- 1Woodwell Climate Research Center, Falmouth, MA, United States
- 2International Institute for Applied Systems Analysis (IIASA), Laxenburg, Austria
The northern permafrost region holds almost half of the world's soil carbon in just 15% of global terrestrial surface area. Between 2007 and 2016, permafrost warmed by an average of 0.29°C, with observations indicating that frozen ground in the more southerly, discontinuous permafrost zone is already thawing. Despite this, our understanding of potential carbon release from this region remains not only uncertain, but incomplete. SROCC highlights that global-scale models represent carbon loss from permafrost only through gradual, top-down thaw. This excludes “pulse” disturbances – namely abrupt thaw, in which frozen ground with high ice content thaws, resulting in subsidence and comparatively rapid ongoing thaw, and fire – both of which are critically important to projecting future permafrost carbon feedbacks. Substantial uncertainty remains around the response of these disturbances to ongoing warming, although both are projected to affect an increasing area of the northern permafrost region. This is of particular concern as recent evidence indicates that pulse disturbances may, in some cases, respond nonlinearly to warming. Even less well understood are the interactions between processes driving loss of permafrost carbon. Fire not only drives direct carbon loss, but can accelerate gradual and abrupt permafrost thaw. However, this important interplay is rarely addressed in the scientific literature. Here, we identify barriers to estimating the magnitude of future emissions from pulse disturbances across the northern permafrost region, including those resulting from interactions between disturbances. We draw on recent advances to prioritize said barriers and suggest avenues for the polar research community to address these.
Introduction
Permafrost soils across the boreal and tundra biomes constitute the world's largest vulnerable carbon pool. Disturbance to this pool through ground thaw, and the subsequent magnitude, timescale, and form of carbon release into the atmosphere, will have a decisive impact on future climate change (Schuur et al., 2015).
Climate change drives disturbance of northern permafrost through a number of distinct processes (Kokelj et al., 2017) (Figure 1). These include gradual warming of the ground and subsequent thickening of the active layer; the upper layer of permafrost soils which undergoes seasonal thaw. This “gradual thaw” is projected to result in the loss of 24 to 69% of near-surface (upper 3m) permafrost this century (likely range; IPCC, 2019). Estimates of carbon release resulting from gradual thaw on the same timescale range from 30Pg C to 150Pg C (Natali et al., 2021); equivalent to the cumulative emissions through 2100 from Japan to those of the United States through 2100, at their current rate of emissions (UCS, 2020).

Figure 1. Conceptual figure illustrating possible changes in permafrost environments due to ongoing climate change, including (a) active layer thickening; (b) combustion of soil organic matter during more frequent and severe fires; (c) accelerated active layer thickening following fire; (d) surface subsidence and development of abrupt thaw features, including in response to increased fire activity; (e) progressive, fire-induced thaw of the full permafrost table in more southerly regions of discontinuous permafrost, including through the formation and lateral expansion of taliks.
Gradual thaw can be likened to a “press” disturbance; a disturbance with a comparatively low magnitude and long duration (Jentsch and White, 2019). Currently, only gradual permafrost thaw is represented in Earth system models (ESMs). This excludes important, relatively slow press disturbances such as the formation of subsurface layers of perennially thawed soil known as taliks, which can drive additional thaw (Devoie et al., 2019), and some gradual thermokarst processes (such as lowland thermokarst lakes), which result from uneven ground subsidence following thaw.
However, climate change is driving not just an acceleration of press disturbance, but also an increase in some types of “pulse” - abrupt, high magnitude per unit duration - disturbance across permafrost regions. This is of concern as pulse disturbances can in many cases result in substantial carbon emissions.
Notable among these pulse disturbances is abrupt permafrost thaw. Abrupt thaw is a collective term encompassing a range of thermokarst processes, where degradation of hillslope permafrost with a high ice content results in comparatively rapid, high magnitude ground disturance and the formation of features (e.g. retrogressive thaw slumps, Kokelj and Jorgenson, 2013). The term also includes the development of some lowland thermokarst landforms, such as thermokarst wetlands and lakes; although some thermokarst features form gradually over decades, and therefore may be better described as press disturbances. While gradual thaw proceeds top-down over years and decades, abrupt thaw can expose several meters of permafrost on a timescale of days to years.
Of the pulse disturbances considered here, fire affects the largest area of the Arctic-boreal zone (Stocks et al., 2002; van der Werf et al., 2017). The incidence of fire has already increased in boreal and tundra biomes since the mid-20th century (Kasischke et al., 2010; Hanes et al., 2019), with evidence suggesting that intensifying fire regimes are linked to exceptionally high fire emissions in recent years (Veraverbeke et al., 2017; Walker et al., 2018; McCarty et al., 2020; Scholten et al., 2021). While some ESMs include fire, they exclude combustion of soil organic matter which is the dominant means of carbon loss at high latitudes. Further, fire can accelerate permafrost thaw following combustion of insulating soil organic layer, both by initiating or accelerating gradual thaw and by promoting abrupt thaw processes in ~20% of the high-latitude region which is prone to abrupt thaw (Olefeldt et al., 2016; Gibson et al., 2018).
Although pulse disturbances occur comparatively infrequently in time and space, they can have impacts equal to those of press disturbances. For example, across the Arctic, abrupt thaw could result in radiative forcing equal to that of gradual thaw, despite affecting less than 20% of the permafrost region (Turetsky et al., 2020).
The body of literature addressing carbon release from pulse disturbances is growing, and in some cases, notably for fire, is already substantial. However, the stochastic nature of pulse disturbances, the complexity underlying both their impacts and responses to climate change, and the challenges inherent in acquiring data across high-latitude regions, are still major obstacles to incorporating these important processes into comprehensive assessments of future carbon release from the northern permafrost region. Here we reflect on these obstacles, with the objective of highlighting priority issues for future research and approaches which - we argue - represent the most efficient means of addressing these.
Barriers to Estimating Carbon LOSS
Of the disturbances considered here, recent carbon loss from fire can be assessed with the most confidence. A number of gridded datasets derived from satellite data report burned area and fire emissions at annual or greater temporal resolution at regional to pan-Arctic scales (Randerson et al., 2015; Veraverbeke et al., 2015; Giglio et al., 2018; Otón et al., 2019; Dieleman et al., 2020). Links with climatic change are also well established, with warming, drying, and increased lightning strike rate all implicated in increasing fire activity (Walsh et al., 2020; York et al., 2020; Chen et al., 2021; McCarty et al., 2021).
However, projected increases in burned area, a primary determinant of fire emissions (Veraverbeke et al., 2015), range from less than 50% to more than 150% per °C of global warming, with similarly wide ranges reported even for well-studied regions such as Alaska (7–93% °C−1) (estimated from: Euskirchen et al., 2009; Eliseev et al., 2014; Genet et al., 2018). The breadth of these ranges demonstrate that future fire activity remains highly uncertain, due both to the complexity of the underlying processes and the challenge of scaling these across large regions (Kitzberger et al., 2017; Boulanger et al., 2018).
In contrast to fire, there is no pan-Arctic assessment of abrupt thaw incidence. This is partly due to the difficulty of detecting abrupt thaw features - which can be comparatively small and, in contrast to the thermal signatures associated with fire, lack a signal that is easily detectable through moderate-resolution satellite remote sensing. Recent studies illustrate that even High Arctic permafrost can rapidly undergo abrupt thaw in response to warming (Farquharson et al., 2019; Jones et al., 2019; Lewkowicz and Way, 2019). However, while local-scale observational studies provide critical insights into (for example) the drivers of different thaw trajectories, an ongoing reliance on local-scale studies alone to quantify abrupt thaw incidence across large and heterogeneous Arctic regions means that the links between climatic change and abrupt thaw rates remain poorly constrained.
Similarly, although regional-scale modeling demonstrates that abrupt thaw can have decisive consequences for carbon vulnerability (Nitzbon et al., 2020), comprehensive assessments of its impact on carbon fluxes are scarce, particularly regarding lateral fluxes, which can account for more than half of total carbon loss (Plaza et al., 2019). The one existing pan-Arctic estimate suggests that abrupt thaw-driven net carbon losses could equal 40% of those for gradual thaw (Turetsky et al., 2020). However, data scarcity remains a limiting factor in this first-order approach.
Quantification of post-fire thaw also relies heavily on single-site studies, often with less than a decade of observational data reported (but see e.g. Jafarov et al., 2013; Gibson et al., 2018). For tundra ecosystems, where the largest proportional increases in fire regimes are expected (Chen et al., 2021), no long-term monitoring of post-fire active layer thickness has been reported to date (Holloway et al., 2020). Therefore, our understanding of post-fire thaw trajectories, and especially their spatial and geographical variation, remains limited.
The obstacles posed by a lack of observational data are compounded by limitations in current modeling approaches. The spatial resolution at which global scale models operate is much coarser than stochastic, fine-scale and highly heterogeneous pulse disturbance processes. Moreover, simplified model structures do not lend themselves to mechanistically simulating these disturbances. For example, ESMs typically employ one soil column per grid cell, and a “big leaf” representation of vegetation; effectively collapsing complex eco-physiological, biogeochemical, and geomorphological processes across thousands of km2 into a single site.
Prioritizing for Better Predictions
Predicting future consequences of pulse disturbances is complex. It requires predictions of climatic changes, identification of the key environmental drivers (including variables describing environmental context, such as those related to soil characteristics, topography and drainage) which govern the incidence of, and recovery from, disturbance, and mechanistic understanding of how climatic changes affect those drivers. Data describing geographical and spatial variation in key environmental drivers, and mechanistic understanding of how those drivers affect the incidence, impacts of, and recovery from disturbance are also required to apply predictions across large spatial scales.
We propose a conceptual framework (Figure 2) to describe these data and knowledge needs. Below, we use this framework to identify priorities to deliver near-term improvements in predicting carbon loss from pulse disturbances. In doing so, we emphasize the need for improvements in: (a) mechanistic understanding of how environmental variables drive disturbance impacts (Figure 2d); and (b) gridded data describing these driving variables (Figure 2c). “Mechanistic understanding” here refers to a predictive understanding of the means through which variation in a given environmental variable drives variation in the impacts of disturbance. “Driving variables” here refers to variables with causal influence over disturbance impacts. Together, improvements in (a) and (b) can deliver better understanding of how heterogeneity across the Arctic currently influences the incidence and consequences of pulse disturbances, and therefore how environmental change will influence pulse disturbances in the future. Addressing each of these concepts (a & b) respectively, we provide specific recommendations and priorities for future research.
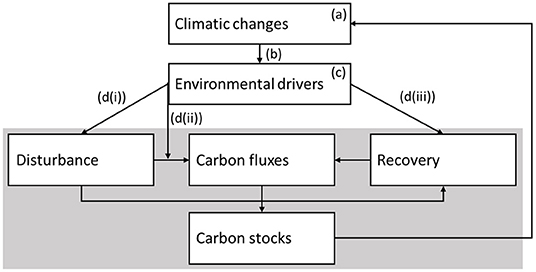
Figure 2. Simplified framework for predicting carbon loss from pulse disturbances under climate change. Predictive understanding relies on a foundational understanding of how the incidence of and recovery from disturbance affects carbon fluxes, and how those carbon flux changes translate to longer-term changes in carbon storage (processes shaded in light gray). Predicting future carbon loss from disturbance under climate change scenarios then requires (a) predictions of climatic changes; (b) mechanistic understanding of how these variables drive (c) key environmental variables that influence incidence of the disturbance in question, and data describing variation in these variables across tundra and boreal biomes; and (d) a mechanistic understanding of how these variables affect (i) disturbance characteristics such as frequency, duration, and spatial distribution; (ii) the severity or intensity of impacts on ecosystem carbon fluxes and (iii) characteristics of recovery such as duration and final state.
Identify Mechanisms
Identifying and quantifying causal processes can highlight variables that influence disturbance characteristics in predictable ways. These variables can then guide ground or remote data collection and/or inform predictive modeling approaches. For example, recent work has highlighted that “bottom-up” drivers, such as fuel availability, can exert a stronger influence over fire emissions compared to “top-down” drivers (such as fire weather; Walker et al., 2020). In this case, site-level drainage played an outsized role in determining fuel availability and carbon emissions, yet large-scale estimates of drainage do not exist. It follows that better quantifying drainage conditions should be a high research priority for understanding and predicting the impact of intensifying fire regimes on carbon cycling.
A mechanistic focus has strong potential to reduce uncertainty around carbon loss due to post-fire permafrost thaw. It has been shown that post-fire organic layer depth is a decisive factor in determining subsequent thaw trajectory (Jafarov et al., 2013). And since belowground carbon pool size, vegetation type, and soil moisture influence organic layer combustion, these variables are likely significant drivers of post-fire thaw (Minsley et al., 2016; Walker et al., 2020).
Mechanisms driving ground thermodynamics are relatively well-understood (Jafarov et al., 2013), allowing robust site-level estimates of gradual ground thaw (e.g., Garnello et al., 2021); yet, pan-Arctic assessments are limited by lack of robust gridded datasets (e.g., snow depth and density). While ground ice content is the primary determinant of ground subsidence following thaw, abrupt thaw processes are also strongly influenced by landscape characteristics such as surface moisture content, hydrological connectivity, soil type, topography, slope and deposit stratigraphy (Shiklomanov and Nelson, 2013; Lara et al., 2015; Olefeldt et al., 2016). Further, the initiation and progression of abrupt thaw may be exacerbated by other pulse disturbances, including fire (Raynolds et al., 2013; Baltzer et al., 2014; Lewkowicz and Way, 2019; Christensen et al., 2021). A causal, quantified understanding of how these landcover characteristics – and interacting disturbance types - influence abrupt thaw processes could therefore inform improved pan-Arctic carbon predictions.
Another priority area for improved mechanistic understanding is post-disturbance changes in vegetation characteristics, such as increased productivity (Reichstein et al., 2013), or dominance of deciduous species (Mack et al., 2021). It is often suggested that these changes may to some extent “offset” soil carbon loss; but long-term, integrated understanding of disturbance-driven changes in carbon uptake is needed to assess how realistic this is – particularly as individual disturbance events can have large, near-term impacts on net carbon loss (Abbott et al., 2016). For example, emissions from the Anaktuvuk River fire, which burned 1,039 km2 of Alaskan tundra in 2007, were equivalent to annual net carbon uptake by the entire tundra biome (Mack et al., 2011). Further, fire has knock-on impacts on permafrost by changing ground surface albedo and removing the vegetation and organic soil that insulate ground temperatures and permafrost from warm summer air temperatures; e.g. via shading and the insulative/conductive capacity of dry/wet moss (O'Donnell et al., 2009). These effects can promote gradual, top-down permafrost thaw over a number of decades, as well initiating the development of thermokarst features, including larger-scale abrupt thaw features (e.g. retrogressive thaw slumps, Liu et al., 2014; Jones et al., 2015). However, the trajectory of post-fire permafrost thaw depends on processes such as the depth of combustion of the soil organic layer and the trajectory of post-fire vegetation recovery; which are in turn mediated by variables such as topography, soil moisture status and ground ice content (Jafarov et al., 2013; Nossov et al., 2013; Gibson et al., 2018).
A process-based approach is also an avenue for addressing nonlinearity. Recent work suggests high-latitude fire regimes may be undergoing a step-change in response to climate change, featuring an abrupt advance in the timing of the fire season. This advance is thought to indicate an increasing role for holdover or “zombie” fires which persist throughout the winter through sub-surface smoldering, before re-commencing their spread the following spring (Scholten et al., 2021). Similarly, the last few years have seen increased burning of traditionally fire-resistant landscapes such as wetlands (McCarty et al., 2020). These phenomena highlight the value of process-level understanding in addition to observations of net impacts; in a rapidly changing and no-analog climate, responses that are unfamiliar and perhaps unexpected are not unlikely. While some changes will inevitably remain unpredictable, a quantified understanding of the variables and thresholds driving key processes such as fire ignition, spread and smoldering behavior may highlight the potential for non-linearity.
Recommendations
While acknowledging that many knowledge gaps exist, here we have identified a sub-set of the processes that determine the net impact of abrupt thaw, fire and fire-mediated thaw on carbon balance that are missing from models, and for which it is feasible to develop mechanistic understanding.
First among these is surface moisture dynamics. Although surface water can significantly alter permafrost temperatures (Langer et al., 2016), how moisture status influences processes such as fire ignition remains poorly quantified, as does its influence over pathways of subsequent carbon release (Plaza et al., 2019). The importance of these processes will only increase as Arctic weather becomes increasingly rain-dominated and permafrost thaw results in complex hydrological change (AMAP, 2017).
A second priority is process-level understanding of vegetation responses and carbon re-accumulation following disturbance (Pizano et al., 2014). For abrupt thaw in particular, these responses are rarely monitored and poorly understood, despite the likelihood of substantial consequences for the net carbon impacts of disturbance (Hugelius et al., 2020). Long-term monitoring of post-disturbance vegetation processes remains a critical avenue for future research.
In addition, a systematic, mechanistic approach to future work on abrupt thaw processes should aim to provide causal insight into how site-specific variables – such as soil and hydrological characteristics, in addition to ground ice – determine the progression and net carbon flux consequences of abrupt thaw (e.g. Kokelj et al., 2017). Such insight, when paired with mapping/quantification of those key variables (see next section) could effectively address this core obstacle to comprehensive estimates of future high-latitude carbon loss (Turetsky et al., 2020).
Similar to ongoing developments in vegetation models (Fisher et al., 2015; Fisher and Koven, 2020), representing patch dynamics (or adaptive tiling) of abrupt permafrost thaw could help bridge the spatial gap between ESMs and abrupt permafrost thaw features. Although spatial resolution is improving, it is unlikely global models will be able to directly simulate these features at their native resolutions (as small as 5–10 m) in the foreseeable future. When developing new algorithms, modelers would also benefit from new benchmarks on abrupt thaw, particularly in terms of spatial distribution, rates of change, stabilization/recovery, and impacts on hydrology and carbon fluxes.
Quantify Drivers
Section Identify Mechanisms emphasizes the importance of identifying variables with causal influence on the net impacts of disturbance. Such variables may then be used in remote sensing and/or predictive modeling approaches to better constrain estimates of future disturbance impacts. For example, parameterizations for abrupt thaw (similar to those developed in Turetsky et al., 2020) could be based on grid cell functions of ground ice, topographic indices, soil properties, and other environmental and climate drivers. Mapping driving variables such as these over space is particularly valuable at high-latitudes, where geographical and spatial heterogeneity enhance the complexity of developing pan-Arctic predictions (Myers-Smith et al., 2020; Virkkala et al., 2021).
However, this approach requires that identified driving variables can be quantified at a sufficient resolution to resolve landscape heterogeneity. Data acquisition across remote Arctic regions remains challenging, and while remote sensing presents a solution for measuring some vegetation characteristics, satellite data can rarely be used to directly observe belowground variables. As a result, many variables fundamental to permafrost processes and belowground carbon cycling remain poorly quantified (Parker et al., 2021).
Notable among the variables which remain poorly-described and have substantial potential to reduce uncertainty is ground ice content: a primary determinant of susceptibility to thermokarst. Other similarly important variables include the depth of the soil organic layer, which plays an important role in determining permafrost thermal dynamics, and those relating to surface and soil moisture, which impact the susceptibility to disturbance, as well as the subsequent form and magnitude of carbon release (Plaza et al., 2019). Mapping these belowground variables could help facilitate the up-scaling of existing observational studies and constrain process-based predictive approaches.
Recommendations
Section Quantify Drivers highlights the underrepresentation of belowground variables in gridded data repositories as a limiting factor in understanding and projecting high-latitude disturbances. Description of belowground variables, and particularly those which are most critical to projecting permafrost changes, is therefore a primary need. Specifically, we recommend gridded data products describing ground ice content, organic layer thickness, and surface moisture at spatial resolutions that facilitate comparison with on-the-ground data as priority research needs.
On a practical level, the inherent difficulty of measuring belowground variables remotely and/or over large areas is a core reason for the comparative lack of data describing them. Therefore, alongside pursuing promising approaches to improving remote sensing of belowground variables, such as use of multi-frequency radar, alternative systematic approaches to collecting these data at a sufficient resolution are required. In particular, the success of initiatives such as ITEX (International Tundra Experiment; Henry and Molau, 1997) highlight the potential for centralized networks to guide efficient collection of comparable datasets at large geographic scales., Centralized protocols may extend these benefits further (Parker et al., 2021), particularly where these a transdisciplinary approach; integrating the knowledge and data needs of the ecosystem ecology, geomorphology and modeling communities.
Discussion
Pulse disturbances present a considerable obstacle to accurately predicting future carbon dynamics, impeding projections of future climate feedbacks from the permafrost region. The body of literature addressing this issue is rapidly increasing, and includes identification of emergent relationships that may help constrain future disturbance rates and aid in up-scaling their impacts (e.g., Walker et al., 2020), as well as useful first-order, pan-Arctic estimates of those impacts (e.g., Turetsky et al., 2020). However, the availability of data required either for constraining such estimates, or scaling mechanistic insights across Arctic regions, remains insufficient. In the case of abrupt thaw and post-fire thaw, the mechanistic insight required to scale these data also limits our predictive capacity. Further, issues such as the disparity between fine-scale pulse disturbances and the relatively coarse spatial scale of existing global scale models, continue to present the modeling community with barriers beyond data availability to integrating these important processes.
We argue that future work should prioritize mechanistic understanding, focussed on identifying key driving variables, and improvements to the availability of data describing those variables. This represents an efficient approach to reducing the uncertainty associated with these important pulse disturbances.
However, the likely relevance of pulse disturbance impacts to near-term mitigation decisions demands not just an efficient approach to improving scientific understanding, but the maximal use of existing information alongside these efforts. This could encompass, for example, novel modeling approaches such as data assimilation (Scholze et al., 2017; Fox et al., 2018), and the incorporation of non-traditional data types such as expert assessments (Abbott et al., 2016; Sayedi et al., 2020). There is broad acceptance within the research community that pulse disturbances will significantly – albeit to an unquantified extent - impact net carbon loss from the permafrost region (Natali et al., 2021). It is therefore appropriate to prioritize effective dissemination of the risks associated with this, alongside longer-term efforts to facilitate a comprehensive, accurate estimate of the net carbon impact of pulse disturbances under ongoing climate change.
Data Availability Statement
The original contributions presented in the study are included in the article/supplementary material, further inquiries can be directed to the corresponding author/s.
Author Contributions
RT, SN, and BR designed research. RT, SN, BR, and EM performed research. RT wrote the paper. RT, SN, BR, EM, and TG provided discussion of themes, ideas, content, and editing. All authors contributed to the article and approved the submitted version.
Funding
RT, BR, SN, and EM were supported by One Earth and by the Gordon and Betty Moore Foundation under grant agreement #8414. TG was supported by the European Union's Horizon 2020 research and innovation programme under grant agreement #773421 (Nunataryuk project).
Conflict of Interest
The authors declare that the research was conducted in the absence of any commercial or financial relationships that could be construed as a potential conflict of interest.
Publisher's Note
All claims expressed in this article are solely those of the authors and do not necessarily represent those of their affiliated organizations, or those of the publisher, the editors and the reviewers. Any product that may be evaluated in this article, or claim that may be made by its manufacturer, is not guaranteed or endorsed by the publisher.
Acknowledgments
Woodwell Climate Research Center is located on the traditional and sacred land of the Wampanoag people who still occupy this land, and whose history, language, traditional ways of life, and culture continue to influence this vibrant community.
References
Abbott, B.W., Jones, J.B., Schuur, E.A., Chapin III, F.S., Bowden, W.B., Bret-Harte, M.S., et al. (2016). Biomass offsets little or none of permafrost carbon release from soils, streams, and wildfire: an expert assessment. Environ. Res. Lett. 11, 034014. doi: 10.1088/1748-9326/11/3/034014
AMAP (2017). Snow, Water, Ice and Permafrost in the Arctic (SWIPA). Available online at: https://www.amap.no/documents/doc/snow-water-ice-and-permafrost-in-the-arctic-swipa-2017/1610. (accessed May 24, 2021)
Baltzer, J. L., Veness, T., Chasmer, L. E., Sniderhan, A. E., and Quinton, W. L. (2014). Forests on thawing permafrost: Fragmentation, edge effects, and net forest loss. Glob. Change Biol. 20, 824–834. doi: 10.1111/gcb.12349
Boulanger, Y., Parisien, M.-A., Wang, X., Boulanger, Y., Parisien, M.-A., and Wang, X. (2018). Model-specification uncertainty in future area burned by wildfires in Canada. Int. J. Wildl Fire. 27, 164–175. doi: 10.1071/WF17123
Chen, Y., Romps, D. M., Seeley, J. T., Veraverbeke, S., Riley, W. J., Mekonnen, Z. A., et al. (2021). Future increases in Arctic lightning and fire risk for permafrost carbon. Nat. Clim. Change. 11, 404–410. doi: 10.1038/s41558-021-01011-y
Christensen, T. R., Lund, M., Skov, K., Abermann, J., Lopez-Blanco, E., Scheller, J., et al. (2021). Multiple Ecosystem Effects of Extreme Weather Events in the Arctic. Ecosystems. 24, 122–136. doi: 10.1007/s10021-020-00507-6
Devoie, É. G., Craig, J. R., Connon, R. F., and Quinton, W. L. (2019). Taliks: a tipping point in discontinuous permafrost degradation in peatlands. Water Resour. Res. 55, 9838–9857. doi: 10.1029/2018WR024488
Dieleman, C. M., Rogers, B. M., Potter, S., Veraverbeke, S., Johnstone, J. F., Laflamme, J., et al. (2020). Wildfire combustion and carbon stocks in the southern Canadian boreal forest: Implications for a warming world. Global Change Biol. 26, 6062–6079. doi: 10.1111/gcb.15158
Eliseev, A. V., Mokhov, I. I., and Chernokulsky, A. V. (2014). An ensemble approach to simulate CO2 emissions from natural fires. Biogeosci. 11, 3205–3223. doi: 10.5194/bg-11-3205-2014
Euskirchen, E. S., McGuire, A. D., Rupp, T. S., Chapin, F. S., and Walsh, J. E. (2009). Projected changes in atmospheric heating due to changes in fire disturbance and the snow season in the western Arctic, 2003–2100. J. Geophys. Res. 114, G04022. doi: 10.1029/2009JG001095
Farquharson, L. M., Romanovsky, V. E., Cable, W. L., Walker, D. A., Kokelj, S. V., and Nicolsky, D. (2019). Climate change drives widespread and rapid thermokarst development in very cold permafrost in the canadian high arctic. Geophys. Res. Lett. 46, 6681–6689. doi: 10.1029/2019GL082187
Fisher, R. A., and Koven, C. D. (2020). Perspectives on the future of land surface models and the challenges of representing complex terrestrial systems. J. Adv. Model. Earth Syst. 12, e2018MS001453. doi: 10.1029/2018MS001453
Fisher, R. A., Muszala, S., Verteinstein, M., Lawrence, P., Xu, C., McDowell, N. G., et al. (2015). Taking off the training wheels: the properties of a dynamic vegetation model without climate envelopes, CLM4.5(ED). Geosci. Model Dev. 8, 3593–3619. doi: 10.5194/gmd-8-3593-2015
Fox, A. M., Hoar, T. J., Anderson, J. L., Arellano, A. F., Smith, W. K., Litvak, M. E., et al. (2018). Evaluation of a data assimilation system for land surface models using CLM4.5. J. Adv. Model. Earth Syst. 10, 2471–2494, doi: 10.1029/2018MS001362
Garnello, A., Marchenko, S., Nicolsky, D., Romanovsky, V., Ledman, J., Celis, G., et al. (2021). Projecting permafrost thaw of sub-Arctic tundra with a thermodynamic model calibrated to site measurements. J. Geophys. Res. Biogeosci. 126:e2020JG006218. doi: 10.1029/2020JG006218
Genet, H., He, Y., Lyu, Z., McGuire, A. D., Zhuang, Q., Clein, J., et al. (2018). The role of driving factors in historical and projected carbon dynamics of upland ecosystems in Alaska. Ecol. Appl. 28, 5–27. doi: 10.1002/eap.1641
Gibson, C. M., Chasmer, L. E., Thompson, D. K., Quinton, W. L., Flannigan, M. D., and Olefeldt, D. (2018). Wildfire as a major driver of recent permafrost thaw in boreal peatlands. Nat. Commun. 9, 3041. doi: 10.1038/s41467-018-05457-1
Giglio, L., Boschetti, L., Roy, D. P., Humber, M. L., and Justice, C. O. (2018). The Collection 6 MODIS burned area mapping algorithm and product. Remote Sens. Environ. 217, 72–85. doi: 10.1016/j.rse.2018.08.005
Hanes, C. C., Wang, X., Jain, P., Parisien, M.-A., Little, J. M., and Flannigan, M. D. (2019). Fire-regime changes in Canada over the last half century. Can. J. For. Res. 49, 256–269. doi: 10.1139/cjfr-2018-0293
Henry, G. H. R., and Molau, U. (1997). Tundra plants and climate change: the international tundra experiment (ITEX). Global Change Biol. 3, 1–9. doi: 10.1111/j.1365-2486.1997.gcb132.x
Holloway, J. E., Lewkowicz, A. G., Douglas, T. A., Li, X., Turetsky, M. R., Baltzer, J. L., et al. (2020). Impact of wildfire on permafrost landscapes: A review of recent advances and future prospects. Permafrost Periglac. Process. 31, 371–382. doi: 10.1002/ppp.2048
Hugelius, G., Loisel, J., Chadburn, S., Jackson, R. B., Jones, M., MacDonald, G., et al. (2020). Large stocks of peatland carbon and nitrogen are vulnerable to permafrost thaw. Proc. National Acad. Sci. 117, 20438–20446. doi: 10.1073/pnas.1916387117
IPCC, . (2019): Summary for Policymakers. In: IPCC Special Report on the Ocean Cryosphere in a Changing Climate. Pörtner H.-O. Roberts D.C. Masson-Delmotte V. Zhai P. Tignor M. Poloczanska E. (eds.). In press .
Jafarov, E. E., Romanovsky, V. E., Genet, H., McGuire, A. D., and Marchenko, S. S. (2013). The effects of fire on the thermal stability of permafrost in lowland and upland black spruce forests of interior Alaska in a changing climate. Environ. Res. Lett. 8, 035030. doi: 10.1088/1748-9326/8/3/035030
Jentsch, A., and White, P. (2019). A theory of pulse dynamics and disturbance in ecology. Ecology. 100, e02734. doi: 10.1002/ecy.2734
Jones, B. M., Grosse, G., Arp, C. D., Miller, E., Liu, L., Hayes, D. J., et al. (2015). Recent Arctic tundra fire initiates widespread thermokarst development. Sci. Rep. 5, 15865. doi: 10.1038/srep15865
Jones, M. K. W., Pollard, W. H., and Jones, B. M. (2019). Rapid initialization of retrogressive thaw slumps in the Canadian high Arctic and their response to climate and terrain factors. Environ. Res. Lett. 14, 055006. doi: 10.1088/1748-9326/ab12fd
Kasischke, E. S., Verbyla, D. L., Rupp, T. S., McGuire, A. D., Murphy, K. A., Jandt, R., et al. (2010). Alaska's changing fire regime - implications for the vulnerability of its boreal forests. Can. J. For. Res. 40, 1313–1324. doi: 10.1139/X10-098
Kitzberger, T., Falk, D. A., Westerling, A. L., and Swetnam, T. W. (2017). Direct and indirect climate controls predict heterogeneous early-mid 21st century wildfire burned area across western and boreal North America. PLoS ONE. 12, e0188486. doi: 10.1371/journal.pone.0188486
Kokelj, S. V., and Jorgenson, M. T. (2013). Advances in thermokarst research. Permafrost Periglac. Process. 24, 108–119. doi: 10.1002/ppp.1779
Kokelj, S. V., Lantz, T. C., Tunnicliffe, J., Segal, R., and Lacelle, D. (2017). Climate-driven thaw of permafrost preserved glacial landscapes, northwestern Canada. Geology. 45, 371–374. doi: 10.1130/G38626.1
Langer, M., Westermann, S., Boike, J., Kirillin, G., Grosse, G., Peng, S., et al. (2016). Rapid degradation of permafrost underneath waterbodies in tundra landscapes—Toward a representation of thermokarst in land surface models. J. Geophys. Res. 121, 2446–2470. doi: 10.1002/2016JF003956
Lara, M. J., McGuire, A. D., Euskirchen, E. S., Tweedie, C. E., Hinkel, K. M., Skurikhin, A. N., et al. (2015). Polygonal tundra geomorphological change in response to warming alters future CO 2 and CH 4 flux on the Barrow Peninsula. Glob. Change Biol. 21, 1634–1651. doi: 10.1111/gcb.12757
Lewkowicz, A. G., and Way, R. G. (2019). Extremes of summer climate trigger thousands of thermokarst landslides in a High Arctic environment. Nat. Commun. 10, 1329. doi: 10.1038/s41467-019-09314-7
Liu, L., Jafarov, E. E., Schaefer, K. M., Jones, B. M., Zebker, H. A., Williams, C. A., et al. (2014). InSAR detects increase in surface subsidence caused by an Arctic tundra fire. Geophys. Res. Lett. 41, 3906–3913. doi: 10.1002/2014GL060533
Mack, M. C., Bret-Harte, M. S., Hollingsworth, T. N., Jandt, R. R., Schuur, E. A. G., Shaver, G. R., et al. (2011). Carbon loss from an unprecedented Arctic tundra wildfire. Nature. 475, 489–492. doi: 10.1038/nature10283
Mack, M. C., Walker, X. J., Johnstone, J. F., Alexander, H. D., Melvin, A. M., Jean, M., et al. (2021). Carbon loss from boreal forest wildfires offset by increased dominance of deciduous trees. Science., 372, 280–283. doi: 10.1126/science.abf3903
McCarty, J. L., Aalto, J., Paunu, V.-V., Arnold, S. R., Eckhardt, S., Klimont, Z., et al. (2021). Reviews andamp; syntheses: arctic fire regimes and emissions in the 21st Century. Biogeosci. Discuss. 18, 5053–5083. doi: 10.5194/bg-18-5053-2021
McCarty, J. L., Smith, T. E. L., and Turetsky, M. R. (2020). Arctic fires re-emerging. Nat. Geosci. 13, 658–660. doi: 10.1038/s41561-020-00645-5
Minsley, B. J., Pastick, N. J., Wylie, B. K., Brown, D. R. N., and Kass, M. A. (2016). Evidence for nonuniform permafrost degradation after fire in boreal landscapes. J. Geophys. Res. 121, 320–335. doi: 10.1002/2015JF003781
Myers-Smith, I. H., Kerby, J. T., Phoenix, G. K., Bjerke, J. W., Epstein, H. E., Assmann, J. J., et al. (2020). Complexity revealed in the greening of the Arctic. Nat. Clim. Change. 10, 106–117. doi: 10.1038/s41558-019-0688-1
Natali, S. M., Holdren, J. P., Rogers, B. M., Treharne, R., Duffy, P. B., Pomerance, R., et al. (2021). Permafrost carbon feedbacks threaten global climate goals. Proc. National Acad. Sci. 118, e2100163118. doi: 10.1073/pnas.2100163118
Nitzbon, J., Westermann, S., Langer, M., Martin, L. C. P., Strauss, J., Laboor, S., et al. (2020). Fast response of cold ice-rich permafrost in northeast Siberia to a warming climate. Nat. 11, 2201. doi: 10.1038/s41467-020-15725-8
Nossov, D. R., Jorgenson, M. T., Kielland, K., and Kanevskiy, M. Z. (2013). Edaphic and microclimatic controls over permafrost response to fire in interior Alaska. Environ. Res. Lett. 8, 035013. doi: 10.1088/1748-9326/8/3/035013
O'Donnell, J. A., Romanovsky, V. E., Harden, J. W., and Mcguire, A. D. (2009). The Effect of moisture content on the thermal conductivity of moss and organic soil horizons from black spruce ecosystems in interior Alaska. Soil Sci. 174, 646–651 doi: 10.1097/SS.0b013e3181c4a7f8
Olefeldt, D., Goswami, S., Grosse, G., Hayes, D., Hugelius, G., Kuhry, P., et al. (2016). Circumpolar distribution and carbon storage of thermokarst landscapes. Nat. Commun. 7, 13043. doi: 10.1038/ncomms13043
Otón, G., Ramo, R., Lizundia-Loiola, J., and Chuvieco, E. (2019). Global Detection of Long-Term (1982–2017) Burned Area with AVHRR-LTDR Data. Remote Sens. 11, 2079. doi: 10.3390/rs11182079
Parker, T. C., Thurston, A. M., Raundrup, K., Subke, J.-A., Wookey, P. A., and Hartley, I. P. (2021). Shrub expansion in the Arctic may induce large-scale carbon losses due to changes in plant-soil interactions. Plant and Soil. 463, 643–651. doi: 10.1007/s11104-021-04919-8
Pizano, C., Barón, A. F., Schuur, E. A. G., Crummer, K. G., and Mack, M. C. (2014). Effects of thermo-erosional disturbance on surface soil carbon and nitrogen dynamics in upland arctic tundra. Environ. Res. Lett. 9, 075006. doi: 10.1088/1748-9326/9/7/075006
Plaza, C., Pegoraro, E., Bracho, R., Celis, G., Crummer, K. G., Hutchings, J. A., et al. (2019). Direct observation of permafrost degradation and rapid soil carbon loss in tundra. Nat. Geosci. 12, 627–631. doi: 10.1038/s41561-019-0387-6
Randerson, J. T., Van Der Werf, G. R., Giglio, L., Collatz, G. J., and Kasibhatla, P. S. (2015). Global Fire Emissions Database. Version 4.1 (GFEDv4). ORNL DAAC.
Raynolds, M. K., Walker, D. A., Verbyla, D., and Munger, C. A. (2013). Patterns of Change within a Tundra Landscape: 22-year Landsat NDVI Trends in an Area of the Northern Foothills of the Brooks Range, Alaska. Arct. Antarct. Alp. Res. 45, 249–260. doi: 10.1657/1938-4246-45.2.249
Reichstein, M., Bahn, M., Ciais, P., Frank, D., Mahecha, M. D., Seneviratne, S. I., et al. (2013). Climate extremes and the carbon cycle. Nature. 500, 287–295. doi: 10.1038/nature12350
Sayedi, S. S., Abbott, B. W., Thornton, B. F., Frederick, J. M., Vonk, J. E., Overduin, P., et al. (2020). Subsea permafrost carbon stocks and climate change sensitivity estimated by expert assessment. Environ. Res. Lett. 15, 124075. doi: 10.1088/1748-9326/abcc29
Scholten, R. C., Jandt, R., Miller, E. A., Rogers, B. M., and Veraverbeke, S. (2021). Overwintering fires in boreal forests. Nature. 593, 399–404. doi: 10.1038/s41586-021-03437-y
Scholze, M., Buchwitz, M., Dorigo, W., Guanter, L., and Quegan, S. (2017). Reviews and syntheses: Systematic Earth observations for use in terrestrial carbon cycle data assimilation systems. Biogeosciences. 14, 3401–3429, doi: 10.5194/bg-14-3401-2017
Schuur, E.a. G, McGuire, A. D., Schädel, C., Grosse, G., Harden, J. W., et al. (2015). Climate change and the permafrost carbon feedback. Nature. 520, 171–179. doi: 10.1038/nature14338
Shiklomanov, N. I., and Nelson, F. E. (2013). “Thermokarst and civil infrastructure,” in Treatise on Geomorphology: Glacial and Periglacial Geomorphology, Vol.8, eds J. Shroder, R. Giardino, and J. Harbor (San Diego, CA: Academic Press), 354–373.
Stocks, B. J., Mason, J. A., Todd, J. B., Bosch, E. M., Wotton, B. M., Amiro, B. D., et al. (2002). Large forest fires in Canada, 1959–1997. J. Geophys. Res. 107, FFR 5-1-FFR 5–12. doi: 10.1029/2001JD000484
Turetsky, M. R., Abbott, B. W., Jones, M. C., Anthony, K. W., Olefeldt, D., Schuur, E. A. G., et al. (2020). Carbon release through abrupt permafrost thaw. Nat. Geosci. 13, 138–143. doi: 10.1038/s41561-019-0526-0
UCS (2020). (Union of Concerned Scientists). 2021. Each Country's Share of CO2 Emissions. Available online at: https://www.ucsusa.org/resources/each-countrys-share-co2-emissions. (accessed May 24, 2021)
van der Werf, G. R., Randerson, J. T., Giglio, L., van Leeuwen, T. T., Chen, Y., Rogers, B. M., et al. (2017). Global fire emissions estimates during 1997–2016. Earth Syst. Sci. Data. 9, 697–720. doi: 10.5194/essd-9-697-2017
Veraverbeke, S., Rogers, B. M., Goulden, M. L., Jandt, R. R., Miller, C. E., Wiggins, E. B., et al. (2017). Lightning as a major driver of recent large fire years in North American boreal forests. Nat. Clim. Change. 7, 529–534. doi: 10.1038/nclimate3329
Veraverbeke, S., Rogers, B. M., and Randerson, J. T. (2015). Daily burned area and carbon emissions from boreal fires in Alaska. Biogeosciences. 12, 3579–3601, doi: 10.5194/bg-12-3579-2015
Virkkala, A.-M., Aalto, J., Rogers, B. M., Tagesson, T., Treat, C. C., Natali, S. M., et al. (2021). Statistical upscaling of ecosystem CO2 fluxes across the terrestrial tundra and boreal domain: Regional patterns and uncertainties. Global Change Biology. 27, 4040–4059. doi: 10.1111/gcb.15659
Walker, X. J., Rogers, B. M., Baltzer, J. L., Cumming, S. G., Day, N. J., Goetz, S. J., et al. (2018). Cross-scale controls on carbon emissions from boreal forest megafires. Global Change Biol. 24, 4251–4265. doi: 10.1111/gcb.14287
Walker, X. J., Rogers, B. M., Veraverbeke, S., Johnstone, J. F., Baltzer, J. L., Barrett, K., et al. (2020). Fuel availability not fire weather controls boreal wildfire severity and carbon emissions. Nat. Clim. Change. 10, 1130–1136. doi: 10.1038/s41558-020-00920-8
Walsh, J. E., Ballinger, T. J., Euskirchen, E. S., Hanna, E., Mård, J., Overland, J. E., et al. (2020). Extreme weather and climate events in northern areas: a review. Earth-Sci. Rev. 209, 103324. doi: 10.1016/j.earscirev.2020.103324
Keywords: Arctic, Boreal, disturbance, fire, permafrost, thaw, carbon, climate
Citation: Treharne R, Rogers BM, Gasser T, MacDonald E and Natali S (2022) Identifying Barriers to Estimating Carbon Release From Interacting Feedbacks in a Warming Arctic. Front. Clim. 3:716464. doi: 10.3389/fclim.2021.716464
Received: 28 May 2021; Accepted: 14 December 2021;
Published: 24 January 2022.
Edited by:
Chris Derksen, Environment and Climate Change, CanadaReviewed by:
Yufei Zou, Independent Researcher, San Francisco Bay Area, United StatesPascale Roy-Léveillée, Université Laval, Canada
Adam Kirkwood, Laurentian University, Canada, in collaboration with reviewer PR-L
Copyright © 2022 Treharne, Rogers, Gasser, MacDonald and Natali. This is an open-access article distributed under the terms of the Creative Commons Attribution License (CC BY). The use, distribution or reproduction in other forums is permitted, provided the original author(s) and the copyright owner(s) are credited and that the original publication in this journal is cited, in accordance with accepted academic practice. No use, distribution or reproduction is permitted which does not comply with these terms.
*Correspondence: Rachael Treharne, cnRyZWhhcm5lQHdvb2R3ZWxsY2xpbWF0ZS5vcmc=