Knockdown of Genes Involved in Transcription and Splicing Reveals Novel RNAi Targets for Pest Control
- 1Department of Bioresources, Fraunhofer Institute for Molecular Biology and Applied Ecology, Giessen, Germany
- 2Corteva Agriscience, Indianapolis, IN, United States
- 3Institute for Insect Biotechnology, Justus Liebig University, Giessen, Germany
RNA interference (RNAi) is a promising next generation technology for the development of species-specific pest management. The key to successful RNAi based-plant protection is dependent in part on data-driven target gene selection, a challenging task due to the absence of laboratory strains and the seasonality of most pest species. In this study, we aimed to identify novel target genes by performing a knowledge-based approach in order to expand the spectrum of known potent RNAi targets. Recently, the protein-coding genes ncm, Rop, RPII-140, and dre4 have been identified as sensitive RNAi targets for pest control. Based on these potent RNAi targets, we constructed an interaction network and analyzed a selection of 30 genes in the model beetle Tribolium castaneum via injection of dsRNA synthesized by in vitro transcription. Nineteen of these targets induced significant mortality of over 70%, including six that caused 100% lethality. Orthologs of active T. castaneum RNAi targets were verified in the economically important coleopteran pests Diabrotica virgifera virgifera and Brassicogethes aeneus. Knockdown of D. v. virgifera genes coding for transcription factor Spt5, Spt6, and RNA polymerase II subunit RPII-33 caused over 90% mortality in larval feeding assays. Injection of dsRNA constructs targeting RPII-215 or the pre-mRNA-processing factor Prp19 into adult B. aeneus resulted in high lethality rates of 93 and 87%, respectively. In summary, the demonstrated knowledge-based approaches increased the probability of identifying novel lethal RNAi target genes from 2% (whole genome) to 36% (transcription- and splicing-related genes). In addition, performing RNAi pre-screening in a model insect increased also the probability of the identification essential genes in the difficult-to-work-with pest species D. v. virgifera and B. aeneus.
Introduction
The world population is expected to reach ~ 10 billion people in 2050, necessitating a substantial crop yield increase to meet the global food demand (Johnson and Jones, 2017; Rohr et al., 2019). An area where significant productivity gains can be made is the reduction of crop losses associated with insect pests, which is estimated at ca. 15% at a multicrop and worldwide scale (Oerke, 2006; Fletcher et al., 2020). The primary solution for insect control, the use of chemical pesticides, is facing challenges like resistance development and growing concerns of undesirable effects on the environment or non-target organisms. Genetically modified (GM) crops expressing insecticidal Bacillus thuringiensis (Bt) proteins provided a technology improvement on pest management that reduced the dependence on chemical insecticides (Phipps and Park, 2002; James, 2009; Areal and Riesgo, 2015). However, wide adoption of Bt trait technology has resulted in field-evolved resistance (Tabashnik et al., 2009, 2013). Consequently, new pest control strategies to overcome these obstacles are urgently required.
A promising alternative insect pest control strategy is the use of double-stranded RNAs (dsRNAs) to knock-down essential pest genes by triggering the conserved eukaryotic RNA interference (RNAi) pathway (Huvenne and Smagghe, 2010; Xue et al., 2012). Upon entry of gene-specific dsRNA into the cell of the intended pest organism, the expression of the targeted endogenous gene transcript will be knocked down post-transcriptionally and can result in insect mortality. Major advantages of this new approach are the rapid biological degradation of dsRNA in the environment and the sequence-specific nature of RNAi, allowing selective pest control that protects non-target organisms like pollinators or predatory insects (Dubelman et al., 2014; Albright et al., 2017; Parker et al., 2019). Baum et al. (2007) demonstrated that transgenic corn expressing a vacuolar ATPase subunit showed protection against feeding damage by Diabrotica virgifera virgifera LeConte (Coleoptera: Chrysomelidae) infestation. Recent examples of successful host-induced gene silencing (HIGS) applications include potato plants resistant to Colorado potato beetles (Zhang et al., 2015), as well as resistant maize plants silencing additional RNAi targets of D. v. virgifera (Hu et al., 2016) (reviewed in Koch and Kogel, 2014). The first commercialized transgenic event that uses HIGS for pest control, jointly developed by Monsanto and Dow AgroSciences, was approved by the three US regulatory agencies (EPA, FDA, and USDA). The combined-trait product known as SmartStax PRO® expresses both Bt toxins and dsRNA targeting the D. v. virgifera Snf7 gene (Head et al., 2017). As RNAi is a very active research area and an emerging pest management tool with potential for plant protection, it is just a question of time before SmartStax Pro and other applied RNA suppression products will appear on the market.
A key step in RNAi based pest control is the identification of novel bioinsecticide targets, a challenging task, as many pests lack genomic data and are often not easy to rear under laboratory conditions (Vogel et al., 2014). Performing large-scale RNAi pre-screens in a model organism was often proposed as a solution for target-site validation in pest insects (Alves et al., 2010; Knorr et al., 2013; Ulrich et al., 2015). Recently, a high throughput RNAi screen functionally analyzed 5000 genes of the model beetle Tribolium castaneum, revealing a panel of 100 lethal target genes that caused ≥ 90% mortality (Dönitz et al., 2014; Ulrich et al., 2015). A selection of these lethal T. castaneum RNAi targets was used to test for efficacy of orthologous genes in D. v. virgifera and the oilseed rape pest Brassicogethes aeneus (previously known as Meligethes aeneus). This approach identified the highly active target genes DNA-directed RNA polymerase II subunit (RPII-140), dre4 (homolog of SPT16), and ncm or nucampholin (homolog of CWC22) (Knorr et al., 2018). These sensitive RNAi targets encode polypeptides with essential functions at different steps of transcription and splicing. More specifically, dre4 is part of the FACT (facilitates chromatin transactions) complex that acts as a general chromatin regulator (Belotserkovskaya and Reinberg, 2004; Yang et al., 2016). The core splicing factor ncm is directing eukaryotic translation initiation factor 4A3 (eIF4AIII) into the exon junction complex (EJC) (Barbosa et al., 2012) and RPII-140 catalyzes the transcription of DNA into RNA (Falkenburg et al., 1987).
The primary goal of the current work was to expand on these potent T. castaneum RNAi targets by silencing functionally connected genes. Target gene selection was based on a knowledge based approach supported by functional Protein-Protein Interaction (PPI) networks generated by the STRING search tool (Mering et al., 2005; Szklarczyk et al., 2015) and KEGG (Kyoto Encyclopedia of Genes and Genomes) pathway information (Ogata et al., 1999). We screened 30 genes in T. castaneum and identified some potent novel RNAi targets that were active in both larval and adult stage. In addition, we evaluated if the newly discovered RNAi targets could be leveraged to the economically important pest species, the Western Corn Rootworm (WCR) D. v. virgifera, a devastating pest of maize in the US Corn Belt, and the pollen beetle B. aeneus, a key pest of oilseed rape. For both of these difficult-to-work with agricultural pest insects we identified novel RNAi target genes that caused more than 90% mortality, suggesting that these genes may be considered as potential RNAi target for RNA-based management.
Materials and Methods
Insect Rearing
Tribolium castaneum (San Bernardino strain) were maintained on wholegrain flour as described previously (Knorr et al., 2009). Adult B. aeneus were collected from flowering Brassica napus fields and reared on greenhouse-grown rape plants in a climate chamber at 24/18°C (day/night), 70% relative humidity and a 16:8 (light:dark) photoperiod. Non-diapausing D. v. virgifera eggs (Crop Characteristics, Inc. Farmington, MN) were washed from soil, followed by surface-sterilization with 10% formaldehyde and subsequent rinsing in water (Števo and Cagán, 2012). The eggs were hatched on artificial diet at 28°C, as described previously (Pleau et al., 2002; Števo and Cagán, 2012; Tan et al., 2016).
Protein-Protein Interaction (PPI) Data
The protein-protein interaction (PPI) data for dre4, ncm, and RPII-140 were imported from the open-source STRING (Search Tool for Recurring Instances of Neighboring Genes) v11.0 database (STRING, https://string-db.org/) (Szklarczyk et al., 2015).
RNA Isolation and Complementary DNA Synthesis
Total RNA was extracted from T. castaneum larvae and B. aeneus adults using Direct-zol™ RNA MiniPrep (Zymo Research, Irvine, California, USA) according to the manufacturer's instructions. cDNA was synthesized from 500 ng RNA using the First Strand cDNA Synthesis Kit (Thermo Fisher Scientific, Waltham, MA).
Total RNA from D. v. virgifera eggs, larvae, and adults was extracted using TRIzol (Life Technologies, Grand Island, NY) according to the manufacturer's instructions. cDNA was synthesized from 1 μg of total RNA using a SuperScript III reverse transcription kit (Thermo Fisher Scientific, Waltham, MA).
Identification of T. castaneum Gene Orthologs in D. v. virgifera and B. aeneus
The T. castaneum genome sequence ver.Tcas5.2 was used for the identification of ortholog proteins of the transcriptome of B. aeneus generated by Vogel et al. (2014) using NCBI BLASTP with an E-value of 0.01. The output were further filtered by a minimum protein identity of 50% as well as a protein coverage of at least 75% and lower than 125%. Resulting hits were ranked by score; redundant and overlapping sequences were removed. D. v. virgifera homologous were identified by performing TBLASTN searches with candidate protein coding sequences that were run against BLASTable databases comprising the unassembled D. v. virgifera sequence reads or the assembled contigs. Significant hits to a D. v. virgifera sequence (defined as lower than <1 × 10–20 for contig homologies and better than E-value of <1 × 10–10 for unassembled sequence reads homologies) were verified using BLASTX against the NCBI non-redundant database. The results of this BLASTX search confirmed that the D. v. virgifera homolog candidate gene sequences identified in the TBLASTN search indeed comprised D. v. virgifera genes, or were the best hits to the non D. v. virgifera candidate gene sequence present in the D. v. virgifera sequences. In the majority of cases, T. castaneum candidate genes that were annotated as encoding a protein, showed clear sequence homology to a sequence or sequences within the D. v. virgifera transcriptome. Sequencher™ v4.9 (Gene Codes Corporation, Ann Arbor, MI) was used in a few cases to assembled contigs that were partially-overlapping into longer contigs.
dsRNA Synthesis
The selected dsRNA sequences were screened against genomic and transcriptomic data to avoid complete matches of more than 19 nt to a potential off-target genes. Gene-specific primers for PCR (Supplementary Table 1) amplification were designed using VectorNTI (Invitrogen, Carlsbad, CA) or Primer3 software and contained a T7 promoter sequence (5′-TAATACGACTCACTATAGGGAGA- 3′) at their 5′ ends. The dsRNAs were synthesized using Ambion MEGAscript T7 (Thermo Fisher Scientific, Waltham, MA) according to the manufacturer's protocol and quantified on a NanoDrop 8000 spectrophotometer (Thermo Fisher Scientific, Waltham, MA).
Injection bioassays
Dorsolateral injection of 150 nl dsRNA [250 ng/μl] into T. castaneum larvae and B. aeneus adults was conducted using a glass capillary and a micromanipulator M3301 (World Precision Instruments, Sarasota, FL) under a dissecting stereomicroscope (n = 12). Negative controls received an equivalent amount of water or dsRNA corresponding to the Galleria mellonella metalloproteinase inhibitor (IMPI) gene (not present in B. aeneus) (Supplementary Table 1). Injected T. castaneum larvae were kept on whole-grain flour, whereas B. aeneus beetles were supplied with dried pollen and wet tissues as water source. Survival rates were monitored and insects counted every 48 h for 10 days.
Feeding bioassays
Brassicogethes aeneus and D. v. virgifera feeding bioassays were performed as described previously (Knorr et al., 2018). In brief, B. aeneus adults ingested dsRNA [500 ng/cm2] using artificial diet to ensure continuous uptake of dsRNA. Negative controls received an equivalent amount of water or dsRNA corresponding to the G. mellonella metalloproteinase inhibitor (IMPI) gene (Supplementary Table 1). The number of dead beetles was counted every 48 h for 10 days.
Neonate D. v. virgifera larvae were fed on artificial diet that was overlaid with dsRNA (500 ng/cm2) (Knorr et al., 2018). Water, 0.1X TE buffer and dsRNA targeting yellow fluorescent protein gene (YFP) were used as negative controls. The number of dead insects, and the weights of surviving insects were recorded after 9 days. Growth Inhibition (GI) was calculated based on the average weights of all controls, as follows: GI = [1 – (TWIT/TNIT)/(TWIBC/TNIBC)], where TWIT is the total weight of insects in the treatment, TNIT is the total number of insects in the treatment, TWIBC is the total weight of insects in the buffer control, and TNIBC is the total number of insects in the buffer control. To estimate the 50% lethal concentration (LC50) and the concentration that causes 50% growth inhibition (GI50) of active RNAi targets, 4-fold serial dilutions of dsRNAs were assayed. Values were calculated using log-logistic regression analysis in JMP® Pro from SAS Institute Inc (Supplementary Table 1).
Statistical Analysis
Bioassays were analyzed using an analyses of variance (ANOVA), followed by a Bonferroni-Holm test with significance threshold of p < 0.05 using Daniel's XL toolbox for Excel, version 7.3.4 68. Each experiment was compared to a control group and all experiments were conducted independently at least three times. LC50 (concentration at which 50% of the insects are dead) and GI50 (concentration that causes 50% growth inhibition or GI) values were calculated as described earlier (Knorr et al., 2018).
Results
Knockdown of Potential Target Genes in T. castaneum via Injection of dsRNA
The three most active RNAi targets RPII-140, Dre4, and ncm described in Knorr et al. (2018) are involved in pre- and post-transcriptional mRNA processing. We mined corresponding String PPI networks and KEGG pathway for potential RNAi targets and selected 30 genes for T. castaneum dsRNA injection assays: 18 genes coding for different RNA polymerase subunits (RP genes) and 12 genes involved in various transcriptional processes (non-RP genes) (Table 1, Figure 1).
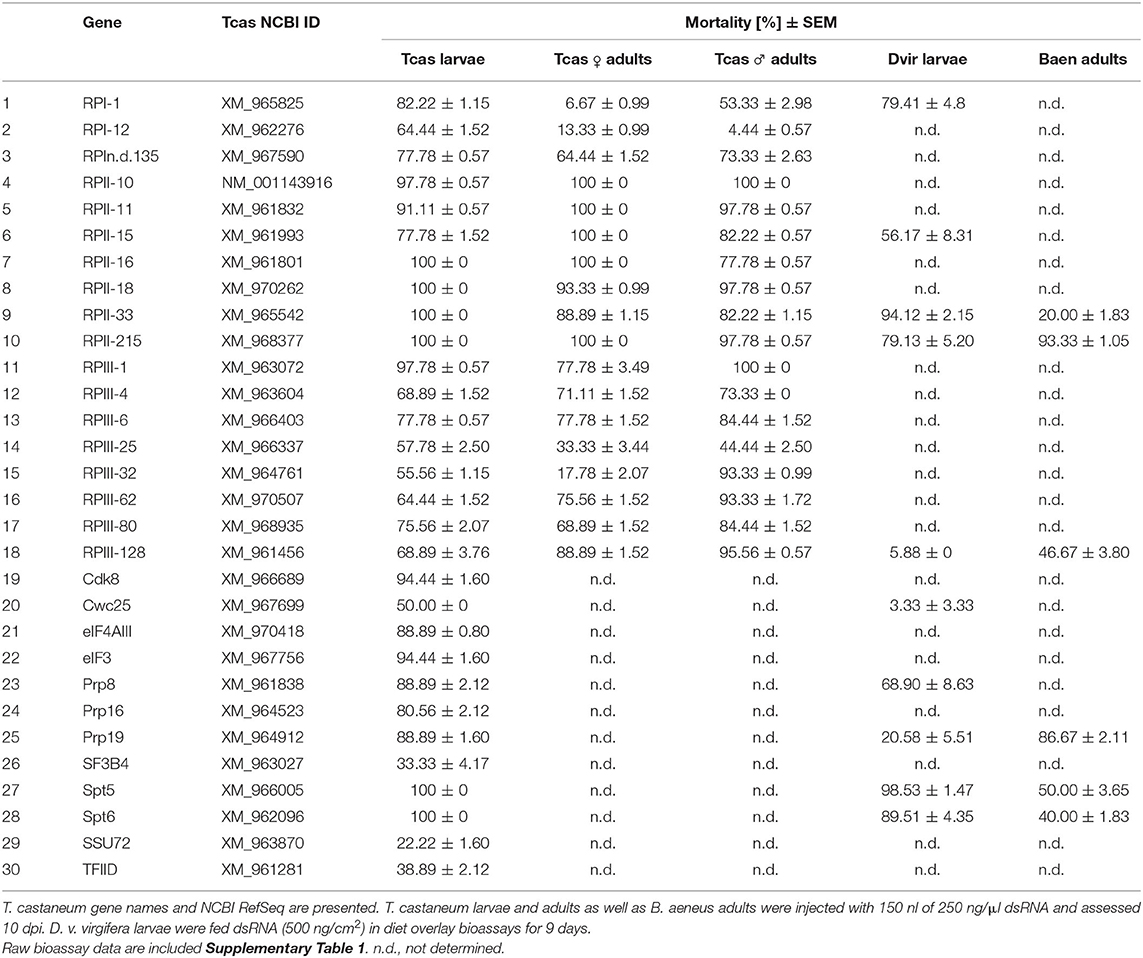
Table 1. Mean mortality (standard error % mortality in three replicates of bioassays) of 30 dsRNA targets 10 dpi in T. castaneum (Tcas), 9 dpf in D. v. virgifera (Dvir) and 10 dpi in B. aeneus (Bean).
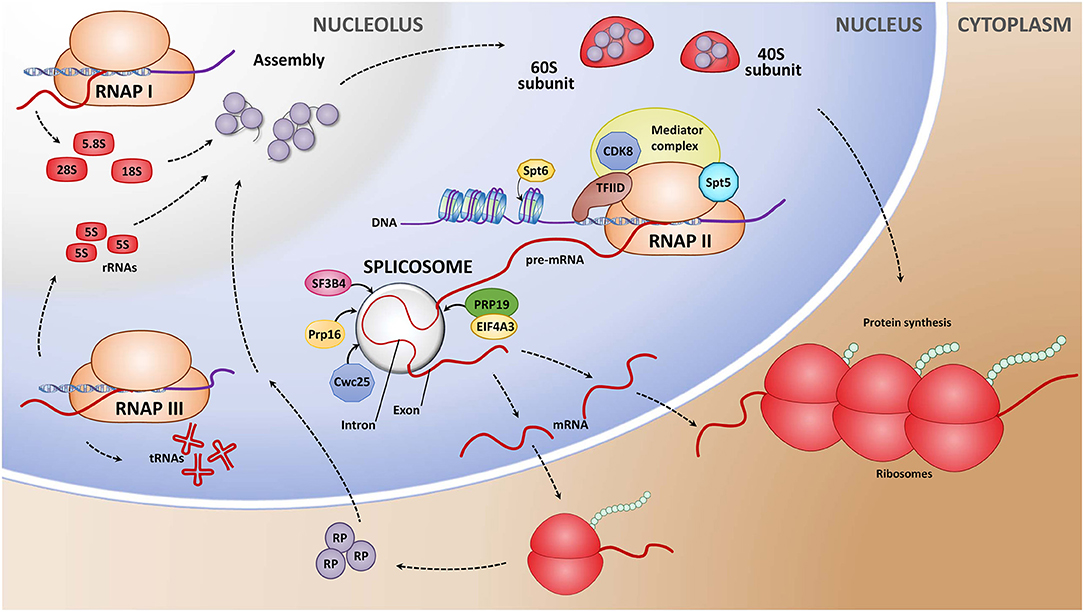
Figure 1. Simplified representation of eukaryotic gene expression and post-transcriptional processing, highlighting the genes that were selected as potential RNAi targets. Gene transcription in eukaryotic cells is carried out by three multisubunit RNA polymerases. RNA polymerase I, II, and III (RNAPI, RNPII, and RNAPIII, respectively). RNAPI synthesizes the larger ribosomal RNA (rRNAs) precursor 28S, 18S, and 5.8S, whereas RNAPIII synthesizes 5S rRNAs, transfer RNAs (tRNAs), and small non-coding RNAs sRNAs (Wild and Cramer, 2012; Yokoyama, 2019). Processed rRNAs form the pre-40S and pre-60S ribosomal subunits, which assemble to mature ribosomes in the cytoplasm. RNAPII transcribes sRNAs and precursor messenger RNA (pre-mRNA). The largest RNAPII subunit, the C-terminal domain (CTD), is flexible in structure and undergoes several conformational changes during gene transcription. These changes are regulated, in part, through alterations of the phosphorylation status that are mediated by phosphatases like SSU72 (Liu et al., 2020). The transcription initiation factor TFIID is a multimeric protein complex and plays a central role in mediating promoter responses to various activators and repressors (Lewis and Reinberg, 2003; Guo et al., 2018). Cyclin-dependent kinase 8 (Cdk8) is part of the mediator complex, a coactivator that is involved in regulating the gene transcription (Leclerc et al., 1996; Yin and Wang, 2014). Transcription elongation factor SPT6 enhances transcription elongation by binding to histone H3 and recruitment of other elongation factors like Spt5 (Ardehali et al., 2009; Duina, 2011). Spt5 is a component of the DRB sensitivity-inducing factor complex (DSIF complex) that both inhibits and stimulates transcription elongation (Jennings et al., 2004). Introns of the pre-mRNA are removed in a two-step transesterification reaction by a large ribonucleoprotein complex called the splicosome. Introns of the pre-mRNA are removed in a two-step transesterification reaction by a large ribonucleoprotein complex called the splicosome. The catalytic core of the spliceosome contains the pre-mRNA processing factor 8 (Prp8), which plays a central role in its molecular interactions during spliceosome formation (Grainger and Beggs, 2005). The pre-mRNA splicing factor Cwc25 is required for the first catalytic reaction within the splicosome (Chiu et al., 2009). The splicing factor 3B subunit 4 (SF3B4) encodes a core subunit of the SF3b complex, a part of the U2-type spliceosome (Xiong and Li, 2020). Structural changes of the spliceosome that allow the second reaction are mediated by the DEAH/H-box ATP-dependent RNA helicase Prp16 (Tseng et al., 2011). The large protein complex associated with the DEAH-box ATPase Prp19, named NTC (NineTeen Complex), stabilizes interactions of the activated splicosome (Tseng et al., 2011). The eukaryotic initiation factor 4A-III (eIF4AIII) is another DEAH-box ATP-dependent RNA helicase and a core component of the splicing-dependent multiprotein exon junction complex (EJC) that forms at the junction of two exons (Barbosa et al., 2012; Le Hir et al., 2015). The mature mRNAs are exported to the cytoplasma, were eukaryotic translation initiation factors (eIFs), like the eIF3 complex, stimulate their recruitment to the 40S ribosomal subunit for translation into protein (Poulin and Sonenberg, 2013, Valášek et al., 2017).
Homologs of the selected 30 genes were identified via BLAST (tblastn) homology searches using Drosophila melanogaster protein isoforms (see Supplementary Table 1 for GenBank IDs) as the queries. To test whether dsRNA targeting these genes may lead to lethal phenotypes in T. castaneum, we designed dsRNA constructs targeting a segment of the open reading frame and applied the dsRNA via injection into the larval hemocoel. Significant mortality of ≥ 50% was observed onset on the 4th day after injection of ~150 nl of 250 ng/μl dsRNA targeting the four genes cdk8, Prp8, Spt5, and Spt6 (Figure 2A). The detected mortality rates were significantly higher compared to the non-target IMPI dsRNA. Significant mortality of over 70% was caused by 19 out of the 30 applied dsRNA constructs 10 days post injection (dpi). The highest mortality of 100% was recorded for the six genes Spt5, Spt6, RPII-16, RPIII-18, RPII-33, and RPII-215 (Table 1, Figure 2). In addition, silencing RNA polymerase subunits (RPs) caused over 50% larval mortality in all 18 treatments 10 dpi (Table 1, Figure 2B).
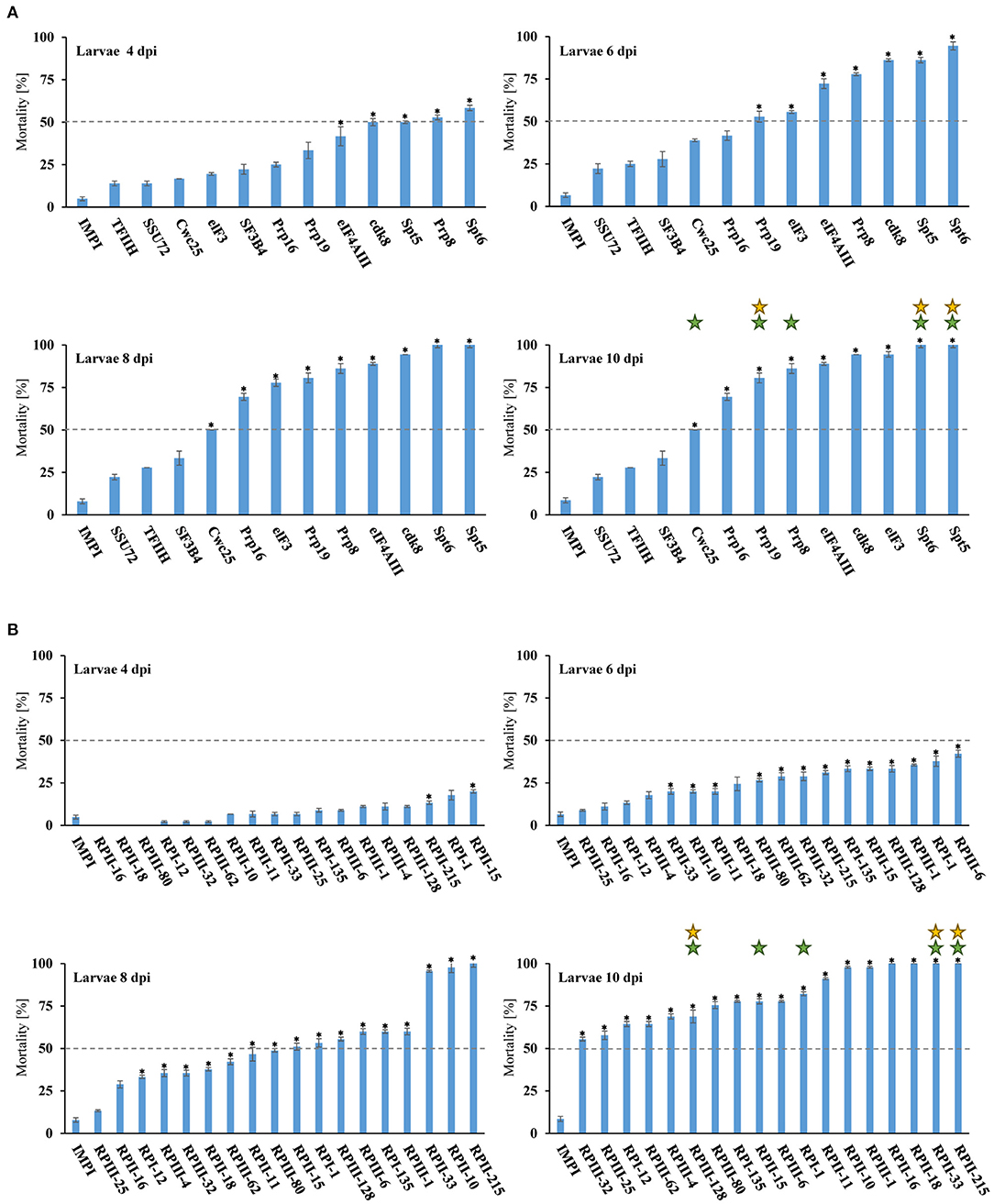
Figure 2. Mortality rates of T. castaneum larvae after knockdown. Injection of 150 nl of dsRNA [250 ng/μl] targeting genes involved in the process of transcription (A) and different RNA polymerase subsunits (B) into T. castaneum larvae. Mortality rates were tracked every 48 h for 10 days. The G. mellonella gene IMPI was used as negative control. The data represent three biological replicates (n = 15 for each replicate). Asterisks show statistical difference (p < 0.001). Green stars represents selected D. v. virgifera and yellow B. aeneus orthologs. The results were analyzed with analysis of variance (ANOVA) (Supplementary Table 1).
We also analyzed the potential lethal effect of the 18 RP dsRNA constructs in T. castaneum female and male adults. Significant mortality rates of over 50% were observed for 14 female and 16 male dsRNA RP constructs 10 dpi (Table 1, Figure 3), including six female and eight treatments that caused over 90% mortality, respectively (Table 1, Figure 3). RPII-215 was one of the most efficacious RNA targets, causing over 75% in females and even 97% mortality in males 6 dpi. Only knock-down of RPI-1 and RPIII-32 resulted in considerably lower female mortality rates of ~7 and ~18%, respectively. Whereas, in males silencing of RPI-1 led to >50% and RPIII-32 to >90% lethality.
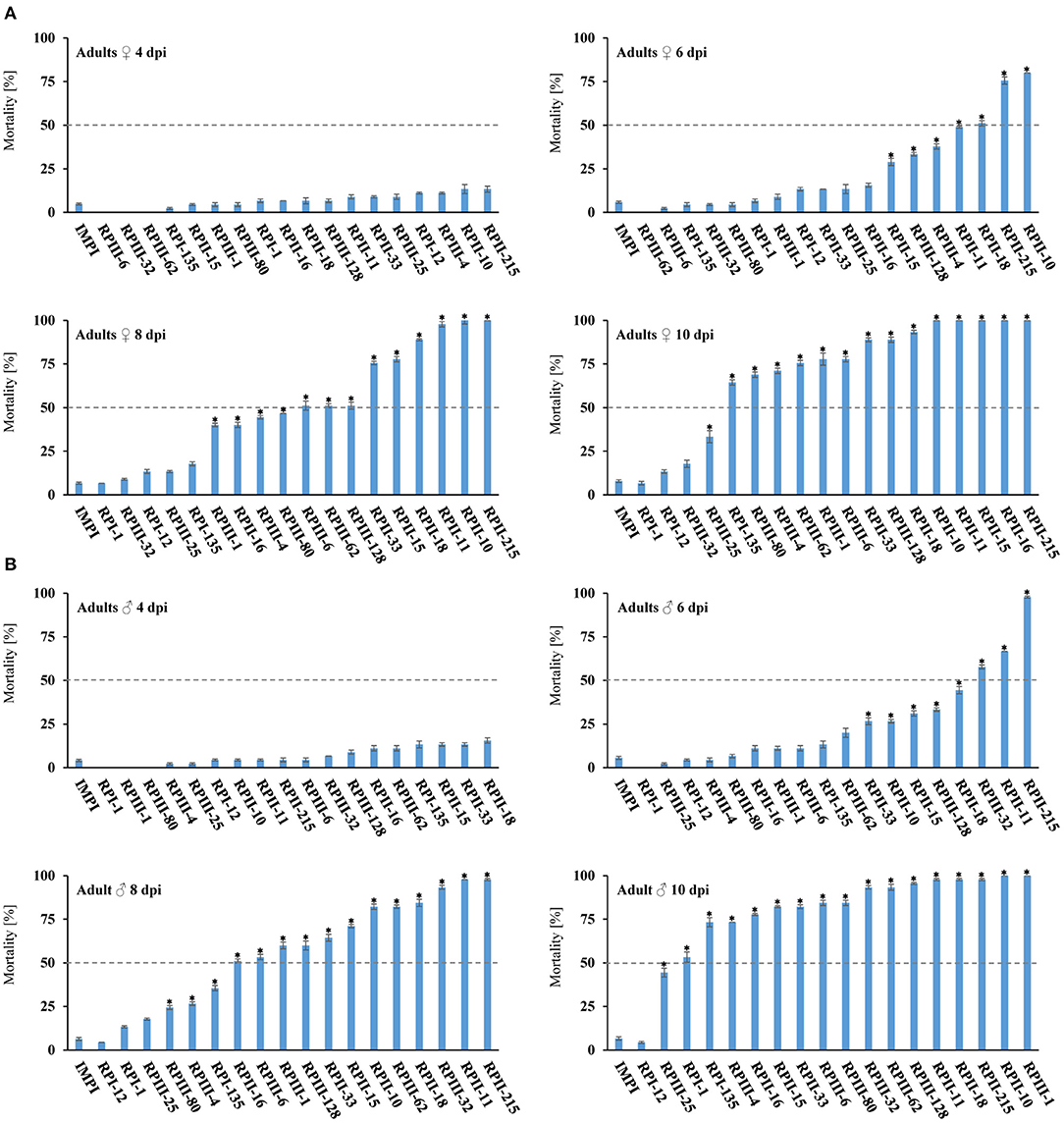
Figure 3. Mortality rates of T. castaneum adults after knockdown. Injection of 150 nl of dsRNA [250 ng/μl] targeting different RNA polymerase subunits into T. castaneum (A) female and (B) male adults. Mortality rates were tracked every 48 h for 10 days. The G. mellonella gene IMPI was used as negative control. The data represent three biological replicates (n = 15 for each replicate). Asterisks show statistical difference (p < 0.001). The results were analyzed with analysis of variance (ANOVA) (Supplementary Table 1).
Knockdown of Potential Target Genes in D. v. virgifera Larvae Upon Feeding
The four most lethal target genes Spt5, Spt6, RPII-215, and RPII-33, as well as the six target genes Prp8, Prp19, Cwc25, RPI-1, RPII-15, and RPIII-128 with moderate activity, were selected from the T. castaneum screen for D. v. virgifera knockdown experiments. Diet overlay bioassays using 500 ng/cm2 dsRNA identified seven out of the 10 dsRNA constructs that caused significant mortality of over 50%. Seven of the tested target genes, caused over 70% mortality (Figure 4A), including dsRNAs targeting Spt5, Spt6, and RPII-33 transcripts, which resulted in the highest mortality rates of 99, 90, and 88%, respectively. In addition, RNAi treated groups showed significant growth inhibition in D. v. virgifera ranging from 0.72 to 0.99 in all treatments, except for the Cwc25 treatment (Figure 4B, Supplementary Table 1).
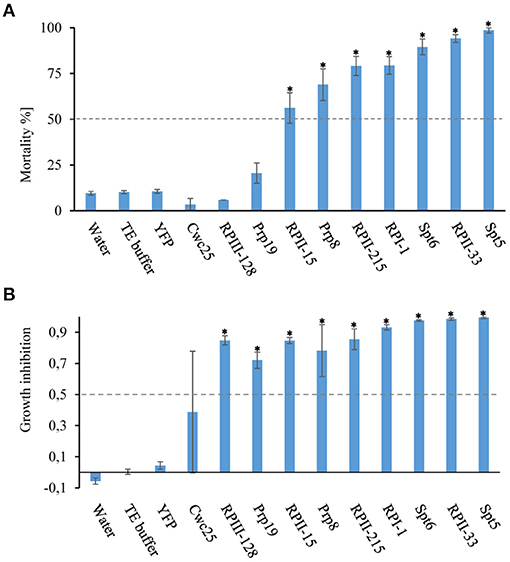
Figure 4. Mortality rates and GI value of D. v. virgifera after knockdown of genes involved in the process of transcription. Neonate D. v. virgifera larvae were fed on artificial diet that was surface-overlaid with dsRNA at 500 ng/cm2. Yellow fluorescent protein gene (YFP) dsRNA, 0.1X TE buffer and water were used as negative controls. (A) Mortality and (B) growth inhibition (GI) were evaluated after 9 days. See Supplementary Table 1 for number of replicates and standard error of the mean. Asterisks show statistical difference (p < 0.001) (Missing, need to be included). The data represent three biological replicates (n = 10 for each replicate). The results were analyzed with analysis of variance (ANOVA) (Supplementary Table 1).
In addition, the median lethal concentration (LC50) and median growth inhibition (GI50) values for dsRNA treatments that caused > 60% mortality were further investigated. A dilution series of dsRNA tested in diet-overlay bioassays led to LC50 values that ranged from 4.33 to 54.26 ng/cm2 (Table 2). The majority of dsRNAs tested resulted in LC50 values of <20 ng/cm2, in particular, the LC50 values of Spt5 and Spt6 were 4.33 and 4.83 ng/cm2, respectively. The GI50 values ranged from 1.38 to 27.92 ng/cm2 (Table 2), whereas Spt5 and Prp8 showed the lowest values of 1.38 and 2.40 ng/cm2.
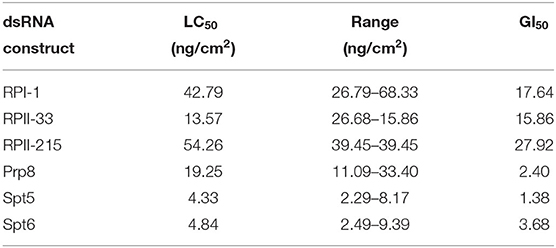
Table 2. LC50 and GI50 for RPI-1, RPII-33, RPII-215, Prp8, Spt5, and Spt6 in larval D. v. virgifera diet-based bioassay 9 dpf.
Knockdown of Potential Target Genes in B. aeneus Adults via Injection of dsRNA
The four most lethal target genes Spt5, Spt6, RPII-215, and RPII-33, as well as Prp19 and RPIII-128 as moderately potent target genes were selected from the T. castaneum screen for B. aeneus knockdown experiments. The ortholog sequences were identified from the B. aeneus transcriptome and gene-specific dsRNAs were designed. Injection of ~150 nl of 250 ng/μl into adult beetles caused significant mortality in five of the six tested dsRNA constructs 10 dpi, including high rates of 87% for the Prp19 and 94% for the RPII-215 dsRNA-treated groups (Figure 5). The most rapid response was observed after silencing RPII-215, which caused significant mortality at four dpi, which increased to over 50% six dpi and reaching 87% mortality on day eight of the bioassay (Figure 5).
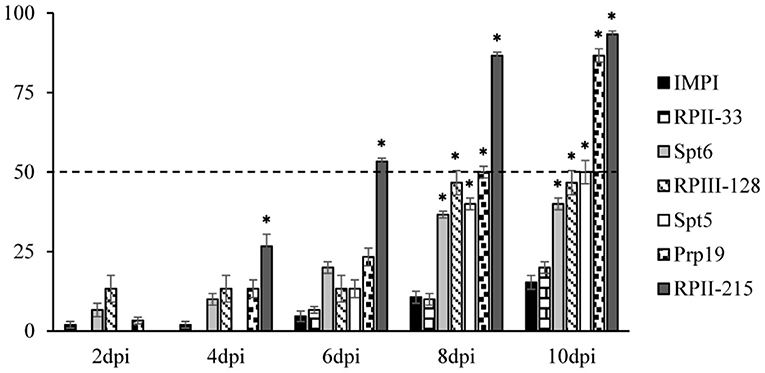
Figure 5. Survival rates of B. aeneus after knockdown of genes involved in the process of transcription. Injection of 150 nl of gene-specific dsRNA [250 ng/μl] was injected into B. aeneus adults. Mortality rates were tracked every 48 h for 10 days (dpi = days post injection). The G. mellonella gene IMPI was used as negative control. The data represent three biological replicates (n = 10 for each replicate). Asterisks show statistical difference (p < 0.001). The results were analyzed with analysis of variance (ANOVA) (Supplementary Table 1).
Discussion
Insect damage to crops along with climate change and the loss of arable land dedicated to agriculture threaten the ability to meet the food demand of a rapidly growing global population (FAO, 2017). Chemical insecticides are still the major control method for insect pests, but widespread resistance development and environmental concerns like non-target toxicity create the need for innovative and sustainable pest control methods (Tabashnik, 1994; Budzinski and Couderchet, 2018). RNAi-based crop protection is a new-generation technology for highly efficient, environmentally soundbiopesticides that target insect pests with great specificity. To date, insecticidal RNAi target genes have been identified in key biological systems of the insect physiology like digestion, defense mechanisms, vesicular trafficking, and detoxification (Mao et al., 2007; Bautista et al., 2009; Kim et al., 2015; Kola et al., 2015; Head et al., 2017; Knorr et al., 2018; Cooper et al., 2019). A major challenge for the development of RNAi applications, however, is the identification of novel sensitive target genes, in particular in non-model organisms that often lack genomic data or laboratory colonies (Vogel et al., 2014). Some researchers take advantage of known RNAi-sensitive genes in model organisms and perform targeted gene mining strategies to verify the orthologous RNAi target in other insects (Pitino et al., 2011; Wang et al., 2011; Zhao et al., 2011; Zhu et al., 2011; Li et al., 2013; Rodrigues et al., 2017; Mogilicherla et al., 2018; Koo et al., 2020). Recently, a knowledge-based approach demonstrated that the spectrum of known lethal RNAi targets could be expanded by silencing genes that are functionally connected (Bingsohn et al., 2017).
In this study, we mined the biological networks of RNA transcription and RNA processing, as silencing of genes with essential functions in these processes have demonstrated high mortality rates in three different coleopteran pests (Knorr et al., 2018). It has been shown that mRNA knockdown efficiency is not necessarily positively related to high mortality rates. Several uncontrollable factors can influence the RNAi response, like protein/mRNA half-life, gene function redundancy or the physiological importance of the targeted gene function (Schwinghammer et al., 2011; Li et al., 2013; Rinkevich and Scott, 2013; Bingsohn et al., 2017; Raje et al., 2018; Howell et al., 2020; Koo et al., 2020). Thus, we focused on organism-level analysis and did not consider gene expression as a key criterion for target selection. Out of 30 genes assayed in T. castaneum, 19 genes caused significant mortality of over 70%, including 11 target genes that lead to >90% mortality at day six post injection. Furthermore, silencing of RNA polymerase subunits in T. castaneum larvae caused significant mortality of at least 50% mortality in all 18 treatments and even 100% when knocking down RPII-215, RPII-33, RPII-18, and RPII-16. RNA polymerases subunits are also sensitive targets in adult beetles, both female and males, as knock down of seven subunits (RPI-1, RPII-10 RPII-11 RPII-15 RPII-16 RPII-215, and RPIII-1) led to 100% mortality 10 days after dsRNA injection. Taken together, 36% of the silenced transcription- and splicing-related genes were identified as highly effective RNAi targets (≥90%. mortality). In comparison, only two percent of 5000 randomly screened Tribolium genes in iBeetle showed mortality rates of over 90% (Dönitz et al., 2014; Ulrich et al., 2015). These results demonstrate that a knowledge-based approach can results in a higher probability of identifying efficient RNAi targets than high-throughput experiments.
To determine if the T. castaneum RNAi-sensitive targets could have a similar effect in other coleopteran pests, we identified orthologous sequences in D. v. virgifera and B. aeneus using transcriptome data mining. Significant growth inhibition was detected for all ten dsRNA constructs that were tested via artificial diet assays using neonate WCR larvae, out of which seven also caused significant mortality of over 50%. Silencing of the transcription elongation factors Spt5 and Spt6, two of the most active T. castaneum RNAi targets in this study, also caused high mortality rates of 99 and 90% in D. v. virgifera, respectively, and the lowest LC50 values of approximately 4 ng/cm2. Due to the lack of B. aeneus lab-colonies, pollen beetle experiments were limited to field collected beetles and focused on injection assays of six active T. castaneum target genes, namely Spt5, Spt6, Prp19, RPII-215, RPII-33, and RIII-128. Knockdown of Prp19 and RPII-215 led to high mortality rates of 87 and 94%, respectively. Silencing of RPII-215, resulted in the most rapid response of 50% mortality 6 days after treatment. Delivering dsRNA via injection is, of course, not a practical technique for RNAi field applications (Huvenne and Smagghe, 2010; Xue et al., 2012). Environmental RNAi response in B. aeneus was previously reported (Knorr et al., 2018; Willow et al., 2020a,b) but might lead to variable knockdown efficiency, which, in this initial study, we wanted to avoid by applying, standardized dsRNA doses to the beetles. Oral uptake of dsRNA might have different dynamics than injection and thus, lethal activity needs to be verified in feeding experiments before implementing results of this study in the greenhouse and the field.
Our study demonstrated a high sensitivity to RNAi of genes involved in RNA transcription and mRNA splicing, providing opportunities to screen for additional targets in these functional classes. In particular, silencing genes that are involved in RNAPII mediated mRNA transcription resulted in severe mortality, revealing a prime source for highly active RNAi targets. The Suppressor of Ty (SPT) genes Spt5 and Spt6, most active targets in T. castaneum and D. v. virgifera, encode polypeptides with essential functions in early transcription elongation. Spt5 forms with Spt4 the conserved heterodimer DSIF (DRB sensitivity-inducing factor) that regulates processive RNAPII transcription elongation (Price, 2000; Winston, 2001; Jennings et al., 2004; Decker, 2020). The histone chaperone Spt6 assembles histones into nucleosomes and is able to escalate the RNAPII elongation rate (Ardehali et al., 2009; McDonald et al., 2010).
Synthetic agrochemicals have traditionally been the method of choice for pollen beetle control in oil seed rape (OSR), but their excessive application, particularly pesticides of the pyrethroid class, has led to the development of populations with high level of resistance (Zimmer et al., 2014). The decreased sensitivity led to the introduction of non-cross-resistant alternative insecticides, such as neonicotinoids. However, adverse effects on non-target organisms like bees and other pollinators resulted in the ban of several neonicotinoids (Bass and Field, 2018). RNAi mediated pollen beetle control via spray-induced gene silencing (SIGS), whereby target-specific dsRNA is sprayed onto host plants, represents a promising, ecologically safe alternative. Foliar applications of naked dsRNA have already shown to be active against different Coleopteran species (e.g., de Andrade and Hunter, 2016; San Miguel and Scott, 2016). But, OSR Brassica napus crops have a short vulnerability window of only a few weeks, as agronomic crop loss is only caused by adults feeding from flower buds (Williams et al., 2010; Hervé and Cortesero, 2016). Thus, dose response studies need to identify most sensitive target genes in order to develop fast acting dsRNA applications. Formulation of dsRNA might further improve potential HIGS applications. dsRNA formulated with nanoparticles like BioClay or Guanylate Polymers demonstrated increased RNAi efficiency by overcoming problems like. stability or cell uptake (Mitter et al., 2017; Christiaens et al., 2018; Parsons et al., 2018). Behavioral studies have shown a preference of B. aeneus for turnip rape (Brassica rapa) over oilseed rape, which can be used for a push-pull strategy (Vinatier et al., 2012; Hervé and Cortesero, 2016; Skellern and Cook, 2018). Push-pull strategies involves integration of stimuli that deter insect pests away from their main crop (push), while simultaneously luring them to trap plants (pull) (Pyke et al., 1987; Cook et al., 2007). Combining HIGS with trap cropping would reduce the treated field area, leading to a more effective outcome of the dsRNA product.
RNAi represents a promising alternative for ecologically sustainable agricultural insect pest. Species-specific dsRNA based insecticides might complement other biosafe measures (e.g., conservation biocontrol) for managing agricultural pests and could become a part of integrated pest management (IPM) approaches. RNAi pre-screening in a model insect significantly increased the probability of identifying high levels of efficacious RNAi targets in pest species that lack genomic information. However, our data highlights the need to validate candidate targets in the insect species of concern because there is not a known correlation of gene RNAi sensitivity across insect species. Of the analyzed genes, 30–40% caused over 90% mortality in D. v. virgifera and B. aeneus. In addition, knockdown of RPII-215 resulted in significant mortality in all three coleopteran species: 100% in T. castaneum, 93% in B. aeneus and 79% in D. v. virgifera assays. These findings suggest that the function of these selected genes might be conserved across coleopterans. However, silencing of Prp19 resulted in high mortality rates of 81% in T. castaneum and 87% B. aeneus, but not in D. v. virgifera. Knockdown of Spt5, Spt6, and RPII-33 in T. castaneum was 100% lethal in all three cases and also caused significant mortality rates of 99, 90, and 98%, respectively, in D. v. virgifera. Targeting RPII-33 in B. aeneus did not led to any significant mortality rates, but silencing of Spt5 and Spt6 did, albeit to a lesser extent.
To summarize, we demonstrate that a knowledge-based approach increased the efficiency in discovering potent RNAi targets in Tribolium. Furthermore, identified T. castaneum RNAi targets were also highly efficacious in two additional coleopteran pest species, D. v. virgifera and B. aeneus, representing promising candidates for RNAi-based pest control. Next steps should focus on greenhouse and field demonstration of RNAi candidates that might become a promising component in future IPM programs.
Data Availability Statement
The original contributions presented in the study are included in the article/Supplementary Material, further inquiries can be directed to the corresponding author/s.
Author Contributions
EK, KN, and AV designed the study with input from other team members. MF, PG, and KA identified the D. v. virgifera target orthologs. AB identified the B. aeneus RNAi target orthologs. EK and LT designed and executed T. castaneum and B. aeneus experiments. MR and WL carried out D. v. virgifera diet bioassay. EK, EF, CG, AB, and KN interpreted the data. EK and KN composed the manuscript. All authors contributed to the article and approved the submitted version.
Funding
This work was funded by the Hessen State Ministry of Higher Education, Research and the Arts (HMWK) via the LOEWE Center for Insect Biotechnology and Bioresources. This research was also supported under a Fraunhofer IME-Corteva Agriscience joint research collaboration.
Conflict of Interest
PG, WL, and CG are employees of Corteva Agriscience. MF, KA, MR, EF, and KN are former employees of Corteva Agriscience.
The remaining authors declare that the research was conducted in the absence of any commercial or financial relationships that could be construed as a potential conflict of interest.
Publisher's Note
All claims expressed in this article are solely those of the authors and do not necessarily represent those of their affiliated organizations, or those of the publisher, the editors and the reviewers. Any product that may be evaluated in this article, or claim that may be made by its manufacturer, is not guaranteed or endorsed by the publisher.
Acknowledgments
We thank Jens Grotmann, Alica Klaus and Jannike Klädtke and Tobias Kessel for excellent technical support. The authors also thank the Corteva Agriscience insectary and insect bioassays teams.
Supplementary Material
The Supplementary Material for this article can be found online at: https://www.frontiersin.org/articles/10.3389/fagro.2021.715823/full#supplementary-material
References
Albright, V. C., Wong, C. R., Hellmich, R. L., and Coats, J. R. (2017). Dissipation of double-stranded RNA in aquatic microcosms. Environ. Toxicol. Chem. 36, 1249–1253. doi: 10.1002/etc.3648
Alves, A. P., Lorenzen, M. D., Beeman, R. W., Foster, J. E., and Siegfried, B. D. (2010). RNA interference as a method for target-site screening in the Western corn rootworm, Diabrotica virgifera virgifera. J. Insect Sci. 10:162. doi: 10.1673/031.010.14122
Ardehali, M. B., Yao, J., Adelman, K., Fuda, N. J., Petesch, S. J., Webb, W. W., et al. (2009). Spt6 enhances the elongation rate of RNA polymerase II in vivo. EMBO J. 28, 1067–1077. doi: 10.1038/emboj.2009.56
Areal, F. J., and Riesgo, L. (2015). Probability functions to build composite indicators. a methodology to measure environmental impacts of genetically modified crops. Ecol. Indicat. 52, 498–516. doi: 10.1016/j.ecolind.2015.01.008
Barbosa, I., Haque, N., Fiorini, F., Barrandon, C., Tomasetto, C., Blanchette, M., et al. (2012). Human CWC22 escorts the helicase eIF4AIII to spliceosomes and promotes exon junction complex assembly. Nat. Struct. Mol. Biol. 19, 983–U29. doi: 10.1038/nsmb.2380
Bass, C., and Field, L. M. (2018). Neonicotinoids. Curr. Biol. 28, R772–R773. doi: 10.1016/j.cub.2018.05.061
Baum, J. A., Bogaert, T., Clinton, W., Heck, G. R., Feldmann, P., Ilagan, O., et al. (2007). Control of coleopteran insect pests through RNA interference. Nat. Biotechnol. 25, 1322–1326. doi: 10.1038/nbt1359
Bautista, M. A. M., Miyata, T., Miura, K., and Tanaka, T. (2009). RNA interference-mediated knockdown of a cytochrome P450, CYP6BG1, from the diamondback moth, Plutella xylostella, reduces larval resistance to permethrin. Insect Biochem. Mol. Biol. 39, 38–46. doi: 10.1016/j.ibmb.2008.09.005
Belotserkovskaya, R., and Reinberg, D. (2004). Facts about FACT and transcript elongation through chromatin. Curr. Opin. Genet. Dev. 14, 139–146. doi: 10.1016/j.gde.2004.02.004
Bingsohn, L., Knorr, E., Billion, A., Narva, K. E., and Vilcinskas, A. (2017). Knockdown of genes in the Toll pathway reveals new lethal RNA interference targets for insect pest control. Insect Mol. Biol. 26, 92–102. doi: 10.1111/imb.12273
Budzinski, H., and Couderchet, M. (2018). Environmental and human health issues related to pesticides: from usage and environmental fate to impact. Environ. Sci. Pollut. Res. 25, 14277–14279. doi: 10.1007/s11356-018-1738-3
Chiu, Y.-F., Liu, Y.-C., Chiang, T.-W., Yeh, T.-C., Tseng, C.-K., Wu, N.-Y., et al. (2009). Cwc25 is a novel splicing factor required after Prp2 and Yju2 to facilitate the first catalytic reaction. Mol. Cell. Biol. 29, 5671–5678. doi: 10.1128/MCB.00773-09
Christiaens, O., Tardajos, M. G., Martinez Reyna, Z. L., Dash, M., Dubruel, P., and Smagghe, G. (2018). Increased RNAi efficacy in Spodoptera exigua via the formulation of dsRNA with guanylated polymers. Front. Physiol. 9:316. doi: 10.3389/fphys.2018.00316
Cook, S. M., Khan, Z. R., and Pickett, J. A. (2007). The use of push-pull strategies in integrated pest management. Annu. Rev. Entomol. 52, 375–400. doi: 10.1146/annurev.ento.52.110405.091407
Cooper, A. M., Silver, K., Zhang, J., Park, Y., and Zhu, K. Y. (2019). Molecular mechanisms influencing efficiency of RNA interference in insects. Pest Manag. Sci. 75, 18–28. doi: 10.1002/ps.5126
de Andrade, E. C., and Hunter, W. B. (2016). “RNA interference – natural gene-based technology for Highly Specific Pest Control (HiSPeC),” in RNA Interference, ed I. Y. Abdurakhmonov (Intech). doi: 10.5772/61612
Decker, T. M. (2020). Mechanisms of transcription elongation factor DSIF (Spt4-Spt5). J. Mol. Biol. 433:166657. doi: 10.1016/j.jmb.2020.09.016
Dönitz, J., Schmitt-Engel, C., Grossmann, D., Gerischer, L., Tech, M., Schoppmeier, M., et al. (2014). iBeetle-base. a database for RNAi phenotypes in the red flour beetle Tribolium castaneum. Nucleic Acids Res. 43, D720–725. doi: 10.1093/nar/gku1054
Dubelman, S., Fischer, J., Zapata, F., Huizinga, K., Jiang, C. J., Uffman, J., et al. (2014). Environmental fate of double-stranded RNA in agricultural soils. PLoS ONE 9:e93155. doi: 10.1371/journal.pone.0093155
Duina, A. A. (2011). Histone chaperones Spt6 and FACT: similarities and differences in modes of action at transcribed genes. Genet. Res. Int. 2011:625210. doi: 10.4061/2011/625210
Falkenburg, D., Dworniczak, B., Faust, D. M., and Bautz, E. K. F. (1987). RNA polymerase II of Drosophila. relation of its 140,000 Mr subunit to the β subunit of Escherichia coli RNA polymerase. J. Mol. Biol. 195, 929–937. doi: 10.1016/0022-2836(87)90496-7
Fletcher, S. J., Reeves, P. T., Hoang, B. T., and Mitter, N. (2020). A perspective on RNAi-based biopesticides. Front. Plant Sci. 11:51. doi: 10.3389/fpls.2020.00051
Grainger, R. J., and Beggs, J. D. (2005). Prp8 protein: at the heart of the spliceosome. RNA 11, 533–557. doi: 10.1261/rna.2220705
Guo, Z., Qin, J., Zhou, X., and Zhang, Y. (2018). Insect transcription factors: a landscape of their structures and biological functions in Drosophila and beyond. Int. J. Mol. Sci. 19:3691. doi: 10.3390/ijms19113691
Head, G. P., Carroll, M. W., Evans, S. P., Rule, D. M., Willse, A. R., Clark, T. L., et al. (2017). Evaluation of SmartStax and SmartStax PRO maize against western corn rootworm and northern corn rootworm. efficacy and resistance management. Pest Manage. Sci. 73, 1883–1899. doi: 10.1002/ps.4554
Hervé, M. R., and Cortesero, A. M. (2016). Potential for oilseed rape resistance in pollen beetle control. Arthropod Plant Interact. 10, 463–475. doi: 10.1007/s11829-016-9438-8
Howell, J. L., Mogilicherla, K., Gurusamy, D., and Palli, S. R. (2020). Development of RNAi methods to control the harlequin bug, Murgantia histrionica. Arch. Insect Biochem. Physiol. 104:e21690. doi: 10.1002/arch.21690
Hu, X., Richtman, N. M., Zhao, J. Z., Duncan, K. E., Niu, X., Procyk, L. A., et al. (2016). Discovery of midgut genes for the RNA interference control of corn rootworm. Sci. Rep. 6:30542. doi: 10.1603/ICE.2016.111203
Huvenne, H., and Smagghe, G. (2010). Mechanisms of dsRNA uptake in insects and potential of RNAi for pest control. a review. J. Insect Physiol. 56, 227–235. doi: 10.1016/j.jinsphys.2009.10.004
James, C. (2009). “Global status of commercialized biotech/GM crops: 2009,” in ISAAA Brief n.41 (Ithaca, NY: ISAAA).
Jennings, B. H., Shah, S., Yamaguchi, Y., Seki, M., Phillips, R. G., Handa, H., et al. (2004). Locus-specific requirements for Spt5 in transcriptional activation and repression in Drosophila. Curr. Biol. 14, 1680–1684. doi: 10.1016/j.cub.2004.08.066
Johnson, S. N., and Jones, T. H. (2017). Global Climate Change and Terrestrial Invertebrates. Chichester: John Wiley & Sons. doi: 10.1002/9781119070894
Kim, E., Park, Y., and Kim, Y. (2015). A transformed bacterium expressing double-stranded RNA specific to integrin β1 enhances Bt toxin efficacy against a polyphagous insect pest, Spodoptera exigua. PLoS ONE 10:e0132631. doi: 10.1371/journal.pone.0132631
Knorr, E., Bingsohn, L., Kanost, M. R., and Vilcinskas, A. (2013). Tribolium castaneum as a model for high-throughput RNAi screening. Adv. Biochem. Eng. Biotechnol. 136, 163–178. doi: 10.1007/10_2013_208
Knorr, E., Fishilevich, E., Tenbusch, L., Frey, M. L. F., Rangasamy, M., Billion, A., et al. (2018). Gene silencing in Tribolium castaneum as a tool for the targeted identification of candidate RNAi targets in crop pests. Sci. Rep. 8:2061. doi: 10.1038/s41598-018-20416-y
Knorr, E., Schmidtberg, H., Vilcinskas, A., and Altincicek, B. (2009). MMPs regulate both development and immunity in the Tribolium model insect. PLoS ONE 4:e4751. doi: 10.1371/journal.pone.0004751
Koch, A., and Kogel, K. H. (2014). New wind in the sails. improving the agronomic value of crop plants through RNAi-mediated gene silencing. Plant Biotechnol. J. 12, 821–831. doi: 10.1111/pbi.12226
Kola, V. S. R., Renuka, P., Madhav, M. S., and Mangrauthia, S. K. (2015). Key enzymes and proteins of crop insects as candidate for RNAi based gene silencing. Front. Physiol. 6:119. doi: 10.3389/fphys.2015.00119
Koo, J., Chereddy, S. C. R. R., and Palli, S. R. (2020). RNA interference-mediated control of cigarette beetle, Lasioderma serricorne. Arch. Insect Biochem. Physiol. 104:e21680. doi: 10.1002/arch.21680
Le Hir, H., Saulière, J., and Wang, Z. (2015). The exon junction complex as a node of post-transcriptional networks. Nat. Rev. Mol. Cell Biol. 17, 41–54. doi: 10.1038/nrm.2015.7
Leclerc, V., Tassan, J. P., O'Farrell, P. H., Nigg, E. A., and Léopold, P. (1996). Drosophila Cdk8, a kinase partner of cyclin C that interacts with the large subunit of RNA polymerase II. Mol. Biol. Cell 7, 505–513. doi: 10.1091/mbc.7.4.505
Lewis, B. A., and Reinberg, D. (2003). The mediator coactivator complex: functional and physical roles in transcriptional regulation. J. Cell Sci. 116, 3667–3675. doi: 10.1242/jcs.00734
Li, H., Jiang, W., Zhang, Z., Xing, Y., and Li, F. (2013). Transcriptome analysis and screening for potential target genes for RNAi-mediated pest control of the beet armyworm, Spodoptera exigua. PLoS ONE 8:e65931. doi: 10.1371/journal.pone.0065931
Liu, C., Zhang, W., and Xing, W. (2020). Diverse and conserved roles of the protein Ssu72 in eukaryotes: from yeast to higher organisms. Curr. Genet. 67, 195–206. doi: 10.1007/s00294-020-01132-5
Mao, Y.-B., Cai, W.-J., Wang, J.-W., Hong, G.-J., Tao, X.-Y., Wang, L.-J., et al. (2007). Silencing a cotton bollworm P450 monooxygenase gene by plant-mediated RNAi impairs larval tolerance of gossypol. Nat. Biotechnol. 25, 1307–1313. doi: 10.1038/nbt1352
McDonald, S. M., Close, D., Xin, H., Formosa, T., and Hill, C. P. (2010). Structure and biological importance of the Spn1-Spt6 interaction, and its regulatory role in nucleosome binding. Mol. Cell 40, 725–735. doi: 10.1016/j.molcel.2010.11.014
Mering, C., von Jensen, L. J., Snel, B., Hooper, S. D., Krupp, M., Foglierini, M., et al. (2005). STRING: known and predicted protein-protein associations, integrated and transferred across organisms. Nucleic Acids Res. 33 (Database issue):D433–7. doi: 10.1093/nar/gki005
Mitter, N., Worrall, E. A., Robinson, K. E., Xu, Z. P., and Carroll, B. J. (2017). Induction of virus resistance by exogenous application of double-stranded RNA. Curr. Opin. Virol. 26, 49–55. doi: 10.1016/j.coviro.2017.07.009
Mogilicherla, K., Howell, J. L., and Palli, S. R. (2018). Improving RNAi in the brown marmorated stink bug: identification of target genes and reference genes for RT-qPCR. Sci. Rep. 8:3720. doi: 10.1038/s41598-018-22035-z
Oerke, E.-C. (2006). Crop losses to pests. J. Agric. Sci. 144, 31–43. doi: 10.1017/S0021859605005708
Ogata, H., Goto, S., Sato, K., Fujibuchi, W., Bono, H., and Kanehisa, M. (1999). KEGG: kyoto encyclopedia of genes and genomes. Nucleic Acids Res. 27, 29–34. doi: 10.1093/nar/27.1.29
Parker, K. M., Barragán Borrero, V., van Leeuwen, D. M., Lever, M. A., Mateescu, B., and Sander, M. (2019). Environmental fate of RNA interference pesticides: adsorption and degradation of double-stranded RNA molecules in agricultural soils. Environ. Sci. Technol. 53, 3027–3036. doi: 10.1021/acs.est.8b05576
Parsons, K. H., Mondal, M. H., McCormick, C. L., and Flynt, A. S. (2018). Guanidinium-functionalized interpolyelectrolyte complexes enabling RNAi in resistant insect pests. Biomacromolecules 19, 1111–1117. doi: 10.1021/acs.biomac.7b01717
Phipps, R. H., and Park, J. R. (2002). Environmental benefits of genetically modified crops. global and European perspectives on their ability to reduce pesticide use. J. Animal Feed Sci. 11, 1–18. doi: 10.22358/jafs/67788/2002
Pitino, M., Coleman, A. D., Maffei, M. E., Ridout, C. J., and Hogenhout, S. A. (2011). Silencing of aphid genes by dsRNA feeding from plants. PLoS ONE 6:e25709. doi: 10.1371/journal.pone.0025709
Pleau, M. J., Huesing, J. E., Head, G. P., and Feir, D. J. (2002). Development of an artificial diet for the western corn rootworm. Entomol. Exp. Appl. 105, 1–11. doi: 10.1046/j.1570-7458.2002.01027.x
Poulin, F., and Sonenberg, N. (2013). “Mechanism of translation initiation in eukaryotes,” in Madame Curie Bioscience Database, eds F. Poulin and H. G. Nahum Sonenberg (Austin, TX: Landes Bioscience).
Price, D. H. (2000). P-TEFb, a cyclin-dependent kinase controlling elongation by RNA polymerase II. Mol. Cell. Biol. 20, 2629–2634. doi: 10.1128/MCB.20.8.2629-2634.2000
Pyke, B., Rice, M., Sabine, B., and Zalucki, M. P. (1987). The push-pull strategy—behavioural control of Heliothis. Aust. Cotton Grow. 9, 7–9.
Raje, K. R., Peterson, B. F., and Scharf, M. E. (2018). Screening of 57 candidate double-stranded RNAs for insecticidal activity against the pest termite Reticulitermes flavipes (Isoptera: Rhinotermitidae). J. Econ. Entomol. 111, 2782–2787. doi: 10.1093/jee/toy294
Rinkevich, F. D., and Scott, J. G. (2013). Limitations of RNAi of α6 nicotinic acetylcholine receptor subunits for assessing the in vivo sensitivity to spinosad. Insect Sci. 20, 101–108. doi: 10.1111/j.1744-7917.2012.01523.x
Rodrigues, T. B., Rieske, L. K. J., Duan, J., Mogilicherla, K., and Palli, S. R. (2017). Development of RNAi method for screening candidate genes to control emerald ash borer, Agrilus planipennis. Sci. Rep. 7:7379. doi: 10.1038/s41598-017-07605-x
Rohr, J. R., Barrett, C. B., Civitello, D. J., Craft, M. E., Delius, B., DeLeo, G. A., et al. (2019). Emerging human infectious diseases and the links to global food production. Nat. Sustain. 2, 445–456. doi: 10.1038/s41893-019-0293-3
San Miguel, K., and Scott, J. G. (2016). The next generation of insecticides. dsRNA is stable as a foliar-applied insecticide. Pest Manag. Sci. 72, 801–809. doi: 10.1002/ps.4056
Schwinghammer, M. A., Zhou, X., Kambhampati, S., Bennett, G. W., and Scharf, M. E. (2011). A novel gene from the takeout family involved in termite trail-following behavior. Gene 474, 12–21. doi: 10.1016/j.gene.2010.11.012
Skellern, M. P., and Cook, S. M. (2018). Prospects for improved off-crop habitat management for pollen beetle control in oilseed rape. Arthropod-Plant Interact. 12, 849–866. doi: 10.1007/s11829-018-9598-9
Števo, J., and Cagán, L. (2012). Washing solutions for the determination of the western corn rootworm eggs in soil. Cereal Res. Commun. 40, 147–156. doi: 10.1556/CRC.40.2012.1.16
Szklarczyk, D., Franceschini, A., Wyder, S., Forslund, K., Heller, D., Huerta-Cepas, J., et al. (2015). STRING v10. protein-protein interaction networks, integrated over the tree of life. Nucleic Acids Res. 43 (Database issue), D447–52. doi: 10.1093/nar/gku1003
Tabashnik, B. E. (1994). Evolution of resistance to Bacillus thuringiensis. Annu. Rev. Entomol. 39, 47–79. doi: 10.1146/annurev.en.39.010194.000403
Tabashnik, B. E., Brevault, T., and Carriere, Y. (2013). Insect resistance to Bt crops. lessons from the first billion acres. Nat. Biotechnol. 31, 510–521. doi: 10.1038/nbt.2597
Tabashnik, B. E., van Rensburg, J. B., and Carriere, Y. (2009). Field-evolved insect resistance to Bt crops. definition, theory, and data. J. Econ. Entomol. 102, 2011–2025. doi: 10.1603/029.102.0601
Tan, S. Y., Rangasamy, M., Wang, H., Velez, A. M., Hasler, J., McCaskill, D., et al. (2016). RNAi induced knockdown of a cadherin-like protein (EF531715) does not affect toxicity of Cry34/35Ab1 or Cry3Aa to Diabrotica virgifera virgifera larvae (Coleoptera: Chrysomelidae). Insect Biochem. Mol. Biol. 75, 117–124. doi: 10.1016/j.ibmb.2016.06.006
Tseng, C.-K., Liu, H.-L., and Cheng, S.-C. (2011). DEAH-box ATPase Prp16 has dual roles in remodeling of the spliceosome in catalytic steps. RNA 17, 145–154. doi: 10.1261/rna.2459611
Ulrich, J., Dao, V. A., Majumdar, U., Schmitt-Engel, C., Schwirz, J., Schultheis, D., et al. (2015). scale RNAi screen in Tribolium reveals novel target genes for pest control and the proteasome as prime target. BMC Genomics 16;9. doi: 10.1186/s12864-015-1880-y
Valášek, L. S., Zeman, J., Wagner, S., Beznosková, P., Pavlíková, Z., Mohammad, M. P., et al. (2017). Embraced by eIF3: structural and functional insights into the roles of eIF3 across the translation cycle. Nucleic Acids Res. 45, 10948–10968. doi: 10.1093/nar/gkx805
Vinatier, F., Gosme, M., and Valantin-Morison, M. (2012). A tool for testing integrated pest management strategies on a tritrophic system involving pollen beetle, its parasitoid and oilseed rape at the landscape scale. Landscape Ecol. 27, 1421–1433. doi: 10.1007/s10980-012-9795-3
Vogel, H., Badapanda, C., Knorr, E., and Vilcinskas, A. (2014). RNA-sequencing analysis reveals abundant developmental stage-specific and immunity-related genes in the pollen beetle Meligethes aeneus. Insect Mol. Biol. 23, 98–112. doi: 10.1111/imb.12067
Wang, Y., Zhang, H., Li, H., and Miao, X. (2011). Second-generation sequencing supply an effective way to screen RNAi targets in large scale for potential application in pest insect control. PLoS ONE 6:e18644. doi: 10.1371/journal.pone.0018644
Wild, T., and Cramer, P. (2012). Biogenesis of multisubunit RNA polymerases. Trends Biochem. Sci. 37, 99–105. doi: 10.1016/j.tibs.2011.12.001
Williams, I. H., Ferguson, A. W., Kruus, M., Veromann, E., and Warner, D. J. (2010). “Ground beetles as predators of oilseed rape pests: incidence, spatio-temporal distributions and feeding,” in Biocontrol-Based Integrated Management of Oilseed Rape Pests, eds I. H. Williams (Hg.) (Dordrecht: Springer), 115–149. doi: 10.1007/978-90-481-3983-5_4
Willow, J., Soonvald, L., Sulg, S., Kaasik, R., Silva, A. I., Taning, C. N. T., et al. (2020b). First evidence of bud feeding-induced RNAi in a crop pest via exogenous application of dsRNA. Insects 11:769. doi: 10.3390/insects11110769
Willow, J., Sulg, S., Taning, C. N. T., Silva, A. I., Christiaens, O., Kaasik, R., et al. (2020a). Targeting a coatomer protein complex-I gene via RNA interference results in effective lethality in the pollen beetle Brassicogethes aeneus. J. Pest Sci. 91, 447–458. doi: 10.1007/s10340-020-01288-6
Winston, F. (2001). Control of eukaryotic transcription elongation. Genome Biol. 2:REVIEWS1006. doi: 10.1186/gb-2001-2-2-reports0004
Xiong, F., and Li, S. (2020). SF3b4: a versatile player in eukaryotic cells. Front. Cell Dev. Biol. 8:14. doi: 10.3389/fcell.2020.00014
Xue, X.-Y., Mao, Y.-B., Tao, X.-Y., Huang, Y.-P., and Chen, X.-Y. (2012). “New approaches to agricultural insect pest control based on RNA interference. Adv. Insect Physiol. 42, 73–117. doi: 10.1016/B978-0-12-387680-5.00003-3
Yang, J., Zhang, X., Feng, J., Leng, H., Li, S., Xiao, J., et al. (2016). The histone chaperone FACT contributes to DNA replication-coupled nucleosome assembly. Cell Rep. 16:3414. doi: 10.1016/j.celrep.2016.08.070
Yin, J., and Wang, G. (2014). The Mediator complex: a master coordinator of transcription and cell lineage development. Development 141, 977–987. doi: 10.1242/dev.098392
Yokoyama, A. (2019). RNA polymerase II-dependent transcription initiated by selectivity factor 1: a central mechanism used by MLL fusion proteins in leukemic transformation. Front. Genet. 9:722. doi: 10.3389/fgene.2018.00722
Zhang, J., Khan, S., her, A, Hasse, C., Ruf, S., Heckel, D. G., and Bock, R. (2015): Full crop protection from an insect pest by expression of long double-stranded RNAs in plastids. Science 347, 991–994. doi: 10.1126/science.1261680
Zhao, Y. Y., Liu, F., Yang, G., and You, M. S. (2011). PsOr1, a potential target for RNA interference-based pest management. Insect Mol. Biol. 20, 97–104. doi: 10.1111/j.1365-2583.2010.01049.x
Zhu, F., Xu, J., Palli, R., Ferguson, J., and Palli, S. R. (2011). Ingested RNA interference for managing the populations of the Colorado potato beetle, Leptinotarsa decemlineata. Pest Manag. Sci. 67, 175–182. doi: 10.1002/ps.2048
Zimmer, C. T., Bass, C., Williamson, M. S., Kaussmann, M., Wolfel, K., and Gutbrod, O. (2014): Molecular functional characterization of CYP6BQ23, a cytochrome P450 conferring resistance to pyrethroids in European populations of pollen beetle, Meligethes aeneus. Insect Biochem. Mol. Biol. 45, 18–29. doi: 10.1016/j.ibmb.2013.11.008.
Keywords: Tribolium castaneum, insect pest control, RNAi target prediction, WCR, Diabrotica v virgifera, Brassicogethes aeneus, pollen beetle, biopesticide
Citation: Knorr E, Billion A, Fishilevich E, Tenbusch L, Frey MLF, Rangasamy M, Gandra P, Arora K, Lo W, Geng C, Vilcinskas A and Narva KE (2021) Knockdown of Genes Involved in Transcription and Splicing Reveals Novel RNAi Targets for Pest Control. Front. Agron. 3:715823. doi: 10.3389/fagro.2021.715823
Received: 27 May 2021; Accepted: 20 July 2021;
Published: 03 September 2021.
Edited by:
Salvatore Arpaia, Italian National Agency for New Technologies, Energy and Sustainable Economic Development (ENEA), ItalyReviewed by:
Luc Swevers, National Centre of Scientific Research Demokritos, GreeceJayendra Nath Shukla, University of Kentucky, United States
Copyright © 2021 Knorr, Billion, Fishilevich, Tenbusch, Frey, Rangasamy, Gandra, Arora, Lo, Geng, Vilcinskas and Narva. This is an open-access article distributed under the terms of the Creative Commons Attribution License (CC BY). The use, distribution or reproduction in other forums is permitted, provided the original author(s) and the copyright owner(s) are credited and that the original publication in this journal is cited, in accordance with accepted academic practice. No use, distribution or reproduction is permitted which does not comply with these terms.
*Correspondence: Eileen Knorr, eileen.knorr@ime.fraunhofer.de