- 1Department of Gastroenterology, Infectious Diseases and Rheumatology, Charité – Universitätsmedizin Berlin, corporate member of Freie Universität Berlin and Humboldt - Universität zu Berlin, Campus Benjamin Franklin, Berlin, Germany
- 2iPATH.Berlin, Campus Benjamin Franklin, Charité – Universitätsmedizin Berlin, corporate member of Freie Universität Berlin and Humboldt - Universität zu Berlin, Berlin, Germany
- 3Institute of Bioinformatics, Biocenter, Medical University of Innsbruck, Innsbruck, Austria
- 4Department of Radiology-Experimental Radiology, Campus Mitte, Charité – Universitätsmedizin Berlin, corporate member of Freie Universität Berlin and Humboldt- Universität zu Berlin, Berlin, Germany
- 5Bundesanstalt für Materialforschung und - Prüfung (BAM), Berlin, Germany
- 6Department of Radiology-Radiology, Campus Benjamin Franklin, Charité – Universitätsmedizin Berlin, corporate member of Freie Universität Berlin and Humboldt - Universität zu Berlin, Berlin, Germany
The field of medical application of organic or inorganic nanoparticles is extensive. Medical nanoparticles offer benefits but pose risks. For safe use in diagnostics and therapy, they should be inert, non-immunogenic, non-aggregating, and avoid long-term accumulation in sensitive tissues like bone marrow or the brain. We have developed in-house very small superparamagnetic iron oxide nanoparticles (VSOP), 7 nm in size, which have been successfully used in preclinical magnetic resonance imaging (MRI) to detect intestinal inflammation, neuroinflammation and atherosclerosis. This study examines nanoparticle effects on human blood cells focusing on monocytes in vitro as a first step toward clinical application. Whole blood and monocytes from healthy donors and patients with inflammatory bowel disease were treated with VSOP in vitro and analyzed for changes in their transcriptome, phenotype and function. RNA sequencing of monocytes identified the transferrin receptor as one of the most significantly downregulated genes after VSOP treatment, likely to limit iron uptake. Whereas whole blood RNA sequencing showed significant changes only in three non-coding genes. CyTOF analysis confirmed that VSOP-treated monocytes remain inactive, with no increased proliferation or altered migration. Metabolically, VSOP uptake enhanced the oxygen consumption rate. This effect was likely due to phagocytosis rather than effects mediated by the VSOP itself, as phagocytosis of latex beads showed comparable results. In summary, the analysis of peripheral blood mononuclear cells and monocytes suggests that VSOP treatment has no major impact on immune cell phenotype or function indicating VSOP as a promising diagnostic tool in MRI for inflammatory bowel disease.
1 Introduction
According to the European Union Observatory for Nanomaterial, nanoparticles are defined as a natural, incidental, or manufactured material containing particles, in an unbound state or as an aggregate or as an agglomerate and where, for 50% or more of the particles in the number size distribution, one or more external dimensions is in the size range 1 nm–100 nm (https://euon.echa.europa.eu/definition-of-nanomaterial). Nanoparticles can be synthesized from various organic or inorganic materials, and both the material and the structure influence their properties and therefore their application. Potential applications of nanotechnology include agriculture, e.g., as nanofertilizers or nanopesticides (Avila-Quezada et al., 2022; Nguyen et al., 2024; Yadav et al., 2023), the food industry (Mohammad et al., 2022; Nile et al., 2020), cosmetics (Sharma et al., 2023; Gupta et al., 2022), and medicine. Nanotechnology in medicine ranges from diagnostics to therapy. The potential for nanotechnology-based therapy includes anti-microbial treatment (Makabenta et al., 2021; Hetta et al., 2023), tissue regeneration and wound healing (Sharifi et al., 2021; Blanco-Fernandez et al., 2021), anticancer therapy (Mosleh-Shirazi et al., 2022; Wang et al., 2024; Gschwend et al., 2021), and sustained drug delivery for long-term therapy of e.g., autoimmune diseases such as psoriasis, rheumatoid arthritis or Crohn’s disease (Gordon et al., 2021; van der Heijde et al., 2022; Loftus et al., 2016). In diagnostics, nanoparticles can be used as biosensors in bioassays for the detection of analytes. Their surface is loaded with binding sites for biomolecules used as disease biomarkers, e.g., SARS-CoV-2 bioassay (Wu et al., 2021). In addition, nanoparticles can also serve as imaging probes, e.g., ultrasmall superparamagnetic iron oxide (USPIO) nanoparticles in MRI for lymph node characterization in the assessment of primary tumor spread in pelvic cancer (Philips et al., 2019). Although the USPIO Simeren® was withdrawn in 2008, the development of advanced USPIO nanoparticles holds great promise for clinical applications (Chen et al., 2022). This illustrates that nanotechnology carries risks as well as opportunities. Approved use of nanoparticles is difficult due to their potential aggregation and accumulation, toxicity, carcinogenicity, and influence on cellular viability and function. With respect to human health, nanoparticles should not accumulate in any organ, such as the brain after crossing the blood-brain barrier (Hersh et al., 2022) or in the spleen or bone marrow, where long-lived immune cells have their niches (Nguyen et al., 2018; Sze et al., 2000; Manz et al., 2002). Thus, nanoparticles should not affect the phenotype and function of immune cells or interfere with their survival. In addition, nanoparticles are potential replacements for substances already in use that accumulate and affect the human body, such as gadolinium-based contrast agents used in medical imaging that accumulate in the brain (Kanda et al., 2015), bone (Darrah et al., 2009) and skin (Roberts et al., 2016; Gathings et al., 2015). Gadolinium accumulation in the liver has been described mainly in animal studies (Frenzel et al., 2017; Bonafe et al., 2023; Di Gregorio et al., 2018) and in pediatric patients with siderosis (Maximova et al., 2016). As mentioned above, nanoparticles come in different flavors and their properties are strongly influenced among others by their size, shape, surface charge (zeta potential), core material, functionalization, and concentration. The nanoparticle size, for example, impacts biodistribution within specific organs and throughout the body (Choi et al., 2011; Sonavane et al., 2008). The shape influences, e.g., gastrointestinal residency after oral application as well as the clearance (Zhao et al., 2017). The study of Zhao et al. showed that long rod nanoparticles achieved longer blood circulation and had the highest bioavailability compared with short rod and spherical nanoparticles (Zhao et al., 2017). In addition, the nanocarrier’s zeta potential influences drug delivery from mucus-rich sites such as the gastrointestinal tract (Maisel et al., 2015). Different core materials have different effects on the phenotype and function of immune cells, especially antigen-presenting cells. For example, iron oxide nanoparticles are generally biocompatible and well-tolerated at low concentrations (Mejias et al., 2013), whereas silver and gold nanoparticles can trigger pro-inflammatory responses (Durocher et al., 2017; Haase et al., 2014). Additionally, silver nanoparticles can have cytotoxic effects, though these are desired in cancer therapy (Surapaneni et al., 2018; Sur et al., 2010). The data on the cytotoxic effects of gold nanoparticles is conflicting but they are promising tools in photothermal therapy (Sani et al., 2021; Baioco et al., 2024). The functionalization of the nanoparticles can also influence their immunogenicity. PEGylation, the coating of nanoparticles with polyethylene glycol, is one of the most popular approaches to control the stability of nanoparticles (Bazile et al., 1995). In mice, though, PEG-coated silver nanoparticles induced acute inflammation and apoptosis in the liver (Cho et al., 2009). Dose-dependent effects of the nanoparticles on immunogenicity were also observed in RAW 264.7 macrophages with hierarchical signaling pathways of cellular responses (Yazdimamaghani et al., 2019). All these examples highlight the vast variety of nanoparticles’ functions.
This study presents ultra-small (7 nm) nanoparticles used in preclinical magnetic resonance imaging (MRI) studies for colitis (Golusda et al., 2022), atherosclerosis (Wagner et al., 2013), and neuroinflammation (Millward et al., 2019). Long-term studies in colitic mice showed no impact on survival, intestinal inflammation or immune-cell composition, with no accumulation in organs such as the liver, spleen, kidney, intestine or bone marrow. These very small superparamagnetic iron oxide nanoparticles (VSOP) were synthesized by the Department of Radiology-Experimental Radiology at the Charité - Universitätsmedizin Berlin. VSOP specifically bind to glucosaminoglycans (GAG) (Ludwig et al., 2013; Berndt et al., 2017; Freise et al., 2024), and their transport into inflamed intestinal tissues depends on hyaluronic acid and monocytes expressing the hyaluronic acid receptor CD44 (Golusda et al., 2022). CD44, TLR2, and 4, RHAMM and Lyve-1 are receptors for hyaluronic acid (Lesley et al., 2000; Scheibner et al., 2006; Riehl et al., 2015; Tolg et al., 2012; Prevo et al., 2001). Previous in vivo studies showed, that monocytes taking up i. v.-injected VSOP expressed CD44 and accumulated in tissues enriched in hyaluronic acid (Golusda et al., 2022).
For clinical diagnostics, VSOP will be administered intravenously. In inflammatory bowel disease (IBD), particularly ulcerative colitis (UC) and Crohn´s disease (CD), complications such as fibrosis, stenosis, and fistulas result from extracellular matrix (ECM) remodeling. Excessive ECM degradation leads to fistulation, while excessive aggregation causes stenosis and fibrosis. Early ECM accumulation prediction is crucial for disease monitoring and personalized treatment, yet current methods, including endoscopy, ultrasound or MRI, fail to detect early-stage ECM alterations. GAG-specific nanoparticles could enhance diagnostic sensitivity. However, intravenous administration raises concerns about VSOP hemocompatibility.
This in vitro study investigates whether VSOP affect human blood cells, particularly monocytes from healthy donors and IBD patients, assessing their impact on cell phenotype and function.
2 Materials and methods
2.1 VSOP
VSOP were provided by the Department of Radiology and produced as previously described (Poller et al., 2016). Electrostatically stabilized VSOP coated with monomeric citrate, featuring a hydrodynamic diameter of 8.1 ± 4 nm and a crystallite size of 6.8 ± 2 nm. Relaxivities in water were r1 = 25 mM−1·s−1 and r2 = 63.8 mM−1·s−1 at 0.94 T at a saturation magnetization of 95 emug−1 iron (Poller et al., 2016). For CyTOF analysis and in vivo visualization of VSOP we made use of europium (Eu)-doped VSOP (de Schellenberger et al., 2017). We detected neither in vitro nor in vivo differences in use of doped or non-doped VSOP.
2.2 Animals and colitis induction
Inbred wildtype (WT) C57/Bl6J mice were obtained from Charles River (Sulzfeld, Germany) and housed under conventional conditions with an enriched environment at the animal facilities of the Research Institute for Experimental Medicine (FEM, Charité - Universitätsmedizin Berlin). Recombination activating gene 1-deficient mice (Rag1tm1Mom) on a C57BL/6 background were bred in-house under SPF conditions at the FEM. All animals were kept in polycarbonate cages and had free access to sterile standard chow and drinking water. All experiments were performed in accordance with the German legislation on the protection of animals and approved by the local authorities (Landesamt für Gesundheit und Soziales, registration number G0117/20).
Transfer colitis was induced in 8-week old Rag1tm1Mom mice by i. p. injection of 4 × 105 CD4+CD45RBhi T cells isolated from WT mice according to Maschmeyer et al. (Maschmeyer et al., 2021). After the onset of colitis on day 14, mice were injected i. v. with 0.03 mM Eu-doped VSOP per kg body weight. The Eu signal can be detected later on in mass spectrometry. Mice were sacrificed on days 3, 10, and 18 after VSOP application.
2.3 LA-ICP-MS (detection of Eu-doped VSOP)
Mice with transfer colitis were analyzed at days 3, 10 or 18 after i. v. application of Eu-doped VSOP. Organs (spleen, liver, colon, caecum, ileum, mesenteric lymph nodes) and tibia were taken out and fixed in 10% formaldehyde overnight at room temperature. After fixation, bones were decalcified with 0.5 M EDTA for 72 h. Formalin-fixed tissue was embedded in paraffin (Histosec, Merck) and tissue microarrays (TMA) were prepared.
For slide preparation, 4 µm thick paraffin sections were cut from paraffin blocks or TMA with respective organs. Paraffin sections were dewaxed and histochemically stained with hematoxylin and eosin to detect a region of interest. This region was assigned to the paraffin block (donor block), where a punch was taken using the Tissue Arrayer MiniCore® 3 (Excilone, Elancourt, France). This punch was transferred to the recipient block, where all punches of the different donor blocks were arranged. This allows for the analysis of multiple individual tissue samples on one slide.
LA-ICP-MS analyses were performed on a NWRimage laser ablation system (266 nm, Elemental Scientific Laser, Bozeman, United States) equipped with a low-dispersion ablation cell in a TwoVol3 ablation chamber attached to an ICP-ToF-MS (Model 2R, Tofwerk AG, Thun, Switzerland) using a 1.016 mm ID PEEK tubing and a Dual Concentric Injector 2 (DCI2) (Schannor et al., 2025). Instrumental settings are summarised in Table 1. Raw data were reduced in the Iolite v4 software (Elemental Scientific Laser, Bozeman, United States) using the “3D Trace Elements” (3D-TE-DRS) data reduction scheme. Gelatin multi-element standards (BIO-logi-CAL standard 2, 48 elements; aemas, Oosterhout, Netherlands) were used as calibration material following established procedures and analyzed before and after samples.
2.4 Patients and healthy volunteers
Heparinized blood (9 mL) and EDTA blood (5 mL), respectively was received from patients with active UC or CD and from healthy donors (Table 2) after giving written consent (EA1/181/17 and EA1/273/21).
2.5 VSOP treatment of whole blood cells and monocytes
Eu-doped VSOP were used for CyTOF analysis to detect VSOP uptake via the Eu mass signal. All other in vitro experiments were conducted with non-doped VSOP.
Five mL of heparinized or EDTA whole blood were treated with 0.75 mM VSOP for 30 min at room temperature under gentle rotation. Treated EDTA blood was subjected to RNA isolation and treated heparinized blood was fixed for CyTOF analysis. Fixation for CyTOF analysis was performed with Proteomic Stabilizer PROT1 (Smart Tube Inc., Las Vegas, NV, United States) at 1:2.5 ratio for 12 min at room temperature. Fixed samples were stored at −80°C until staining and analysis.
Peripheral blood mononuclear cells (PBMC) were isolated from heparinized whole blood via gradient centrifugation using LymphoPrep (STEMCELL Technologies, Köln, Germany). Monocytes were enriched from PBMC using CD14 magnetic microbeads (Miltenyi Biotec, Bergisch Gladbach, Germany). Purity was evaluated by flow cytometry employing APC-conjugated anti-CD14 (clone CHD14, BioLegend, San Diego, United States). Monocytes (>95% purity) were treated with 0.5–1 mM VSOP for 30–90 min.
2.6 Cytokine detection and flow cytometry
CD14+ monocytes were cultured in RPMI media (Gibco by ThermoFisher Scientific, Darmstadt, Germany) supplemented with 1% Penicillin/Streptomycin (105 U/mL and 105 μg/L, respectively; Biochrom, Berlin, Germany), heat-inactivated 10% FBS (Corning, NY, United States) and 20 ng/mL M-CSF (PeproTech by ThermoFisher Scientific). Monocytes were seeded in a 24-well plate (ThermoFisher Scientific) at a density of 4.5 × 105 cells per well and rested overnight at 37°C with 5% CO2. On the next day, monocytes were treated with VSOP at different concentrations (0.5 mM, 0.75 mM or 1 mM per well). Cells were incubated with VSOP for either 30 or 90 min. After each incubation time, the VSOP-containing media was removed, and wells were washed once with RPMI. Complete medium was added, and cells were incubated at 37°C with 5% CO2. After 24 h, supernatants were removed and stored at −80°C for cytokine analysis. For analysis of cell surface marker expression, monocytes were detached from the plates using trypsin/EDTA (0.25%) (Gibco) and stained with fluorochrome-labeled antibodies directed against CD14, CD16, and viability (Table 3) for flow cytometric measurement. Cytokines in the supernatant were detected by LEGENDplex Human Macrophage/Microglia Panel (Biolegend) as described in the user´s manual.
2.7 CyTOF
Isotope-tagged antibodies were purchased from Standard BioTools (CA, United States) (Table 3). Unlabeled antibodies were purchased in carrier-free buffer as indicated in Table 3 and conjugated to metal isotopes with Maxpar® X8 antibody labeling kit (Standard BioTools) following the user´s manual.
Fixed whole blood samples were thawed and incubated two times in Thaw-Lyse Buffer (Smart Tube Inc.) for 10 min for erythrocyte lysis. Samples were barcoded, pooled, and stained with a cocktail of metal-conjugated antibodies for 30 min at 4°C in Maxpar Cell Staining Buffer (Standard BioTools). An anchor sample was included in each staining for quality control and normalization. Measurement was performed at the BIH Cytometry Core Facility (Charité–Universitätsmedizin Berlin) employing CyTOF2/Helios (Standard BioTools) with CyTOF software (version 6.5.236). Flow cytometry standard files (.fcs) were analyzed using Cytobank platform (Kotecha et al., 2010) or FlowJo software (version 10.1).
2.8 RNA isolation, sequencing and analysis
RNA was isolated using RNeasy for RNA isolation from whole blood (Qiagen, Hilden, Germany). Isolated RNA was further cleaned and concentrated using Clean and Concentrator Kit (Zymo Research, Freiburg, Germany). Concentration and purity of the isolated RNA was determined by the ratios of absorbance (A260:280 and A260:230) using NanoDrop 1,000 (ThermoFisher Scientific). The RNA Integrity Number (RIN) was determined using the Agilent RNA ScreenTape System (Agilent Technologies, Santa Clara, CA, United States) employing the Agilent 2200 TapeStation. RNA library preparation and sequencing were performed by BIH/MDC Genomics Platform using Illumina library and Illumina High-Troughput Sequencer.
RNA-sequencing samples were preprocessed and mapped to the human reference genome with the nf-core RNA-sequencing pipeline (v.3.14.0) (Ewels et al., 2020). In brief, sequencing reads were aligned to the hg38/GRCh38 reference genome with GENCODE v.46 annotations using STAR (Dobin et al., 2013) and quantified using Salmon (Patro et al., 2017). Raw gene count tables from paired samples were then used for differential expression analysis with DESeq2 v.1.44.0 in R (version 4.4.1) (Love et al., 2014). Genes were defined as differentially expressed when meeting a |log2 fold change| threshold of >1 and an adjusted p-value of <0.1. For visualization of the results, we used the ggplot2 and the EnhancedVolcano R-packages.
2.9 Metabolic measurement (seahorse)
CD14+ monocytes were seeded in a 96-well Seahorse culture microplate (Agilent, Santa Clara, CA, United States). at a density of 104 cells/well and incubated at 37°C with 5% CO2 in complete media supplemented with 20 ng/ml M-CSF (PeproTech by ThermoFisher Scientific) for 2 days before the assay. To assess the acute response to VSOP uptake on the oxygen consumption rate (OCR) and extracellular acidification rate (EACR), we utilized the T Cell Metabolic Profiling Kit (Agilent). Cells were treated with VSOP or Latex beads (30 nm size) by acute injections during the assay or VSOP treated 18 h before the assay. The injection ports were loaded as follows: VSOP (0.75 mM), Latex beads (Sigma-Aldrich by Merck KGaA, Darmstadt, Germany) (1.6 μL/mL)], Oligomycin (1 µM), BAM15 (5 µM), and Rotenone/Antimycin A (0.5 µM each). The assay was performed according to the manufacturer’s instructions. The XFe96 Seahorse Analyzer (Agilent) was run for 200 min, consisting of an 18-min basal measurement period prior to acute injections, 150 min during the acute injection phase, and 60 min post-injection with inhibitors. Spare respiratory capacity (SRC) was defined as the difference between maximal and basal respiration as % OCR.
2.10 Migratory behavior
For the migration assay, 1.5 × 104 CD14+ monocytes in 100 µL were seeded in a 96-well ImageLock plate (Sartorius AG, Göttingen, Germany) and allowed to reach confluency at 37°C with 5% CO2 over 3 days. The cells were then treated with VSOP (0.75 mM) or left untreated. After 3 hours, the cells were washed once with warm 1x PBS (Gibco by ThermoFisher Scietific) before introducing a scratch using the 96-Well WoundMaker Tool (Essen BioScience, Ann Arbor, United States). The cells were washed twice with 1x PBS to remove any floating cells, and fresh complete media was added. In an IncuCyte® S3 live-cell imaging system (Sartorius) images were captured using a ×20 objective in wide mode, with two images per well taken every 3 h for 3 days.
2.11 Statistical analysis
Statistical significance was determined using Kruskal-Wallis test followed by Dunn´s multiple comparison, Mann-Whitney U test and 2way ANOVA with p ≤ 0.05 defining it as statistically significant. Results are displayed as mean ± SD using GraphPad Prism (Version 6).
3 Results
3.1 Distribution and clearance of VSOP after intravenous administration
Previous studies on intestinal inflammation in mice demonstrated that intravenously (i.v.) administered VSOP serve as MRI contrast agents in the DSS-induced colitis model (Golusda et al., 2022). Since VSOP retention in the body is unknown, their accumulation and elimination were investigated. Transfer colitis was induced in mice, and after colitis onset on day 14, Eu-doped VSOP were injected i. v., allowing detection of VSOP via laser ablation-inductively coupled plasma-mass spectrometry (LA-ICP-MS). On days 3, 10, and 18 post-injection, the spleen, kidney, liver, ileum, cecum, colon, mesenteric lymph nodes, and tibia were excised for Eu analysis. As shown in Figure 1, on day 3, Eu-doped VSOP were detected in the spleen, liver, mesenteric lymph nodes, and tibia bone marrow but not in the kidney or intestine. By day 18, the Eu signal was undetectable in all organs, indicating complete VSOP clearance from the body.
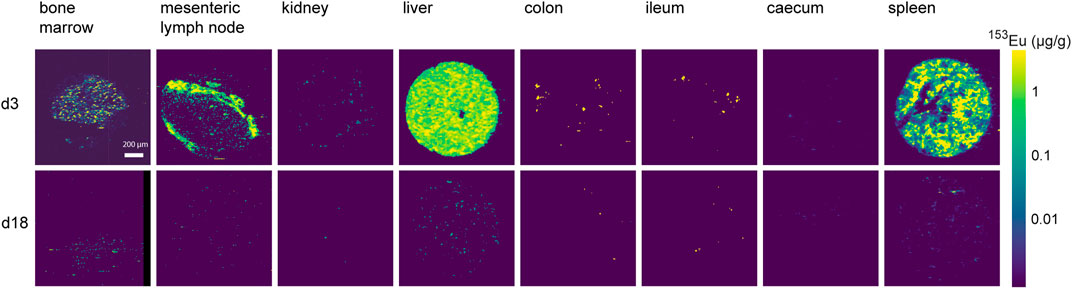
Figure 1. VSOP have no long-term persistence in the organs of mice. The Eu signal of Eu-doped VSOP was detected in various mouse organs by LA-ICP-MS. The upper row shows day 3, and the bottom row shows day 18 after i. v. injection. Data are representative for n = 3.
3.2 Monocytes are the main cell population interacting with VSOP
To identify immune cells interacting with the nanoparticles, whole blood from healthy volunteers was treated with Eu-doped VSOP, stained with a metal-conjugated antibody cocktail (Table 3), and analyzed by mass cytometry. Data analysis revealed monocytes as the primary interacting cells, with 70% ± 25% showing a positive signal for Eu (Figures 2A,B). In addition, 24% ± 12% of B cells interacted with VSOP (Figures 2A,B). In contrast, almost no Eu signal was detected in T cells, granulocytes (Figures 2A,B) or NK cells (not shown).
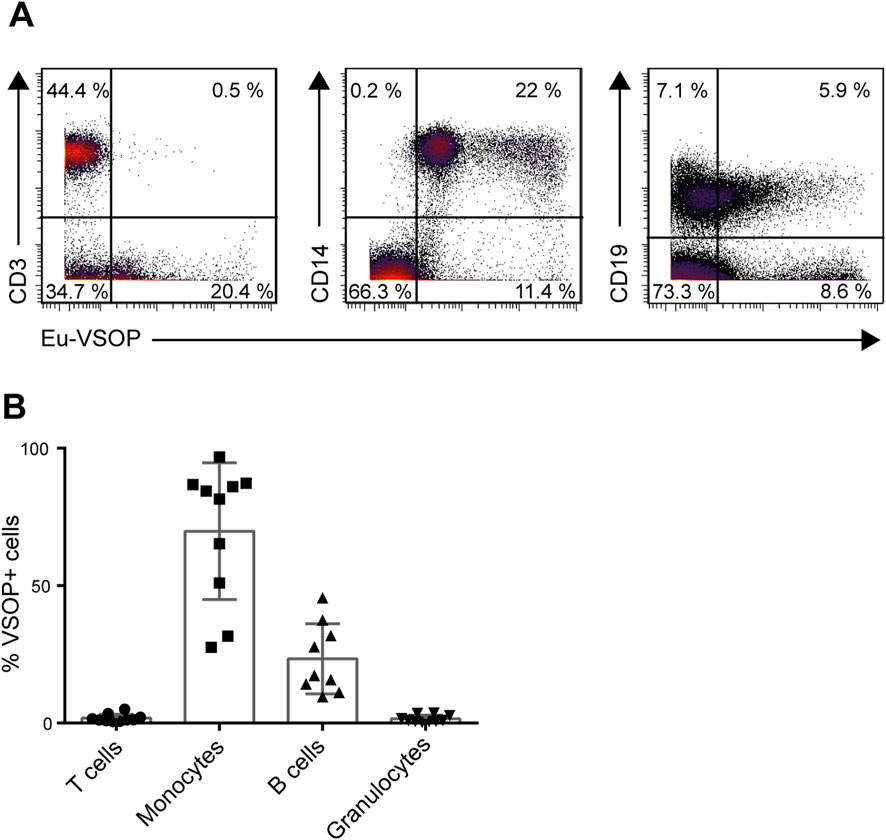
Figure 2. Monocytes and B cells incorporate or interact with VSOP. Whole blood was incubated with VSOP, stained for granulocytes, T cells, monocytes and B cells and analyzed by mass cytometry. (A) Cells were gated for CD45+ CD66b−. Plots are representative of n = 9-10 samples. (B) Percentage of cells incorporating VSOP in each immune subset. Data represent mean ± SD, n = 9-10 samples.
3.3 VSOP uptake preserves monocyte viability but alters IL-1β production
Monocytes, the primary immune cell subset interacting with VSOP, may bind to the glycocalyx or undergo phagocytosis. To assess whether VSOP affect monocyte viability or cytokine production, whole blood CD14+ monocytes were incubated with various VSOP concentrations, and cell viability was measured. VSOP treatment did not alter monocyte viability at any concentration (Figure 3A). Cytokine levels in the culture supernatant after 24 h showed that TNFα, IL-6, and IL-10 concentrations were similar in VSOP-treated and untreated monocytes (Figures 3B,C,E). However, IL-1β levels significantly decreased with VSOP treatment at all concentrations, though the effect was not dose-dependent (Figure 3D).
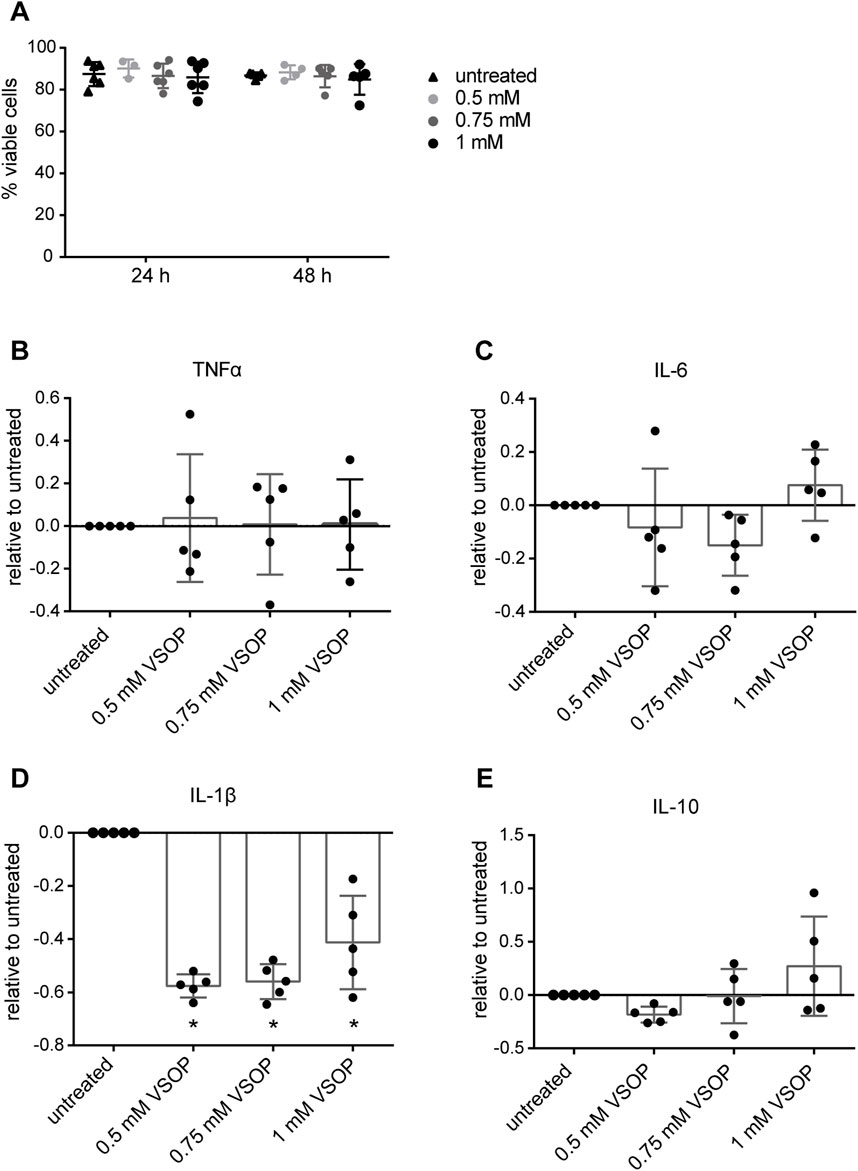
Figure 3. VSOP treatment does not affect monocyte viability and has minor effects on cytokine production. Primary human monocytes were incubated for 90 min with or without VSOP and cultured for 2 days. After 24 or 48 h of culture, monocytes were stained for viability and supernatant was taken for cytokine detection. (A) Shows monocyte viability (%) 24 and 48 h after VSOP treatment at different concentrations (circles) compared to untreated controls (triangle). (B–E) The supernatant of monocytes was collected 24 h after VSOP incubation and cytokines were detected by LegendPlex. Data show mean ± SD, n = 5. Statistical significance was tested with 2-way ANOVA relative to control (*p ≤ 0.05).
3.4 Transcriptional changes in monocytes after VSOP treatment
RNA sequencing of primary human monocytes treated with VSOP revealed transcriptional changes, with the transferrin receptor (TFRC) being one of the most significantly downregulated genes. The downregulation of TFRC likely restricts further iron uptake in response to increased intracellular iron from VSOP treatment (Figure 4A).
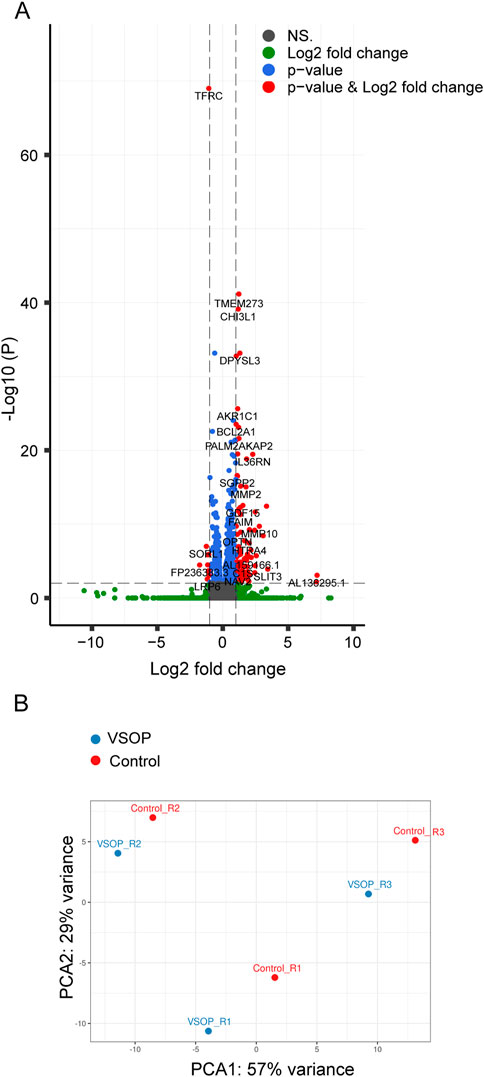
Figure 4. VSOP do not significantly affect the RNA expression profile of monocytes. (A) Volcano plot of RNA sequencing from monocytes of healthy volunteers incubated with or without VSOP. (B) Principal component analysis (PCA) of RNA-seq data from monocytes from healthy volunteers incubated with (blue) or without (red) VSOP. Data represent n = 3.
Principal component analysis showed distinct clustering between donors, with treated and untreated samples from the same donor, indicating minimal variation in transcriptomes due to VSOP (Figure 4B). Notable changes included increased expression of MMP2, MMP10, IL36B, IL36RN, IL24, CCL2, CCL7, and CHI3L1.
3.5 VSOP-treated monocytes exhibit neither increased activation nor proliferation
Small effects of VSOP on transcriptomics of monocytes from healthy volunteers have already been observed. In chronic inflammation, such as in IBD patients, the immune system is often activated, raising the question of whether VSOP treatment affects immune cells, particularly monocytes, in IBD patients. To investigate this, blood from IBD patients with ulcerative colitis (n = 6) and Crohn´s disease (n = 4) was incubated with VSOP for 30 min, and whole blood cells were analyzed using CyTOF. Clustering of immune cells from the blood shows no major differences in the composition of cell clusters (Figure 5A). Furthermore, gating on the major immune cell subsets revealed no differences in the frequencies of B cells, T cells, monocytes and granulocytes (Figure 5B). A deeper analysis of the monocyte compartment revealed no significant differences in cell proliferation (Ki67 expression) (Figure 5C). In addition, markers of monocyte activation (HLA-DR, CD80, CD86, CD38, CX3CR1, CD11c, CD62L) were not differentially expressed by VSOP-treated monocytes (Figure 5C). The expression of markers indicating a shift towards classically or alternatively activated macrophages did not change after VSOP treatment. This was also true for B cells, T cells, and granulocytes, which showed no significant regulation (data not shown).
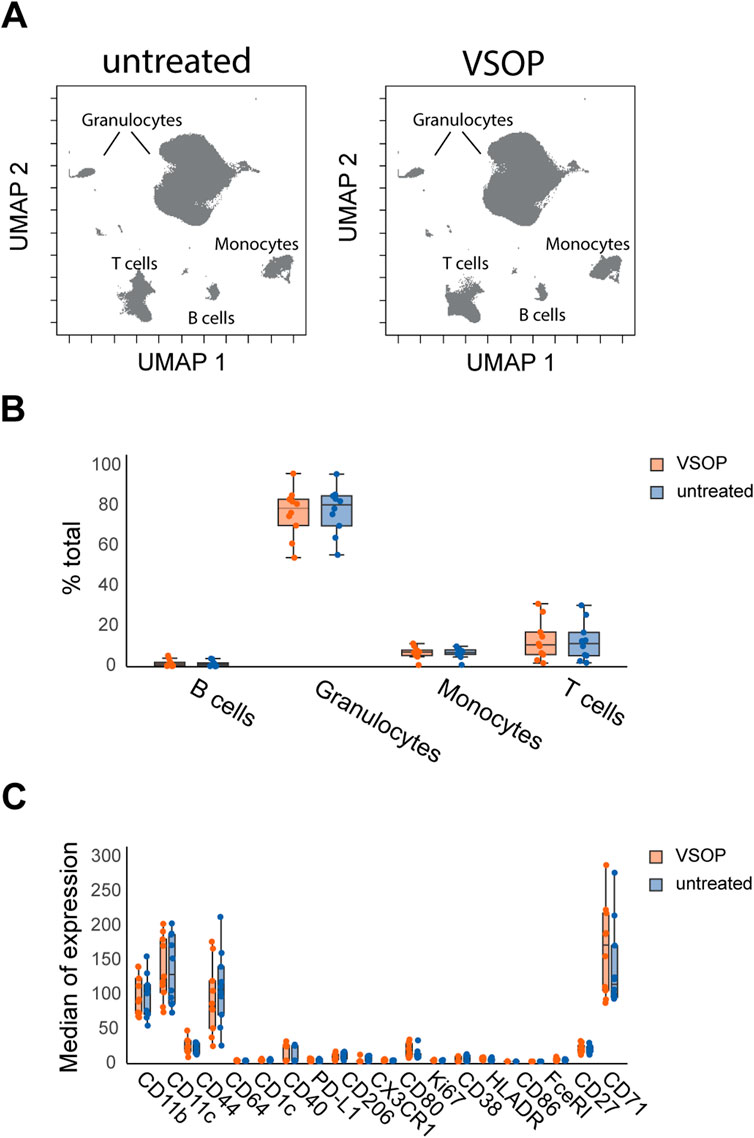
Figure 5. VSOP do not affect immune cell abundance, activation or proliferation. (A) Uniform manifold approximation and projection (UMAP) of whole blood cells treated with or without VSOP. (B) Immune cell abundance of whole blood after treatment with (red) or without (blue) VSOP. Boxes extend from first to third percentiles and the line shows the median cell frequencies. Whiskers show min/max values; n = 10. (C) Marker expression of monocytes treated with (red) or without (blue) VSOP. Boxes extend from first to third percentiles and the line shows the median expression. Whiskers show min/max values; n = 10. Statistical significance was tested with Mann-Whitney U test.
3.6 Minor influence of VSOP on the transcriptome of whole blood cells from IBD patients
The impact of VSOP on the transcriptome of monocytes from healthy donors was minimal, but may be more pronounced in IBD patients due to pre-activation. To assess this, whole blood from IBD patients with active UC and CD (n = 3 each) was analyzed, as CyTOF showed interactions between VSOP and not only monocytes but also B cells, T cells, granulocytes and NK cells, though to a lesser extent. Only three non-coding genes were differentially regulated (C3orf86P, ENSG00000278996, ENSG00000259002) (Figure 6A). Principal component analysis revealed distinct clustering between donors, with treated and untreated samples from the same donor clustering together (Figure 6B).
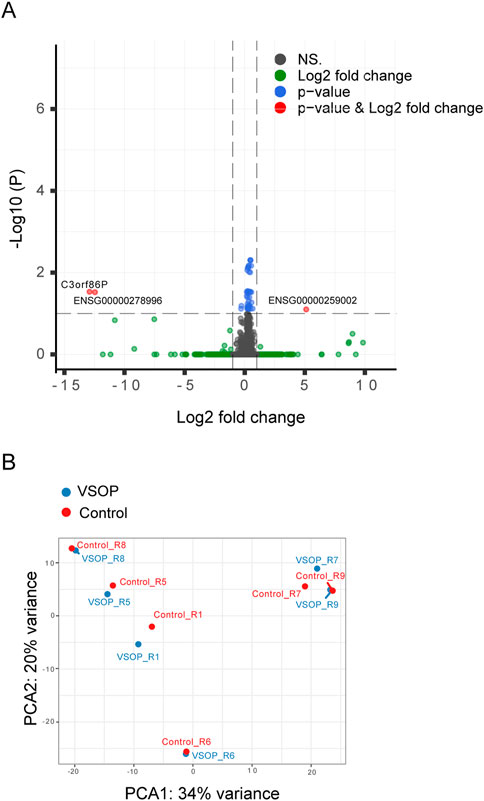
Figure 6. VSOP have minimal impact on the RNA expression profile of cells from whole blood of IBD patients. (A) Volcano plot of RNA sequencing from whole blood cells of IBD patients with active disease incubated with or without VSOP. (B) PCA analysis of RNA sequencing from whole blood cells from IBD patients with active disease incubated with (red) or without (blue) VSOP. Data represent n = 6.
3.7 Monocyte metabolism is primarily driven by phagocytic activity, not VSOP treatment
Treatment with VSOP (n = 9) and latex beads (n = 3) as a phagocytosis control increased both oxygen consumption rate (OCR) and extracellular acidification rate (EACR) in monocytes. Maximal respiratory capacity remained unchanged, while spare respiratory capacity (SRC) significantly decreased (Figures 7A,B). In addition, increased glycolytic and mitochondrial ATP production, along with higher OCR after rotenone/antimycin A injection, indicated enhanced non-mitochondrial oxygen consumption. Both VSOP or latex beads shifted monocyte metabolism to a more energetic state, utilizing both mitochondrial oxidative phosphorylation (OXPHOS) and glycolysis (Figure 7C). When VSOP treatment was administered 18 h before the assay, the SRC decrease was balanced to the level of untreated monocytes, suggesting that the shift in metabolism was due to phagocytosis (Figure 7D).
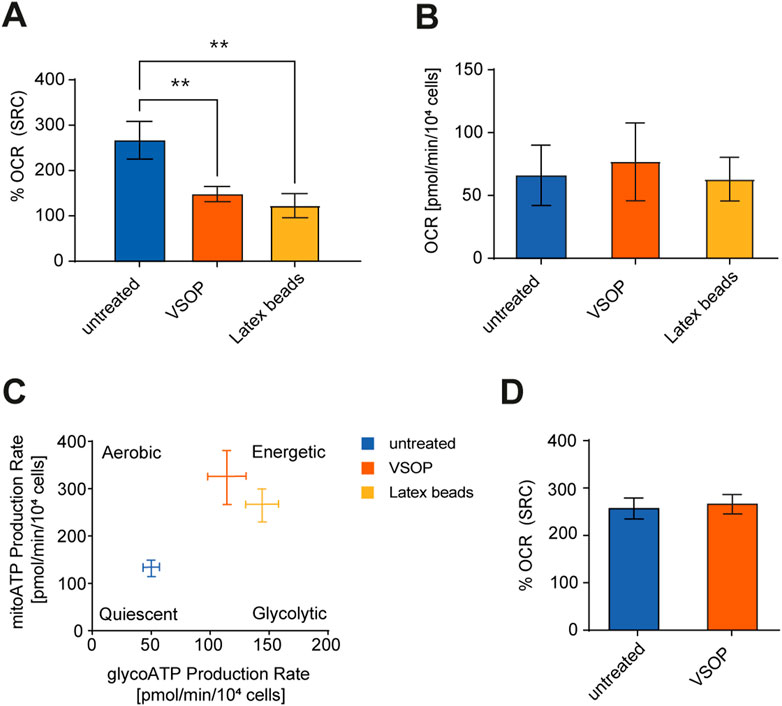
Figure 7. VSOP treatment alters monocyte respiration due to phagocytic activity of the cells. (A) Spare respiratory capacity of monocytes with (red) or without (blue) acute injection of VSOP or latex beads (yellow). (B) Maximal respiration of monocytes with (red) or without (blue) acute injection of VSOP or latex beads (yellow). (C) Energetic map of the metabolic status of monocytes treated with (red) or without (blue) VSOP or latex beads (yellow) by acute injection. Data in A, B, C show mean ± SD n = 9 (untreated, VSOP) or n = 3 (latex beads). (D) Spare respiratory capacity of monocytes with (red) or without (blue) VSOP pretreatment. Data show mean ± SD n = 3. Statistical significance was tested using Kruskal-Wallis test followed by Dunn´s multiple comparison; *p < 0.05, **p < 0.01.
3.8 VSOP treatment does not affect monocyte migratory behavior
Since VSOP increased glycolysis, we hypothesized they might affect CD14+ monocyte migration. To test this, CD14+ monocytes were treated with VSOP or left untreated, and a wound scratch was made on the cell monolayer. Cells were imaged for 3 days, and wound closure was calculated. The results showed no significant difference in migratory ability between the treatment groups (Figure 8).
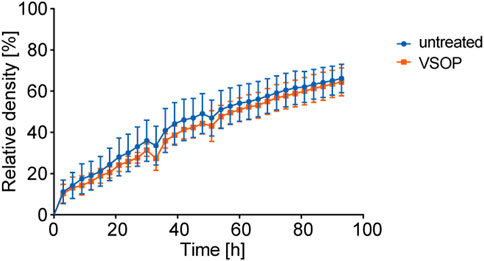
Figure 8. Migratory behavior of monocytes remains unaffected by VSOP treatment. Increase of relative cell density (%) after scratching the monolayer of monocytes treated with VSOP (red) or left untreated (blue). Shown are mean ± SD for n = 5.
4 Discussion
Iron oxide nanoparticles (IONP) have garnered significant attention in biomedical research and clinical applications, including cancer therapy (Shestovskaya et al., 2023; Shubhra, 2023), cell labeling (Pohland et al., 2022; Tefft et al., 2015) targeted drug delivery (Kumar et al., 2019), and MRI (Barrow et al., 2017). Inflammatory diseases such as atherosclerosis (Wagner et al., 2013; Haase et al., 2024) multiple sclerosis (Millward et al., 2013), and IBD (Golusda et al., 2022) are key areas where IONP may offer diagnostic or therapeutic benefits.
Studies exploring the interaction of IONP with macrophages have yielded conflicting results, largely due to variability in nanoparticle size, coating, concentration, exposure time, and experimental conditions, including the type and activation state of immune cells (Chen et al., 2018; Wu et al., 2013; Laskar et al., 2013; Mulens-Arias et al., 2021). Upon intravenous administration, VSOP interact primarily with monocytes and macrophages, raising questions about potential cytotoxicity, phenotypic alterations, and clearance mechanisms (Ludwig et al., 2013; Berndt et al., 2017).
Using a murine model of intestinal inflammation, we analyzed the accumulation and retention of VSOP in vivo. While VSOP were undetectable after 18 days, a previous study found positive iron staining of VSOP-labeled adipose tissue-derived stromal cells after 28 days (Radeloff et al., 2020). However, those in vitro findings differ from our in vivo results, where colitic mice received VSOP i. v. Given that monocytes and, to a lesser extent, B cells are the primary blood cells interacting with VSOP, this difference may be due to administration routes. Stromal cells in vivo may not encounter i. v. applied VSOP. Similarly, while a study showed that T cells from mycobacteria-infected mice could be labeled with VSOP (Wuerfel et al., 2011), our in vitro whole blood experiments found no VSOP interaction with T cells. Discrepancies may stem from different experimental conditions, as the cited study used up to 9 mM iron per mL for 4 days (Wuerfel et al., 2011), whereas whole blood was incubated with 0.75 mM VSOP for 30 min–a concentration successfully applied in mouse MRI (Golusda et al., 2022). These variations highlight how cell composition, culture conditions, and administration routes influence VSOP interactions. Regarding cell composition in the study by Wuerfel et al. isolated murine T cells were used. Whereas in our study whole human blood was treated with VSOP. Since monocytes phagocytose VSOP within minutes to hours the cells compete with the substrate. The culture conditions rather than incubation time (4 days versus 30 min) as well as the amount of iron particles allow for interaction with T cells.
Research on VSOP’s effect on the immune system remains limited. To investigate potential immune modulation, bulk RNA sequencing was performed on human monocytes, given their primary interaction with VSOP in vitro and in vivo. Surprisingly, transcriptomic analysis revealed only minor effects, with the most significantly downregulated gene encoding for the TFRC. This likely reflects an adaptive response to increased intracellular iron, and feedback regulation to prevent iron overload (Rojas et al., 2016; Wu et al., 2017; Yi et al., 2022). However, TFRC is not involved in VSOP uptake as citrate-coated VSOP have been shown to be internalized via electrostatic interactions with sulfated GAGs on cell surfaces (Ludwig et al., 2013; Berndt et al., 2017). Once internalized, these nanoparticles are trafficked to lysosomes, where the acidic environment facilitates their degradation, contributing to the systemic iron pool (Maraloiu et al., 2016; Malvindi et al., 2014; Portilla et al., 2022). Simultaneously, upregulation of genes linked to tissue remodeling (MMP2, MMP10), immunoregulation (IL36RN, IL24), and cell migration (CCL2, CCL7) was observed, without suppression of pro-IL-1β transcripts. This reinforces the idea that cytokine modulation occurs post-transcriptionally.
Previous studies suggest that cell activation and inflammation enhance VSOP interactions due to altered GAG expression (Golusda et al., 2022; Berndt et al., 2017). To examine whether inflammation amplifies VSOP effects, bulk RNA sequencing was performed on whole blood cells from IBD patients with active ulcerative colitis or Crohn´s disease. Given that monocytes from these patients are already activated (Andus et al., 1991; Mazlam and Hodgson, 1992; Aschenbrenner et al., 2021), they may respond differently to VSOP than monocytes from healthy donors. Other immune cells, including B cells, which showed slight uptake of VSOP in vitro, and T cells, which are key players in IBD (Gomez-Bris et al., 2023), are also activated in the blood of IBD patients with active disease (Funderburg et al., 2013; Rabe et al., 2019; Noronha et al., 2009). Surprisingly, only three non-coding genes were significantly different after VSOP treatment (C3orf86P, ENSG00000278996, ENSG00000259002). The functional relevance of these remains unclear and warrants further study. In general, there are many non-coding RNAs identified, differing in size and type. This includes long non-coding RNAs, circular RNAs, PIWI-interacting RNAs, ribosomal RNAs, small nuclear RNAs, transfer RNAs and micro RNAs (Nemeth et al., 2024). But the function of the majority of non-coding RNAs still needs to be elucidated. Thus, VSOP treatment of monocytes or whole blood in vitro did not alter the transcription of functionally relevant genes. A limitation of this transcriptomic analysis was the small sample size and inter-individual variability, which may have masked subtle changes. Future studies with larger, stratified cohorts will be needed to explore this further.
Functional analyses further confirmed minimal VSOP effects on immune cells. VSOP did not impact immune cell survival, indicating no cytotoxicity, consistent with other studies (Remmo et al., 2022; Boosz et al., 2021; Radeloff et al., 2021). While one study reported dose-dependent cytotoxic effect of citrate-coated VSOP (8.6 nm diameter) on mouse C17.2 cells (Soenen et al., 2011), those results may reflect differences in VSOP synthesis, treatment conditions and cell type. Unlike primary human monocytes, immortalized C17.2 cells may not accurately model normal cell behavior (Snyder et al., 1992). In murine organotypic hippocampal slice cultures, VSOP treatment altered cell viability within the dentate gyrus and the hippocampus (Pohland et al., 2017). Again, the use of slice cultures does not reflect the behavior of primary cells. Organotypic culture on porous membranes may be well suited for developmental studies of synaptic connectivity and plasticity in hippocampus as well as for drug distribution (Stoppini et al., 1991; Loryan et al., 2013). For assessing neurotoxicity, other models seem more appropriate e.g., using human induced pluripotent stem cell-derived neurons, co-culture of human neurons and astrocytes, or human brain organoids (Scholz et al., 2022; De Simone et al., 2017; Liu et al., 2023). Cytotoxicity of the fast-growing mouse neuroblastoma cell line N2a induced by superparamagnetic iron oxide nanoparticles in increasing concentrations (0, 25, 50, 100, 200 and 300 μg/mL) was detected after 72 h by trypan blue exclusion method (Abad Tan et al., 2019). Cytotoxic effects were detected at concentrations of ≥100 μg/mL (Abad Tan et al., 2019). Again, comparability to VSOP used in our study is difficult as their superparamagnetic iron oxide nanoparticles were of spherical shape, had a size of 10–15 nm and were incubated for 72 h on a fast-growing cell line.
VSOP also had no effect on immune cell abundance or proliferation. Current literature on nanoparticle-induced proliferation varies widely depending on nanoparticle type and application. Some nanoparticles inhibit cancer cell proliferation–a desired effect that depends on their composition, coating, and size (Villalobos Gutierrez et al., 2023). For example, BiFe2O4@Ag nanoparticles were designed for gastric cancer therapy due to their silver and bismuth content (Shamsi et al., 2024). In contrast, VSOP were specifically developed for inert behavior in diagnostic applications. In line with this, proliferation and migration capability were also studied in human adipose tissue-derived stromal cells after labeling with VSOP for potential cell-based therapeutic approaches (Radeloff et al., 2021). Proliferation, migration capability and differentiation potential were not affected by the procedure (Radeloff et al., 2021). Additionally, VSOP labeling of human mesenchymal stem cells did not affect viability or proliferation potential (Heymer et al., 2008). Furthermore, iron incorporation had no impact on human mesenchymal stem cells’ ability to undergo adipogenic, osteogenic or chondrogenic differentiation (Heymer et al., 2008).
Phenotypic analysis of immune cell subsets, particularly monocytes, revealed no change in activation marker expression. Correspondingly, IL-1β secretion was attenuated, while TNFα and IL-6 levels remained unchanged regardless of concentration. This aligns with previous studies where both inhibitory and stimulatory effects of IONP on IL-1β, depending on size and cell type were demonstrated (Chen et al., 2018; Wu et al., 2013).
Our mitochondrial stress assay showed elevated OCR in acutely treated cells, consistent with increased energy demand for phagocytosis, likely via glycolysis and fatty acid oxidation (Cline and Lehrer, 1968; Reiss and Roos, 1978; Zhu et al., 2019). Notably, basal respiration remained unchanged in pre-treated monocytes, suggesting the absence of ROS-induced stress. Additionally, evidence suggests that ROS (e.g., NO, O2−) interfere with OCR, rendering cells unresponsive to OXPHOS modulators and can drive inflammasome activation (Christopoulos et al., 2021). Taken together, our data suggest that VSOP inhibit IL-1β post-transcriptionally, likely via inflammasome inhibition.
Collectively, our findings contrast with studies using larger or differently coated IONP (e.g., DMSA, APS, PEI), which have shown phenotype alterations in macrophage subtypes (Mulens-Arias et al., 2021; Rojas et al., 2016). Our results support the notion that coating and size critically influence biological effects, with our citrate-coated VSOP demonstrating a favorable interaction profile.
Overall, our findings demonstrate that VSOP are efficiently cleared through physiological iron-handling mechanisms without inducing toxicity or inflammatory exacerbation. The selective IL-1β reduction without broader immune activation along with the absence of phenotypic or migratory changes, underscores their safety. Their favorable size and coating properties minimize off-target immune activation while allowing for effective labeling and imaging of monocytes/macrophages in inflammatory disease contexts such as atherosclerosis and multiple sclerosis. Future studies will aim to elucidate the molecular VSOP uptake mechanism and metabolic fate in long-term exposure in chronic inflammation models.
Data availability statement
The data presented in this study are deposited on Zenodo repository; DOI: 10.5281/zenodo.15388312.
Ethics statement
Experiments with human material was performed according to The Code of Ethics of the World Medical Association (Declaration of Helsinki) and has been approved of by the Ethics Committee of the Charité – Universitätsmedizin Berlin (EA1/181/17 and EA1/273/21). The studies were conducted in accordance with the local legislation and institutional requirements. The participants provided their written informed consent to participate in this study. The animal study was approved by Landesamt für Gesundheit und Soziales, registration number G0117/20. The study was conducted in accordance with the local legislation and institutional requirements.
Author contributions
SH: Formal Analysis, Investigation, Writing – original draft. AK: Conceptualization, Funding acquisition, Supervision, Writing – original draft, Formal Analysis. LG: Investigation, Writing – review and editing, Conceptualization, Formal Analysis. CP: Formal Analysis, Writing – review and editing, Data curation. NH: Investigation, Writing – review and editing. GS: Writing – review and editing, Data curation, Formal Analysis. CF: Resources, Writing – review and editing. HT: Formal Analysis, Writing – review and editing, Data curation. MS: Formal Analysis, Writing – review and editing, Data curation, Investigation. ZT: Writing – review and editing, Supervision. MT: Writing – review and editing, Project administration. BS: Conceptualization, Funding acquisition, Supervision, Writing – review and editing. DP: Conceptualization, Formal Analysis, Supervision, Visualization, Writing – original draft, Investigation, Writing – review and editing.
Funding
The author(s) declare that financial support was received for the research and/or publication of this article. The study was supported by the German Research Foundation: CRC 1340 (project number 372486779) (to AK, CF, DP, HT, MT, SH), and TRR 241 (project number 375876048) (to AK, CP, GS, ZT). BS is supported by the German Research Foundation: CRC-TRR 241-B01 and Z02 (project number 375876048), CRU 5023 (project number 50474582), CRC 1449-B04 and Z02 (project number 431232613), CRC 1340-B06 (project number 372486779).
Acknowledgments
The authors would like to thank the BIH/MDC Genomics Platform and BIH Cytometry Core Facility for technical support.
Conflict of interest
The authors declare that the research was conducted in the absence of any commercial or financial relationships that could be construed as a potential conflict of interest.
Generative AI statement
The authors declare that no Generative AI was used in the creation of this manuscript.
Publisher’s note
All claims expressed in this article are solely those of the authors and do not necessarily represent those of their affiliated organizations, or those of the publisher, the editors and the reviewers. Any product that may be evaluated in this article, or claim that may be made by its manufacturer, is not guaranteed or endorsed by the publisher.
References
Abad Tan, S., Zoidl, G., and Ghafar-Zadeh, E. (2019). A multidisciplinary approach toward High throughput label-free cytotoxicity monitoring of superparamagnetic iron oxide nanoparticles. Bioeng. (Basel) 6 (2), 52. doi:10.3390/bioengineering6020052
Andus, T., Gross, V., Casar, I., Krumm, D., Hosp, J., David, M., et al. (1991). Activation of monocytes during inflammatory bowel disease. Pathobiology 59 (3), 166–170. doi:10.1159/000163637
Aschenbrenner, D., Quaranta, M., Banerjee, S., Ilott, N., Jansen, J., Steere, B., et al. (2021). Deconvolution of monocyte responses in inflammatory bowel disease reveals an IL-1 cytokine network that regulates IL-23 in genetic and acquired IL-10 resistance. Gut 70 (6), 1023–1036. doi:10.1136/gutjnl-2020-321731
Avila-Quezada, G. D., Ingle, A. P., Golinska, P., and Rai, M. (2022). Strategic applications of nano-fertilizers for sustainable agriculture: benefits and bottlenecks. Nanotechnol. Rev. 11 (1), 2123–2140. doi:10.1515/ntrev-2022-0126
Baioco, K. S., Pereira, R., Ferreira-Goncalves, T., Coelho, J. M. P., Gaspar, M. M., and Reis, C. P. (2024). Combining phototherapy and gold-based nanomaterials: a breakthrough in basal cell carcinoma treatment. Int. J. Mol. Sci. 25 (21), 11494. doi:10.3390/ijms252111494
Barrow, M., Taylor, A., Fuentes-Caparros, A. M., Sharkey, J., Daniels, L. M., Mandal, P., et al. (2017). SPIONs for cell labelling and tracking using MRI: magnetite or maghemite? Biomater. Sci. 6 (1), 101–106. doi:10.1039/c7bm00515f
Bazile, D., Prud'homme, C., Bassoullet, M. T., Marlard, M., Spenlehauer, G., Veillard, M., et al. (1995). PEG-PLA nanoparticles avoid uptake by the mononuclear phagocytes system. J. Pharm. Sci. 84 (4), 493–498. doi:10.1002/jps.2600840420
Berndt, D., Millward, J. M., Schnorr, J., Taupitz, M., Stangl, V., Paul, F., et al. (2017). Inflammation-induced brain endothelial activation leads to uptake of electrostatically stabilized iron oxide nanoparticles via sulfated glycosaminoglycans. Nanomedicine 13 (4), 1411–1421. doi:10.1016/j.nano.2017.01.010
Blanco-Fernandez, B., Castano, O., Mateos-Timoneda, M. A., Engel, E., and Perez-Amodio, S. (2021). Nanotechnology approaches in chronic wound healing. Adv. Wound Care (New Rochelle) 10 (5), 234–256. doi:10.1089/wound.2019.1094
Bonafe, R., Coppo, A., Queliti, R., Bussi, S., Maisano, F., Kirchin, M. A., et al. (2023). Gadolinium retention in a rat model of subtotal renal failure: are there differences among macrocyclic GBCAs? Eur. Radiol. Exp. 7 (1), 7. doi:10.1186/s41747-023-00324-1
Boosz, P., Pfister, F., Stein, R., Friedrich, B., Fester, L., Band, J., et al. (2021). Citrate-coated superparamagnetic iron oxide nanoparticles enable a stable non-spilling loading of T cells and their magnetic accumulation. Cancers (Basel) 13 (16), 4143. doi:10.3390/cancers13164143
Chen, C., Ge, J., Gao, Y., Chen, L., Cui, J., Zeng, J., et al. (2022). Ultrasmall superparamagnetic iron oxide nanoparticles: a next generation contrast agent for magnetic resonance imaging. Wiley Interdiscip. Rev. Nanomed Nanobiotechnol 14 (1), e1740. doi:10.1002/wnan.1740
Chen, S., Chen, S., Zeng, Y., Lin, L., Wu, C., Ke, Y., et al. (2018). Size-dependent superparamagnetic iron oxide nanoparticles dictate interleukin-1β release from mouse bone marrow-derived macrophages. J. Appl. Toxicol. 38 (7), 978–986. doi:10.1002/jat.3606
Cho, W. S., Cho, M., Jeong, J., Choi, M., Cho, H. Y., Han, B. S., et al. (2009). Acute toxicity and pharmacokinetics of 13 nm-sized PEG-coated gold nanoparticles. Toxicol. Appl. Pharmacol. 236 (1), 16–24. doi:10.1016/j.taap.2008.12.023
Choi, C. H., Zuckerman, J. E., Webster, P., and Davis, M. E. (2011). Targeting kidney mesangium by nanoparticles of defined size. Proc. Natl. Acad. Sci. U. S. A. 108 (16), 6656–6661. doi:10.1073/pnas.1103573108
Christopoulos, P. F., Grigalavicius, M., Corthay, A., Berg, K., and Theodossiou, T. A. (2021). Reactive species from two-signal activated macrophages interfere with their oxygen consumption measurements. Antioxidants (Basel) 10 (7), 1149. doi:10.3390/antiox10071149
Cline, M. J., and Lehrer, R. I. (1968). Phagocytosis by human monocytes. Blood 32 (3), 423–435. doi:10.1182/blood.v32.3.423.423
Darrah, T. H., Prutsman-Pfeiffer, J. J., Poreda, R. J., Ellen Campbell, M., Hauschka, P. V., and Hannigan, R. E. (2009). Incorporation of excess gadolinium into human bone from medical contrast agents. Metallomics 1 (6), 479–488. doi:10.1039/b905145g
de Schellenberger, A. A., Hauptmann, R., Millward, J. M., Schellenberger, E., Kobayashi, Y., Taupitz, M., et al. (2017). Synthesis of europium-doped VSOP, customized enhancer solution and improved microscopy fluorescence methodology for unambiguous histological detection. J. Nanobiotechnol. 15 (1), 71. doi:10.1186/s12951-017-0301-6
De Simone, U., Caloni, F., Gribaldo, L., and Coccini, T. (2017). Human Co-culture model of neurons and astrocytes to test acute cytotoxicity of neurotoxic compounds. Int. J. Toxicol. 36 (6), 463–477. doi:10.1177/1091581817739428
Di Gregorio, E., Ferrauto, G., Furlan, C., Lanzardo, S., Nuzzi, R., Gianolio, E., et al. (2018). The issue of gadolinium retained in tissues: insights on the role of metal complex stability by comparing metal uptake in murine tissues upon the concomitant administration of lanthanum- and gadolinium-diethylentriamminopentaacetate. Invest Radiol. 53 (3), 167–172. doi:10.1097/rli.0000000000000423
Dobin, A., Davis, C. A., Schlesinger, F., Drenkow, J., Zaleski, C., Jha, S., et al. (2013). STAR: ultrafast universal RNA-seq aligner. Bioinformatics 29 (1), 15–21. doi:10.1093/bioinformatics/bts635
Durocher, I., Noel, C., Lavastre, V., and Girard, D. (2017). Evaluation of the in vitro and in vivo proinflammatory activities of gold (+) and gold (-) nanoparticles. Inflamm. Res. 66 (11), 981–992. doi:10.1007/s00011-017-1078-7
Ewels, P. A., Peltzer, A., Fillinger, S., Patel, H., Alneberg, J., Wilm, A., et al. (2020). The nf-core framework for community-curated bioinformatics pipelines. Nat. Biotechnol. 38 (3), 276–278. doi:10.1038/s41587-020-0439-x
Freise, C., Biskup, K., Blanchard, V., Schnorr, J., and Taupitz, M. (2024). Inorganic phosphate-induced extracellular vesicles from vascular smooth muscle cells contain elevated levels of hyaluronic acid, which enhance their interaction with very small superparamagnetic iron oxide particles. Int. J. Mol. Sci. 25 (5), 2571. doi:10.3390/ijms25052571
Frenzel, T., Apte, C., Jost, G., Schockel, L., Lohrke, J., and Pietsch, H. (2017). Quantification and assessment of the chemical form of residual gadolinium in the brain after repeated administration of gadolinium-based contrast agents: comparative study in rats. Invest Radiol. 52 (7), 396–404. doi:10.1097/rli.0000000000000352
Funderburg, N. T., Stubblefield Park, S. R., Sung, H. C., Hardy, G., Clagett, B., Ignatz-Hoover, J., et al. (2013). Circulating CD4(+) and CD8(+) T cells are activated in inflammatory bowel disease and are associated with plasma markers of inflammation. Immunology 140 (1), 87–97. doi:10.1111/imm.12114
Gathings, R. M., Reddy, R., Santa Cruz, D., and Brodell, R. T. (2015). Gadolinium-Associated plaques A new, distinctive clinical entity. Jama Dermatol. 151 (3), 316–319. doi:10.1001/jamadermatol.2014.2660
Golusda, L., Kuhl, A. A., Lehmann, M., Dahlke, K., Mueller, S., Boehm-Sturm, P., et al. (2022). Visualization of inflammation in experimental colitis by magnetic resonance imaging using very small superparamagnetic iron oxide particles. Front. Physiol. 13, 862212. doi:10.3389/fphys.2022.862212
Gomez-Bris, R., Saez, A., Herrero-Fernandez, B., Rius, C., Sanchez-Martinez, H., and Gonzalez-Granado, J. M. (2023). CD4 T-cell subsets and the pathophysiology of inflammatory bowel disease. Int. J. Mol. Sci. 24 (3), 2696. doi:10.3390/ijms24032696
Gordon, K. B., Warren, R. B., Gottlieb, A. B., Blauvelt, A., Thaci, D., Leonardi, C., et al. (2021). Long-term efficacy of certolizumab pegol for the treatment of plaque psoriasis: 3-year results from two randomized phase III trials (CIMPASI-1 and CIMPASI-2). Br. J. Dermatol 184 (4), 652–662. doi:10.1111/bjd.19393
Gschwend, P. M., Hintze, J. M., Herrmann, I. K., Pratsinis, S. E., and Starsich, F. H. L. (2021). Precision in thermal therapy: clinical requirements and solutions from nanotechnology. Adv. Ther-Germany 4 (2). doi:10.1002/adtp.202000193
Gupta, V., Mohapatra, S., Mishra, H., Farooq, U., Kumar, K., Ansari, M. J., et al. (2022). Nanotechnology in cosmetics and cosmeceuticals-A review of latest advancements. Gels 8 (3), 173. doi:10.3390/gels8030173
Haase, H., Fahmi, A., and Mahltig, B. (2014). Impact of silver nanoparticles and silver ions on innate immune cells. J. Biomed. Nanotechnol. 10 (6), 1146–1156. doi:10.1166/jbn.2014.1784
Haase, T., Ludwig, A., Stach, A., Mohtashamdolatshahi, A., Hauptmann, R., Mundhenk, L., et al. (2024). Repeated injection of very small superparamagnetic iron oxide particles (VSOPs) in murine atherosclerosis: a safety study. Nanomater. (Basel) 14 (9), 773. doi:10.3390/nano14090773
Hersh, A. M., Alomari, S., and Tyler, B. M. (2022). Crossing the blood-brain barrier: advances in nanoparticle technology for drug delivery in neuro-oncology. Int. J. Mol. Sci. 23 (8), 4153. doi:10.3390/ijms23084153
Hetta, H. F., Ramadan, Y. N., Al-Harbi, A. I., E, A. A., Battah, B., Abd Ellah, N. H., et al. (2023). Nanotechnology as a promising approach to combat multidrug resistant bacteria: a comprehensive review and future perspectives. Biomedicines 11 (2), 413. doi:10.3390/biomedicines11020413
Heymer, A., Haddad, D., Weber, M., Gbureck, U., Jakob, P. M., Eulert, J., et al. (2008). Iron oxide labelling of human mesenchymal stem cells in collagen hydrogels for articular cartilage repair. Biomaterials 29 (10), 1473–1483. doi:10.1016/j.biomaterials.2007.12.003
Kanda, T., Fukusato, T., Matsuda, M., Toyoda, K., Oba, H., Kotoku, J., et al. (2015). Gadolinium-based contrast agent accumulates in the brain even in subjects without severe renal dysfunction: evaluation of autopsy brain specimens with inductively coupled plasma mass spectroscopy. Radiology 276 (1), 228–232. doi:10.1148/radiol.2015142690
Kotecha, N., Krutzik, P. O., and Irish, J. M. (2010). Web-based analysis and publication of flow cytometry experiments. Curr. Protoc. Cytom. 53. doi:10.1002/0471142956.cy1017s53
Kumar, R., Nayak, M., Sahoo, G. C., Pandey, K., Sarkar, M. C., Ansari, Y., et al. (2019). Iron oxide nanoparticles based antiviral activity of H1N1 influenza A virus. J. Infect. Chemother. 25 (5), 325–329. doi:10.1016/j.jiac.2018.12.006
Laskar, A., Eilertsen, J., Li, W., and Yuan, X. M. (2013). SPION primes THP1 derived M2 macrophages towards M1-like macrophages. Biochem. Biophys. Res. Commun. 441 (4), 737–742. doi:10.1016/j.bbrc.2013.10.115
Lesley, J., Hascall, V. C., Tammi, M., and Hyman, R. (2000). Hyaluronan binding by cell surface CD44. J. Biol. Chem. 275 (35), 26967–26975. doi:10.1016/s0021-9258(19)61467-5
Liu, L., Wang, J., Zhang, J., Huang, C., Yang, Z., and Cao, Y. (2023). The cytotoxicity of zinc oxide nanoparticles to 3D brain organoids results from excessive intracellular zinc ions and defective autophagy. Cell Biol. Toxicol. 39 (1), 259–275. doi:10.1007/s10565-021-09678-x
Loftus, E. V., Colombel, J. F., Schreiber, S., Randall, C. W., Regueiro, M., Ali, T., et al. (2016). Safety of long-term treatment with certolizumab pegol in patients with crohn's disease, based on a pooled analysis of data from clinical trials. Clin. Gastroenterol. Hepatol. 14 (12), 1753–1762. doi:10.1016/j.cgh.2016.07.019
Loryan, I., Friden, M., and Hammarlund-Udenaes, M. (2013). The brain slice method for studying drug distribution in the CNS. Fluids Barriers CNS 10 (1), 6. doi:10.1186/2045-8118-10-6
Love, M. I., Huber, W., and Anders, S. (2014). Moderated estimation of fold change and dispersion for RNA-seq data with DESeq2. Genome Biol. 15 (12), 550. doi:10.1186/s13059-014-0550-8
Ludwig, A., Poller, W. C., Westphal, K., Minkwitz, S., Lattig-Tunnemann, G., Metzkow, S., et al. (2013). Rapid binding of electrostatically stabilized iron oxide nanoparticles to THP-1 monocytic cells via interaction with glycosaminoglycans. Basic Res. Cardiol. 108 (2), 328. doi:10.1007/s00395-013-0328-2
Maisel, K., Ensign, L., Reddy, M., Cone, R., and Hanes, J. (2015). Effect of surface chemistry on nanoparticle interaction with gastrointestinal mucus and distribution in the gastrointestinal tract following oral and rectal administration in the mouse. J. Control Release 197, 48–57. doi:10.1016/j.jconrel.2014.10.026
Makabenta, J. M. V., Nabawy, A., Li, C. H., Schmidt-Malan, S., Patel, R., and Rotello, V. M. (2021). Nanomaterial-based therapeutics for antibiotic-resistant bacterial infections. Nat. Rev. Microbiol. 19 (1), 23–36. doi:10.1038/s41579-020-0420-1
Malvindi, M. A., De Matteis, V., Galeone, A., Brunetti, V., Anyfantis, G. C., Athanassiou, A., et al. (2014). Toxicity assessment of silica coated iron oxide nanoparticles and biocompatibility improvement by surface engineering. PLoS One 9 (1), e85835. doi:10.1371/journal.pone.0085835
Manz, R. A., Arce, S., Cassese, G., Hauser, A. E., Hiepe, F., and Radbruch, A. (2002). Humoral immunity and long-lived plasma cells. Curr. Opin. Immunol. 14 (4), 517–521. doi:10.1016/s0952-7915(02)00356-4
Maraloiu, V. A., Appaix, F., Broisat, A., Le Guellec, D., Teodorescu, V. S., Ghezzi, C., et al. (2016). Multiscale investigation of USPIO nanoparticles in atherosclerotic plaques and their catabolism and storage in vivo. Nanomedicine 12 (1), 191–200. doi:10.1016/j.nano.2015.08.005
Maschmeyer, P., Zimmermann, J., and Kuhl, A. A. (2021). Murine T-cell transfer colitis as a model for inflammatory bowel disease. Methods Mol. Biol. 2285, 349–373. doi:10.1007/978-1-0716-1311-5_26
Maximova, N., Gregori, M., Zennaro, F., Sonzogni, A., Simeone, R., and Zanon, D. (2016). Hepatic gadolinium deposition and reversibility after contrast agent-enhanced MR imaging of pediatric hematopoietic stem cell transplant recipients. Radiology 281 (2), 418–426. doi:10.1148/radiol.2016152846
Mazlam, M. Z., and Hodgson, H. J. (1992). Peripheral blood monocyte cytokine production and acute phase response in inflammatory bowel disease. Gut 33 (6), 773–778. doi:10.1136/gut.33.6.773
Mejias, R., Gutierrez, L., Salas, G., Perez-Yague, S., Zotes, T. M., Lazaro, F. J., et al. (2013). Long term biotransformation and toxicity of dimercaptosuccinic acid-coated magnetic nanoparticles support their use in biomedical applications. J. Control Release 171 (2), 225–233. doi:10.1016/j.jconrel.2013.07.019
Millward, J. M., Ariza de Schellenberger, A., Berndt, D., Hanke-Vela, L., Schellenberger, E., Waiczies, S., et al. (2019). Application of europium-doped very small iron oxide nanoparticles to visualize neuroinflammation with MRI and fluorescence microscopy. Neuroscience 403, 136–144. doi:10.1016/j.neuroscience.2017.12.014
Millward, J. M., Schnorr, J., Taupitz, M., Wagner, S., Wuerfel, J. T., and Infante-Duarte, C. (2013). Iron oxide magnetic nanoparticles highlight early involvement of the choroid plexus in central nervous system inflammation. ASN Neuro 5 (1), e00110. doi:10.1042/an20120081
Mohammad, Z. H., Ahmad, F., Ibrahim, S. A., and Zaidi, S. (2022). Application of nanotechnology in different aspects of the food industry. Discov. Food 2 (1), 12. doi:10.1007/s44187-022-00013-9
Mosleh-Shirazi, S., Abbasi, M., Moaddeli, M. R., Vaez, A., Shafiee, M., Kasaee, S. R., et al. (2022). Nanotechnology advances in the detection and treatment of cancer: an overview. Nanotheranostics 6 (4), 400–423. doi:10.7150/ntno.74613
Mulens-Arias, V., Rojas, J. M., and Barber, D. F. (2021). The use of iron oxide nanoparticles to reprogram macrophage responses and the immunological tumor microenvironment. Front. Immunol. 12, 693709. doi:10.3389/fimmu.2021.693709
Nemeth, K., Bayraktar, R., Ferracin, M., and Calin, G. A. (2024). Non-coding RNAs in disease: from mechanisms to therapeutics. Nat. Rev. Genet. 25 (3), 211–232. doi:10.1038/s41576-023-00662-1
Nguyen, D. C., Garimalla, S., Xiao, H., Kyu, S., Albizua, I., Galipeau, J., et al. (2018). Factors of the bone marrow microniche that support human plasma cell survival and immunoglobulin secretion. Nat. Commun. 9 (1), 3698. doi:10.1038/s41467-018-05853-7
Nguyen, D. T. C., Nguyen, N. T. T., Nguyen, T. T. T., and Tran, T. V. (2024). Recent advances in the biosynthesis of ZnO nanoparticles using floral waste extract for water treatment, agriculture and biomedical engineering. Nanoscale Adv. 6 (16), 4047–4061. doi:10.1039/d4na00133h
Nile, S. H., Baskar, V., Selvaraj, D., Nile, A., Xiao, J. B., and Kai, G. Y. (2020). Nanotechnologies in food science: applications, recent trends, and future perspectives. Nano-Micro Lett. 12 (1), 45. doi:10.1007/s40820-020-0383-9
Noronha, A. M., Liang, Y., Hetzel, J. T., Hasturk, H., Kantarci, A., Stucchi, A., et al. (2009). Hyperactivated B cells in human inflammatory bowel disease. J. Leukoc. Biol. 86 (4), 1007–1016. doi:10.1189/jlb.0309203
Patro, R., Duggal, G., Love, M. I., Irizarry, R. A., and Kingsford, C. (2017). Salmon provides fast and bias-aware quantification of transcript expression. Nat. Methods 14 (4), 417–419. doi:10.1038/nmeth.4197
Philips, B. W. J., Stijns, R. C. H., Rietsch, S. H. G., Brunheim, S., Barentsz, J. O., Fortuin, A. S., et al. (2019). USPIO-enhanced MRI of pelvic lymph nodes at 7-T: preliminary experience. Eur. Radiol. 29 (12), 6529–6538. doi:10.1007/s00330-019-06277-7
Pohland, M., Glumm, R., Wiekhorst, F., Kiwit, J., and Glumm, J. (2017). Biocompatibility of very small superparamagnetic iron oxide nanoparticles in murine organotypic hippocampal slice cultures and the role of microglia. Int. J. Nanomed. 12, 1577–1591. doi:10.2147/ijn.s127206
Pohland, M., Pohland, C., Kiwit, J., and Glumm, J. (2022). Magnetic labeling of primary murine monocytes using very small superparamagnetic iron oxide nanoparticles. Neural Regen. Res. 17 (10), 2311–2315. doi:10.4103/1673-5374.336873
Poller, W. C., Ramberger, E., Boehm-Sturm, P., Mueller, S., Möller, K., Löwa, N., et al. (2016). Erratum to: uptake of citrate-coated iron oxide nanoparticles into atherosclerotic lesions in mice occurs via accelerated transcytosis through plaque endothelial cells. Nano Res. 9 (12), 3938. doi:10.1007/s12274-016-1337-x
Portilla, Y., Mulens-Arias, V., Paradela, A., Ramos-Fernandez, A., Perez-Yague, S., Morales, M. P., et al. (2022). The surface coating of iron oxide nanoparticles drives their intracellular trafficking and degradation in endolysosomes differently depending on the cell type. Biomaterials 281, 121365. doi:10.1016/j.biomaterials.2022.121365
Prevo, R., Banerji, S., Ferguson, D. J., Clasper, S., and Jackson, D. G. (2001). Mouse LYVE-1 is an endocytic receptor for hyaluronan in lymphatic endothelium. J. Biol. Chem. 276 (22), 19420–19430. doi:10.1074/jbc.m011004200
Rabe, H., Malmquist, M., Barkman, C., Östman, S., Gjertsson, I., Saalman, R., et al. (2019). Distinct patterns of naive, activated and memory T and B cells in blood of patients with ulcerative colitis or Crohn's disease. Clin. Exp. Immunol. 197 (1), 111–129. doi:10.1111/cei.13294
Radeloff, K., Radeloff, A., Ramos, T. M., Scherzad, A., Hagen, R., Kleinsasser, N. H., et al. (2020). Toxicity and functional impairment in human adipose tissue-derived stromal cells (hASCs) following long-term exposure to very small iron oxide particles (VSOPs). Nanomater. (Basel) 10 (4), 741. doi:10.3390/nano10040741
Radeloff, K., Ramos, T. M., Haddad, D., Breuer, K., Muller, J., Hochmuth, S., et al. (2021). Superparamagnetic iron oxide particles (VSOPs) show genotoxic effects but No functional impact on human adipose tissue-derived stromal cells (ASCs). Mater. (Basel) 14 (2), 263. doi:10.3390/ma14020263
Reiss, M., and Roos, D. (1978). Differences in oxygen metabolism of phagocytosing monocytes and neutrophils. J. Clin. Invest 61 (2), 480–488. doi:10.1172/jci108959
Remmo, A., Lowa, N., Kosch, O., Eberbeck, D., Ludwig, A., Kampen, L., et al. (2022). Cell tracking by magnetic particle imaging: methodology for labeling THP-1 monocytes with magnetic nanoparticles for cellular imaging. Cells 11 (18), 2892. doi:10.3390/cells11182892
Riehl, T. E., Santhanam, S., Foster, L., Ciorba, M., and Stenson, W. F. (2015). CD44 and TLR4 mediate hyaluronic acid regulation of Lgr5+ stem cell proliferation, crypt fission, and intestinal growth in postnatal and adult mice. Am. J. Physiol. Gastrointest. Liver Physiol. 309 (11), G874–G887. doi:10.1152/ajpgi.00123.2015
Roberts, D. R., Lindhorst, S. M., Welsh, C. T., Maravilla, K. R., Herring, M. N., Braun, K. A., et al. (2016). High levels of gadolinium deposition in the skin of a patient with normal renal function. Invest Radiol. 51 (5), 280–289. doi:10.1097/rli.0000000000000266
Rojas, J. M., Sanz-Ortega, L., Mulens-Arias, V., Gutierrez, L., Perez-Yague, S., and Barber, D. F. (2016). Superparamagnetic iron oxide nanoparticle uptake alters M2 macrophage phenotype, iron metabolism, migration and invasion. Nanomedicine. 12 (4), 1127–1138. doi:10.1016/j.nano.2015.11.020
Sani, A., Cao, C., and Cui, D. (2021). Toxicity of gold nanoparticles (AuNPs): a review. Biochem. Biophys. Rep. 26, 100991. doi:10.1016/j.bbrep.2021.100991
Schannor, M., Oelze, M., Traub, H., He, Y., Schmidt, R., Heidemann, L., et al. (2025). Advancing biomarker research: in situ Cu isotope analysis in liver tumors by LA-MC-ICP-MS. Anal. Chem. 97 (8), 4425–4432. doi:10.1021/acs.analchem.4c05626
Scheibner, K. A., Lutz, M. A., Boodoo, S., Fenton, M. J., Powell, J. D., and Horton, M. R. (2006). Hyaluronan fragments act as an endogenous danger signal by engaging TLR2. J. Immunol. 177 (2), 1272–1281. doi:10.4049/jimmunol.177.2.1272
Scholz, S., Lewis, K., Saulich, F., Endres, M., Boehmerle, W., and Huehnchen, P. (2022). Induced pluripotent stem cell-derived brain organoids as potential human model system for chemotherapy induced CNS toxicity. Front. Mol. Biosci. 9, 1006497. doi:10.3389/fmolb.2022.1006497
Shamsi, H., Yari, R., and Salehzadeh, A. (2024). Biosynthesized BiFe(2)O(4)@Ag nanoparticles mediated Scenedesmus obliquus induce apoptosis in AGS gastric cancer cell line. Sci. Rep. 14 (1), 10284. doi:10.1038/s41598-024-57157-0
Sharifi, S., Hajipour, M. J., Gould, L., and Mahmoudi, M. (2021). Nanomedicine in healing chronic wounds: opportunities and challenges. Mol. Pharm. 18 (2), 550–575. doi:10.1021/acs.molpharmaceut.0c00346
Sharma, A., Agarwal, P., Sebghatollahi, Z., and Mahato, N. (2023). Functional nanostructured materials in the cosmetics industry: a review. Chemengineering 7 (4), 66. doi:10.3390/chemengineering7040066
Shestovskaya, M. V., Luss, A. L., Bezborodova, O. A., Makarov, V. V., and Keskinov, A. A. (2023). Iron oxide nanoparticles in cancer treatment: cell responses and the potency to improve radiosensitivity. Pharmaceutics 15 (10), 2406. doi:10.3390/pharmaceutics15102406
Shubhra, Q. T. H. (2023). Iron oxide nanoparticles in magnetic drug targeting and ferroptosis-based cancer therapy. Med. Rev. (2021) 3 (5), 444–447. doi:10.1515/mr-2023-0029
Snyder, E. Y., Deitcher, D. L., Walsh, C., Arnold-Aldea, S., Hartwieg, E. A., and Cepko, C. L. (1992). Multipotent neural cell lines can engraft and participate in development of mouse cerebellum. Cell 68 (1), 33–51. doi:10.1016/0092-8674(92)90204-p
Soenen, S. J., Himmelreich, U., Nuytten, N., and De Cuyper, M. (2011). Cytotoxic effects of iron oxide nanoparticles and implications for safety in cell labelling. Biomaterials 32 (1), 195–205. doi:10.1016/j.biomaterials.2010.08.075
Sonavane, G., Tomoda, K., and Makino, K. (2008). Biodistribution of colloidal gold nanoparticles after intravenous administration: effect of particle size. Colloids Surf. B Biointerfaces 66 (2), 274–280. doi:10.1016/j.colsurfb.2008.07.004
Stoppini, L., Buchs, P. A., and Muller, D. (1991). A simple method for organotypic cultures of nervous tissue. J. Neurosci. Methods 37 (2), 173–182. doi:10.1016/0165-0270(91)90128-m
Sur, I., Cam, D., Kahraman, M., Baysal, A., and Culha, M. (2010). Interaction of multi-functional silver nanoparticles with living cells. Nanotechnology 21 (17), 175104. doi:10.1088/0957-4484/21/17/175104
Surapaneni, S. K., Bashir, S., and Tikoo, K. (2018). Gold nanoparticles-induced cytotoxicity in triple negative breast cancer involves different epigenetic alterations depending upon the surface charge. Sci. Rep. 8 (1), 12295. doi:10.1038/s41598-018-30541-3
Sze, D. M., Toellner, K. M., Garcia de Vinuesa, C., Taylor, D. R., and MacLennan, I. C. (2000). Intrinsic constraint on plasmablast growth and extrinsic limits of plasma cell survival. J. Exp. Med. 192 (6), 813–821. doi:10.1084/jem.192.6.813
Tefft, B. J., Uthamaraj, S., Harburn, J. J., Klabusay, M., Dragomir-Daescu, D., and Sandhu, G. S. (2015). Cell labeling and targeting with superparamagnetic iron oxide nanoparticles. J. Vis. Exp. (105), e53099. doi:10.3791/53099
Tolg, C., Hamilton, S. R., Zalinska, E., McCulloch, L., Amin, R., Akentieva, N., et al. (2012). A RHAMM mimetic peptide blocks hyaluronan signaling and reduces inflammation and fibrogenesis in excisional skin wounds. Am. J. Pathol. 181 (4), 1250–1270. doi:10.1016/j.ajpath.2012.06.036
van der Heijde, D., Gensler, L. S., Maksymowych, W. P., Landewe, R., Rudwaleit, M., Bauer, L., et al. (2022). Long-term safety and clinical outcomes of certolizumab pegol treatment in patients with active non-radiographic axial spondyloarthritis: 3-year results from the phase 3 C-axSpAnd study. RMD Open 8 (1), e002138. doi:10.1136/rmdopen-2021-002138
Villalobos Gutierrez, P. T., Munoz Carrillo, J. L., Sandoval Salazar, C., Viveros Paredes, J. M., and Gutierrez Coronado, O. (2023). Functionalized metal nanoparticles in cancer therapy. Pharmaceutics 15 (7), 1932. doi:10.3390/pharmaceutics15071932
Wagner, S., Schnorr, J., Ludwig, A., Stangl, V., Ebert, M., Hamm, B., et al. (2013). Contrast-enhanced MR imaging of atherosclerosis using citrate-coated superparamagnetic iron oxide nanoparticles: calcifying microvesicles as imaging target for plaque characterization. Int. J. Nanomed. 8, 767–779. doi:10.2147/ijn.s38702
Wang, Q., Liang, Q., Dou, J., Zhou, H., Zeng, C., Pan, H., et al. (2024). Breaking through the basement membrane barrier to improve nanotherapeutic delivery to tumours. Nat. Nanotechnol. 19 (1), 95–105. doi:10.1038/s41565-023-01498-w
Wu, H. Y., Chung, M. C., Wang, C. C., Huang, C. H., Liang, H. J., and Jan, T. R. (2013). Iron oxide nanoparticles suppress the production of IL-1beta via the secretory lysosomal pathway in murine microglial cells. Part Fibre Toxicol. 10, 46. doi:10.1186/1743-8977-10-46
Wu, K., Chugh, V. K., V, D. K., di Girolamo, A., Wang, Y. A., Saha, R., et al. (2021). One-step, wash-free, nanoparticle clustering-based magnetic particle spectroscopy bioassay method for detection of SARS-CoV-2 spike and nucleocapsid proteins in the liquid phase. ACS Appl. Mater Interfaces 13 (37), 44136–44146. doi:10.1021/acsami.1c14657
Wu, Q., Jin, R., Feng, T., Liu, L., Yang, L., Tao, Y., et al. (2017). Iron oxide nanoparticles and induced autophagy in human monocytes. Int. J. Nanomed. 12, 3993–4005. doi:10.2147/ijn.s135189
Wuerfel, E., Smyth, M., Millward, J. M., Schellenberger, E., Glumm, J., Prozorovski, T., et al. (2011). Electrostatically stabilized magnetic nanoparticles - an optimized protocol to label murine T cells for in vivo MRI. Front. Neurol. 2, 72. doi:10.3389/fneur.2011.00072
Yadav, A., Yadav, K., and Abd-Elsalam, K. A. (2023). Exploring the potential of nanofertilizers for a sustainable agriculture. Plant Nano Biol. 5, 100044. doi:10.1016/j.plana.2023.100044
Yazdimamaghani, M., Moos, P. J., and Ghandehari, H. (2019). Time- and dose-dependent gene expression analysis of macrophage response as a function of porosity of silica nanoparticles. Nanomedicine 21, 102041. doi:10.1016/j.nano.2019.102041
Yi, L., Hu, Y., Wu, Z., Li, Y., Kong, M., Kang, Z., et al. (2022). TFRC upregulation promotes ferroptosis in CVB3 infection via nucleus recruitment of Sp1. Cell Death Dis. 13 (7), 592. doi:10.1038/s41419-022-05027-w
Zhao, Y., Wang, Y., Ran, F., Cui, Y., Liu, C., Zhao, Q., et al. (2017). A comparison between sphere and rod nanoparticles regarding their in vivo biological behavior and pharmacokinetics. Sci. Rep. 7 (1), 4131. doi:10.1038/s41598-017-03834-2
Keywords: superparamagnetic iron oxide nanoparticles, molecular imaging, diagnostics, inflammatory bowel disease, monocytes
Citation: Hussein S, Kühl AA, Golusda L, Plattner C, Heinze N, Sturm G, Freise C, Traub H, Schannor M, Trajanoski Z, Taupitz M, Siegmund B and Paclik D (2025) Phenotype and function of human monocytes remain mainly unaffected by very small superparamagnetic iron oxide particles. Front. Nanotechnol. 7:1584000. doi: 10.3389/fnano.2025.1584000
Received: 26 February 2025; Accepted: 30 April 2025;
Published: 23 May 2025.
Edited by:
Hayrunnisa Nadaroglu, Atatürk University, TürkiyeReviewed by:
Christina Janko, Universitätsklinikum Erlangen, GermanyRebeca Gonzalez-Pastor, Universidad Tecnológica Equinoccial, Ecuador
Sandipan Mukherjee, University of Washington, United States
Ashish Tiwari, University of Delaware, United States
Alper Baran, Atatürk University, Türkiye
Copyright © 2025 Hussein, Kühl, Golusda, Plattner, Heinze, Sturm, Freise, Traub, Schannor, Trajanoski, Taupitz, Siegmund and Paclik. This is an open-access article distributed under the terms of the Creative Commons Attribution License (CC BY). The use, distribution or reproduction in other forums is permitted, provided the original author(s) and the copyright owner(s) are credited and that the original publication in this journal is cited, in accordance with accepted academic practice. No use, distribution or reproduction is permitted which does not comply with these terms.
*Correspondence: Daniela Paclik, RGFuaWVsYS5wYWNsaWtAY2hhcml0ZS5kZQ==
†These authors have contributed equally to this work and share first authorship
‡These authors have contributed equally to this work and share last authorship