Stannic Oxide Nanoparticle Regulates Proliferation, Invasion, Apoptosis, and Oxidative Stress of Oral Cancer Cells
- 1Department of Stomatology, China-Japan Union Hospital, Jilin University, Changchun, China
- 2VIP Integrated Department, School and Hospital of Stomatology, Jilin University, Changchun, China
- 3Department of Pediatrics, The Second Hospital of Jilin University, Changchun, China
Objective: To explore the effects of SnO2 nanoparticles (NPs) on proliferation, invasion, apoptosis, and oxidative stress of oral cancer.
Methods: SnO2 NPs were prepared and characterized. Oral cancer cell lines CAL-27 and SCC-9 were cultured in vitro. We detected the effects of various concentrations of SnO2 NPs (0, 5, 25, 50, 100, 200 μg/mL) on the proliferation of oral cancer cells, and observed the morphological changes, and measured the cells ability of migration, invasion and apoptosis condition, and the levels of oxidative stress were measured by detecting malondialdehyde (MDA) and reactive oxygen species (ROS). Besides, we also measured the changes of mRNA and protein levels of factors related to cell proliferation, migration, invasion, apoptosis, and oxidative stress.
Results: SnO2 NPs inhibited the proliferation of oral cancer cells in a concentration-dependent manner (all P < 0.05). And SnO2 NPs treatment could reduce the migration and invasion ability of cells (all P < 0.05), induce apoptosis, and those effects were better when treated for 48 h than 24 h (all P < 0.05). And SnO2 NPs could induce oxidative stress in cells (all P < 0.05). Besides, the concentrations of cyclin-D1, C-myc, matrix MMP-9, and MMP-2 in SnO2 NPs treated group was decreased (all P < 0.05), and the expression levels of cleaved Caspase-3, cleaved Caspase-9, and Cytochrome C were increased (all P < 0.05).
Conclusion: In the present study, we found that SnO2 NPs could play a cytotoxic role in oral cancer cells, and inhibit cell proliferation, migration, and invasion, and induce oxidative stress and apoptosis, which suggests that SnO2 NPs may have the effects of anti-oral cancer. However, a more in-depth study is needed to determine its roles.
Introduction
Oral cancer is one of the most common human malignant tumors in the world (Shiga et al., 2011; Omori et al., 2020). Despite progress in cancer treatment, for patients with oral cancer, the 5-year survival rate is still less than 50% (Cristaldi et al., 2019). At present, the available treatments for oral cancer mainly include surgery, radiotherapy, and chemotherapy. After surgical treatment, it is easy to relapse, and the prognosis is poor. Although chemotherapy has effects to some extent, the tolerance and drug resistance of chemotherapy drugs are still severe problems faced by oral cancer patients. Therefore, it is necessary to find and develop a more effective and safer chemical molecular drug for treating the disease.
With the continuous development of nanomedicines, nanoparticles are now considered as a potential cancer treatment with bright future (Ding et al., 2019; Feng et al., 2019; Jiang et al., 2019; Li et al., 2019; Qi et al., 2019; Sun et al., 2019; Wang et al., 2019; Xu et al., 2019; Xiong et al., 2020; Yang et al., 2020). The nanoparticle is small and can facilitate the various biological reactions (Behzadi et al., 2017; Chen et al., 2017a). Tin oxide (SnO2) is an essential n-type wide bandgap (3.6 eV) semiconductor material. Currently, SnO2 nanoparticles are being used in different fields, including solar cells, gas sensors, catalysts, lithium-ion batteries, solid-state chemistry, etc. (Cheng et al., 2014). Increasingly, SnO2 NP has more and more extensive use in the field of biomedicine, and studies have shown that SnO2 NP has antibacterial and antioxidant activity (Vidhu and Philip, 2015). For examples, in cell-related research, Tammina et al. (2017) observed that SnO2 NP can exert anti-human colorectal cancer and anti-lung cancer effects through cytotoxicity; Roopan et al. (2015) reported the cytotoxic response of SnO2 NP to hepatocellular carcinoma (Roopan et al., 2015); Ahamed et al. (2018) demonstrated that SnO2 NP could induce breast cancer cell toxicity through oxidative stress. In animal-related research, Yang et al. (2017) found that SnO2 NP had an inhibitory effect on the weight gain of newborn rats. However, there is still little research on the mechanism of toxic effects of SnO2 NP at the cellular and molecular levels. Therefore, before SnO2 NP can be applied in the field of biomedicine, in-depth research on the possible biological roles of SnO2 is essential (Dong et al., 2012).
Since there is no relevant research on the role of SnO2 NP in the initialization and progression of oral cancer, two oral cancer cells CAL-27 and SCC-9 were selected in this study to explore the possibility of SnO2 NP in treating oral cancer by observing its effect on proliferation, invasion, apoptosis, and oxidative stress of the cells.
Materials and Methods
Synthesis of SnO2 NP
SnO2 NP was synthesized by the sol-gel method, see reference for details (Aziz et al., 2013). First, 5.0 g of SnCl4⋅5H2O was dissolved in 200.0 mL of ethanol (C2H5OH) and stirred for 30 min by a magnetic stirrer. Then, 6.0 mL of acetylacetone was added to hydrolyze SnO2, and the solution was refluxed through an 80°C condenser. After that, the solution was further dried for 3 h in a 90°C hot air oven (Thermo Fisher Scientific, United States) to obtain SnO2 gel, which was then calcined at 400-500°C for 2 h to obtain SnO2 NP.
Characterizations of SnO2 NP
The absorption spectrum of SnO2 NP was measured using a spectrometer (UV-1800, Shimadzu, Japan) at a resolution of 0.5 nm and a wavelength range of 300–900 nm. The structure of SnO2 NP was analyzed by using Ku-Cα ray powder X-ray diffraction (XRD) with a wavelength of 1.54056 Å, and the XRD spectrum in the surrounding environment was recorded at a scan rate of 0.02°/s in the 2θ range of 20–80°. Besides, the characteristics of SnO2 NP were observed with a transmission electron microscope (TEM).
Cell Culture
Human oral cancer cell lines CAL-27 and SCC-9 were purchased from ATCC (United States). The cells were incubated in DMEM medium containing 10% fetal bovine serum (Gibco, United States), 100 U/mL penicillin, and 100 μg/mL streptomycin (Invitrogen, United States), with 5% carbon dioxide (CO2) at 37°C.
Experimental Grouping and Treatment of Cells Exposed to SnO2 NP
DMEM was used to prepare a SnO2 NP storage solution (1.0 mg/mL), which was then diluted to an appropriate concentration (5–200 μg/mL). Before the experiment, SnO2 NP of different concentrations was placed in the ultrasonic bath with 40 W for 15 min at room temperature, which could maintain nanoparticles being evenly distributed in DMEM and prevent aggregation. The cells were divided into the control group (DMEM group, 0 μg/mL) and experimental groups (SnO2 NP concentration was 5, 25, 50, 100, and 200 μg/mL), and two experimental treatment time points of 24 and 48 h were set.
Detection of Cell Proliferation Behaviors
Cell Counting Kit-8 (CCK-8, Donjindo, Japan) was used for the measurement. CAL-27 and SCC-9 cells were inoculated into 96-well plates at a density of 1 × 104 cells/well. After 24 or 48 h of treatment for control and experimental groups, 10 μL of CCK-8 solution was added to each well for another 1 h of incubation at 37°C. After that, the absorbance at 450 nm (Absorbance, A) was measured with a multifunctional microplate reader (Bio-Rad, Untied States). A blank well (only containing medium and CCK-8 solution) was set in the experiment to counteract background interference. The formula for calculating cell proliferation activity is as follows (Chen et al., 2017b; Li et al., 2018):
Each group has four replicates.
Cell Morphology Observation
The morphological changes of cells in treatment groups were determined by a phase-contrast inverted microscope (Leica Microsystems Inc., United States).
Cell Scratch Test
First, cells were prepared for the control and experimental groups respectively, and then CAL-27 and SCC-9 cells were seeded on 6-well plates at a density of 2 × 105 cell/well and cultured for 24 h. Straight lines parallel to the cell layer were then drawn using a sterile pipette tip. After that, the control and experimental groups were treated accordingly, and the cells were photographed at 0 and 24 h with the use of a phase-contrast inverted microscope. Finally, ImageJ was used to measure the width of the scratch area and calculate the cell migration rate. The formula is as follows: cell migration rate = (scratch width at 0 h−scratch width at 24 h) / scratch width at 0 h × 100%.
Cell Invasion Experiment
Transwell was used for the cell invasion test (Chen et al., 2017b). First, cells were prepared for the control group and the experimental group. Then CAL-27 (6 × 104 cell/well) and SCC-9 cells (8 × 104 cell/well) inoculated into 200 μL of serum-free medium were plated in the upper chamber coated with Matrigel, and 500 μL of medium containing 10% fetal calf serum was added in the lower chamber. The cells were incubated at 37°C for 24 h, after which the cells on the membrane surface were fixed with 4% paraformaldehyde, stained with 0.1% crystal violet, and placed in a phase-contrast inverted microscope for cell counting.
Detection of Oxidative Stress Indicators
The oxidative stress was evaluated by determining the levels of malondialdehyde (MDA) and reactive oxygen species (ROS). According to the instructions, the MDA detection kit for lipid peroxidation (S0131, Biyuntian, China) and the active oxygen detection kit (S0033, Biyuntian, China) were used for detection, respectively.
Western Blot to Detect Protein Expression
The cells after the experimental treatment were washed with pre-cooled PBS and lysed with a strong RIPA lysis buffer (Biyuntian, China) containing protease inhibitors, which was subsequently centrifuged at 16,000 g at 4°C for 20 min to retain the supernatant. Then, the protein quantification was measured by the BCA protein concentration detection kit (Biyuntian, China). After that, the protein was denatured by heating at 98°C for 10 min, and separated by SDS-PAGE gel electrophoresis. After electrophoresis, proteins on the gel were transferred to PVDF membrane (Millipore, United States), and the membrane was then blocked with a blocking solution (Biyuntian, China) for 1 h after the transfer. Subsequently, the membrane was incubated overnight at 4°C after the addition of the corresponding primary antibody. On the next day, the membrane was washed with TBST three times, and the secondary antibody conjugated with the corresponding horseradish peroxidase (HRP) was then incubated at room temperature for 2 h. After that, the membrane was washed three times with TBST, and the Western blot was developed with ECL color developing solution (Biyuntian, China). Finally, the grayscale analysis was performed with Photoshop CS6. The primary antibodies used in this experiment were cleaved Caspase-3 (ab2302 17 kDa), Caspase-3 (ab13847 17 kDa), cleaved Caspase-9 (ab2324 46 kDa), Caspase-9 (ab202068 46 kDa), matrix metalloproteinase 9 (Matrix metalloproteinase-9, MMP-9) (ab38898 92 kDa), MMP-2 (ab97779 74 kDa), G1/S-specific cyclin-D1 (Cyclin D1, CCND1) (ab134175 34 kDa), c-myc (Ab32072 57 kDa), Cytochrome C (ab133504 14 kDa), and β-actin (ab227387 42 kDa), of which β-actin serves as an internal reference protein.
Flow Cytometry to Detect Apoptosis
The flow cytometer Annexin V-FITC/PI double staining method was used for the detection. CAL-27 and SCC-9 cells were seeded on 6-well plates at a density of 2 × 105 cells/well. After treatment for 24 or 48 h in the control and experimental groups, cells were digested with trypsin digestion solution without EDTA and centrifuged at 1,000 rpm for 5 min at room temperature to retain the cell pellet. Then, the cells washed with 1 mL of pre-chilled PBS were centrifuged at 3,000 rpm for 5 min at room temperature to retain the cell pellet, which was followed by PBS washing twice. After that, the cell pellet was added with 500 μL of binding buffer to resuspend, 10 μL PI and 5 μL Annexin V-FITC were added, and cultured in the dark. After incubation, the apoptosis was immediately analyzed using flow cytometry (BD Biosciences, United States).
Real-Time Fluorescence Quantitative PCR to Detect Expression Level of Target Genes
The cells after the experimental treatment were washed with pre-cooled PBS, and the total RNA was extracted from the cell line using TRIzol® reagent (Invitrogen, United States). After that, the concentration and purity of the RNA were measured with a multifunctional microplate reader. According to the instructions of the reverse transcription kit (Takara, Japan), 1 μg of total RNA was used for PCR to obtain cDNA. Then, the SYBR Green kit (Takara, Japan) and target gene primers or internal reference gene (β-actin) primers were used to perform real-time fluorescence quantitative PCR (RT-qPCR). Finally, the expression cycle Ct value of each gene was measured, and the relative expression level was calculated according to this formula 2–ΔΔCt. Primer sequences are shown in Table 1.
Statistical Analysis
All experiments were independently repeated three times. The measurement data obtained in the experiment are expressed as mean ± standard deviation, and software SPSS 18.0 was used for statistical analysis. Student’s t-test was used for comparison between the two groups, and one-way ANOVA was used for comparison within the group when considering the single factors. P < 0.05 was considered statistically significant.
Results
Physicochemical Characterization of SnO2 NP
As shown in Figure 1A, the absorption spectrum of SnO2 NP ranges from 200 to 700 nm. The formula calculates the absorption coefficient (α) of SnO2 NP: α = A/d (A: absorbance, d: cuvette thickness) (Khan et al., 2014). According to the formula: (αhν) = A (hν−Eg) (where hν is the photon energy, A is a constant that does not depend on the photon energy), the absorption coefficient α was used to make Tauc plots (Khan et al., 2017). The analysis found that the energy band gap of SnO2 NP is 3.50 eV, which is consistent with other reports (Gattu et al., 2015; Mohanta et al., 2017). It is also known that the energy band gap of semiconductor NP plays a critical role in the toxicity of tumor cells (Rasmussen et al., 2010).
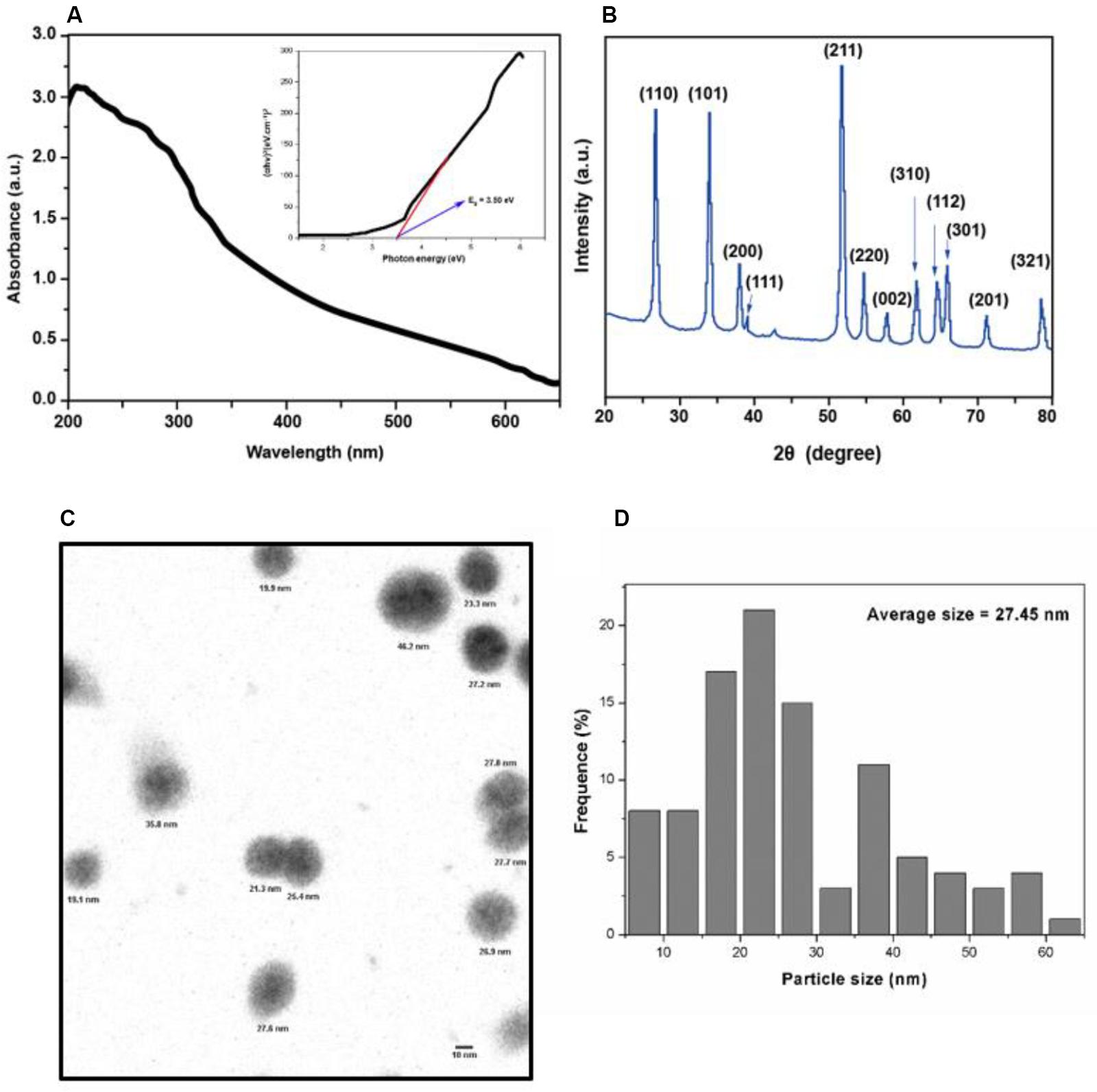
Figure 1. Characterization of SnO2 NP. (A) Absorption spectra of SnO2 NP. Figure inset represents the bandgap energy of SnO2 NP. (B) XRD spectra of SnO2 NP. (C,D) TEM micrograph of SnO2 NP and a histogram of the size distribution of SnO2 NP.
X-ray diffraction analyzed the crystal structure of SnO2 NP. As shown in Figure 1B, all peaks in XRD are related to the rutile structure of SnO2 (JCPDS No. 41-1445). According to the Scherrer equation: d = Kλ / βCos θ (where K = 0.9 is the shape factor, λ is the X-ray wavelength of Cu Kα rays (1.54 Å), θ is the Bragg diffraction angle, and β is the diffraction line at its maximum intensity (broadness) measured at half a radian), it is found that the average size of SnO2 NP is about 13 nm, and the XRD results are consistent with the results reported by other studies (Chen et al., 2014).
The appearance of SnO2 NP was detected by TEM and shown in Figure 1C. The average TEM size of SnO2 NP was calculated based on more than 300 nanoparticles and found that the average TEM size was 27.45 ± 13.4 nm (Figure 1D).
SnO2 NP Inhibit Proliferation of Oral Cancer Cells
Figure 2A showed that compared with the control group, SnO2 NP induced a significant decrease in the proliferation activity of CAL-27 cells in a concentration-dependent manner (50, 100, and 200 μg/mL) (all P < 0.05), and the inhibition effect was more significant at 48 h than that at 24 h. When the concentration of SnO2 NP was lower than 50 μg/mL (5, 25 μg/mL), there was no significant effect on cell proliferation activity (all P > 0.05). Figure 2B showed that compared with the control group, SnO2 NP induced a significant decrease in the proliferation activity of SCC-9 cells in a concentration-dependent manner (25, 50, 100, and 200 μg/mL) (all P < 0.05), and the treatment at 48 h has stronger effects on cell proliferation activity than that at 24 h. When the concentration of SnO2 NP was less than 5 μg/mL, there was no significant effect on cell proliferation activity (P > 0.05). Since SnO2 can exert toxicity on oral cancer cells when the concentration greater than 50, 100 μg/mL was used as the treatment concentration in the SnO2 NP group.
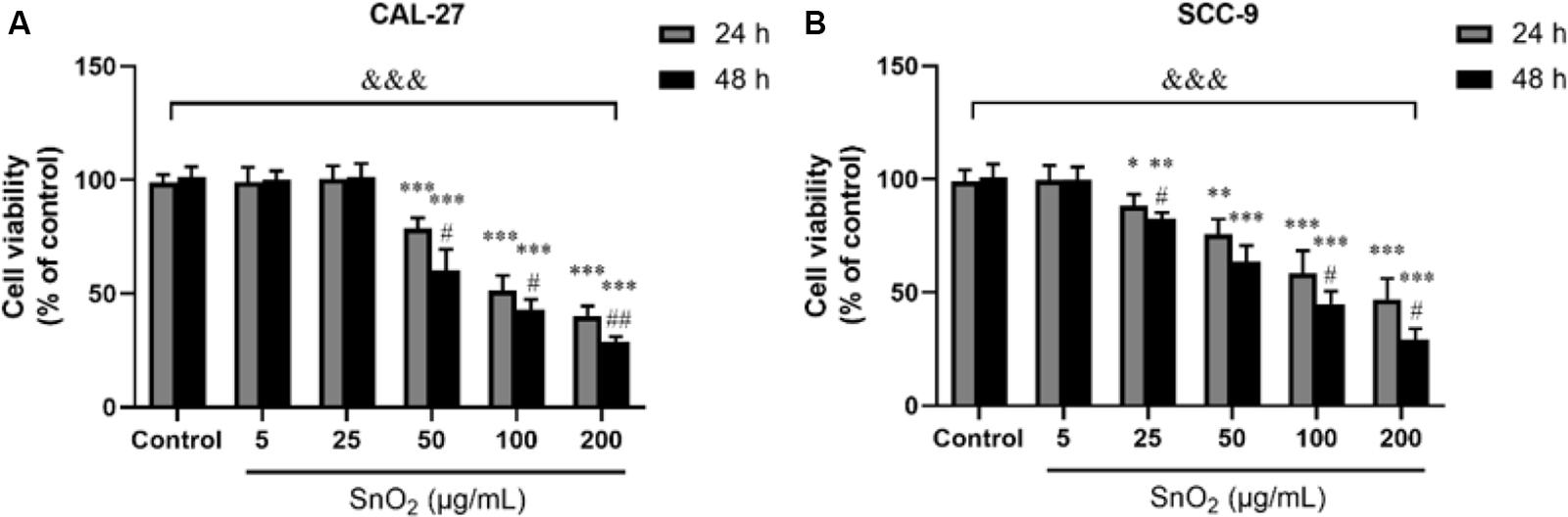
Figure 2. SnO2 NP inhibited cell viability in oral cancer cells. (A,B) The viability of CAL-27 and SCC-9 cells was measured after treatment of different concentrations of SnO2 NP at 24 or 48 h. n = 4, compared with control group, *P < 0.05, **P < 0.01, ***P < 0.001; compared with 24 h treated group, #P < 0.05, ##P < 0.01; compared in groups, &&&P < 0.001.
SnO2 NP Changed Density Morphology of Oral Cancer Cells
Figure 3A showed that compared with the control group, the treatment of 100 μg/mL SnO2 NP can significantly reduce the CAL-27 cell density, and the cell density was changed more significantly with the treatment of SnO2 NP at 48 h than that at 24 h. As shown in Figure 3B, the changes caused by SnO2 NP on SCC-9 cells are consistent with the results from CAL-27 cells.
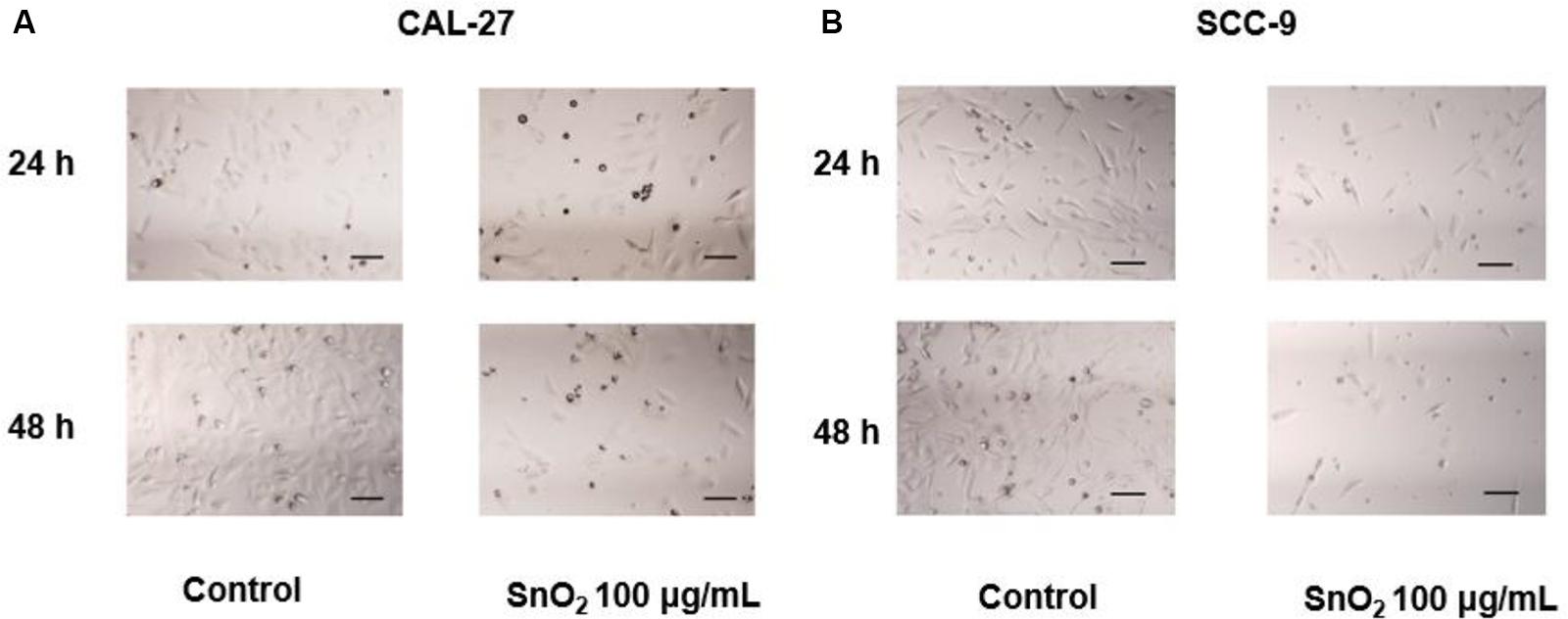
Figure 3. SnO2 NP alters cell density and morphology in oral cancer cells (100×, 100 μm). (A,B) The changes in cell density and morphology in CAL-27 and SCC-9 cells.
SnO2 NP Inhibited Migration and Invasion of Oral Cancer Cells
As shown in Figures 4A,B, compared with the control group, SnO2 NP treatment significantly suppressed the migration of CAL-27 and SCC-9 cells (both P < 0.05), and cells treated with SnO2 NP for 48 h has a weaker migration ability than those treated for 24 h (all P < 0.05).
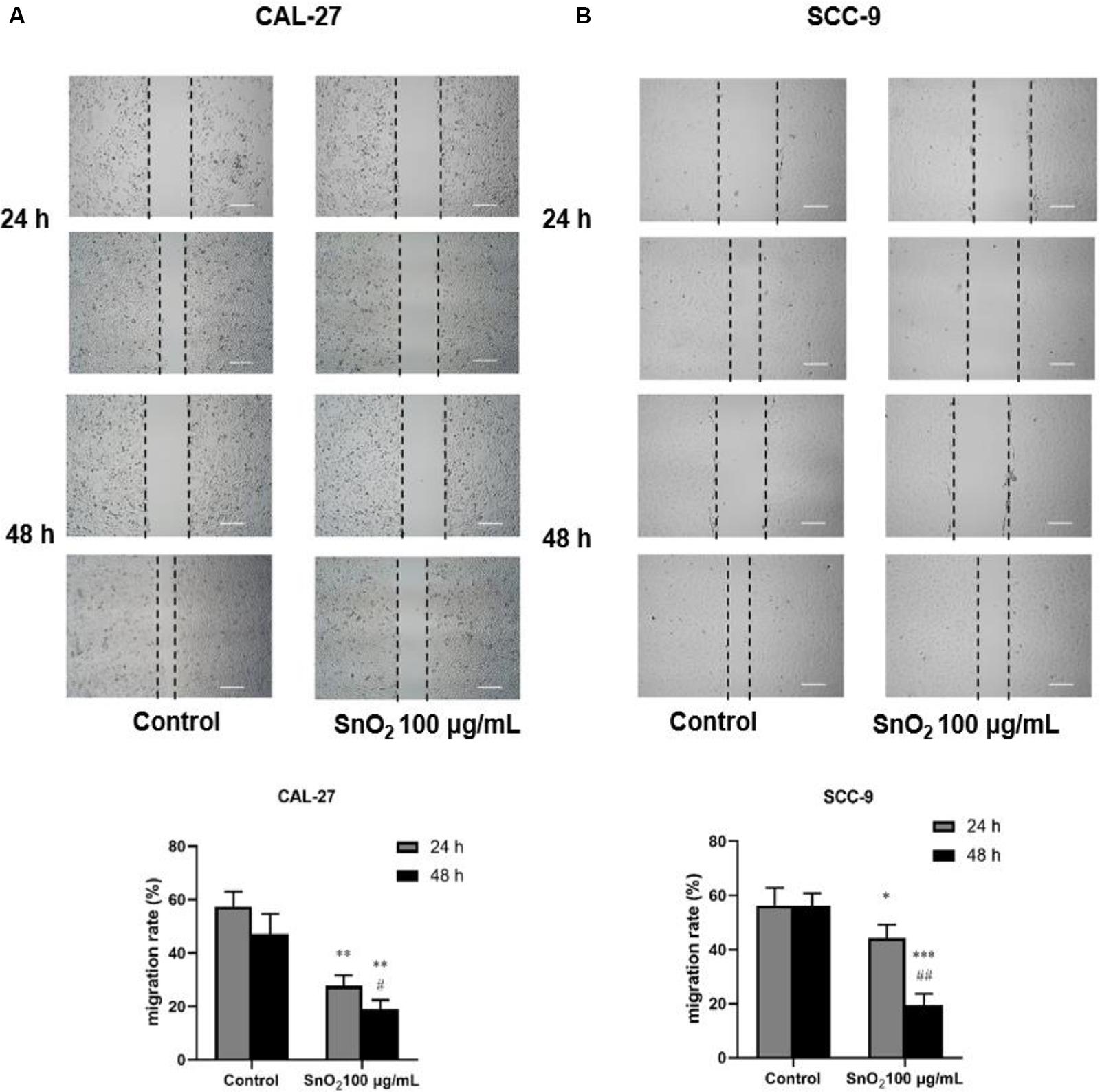
Figure 4. SnO2 NP inhibited cell migration in oral cancer cells (50×, 200 μm). (A,B) The ability of cell migration was measured in CAL-27 and SCC-9 cells after treatment of 0 or 100 μg/mL SnO2 NP at 24 or 48 h. Compared with control group, *P < 0.05, **P < 0.01, ***P < 0.001; compared with 24 h treated group, #P < 0.05, ##P < 0.01.
As shown in Figures 5A,B, compared with the control group, SnO2 NP treatment significantly reduced the invasive ability of CAL-27 and SCC-9 cells (both P < 0.05), and the effect on cell invasion was more significant with the treatment of SnO2 NP at 48 h than at 24 h (P < 0.05).
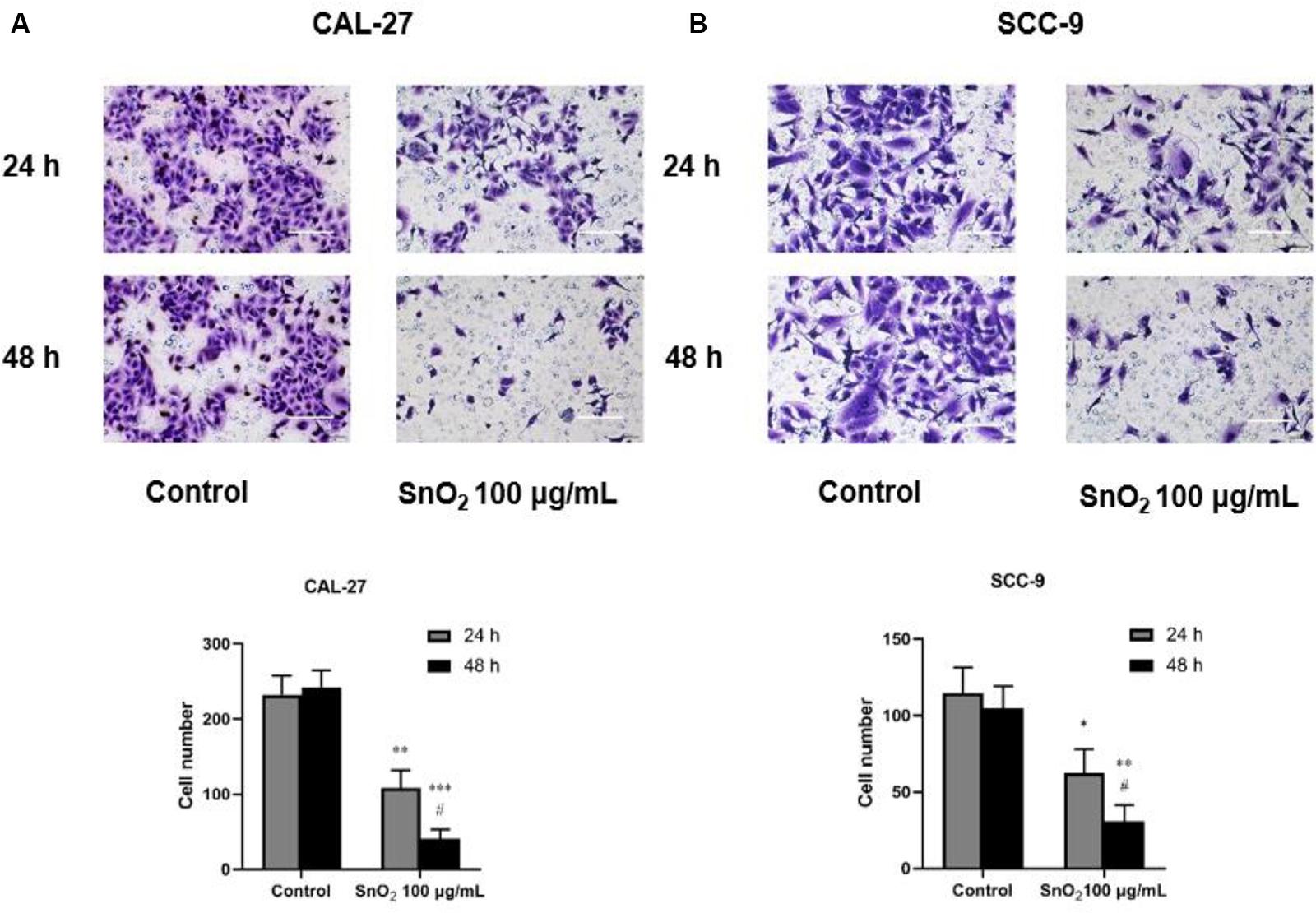
Figure 5. SnO2 NP inhibited cell invasion of oral cancer cells (100×, 100 μm). (A,B) The ability of cell invasion was measured in CAL-27 and SCC-9 cells after treatment of 0 or 100 μg/mL SnO2 NP at 24 or 48 h. Compared with control group, *P < 0.05, **P < 0.01, ***P < 0.001; compared with 24 h treated group, #P < 0.05.
SnO2 NP Induced Apoptosis of Oral Cancer Cells
Figure 6A indicated that compared with the control group, 100 μg/mL SnO2 NP can significantly induce apoptosis of CAL-27 cells (P < 0.05), and the cells treated with SnO2 NP at 48 h have more significant change than that at 24 h. As shown in Figure 6B, the apoptosis induced by SnO2 NP on SCC-9 cells was consistent with results with CAL-27 cells (P < 0.05).
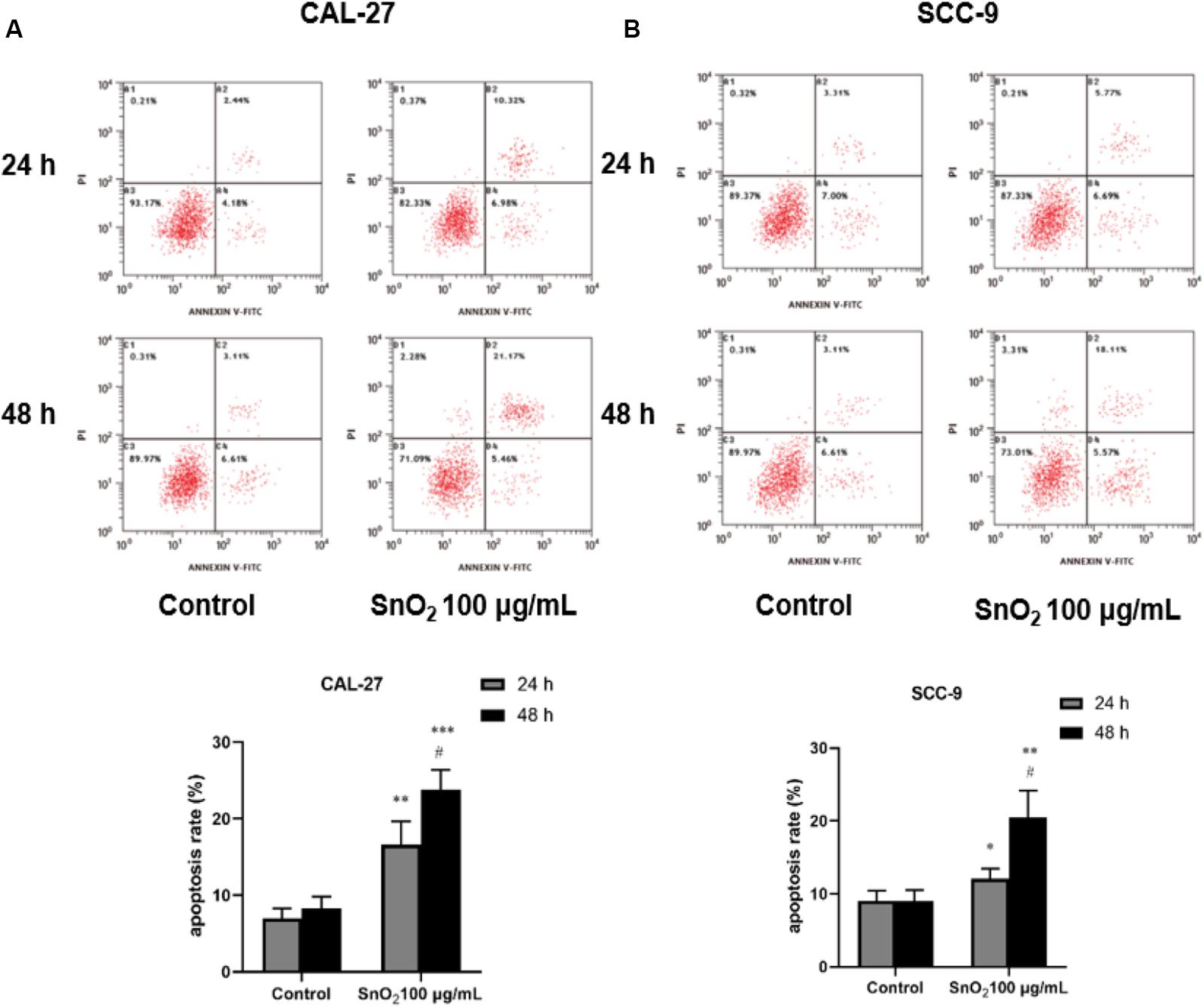
Figure 6. SnO2 NP induced cell apoptosis in oral cancer cells. (A,B) Apoptosis was determined in CAL-27 and SCC-9 cells after treatment of 0 or 100 μg/mL SnO2 NP at 24 or 48 h. Compared with control group, *P < 0.05, **P < 0.01, ***P < 0.001; compared with 24 h treated group, #P < 0.05.
SnO2 NP Induced Oxidative Stress in Oral Cancer Cells
As shown in Figures 7A,C, CAL-27 cells treated with 100 μg/mL SnO2 NP, compared with those of the control group, significantly increased the levels of oxidative stress-related factors MDA and ROS (both P < 0.05), and the cells treated with at 48 h changed more significant than at 24 h. As shown in Figures 7B,D, the SnO2 NP-induced oxidative stress of SCC-9 cells was consistent with results from CAL-27 cells (P < 0.05).
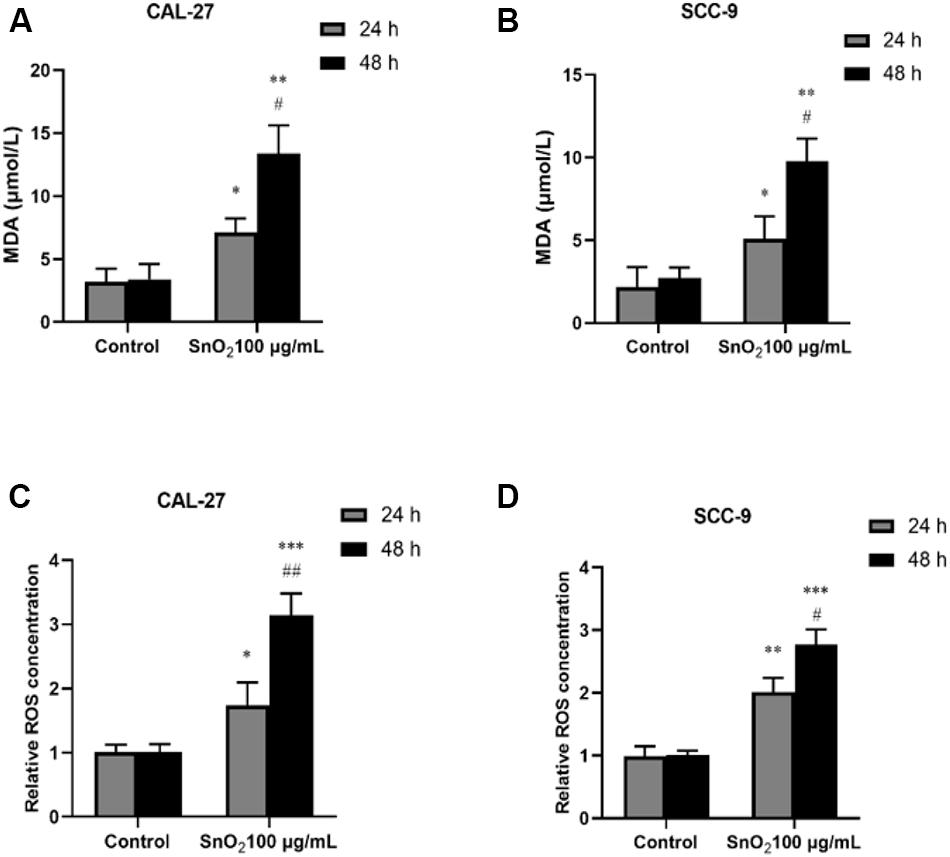
Figure 7. SnO2 NP induced cell apoptosis in oral cancer cells. (A,B) MDA levels were determined in CAL-27 and SCC-9 cells after treatment of 0 or 100 μg/mL SnO2 NP at 24 or 48 h. (C,D) The effects of 0 or 100 μg/mL SnO2 NP on ROS concentrations were tested in CAL-27 and SCC-9 cells at 24 or 48 h. Compared with control group, *P < 0.05, **P < 0.01, ***P < 0.001; compared with 24 h treated group, #P < 0.05, ##P < 0.05.
SnO2 NP Altered mRNA and Protein Expression of Oral Cancer Cell Proliferation, Migration, Invasion, Apoptosis, and Oxidative Stress-Related Genes
CAL-27 cells are more sensitive to SnO2 NP stimulation in terms of migration, invasion, and apoptosis, and were selected in the study for the Western blot and RT-qPCR experiments.
As shown in Figures 8A,B, compared with the control group, 100 μg/mL SnO2 NP treatment at both 24 and 48 h could not only significantly inhibit the expression of proliferation-related factors CCND1 and c-myc in CAL-27 cells to varying degrees, but also decrease the mRNA levels of migration and invasion related factors MMP-2 and MMP-9 (both P < 0.05), to promote the mRNA expression of oxidative stress-related factors Cytochrome C (both P < 0.05).
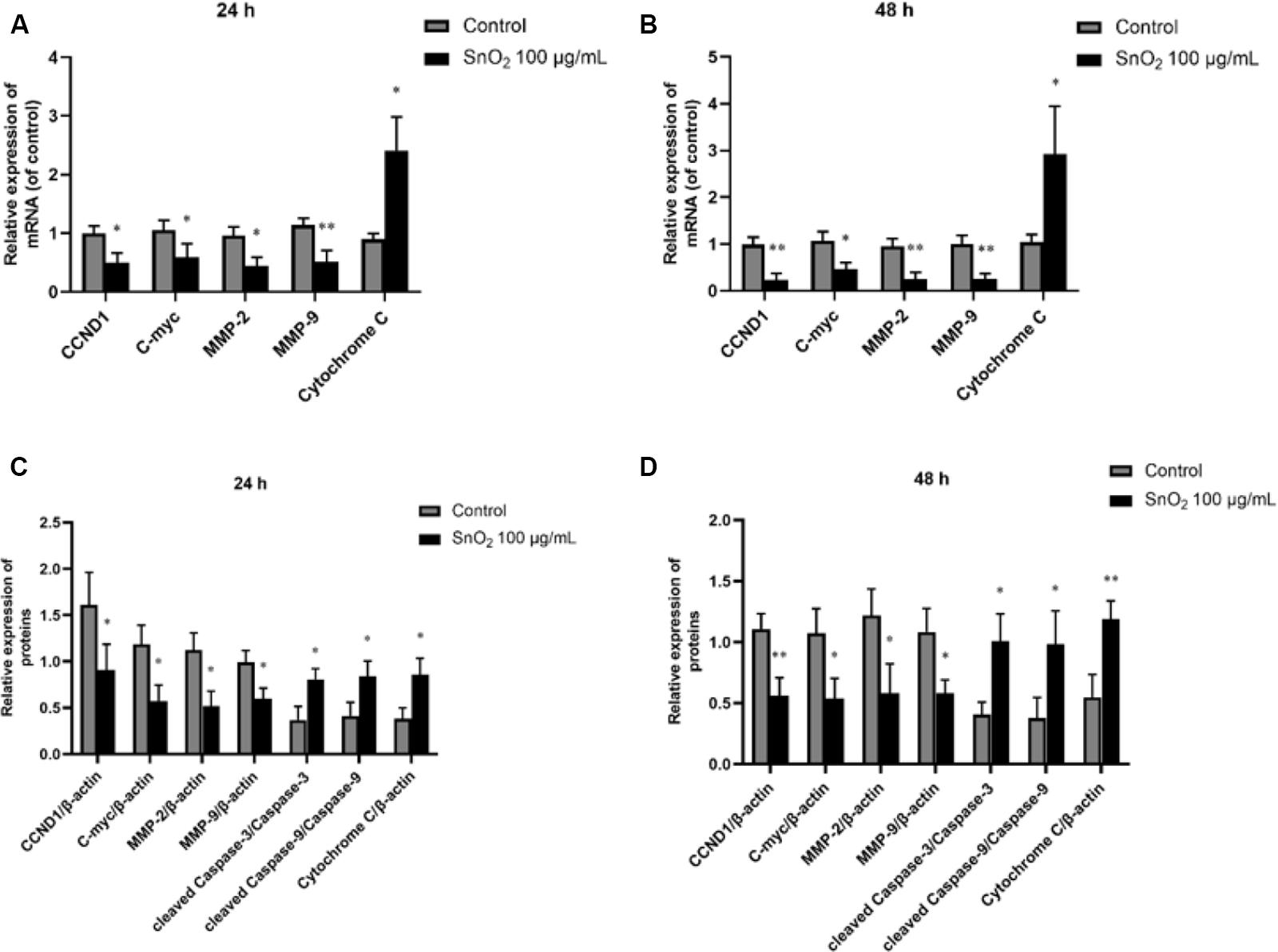
Figure 8. SnO2 NP changed expression of proliferation, migration, invasion, apoptosis, and oxidative stress-related protein. (A,B) The changes of target mRNA levels in cells after treatment of 0 or 100 μg/mL SnO2 NP at 24 or 48 h. (C,D) The changes of target protein levels in cells after treatment of 0 or 100 μg/mL SnO2 NP at 24 or 48 h. Compared with a control group, *P < 0.05, **P < 0.01.
Moreover, as shown in Figures 8C,D, compared with the control group, 100 μg/mL SnO2 NP treatment at 24 and 48 h can not only significantly inhibit the expression of proliferation-related factors CCND1, c-myc in CAL-27 cells, but also decrease the protein levels of migration and invasion related factors MMP-2 and MMP-9 (both P < 0.05), promote the expression of such apoptosis-related proteins as cleaved Caspase-3, cleaved Caspase-9 and oxidative stress-related factor Cytochrome C (all P < 0.05).
Discussion
SnO2 is a multifunctional metal oxide. Since SnO2 NP can be used as antibacterial agents, disinfectants, and other products with excellent performance, SnO2 NP has been gradually applied in the medical field. Recently, the critical role of SnO2 NP in cancer treatment has attracted widespread attention. Some studies have confirmed that SnO2 NP had a robust anti-cancer effect on specific cancer cells. For instance, SnO2 NP could induce human HCT116 and A549 cells to produce ROS in vitro, which leads to cell death (Tammina et al., 2017); besides, it is found that SnO2 NP treatment can cause HepG2 cells to occur Morphological changes and cytotoxic effects (Roopan et al., 2015); it is also demonstrated that SnO2 NP can induce oxidative stress in MCF-7 cells, prompting the cells to synthesize ROS, increasing H2O2 levels, triggering lipid peroxidation, reducing glutathione and antioxidant enzymes levels, and finally causing cell damage (Ahamed et al., 2018); Moreover, Lv found that SnO2 nanofibers can not only reduce the cell activity of hepatocellular carcinoma SMMC-7721 but also induce apoptosis. Western blot detection found that the expression of Caspase-3, the apoptosis-related protein, was increased (Lv et al., 2018).
In this study, SnO2 NP was prepared by the sol-gel method. Spectral analysis, XRD analysis, and TEM observation confirmed that the SnO2 NP that we produced were consistent with literature records and could be used for experiments. This study first found that SnO2 NP had anti-proliferative effects on two different kinds of oral cancer cells. Specifically, SnO2 NP induced a decrease in the activity of oral cancer cells in a dose-dependent manner, which became more evident with longer exposure time. This result is consistent with previous studies on the effects of SnO2 NP on other cancers (Roopan et al., 2015; Tammina et al., 2017). At the same time, the protein and mRNA expressions of CCND1 and c-myc, the cell proliferation-related genes, were also detected. Some studies have found that CCND1 can regulate the cell cycle by controlling the G1/S phase transition, and increased expression of CCND1 will lead to the occurrence of cancer (Musgrove et al., 2011). As a carcinogenic transcription factor, C-Myc recognizes E-box and its related sequences in the target gene promoter. The increase of C-Myc level significantly improves the tumor proliferation ability (Liu et al., 2015). The study indicated that SnO2 NP could inhibit the expression of CCND1 and c-myc in oral cancer cells, thereby exerting an inhibitory effect on cell proliferation. Also, the morphological changes in oral cancer cells after being treated with SnO2 NP were observed and found that the cells shrank significantly. The cell density decreased, which further demonstrated that SnO2 NP could exert cytotoxicity on oral cancer cells.
It is known that the cytotoxicity induced by SnO2 NP is involved in the generation of oxidative stress, and Ahamed et al. (2018) demonstrated that in breast cancer cells SnO2 NP induced a dose-dependent increase in the production of oxidative products. As we all know, the production and removal of ROS in a normal healthy body are in a dynamic balance. When harmful factors destroy this balance, the level of free radical scavenging enzymes in the body will increase the production of ROS. Besides, it may also trigger the cleavage of lipid peroxides to MDA, which plays a cytotoxic role (Kannan and Jain, 2000). Therefore, MDA and ROS are effective markers of the occurrence of oxidative stress in the body. This study found that SnO2 NPs increased MDA and ROS levels in CAL-27 and SCC-9 cells, and by detecting the expression of oxidative stress-related factors Cytochrome C, it was found that SnO2 NPs can induce oral cancer cells to produce an increased amount of Cytochrome C, thereby increasing oxidative stress. The above results indicated that SnO2 NP could cause cell damage by inducing oxidative stress.
Cytochrome c plays a vital role in apoptosis in addition to its role in oxidative stress. It is known that caspase-3 and caspase-9 belong to the cysteine aspartic protease family and act as intermediary proteins in the degradation of proteolytic enzymes during apoptosis. In mammals, cytochrome c together with apoptosis-activating factor 1 activates caspase 9, which subsequently leads to caspase-3 activation, finally triggering a cascading waterfall effect and inducing apoptosis. This study found that SnO2 NPs can significantly increase the number of apoptosis of oral cancer cells, and the expression levels of apoptosis-related proteins Cleared Caspase-3 and Cleared Caspase-3 were also increased at different levels. These results suggest that SnO2 NP can cause cell damage by inducing apoptosis.
In addition to apoptosis and oxidative stress, the effects of SnO2 NP treatment on migration and invasion of oral cancer cells were also examined in this study. The results showed that oral cancer cells exposed to SnO2 NP can significantly reduce their migration and invasion ability, and this inhibitory effect became more apparent, with the prolonged exposure time. In addition to visually observing changes in cell function, the mRNA and protein expression of migration and invasion related genes MMP-2 and MMP-9 were also detected. MMPs are known to be essential for tumor angiogenesis and metastasis, and the destruction of the basement membrane by activating MMPs is a crucial step for cancer invasion and metastasis (Lin et al., 2014). Therefore, inhibition of MMPs expression can provide an initial target for preventing tumor metastasis. This study found that after treatment with SnO2 NP, both MMP-2 and MMP-9 protein and mRNA expressions in oral cancer cells were down-regulated by varying degrees. This result is consistent with the results of migration and invasion experiments, suggesting that SnO2 NP can inhibit the migration and invasion of oral cancer cells.
Despite the results shown in this study, because anti-tumor polymer nano-drugs are a new research hotspot and the research history is still relatively short, there are still a series of problems in this research, such as the lack of in-depth understanding of metabolic kinetics and biodistribution of the nano-drugs. For targeted tumor therapy, key factors (such as particle size, charge, surface chemistry) are still lacking in-depth exploration. Furthermore, due to the complexity of tumors, the therapeutic effect of nanomedicine in different tumor types and tumors with different stages of progressions may be different. Nevertheless, nanotechnology is still a very important hot subject that affects medicine. Although the research and development of nanotechnology-applied medicine are still only in its early stages, due to its rapid development, it will surely develop into a new discipline and new industry.
Conclusion
In summary, we found in this study that SnO2 NP can exert cytotoxic effects on oral cancer cells by inhibiting cell proliferation, migration, and invasion abilities, and can also induce oxidative stress and apoptosis. Therefore, it is indicated that SnO2 NP may have anti-oral cancer effects, which can provide a basis for future research and clinical application and has important theoretical value. However, more in-depth animal experiments and clinical trials are still needed to confirm its clinical value. This is a major limitation of this study and a direction for future research.
Data Availability Statement
The original contributions presented in the study are included in the article/supplementary material, further inquiries can be directed to the corresponding author/s.
Ethics Statement
The animal study was reviewed and approved by the Animal Care and Use Committee at Jilin University.
Author Contributions
HY and BZ proposed and designed the experiments. HL and QL carried out the experiments with the help of YL and XS. HL and QL drafted the manuscript and interpreted the data. HL, QL, HY, and BZ revised the manuscript. All authors contributed to the article and approved the submitted version.
Funding
The study was financially supported by the Science and Technology Development Program of Jilin Province (Grant No. 20200201519JC).
Conflict of Interest
The authors declare that the research was conducted in the absence of any commercial or financial relationships that could be construed as a potential conflict of interest.
References
Ahamed, M., Akhtar, M. J., Khan, M. M., and Alhadlaq, H. A. (2018). Oxidative stress mediated cytotoxicity of tin (IV) oxide (SnO2) nanoparticles in human breast cancer (MCF-7) cells. Coll. Surf. B Biointerf. 172, 152–160. doi: 10.1016/j.colsurfb.2018.08.040
Aziz, M., Abbas, S. S., and Baharom, W. R. W. (2013). Size-controlled synthesis of SnO2 nanoparticles by sol–gel method. Mater. Lett. 91, 31–34. doi: 10.1016/j.matlet.2012.09.079
Behzadi, S., Serpooshan, V., Tao, W., Hamaly, M. A., Alkawareek, M. Y., Dreaden, E. C., et al. (2017). Cellular uptake of nanoparticles: journey inside the cell. Chem. Soc. Rev. 46, 4218–4244. doi: 10.1039/c6cs00636a
Chen, H., Wang, Q., Kou, C., Sui, Y., Zeng, Y., and Du, F. (2014). One-pot synthesis and improved sensing properties of hierarchical flowerlike SnO2 assembled from sheet and ultra-thin rod subunits. Sens. Actuat. B Chem. 194, 447–453. doi: 10.1016/j.snb.2013.12.119
Chen, J., Ding, J., Wang, Y., Cheng, J., Ji, S., Zhuang, X., et al. (2017a). Sequentially responsive shell-stacked nanoparticles for deep penetration into solid tumors. Adv. Mater. 29:1701170. doi: 10.1002/adma.201701170
Chen, J., Ding, J., Xu, W., Sun, T., Xiao, H., Zhuang, X., et al. (2017b). Receptor and microenvironment dual-recognizable nanogel for targeted chemotherapy of highly metastatic malignancy. Nano Lett. 17, 4526–4533. doi: 10.1021/acs.nanolett.7b02129
Cheng, L., Ma, S., Wang, T., Li, X., Luo, J., Li, W., et al. (2014). Synthesis and characterization of SnO2 hollow nanofibers by electrospinning for ethanol sensing properties. Mater. Lett. 131, 23–26. doi: 10.1016/j.matlet.2014.05.151
Cristaldi, M., Mauceri, R., Di Fede, O., Giuliana, G., Campisi, G., and Panzarella, V. (2019). Salivary biomarkers for oral squamous cell carcinoma diagnosis and follow-up: current status and perspectives. Front. Physiol. 10:1476. doi: 10.3389/fphys.2019.01476
Ding, J., Chen, J., Gao, L., Jiang, Z., Zhang, Y., Li, M., et al. (2019). Engineered nanomedicines with enhanced tumor penetration. Nano Today 29:100800. doi: 10.1016/j.nantod.2019.100800
Dong, H., Lei, J., Ju, H., Zhi, F., Wang, H., Guo, W., et al. (2012). Target-cell-specific delivery, imaging, and detection of intracellular MicroRNA with a multifunctional SnO2 nanoprobe. Angew. Chem. Intern. Edn. 51, 4607–4612. doi: 10.1002/anie.201108302
Feng, X., Xu, W., Li, Z., Song, W., Ding, J., and Chen, X. (2019). Immunomodulatory nanosystems. Adv. Sci. 6:1900101. doi: 10.1002/advs.201900101
Gattu, K. P., Ghule, K., Kashale, A. A., Patil, V., Phase, D., Mane, R., et al. (2015). Bio-green synthesis of Ni-doped tin oxide nanoparticles and its influence on gas sensing properties. RSC Adv. 5, 72849–72856. doi: 10.1039/c5ra13513c
Jiang, Z., Liu, Y., Feng, X., and Ding, J. (2019). Functional polypeptide nanogels. J. Funct. Poly. 32, 13–27.
Khan, M. M., Khan, W., Ahamed, M., and Alhazaa, A. N. (2017). Microstructural properties and enhanced photocatalytic performance of Zn doped CeO 2 nanocrystals. Sci. Rep. 7, 1–11.
Khan, M. M., Kumar, S., Khan, M. N., Ahamed, M., and Al Dwayyan, A. (2014). Microstructure and blueshift in optical band gap of nanocrystalline AlxZn1- xO thin films. J. Luminescen. 155, 275–281. doi: 10.1016/j.jlumin.2014.06.007
Li, S., Feng, X., Wang, J., Xu, W., Islam, M. A., Sun, T., et al. (2019). Multiantigenic nanoformulations activate anticancer immunity depending on size. Adv. Funct. Mater. 29:1903391. doi: 10.1002/adfm.201903391
Li, S., Zhang, T., Xu, W., Ding, J., Yin, F., Xu, J., et al. (2018). Sarcoma-targeting peptide-decorated polypeptide nanogel intracellularly delivers shikonin for upregulated osteosarcoma necroptosis and diminished pulmonary metastasis. Theranostics 8, 1361–1375. doi: 10.7150/thno.18299
Lin, C.-W., Chou, Y.-E., Chiou, H.-L., Chen, M.-K., Yang, W.-E., Hsieh, M.-J., et al. (2014). Pterostilbene suppresses oral cancer cell invasion by inhibiting MMP-2 expression. Expert Opin. Therap. Targ. 18, 1109–1120. doi: 10.1517/14728222.2014.947962
Liu, Z., He, Q., Ding, X., Zhao, T., Zhao, L., and Wang, A. (2015). SOD2 is a C-myc target gene that promotes the migration and invasion of tongue squamous cell carcinoma involving cancer stem-like cells. Intern. J. Biochem. Cell Biol. 60, 139–146. doi: 10.1016/j.biocel.2014.12.022
Lv, H., Wu, C., Liu, X., Bai, A., Cao, Y., Shang, W., et al. (2018). Folate-functionalized mesoporous hollow SnO2 nanofibers as a targeting drug carrier to improve the antitumor effect of paclitaxel for liver cancer therapy. Biomed Res. Intern. 2018:8526190.
Mohanta, D., Barman, K., Jasimuddin, S., and Ahmaruzzaman, M. (2017). MnO doped SnO2 nanocatalysts: activation of wide band gap semiconducting nanomaterials towards visible light induced photoelectrocatalytic water oxidation. J. Coll. Interf. Sci. 505, 756–762. doi: 10.1016/j.jcis.2017.06.064
Musgrove, E. A., Caldon, C. E., Barraclough, J., Stone, A., and Sutherland, R. L. (2011). Cyclin D as a therapeutic target in cancer. Nat. Rev. Cancer 11, 558–572. doi: 10.1038/nrc3090
Omori, H., Nishio, M., Masuda, M., Miyachi, Y., Ueda, F., Nakano, T., et al. (2020). YAP1 is a potent driver of the onset and progression of oral squamous cell carcinoma. Sci. Adv. 6:eaay3324. doi: 10.1126/sciadv.aay3324
Qi, B., Wang, C., Ding, J., and Tao, W. (2019). Editorial: applications of nanobiotechnology in pharmacology. Front. Pharmacol. 10:1451. doi: 10.3389/fphar.2019.01451
Rasmussen, J. W., Martinez, E., Louka, P., and Wingett, D. G. (2010). Zinc oxide nanoparticles for selective destruction of tumor cells and potential for drug delivery applications. Expert Opin. Drug Deliv. 7, 1063–1077. doi: 10.1517/17425247.2010.502560
Roopan, S. M., Kumar, S. H. S., Madhumitha, G., and Suthindhiran, K. (2015). Biogenic-production of SnO 2 nanoparticles and its cytotoxic effect against hepatocellular carcinoma cell line (HepG2). Appl. Biochem. Biotechnol. 175, 1567–1575. doi: 10.1007/s12010-014-1381-5
Shiga, K., Tateda, M., Katagiri, K., Nakanome, A., Ogawa, T., Asada, Y., et al. (2011). Distinct features of second primary malignancies in head and neck cancer patients in Japan. Tohoku J. Exper. Med. 225, 5–12. doi: 10.1620/tjem.225.5
Sun, Y., Ma, W., Yang, Y., He, M., Bai, L., Yu, Z., et al. (2019). Cancer nanotechnology: enhancing tumor cell response to chemotherapy for hepatocellular carcinoma therapy. Asian J. Pharm. Sci. 14, 581–594. doi: 10.1016/j.ajps.2019.04.005
Tammina, S. K., Mandal, B. K., Ranjan, S., and Dasgupta, N. (2017). Cytotoxicity study of Piper nigrum seed mediated synthesized SnO2 nanoparticles towards colorectal (HCT116) and lung cancer (A549) cell lines. J. Photochem. Photobiol. B Biol. 166, 158–168. doi: 10.1016/j.jphotobiol.2016.11.017
Vidhu, V., and Philip, D. (2015). Biogenic synthesis of SnO2 nanoparticles: evaluation of antibacterial and antioxidant activities. Spectroch. Acta Part A Mol. Biomol. Spectrosc. 134, 372–379. doi: 10.1016/j.saa.2014.06.131
Wang, Q., Zhang, P., Li, Z., Feng, X., Lv, C., Zhang, H., et al. (2019). Evaluation of polymer nanoformulations in hepatoma therapy by established rodent models. Theranostics 9, 1426–1452. doi: 10.7150/thno.31683
Xiong, Q., Li, Y., Zhou, K., Chen, P., Guo, H., Chen, L., et al. (2020). Optimized fluorodendrimer-incorporated hybrid lipid–polymer nanoparticles for efficient siRNA delivery. Biomater. Sci. 8, 758–762. doi: 10.1039/c9bm01738k
Xu, J., Wang, H., Hu, Y., Zhang, Y. S., Wen, L., Yin, F., et al. (2019). Inhibition of CaMKIIα activity enhances antitumor effect of fullerene C60 nanocrystals by suppression of autophagic degradation. Adv. Sci. 6:1801233. doi: 10.1002/advs.201801233
Yang, C. X., Xing, L., Chang, X., Zhou, T. J., Yang, B., Jiang, H. L., et al. (2020). Synergistic platinum(II) prodrug nanoparticles for enhanced breast cancer therapy. Mol. Pharm. 17, 1300–1309. doi: 10.1021/acs.molpharmaceut.9b01318
Keywords: SnO2 nanoparticles, oral cancer, proliferation, invasion, apoptosis, oxidative stress
Citation: Li H, Li Q, Li Y, Sang X, Yuan H and Zheng B (2020) Stannic Oxide Nanoparticle Regulates Proliferation, Invasion, Apoptosis, and Oxidative Stress of Oral Cancer Cells. Front. Bioeng. Biotechnol. 8:768. doi: 10.3389/fbioe.2020.00768
Received: 22 April 2020; Accepted: 18 June 2020;
Published: 17 July 2020.
Edited by:
Mingqiang Li, Sun Yat-sen University, ChinaReviewed by:
Zhiqiang Yu, Southern Medical University, ChinaChao Zhao, The University of Alabama, United States
Jin Zhang, Fuzhou University, China
Copyright © 2020 Li, Li, Li, Sang, Yuan and Zheng. This is an open-access article distributed under the terms of the Creative Commons Attribution License (CC BY). The use, distribution or reproduction in other forums is permitted, provided the original author(s) and the copyright owner(s) are credited and that the original publication in this journal is cited, in accordance with accepted academic practice. No use, distribution or reproduction is permitted which does not comply with these terms.
*Correspondence: Haotian Yuan, dentantony@163.com; Baihong Zheng, zhengbh@jlu.edu.cn; hengbh@jlu.edu.cn
†These authors have contributed equally to this work