- Institute of Crop Science and Plant Breeding, Christian-Albrechts-University, Kiel, Germany
Nitrogen (N) loss by leaching is a major concern in common crop rotations in Central Europe due to high post-harvest soil mineral N, low N uptake in autumn/winter, and percolation. This can lead to eutrophication of water bodies and indirect greenhouse gas emissions. One potential solution is to control microbial immobilization through the incorporation of organic matter. To examine the effectiveness of this approach, we compared the incorporation of crop residues from winter oilseed rape, faba beans, and winter wheat with the application of sawdust in a field-scale experiment. Field observations of the impact on autumn/winter N-dynamics were supplemented with model-derived parameters. Our results showed that the incorporation of crop residues significantly reduced autumn net mineralization in topsoil, with the largest reduction seen with cereal straw. However, the temporal dynamics of the effect did not meet the desired time frame, and the impact on N leaching was low. Field data, modeling processes, and a theoretical amendment assessment suggested that resistant organic matter accumulated under the given environmental conditions, probably resulting in a long-term effect on nutrient dynamics. In conclusion, incorporating crop residues from the preceding crop is the best option to date for mitigating post-harvest N losses and improving soil health with minimal crop management effort. However, controlling microbial immobilization through organic matter incorporation can be a useful measure whose effectiveness depends on the amendment used and field conditions.
1 Introduction
The combination of high post-harvest soil mineral N (SMN), low nitrogen (N) uptake in autumn/winter, and percolation causes a serious risk of N losses (Justes et al., 1999; Sieling and Kage, 2006; Engström and Lindén, 2012). In arable cropping systems of central Europe, these factors are for instance realized after winter oilseed rape (WOSR) and faba beans (FB), followed by winter wheat (WW) (Stützel and Kage, 1998; Sieling and Kage, 2010). In addition to direct N2O emissions (Bouwman, 1996) and ammonia volatilization from decomposing leaves (Nemitz et al., 2000), nitrate ( ) leaching is one of the most important pathways for N losses in autumn/winter (Di and Cameron, 2002). N leaching contributes to the eutrophication of water bodies and indirect N2O emissions (IPCC, 2006).
Ideally, N supply by the soil should be synchronized with N demand by the crop. In the case of WW in Northern Germany, substantial N uptake does not start before March, and the N transfer from crop residues and unused autumnal soil N mineralization into wheat is often low. In addition to lowering the overall mineralization intensity by reducing tillage operations after harvest (Goss et al., 1993; Di and Cameron, 2002; Beaudoin et al., 2005) or introducing catch crops, microbial N immobilization can reduce leaching losses (Nicholson et al., 1997; Trinsoutrot et al., 2000). The idea is that microbes degrading carbon-rich organic matter, so-called high carbon amendments (HCA), consume SMN to build enzymes and microbial biomass (Hoffmann et al., 1997; Yansheng et al., 2020). Nitrogen would be conserved in organic compounds and at best re-mineralized, when the crop N uptake increases, leading to fertilizer savings, increased nutrient use efficiency, and lower greenhouse gas emissions. Many studies proved this mechanism in lab and field experiments (Jensen, 1994; Mary et al., 1996; Henke et al., 2008; Engström and Lindén, 2012; Masunga et al., 2016). Whereas the findings of the first are clearly related to controlled lab conditions, the results of the latter are also often limited in their applicability to certain local field conditions (climate, soil, and crops).
The development of (regional) stakeholder recommendations requires detailed information about the consequences of amendment application for a specific site and a certain variety of weather conditions. To adjust fertilization in spring and summer, a farmer needs to know how prior management options affect the soil N status. To assess the environmental impact of cropping techniques, it is essential to know in which way they affect the fate of N from different sources (residual mineral fertilizer, soil organic matter (SOM), or residues). Another factor to ensure the feasibility of proposed measures is the local availability of the resources.
Therefore, we decided to incorporate crop residues of WOSR, FB, and WW as well as sawdust in a 3-year field trial. The aim was to quantify the impact of these amendments on the autumn SMN dynamics and their potential to reduce N leaching. In the current context, nitrate translocated below 120 cm till March is assumed to be out of reach of the wheat roots and therefore considered leached.
Provided rainfall and percolating water, most N leaching from arable soils occurs when N supply (sum of mineral N) in autumn and early winter exceeds N uptake by the crop (Sieling and Kage, 2006). Therefore, this period will be the focus of the following examination.
Measurements were supplemented with model-derived estimates of N leaching and mineralization dynamics and analyzed for the impact on the autumn/winter N-dynamics. Although the resulting selection differs in many aspects, the amendments are mainly characterized by a C:N gradient among them. Based on the literature, we hypothesized that the more C and the less N are applied (HCA = wide C:N ratio), the more SMN will be retained in topsoil. A model-based estimation of the immobilization potential confirmed this assumption. Decreasing SMN content or at least a buffered increase was expected. The more N is fixed in organic compounds, the less mineral N is at risk of translocation, and consequently, N leaching should be reduced.
2 Material and methods
2.1 Site description
The field trial was conducted from 2015 to 2018 at the experimental station Hohenschulen near Kiel (Northern Germany), situated in a young moraine drift landscape. The soil is a sandy to clayey loam with high small-scale heterogeneity. The average pH (CaCl2) was 6.5 ± 0.17, and soil organic carbon (SOC) content was 1.3 ± 0.1% (Reichel et al., 2018). The climate is classified as fully humid warm temperate with a long-term average annual temperature of 8.8°C and average annual precipitation of 718 mm (1993–2017).
The experimental years were quite contrasting in precipitation and temperature. The precipitation sums of the focus periods (1 August to 15 March) were in the interquartile range (IQR) of the long-term average (Supplementary Figure 1). In 2015/2016 and 2017/2018, the upper boundaries were reached. Whereas in 2016/2017, the precipitation sum in March was the lowest of all three experimental years. Regarding the average weekly temperatures, in all 3 years, the upper quartile of the average was exceeded far for 5–8 weeks in fall/winter. In contrast, only in 3–4 weeks per period, the value fell a little below the lower quartile.
2.2 Experiment design
For the field trial, the two common local crop rotations of WOSR as the preceding crop to WW followed by winter barley (WB) and FB–WW–WB were established (Figure 1). In all years, each crop of both rotations was cultivated on main plots (33 × 30 m2) in fourfold replication (n = 24 main plots). Within a quarter of each main plot, a sub-plot of 3 × 15 m2 was treated with one of four amendment treatments (Table 1) prior to the sowing of WW.
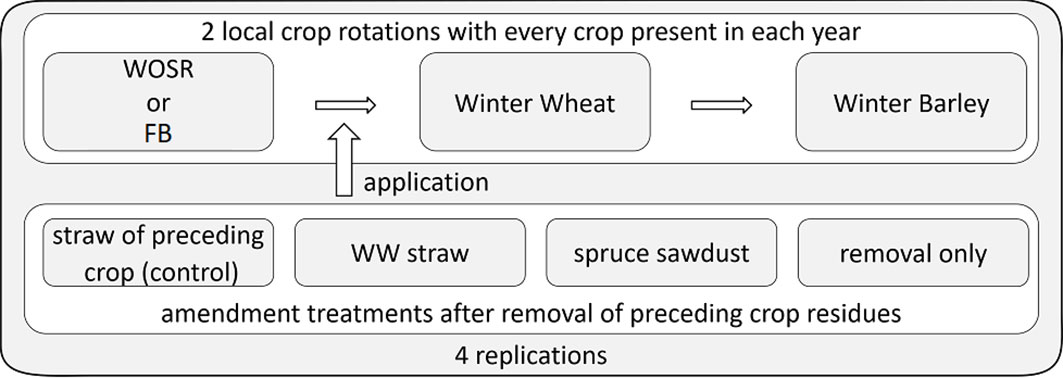
Figure 1 Scheme of the experimental setup. WOSR, winter oilseed rape; FB, faba beans; WW, winter wheat.
Thus, the design was crop (n = 2 rotations × 3 species), post-harvest amendment (n = 4), and replication (n = 4) summing up to 96 plots. However, this study comprises only data from WW, which was treated in the current year. The treatment sub-plots were separated from each other and the surrounding area by buffer plots receiving the same treatment except for the HCA application.
The selection of amendments was governed by practical aspects, i.e., a notable potential to immobilize N, local availability, and the possibility for coupled use of (agricultural) resources. The common local practice to retain the preceding crop residues on the field was chosen as the control treatment. Although there is typically no alternative use for WOSR and FB straw, they were considered for biogas production (Lönnqvist et al., 2013) and dairy cow fodder (Moss, 2002). Therefore, the mere removal of above-ground residues without any amendment was the contrast treatment at the lower end of the C:N gradient. WW straw could be procured most efficiently through farm-internal displacement. Additionally, the cereal straw shows a considerably wider C:N ratio than both preceding crop residues, promising an increased immobilization effect. Sawdust was chosen due to its extremely wide C:N ratio. Local availability should be given in wood-rich Central Europe. The applied amounts of residues were based on the actual yield (for the preceding crops averaged per year over the entire experimental site, for WW it was set to 10 t ha−1). Regarding sawdust, the dry matter equivalent to wheat straw was applied. Consequently, amendment treatments differed in C:N ratio as well as in absolute amounts of C and N applied.
With the use of a proven basic C/N turnover modeling approach (Verberne et al., 1990), the potential, stoichiometric N immobilization (Ipot) for the different HCAs was calculated (Equation 1, Table 1). The parameter represents an estimate of the HCA’s impact on C/N mineralization, thereby not considering the temporal component. Since most of the parameters are well established in mineralization modeling, the outcome is mainly affected by the total biomass of the input (CResidues) as well as by its C:N ratio.
with decomposition efficiency of plant matter to soil biome E = 0.4 (Verberne et al., 1990) and C:N ratio of the soil microbiome CNbiom = 8 (Hassink (unpublished) in Verberne et al. (1990).
As preparation for the treatments, above-ground residues of WOSR and FB were removed after harvest. Amendments were applied shortly after that (2–3 weeks) and prior to the sowing of WW. Due to chopping during removal and application, the resulting particle size of the straws varied between 1 and 10 cm in length. Sawdust particles were approximately 2–5 mm in diameter. Amendments were incorporated immediately after application in the top 10 cm of soil using a short disk harrow. Seed-bed preparation required plowing (30 cm deep) shortly before WW sowing. The preceding WOSR was fertilized with two applications of calcium ammonium nitrate (120 kg N ha−1 each) at EC27 and EC31, applied dry with a spreader. This amount of N is intentionally higher than the local optimum to induce high residual N levels after harvest. As a legume, FB was not fertilized.
2.3 Soil sampling and mineral N estimation
To monitor N dynamics and fate, soil samples down to 90-cm depth were taken and divided into 30-cm layers at decisive time points (after harvest, at the end of autumn growth, and prior to spring fertilization). Additional samples, mostly taken down to only 30 cm, were collected at irregular intervals between these dates. While isotopic labeling and lysimeters allow for more accurate tracking of N fate, their scalability is limited due to their high cost. Therefore, in this study, at least three cores per plot were pooled, and samples were immediately kept cool in the field in insulated ice boxes. Subsequently, the samples were stored frozen at −18°C until processing in the lab. Soil mineral N (SMN, the sum of and ) was determined with a spectrophotometer after extraction of 50 g soil with 400 ml of a 0.0125 M CaCl2 solution and 45 min of mechanical shaking followed by centrifugation (VDLUFA, 2002). Despite the potential underestimation of levels, as reported in the literature (Mulvaney et al., 2016), the 0.0125 M CaCl2 extraction method was used in this study. This method is widely accepted as the standard for determining SMN in arable soils in Germany, ensuring high comparability with studies from the same context.
A subsample was dried at 105°C to determine the gravimetric soil water content (SWC). The latter was converted to volumetric SWC via multiplication with estimated bulk densities of the three soil layers (1.45 g cm−3 for 0–30 cm, 1.60 g cm−3 for 30–60 cm, and 1.70 g cm−3 for 60–90 cm).
2.4 Model
The experiment was numerically simulated on daily time steps with a modular plant–soil–atmosphere model (PSAM) implemented in the HUME modeling environment (Kage and Stützel, 1999; Kage et al., 2003; Henke et al., 2008). High soil heterogeneity at the site caused a large variance in the soil parameters; therefore, we decided to simulate on a single plot scale (=four replications per treatment and year). Although this required an increased effort of parameterization, the approach enables statistical analysis for the modeled results as for the directly measured field data.
A detailed description of the model is given in Räbiger et al. (2020). The basic approach is described briefly in the following: based on daily climate data (i.e., precipitation, air temperature, humidity, wind speed, and global radiation), a crop growth model quantifies transpiration and N uptake by WW.
A mineralization submodel simulates the C and N turnover of soil organic matter and added amendments. The mineralization mechanics are based on the approach of DAISY (Hansen et al., 1990); i.e., dynamics are calculated as first-order kinetics of four organic C pools (stable organic matter (SOM), soil microbiome (BIOM), decomposable plant matter (DPM), and recalcitrant plant matter (RPM)) with corresponding N compartments (related via specific C:N ratios). The non-linear impact of abiotic conditions on mineralization (fabiot) was assessed with empirical functions according to Hansen et al. (1990): assuming maximal mineralization at 28°C and 20 vol% water content, a reduction factor was calculated for the current conditions (i.e., soil temperature, soil moisture, and fraction of clay) resulting in daily mineralization rates below maximum. The cumulative sum of fabiot for a certain period represents days of optimal mineralization conditions, which were used in further analyses.
The general formula for the impact rating is expressed in Equation 1, assuming that there is no interaction between the effect of soil temperature and soil water status and that their combined effect is multiplicative (Hansen et al., 1990).
The functions f(W), f(T), and f(Clay) (Supplementary Equations I–III) reflect the limitations imposed by moisture and temperature in dependency on the soil’s clay content.
Vertical water transport, losses via evapotranspiration, and accumulated N leaching were calculated by further specific submodels relying on a numerical solution of the Richards equation and the convection–dispersion equation (Richards, 1931; Feddes and Zaradny, 1978).
The accuracy of the prediction of parameters such as N leaching essentially depends on the simulation quality of the vertical soil water flow and SMN dynamics. Therefore, model parameterization and evaluation were conducted with care, as follows. Whereas most of the relevant parameters were adopted from the literature (Supplementary Table A.1), only a few had to be adjusted in regard to the site and amendment properties.
Initial values of SMN and SWC were derived from the field measurements on the plot level. Hence, the start of the simulation period was set at the date of the first soil sampling after the preceding crop harvest. Initial SMN values below the measurement depth of 90 cm (100 to 200 cm) were initialized with 90% of SMN60–90cm but with at least 2 kg N ha−1 in 10-cm layers. Initial SWC below 90 cm was calculated with a water potential gradient of −10 hPa per 10 cm on the basis of the water potential at 90 cm.
Measurement values of SMN and SWC from other than the first samplings per season were used to parameterize the model. Starting with established parameters from previous studies at the same site (Henke et al., 2008; Räbiger et al., 2020), the Levenberg–Marquardt algorithm was applied to adjust the selected values of the model (Supplementary Table A.1).
Soil textures were fitted on the main plot level, i.e., overarching amendment treatments, which is therefore the smallest unit of uniform soil type. As especially soil textures below 30 cm varied, a more small-scale, plot-specific adaptation of the van Genuchten parameter θs (water content at saturation, (van Genuchten, 1980) was necessary as a small-scale refinement of the assigned textures to improve the fit to measured SWC.
The initial organic C contents of SOM and the BIOM were estimated from humus content by constant factors. To avoid potential interaction with the amendment effect, humus content was fitted from an initial value of 1.5% on the main plot level using only the validation measurements of the respective plot without amendment.
Furthermore, amendment-specific adaptations were required: C and N from added biomass (HCA application) are represented by the RPM (lignified components) and DPM (carbohydrates and proteins) pools. The C:N ratio of the added matter determines the initial quantitative distribution to the compartments (Supplementary Equations IV–VI). With a C:N ratio of DPM set to 6 (proven reliable in numerous studies), to avoid negative DPM values, the C:N ratio of the RPM pool must exceed the C:N ratio of the respective amendment. For the preceding crop residues as well as for WW straw, a value of 150 resulted in a plausible distribution of organic C and N. For sawdust, it had to be increased to 792. It should be noted that compartment C:N only concerns the initial load after amendment incorporation, not the subsequent mineralization processes.
In contrast, turnover parameters have a decisive impact on biomass decomposition, which in turn depends on the biochemical composition of the organic material. As a reference to the amendment properties, we decided to also adjust the turnover rate of RPM to BIOM, kRPM, HCA specifically. The underlying assumption is that the higher the proportion of hard-to-decompose molecules such as lignin and phenols, the longer it takes for microbes to mineralize C (and thus N), which is reflected in a smaller k-value. Optimization of kRPM resulted in 0.0598 for WOSR residues, 0.0326 for FB residues, 0.0058 for WW straw, and just 0.0014 for sawdust. Thus, C:NRPM and kRPM refer to the material’s biochemical composition and therefore mainly determine HCA-specific mineralization/immobilization dynamics.
Model performance was examined with methods described by Smith et al. (1996), i.e., inspection of root mean square error (RMSE), maximum error (ME), and modeling efficiency (EF).
The cumulative sum of daily fabiot of a specific number of simulated days can be interpreted as homogenous days of optimal mineralization of that particular period. From the residue C decomposition per mineralization days from amendment incorporation until 1 March, the decay rate k was derived from the half-life equation (Equation 3).
with the remaining amendment C (sum of RPM and DPM) as a decimal part of the introduced organic C and t in days.
With the known decay rate per amendment, half-life t0.5 was calculated. The 25-year weather data of the experimental site were used to calculate the average number of days of optimal mineralization per year. Subsequently, half-lives were converted to site-specific calendar years by division with this value. It should be noted that after the half-life of an amendment, still half of the Ipot is remaining, and decomposition will continue at a decreasing rate, thus illustrating the temporal extent of the effects.
2.5 Statistical analysis
All data operations, visualizations, and statistical procedures were realized with R, RStudio, and tools from the tidyverse-package (Wickham et al., 2019; R Core Team, 2020; RStudio Team, 2020). Cumming plots were produced with the dabestr package (Ho et al., 2019). The sampling distribution and the confidence interval (CI) of the effect size plot were calculated by bootstrapping (n = 5000, Efron and Tibshirani, 1994). The CI is bias-corrected and accelerated (Efron, 1987).
In general, for the study data, linear-mixed effect models proved to have the best explanatory power in regression analysis (Pinheiro et al., 2019). Subsequently, multiple comparisons of means from the multcomp package were used to determine significant differences between groups (Hothorn et al., 2008). A generic code example of the model formulation can be found in the Supplementary Material. Results are presented as arithmetic mean values ±1 standard deviation or else as indicated in the text. Statistical significance was stated with p< 0.05.
3 Results
A comprehensive ANOVA report table for the main effects and interactions of the regression analysis of each variable is available in the Supplementary Material (Table A.2).
3.1 Evaluation of soil water and soil mineral N dynamics simulation
The process model predicted SWC and SMN for different soil layers on a single plot level. The soil textures were uniform for each replication, but the initial values were set plot-specific. The simulation quality is assessed by comparing the measured values with the modeled equivalents using major axis regression analysis. All observed relationships were found to be significant (p< 0.001), with slopes ranging from 0.68 to 0.97 (Table 2). The R2 values ranged from 0.38 to 0.57, indicating that the model explains one-third to half of the variation in the modeled variables based on the measured values. Correlation coefficients (r) for the six parameters ranged from 0.62 to 0.78, indicating a strong positive correlation between the predicted and measured values. The RMSE for SWC was approximately equal to the standard deviation (SD) of the data, while for SMN, it was even lower. The maximum error (ME) was within an acceptable range considering the variability of the field measurements due to heterogeneous conditions. It should be noted that the prediction quality for SWC decreased with depth, whereas it was more stable for SMN. Please refer to Supplementary Figure 3 for a graphical illustration of the model accuracy (1:1 plots).
3.2 Soil water dynamics and temperature sums
In dependency on the year and the preceding crop, simulations started with different initial SWCs. However, regular rainfall in the post-harvest period quickly led to SWC in 0–30 cm leveling off around saturation every year (Figure 2). Hence, the soil profiles were filled and started to drain water from 120-cm depth immediately. This dynamic lasted in all experimental years until mid-April. In 2015/2016 and 2017/2018, almost 400 mm was drained from harvest till March, whereas in 2016/2017, only approximately 150 mm left the defined drainage depth. When soil water is not a limiting factor, the rate of evaporation is primarily determined by the temperature of the topsoil. Therefore, it is not surprising that the values for evaporation were similar across all years, as the difference in the temperature sum during the simulation was not significant for either the crop rotations or the individual years.
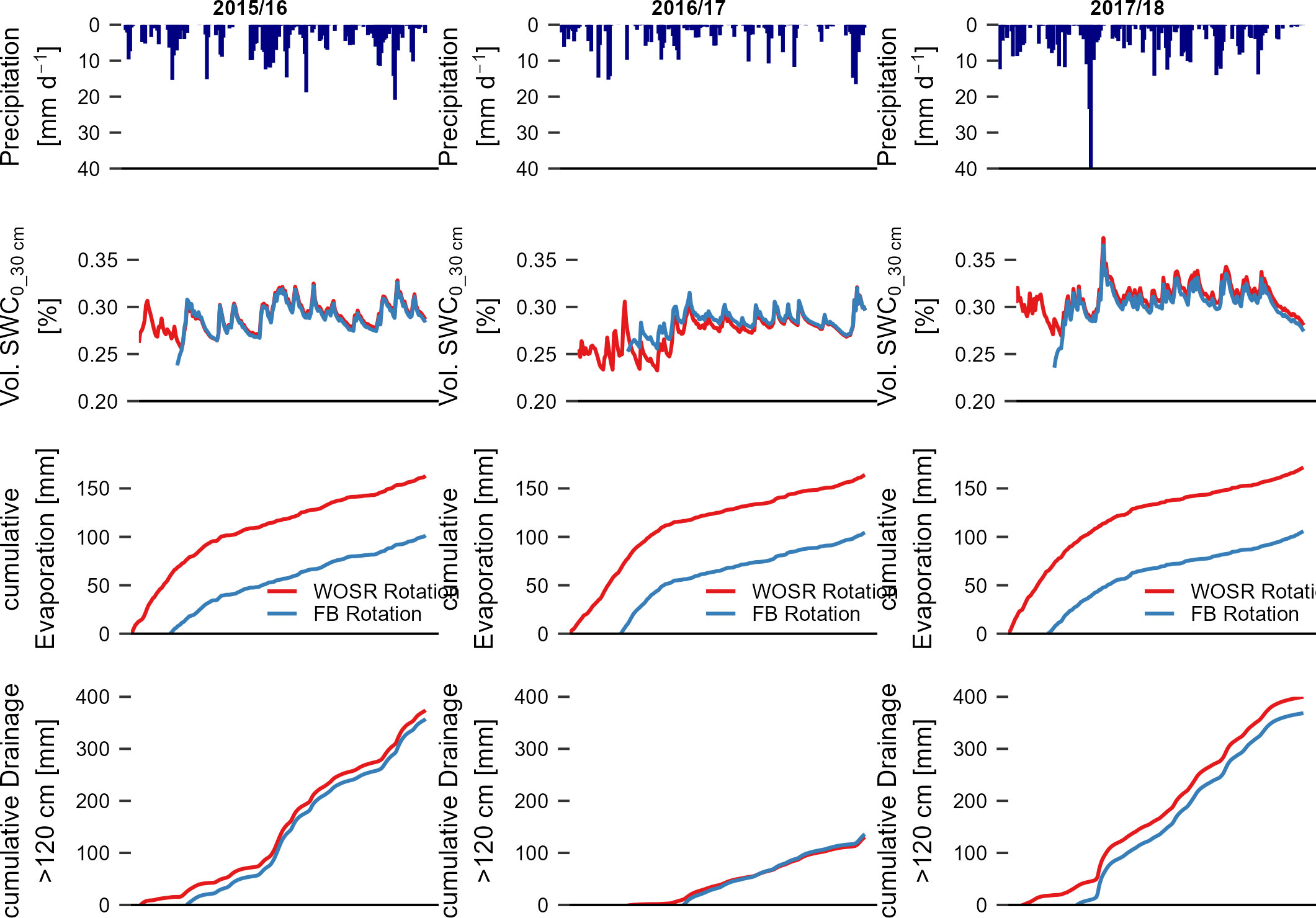
Figure 2 Measured daily precipitation, modeled topsoil water content (SWC), evaporation, and drainage water on average per crop rotation and experimental period.
The period between the simulation start of WOSR and FB rotation ranged from 28 to 39 days. For the WOSR rotation, the simulation period experienced an average of 73 mm more precipitation than the FB rotation (p< 0.001). The mineralization time, expressed as the cumulative sum of fabiot per season (amendment incorporation until 1 March), was on average 43.2 ± 2.8 days for the WOSR rotation and after FB 33.5 ± 2 days. Consequently, depending on the crop rotation, the amendments were exposed to active decomposition for periods of different lengths, and this difference exerts the greatest impact on the parameters shown in Figure 2.
3.3 Carbon and nitrogen mineralization dynamics
The initial SMN content in 0–90 cm (SMN0–90) after harvest of WOSR and FB was on average 74 ± 17 kg N ha−1 (Figure 3), whereas the preceding crops did not imply a significant difference. However, there was an effect of the year, mostly due to the climatic conditions of the vegetational period: in 2016, the average residual N after harvest was 14 kg N ha−1 higher than in 2015 (p< 0.01). Each year, almost 50% of the SMN could be found in topsoil (0–30 cm). Faba beans accumulated in topsoil on average 4 kg N ha−1 more than WOSR (p< 0.05).
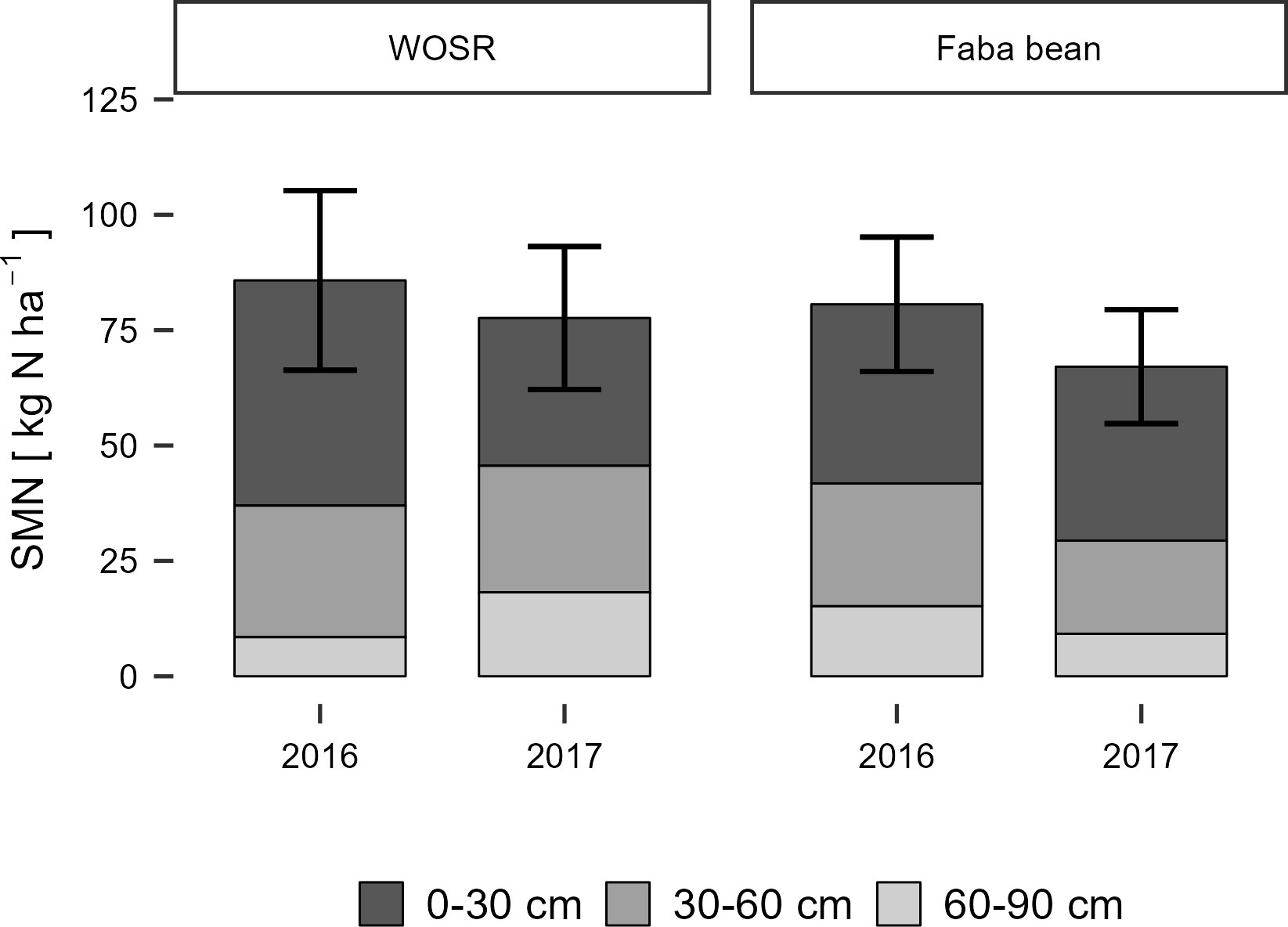
Figure 3 Soil mineral N (SMN) in three layers of the 0–90-cm soil profile after harvest of winter oilseed rape (WOSR) and faba beans (FB) in advance of treatment application; error bars indicate standard deviation of SMN0–90; differences are rooted in soil heterogeneity among the replications.
The mineralization dynamics of all organic C pools (and corresponding N) were mainly driven by soil cultivation. Therefore, modeled N mineralization rates peaked right after plowing (SOM, 1.5 ± 0.6 kg N ha−1 day−1; BIOM, 0.4 ± 0.2 kg N ha−1 day−1; DPM, 2.4 ± 0.4 kg N ha−1 day−1; RPM: −3 ± 1.3 kg N ha−1 day−1) due to the short-term oxygenation. Negative net mineralization of the RPM indicated N immobilization by SMN consumption. Amendment C (DPM+RPM) over mineralization time illustrates the first-order kinetics used for the model and the different durations of active decomposition in dependency on the preceding crop (Figure 4). The rapid decline in decomposition activity after the peak was induced by falling temperatures and the fading tillage effect.
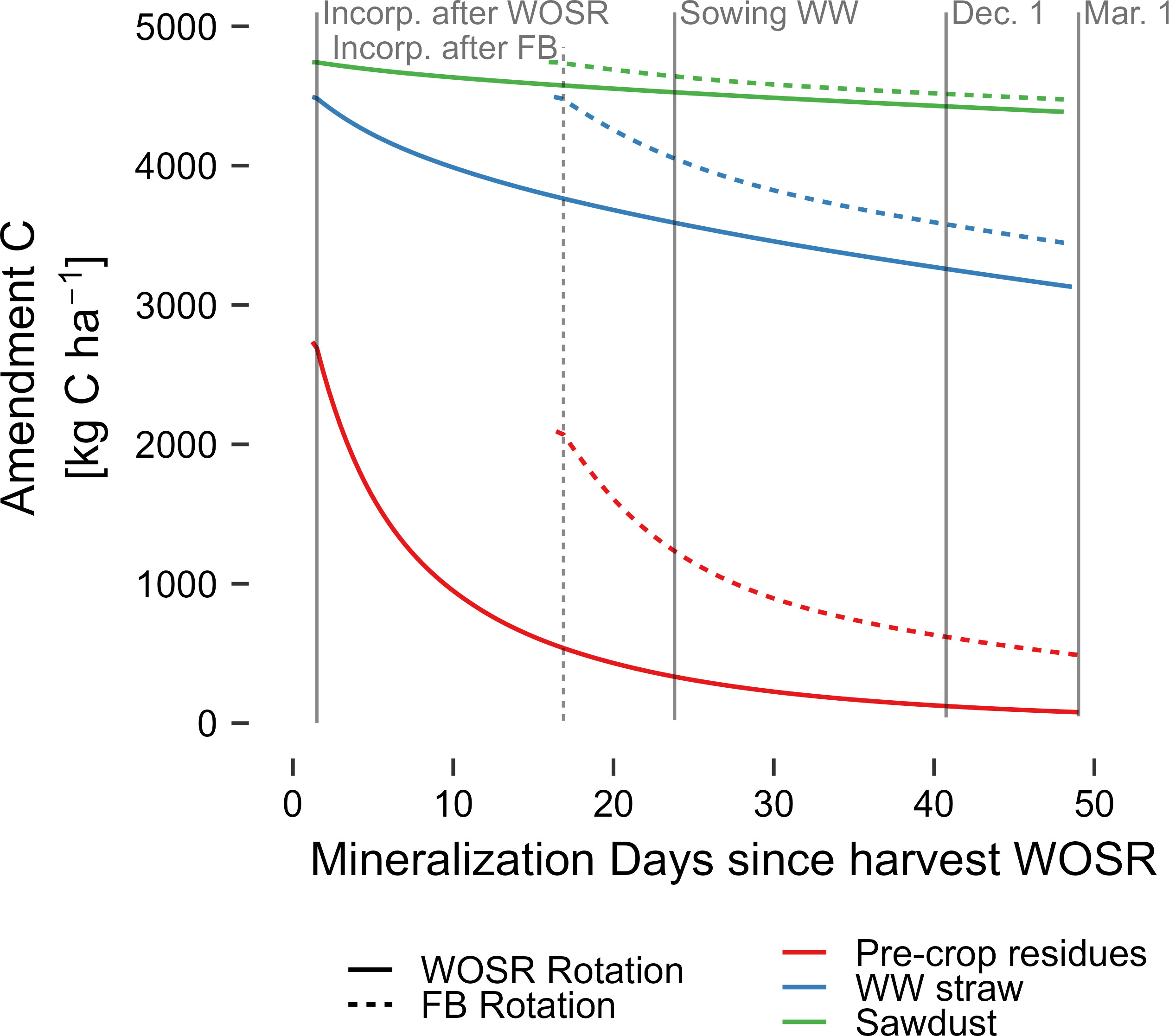
Figure 4 Modeled amendment C decomposition 2016/2017 per day of optimal mineralization conditions (cumulative fabiot from harvest winter oilseed rape (WOSR)); vertical lines indicate corresponding calendar dates.
Also, measured SMN contents of the topsoil layer (0–30 cm) increased to a peak of an average of 39.8 ± 11.4 kg N ha−1 shortly after amendment incorporation by plowing (Figure 5). These peaks were on plots with HCA less pronounced compared to those without residues (on average −5.7 kg N ha−1, n.s.). WW straw had the strongest effect: after WOSR, the maximum SMN content was an average of almost 14 kg N ha−1 lower than without residues (p< 0.05). A subtle temporal delayed occurrence of the SMN peaks in deeper soil layers indicated the translocation of th percolation.
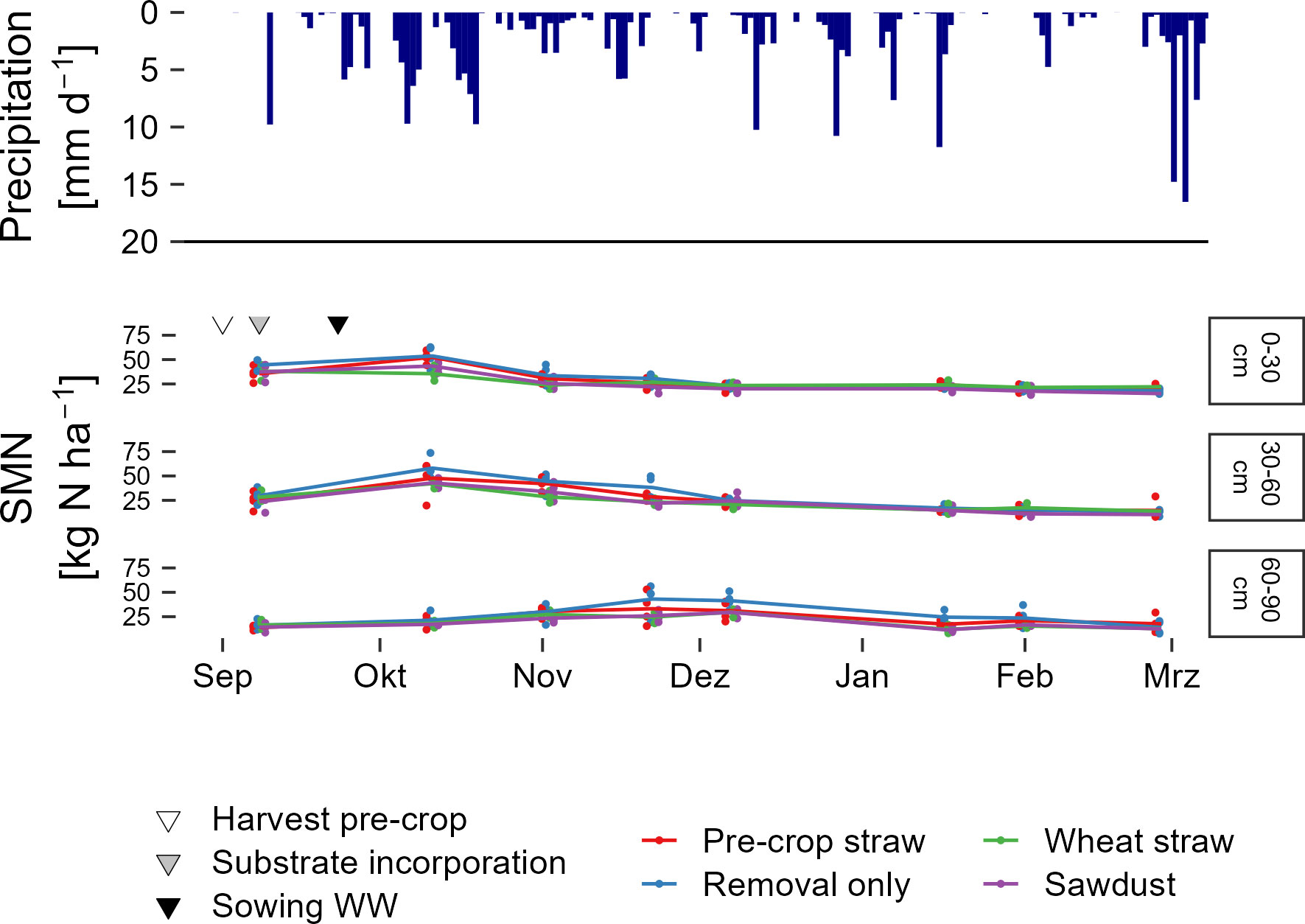
Figure 5 Example soil mineral N (SMN) dynamics from 2016/2017 after faba beans (FB) for the three soil layers 0–30-, 30–60-, and 60–90-cm depth; points indicate measured values; lines indicate linear interpolated means.
Looking at the mineralization balance at the end of the percolation period in March (Figure 6A), net N mineralization from organic inputs differed in dependency on the preceding crop and the amendment. The difference in total net N mineralization with and without HCA application can be considered as relative net immobilization (Figure 6B). After WOSR, relative net N immobilization by organic input was an average of 2 kg N ha−1 higher than after FB (n.s., p = 0.09). Separated per rotation, the WW straw application immobilized almost 8 kg N ha−1 after WOSR and more than 9 kg N ha−1 after FB compared to the corresponding preceding crop residues (p< 0.001). In the WOSR rotation, the difference of relative net N mineralization with WW straw to sawdust was still 5 kg N ha−1. Compared to FB residues, sawdust reduced net mineralization by a further 5 kg N ha−1 (p< 0.05).
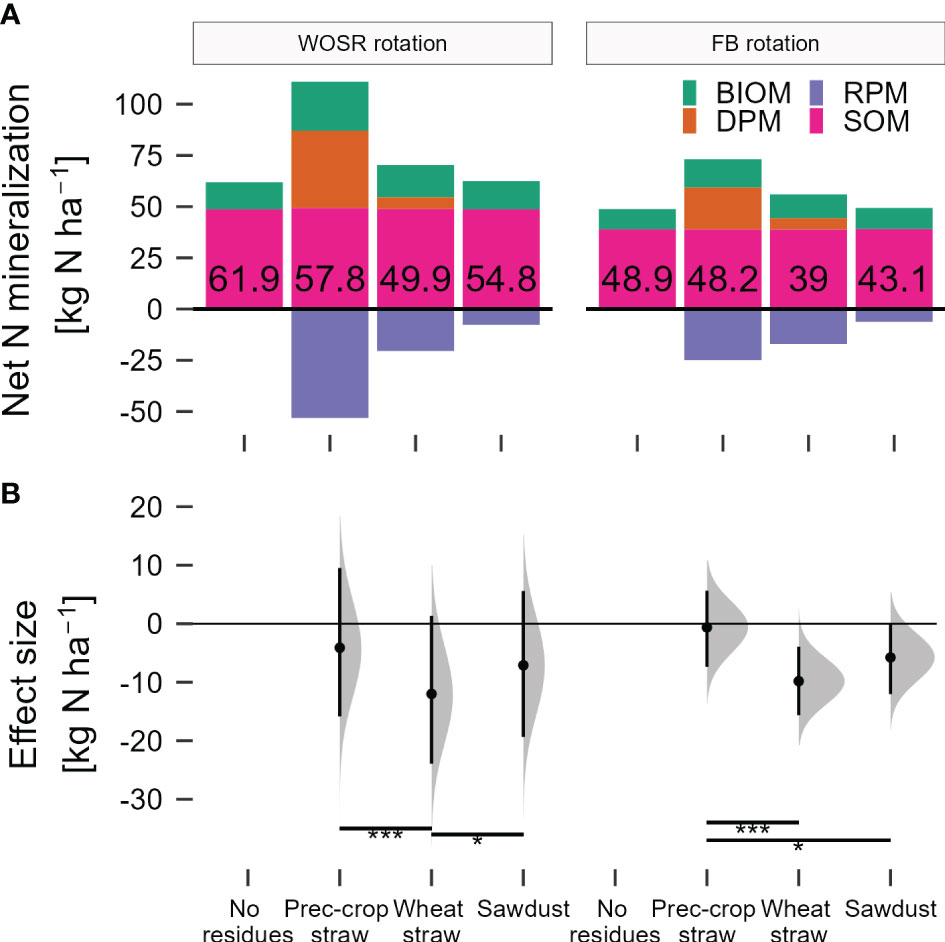
Figure 6 (A) Average cumulative modeled net N mineralization per organic compartment at March 1st; negative values represent net immobilization of soil mineral N (SMN); numbers in the bars indicate the total net balance ±1 sd; error bars were omitted in favor of better readability. (B) Effect size expressed as mean difference of total net N mineralization from amendment addition to the shared control of residue removal plotted as bootstrap sampling distributions. Each 95% confidence interval is indicated by the ends of the vertical error bars. Asterisk marked lines indicate significant differences between the treatments (‘*’ p < 0.05, ‘***’ p < 0.001).
SMN at the end of the percolation period was on average 39.3 ± 13.5 kg N ha−1 and shows only small variation in regard to the different seasons. Also, rotations and treatments differ only slightly. After WOSR with HCAs, there was on average slightly less mineral N in the 60–90-cm layer, but not significantly.
The simulated remaining amendment C at the beginning of March (Supplementary Figure 4) can be considered as an indicator of an untapped Ipot. With WOSR and FB residues 4.4% ± 1%, 23.2% ± 1% of the introduced C remained in the topsoil layer. With WW straw and sawdust, even 76% ± 3% and 94% ± 1% respectively remained. Based on these remains, site-specific half-lives were calculated as described above. An average year at the experimental farm equals 75 ± 6 mineralization days. Thus, 50% of the introduced crop residues of WOSR and FB would be decomposed after 0.15 ± 0.02 years (=11.25 mineralization days) and 0.23 ± 0.01 years (=17 mineralization days), respectively. WW straw average exceeds this by eight times (1.42 ± 0.2 years) and sawdust by 34 times (6.19 ± 0.66 years).
3.4 N leaching
On average, of all treatments and years, 63 ± 17 kg N leached in the period from preceding-crop harvest till March. The average difference between the crop rotations of 6 kg N ha−1 was not significant (p = 0.08). As a direct consequence of the total amount of rainfall and thus percolation water, leached N differs in dependency of the year (Figure 7): high N losses of 74.2 ± 13.8 kg N ha−1 in 2017/2018 can be attributed to high precipitation during fall and winter (429 mm in October to February). Slightly lower losses (67.6 ± 11.7 kg N ha−1) were observed in 2015/2016 (441 mm). Still, losses in both years were significantly higher than in 2016/2017 (p< 0.001), which was characterized by an extraordinary dry fall/winter at the site: only 46.3 ± 11.6 kg N ha−1 was lost with 250-mm precipitation. However, the average rate was quite constant at 0.14 ± 0.03 kg N ha−1 mm−1.
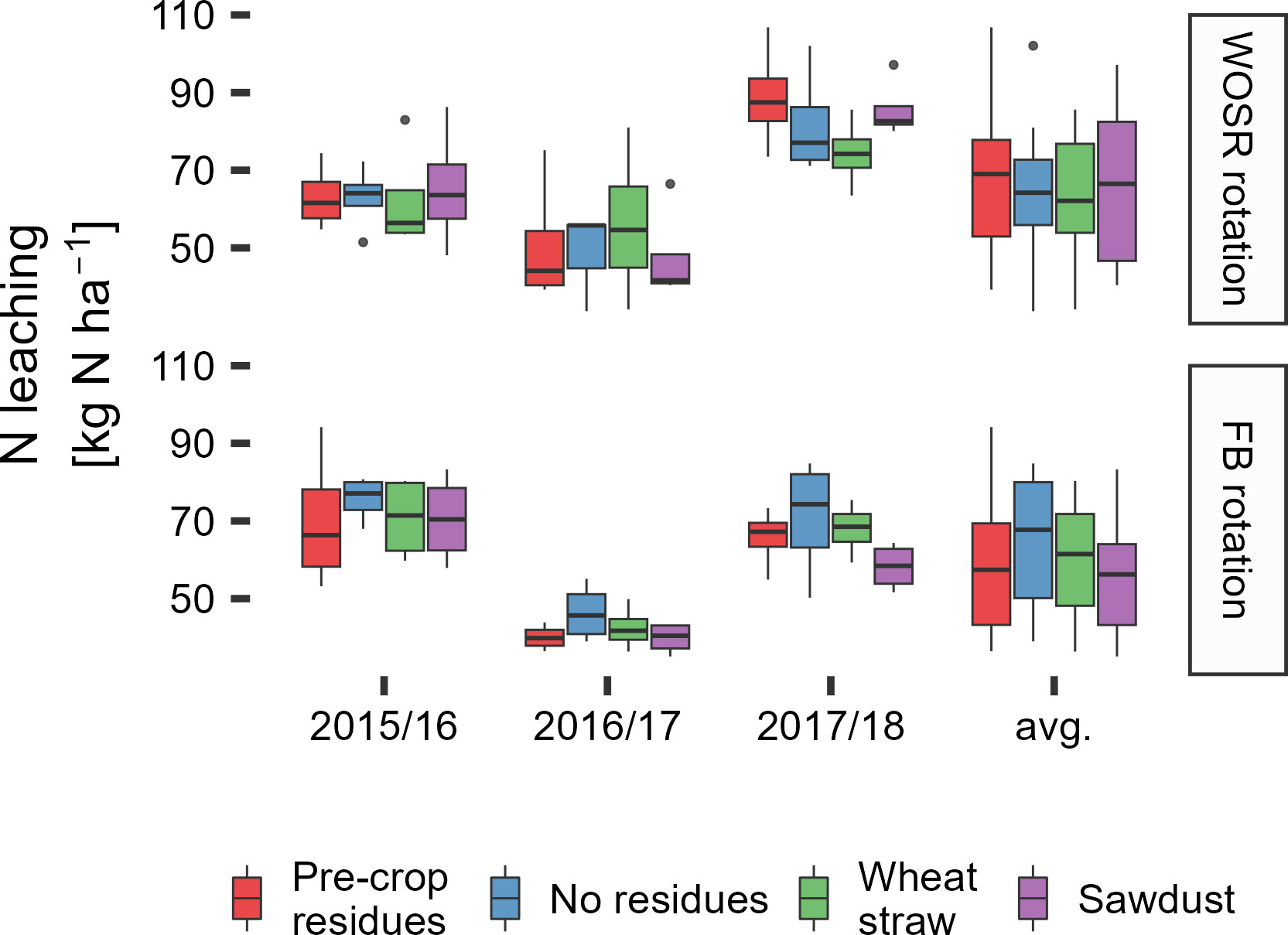
Figure 7 Model derived N leaching losses per amendment treatment and year as well as on average of all experimental years (n = 4 per year and treatment).
Considering the crop rotations and amendments separately, averaged N losses differed between 0.5 and 12 kg N ha−1 (Figure 7, Supplementary Table A.4). Losses after FB without amendment were significantly higher (6 to 12 kg N ha−1, adj. p< 0.05) than in all other treatments. Contrary to the prior assumption, N leaching losses did not correlate clearly with the C:N ratio of the amendments.
It became obvious that N leaching occurred only while crop N uptake was low and precipitation exceeded evapotranspiration (Figures 8A, B). As SMN in topsoil was reduced by WW straw incorporation, also total leaching at the end of the percolation period was decreased. The modeling results made it also evident that N from fertilizer applied to WW was not translocated below 60 cm since it was taken up by the wheat roots (Figures 8C, D). Therefore, this period was not of interest for further examination of the proposed approach.
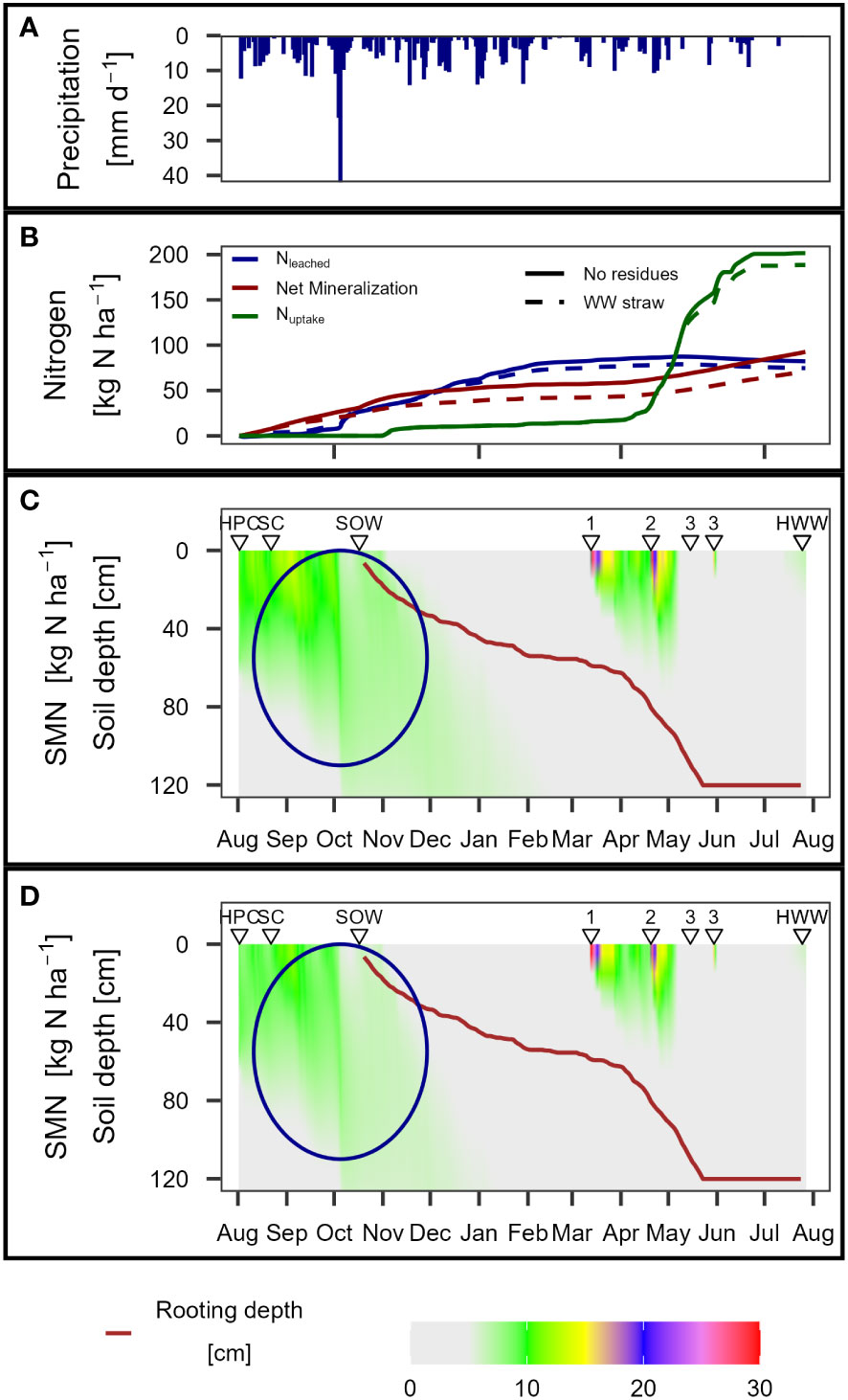
Figure 8 Precipitation (A) and mean modeled values for (leached) soil mineral N (SMN), net mineralization, crop N uptake (B–D), and rooting depth of wheat (C, D) in 2017/2018 after winter oilseed rape (WOSR). (C) Without residues. (D) With winter wheat (WW) straw applied after WOSR harvest. HPC, harvest of pre-crop, SC, soil cultivation; SOW, sowing of WW; 1 to 3, fertilizer applications; HWW, harvest of WW.
4 Discussion
In humid climates, residual N as SMN or crop residues and SMN mineralized after harvest from SOM are often lost from the cropping system by the process of leaching. The amount of leached N depends on rainfall (percolation water), mineral N concentration, and crop N uptake. Latter is generally low in winter wheat until March (up to 20 kg N ha−1). Residual N in soil is related to the crop yield (N uptake) and climatic conditions and therefore shows high inter-annual variability. Additionally, spatial heterogeneity of soil conditions causes a high SMN variance across the site each year. Still, in all 3 years, we found SMN concentrations in 0–90 cm after WOSR in the same range as found by Sieling et al. (1999) at the same site. Aufhammer et al. (1992) reported comparable N contents in Southern Germany after FB. The situation of post-harvest SMN values may therefore be seen as typical for both crops.
Common practice encounters this loss pathway inter alia by retaining the preceding crop residues in order to immobilize excess N. Our study strives to analyze the effects of the N immobilization potential of alternative amendments in order to minimize nitrate leaching losses. Specifically, we tested in field scale the effects and practical relevance of HCAs on N immobilization/mineralization processes and potential benefits for N use efficiency by reduced leaching and improved N transfer within the cropping system. Thereby, we combined experimental data from a 3-year field experiment and analyzed it with a simulation modeling approach.
4.1 Model performance
Overall, the major axis regression analysis conducted for measured and modeled SWC and SMN values suggests that the process model provides a reasonable fit to the observed data, with a strong positive correlation and relatively low error rates. Although there was little variation in SWC during the period of interest, the fall SWC dynamics were simulated comparatively well. In fact, the model performance in this study is even slightly better than in a study using the same modeling environment (Räbiger et al., 2020). The model also estimated correctly the magnitude and timing of SMN dynamics within the top 90 cm of soil. Thus, maximum reliability was achieved for model-derived parameters, i.e., N leaching and mineralization. However, model-derived data remain an estimation, and further research may be needed to refine the model and improve its accuracy in predicting SWC at greater depths.
4.2 N leaching
The effect of the amendments on N leaching falls short of expectations. In contrast, Mitchell et al. (2001) found a reduction by cereal straw of up to 19 kg N ha−1. Although mean differences of leached N are in the same range of the effect size on total net mineralization, statistically, it was not possible to prove the same significant contrasts. Moreover, dilution of the effect in the interaction of immobilization/mineralization, N uptake and leaching, precipitation was probably a major cause for the reduced impact of the HCA on N leaching. In the rather wet years, the HCA-induced reduction of leached N was pronounced. This circumstance points out that it needs a certain amount of percolating water to translocate from the affected horizon in depths > 120 cm. If this threshold is not reached, the N potentially affected by amendment-induced immobilization does not even contribute to N leaching in the variant without residues; i.e., the amendment effect persists at depths< 120 cm.
4.3 C and N dynamics
The amendment-induced microbial immobilization was observable in buffered SMN increases in the field data as well as in reduced total net mineralization in the modeling data. This finding corresponds well with other studies (Justes et al., 1999; Congreves et al., 2013; Chen et al., 2014; Chaves et al., 2021).
Despite the immobilization potentials of all amendments exceeding the post-harvest SMN, the temporal dynamics of the effect did not meet the desired time frame; neither could the alternatives to the preceding crop residues fully realize their immobilization potential when N availability was high. On the contrary, initially, SMN increases in fall in all treatments. Nor do the derived half-lives of WW straw and sawdust indicate a prompt re-mineralization at times of increased N demand by the crop.
Modeled dynamics of the organic C pools confirmed the following: positive net mineralization from all other sources exceeded the N immobilized by RPM decomposition. High temperatures, sufficient soil moisture, and aeration by soil cultivation are the drivers of general excellent mineralization conditions in fall (Engström and Lindén, 2012). Nevertheless, the application of wheat straw and sawdust induced N limitation (<<30 mg N per kg C; Recous et al., 1995; Mary et al., 1996), resulting in decelerated mineralization rates (Chaves et al., 2021). This can be considered as relative net N retention compared to the control and especially compared to the withdrawal of organic C inputs.
The observed general decline in mineralization activity and SMN content after the peak in late October is typical, as low soil temperatures, which slow down the mineralization and nitrification processes, are characteristic of Central European winter (Garnier et al., 2003). Additionally, at this time, the easily decomposable compartments (simple carbohydrates) were largely decomposed. Trinsoutrot et al. (2000) described similar patterns after the incorporation of WOSR residues. The stable parts (lignified material), however, were degraded much slower, which led to the high residual amounts of RPM-C in March after WW straw and sawdust application. This points out the long-term residence of incorporated organic matter as stated by Mitchell et al. (2001) and may lead to C-sequestration (Stockmann et al., 2013).
4.4 Ipot and HCA half-lives
For the average of all three trial years, the amendments’ effect on mineralization and hence N leaching does not immediately correlate with the C:N ratio of the tested HCAs. Although the C:N ratios of the two pre-crop residues are above the proclaimed limit for immobilization (C:N = 25, Verberne et al., 1990), their incorporation caused mixed effects. This may be due to the fact that their biochemical composition is closer to the limit of mineralization/immobilization equilibrium than expected. This would explain, depending on the year, i.e., on the absolute amount of residues and their exact C:N ratio, why the autumn mineralization is sometimes higher and sometimes lower than in the variant without an amendment. Also, in regard to the whole choice of amendments, the short-term impact did not simply scale up with the C:N ratio as proposed by older literature (e.g., Mueller et al., 1998). Rather, recent studies suggest further amendment properties affecting N dynamics after incorporation. Chen et al. (2014) recommended, in addition to the C:N ratio, considering the explicit biochemical compounds of C and N (e.g., lignin) to estimate their effect on the mineralization–immobilization progress. Lashermes et al. (2021) pointed out that the initial soluble fraction of biomass is linked to early C mineralization.
In contrast to Ipot, half-lives derived from the model results give a good impression of the temporal dimension of the HCA effect on mineralization dynamics. For WW straw and sawdust, Ipot was not nearly realized at the end of the drainage period, which is confirmed by the determined half-lives, thus indicating a long-term effect and even organic C accumulation. As far as the authors know, an estimation like this was never given before in the context of biomass degradation in soils. Consequently, in dependency on the sites’ climatic and soil conditions, the effects of resistant amendments tend to be less pronounced but subtle and longer lasting. Regarding long-term effects, Paustian et al. (1992) observed SOM accumulation of up to 15% over 30 years by comparable biomass inputs of cereal straw and sawdust, though this relates to a colder and drier climate.
However, the disadvantages of the method presented here are that the half-lives were derived ex post from modeling data and that they are also highly site- and amendment-specific. By exploring the underlying model mechanics and by adjoining further amendment specifications, it might be possible to develop a general half-life estimation.
4.5 Conclusion
In conclusion, the tested amendments alone did not meet the short- to medium-term aims under the given soil and climatic conditions. The observed mean differences even in comparison to the treatment without amendment can be considered as practically irrelevant and do not justify any additional effort (time, money, and manpower) from farmers. Hence, further research should aim at increasing the extent of immobilization and/or accelerating re-mineralization. Unfortunately, as far as the authors know, the choice of materials in terms of their biochemical suitability is scarce while also considering local availability and cost efficiency. More promising is the optimization of the method in regard to the application date, particle size, and placement (Jensen, 1994; Chen et al., 2014; Toenshoff et al., 2014; Chaves et al., 2021). Also, the long-term effects of the tested options still need to be investigated. C-sequestration, soil structure improvements, and the adaption of the microorganism spectrum might contribute to better soil health and climate-smart agriculture.
The strong dependency of the amendment effect on site-specific properties such as soil and climate conditions makes it hard to transfer the recent findings to differing sites. Therefore, it is necessary to either obtain field data from comparable experiments at various sites or to evaluate modeled extrapolation of climate scenarios under varying soil conditions. The only general recommendation on residue management that can be made to date is to maintain the practice of retaining the preceding crop’s straw. Nevertheless, since we could basically confirm the immobilization effect, the approach of the HCA application remains relevant and could be part of more complex site-specific management to mitigate post-harvest N losses.
Data availability statement
The original contributions presented in the study are included in the article/Supplementary Material, further inquiries can be directed to the corresponding author.
Author contributions
HK conceived the presented approach and the experimental setup and supervised the study. SR carried out the fieldwork and organized sampling and laboratory analyses. SR performed the model runs and the analytical calculations. SR wrote the manuscript and produced all graphs, supported by the feedback of HK. All authors contributed to the article and approved the submitted version.
Funding
This work was supported by the German Federal Ministry of Education and Research (BMBF) (grant number 031B1062E). We also acknowledge the financial support for publication fees provided by the German Research Foundation (DFG) as part of its Open Access Publishing program.
Acknowledgments
We would like to thank our technical assistant Anja Wolff and the numerous research assistants for their help with the fieldwork. Thanks also to the R developer and user community for providing wonderful software and solutions for nearly all issues related to data management, analysis, and graph production.
Conflict of interest
The authors declare that the research was conducted in the absence of any commercial or financial relationships that could be construed as a potential conflict of interest.
Publisher’s note
All claims expressed in this article are solely those of the authors and do not necessarily represent those of their affiliated organizations, or those of the publisher, the editors and the reviewers. Any product that may be evaluated in this article, or claim that may be made by its manufacturer, is not guaranteed or endorsed by the publisher.
Supplementary material
The Supplementary Material for this article can be found online at: https://www.frontiersin.org/articles/10.3389/fagro.2023.1155187/full#supplementary-material
References
Aufhammer W., Stützel H., Kübler E., Fiegenbaum A. (1992). Interplanting of non-legumes into faba bean (Vicia faba L.) to reduce the risk of nitrate contamination of the ground water. Euro. J. Agro. 1 (2), 59–69. doi: 10.1016/S1161-0301(14)80002-6
Beaudoin N., Saad J. K., Van Laethem C., Machet J. M., Maucorps J., Mary B. (2005). Nitrate leaching in intensive agriculture in northern France: effect of farming practices, soils and crop rotations. Agriculture Ecosyst. Environ. 111, 292–310. doi: 10.1016/j.agee.2005.06.006
Bouwman A. F. (1996). Direct emission of nitrous oxide from agricultural soils. Nutr. Cycl Agroecosyst 46, 53–70. doi: 10.1007/BF00210224
Chaves B., Redin M., Giacomini S. J., Schmatz R., Léonard J., Ferchaud F., et al. (2021). The combination of residue quality, residue placement and soil mineral n content drives c and n dynamics by modifying n availability to microbial decomposers. Soil Biol. Biochem. 163, 108434. doi: 10.1016/j.soilbio.2021.108434
Chen B., Liu E., Tian Q., Yan C., Zhang Y. (2014). Soil nitrogen dynamics and crop residues. A review. Agron. Sustain. Dev. 34, 429–442. doi: 10.1007/s13593-014-0207-8
Congreves K., Vyn R., Van Eerd L. (2013). Evaluation of post-harvest organic carbon amendments as a strategy to minimize nitrogen losses in Cole crop production. Agronomy 3, 181–199. doi: 10.3390/agronomy3010181
Di H. J., Cameron K. C. (2002). Nitrate leaching in temperate agroecosystems: sources, factors and mitigating strategies. Nutrient Cycling Agroecosystems 64, 237–256. doi: 10.1023/A:1021471531188
Efron B. (1987). Better bootstrap confidence intervals. J. Am. Stat. Assoc. 82, 171–185. doi: 10.2307/2289144
Efron B., Tibshirani R. J. (1994). An introduction to the bootstrap (Boca Raton, Florida, USA: CRC Press).
Engström L., Lindén B. (2012). Temporal course of net n mineralization and immobilization in topsoil following incorporation of crop residues of winter oilseed rape, peas and oats in a northern climate. Soil Use Manage. 28, 436–447. doi: 10.1111/sum.12004
Feddes R. A., Zaradny H. (1978). Model for simulating soil-water content considering evapotranspiration {{/amp]]mdash; comments. J. Hydrology 37, 393–397. doi: 10.1016/0022-1694(78)90030-6
Garnier P., Néel C., Aita C., Recous S., Lafolie F., Mary B. (2003). Modelling carbon and nitrogen dynamics in a bare soil with and without straw incorporation. Eur. J. Soil Sci. 54, 555–568. doi: 10.1046/j.1365-2389.2003.00499.x
Goss M. J., Howse K. R., Lane P. W., Christian D. G., Harris G. L. (1993). Losses of nitrate-nitrogen in water draining from under autumn-sown crops established by direct drilling or mouldboard ploughing. J. Soil Sci. 44, 35–48. doi: 10.1111/j.1365-2389.1993.tb00432.x
Hansen S., Jensen H. E., Nielsen N. E., Svendsen H. (1990). NPo-research, A10: DAISY: soil plant atmosphere system model (Copenhagen, Denmark: Miljoestyrelsen).
Henke J., Böttcher U., Neukam D., Sieling K., Kage H. (2008). Evaluation of different agronomic strategies to reduce nitrate leaching after winter oilseed rape (Brassica napus l.) using a simulation model. Nutrient Cycling Agroecosystems 82, 299–314. doi: 10.1007/s10705-008-9192-0
Ho J., Tumkaya T., Aryal S., Choi H., Claridge-Chang A. (2019). Moving beyond p values: data analysis with estimation graphics. Nat. Methods 16, 565–566. doi: 10.1038/s41592-019-0470-3
Hoffmann C., Platte H., Lickfett T., Koch H.-J. (1997). Microbial biomass and n mineralization in relation to n supply of sugar beet under reduced tillage. Z. für Pflanzenernährung und Bodenkunde 160, 187–193. doi: 10.1002/jpln.19971600211
Hothorn T., Bretz F., Westfall P. (2008). Simultaneous inference in general parametric models. Biometrical J. 50, 346–363. doi: 10.1002/bimj.200810425
IPCC (2006). IPCC guidelines for national greenhouse gas inventories prepared by the national greenhouse gas inventories programme (Japan: Institute for Global Environmental Strategies (IGES).
Jensen E. S. (1994). Mineralization-immobilization of nitrogen in soil amended with low C:N ratio plant residues with different particle sizes. Soil Biol. Biochem. 26, 519–521. doi: 10.1016/0038-0717(94)90185-6
Justes E., Mary B., Nicolardot B. (1999). Comparing the effectiveness of radish cover crop, oilseed rape volunteers and oilseed rape residues incorporation for reducing nitrate leaching. Nutrient Cycling Agroecosystems 55, 207–220. doi: 10.1023/A:1009870401779
Kage H., Alt C., Stützel H. (2003). Aspects of nitrogen use efficiency of cauliflower i. a simulation modelling based analysis of nitrogen availability under field conditions. J. Agric. Sci. 141, 1–16. doi: 10.1017/S0021859603003344
Kage H., Stützel H. (1999). HUME: an object-oriented component library for generic modular modelling of dynamic systems. (Lleida, Spain: European Society of Agronomy) Model. Cropping Syst. 299–300.
Lashermes G., Recous S., Alavoine G., Janz B., Butterbach-Bahl K., et al. (2021). N2O emissions from decomposing crop residues are strongly linked to their initial soluble fraction and early C mineralization. Sci. Total Environ. 150883. doi: 10.1016/j.scitotenv.2021.150883
Lönnqvist T., Silveira S., Sanches-Pereira A. (2013). Swedish Resource potential from residues and energy crops to enhance biogas generation. Renewable Sustain. Energy Rev. 21, 298–314. doi: 10.1016/j.rser.2012.12.024
Mary B., Recous S., Darwis D., Robin D. (1996). Interactions between decomposition of plant residues and nitrogen cycling in soil. Plant Soil 181, 71–82. doi: 10.1007/BF00011294
Masunga R. H., Uzokwe V. N., Mlay P. D., Odeh I., Singh A., Buchan D., et al. (2016). Nitrogen mineralization dynamics of different valuable organic amendments commonly used in agriculture. Appl. Soil Ecol. 101, 185–193. doi: 10.1016/j.apsoil.2016.01.006
Mitchell R., Webb J., Harrison R. (2001). Crop residues can affect n leaching over at least two winters. Eur. J. Agron. 15, 17–29. doi: 10.1016/S1161-0301(00)00088-5
Moss A. (2002). The effects of long-term feeding of extracted rapeseed meal and whole rapeseed on the physical and financial performance, health and welfare of high yielding dairy cows (No. OS59) (Reading, GB: ADAS).
Mueller T., Jensen L. S., Nielsen N. E., Magid J. (1998). Turnover of carbon and nitrogen in a sandy loam soil following incorporation of chopped maize plants, barley straw and blue grass in the field. Soil Biol. Biochem. 30, 561–571. doi: 10.1016/S0038-0717(97)00178-8
Mulvaney R. L., Otto R., Griesheim K. L., Su K., Trivelin P. C. O. (2016). Leaching methods can underestimate mineralization potential of soils. Commun. Soil Sci. Plant Anal. 47, 1701–1708. doi: 10.1080/00103624.2016.1206561
Nemitz E., Sutton M., Gut A., García R., Husted S., Schjoerring J. (2000). Sources and sinks of ammonia within an oilseed rape canopy. Agric. For. Meteorology 105, 385–404. doi: 10.1016/S0168-1923(00)00205-7
Nicholson F. A., Chambers B. J., Mills A. R., Strachan P. J. (1997). Effects of repeated straw incorporation on crop fertilizer nitrogen requirements, soil mineral nitrogen and nitrate leaching losses. Soil Use Manage. 13, 136–142. doi: 10.1111/j.1475-2743.1997.tb00574.x
Paustian K., Parton W. J., Persson J. (1992). Modeling soil organic matter in organic-amended and nitrogen-fertilized long-term plots. Soil Sci. Soc. America J. 56, 476. doi: 10.2136/sssaj1992.03615995005600020023x
Pinheiro J., Bates D., DebRoy S., Sarkar D. (2019). Nlme: linear and nonlinear mixed effects models. R Package version 3, 1–131.
Räbiger T., Andres M., Hegewald H., Kesenheimer K., Köbke S., Quinones T. S., et al. (2020). Indirect nitrous oxide emissions from oilseed rape cropping systems by NH3 volatilization and nitrate leaching as affected by nitrogen source, n rate and site conditions. Eur. J. Agron. 116, 126039. doi: 10.1016/j.eja.2020.126039
R Core Team (2020). R: a language and environment for statistical computing (Vienna, Austria: R Foundation for Statistical Computing).
Recous S., Robin D., Darwis D., Mary B. (1995). Soil inorganic n availability: effect on maize decomposition. Soil Biol. Biochem. 27, 1529–1538. doi: 10.1016/0038-0717(95)00096-W
Reichel R., Wei J., Islam M. S., Schmid C., Wissel H., Schröder P., et al. (2018). Potential of wheat straw, spruce sawdust, and lignin as high organic carbon soil amendments to improve agricultural nitrogen retention capacity: an incubation study. Front. Plant Sci. 9. doi: 10.3389/fpls.2018.00900
Richards L. A. (1931). Capillary conduction of liquids through porous mediums. Physics 1, 318–333. doi: 10.1063/1.1745010
Sieling K., Kage H. (2006). N balance as an indicator of n leaching in an oilseed rape - winter wheat - winter barley rotation. Agriculture Ecosyst. Environ. 115, 261–269. doi: 10.1016/j.agee.2006.01.011
Sieling K., Kage H. (2010). Efficient n management using winter oilseed rape. A review. Agron. Sustain. Dev. 30, 271–279. doi: 10.1051/agro/2009036
Sieling K., Günther-Borstel O., Teebken T., Hanus H. (1999). Soil mineral N and N net mineralization during autumn and winter under an oilseed rape-winter wheat-winter barley rotation in different crop management systems. J. Agricult. Sci. 132, 127–137. doi: 10.1017/S0021859698006273
Smith J., Smith P., Addiscott T. (1996). Quantitative methods to evaluate and compare soil organic matter (SOM) models, in: evaluation of soil organic matter models, NATO ASI series. Springer Berlin Heidelberg 38, 181–199. doi: 10.1007/978-3-642-61094-3_13
Stockmann U., Adams M. A., Crawford J. W., Field D. J., Henakaarchchi N., Jenkins M., et al. (2013). The knowns, known unknowns and unknowns of sequestration of soil organic carbon. Agriculture Ecosyst. Environ. 164, 80. doi: 10.1016/j.agee.2012.10.001
Stützel H., Kage H. (1998). Lnfluence of tillage and nitrogen fertilisation within the rotation on the nitrogen dynamics after faba beans. Pflanzenbauwissenschaften 2, 199–206.
Toenshoff C., Joergensen R. G., Stuelpnagel R., Wachendorf C. (2014). Initial decomposition of post-harvest crown and root residues of poplars as affected by n availability and particle size. Biol. Fertility Soils 50, 675–683. doi: 10.1007/s00374-013-0882-7
Trinsoutrot I., Nicolardot B., Justes E., Recous S. (2000). Decomposition in the field of residues of oilseed rape grown at two levels of nitrogen fertilisation. effects on the dynamics of soil mineral nitrogen between successive crops. Nutrient Cycling Agroecosystems 56, 125–137. doi: 10.1023/A:1009838618133
van Genuchten M. (1980). A closed-form equation for predicting the hydraulic conductivity of unsaturated soils. Soil Sci. Soc. America J. 44, 892–898. doi: 10.2136/sssaj1980.03615995004400050002x
Verberne E. L. J., Hassink J., De Willigen P., Groot J. J. R., Van Veen J. (1990). Modelling organic matter dynamics in different soils. Netherlands J. Agric. Sci. 38, 221–238. doi: 10.18174/njas.v38i3A.16585
Wickham H., Averick M., Bryan J., Chang W., McGowan L. D., François R., et al. (2019). Welcome to the tidyverse. J. Open Source Software 4, 1686. doi: 10.21105/joss.01686
Keywords: residue management, plant-soil-atmosphere model, soil health, organic amendments, carbon sequestration, N-leaching
Citation: Rothardt S and Kage H (2023) Model-based assessment of biomass incorporation to mitigate nitrogen losses from arable fields in Central Europe. Front. Agron. 5:1155187. doi: 10.3389/fagro.2023.1155187
Received: 31 January 2023; Accepted: 28 April 2023;
Published: 17 May 2023.
Edited by:
Gian Franco Capra, University of Sassari, ItalyReviewed by:
Tao Ren, Huazhong Agricultural University, ChinaEleonora Grilli, University of Campania Luigi Vanvitelli, Italy
Copyright © 2023 Rothardt and Kage. This is an open-access article distributed under the terms of the Creative Commons Attribution License (CC BY). The use, distribution or reproduction in other forums is permitted, provided the original author(s) and the copyright owner(s) are credited and that the original publication in this journal is cited, in accordance with accepted academic practice. No use, distribution or reproduction is permitted which does not comply with these terms.
*Correspondence: Steffen Rothardt, cm90aGFyZHRAcGZsYW56ZW5iYXUudW5pLWtpZWwuZGU=