstrated that carbon has the largest percentage, which is at 76.15%, confirming the graphitization of the sand surface. Moreover, the reduction in the percentage of silica from 52.3% in sand to only 0.9% in graphene-coated sand indicates that the surface of the sand has been covered with graphene. Also, there is 0.7% sulfur on the surface of graphene-coated sand which could be a result of the activation with H2SO4.
Graphene sand hybrid was investigated utilizing a Raman spectrometer and the results showed three peaks. The three peaks were the D band at 1,370 cm-1, the G band at 1,580 cm-1, and the 2D band at 2,760 cm-1. These peaks confirm that date syrup components have successfully converted to graphene carbon material (Khan et al., 2019). Only in graphene with more crystallinity or fewer imperfections is the 2D band clearly visible. As the degree of oxidation rises, the disorder in the material structure also rises. As a result, the intensity of the 2D bands gradually decreases and widens, resulting in their assignment to other bands (Tinh et al., 2023). Moreover, these values are very close to those of conventional graphene reported in (Mbayachi et al., 2021). Table 6 presents an overview of the Raman spectroscopy results for traditional graphene and graphene sand hybrid.

Table 6. Summary of Raman spectroscopy results of graphene materials produced from date palm waste and oil palm waste using sand and glass as supporting material.
On the other hand, the XRD results showed a distinct peak at around 23.2
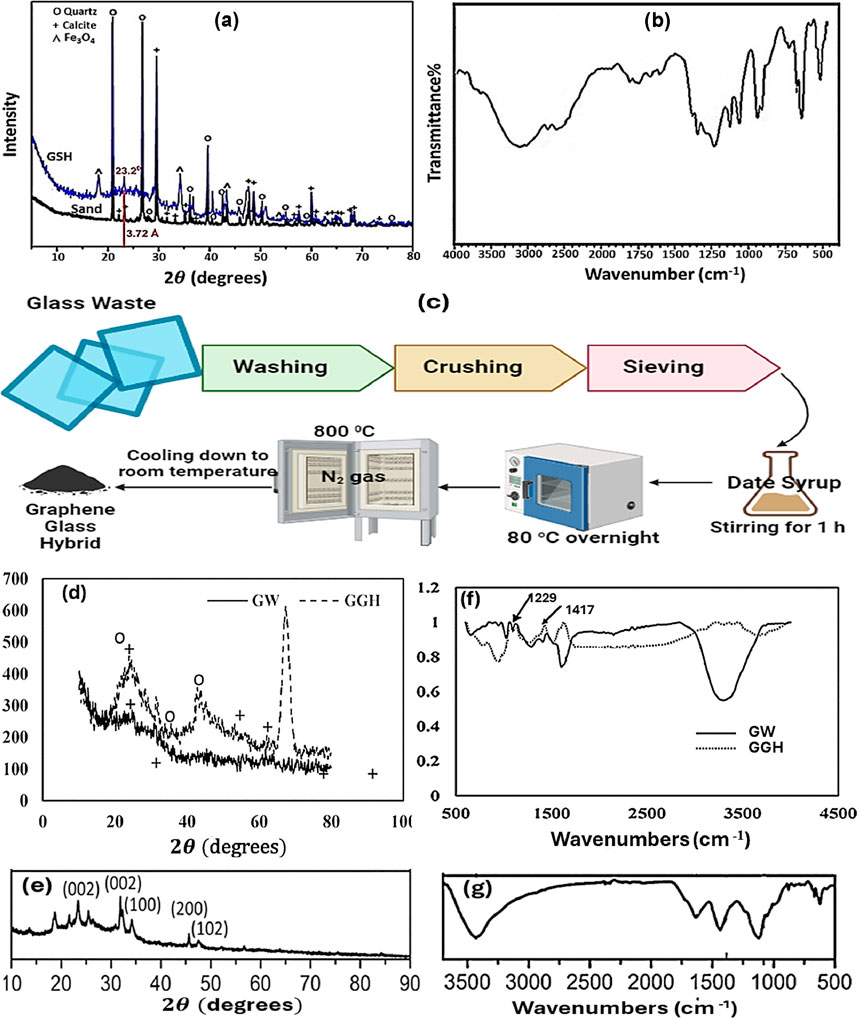
Figure 9. (a) XRD of sand and graphene sand hybrid (GSH) (Khan et al., 2019), (b) FTIR of graphene-coated sand (Reproduced with permission from Abd Ali, 2019 ©Taylor and Francis), (c) schematic representation of graphene glass hybrid synthesis process. XRD and FTIR of graphene materials containing glass as supporting material and produced from (d, f) date palm (Reproduced with permission from Graimed and Tark Abd Ali, 2022 ©Elsevier), and (e, g) oil palm (Reproduced with permission from Vasudevan et al., 2023 ©Elsevier), respectively.
FTIR measurements of graphene sand hybrid revealed peaks at 1796 and 1,451 cm-1 and they were ascribed to C=O stretching of the–COOH groups and C–H stretching vibrations. These findings provided proof that the sucrose and fructose particles completely graphitize on the sand surface, producing a carbonaceous substance with several oxygen functional groups. Conversely, the sucrose functional groups in the graphene-coated sand were represented by the adsorption bands at 1,346 cm-1, 2,721 cm-1, 2,937 cm-1, and 3,336 cm-1 and the graphitization of date syrup was confirmed by the peaks at 1,600 cm1, 1,685 cm-1, 1749 cm-1, and 3,525 cm-1 (Figure 9b).
Glass waste was utilized as supporting material and low-quality dates were used as a sustainable carbon source to create a graphene glass hybrid (Graimed and Tark Abd Ali, 2022). Date syrup was graphitized to create the graphene-glass hybrid through the pyrolysis process. The glass trash was collected, cleaned, sieved, and then added to the date syrup at the ratio of 2:5. After stirring for an hour and drying overnight at 80°C, the uniform solid mixture was loaded onto a ceramic boat and placed in a tube furnace for carbonization at 800°C (under the flow of 100 mL/min N2). Figure 9c shows a schematic of the synthesis of graphene glass hybrid. Glass was also employed by (Vasudevan et al., 2023) in the manufacture of graphene as a covering material for oil palm lignin solution. The lignin that was taken out of the empty fruit bunch of the oil palm was used to create graphene. First, 50 mL of distilled water and 10 g of lignin powder were combined, and the mixture was stirred until a uniform lignin solution was created. After coating a glass substrate with the uniform lignin solution, the substrate was placed in an oven at 50°C for 30 min to dry. Next, the dried lignin glass substrate was placed into a laser-scribed machine and after 30 min, a lignin-coated glass substrate was penetrated by a CO2 laser to generate graphene.
Defect quantification is essential for understanding the basic characteristics of graphene-related systems. Using Raman spectroscopy, significant efforts have been made to measure flaws and disorders. Since the area beneath each peak indicates the probability of the entire process, taking uncertainty into account, the area ratio should, in theory, always be considered for minor disorders or perturbations. However, decoupling the information at full width at half-maximum and peak intensity is significantly more revealing for a huge disorder. The intensity indicates the phonon modes or molecular vibrations participating in the most resonant Raman processes, whereas full width at half-maximum is a measure of structural disorder (Cançado et al., 2011). By measuring the intensity ratio of the D band to the G band (ID/IG ratio), Raman spectrum analysis enables the evaluation of disorder and flaws in carbon materials (Lee et al., 2021). Raman spectroscopy results of graphene glass hybrid (Graimed and Tark Abd Ali, 2022) showed two peaks at 1,110 cm-1 and 1,632 cm-1. The first peak is the defects and disorder D peak, and the second is the graphics G peak. These findings demonstrated that the graphene glass hybrid contains a significant amount of the sp2 graphene carbon material, which was produced by the conversion of the sucrose and fructose molecules in the date syrup. On the other hand, graphene produced from oil palm waste and supported by glass had values of D and G peaks closer to the ones reported for conventional graphene (Table 6). This can indicate that it has a similar structure and crystallinity to the conventional graphene more than the date palm graphene glass hybrid. The intensity ratio of graphene glass hybrid and oil palm graphene (with glass support) is 0.775 and 0.7, respectively indicating the existence of the same disorder degree. The ratio ID/IG rises with increasing graphene disorder because of elastic scattering brought on by increased defect intensity. Nonetheless, the more amorphous the carbon structure, the lower the ID/IG ratio (Mbayachi et al., 2021). An ID/IG ratio of 0.94 was reported by (Tinh et al., 2023) for graphite synthesized from the cellulose of corncob indicating the sp2 hybrid carbon structure; following reflux heating and synthesis using the improved Hummers' method, this ratio rose to 1.003 for GO sample, confirming that oxidation has increased the material’s degree of structural defects. Furthermore, rGO synthesized from sugarcane dry leaves showed an ID/IG ratio of 1.24. When compared to the G band, the D band’s intensity is higher, suggesting that there is more disordered phase in rGO (Thangaraj et al., 2024). The maximal value of ID/IG is approximately 13, and it denotes that the defect is associated with sp3 hybridization (Gupta et al., 2025).
According to X-ray diffraction results, the glass waste had peaks that most likely fit the distinctive quartz and silica diffraction patterns (symbols o and + in Figure 9d). Furthermore, graphene glass hybrid’s results revealed clear peaks at 24.4
Regarding the FTIR analysis of the graphene glass hybrid derived from date palm syrup (Figure 9f), the presence of quartz on the surface of graphene was confirmed by a bending that occurred at 1,229 cm-1 and was attributed to Si–O vibration. Additionally, a peak at 1,417 cm-1 was identified as the result of the vibratory vibrations' C-H expansion and the C-O distribution of the–COOH groups. The results indicated the complete graphitization of sucrose and fructose on the glass waste surface. On the other hand, the graphene prepared from oil palm waste with glass as supporting material showed peaks at 3,433.5 cm-1, 1722.5 cm-1, and 1,165.4 cm-1, which are assigned O-H, C=C, and C-O groups, respectively (Figure 9g).
The surface area of the graphene produced using dates syrup has a low surface area in comparison to the conventional graphene which has a reported surface area of 2,630 m2/g (Abdellah et al., 2023). This decrease in surface area is probably due to the coating of sand and glass. Regardless of this low surface area, these date palm graphene materials showed superior performance when applied in pollutant removal. However, the graphene-coated sand of (Abd Ali, 2019) showed a greater surface area (175 m2/g) than the graphene-sand hybrid of (Khan et al., 2019) and graphene glass hybrid of (Graimed and Tark Abd Ali, 2022). The reason for this increased surface area can be the activation of the produced graphene with H2SO4. This activation was not carried out in the case of the other graphene materials. The BET surface area of some biomass-derived graphene materials, and date-palm graphene materials in comparison to conventional graphene is summarized in Table 7. It can be observed that many biomass-derived graphene materials have surface areas that are significantly smaller than the theoretical value, thus, chemical activation using KOH, ZnCl2, and H3PO4 as well as physical activation using CO2 and steam is required to enhance surface area (Safian et al., 2021; Lin et al., 2021; Ekhlasi et al., 2018) reported an exceptional surface area of 1,317.1 m2/g for graphene material prepared from powdered Populus wood as a carbon precursor via carbonization and KOH activation under N2 gas at temperatures ranging from 750 to 950°C. Although similar synthesis procedure of carbonization and activation has been followed utilizing other different biomass sources, however, the surface area of the produced graphene materials was far less than the one produced from Populus wood. Thus, it can be hypothesized that the origin of the biomass source plays a significant role in determining the surface area of the produced graphene.
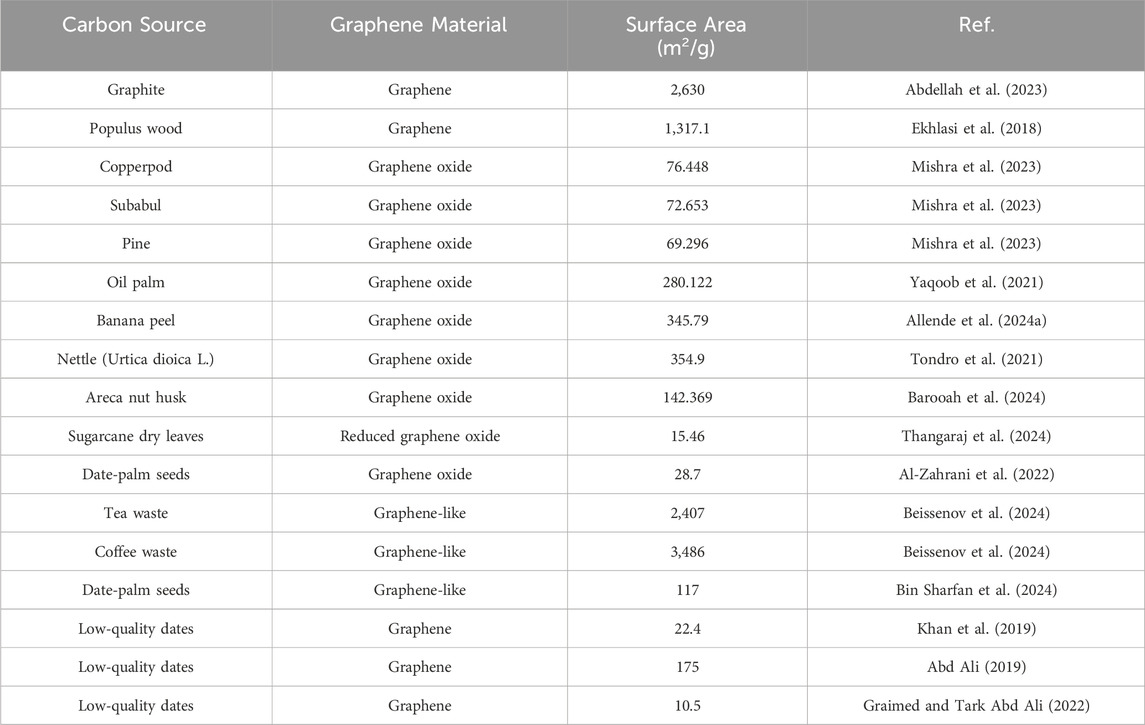
Table 7. Summary of surface area of biomass-derived graphene, date-palm-derived graphene and conventional graphene.
3.4 Graphene oxide-reducing agents from date-palm fruit
The chemical reduction technique is the most effective way to synthesize rGO quickly and on a large scale. However, there are many challenges in the process of turning graphitized carbon into rGO. The present chemical approach, however, uses hydrazine as a reducing agent which is a dangerous, poisonous, and corrosive chemical. As a result, the numerous applications of graphene will be limited because of the environmental impact and safety concerns (Amir Faiz et al., 2020).
Lately, efforts have been focused on reducing graphene oxide using ecologically friendly solutions. Additionally, applying these compounds to reduce graphene oxide yields graphene with a higher carbon content. The rich and varied makeup of natural plants includes polyphenols, terpene, alkaloids, tannins, polysaccharides, and other substances. During the reduction process, these ingredients function as antioxidants (Ousaleh et al., 2020). The production of rGO has involved the use of a number of natural sources as green reducing agents, such as green tea extract (Vatandost et al., 2020), sugarcane bagasse (Gan et al., 2019), Caesalpinia sappan L. (Destiarti et al., 2024) and oil palm leaves (Amir Faiz et al., 2020). Table 8 provides an overview of the various biomass sources, reduction techniques, and applications. In general, the green reduction steps of graphene oxide include the stirring, heating, refluxing and filtration of the plant to produce extract which is further used as a green reducing agent for graphene oxide.
Fruit from the date palm is abundant in active phytochemicals, including flavonoids, carotenoids, and phenolic acids. Phenolic acids and flavonoids isolated from dates were utilized as reducing and stabilizing agents. The date fruit extract containing flavonoids and phenolic acids has the unique ability to chelate metal ions, which promotes the reduction and stability of metal ions into nanoparticles or nanocomposites. Also, phenolic compounds have a large amount of -OH functional groups, which is important for the reduction process. Mo-ZnO/reduced graphene oxide nanocomposites (Mo-ZnO/RGO NCs) was prepared from sodium molybdate (Na2MoO4), zinc nitrate (Zn(NO3)2), and graphene oxide. Reduced graphene oxide was produced from graphene oxide using the bioactive phytochemicals of date fruit extract. Free-moving Zn2+ and Co3+ ions were released from Zn(NO3)2 and Na2MoO4 after dissolving in date fruit extract. These free-moving ions target the extract’s bioactive phenolic compounds' active sites to be stabilized and reduced into nano complexes. The Mo-ZnO/reduced graphene oxide nanocomposites were produced from the nano complexes through heat treatment (Ahamed et al., 2022). Diagram illustrating the use of date palm fruits as a reducing agent in the preparation of Mo-ZnO/rGO is shown in Figure 10a.
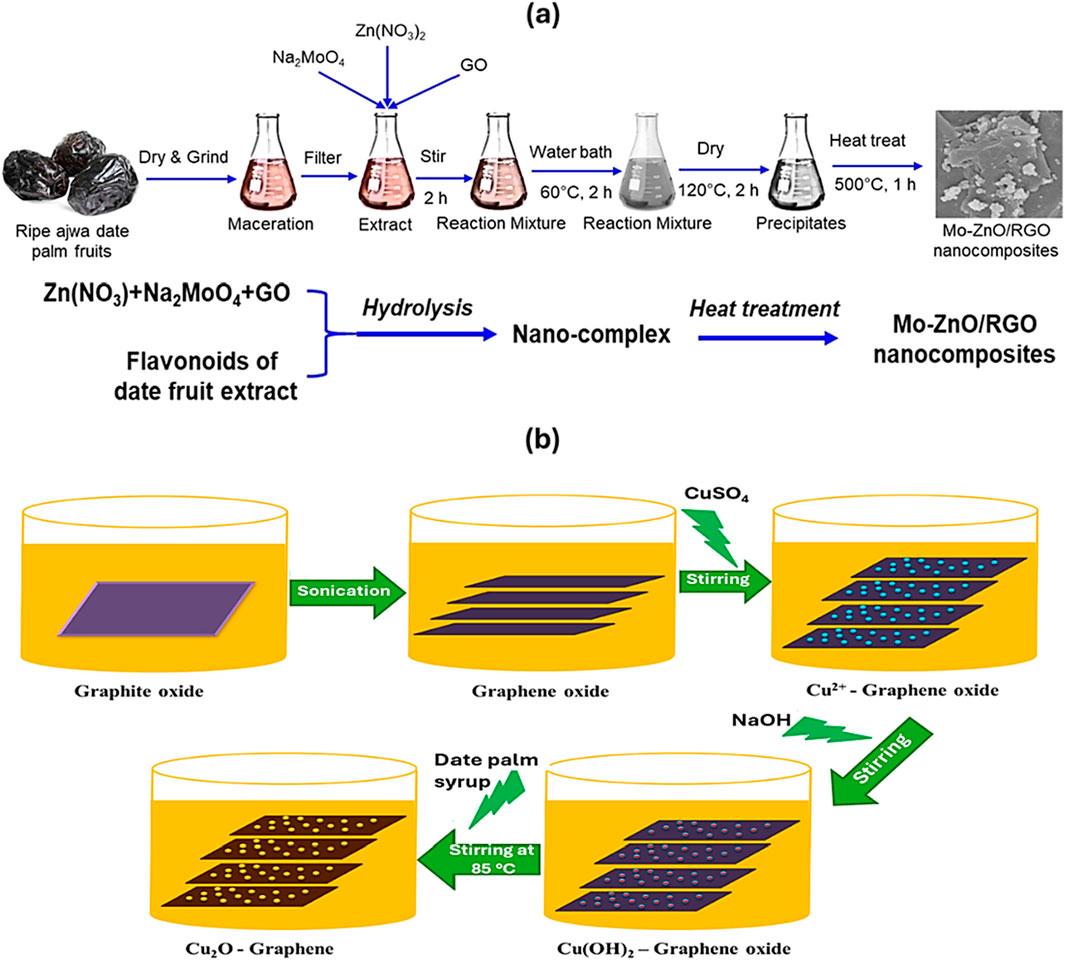
Figure 10. Schematic representation of using date palm fruits as a reducing agent in the preparation of (a) Mo-ZnO/RGO nanocomposite (Ahamed et al., 2022), and (b) Cu2O graphene nanocomposite (Reproduced with permission from Athinarayanan et al., 2018 ©Elsevier).
In another study, date fruit extract was used as a reducing agent to synthesize Cu2O/graphene nanocomposites (Athinarayanan et al., 2018). To synthesize Cu2O/graphene nanocomposites; Cu(OH)2 was used to anchor the graphene oxide surface, and date palm fruit syrup was used to reduce it. After creating the copper sulfate solution, it was stirred into the graphene oxide solution. After adding 5 mL of date palm syrup to the mixture, the mixture was incubated at 85°C. The liquid was then allowed to cool to ambient temperature, and the precipitate that formed was centrifuged, carefully cleaned, and dried out at 50°C (Figure 10b). Graphene oxide was successfully reduced to crystalline graphene, as evidenced by the XRD patterns of the Cu2O/G nanocomposite, which had a diffraction peak at 2θ = 22.2
4 Date palm-derived graphene applications
The use of graphene materials derived from carbonaceous wastes in fuel cells, solar cells, supercapacitors, electrochemical sensors, polymer composites, energy storage, and medical applications has been the subject of numerous investigations. A wide range of research and development disciplines are particularly interested in the potential applications of graphene nanoparticles. Excellent qualities and low cost make graphene-based nanomaterials ideal for use in photocatalysis, antimicrobial devices, sensors, and water treatment (Kumari and Samadder, 2022; Manikandan and Lee, 2023). Heavy metal adsorption in soil and water settings is one use for graphene derived from agricultural waste. Oil-water separation, sensors to identify specific trace substances, and the production of fuel cells and supercapacitor electrode materials are also amongst the applications of graphene derived from agricultural waste (Li L. et al., 2024). Additionally, biomedical applications like bioimaging, biosensing, tissue engineering, medication delivery, and antibacterial agents have made use of graphene derived from biomass (Figure 11a) (Blessy Rebecca et al., 2022).
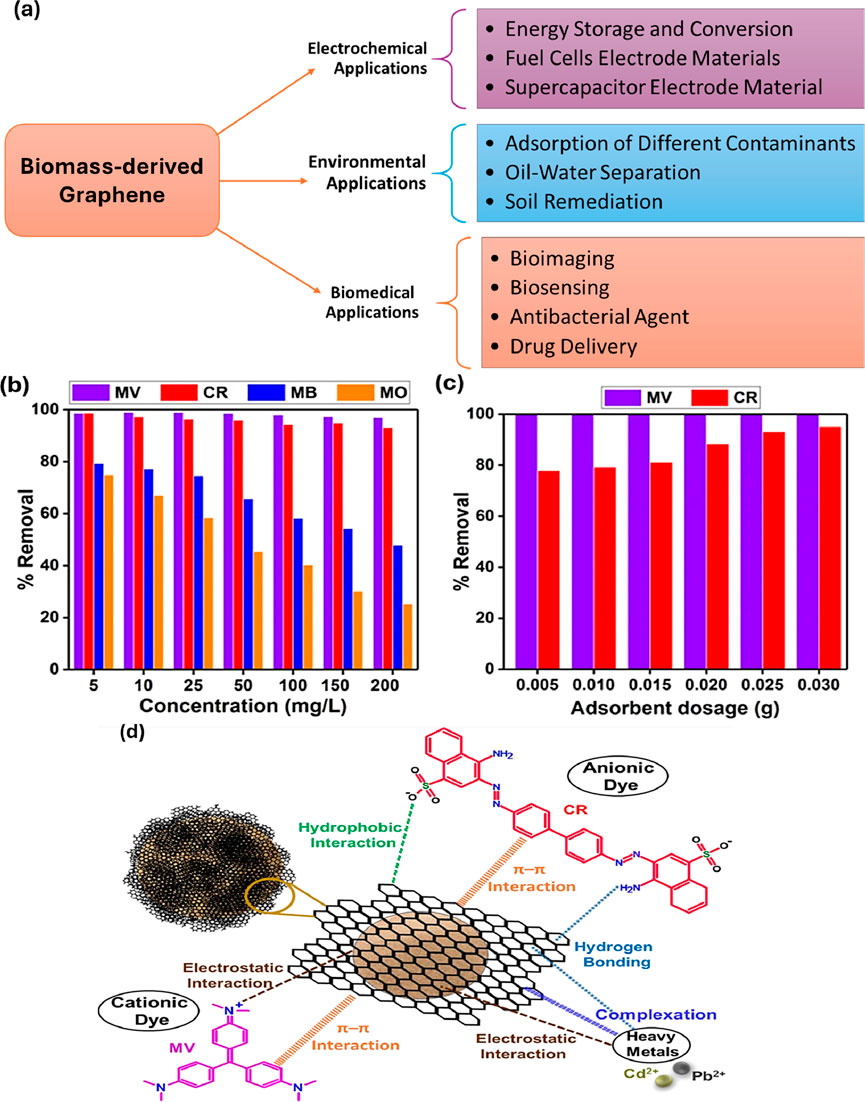
Figure 11. (a) Applications of biomass-derived graphene in different areas. Effect of (b) concentration and (c) dosage on the adsorption of dyes onto graphene sand hybrid prepared from low-quality dates syrup, and (d) adsorption mechanism onto the graphene sand hybrid (Khan et al., 2019).
Contaminants such as organic pollutants, and heavy metal ions pose a major threat to soil, water, and groundwater pollutants and it is essential to remove them. Because of their large surface area and strong pollutant interactions, which subsequently increase adsorption effectiveness, carbon composites generated from biomass showed a lot of promise (Bezazi et al., 2024). The carbon materials function as adsorbents to move ion pollutants in water from the liquid phase to the solid phase, following the theory of phase transition (Avornyo and Chrysikopoulos, 2024). The principal pollutants found in industrial effluents include different heavy metal ions, various dyes, insecticides, herbicides, and fungicides. These compounds are all carcinogenic, mutagenic, and non-biodegradable (Saini et al., 2024). The graphene obtained from date palms has been used to remove a variety of contaminants (both organic and inorganic) from contaminated water.
4.1 Organic pollutants removal
Dyes, antibiotics, and petroleum hydrocarbons are examples of organic contaminants that are extremely harmful to both human health and aquatic organism life. For instance, due to their strong coloring power, low biodegradability, and high carcinogenicity, organic dyes dumped straight into fresh water might alter human cells through the food chain and harm aquatic organisms' immune systems (Qu et al., 2023). However, antibiotics originating from factories, homes, hospital discharges, livestock farming, and runoff from agricultural and aquaculture locations can typically lead to the creation of antibiotic-resistant genes and bacteria, which is extremely dangerous for human health as well as the health of other living things (Fayaz et al., 2024). Graphene-based adsorbents have recently studied for the treatment of wastewater. Graphene is a graphite single-atomic layer with a unique two-dimensional (2D) structure that allows the adsorption process to occur on both sides of flake sheets (Azam et al., 2022; Rezania et al., 2022).
The graphene sand hybrid developed by (Khan et al., 2019) from low-quality dates syrup was investigated on a series of cationic and anionic dye solutions including methyl violet (MV), Congo red (CR), methylene blue (MB) and methyl orange (MO) with initial concentrations ranging from 5 to 200 mg/L using 25 mg of the adsorbent for 4 h. Figure 11b shows that graphene sand hybrid was able to remove more than 90% of MV and CR in the whole range of initial concentrations. However, the removal of MB and MO was only 75% and 57%, respectively at initial concentration 25 mg/L and as concentration increased, the removal percentage decreased. The effect of adsorbent dosage on the removal of CR and MV is shown in Figure 11c. It is clearly seen that increasing the dosage of graphene sand hybrid had negligible effect on MV adsorption. However, for CR, the removal percentage increased with the increase in adsorbent dosage and the highest removal efficiency of 93% was reported at a dose of 25 mg. Further increase in dosage above 25 mg showed no effect on removal efficiency, and this was attributed to the agglomeration of the adsorbent particles. Results showed that the graphene sand hybrid had a strong ability to adsorb MV dye and CR dye with adsorption capacities of 2,564 mg/g and 333 mg/g, respectively. The excellent adsorption performance of graphene sand hybrid was attributed to the fact that graphene’s layered interlamination is opened by wrapping it around sand, revealing the potent adsorption sites found in the interlayers. Moreover, the hydrophilic sand surface turns hydrophobic, negatively charged, and has π-electron conjugated structure when a hydrophobic graphene sheet with sp2 hybridized structure is present on it. The anionic graphene sand hybrid demonstrated high extraction efficiencies for cationic dye pollutants like MV because of its strongly negatively charged surface. In addition to electrostatic interaction, π-π bonding was also a significant factor in dye adsorption (Figure 11d). The Congo red dye adsorption capacity using graphene sand hybrid material is slightly higher than the adsorption capacity of Congo red dye using graphene synthesized from rubber seed shells (Nizam et al., 2022). According to this study, functional groups like hydroxyl and carboxylic have a major impact on the process via which dye molecules connect with the graphene oxide surface through π-π interaction and electrostatic contact. Regardless, the adsorption equilibrium data of Congo red adsorption using both adsorbents were fitted by Langmuir model, while the kinetic data were fitted by the pseudo-second order kinetic model. Teow et al. (2020) created graphene shell composite, which was utilized to remove methylene blue (MB), utilizing oil palm kernel shell as the basis material and oil palm frond juice as the natural carbon source. They reported that after 20 h, the removal was more than 80% for a range of different initial concentrations. Although, this percentage is higher when compared to date palm-derived graphene sand hybrid, the contact time of 20 h is 5 times more than the contact time in the case of date palm-derived graphene sand hybrid which was only 4 h.
Graphene oxide produced from raw date seeds was utilized in the adsorption of the insoluble phenothiazine-derived dye. The adsorption capacity was 4.88 mg/g at optimum conditions of 11 mg/L initial dye concentration, pH 9, and contact time of 30 min (Al-Zahrani et al., 2022). On the other hand, the graphene glass hybrid prepared from the syrup of dates with low quality was utilized as a reactive substance in permeable barrier technology to treat groundwater contaminated with tetracycline (Graimed and Tark Abd Ali, 2022). The Langmuir model was used to fit the equilibrium data, the maximum adsorption capacity was determined to be 17.122 mg/g, and the kinetic data were fitted by the pseudo-second order kinetic model. Optimum adsorption conditions, equilibrium, kinetics, and thermodynamics of different organic pollutants using date palm-derived graphene materials are summarized in Table 9. To improve graphene’s potential for water treatment, a composite formed of graphene functionalized with ferrocene was produced using byproducts of palm tree as a carbon source (El-Maghrabi et al., 2022). In batch adsorption tests, several parameters such as pH (Figure 12a), time (Figure 12b), adsorbent dose (Figure 12c) and starting concentration (Figure 12d) were tested to determine the best conditions for removing PO4−3 from wastewater. The best adsorption capacity was found to be 58.93 mg/g when graphene produced from palm fibers was used at an initial concentration of 15 mg/L, pH = 3, dose of 10 mg, and contact duration of 60 min. The data derived from the pH effect confirmed that the phosphate ions adsorption mechanism is ion exchange (Figure 12e). This was ascribed to the rise in pH associated with an increase in hydroxyl ions, which lowered the removal % by competing with phosphate ions for the active sites on the graphene surface. Because the Langmuir model fits the adsorption process, this interpretation is also consistent with the results of the adsorption isotherm.
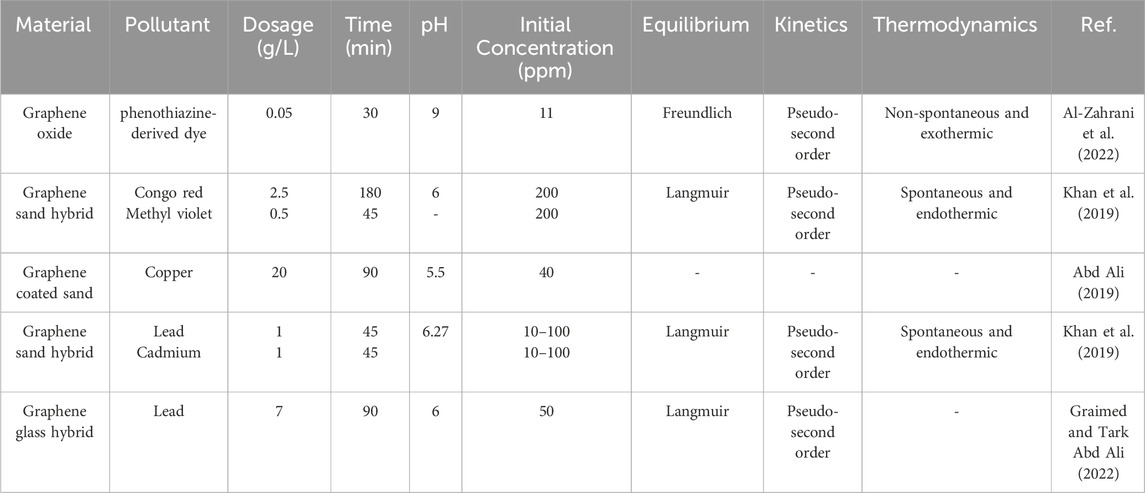
Table 9. Optimum adsorption conditions, equilibrium, kinetics, and thermodynamics of different organic and inorganic pollutants using graphene materials derived from low-quality dates syrup.
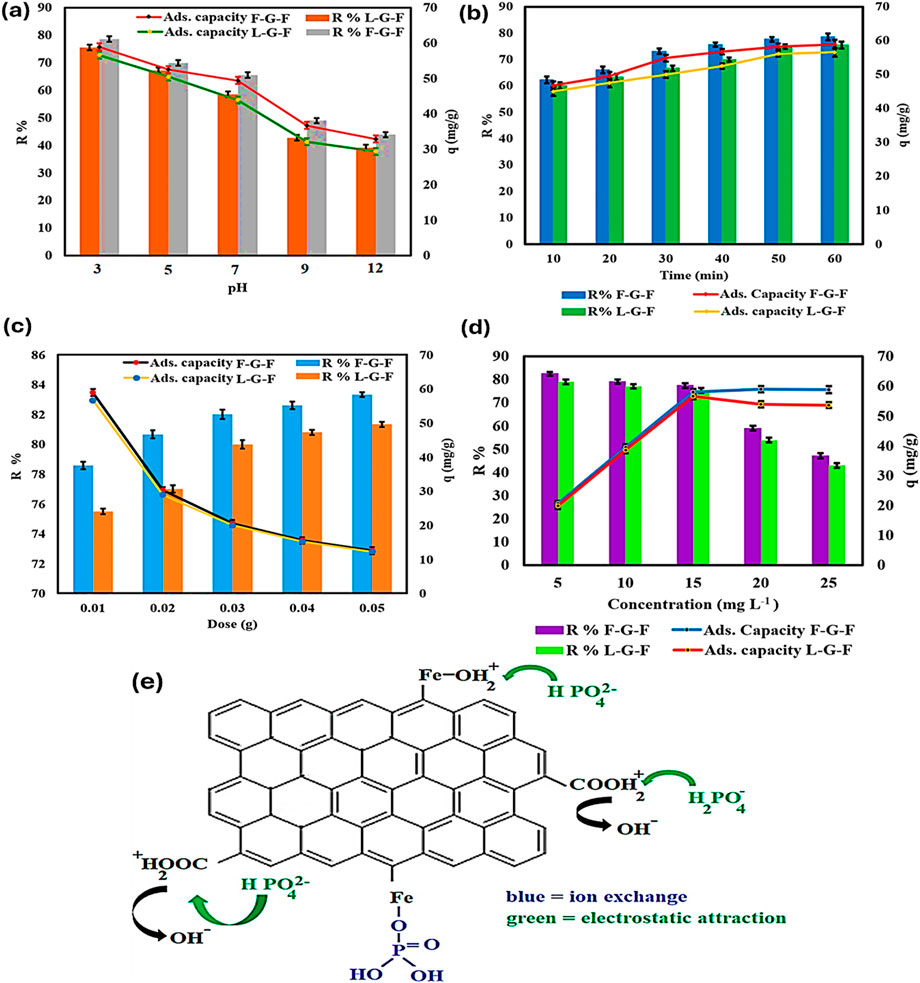
Figure 12. Effect of (a) pH, (b) time, (c) dose and (d) concentration on the adsorption of phosphate onto ferrocene-functionalized graphene prepared from palm fibres (F-G-F) and palm leaves (L-G-F), and (e) adsorption mechanism onto the ferrocene-functionalized graphene (El-Maghrabi et al., 2022).
Adsorbent materials must be able to be successfully regenerated and reused for several treatment cycles in order to be economically viable. The optimal adsorbent regeneration process should remove impurities and competing species such as dissolved organic matter and background ions, preserve the original surface functional groups and structure, and allow for additional adsorption-desorption cycles without requiring the replacement of media (Vakili et al., 2024). Through the use of several solvents, including acetone, HNO3, and NaOH, the reusability of graphene sand hybrid prepared from low-quality dates syrup was conducted. A 0.1 M NaOH was able to effectively desorb methyl violet where there was no discernible decrease in adsorption capacity even after three cycles (Figure 13a). When Congo red-adsorbed graphene sand hybrid was regenerated using acetone and NaOH, a similar pattern was observed (Figure 13b) (Khan et al., 2019). On the other, GO derived from date palm seeds which was used for the adsorption of phenothiazine-derived dye was regenerated through rinsing with deionized water and employed in the following adsorption experiment. 90% of the regenerated GO’s capacity was preserved after four adsorption cycles (Figure 13c) (Al-Zahrani et al., 2022). The reusability of ferrocene-functionalized graphene derived from palm fibres (F-G-F) and palm leaves (L-G-F) in the adsorption of phosphate were also investigated and results showed significant decrease in the removal percentage after four consecutive adsorption/desorption cycles (Figure 13d) (El-Maghrabi et al., 2022).
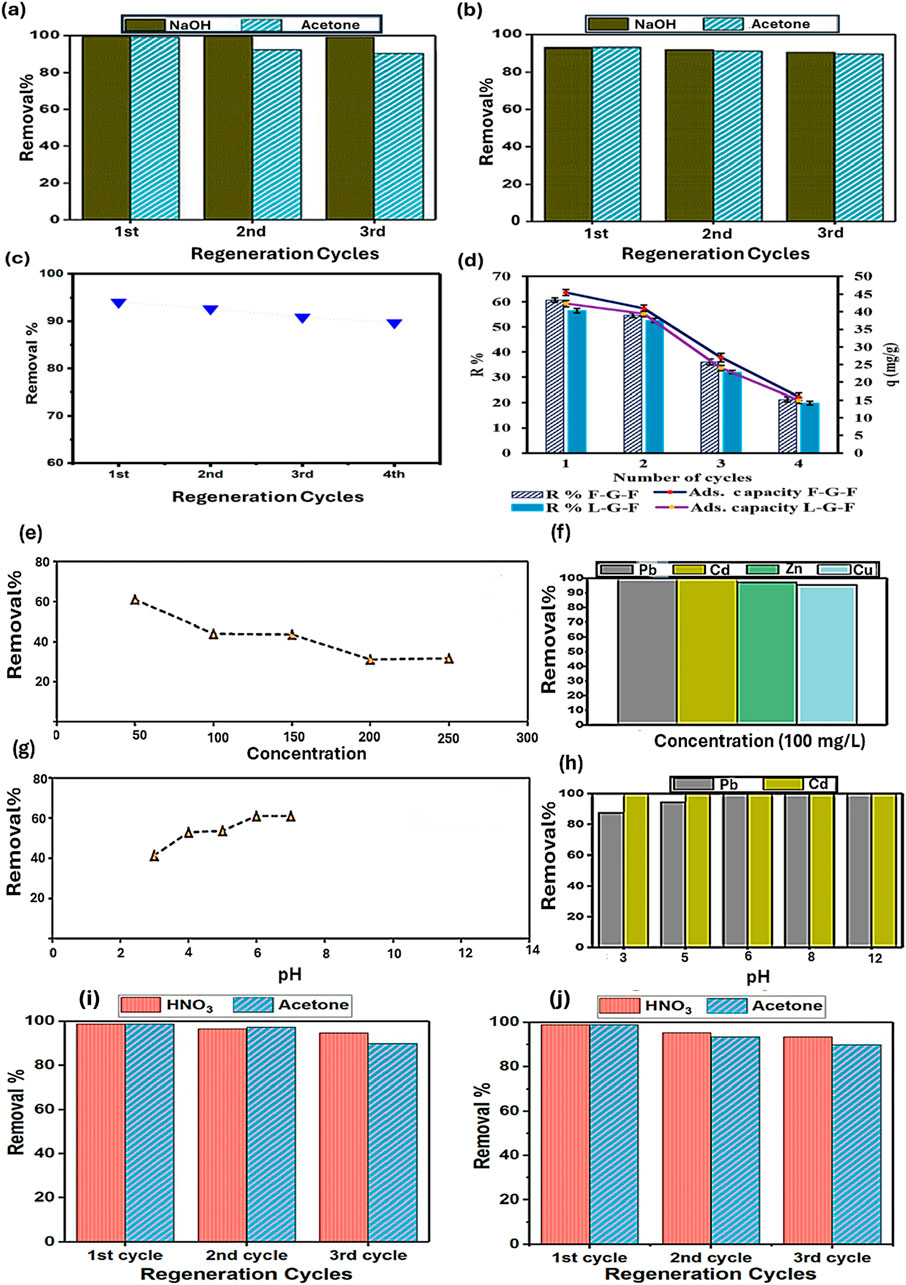
Figure 13. Regeneration effect on the adsorption of (a) methyl violet and (b) Congo red on graphene sand hybrid derived from low-quality dates syrup, (c) phenothiazine-derived dye on GO derived from dates seeds (Al-Zahrani et al., 2022), (d) phosphate on ferrocene-functionalized graphene derived from palm fibres (F-G-F) and palm leaves (L-G-F) (El-Maghrabi et al., 2022). Effect of: (e, f) initial concentration, (g, h) pH on the adsorption of lead onto graphene glass hybrid prepared from low-quality dates syrup (Reproduced with permission from Graimed and Tark Abd Ali, 2022 ©Elsevier) and graphene sand hybrid prepared from low-quality dates syrup, respectively. Regeneration effect on the adsorption of (i) lead and (j) cadmium on graphene sand hybrid prepared from low-quality date syrup (Khan et al., 2019).
4.2 Inorganic pollutants removal
Inorganic pollutants have the potential to seriously harm both human health and the health of all living creatures. Inorganic pollutants, such as lead, mercury, arsenic, zinc, and other heavy metals, can have harmful impacts on the environment and public health if their concentration rises above the World Health Organization’s (WHO) recommended threshold (Al-Raad and Hanafiah, 2021). Overexposure of biological organisms to heavy metals can damage blood compositions, livers, kidneys, lungs, and other vital organs in addition to having certain negative impacts on mental and central nervous system functions (Yu et al., 2024). Graphene-coated sand prepared by (Abd Ali, 2019) - employing syrup made from dates with low quality - was used in a permeable reactor barrier for the removal of copper from contaminated groundwater. At pH = 5.5, 2 g of graphene-coated sand was able to remove the copper ions from 100 mL solutions with concentrations ranging from 5 to 100 mg/L with a maximum removal efficiency of 90%. It was observed that graphene-coated sand has the ability to remove the copper ions despite having a smaller surface area (175 m2/g) than other sorbents. The presence of graphene sheets on sand surfaces offers multiple active sites for adsorption and enables a high percentage of copper removal. Oil palm leaves were utilized to synthesize graphene oxide using the catalytic acid spray method by aqueous acid exfoliation of cellulose in the presence of Co silicate as a catalyst (Fathy et al., 2019). The produced graphene oxide was used as an adsorbent for copper ions and the optimum conditions were 2.22 g/L adsorbent dosage, initial pH of 6 and initial concentration of 259 mg/L. Optimum adsorption conditions, equilibrium, kinetics, and thermodynamics of different inorganic pollutants using date palm-derived graphene materials are summarized in Table 9. The graphene glass hybrid prepared by (Graimed and Tark Abd Ali, 2022) from the syrup of low-quality dates was also used as a reactive material in permeable barrier technology to treat groundwater contaminated with lead. The maximum adsorption capacity was found to be 38.639 mg/g.
On the other hand, the graphene sand hybrid material demonstrated exceptional adsorption capacity and regeneration ability for heavy metal pollutants. Lead ions and cadmium ions had adsorption capacities of 781, and 793 mg/g at 25°C, respectively. A comparative overview of the performance of different biomass graphene materials in the removal of different organic and inorganic pollutants is provided in Table 10. It was reported that only 10 mg of date palm-derived graphene sand hybrid was able to completely remove both Pb and Cd ions regardless of the initial metal concentration. While the removal of Cd ions remained constant regardless of the starting solution pH, the Pb adsorption increased with increasing pH which suggested that the ion-chelating negatively charged functional groups on the surface of date palm-derived graphene sand hybrid play a major role in the removal of heavy metals (Khan et al., 2019). A similar relationship with pH was also reported for the adsorption of Pb ions using date palm-derived graphene glass hybrid. The effect of initial concentration and pH on the adsorption process using graphene glass hybrid and graphene sand hybrid is shown in Figures 13e–h. The Pb adsorption capacity using date palm-derived graphene sand hybrid is almost 20 times the Pb adsorption capacity using date palm-derived graphene glass hybrid. This can be attributed to the fact that the surface area of the graphene sand hybrid is almost twice the surface area of the graphene glass hybrid. Regarding the regeneration of heavy metals-loaded graphene sand hybrid, using 0.1 M HNO3 resulted in less than 5% drop in the adsorption capacity after the third cycle, while using acetone resulted in less than 10% drop in the adsorption capacity after the third cycle (Figures 13i,j).
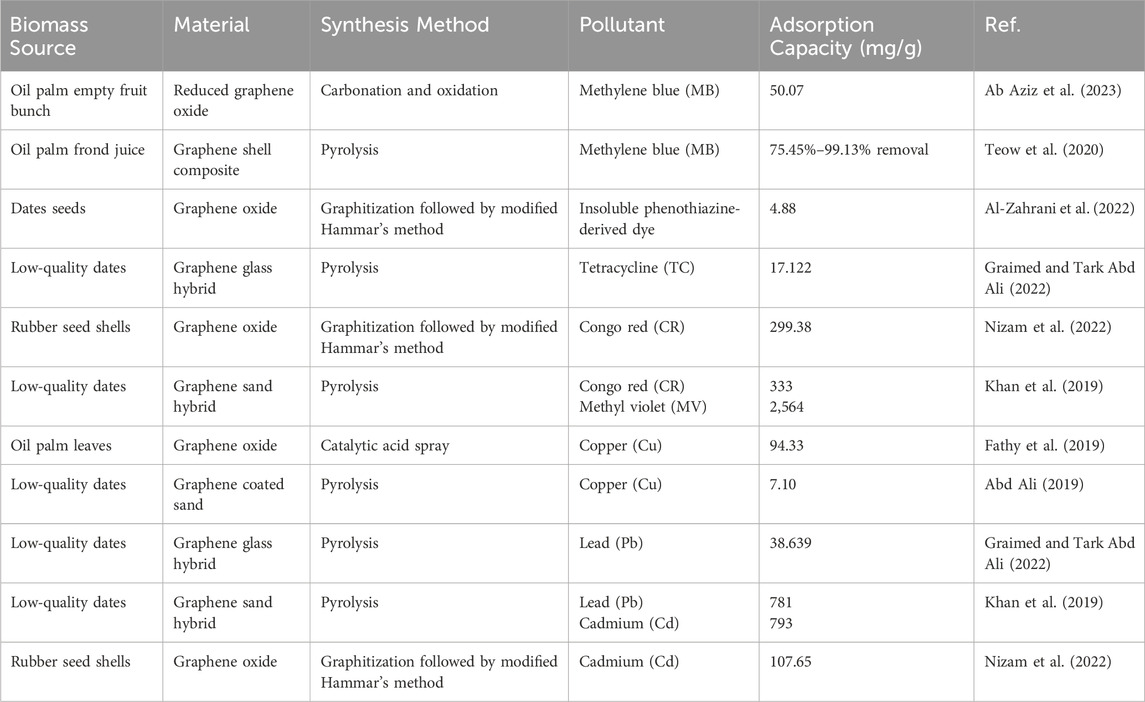
Table 10. Comparison of the adsorption performance of biomass graphene materials for different pollutants.
5 Environmental fate and economic assessment
5.1 Toxicity and environmental fate
After being released, graphene materials eventually find their way into the environment, with the three most crucial systems being the air, soil, and water. Graphene materials will undergo a number of physical, chemical, and biological changes as a result of their interactions with various environmental media, such as ions, dissolved organic matter, UV light, and microbes. These changes will impact their biological impacts. The change of graphene materials in water, which primarily comprises colloidal behavior and chemical transformation brought on by sunlight, chemicals, and microbes, has drawn the greatest attention because the aquatic environment is the primary recipient of graphene (Ding et al., 2022).
According to earlier research, GO is cytotoxic to human red blood cells, mammalian cells, erythrocytes, and skin fibroblasts in a dose-and size-dependent manner. The toxicity of 50 μg/mL GO to human fibroblast cells was shown to be evident. Extensive research on the colloidal properties of graphene-related nanomaterials, which are crucial to their application, reactivity, destiny, transport, bioavailability, and toxicity, has been spurred by worries about the possible detrimental effects on human health, the environment, and the ecosystem (Gao et al., 2019). GO’s strong hydrophilicity and water mobility, which are among its greatest benefits, can easily become an ecological problem. According to earlier reports, elements belonging to the graphene family have a significant propensity to collect in living things' bodies and have an impact on the aquatic ecosystem’s food chain. Given its extensive interactions with aquatic creatures, GO is likely to exhibit similar behavior. Understanding GO’s possible harmful mechanism against aquatic creatures is crucial to addressing concerns about its ecotoxicity and fate in aquatic environments. However, there has not been much research done on how GO interacts with species in watery environments (Malina et al., 2020). Conversely, multiple studies have indicated that GQDs derived from various biomass sources exhibit reduced toxicity. This observation extended to GQDs synthesized from date palm leaves. Generally, GQDs are considered non-toxic, environmentally benign, and biologically inert materials when compared to GO (Saleem et al., 2023).
While date-palm graphene materials offer potential green chemistry-aligned and environmentally friendly solutions in terms of sustainable raw materials, green synthesis, and cost-efficiency, the extensive ongoing research and development aimed at fully realizing their potential in real-world applications by improving their characteristics to match those of conventional graphene may lead to increased toxicity. The physiochemical characteristics of graphene materials, such as size, shape, oxidation level, dispersion quality, and surface area, determine their toxicity (Goodwin et al., 2018; Koyyada and Orsu, 2020). For instance, (Taşdemir et al., 2023), studied the cytotoxic and genotoxic effects of two graphene materials with two different surface areas (150 and 750 m2/g) and proved that cell damage increased with higher surface area. It was observed that the developed date palm-derived graphene materials have surface areas that are significantly smaller than the conventional graphene, thus, their toxicity is expected to be far less than the conventional graphene. Therefore, the lower surface area of date palm graphene can provide a positive impact in enhancing its environmental fate. Regardless, the great adsorption capacity of graphene for persistent organic pollutants (POPs), can lead to Trojan horse effect which is a phenomena whereby organic contaminants accumulate on the nanomaterial’s surface as a result of this adsorption, potentially increasing their uptake by aquatic species and its negative impacts (González-Soto et al., 2023). Despite the lower surface area of date palm graphene materials compared to conventional ones, they exhibited superior adsorption capacities, particularly for organic pollutants, which may render them susceptible to developing a Trojan horse effect when released into aquatic environments. Graphene properties affecting its toxicity as well as possible interactions with various environmental media are presented in Figure 14.
An issue of great importance is the biodegradation of graphene materials. Even though graphene-based materials have been the subject of some research in this area, it is essential to describe the structural features of the various materials employed in each biodegradation study. Recent research has unequivocally demonstrated that immune cells are capable of carrying out the intracellular and extracellular destruction of GO. To better understand the potential dangers of long-term bio-persistence of such materials and to make them safer by design, more study is needed on the degradation of other graphene-based materials with defined features using pertinent in vitro and in vivo models (Fadeel et al., 2018). In summary, concerns about potential environmental costs arise from the increasing use of graphene-based products. To detect possible repercussions, assessing the life cycle environmental impacts of applications from current and anticipated graphene technology advancements is necessary. However, environmental impact assessment models have not been developed. Therefore, the environmental fate of biomass-derived graphene and its applications during usage and final disposal stages should receive more attention from researchers (Li X. et al., 2023).
5.2 Economic assessment
Compared to the other nanomaterials, graphene production is higher, and it is predicted to keep increasing as the costly processes currently employed to produce graphene are improved. Accordingly, it is anticipated that by 2027, 3,800 tons of graphene will be produced, valued at $300 million (González-Soto et al., 2023). The process of producing graphene is costly, and complicated. However, the utilization of far less costly biomass as a carbon source has solved this problem. A variety of biomass sources can be effectively used to produce graphene and nanoporous carbons. Additionally, controlling the textural characteristics and the kind and amount of functional groups on the surface is made possible by thorough research into the synthesis parameters of carbons from biomass wastes (Urzúa et al., 2024).
The process of large-scale production involves many economic considerations, including the need to obtain large quantities of starting materials at low cost. The source of graphite is a crucial factor because it will impact not only the operation’s cost but also the quality of the final product and the necessary subsequent processing steps (Lowe et al., 2016). Locally available biomass sources can provide a low-cost and eco-friendly raw material for graphene production. Research studies employing date palm components for the synthesis of graphene materials exhibit a limitation of not studying the cost-effectiveness in comparison to conventional graphene materials. However, the cost analysis of graphene synthesis from other biomass sources can be considered a representative initial cost estimation for the production of date palm graphene materials. Nevertheless, the pretreatment required for the biomass raw material may vary depending on the type of biomass source.
Detailed cost analysis for large scale production was provided for graphene production from populus wood biomass following carbonization and activation processes where it was estimated that the production cost of each 1 kg of graphene is almost $25 (Ekhlasi et al., 2018). Commercial graphene exhibited higher prices ranging from $30 to $120 per kg (Ban et al., 2024). For graphene production from populus wood biomass with guaranteed viability, the cost analysis considered an underestimated yield of 17%, 300 days a year, and a staff of five employees each shift for 24 h a day. According to the results, 1 kg of graphene required an average of 81 MJ of energy to generate. About 5.25, 6.13, and 40% of energy is used in the crushing of biomass residue, ball milling of the crushed biomass to a fine powder, and carbonization of the powdered populus wood, respectively. Furthermore, 0.033 kg of N2 is needed for each 1 kg of populus wood, and about 12 kg of HCl (10 wt. %) and 5.6 kg of KOH are needed for every 1 kg of graphene sample that is produced.
Regarding GO costs, it was revealed that the total cost of synthesizing GO on a laboratory scale from 3 g of graphite flakes and 3 g of graphite from nettle (Urtica dioica L.) was estimated to be $4.57 $ and $5.60 per gram, respectively, producing 5.5 and 4 g of products (Tondro et al., 2021). By synthesizing high-purity graphite with a carbon concentration of >99.9% from easily accessible lignocellulosic biomass, including nettle (Urtica dioica L.), the production yield of GO from nettle can be further enhanced. However, the filtration methods', significant energy consumption and acid release in wastewater have raised serious environmental concerns. On the other hand, the cost for producing 0.3 g of GO obtained from corncob was found to be $25.20. In the synthesis of GO from corncob, cellulose was initially isolated and subsequently utilized for graphite synthesis. The extraction of cellulose necessitated additional chemical reagents such as NaOH and NaClO2, which incurred additional costs. Therefore, extra costs may be applied to GO produced from date palm seeds as it initially required the extraction of lignin. Concerning the commercial GO, (Donato et al., 2023), reported the absence of standardization in GO’s pricing as well as the lack of information regarding the synthesis methodology employed by the majority of GO producers. A comparative analysis of 34 distinct commercially available GO samples from 25 companies across 11 countries revealed prices ranging from $0.40 to $2,300 per gram. This substantial price variation presents a significant challenge in conducting meaningful comparative cost-efficiency analyses between commercial and biomass-derived graphene.
On the other hand, non-conventional synthesis methods showed promising results in decreasing graphene production costs. Using coal as a carbon source, flash Joule heating costs $130 to $135 per ton which is equivalent to only $0.13–$0.135 per kg. It can handle a variety of waste materials without the need for solvents or catalysts, and it only produces roughly 10 kg of carbon dioxide for every kg of graphene, as opposed to 400–500 kg released from conventional synthesis (Hosny et al., 2025). Although flash Joule heating has not been employed in the synthesis of graphene from date palm, it can provide a scalable and economical approach compared to conventional synthesis methods. In another research, GO produced from banana peel employing microwave pyrolysis method was found to be $14 less expensive than the market price for producing 1 kg of GO (Allende et al., 2024b). Therefore, it is advisable to explore alternative synthesis methods, such as flash Joule heating and microwave-assisted pyrolysis, in the field of date palm graphene synthesis. Furthermore, a comparative cost analysis of date palm graphene in relation to conventional graphene is necessary, as the majority of studies on biomass-derived graphene focus primarily on synthesis and characterization, neglecting cost analysis and life cycle assessment, despite these being crucial factors for practical applications.
6 Conclusions and future prospects
Date palm trees are one of the trees that produce significant amounts of waste annually. This waste is usually burned; although it is carbon-rich and can be converted into valuable products. This novel review paper has comprehensively summarized the advances in the synthesis and reduction of graphene materials using different types of date palm waste. Different types of date palm waste such as seeds, leaves and low-quality dates were utilized in different studies to produce graphene materials. Although graphene materials produced from low-quality dates had low surface area when compared to conventional graphene; they showed an exceptional performance in the removal of some pollutants from contaminated water. Date palm fruit was also used to prepare extracts to serve as a green reducing agent for graphene oxide. Although this extract can avoid the usage of harmful and expensive chemicals; this trend does not solve the environmental issue of date palm waste as the fruit part is consumed by humans and is considered an important source of nutrients. Therefore, the applicability of spoiled date fruit or other parts of the date palm tree which are considered as a waste in graphene reduction should be investigated. The research of date palm-derived graphene is still in its early stages. However, the urgent need to find sustainable routes for graphene production will lead to growth in this new aspect of research. Several challenges and future research directions are pointed out below for date palm-derived graphene materials and shown in Figure 15 to help researchers in advancing more novel synthesis routes and diverse applications.
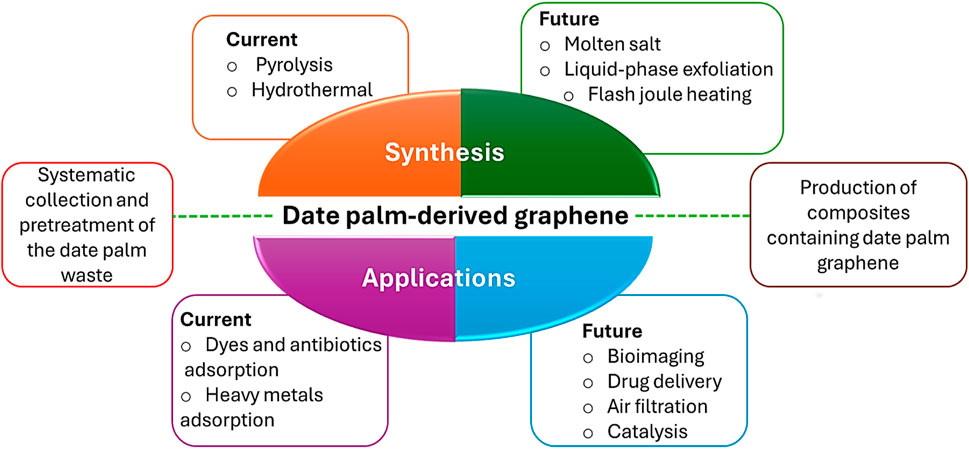
Figure 15. Summary of the current and recommended future prospectives in the synthesis and applications of date palm-derived graphene materials.
There is a challenge related to the systematic collection and classification of the raw material itself which is the date palm waste. Although this waste is abundantly available; it is very important to have a standard specification for the collected raw material. The pre-treatment of the collected waste is also important because agricultural wastes in general contain unwanted impurities. Regarding the synthesis methods, only pyrolysis and hydrothermal techniques have been investigated in the production of graphene from date palm waste. Thus, other synthesis methods such as molten salt, liquid-phase exfoliation and flash Joule heating should be investigated, and the quality of graphene produced from each method should be compared. Additionally, the synthesis conditions need to be optimized to get a stable and reproducible product which is required for the control of the structure and properties.
Date palm-derived graphene materials have immense possibilities for applications in different areas. For instance, the graphene-like material derived from date palm seeds demonstrated porous structure and high surface area, which make it a good candidate in applications where porous structure and high surface area are required. Furthermore, the GQD prepared from date palm leaves showed biocompatibility with minimum probability of cellular toxicity which gives them a great potential for biomedicine applications. Finally, the production of composites consisting of date palm graphene along with conventional graphene or other chemically produced materials should also be investigated. Such composite can have excellent characteristics and performance in different applications as well as lower production costs and more environment-friendly synthesis procedures. Finally, although date palm components offer potential environmentally sustainable and cost-effective starting materials for the production of graphene materials aligned with green chemistry principles, life cycle assessment and detailed comparative cost analysis remain critical factors for large-scale production that require further investigation by researchers to fully realize the potential of date palm-derived graphene materials in practical applications.
Author contributions
MS: Formal Analysis, Methodology, Writing – original draft. MT: Conceptualization, Funding acquisition, Resources, Supervision, Writing – review and editing.
Funding
The author(s) declare that financial support was received for the research and/or publication of this article. United Arab Emirates University.
Acknowledgments
The financial support for this work is provided by United Arab Emirates University (UAEU), under research grant vote no. 12R228.
Conflict of interest
The authors declare that the research was conducted in the absence of any commercial or financial relationships that could be construed as a potential conflict of interest.
Generative AI statement
The author(s) declare that no Generative AI was used in the creation of this manuscript.
Publisher’s note
All claims expressed in this article are solely those of the authors and do not necessarily represent those of their affiliated organizations, or those of the publisher, the editors and the reviewers. Any product that may be evaluated in this article, or claim that may be made by its manufacturer, is not guaranteed or endorsed by the publisher.
References
Ab Aziz, N. A. H., Md Ali, U. F., Ahmad, A. A., Mohamed Dzahir, M. I. H., Khamidun, M. H., and Abdullah, M. F. (2023). Non-functionalized oil palm waste-derived reduced graphene oxide for methylene blue removal: isotherm, kinetics, thermodynamics, and mass transfer mechanism. Arab. J. Chem. 16 (1), 104387. doi:10.1016/j.arabjc.2022.104387
Abbas, A., Rubab, S., Rehman, A., Irfan, S., Sharif, H. M. A., Liang, Q., et al. (2023). One-step green synthesis of biomass-derived graphene quantum dots as a highly selective optical sensing probe. Mater. Today Chem. 30, 101555. doi:10.1016/j.mtchem.2023.101555
Abd Ali, Z. T. (2019). Green synthesis of graphene-coated sand (GCS) using low-grade dates for evaluation and modeling of the pH-dependent permeable barrier for remediation of groundwater contaminated with copper. Sep. Sci. Technol. 56 (1), 14–25. doi:10.1080/01496395.2019.1708937
Abdellah, M. Y., Sadek, M. G., Alharthi, H., Abdel-Jaber, G. T., and Backar, A. H. (2023). Characteristic properties of date-palm fibre/sheep wool reinforced polyester composites. J. Bioresour. Bioprod. 8 (4), 430–443. doi:10.1016/j.jobab.2023.09.003
Ahamed, M., Akhtar, M. J., Khan, M. A. M., and Alhadlaq, H. A. (2022). Enhanced anticancer performance of eco-friendly-prepared Mo-ZnO/RGO nanocomposites: role of oxidative stress and Apoptosis. ACS Omega 7 (8), 7103–7115. doi:10.1021/acsomega.1c06789
Ahmad Farid, M. A., and Andou, Y. (2022). A route towards graphene from lignocellulosic biomass: Technicality, challenges, and their prospective applications. J. Clean. Prod. 380, 135090. doi:10.1016/j.jclepro.2022.135090
Al-Awa, Z. F. A., Sangor, F. I. M. S., Babili, S. B., Saud, A., Saleem, H., and Zaidi, S. J. (2023). Effect of leaf powdering technique on the characteristics of date palm-derived cellulose. ACS Omega 8 (21), 18930–18939. doi:10.1021/acsomega.3c01222
Ali, M. M., Abu Al-Rub, R. K., Banat, F., and Kim, T.-Y. (2024). Enhancing the printing quality and mechanical properties of 3D-printed cement composites with date syrup-based graphene coated sand hybrid. Dev. Built Environ. 20, 100582. doi:10.1016/j.dibe.2024.100582
Alkhalaf, M. I., Churchill, G. C., and Mirghani, M. E. S. (2023). Chemical composition and antioxidant/antibacterial depictions of Zahidi date palm (Phoenix dactylifera) kernel Oil. J. King Saud. Univ. Sci. 35 (7), 102817. doi:10.1016/j.jksus.2023.102817
Allende, S., Liu, Y., Zafar, M. A., and Jacob, M. V. (2024a). Synthesis and application of biomass-based graphene oxide using microwave-assisted pyrolysis method. Nano-Struct. Nano-Objects 40, 101338. doi:10.1016/j.nanoso.2024.101338
Allende, S., Liu, Y., Zafar, M. A., and Jacob, M. V. (2024b). Synthesis and application of biomass-based graphene oxide using microwave-assisted pyrolysis method. Nano-Structures and Nano-Objects 40, 101338. doi:10.1016/j.nanoso.2024.101338
Al-Raad, A. A., and Hanafiah, M. M. (2021). Removal of inorganic pollutants using electrocoagulation technology: a review of emerging applications and mechanisms. J. Environ. Manage 300, 113696. doi:10.1016/j.jenvman.2021.113696
Al-Zahrani, F. A. M., Al-Shehri, B. M., El-Shishtawy, R. M., Awwad, N. S., Khan, K. A., Sayed, M. A., et al. (2022). Characterization of date seed powder derived porous graphene oxide and its application as an environmental functional material to remove dye from aqueous solutions. Mater. (Basel) 15 (22), 8136. doi:10.3390/ma15228136
Amir Faiz, M. S., Che Azurahanim, C. A., Raba'ah, S. A., and Ruzniza, M. Z. (2020). Low cost and green approach in the reduction of graphene oxide (GO) using palm oil leaves extract for potential in industrial applications. Results Phys. 16, 102954. doi:10.1016/j.rinp.2020.102954
Atakoohi, S. E., Spennati, E., Riani, P., Carpanese, M. P., and Garbarino, G. (2024). Graphene-based materials for wastes, biomass and CO2 valorization in catalysis: a technological perspective via molten salt synthesis. Catal. Today 441, 114848. doi:10.1016/j.cattod.2024.114848
Athinarayanan, J., Periasamy, V. S., Krishnamoorthy, R., and Alshatwi, A. A. (2018). Evaluation of antibacterial and cytotoxic properties of green synthesized Cu2O/Graphene nanosheets. Mater Sci. Eng. C Mater Biol. Appl. 93, 242–253. doi:10.1016/j.msec.2018.07.073
Avornyo, A., and Chrysikopoulos, C. V. (2024). Applications of graphene oxide (GO) in oily wastewater treatment: recent developments, challenges, and opportunities. J. Environ. Manage 353, 120178. doi:10.1016/j.jenvman.2024.120178
Azam, M. G., Kabir, M. H., Shaikh, M. A. A., Ahmed, S., Mahmud, M., and Yasmin, S. (2022). A rapid and efficient adsorptive removal of lead from water using graphene oxide prepared from waste dry cell battery. J. Water Process Eng. 46, 102597. doi:10.1016/j.jwpe.2022.102597
Balandin, A. A., Ghosh, S., Bao, W., Calizo, I., Teweldebrhan, D., Miao, F., et al. (2008). Superior thermal conductivity of single-layer graphene. Nano Lett. 8 (3), 902–907. doi:10.1021/nl0731872
Balasubramanian, R., and Chowdhury, S. (2015). Recent advances and progress in the development of graphene-based adsorbents for CO2 capture. J. Mater. Chem. 3 (44), 21968–21989. doi:10.1039/c5ta04822b
Balqis, N., Mohamed Jan, B., Simon Cornelis Metselaar, H., Sidek, A., Kenanakis, G., and Ikram, R. (2023). An overview of Recycling wastes into graphene derivatives using microwave synthesis; trends and prospects. Mater. (Basel) 16 (10), 3726. doi:10.3390/ma16103726
Ban, T., Qiao, W., Hou, B., Zhang, M., Zhang, W., and Kong, X. (2024). Low-cost in-situ preparation of highly dispersed graphene for high-performance cement-based composites. Constr. Build. Mater. 452, 138900. doi:10.1016/j.conbuildmat.2024.138900
Barooah, P., Mushahary, N., Das, B., and Basumatary, S. (2024). Waste biomass-based graphene oxide decorated with ternary metal oxide (MnO-NiO-ZnO) composite for adsorption of methylene blue dye. Clean. Water 2, 100049. doi:10.1016/j.clwat.2024.100049
Beissenov, R., Duisenbek, A., Beisenova, Y., Askaruly, K., Yeleuov, M., and Abdisattar, A. (2024). Activated biomass-derived 3-dimensional porous graphene-like carbon for high-performance energy storage electrode materials. Diam. Relat. Mater. 149, 111588. doi:10.1016/j.diamond.2024.111588
Benallel, A., Tilioua, A., and Garoum, M. (2024). Development of thermal insulation panels bio-composite containing cardboard and date palm fibers. J. Clean. Prod. 434, 139995. doi:10.1016/j.jclepro.2023.139995
Bezazi, A., Bouhemame, N., Reis, P. N. B., Santos, P., Boumediri, H., and Scarpa, F. (2024). Viscoelasticity and impact behaviour of green epoxy bio-composites made of date palm leaflets. Compos. Struct. 344, 118347. doi:10.1016/j.compstruct.2024.118347
Bin Sharfan, I. I., Norrman, K., and Abdulhamid, M. A. (2024). Thermal transformation of date seeds from nonporous to porous materials: Insights on structural changes using chemical and physical characterization. J. Anal. Appl. Pyrolysis 177, 106353. doi:10.1016/j.jaap.2024.106353
Blessy Rebecca, P. N., Durgalakshmi, D., Balakumar, S., and Rakkesh, R. A. (2022). Biomass-derived graphene-based nanocomposites: a Futuristic material for biomedical applications. ChemistrySelect 7 (5), e202104013. doi:10.1002/slct.202104013
Bourgis, F., Kilaru, A., Cao, X., Ngando-Ebongue, G. F., Drira, N., Ohlrogge, J. B., et al. (2011). Comparative transcriptome and metabolite analysis of oil palm and date palm mesocarp that differ dramatically in carbon partitioning. Proc. Natl. Acad. Sci. U. S. A. 108 (30), 12527–12532. doi:10.1073/pnas.1106502108
Cançado, L. G., Jorio, A., Ferreira, E. H. M., Stavale, F., Achete, C. A., Capaz, R. B., et al. (2011). Quantifying defects in graphene via Raman spectroscopy at different Excitation Energies. Nano Lett. 11 (8), 3190–3196. doi:10.1021/nl201432g
Casallas Caicedo, F. M., Vera López, E., Agarwal, A., Drozd, V., Durygin, A., Franco Hernandez, A., et al. (2020). Synthesis of graphene oxide from graphite by ball milling. Diam. Relat. Mater. 109, 108064. doi:10.1016/j.diamond.2020.108064
Chakraborty, M., and Hashmi, M. S. J. (2018). Wonder material graphene: properties, synthesis and practical applications. Adv. Mater. Process. Technol. 4 (4), 573–602. doi:10.1080/2374068x.2018.1484998
Chandrasekaran, M., and Bahkali, A. H. (2013). Valorization of date palm (Phoenix dactylifera) fruit processing by-products and wastes using bioprocess technology - review. Saudi J. Biol. Sci. 20 (2), 105–120. doi:10.1016/j.sjbs.2012.12.004
Chang, H., and Wu, H. (2012). Graphene-based nanomaterials: synthesis, properties, and optical and optoelectronic applications. Adv. Funct. Mater. 23 (16), 1984–1997. doi:10.1002/adfm.201202460
Chaturvedi, A. K., Pappu, A., and Gupta, M. K. (2022). Unraveling the role of agro waste-derived graphene quantum dots on dielectric and mechanical property of the fly ash based polymer nanocomposite. J. Alloys Compd. 903, 163953. doi:10.1016/j.jallcom.2022.163953
Chen, J., and Li, L. (2020). Effect of oxidation degree on the thermal properties of graphene oxide. J. Mater. Res. Technol. 9 (6), 13740–13748. doi:10.1016/j.jmrt.2020.09.092
Chiang, A. K. M., Ng, L. Y., Ng, C. Y., Lim, Y. P., Mahmoudi, E., Tan, L. S., et al. (2023). Conversion of palm oil empty fruit bunches to highly stable and fluorescent graphene oxide quantum dots: an eco-friendly approach. Mater. Chem. Phys. 309, 128433. doi:10.1016/j.matchemphys.2023.128433
Chkala, H., Ighir, S., Ettahiri, W., Taleb, M., Chigr, M., and El Mansouri, N.-E. (2024). Feasibility of incorporating leaf date palm fibers in geopolymer composites made from mining waste. Constr. Build. Mater. 428, 136188. doi:10.1016/j.conbuildmat.2024.136188
Chua, C. K., Sofer, Z., Šimek, P., Jankovský, O., Klímová, K., Bakardjieva, S., et al. (2015). Synthesis of strongly fluorescent graphene quantum dots by Cage-Opening Buckminsterfullerene. ACS Nano 9 (3), 2548–2555. doi:10.1021/nn505639q
Chung, S., Revia, R. A., and Zhang, M. (2021). Graphene quantum dots and their applications in bioimaging, biosensing, and Therapy. Adv. Mater 33 (22), e1904362. doi:10.1002/adma.201904362
Da'na, D. A., Shoshaa, R., Ashfaq, M. Y., and Al-Ghouti, M. A. (2023). Remediation of boron, lithium, and molybdenum by date pits modified with graphene oxide and cellulose nanocrystals: Mechanistic studies. Groundw. Sustain. Dev. 23, 101008. doi:10.1016/j.gsd.2023.101008
Dawood Saleh, B., Hasan Abdulwahhab, G., and Mohammad rashid Ahmed, S. (2023). Preparation and characterization of graphene oxide nanoparticles derived from wheat straw. Mater. Today Proc. 80, 860–869. doi:10.1016/j.matpr.2022.11.320
Demirbas, A. (2017). Utilization of date biomass waste and date seed as bio-fuels source. Energy Sources Part A 39 (8), 754–760. doi:10.1080/15567036.2016.1261208
Destiarti, L., Riyanto, R., Roto, R., and Mudasir, M. (2024). Facile synthesis of reduced graphene oxide using Caesalpinia sappan L. extract as green reducing agent. Next Mater. 2, 100134. doi:10.1016/j.nxmate.2024.100134
Ding, X., Pu, Y., Tang, M., and Zhang, T. (2022). Environmental and health effects of graphene-family nanomaterials: potential release pathways, transformation, environmental fate and health risks. Nano Today 42, 101379. doi:10.1016/j.nantod.2022.101379
Divyabharathi, R., Komalabharathi, P., and Subramanian, P. (2024). “Chapter 3 - microwave-assisted hydrothermal carbonization for biochar production: potential application and limitations,” in Biochar production for green Economy, 43–56.
Donato, K. Z., Tan, H. L., Marangoni, V. S., Martins, M. V., Ng, P. R., Costa, M. C., et al. (2023). Graphene oxide classification and standardization. Sci. Rep. 13 (1), 6064. doi:10.1038/s41598-023-33350-5
Dong, W.-X., Qu, Y.-F., Liu, X., and Chen, L.-F. (2023). Biomass-derived two-dimensional carbon materials: synthetic strategies and electrochemical energy storage applications. FlatChem 37, 100467. doi:10.1016/j.flatc.2022.100467
Ekhlasi, L., Younesi, H., Rashidi, A., and Bahramifar, N. (2018). Populus wood biomass-derived graphene for high CO2 capture at atmospheric pressure and estimated cost of production. Process Saf. Environ. Prot. 113, 97–108. doi:10.1016/j.psep.2017.09.017
El-Maghrabi, N., Fawzy, M., and Mahmoud, A. E. D. (2022). Efficient removal of phosphate from wastewater by a novel Phyto-graphene composite derived from palm byproducts. ACS Omega 7 (49), 45386–45402. doi:10.1021/acsomega.2c05985
El-Shekeil, Y. A., Al-Oqla, F. M., Refaey, H. A., Bendoukha, S., and Barhoumi, N. (2024). Investigating the mechanical performance and characteristics of nitrile butadiene rubber date palm fiber reinforced composites for sustainable bio-based materials. J. Mater. Res. Technol. 29, 101–108. doi:10.1016/j.jmrt.2024.01.092
Ercan, B., Alper, K., Ucar, S., and Karagoz, S. (2023). Comparative studies of hydrochars and biochars produced from lignocellulosic biomass via hydrothermal carbonization, torrefaction and pyrolysis. J. Energy Inst. 109, 101298. doi:10.1016/j.joei.2023.101298
Fadeel, B., Bussy, C., Merino, S., Vázquez, E., Flahaut, E., Mouchet, F., et al. (2018). Safety assessment of graphene-based materials: focus on human health and the environment. ACS Nano 12 (11), 10582–10620. doi:10.1021/acsnano.8b04758
Faghiri, F., and Ghorbani, F. (2020). Synthesis of graphene oxide nanosheets from sugar beet bagasse and its application for colorimetric and naked eye detection of trace Hg-2+ in the environmental water samples. Microchem. J. 152, 104332. doi:10.1016/j.microc.2019.104332
Fathy, M., Hosny, R., Keshawy, M., and Gaffer, A. (2019). Green synthesis of graphene oxide from oil palm leaves as novel adsorbent for removal of Cu(II) ions from synthetic wastewater. Graphene Technol. 4 (1-2), 33–40. doi:10.1007/s41127-019-00025-w
Fayaz, T., Renuka, N., and Ratha, S. K. (2024). Antibiotic occurrence, environmental risks, and their removal from aquatic environments using microalgae: advances and future perspectives. Chemosphere 349, 140822. doi:10.1016/j.chemosphere.2023.140822
Freitag, M. (2010). Optical and thermal properties of graphene field-effect transistors. Phys. Status Solidi B 247 (11-12), 2895–2903. doi:10.1002/pssb.201000173
Galiwango, E., Al-Marzouqi, A. H., Abu-Omar, M. M., Khaleel, A. A., and Rahman, N. S. A. (2017). Estimating Combustion kinetics of UAE date palm tree biomass using Thermogravimetric analysis. J. Nat. Sci. Res. 7, 2224–3186.
Gan, L., Li, B., Chen, Y., Yu, B., and Chen, Z. (2019). Green synthesis of reduced graphene oxide using bagasse and its application in dye removal: a waste-to-resource supply chain. Chemosphere 219, 148–154. doi:10.1016/j.chemosphere.2018.11.181
Gao, Y., Ren, X., Zhang, X., and Chen, C. (2019). Environmental fate and risk of ultraviolet- and visible-light-transformed graphene oxide: a comparative study. Environ. Pollut. 251, 821–829. doi:10.1016/j.envpol.2019.05.010
Gao, Y., Wang, J., Huang, Y., Zhang, S., Zhang, S., and Zou, J. (2023). Rational design of N-doped porous biomass carbon nanofiber electrodes for flexible asymmetric supercapacitors with high-performance. Appl. Surf. Sci. 638, 158137. doi:10.1016/j.apsusc.2023.158137
González-Soto, N., Blasco, N., Irazola, M., Bilbao, E., Guilhermino, L., and Cajaraville, M. P. (2023). Fate and effects of graphene oxide alone and with sorbed benzo(a)pyrene in mussels Mytilus galloprovincialis. J. Hazard. Mater. 452, 131280. doi:10.1016/j.jhazmat.2023.131280
Goodwin, D. G., Adeleye, A. S., Sung, L., Ho, K. T., Burgess, R. M., and Petersen, E. J. (2018). Detection and quantification of graphene-family nanomaterials in the environment. Environ. Sci. Technol. 52 (8), 4491–4513. doi:10.1021/acs.est.7b04938
Gozali Balkanloo, P., Mohammad Sharifi, K., and Poursattar Marjani, A. (2023). Graphene quantum dots: synthesis, characterization, and application in wastewater treatment: a review. Mater. Adv. 4 (19), 4272–4293. doi:10.1039/d3ma00372h
Graimed, B. H., and Tark Abd Ali, Z. (2022). Green approach for the synthesis of graphene glass hybrid as a reactive barrier for remediation of groundwater contaminated with lead and tetracycline. Environ. Nanotechnol. Monit. Manage. 18, 100685. doi:10.1016/j.enmm.2022.100685
Gupta, A., Kumar, A., Jaidka, S., Ajravat, K., and Brar, L. K. (2025). Graphene oxide from biomass waste: a pathway to electrochemical hydrogen production and capacitive applications. Phys. B 698, 416765. doi:10.1016/j.physb.2024.416765
Hao, H.-C., Chen, S., Tan, Z.-X., and Jiang, H. (2023). Preparation of high-yield carbon quantum dots and paper-based sensors from biomass wastes by mechano-chemical method. J. Environ. Chem. Eng. 11 (6), 111406. doi:10.1016/j.jece.2023.111406
Hosny, M., Elbay, A. S., Abdelfatah, A. M., El-Maghrabi, N., and Fawzy, M. (2025). Recent trends in transforming different waste materials into graphene via Flash Joule Heating. Environ. Res. 270, 121033. doi:10.1016/j.envres.2025.121033
Hussain, A., Farooq, A., Bassyouni, M., Sait, H. H., El-Wafa, M. A., Hasan, S. W. u., et al. (2014). Pyrolysis of Saudi Arabian date palm waste: a viable option for converting waste into Wealth. Life Sci. 11, 667–671.
Idris, M. O., Guerrero-Barajas, C., Kim, H.-C., Yaqoob, A. A., and Ibrahim, M. N. M. (2023). Scalability of biomass-derived graphene derivative materials as viable anode electrode for a commercialized microbial fuel cell: a systematic review. Chin. J. Chem. Eng. 55, 277–292. doi:10.1016/j.cjche.2022.05.009
Jaafar, E., Kashif, M., Sahari, S. K., and Ngaini, Z. (2018). Study on morphological, optical and electrical properties of graphene oxide (GO) and reduced graphene oxide (rGO). Mater. Sci. Forum 917, 112–116. doi:10.4028/www.scientific.net/MSF.917.112
Jonoobi, M., shafie, M., Shirmohammadli, Y., Ashori, A., Zarea-Hosseinabadi, H., and Mekonnen, T. (2019). A review on date palm tree: properties, characterization and its potential applications. J. Renew. Mater. 7 (11), 1055–1075. doi:10.32604/jrm.2019.08188
Joshi, S., Bobade, H., Sharma, R., and Sharma, S. (2023). Graphene derivatives: properties and potential food applications. J. Ind. Eng. Chem. 123, 1–18. doi:10.1016/j.jiec.2023.03.047
Kalita, H., Mohapatra, J., Pradhan, L., Mitra, A., Bahadur, D., and Aslam, M. (2016). Efficient synthesis of rice based graphene quantum dots and their fluorescent properties. RSC Adv. 6 (28), 23518–23524. doi:10.1039/C5RA25706A
Khan, S., Achazhiyath Edathil, A., and Banat, F. (2019). Sustainable synthesis of graphene-based adsorbent using date syrup. Sci. Rep. 9 (1), 18106. doi:10.1038/s41598-019-54597-x
Khoei, A. R., and Khorrami, M. S. (2016). Mechanical properties of graphene oxide: a molecular dynamics study. Fuller. nanotub. Carbon Nanostructures 24 (9), 594–603. doi:10.1080/1536383x.2016.1208180
Korkmaz, S., and Kariper, İ. A. (2020). Graphene and graphene oxide based aerogels: synthesis, characteristics and supercapacitor applications. J. Energy Storage 27, 101038. doi:10.1016/j.est.2019.101038
Koyyada, A., and Orsu, P. (2020). “Chapter Thirteen - safety and toxicity concerns of graphene and its composites,” in Comprehensive analytical chemistry, 327–353.
Kumar, A., Sharma, K., and Dixit, A. R. (2019). A review on the mechanical and thermal properties of graphene and graphene-based polymer nanocomposites: understanding of modelling and MD simulation. Mol. Simul. 46 (2), 136–154. doi:10.1080/08927022.2019.1680844
Kumar, K., Kumar, R., Kaushal, S., Thakur, N., Umar, A., Akbar, S., et al. (2023). Biomass waste-derived carbon materials for sustainable remediation of polluted environment: a comprehensive review. Chemosphere 345, 140419. doi:10.1016/j.chemosphere.2023.140419
Kumari, P., and Samadder, S. R. (2022). Valorization of carbonaceous waste into graphene materials and their potential application in water and wastewater treatment: a review. Mater. Today Chem. 26, 101192. doi:10.1016/j.mtchem.2022.101192
Kwaasi, A. A. A. (2003). “DATE PALMS,” in Encyclopedia of food sciences and nutrition. Second Edition (Oxford: Academic Press), 1730–1740.
Lee, A. Y., Yang, K., Anh, N. D., Park, C., Lee, S. M., Lee, T. G., et al. (2021). Raman study of D* band in graphene oxide and its correlation with reduction. Appl. Surf. Sci. 536, 147990. doi:10.1016/j.apsusc.2020.147990
Li, J., Zhong, D., Zeng, K., Chen, X., Wu, B., Liu, T., et al. (2024a). Co-pyrolysis of algae and lignocellulosic biomass in molten salts to produce N-doped carbon for supercapacitor application. Energy 305, 132127. doi:10.1016/j.energy.2024.132127
Li, L., Tang, Y., Bao, Z., Tu, W., Peng, L., Zou, L., et al. (2024b). When graphene meets circular agriculture: insights into agricultural sustainable development. Biosyst. Eng. 237, 92–117. doi:10.1016/j.biosystemseng.2023.12.002
Li, Q., Wang, Y., Zhu, R., Wu, J., Zhang, W., and Lu, H. (2024c). Rapid preparation of porous carbon by Flash Joule heating from bituminous coal and its adsorption mechanism of methylene blue. Colloids Surf. A 682, 132900. doi:10.1016/j.colsurfa.2023.132900
Li, X., Jin, H., Chan, Y., Guo, H., and Ma, W. (2023a). Environmental impacts of graphene at industrial production scale and its application in electric heating technology. Resour. Conserv. Recycl. 199, 107250. doi:10.1016/j.resconrec.2023.107250
Li, Z., Deng, L., Kinloch, I. A., and Young, R. J. (2023b). Raman spectroscopy of carbon materials and their composites: graphene, nanotubes and fibres. Prog. Mater Sci. 135, 101089. doi:10.1016/j.pmatsci.2023.101089
Lin, Y., Tian, Y., Sun, H., and Hagio, T. (2021). Progress in modifications of 3D graphene-based adsorbents for environmental applications. Chemosphere 270, 129420. doi:10.1016/j.chemosphere.2020.129420
Lowe, S. E., and Zhong, Y. L. J. G. o.f.applications (2016). Challenges of industrial-scale graphene oxide production, 410–431.
Mahalingam, S., Manap, A., Lau, K. S., Omar, A., Chelvanathan, P., Chia, C. H., et al. (2022). Mixture deposition method for graphene quantum dots-based dye-sensitized solar cell. Electrochim. Acta 404, 139732. doi:10.1016/j.electacta.2021.139732
Mahor, A., Singh, P. P., Bharadwaj, P., Sharma, N., Yadav, S., Rosenholm, J. M., et al. (2021). Carbon-based nanomaterials for delivery of biologicals and Therapeutics: a Cutting-edge technology. C 7 (1), 19. doi:10.3390/c7010019
Makkawi, Y., El Sayed, Y., Salih, M., Nancarrow, P., Banks, S., and Bridgwater, T. (2019). Fast pyrolysis of date palm (Phoenix dactylifera) waste in a bubbling fluidized bed reactor. Renew. Energy 143, 719–730. doi:10.1016/j.renene.2019.05.028
Malina, T., Maršálková, E., Holá, K., Zbořil, R., and Maršálek, B. (2020). The environmental fate of graphene oxide in aquatic environment—complete mitigation of its acute toxicity to planktonic and benthic crustaceans by algae. J. Hazard. Mater. 399, 123027. doi:10.1016/j.jhazmat.2020.123027
Manai, S., Boulila, A., Silva, A. S., Barbosa-Pereira, L., Sendón, R., and Khwaldia, K. (2024). Recovering functional and bioactive compounds from date palm by-products and their application as multi-functional ingredients in food. Sustain. Chem. Pharm. 38, 101475. doi:10.1016/j.scp.2024.101475
Manikandan, V., and Lee, N. Y. (2023). Reduced graphene oxide: Biofabrication and environmental applications. Chemosphere 311 (Pt 1), 136934. doi:10.1016/j.chemosphere.2022.136934
Mao, X., Zhang, Y., Li, H., Zhao, N., Zhang, H., Zhao, P., et al. (2025). Ultrafast synthesis of lignin-based graphene by flash joule heating for multifunctional applications. J. Power Sources 631, 236211. doi:10.1016/j.jpowsour.2025.236211
Mbayachi, V. B., Ndayiragije, E., Sammani, T., Taj, S., Mbuta, E. R., and khan, A. u. (2021). Graphene synthesis, characterization and its applications: a review. Results Chem. 3, 100163. doi:10.1016/j.rechem.2021.100163
Mishra, R., Kumar, A., Singh, E., Kumari, A., and Kumar, S. (2023). Synthesis of graphene oxide from biomass waste: characterization and volatile organic compounds removal. Process Saf. Environ. Prot. 180, 800–807. doi:10.1016/j.psep.2023.10.048
M K, K., and Jaiswal, M. (2016). Graphene: a review of optical properties and photonic applications. Asian J. Phys. 25, 809–831.
Muthalagu, R., Murugesan, J., Sathees Kumar, S., and Sridhar Babu, B. (2021). Tensile attributes and material analysis of kevlar and date palm fibers reinforced epoxy composites for automotive bumper applications. Mater. Today Proc. 46, 433–438. doi:10.1016/j.matpr.2020.09.777
Nizam, N. U. M., Hanafiah, M. M., Mahmoudi, E., Mohammad, A. W., and Oyekanmi, A. A. (2022). Effective adsorptive removal of dyes and heavy metal using graphene oxide based Pre-treated with NaOH/H2SO4 rubber seed shells synthetic graphite Precursor: equilibrium Isotherm, kinetics and thermodynamic studies. Sep. Purif. Technol. 289, 120730. doi:10.1016/j.seppur.2022.120730
Nusair, A., Barber, M., Pramanik, A., Ethridge, C., William, C., Alkhateb, H., et al. (2024). Graphene-coated sand for enhanced water reuse: impact on water quality and chemicals of emerging concern. Sci. Total Environ. 945, 174078. doi:10.1016/j.scitotenv.2024.174078
Ousaleh, H. A., Charti, I., Sair, S., Mansouri, S., Abboud, Y., and Bouari, A. E. (2020). Green and low-cost approach for graphene oxide reduction using natural plant extracts. Mater. Today Proc. 30, 803–808. doi:10.1016/j.matpr.2020.03.640
Ouyang, D.-d., Hu, L.-b., Wang, G., Dai, B., Yu, F., and Zhang, L.-l. (2021). A review of biomass-derived graphene and graphene-like carbons for electrochemical energy storage and conversion. New Carbon Mater 36 (2), 350–372. doi:10.1016/s1872-5805(21)60024-0
Pandey, M., Deshmukh, K., Raman, A., Asok, A., Appukuttan, S., and Suman, G. R. (2024). Prospects of MXene and graphene for energy storage and conversion. Renew. Sustain. Energy Rev. 189, 114030. doi:10.1016/j.rser.2023.114030
Papageorgiou, D. G., Kinloch, I. A., and Young, R. J. (2017). Mechanical properties of graphene and graphene-based nanocomposites. Prog. Mater Sci. 90, 75–127. doi:10.1016/j.pmatsci.2017.07.004
Phiri, J., Gane, P., and Maloney, T. C. (2017). General overview of graphene: production, properties and application in polymer composites. Mater. Sci. Eng. B 215, 9–28. doi:10.1016/j.mseb.2016.10.004
Qu, J., Meng, Q., Peng, W., Shi, J., Dong, Z., Li, Z., et al. (2023). Application of functionalized biochar for adsorption of organic pollutants from environmental media: synthesis strategies, removal mechanisms and outlook. J. Clean. Prod. 423, 138690. doi:10.1016/j.jclepro.2023.138690
Rezania, S., Mojiri, A., Park, J., Nawrot, N., Wojciechowska, E., Marraiki, N., et al. (2022). Removal of lead ions from wastewater using lanthanum sulfide nanoparticle decorated over magnetic graphene oxide. Environ. Res. 204 (Pt A), 111959. doi:10.1016/j.envres.2021.111959
Safian, M.T.-u., Raja, P. B., Shen, C. Y., and Mohamad Ibrahim, M. N. (2023). A novel preparation of bio-based graphene from oil palm biomass as a fluid loss additive in water-based drilling fluid. Geoenergy Sci. Eng. 231, 212321. doi:10.1016/j.geoen.2023.212321
Safian, M.T.-u., Umar, K., and Mohamad Ibrahim, M. N. (2021). Synthesis and scalability of graphene and its derivatives: a journey towards sustainable and commercial material. J. Clean. Prod. 318, 128603. doi:10.1016/j.jclepro.2021.128603
Saini, K., Singh, J., Malik, S., Saharan, Y., Goyat, R., Umar, A., et al. (2024). Metal-Organic Frameworks: a promising solution for efficient removal of heavy metal ions and organic pollutants from industrial wastewater. J. Mol. Liq. 399, 124365. doi:10.1016/j.molliq.2024.124365
Saleem, H., Saud, A., and Zaidi, S. J. (2023). Sustainable preparation of graphene quantum dots from leaves of date palm tree. ACS Omega 8 (31), 28098–28108. doi:10.1021/acsomega.3c00694
Sarmin, S. N., Jawaid, M., Ismail, A. S., Hashem, M., Fouad, H., Midani, M., et al. (2023). Effect of chitosan filler on the thermal and viscoelasticity properties of bio-epoxy/date palm fiber composites. Sustain. Chem. Pharm. 36, 101275. doi:10.1016/j.scp.2023.101275
Sawant, S. A., Patil, A. V., Waikar, M. R., Rasal, A. S., Dhas, S. D., Moholkar, A. V., et al. (2022). Advances in chemical and biomass-derived graphene/graphene-like nanomaterials for supercapacitors. J. Energy Storage 51, 104445. doi:10.1016/j.est.2022.104445
Shahil, K. M. F., and Balandin, A. A. (2012). Thermal properties of graphene and multilayer graphene: applications in thermal interface materials. Solid State Commun. 152 (15), 1331–1340. doi:10.1016/j.ssc.2012.04.034
Sharma, N., Tomar, S., Shkir, M., Kant Choubey, R., and Singh, A. (2021). Study of optical and electrical properties of graphene oxide. Mater. Today Proc. 36, 730–735. doi:10.1016/j.matpr.2020.04.861
Suk, J. W., Piner, R. D., An, J., and Ruoff, R. S. (2010). Mechanical properties of monolayer graphene oxide. ACS Nano 4 (11), 6557–6564. doi:10.1021/nn101781v
Supian, A. B. M., Jawaid, M., Rashid, B., Fouad, H., Saba, N., Dhakal, H. N., et al. (2021). Mechanical and physical performance of date palm/bamboo fibre reinforced epoxy hybrid composites. J. Mater. Res. Technol. 15, 1330–1341. doi:10.1016/j.jmrt.2021.08.115
Tahir, A. H. F., Al-Obaidy, A. H. M. J., and Mohammed, F. H. (2020). Biochar from date palm waste, production, characteristics and use in the treatment of pollutants: a Review. IOP Conf. Ser. Mater. Sci. Eng. 737, 012171. doi:10.1088/1757-899x/737/1/012171
Tajik, S., Dourandish, Z., Zhang, K., Beitollahi, H., Le, Q. V., Jang, H. W., et al. (2020). Carbon and graphene quantum dots: a review on syntheses, characterization, biological and sensing applications for neurotransmitter determination. RSC Adv. 10 (26), 15406–15429. doi:10.1039/d0ra00799d
Tamilselvi, R., Ramesh, M., Lekshmi, G. S., Bazaka, O., Levchenko, I., Bazaka, K., et al. (2020). Graphene oxide – based supercapacitors from agricultural wastes: a step to mass production of highly efficient electrodes for electrical transportation systems. Renew. Energy 151, 731–739. doi:10.1016/j.renene.2019.11.072
Taşdemir, Ş., Morçimen, Z. G., Doğan, A. A., Görgün, C., and Şendemir, A. (2023). Surface area of graphene Governs its Neurotoxicity. ACS Biomater. Sci. Eng. 9 (6), 3297–3305. doi:10.1021/acsbiomaterials.3c00104
Teow, Y. H., Tajudin, S. A., Ho, K. C., and Mohammad, A. W. (2020). Synthesis and characterization of graphene shell composite from oil palm frond juice for the treatment of dye-containing wastewater. J. Water Process Eng. 35, 101185. doi:10.1016/j.jwpe.2020.101185
Thangaraj, B., Solomon, P. R., Wongyao, N., Helal, M. I., Abdullah, A., Abedrabbo, S., et al. (2024). Synthesis of reduced graphene oxide nanosheets from sugarcane dry leaves by two-stage pyrolysis for antibacterial activity. Nano Mater. Sci. 6, 625–634. doi:10.1016/j.nanoms.2024.01.006
Tinh, N. T., Dat, N. M., Hieu, L. M., Bao, L. M., Khoa, N. N., Nhan, D. D., et al. (2023). Sustainable synthesis and characterization of corncob-derived graphene oxide. ChemistrySelect 8 (29), e202301537. doi:10.1002/slct.202301537
Tondro, H., Zilouei, H., Zargoosh, K., and Bazarganipour, M. (2021). Nettle leaves-based sulfonated graphene oxide for efficient hydrolysis of microcrystalline cellulose. Fuel 284, 118975. doi:10.1016/j.fuel.2020.118975
Torrisi, L., Cutroneo, M., Torrisi, A., and Silipigni, L. (2022). Measurements on five characterizing properties of graphene oxide and reduced graphene oxide Foils. Phys. Status Solidi A 219 (6), 2100628. doi:10.1002/pssa.202100628
Tucek, J., Blonski, P., Ugolotti, J., Swain, A. K., Enoki, T., and Zboril, R. (2018). Emerging chemical strategies for imprinting magnetism in graphene and related 2D materials for spintronic and biomedical applications. Chem. Soc. Rev. 47 (11), 3899–3990. doi:10.1039/c7cs00288b
Urzúa, J., Poon, P. S., and Matos, J. (2024). Biomass-derived graphene and nanostructured carbons: a review for electrochemical applications. J. Non-Cryst. Solids 626, 122779. doi:10.1016/j.jnoncrysol.2023.122779
Vakili, M., Cagnetta, G., Deng, S., Wang, W., Gholami, Z., Gholami, F., et al. (2024). Regeneration of exhausted adsorbents after PFAS adsorption: a critical review. J. Hazard Mater 471, 134429. doi:10.1016/j.jhazmat.2024.134429
Vasudevan, M., Remesh, S., Perumal, V., Raja, P. B., Ibrahim, M. N. M., Gopinath, S. C. B., et al. (2023). Lignin derived nanoparticle intercalation on nitrogen-doped graphene quantum dots for electrochemical sensing of cardiac biomarker. Microchem. J. 195, 109405. doi:10.1016/j.microc.2023.109405
Vatandost, E., Ghorbani-HasanSaraei, A., Chekin, F., Naghizadeh Raeisi, S., and Shahidi, S. A. (2020). Green tea extract assisted green synthesis of reduced graphene oxide: application for highly sensitive electrochemical detection of sunset yellow in food products. Food Chem. X 6, 100085. doi:10.1016/j.fochx.2020.100085
Wang, F., Qi, X., Zhang, H., and Yang, Z. (2025). Innovative molten salt techniques for biomass valorization: transforming biomass into advanced carbon materials. Carbon 234, 119999. doi:10.1016/j.carbon.2025.119999
Wu, W., and Yu, B. (2020). Corn flour nano-graphene prepared by the Hummers redox method. ACS Omega 5 (46), 30252–30256. doi:10.1021/acsomega.0c04722
Yang, S., Wang, Z., Xie, Y., Gao, X., Yao, F., Bai, H., et al. (2024). Polyaniline nanowire arrays on biomass-derived carbon nanotubes with typha longbracteata for high-performance symmetric supercapacitors. Diam. Relat. Mater. 141, 110608. doi:10.1016/j.diamond.2023.110608
Yaqoob, A. A., Ibrahim, M. N. M., and Umar, K. (2021). Biomass-derived composite anode electrode: synthesis, characterizations, and application in microbial fuel cells (MFCs). J. Environ. Chem. Eng. 9 (5), 106111. doi:10.1016/j.jece.2021.106111
Yoo, S., Chung, C.-C., Kelley, S. S., and Park, S. (2018). Graphitization behavior of loblolly pine wood investigated by in situ high temperature X-ray diffraction. ACS Sustain. Chem. Eng. 6 (7), 9113–9119. doi:10.1021/acssuschemeng.8b01446
Yoon, D., Son, Y.-W., and Cheong, H. (2011). Negative thermal expansion coefficient of graphene measured by Raman spectroscopy. Nano Lett. 11 (8), 3227–3231. doi:10.1021/nl201488g
Younis, M., Farag, H. A., Alhamdan, A., Aboelasaad, G., Zein El-Abedein, A. I., and Kamel, R. M. (2023). Utilization of palm residues for biochar production using continuous flow pyrolysis unit. Food Chem. X 20, 100903. doi:10.1016/j.fochx.2023.100903
Yu, S., Wang, L., Li, Q., Zhang, Y., and Zhou, H. (2022). Sustainable carbon materials from the pyrolysis of lignocellulosic biomass. Mater. Today Sustain. 19, 100209. doi:10.1016/j.mtsust.2022.100209
Yu, T., Wang, J., Ding, N., Guo, X., Wang, M., and Chen, Y. (2024). Tourmaline for heavy metals removal in wastewater treatment: a review. J. Ind. Eng. Chem. 131, 44–53. doi:10.1016/j.jiec.2023.10.048
Zakaria, N. Z. J., Rozali, S., Mubarak, N. M., and Ibrahim, S. (2022). A review of the recent trend in the synthesis of carbon nanomaterials derived from oil palm by-product materials. Biomass Convers. Biorefin 14, 13–44. doi:10.1007/s13399-022-02430-3
Zeng, Y., Li, T., Yao, Y., Li, T., Hu, L., and Marconnet, A. (2019). Thermally conductive reduced graphene oxide thin films for extreme temperature sensors. Adv. Funct. Mater. 29 (27), 1901388. doi:10.1002/adfm.201901388
Zhang, B., Biswal, B. K., Zhang, J., and Balasubramanian, R. (2023). Hydrothermal treatment of biomass feedstocks for sustainable production of chemicals, fuels, and materials: progress and perspectives. Chem. Rev. 123 (11), 7193–7294. doi:10.1021/acs.chemrev.2c00673
Zhao, T., Wang, K., Liu, F., Zhang, S., and Ho, S.-H. (2024). Recent progress of tailoring valuable graphene quantum dots from biomass. Chin. Chem. Lett., 110321. doi:10.1016/j.cclet.2024.110321
Zheng, X. T., Ananthanarayanan, A., Luo, K. Q., and Chen, P. (2015). Glowing graphene quantum dots and carbon dots: properties, syntheses, and biological applications. Small 11 (14), 1620–1636. doi:10.1002/smll.201402648
Keywords: graphene and derivatives, date-palm tree derived graphene, syrup derived graphene, synthesis process of graphene, characterization and identification, pollutants removal
Citation: Suliman M and Tahir M (2025) Date palm-derived graphene materials: a review on recent advances in synthesis, characterization and environmental applications. Front. Nanotechnol. 7:1518715. doi: 10.3389/fnano.2025.1518715
Received: 28 October 2024; Accepted: 11 March 2025;
Published: 15 May 2025.
Edited by:
Sandeep Kumar, Punjab engineering college (Deemed to be University), IndiaReviewed by:
Priya Banerjee, Rabindra Bharati University, IndiaSahil Tahiliani, Applied Materials, United States
Copyright © 2025 Suliman and Tahir. This is an open-access article distributed under the terms of the Creative Commons Attribution License (CC BY). The use, distribution or reproduction in other forums is permitted, provided the original author(s) and the copyright owner(s) are credited and that the original publication in this journal is cited, in accordance with accepted academic practice. No use, distribution or reproduction is permitted which does not comply with these terms.
*Correspondence: Muhammad Tahir, bXVoYW1tYWQudGFoaXJAdWFldS5hYy5hZQ==