- 1Department of Biological and Pharmaceutical Science, Munster Technological University (MTU), Tralee, Kerry, Ireland
- 2Marigot Research Centre, Sycamore Court, Clash, Tralee, Co. Kerry, Ireland
- 3Centre for Applied Bioscience Research, Munster Technological University (MTU), Tralee, Kerry, Ireland
Feed additives to reduce enteric methane (CH4) emissions from ruminants are gaining attention to help curb agriculture’s 24% share of global CH4 emissions. Several mechanisms of action of feed additives for mitigating rumen methanogenesis have been identified from ongoing research, however, there is still a need to determine the most effective method and explore potential synergies between these different approaches. This study evaluates the CH4 mitigation potential of nine natural feed additives, focusing on their mode of action in reducing CH4 emissions during in vitro fermentation. The natural feed additives assessed include garlic oil (GO), garlic powder (GP), allicin (ALL), yucca schidigera plant extract (Yucca), and an essential oil blend (EO), all functioning as rumen microbiome modifiers. Calcareous marine algae rumen buffer (CMA) and its magnesium oxide-fortified form (CMA.MgO) acted as hydrogen sinks, while Asparagopsis taxiformis (cultured and ocean forms) inhibited the central enzyme involved in methane metabolism. Total gas, CH4, and volatile fatty acid (VFA) outputs were recorded after in vitro batch fermentations simulating rumen 24 h metabolic events. The CMA.MgO rumen buffer displayed significant reductions (P=0.02) in both CH4 emissions relative to the control (Rel % CH4; 40.1%) and total gas production relative to the control (Rel % Total gas; 22.9%). The greatest synergistic effect on gas emissions was achieved by combining GP with the CMA buffer treatment, leading to significant reductions (P<0.05) in Rel % Total gas by 37% and Rel % CH4 by 64.5%. Additionally, sole supplementation of CMA.MgO, GO, and Yucca demonstrated improved rumen productivity by increasing total VFAs by 39.8%, 24.4%, and 22.6% compared to the control result, respectively. Feed additives altering rumen microbial populations by reducing methanogens and promoting VFA production increase readily available energy for the animal while reducing CH4 generation significantly. Semi-continuous rumen culture fermentations or in vivo studies can confirm the long-term stability of synergistic antimethanogenic mechanisms, potentially optimizing CH4 inhibitors like A. taxi and EO for commercial use.
1 Introduction
There is considerable interest in identifying natural bioactive compounds that have the potential to manipulate the rumen microbiome and fermentation processes. Manipulation of the rumen environment has the potential to protect animals against prevalent diseases, improve energy by increasing volatile fatty acids (VFAs) availability (Huang et al., 2021), increase product yield by elevating organic matter digestibility (Campanile et al., 2008), and minimize the production of enteric methane (CH4) (Tong et al., 2020; Wasson et al., 2023). Mitigation strategies implemented to reduce the increase in enteric gases, especially CH4, include dietary supplementation, animal management, and rumen manipulation. This study was aimed at investigating dietary supplementation approaches to support ruminant productivity and mitigate enteric CH4 in line with emission reduction targets (Andersen et al., 2005; Masson-Delmotte et al., 2018).
Commercially available feed additive products claiming to mitigate enteric CH4 emissions include red seaweed Asparagopsis taxiformis (LomeTM, Solna, Sweden) and 3-Nitroooxypropanol (3-NOP), marketed as Bovaer (DSM, Kaiseraugst, Switzerland). Both additives work by inhibiting the central methyl-coenzyme M reductase (MCR) enzyme in the methanogenesis pathway, effectively inhibiting CH4 production. Methanogenesis inhibitors provided as feed additives to ruminants have been the best performers thus far, with the highly bioactive seaweeds from the Asparagopsis genus demonstrating the greatest antimethanogenic potential (Roque et al., 2019, 2021). Recent reviews by Hristov (2024) and Arndt et al. (2022) summarize the CH4 mitigation potential of enzyme inhibitors exceeds 28% in daily CH4 emission yield and intensity. Despite the significant reduction in enteric CH4 achieved with both enzyme inhibiting additives, questions remain regarding their consistency and the potential for rumen ecosystem adaptation (Hristov, 2024). This underscores the need for continued research into alternative feed additive CH4 mitigating strategies and the exploration of ruminal adaptation to commercial feed additives during extended supplementation periods.
An essential oil blend of coriander (Coriandrum sativum) seed oil (up to 10%), eugenol (up to 7%), geranyl acetate (up to 7%), and geraniol (up to 6%) (Agolin SA, Bière, Switzerland), as well as a mix of garlic and citrus extract (Mootral SA, Rolle, Switzerland) are both labeled as antimicrobial agents in the rumen against methanogens. Essential oils demonstrate initial antibacterial activity by inhibiting the growth of gram-positive ruminal microorganisms and antiprotozoal activity (Patra and Yu, 2012), thus, reducing CH4 emissions from dairy cattle (McIntosh et al., 2003). In recent reviews investigating effective strategies for mitigating enteric CH4 emissions, oil and lipid supplementation displayed an average decrease of 12.9% in CH4 intensity for dairy and beef cattle (Arndt et al., 2022; Hristov, 2024). Furthermore, in a literature review by Honan et al. (2022), the garlic and citrus extract mix demonstrated greater average CH4 reduction potential in beef and dairy cattle studies compared to the commercial essential oil blend at an increased average dose rate of 1.14 g/kg DM compared to 0.07 g/kg DM, respectively. However, evidence of rumen adaptation to the supplementation of the garlic and citrus extracts is evident in trials by Klop et al. (2017) and Hart et al. (2019) that lasted 10 and 23 weeks, respectively. Rotation of rumen microbial modifying essential oils (EO) and lauric acid supplementation to lactating dairy cows on a weekly basis showed no improvement in CH4 mitigation in contrast to sole supplementation of EO over a 10-week period (Klop et al., 2017). Alternatively, rotation of rumen feed additives with different modes of action for CH4 mitigation may reduce the occurrence of rumen adaptation and possibly induce greater CH4 mitigation compared to individual feed additive supplementation. Rotational supplementation of feed additives targeting different CH4 production pathways may allow for the overlap in their antimethanogenic effects, preventing microbial adaption and maintaining the treatments effectiveness. Therefore, implementing a similar 2-week or weekly supplementation rotation structure, as described by Guan et al. (2006) and Klop et al. (2017) respectively, may benefit from rotating feed additives with different modes of antimethanogenic action. Antimicrobial rumen modifiers supplemented as mixtures to the rumen appear to induce enteric CH4 mitigation without impacting ruminant feeding and productivity behavior (Khurana et al., 2023; Miller et al., 2023), suggesting the opportunity to apply further feed supplementation with an alternative CH4 mitigating approach to explore possible synergistic effects.
Additionally, feed grade calcium nitrate (Bolifor, York, United Kingdom) is implemented as an additional hydrogen sink in the rumen to divert free hydrogen away from methanogenesis. Calcium nitrate has previously displayed a linear decrease in CH4 emissions with increasing inclusion rates (Olijhoek et al., 2016). Hydrogen sinks that act on rumen conditions to demote methanogenesis display an average decrease of 15% in CH4 yield in beef and dairy animals as seen in the meta-analysis of CH4 mitigation strategies by Arndt et al. (2022). Nitrate appears to be the most effective electron sink treatment for mitigating enteric methane without transient effect over long-term studies (Feng et al., 2020; Van Zijderveld et al., 2011). However, adaptation periods and gradual build-up of nitrate dietary inclusion are required to reduce the risk of nitrite build-up in the rumen and absorption into the bloodstream resulting in the development of methemoglobinemia (Nolan et al., 2016). Alternative rumen hydrogen-sequestering approaches, such as calcareous marine algae (CMA), have demonstrated hydrogen ion neutralization and stability within the rumen physiology, as reported in previous studies (Neville et al., 2019; Rossi et al., 2019). Therefore, CMA may serve as an effective alternative to nitrate-based feed additives for methanogenesis inhibition. This study follows on from previous in vitro research by Durmic et al. (2014), where a moderate reduction in methane production was found with calcareous marine algae supplementation.
To identify natural bioactive compounds with the most effective mode of action for enhancing CH4 mitigation and ruminant productivity, a high throughput in vitro batch culture method for simulating rumen enteric fermentation products was assembled. This method was adapted from existing methodologies in the literature to screen CH4 mitigating compounds, categorized by their mechanisms of action, for use in ruminant feed supplementation (Brooke et al., 2020; Sarker et al., 2018). The feed supplements were selected for further in vitro investigation based on a meta-analysis originating from literature-based candidates with CH4-mitigating potential by Hodge et al. (2024) and existing literature. The selected feed additives underwent in vitro screening to collect fermentation gas levels and profiles of the primary VFAs, acetate (or acetic acid), propionate (or propanoic acid), and butyrate (or butyric acid), through corresponding fermentation runs. This method was employed to facilitate direct comparisons and evaluate the effectiveness of each CH4 reduction strategy across various representative treatments.
2 Materials and methods
2.1 Donor animals and rumen fluid collection
All procedures described in this experiment were approved by the Animal Welfare Body (AWB) at Munster Technological University (MTU), Kerry, Ireland. It was concluded that the animals in this project were slaughtered for another purpose before sampling. As the animals were not alive at the point of scientific sampling, there was no requirement for a Health Products Regulatory Authority (HPRA) licence, nor does it come under the remit of the AWB. A certificate of registration was obtained from the Department of Agriculture, Food and the Marine (DAFM) which authorized the transport and use of cattle rumen fluid obtained from ABP in accordance with Regulation (EC) No. 1069 of 2009 and Regulation (EU) No. 142 of 2011, Registration No. HAC2426. Procedures related to the care and handling of the experimental animals were conducted under protocols approved by the ABP food group quality team. Prior to every in vitro run, all glassware was autoclaved to achieve steam sterilization. Rumen fluid samples were collected from different areas of the rumen within Friesian and Friesian cross animals. The donor animals with a mean age of 4.3 ± 0.5 years and mean hot weight of 318.1 ± 21.0 kg were managed in ABP Food Group, Bandon and the rumen fluid was obtained within 2 minutes of each donor animal’s time of death. The rumen digesta was strained over two stainless steel sieves with a pore aperture of 1.18 mm and 600 μm and the fluid was directly funneled into a sterilized 1.2 L thermos flask for insulated transportation. The thermos flask was filled 1 inch from the top of the flask and purged with CO2 for 20 seconds before being tightly sealed in an upright position for transportation to the fermentation lab set-up, preventing spills and maintaining the CO2 headspace. A second sterilized thermos flask was used to collect filtered rumen fluid through two layers of cheesecloth, removing larger particles of rumen digesta. The pH and temperature of the rumen fluid were recorded upon arrival at the laboratory after each collection. The headspace of the thermos flask was again purged with CO2 for 20 seconds and sealed for relocation to a vertical laminar flow hood to prevent contamination of fermentation vessel contents during filling.
2.2 Experimental design
Following collection, rumen fluid was diluted (1:2 v/v) with pre-heated (39°C) artificial saliva buffer (pH 8 ± 0.015) (Cone, 1998) to closely resemble realistic ruminal pH conditions in the preheated septa port glass bottles over the 24 h fermentation period (see Supplementary Figure S1A). Fresh forage was added to each 250 mL bottle contents at 1.15 g dry matter (DM) dosage simulating the Bord Bia grass-fed standard conformance by ABP food group, Bandon (Bord Bia, 2020) and the average dry matter intake of 16.7± 0.25 kg of DM/d investigated for grazing cattle (Coleman et al., 2010). The fresh material was 3-leaf stage perennial ryegrass collected fresh on the morning of each in vitro incubation. Composition analysis of the grass diet was carried out by a forage analysis laboratory (FBA Laboratories, Waterford, Ireland) for wet chemistry-based analysis (Table 1). The procedures used in the wet-chemistry analysis of the forage portion used in this experiment is detailed in the next section (section 2.3).
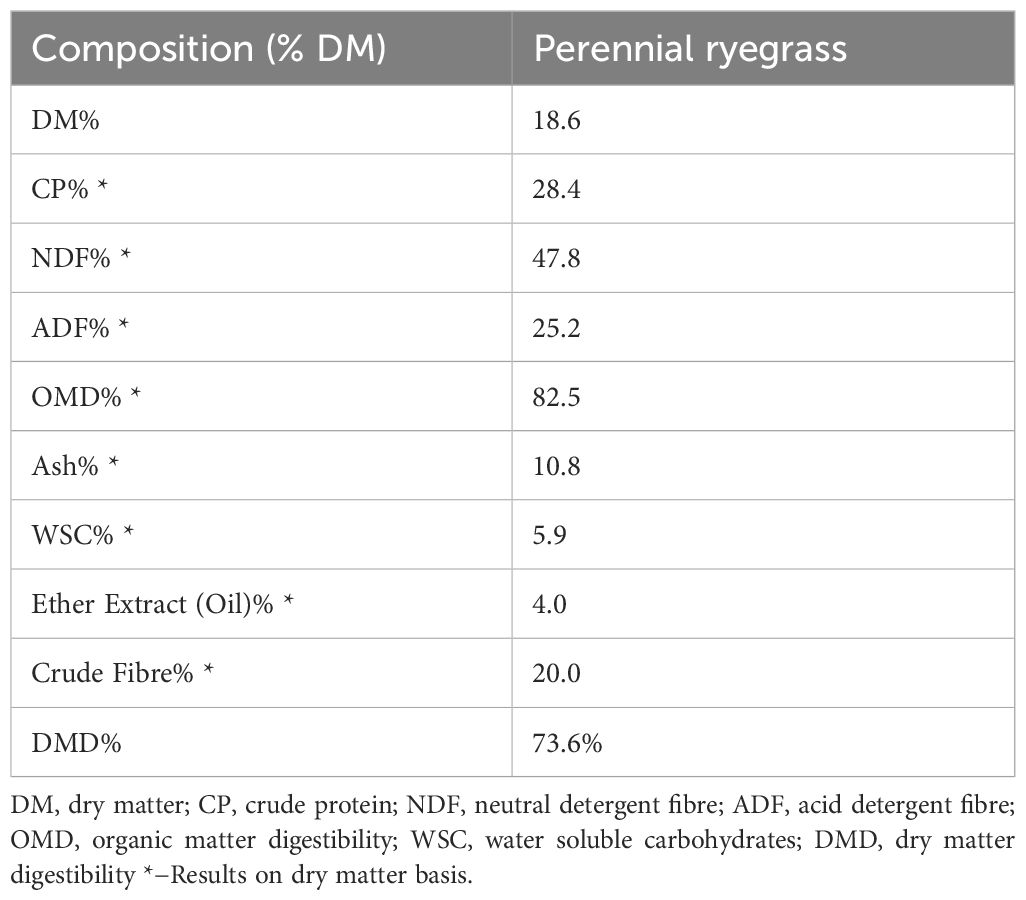
Table 1. Composition of perennial ryegrass fed to in vitro fermentation vessels at the beginning of each 24 h fermentation run.
Fresh forage was sealed in Ankom 5cm x 5cm 50-micron porosity feed bags (ANKOM Technology Ltd., New York, United States) and submerged in the buffered rumen fluid vessel contents to allow for gradual digestion within the fermentation units (see Supplementary Figure S1A). Treatments assigned to the 250 mL vessels included the following: rumen microbiome modifying Allium sativum derived garlic oil (GO), garlic powder (GP) and the plants bioactive compound allicin (ALL) (Axenic health solutions, Texas, US), Yucca schidigera plant extract (Yucca) (Agroin, Baja California, México), and an essential oil blend (EO) (Agolin SA, Bière, Switzerland), hydrogen-sequestering rumen buffers CMA (Lithothamnion glaciale) and the algae’s magnesium oxide-fortified form (CMA.MgO) (Celtic Sea Minerals, Cork, Ireland), and the MCR inhibitor Asparagopsis taxiformis, in both its cultured form (A. taxi cultured) and its ocean form (A. taxi ocean) (LomeTM, Solna, Sweden).The screening period included a total of 15 treatment variations and combinations that were investigated in at least 3 biological replicates. All individual biological replicates are the average of duplicate technical replicates for 24 h in vitro incubation. Optimum doses for both the marine based rumen buffer (CMA) and the essential oil (GO) were determined for combination treatment investigations based on dose response results and suitable doses for on-farm applications. Once the vessel filling was completed an airtight seal was established with the numbered ANKOM RF modules (ANKOM Technology Ltd., New York, United States). The sealed 250 ml bottles were incubated in a temperature-controlled orbital shaking water bath with pre-set parameters of 39°C and 85 rpm (see Supplementary Figure S1B). The current in vitro setup was adapted for optimum fermentation conditions based on previous in vitro methodologies (Brooke et al., 2020; Sarker et al., 2018), and to simulate rumen temperature conditions, rumination and, animal activity (Abeni and Galli, 2017). Initially, 99.9% CO2 was flushed through the headspace of each fermentation bottle and evacuated through the septa port for 10 seconds. The septa ports were then sealed before being purged with 99.9% CO2 to allow for a build-up of 5 pounds per square inch of CO2 within the headspace of each sealed bottle. The CO2 gas was held within the headspace for 30 minutes while incubated and in orbital motion to allow for absorption of the CO2 gas within the rumen fluid inoculum. Venting valves for each of the modules were opened in unison to allow for a controlled release of the CO2 purge gas. The valves were then sealed after purge gas evacuation to allow for the collection and recording of enteric gas. Headspace gas readings are detected via radio frequency technology which is transmitted to a base coordinator and recorded on ANKOM software as raw data in an Excel file. Total gas production was measured continuously for a maximum of 24 h with pressure readings for each module being recorded in 10-minute intervals. Enteric gas values expressed in mL/g DM were determined by application of the natural gas law to the final gas pressures while accounting for individual vessel headspace volumes.
2.3 Feed sample analysis
Subsamples of the fresh perennial ryegrass were dried and ground for wet chemistry analysis. The gravimetric method with sample drying in a Genlab fan oven at 103°C for 4 hours was used to determine DM by difference in initial weight vs final weight. Crude protein was determined using a LECO ®928 series carbon and nitrogen determinator instrument (LECO Instruments UK Ltd., Stockport, United Kingdom). Ether extract (oil) was extracted using a Gerhardt Soxtherm Variostat extraction system (Gerhardt UK Ltd., Northamptonshire, United Kingdom) by weighing sample into cellulose thimbles and subjected to an automated extraction in petroleum ether. Petroleum ether was selected over alternative solvents such as diethyl ether for lipid extraction due to its greater selectivity for more nonpolar lipids. The percentage crude fibre was determined by digesting the silage sample in 0.26N H2SO4 and 0.31N NaOH solutions under specific conditions. Subsequently, the digested sample was dried in a Genlab fan oven. The crude fibre content was then determined by measuring the loss of ignition of the remaining dried residue in a Carbolite muffle furnace (Carbolite Gero Ltd., Hope, United Kingdom) kept at 500°C for at least 4 hours. Acid detergent fibre (ADF) was reported using the filter bag technique in an Ankom 220 Fibre Analyser digestion instrument using acid detergent solution (20 g cetyltrimethylammonium bromide (CTAB) to 1 L of 1N H2SO4 previously standardized) (Ankom Technology, New York, United States). The neutral detergent fibre (NDF) procedure was carried out using the filter bag technique in an Ankom 220 Fibre Analyser digestion instrument treated with neutral detergent solution with an average pH value of 7.0 ± 0.1. The neutral detergent solution contained 30 g of Sodium dodecyl sulfate (USP), 18.61 g of Ethylenediaminetetraacetic disodium salt (dehydrate), 6.81 g of Sodium borate, 4.56 g of Sodium phosphate dibasic (anhydrous), and 10.0 ml of Triethylene glycol in 1 L of distilled H2O. To determine the digestible organic matter percentage (OMD%), samples were placed into an Ankom Daisy Incubator digestion jar along with cellulase and acetate buffer solution. The digestion was run for 48 hours at 39°C, followed by removing the sample bags, washing with water, and subject to NDF digestion as discussed above. Ash was determined with the loss on ignition method using a Carbolite muffle furnace at 500°C. Water soluble carbohydrates (WSC) were determined by phenol-sulfuric acid sample preparation followed by UV spectrophotometry using a Genesys 150 UV-Visible Spectrophotometer (Genesys, California, United States) and 10 mm cuvettes. Absorbance was measured at 485 nm as it corresponds to the distinct absorption peak of the phenol-sulfuric acid complex.
2.4 Determination of methane and VFA production
In vitro end products (enteric gas and volatile fatty acids) collected post-24-hour incubations were analzed via gas chromatography. Headspace gas was collected using 12 mL disposable syringes with removable needles via the septa port of each 250 mL bottle after 24 h incubation (see Supplementary Figure S1A). A total of 12 mL was extracted from each headspace and dispensed into separate 12 mL pre-evacuated glass vials with double-wadded PTFE/silicone septa (Labco Ltd., Lampeter, United Kingdom). The vials were labeled with the date, headspace pressure in psi, treatment added, and module head number for identification. Gas chromatography (GC) (Shimadzu GC-2010, Shimadzu Corporation, Kyoto, Japan) equipped with a 30 m x 0.53 mm Carboxen 1010 capillary column with a flame ionization detector (FID) was used to determine the amount of CH4 in each of the samples. 150 μL samples were manually injected into the column. The oven temperature was held at a 75°C iso thermic temperature. The FID was held at 270°C and the injector was set to 200°C. Nitrogen was used as a carrier gas with a total flow rate of 21.0 mL/min and a column flow of 3 mL/min. The average DM supplemented (1.15 g) to fermentation vessels throughout the in vitro treatment screening period was used to standardize gas concentrations. Accumulated control total gas and CH4 values (mL) achieved were set to 100% and the other treatment values were presented as a percentage of total gas and CH4 relative to the untreated control in each 24-hour run (Tables 2, 3). The control in each run was set as the benchmark 24 h gas accumulation to ensure comparability between novel batches of rumen fluid each week. Standard calibration curves were generated using standards prepared manually using Nitrogen and CH4 certified by Air Products (Air Products Plc, Cheshire, England) (see Supplementary Figure 2).
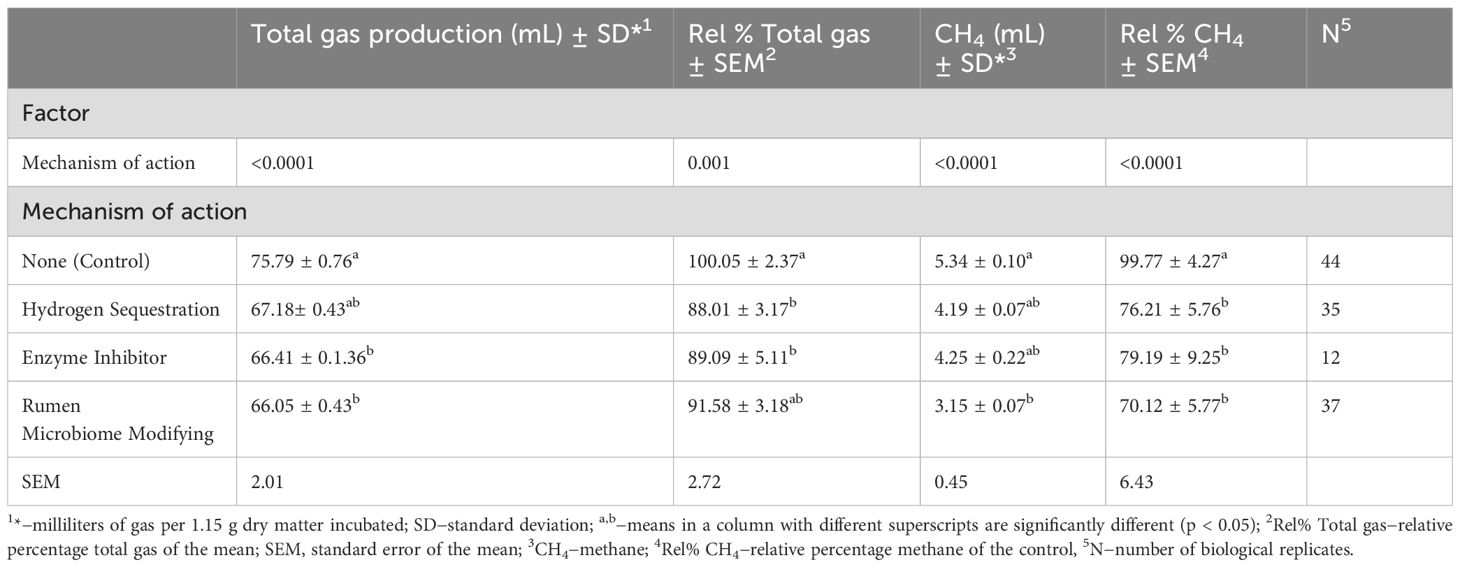
Table 2. Effect of additive’s mechanisms of action on gas production post 24 h incubation in buffered rumen fluid from Friesian cow donors.
Seven treatments that exhibited the greatest CH4 mitigation capabilities which were inoculated at doses feasible for on-farm supplementation were analyzed for VFA profiles. Volatile fatty acid sample deproteinization was performed by adding 200 μL of 25% metaphosphoric acid and formic acid (3:1) mixture to 1 mL of rumen fluid from each fermentation vessel post-24-hour incubation, based on a method adapted from Poblete et al. (2020). The mentioned ratio and solutions were selected based on the ability for 25% metaphosphoric acid to precipitate the proteins and the prevention of ghost peaks appearing on the chromatogram by using formic acid (Cottyn and Boucque, 1968). Samples were allowed to sit for 30 minutes before being centrifuged twice for 30 minutes at 3900 rpm in a benchtop centrifuge (Centrifuge 5810, Eppendorf) to separate the precipitated protein in the clear supernatant from the particulate debris pellet. Each supernatant (1 mL) was stored in 1.5 mL Eppendorf tubes at 4°C until GC analysis. The samples were diluted 10-fold with 3:1 methanol to deionized water in 1.5 mL chromatography vials to prevent the smearing phenomenon in chromatographs described by Luo et al. (2015). After dilution, the samples were injected from 1.5 mL chromatography vials (1.0 μL quantities, in split mode with a ratio of 5.0) via an AOC-20i autosampler into a Shimadzu GC-2010 gas chromatography unit equipped with a capillary column (SH-Stabilwax-DA, 30 m x 0.53 mmID x 0.25 μm, Shimadzu) with nitrogen as the carrier gas. The GC set-up was adapted from Luo et al. (2015), the flow rate was 37.4 mL/min, column temperature was held at 100°C for 2 minutes, increased at a rate of 8°C/min to 164°C and held for 1 minute, followed by an increase in temperature by 20°C/min to 200°C and held for 1 minute. The temperatures for the injector and the FID were held at 280°C and the cycle time for each analysis was 21 minutes. Total VFA concentrations are measured in mmol VFA/L of rumen fluid incubated and the individual acid concentrations are recorded in percentage mmol/mmol total VFA. An external standard column test mixture of free fatty acids (Thames Restek Ltd., Wycombe, United Kingdom) was prepared before the beginning of the sample analysis. The standard mix underwent a ten-fold dilution in a 3:1 solution of methanol and deionized water and was analyzed to achieve a good correlation between the measured response and concentrations of each acid (R2 ≥ 0.99) (see Supplementary Figures 3–8). The acids included acetic, propanoic, isobutyric, butyric, isovaleric, and valeric acid which are commonly found in the rumen (Lee et al., 2021; Luo et al., 2015).
2.5 Statistical analysis
Enteric gas expressed as a percentage of the control vials, and VFA level changes were analyzed using the repeated measures analysis of variance using mixed models via Addinsoft XLSTAT-Basic+ software (version 2021.2.2). The model included the fixed effect of treatment, and the random effect of the rumen fluid inoculum used in each fermentation run to determine the presence of significant influence induced by both fixed and random effects. Significance of fixed effects was determined using Fisher’s F-test with consideration for the random effect of rumen fluid variation (via orthogonal decomposition) to enable the study of the unbalanced dataset. The Tukey honestly significant difference (HSD) test was used to test differences between sample means for significance. For non-parametric enteric gas production data (milliliters of gas per 1.15 g DM incubated), the Kruskal-Wallis test was used for comparison of k samples. Dunn’s post-hoc test for multiple comparisons was used between each independent treatment to identify which treatments were significantly different. Differences were declared significant at P < 0.05.
3 Results
3.1 In vitro gas production
Initially the treatments were categorized based on their mechanism of action and analyzed for their corresponding gas production response, as shown in Table 2. The results indicate significant reduction potential in CH4 emissions for hydrogen sequestering (−23.6%; P=0.01), enzyme inhibiting (−20.6%; P=0.03), and rumen microbiome modifying additives (−29.6%; P<0.01) compared to the control values (Rel % CH4). Additionally, hydrogen sequestering (−12%) and enzyme inhibiting (−11%) categories showed significant reductions (P<0.03) in total gas production relative to the control values (Rel % Total gas), while a trend towards significance (−8.5%) was observed for rumen microbiome modifying additives. Subsequently, the investigation into each treatment’s optimal dose–response on in vitro gas emissions are presented in Table 3 and discussed under the related subheadings of this section. Dose-response curves for the hydrogen-sequestering CMA treatment and rumen microbiome-modifying GO treatment are shown in Figures 1, 2, respectively.
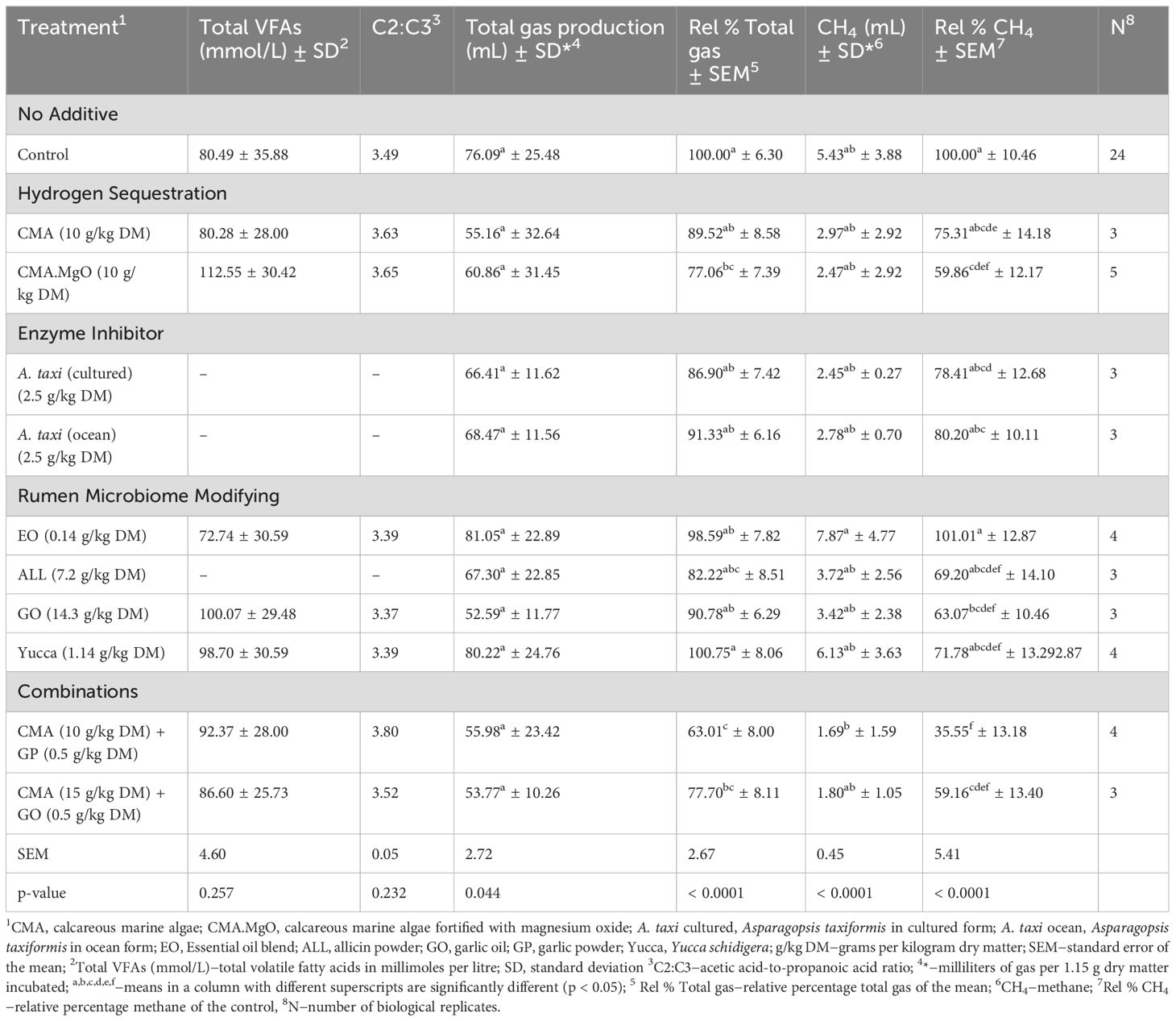
Table 3. Effect of additives on volatile fatty acid concentrations and gas production post 24 h incubation in buffered rumen fluid from Friesian cow donors.
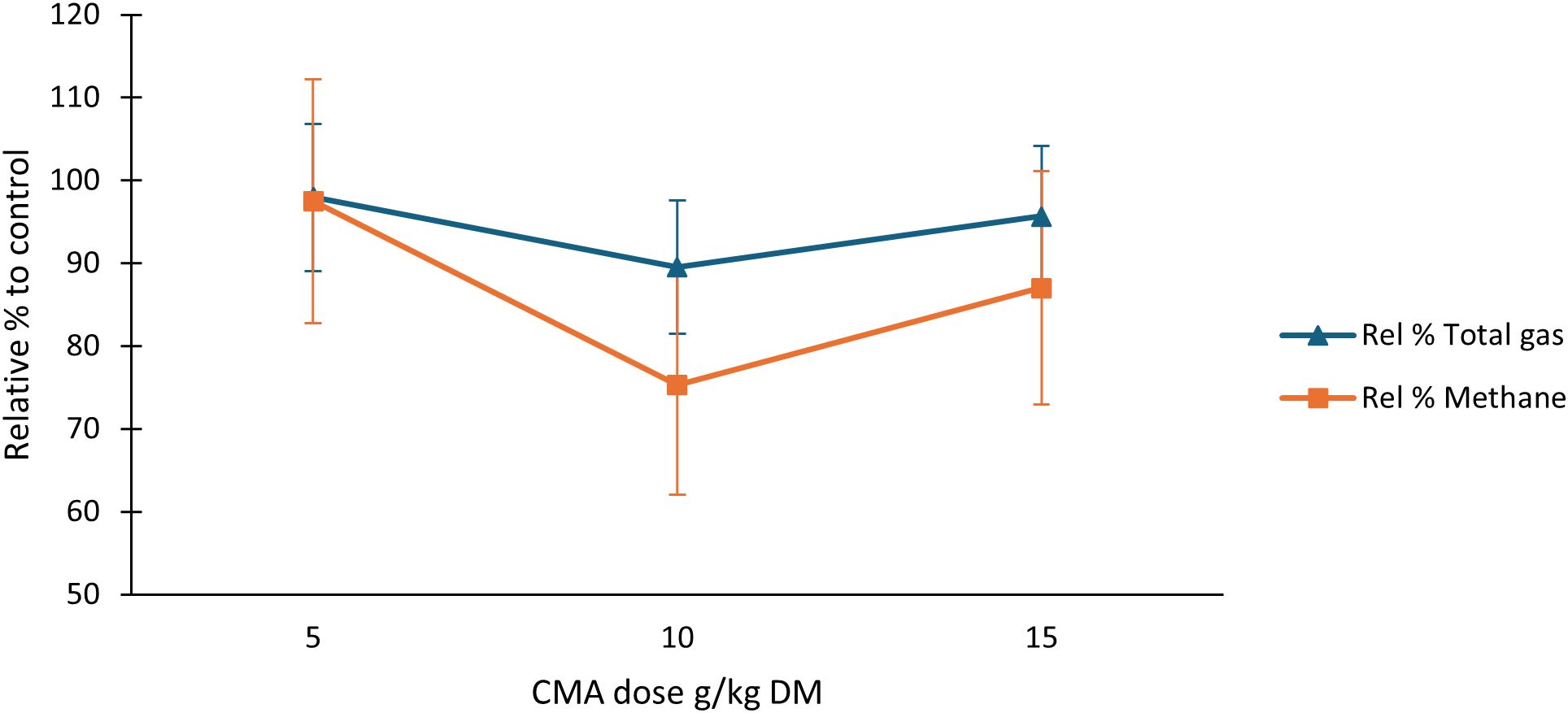
Figure 1. Dose response of CMA for total gas and methane post 24 h rumen simulation. n ≥ 3. CMA, calcareous marine algae; Rel % Total gas, relative percentage total gas to control; Rel % CH4, relative % methane to control.
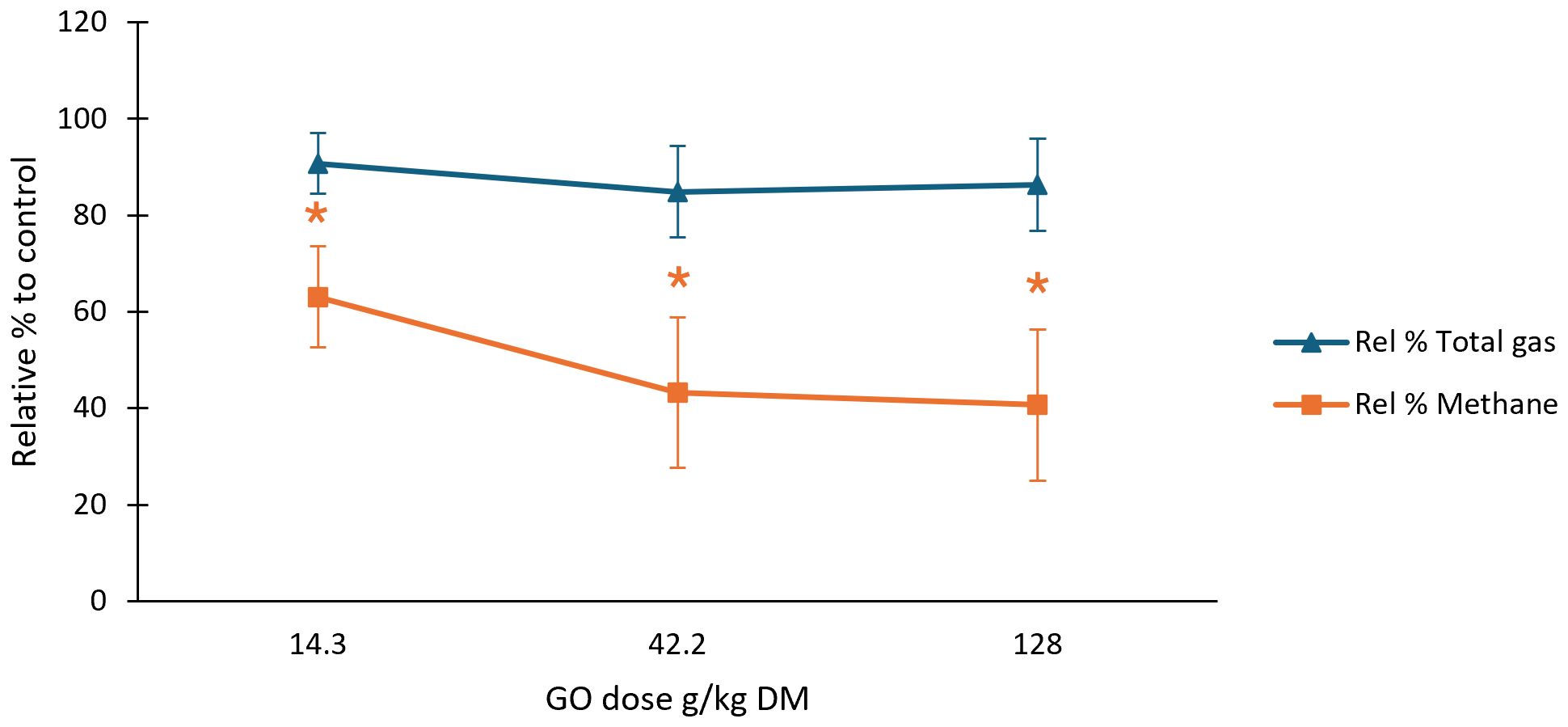
Figure 2. Dose response of GO for total gas and methane post 24 h rumen simulation. n=3. GO, garlic oil; colour coordinated four-point shapes (*) signify significant differences (p < 0.05); Rel % Total gas, relative percentage total gas to control; Rel % CH4, relative % methane to control.
3.1.1 Rumen microbiome modifying bioactives
Maintenance of Rel % Total gas production and significant reductions in Rel % CH4 with GO at its optimum dose depicted from literature (42.2 g/kg DM), facilitated the dose response investigation seen in Figure 2. The lower dose of GO (14.3 g/kg DM) was identified as a more practical level of GO for on-farm supplementation based on gas production results (Table 3, Figure 2) and was therefore the selected dose for VFA analysis, as discussed in later sections. Supplementation with GO resulted in reduction in total gas compared to the control equating to 9.2, 15.1, and 13.7% when the in vitro administration dosage was increased in increments of 14.3, 42.2, and 128 g/kg DM (Figure 2), respectively. There was a trend between GO dosage and significant reduction (P < 0.04) in Rel % CH4 of 36.9, 56.7, and 59.3% as the concentration of GO was increased to 14.3, 42.2, and 128 g/kg DM, respectively (Figure 2). The plant active component ALL was investigated at a concentration of 7.2 g/kg DM, to simulate the natural amount found in the high dose of garlic oil (128 g/kg DM) extracted from Allium sativum (Busquet et al., 2005; Rahman, 2007). The plant component induced a reduction of 17.8 in Rel % total gas and 30.8 in Rel % CH4 production compared to control values (Table 3). The essential oil blend (EO) when supplemented at 0.14 g/kg DM, demonstrated a decrease of 1.4% accumulated total gas from the control value and induced an increase of 1% in Rel % CH4 to the control across the four biological replicate in vitro runs (Table 3). Yucca plus powder derived from the Yucca schidigera plant was supplemented at a singular dose of 1.14 g/kg DM. The yucca plant supplementation was the only investigated treatment that resulted in increased Rel % Total gas production (0.8%). However, the associated percentage CH4 amount (Rel % CH4) was reduced by 28.2% when Yucca was supplemented over 24 hours.
3.1.2 Hydrogen sequestering bioactives
The selected optimum dose of CMA at 10 g/kg DM maintained Rel % Total gas (89.5%) and induced a 24.7% reduction in Rel % CH4 (Table 3) leading to the dose response graphed in Figure 1. The CMA induced a decrease of 2.1% in Rel % Total gas production when supplemented at the lower dose of 5 g/kg DM. Total gas production declined with respect to the control value as CMA dosage was increased from 5 to 10 g/kg DM, producing an 8.4% reduction (Figure 1). Conversely, Inclusion of 15 g/kg DM CMA elicited a total gas decline of 4.3%. The CMA middle dose (10 g/kg DM) caused the greatest drop of 24.7% CH4 compared to the control (Figure 1). A 13% decrease in CH4 in relation to the control value was identified after 15 g/kg DM supplementation of CMA, surpassing the recommended dose for rumen buffering (5 g/kg DM) with five times its CH4 reduction compared to the control (2.5%).
The supplemented CMA.MgO is a magnesium ion-fortified calcareous marine algae that was investigated for rumen productivity and CH4 mitigation in five separate rumen fluid runs. Average total gas volumes after supplementation of 10 g/kg DM CMA.MgO were decreased significantly (P = 0.02) by 22.9% relative to the control (Table 3). A significant drop (P = 0.02) in CH4 production (40.1%) was observed across in vitro runs after 10 g/kg DM of CMA.MgO was included. The CMA.MgO replicated the middle dose of the CMA but induced a greater as well as significant total gas and CH4 reduction compared to the reference control values.
3.1.3 Enzyme inhibiting bioactives
The red seaweed (A. taxi) was supplemented in both its freeze-dried oceanic and cultured forms, with each form included at the same dose (2.5 g/kg DM). Asparagopsis taxiformis from cultured origin caused a 13.1% decrease in total gas compared to its oceanic form which decreased Rel % total gas by 8.7%. Similarly, the cultured A. taxi induced a greater reduction in Rel % CH4 production (21.6%) in contrast to the capabilities of CH4 reduction with supplementation of the A. taxi in its ocean form (19.8%).
3.1.4 Combination bioactives
The combination of GP (0.5 g/kg DM) with the middle dose of CMA (10 g/kg DM) significantly decreased (P < 0.001) total gas production by 37% in comparison to the control, correspondingly, the CMA (15 g/kg DM) and GO (0.5 g/kg DM) combination induced a significant 22.3% reduction in Rel % Total gas (P = 0.04; Table 3). The combination supplementation of CMA and Allium sativum derivatives induced significant CH4 reductions (P < 0.01; Rel % CH4) of 64.5% and 40.8% with GP and GO supplementation, respectively, suggesting a synergistic effect (Table 3).
3.2 In vitro VFA profiles
The total VFA concentrations and acetic acid-to-propanoic acid ratios (C2:C3) collected from the treatment vessels are presented in Table 3 and discussed under the corresponding mode of action subheadings. Individual VFA proportions in percentage millimole per millimole of total VFA corresponding to each treatment VFA profile are presented in Table 4. The levels of acetic acid, propanoic acid, and butyric acid can collectively account for 95% of the total VFAs found in rumen fluid (Luo et al., 2015). Among the six types of VFA analzed in this study, acetic acid, propanoic acid, and butyric acid accounted for 87.1% of the VFAs analzed. No significant differences were observed between the control mean values and the treatment values concerning total VFA concentrations, whereas significance was identified with individual VFA component propanoic acid and pairwise comparison significance for n-valeric acid.
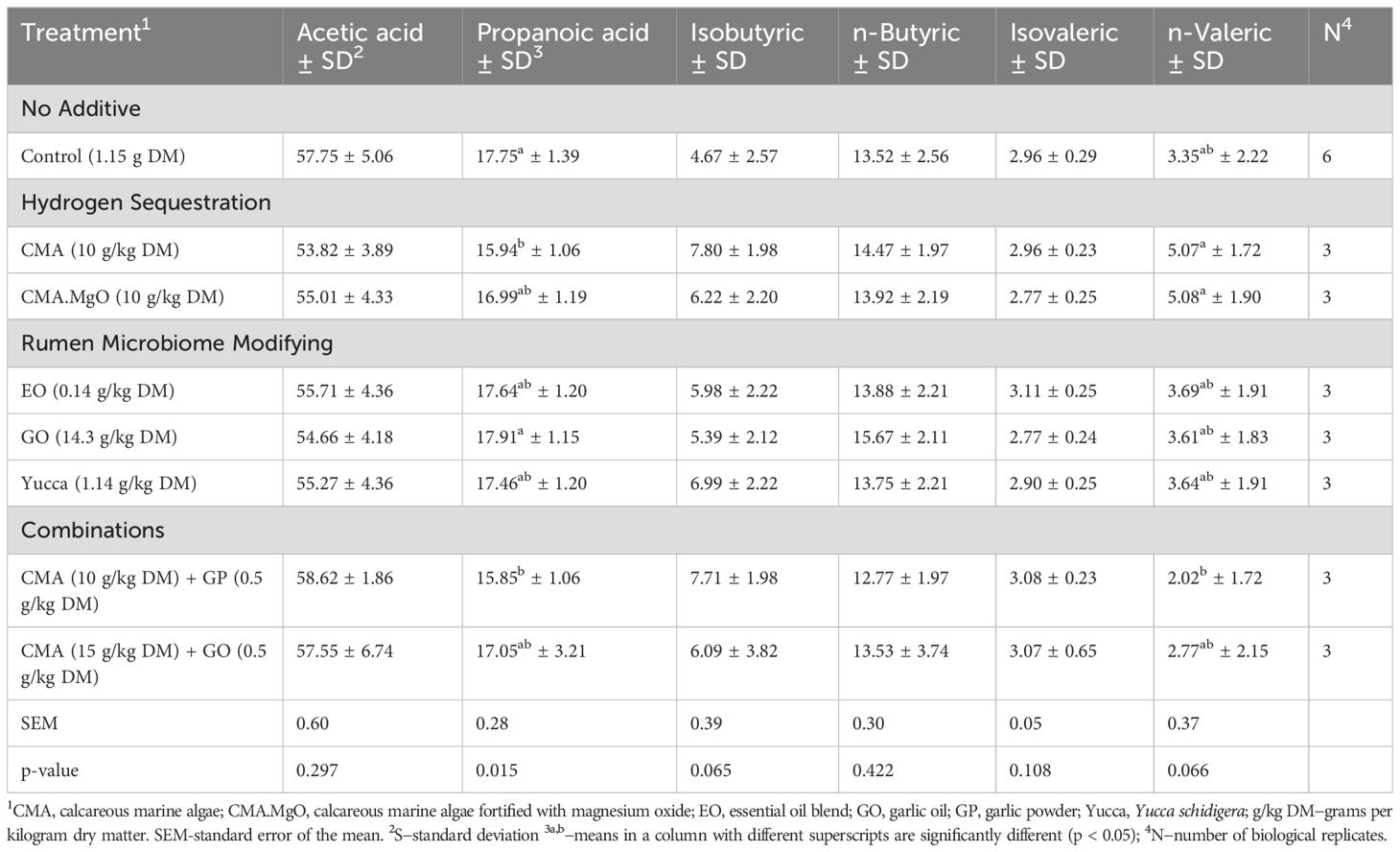
Table 4. Effect of additives on individual VFA millimolar proportions (Percentage mmol/mmol total VFA) of acetic acid, propanoic acid, isobutyric acid, n-butyric acid, isovaleric acid, and n-valeric acid after 24 h incubation.
3.2.1 Rumen microbiome modifying bioactives
Volatile fatty acid profiling of the lower GO dosage (14.3 g/kg DM) generated the second highest concentration of total VFAs (100.1 mmol/L RF; Table 3), 24.4% greater than the control concentration. There were no significant differences in individual VFA concentrations between GO (14.3g/kg DM) and the control after 24 h fermentation. Total VFA’s were decreased by 9.6%, with no instances of significant changes between control and EO treated individual VFA concentrations. Volatile fatty acid profiling of Yucca-treated rumen fluid revealed a 22.6% rise in the total concentration of VFAs. Individual VFA proportions were not significantly influenced by Yucca supplementation.
3.2.2 Hydrogen sequestering bioactives
The middle dose of CMA significantly decreased (P = 0.05) propanoic acid concentrations by 10.2% in contrast to the control (Table 4). The total VFAs and remaining individual VFA concentrations remained largely unchanged by CMA (10 g/kg DM) supplementation. The overall VFA concentration experienced a 39.8% increase compared to the control when fermentation vessels were supplemented with 10 g/kg DM of CMA.MgO. However, the increase in total VFAs associated with CMA.MgO was not significant, with similar trends observed in the individual VFA percentages.
3.2.3 Bioactive combinations
Combining CMA (10 g/kg DM) and GP (0.5 g/kg DM) in the simulated rumen led to an increase in total VFA concentration (14.8%) with a significant reduction (p = 0.02) in propanoic acid (10.7%) compared to the control. Additionally, the second combination treatment of CMA (15 g/kg DM) and GO (0.5 g/kg DM) tended to increase total VFA concentration by 7.6% and inflicted no significant influence on individual VFAs compared to the control value.
4 Discussion
This study investigates the potential of natural bioactive compounds as feed additives, examining their diverse mechanisms of action to enhance ruminal productivity and reduce enteric CH4 emissions, supporting further in vivo trials and potential on-farm applications. The effectiveness of the mentioned feed additives and combinations on rumen productivity was evaluated based on total gas volumes, enteric CH4 levels, and VFA profiles collected and analyzed at the end of each fermentation run. A similar in vitro study achieved similar rumen fermentation gas levels and included a comparable scale fermentation system fed a high forage diet (Ahmed et al., 2021). This study also used the 2:1 ratio of buffer to rumen fluid with a grass-dominant diet for control vessels which was also closely reproduced in the current in vitro study. The control vessels set the benchmark for enteric gas levels and VFA concentrations when investigating treatments for effects on the simulated rumen fermentation and outputs. Total gas production in control vessels was comparable to the headspace enteric gas volumes obtained by Ahmed et al. (2021) using the same forage dominant diet composition, reinforcing the reproducibility and robustness of the experimental setup. This consistency enhances confidence in the methodology and strengthens the credibility of novel treatments and treatment combinations for their observed effects in the current study.
The results in Table 2 support the hypothesis that the bioactive mechanisms of action play a significant role in CH4 abatement, while significant reductions in Rel % Total gas were observed with hydrogen sequestering and enzyme inhibiting mechanisms (Table 2). The promising reductions in Rel % CH4 observed across the three investigated mechanisms, along with the need to identify treatments that enhance fermentation productivity, prompted further analysis of treatments at optimal doses to evaluate their individual and combined impact on fermentation parameters (Tables 3, 4). The inclusion of GO resulted in comparable overall gas percentages, amounting to 90.8%, 84.9%, and 86.3% in relation to the control, as the concentrations of 14.3, 42.2, and 128 g/kg DM GO increased. A corresponding trend with GO inclusion was observed by Patra and Yu (2012), and they reported that as GO was increased in dosage increments there was a linear decrease in CH4 emissions. There were numerical increases in total VFAs, propanoic acid and butyric acid with drops in acetic acid in fermentations supplemented with GO (14.3 g/kg DM). The detected reduction in acetic acid (3.1%) may be credited to the antimicrobial properties of GO, directly inhibiting methanogenic Archaea (Busquet et al., 2006; Kim et al., 2012). As a result, the increased intensity of dissolved hydrogen in the buffered rumen fluid due to methanogenesis suppression, may have suppressed acetic acid production based on the expected stoichiometry of acetate, as reported by Wang et al. (2017, 2018). Hence, hydrogen ions that would have been utilized in the formation of CH4, could have been redirected towards hydrogen sinks, such as propanoic acid. A meta-analysis by Ungerfeld (Ungerfeld, 2015) supporting this concept found that inhibiting CH4 production in ruminal fermentation systems increased hydrogen availability, which was subsequently utilized in the formation of VFAs, particularly propionate. The strength of the rumen buffer used across the in vitro fermentations may have suppressed the potential production of propanoic acid with treatment supplementations. Evidence of VFA shifts to higher levels of propanoic acid in lower ruminal pH conditions supports the theory (Morgante et al., 2007). Similarly, ruminal VFA concentration has been shown to be related negatively to ruminal pH (Dijkstra et al., 2012), suggesting the opportunity to increase total VFAs and decrease acetic acid to propanoic acid concentrations in higher fermentable diets with supplemented treatments such as GO. Intensifying sulfhydryl-modifying activity linked with GO’s bioactive compounds and the GO dosage increase in this study support the possible inhibition of SH-containing enzymes involved in the synthesis of isoprenoid chains found on the cell membrane of methanogenic archaea (Busquet et al., 2006; Patra and Yu, 2012). Steady decreases in CH4 volumes with increasing GO doses may signify the specific targeting of GO’s active components on the rumen archaea domain. Stability in total gas production, as the concentration is increased, may be explained by the predominance of gram-negative bacteria in animals being fed high forage diets (Seshadri et al., 2018; Ternouth, 1968) and GO’s antibacterial activity against susceptible gram-positive bacteria is suspected to be due to the lack of a protectant outer membrane of the cell wall (Burt, 2004; Yasin et al., 2022). Microbial profiling and inclusion of the current GO dosages to rumen fluid fed a high grain diet in vitro could uncover the versatility of GO as a CH4 mitigating feed additive in livestock provided with diverse diets.
The integration of garlic-derived treatments with CMA as an additional antimethanogenic agent broadens the potential of garlic beyond its use as a standalone strategy for enteric methane mitigation as discussed in previous research (Patra and Yu, 2012). Co-dosage of GO with CMA evoked a synergistic effect in terms of CH4 mitigation. GO was supplemented at a much lower dose (0.5 g/kg DM) with 15 g/kg DM of CMA and caused a significant decrease (P = 0.01) in Rel % CH4 (40.8%) (Table 3). In correspondence with this result, at a lower dose of CMA (10 g/kg DM) co-dosed with GP (0.5 g/kg DM) also induced a significant reduction (P < 0.001) in CH4 with respect to the control (64.5%). The significant drops in CH4 levels appear to correlate with significant drops in total gas with reference to the control values, as a greater drop in total gas was observed with CMA and GP (37%) compared to the CMA and GO combination (22.3%) (Table 3). Increases in total VFA concentrations in both combination treatments compared to the control suggest the redirection of metabolic hydrogen in the rumen away from enteric CH4 production and toward VFAs (Ungerfeld, 2015), this in turn would increase the efficiency of ruminant production and decrease environmental impact from rumen fermentation. Additionally, the direct inhibition of methanogens as a hydrogen sink by garlic-derived compounds can increase hydrogen availability (Sari et al., 2022), potentially enhancing CMA’s buffering capacity. This shift in hydrogen utilization may favour pH stabilization over its role in branched-chain fatty acid synthesis, such as valeric acid. This is supported by the significant reduction in valeric acid observed in the CMA with GP treatment compared to CMA and CMA.MgO treatments (P = 0.02). Further research on dosage rates for both CMA and GO is necessary to find optimum levels of both but a potential explanation for this synergistic effect with CMA addition may be the rumen buffer’s ability to neutralize the acidic rumen environment (Cruywagen et al., 2015; Rossi et al., 2019) caused by the GO supplement (pH 4.91). The rumen buffers’ ability to reduce pH fluctuation and improve overall digestion described by Rossi et al. (2019) might explain the maintenance of total gas production as the concentration of CMA was increased. The introduction of CMA with Allium sativum derivatives in vitro may maintain the rumen fluid at a more stable range of pH 6 to 7 when supplemented with more acidic diets, preventing acidosis-like conditions, and providing an optimum pH range for microorganisms (Krause and Oetzel, 2006), including those that are affected by GO’s inhibitory effects. Further in vitro studies with a rapidly fermentable acidic diet and less potent buffer solution are necessary to unveil the potential of CMA in mitigating enteric CH4 and influencing fermentation outcomes in rumen inoculum susceptible to lower ruminal pH conditions.
The supplementation of three CMA doses (5, 10, and 15 g/kg DM) induced non-significant reductions in total gas percentages relative to the mean, with the greatest reduction in 10 g/kg DM CMA supplemented vessels (10.5%). The middle CMA dose (10 g/kg DM) also caused the greatest CH4 reductions relative to the control mean (24.7%) out of the three dosages administered (Figure 1). The middle CMA dose appears to be the optimum amount for enteric CH4 mitigation as improvements in mitigation were not observed as CMA introduction was increased to 15 g/kg DM. Based on CH4 percentages relative to control values in Figure 1, the middle and higher CMA quantities appear to provide adequate buffering capacity to act as sufficient proton acceptors to sequester H2 from methanogenesis over the 24-hour in vitro period. Increasing the dosage of CMA has previously shown improvements in rumen pH ranges when the dose was increased from 0.125% to 1.2% dietary DM (Cruywagen et al., 2004). Similarly, the CMA dose increase from 5 to 10 g/kg DM enhanced rumen fermentation parameters in this in vitro study by mitigating methanogenesis without significantly reducing total gas volume and total VFA concentrations after 24 h incubation. The significant reduction in propanoic acid proportions influenced by CMA at the middle dose may be due to its buffering properties, which stabilize ruminal pH in conjunction with the artificial saliva buffer. This stabilising effect likely inhibits the proliferation of propionate-producing bacteria, as lower ruminal pH has been shown to favour these bacteria (Russell, 1998). Subsequent research may involve conducting additional tests with diets that challenge rumen pH, aiming to investigate the CH4 mitigating capabilities of rumen buffers under conditions resembling rumen fluid susceptibility to acidosis.
The CMA with additional magnesium oxide (CMA.MgO) was supplemented at 10 g/kg DM. CMA.MgO reduced Rel % total gas production by 22.9%, 12.5% more than that observed by CMA alone, and caused a 15.5% greater reduction in Rel % CH4 levels when compared to CMA at the same concentration (10 g/kg DM). Magnesium (Mg) has a higher cofactor capacity than Ca due to its higher coordination number as a central atom for free ions, and greater surface area may stimulate additional enzymes within the rumen. Considering Mg’s cofactor abilities, Mg is linked with promoting approximately 300 cellular enzymes which are responsible for oxidative phosphorylation and metabolism of carbohydrates, lipids, and proteins in the rumen (Ebel, 1980). Promotion of the fermenting rumen species through increased enzymatic action could increase interaction with H2 – utilizing bacteria through “interspecies hydrogen transfer” (Iannotti et al., 1973), thus, maintaining rumen fermentation pathways, pH, and synthesis of VFA’s. A shift towards a 40% increase in total VFAs produced compared to CMA at the same concentration and the control total VFA result (Table 2), strongly suggests enhanced enzymatic catalysis and an increased supply of the primary source of metabolizable energy in ruminants (Bergman, 1990). This, in turn, promotes higher levels of productivity. Furthermore, the carbonate ion (CO3−) found in the CMA and CMA.MgO calcium carbonate structure can utilize hydrogen byproducts, such as those of pyruvate metabolism, in the reduction reaction to bicarbonate (HCO3−) and ultimately carbonic acid (H2CO3). Therefore scavenging H2 from the largest H2 sink being the formation of CH4 where 4 moles of H2 are consumed through the following reaction:
The in vitro results in this study point toward the optimum effect of the CMA on rumen productivity and H2 redirection from CH4 production using the middle dose of 10 g/kg DM.
The principal bioactive component ALL, obtained from Allium sativum, induced a 17.8% decrease in Rel % total gas production when supplemented at a concentration of 7.2 g/kg DM. An average decrease in Rel % CH4 of 30.8% was observed when ALL was supplemented at concentrations resembling the higher GO treatment allicin content (1.4%) typically found in GO (Ahmed et al., 2021; Busquet et al., 2005). In terms of enteric CH4 reduction relative to the control, the GO higher dose with expected equivalent allicin content induced a 28.5% greater reduction compared to the ALL 25% allicin concentrated powder suggesting the importance of the synergistic CH4 mitigating capabilities of GO’s compounds diallyl sulfide, diallyl disulfide, and allyl mercaptan. Busquet et al. (2005) identified significant CH4 mitigation by diallyl disulfide and allyl mercaptan in a separate in vitro microbial fermentation, suggesting the requirement of the primary GO components for optimal anti-methanogenic activity. Our study results display greater anti-methanogenic activity and total gas maintenance across all three GO doses with suspected lower allicin levels in the lower and middle GO dose than the 25% allicin powder supplementation.
Cultured A. taxi provided by a Swedish Greentech company (Volta Greentech, Stockholm, Sweden), showed comparable results with ocean-sourced A. taxi in terms of enteric gas production post-24-hour incubation when supplemented at the same dose rate (2.5 g/kg DM). A. taxiformis halogenated CH4 analogue, bromoform (Merck group Ltd., Darmstadt, Germany), was initially investigated in this in vitro system for its ability to inhibit the MCR enzyme required for methanogenesis in rumen archaea (Duin et al., 2016) and proved very effective with almost complete CH4 inhibition but significantly reduced total gas yield (data not shown). The bromoform concentration of A. taxi is linked to the CH4-mitigating efficacy of this additive (Kinley et al., 2020; Roque et al., 2019, 2021), and the active compound has been shown to deliver the greatest suppression of MCR synthesis in the final step of methanogenesis (Alvarez-Hess et al., 2023; Machado et al., 2016). Similarly, 3-NOP inhibits enteric CH4 emissions by binding to the nickel cofactor active site of MCR in a position that allows for electron transfer with 3-NOPs nitrate group, resulting in MCR inactivation (Duin et al., 2016). Providing A. taxi and 3-NOP containing halogenated methane analogue (HMA) components at 1% organic matter inclusion increases ruminal H2 production (Kirwan et al., 2024; Roque et al., 2019), probably due to the suppression of methanogenesis as the largest H2 sink in the rumen. Based on the current results, bromoform may be at a higher concentration in the cultured seaweed (unspecified) compared to the ocean-sourced seaweed (6–10 mg/g DM) due to the greater reduction induced by the seaweed cultured form on gas production (3%) and CH4 emissions (11.9%) in this study.
The essential oil blend (EO) formulated for direct ruminant consumption caused a 1% increase on CH4 emissions and resulted in a 1.4% decrease in overall gas production, suggesting negligible influence with the recommended dose of EO on in vitro fermentation over 24 hours. The inclusion of the essential oil blend over 6 and 22-week-long studies in vivo appear to allow the essential oils anti-methanogenic properties to unfold by inducing a 14.7% and 6% decrease in CH4 g/day with its recommended dose, respectively (Castro-Montoya et al., 2015; Hart et al., 2019). An in vitro system with continuous culture capabilities such as the rumen simulation technique (RUSITEC) used in a recent study by Foggi et al. (2024), may be more suited for EO treatment investigation to reveal the ruminal changes in metabolite concentrations and abundance of bacteria that occur when the essential oil blend is applied for extended periods.
Supplementation with Yucca slightly (0.8%) increased total gas production compared to the control benchmark value – the only feed additive to do so in this study. The adequacy of Yucca extracts in maintaining overall gas levels and increasing total VFA concentration compared to the control (22.6%) in this study reinforce the proposed ability of saponins to enhance the growth of specific rumen microbes associated with aiding digestion (Goel et al., 2008; Wang et al., 2000). Furthermore, investigated cultures in the study by Wang et al. (2000) showed a sustained higher optical density at 14 and 24 hours during in vitro incubation when yucca extract was included. This implies a flourishing population of rumen microbes through increased metabolism of complex carbohydrates which is supported by improved total gas and VFA production with the current in vitro Yucca supplementation. The Yucca extract caused a decrease of CH4 emissions by 28.2% which may be explained by linkages to protozoa and methanogen reduction with saponin supplementation (Wina et al., 2005; Goel et al., 2008). While the preservation of total gas production suggests the absence of an impact on in vitro ruminal fermentation, the present dosage of Yucca necessitates additional in vivo validation for beneficial microbial community proliferation and the described CH4 mitigating effect that was not observed when the plant extract was fed at a similar dose in vivo (Holtshausen et al., 2009). In alignment with our findings, numerous authors have consistently reported reductions ranging from 22% to 27% in CH4 concentration in vitro through the supplementation of saponins (Anele et al., 2022; Hess et al., 2003; Hu et al., 2005). These findings reinforce the effectiveness of the current in vitro rumen simulation system to accurately replicate rumen fermentation and supplement effects. Simultaneously, it emphasizes the need for additional research on Yucca supplements for CH4 mitigation in live animals.
Trends towards significant CH4 reduction with ALL further support garlic’s antimicrobial activity. Results from this 24-hour batch fermentation study indicate that rumen microbiome modifiers and hydrogen-sequestering bioactive compounds are the most effective CH4 mitigators. However, it is worth noting that the enzyme-inhibiting compound bromoform, found in A. taxi and the rumen modifying saponins in Yucca as well as the essential oil mixture (EO) may demonstrate greater efficiency in extended fermentation cycles. This study aligns with the imperative to achieve greenhouse gas reduction targets, as outlined in the Global Methane Pledge launched at COP 26 in November 2021 in Glasgow to reduce global methane emissions by at least 30% from 2020 levels by 2030 (UN Climate Change Conference UK, 2021). In accordance with these objectives, this high throughput in vitro system has demonstrated its capability to accurately replicate rumen conditions allowing for the swift identification of treatments with CH4 mitigating potential that enhance rumen productivity. The development of in vitro screening systems offers a cost-effective and ethically responsible approach to evaluating CH4 reduction strategies before in vivo testing, providing a practical pathway for the livestock industry to achieve its methane reduction targets. Limitations associated with this set-up include the absence of host palatability influence for higher GO dosages, the short duration of in vitro screening fermentations in comparison to RUSITEC fermentations, and the accompanying costs associated with routine feeding of higher dose supplements to animals. Nevertheless, a potential effective strategy for mitigating enteric CH4 could involve combining lower GO concentrations with rumen buffers or sole supplementation of rumen buffers fortified with magnesium oxide.
5 Conclusion
The in vitro fermentation analysis of potential rumen feed additives with CH4 mitigating capabilities revealed mechanisms that either enhanced or inhibited rumen metabolism. These variations were supported by noticeable fluctuations in gas emissions as well as distinct comparative patterns in concentrations of total VFAs. Supplementation of rumen modifying garlic derivatives GO and GP, as well as hydrogen sequestering rumen buffers CMA and CMA.MgO induced CH4 reductions that were backed up by ruminal VFA fluctuations indicative of rumen functionality and trends towards increased total VFA concentrations. Conducting additional in vitro research with CMA and CMA.MgO rumen buffers added to rumen fluid at lower pH ranges may elucidate the acetic acid to propanoic acid ratios linked to the inhibited methanogenesis observed in this study. Combining the antimicrobial garlic derivatives at lower concentrations with the hydrogen sink rumen buffers implied synergistic effects with significant CH4 mitigation and improved VFA profiles at concentrations that are suitable for in vivo investigations.
Data availability statement
The raw data supporting the conclusions of this article will be made available by the authors, without undue reservation.
Ethics statement
The animal study was approved by Animal Welfare Body (AWB) at Munster Technological University (MTU), Kerry, Ireland. The study was conducted in accordance with the local legislation and institutional requirements.
Author contributions
IH: Writing – original draft, Formal analysis, Investigation. PQ: Writing – review & editing, Conceptualization, Methodology, Supervision. MA: Writing – review & editing, Methodology. SO: Writing – review & editing, Conceptualization, Methodology, Supervision.
Funding
The author(s) declare that financial support was received for the research and/or publication of this article. This research received funding support from Enterprise Ireland as part of the company research fund scheme under grant number 164475.
Acknowledgments
We thank the Quality and Procurement teams at ABP Food Group Bandon for their assistance in providing rumen fluid used in this study.
Conflict of interest
Authors MA and SC were employed by the company Marigot Ltd.
The remaining authors declare that the research was conducted in the absence of any commercial or financial relationships that could be construed as a potential conflict of interest.
The authors declare that this study received funding from Marigot Ltd. The funder had the following involvement in the study: Selection of natural bioactives for screening in the study, and review of the drafted article.
Generative AI statement
The author(s) declare that no Generative AI was used in the creation of this manuscript.
Publisher’s note
All claims expressed in this article are solely those of the authors and do not necessarily represent those of their affiliated organizations, or those of the publisher, the editors and the reviewers. Any product that may be evaluated in this article, or claim that may be made by its manufacturer, is not guaranteed or endorsed by the publisher.
Supplementary material
The Supplementary Material for this article can be found online at: https://www.frontiersin.org/articles/10.3389/fanim.2025.1546486/full#supplementary-material
References
Abeni F., Galli A. (2017). Monitoring cow activity and rumination time for an early detection of heat stress in dairy cow. Int. J. Biometeorol. 61, 417–425. doi: 10.1007/S00484-016-1222-Z/TABLES/3
Ahmed E., Fukuma N., Hanada M., Nishida T. (2021). The efficacy of plant-based bioactives supplementation to different proportion of concentrate diets on methane production and rumen fermentation characteristics in vitro. Animals 11. doi: 10.3390/ani11041029
Alvarez-Hess P. S., Jacobs J. L., Kinley R. D., Roque B. M., Neachtain A. S. O., Chandra S., et al. (2023). Twice daily feeding of canola oil steeped with Asparagopsis armata reduced methane emissions of lactating dairy cows. Anim. Feed Sci. Technol. 297, 115579. doi: 10.1016/j.anifeedsci.2023.115579
Andersen S. O., Davidson O., Kuijpers L., Metz B. (2005). Safeguarding the Ozone Layer and the Global Climate System. (Intergovernmental Panel on Climate Change/Technological and Economic Assessment Panel Special Report). Available online at: https://www.ipcc.ch/report/safeguarding-the-ozone-layer-and-the-global-climate-system/.
Anele U. Y., Crumel X., Olagunju L., Compart D. P. (2022). Effects of yucca schidigera based feed additive on in vitro dry matter digestibility, efficiency of microbial production, and greenhouse gas emissions of four dairy diets. Dairy 3, 326–332. doi: 10.3390/dairy3020025
Arndt C., Hristov A. N., Price W. J., McClelland S. C., Pelaez A. M., Cueva S. F., et al. (2022). Full adoption of the most effective strategies to mitigate methane emissions by ruminants can help meet the 1.5°C target by 2030 but not 2050. Proc. Natl. Acad. Sci. U. S. A. 119, e2111294119. doi: 10.1073/PNAS.2111294119/SUPPL_FILE/PNAS.2111294119.SD01.XLSX
Bergman E. N. (1990). “Energy contributions of volatile fatty acids from the gastrointestinal tract in various species,” in Physiological Reviews, American Physiological Society. vol. 70. (American Physiological Society), 567–590. doi: 10.1152/physrev.1990.70.2.567
Bord Bia (2020). Grass Fed Beef Standard. Available online at: https://www.bordbia.ie/globalassets/bordbia2020/farmers--growers/grass-fed-standard/grass-fed-beef-standard-revision-02-draft1.pdf (Accessed February 27, 2023).
Brooke C. G., Roque B. M., Shaw C., Najafi N., Gonzalez M., Pfefferlen A., et al. (2020). Methane reduction potential of two pacific coast macroalgae during in vitro ruminant fermentation. Front. Marine Sci. 7. doi: 10.3389/fmars.2020.00561
Burt S. (2004). “Essential oils: Their antibacterial properties and potential applications in foods - A review,” in International Journal of Food Microbiology, vol. 94. (Elsevier), 223–253. doi: 10.1016/j.ijfoodmicro.2004.03.022
Busquet M., Calsamiglia S., Ferret A., Carro M. D., Kamel C. (2005). Effect of garlic oil and four of its compounds on rumen microbial fermentation. J. Dairy Sci. 88, 4393–4404. doi: 10.3168/jds.S0022-0302(05)73126-X
Busquet M., Calsamiglia S., Ferret A., Kamel C. (2006). Plant extracts affect in vitro rumen microbial fermentation. J. Dairy Sci. 89, 761–771. doi: 10.3168/jds.S0022-0302(06)72137-3<au>
Campanile G., Zicarelli F., Vecchio D., Pacelli C., Neglia G., Balestrieri A., et al. (2008). Effects of Saccharomyces cerevisiae on in vivo organic matter digestibility and milk yield in buffalo cows. Livestock Sci. 114, 358–361. doi: 10.1016/j.livsci.2007.11.002
Castro-Montoya J., Peiren N., Cone J. W., Zweifel B., Fievez V., De Campeneere S. (2015). In vivo and in vitro effects of a blend of essential oils on rumen methane mitigation. Livestock Sci. 180, 134–142. doi: 10.1016/j.livsci.2015.08.010
Coleman J., Berry D. P., Pierce K. M., Brennan A., Horan B. (2010). Dry matter intake and feed efficiency profiles of 3 genotypes of Holstein-Friesian within pasture-based systems of milk production. J. Dairy Sci. 93, 4318–4331. doi: 10.3168/jds.2009-2686
Cone J. W. (1998). The development, use and application of the gas production technique at the DLO Institute for Animal Science and Health (ID-DLO), Lelystad, The Netherlands. BSAP Occasional Publ. 22, 65–78. doi: 10.1017/s0263967x00032286
Cottyn B. G., Boucque C. V. (1968). Rapid method for the gas-chromatographic determination of volatile fatty acids in rumen fluid. J. Agric. Food Chem. 16, 105–107. doi: 10.1021/JF60155A002
Cruywagen C. W., Swiegers J. P., Taylor S. J., Coetzee E. (2004). The effect of Acid Buf in dairy cow diets on production response and rumen parameters. J. Anim. Sci. 87. Available online at: https://www.researchgate.net/publication/284260848_The_effect_of_Acid_Buf_in_dairy_cow_diets_on_production_response_and_rumen_parameters.
Cruywagen C. W., Taylor S., Beya M. M., Calitz T. (2015). The effect of buffering dairy cow diets with limestone, calcareous marine algae, or sodium bicarbonate on ruminal pH profiles, production responses, and rumen fermentation. J. Dairy Sci. 98, 5506–5514. doi: 10.3168/jds.2014-8875
Dijkstra J., Ellis J. L., Kebreab E., Strathe A. B., López S., France J., et al. (2012). Ruminal pH regulation and nutritional consequences of low pH. Anim. Feed Sci. Technol. 172, 22–33. doi: 10.1016/j.anifeedsci.2011.12.005
Duin E. C., Wagner T., Shima S., Prakash D., Cronin B., Yáñez-Ruiz D. R., et al. (2016). Mode of action uncovered for the specific reduction of methane emissions from ruminants by the small molecule 3-nitrooxypropanol. Proc. Natl. Acad. Sci. U. S. A. 113, 6172–6177. doi: 10.1073/pnas.1600298113
Durmic Z., Moate P. J., Eckard R., Revell D. K., Williams R., Vercoe P. E. (2014). In vitro screening of selected feed additives, plant essential oils and plant extracts for rumen methane mitigation. J. Sci. Food Agric. 94 (6), 1191–1196. doi: 10.1002/JSFA.6396
Ebel H. (1980). Magnesium metabolism: A review. J. Clin. Chem. Clin. Biochem. 18, 257–270. doi: 10.1515/cclm.1980.18.5.257
Feng X. Y., Dijkstra J., Bannink A., van Gastelen S., France J., Kebreab E. (2020). Antimethanogenic effects of nitrate supplementation in cattle: A meta-analysis. J. Dairy Sci. 103, 11375–11385. doi: 10.3168/jds.2020-18541
Foggi G., Terranova M., Daghio M., Amelchanka S. L., Conte G., Ineichen S., et al. (2024). Evaluation of ruminal methane and ammonia formation and microbiota composition as affected by supplements based on mixtures of tannins and essential oils using Rusitec. J. Anim. Sci. Biotechnol. 15, 1–16. doi: 10.1186/S40104-024-01005-8
Goel G., Makkar H. P. S., Becker K. (2008). Changes in microbial community structure, methanogenesis and rumen fermentation in response to saponin-rich fractions from different plant materials. J. Appl. Microbiol. 105, 770–777. doi: 10.1111/J.1365-2672.2008.03818.X
Guan H., Wittenberg K. M., Ominski K. H., Krause D. O. (2006). Efficacy of ionophores in cattle diets for mitigation of enteric methane. J. Anim. Sci. 84, 1896–1906. doi: 10.2527/JAS.2005-652
Hart K. J., Jones H. G., Waddams K. E., Worgan H. J., Zweifel B., Newbold C. J. (2019). An essential oil blend decreases methane emissions and increases milk yield in dairy cows. Open J. Anim. Sci. 09, 259–267. doi: 10.4236/ojas.2019.93022
Hess H. D., Kreuzer M., Díaz T. E., Lascano C. E., Carulla J. E., Soliva C. R., et al. (2003). Saponin rich tropical fruits affect fermentation and methanogenesis in faunated and defaunated rumen fluid. Anim. Feed Sci. Technol. 109, 79–94. doi: 10.1016/S0377-8401(03)00212-8
Hodge I., Quille P., O’Connell S. (2024). A review of potential feed additives intended for carbon footprint reduction through methane abatement in dairy cattle. Animals 14, 568. doi: 10.3390/ANI14040568
Holtshausen L., Chaves A. V., Beauchemin K. A., McGinn S. M., McAllister T. A., Odongo N. E., et al. (2009). Feeding saponin-containing Yucca schidigera and Quillaja saponaria to decrease enteric methane production in dairy cows. J. Dairy Sci. 92, 2809–2821. doi: 10.3168/jds.2008-1843
Honan M., Feng X., Tricarico J. M., Kebreab E. (2022). Feed additives as a strategic approach to reduce enteric methane production in cattle: modes of action, effectiveness and safety. Anim. Product Sci. 62, 1303–1317. doi: 10.1071/AN20295
Hristov A. N. (2024). Invited review: Advances in nutrition and feed additives to mitigate enteric methane emissions. J. Dairy Sci. 107, 4129–4146. doi: 10.3168/JDS.2023-24440
Hu W.-L., Liu J.-X., Ye J.-A., Wu Y.-M., Guo Y.-Q. (2005). Effect of tea saponin on rumen fermentation in vitro. Anim. Feed Sci. Technol. 120, 333–339. doi: 10.1016/j.anifeedsci.2005.02.029
Huang S., Ji S., Suen G., Wang F., Li S. (2021). The rumen bacterial community in dairy cows is correlated to production traits during freshening period. Front. Microbiol. 12. doi: 10.3389/fmicb.2021.630605
Iannotti E. L., Kafkewitz D., Wolin M. J., Bryant M. P. (1973). Glucose fermentation products of Ruminococcus albus grown in continuous culture with Vibrio succinogenes: changes caused by interspecies transfer of H2. J. Bacteriol. 114, 1231–1240. doi: 10.1128/jb.114.3.1231-1240.1973
Khurana R., Brand T., Tapio I., Bayat A. R. (2023). Effect of a garlic and citrus extract supplement on performance, rumen fermentation, methane production, and rumen microbiome of dairy cows. J. Dairy Sci. 106, 4608–4621. doi: 10.3168/jds.2022-22838
Kirwan S. F., Tamassia L. F. M., Walker N. D., Karagiannis A., Kindermann M., Waters S. M. (2024). Effects of dietary supplementation with 3-nitrooxypropanol on enteric methane production, rumen fermentation, and performance in young growing beef cattle offered a 50:50 forage:concentrate diet. J. Anim. Sci. 102. doi: 10.1093/jas/skad399
Kim E. T., Kim C. H., Min K. S., Lee S. S. (2012). Effects of plant extracts on microbial population, methane emission and ruminal fermentation characteristics in in vitro. Asian-Australasian J. Anim. Sci. 25, 806–811. doi: 10.5713/ajas.2011.11447
Kinley R. D., Martinez-Fernandez G., Matthews M. K., de Nys R., Magnusson M., Tomkins N. W., et al. (2020). Mitigating the carbon footprint and improving productivity of ruminant livestock agriculture using a red seaweed. J. Clean. Prod. 259. doi: 10.1016/j.jclepro.2020.120836
Klop G., Dijkstra J., Dieho K., Hendriks W. H., Bannink A. (2017). Enteric methane production in lactating dairy cows with continuous feeding of essential oils or rotational feeding of essential oils and lauric acid. J. Dairy Sci. 100, 3563–3575. doi: 10.3168/jds.2016-12033
Krause K. M., Oetzel G. R. (2006). “Understanding and preventing subacute ruminal acidosis in dairy herds: A review,” in Animal Feed Science and Technology, vol. 126. (Animal Feed Science and Technology), 215–236. doi: 10.1016/j.anifeedsci.2005.08.004
Lee S.-R., Cho Y., Ju H. K., Kim E. (2021). Theoretical methane emission estimation from volatile fatty acids in bovine rumen fluid. Appl. Sci. 11, 7730. doi: 10.3390/app11167730
Luo C., Cai S., Jia L., Tang X., Zhang R., Jia G., et al. (2015). Study on accurate determination of volatile fatty acids in rumen fluid by capillary gas chromatography. Proc. 5th Int. Conf. Inf. Eng. Mech Mater 21, 386–391. doi: 10.2991/icimm-15.2015.73
Machado L., Magnusson M., Paul N. A., Kinley R., de Nys R., Tomkins N. (2016). Identification of bioactives from the red seaweed Asparagopsis taxiformis that promote antimethanogenic activity in vitro. J. Appl. Phycol 28, 3117–3126. doi: 10.1007/s10811-016-0830-7
Masson-Delmotte V., Zhai P., Pörtner H.-O., Roberts D., Skea J., Shukla P. R., et al. (2018). Global warming of 1.5°C. An IPCC Special Report on the impacts of global warming of 1.5°C above pre-industrial levels and related global greenhouse gas emission pathways, in the context of strengthening the global response to the threat of climate change. Ipcc - Sr15 2, 17–20. Available online at: www.environmentalgraphiti.org (Accessed March 20, 2023).
McIntosh F. M., Williams P., Losa R., Wallace R. J., Beever D. A., Newbold C. J. (2003). Effects of essential oils on ruminal microorganisms and their protein metabolism. Appl. Environ. Microbiol. 69, 5011–5014. doi: 10.1128/AEM.69.8.5011-5014.2003
Miller G. A., Bowen J. M., Dewhurst R. J., Zweifel B., Spengler K., Duthie C. A. (2023). Enteric methane emissions from dairy–beef steers supplemented with the essential oil blend agolin ruminant. Animals 13, 1826. doi: 10.3390/ANI13111826
Morgante M., Stelletta C., Berzaghi P., Gianesella M., Andrighetto I. (2007). Subacute rumen acidosis in lactating cows: An investigation in intensive Italian dairy herds. J. Anim. Physiol. Anim. Nutr. 91, 226–234. doi: 10.1111/j.1439-0396.2007.00696.x
Neville E. W., Fahey A. G., Gath V. P., Molloy B. P., Taylor S. J., Mulligan F. J. (2019). The effect of calcareous marine algae, with or without marine magnesium oxide, and sodium bicarbonate on rumen pH and milk production in mid-lactation dairy cows. J. Dairy Sci. 102, 8027–8039. doi: 10.3168/jds.2019-16244
Nolan J. V., Godwin I. R., De Raphélis-Soissan V., Hegarty R. S. (2016). “Managing the rumen to limit the incidence and severity of nitrite poisoning in nitrate-supplemented ruminants,” in Animal production science, vol. 56. (CSIRO), 1317–1329. doi: 10.1071/AN15324
Olijhoek D. W., Hellwing A. L. F., Brask M., Weisbjerg M. R., Højberg O., Larsen M. K., et al. (2016). Effect of dietary nitrate level on enteric methane production, hydrogen emission, rumen fermentation, and nutrient digestibility in dairy cows. J. Dairy Sci. 99, 6191–6205. doi: 10.3168/jds.2015-10691
Patra A. K., Yu Z. (2012). Effects of essential oils on methane production and fermentation by, and abundance and diversity of, rumen microbial populations. Appl. Environ. Microbiol. 78, 4271–4280. doi: 10.1128/AEM.00309-12
Poblete J. B., Angeles A. A., Agbisit E. M., Espaldon M. V. O. (2020). Response of growing dairy bulls to dietary tannin in rations with varying energy levels. Trop. Anim. Sci. J March. 43, 50–56. doi: 10.5398/tasj.2020.43.1.50
Rahman M. S. (2007). Allicin and other functional active components in garlic: Health benefits and bioavailability. Int. J. Food Proper 10, 245–268. doi: 10.1080/10942910601113327
Roque B. M., Salwen J. K., Kinley R., Kebreab E. (2019). Inclusion of Asparagopsis armata in lactating dairy cows’ diet reduces enteric methane emission by over 50 percent. J. Clean. Prod. 234, 132–138. doi: 10.1016/j.jclepro.2019.06.193
Roque B. M., Venegas M., Kinley R. D., De Nys R., Duarte T. L., Yang X., et al. (2021). Red seaweed (Asparagopsis taxiformis) supplementation reduces enteric methane by over 80 percent in beef steers. PLoS One 16, 1–20. doi: 10.1371/journal.pone.0247820
Rossi C. A. S., Compiani R., Baldi G., Taylor S. J., Righi F., Simoni M., et al. (2019). Replacing sodium bicarbonate with half amount of calcareous marine algae in the diet of beef cattle. Rev. Bras. Zootecnia 48. doi: 10.1590/rbz4820180129
Russell J. B. (1998). The importance of pH in the regulation of ruminal acetate to propionate ratio and methane production in vitro. J. Dairy Sci. 81, 3222–3230. doi: 10.3168/jds.S0022-0302(98)75886-2
Sari N. F., Ray P., Rymer C., Kliem K. E., Stergiadis S. (2022). “Garlic and its bioactive compounds: implications for methane emissions and ruminant nutrition,” in Animals, vol. 12. (Multidisciplinary Digital Publishing Institute: Animals), 2998. doi: 10.3390/ani12212998
Sarker N. C., Keomanivong F., Borhan M., Rahman S., Swanson K. (2018). In vitro evaluation of nano zinc oxide (nZnO) on mitigation of gaseous emissions. J. Anim. Sci. Technol. 60, 27. doi: 10.1186/s40781-018-0185-5
Seshadri R., Leahy S. C., Attwood G. T., Teh K. H., Lambie S. C., Cookson A. L., et al. (2018). “Cultivation and sequencing of rumen microbiome members from the Hungate1000 Collection,” in Nature Biotechnology, vol. 36. (Nature Biotechnology: Nature Publishing Group), 359–367. doi: 10.1038/nbt.4110
Ternouth J. H. (1968). The rumen and its microbes. Aust. Veter J. 44, 47–47. doi: 10.1111/j.1751-0813.1968.tb04952.x
Tong J.j., Zhang H., Wang J., Liu Y., Mao S.y., Xiong B.h., et al. (2020). Effects of different molecular weights of chitosan on methane production and bacterial community structure in vitro. J. Integr. Agric. 19, 1644–1655. doi: 10.1016/S2095-3119(20)63174-4
UN Climate Change Conference UK (2021). “Cop26: the Glasgow climate pact,” in Cop26: The Glasgow Climate Pact, (United Nations), 28. Available online at: https://www.un.org/en/climatechange/cop26
Ungerfeld E. M. (2015). Shifts in metabolic hydrogen sinks in the methanogenesis-inhibited ruminal fermentation: A meta-analysis. Front. Microbiol. 6. doi: 10.3389/fmicb.2015.00037
Van Zijderveld S. M., Gerrits W. J. J., Dijkstra J., Newbold J. R., Hulshof R. B. A., Perdok H. B. (2011). Persistency of methane mitigation by dietary nitrate supplementation in dairy cows. J. Dairy Sci. 94, 4028–4038. doi: 10.3168/jds.2011-4236
Wang Y., McAllister T. A., Yanke L. J., Cheeke P. R. (2000). Effect of steroidal saponin from Yucca schidigera extract on ruminal microbes. J. Appl. Microbiol. 88, 887–896. doi: 10.1046/j.1365-2672.2000.01054.x
Wang R., Wang M., Ungerfeld E. M., Min Zhang X., Lei Long D., Xiang Mao H., et al. (2018). Nitrate improves ammonia incorporation into rumen microbial protein in lactating dairy cows fed a low-protein diet. J. Dairy Sci. 101 (11), 9789–9799. doi: 10.3168/jds.2018-14904
Wang M., Wang R., Zhang X., Ungerfeld E. M., Long D., Mao H., et al. (2017). Molecular hydrogen generated by elemental magnesium supplementation alters rumen fermentation and microbiota in goats. Br J Nutr. 118 (6), 401–410. doi: 10.1017/S0007114517002161
Wasson D. E., Stefenoni H., Cueva S. F., Lage C., Räisänen S. E., Melgar A., et al. (2023). Screening macroalgae for mitigation of enteric methane in vitro. Sci. Rep. 13, 1–15. doi: 10.1038/s41598-023-36359-y
Wina E., Muetzel S., Hoffmann E., Makkar H. P. S., Becker K. (2005). Saponins containing methanol extract of Sapindus rarak affect microbial fermentation, microbial activity and microbial community structure in vitro. Anim. Feed Sci. Technol. 121, 159–174. doi: 10.1016/J.ANIFEEDSCI.2005.02.016
Keywords: CMA, essential oils, asparagopsis, methane reduction, enzyme inhibitor, microbiome modifying, buffering, Ankom
Citation: Hodge I, Quille P, Ayyachamy M and O'Connell S (2025) In vitro comparison of naturally bioactive plant extracts, essential oils, and marine algae targeting different modes of action for mitigation of enteric methane emissions in ruminants. Front. Anim. Sci. 6:1546486. doi: 10.3389/fanim.2025.1546486
Received: 17 December 2024; Accepted: 10 April 2025;
Published: 07 May 2025.
Edited by:
Yosra Ahmed Soltan, Alexandria University, EgyptReviewed by:
Ravikanthreddy Poonooru, University of Missouri, United StatesRittikeard Prachumchai, Rajamangala University of Technology Thanyaburi, Thailand
Copyright © 2025 Hodge, Quille, Ayyachamy and O'Connell. This is an open-access article distributed under the terms of the Creative Commons Attribution License (CC BY). The use, distribution or reproduction in other forums is permitted, provided the original author(s) and the copyright owner(s) are credited and that the original publication in this journal is cited, in accordance with accepted academic practice. No use, distribution or reproduction is permitted which does not comply with these terms.
*Correspondence: Ian Hodge, aWFuaG9kZ2UyNTVAZ21haWwuY29t