- 1SUBATECH (IMT Atlantique, CNRS/IN2P3, Nantes Université), Nantes, France
- 2Mokili Consulting, Nantes, France
The sorption properties of I2 and CH3I on activated carbon were reviewed. The dependence of the sorption capacity on activated carbon of iodine and methyl iodide in air or effluent gas on the sampling operating conditions (temperature, relative humidity, linear velocity and bed length) was investigated. A compilation of experimental data on the sorption efficiency, penetration or decontamination factor of gaseous radioactive iodine species was carried out for nuclear-grade activated carbon. Non-linear surface fitting of experimental data of I2 and CH3I sorption efficiencies by activated carbon at different values of temperature and relative humidity shows that there are ranges of variation in both parameters where the removal capacity remains relatively constant. The values and associated uncertainties of the sorption efficiencies of I2 and CH3I by activated carbon cartridges with bed lengths of 25 and 50 mm were determined for different specified ranges of temperature, relative humidity and linear gas flow velocity. The Sorption Efficiency (SE) values can be used to determine the concentration of iodine radioisotopes in the gaseous effluent or ambient air in Bq/m3 from the results of radionuclide activity in the cartridge measured by gamma spectrometry.
1 Introduction
Radioactive isotopes of iodine are potentially hazardous fission products that could be generated as a uranium fission product during the nuclear power plants’ operations, as they can critically impact the workers and the environment. A number of isotopes of iodine, notably 131I to 135I and 132I from the parent 132Te, are produced in considerable quantities in the fuel of nuclear reactors. Among the various types of isotopes, iodine-131 (131I) released as gaseous effluents from the nuclear power plants (NPPs) has been a critical environmental concern.
The main forms of radioactive iodine present in the atmospheric environment as a result of human activity are: 1) gaseous iodine, in organic (CH3I) or elemental form (I2), as inorganic compounds such as HI and HIO, 2) particulate iodine (also known as attached iodine), whose particle diameter varies with the level of aerosol aggregation.
Thyroid and whole-body exposure to atmospheric release of radioiodine can occur through several pathways including: (1) ingestion of foodstuffs such as milk; (2) inhalation; and (3) air submersion. The inhalation pathway is normally assessed by air sampling. Species identification allows differentiation of those forms of iodine that are prone to deposition on vegetation and soil (elemental iodine) from those that are not (CH3I and HIO). All chemical forms can be readily inhaled and contribute to thyroid exposure; however, it is primarily the elemental form that enters the food chain. The manner in which radioiodine concentrations are distributed among the various chemical forms is key input information for accurate environmental dose estimates (DOE, 2015; IAEA, 1973).
Elemental iodine and methyl iodide are today considered the most important forms of iodine for the design of absorbing devices in nuclear power plants. The distribution of gaseous radioactive iodine species in gaseous releases from nuclear power plants was studied for 1,300 MWe nuclear reactors during the period of full power operation and during the period of shut down for maintenance or nuclear fuel loading (KfK, 1979). The results of these 1-year studies on 3 similar PWRs showed that the distribution of organic (CH3I) and inorganic (I2) radioactive iodine species can differ from one nuclear plant to another, and varies according to whether the nuclear reactor is operating at full power or in shutdown. With PWR-2, the ratio of organic 131I was higher than that of inorganic 131I (63% and 37% respectively calculated from rejection rates). PWR-1 and PWR-2 had a similar distribution of iodine species. Whereas for PWR-3, the inorganic 131I ratio was highest during full-power operation, and the organic 131I ratio was highest during shutdown. Overall, the organic 131I ratio was lower than the inorganic 131I ratio (41% and 59% respectively for the calculation based on release rates) for PWR-3. The following average distribution of radioiodine compounds has been determined in the off-gas from BWR nuclear reactors: 7% for radioiodine associated with particulates, 36% for elemental iodine (I2), 26% for hypoiodous acid (HIO) and 31% for organic iodides such as CH3I (SAIC, 1989). During environmental monitoring for incremental exposure in the United States, and for air sampling and analysis to estimate the Estimated Total Effective Dose (TED) from gaseous effluent releases for the Maximally Exposed Individual (MEI) or Representative Person, the analysis with differentiation of the following species I2 + CH3I + HIO is required when TED> 5 mrem/yr (>0.05 Sv/yr) (DOE, 2015). The chemical change in iodine species has been reported by Kabat (Kabat, 1988), who shows that as aging of radioactive iodine occurs, the chemical species change from reactive forms (I2 and HIO) to the less reactive organic form (CH3I). He also noted that in 27 days the proportion of HIO species decreased from 54% to 9%, while the proportion of organic forms increased from 35% to 86%. A similar observation was made at CANDU nuclear power plants, showing that the initial HOI species portion range of 55%–75% decreased within a few weeks to the 30%–40% range, while the organic iodine portion increased from 10%-20% to 40%–60%. The main physical and chemical forms of iodine that can be found in the containment after a core melt accident are gaseous elemental iodine I2, particulate iodine (i.e., in aerosol form, such as cesium iodide CsI) and gaseous organic iodine (e.g., methyl iodide CH3I). According to the 3 source terms corresponding to the 3 major nuclear reactor disaster scenarios designated S1 (short-term containment failure occurring no more than a few hours), S2 (direct releases to the atmosphere after one or more days loss of containment) and S3 (indirect, delayed releases to the atmosphere) envisaged for the 900 MWe PWR, the percentages of initial activity of radioactive substances present in the reactor core that can be released into the environment are in a ratio of inorganic/organic iodine of (60/0.7), (5.7/0.55) and (0.3/0.55) respectively for S1, S2 and S3 (Jacquemain et al., 2015).
The general ultimate purpose of the monitoring is to provide means of checking the release of iodine. Monitoring is also necessary for checking filter and process operation of a nuclear plant. It can sometimes be advantageous to monitor the presence of iodine in the environment around nuclear power plants as a final check that releases are within authorized limits. Monitoring systems for radioactive iodine in air can be either continuous or based on periodic sampling at certain time intervals. Under normal nuclear reactor operating conditions, release rates of radioactive iodine from the reactor core and coolant are very low. Volumetric activities of less than 400 Bq/m3 were detected at the inlets to the air exhaust filters of the reactor containment systems. Typical annual discharge rates for European reactor stations are in the range of 107–109 Bq/yr of iodine-131 (Choi et al., 2021). An average 131I discharge of 5.6 × 109 Bq/yr is usual for pressurized water reactors (PWR) in the United States (Choi et al., 2021; IAEA, 1987). In accidental situations, the amounts of iodine released into the containment systems and the environment depend on many factors and the quantities released may represent a significant percentage of the core inventory. In existing nuclear power plants, the continuous discharges and the air of the reactor building are currently monitored by the radioprotection devices and sampling is carried out by the Plant Radiation Monitoring System PRMS (or KRT) lines at the stack of the Nuclear Auxiliary Building, and sampling of the radio-halogens is often carried out on activated carbon cartridges (AREVA/EDF, 2012).
Activated carbon (activated charcoal) is a widely used adsorbent for trapping radioactive iodine gases to protect personnel and the environment of nuclear facilities, including nuclear power plants (NPPs), and is also used in military protective respirators and purification devices for various toxic gases, vapors and liquid chemicals, as it has high porosity and specific surface area (Choi et al., 2021). Activated carbon cartridges are replaced periodically, and the activities of iodine radioisotopes contained in activated carbon are determined by gamma spectrometry (AFNOR, 2005; Nolan et al., 2021; Kravchik et al., 2008; Montgomery, 1990). Bear in mind that activated carbon can retain other gaseous compounds that may contain radionuclides and noble gases, some isotopes of which are radioactive. In some papers, it was shown that the parameters for adsorption capacity are influenced by different physical and environmental conditions. Thus, the sorption efficiencies of the various radioactive iodine species retained in the activated carbon cartridges under the sampling conditions are important for determination of radionuclide concentrations in the gaseous discharge or ambient air.
A review of studies on the retention of gaseous iodine species by activated carbon was carried out in order to compile experimental data from work carried out on iodine retention as a function of variation in different factors, and to assess the amplitude of impact of certain factors such as temperature and humidity.
2 Activated carbon adsorbers
2.1 Activated carbon
Activated carbons, also called activated charcoals, are materials that are essentially composed of carbonaceous matter with high specific surface area and high porosity structure. Activated carbon is usually derived from waste products such as coconut husks; walnuts; charcoal; anthracite; bamboo, oil-palm shells, waste from paper mills, tyres and bituminous coal has been studied as a source. Activated carbons are generally produced in two steps: firstly, by carbonization or pyrolysis, and secondly by an activation step.
The carbonization step generates porosity in the raw carbonaceous material by removing elements other than carbon. The porous structure is defined by pore size distribution and can be classified into three categories. The first category includes those that have small pores to allow one or two molecules to be adsorbed per pore with strong interaction between the adsorbed molecules and the pore wall. The secondary category has mesopores that may demonstrate capillary phenomena and characteristics of hysteresis loops under relatively high pressure (Chien et al., 2011). The third category consists of macroporal sizes with relatively lower ratio of surface area to porosity. Although macropores have a low specific surface area, pores can act as channels between mesopores and micropores (Ho et al., 2019).
The activation procedure is performed to increase the material’s retention capacity. Two types of activation procedures can be carried out: physical activation in the presence of water vapor and pressurized air at temperature of about 800°C–1,000°C or chemical activation generally carried out by phosphoric acid treatment.
The heat activation and vacuum activation have been used by Ji (Ji, 2024) to re-increase the adsorbed amount of Xe and Kr from activated charcoal used in radioactive off-gas treatment systems in nuclear power plants. Their relatively large specific surface area of activated carbon varies between 500 and 1,800 m2/g with an average of around 1,000 m2/g (Lin et al., 2022; Chebbi et al., 2022; Qi-dong and Sui-yuang, 1984; ANDRA, AREVA, CEA, EDF, Marine Nationale, 2011; González-García et al., 2011; Lin, 2022; Chebbi et al., 2024). The pore size ranging from 4.5 to 60 Å and charcoal particle size ranging from 0.5 to 5.0 mm (Huve et al., 2018).
The tests to assess the retention of radioactive methyl iodide (CH3I) or radioactive elemental iodine gas (I2) under various conditions are generally carried out using standard methods (Table 1) such as ASTM-D3803-91 (ASTM, 2022), ANSI/ASME N509 (ANSI/ASME, 1976; ANSI/ASME, 2002), ISO 18417 (ISO, 2019) or NF M62-206 (AFNOR, 1984). These standards cover test methods to establish the ability of new and used activated carbon to remove gaseous radioactive iodine and iodine compounds from air and gas streams.
The Sorption Efficiency (also referred to as the retention efficiency) expressed in % [SE (%)] is defined as the fraction of iodine captured by the material and is calculated by the following relationship (Equation 1):
The decontamination factor DF (Equation 2) is defined as the ratio of the original concentration of the contaminant to the concentration that remains after removal.
The sorption efficiency SE (%) is can be related to the penetration expressed in % [P (%)] and the decontamination factor DF by the (Equation 3):
The performance factor K is calculated according to the relationship (Equation 4):
τ = Residence time (or contact time) in sec.
The sorption capacity index ξ is defined according to the ISO 18417 (ISO, 2019) by the relationship (Equation 5):
Some authors have used the removal coefficient μ to quantify the removal capacity of an activated carbon (Shiomi et al., 1982). The sorption efficiency SE (%) is can be related to the removal coefficient by the (Equation 6):
μ = Removal coefficient (cm−1), l = bed length (cm).
2.2 Impregnated activated carbons
To enhance the radioactive iodine trapping efficiency, many researchers have attempted to modify the surface functional groups and porosity of activated carbon or by impregnating it with other specific molecules. The studies have focused mainly on activated carbons and their forms impregnated with KI and/or TEDA (Triethylenediamine). Other inorganic impregnants have been used, such as KOH (Ramarathinam et al., 1989), KIO3, SnI2, KSCN (Kitani et al., 1971), BaI2 (Rastunov et al., 2010) or organic impregnants, such as, Quinuclidine (Choi et al., 2021; Ho et al., 2019), Hexamethylenetetramine (Huve et al., 2018; Kitani et al., 1971; Evans, 1976a). The effects of the nature of the various inorganic and organic impregnants on CH3I trapping by activated carbon produced from coconuts had been studied by Kitani et al. (1971) under the following experimental conditions: temperature = 70°C, relative humidity = 90%, flow velocity = 24 cm/sec, residence time = 0.10 s, charcoal grain size = 0.5–1.19 mm. They concluded that stannous iodide, potassium thiocyanate, potassium iodide, iodine, bromine and triethylenediamine (TEDA), were found to be effective for trapping radioiodine in the form of methyl iodide. According to Ho et al. (2019), activated carbon impregnated with quinuclidine (QD) has a much higher CH3I removal efficiency than activated carbon impregnated with (TEDA) with the same impregnant content.
The amount of KI in activated carbon ranges from 0% to 5% wt and from 0% to 10% wt for TEDA. In the case of activated carbon impregnated with a KI + TEDA mixture, the ratios of these two compounds are highly variable. The impact on DF of varying the proportions of KI and TEDA in activated carbons impregnated at 40% and 90% RH was extensively studied by Lin et al. (2022). The effect of the presence of impregnant in the internal porosity of several activated carbons has been studied and discussed in terms of pore characteristics in some papers (Lin et al., 2022; Chebbi et al., 2022; Lin, 2022). The tested activated carbon compounds exhibit in general high specific surface area and an important contribution due to the microporous volume (Vmicropore/Vpore > 90%) (Lin et al., 2022; Lin, 2022; Chebbi et al., 2024). The microporous characteristics observed are in agreement with activated carbon made from coconut shells, which are generally used in the nuclear field. However, different activated carbon characteristics can be observed from one batch to the next during manufacture. The CH3I adsorption isotherms and the overall adsorption capacities (qm) of commercial impregnated activated carbons have been studied by some researchers and the determined qm values are ranged between 0.117 and 0.204 g/g (adsorbed species/adsorbent) (Chebbi et al., 2022); Chebbi et al., 2024). The authors observed that the decrease in overall adsorption capacity with increasing temperature, also reported in several other studies, can be explained by the reduction in physisorption phenomena, which are preferentially favored at low temperatures. The study of Park et al. (2001) have reported a progressive decrease in the physisorbed proportion of CH3I by an impregnated activated carbon (6.5% wt TEDA) from 58% to 34% for temperatures ranging from 35°C to 75°C. The decrease extent in adsorption capacities (qm) depends on the activated carbon nature. The decrease in global adsorption capacity from 35°C to 75°C for the non-impregnated activated carbon was around 76% and this decrease was all the lower (32%–53%) as the amount of TEDA in the impregnated activated carbon increased (Chebbi et al., 2022).
The reactions of iodine species with activated carbon can be classified into 3 main reactions: physisorption, chemisorption and isotope exchange. The main iodine species (I2, CH3I) are generally retained by activated carbon micropores close to 0.5 nm in size, by a physisorption mechanism. Due to the fact that activated carbons retain relatively high quantities of elemental iodine I2, both 127I and 131I will be retained on the carbon surface, mainly through a physisorption mechanism. Porosity changes upon impregnation with tertiary amine affect the adsorption rate and physisorption, thereby affecting the adsorption capacity.
The mechanisms of CH3I/Activated Carbon (AC) interaction continue to be investigated, but the most widely accepted mechanism is that organic compounds iodides are chemisorbed on activated carbon that has been impregnated with iodides by an isotopic exchange reaction. For example, for a K127I impregnation, we have the following reaction:
Although only the iodine of the CH3I compound is involved in the isotopic exchange 131I mechanism, the removal efficiency of CH3131I is assumed to be the same as that of 131I. If it is assumed that at the beginning no 131I is present on the surface of the activated carbon, but is introduced with CH3131I in vapor form, then the surface concentrations of each of the four types of species will evolve to reach equilibrium values. The isotope exchange reaction cannot be completed until all the CH3131I has been adsorbed and desorbed. The only factor that reduces the exchange rate from the initial value is the increase in 131I concentration compared with 127I concentration. Thus, the rate determining step in isotope exchange is the rate of adsorption and desorption of methyl iodide, and as long as an excess of 127I is present the overall isotope exchange balance is displaced towards the formation of K131I. In the case of methyl iodide, no difference in adsorption rate can be expected between CH3131I and CH3127I, as the relative size and polarity of both molecules are identical.
For an amine impregnation, heterocyclic amines such as TEDA seem to be excellent molecules for sorption of organic iodides because the amine is more reactive with organic iodide than with water, and the double amine groups provide a strong attachment to both the activated charcoal (AC) and the organic iodide. The chemical reaction is usually represented as:
The quaternary amine produced is then strongly adsorbed onto activated charcoal. The reaction mechanisms of TEDA and QD with CH3I have been extensively studied by Ho et al. (2019) and Ho et al. (2019). The adsorption kinetics of CH3I on activated carbon had been investigated by Friedrich (Friedrich, 1988) in the temperature range from 17°C to 80°C. He showed from the temperature dependence of the kinetic parameters that the CH3I adsorption process is exothermic, and the activation energy calculated using the Arrhenius equation is 67 kJ/mol (16 kcal/mol). The adsorption rate constant (KF), desorption rate constant (KB) and “active site concentration” (A0) were determined by fitting theoretical curves to experimentally measured breakthrough curves. The values for KF, KB and A0 were 3 × 103 cm3. mol−1. sec−1, 2.5 × 10−4 sec−1 and 2.3 × 10−3 mol.cm−3, respectively for the system composed of methyl iodide vapor as adsorbate and granulated activated carbon as adsorbent. His observation from the curve of the temperature dependence of the concentration of active sites suggests that the number of active sites available for adsorption under given thermodynamic conditions also has a dynamic character and is influenced by temperature via the two-dimensional movement of molecules adsorbed on the surface of the active sites.
In the USA, impregnated charcoals are required to trap highly penetrating organic iodides and the performance of the activated charcoals for organic iodides must be qualified under a range of operating conditions specified in RDT/M/16-1T. The minimum accepted efficiencies for I2 and CH3I at low (21°C) and high (80°C) temperatures and low (70%) and high (>95%) relative humidity are specified in ANSI N509 and RDT/M/16-1T (Holladay, 1979). Any good grade of activated carbon, with or without impregnant, will deliver minimum decontamination factor (DF) of 99.5 for I2 and 95.0 for CH3I with any combination of temperature and humidity that would be encountered in a nuclear air-cleaning system (AEC, 1973). In Europe, the testing of activated carbon filters used in nuclear facilities is achieved in situ for the industrial ventilation system by periodic injection of CH3I and/or I2 and can be defined according to different methods (ISO, 2019; Holladay, 1979). The decontamination efficiency will depend mainly on device construction, adsorbent characteristics, temperature, relative humidity and gas flow rate, bed length and adsorbent layer packing density, as well as other external factors. According to ISO 18417 (ISO, 2019) and Rastunov et al. (2011), the performance of nuclear-grade activated carbon used for CH3131I removal from gaseous radioactive wastes at nuclear power plants is satisfactory if ξ > 4.605/τ at 30°C, 90% RH and τ = 0.2–0.4 s. In practice, this means that DF > 100 under the same operating conditions.
2.3 Influence of different parameters on the capture performance of activated carbon
The radioactive iodine removal efficiency of impregnated activated carbons is a complex process, which is influenced by several parameters. Many previous studies were carried out to evaluate the removal efficiencies of activated carbon by changing various test conditions such as temperature, relative humidity, linear velocity, bed depth, particle size distribution, impregnant, run duration, and aging, weathering, and poisoning. In general, it has been demonstrated under similar experimental conditions, I2 trapping by impregnated activated carbon is systematically higher than CH3I trapping. As a result, much work has focused on CH3I removal studies and evaluating the impact of various factors on activated carbon trapping.
2.3.1 The influence of charcoal grain size and linear velocity on the performance of activated carbon adsorbent
The effective density of activated carbon depends on grain size and varies from 0.48 to 0.66 g/cm3. The effects of particle size on CH3I removal were studied by Kovach (Kovach, 1992; Kovach, 1998) using activated carbon impregnated with 2% KI and 2% TEDA +2% KI for 50 mm and 100 mm of activated carbon layer. Each sample was tested according to the ASTM D3803 standard at 30°C and 95% RH. The CH3131I penetration and sorption efficiency data for each of the two impregnant types are shown on Tables 2, 3. The sorption efficiency is always improved by using a smaller particle size adsorbent. In all cases for both impregnants (KI and KI + TEDA) the CH3131I sorption efficiency increased with decreasing particle size. If the average size of the adsorbent particles becomes very small, and the particles are well packed in the cartridge, the increase in pressure drop between the cartridge inlet and outlet may lead to additional difficulties in the sampling device.
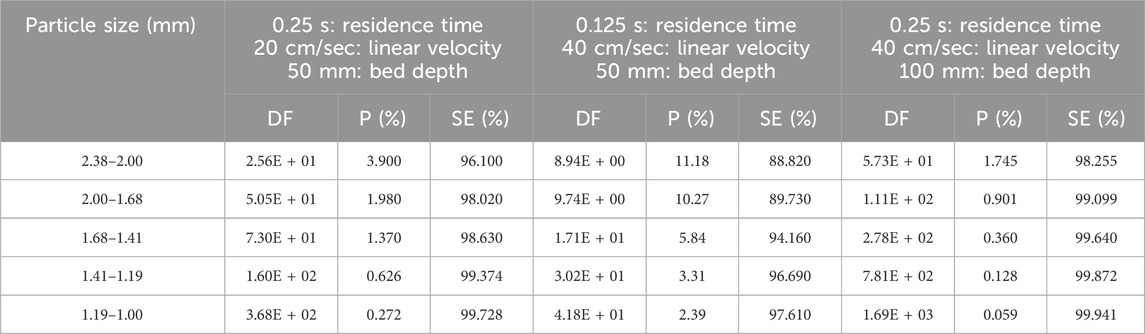
Table 2. Parametric study of particle size effects on CH3131I Penetration and Sorption Efficiency at 30°C, 95% RH for coconut-based activated carbon impregnated with 2% KI. Data from references (Kovach, 1992; Kovach, 1998).
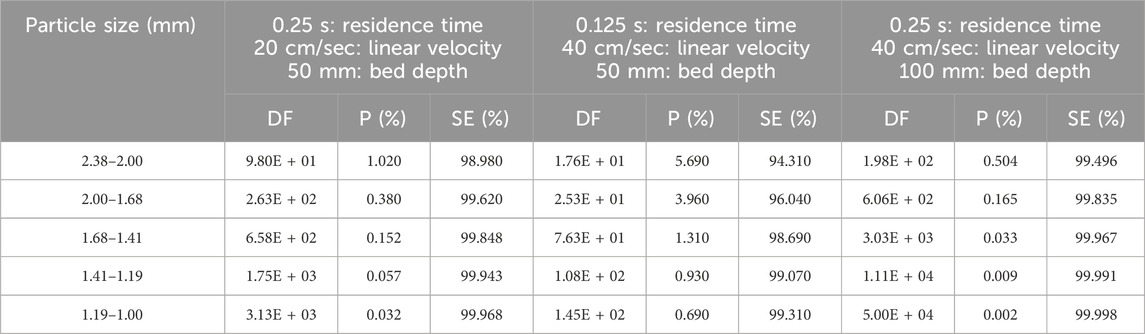
Table 3. Parametric study of particle size effects on CH3131I Penetration and Sorption Efficiency at 30°C, 95% RH for coconut-based activated carbon impregnated with 2% KI + 2% TEDA. Data from references (Kovach, 1992; Kovach, 1998).
The effects of linear velocity on CH3I removal were studied using commercial NUSORB KITEG II adsorbent (co-impregnated with KI and TEDA) for 25 mm, 50 mm and 100 mm of activated carbon layer (Kovach, 1992; Kovach, 1998). Each sample was tested according to the ASTM D3803 standard at 30°C and 95% RH. The CH3131I penetration and sorption efficiency data are shown on Table 4 and the results are plotted on Figure 1.
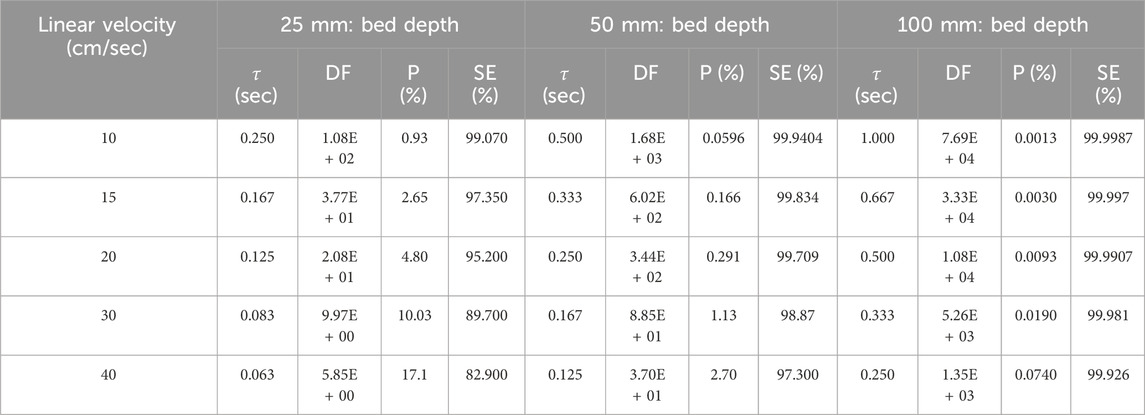
Table 4. Parametric study of linear velocity effects on CH3131I Penetration and Sorption Efficiency at 30°C, 95% RH for commercial NUSORB KITEG II adsorbent. Data from references (Kovach, 1992; Kovach, 1998).
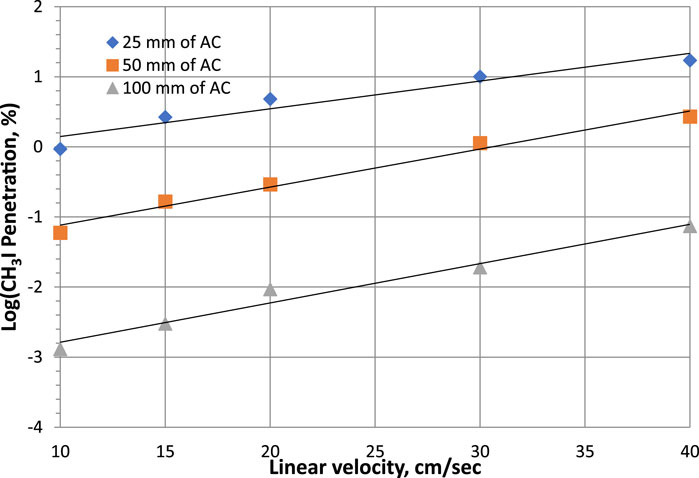
Figure 1. Parametric study of linear velocity effects on CH3131I Penetration at 30°C, 95% RH for commercial NUSORB KITEG II adsorbent. Data from Table 4 and references (Kovach, 1992; Kovach, 1998).
The parametric study of activated carbon performance is sometimes made by plotting the variation of the logarithm of penetration as a function of certain variables such as particle size, gas flow, temperature or relative humidity, as Kovach (Kovach, 1992) observed that the data generally line up on a straight line, which facilitates interpolations. For the 3 bed depth values, the sorption efficiency decreases with increasing linear velocity for the same bed depth. The iodine removal increases as the sorbent thickness increases for the same linear gas velocity.
Both observations (particle size and linear velocity effects) indicate that diffusion is a step controlling the kinetics of the CH3131I removal mechanism. Although there are differences in the mechanism of iodine retention between single KI and co-impregnated KI + TEDA activated carbons, this difference indicates that KI and TEDA are deposited in different parts of the pore structure, and that the rate of isotope exchange also affects the removal process. The main removal mechanism, even for TEDA impregnants, is isotope exchange, because the excess non-radioactive CH3127I first reacts with TEDA, and then CH3127I-CH3131I exchange can take place. The data also show the difficulty of comparing adsorbent materials for CH3131I removal when the particle sizes of the adsorbents are not identical.
According to Chang’s findings (Chang, 1989), the analysis of these experimental data indicates that pore diffusion is the controlling step when gas velocity is over 20 cm/sec, and both pore diffusion and external mass transfer resistance are contributed to the overall resistance when the gas velocity is 10 cm/sec. Thus, the reduction of removal efficiency under humid condition should be considered in view of the reduction of equilibrium adsorption capacity and the decrease of effective pore diffusivity. When the air velocity through the activated carbon layer is very low, the exchange kinetics between the reactive species in the gas phase and the reaction sites in the adsorbed phase leads to a static equilibrium state that depends only on the concentration of the reagents and the thickness of the activated carbon layer. On the other hand, when the air velocity through the layer becomes greater than the reaction kinetics of the species at the gas-solid interfaces, the previous static equilibrium state is never reached, and a dynamic equilibrium state is reached which depends only on the residence time of the gas in the adsorbent (Pradel et al., 1962). The linear velocity of the gas and the bed depth determine the residence time in the activated carbon. Sorption efficiency therefore depends on the gas residence time in the bed. The effects of residence time and linear CH3I velocity on the velocity constant and gas penetration in different activated carbon bed lengths have been extensively studied by Deitz and Blachly (1974). The general conclusion of their studies is that surface linear velocity plays a dominant role, while residence time plays a more subsidiary role, in influencing the velocity rate. In addition, the catalytic process appears to be more effective than simple adsorption in trapping methyl radioiodide. If the residence time is increased, radioactive iodine removal efficiency will be improved. For filtering or removal purposes the residence time must be long enough (0.1–1 s) to assure the required removal efficiency but, for monitoring, a lower efficiency and a shorter residence time (0.01–0.1 s) can often be tolerated. The residence time value τ ≥ 0.2 s is recommended by some standards for more efficient removal (ISO, 2019; AFNOR, 2005; IEC, 2002). However, τ ≥ 0.25 s is recommended by two other standard methods to determine the radioactive iodine trapping performance of nuclear grade activated carbon (ASTM, 2022; AFNOR, 1984).
2.3.2 The influence of temperature on the performance of activated carbon adsorbent
The temperature of the gas flow can affect the removal efficiency of radioactive iodine by activated carbon. Increasing temperature should increase both diffusion rates and chemical reactions, and thus improve CH3131I removal over the temperature range tested. The physicochemical reactions for removal of CH3131I are chemisorption and 131I-127I isotope exchange by the impregnants which are deposited on the activated carbon. Since chemical reactions and isotope exchange proceed more efficiently at higher than room temperatures, the removal efficiency is improved at slightly elevated temperatures. Kovach’s data (Kovach, 1992; Kovach, 1998) on the effect of temperature on sorption efficiency or penetration are shown in Table 5; Figure 2.
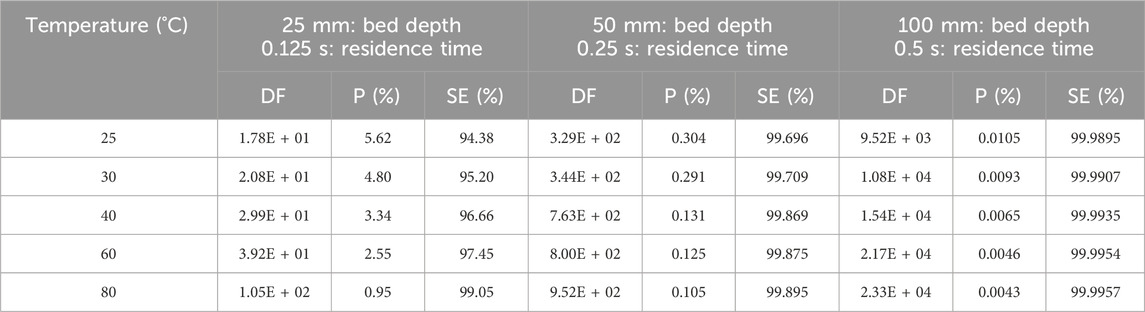
Table 5. Parametric study of temperature effects on CH3131I Penetration and Sorption Efficiency at 95% RH, 20 cm/sec for commercial NUSORB KITEG II adsorbent. Data from references (Kovach, 1992; Kovach, 1998).
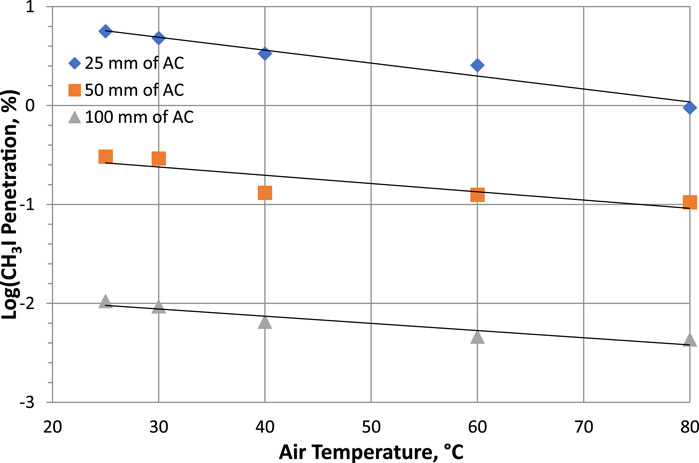
Figure 2. Parametric study of temperature effects on CH3131I Penetration at 95% RH, 20 cm/sec for commercial NUSORB KITEG II adsorbent. Data from Table 5 and references (Kovach, 1992; Kovach, 1998).
The improvement in performance is much more significant for shallow adsorbent beds (25 mm) than for deeper beds such as 50 mm and 100 mm. However, in all conditions, performance improved with increasing temperature. Other studies on the effect of temperature on the retention of I2 and CH3I by activated carbons confirm the improvement in removal with increasing temperature (Ramarathinam et al., 1989; Qi-dong and Sui-yuang, 1984; Suhariyono et al., 2014; Billinge and Broadbent, 1989). From the data in Table 5, it can be seen that for a temperature increase of 55°C (from 25°C to 80°C), the CH3I sorption efficiencies SE (%) increase of 4.67, 0.199 and 0.0062 respectively for activated carbon bed lengths of 25, 50 and 100 mm. Considering a wide range of temperature variations, the improvement in sorption efficiency can be considered significant for a bed length of 25 mm, whereas the variations in sorption efficiency are very small for 50 and 100 mm bed lengths, and the values can be considered practically constant compared to their respective average values. The results of CH3I retention studies carried out by Ramarathinam et al. with 50.8 mm activated carbon bed length and at very low relative humidity (<10% RH) for temperature variations ranging from 40°C to 100°C also show a very small SE (%) increase of 0.007 (Ramarathinam et al., 1989). On the other hand, other experiments carried out by the same authors at high relative humidity (≥97% RH) for a temperature increase from 35°C to 90°C show a different behavior, with a progressive decrease in sorption capacity (ΔSE (%) = −1.99). With a bed length of 25.4 mm, 90% RH and a temperature increase from 30°C to 45°C, Suhariyono et al. observe a significant increase in CH3I SE (%) of 10.9 (Suhariyono et al., 2014). One of the explanations given is the increase in water desorption from the micropores of the active carbon as the temperature rises, and this phenomenon results in the increasing sorption of methyl iodide in the micropores released by the water molecules (water desorption process). Using the removal coefficient μ, studies by Shiomi et al. show a slight increase in the μ values of CH3I for various sorbents tested with increasing temperature from 20°C to 85°C (Shiomi et al., 1982).
The melting and boiling points of TEDA are 158°C and 174°C respectively, and this compound has a vapor pressure that cannot be ignored, especially at high temperatures. The vapor pressure of TEDA impregnated on activated carbon is not known, but values for the pure compound for temperatures ranging from 25°C to 70°C have been reported by Billinge and Broadbent (1989) and are shown in Table 6.
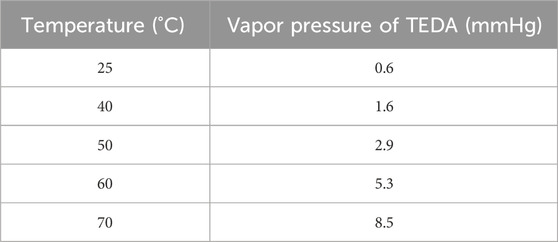
Table 6. Vapor pressure of TEDA at different temperatures. Data from reference (Billinge and Broadbent, 1989).
We can therefore assume that when a high-temperature gas stream flows through an activated carbon impregnated with TEDA over a long period of time, there will be a risk of altering the removal capacity of the activated carbon. But other chemical reactions can occur, notably with water molecules to give quaternary amine hydroxides as reported by Billinge and Broadbent which limits the loss of TEDA by evaporation and makes predictions of activated carbon degradation very difficult.
Park et al. (2001) mentioned that due to the chemical reaction, the TEDA-methyl iodide complex is very stable, and pure TEDA begins to vaporize at 174°C, the TEDA-methyl iodide complex begins at 270°C and a TEDA-methyl iodide on TEDA-AC begin to decompose at 200°C. Based on these results, they concluded that activated carbon impregnated with TEDA can be used to remove radioactive iodine below 150°C. However, a change in the sorption capacity of activated carbon impregnated with TEDA (commercial type SKT-3I) has been reported by Rastunov et al. due to the partial removal of triethylenediamine (TEDA) as a result of the relatively high vapor pressure of this impregnant (Rastunov et al., 2011). The loss of impregnant as a result of evaporation should not be observed with activated carbon impregnated only with KI. Thus, all tests to assess the performance of impregnated activated carbons are carried out at temperatures not exceeding 150°C.
Qi-dong et al. studied the retention of radioactive elemental iodine for different temperatures (40°C–70°C) with different activated carbon bed lengths from 5 to 50 mm (Qi-dong and Sui-yuang, 1984). A different behavior was observed for I2 retention, where the data in Table 7 show that decontamination factors tend to decrease with increasing temperature for 2 activated carbon bed lengths impregnated with 2% TEDA-2% KI and the variations in Log10(P %) as a function of temperature are plotted in Figure 3.
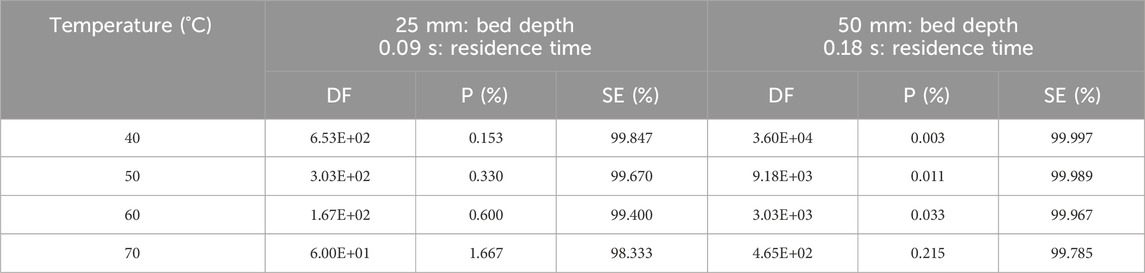
Table 7. Parametric study of temperature effects on Decontamination Factor, Penetration and Sorption Efficiency of radioactive elemental iodine I2 at 95% RH, 28 cm/sec for activated carbon with 2% TEDA - 2% KI impregnation. Data from reference (Qi-dong and Sui-yuang, 1984).
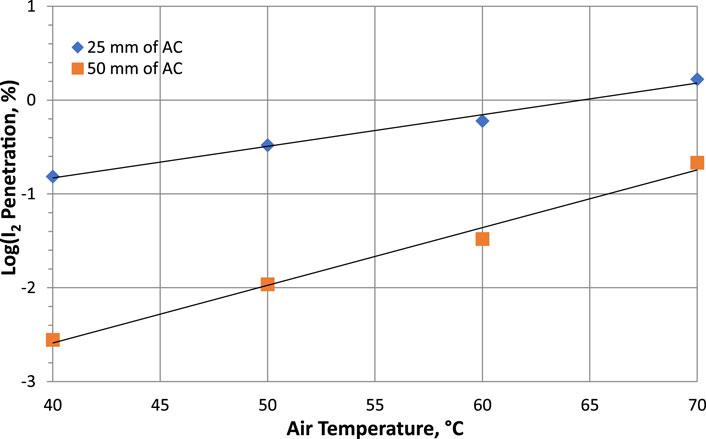
Figure 3. Parametric study of temperature effects on Penetration of radioactive elemental iodine I2 at 95% RH, 28 cm/sec for activated carbon with 2% TEDA - 2% KI impregnation. Data from Table 7 and reference (Qi-dong and Sui-yuang, 1984).
On the other hand, sorption efficiency values change only slightly (ΔSE (%) = 1.514 and 0.212 for 25- and 50-mm bed lengths respectively) over the temperature range studied (40°C–70°C). For the removal radioiodine at very high temperatures (superheated steam over 150°C) and in oxidizing gases, inorganic, non-flammable adsorber materials should be used instead of activated charcoal, according to Wilhelm and Schuettelkopf (1973). The increase of temperature is known to favor the carbon oxidation phenomena leading to sorbent ignition at temperatures higher than 300°C generally for basic activated charcoal or for activated charcoal impregnated only with KI. On the contrary, activated charcoal impregnated with TEDA display lower ignition temperature due to the low flash point of the TEDA molecule. Some examples of ignition temperatures are reported by Lin (2022) for unimpregnated activated carbon and activated carbon impregnated with KI or TEDA. The ignition temperatures range from 190°C to 340°C, depending on impregnant composition. The ignition temperature of coconut-based activated carbons had been determined by Evans (1976b) in a quartz apparatus at a linear face velocity of 27.94 cm/sec. Ignition temperatures ranged from 275°C to 340°C for pure activated carbon and from 312°C to 437°C for activated carbons impregnated with 1%–2% TEDA +2% KI and additionally containing 1% flame retardant. However, some work has been carried out by Collinson and Taylor (1988) on the trapping performance of methyl iodide in CO2 at pressures up to 42 bar and temperatures up to 275°C. The aim of their studies was to underwrite the performance of iodine adsorption facilities in the purge systems of gas-cooled reactors in the UK. They reported that the high-temperature trapping process is controlled by the desorption of iodine species from the charcoal, and when the temperature is reduced below 200°C, there is a progressive reduction in charcoal performance with increasing pressure. A loss of impregnant and the presence of iodine species other than incident methyl iodide were observed.
In general, large variations in airflow temperature do not necessarily lead to significant variations in sorption efficiency for good quality activated carbons, as long as the residence time remains within the recommended range and moisture saturation is not approached.
2.3.3 The influence of air humidity on the performance of activated carbon adsorbent
Activated carbon is hygroscopic and adsorbs large quantities of water in high humidity. The adsorbed water prevents radioactive iodine compounds from being adsorbed by the activated carbon because the presence of water vapor leads to the competitive adsorption between iodine species and the water molecules. Although in most studies of the effect of air humidity on iodine retention, air humidity is expressed in relative humidity (% RH) and not in absolute humidity (g/m3), it is important to remember that the chemical reaction equilibria for sorption of gaseous species such as H2O, I2 or CH3I molecules at air/activated carbon interfaces only take into account the volumetric concentrations (moles/m3, g/m3 or Bq/m3) of the reagents in the air (Friedrich, 1988; Shiomi et al., 1982), and therefore depend on the absolute humidity of the air in the case of water adsorption. Some studies on the variation in sorption capacity as a function of absolute air humidity have been reported (ISO, 2019; Rastunov et al., 2011). The mechanism of water adsorption in activated carbon micropores and mesopores had been investigated by Alcañiz-Monge et al. (2001) over a wide range of water partial pressures. These authors showed that the shape of the water isotherm has a clear correlation with the pore size distribution and at relative humidities of between 40% and 70%, water molecules progressively fill the micropores via a clustering mechanism. Micropore filling is not complete until around 0.82, when capillary condensation begins in activated carbons with relatively low surface polarity. For RH = 70–95%, they observe mesopore filling by the capillary condensation mechanism. At high RH > 95%, macropores are filled by capillary condensation. It should be noted that the retention of iodine species by activated carbon remains efficient at RH<40%, due to the hydrophobic nature of carbon, which limits water adsorption. The presence of surface groups affects the water isotherm in two ways: by controlling the relative pressure at the start of water adsorption in micropores and the filling of mesopores and macropores by capillary condensation through their effect on the contact angle with the adsorbing liquid prevailing on the pore wall. Studies by Velasco et al. (2016) have provided further insights into the influence of the surface oxygen content of porous carbonaceous materials on the mechanisms prevailing at the start of the water sorption process. It has been shown that above a certain surface oxygen concentration (≈16 wt%), there is a change in the sorption mechanism (from clustering to layering), reinforced by steric effects. The size of the water cluster, formed before micropore filling begins, decreases steadily from the initial value. In addition, the presence of very large quantities of oxygenated groups on the sorbent surface inhibits the appearance of water-water interactions and hence the formation of water clusters.
Experimental data from the Kovach study (Kovach, 1992; 1998; Scarpellino and Sill, 1987) on the effect of relative humidity on the CH3131I penetration and sorption efficiency data are shown on Table 8 and the results are plotted on Figure 4. A progressive decrease of sorption efficiency with increase of relative humidity was observed up to 95% RH. The same observation was made with H131I species for two activated carbon bed lengths (Table 9). Other experimental data from Kavach’s work on the water adsorbed ant the CH3I retention up to very high relative humidity are presented in Table 10 and the results are plotted in Figures 5, 6.
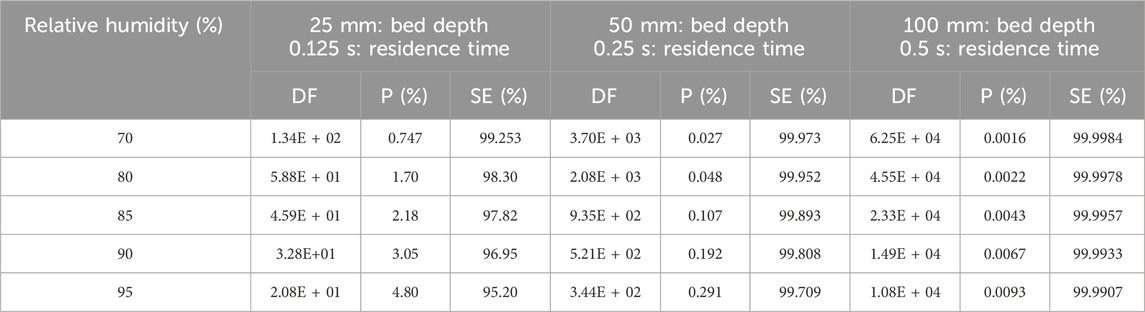
Table 8. Parametric study of relative humidity effects on CH3131I Penetration and Sorption Efficiency at 30°C, 20 cm/sec for commercial NUSORB KITEG II adsorbent. Data from references (Kovach, 1992; Kovach, 1998).
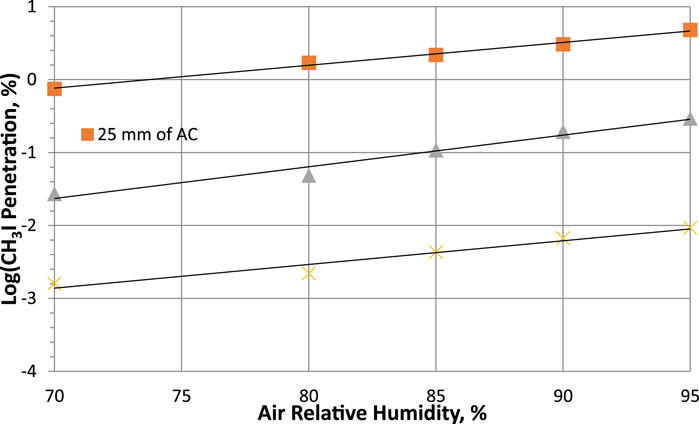
Figure 4. Parametric study of relative humidity effects on CH3131I Penetration at 30°C, 20 cm/sec for commercial NUSORB KITEG II adsorbent. Data from Table 8 and references (Kovach, 1992; Kovach, 1998).

Table 9. Parametric study on H131I Penetration and Sorption Efficiency for commercial NUSORB KITEG II adsorbent. Data from references (Kovach, 1992; Kovach, 1998).
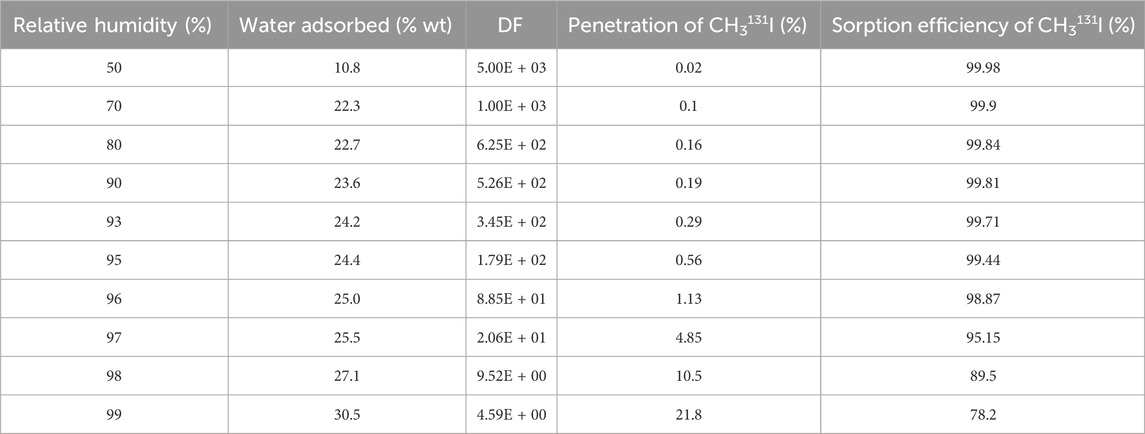
Table 10. The water adsorbed ant the effect of relative humidity on methyl iodide removal for 50 mm bed depth of impregnated activated carbon (2% KI + 2% TEDA) operated at 30°C and 20 cm/sec. Data from references (Kovach, 1998; Scarpellino and Sill, 1987).
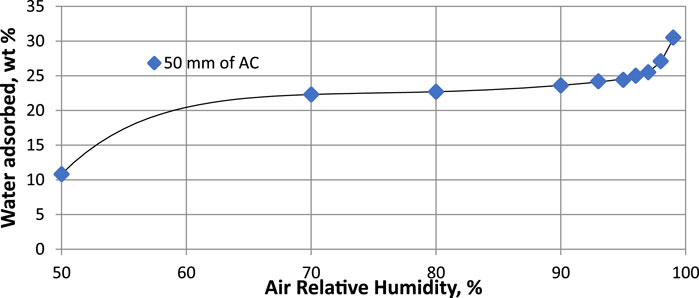
Figure 5. Water adsorbed during the methyl iodide removal for 50 mm bed depth of activated carbon (2% KI + 2% TEDA) operated at 30°C and 20 cm/s. Data from Table 10 and references (Kovach, 1998; Scarpellino and Sill, 1987).
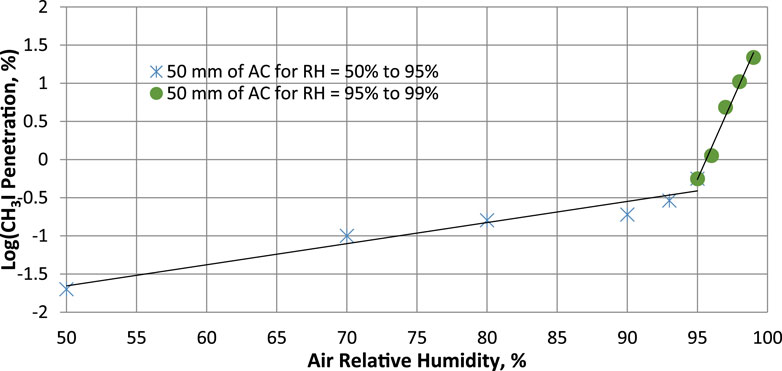
Figure 6. The effect of relative humidity ranging from 50% to 99% on methyl iodide removal for 50 mm bed depth of activated carbon (2% KI + 2% TEDA) operated at 30°C and 20 cm/s. Data from Table 10 and references (Kovach, 1998; Scarpellino and Sill, 1987).
From the data in Table 8, it can be observed that for a 25% increase in relative humidity SE (%) in the 70%–95% range, CH3I sorption efficiencies SE (%) decrease of 4.053, 0.263 and 0.0077 respectively for activated carbon bed lengths of 25, 50 and 100 mm. A significant drop in sorption efficiency is observed for a bed length of 25 mm, while for bed lengths of 50 and 100 mm the variations in sorption efficiencies are very small and the values can be considered relatively constant within the measurement uncertainties of their averages. When the relative humidity of the air flow is above 95%, performance is dramatically reduced.
The data in Table 10 show that in a reduced range of very high humidity such as the 95%–99% RH range, there is a sharp drop of 21.24 units (from 99.44% to 78.2%) in the CH3I sorption efficiency value for an active carbon bed length of 50 mm, whereas for RH <95% and the same bed length value, the data in Table 8 show very small retention variations. Figure 6 shows that the points do not line up on the same straight line throughout the RH range. Above 95% RH, the data align on another line with a higher slope. The significant change in activated carbon behavior occurs at around 95% RH. At high relative humidity, physical adsorption of water vapor can influence the available porosity and block some adsorption sites for trapping iodine. However, prolonged exposure to water vapor has additional adverse effects, and these can continue to degrade the activated carbon.
The performance of activated carbon impregnated with different moisture loads due to increased condensed water was carried out by Ramarathinam et al. (1989) to estimate the extent of deterioration in CH3I retention performance (see Table 11). Continuous condensation of moisture on the adsorbent bed initially leads to a gradual decline in retention, which becomes more significant as moisture saturation in the adsorbent medium is approached. Above 34% wt condensed water content, surface wetting begins to block almost all available pores, resulting in CH3I removal performance fluctuating between 80% and 86%. Some studies carried out with impregnated activated carbons have shown that direct spraying of water onto activated carbon beds reduces CH3I removal efficiency from over 90% to around 60%; however, within 1-h, instantaneous efficiency has returned to 78%–79% again. It is also important to note that physical adsorption is accompanied by a decrease in the free energy of the system. This process involves a loss of degrees of freedom, and is therefore always exothermic. Water adsorption on activated carbon is therefore an exothermic process. Table 12 shows the isosteric heat of adsorption of water on activated carbon at different temperatures (AEC, 1968). The researchers noted that the heat of adsorption of water by activated carbon is slightly lower than the heat of liquefaction at identical temperatures, but is still significant. Both mechanisms take place simultaneously in activated carbon, although one is slightly favored due to the small energy difference. The water adsorption rate will be higher at higher temperatures due to the increased diffusion rate within the activated carbon.
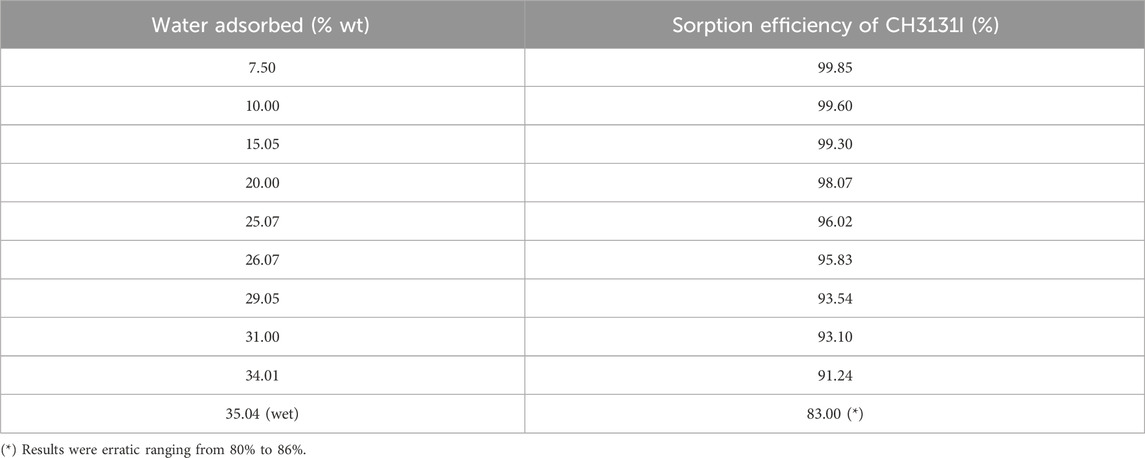
Table 11. The effect of water adsorbed on methyl iodide removal for 50 mm bed depth of impregnated activated carbon operated at 28°C and 0.24 s contact time. Data from reference (Ramarathinam et al., 1989).
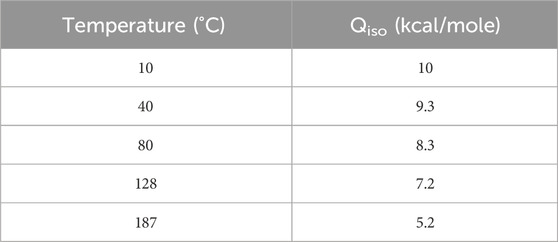
Table 12. Isosteric heat of adsorption (Qiso) of water on activated carbon at various temperatures. Data from reference (AEC, 1968).
Deitz (Deitz, 1985) carried out studies with seven commercial activated carbons were exposed to filtered air flows at three levels of relative humidity (50%, 70% and 90% RH) for a total volume of 600 m3. The purified air flow was continuous at a rate of 100 L/min for 100 h and the residence time in the activated carbon bed was 0.25 s with the linear flow rate was 20.5 cm/sec. The methyl iodide-131 penetrations increased for all seven carbons with increase in the relative humidity of the air flow. The increased penetration from 50% to 70% RH was greater than the increase from 70% to 90% RH.
Data from Shiomi et al. (1982) show a decrease in the μ values of the different activated carbons tested is observed with increasing relative humidity from 30% to 95%. According to Kovach’s data, 30.5 % wt water is reached at 99% relative humidity, and the value of 35.04 % wt is measured at water saturation of the activated carbon according to Ramarathinam et al. (1989). Bulk condensation of moisture on the activated carbon results in the rapid decrease in retention. The reaction mechanisms of TEDA and QD with CH3I under dry and wet conditions have been extensively studied by Ho et al. (2019). QD forms products with higher thermodynamic stability than TEDA. The chemical interactions of TEDA and QD with CH3I are considerably enhanced if TEDA and QD adsorb water molecules onto the nucleophilic nitrogen site.
According to studies carried out by Qi-dong and Sui-yuang (1984) on the retention of radioactive elemental iodine for different relative humidities at different activated carbon bed lengths, their experimental data, presented in Table 13 and plotted in Figure 7, show that decontamination factors decrease (or penetration increase) with increasing relative humidity for 2 activated carbon bed lengths impregnated with 2% TEDA- 2% KI, while the other variables remain constant. However, despite a large drop in decontamination factor, DF values remain above 100 and the sorption efficiency values decrease very slightly [ΔSE (%) = −0.349 and −0.038 for 25- and 50-mm bed lengths respectively] and can be considered to remain relatively constant within the uncertainties over the range of relative humidities studied. It should be noted that the sorption efficiency of radioactive elemental iodine remains very high even at 100% RH.
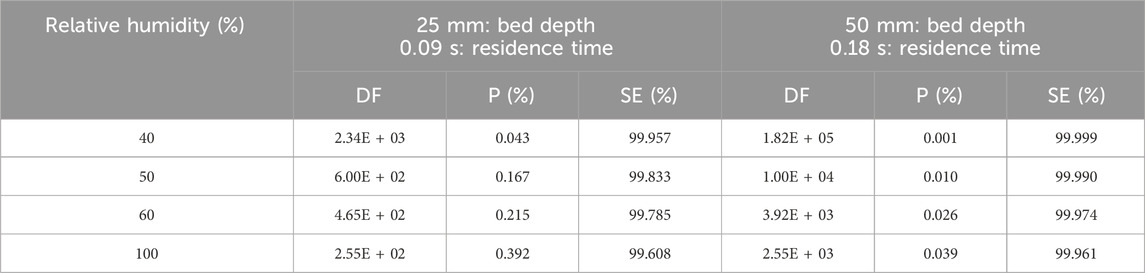
Table 13. Parametric study of relative humidity effects on Decontamination Factor, Penetration and Sorption Efficiency of radioactive elemental iodine I2 at 40°C, 28 cm/sec for activated carbon with 2% TEDA - 2% KI impregnation. Data from reference (Qi-dong and Sui-yuang, 1984).
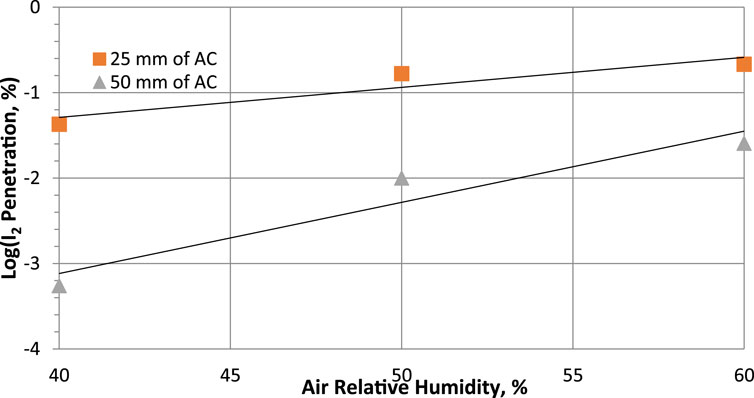
Figure 7. Parametric study of relative humidity effects on Penetration of radioactive elemental iodine I2 at 40°C, 28 cm/sec for activated carbon with 2% TEDA - 2% KI impregnation. Data from Table 13 and reference (Qi-dong and Sui-yuang, 1984).
2.3.4 Inhibition/poisoning, aging and disadvantages of activated carbon adsorbent
Sorbent degradation mechanisms can be classified into 3 main contribution classes: static, dynamic and weathering (Deitz, 1981). Activated carbon aging refers to the possible shelf-life of carbon, stored as it must be in closed containers in a given gaseous environment. Thus, aging would be limited to a static test operation. In the case of “service aged” or dynamic aging, the activated carbon is exposed to the actual air flow of a confined system such as a reactor building, fuel storage building, atmospheric air, etc. The term itself suggests exposure under a set of relevant conditions. Weathered activated carbon means exposure to ambient contaminants in an outside air stream at a given location, or in a set of laboratory conditions, where controlled exposure to a given contaminant can be achieved.
It is well known that the presence in the gas stream of other, and for the most part unspecified, substances may impair the performance of sorption devices. Published data on weathering have generally been presented in the form of CH3I removal efficiencies, measured after selected intervals of exposure time and sometimes in the presence of certain contaminants. Exposure of adsorbents to organic solvent vapors such as xylene, carbon tetrachloride, methylethylketone, methyl alcohol, hexane or hydrocarbon vapor in general will reduce performance (Broadbent, 1985; IAEA, 1973; IAEA, 1989). The major air quality contaminants that degrade nuclear-grade activated carbon are ozone, nitric oxides, sulfur dioxide, carbon dioxide particularly when combined in synergy with water vapor (Deitz, 1981; 1985; Rastunov et al., 2011). A contaminant concentration of 1 ppm becomes a significant concern on removal performance when it is present in the gas flow over the lifetime of the activated carbon filter (Deitz, 1981). Hence, when a KI impregnation on activated carbon is oxidized during weathering, or high temperature loss or degradation of TEDA occurs, the methyl iodide-131 trapping efficiency of the activated carbon can be seriously impaired. A chemical modification of the carbon network and/or iodine-containing impregnants by weathering can also adversely influence the chemical retention capability since the carbon surface chemical composition is modified by the contaminants.
According to studies by Deitz (1985), mixtures of sulfur dioxide + water vapor and ozone + water vapor exceed the influence of water vapor alone. Exposure to mixtures of ozone or sulfur dioxide with water vapor leads to strong adsorption of water vapor in the deeper layers of the activated carbon, but the interaction of ozone or sulfur dioxide is limited to the first layer. This is due, in part, to the large difference in concentration of the two components introduced; ozone about 2.5 ppm, sulfur dioxide 0.5 ppm, and water vapor about 200,000 ppm. However, it does demonstrate that humidified carbons present strong adsorption sites for the two contaminants considered. Carbon monoxide appears indifferent and does not influence the methyl iodide-131 penetration.
At very low gas temperatures in the range ≤0°C, and in the presence of high humidity in activated carbons, a thin layer of water ice can form on the surface of the carbon grains and pores, preventing the activated carbon’s ability to retain gaseous iodine species.
Aging, which is defined as a deterioration in carbon performance due to exposure to humid air, is a slow process that occurs on activated carbon with KI impregnation under normal conditions of <50% RH. At higher relative humidities aging of these activated carbons can be rapid so that use of activated carbon with KI impregnation for continuous operation in high relative humidity air is not consistent with economic carbon filter life. On activated carbon with TEDA impregnation the aging rate is slow at high relative humidities and temperatures below 50°C. Above 50°C the rate of TEDA aging increases rapidly with relative humidity due either to vapor phase removal of the impregnant or its conversion to a less efficient compound (IAEA, 1989). In the case of continuous gas sampling over very long periods at high relative humidity and temperatures in excess of 50°C, it is therefore important to ensure that the activated carbon performs (dynamic aging over time) according to the desired requirements under these conditions. Using the performance factor K (Equation 4), some researchers have observed a K decrease with increasing time, and this decrease is greater the higher the relative humidity (Billinge and Broadbent, 1989; 1988; Evans and Hillary, 1985; Hyder, 1985). Billinge and Broadbant had concluded that the value of K decreases linearly with increasing water partial pressure, favoring a TEDA removal mechanism by physical steam distillation linked to the combined water + TEDA vapor pressure over the activated carbon surface as the most probable cause of sorbent aging. Another possibility is that the chemical reaction, either by hydroxylation of TEDA or by oxidation of TEDA catalyzed by water, forms a less efficient product. Studies of the decline in performance of activated carbons (K decay) through ageing under different conditions of temperature and relative humidity over time had also been studied by Billinge et al. up to 1,000 h. The rate of decrease of the K value (from 14.05 to 11.05–4.56) with ageing time follows the sequence 40°C/90% RH < 60°C/50% RH < 70°C/50% RH < 60°C/90% RH < 70°C/90% RH.
Aging studies carried out by Rastunov et al. (2011) on a few of activated carbons using the sorption capacity index ξ (Equation 5) have shown that storage for less than a year maintains the same sorption capacity index ξ value. However, if the storage time is longer (4 years), the sorption capacity index decreases considerably (Δξ = − 65.04% compared with the initial state). It should be noted that 2 shelf lives (9 and 48 months), which are very distant in time from each other, were evaluated, and it is not possible to know at what time the index ξ begins to deviate significantly from the initial value. A model for evaluating activated carbon weathering using a single parameter, the Effective Weathering Rate (EWR), which takes into account several factors (bed length, linear velocity, etc.), was considered by Parish and Muhlenhaupt (1974). Data from various curves showing the decrease in removal efficiency with ageing time up to 700 days show that activated carbon degradation is higher the shorter the bed length (degradation level for 2.5 > 5.0 > 7.5 > 10 > 12.5 > 15.0 cm bed length) at 25.0 cm/sec. Lee et al. (2020) evaluated the performance of TEDA-impregnated activated carbon under simulated long-term operating conditions (15 months) of a nuclear power plant by analyzing various parameters such as BET specific surface area, TEDA contents, pore volume of TEDA-AC samples, CH3I removal efficiency, etc. More than 99% of CH3I removal performance of TEDA-AC was observed in the TEDA-AC samples during 15 months of long-term operation under the simulated NPP operating conditions including the ppb level of organic and oxide form of poisoning gases.
The shelf life of recommended radioactive iodine adsorbents varies according to the supplier specifications. If the cartridges are stored (static aging) in good conditions, NUCON, for example, recommends a shelf life of 5 years for activated carbon cartridges according to ANSI/ASME N45.2.2, Level B storage conditions (NUCON, 2011). Cartridge shelf life is 10 years from date of manufacture, in compliance with RADECO (RADECO, 2021) and SDEC (SDEC, 2024) storage requirements. MIRION (MIRION, 2024), which markets MGP Instruments cartridges, and APVL (APVL, 2024) recommend a shelf life for their activated carbon cartridges of 2 and 4 years respectively if the manufacturers' storage conditions are respected.
3 Synthesis, data analysis and processing of sorption efficiency data
A number of documents summarizing experimental observations from various studies on iodine gas retention by activated carbon can be found in the literature (Lin, 2022; Deitz, 1985; IAEA, 1989; Huve et al., 2018). Some of the following general conclusions have been announced:
a) The retention performances are enhanced for increasing adsorbent bulk densities. Indeed, the quantity of available adsorption sites will be enhanced for higher packing densities.
b) The activated carbons performance decreases with increasing linear velocity.
c) The increase of bed depth will also account for an enhancement of the retention performances in general, since the residence time is higher for a fixed linear velocity.
d) For the effects of high temperature at low and medium relative humidity:
− Activated carbons impregnated with KI and TEDA are effective up to 180°C and 100°C under dry conditions respectively.
e) For the effects of high temperature at high relative humidity:
− All granular activated carbons have a high capacity for water vapor at high RH, and any resulting loss of CH3I removal efficiency can be reduced by the addition of chemical impregnants.
− Activated carbons impregnated with Kl show a progressive decrease in sorption efficiency with increasing humidity. The presence of the impregnant, however, maintains sufficient efficiency even at 98% RH at room temperature. Other researchers found that activated carbons impregnated with KI performed slightly better at higher temperatures.
−Activated carbons impregnated with TEDA showed a constant initial CH3I sorption efficiency independent of humidity up to 70°C and 90% RH.
− Evaluation of impregnated charcoal, under exposure to high temperature and humidity (90°C, 90% RH) over a period of 8 h showed marginal decrease in the CH3I removal efficiency. The effect was interpreted as due to the adsorption of moisture from the flow of high humidity air.
− A significant decrease in CH3I removal is observed when relative humidity is above 95% and the proportion of condensed water in the activated carbon approaches saturation.
− The effect of high humidity on the retention of I2 species is minimal, and retention efficiency remains very high using impregnated activated carbon. Under the same operating conditions, I2 removal efficiency will be higher than CH3I removal efficiency due to the very strong interaction of elemental iodine with activated carbon.
− The rate of activated carbon ageing increased considerably above 50°C.
The aim of this section is to summarize data on the retention of iodine species by activated carbon cartridges used to monitor gaseous effluent releases from nuclear facilities, in terms of radiological composition. The widely marketed activated carbon cartridges used for monitoring gaseous radioiodines have cylindrical shapes with activated carbon layers of 25 ± 1 mm and 50 ± 1 mm and an outer cartridge diameter and thickness of around 26.3 mm and 57.4 mm respectively. Extensive studies on the removal efficiency of radioactive I2 by activated carbon as a function of flow velocity and thickness have shown that a sampling device using a homogeneous, well-packed activated carbon thickness of 30 mm and an air flow velocity of 86 cm/sec gave a decontamination factor of over 1,000, i.e., a removal efficiency of over 99.9% (Pradel et al., 1962; Billard et al., 1964). A plot of adsorption efficiency versus bed depth ranging from 5 to 50 mm was made by Qi-dong and Sui-yuang (1984) for superficial velocities of 28, 40, and 50 cm/sec shows that adsorption efficiency tends to decrease with increase in superficial velocity at lower bed depth. It has been observed that adsorption efficiencies increase with increasing bed depth and tends to keep constant at a bed depth above 30 mm. A bed depth of 30 mm therefore seems to be the optimized minimum value for an activated carbon cartridge for a wide range of gas velocities, instead of the 25 mm commonly used today.
Several literature studies have demonstrated that the retention capacity parameters of inorganic and organic species of gaseous iodine radioisotopes on activated carbon cartridges are influenced by various factors related to activated carbon characteristics, sampling operating conditions and environmental parameters. Thus, the sampling conditions of gaseous effluent or environmental air are important and will determine the retention efficiency for future radiological analyses in the laboratory of activated carbon cartridges. Knowledge of the sorption efficiency of radioactive iodine species by the cartridge used at the time of sampling is necessary to determine the radioactivity concentration of iodine radioisotopes in the gaseous effluent or environmental air in Bq/m3 from the results of radionuclide measurements in Becquerel (Bq) determined on the cartridge by gamma spectrometry.
Sorption studies on activated carbon are practically all carried out with a single species of iodine gas, whereas gaseous emissions from nuclear facilities often contain a mixture of two or more iodine species. This raises the question of whether the sorption efficiency data for each iodine species is not significantly altered in the case of a mixture of several species, or whether there is sorption competition between the different species for a given sampling operating condition. Studies by Chang have shown that the variation of multi-component sorption breakthrough curves as a function of time is totally different from that of a single-component system (Chang, 1989). A peak is observed only on the CH3I breakthrough curve showing competitive sorption between CH3I and I2 in the first adsorption stages for a two-component system. In the early stages of adsorption, CH3I and I2 are adsorbed on the activated carbon and later, as I2 has the highest affinity, it gradually replaces CH3I. As a result, the concentration profile (flow curve) shows a peak for CH3I adsorption, indicating a higher concentration than the inlet concentration. The author concludes that the kinetics of CH3I adsorption in multicomponent system is faster than that of single-component adsorption.
A compilation of data from experiments published in the literature on the sorption efficiency (SE), penetration (P) or decontamination factor (DF) of I2 and CH3I as a function of temperature and relative humidity was carried out for impregnated and unimpregnated activated carbon (ANDRA, AREVA, CEA, EDF, Marine Nationale, 2011; Choi et al., 2021; Chien et al., 2011; Lin et al., 2022; Qi-dong and Sui-yuang, 1984; Lin, 2022; Ramarathinam et al., 1989; Kitani et al., 1971; Kovach, 1992; Kovach, 1998; Suhariyono et al., 2014; Deitz, 1987; Zhou et al., 2014; Wood, 1985; RADECO, 2021). Additional information (bed depth, linear velocity, residence time, granulometry, standard methods, etc.) on the operating conditions of the analyses was also collected to better assess the quality and grouping of the data. The selected data experiments were carried out at a pressure of 1 atm (1,013.25 hPa). Available iodine retention data that do not contain information on activated carbon bed depth or data to calculate this parameter, or a lack of information on the gaseous iodine species used, have been excluded from this data compilation. All these data were grouped into two categories of activated carbon bed depth (25 and 50 mm) for the two gaseous iodine species I2 and CH3I, giving a total of 4 series of data. For species I2, 20 and 17 experimental sorption efficiency data were initially selected for 25- and 50-mm bed length respectively, while for the CH3I, 55 and 129 data were initially considered for 25- and 50-mm activated carbon bed length respectively. As can be noted, the experimental results available contain more data from studies carried out with CH3I than with I2. There is little information available on the retention efficiency of activated carbon for other iodine species such as HI and HIO. A few studies have been carried out on the removal of HI (Kovach, 1992; 1998) and HIO (Kabat, 1974). For both species, acceptable sorption efficiencies have been observed even at high relative humidity values.
When a type of commercial activated carbon cartridge is selected for sampling, certain parameters related to the cartridge’s manufacturing characteristics are automatically fixed, such as cartridge dimensions, grain size, specific surface area and pore size of the activated carbon, impregnant composition, etc. The sorption efficiency will depend on the temperature, relative humidity, flow rate and duration of air sampling. The values of the last two parameters are often determined during sampling operations according to the analytical performances and detection limit to be achieved. Temperature and relative humidity of air or gaseous effluent are two important parameters that can vary over a wide range depending on weather conditions or conditions of gas discharges or filtrations from the nuclear power plant, and they can significantly modify iodine sorption efficiency, especially in the case of environmental air monitoring. These two parameters (temperature and humidity) were also considered as important variables to assess activated carbon performance over a relatively wide range of changing weather conditions, using the sorption capacity index ξ (ISO, 2019; Rastunov et al., 2011). In practice, the relative humidity often encountered varies from a minimum of 40% to a maximum of 90%, corresponding respectively to optimum and most unfavorable conditions (CEA/IPSN, 1992), excluding conditions of heavy rainfall. Germany’s Nuclear Safety Standards Commission (KTA) has defined the nominal operating ranges and reference values of the physical parameters of influence for monitoring the release of radioactive gases and airborne radioactive particles from nuclear facilities (KTA, 2022a). The following ranges have been specified: Temperature of the measurement medium: 15°C–40°C; Relative humidity of the measurement medium: 10%–95%, non-dewing; Pressure of the measurement medium: 700 hPa to 1,100 hPa. In the event of an accident at the nuclear facility, different evaluation ranges for certain physical parameters are recommended, such as the relative humidity range (KTA, 2020b).
Experimental data systematically containing 3 measured variables [temperature (T °C), relative humidity (RH %), sorption efficiency (SE %)] from each of the 4 data sets were mathematically processed using a linear and non-linear surface fitting software (TableCurve 3D marketed by Grafiti LLC) with 3D graphic visualization to assess areas of large variation and small change in sorption efficiency as a function of temperature and relative humidity.
An initial sorting of the data was carried out by removing from the sorption efficiency modelling process DF values lower than one-10th of those recommended (e.g., DF > 100 under reference conditions) for nuclear-grade activated carbons, or data far from the limits of the confidence interval [lower confidence limit for 95% probability; upper confidence limit for 95% probability]. Discarded data often corresponded to SE values (%) of CH3I species that deviated significantly from the performance generally expected of nuclear-grade activated carbons for given values of temperature or relative humidity. Data retained at the end of the treatment process by mathematical modeling for each series are presented in Supplementary Tables S1–S4, and 3D plots of the fitting results are shown in Figure 8.
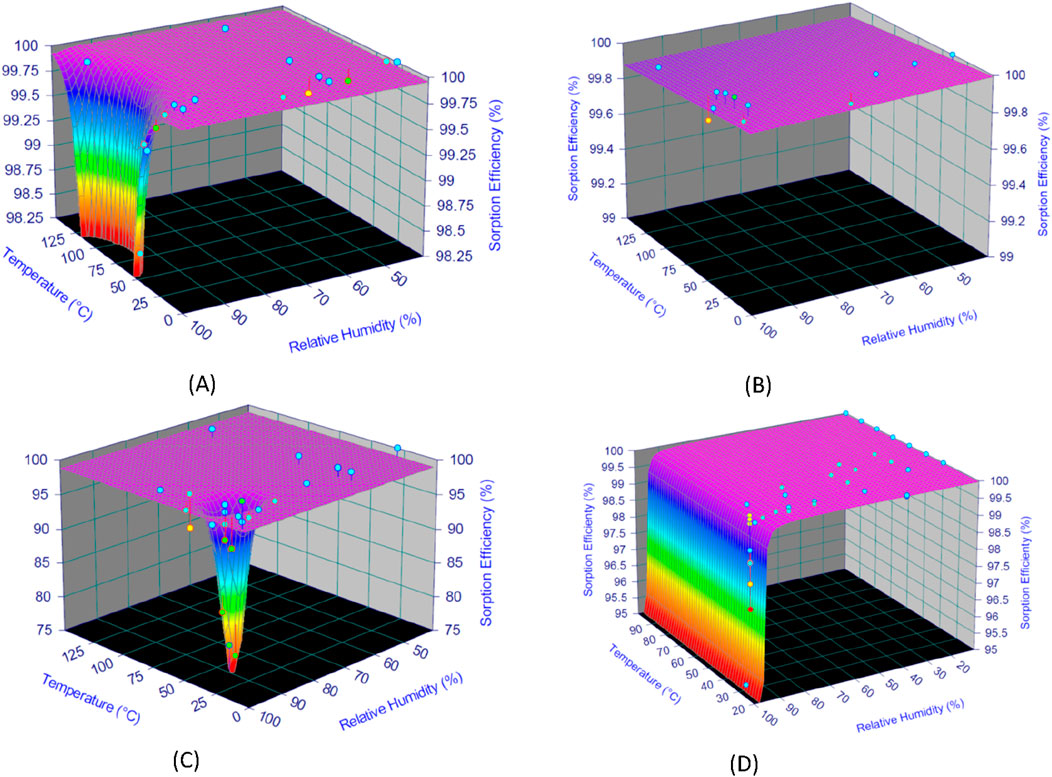
Figure 8. 3D fitting plot of selected SE (%) data as a function of temperature and humidity for I2 [(A, B)] and CH3I [(C, D)] for 25 mm and 50 mm activated carbon bed lengths.
Tables 14, 15 contain the determined values of sorption efficiencies and their associated uncertainties at k = 1 for I2 and CH3I species for activated carbon bed lengths of 25 mm and 50 mm for the temperature, relative humidity and linear velocity ranges. Sorption efficiency uncertainty is calculated from confidence interval data derived from linear and non-linear equations fitting of experimental data [T °C, RH (%), SE (%)] according to ISO 8466 (ISO, 1990; ISO, 2001). The range of parameter variations reported in these tables corresponds to the extremes of the experimental data retained at the end of the mathematical fitting process, or to the routine monitoring range.
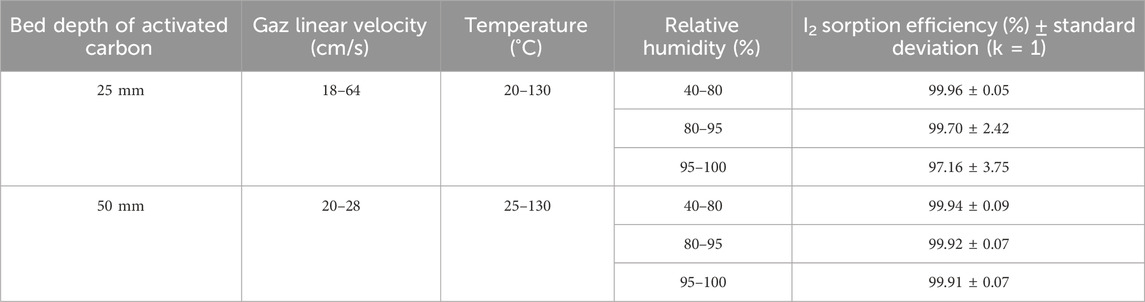
Table 14. I2 sorption efficiency of activated carbon for different temperature and relative humidity ranges.
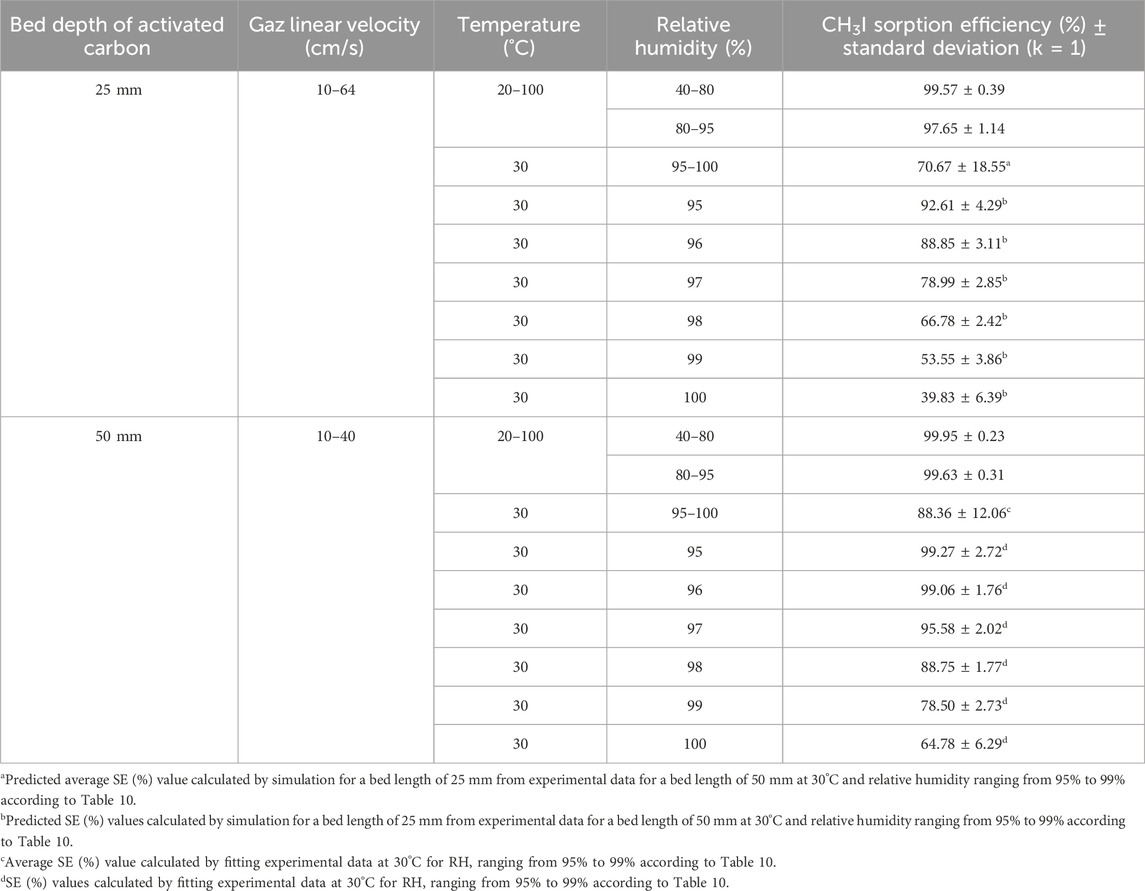
Table 15. CH3I sorption efficiency of activated carbon for different temperature and relative humidity ranges.
The sorption efficiency values determined should be used as long as temperature, relative humidity and gas velocity remain within the defined parameter ranges throughout the sampling period. SE (%) values remain relatively constant for a gas sampling duration of 1 week at room temperature and for RH ≤90%. For environmental monitoring, it is also important to ensure that the adsorbed water content does not saturate the activated carbon (around 35% wt, see Table 11) or approach saturation, and in the absence of significant concentrations of contaminants (poisons/inhibitors) that could alter activated carbon removal performance. Sampling time must be adapted to avoid saturation of the activated carbon by adsorbed water, particularly when air sampling in rainy conditions or in the presence of airborne fog. The difference between the mass of the cartridge before and after sampling, divided by the mass of activated carbon in the cartridge, will provide information on the percentage of water condensed in the sorbent. The installation of equipment for measuring these parameters and the recording of experimental data should enable compliance with recommended parameter ranges to be verified. Although Tables 14, 15 show high values of T ≥ 100°C for a selected range of gas flow temperatures, it is important to follow the activated carbon supplier’s instructions for use. According to several activated carbon manufacturers, activated carbon can only be validly used at temperatures not exceeding 80°C, although its flash point is always above 200°C. Caution should always be exercised when extrapolating sorption efficiency values to conditions other than those of the parameters under which the tests were carried out. According to the findings of Ramarathinam et al. (1989), the combined effect of high temperature and high humidity showed that there is a net decrease in overall CH3I removal performance indicating that with increasing humidity, the rate of fall in efficiency is much higher than that of temperature, excluding condensation in the adsorbent medium. The reduced performance of activated charcoal is due to loading of the charcoal surface with water and the reduced diffusion velocity in the macropores. This significant drop in removal is not observed for species I2 even at very high humidity (see Table 13), based on data from Qi-dong and Sui-yuang (1984) showing a stronger interaction of this chemical species with impregnated activated carbon than with CH3I, which confirms the conclusions of Chang (1989) on simultaneous sorption studies of I2 and CH3I. A significant drop in retention can also occur when a thin layer of water ice forms around activated carbon particles for gas stream temperatures ≤0°C. For CH3I removal at RH > 95% using cartridges with 50 mm activated carbon bed length, the SE (%) values given in Table 10 can be used for CH3I. Extrapolation of these data at RH = 100% should give the value of SE = 64.78% as long as the activated carbon is not wet. The SE (%) experimental data for CH3I removal by cartridges with 25 mm activated carbon bed length for RH ranging from 95% to 100% was not available in the literature. For the determination of iodine removal efficiency, a note from the German Nuclear Safety Standards Commission (KTA) specifies that the organic form of the methyl iodide compound is generally taken as the reference (KTA, 2022a). Due to the wide variation in CH3I sorption efficiency in the near-saturation range of relative humidity (RH > 95%) in air, the absence of sufficient experimental data for a wide temperature range and different activated carbon lengths makes it difficult to carry out environmental monitoring of radioactive methyl iodides in air under heavy rainfall conditions. Given the importance of having these data available, SE (%) values for CH3I were calculated by simulation for a bed length of 25 mm from experimental sorption efficiency data determined by Kovach for an activated carbon bed length of 50 mm at 30°C and for relative humidity values ranging from 95% to 99% shown in Table 10. The validation test of the method for calculating predicted SE values had previously been validated on the experimental data in Table 8, and the relative deviations observed with experimental SE values for 25 mm bed length were <1.5%. These theoretical values of CH3I sorption efficiency calculated for RH values ranging from 95% to 100%, which are shown in Table 15, should be compared with future results from experiments carried out at very high relative humidity up to RH = 100%.
In existing nuclear power plants, generally speaking, isokinetic sampling is essential in order to obtain a representative sample and two cartridges in series are used for each sampling, in order to ensure maximum retention of the iodine in the gaseous effluents. The use of two cartridges in series with a 25 mm activated carbon bed length ensures over 99% iodine retention. In order to prevent any distortion of the measurement of gaseous radioiodine activities, a high-efficiency particulate air filter of at least Filter Class E12 in accordance with EN 1822–1 (ECS, 2019) is usually installed inline before the cartridges to retain any particulate halogens or aerosols, and the direction of the gaseous flow is recorded on the cartridges, as retention will be higher on the first cartridge than on the second one. The distribution of radioactive iodine species retained in the activated carbon cartridge is not homogeneous along the length of the bed. When large volumes of air are sampled, the activated carbon at the front of the cartridge becomes saturated within a relatively short time, and radioactive iodine is thus found in deeper parts of the activated carbon cartridge. Depending on the length of the activated carbon bed, a fraction of the radionuclides may even breakthrough and exit the cartridge. The variation curve for iodine radioactivity within the thickness of the adsorbent is an exponential decrease in the direction of gas flow (Kravchik et al., 2008; ISO, 2019; Shiomi et al., 1982). The curve decreases more rapidly at lower gas velocities, leading to higher residence times.
The sampling time must be adapted to the level of radioactivity in the medium being monitored, the characteristics of the activated carbon cartridge and the detection limit to be achieved. In France, the sampling time varies according to the control objectives, which can be divided into 3 categories: 30 min for radioactive air plume monitoring; 30 min to a few hours for spot sample measurement; and from 1 day to 1 month for measurement of the sample from integrated sampling intended for monitoring around nuclear facilities (Pradel et al., 1962). In general, and taking into account the short half-lives of some iodine radioisotopes such as 131I (8.0233 days), a continuous sampling time of 3–10 days is often applied for environmental monitoring around nuclear facilities or for monitoring radioactive gaseous releases. Air sampling rates with activated carbon cartridges range from 1.2 to 6 m3/h. With available data on the variation of iodine radioisotope DF or SE (%) as a function of linear velocity or residence time (τ) for a given length of activated carbon and fixed values of temperature and relative humidity, it should be possible to predict the gas removal flow required to achieve the desired performance. Figure 9 shows that to ensure DF >100 or >1,000 (SE > 99% or >99.9%), τ >0.24 s or >0.39 s respectively is required for an activated carbon bed length of 25 mm. For a bed length of 50 mm, τ >0.18 or >0.41 s respectively under the same conditions. For a longer activated carbon bed, higher gas flow rates can be used because low residence times ensure high DF values.
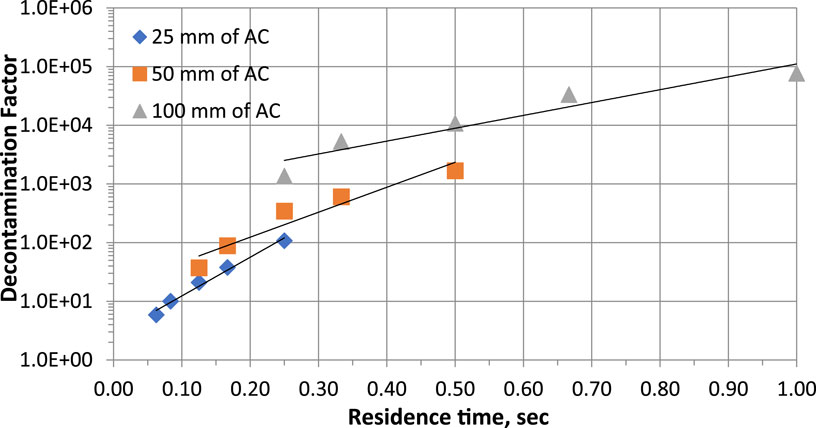
Figure 9. Parametric study of residence time effects on CH3131I Decontamination factor at 30°C, 95% RH for commercial NUSORB KITEG II adsorbent. Data from Table 4 and references (Kovach, 1992; Kovach, 1998).
4 Conclusion
The sorption efficiency of gaseous iodine species by activated carbon depends on several parameters, some of which are fixed by the operating conditions during sampling, other parameters can vary significantly depending on the weather conditions, and efficiency may be affected by the presence of retention inhibitors in the air or gaseous effluents.
Linear velocity and residence time are important parameters which can affect the removal of radioactive iodine species from air or off-gas. Their values should be chosen to optimize retention efficiency, taking into account the objectives to be achieved (detection limit, volume of air to be filtered, desired level of removal, etc.).
The removal efficiency of the activated carbon increases moderately with increasing temperature of the air stream at low and medium relative humidity. This is due to the increased kinetics of almost all species reaction steps at gas-solid interfaces. At constant temperature the removal efficiency of activated carbon decreases with increasing relative humidity. Sorption efficiency drops rapidly as we approach moisture saturation in the air. The combined effect of high temperature and high humidity leads to a net decrease in the overall performance of the activated carbon, mainly with regard to the CH3I species. The level of decrease in efficiency was much higher with increasing humidity than with increasing temperature. The effect of high humidity on the retention of I2 species is minimal due to the very strong interaction of this compound with activated carbon. The reduced performance of activated carbon is due to the water load on the adsorbent surface and the reduced diffusion rate in the macro-pores. The water condensation in the adsorbent is an important factor in the performance of impregnated activated carbon. The CH3I removal efficiency decreases steadily as the moisture content of the activated carbon increases, up to 34% wt. Above 34% wt, surface wetting begins to block almost all available pores, resulting in a rapid and random drop in performance. This can also occur when condensed water in the activated carbon forms a thin layer of ice around the grains for gas flow temperatures ≤0°C. In order to prevent water condensation or water ice formation in the air sampling device, the supply air flow must be heated.
Non-linear surface fitting of experimental data on the sorption efficiencies of I2 and CH3I by nuclear-grade activated carbon cartridges for different temperature and relative humidity ranges shows that there are ranges of variation of both parameters where the removal capacity remains relatively constant. Sorption efficiency values have been determined for activated carbon bed lengths of 25 and 50 mm for ranges of some important factors that can have an impact on iodine retention. Under the same sampling conditions, the retention of I2 is higher than that of CH3I. The sorption efficiency values determined should be used with caution, ensuring that temperature, relative humidity and gas velocity remain within the defined parameter range throughout the sampling period.
Author contributions
BM: Conceptualization, Formal Analysis, Investigation, Methodology, Validation, Visualization, Writing – original draft, Writing – review and editing. AA: Methodology, Validation, Writing – review and editing.
Funding
The author(s) declare that no financial support was received for the research and/or publication of this article.
Acknowledgments
The authors would like to thank MIRION Technologies, APVL Ingénierie and SDEC France for providing some technical information on the activated carbon cartridges marketed by their companies. We would like to express our gratitude to Mr. A. Ratsirahonana and Mr. S. Brun of CEA Saclay for his help in participating in discussions on the results of the data synthesis and on the general conclusions resulting from data processing.
Conflict of interest
Author BM was employed by Mokili Consulting.
The remaining author declares that the research was conducted in the absence of any commercial or financial relationships that could be construed as a potential conflict of interest.
Generative AI statement
The author(s) declare that no Generative AI was used in the creation of this manuscript.
Publisher’s note
All claims expressed in this article are solely those of the authors and do not necessarily represent those of their affiliated organizations, or those of the publisher, the editors and the reviewers. Any product that may be evaluated in this article, or claim that may be made by its manufacturer, is not guaranteed or endorsed by the publisher.
Supplementary material
The Supplementary Material for this article can be found online at: https://www.frontiersin.org/articles/10.3389/fnuen.2025.1553446/full#supplementary-material
References
AEC (1968). Sevent supplement to preliminary safety analysis report to Indian point nuclear generating unit no 3 PSAR. Consolidated EDISON Company of New York, Inc. Docket N° 50-286 - Exhibit B-7, December 9, 1968. United States: U.S. Atomic Energy Commission.
AEC (1973). Gas-phase adsorbents for trapping radioactive iodine and iodine compounds, RDT-M-16-1T, October 1973, United States: U.S. Atomic Energy Commission.
AFNOR (1984). NF M62-206 (1984) - Énergie Nucléaire Installations de Ventilation Nucléaire: Méthode de contrôle du coefficient d'épuration des pièges à iode. Paris, France: Association Française de Normalisation.
AFNOR (2005). NF M60-759 (Avril 2005): Mesure de la radioactivité dans l'environnement - Air - Détermination de l'activité volumique des iodes atmosphériques. Paris, France: Association Française de Normalisation.
Alcañiz-Monge, J., Linares-Solano, A., and Rand, B. (2001). Water adsorption on activated carbons: study of water adsorption in micro- and mesopores. J. Phys. Chem. B 105, 7998–8006. doi:10.1021/jp010674b
ANDRA, AREVA, CEA, EDF, Marine Nationale (2011). Les prélèvements d’air - Guide méthodologique inter-exploitants relatif à la qualité des prélèvements pour les mesures de radioactivité dans l’environnement, ANDRA, AREVA, CEA, EDF, Marine Nationale, Octobre 2011, pp. 23–26. CALATHEA Ed.
ANSI/ASME (1976). N509 - nuclear power plant air cleaning units and components. American National Standards Institute/American Society of Mechanical Engineers.
ANSI/ASME (2002). N509 - nuclear power plant air cleaning units and components. American National Standards Institute/American Society of Mechanical Engineers.
APVL (2024). Activated carbon cartridge technical data supplied by APVL Ingénierie, 37540 Saint-Cyr-sur-Loire, France. Available online at: https://www.apvl.com/(Accessed October 18, 2024).
AREVA/EDF (2012). Monitoring of liquid and gaseous discharges: prospective arrangements for the UK EPR. Paris, France: AREVA EDF Rep. UKEPR-0007-001 - Issue 03, 26–28. Available online at: https://www.edfenergy.com/sites/default/files/monitoring_of_liquid_and_gaseous_discharges.pdf (Accessed October 18, 2024).
ASTM (2022). ASTM-D3803-91 (reapproved 2022): standard test method for nuclear-grade activated carbon. West Conshohocken, PA: ASTM International.
Billard, F., Chevalier, G., Gaillard, P., and Pradel, J. (1964). Quelques méthodes de détection de contaminations atmosphériques par l’iode 131. Note CEA no 480. France: CEA Saclay.
Billinge, B. H. M., and Broadbent, D. (1988). “The effects of temperature and humidity on the ageing of TEDA impregnated charcoals,” in Proceedings of the 20th DOE/NRC nuclear air cleaning conference, Boston, Massachusetts, august 22-25 1988. Editor W. Melvin (Boston, United States: First, The Harvard Air Cleaning Laboratory), 572–587.
Billinge, B. H. M., and Broadbent, D. (1989). “The effects of temperature and humidity on the ageing of TEDA impregnated charcoals. In: retention of iodine and other Airborne Radionuclides,” in Nuclear Facilities during Abnormal and Accident conditions, TECDOC-521 (Vienna, Austria: International Atomic Energy Agency), 151–167.
Broadbent, D. (1985). “The ageing and poisoning of charcoal used in nuclear plant air cleaning systems,” in Gaseous effluent treatment in nuclear installations, Proceeding of European Conference. Editors G. Fraser, and F. Luykx (Luxembourg: Graham & Trotinan, Commission of the European Communities), 463–474. 14-18 October 1985.
CEA/IPSN (1992). Cartouche pour prélèvement d’iode gazeux « Fernez », IPSN-DPEI-STESR-CTHEN CHN-PV.02 indice B du 08-03-1992. Fontenay-aux-Roses, France: CEA - IPSN.
Chang, S. H. (1989). “Experimental, analytical and numerical study on the removal efficiency of a charcoal bed for methyl-iodide under humid conditions. In: retention of iodine and other Airborne Radionuclides,” in Nuclear Facilities during Abnormal and Accident conditions, TECDOC-521 (Vienna, Austria: International Atomic Energy Agency), 143–148.
Chebbi, M., Marcillaud, B., Roynette, A., Monsanglant-Louvet, C., Dougniaux, G., Nerisson, P., et al. (2024). Optimisation des conditions de prélèvement des iodes dans l’atmosphère en vue d’une meilleure représentativité des différentes formes physico-chimiques. Paris: Séminaire sur L’IODE dans tous ses état, Journées Techniques SFRP. 26-27 mars 2024.
Chebbi, M., Monsanglant-Louvet, C., Parent, P., Gerente, C., Le Coq, L., and Mokili, B. M. (2022). Sorption properties of activated carbons for the capture of methyl iodide in the context of nuclear industry. Carbon Trends 7, 100164. doi:10.1016/j.cartre.2022.100164
Chien, C.-C., Huang, Y.-P., Wang, W.-C., Chao, J.-H., and Wei, Y.-Y. (2011). Efficiency of moso bamboo charcoal and activated carbon for adsorbing radioactive iodine. Clean- Soil, Air, Water 39 (2), 103–108. doi:10.1002/clen.201000012
Choi, B.-S., Kim, S.-B., Moon, J., and Seo, B.-K. (2021). Evaluation of decontamination factor of radioactive methyl iodide on activated carbons at high humid conditions. Nucl. Eng. Technol. 53, 1519–1523. doi:10.1016/j.net.2020.10.020
Collinson, B., and Taylor, L. R. (1988). “Trapping performance of 1.5% KI 207B charcoal for methyl iodide in CO2 at high temperature and pressure,” in 20th DOE/NRC nuclear air cleaning conference, Boston, Massachusetts, august 22-25 1988. Editor W. Melvin (Boston, United States: First, The Harvard Air Cleaning Laboratory), 537–559.
Deitz, V. R. (1981). Effect of weathering on impregnated charcoal performance. Washington, DC, United States: Naval Research Laboratory.
Deitz, V. R. (1985). Charcoal performance under accident conditions in light-water reactors, final report, NUREG CR-3990, NRL memo rpt 5528, march 1985. Naval research laboratory, Washington, DC, United States: Report prepared for U.S. Nuclear Regulatory Commission Washington, D.C. 20555.
Deitz, V. R. (1987). Interaction of radioactive iodine gaseous species with nuclear-grade activated carbons. Carbon 25 (1), 31–38. doi:10.1016/0008-6223(87)90037-6
Deitz, V. R., and Blachly, C. H. (1974). “Dependence of gas penetration of charcoal beds on residence time and linear velocity,” in Proceedings of the 14th ERDA air cleaning conference, sun valley, Idaho, 2-4 august, 1974. Editor W. Melvin (Boston, United States: First, The Harvard Air Cleaning Laboratory), 233–248.
DOE (2015). DOE handbook, environment radiological effluent Monitoring and environmental surveillance, DOE-HDBK-1216-2015. Washington, D.C: U.S. Department of Energy.20585
ECS (2019). EN 1822-1 (April 2019): high efficiency air filters (EPA, HEPA and ULPA) - Part 1 -Classification, performance testing, marketing. Brussels, Belgium: European Committee for Standardization ECS.
Evans, A. G. (1976a). “New charcoal impregnants for trapping methyl iodide - Part II, applications to a variety of base charcoals,” in Proceedings of the 14th ERDA air cleaning conference, sun valley, Idaho, august 2-4, 1976. Editor W. Melvin (Boston, United States: First, The Harvard Air Cleaning Laboratory), 310–321.
Evans, A. G. (1976b). “Effect of service aging on iodine retention of activated charcoals,” in Proceedings of the 14th ERDA air cleaning conference, sun valley, Idaho, august 2-4, 1976. Editor W. Melvin (Boston, United States: First, The Harvard Air Cleaning Laboratory), 251–264.
Evans, M. G., and Hillary, J. J. (1985). “Recent studies on the performance of impregnated charcoals for trapping methyl iodide, after exposure to moist air,” in Gaseous effluent treatment in nuclear installations, Proceeding of European Conference. Editors G. Fraser, and F. Luykx (Luxembourg: Graham & Trotinan, Commission of the European Communities), 475–499. 14-18 October 1985.
Friedrich, V. (1988). “Kinetic studies of the retention of radioactive gazes by activated carbon adsorbers,” in Proceedings of the 20th DOE/NRC nuclear air cleaning conference, Boston, Massachusetts, august 22-25 1988. Editor W. Melvin (Boston, United States: First, The Harvard Air Cleaning Laboratory), 512–521.
González-García, C. M., González, J. F., and Román, S. (2011). Removal efficiency of radioactive methyl iodide on TEDA-impregnated activated carbons. Fuel Process. Technol. 92, 247–252. doi:10.1016/j.fuproc.2010.04.014
Ho, K., Chuna, H., Leeb, H. C., Lee, Y., Lee, S., Junga, H., et al. (2019). Design of highly efficient adsorbents for removal of gaseous methyl iodide using tertiary amine-impregnated activated carbon: integrated experimental and first-principles approach. Chem. Eng. J. 373, 1003–1011. doi:10.1016/j.cej.2019.05.115
Holladay, D. W. (1979). A literature survey: methods for the removal of lodine species from off-gases and liquid waste streams of nuclear power and nuclear fuel reprocessing plants, with emphasis on solid sorbents. United States: Oak Ridge National Laboratory. ORNL-TM-6350, January 1979.
Huve, J., Ryzhikov, A., Nouali, H., Lalia, V., Augé, G., and Daou, T. J. (2018). Porous sorbents for the capture of radioactive iodine compounds: a review. RSC Adv. 8, 29248–29273. doi:10.1039/c8ra04775h
Hyder, M. L. (1985). “Mechanisms of carbon aging and their effects on the retention of organic iodides,” in Gaseous effluent treatment in nuclear installations, Proceeding of European Conference. Editors G. Fraser, and F. Luykx (Luxembourg: Graham & Trotinan, Commission of the European Communities), 451–462. 14-18 October 1985.
IAEA (1973). “Control of iodine in the nuclear industry,”. IAEA Technical Reports Series no 148, 1973. Vienna, Austria: International Atomic Energy Agency, 3–26.
IAEA (1987). “Design of off-gas and air cleaning system at nuclear power plant,”. IAEA Technical Reports Series No 274, 1987. Vienna, Austria: International Atomic Energy Agency, 89–113.
IAEA (1989). “Retention of iodine and other airborne radionuclides,” in Nuclear facilities during abnormal and accident conditions, TECDOC-521, 1989 (Vienna, Austria: International Atomic Energy Agency), 18–21.
IEC (2002). IEC 60761 (in five parts) (2002): equipment for continuous monitoring of radioactivity in gaseous effluents. Geneva, Switzerland: International Electrotechnical Commission.
ISO (1990). ISO 8466-1 (1990): water quality - calibration and evaluation of analytical methods and estimation of performance characteristics - Part 1: statistical evaluation of the linear calibration function. Geneva, Switzerland: International Organization for Standardization.
ISO (2001). ISO 8466-2 (2001): water quality - calibration and evaluation of analytical methods and estimation of performance characteristics - Part 2: calibration strategy for non-linear second-order calibration functions. Geneva, Switzerland: International Organization for Standardization.
ISO (2019). ISO 18417 (2019) - lodine charcoal sorbents for nuclear facilities - method for defining sorption capacity. Geneva, Switzerland: International Organization for Standardization.
Jacquemain, D., Bentaïb, A., Bonneville, H., Cénérino, G., Clément, B., Corenwinder, F., et al. (2015). Nuclear power reactor core melt accidents - current State of knowledge, IRSN book. France: EDP Sciences, 70–71.
Ji, Y. (2024). The activation study of activated charcoal for radioactive off-gas treatment systems in NPPs. Results Eng. 21, 101831. doi:10.1016/j.rineng.2024.101831
Kabat, M. J. (1974). “Testing and evaluation of absorbers for gaseous penetrative forms of radioiodine,” in Proceedings of the 13th AEC air cleaning conference, San Francisco, California, 12-15 august 1974. Editor W. Melvin (Boston, United States: First, The Harvard Air Cleaning Laboratory), 765–800.
Kabat, M. J. (1988). “Current problems in the assessment of the effectiveness of radioiodine release control,” in 20th DOE/NRC nuclear air cleaning conference, Boston, Massachusetts, august 22-25 1988. Editor W. Melvin (Boston, United States: First, The Harvard Air Cleaning Laboratory), 524–533.
KfK (1979). Kernforschungszentrum Karlsruhe, Ergebnisbericht über Forschungs - und Entwicklungsarbeiten (1978) des Laboratoriums für Aerosolphysik und Filtertechnik, KfK 2743, Februar 1979 Karlsruhe Nuclear Research Center, Report on the results of research and development studies (1978) of the Laboratory for Aerosol Physics and Filter Technology, KfK 2743, February 1979
Kitani, S., Noro, T., and Kohara, T. (1971). Removal of methyl iodide by impregnated charcoals from flowing air under humid condition. J. Nucl. Sci. Technol. 9 (4), 197–202. doi:10.1080/18811248.1972.9734831
Kovach, J. L. (1992). “Parametric studies of radioactive iodide, hydrogen iodide and methyl iodide removal,” in Proceedings of the 22nd DOE-NRC nuclear air cleaning and treatment conference. Editor W. Melvin (Denver, Colorado: First, The Harvard Air Cleaning Laboratory), 646–660. August 24-27, 1992.
Kovach, J. L. (1998). “History of radioiodine control,” in Proceedings of the 25th DOE-NRC nuclear air cleaning and treatment conference. Editor W. Melvin (Minneapolis, Minnesota: First, The Harvard Air Cleaning Laboratory), 304–319. August 3 - 6, 1998.
Kravchik, T., Levinson, S., Oved, S., Tsroya, S., Pelled, O., Haim, M., et al. (2008). Determination of radioiodine activity in charcoal cassettes. Appl. Radiat. Isotopes 66 (issues 6-7), 972–975. doi:10.1016/j.apradiso.2008.02.043
KTA (2022a). KTA 1503.1 (2022-11): monitoring the discharge of radioactive gases and airborne radioactive particulates; Part 1: monitoring the discharge of radioactive matter with the stack exhaust air during specified normal operation. Nucl. Saf. Stand. Comm. (KTA) - Geschaeftsstelle, 38226 - Salzgitter, Ger. Available online at: https://www.kta-gs.de/e/standards/1500/1503_2_engl_2022_11.pdf (Accessed October 18, 2024).
KTA (2020b). KTA 1503.1 (2022-11): monitoring the discharge of radioactive gases and airborne radioactive particulates; Part 2: monitoring the discharge of radioactive matter with the vent stack exhaust air during design-basis accidents. Nucl. Saf. Stand. Comm. (KTA) - Geschaeftsstelle, 38226 - Salzgitter, Ger. Available online at: https://www.kta-gs.de/e/standards/1500/1503_2_engl_2022_11.pdf (Accessed October 18, 2024).
Lee, H. C., Lee, D. Y., Kim, H. S., and Kim, C. R. (2020). Performance evaluation of TEDA impregnated activated carbon under long term operation simulated NPP operating condition. Nucl. Eng. Technol. 52, 2652–2659. doi:10.1016/j.net.2020.04.020
Lin, H. (2022). “Evaluation of the contribution of the isotopic exchange mechanism to the retention of radioactive iodine,”. France: Sorbonne Université. Doctoral thesis.
Lin, H., Chebbi, M., Monsanglant-Louvet, C., Marcillaud, B., Roynette, A., Doizi, D., et al. (2022). KI and TEDA influences towards the retention of radiotoxic CH3I by activated carbons. J. Hazard. Mater. 431, 128548. doi:10.1016/j.jhazmat.2022.128548
MIRION (2024). Absorbent cartridge 12217, Activated carbon cartridge technical data supplied by Mirion Technologies. France: Inc. 18110 Fussy. Available online at: https://www.mirion.com/(Accessed October 18, 2024).
Montgomery, D. M. (1990). Calibrating germanium detectors for assaying radioiodine in charcoal cartridges. Radioact. & Radiochem. Count. Room 1 (2), 47–51. Available online at: https://www.ezag.com/fileadmin/ezag/user-uploads/isotopes/pdf/Calibrating_Germanium_Detectors_for%20Assaying_Radioiodine.pdf (Accessed October 18, 2024).
Nolan, L., Murphy, N. M., Burbidge, C. I., Brien, D. O., Leon Vintro, L., Hanley, O., et al. (2021). Gamma spectrometry analysis of radioiodine in charcoal from high volume aerosol samples. Appl. Radiat. Isotopes 178, 109984. doi:10.1016/j.apradiso.2021.109984
NUCON (2011). Radioiodine adsorbents, NUCON bulletin 11B31-april 2006 (revised january 2011). Columbus, OH: NUCON International, Inc. Available online at: http://nucon-int.com/wp-content/uploads/2020/04/NUCON-Radioiodine-Adsorbents-Bulletin-11B31.pdf (Accessed October 18, 2024).
Parish, H. C., and Muhlenhaupt, R. C. (1974). “A method for correlating weathering data on adsorbents used for removal of CH3I,” in Proceedings of the 14th ERDA air cleaning conference, sun valley, Idaho, 2-4 august, 1974. Editor W. Melvin (Boston, United States: First, The Harvard Air Cleaning Laboratory), 266–285.
Park, G.-I., Kim, I.-T., Lee, J. K., Ryu, S. K., and Kim, J. H. (2001). Effect of temperature on the adsorption and desorption characteristics of methyl Iodide over TEDA-impregnated activated carbon, Carbon letters (Korean Carbon Society). 2 (1), 9–14. Available online at: https://scispace.com/papers/effect-of-temperature-on-the-adsorption-and-desorption-1qnegedllx (Accessed October 18, 2024).
Pradel, J., Chevalier, G., and Billard, F. (1962). Etudes de l'arrêt de l'iode par les charbons actifs, Rapport CEA n°2237. France: CEA Saclay.
Qi-dong, L., and Sui-yuang, H. (1984). “A study of adsorption properties of impregnated charcoal for airborne iodine and methyl iodide,” in Proceedings of the 18th DOE nuclear airborne waste management and air cleaning, 12-16 august, 1984. Editor W. Melvin (Boston, United States: First, The Harvard Air Cleaning Laboratory), 78–92.
RADECO (2021). CP-100 and BG-300 radioiodine samplers, RADECO Inc. Plainfield, CT 06374, USA. Available online at: https://radecoinc.com/products/cp-100-and-bg-300-radioiodine-samplers/[Accessed October 18, 2024].
Ramarathinam, K., Kumar, S., Gandhi, K. G., and Ramachandran, S. (1989). “Evaluation of high efficiency particulate air (HEPA) and iodine filters under high temperature, humidity and radiation. In: retention of iodine and other Airborne Radionuclides,” in Nuclear facilities during abnormal and accident conditions, TECDOC-521 (Vienna, Austria: International Atomic Energy Agency), 113–142.
Rastunov, L. N., Magomedbekov, E. P., Obruchikov, A. V., and Lomazova, L. A. (2010). Sorption power - a control test for impregnated carbons for nuclear power plants. At. Energy 109 no 1, 1–6. doi:10.1007/s10512-010-9315-y
Rastunov, L. N., Magomedbekov, E. P., Obruchikov, A. V., and Lomazova, L. A. (2011). Evaluation of the sorbent layer thickness in iodine filters. At. Energy 110 no 1, 68–72. doi:10.1007/s10512-011-9392-6
SAIC (1989). Science applications international corporation radioiodine and particle transmission through sampling lines for SGTS effluents at fermi 2. Rockville, Maryland United States: Utility Services Department/SAIC.
Scarpellino, C. D., and Sill, C. W. (1987). Final technical evaluation report for the nuclear regulatory commission, Idaho national engineering, ECG-CS-7653, april 1987. Idaho National Engineering Laboratory. Idaho, United States: EG&G Idaho Inc. Idaho Falls, Idaho 83415, 10–12.
SDEC (2024). Activated carbon cartridge technical data supplied by SDEC France, 37310 Reignac-sur-Indre, France. Available online at: https://fr.sdec-france.com/produit/as-3000/(Accessed October 18, 2024).
Shiomi, H., Yuasa, Y., Tani, A., Ohki, M., and Nakagawa, T. (1982). “A Parametric study on removal efficiency of impregnated activated charcoal and silver zeolite for radioactive methyl iodide,” in Proceedings of the 17th DOE nuclear air cleaning conference, Denver, Colorado, 2-5 august 1982. Editor W. Melvin (Boston, United States: First, The Harvard Air Cleaning Laboratory), 199–221. Conf. 820833.
Suhariyono, G., Kusnoputranto, H., and Kardono, S. (2014). “Absorption life time of iodine-131 in charcoal filter on the airborne measurement in stack before released into the environment,” in Proceedings of the 3rd applied science for technology innovation, ASTECHNOVA 2014, international energy conference (Yogyakarta, Indonesia), 115–124. 13-14 August 2014.
Velasco, L. F., Snoeck, D., Mignon, A., Misseeuw, L., Ania, C. O., Van Vlierberghe, S., et al. (2016). Role of the surface chemistry of the adsorbent on the initialization step of the water sorption process. Carbon 106, 284–288. doi:10.1016/j.carbon.2016.05.042
Wilhelm, J. G., and Schuettelkopf, H. (1973). “Inorganic adsorber materials for trapping fission product iodine,” in Control of Iodine in the nuclear industry, technical reports series n°148 (Vienna, Austria: IAEA), 47–57.
Wood, G. O. (1985). Effects of air temperatures and humidities on efficiencies and lifetimes of air-purifying chemical respirator cartridges tested against methyl iodide. Am. Industrial Hyg. Assoc. J. 46, 251–256. doi:10.1080/15298668591394761
Keywords: activated carbon, sorption efficiency, radioactive methyl iodide, radioactive elemental iodine, radioiodine removal, activated charcoal
Citation: Mokili BM and Abdelouas A (2025) Sorption efficiency of radioactive iodine compounds by nuclear-grade activated carbon - a review and processing of literature data. Front. Nucl. Eng. 4:1553446. doi: 10.3389/fnuen.2025.1553446
Received: 30 December 2024; Accepted: 11 March 2025;
Published: 01 May 2025.
Edited by:
Khawaja Muhammad Rafique Mir, University of Azad Jammu and Kashmir, PakistanReviewed by:
Jun-Yeop Lee, Pusan National University, Republic of KoreaMouheb Chebbi, Institut de Radioprotection et de Sûreté Nucléaire, France
Copyright © 2025 Mokili and Abdelouas. This is an open-access article distributed under the terms of the Creative Commons Attribution License (CC BY). The use, distribution or reproduction in other forums is permitted, provided the original author(s) and the copyright owner(s) are credited and that the original publication in this journal is cited, in accordance with accepted academic practice. No use, distribution or reproduction is permitted which does not comply with these terms.
*Correspondence: Bandombele Marcel Mokili, Ym1tb2tpbGlAZ21haWwuY29t