- 1Department of Biotechnology and Food Science, Norwegian University of Science and Technology, Trondheim, Norway
- 2SINTEF Industry, Trondheim, Norway
Azotobacter vinelandii produces the biopolymer alginate, which has a wide range of industrial and pharmaceutical applications. A random transposon insertion mutant library was constructed from A. vinelandii ATCC12518Tc in order to identify genes and pathways affecting alginate biosynthesis, and about 4,000 mutant strains were screened for altered alginate production. One mutant, containing a mucA disruption, displayed an elevated alginate production level, and several mutants with decreased or abolished alginate production were identified. The regulatory proteins AlgW and AmrZ seem to be required for alginate production in A. vinelandii, similarly to Pseudomonas aeruginosa. An algB mutation did however not affect alginate yield in A. vinelandii although its P. aeruginosa homolog is needed for full alginate production. Inactivation of the fructose phosphoenolpyruvate phosphotransferase system protein FruA resulted in a mutant that did not produce alginate when cultivated in media containing various carbon sources, indicating that this system could have a role in regulation of alginate biosynthesis. Furthermore, impaired or abolished alginate production was observed for strains with disruptions of genes involved in peptidoglycan biosynthesis/recycling and biosynthesis of purines, isoprenoids, TCA cycle intermediates, and various vitamins, suggesting that sufficient access to some of these compounds is important for alginate production. This hypothesis was verified by showing that addition of thiamine, succinate or a mixture of lysine, methionine and diaminopimelate increases alginate yield in the non-mutagenized strain. These results might be used in development of optimized alginate production media or in genetic engineering of A. vinelandii strains for alginate bioproduction.
Introduction
Alginate is the collective term for a family of linear polysaccharides consisting of varying amounts of β-D-mannuronic acid (M) and α-L-guluronic acid (G) (Haug et al., 1966). In nature alginates are produced by brown seaweeds and by several bacteria in the genera Pseudomonas and Azotobacter, among them the soil bacterium A. vinelandii (Gorin and Spencer, 1966). Bacterial alginate is produced as an exopolysaccharide, and the biosynthetic apparatus is similar in both genera (Ertesvåg, 2015). However, A. vinelandii produces alginate constitutively, while biosynthesis is activated only under certain environmental conditions in Pseudomonas spp.
Alginates are commercially important biopolymers with a wide range of industrial and technological applications (Skjåk-Bræk et al., 2015). All commercial alginate production is currently based on extraction from brown algae, which yields complex mixtures of alginates with regard to both chain composition and molecular weight (Andersen et al., 2012). The bulk alginates used in for example food and cosmetic industry can be acquired at prices as low as 5 USD per kilogram, while pharmaceutical or medical grade alginates with defined molecular weights and M/G profiles, and thus more defined material properties, cost about 100 USD per gram (NovaMatrix web catalog prices, September 2019). New pharmaceutical and medical applications are being developed, and this increases the demand for high-end alginates. Because engineered bacteria have the potential for in vivo production of homogeneous alginates with specialized compositions (Remminghorst and Rehm, 2006), there is considerable interest in microbial bioproduction of these polymers. Furthermore, as most current applications rely on the gelling properties of G-blocks (stretches of consecutive G residues) (Andersen et al., 2012), A. vinelandii is an attractive candidate for strain engineering due to its innate ability to introduce G-blocks in the alginate chains (Ertesvåg et al., 1995; Svanem et al., 1999). No strains producing G-block alginates have been identified among the Pseudomonas species studied so far.
In order to optimize bacterial production processes, it is important to understand how alginate biosynthesis is regulated as well as elucidate which other metabolic aspects have an influence on production levels. The precursor for the alginate monomers is fructose 6-phosphate, so alginate biosynthesis is closely connected to the central carbohydrate metabolism of the cells (Maleki et al., 2015, 2017; Ertesvåg et al., 2017). Alginate production is furthermore an energy demanding process and is tightly controlled by a complex network of regulators which also influence other cellular processes (Urtuvia et al., 2017). The state of several cellular processes can therefore be expected to affect the biosynthesis of alginate. So far, the regulatory network controlling alginate biosynthesis has mainly been studied in P. aeruginosa. Regulatory differences between P. aeruginosa and A. vinelandii have already been observed (Núñez et al., 1999; López-Pliego et al., 2018), demonstrating a need for further investigations of these mechanisms in the latter organism. In addition, previous investigations of A. vinelandii have mainly been directed toward gene disruptions or environmental factors leading to an increase in alginate biosynthesis (Núñez et al., 2013; Ahumada-Manuel et al., 2017; Quiroz-Rocha et al., 2017). Identification of genes and processes which influence alginate biosynthesis negatively is however equally important in order to achieve a better understanding of limiting factors, which is a central issue when industrial production purposes are considered.
The aim of this study was to investigate factors affecting alginate biosynthesis in A. vinelandii by screening of a transposon insertion library of strain ATCC12518Tc, a tetracycline-resistant derivative of ATCC12518. The genome of A. vinelandii DJ, also a derivative of ATCC12518, has been sequenced (Setubal et al., 2009), thus greatly simplifying identification of the affected genes in transposon insertion mutants with interesting phenotypes. We have previously screened a Pseudomonas fluorescens transposon library (Ertesvåg et al., 2017), and an additional aim of the current study was to compare the results from the two bacteria in order to detect similarities and differences between the two genera.
Materials and Methods
General Cultivation of Bacteria
The bacterial strains and plasmids used in this work are described in Table 1. Escherichia coli strains were routinely grown in LB broth (10 g/l tryptone, 5 g/l yeast extract, 5 g/l NaCl) or on LB agar at 37°C. A. vinelandii strains were routinely grown in liquid Burk's medium (BM) or RA1 medium (Gimmestad et al., 2006) supplemented with 3.0 ml/l TMS1 (Wentzel et al., 2012) or on BM or RA1 agar at 30°C. The media contained 20 g/l of the relevant carbon source unless stated otherwise. Biotin (1.6 μM), lysine (0.5 mM), methionine (0.6 mM), diaminopimelate (0.2 mM), adenine (0.8 mM), thiamine (2 μM), pyridoxine (5 μM), pyridoxal (5 μM), or succinate (30 mM) were added in some experiments. Antibiotics were present in the following concentrations when used in cultivations: ampicillin 200 μg/ml, tetracycline 15 μg/ml, spectinomycin 20 μg/ml, apramycin 25 μg/ml (A. vinelandii) or 50 μg/ml (E. coli), kanamycin 2 μg/ml (A. vinelandii) or 50 μg/ml (E. coli).
Standard Procedures
Plasmid isolations, enzymatic DNA manipulations and agarose gel electrophoresis were performed according to Sambrook and Russell (2001). Transformation and conjugation was performed as described earlier (Gimmestad et al., 2009). Pwo SuperYield DNA Polymerase (Roche Diagnostics) or Q5 polymerase (New England Laboratories) was used to amplify DNA from A. vinelandii. The QIAquick Gel Extraction Kit and QIAquick PCR Purification Kit (QIAGEN) were used for DNA purifications from gel electrophoresis and enzymatic reactions, respectively. Chromosomal DNA was isolated from A. vinelandii strains using the PurElute™ Bacterial Genomic Kit (EdgeBio). Cells were washed with 0.9% NaCl, 10 mM EDTA (pH 8.0) prior to DNA isolation in order to remove extracellular alginate. DNA sequencing was performed using the BigDye® Terminator v1.1 Cycle Sequencing Kit (Applied Biosystems). Transposon insertion sites were identified using primers that are complementary to the ends of the inserted fragment, and genomic DNA isolated from each mutant as the template, thus allowing direct sequencing of the genomic regions flanking the insertion. Primer sequences for PCR and sequencing are available upon request.
Construction of a Transposon Insertion Mutant Library
The mini-Tn5 transposon delivery vector pCAM140 which encodes a spectinomycin resistance gene flanked by transcriptional terminators to avoid read-through from the transposon (Wilson et al., 1995) was introduced to A. vinelandii ATCC12518Tc by conjugation. Mating was performed at 30°C on LB agar and transconjugants were selected on RA1 agar containing tetracycline and spectinomycin. Selection plates also contained alginate lyase AlgL (6.5 mU/cm2) (Ertesvåg et al., 1998); the enzyme was applied to the plates before spreading the cells in order to reduce the colony mucoidicity of alginate-producing transconjugants. Transconjugant colonies were picked using a Genetix Q-Pix2 robotic colony picker and transferred to 96-well microtiter plates containing 110 μl liquid 0.5x RA1 medium (CaCl2·2H2O and MOPS concentrations as for 1x medium, and 10 g/l fructose) with tetracycline and spectinomycin. A. vinelandii insertion mutants are known to sometimes contain copies of both mutant and wild-type alleles, so to eliminate wild-type chromosome copies the transconjugants were grown by repeated transfers in selective media. All liquid and microtiter plate handling were performed by a Beckman Coulter Core robotic equipped with a Beckma Coulter NXP liquid handling unit.
Alginate Analyses
Culture samples (diluted in 0.2 M NaCl when necessary to reduce viscosity) were centrifuged to remove bacterial cells, and the alginates in the cell-free supernatants were deacetylated by mild alkaline treatment as described previously (Ertesvåg and Skjåk-Bræk, 1999). The alginate content in the deacetylated samples was determined enzymatically as described earlier (Østgaard, 1992; Maleki et al., 2015). Samples for alginate quantification were collected from transconjugants cultivated in 96-well microtiter plates with 110 μl selective 0.5x RA1 medium in each well. Mutants displaying a potential increase or decrease in alginate production were verified by assessing alginate production in triplicate cultures in 96-deepwell plates with 600 μl selective 0.2x RA1 medium in each well, or in 250 ml shake flask with 30 ml 0.5x RA1 medium. In all experiments, A. vinelandii ATCC12518Tc was included as a reference. The reference strain displayed similar alginate production and growth characteristics in deepwell plates and in shake flasks. The final alginate production and cell densities reported in this study are based on measurements of extracellular alginate concentration (described above) and OD660 respectively, sampled in stationary phase.
For qualitative analyses of complemented strains, 1 ml culture was centrifuged, NaCl was added to the supernatant (0.1 M) and the alginate was precipitated with an equal amount of isopropanol. Only alginate-producing strains produce a pellet in this assay. The mutant strain, or mutant strain with an empty vector (pIB11), was always used as a control.
Fermentations
Fermentations of A. vinelandii strains were performed as described by Steigedal et al. (2008) except that the inoculum was cultivated in two steps in 0.5x RA1 medium in shake flasks. The first culture was cultivated until visible growth, followed by inoculating 3% into fresh medium for the second stage inoculum, which was then cultivated over night before being transferred, 3%, to 3 l Applikon fermentors with 1 l of 0.5x PM1 containing the following ingredients per liter: fructose (50 g), peptone (4.75 g), MgSO4·7H2O (0.3 g), KH2PO4 (65 mg), K2HPO4 (16 mg), NaCl (0.2 g), CaCl2·2H2O (0.29 g), FeSO4·7H2O (20 mg), and Clerol FBA622 (antifoam, 0.5 g). The fermentations were performed at 30°C. pH was adjusted to 7.2 from start and controlled at this pH by addition of NaOH. The dissolved oxygen was controlled to 10% of saturation by automatic control of the stirrer speed. Aeration rate in the culture medium in fermentors was initially 0.25 vvm (lgas/lliquid per minute) and was increased up to 1.0 vvm when required for maintaining dissolved oxygen without exceeding the maximum stirrer speed of the fermentor (2,000 rpm).
Results
Construction and Screening of the Transposon Insertion Mutant Library
In order to obtain an unbiased transposon insertion library allowing for auxotrophic mutants, the strains have to be maintained on a rich medium. This required insertion of a resistance marker into the A. vinelandii ATCC12518 strain, to allow for counter selection of E. coli after conjugation. Using the gene replacement vector pHE208, we therefore constructed a tetracycline resistant derivative of ATCC12518, designated ATCC12518Tc, in which a putative transposase gene is disrupted by insertion of the tetA-tetR genes (Table 1). Strain ATCC12518Tc produced 8.3 (±0.9) g/l alginate in batch fermentations, which is about 70% of that observed for the wild type. The reason for this reduction is not known. The production level is however still sufficient to allow for identification of mutants with lowered alginate production, and the decrease could potentially be beneficial for identification of mutants with increased production. A transposon insertion library of ~4,000 transconjugants was made from A. vinelandii ATCC12518Tc as described in the Materials and Methods section.
The mutant strains from the library were cultivated in 96-well microtiter plates and screened with regard to alginate production levels compared to the reference strain ATCC12518Tc (see Materials and Methods section). Mutants that failed to grow (~1,000 mutants) were disregarded. Among the viable mutants, the screen identified 56 mutants with potentially increased alginate production (>125% alginate concentration relative to the reference strain) and 241 mutants with potentially lowered alginate production (<50% relative to the reference).
Verification of Mutants With Altered Alginate Production Levels
Selected mutants with potentially altered alginate production were recultivated in triplicates in deepwell plates and/or shake flasks (see Materials and Methods section) for verification of the initial screening results. A total of 17 mutants with potentially increased alginate production and 109 mutants with potentially lowered alginate production were chosen for verification experiments. Using the same criteria as above, an increase or decrease in alginate production relative to the reference strain was affirmed for 2 and 68 of these candidates, respectively. The verified mutants showing increased or decreased alginate production are hereafter referred to as up-mutants and down-mutants, respectively, and their alginate production and growth levels relative to the reference strain is given in Table 2. Several of the mutant strains were observed to aggregate in liquid culture. Thus, growth measured as OD660 could not always be considered a reliable measure and is therefore not discussed in more detail. It should however be noted that for one of the up-mutants, with a transposon insertion in pgm-2, the apparent increase in alginate yield is most likely caused by an increased cell density in the cultures (Table 2).
Validation of the Verification Process by Batch Fermentation Trials
To further confirm the alginate phenotypes observed in the verification experiments, fermentations were performed for 12 of the down-mutants and one up-mutant (36C11) in order to obtain growth and alginate production data under highly controllable conditions. Very low or no alginate production (<10% relative to the reference strain) was confirmed for 10 of the down-mutants (27H10, 33A03, 20A04, 22B07, 08E05, 20A06, 36F10, 22G07, 39H08, and 21C03), while the remaining two were shown to produce 67% (03E03) and 35% alginate (45G12) compared to the reference strain. Batch fermentation of the up-mutant 36C11 confirmed a significant increase in alginate production; 180% relative to ATCC12518Tc. The general agreement between the fermentation results and the alginate production levels observed in deepwell plates indicate that verification experiments with triplicate deepwell plate cultivations can be regarded as a reliable method for evaluating large numbers of A. vinelandii strains with regard to alginate production.
Identification of Disrupted Genes in Mutants With Altered Alginate Production
The transposon insertion points were identified in the genomes of the verified up- and down-mutants (Table 2) by determining the chromosomal DNA sequences flanking the transposons and performing BLAST (Altschul et al., 1997) searches against the A. vinelandii DJ genome (Setubal et al., 2009). The affected genes include genes involved in metabolism, transport, translation and gene regulation (Table 2). For 11 of the mutants in Table 2, the affected genes were annotated as hypothetical. Ten of the mutants have insertions in structural genes directly involved in alginate biosynthesis (algD, algK, algJ, algI, and algA) (Rehm et al., 1996), and show the expected alg− phenotype. For further investigations of selected mutants, we chose to focus on some of the genes with known or putative regulatory functions. Moreover, in an earlier study on the alginate-producing mutant of P. fluorescens, it was reported that inactivation of many genes in the central metabolism resulted in lower alginate production. We therefore wanted to investigate whether this appeared to be the case for A. vinelandii as well.
Transposon Insertions in Regulatory Genes Presumed to Be Involved in Alginate Biosynthesis
The regulation of alginate biosynthesis has mostly been studied in P. aeruginosa (reviewed in Hay et al., 2014; Urtuvia et al., 2017). The phenotypes of A. vinelandii mutants found to have insertions in such genes are therefore discussed taking P. aeruginosa strains with disruptions in homologous genes into consideration. One mutant was found to have the transposon inserted in the known A. vinelandii alginate regulatory gene mucA, and five mutants had insertions in homologs of alginate regulatory genes known from P. aeruginosa: algW, amrZ (three independent mutants), and algB. These mutants are described below. The gene context of the genes discussed below are shown in Supplementary Figure S1. In addition, several other genes putatively involved in transcription regulation were identified in the screen (Table 2), but since their targets are unknown, they were not studied further.
MucA is an anti-sigma factor that represses sigma factor AlgU, which is needed for expression of alginate biosynthetic gene algD (and possibly other alginate biosynthetic genes). A. vinelandii mucA mutants have previously been shown to display increased alginate production (Martínez-Salazar et al., 1996; Núñez et al., 2000). As could be expected, this is also the case for the mucA::TnCAM140 mutant identified in this work.
The protease AlgW has been shown to be required for activation of alginate biosynthesis in P. aeruginosa, by degrading MucA in a MucE-dependent manner (Wood et al., 2006; Qiu et al., 2007; Cezairliyan and Sauer, 2009). The algW::TnCAM140 mutant identified in this work does not produce alginate, which indicates that the A. vinelandii AlgW homolog has a function similar to the P. aeruginosa protease even though there is no mucE homolog in A. vinelandii (Setubal et al., 2009). However, when complemented with algW encoded on plasmid pHE536, the alginate production was not restored.
An algB::TnCAM140 mutant was identified among the mutants with lowered alginate production in the initial screen, but further evaluations showed that the production level of this mutant is actually comparable to that of the reference strain. The two-component response regulator AlgB is required for alginate production in P. aeruginosa (Goldberg and Ohman, 1987; Goldberg and Dahnke, 1992; Wozniak and Ohman, 1994) where it positively regulates expression of biosynthetic genes by direct binding to PalgD (Leech et al., 2008). Our results thus indicate that the role of the AlgB homolog in A. vinelandii differs from that of P. aeruginosa AlgB. PCR on chromosomal DNA from the transposon mutant confirmed the absence of wild type copies of the algB gene. Thus, it appears that AlgB is not required for alginate production in A. vinelandii.
AmrZ (AlgZ) is a DNA binding protein required for transcription from PalgD in P. aeruginosa (Baynham et al., 1999), and has also been shown to negatively regulate motility in both P. aeruginosa and P. fluorescens via FleQ (Baynham et al., 2006; Tart et al., 2006; Martínez-Granero et al., 2012). Expression of P. aeruginosa amrZ is controlled by the sigma factor AlgU (AlgT) (Wozniak et al., 2003). AlgU negatively regulates motility in A. vinelandii as well, but via CydR and FlhDC instead of AmrZ and FleQ (León and Espín, 2008). An amrZ mutant has not previously been described in Azotobacter. The alginate production in our amrZ::TnCAM140 mutant was restored by complementation using plasmid pHE537, which encodes amrZ. This confirms that AmrZ is necessary for alginate production in A. vinelandii, like it is in P. aeruginosa.
A fruA Transposon Insertion Abolishes Alginate Production in A. vinelandii
Alginate production was absent in a mutant with the transposon insertion in an E. coli fruA homolog. Complementation with plasmid pHE542 encoding fruA restored the strain's ability to produce alginate (data not shown), demonstrating that the observed phenotype was indeed caused by the fruA disruption. As FruA is a part of the fructose phosphoenolpyruvate phosphotransferase system (PTS) (Prior and Kornberg, 1988), the lack of alginate synthesis could simply be caused by limited carbon uptake, since fructose was used as the carbon source in cultivations. The fruA::TnCAM140 mutant was therefore evaluated in media containing other carbon sources, but still did not produce alginate when fructose was replaced with glucose, glycerol or sucrose (data not shown).
Mutations in Genes Encoding Proteins Involved in Biosynthesis of Vitamins, Cofactors and Biosynthetic Precursors Affect Alginate Production Levels
Transposon insertions in genes involved in isoprenoid, purine and thiamine biosynthesis resulted in decreased alginate production in A. vinelandii, and the same has previously been observed for P. fluorescens (Figure 1). Several other A. vinelandii mutants shown to produce very little or no alginate have transposon insertions in genes involved in the biosynthesis of cofactors (vitamins) or central metabolic precursors (Table 2). Selected mutants in these categories are described below.
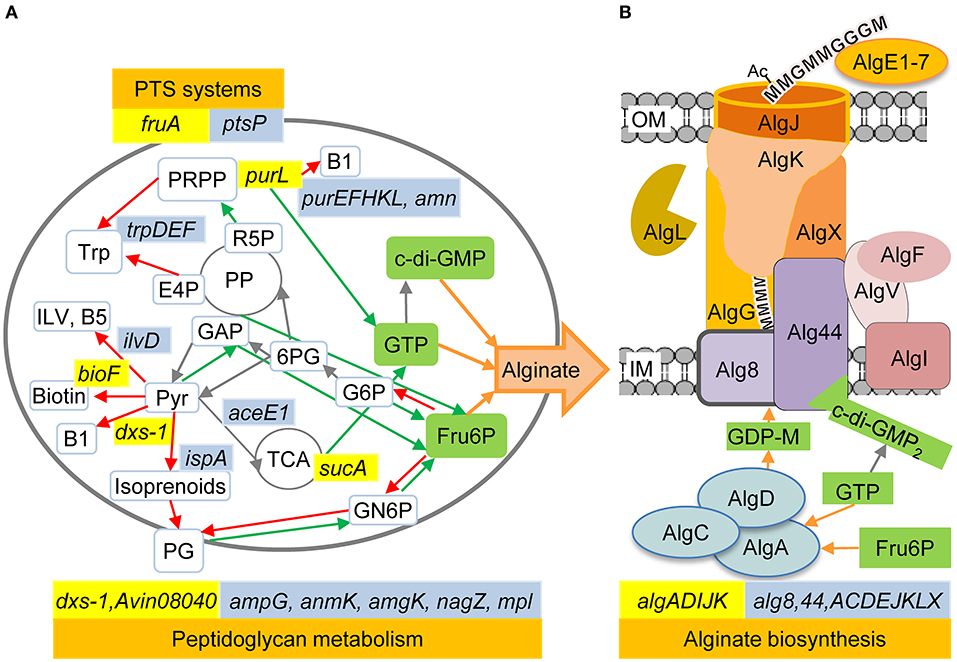
Figure 1. The relationship between alginate biosynthesis and the cellular metabolism in A. vinelandii and P. fluorescens. (A) A simplified model of the cell's metabolism highlighting the processes identified in the present study as being important for full alginate biosynthesis levels. (B) The proteins and metabolites directly needed for alginate biosynthesis (adapted from Ertesvåg et al., 2017). A. vinelandii genes discussed in the current paper are highlighted in yellow, while the previously identified P. fluorescens genes are highlighted in blue. In P. fluorescens, the AlgJ homolog is named AlgE, while the AlgV homolog is named AlgJ. Red arrows indicate pathways competing with accumulation of the three metabolites Fru6P, GTP, and c-di-GMP, which are essential for alginate biosynthesis, while green arrows indicate pathways that would increase the synthesis of one of these three metabolites. Each arrow may represent several enzymatic steps. OM, Outer membrane; IM, Inner membrane; 6PG, 6-Phosphogluconate; Ac, Acetyl; B1, Thiamine; B5, Pantothenate; E4P, Erythrose 4-phosphate; G, Guluronic acid residue; GN6P, Glucosamine 6-phosphate; G6P, Glucose 6-phosphate; ILV, Isoleucine Leucine Valine; M, Mannuronic acid residue; PG, Peptidoglycan; PP, Pentose-phosphate cycle; PRPP, Phosphoribosyl pyrophosphate; Pyr, Pyruvate; R5P, Ribose 5-phosphate; TCA, Tricarboxylic acid cycle; Trp, Tryptophan.
1-deoxy-D-xylulose-5-phosphate synthase (Dxs) synthesizes the common precursor for thiamine, pyridoxine, and isoprenoid biosynthesis (Sprenger et al., 1997). An A. vinelandii dxs-1 mutant identified in our screen was found to produce no or very low amounts of alginate. A. vinelandii has two nearly identical dxs genes, but the phenotype of the dxs-1 mutant indicates that the expression of dxs-2 alone is not sufficient for alginate production. Production was restored by cloning wt dxs-1 on a transposon and transferring it back to the dxs-1::TnCAM140 mutant (data not shown), thus confirming that dxs-1 is required for alginate production in A. vinelandii. Cultivating the dxs-1::TnCAM140 mutant with pyridoxine, pyridoxal or thiamine added to the growth medium did however not restore alginate production (data not shown).
The phosphoribosylformylglycinamidine synthase PurL is involved in de novo biosynthesis of purines (Sampei and Mizobuchi, 1989). A purL mutation abolished alginate production in both A. vinelandii (this study) and P. fluorescens (Ertesvåg et al., 2017). For P. fluorescens this was also observed for several additional mutants where genes involved in either de novo or salvage pathways for purine synthesis were disrupted (Figure 1). Some of these P. fluorescens mutants were complemented, and complementation was shown to restore alginate biosynthesis (Ertesvåg et al., 2017).
PurL is also involved in biosynthesis of thiamine, as thiamine is derived from the purine biosynthesis intermediate 5-amino-1-(5-phospho-D-ribosyl)imidazole (AIR) (Begley et al., 1999). Thiamine is an essential cofactor for a variety of enzymes, several of which play important roles in carbohydrate metabolism. Due to the central roles of purines and thiamine, A. vinelandii cells that do not synthesize these compounds would be expected to be unable to grow in the media used in this study. A. vinelandii insertion mutants are however known to sometimes contain copies of both mutant and wild-type alleles. This was shown to be the case for the purL::TnCAM140 mutant, which explains how the strain was still able to grow normally.
Likewise, a low-alginate-producing bioF::TnCAM140 mutant was shown to retain wild-type alleles. A genetically pure bioF mutant would not be expected to grow in unsupplemented media, as BioF is an 8-amino-7-oxononanoate synthase involved in the synthesis of biotin, a cofactor required for cellular growth (Marquet et al., 2001).
Our results also revealed that alginate production was abolished in an A. vinelandii sucA::TnCAM140 mutant. SucA is part of the 2-oxoglutarate dehydrogenase complex, which catalyzes the irreversible conversion of 2-oxoglutarate to succinyl-CoA in the tricarboxylic acid (TCA) cycle. In addition to being an intermediate in the TCA cycle, succinyl-CoA is a precursor for several important biomolecules. Inactivation of sucA would be expected to reduce growth rate (Yu et al., 2006), but PCR showed that the sucA mutant, like the purL and bioF mutants, has retained both disrupted and wild-type alleles, which can explain why growth was unimpaired (Table 2).
Alginate Production Is Limited by Access to Key Metabolites in A. vinelandii
As mentioned earlier, A. vinelandii has multiple copies of its chromosome and will use this to retain wild type copies of genes necessary to maintain an adequate growth rate even if the inactivated version is selected for by an antibiotic. As described above, the purL, bioF, and sucA mutants all contained wild type copies of their chromosomes in addition to chromosomes where the gene was inactivated by the transposon. Such mutants may easily revert to the wild type, and any analyses of growth or alginate production would be hampered by perceivable fluctuations of the relative copy-number of each chromosome between different cells in the population. Moreover, complementation results of such mutants are not easily interpreted, since any perceived wild-type phenotype theoretically could be caused by a high copy-number of the wild type chromosome rather than by the complementing plasmid.
Still, the phenotypes of the dxs-1, purL, bioF, and sucA mutants indicate that a lack of essential vitamins or other key metabolites negatively affects A. vinelandii alginate production, even when growth is only mildly affected or not affected at all. If this is the case, it is also possible that insufficient access to such compounds is a limiting factor for alginate biosynthesis in the reference strain.
In light of this, we settled on an alternative approach to further assess the impact of these mutations. We hypothesized that sufficient availability of compounds like succinate, biotin, thiamine, or purines could be necessary for alginate biosynthesis, and thus also be a limiting factor for alginate biosynthesis in the reference strain. To investigate this, strain ATCC12518Tc was cultivated in RA1 medium with different supplements: succinate, the four vitamins pyridoxal, pyridoxine, thiamine and biotin, the purine adenine, and a mixture of the three amino acids diaminopimelate, lysine, and methionine (Table 3). The amino acids were chosen due to their dependence on the succinate-derivative succinyl CoA for biosynthesis. While this is true for diaminopimelate and lysine; in A. vinelandii methionine biosynthesis is catalyzed by MetX instead of MetA, and probably utilizes acetyl-CoA and not succinyl-CoA as an acyl-donor (Ferla and Patrick, 2014).
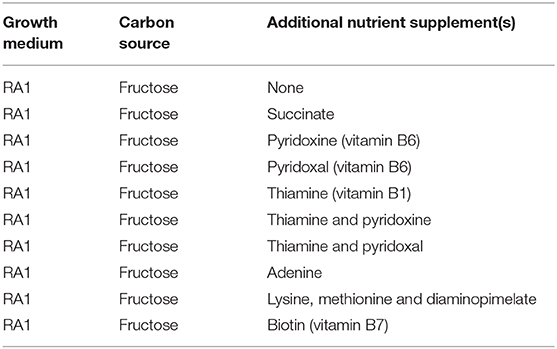
Table 3. Growth medium combinations used for cultivation experiments to assess nutrient supplements.
The cultivation results showed that while cell growth was not significantly affected by any of the added nutrients (Figure 2A), addition of succinate, thiamine or a mixture of lysine, methionine, and diaminopimelate increased the amount of measured alginate in the cultures by ~40% (Figure 2B). The observed effect of succinate supplementation could be due to the added carbon, since ~5 g/l was added to the medium (in addition to the main carbon source; 20 g/l fructose), but cell growth did not increase relative to the reference. The effect of the amino acid supplement could also be related to the role of diaminopimelate as a precursor in peptidoglycan biosynthesis (Mengin-Lecreulx et al., 1996), as there appears to be a connection between peptidoglycan metabolism and alginate biosynthesis (Figure 1). Thus, the availability of thiamine and possibly TCA cycle intermediates appears to be limiting for alginate production in A. vinelandii.
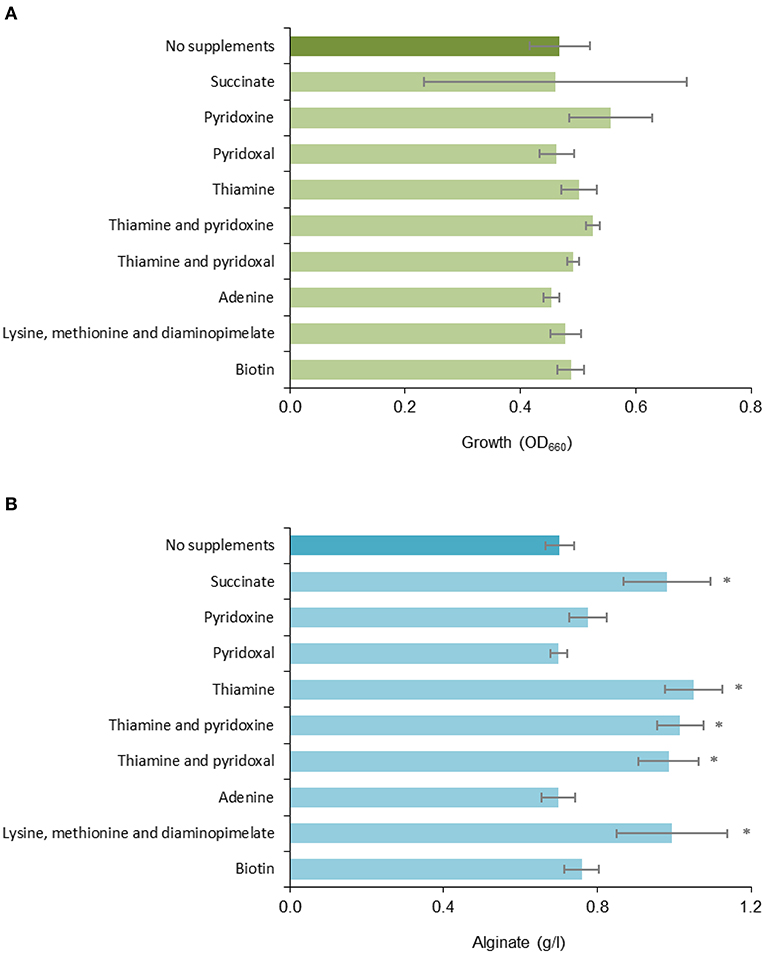
Figure 2. Effect of different media supplements on (A) growth and (B) alginate production. Triplicate cultures of A. vinelandii ATCC12518Tc was grown in deepwell plates containing RA1 with TMS1, fructose and different supplements for 48 h before sampling. Cultures without supplements were included as a reference. Error bars represent one standard deviation. *denotes a statistically significant increase in alginate concentration relative to the reference (p ≤ 0.05).
Discussion
The presented screen had limited coverage, since several genes known to be necessary for alginate biosynthesis were not identified (confer Figure 1B). Still, genes not previously known to be required for alginate production were identified as necessary, the most interesting of which may be fruA. The scarcity of mutants with a reproducible increase in alginate production could reflect the fact that the reference strain, ATCC12518Tc, produces quite large amounts of the polysaccharide, so there might be fewer single gene mutations that will have a pronounced positive effect on production levels. The current study focused on two groups of genes; genes with assumed regulatory functions and genes connected to central metabolism.
Mutations in Regulatory Genes
Alginate production is dependent on the RpoE-like sigma factor AlgU. MucA is an anti-sigma factor, which sequesters AlgU. A mucA mutant was identified in the present study, and as could be expected, this mutant showed an increase in alginate production levels. In P. aeruginosa, release of AlgU is controlled by a proteolytic cascade similar to the activation of RpoE in E. coli, and AlgW is needed for the first proteolytic cleavage (Delgado et al., 2018). A non-alginate producing algW mutant was identified in the present study but could not be complemented by plasmid pHE536. More studies on the role of AlgW and other proteases in A. vinelandii are needed in order to elucidate the proteolytic cascade for AlgU activation in this organism.
While the two-component response regulator AlgB is necessary for alginate production in P. aeruginosa, our study indicates that this is not the case in A. vinelandii. A possible explanation would be that the mutant expresses a truncated AlgB protein which has retained sufficient function to carry out its role in alginate synthesis. This is however not very likely, since the transposon insertion splits the gene almost evenly in half, and a specific amino acid in the C-terminal end has previously been shown to be necessary for the binding of AlgB to PalgD in P. aeruginosa (Ma et al., 1998; Leech et al., 2008). The AlgB proteins from P. aeruginosa PAO1 and A. vinelandii DJ are of equal length and share 75% sequence identity.
In P. aeruginosa the cognate histidine kinase of AlgB is KinB, but AlgB phosphorylation is not needed for its role in alginate production (Ma et al., 1998). KinB has recently been shown to be a negative regulator of alginate biosynthesis in this organism, as AlgW-dependent proteolysis of MucA does not occur in the presence of KinB (Damron et al., 2009). However, no histidine kinase gene is found in the vicinity of algB in A. vinelandii, and a homology search of the A. vinelandii DJ genome revealed that it does not encode a KinB homolog. The phenotype of our mutant and the absence of a KinB homolog indicate that the algB gene may not have a role in the regulation of alginate biosynthesis in A. vinelandii.
AmrZ was originally identified as a positive regulator for alginate biosynthesis in P. aeruginosa (Baynham and Wozniak, 1996). The protein was later found to also act as a positive or negative regulator for several other genes, that encode proteins involved in motility, polysaccharide biosynthesis, iron homeostasis, c-di-GMP production etc., in various species of Pseudomonas (See Martínez-Granero et al., 2014; Prada-Ramírez et al., 2016; Hou et al., 2019, and references therein). Interestingly, the effect of AmrZ differs between species; while a P. aeruginosa amrZ mutant had an increased level of c-di-GMP (Hou et al., 2019), a P. fluorescens amrZ mutant displayed severely reduced levels (Muriel et al., 2018). The effect on motility is also species dependent, from severe defects to hypermotility (Baltrus et al., 2018). The present study shows that amrZ is necessary for alginate production in A. vinelandii. AmrZ has not previously been studied in A. vinelandii, and given the pleiotropic effects of its homolog in Pseudomonas spp., it would be interesting to further investigate the role of AmrZ in A. vinelandii.
FruA Is Necessary for Alginate Biosynthesis
Our growth studies showed that FruA is necessary for alginate production on several carbon sources, also those that do not require this protein for uptake. Many carbohydrate PTS systems have regulatory roles besides their function in sugar uptake and phosphorylation (reviewed in Deutscher et al., 2006), which is another possible explanation for the absence of alginate production in this mutant. Similarly to P. putida (Velázquez et al., 2007), A. vinelandii encodes two PTS systems; FruA-FruB (PTSFru) and PtsO-PtsN-PtsP (PTSNtr). The latter is not involved in carbohydrate uptake, but is believed to be involved in coordinating nitrogen and carbon metabolism in several bacteria and has been shown to affect various metabolic processes (reviewed in Deutscher et al., 2006). Furthermore, cross-talk between the PTSFru and PTSNtr systems has been demonstrated in P. putida (Pflüger-Grau et al., 2011). In A. vinelandii, the PTSNtr system has been shown to be involved in regulation of polyhydroxybutyrate and alkylresorcinol synthesis (Segura and Espín, 1998; Noguez et al., 2008; Muriel-Millán et al., 2015). As these studies were carried out on derivatives of the A. vinelandii UW strain, which does not produce alginate, it is not known whether PTSNtr mutations also have an effect on alginate synthesis. However, the screening of a P. fluorescens transposon insertion library (Figure 1) revealed that inactivation of ptsP does affect alginate production negatively in this strain. Thus, our results indicate that the A. vinelandii PTSFru system could have a role in regulation of alginate biosynthesis, possibly via interaction with the PTSNtr system.
Alginate Biosynthesis Seems to Be Regulated by the Metabolic Status of the Cell
Our earlier studies in P. fluorescens indicated that even in a mucA mutant, alginate biosynthesis does not start immediately after the first alginate biosynthetic complexes have been assembled (Maleki et al., 2016). Moreover, both the P. fluorescens transposon insertion study (Ertesvåg et al., 2017) and studies focusing on the synthesis of fructose 6-phosphate (Maleki et al., 2015, 2017) clearly indicated that the cells prioritize growth and survival over alginate production. The present study indicates that the same is true for A. vinelandii.
Some of the mutants identified in our screen had insertions in genes encoding proteins homologous to or in the same metabolic pathways as proteins identified and confirmed as relevant for alginate biosynthesis in the P. fluorescens screen (Figure 1). Even if many of these A. vinelandii mutants were not complemented, the concurrence makes it more plausible that the observed phenotypes are due to the introduced mutations.
A. vinelandii Avin08040 and an mpl homolog appear to form an operon, with Avin08040 located upstream of mpl. The transposon insertion in Avin08040 resulted in decreased alginate production, and a slight decrease has also been observed for a P. fluorescens mpl mutant (Ertesvåg et al., 2017). The murein peptide ligase Mpl is involved in the recycling of cell wall peptidoglycan (Mengin-Lecreulx et al., 1996), a process where degradation products from peptidoglycan can be used to resynthesize more peptidoglycan or be utilized as an energy source (Park and Uehara, 2008). Avin08040 encodes a probable aromatic acid decarboxylase of unknown function, but the observed phenotype of this mutant could result from polar effects on mpl expression; a defect in peptidoglycan recycling would impose a drain on fructose-6-phosphate, making less available for alginate biosynthesis. It is also possible that Avin08040 plays a hitherto unknown role in utilization of peptidoglycan degradation products.
Results obtained in the current study show that dxs-1 is necessary for alginate biosynthesis in A. vinelandii. Negative impact on alginate production has also been reported for a P. fluorescens ispA mutant (Ertesvåg et al., 2017). For P. fluorescens this could be due to a polar effect on dxs expression, as these genes are part of the xseB-ispA-dxs gene cluster encoding exodeoxyribonuclease VII small subunit, geranyl transferase (isoprenoid biosynthesis), and 1-deoxy-D-xylulose-5-phosphate synthase (thiamine, pyridoxine, and isoprenoid biosynthesis). P. fluorescens has one such cluster (Winsor et al., 2016) while the A. vinelandii genome contains two nearly identical copies (Setubal et al., 2009). The two A. vinelandii Dxs proteins are identical except for one Pro to Ala substitution, but according to our results the bacterium does not produce alginate when only dxs-2 is intact. Alginate production was restored by complementation with wt dxs-1, but not by addition of pyridoxine, pyridoxal or thiamine to the growth medium. This suggests that the role of Dxs-1 in alginate biosynthesis could be related to its role in isoprenoid biosynthesis, or to some yet unknown function of this protein.
We were unable to isolate pure mutants with insertions in the sucA, purl, and bioF genes. Still, in light of the results from P. fluorescens, the phenotype of the A. vinelandii purL mutant was as expected and demonstrates that A. vinelandii mutants can exhibit changed alginate phenotypes even if the mutant strain is not genetically pure. Based on the expected deficiencies in the identified metabolic mutants, cultivation of the parent strain was performed with addition of selected medium supplements (Table 3). The results (Figure 2) show that adding thiamine, succinate or a mixture of lysine, methionine, and diaminopimelate resulted in increased alginate biosynthesis for the wild type strain. There is no obvious connection between thiamine or succinate levels and alginate biosynthesis, but both compounds are essential for several central metabolic processes. A deficiency can thus be expected to cause suboptimal performance of the cellular metabolism, which could render the cell unable to carry out certain energy-demanding secondary processes, such as biosynthesis of exopolysaccharides.
Furthermore, it was recently shown that A. vinelandii cells cultivated using succinate as the sole carbon source produce more alginate and also show differential expression of sRNA genes belonging to the GacS/A-Rsm regulatory system (affects algD expression) compared to cells cultivated with glucose or fructose (López-Pliego et al., 2018). Interestingly, succinate, methionine, diaminopimelate and lysine have been shown to lower the thiamine requirement in Salmonella typhimurium, probably by reducing the need for succinyl-CoA synthase (Enos-Berlage and Downs, 1997). Since thiamine biosynthesis is competing with purine biosynthesis for precursors, this is an indirect link to alginate biosynthesis (Figure 1). Succinyl CoA is also a precursor for protein succinylation, and such posttranslational modification is used to fine-tune the metabolism (Yang et al., 2015). In addition to proteins involved in TCA and gluconeogenesis, four proteins necessary for alginate biosynthesis, namely AlgU, MucB, AlgR, and AlgC, were found to be succinylated in P. aeruginosa (Gaviard et al., 2018).
Conclusions
Screening of the described A. vinelandii transposon mutant library identified only one strain, a mucA::TnCAM140 mutant, which produces significantly more alginate per cell than the reference strain. Several gene disruptions resulting in decreased or abolished alginate production were however identified, including genes not previously known to affect alginate biosynthesis. The data also provided new insights regarding alginate regulatory genes in A. vinelandii and the cellular processes and metabolites that influence alginate synthesis in this organism. Finally, analyses of the data resulted in the identification of nutrients that limit alginate production in the reference strain, which would not have been easily found using metabolic modeling. The results obtained in the present study will be valuable both for development of alginate bioproduction media and for genetic engineering of A. vinelandii toward optimization of alginate production and yield.
Data Availability Statement
All relevant data is contained within the manuscript.
Author Contributions
MM, HS, TE, SV, and HE participated in the design of the study. MM, ØJ, HS, GK, and AT performed the experiments. MM and HE reviewed bioinformatic information and literature on relevant genes and drafted the manuscript. All authors read and approved the manuscript.
Funding
This work was supported by the Research Council of Norway and FMC Biopolymers. Open access publication was supported by NTNU's publishing fund.
Conflict of Interest
The authors ØJ, HS, GK, AT, and TE were employed by the Research Foundation SINTEF Industry.
All authors declare that the research was conducted in the absence of any commercial or financial relationships that could be construed as a potential conflict of interest.
Acknowledgments
The authors thank Tone Haugen and Vu To for assisting with cultivations, fermentations, and alginate assays and Margit Dagsdatter Haugsnes for constructing the transposon vector pMH13.
Supplementary Material
The Supplementary Material for this article can be found online at: https://www.frontiersin.org/article/10.3389/fbioe.2019.00475/full#supplementary-material
Supplementary Figure S1. Gene context of the genes discussed in the Paper: The discussed genes are shown as blue arrows, neighboring genes as red arrows, and the transposon insertions by bars. The genes are sorted by their mutation identifier.
References
Ahumada-Manuel, C. L., Guzmán, J., Peña, C., Quiroz-Rocha, E., Espín, G., and Núñez, C. (2017). The signaling protein MucG negatively affects the production and the molecular mass of alginate in Azotobacter vinelandii. Appl. Microbiol. Biotechnol. 101, 1521–1534. doi: 10.1007/s00253-016-7931-8
Altschul, S. F., Madden, T. L., Schaffer, A. A., Zhang, J., Zhang, Z., Miller, W., et al. (1997). Gapped BLAST and PSI-BLAST: a new generation of protein database search programs. Nucleic Acids Res. 25, 3389–3402. doi: 10.1093/nar/25.17.3389
Andersen, T., Strand, B. L., Formo, K., Alsberg, E., and Christensen, B. E. (2012). Alginates as biomaterials in tissue engineering. Carbohydr. Chem. 37, 227–258. doi: 10.1039/9781849732765-00227
Bakke, I., Berg, L., Aune, T. E., Brautaset, T., Sletta, H., Tøndervik, A., et al. (2009). Random mutagenesis of the Pm promoter as a powerful strategy for improvement of recombinant-gene expression. Appl. Environ. Microbiol. 75, 2002–2011. doi: 10.1128/AEM.02315-08
Bakkevig, K., Sletta, H., Gimmestad, M., Aune, R., Ertesvåg, H., Degnes, K., et al. (2005). Role of the Pseudomonas fluorescens alginate lyase (AlgL) in clearing the periplasm of alginates not exported to the extracellular environment. J. Bacteriol. 187, 8375–8384. doi: 10.1128/JB.187.24.8375-8384.2005
Baltrus, D. A., Dougherty, K., Diaz, B., and Murillo, R. (2018). Evolutionary plasticity of AmrZ regulation in Pseudomonas. mSphere 3. doi: 10.1128/mSphere.00132-18
Baynham, P. J., Brown, A. L., Hall, L. L., and Wozniak, D. J. (1999). Pseudomonas aeruginosa AlgZ, a ribbon-helix-helix DNA-binding protein, is essential for alginate synthesis and algD transcriptional activation. Mol. Microbiol. 33, 1069–1080. doi: 10.1046/j.1365-2958.1999.01550.x
Baynham, P. J., Ramsey, D. M., Gvozdyev, B. V., Cordonnier, E. M., and Wozniak, D. J. (2006). The Pseudomonas aeruginosa ribbon-helix-helix DNA-binding protein AlgZ (AmrZ) controls twitching motility and biogenesis of type IV pili. J. Bacteriol. 188, 132–140. doi: 10.1128/JB.188.1.132-140.2006
Baynham, P. J., and Wozniak, D. J. (1996). Identification and characterization of AlgZ, an AlgT-dependent DNA-binding protein required for Pseudomonas aeruginosa algD transcription. Mol. Microbiol. 22, 97–108. doi: 10.1111/j.1365-2958.1996.tb02659.x
Begley, T. P., Downs, D. M., Ealick, S. E., McLafferty, F. W., Van Loon, A. P. G. M., Taylor, S., et al. (1999). Thiamin biosynthesis in prokaryotes. Arch. Microbiol. 171, 293–300. doi: 10.1007/s002030050713
Cezairliyan, B. O., and Sauer, R. T. (2009). Control of Pseudomonas aeruginosa AlgW protease cleavage of MucA by peptide signals and MucB. Mol. Microbiol. 72, 368–379. doi: 10.1111/j.1365-2958.2009.06654.x
Damron, F. H., Qiu, D., and Yu, H. D. (2009). The Pseudomonas aeruginosa sensor kinase KinB negatively controls alginate production through AlgW-dependent MucA proteolysis. J. Bacteriol. 191, 2285–2295. doi: 10.1128/JB.01490-08
de Lorenzo, V., Cases, I., Herrero, M., and Timmis, K. N. (1993). Early and late response of TOL promoters to pathway inducers: identification of postexponential promoters in Pseudomonas putida with lacZ-tet bicistronic reporters. J. Bacteriol. 175, 6902–6907. doi: 10.1128/jb.175.21.6902-6907.1993
Delgado, C., Florez, L., Lollett, I., Lopez, C., Kangeyan, S., Kumari, H., Mathee, K., et al. (2018). Pseudomonas aeruginosa regulated intramembrane proteolysis: protease MucP can overcome mutations in the AlgO periplasmic protease to restore alginate production in nonmucoid revertants. J. Bacteriol. 200:e00215–18. doi: 10.1128/JB.00215-18
Deutscher, J., Francke, C., and Postma, P. W. (2006). How phosphotransferase system-related protein phosphorylation regulates carbohydrate metabolism in bacteria. Microbiol. Mol. Biol. Rev. 70, 939–1031. doi: 10.1128/MMBR.00024-06
Enos-Berlage, J. L., and Downs, D. M. (1997). Mutations in sdh (succinate dehydrogenase genes) alter the thiamine requirement of Salmonella typhimurium. J. Bacteriol. 179, 3989–3996. doi: 10.1128/jb.179.12.3989-3996.1997
Ertesvåg, H. (2015). Alginate-modifying enzymes: biological roles and biotechnological uses. Front. Microbiol. 6:523. doi: 10.3389/fmicb.2015.00523
Ertesvåg, H., Erlien, F., Skjåk-Bræk, G., Rehm, B. H., and Valla, S. (1998). Biochemical properties and substrate specificities of a recombinantly produced Azotobacter vinelandii alginate lyase. J. Bacteriol. 180, 3779–3784.
Ertesvåg, H., Høidal, H. K., Hals, I. K., Rian, A., Doseth, B., and Valla, S. (1995). A family of modular type mannuronan C-5-epimerase genes controls alginate structure in Azotobacter vinelandii. Mol. Microbiol. 16, 719–731. doi: 10.1111/j.1365-2958.1995.tb02433.x
Ertesvåg, H., and Skjåk-Bræk, G. (1999). “Modification of alginate using mannuronan C-5-epimerases,” in Methods in Biotechnology 10, Carbohydrate Biotechnology Protocols, ed C. Bucke (Totowa, NJ: Humana Press Inc.), 71–78. doi: 10.1007/978-1-59259-261-6_6
Ertesvåg, H., Sletta, H., Senneset, M., Sun, Y. Q., Klinkenberg, G., Konradsen, T. A., et al. (2017). Identification of genes affecting alginate biosynthesis in Pseudomonas fluorescens by screening a transposon insertion library. BMC Genomics 18:11. doi: 10.1186/s12864-016-3467-7
Ferla, M. P., and Patrick, W. M. (2014). Bacterial methionine biosynthesis. Microbiology 160, 1571–1584. doi: 10.1099/mic.0.077826-0
Gaviard, C., Broutin, I., Cosette, P., Dé, E., Jouenne, T., and Hardouin, J. (2018). Lysine succinylation and acetylation in Pseudomonas aeruginosa. J. Proteome Res. 17, 2449–2459. doi: 10.1021/acs.jproteome.8b00210
Gimmestad, M., Ertesvåg, H., Heggeset, T. M. B., Aarstad, O., Svanem, B. I. G., and Valla, S. (2009). Characterization of three new Azotobacter vinelandii alginate lyases, one of which is involved in cyst germination. J. Bacteriol. 191, 4845–4853. doi: 10.1128/JB.00455-09
Gimmestad, M., Steigedal, M., Ertesvåg, H., Moreno, S., Christensen, B. E., Espín, G., et al. (2006). Identification and characterization of an Azotobacter vinelandii Type I secretion system responsible for export of the AlgE-type mannuronan C5-epimerases. J. Bacteriol. 188, 5551–5560. doi: 10.1128/JB.00236-06
Goldberg, J. B., and Dahnke, T. (1992). Pseudomonas aeruginosa AlgB, which modulates the expression of alginate, is a member of the NtrC subclass of prokaryotic regulators. Mol. Microbiol. 6, 59–66. doi: 10.1111/j.1365-2958.1992.tb00837.x
Goldberg, J. B., and Ohman, D. E. (1987). Construction and characterization of Pseudomonas aeruginosa algB mutants: role of algB in high-level production of alginate. J. Bacteriol. 169, 1593–1602. doi: 10.1128/jb.169.4.1593-1602.1987
Gorin, P., and Spencer, J. F. (1966). Exocellular alginic acid from Azotobacter vinelandii. Can. J. Chem. 44, 993–998. doi: 10.1139/v66-147
Haug, A., Larsen, B., and Smidsrød, O. (1966). A study of the constitution of alginic acid by partial acid hydrolysis. Acta Chem. Scand. 20, 183–190. doi: 10.3891/acta.chem.scand.20-0183
Hay, I. D., Wang, Y., Moradali, M. F., Rehman, Z. U., and Rehm, B. H. (2014). Genetics and regulation of bacterial alginate production. Environ. Microbiol. 16, 2997–3011. doi: 10.1111/1462-2920.12389
Hou, L. L., Debru, A., Chen, Q. Q., Bao, Q. Y., and Li, K. W. (2019). AmrZ regulates swarming motility through cyclic di-GMP-dependent motility inhibition and controlling pel polysaccharide production in Pseudomonas aeruginosa PA14. Front. Microbiol. 10:1847. doi: 10.3389/fmicb.2019.01847
Leech, A. J., Sprinkle, A., Wood, L., Wozniak, D. J., and Ohman, D. E. (2008). The NtrC family regulator AlgB, which controls alginate biosynthesis in mucoid Pseudomonas aeruginosa, binds directly to the algD promoter. J. Bacteriol. 190, 581–589. doi: 10.1128/JB.01307-07
León, R., and Espín, G. (2008). flhDC, but not fleQ, regulates flagella biogenesis in Azotobacter vinelandii, and is under AlgU and CydR negative control. Microbiology 154, 1719–1728. doi: 10.1099/mic.0.2008/017665-0
López-Pliego, L., García-Ramírez, L., Cruz-Gómez, E. A., Domínguez-Ojeda, P., López-Pastrana, A., Fuentes-Ramírez, L. E., et al. (2018). Transcriptional study of the RsmZ-sRNAs and their relationship to the biosynthesis of alginate and alkylresorcinols in Azotobacter vinelandii. Mol. Biotechnol. 60, 670–680. doi: 10.1007/s12033-018-0102-7
Ma, S., Selvaraj, U., Ohman, D. E., Quarless, R., Hassett, D. J., and Wozniak, D. J. (1998). Phosphorylation-independent activity of the response regulators AlgB and AlgR in promoting alginate biosynthesis in mucoid Pseudomonas aeruginosa. J. Bacteriol. 180, 956–968.
Maleki, S., Almaas, E., Zotchev, S. B., Valla, S., and Ertesvåg, H. (2016). Alginate biosynthesis factories in Pseudomonas fluorescens: localization and correlation with alginate production level. Appl. Environ. Microbiol. 82, 2027–2036. doi: 10.1128/AEM.03114-15
Maleki, S., Hrudikova, R., Zotchev, S. B., and Ertesvåg, H. (2017). Identification of a new phosphatase enzyme potentially involved in sugar-phosphate stress response in Pseudomonas fluorescens. Appl. Environ. Microbiol. 83:e02361–e02316. doi: 10.1128/AEM.02361-16
Maleki, S., Mærk, M., Valla, S., and Ertesvåg, H. (2015). Mutational analyses of glucose dehydrogenase and glucose-6-phosphate dehydrogenase genes in Pseudomonas fluorescens reveal their effects on growth and alginate production. Appl. Environ. Microbiol. 81, 3349–3356. doi: 10.1128/AEM.03653-14
Marquet, A., Bui, B. T., and Florentin, D. (2001). Biosynthesis of biotin and lipoic acid. Vitam. Horm. 61, 51–101. doi: 10.1016/S0083-6729(01)61002-1
Martínez-Granero, F., Navazo, A., Barahona, E., Redondo-Nieto, M., Rivilla, R., and Martín, M. (2012). The Gac-Rsm and SadB signal transduction pathways converge on AlgU to downregulate motility in Pseudomonas fluorescens. PLoS ONE 7:e31765. doi: 10.1371/journal.pone.0031765
Martínez-Granero, F., Redondo-Nieto, M., Vesga, P., Martín, M., and Rivilla, R. (2014). AmrZ is a global transcriptional regulator implicated in iron uptake and environmental adaption in P. fluorescens F113. BMC Genomics 15:237. doi: 10.1186/1471-2164-15-237
Martínez-Salazar, J. M., Moreno, S., Nájera, R., Boucher, J. C., Espín, G., Soberón-Chávez, G., et al. (1996). Characterization of the genes coding for the putative sigma factor AlgU and its regulators MucA, MucB, MucC, and MucD in Azotobacter vinelandii and evaluation of their roles in alginate biosynthesis. J. Bacteriol. 178, 1800–1808. doi: 10.1128/jb.178.7.1800-1808.1996
Mengin-Lecreulx, D., van Heijenoort, J., and Park, J. T. (1996). Identification of the mpl gene encoding UDP-N-acetylmuramate: L-alanyl-gamma-D-glutamyl-meso-diaminopimelate ligase in Escherichia coli and its role in recycling of cell wall peptidoglycan. J. Bacteriol. 178, 5347–5352. doi: 10.1128/jb.178.18.5347-5352.1996
Muriel, C., Arrebola, E., Redondo-Nieto, M., Martínez-Granero, F., Jalvo, B., Pfeilmeier, S., et al. (2018). AmrZ is a major determinant of c-di-GMP levels in Pseudomonas fluorescens F113. Sci. Rep. 8:1979. doi: 10.1038/s41598-018-20419-9
Muriel-Millán, L. F., Moreno, S., Romero, Y., Bedoya-Peréz, L. P., Castañeda, M., Segura, D., et al. (2015). The unphosphorylated EIIA(Ntr) protein represses the synthesis of alkylresorcinols in Azotobacter vinelandii. PLoS ONE 10:e0117184. doi: 10.1371/journal.pone.0117184
Noguez, R., Segura, D., Moreno, S., Hernandez, A., Juarez, K., and Espín, G. (2008). Enzyme I NPr, NPr and IIA Ntr are involved in regulation of the poly-beta-hydroxybutyrate biosynthetic genes in Azotobacter vinelandii. J. Mol. Microbiol. Biotechnol. 15, 244–254. doi: 10.1159/000108658
Núñez, C., Leon, R., Guzman, J., Espín, G., and Soberon-Chavez, G. (2000). Role of Azotobacter vinelandii mucA and mucC gene products in alginate production. J. Bacteriol. 182, 6550–6556. doi: 10.1128/JB.182.23.6550-6556.2000
Núñez, C., Moreno, S., Soberon-Chavez, G., and Espín, G. (1999). The Azotobacter vinelandii response regulator AlgR is essential for cyst formation. J. Bacteriol. 181, 141–148.
Núñez, C., Peña, C., Kloeckner, W., Hernández-Eligio, A., Bogachev, A. V., Moreno, S., et al. (2013). Alginate synthesis in Azotobacter vinelandii is increased by reducing the intracellular production of ubiquinone. Appl. Microbiol. Biotechnol. 97, 2503–2512. doi: 10.1007/s00253-012-4329-0
Østgaard, K. (1992). Enzymatic microassay for the determination and characterization of alginates. Carbohydr. Polym. 19, 51–59. doi: 10.1016/0144-8617(92)90054-T
Park, J. T., and Uehara, T. (2008). How bacteria consume their own exoskeletons (turnover and recycling of cell wall peptidoglycan). Microbiol. Mol. Biol. Rev. 72, 211–227. doi: 10.1128/MMBR.00027-07
Pflüger-Grau, K., Chavarría, M., and de Lorenzo, V. (2011). The interplay of the EIIA(Ntr) component of the nitrogen-related phosphotransferase system (PTS(Ntr)) of Pseudomonas putida with pyruvate dehydrogenase. Biochim. Biophys. Acta 1810, 995–1005. doi: 10.1016/j.bbagen.2011.01.002
Prada-Ramírez, H. A., Pérez-Mendoza, D., Felipe, A., Martínez-Granero, F., Rivilla, R., Sanjuán, J., et al. (2016). AmrZ regulates cellulose production in Pseudomonas syringae pv. tomato DC3000. Mol. Microbiol. 99, 960–977. doi: 10.1111/mmi.13278
Prior, T. I., and Kornberg, H. L. (1988). Nucleotide sequence of fruA, the gene specifying enzyme IIfru of the phosphoenolpyruvate-dependent sugar phosphotransferase system in Escherichia coli K12. J. Gen. Microbiol. 134, 2757–2768. doi: 10.1099/00221287-134-10-2757
Qiu, D., Eisinger, V. M., Rowen, D. W., and Yu, H. D. (2007). Regulated proteolysis controls mucoid conversion in Pseudomonas aeruginosa. Proc. Natl. Acad. Sci. U.S.A. 104, 8107–8112. doi: 10.1073/pnas.0702660104
Quiroz-Rocha, E., Bonilla-Badía, F., Garcia-Aguílar, V., López-Pliego, L., Serrano-Román, J., Cocotl-Yañez, M., et al. (2017). Two-component system CbrA/CbrB controls alginate production in Azotobacter vinelandii. Microbiology 163, 1105–1115. doi: 10.1099/mic.0.000457
Rehm, B. H., Ertesvåg, H., and Valla, S. (1996). A new Azotobacter vinelandii mannuronan C-5-epimerase gene (algG) is part of an alg gene cluster physically organized in a manner similar to that in Pseudomonas aeruginosa. J. Bacteriol. 178, 5884–5889. doi: 10.1128/jb.178.20.5884-5889.1996
Remminghorst, U., and Rehm, B. H. (2006). Bacterial alginates: from biosynthesis to applications. Biotechnol. Lett. 28, 1701–1712. doi: 10.1007/s10529-006-9156-x
Sambrook, J., and Russell, D. W. (2001). Molecular Cloning: A Laboratory Manual. New York, NY: Cold Spring Harbor Laboratory Press.
Sampei, G., and Mizobuchi, K. (1989). The organization of the purL gene encoding 5'-phosphoribosylformylglycinamide amidotransferase of Escherichia coli. J. Biol. Chem. 264, 21230–21238.
Segura, D., and Espín, G. (1998). Mutational inactivation of a gene homologous to Escherichia coli ptsP affects poly-beta-hydroxybutyrate accumulation and nitrogen fixation in Azotobacter vinelandii. J. Bacteriol. 180, 4790–4798.
Setubal, J. C., dos Santos, P., Goldman, B. S., Ertesvåg, H., Espín, G., Rubio, L. M., Wood, D., et al. (2009). The genome sequence of Azotobacter vinelandii, an obligate aerobe specialized to support diverse anaerobic metabolic processes. J. Bacteriol. 191, 4534–4545. doi: 10.1128/JB.00504-09
Simon, R., Priefer, U., and Pühler, A. (1983). A broad host range mobilization system for in vivo genetic engineering: transposon mutagenesis in Gram negative bacteria. Biotechnology 1, 784–791. doi: 10.1038/nbt1183-784
Skjåk-Bræk, G., Donati, I., and Paoletti, S. (2015). “Alginate hydrogels: properties and applications,” in Polysaccharide Hydrogels: Characterization and Biomedical Applications, eds P. Matricardi, F. Alhaique, and T. Coviello (Boca Raton, FL: Pan Stanford Publishing Pte Ltd), 449–498. doi: 10.1201/b19751-14
Sprenger, G. A., Schorken, U., Wiegert, T., Grolle, S., de Graaf, A. A., Taylor, S. V., et al. (1997). Identification of a thiamin-dependent synthase in Escherichia coli required for the formation of the 1-deoxy-D-xylulose 5-phosphate precursor to isoprenoids, thiamin, and pyridoxol. Proc. Natl. Acad. Sci. U.S.A. 94, 12857–12862. doi: 10.1073/pnas.94.24.12857
Steigedal, M., Sletta, H., Moreno, S., Mærk, M., Christensen, B. E., Bjerkan, T., et al. (2008). The Azotobacter vinelandii AlgE mannuronan C-5-epimerase family is essential for the in vivo control of alginate monomer composition and for functional cyst formation. Environ. Microbiol. 10, 1760–1770. doi: 10.1111/j.1462-2920.2008.01597.x
Svanem, B. I. G., Skjåk-Bræk, G., Ertesvåg, H., and Valla, S. (1999). Cloning and expression of three new Azotobacter vinelandii genes closely related to a previously described gene family encoding mannuronan C-5-epimerases. J. Bacteriol. 181, 68–77.
Tart, A. H., Blanks, M. J., and Wozniak, D. J. (2006). The AlgT-dependent transcriptional regulator AmrZ (AlgZ) inhibits flagellum biosynthesis in mucoid, nonmotile Pseudomonas aeruginosa cystic fibrosis isolates. J. Bacteriol. 188, 6483–6489. doi: 10.1128/JB.00636-06
Urtuvia, V., Maturana, N., Acevedo, F., Peña, C., and Díaz-Barrera, A. (2017). Bacterial alginate production: an overview of its biosynthesis and potential industrial production. World J. Microbiol. Biotechnol. 33:198. doi: 10.1007/s11274-017-2363-x
Velázquez, F., Pflüger, K., Cases, I., De Eugenio, L. I., and de Lorenzo, V. (2007). The phosphotransferase system formed by PtsP, PtsO, and PtsN proteins controls production of polyhydroxyalkanoates in Pseudomonas putida. J. Bacteriol. 189, 4529–4533. doi: 10.1128/JB.00033-07
Wentzel, A., Bruheim, P., Øverby, A., Jakobsen, Ø. M., Sletta, H., Omara, W. A., et al. (2012). Optimized submerged batch fermentation strategy for systems scale studies of metabolic switching in Streptomyces coelicolor A3(2). BMC Syst. Biol. 6:59. doi: 10.1186/1752-0509-6-59
Wilson, K. J., Sessitsch, A., Corbo, J. C., Giller, K. E., Akkermans, A. D., and Jefferson, R. A. (1995). Beta-Glucuronidase (GUS) transposons for ecological and genetic studies of Rhizobia and other Gram-negative bacteria. Microbiology 141, 1691–1705. doi: 10.1099/13500872-141-7-1691
Winsor, G. L., Griffiths, E. J., Lo, R., Dhillon, B. K., Shay, J. A., and Brinkman, F. S. (2016). Enhanced annotations and features for comparing thousands of Pseudomonas genomes in the Pseudomonas genome database. Nucleic Acids Res. 44, D646–D653. doi: 10.1093/nar/gkv1227
Wood, L. F., Leech, A. J., and Ohman, D. E. (2006). Cell wall-inhibitory antibiotics activate the alginate biosynthesis operon in Pseudomonas aeruginosa: roles of sigma (AlgT) and the AlgW and Prc proteases. Mol. Microbiol. 62, 412–426. doi: 10.1111/j.1365-2958.2006.05390.x
Wozniak, D. J., and Ohman, D. E. (1994). Transcriptional analysis of the Pseudomonas aeruginosa genes algR, algB, and algD reveals a hierarchy of alginate gene expression which is modulated by algT. J. Bacteriol. 176, 6007–6014. doi: 10.1128/jb.176.19.6007-6014.1994
Wozniak, D. J., Sprinkle, A. B., and Baynham, P. J. (2003). Control of Pseudomonas aeruginosa algZ expression by the alternative sigma factor AlgT. J. Bacteriol. 185, 7297–7300. doi: 10.1128/JB.185.24.7297-7300.2003
Yang, M. K., Wang, Y., Chen, Y., Cheng, Z. Y., Gu, J., Deng, J. Y., et al. (2015). Succinylome analysis reveals the involvement of lysine succinylation in metabolism in pathogenic Mycobacterium tuberculosis. Mol. Cell. Proteomics 14, 796–811. doi: 10.1074/mcp.M114.045922
Keywords: alginate, Azotobacter vinelandii, amrZ, fruA, algB, medium supplements
Citation: Mærk M, Jakobsen ØM, Sletta H, Klinkenberg G, Tøndervik A, Ellingsen TE, Valla S and Ertesvåg H (2020) Identification of Regulatory Genes and Metabolic Processes Important for Alginate Biosynthesis in Azotobacter vinelandii by Screening of a Transposon Insertion Mutant Library. Front. Bioeng. Biotechnol. 7:475. doi: 10.3389/fbioe.2019.00475
Received: 07 October 2019; Accepted: 23 December 2019;
Published: 17 January 2020.
Edited by:
Bruce Ramsay, Queen's University, CanadaReviewed by:
Alvaro Diaz, Pontificia Universidad Católica de Valparaíso, ChileMIguel Castañeda, Meritorious Autonomous University of Puebla, Mexico
Copyright © 2020 Mærk, Jakobsen, Sletta, Klinkenberg, Tøndervik, Ellingsen, Valla and Ertesvåg. This is an open-access article distributed under the terms of the Creative Commons Attribution License (CC BY). The use, distribution or reproduction in other forums is permitted, provided the original author(s) and the copyright owner(s) are credited and that the original publication in this journal is cited, in accordance with accepted academic practice. No use, distribution or reproduction is permitted which does not comply with these terms.
*Correspondence: Helga Ertesvåg, aGVsZ2EuZXJ0ZXN2YWdAbnRudS5ubw==
†Present address: Mali Mærk, PHARMAQ AS, Overhalla, Norway
Øyvind M. Jakobsen, NTNU Social Research, Trondheim, Norway
‡Deceased