- 1Budker Institute of Nuclear Physics, Novosibirsk, Russia
- 2Novosibirsk State University, Novosibirsk, Russia
- 3Tartous University, Tartous, Syria
- 4Novosibirsk State Technical University, Novosibirsk, Russia
The method of boron neutron capture therapy for malignant tumors, proposed in 1936, is beginning to enter clinical practice. The development of dosimetry tools for characterization of therapeutic mixed neutron-photon beam and assessing the patient’s response to treatment is becoming relevant. In this work, a number of dosimetric techniques have been developed: a compact neutron detector with a pair of cast scintillators, one of which is enriched with boron, to measure the boron dose and the γ-ray dose; cell dosimeter for measuring the sum of the equivalent dose of fast neutrons and the equivalent nitrogen dose; prompt γ-ray spectroscopy for in situ measurement of boron dose in real time; epithermal neutron flux monitor for measuring the epithermal neutron flux. Their verification carried out on the accelerator based neutron source VITA showed that they can become convenient and reliable tools for characterization of neutron beam and assessing the patient’s response to treatment.
1 Introduction
Boron neutron capture therapy (BNCT) (Sauerwein et al., 2012; Dymova et al., 2020; Ahmed et al., 2023)–selective destruction of tumor cells by accumulating boron-10 stable isotopes in them followed by neutron irradiation–is considered to be one of the promising methods to treat malignant tumors. As a result of neutron absorption by boron, a nuclear reaction 10B(n,α)7Li occurs with high energy release in the cell, which leads to its destruction. Recently, significant progress has been made in the development of accelerator based neutron sources, a large number of them have been put into operation, and clinical trials of the BNCT technique and treatment of patients are being carried out on some of them (bnct-bor on-neutron, 2021). The main parameters for neutron source are as follows: therapeutic epithermal flux ≥5 × 108 cm–2 s–1, fast neutron dose per unit epithermal fluence ≤7 × 10−13 Gy cm2, γ-ray dose per unit epithermal fluence ≤2 × 10−13 Gy cm2 (Ahmed et al., 2023). The development of dosimetry tools for characterization of therapeutic mixed neutron-photon beam and assessing the patient’s response to treatment is becoming relevant. The purpose of this work was the development of dosimetry tools for BNCT and their verification on the accelerator base neutron source VITA (Taskaev et al., 2021a).
2 Materials and methods
In BNCT, the total absorbed dose is the sum of four dose components: boron dose; nitrogen dose; fast neutron dose; γ-ray dose. Before describing the proposed measurement approaches, we detail the processes leading to these four doses.
• Boron dose DB: 10B(n,α)7Li reaction produces two high-LET particles: 4He and 7Li.
• Nitrogen dose DN: 14N(n,p)14C reaction produces high-LET proton.
• Fast neutron dose Df: elastic scattering of neutrons by atomic nuclei of matter, mainly hydrogen, produces high-LET recoil nuclei, mainly protons.
• γ-ray dose Dγ: there are many sources of γ-rays here, including emission of 2.2 MeV photons in 1H(n,γ)2H reaction in patient’s body, 478 keV photons in 10B(n,α)7Li reaction, 478 keV photons in 7Li(p,p’γ)7Li reaction (Taskaev et al., 2021b) in the case of using a lithium target for neutron generation, and emission of photons due to interaction of neutrons with structural materials of a neutron beam shaping assembly.
The total absorbed dose DT is the sum of these four dose components: DT = DB + DN + Df + Dγ.
“The first two dose components cannot be measured in principle”, as previously was written in [Sauerwein et al., 2012, p. 279]. The methods for measuring the fast neutron dose for BNCT are absent also, as the energy of neutrons is obviously lower than 1 MeV and, for example, fission ionization chambers are not applicable. There are quite a few proven approaches for measuring of γ-ray dose alone.
When evaluating the patient’s response to the therapy, it is customary to analyze the equivalent (biological) dose, since the destructive effect of different ionizing particles can be different. The damage of tissue in BNCT is due to three types of directly ionizing radiations that differ in their linear energy transfer (LET) characteristics: low-LET γ-rays, high-LET protons and high-LET heavier charged particles 4He, 7Li, and 14C. The equivalent dose Dw is the sum of four dose components each of which is multiplied by a weighted factor: Dw = wB DB + wN DN + wf Df + wγ Dγ. Some of these weighted factors are not well defined, and the weighted factor wB for DB depends on the 10B carrier used and the tissue.
All this demonstrates how dosimetry in BNCT is more complicated than in photon and electron therapy, which both act by energy transfer via electrons.
We have developed and verified the following dosimetry techniques: 1) a compact neutron detector with a pair of cast scintillators, one of which is enriched with boron, to measure the boron dose and the γ-ray dose; 2) cell dosimeter for measuring the sum of the equivalent dose of fast neutrons and the equivalent nitrogen dose. We also implement the well-known prompt γ-ray spectroscopy for in situ measurement of boron dose in real time and epithermal neutron flux monitor for measuring the epithermal neutron flux.
2.1 Boron neutron detector
The sensitive element of the detector is a cylinder with diameter of 1 mm and length of 1 mm made of cast polystyrene scintillator (Bykov et al., 2021). It is placed inside of a light-reflecting cylinder made of hard Teflon with wall thickness of 1 mm. The top side of the scintillator is painted with custom-made MgO-based white paint. The scintillator is glued to a plastic optical fiber with epoxy, and all components are placed inside of a black plastic case. Two sensors, one with a boron enriched scintillator and a boron-free one, are combined in one detector head. Simultaneous application of two different registration channels: the first one, sensitive to γ-radiation, and the second one, sensitive to γ-radiation and neutrons, allows us to estimate the contribution of the neutron component accurately.
2.2 Cell dosimeter
The idea to measure fast neutron dose and nitrogen dose is the following (Taskaeva and Taskaev, 2021). The cell lines are exposed to γ-radiation and mixed radiation (neutrons and γ-radiation) measuring the γ-ray dose Dγ. The γ-ray doses that cause the same effect, for example, cell survival, are compared. When cells are irradiated with γ-radiation, only a γ-ray dose is received, when they are irradiated with mixed radiation, a fast neutron dose and a nitrogen dose are added (there is no boron dose, since the boron drug is not used). The equivalent dose of high-LET particles (sum of the fast neutron dose and the nitrogen dose) is calculated by the formula: Dn = Dγ standard–Dγ mixed, where Dn–the equivalent dose of high-LET particles; Dγ standard–the dose of γ-radiation when the cells are exposed to γ-radiation; Dγ mixed–the dose of γ-radiation when the cells are exposed to mixed radiation.
It is well known (Wang et al., 2010; Faião-Flores et al., 2013; Yura and Fujita, 2013), that cell survival depends on the cell line culture, the absorbed dose, the type of ionizing radiation and its energy spectrum, time of the irradiation. The proposed method of dose measurement is free from the need to take into account all these factors. In the method, cell cultures placed in the same place are irradiated with two different types of ionizing radiation (γ-radiation and mixed radiation) for the same time and achieve the same survival rate. If irradiation of cell cultures of the same line with two different types of radiation for the same time leads to the same cell survival, then the equivalent doses are equal.
2.2.1 Cell lines
The human glioblastoma multiforme cell line U251 obtained from the Russian cell culture collection (Russian Branch of the, ETCS, St. Petersburg, Russia) was used for research. Cells were cultured in Minimum Essential Media (MEM) (Gibco, United States) supplemented with 10% (v/v) of fetal bovine serum (Gibco, United States) and 1% (v/v) antibiotic-antimycotic solution (Gibco, United States). Cells were maintained at 37°C in a 5% CO2 atmosphere.
2.2.2 Clonogenic assay
After irradiation, cells were seeded in 6-well plates (TPP, Switzerland) at a density of 300 cells per well and incubated for a week in a humidified incubator under 5% (v/v) CO2 at 37°C. Colonies were fixed with glutaraldehyde (6.0% v/v), stained with crystal violet (0.5% w/v) (Franken et al., 2006). Colonies of more than 50 cells were counted. The percent plating efficiency and surviving fraction were calculated based on formula described earlier (Franken et al., 2006).
2.3 Prompt γ-ray spectroscopy
The principle of prompt γ-ray spectroscopy is based on γ-ray spectroscopy following neutron capture in 10B. In 93.9% of cases, a10B(n,α)7Li reaction proceeds with the emission of a recoiling 7Li nuclei in an excited state. Excited nuclei go to the ground state by the emission of 478 keV photons. The emission rate of the photons is proportional to the neutron capture reaction rate. This method is a direct method for measuring boron dose (Kobayashi and Kanda, 1983).
The complexity of the implementation is that the γ-ray spectrometer must be in the neutron flux, have a good energy resolution, while photons with the same energy are emitted from the lithium target as a result of inelastic scattering of protons on lithium atomic nuclei. If we use a γ-spectrometer, that is, relatively stable in the neutron flux, then the energy resolution does not allow us to separate the 478 keV line from the more powerful 511 keV line. The HPGe γ-ray spectrometer separates these lines, but it is not resistant to neutrons.
2.4 Epithermal neutron flux monitor
The principle of the epithermal neutron flux monitor is based on the activation method using 71Ga(n,γ)72Ga reaction (Guan et al., 2015). In the monitor, the activation material is positioned in the geometrical center of the polymethyl methacrylate (PMMA) cylinder used for neutron moderation and covered with Cd foil for thermal neutron absorption. Gallium foil has a diameter of about 10 mm and a mass of about 50 mg; PMMA cylinder is 63 mm in height and 65 mm in diameter; Cd foil thickness is 0.1 mm. The detector is made collapsible in order to remove activated gallium foil and measure its activation by the HPGe γ-ray spectrometer. Of course, it is possible not to disassemble the detector. However, in this case, an intense cadmium emission line gives an additional load to the detector, which leads to an increase in the dead time of the spectrometer and to a systematic error in the intensity of γ-radiation measurement.
This monitor design allows you to achieve a flat sensitivity curve in the epithermal neutron energy range, while its sensitivities to fast and thermal neutrons are low.
2.5 Neutron source
Neutron irradiation was performed at the accelerator based neutron source VITA in the Budker Institute of Nuclear Physics, Novosibirsk, Russia (Taskaev et al., 2021a). The layout of the facility is shown in Figure 1.
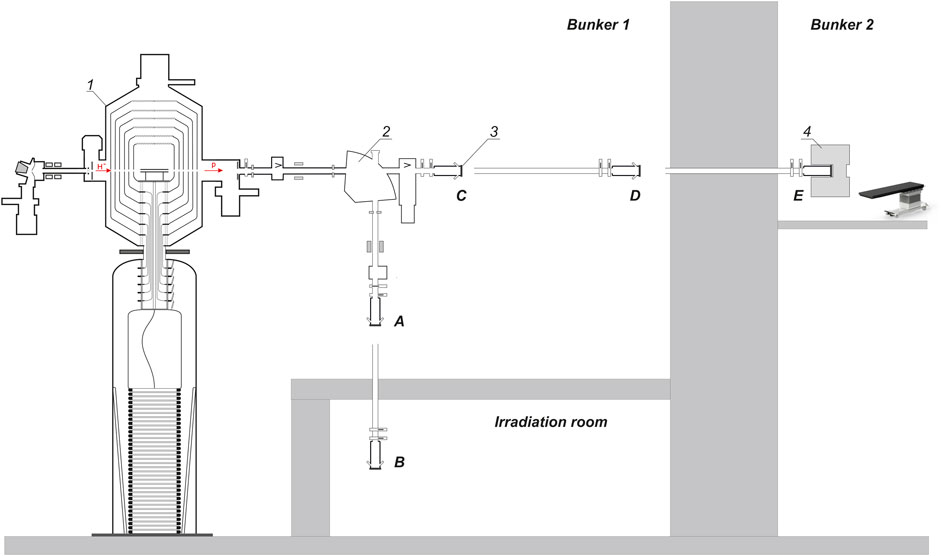
FIGURE 1. Layout of the experimental facility: 1–vacuum insulated tandem accelerator, 2—bending magnet, 3—lithium target, 4—beam shaping assembly. A, B, C, D, E—lithium target placement positions.
The neutron source comprises a tandem electrostatic particle accelerator of original design (named Vacuum Insulated Tandem Accelerator–VITA) to produce a beam of protons or deuterons, thin solid lithium target to generate neutrons, a neutron beam shaping assembly to form a neutron beam. It is placed in two bunkers. The facility has the ability to place a lithium target in 5 positions; in Figure 1 they are marked as positions A, B, C, D, E. In these experiments, the lithium target was placed in positions A, C, or D.
In the accelerator, the energy of protons can be changed within a range of 0.6 MeV–2.3 MeV keeping a high-energy stability 0.1%. The beam current can also be changed in a range of 0.3–10 mA keeping a high current stability 0.4%.
Lithium target 10 cm in diameter has three layers: a thin layer of pure lithium to generate neutrons in 7Li(p,n)7Be reactions; a thin layer of material totally resistant to radiation blistering; and a thin copper substrate for efficient heat removal. This target provides a stable neutron yield for a long time with an acceptably low level of contamination of the beam transport path by the inevitably formed radioactive isotope beryllium-7.
A Beam Shaping Assembly (BSA) is applied to convert neutron flux into a beam of epithermal neutrons with characteristics suitable for clinical applications (Zaidi et al., 2018). The BSA consists of a magnesium fluoride moderator 210 mm thick, a composite reflector (graphite in the front hemisphere and lead in the back), an absorber, and a filter.
A polymethyl methacrylate (PMMA) moderator 72 mm thick is used to produce a thermal neutron beam suitable for irradiation of cell cultures and laboratory animals for development the BNCT technique. Compared to MgF2 BSA this moderator provides a higher neutron flux, but slightly worse therapeutic ratio and depth of therapy.
3 Results
All proposed dosimetry tools were tested on the accelerator based neutron source VITA.
3.1 Boron neutron detector
The spatial distribution of the boron dose and the γ-ray dose in air and in a water phantom was measured by the small-sized boron neutron detector with a pair of cast polystyrene scintillators, one of which is enriched with boron.
For the treatment of patients, a BSA with a magnesium fluoride moderator and a composite reflector (graphite in the front hemisphere, lead in the back) was developed and manufactured. The results of simulating and measuring the dependence of the dose component rate considered in BNCT on the depth in the phantom using this BSA are shown in Figure 2. The energy of the proton beam is 2.2 MeV, the current is 1 mA. The phantom is a cube with a side of 15 cm, placed with 5 cm clearance from the moderator. In simulations, the phantom is filled with tissue-equivalent contents; in measurements, the phantom is filled with water. Measurements of boron dose and γ-ray dose were carried out by the developed boron neutron detector. The boron concentration is assumed to be 40 ppm. Figure 2 also shows the depth distribution of the so-called therapeutic coefficient TC–the ratio of the “useful” dose (boron dose) to the “harmful” dose (the sum of the γ-ray dose, the fast neutron dose and the nitrogen dose).
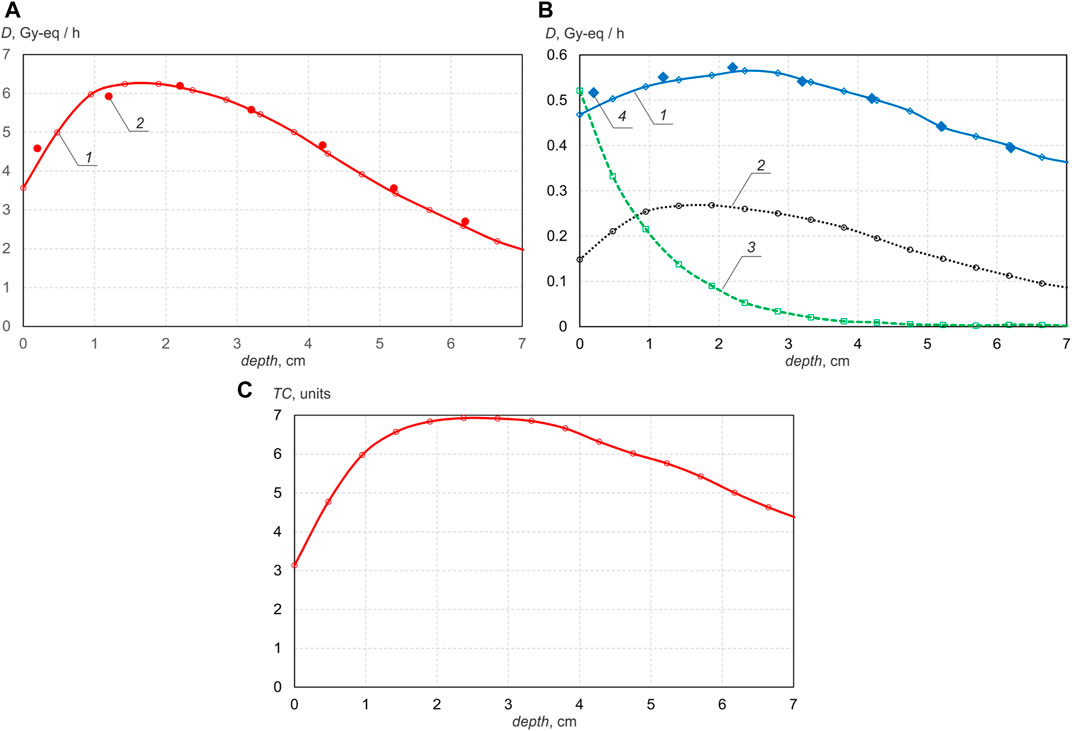
FIGURE 2. Deep propagation dependence using BSA with MgF2 moderator: (A)—boron dose rate (1—calculated, 2—measured); (B)—γ-ray dose rate (1—calculated, 4—measured), simulated nitrogen dose rate 2, simulated fast neutron dose rate 3, (C) therapeutic coefficient.
It can be seen that the formed beam of epithermal neutrons penetrates deep enough, providing the maximum boron dose at a depth of 2 cm and 1/e times less at a depth of 6.6 cm. If the phantom is placed close to the moderator, then the boron dose increases by 1.5 times, but the therapeutic coefficient is reduced by 8%. Since simulations show that an increase in the proton energy from 2.2 to 2.3 MeV leads to an increase in both the boron dose and the therapeutic coefficient, it is planned to test this experimentally after replacing the accelerator electrodes damaged by operation at the extremely high parameters with new ones.
The facility also uses a PMMA moderator, which ensures an acceptable neutron beam quality when irradiating cell cultures and laboratory animals, when testing targeted boron delivery drugs, and when treating domestic animals with spontaneous tumors.
The results of simulating and measuring the dependence of the dose component rate on depth in a phantom placed close to a 72 mm thick PMMA moderator are shown in Figure 3. In this case, the energy of the proton beam is 2.1 MeV, the current is 1 mA. Since the neutron spectrum is shifted to the region of thermal energies due to the hydrogen-containing moderator, the maximum boron dose is realized on the surface and the neutrons penetrate into the phantom by a smaller one–e times the boron dose rate drops at a depth of 3.4 cm. It is also seen that the therapeutic coefficient of such the neutron beam is 1.5 times smaller. However, such a beam provides 2.7 times the dose rate at a proton energy of 2.1 MeV and 4.4 times at an energy of 2.2 MeV compared to a magnesium fluoride moderator, if the phantom is also placed close to the moderator.
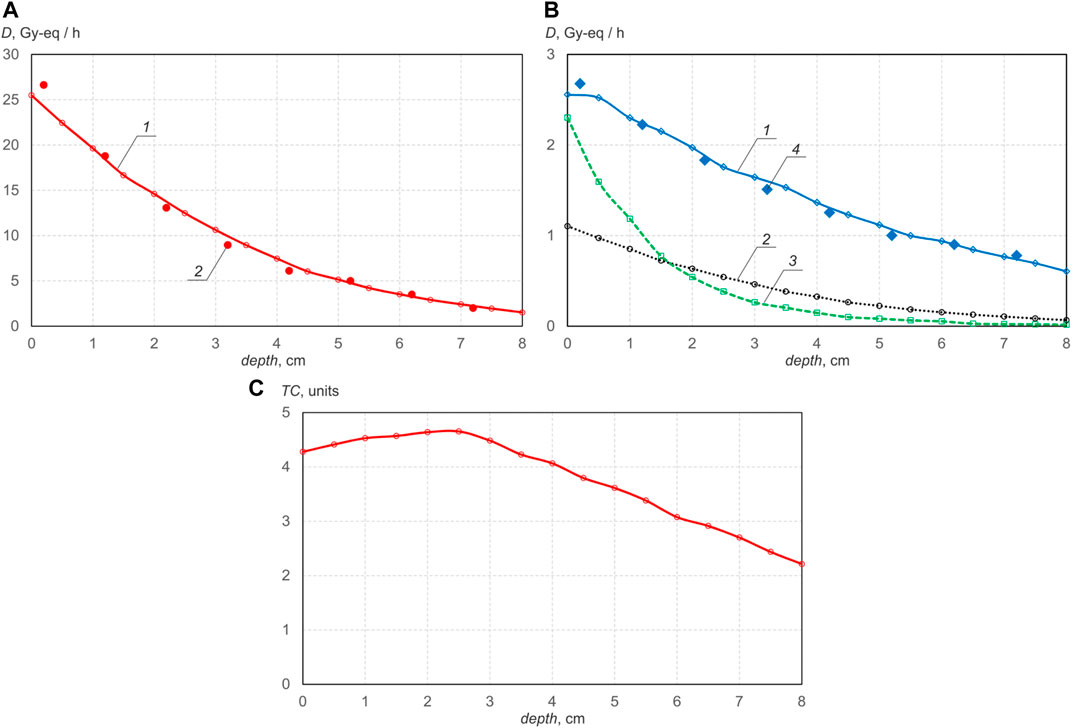
FIGURE 3. Deep propagation dependenceusing BSA with PMMA moderator: (A)—boron dose rate (1—calculated, 2—measured); (B)—γ-ray dose rate (1—calculated, 4—measured), simulated nitrogen dose rate 2, simulated fast neutron dose rate 3, (C) therapeutic coefficient.
To improve the quality of the neutron beam using a PMMA moderator, it is proposed to make the moderator thicker in the forward direction–this will reduce the fast neutron dose, and to sprinkle bismuth inside the PMMA–this will reduce the contribution of the γ-ray dose. Such a change, as shown by the simulation results, will improve the therapeutic coefficient by a third–up to 6, with a slight decrease in the boron dose rate.
3.2 Cell dosimeter
The glioblastoma cell line U251 and the clonogenic test were used to implement the dose measurement method. At first, U251 cells were irradiated only with γ-radiation and their survival was determined. A γ-radiation was produced in the 7Li(p,p’γ)7Li reaction at a proton beam energy below 1.882 MeV–threshold of the 7Li(p,n)7Be reaction. The photon energy in this reaction is 478 keV. U251 cell line was irradiated for 1.5 h with photons at proton energy 1.800 ± 0.002 MeV and proton current 2.17 ± 0.03 mA. The dose absorbed by the cells was 5.21 Gy. After γ-radiation the surviving fractions of U251 cell line were examined using a clonogenic assay. Cell survival as a result of photon irradiation was 34% ± 4%.
To generate mixed radiation, neutron and γ-radiation, the proton energy was increased to 2.05 MeV–above the threshold of the 7Li(p,n)7Be reaction, the proton beam current was reduced. The experiment was carried out according to the following scenario. A certain value of the proton beam current was set, the cells were irradiated for 1.5 h, and then their survival was determined. If the cell survival is more than 34%, then in the next irradiation the beam current will be increased, if it is less it will be reduced. After several attempts the cell survival fraction was achieved exactly 34%. The dose measured with a γ-dosimeter was 3.98 Gy.
Since the survival rate of cells after irradiation with mixed radiation is the same as that of γ-radiation and the irradiation times were equal, the cells received the same equivalent dose—5.21 Gy-Eq. When irradiated with mixed radiation, γ-radiation gave 3.98 Gy-Eq at this dose, which means that the remaining 1.23 Gy-Eq were due to recoil nuclei caused by the absorption of thermal neutrons and elastic scattering of fast neutrons.
3.3 Prompt γ-ray spectroscopy
In this experiment, the lithium target was placed in position D, and the HPGe γ-spectrometer in bunker 2 (see Figure 1). A 50 mL test tube with boron of various concentrations is placed in a neutron beam, and the γ-ray spectrum is measured with the HPGe γ-spectrometer within 15 min. The result obtained is shown in Figure 4. It can be seen that the 478 keV photon line broadened by the Doppler effect is reliably measured and the boron content is determined starting from a 20 ppm content. Measuring this concentration of boron is sufficient to estimate the boron dose during therapy. Note that the 511 keV photon line due to neutron absorption by hydrogen is separated from the 478 keV photon line and can be used to monitor the neutron flux.
3.4 Epithermal neutron flux monitor
The epithermal neutron flux monitor is placed along the proton beam axis at a distance of 100 mm from the lithium target placed in position A (see Figure 1). Discs made of PMMA with a diameter of 200 mm and a thickness of 12 mm are placed closely below the target in an amount of 0–6 pcs. The target is irradiated to a fluence of 2.5 C for 1 h at a proton energy of 2 MeV. After irradiation, the monitor is disassembled, the gallium foil is removed and the HPGe γ-spectrometer measures the activation of the gallium foil. The measured activation of the gallium foil, taking into account its mass, makes it possible to reconstruct the epithermal neutron flux density.
The results are shown in Figure 5. It can be seen that the experimental results are very different from the simulation results in the absence of disks and with a single disk 12 mm thick, but are consistent with for a PMMA thickness of more than 24 mm. The simulated neutron energy spectrum for several PMMA thicknesses is shown in Figure 6. It can be seen that without the use of a moderator, fast neutrons predominate in the energy spectrum of neutrons. Although the sensitivity of the monitor to fast neutrons is low, they make a significant contribution to the activation of gallium due to their large number. As the moderator thickness increases the energy spectrum shifts towards the epithermal energy range and the measurement results become closer to the simulation results.
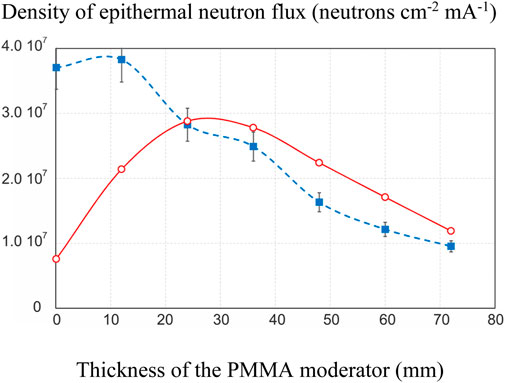
FIGURE 5. Dependence of the epithermal neutron flux density on the thickness of the PMMA moderator: blue dashed line–measured, red solid line–simulated.
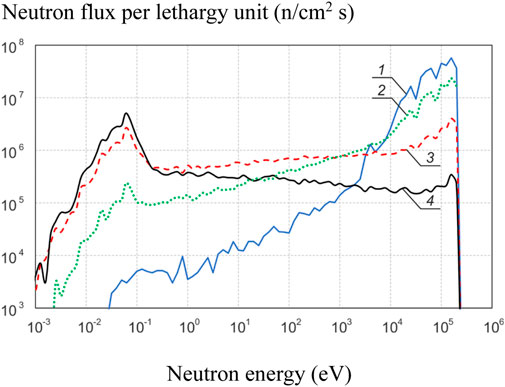
FIGURE 6. Simulated neutron energy spectrum for several PMMA thicknesses: 1—0 mm, 2–12 mm, 3–36 mm, 4–72 mm.
4 Discussion
The compact scintillator detector turned out to be a very convenient and simple tool for measuring boron dose and γ-ray dose in air, in a phantom, and even in the treatment of pets with spontaneous tumors. However, the boron dose requires absolute calibration.
The cell dosimeter provides reliable information on the sum of fast neutron dose and nitrogen dose, but requires large resources. Nevertheless, we consider it appropriate to carry out these measurements on accelerator based neutron sources with lithium targets, when cell cultures can be placed in the same place and irradiated from the same direction.
We plan to continue research on the implementation of prompt γ-ray spectroscopy to determine the boron dose, since this is the most direct and reliable method. We consider it is a good idea to place the HPGe γ-ray spectrometer in an adjacent bunker, but we need to optimize the collimator for γ-rays and the scatterer and absorber for neutrons.
The epithermal neutron flux monitor needs additional verification. Monitor efficiency calculated by us (Byambatseren et al., 2023) and presented in the article (Guan et al., 2019) differ significantly. To use the detector in practice, it is necessary to eliminate the discrepancy in the monitor efficiency, which can be achieved both by joint research and by independent calculations.
5 Conclusion
Recently, significant progress has been made in the development of accelerator based neutron sources. The method of boron neutron capture therapy for malignant tumors is beginning to enter clinical practice now. The development of dosimetry tools for characterization of therapeutic mixed neutron-photon beam and assessing the patient’s response to treatment is becoming relevant.
We have developed and verified a number of diagnostic methods that allow us to evaluate all four components of doses considered in BNCT: compact neutron detector, cell dosimeter, prompt γ-ray spectroscopy and epithermal neutron flux monitor. The developed compact neutron detector measures the spatial distribution of boron dose and γ-ray dose in air and in a water phantom with a resolution of 1 mm. The proposed cell dosimeter provides measurement of the sum of fast neutron doses and nitrogen dose using cell cultures irradiated with γ-radiation and mixed radiation. The paper shows that providing effective protection of the HPGe γ-spectrometer, it is possible to implement the prompt γ-ray spectroscopy for real-time measurement of the boron dose during therapy. The manufactured epithermal neutron flux monitor provides measurement of the epithermal neutron flux, but requires verification due to the difference in cross sections in different databases.
It is desirable to use all the developed methods in therapeutic facilities for treatment planning and evaluation of its results.
Data availability statement
The original contributions presented in the study are included in the article/Supplementary Material, further inquiries can be directed to the corresponding author.
Author contributions
MB: Investigation, Writing–original draft. TB: Software, Writing–original draft. II: Validation, Writing–original draft. AK: Investigation, Writing–original draft. DK: Investigation, Methodology, Writing–original draft. IK: Formal Analysis, Investigation, Writing–original draft. VK: Investigation, Writing–original draft. TK: Investigation, Writing–original draft. AKo: Data curation, Software, Writing–original draft. AKu: Software, Visualization, Writing–original draft. VP: Software, Methodology, Writing–original draft. SS: Investigation, Resources, Writing–original draft. IS: Data curation, Investigation, Writing–original draft. NS: Investigation, Writing–original draft. ES: Investigation, Methodology, Writing–original draft. TS: Formal Analysis, Software, Writing–original draft. IT: Investigation, Methodology, Writing–original draft. GV: Validation, Writing–original draft. ST: Conceptualization, Funding acquisition, Project administration, Resources, Supervision, Writing–review and editing.
Funding
The author(s) declare financial support was received for the research, authorship, and/or publication of this article. This research was funded by Russian Science Foundation, grant number 19-72-30005, https://rscf.ru/project/19-72-30005/.
Conflict of interest
The authors declare that the research was conducted in the absence of any commercial or financial relationships that could be construed as a potential conflict of interest.
Publisher’s note
All claims expressed in this article are solely those of the authors and do not necessarily represent those of their affiliated organizations, or those of the publisher, the editors and the reviewers. Any product that may be evaluated in this article, or claim that may be made by its manufacturer, is not guaranteed or endorsed by the publisher.
References
Ahmed, M., Alberti, D., Altieri, S., et al. (2023). Advances in boron neutron capture therapy. Vienna, Austria: International Atomic Energy Agency. CRCP/BOR/002, ISBN: 978-92-0-132723-9.
bnct-boron-neutron (2021). Accelerator-based BNCT projects. https://isnct.net/bnct-boron-neutron-capture-therapy/accelerator-based-bnct-projects-2021/.
Byambatseren, E., Burdakov, A., Bykov, T., Kasatov, D., Kolesnikov, I., Savinov, S., et al. (2023). Validation and optimization of the epithermal neutron flux detector using the 71Ga(n,γ)72Ga reaction. JINST 18, doi:P02020doi:10.1088/1748-0221/18/02/P02020
Bykov, T., Kasatov, D., Koshkarev, A., Makarov, A., Porosev, V., Savinov, G., et al. (2021). Initial trials of a dose monitoring detector for boron neutron capture therapy. JINST 16, P01024. doi:10.1088/1748-0221/16/01/P01024
Dymova, M., Taskaev, S. Y., Richter, V. A., and Kuligina, E. V. (2020). Boron neutron capture therapy: current status and future perspectives. Cancer Commun. 40, 406–421. doi:10.1002/cac2.12089
Faião-Flores, F., Coelho, P. R. P., Arruda-Neto, J. D. T., Maria-Engler, S. S., and Maria, D. A. (2013). Cell cycle arrest, extracellular matrix changes and intrinsic apoptosis in human melanoma cells are induced by Boron Neutron Capture Therapy. Toxicol. Vitr. 27, 1196–1204. doi:10.1016/j.tiv.2013.02.006
Franken, N. A. P., Rodermond, H. M., Stap, J., Haveman, J., and van Bree, C. (2006). Clonogenic assay of cells in vitro. Nat. Protoc. 1, 2315–2319. doi:10.1038/nprot.2006.339
Guan, X. C., Gong, Y., Murata, I., and Wang, T. (2019). The new design and validation of an epithermal neutron flux detector using 71Ga(n,γ)72Ga reaction for BNCT. JINST 14, P06016. doi:10.1088/1748-0221/14/06/P06016
Guan, X. C., Manabe, M., Murata, I., and Wang, T. (2015). Design of an epi-thermal neutron flux intensity monitor with GaN wafer for boron neutron capture therapy. J. Nucl. Sci. Technol. 52, 1–6. doi:10.1080/00223131.2014.956831
Kobayashi, T., and Kanda, K. (1983). Microanalysis system of ppm-order 10B concentrations in tissue for neutron capture therapy by prompt gamma-ray spectrometry. Nucl. Instrum. Methods Phys. Res. 204, 525–531. doi:10.1016/0167-5087(83)90082-0
Sauerwein, W. A. G., Wittig, A., Moss, R., and Nakagawa, Y. (2012). Neutron capture therapy: Principles and applications. Berlin, Germany: Springer.
Taskaev, S., Berendeev, E., Bikchurina, M., Bykov, T., Kasatov, D., Kolesnikov, I., et al. (2021a). Neutron source based on vacuum insulated tandem accelerator and lithium target. Biology 10, 350. doi:10.3390/biology10050350
Taskaev, S., Bykov, T., Kasatov, D., Kolesnikov, I., Koshkarev, A., Makarov, A., et al. (2021b). Measurement of the 7Li(p,p'γ)7Li reaction cross-section and 478 keV photon yield from a thick lithium target at proton energies from 0.65 MeV to 2.225 MeV. Nucl. Inst. Methods Phys. Res. 502, 85–94. doi:10.1016/j.nimb.2021.06.010
Taskaeva, I., and Taskaev, S. Method Of Measuring Of Dose Produced By Recoil Nuclei. Patent for invention No. 2743417, 2021.
Wang, P., Zhen, H., Jiang, X., Zhang, W., Cheng, X., Guo, G., et al. (2010). Boron neutron capture therapy induces apoptosis of glioma cells through Bcl-2/Bax. BMC Cancer 10, 661. doi:10.1186/1471-2407-10-661
Yura, Y., and Fujita, Y. (2013). Boron neutron capture therapy as a novel modality of radiotherapy for oral cancer: principle and antitumor effect. Oral Sci. Int. 10, 9–14. doi:10.1016/S1348-8643(12)00046-8
Keywords: boron neutron capture therapy, charge particle accelerator, lithium target, dose, neutron detector
Citation: Bikchurina M, Bykov T, Ibrahim I, Kasatova A, Kasatov D, Kolesnikov I, Konovalova V, Kormushakov T, Koshkarev A, Kuznetsov A, Porosev V, Savinov S, Shchudlo I, Singatulina N, Sokolova E, Sycheva T, Taskaeva I, Verkhovod G and Taskaev S (2023) Dosimetry for boron neutron capture therapy developed and verified at the accelerator based neutron source VITA. Front. Nucl. Eng. 2:1266562. doi: 10.3389/fnuen.2023.1266562
Received: 25 July 2023; Accepted: 22 September 2023;
Published: 03 October 2023.
Edited by:
Fuminobu Sato, Osaka University, JapanReviewed by:
Shingo Tamaki, Osaka University, JapanJacob G. Fantidis, International Hellenic University, Greece
Copyright © 2023 Bikchurina, Bykov, Ibrahim, Kasatova, Kasatov, Kolesnikov, Konovalova, Kormushakov, Koshkarev, Kuznetsov, Porosev, Savinov, Shchudlo, Singatulina, Sokolova, Sycheva, Taskaeva, Verkhovod and Taskaev. This is an open-access article distributed under the terms of the Creative Commons Attribution License (CC BY). The use, distribution or reproduction in other forums is permitted, provided the original author(s) and the copyright owner(s) are credited and that the original publication in this journal is cited, in accordance with accepted academic practice. No use, distribution or reproduction is permitted which does not comply with these terms.
*Correspondence: Sergey Taskaev, dGFza2FldkBpbnAubnNrLnN1