- 1Department of Animal, Veterinary and Food Sciences, University of Idaho, Moscow, ID, United States
- 2Department of Dairy and Food Sciences, South Dakota State University, Brookings, SD, United States
- 3Department of Animal Science, University of Connecticut, Storrs, CT, United States
Introduction: Health and performance of dairy calves can be influenced by the intra-uterine environment. Environmental stressors such as heat stress, maternal inflammatory status, and nutrient deficiencies have been shown to impair intra-uterine and postnatal development in dairy cattle, with implications for long-term productivity. Recent studies in primates have reported lower birth weights and altered infant inflammatory markers associated with maternal exposure to wildfire smoke particulate matter (wildfire-PM2.5) during pregnancy. However, the impact of intra-uterine exposure to wildfire-PM2.5 on dairy calf growth and health is unknown.
Methods: Holstein heifer calves were born to cows exposed (WFS, n = 17) or unexposed (CON, n = 26) to wildfire smoke during mid gestation. Calves were monitored from birth through 6 months of life to evaluate growth patterns, health, and basal metabolic and inflammatory markers.
Results: Although there was no difference detected between groups in gestation length or calf frame size at birth, WFS calves had lower birth weights compared with that of CON (36.87 ± 0.87 kg vs. 40.82 ± 0.80 kg). WFS calves had greater average daily gain in the pre-weaning period but lower gain in the post-weaning period compared with CON. WFS calves also had greater plasma glucose concentrations in the pre-weaning period, lower β-hydroxybutyrate concentrations in the early post-weaning period, and greater non-esterified fatty acid concentrations for much of the pre-weaning and the early post-weaning period relative to those for CON calves. In addition, WFS calves had lower white blood cell counts throughout the preweaning period, coupled with greater serum albumin and lower serum amyloid A through the study, as well as lower haptoglobin concentrations at approximately 3 months of age. All calves received adequate passive transfer of immunity, but WFS calves had greater serum IgG concentrations, yet no difference was detected in apparent efficiency of IgG absorption compared with that for CON. WFS calves had lower odds of lung consolidation throughout the study.
Discussion: Overall, these results indicate that prenatal exposure to wildfire particulates restricts fetal growth and programs postnatal calf metabolic and immune systems, which may have implications for future health and productivity.
1 Introduction
Throughout the past century, changes in climate and historical fire suppression have fueled changes in wildfire activity such as increased wildfire burned area and severity (Harris and Taylor, 2015; Littell et al., 2009), and this trend is predicted to continue in future decades (Abatzoglou and Williams, 2016). Wildfires have repercussions in locations well beyond their vicinity (Burke et al., 2021) as they expel large quantities of combustion pollutants comprised of noxious gases and particulates, which threaten human and animal health (Black et al., 2017; Chen et al., 2021; Dickinson et al., 2022; Heaney et al., 2022; Pace et al., 2023). Cattle are particularly susceptible to pulmonary pathologies because of their respiratory anatomy (Ackermann et al., 2010), which also may make them prone to morbidities and mortalities related to airborne particulates (Cox et al., 2016; Beaupied et al., 2021; Egberts et al., 2019; van Leenen et al., 2021). Indeed, we reported that wildfire smoke-derived fine particulate matter (wildfire-PM2.5) is linked to altered inflammatory markers, metabolism, and respiratory irritation in young calves (Pace et al., 2023), as well as inflammation, altered metabolism, and reduced milk production in lactating cows (Anderson et al., 2022). Several top-producing states in dairy production are also frequently affected annually by wildfires (National Interagency Coordination Center (NICC), 2022; USDA National Agricultural Statistics Service, 2022), which further underscores the growing need to reduce impacts of wildfire emissions on the dairy industry.
Wildfire-PM2.5 is considered one of the most detrimental components of wildfire smoke, owing in part to its small diameter (< 2.5µm). Upon inhalation, aerosolized particulates may be deposited throughout the airways and pass through the alveoli to the bloodstream, where they can enter extra-pulmonary tissues (Li et al., 2019). These interactions and resulting inflammatory responses are thought to facilitate direct and indirect wildfire-PM2.5 impacts within the lung and other organ systems. Many studies have documented negative health effects of wildfire-PM2.5 inhalation on both mature and immature humans and animals, including, but not limited to, increased risk of mortality (Ma et al., 2024), respiratory infections (Tinling et al., 2016), epigenetic modifications (Brown et al., 2022; Xu et al., 2023), and cardiovascular and respiratory decrements (Chen et al., 2021).
The theory of the Developmental Origins of Health and Disease (DOHaD) (Barker and Osmond, 1986; Barker, 1990; Barker, 2007) states that the prenatal environment has long-term consequences on postnatal development and predisposition to disease. A few recent studies have reported that exposure to wildfire-PM2.5 during pregnancy may affect fetal development and program postnatal phenotype (Capitanio et al., 2022; Abdo et al., 2019; Breton et al., 2011; Holstius et al., 2012; O’Donnell and Behie, 2013; Yue et al., 2020; Lan et al., 2024). Several investigators have linked reduced birth weights in human newborns to wildfire-PM2.5 exposure in utero (Abdo et al., 2019; Breton et al., 2011; Holstius et al., 2012; O’Donnell and Behie, 2013). Furthermore, mice whose mothers aspirated ambient PM2.5 while pregnant showed evidence of altered lung development (Yue et al., 2020) Wildfire-PM2.5-induced alterations in prenatal lung development may increase risk of respiratory infections during postnatal life (Lan et al., 2024).
Although it is known that neonatal and adult dairy cattle are impacted by wildfire-PM2.5, the effects of prenatal exposure to wildfire-PM2.5 on calves postnatally are unknown. The aim of the present study was to investigate the effects of natural prenatal wildfire-PM2.5 exposure on postnatal calf growth, health, nutrient metabolism, and inflammatory status. We hypothesized that mid-gestation wildfire-PM2.5 exposure would result in immunodepression, compromised health, growth restriction, and metabolic deficiencies in the postnatal calf.
2 Materials and methods
All procedures were conducted according to the University of Idaho Institutional Animal Care and Use Committee protocol #IACUC-2022-46. Two groups of Holstein cows were either exposed (0.88 ± 1.15 lactations) or unexposed (1.12 ± 0.95 lactations) to naturally-occurring wildfire-PM2.5 in mid-gestation. Therefore, Holstein heifer calves were either born to dams exposed to wildfire-PM2.5 in mid-gestation (WFS, n = 17) or unexposed for the entirety of the gestational period (CON, n = 26). The calves were followed from birth (week 0) to 6 months of age (week 24). Postnatal calf management was matched between the two groups (see Postnatal Calf Management). Cows were from two geographically distinct farms in order to observe the effects of natural wildfire-PM2.5 exposure and the calves remained at the same location as their dams for the duration of the study to avoid transportation effects. Wildfire-PM2.5 exposed cows and WFS calves were housed at the University of Idaho Dairy Center in Moscow, ID, while cows that were not exposed to wildfire-PM2.5 during gestation, as well as CON calves, were housed at a private farm near Sioux Falls, SD. These farms were selected based on 1) historical air quality measurements in both locations, as air quality is typically affected by wildfire smoke in Moscow, ID annually, whereas Sioux Falls, SD is less frequently affected by wildfire smoke, 2) similarity of seasonal patterns for temperature-humidity index (THI) between both locations, and 3) ability to match management protocols of calves on both farms.
Dam management was similar between the two farms throughout gestation. During gestation, dams were fed a total mixed ration formulated according to the National Academies of Sciences, Engineering, and Medicine (NASEM) Nutrient Requirements of Dairy Cattle (NRC). Lactating cows were dried off 60 d prior to the expected calving date and fed a dry cow ration.
2.1 Environmental conditions
Air quality and climate conditions were monitored for both farms. PM2.5 concentrations (µg/m3) were retrieved from the U.S. EPA Air Quality System database tool (United States Environmental Protection Agency, 2024). Heightened PM2.5 concentrations were confirmed to be derived from active wildfires using AirNow-Tech Navigator and the National Oceanic and Atmospheric Administration’s (NOAA) HSYPLIT atmospheric transport and dispersion modeling, which considers air mass trajectories tracked 72 h back to their source at three atmospheric heights: 50, 100, and 150 m (Draxler and Hess, 1997, 1998; Draxler, 1999; Stein et al., 2015). Thus, users can visualize the origin of air masses overlaid with active wildfires, to determine if air masses were wildfire-derived. Exposure to wildfire-PM2.5 was determined using the following criteria, as previously described by our group (Anderson et al., 2022; Pace et al., 2023; Pace et al., 2024): 1) daily average PM2.5 concentrations surpassed 35µg/m3, and 2) PM2.5 was confirmed to have originated from active wildfires using HYSPLIT modeling. A wildfire event in Idaho resulted in exposure to elevated PM2.5 concentrations for cows several days between 148 to 158 days in gestation (Figure 1).
Climate conditions for both locations were retrieved from NOAA’s Local Climatology, Version 2 datasets (https://www.ncei.noaa.gov/access/search/data-search/local-climatological-data-v2). Weekly temperature and relative humidity data were used to calculate weekly temperature–humidity index (THI) according to the following equation (Dikmen 2008):
2.2 Postnatal calf management
All calves were born in January of 2023 without difficulty and thus required no human intervention in the parturition process. The calves were all singleton heifers, except for one set of heifer twins in the CON group. Calf conditions and management (e.g., nutrition, weaning protocol, disbudding) were matched between the two farms. Heifer calves were fed 3.8 L of colostrum within 1 h of birth, and an additional 1.9 L of colostrum within 12 h of birth. Samples of colostrum fed to each calf were collected at the time of feeding and stored at −20°C for future analyses.
Calves within each group were born within 12 d of each other and were raised as single cohorts within each farm; time of management changes, such as weaning, and sampling dates were based on the average age of each cohort. On average, the CON calves were born approximately 9 days after WFS were born. From birth until 73 days of age (± 2 days), calves were housed in individual hutches. For the remainder of the study, calves within each farm were housed as groups. Although health events were recorded, diagnoses and treatment decisions were made by each farm. Calves identified by farm management as ill were allowed up to three separate treatments prior to being removed from the study. In the CON group, 13 calves were diagnosed with respiratory or enteric illness, and in the WFS group, 8 calves were diagnosed with enteric illnesses. A total of 2 calves were removed from the herd after 3 unsuccessful treatments for respiratory illness, both from the CON group. These data were included in the analyses. No WFS calves were removed from the study.
Nutrition was matched between the two groups. For the preweaning phase, calves were fed a combination of 60% Land O’ Lakes® Amplifier ® Max Protein Blend milk replacer (Land O’ Lakes, Inc., Arden Hills, Mn), 40% waste milk, and supplemented with 0.25 lb Land O’ Lakes® Pasteurized Milk Balancer® (Land O’ Lakes, Inc., Arden Hills, Minnesota) per day. From 1–14 days of age, calves were fed 3.41 L 3X/day, then increased to 3.8 L 3X per day from 15–35 days of age. The first milk stepdown occurred from days 36–45, when 3.3 L of milk was provided 2X per day. The second stepdown was to 3.3L 1X per day from 46–56 days of age, after which calves were fully weaned. Calves had ad libitum access to water and Purina® AMPLI-CALF® Starter 20 calf starter grain (Land O’ Lakes, Inc., Arden Hills, Mn) beginning at three days of age. Following weaning, calves were slowly transitioned from starter grain to a total mixed ration that was formulated according to NASEM guidelines and matched between the two groups from 56 days of age to 105 days of age (Supplementary Table S1).
2.3 Growth and gestation length
Calf body weights and wither and hip heights were measured at birth and 2X per month for the first 6 months of life. Average daily gains (ADG) of these variables were calculated at two periods: 1) from birth to weaning (pre-weaning ADG), and 2) from weaning to 6 months of age (post-weaning ADG). Growth measurements of the twin calves in the CON group were excluded from body measurement and growth analyses as they were outliers. Gestation length was calculated as the number of days between the date the dam was bred and the date of birth for each calf. Sire predicted transmitting ability of gestation length was not different between the two groups (−2.35 ± 0.87 days: vs. CON: −1.88 ± 0.91 days; P = 0.12).
2.4 Blood sampling
Blood was collected immediately following birth (prior to first colostrum feeding), at 48 h, and every other week for the first 6 months of life. For all time points except for the 48 h time point, a total of 20 mL of postprandial whole blood was collected, with 10 mL collected in serum tubes with a clot-activating agent, and 10 mL in ethylenediaminetetraacetic acid (EDTA) tubes. The 48 h blood sample was collected in serum tubes and used for measurement of IgG. EDTA tubes were inverted immediately following collection, and stored on ice until processing, while serum tubes were stored at room temperature for at least 20 minutes to allow for clotting. A portion of whole blood collected in EDTA tubes was used for hematological analyses. The remainder of the whole blood in EDTA tubes, along with clotted blood in serum tubes, were centrifuged at 3,000 × g for 17 minutes at 24°C, after which plasma and serum, respectively, were removed and stored at −20°C for further analysis.
2.5 Blood metabolites
Blood metabolites were assessed at birth and every other week thereafter for the first 6 months following birth. Plasma β-Hydroxybutyrate (BHB) was measured using methods described by Tsai, 2021. In brief, this in-house protocol involves quantification of BHB by measurement of NADH, which is produced in proportion to the concentration of BHB in each sample. The enzyme 3β-dehydrogenase within a buffer (33μg/mL 3β-dehydrogenase (no. 3HBDB-RO), 0.1M Tris, 0.1 M oxalic acid, 2mM EDTA, 2.25 mM NADH) is added to each triplicate sample or calibrator (no. 421-73791, FujiFilm Medical Systems, Lexington, MA), and incubated at 37°C for 1 minute prior to measurement on a Spectramax ID3 spectrophotometer (Molecular Devices, San Jose, California). Measurements of NADH were taken at 0, 1, and 2 minutes at a wavelength of 340 nm, which represented the proportional concentration of BHB in the sample. The change in absorbance per minute was used to calculate the concentration of BHB in the samples.
Commercial kits were used to quantify plasma concentrations of non-esterified fatty acids (NEFA) and glucose. NEFA concentrations were measured in duplicate using the colorimetric Wako HR series NEFA-HR(2) assay (Color reagent A: 999-34691; Solvent A: 995-34791; Color reagent B: 991-34891; Solvent B: 993-35191; NEFA Standard Solution: 276-76491; FUJIFILM Medical Systems), following manufacturer protocols. Glucose concentrations were measured using a microtiter Autokit Glucose assay (Fujifilm Autokit Glucose, no. 99703001), following manufacturer protocols. Intra-assay CVs for BHB, NEFA, and Glucose were 6.64, 5.66, and 5.69% respectively, while inter-assay CVs were 19.89%, 14.08, and 8.2%, respectively.
2.6 IgG and apparent efficiency of absorption
Serum collected at 48 h after birth and colostrum were used to measure concentration of Immunoglobulin-gamma (IgG). For each calf, colostrum fed at the 1st and 2nd feedings were pooled. IgG was measured in duplicate using a Kent Laboratories bovine IgG radial immunodiffusion test kit (no. 728411; Triple J Farms, Bellingham, WA) according to manufacturer protocol. Colostrum samples were diluted 9:1, and 48 h serum samples were diluted between 1:2 to 2:1 for each calf. Inter-assay CV was 8.81% and intra-assay CV was 3.57%. Apparent efficiency of IgG absorption (AEA) was calculated using the following equation from Skibiel et al. (2017):
2.7 Hematology, acute phase proteins, and cortisol
Whole blood was used to determine complete blood counts and differential cell counts at birth and every other week for 6 months following birth. Complete cell counts were assessed for 13 time points, while differential cell counts were assessed for 12 time points, as differential cell counts could not be measured for CON calves at 2 wk of age. For WFS, hematology was analyzed using a VetScan HM5C Hematology System (Abaxis, Union City, CA) according to manufacturer protocols. For CON, hematology was analyzed on an ADVIA 2120i hematology analyzer (Siemens Healthcare Diagnostics, Inc. Tarrytown, NY, USA). Whole blood internal controls were used to calculate CV between machines; only outputs with CV ≤ 20% were used. Output used in this study include total white blood cell concentrations (WBC), and concentrations of lymphocytes (LYMPH), red blood cells (RBC), hemoglobin (HB), and hematocrit (HCT).
To assess differences in cortisol concentrations in the early pre-weaning period, plasma cortisol was measured at birth (prior to colostrum feeding) and at 2 wk of age. Cortisol was measured in duplicate using DetectX® Enzyme Cortisol ImmunoAssay Kits (no. K003-H1W/H5W, Arbor Assays, Ann Arbor, Michigan), according to manufacturer protocols. Samples were either undiluted or diluted 1:100.
Acute phase proteins, serum albumin (SA), serum amyloid A (SAA), and haptoglobin (Hp) were quantified at birth and 1X monthly for the duration of the study. Plasma SAA was measured in duplicate, diluted 1:1,500 or 1:1,750, using a commercial multispecies solid phase sandwich ELISA, according to manufacturer instructions (no. TP-802, Tridelta Development LTD, Kildare, Ireland). Serum albumin was measured in plasma in duplicate with a 1:1 dilution using a commercial kit (no. MAK124; Sigma Aldrich, UK) according to the manufacturer’s protocol. Plasma haptoglobin was analyzed in duplicate, undiluted or diluted 1:1, using an in-house colorimetric assay adapted from Cooke and Arthington (2013). This assay involves measurement of peroxidase activity, which occurs in conjunction with haptoglobin-hemoglobin binding. Briefly, bovine hemoglobin (CAS no. 9008-02-0) was added to samples, facilitating peroxidase activity. A color reagent [O-dianisidine (CAS no. 119-90-4), disodium EDTA (CAS no. 6381-92-6), and sodium phosphate monobasic (CAS no. 10049-21-5)] were added to each sample, then incubated at 37°C for 45 minutes in a water bath. 156 mM Hydrogen Peroxide was then added to facilitate a colorimetric reaction, and absorbance measured at 450 nm. Concentration of haptoglobin was calculated using a standard curve generated using an 8-point serial dilution with a commercial haptoglobin calibrator (no. TP-801-CAL, Tridelta Development, LTD, Kildare, Ireland). For all assays, intra-assay CVs were < 7.81%, while inter-assay CVs were < 18.74%.
2.8 Health and vitals
Vital signs [heart rate (HR) and rectal temperature (RT)] were taken at birth and 1X every 2 wk thereafter. RT was measured using a calf rectal thermometer. HR was assessed using a stethoscope and counting the number of heart beats in one minute. Health scores were recorded at 2 wk of age and 2X per month thereafter using the University of Wisconsin-Madison calf health scorer (McGuirk, 2008), which assigns scores to calves based on severity of coughing, eye discharge, nasal discharge, ear droopiness/head tilt, fecal consistency, attitude, and naval inflammation. These scores range from 0–3, with scores increasing with severity of symptom for each variable.
To assess respiratory health, thoracic ultrasound scores were recorded for each calf 1X per month beginning at 4 wk of age. Thoracic ultrasounds were conducted using an ExaGo veterinary ultrasound scanner with a linear probe (IMV Imaging, Bellshill, United Kingdom). A standardized scoring system (Ollivett and Buczinski, 2016; https://www.vetmed.wisc.edu/fapm/svm-dairy-apps/calfscan-calf-lung-ultrasound-scorer/) was used to assign thoracic ultrasound scores to each lung lobe, identifying lesions including “comet tail” artifacts and lung consolidation, which are commonly observed in calves afflicted with bovine respiratory disease (Ollivett and Buczinski, 2016).These lesions appear when fluid or products of inflammatory processes replace air in normally aerated lung tissue, and, when they reach the pleura, are visible via ultrasonography (Peek et al., 2018). Greater lung consolidation scores are associated with greater severity and extent of lung consolidation. Each lung lobe received a score ranging from 0 to 3, and a cumulative score was assigned based on these scores. A cumulative score of 0 is indicative of no detected consolidation on any lung lobes, while a score of 1 is indicative of severe diffuse comet tails on one or more lobe. A score of 2 indicates lobular consolidation in one or more lung lobe, and a score of 3 indicates lobar consolidation in one or more lobe. Scores of 4 are assigned when two lobes are afflicted with lobar consolidation, while a score of 5 is assigned when three or more lobes exhibit lobar consolidation.
2.9 Statistical analysis
Statistical analyses were conducted in SAS V. 9.4. Residuals were checked for normality using Kolmogorov-Smirnov tests and visual assessment. To detect differences in THI between the two locations, paired t-tests using PROC TTEST was used. Gestation length, IgG concentration, and AEA were assessed with unpaired T-tests using PROC TTEST.
Health and thoracic ultrasound scores were analyzed using generalized linear mixed models with a multinomial distribution and cumulative link function for ordinal data using PROC GLIMMIX. Time (age in wks), group (WFS or CON), and their interaction were fixed effects, and a random intercept was calculated for each individual calf. Group and time increments were represented by dummy variables, and the last time point (12 wks of age) and CON group were used as references for calculating estimates; therefore, estimates are not calculated for the main effect of CON or 12 weeks. To obtain estimates for significant interactive effects, separate models were run for each time point, with treatment alone as a fixed effect, and random effect of calf. Global false discovery rate for global P-value adjustments were applied to account for type I error. The multinomial distribution with a cumulative logit link function of PROC GLIMMIX uses log-likelihood tests to estimate log-odds ratios. The inverse link function of the estimates was applied to provide the odds ratios (OR) as estimates with 95% confidence intervals. Score data are therefore shown as OR, representing the odds of WFS receiving a higher score (more severe symptoms) relative to CON, along with 95% confidence intervals.
Body measurements and ADG, hematological variables, blood metabolites, acute phase proteins, and cortisol concentrations were analyzed using either general (PROC MIXED) or generalized (PROC GLIMMIX) linear mixed modes with a negative binomial distribution for count data. Fixed effects were group, time, and their interaction for all models, except for ADG, in which fixed effects were period (pre-weaning vs post-weaning), group, and their interaction. For PROC MIXED, repeated effects were modeled for time within calf, or period within calf for ADG. For PROC GLIMMIX, random effects were modeled for individual calf, with separate intercepts calculated to control for individual variation among repeated measures. Nonsignificant interactions (P > 0.05) were removed from all final models. If necessary to meet normality assumptions, data were log transformed. In these cases, the data presented are back-transformed least squares means (LSM) with standard error of means (SEM) calculated using the delta method.
3 Results
3.1 Environmental conditions
Wildfire-PM2.5 concentrations were elevated for approximately 2 wk during mid-gestation for WFS dams, peaking at 113.5 µg/m3 at approximately 164 days in gestation (Figure 1B). Within those 2 wk, there were five non-consecutive days when daily average PM2.5 concentrations were greater than 35 µg/m3 because of wildfires. For the CON dams, the maximum daily average PM2.5 concentrations reached 25.2 µg/m3 which occurred around the time of conception. Unexpectedly, the CON calves were exposed to 1 d of wildfire-PM2.5 that reached a daily average of 98.0 µg/m3 postnatally at approximately 4 mo of age and 2 d of wildfire-PM2.5 at approximately 5.5 mo of age that reached a daily average up to 64.5 µg/m3. Thus, the last four time points at which samples were taken for CON calves (18 through 24 wk of age) occurred after postnatal smoke exposure. WFS calves were not exposed to daily average PM2.5 greater than 27.8 µg/m3 in the postnatal life. Weekly THI did not differ between the two locations throughout the study (Figure 1A, P = 0.11).
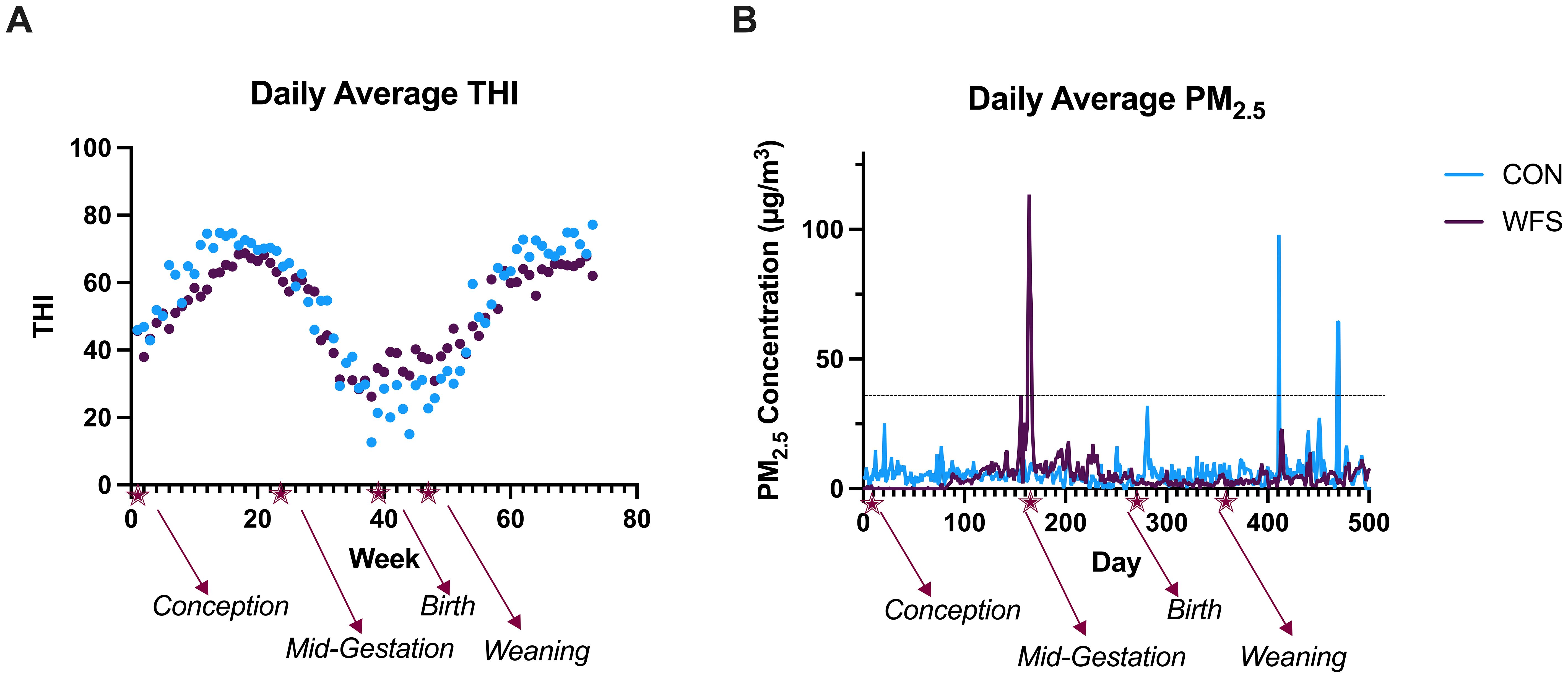
Figure 1. Weekly average temperature–humidity index (THI) and daily average PM2.5 concentrations from calf conception through 6 months after birth. Holstein heifer calves were conceived at week or d 0, and their dams were either exposed (WFS, n=17) or not exposed (CON, n=26) to a natural wildfire smoke event during mid-gestation. (A) THI did not differ between WFS and CON (P = 0.11). (B) Animals were considered exposed to wildfire smoke if wildfire smoke-derived PM2.5 (wildfire-PM2.5) concentrations surpassed a daily average concentration of 35µg/m3, indicated by the dashed line. Dams of WFS calves were exposed to daily average PM2.5 concentrations of up to 113.5 µg/m3 during mid-gestation whereas dams of CON calves were not exposed to PM2.5 levels greater than 35 µg/m3 during gestation (maximum daily average PM2.5 concentration = 25.2 µg/m3 around the time of conception). However, between approximately 4 and 6 mo of age, CON calves were unexpectedly exposed to two wildfire smoke events that reached daily averages of up to 98.0 µg/m3 and 64.5 µg/m3, respectively.
3.2 Growth and gestation length
There was no difference detected in gestation length between the two groups (Table 1, P = 0.30). There was an interactive effect of group and time on body weight, wither height, and hip height (Figure 2). WFS calves were lighter at birth (Figure 2A, P = 0.002). No difference in body weight was detected again until 24 wk of age when CON calves had greater body weights than WFS calves (Figure 2D, P = 0.01). WFS calves also tended to be larger at 10 and 14 wk of age (Figure 2D, P = 0.05 and P = 0.06, respectively). Wither height was greater in CON calves at 2, 4, and 22 wk of age but was higher in WFS calves at 14 wk (Figures 2B, E, all P < 0.03). There was an additional tendency for wither height to be lower in WFS calves at 6 wk (Figure 2B, P = 0.09). WFS calves were shorter at the hip at 2, 4, 8, and 10 wk of age (Figures 2C, F, all P < 0.03), but had higher hip heights by 18 wk of age (Figure 2F, P = 0.04). There was a tendency for WFS calves to have shorter hip heights at 24 wk of age (Figure 2F, P = 0.08).
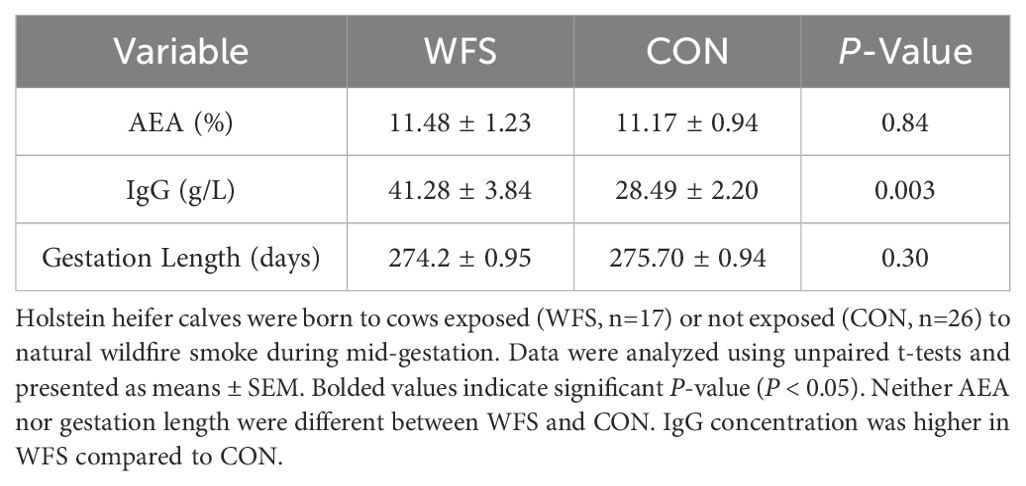
Table 1. Apparent efficiency of IgG absorption (AEA), serum IgG concentration, gestation length in calves after prenatal exposure to wildfire PM2.5.
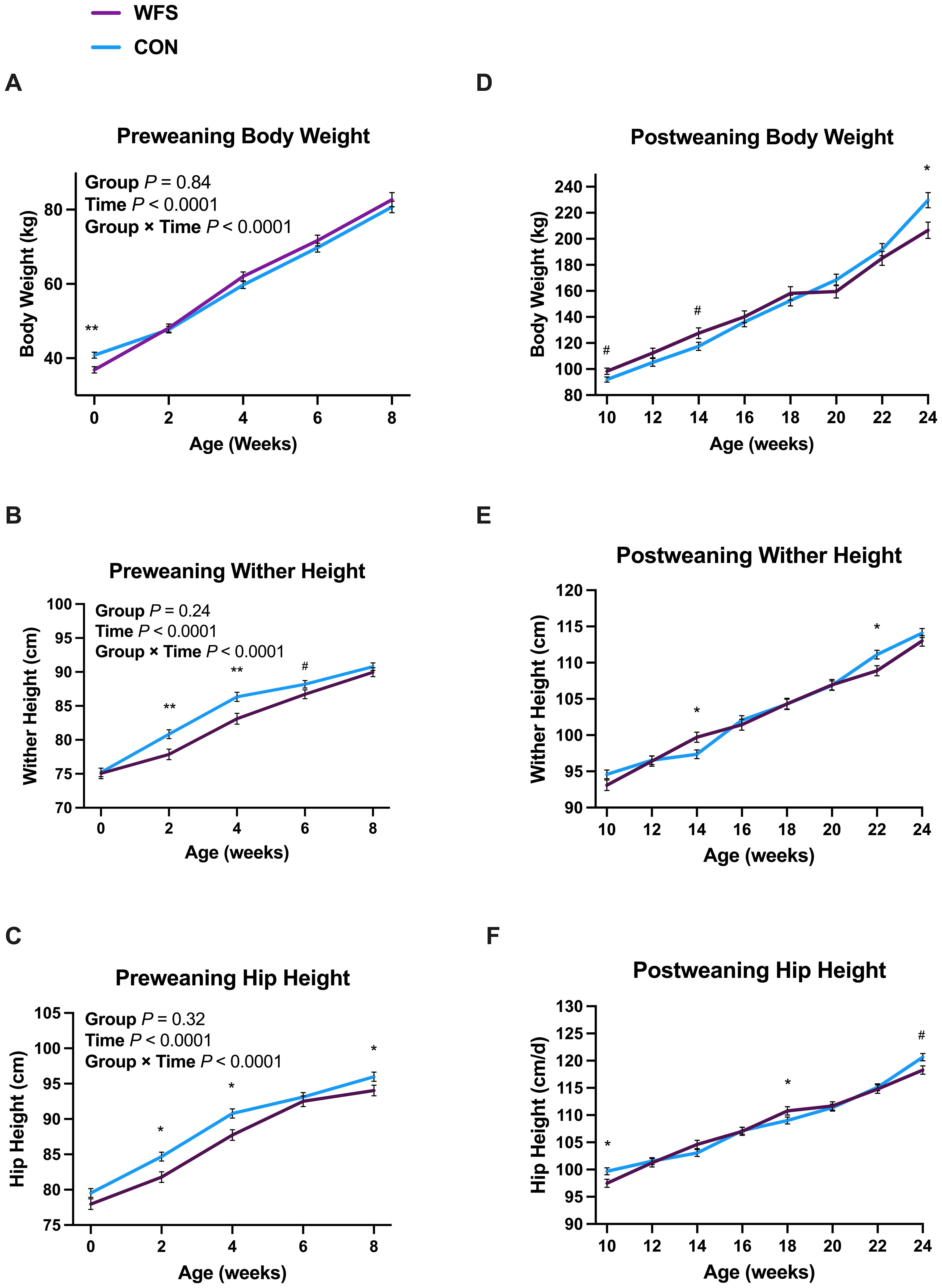
Figure 2. Effects of prenatal wildfire-PM2.5 exposure on calf growth. Pre-weaning (A) body weight, (B) wither height, and (C) hip height and post-weaning (D) body weight, (E) wither height, and (F) hip height. Holstein heifer calves were born to cows exposed (WFS, n=17) or not exposed (CON, n=26) to a natural wildfire smoke event during mid-gestation. General linear mixed models were used to assess group (WFS or CON), time (birth through 24 wk), and their interaction as fixed effects, and time within calf as a repeated effect. Data are LSM ± SEM. Preweaning and postweaning measurements are separated graphically for improved visualization, but all time-points were statistically analyzed together. P-values shown on preweaning graphs reflect the overall effects of group, time, and their interaction on body measurements across all timepoints. *P < 0.05; **P < 0.01, #0.05 < P < 0.1. WFS body weight was lower at birth and at 24 wk of age compared to CON. Wither height was lower in WFS calves from 2 through 6 weeks of age but higher at 14 wk of age. Hip height was lower in WFS calves in early postnatal life, but higher at 18 wk of age.
Differences in ADG were observed in both the pre-weaning and post-weaning periods. Body weight ADG was greater in the WFS group relative to that for CON from birth through weaning (Figure 3A, P = 0.01), but was lower in WFS from weaning through 6 months of age (Figure 3A; P = 0.003). There were no differences detected between groups for wither and hip height average daily gains, but both decreased overall after weaning (Figures 3B, C, both P < 0.0001).
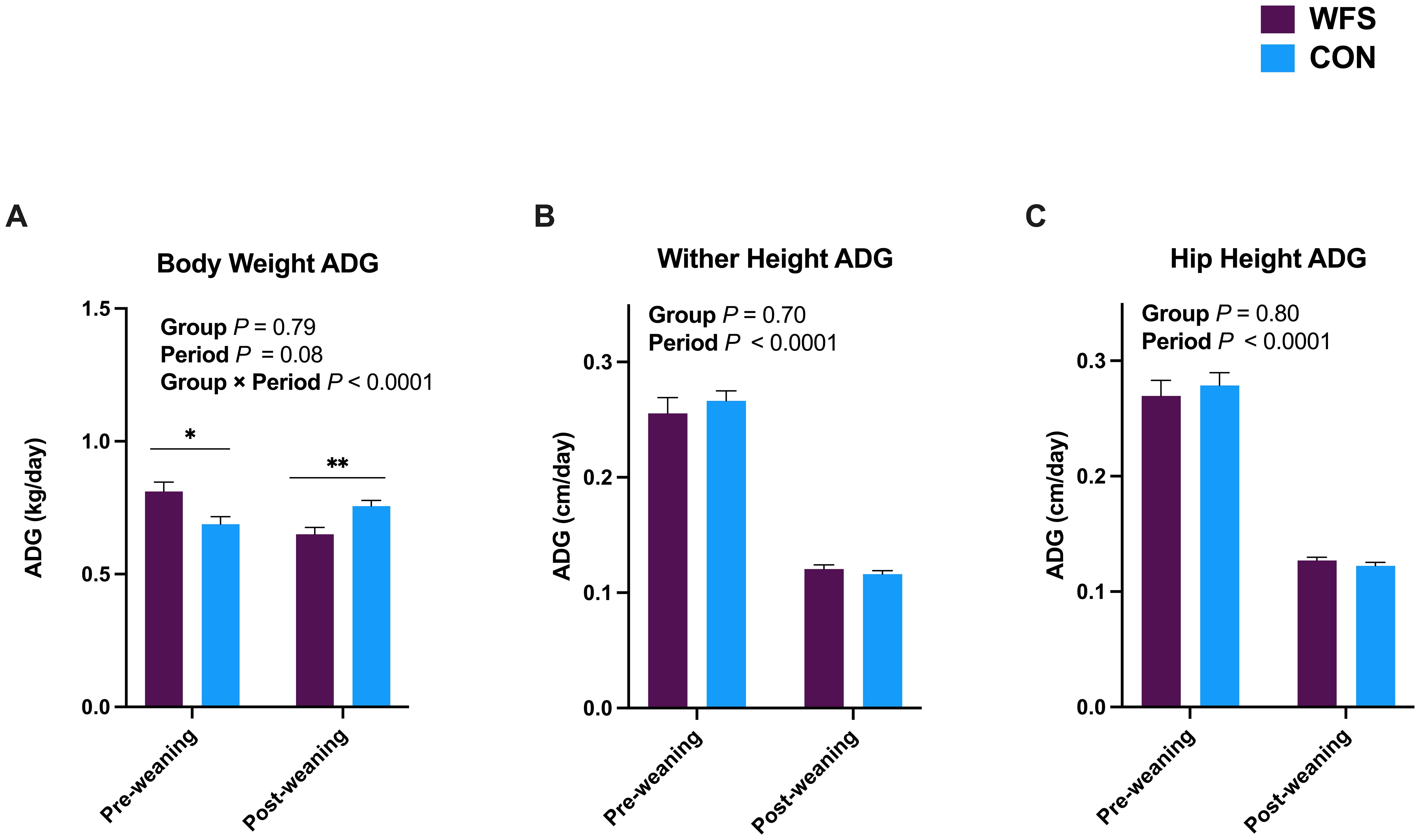
Figure 3. Average daily gain (ADG) of (A) body weights, (B) wither heights, (C) hip heights in Holstein heifer calves born to dams exposed (WFS, n=17) or not exposed (CON, n=26) to a natural wildfire smoke event during mid-gestation. General linear mixed models were used to assess the effects of group (WFS or CON), period (preweaning vs. post-weaning) and their interaction as fixed effects, and period within calf as a repeated effect. Nonsignificant interactions were removed from the final model. Data are presented as LSM ± the SEM. *P < 0.05; **P < 0.01. Pre-weaning body weight ADG was higher in WFS, wheareas post-weaning body weight ADG was lower compared to CON.
3.3 Metabolites
There were interactions between group and time on plasma glucose, BHB, and NEFA concentrations. WFS had greater blood glucose concentrations at 2, 6, 8, and 10 wk of age, and lower concentrations at 18 wk of age (Figure 4A, all P < 0.05). Glucose tended to be lower in WFS calves at wk 14 and higher at wk 20 (Figure 4A, P = 0.06 and P = 0.08, respectively). WFS had lower BHB concentrations relative to CON at 12, 14, and 22 wk of age (Figure 4B, all P < 0.03), and greater BHB concentrations at 2, 16 and 18 wk (Figure 4B, all P < 0.05). NEFA concentrations were greater in WFS at 4 and 6 wk, lower in WFS at 8 wk, and greater again in WFS at 14–20 wk (Figure 4C, all P < 0.03).
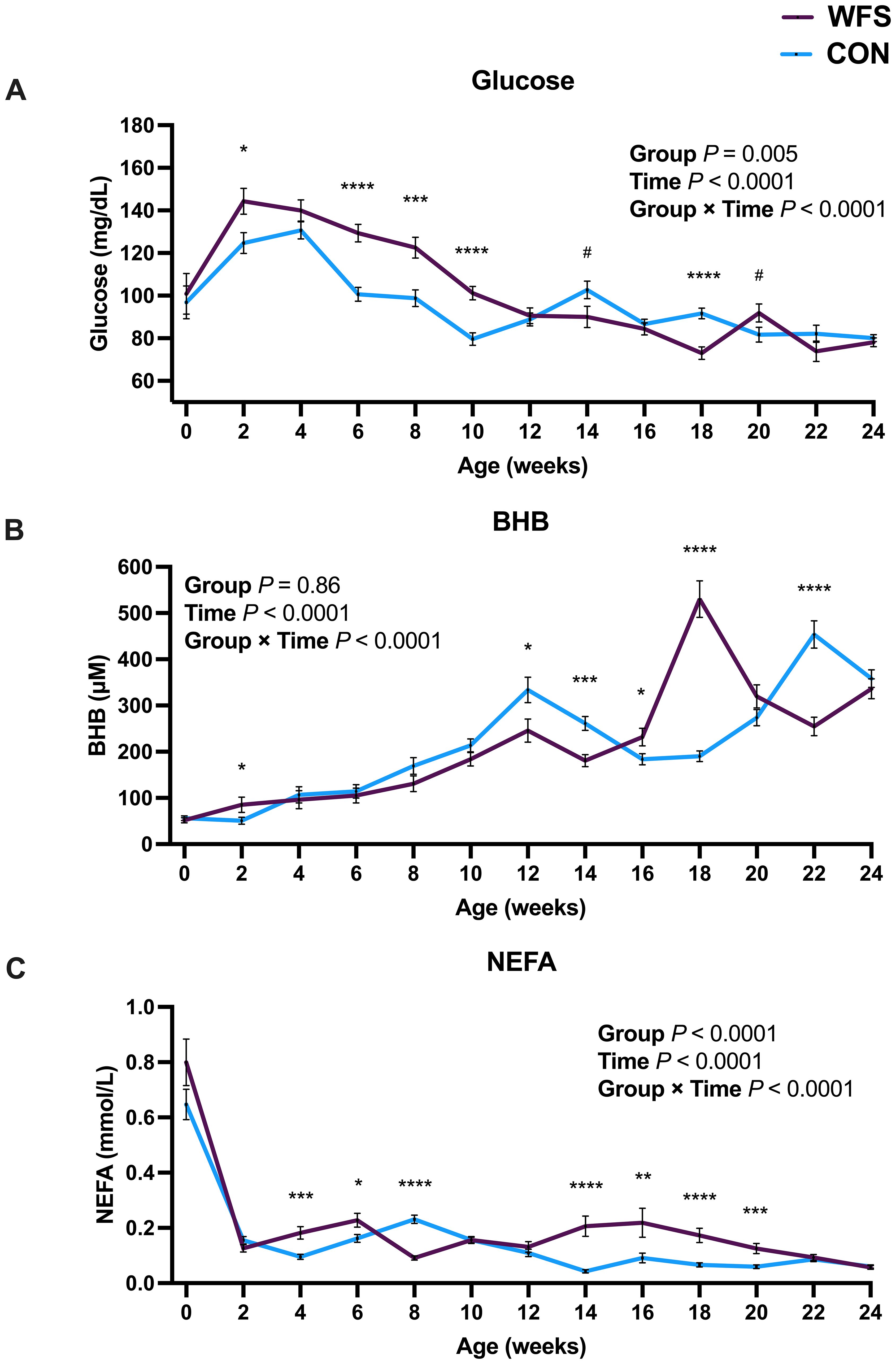
Figure 4. Effects of prenatal wildfire-PM2.5 exposure on postnatal calf blood metabolites; blood (A) glucose, (B) β-Hydroxybutyrate (BHB), (C) non-esterified fatty acids (NEFA). Holstein heifer calves were born to dams exposed (WFS, n=17) or not exposed (CON, n=26) to a natural wildfire smoke event during mid-gestation. Data were analyzed using general linear mixed models with group (WFS or CON), time (birth through 24 wk), and their interaction as fixed effects, and time within calf as a repeated effect. Data are LSM ± SEM. *P < 0.05; **P < 0.01; ***P < 0.001; ****P < 0.0001; #0.05 < P < 0.1. Glucose was higher in WFS in early postnatal life, but was lower at 18 wk of age. In WFS calves compared to CON calves, BHB was higher at 2, 16, and 18 wk of age, and lower at 12, 14, and 22 wk of age. NEFA was higher in WFS calves at wk 4–6 and 14–20, but lower at 8 wk of age compared to CON.
3.4 Acute phase proteins
From birth to 24 wk of age, SAA was lower in the WFS group, whereas SA was greater compared to that for CON (Figure 5A, P < 0.001 and Figure 5C, P = 0.001, respectively). Time alone also influenced both SAA and SA (Figure 5A, P < 0.0001 and Figure 5C, P = 0.0005, respectively). There was a group × time effect for Hp, with Hp concentrations being lower in WFS calves only at 12 wk of age (Figure 5B, P < 0.0001).
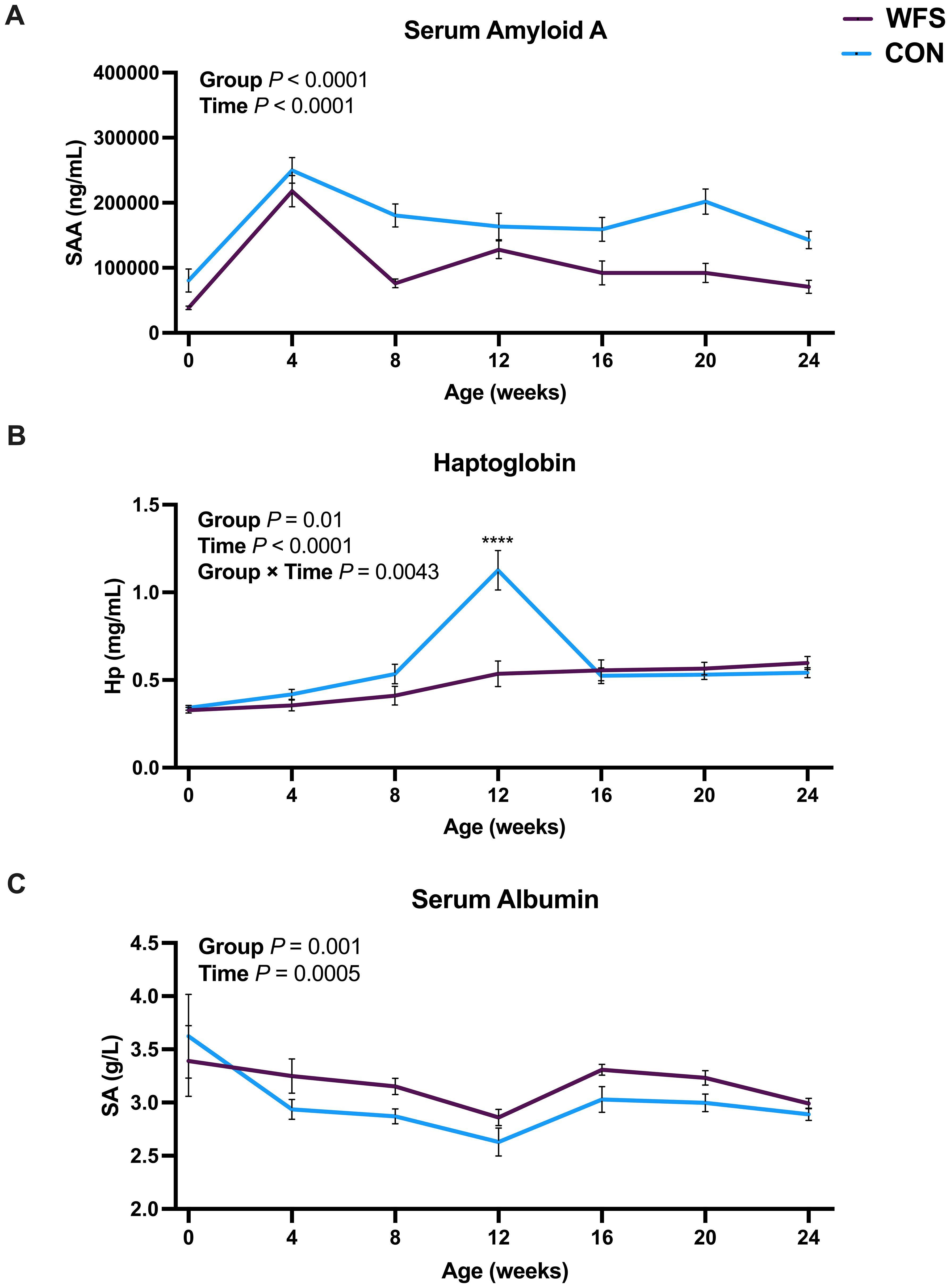
Figure 5. Effects of prenatal wildfire-PM2.5 exposure on postnatal calf (A) Serum amyloid A (SAA), (B) Haptoglobin (Hp), (C) Serum albumin (SA). Holstein heifer calves were born to cows exposed (WFS, n=17) or not exposed (CON, n=26) to a natural wildfire smoke event during mid-gestation. General linear mixed models were used to assess group (WFS or CON), time (birth through 24 wk), and their interaction as fixed effects, and time within calf as a repeated effect. Nonsignificant interactions were removed from the final model. Data are presented as LSM ± SEM. ****P < 0.0001. WFS had lower serum amyloid A, but higher serum albumin throughout the study. CON calves had elevated haptoglobin at 12 wk of age.
3.5 IgG And AEA
AEA was not different between WFS and CON (Table 1, P = 0.84). However, WFS calves had greater serum IgG relative to CON (Table 1; P < 0.01).
3.6 Hematology and cortisol
There were differences in hematology variables between groups at several time points. Total white blood cells (WBC) were lower in WFS relative to CON from birth through 8 wk of age, and at 16 and 18 wk of age (Table 2, all P < 0.03). WBC tended to be lower than CON at 22 wk (Table 2, P = 0.09). Total red blood cell counts were lower in WFS compared to CON from birth through 2 wk of age, higher at 4 wk of age, and lower than CON again at 12 and 16 wk of age (Table 2, all P < 0.02). Hemoglobin was lower in WFS relative to CON from birth through 2 wk, and again at 8–24 wk of age (Table 2, all P < 0.05). Hematocrit was lower in WFS at birth, but was higher at 4 wk, 8 to 14 wk, and 18 to 24 wk compared to CON (Table 2, all P < 0.05). Lymphocytes were lower in WFS calves compared with that for CON at 4 wk, 8 wk, and higher in WFS than CON at 16 wk of age (Table 2, all P < 0.02). Lymphocytes also tended to be lower in WFS calves at 6 wk and tended to be higher in WFS at 10 wk compared to CON (Table 2, P = 0.07 and P = 0.10, respectively).
There was a tendency for cortisol to be lower in WFS calves compared to CON (51,200 ± 8,873 vs. 74,968 ± 8,728 pg/mL; P = 0.06). Overall, cortisol concentration decreased from birth to 2 wk of age (99,989 ± 11,232 pg/mL vs. 26,178 ± 9,497 pg/mL; P < 0.0001).
3.7 Vital signs, thoracic ultrasound scores, and health scores
There was an interaction between group and time on both rectal temperature and heart rate. Rectal temperature was lower in WFS calves at 4 wk and 10 wk of age (Table 3, P = 0.04 and P = 0.0002, respectively), and higher than CON at wk 22 and wk 24 (Table 3, all P = 0.03 and P = 0.04, respectively). Heart rate was higher in WFS calves at birth, but lower at wk 4, 8, 14 and 20 (Table 3, all P < 0.05).
TUS scores were different between groups. WFS calves were less likely to have higher TUS scores as compared to that for CON calves, indicating greater likelihood of increased severity or extent of lung consolidation in CON calves (Table 4, P < 0.0001). Similarly, WFS calves were less likely to have high nasal scores relative to CON calves across the study, indicating increased nasal discharge in CON calves (Table 4, P < 0.0001). There was an additional effect of time on nasal scores (Table 4, P < 0.0001). Time alone impacted cough and attitude scores (Table 4, both P < 0.0001). There was no discernible effect of group or time on naval scores (Table 4, P = 0.12 and 0.67, respectively) or joint scores (Table 4, P = 0.96 and 1.00, respectively. There was an interactive effect between group and time on eye scores, with WFS calves being less likely to have higher eye scores at 12 wk of age (Table 5, P < 0.002) and more likely to have higher eye scores, at 18 wk of age (Table 5, P = 0.03). Group and time interactions explained fecal scores, as there were greater odds of WFS having higher fecal scores than CON calves at 2 wk of age (Table 5, P = 0.001).
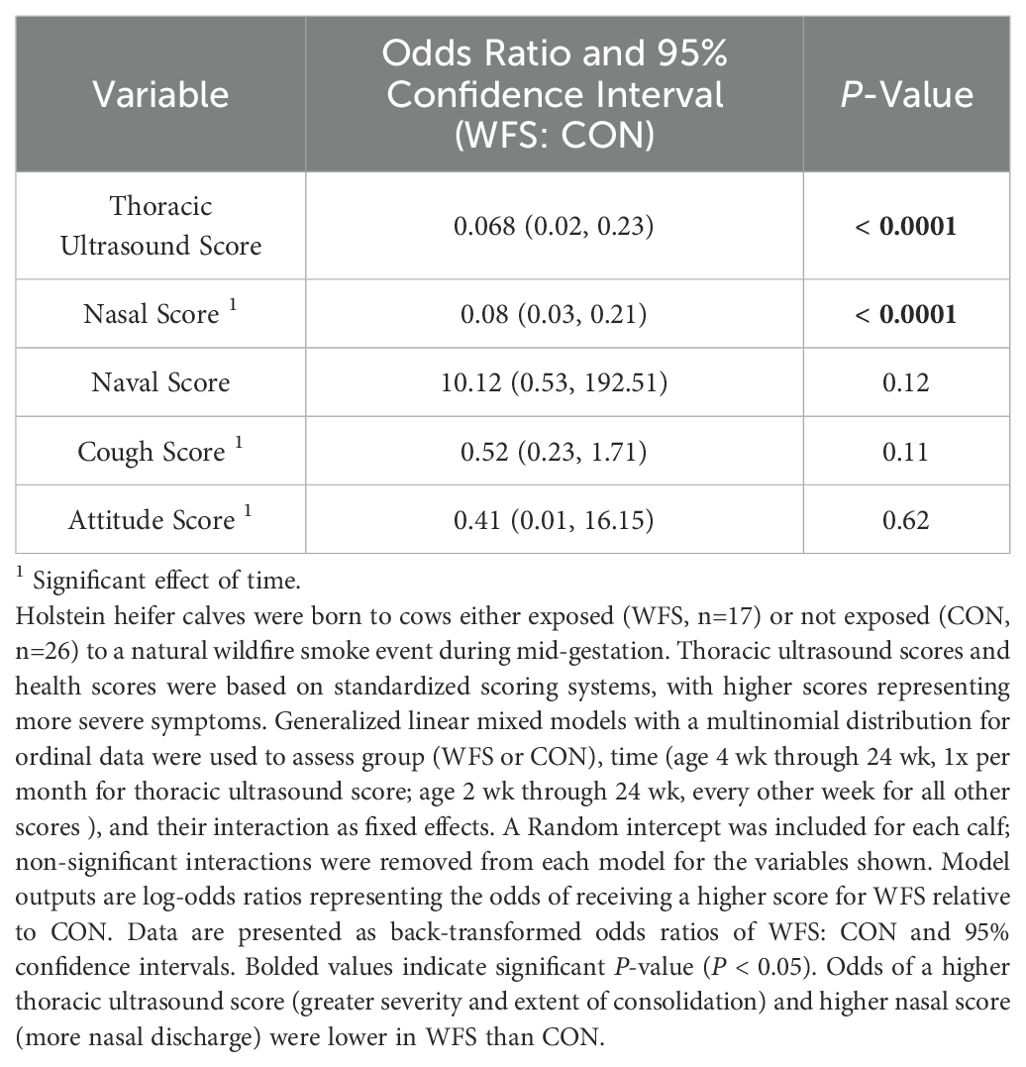
Table 4. Thoracic ultrasound, nasal, and cough scores in the postnatal calf after exposure to wildfire-PM2.5 in utero.
4 Discussion
The dairy industry faces the challenge of maximizing health and efficiency in cattle while combatting severe climates and climatic events, such as wildfires. It is well-established that the intra-uterine environment influences postnatal phenotype. For example, heat stress in late gestation cattle has been associated with many negative effects on postnatal calves, including, but not limited to, reduced efficiency of absorption of IgG, lower birth weights, reduced gestation length, epigenetic alterations, and compromised immune function (Davidson et al., 2021; Laporta et al., 2017; Skibiel et al., 2018; Tao et al., 2012). Although wildfire-PM2.5 is known to impact metabolic and inflammatory statuses of dairy calves and cows (Anderson et al., 2022; Pace et al., 2023), the effects of prenatal exposure to wildfire-PM2.5 on calf development has remained unexplored until now. Herein, we investigated whether prenatal wildfire-PM2.5 exposure causes altered growth, metabolism, and health in the first 6 months of postnatal life for dairy calves.
WFS calves had lower body weights at birth than CON, which were not attributed to frame size or gestation length. WFS calves also had greater preweaning ADG, resulting in a tendency for greater body weights soon after weaning, but this effect did not persist as WFS had lower ADG in the post weaning phase relative to CON. These findings corroborate observations in humans linking maternal wildfire-PM2.5 exposure during pregnancy to lower birth weights (Abdo et al., 2019; Breton et al., 2011; Holstius et al., 2012; O’Donnell and Behie, 2013). Additionally, inhalation of ambient particulate matter < 10 µm in diameter in pregnant mothers was associated with altered ultrasound-detected fetal growth measurements (Hansen et al., 2008; Zhao et al., 2018; Zhou et al., 2022). Our results suggest that WFS calves may have experienced intra-uterine growth restriction (IUGR), followed by accelerated growth during the pre weaning phase, although this was a transient effect. Compensatory growth, or catch-up growth, can occur in animals exposed to conditions, such as nutritional or environmental stressors, that result in IUGR (reviewed by Wathes, 2022), and may explain the increased ADG observed in WFS calves during the pre weaning phase. The mechanisms by which wildfire smoke-induced IUGR may take place are unclear, but could include both consequences of maternal responses to PM2.5 inhalation and interactions of wildfire-PM2.5 with the placental tissue (Bové et al., 2019; Bongaerts et al., 2021). Studies in pregnant mice suggest impaired placental angiogenesis (Yue et al., 2019) and altered expression of placental nutrient transporters after exposure to non-wildfire derived PM2.5 (Zhu et al., 2021). Of note, IUGR may result in disparities in tissue and organ development. For example, in cattle, maternal nutritional stress during early and mid-gestation causes altered calf tissue and organ mass including a larger proportion of perirenal adipose tissue depots relative to empty body weight (Taylor et al., 2018). Furthermore, maternal heat stress in late gestation has been associated with altered gross organ masses (Dado-Senn et al., 2021) and growth patterns of the postnatal calf (Laporta et al., 2017; Tao et al., 2012). Although tissue and organ system growth and development were not investigated in the present study, it is possible that prenatally exposed calves may have modified tissue composition and organ development that contributed to postnatal growth patterns.
At a number of time points in the pre-weaning period, plasma glucose, the main energy substrate for pre-weaned calves (Hammon et al., 2012) was greater in WFS than CON calves. There may be several reasons for elevated blood glucose including over- or under- expression of insulin or non-insulin dependent glucose transporters, differences in hormone sensitivity or endocrine regulation of glucose uptake, endogenous glucose production, or altered tissue metabolic demands. Indeed, diminished glucose tolerance and reduced glucose-induced insulin secretion were observed in male mice born to dams exposed to diesel exhaust PM2.5 before and during gestation (Chen et al., 2018). Additionally, systemic sensitivity to insulin may be diminished in mice postnatally exposed to ambient PM2.5 (Li et al., 2020). Human mortalities caused by endocrine and digestive diseases, and specifically diabetes, have been linked to long-term postnatal wildfire-PM2.5 exposure (Ma et al., 2024). In ruminants, there are marked changes that occur in glucose metabolism during prenatal development (Hocquette et al., 2006) and disrupting that development may lead to changes in postnatal glucose metabolism. For example, heat stressed sheep have reduced placental permeability to nutrients and subsequent fetal IUGR (Limesand et al., 2018). Heat stress-induced IUGR lambs have greater glucose-induced insulin secretion, as well as greater rates of glucose utilization and increased insulin sensitivity for glucose utilization during a hyper insulinemic-euglycemic clamp relative to non-IUGR lambs, despite having similar fasting plasma glucose and insulin concentrations (Camacho et al., 2017). Although the present study was limited to investigation of post prandial blood glucose concentrations, our findings suggest disruptions in glucose metabolism in dairy calves whose dams were exposed to wildfire-PM2.5 during mid-gestation.
Following weaning, NEFA and BHB were greater and lower, respectively, at several time points in WFS relative to CON. Although BHB may be produced because of hepatic ketogenesis, an increase in the oxidation of butyrate to BHB occurs following ruminal development as microbial fermentation increases (Deelen et al., 2016; Quigley et al., 1991). Thus, greater BHB concentrations may indicate CON calves had more developed rumens after weaning or an increased rate of ketogenesis. Consistently greater NEFA in WFS calves suggests that WFS calves may have catabolized fat stores more than CON calves. This could have implications on feed efficiency for the WFS calves, as they also had lower average daily gains post-weaning. However, because solid feed intake was not measured in the present study, it is unclear whether wildfire-PM2.5-exposed calves are more or less feed efficient than their non-exposed counterparts.
The mechanisms underpinning altered postnatal metabolism in WFS calves require further investigation. Adult cows exposed to wildfire-PM2.5 experience shifts in energy metabolism related to exposure to concomitant elevated wildfire-PM2.5 and THI (Anderson et al., 2022). Providing a lower protein in the diet of late gestation beef cows results in altered offspring glucose metabolism (Maresca et al., 2018). Additionally, inflammatory responses in pregnant ewes impacts fetal glucose metabolism (Cadaret et al., 2019). Thus, fetal responses may be related to changes in maternal metabolic or inflammatory responses and may have prolonged effects on offspring metabolism. Altered placental function may play an important role in programming fetal metabolism. For example, placental nutrient absorption or tissue metabolic changes may be linked to PM2.5 exposure and may be modulated by altered placental expression of genes related to metabolic function. For example, higher PM2.5 exposure within sensitive developmental windows was associated with altered placental genes involved in cellular respiration processes and amino acid transport in humans (Deyssenroth et al., 2021). Using a similar study population, Kaur et al. (2022) found that several genes involved in lipid metabolism were either downregulated (ex. ABHD3) or upregulated (ex. ATP11A, PSCA, and ST6GALNAC4) with gestational PM2.5 exposure. Further, placental nutrient transfer may be impacted by PM2.5 exposure. Mice that aspirated ambient-sourced PM2.5 during pregnancy had altered placental nutrient transporter gene expression (ex. GLUT3, FATP1, FATP4, SNAT1, SNAT4) in late gestation relative to mice that aspirated vehicle, which may have consequences for maternal-fetal nutrient transfer capacity, and therefore, offspring growth and development (Zhu et al., 2021). Air quality-induced changes in fetal growth and metabolism may have implications on long-term postnatal metabolism; a recent study found that fecal metabolic markers in babies up to 1 year of age are altered after exposure to non-wildfire derived ambient air pollutants (Holzhausen et al., 2024). Thus, the mechanisms underlying the changes in postnatal metabolism of calves exposed to wildfire-PM2.5 may involve interactions among maternal, placental, and fetal factors.
In the present study, we also found evidence of altered immune status in calves exposed to wildfire-PM2.5 in utero. WFS calves had greater concentrations of the negative acute phase protein SA, and lower concentrations of the positive acute phase proteins SAA and Hp at one time point in the post weaning phase, along with lower overall WBC concentrations, and several leukocyte subpopulations. There are notable changes in locations in which hematopoietic development occurs throughout embryonic and fetal development (Ciriza et al., 2013). Disturbances to the migration and colonization of fetal hematopoietic stem cells in different tissues, such as from the fetal liver to bone marrow, may be a possible mechanism for leukocyte disparities seen in the present study. Indeed, bovine hematopoietic stem cells are found in the fetal bone marrow around mid to late gestation (Pessa-Morikawa et al., 2012), the approximate timeframe in which prenatal WFS calves were exposed to wildfire-PM2.5. Previous studies in humans have demonstrated that wildfire-PM2.5 exposure during early, and possibly mid-gestation, is associated with lower fetal Haufbour cells (Basilio et al., 2023), while traffic-derived air pollutants, including PM2.5, affects concentrations of neonatal cord blood leukocytes (García-Serna et al., 2021) and cytokines (García-Serna et al., 2022). Growth restriction could also be an important factor in immune cell generation, as neutropenia has been observed in human neonates born small for their gestational age (Christensen et al., 2015). It is also known that ambient PM2.5 causes systemic inflammatory responses in humans (Dabass et al., 2018; Zhang et al., 2022) and wildfire-PM2.5 causes changes in inflammatory markers in cattle (Anderson et al., 2022; Pace et al., 2023). However, hematopoietic impairment or altered inflammatory signaling may not be the only explanations for the differences we observed in blood hematological parameters in WFS vs. CON calves. At birth, WFS calves had lower HCT than CON calves, but this pattern was opposite in the following weeks. Although RBC production could alter HCT, it is also possible that HCT was affected by altered water balance, which is, in part, regulated by the placenta during fetal development, and through hormones such as vasopressin (Lindower, 2017). Although the placenta serves a protective role against fetal insults (Hsiao and Patterson, 2012), exposure to ambient air pollutants during pregnancy has been associated with black carbon accumulation in placental tissue, which could facilitate direct contact with the fetus (Bové et al., 2019; Bongaerts et al., 2021). Taken together, these studies suggest that wildfire-PM2.5 may program the fetal immune system, altering responses to environmental and pathogenic insults in the future. Future research is needed to determine whether immune cell and protein production, water balance, or both, drive the differences in hematological parameters seen in postnatal calves in the present study.
Whether differences in white blood cells and inflammatory markers observed in WFS calves provide a physiological advantage or suggest developmental impairment is currently unknown. In the present study however, based on health scoring and thoracic ultrasounds, WFS respiratory health appeared to be better than CON overall. WFS calves had lower odds of eye discharge at approximately 12 wk of age, and lower odds of ultrasound-detected lung consolidation throughout the study. However, WFS calves had higher odds of enteric symptoms at 2 wk of age. All calves received adequate passive transfer of immunity at birth and no difference in apparent efficiency of IgG absorption was detected. WFS calves however, had greater serum IgG concentrations at 48 hr, likely because of greater concentrations of IgG provided in the colostrum. It is possible that greater IgG concentrations could have conferred a superior protection against postnatal respiratory pathogens for WFS calves. Although odds of TUS-detected lung consolidation were lower in the WFS calves, lung lesions must reach the pleura to be visible using thoracic ultrasonography. Thus, pathologies that do not reach the pleura may have been present but unobserved (Ollivett and Buczinski, 2016). Lung consolidation observed in the present study may have arisen from unmeasured factors between the farms, including pathogen exposure. Alternatively, from a programming perspective, it is possible that prenatal exposure to wildfire-PM2.5 influences the extent of responses to postnatal immune challenges. Although the lesser lung consolidation present in the WFS calves suggests a beneficial effect, these same calves were afflicted with enteric symptoms and altered growth patterns, so if programming played a role in WFS lung responses, it may not confer an overall production advantage. However, to assess what mechanisms are controlling these responses, experimental studies including matched immune challenges between prenatal wildfire-PM2.5 exposed and unexposed calves are needed.
Several of the responses observed herein corroborate previous studies on the impacts of wildfire-PM2.5 inhaled by adult cows and young calves. We have previously shown that dairy calves exposed to elevated wildfire-PM2.5 during the pre weaning phase had shifts in circulating glucose, BHB, and NEFA, acute phase proteins, and leukocytes (Pace et al., 2023). In addition, lactating dairy cows exposed to high concentrations (daily average up to 282.5 µg/m3 for 7 d) of wildfire-PM2.5 had reduced milk production, and altered concentrations of several blood components including blood CO2, hemoglobin, red blood cell and leukocyte concentrations, and metabolic markers such as NEFA (Anderson et al., 2022). Furthermore, these effects continued beyond the exposure period in both cows and calves; thus, acute exposures may result in delayed and persistent effects. The mechanisms linking maternal exposure to postnatal calf phenotype are not entirely clear, but could be related to maternal physiology such as activation of inflammatory cascades, oxidative stress, endocrine disruption, and hemodynamic modulations (reviewed by Basilio et al., 2022). Additionally, inhaled particulates may interact directly with placental and fetal tissues (Bové et al., 2019; Bongaerts et al., 2021). Future studies should investigate concomitant maternal and fetal responses during and after wildfire-PM2.5 exposure, and their effects on postnatal outcomes. Furthermore, investigations into interventions and management strategies to mitigate animal exposures and responses to wildfire-PM2.5 (ie., nutritional modifications and supplementations, improvements to housing, animal health assessments and treatment protocols) are needed. Farm managers may consider limiting stressful management events, such as transportation, during episodes of poor air quality and closely monitoring health of animals, especially susceptible groups like pregnant cows and newborn calves, when air quality is degraded by wildfire smoke.
A limitation of the present study is the geographically distinct location of each calf group, which was necessary to study natural wildfire smoke exposure. Although management and other conditions were matched between the two farms, other external unmeasured factors could exist. This includes the two unexpected smoke events that affected the CON calves in the late postnatal period. These events may have affected CON calf variables after 4 months of age. CON calves were sampled approximately 3 days after smoke exposure, and at 4 months of age, although there was no statistical difference detected in WFS vs CON calves, blood glucose concentrations appeared to decrease and BHB increased in CON from the 4-month mark. These results are similar to the responses we have seen in pre-weaned calves 3 days after simultaneously elevated wildfire-PM2.5 and THI, in which we hypothesized that calves may have been mobilizing body reserves for energy (Pace et al., 2023). Additionally, in the present study, circulating lymphocyte concentrations were lower in CON calves relative to WFS calves at 4 months, and were almost half that of concentrations seen at 3.5 months. This response, too, is similar to that seen in our previous study, in which we observed lower lymphocyte concentrations 2 days after exposure of pre-weaned calves to simultaneously elevated PM2.5 and THI (Pace et al., 2023). Another limitation of the study is that although diets were matched to limit differences between calf groups in nutritional status, intake of solid feed or water were not controlled or measured. Thus, disparities between groups in metabolism or growth could be at least in part attributed to different feed and water intakes. A third limitation to the present study is the duration of time calves were monitored. The impacts of prenatal exposure to natural wildfire-PM2.5 on postnatal outcomes in young calves has not, to our knowledge, been previously investigated. However, impacts on future production and calf development beyond 6 months of age should be explored. Finally, farm staff-level assessments of calf health were made by different individuals, which may have influenced propensity for treatments provided to calves on either farm. However, staff are trained to follow standard protocols to determine whether treatment for a calf is necessary, thus reducing the likelihood of treatment differences as a confounding factor between farms.
To our knowledge, this is the first study of prenatal exposure to natural wildfire-PM2.5 in a non-rodent or non-primate mammal in which nutrition, age and sex were experimentally controlled. Herein, we found evidence of PM2.5-induced IUGR, characterized by lower birth weights and accelerated postnatal growth in WFS calves. Furthermore, WFS and CON calves appear to have disparities in basal inflammatory status and metabolism, which could impact future health and production. Further investigation is necessary to pinpoint causal mechanisms through which prenatal wildfire-PM2.5 exposure programs postnatal calf phenotype. For example, future studies could investigate prenatal factors such as the relationship between maternal-fetal inflammatory cascades, endocrine signaling, placental nutrient transportation, and effects on tissue-level ontogeny, during and after wildfire-PM2.5 exposure. Investigating longer-term phenotypic effects, such as impacts on calf growth to puberty, feed efficiency, and first-lactation milk production would also provide more comprehensive insights into production implications. Understanding the relationship between wildfire-PM2.5 exposure and calf ontogeny may aid development of interventions to maximize dairy cattle health, productivity, and welfare at vulnerable life stages.
Data availability statement
The original contributions presented in the study are included in the article/Supplementary Material. Further inquiries can be directed to the corresponding author.
Ethics statement
The animal studies were approved by Institutional Animal Care and Use Committee. The studies were conducted in accordance with the local legislation and institutional requirements. Written informed consent was obtained from the owners for the participation of their animals in this study.
Author contributions
AP: Conceptualization, Formal analysis, Investigation, Methodology, Validation, Visualization, Writing – original draft. PV: Investigation, Resources, Writing – review & editing. MR: Resources, Writing – review & editing, Investigation. GP: Investigation, Writing – review & editing. NE: Investigation, Writing – review & editing. ASt: Writing – review & editing, Investigation. ML: Investigation, Writing – review & editing. DK: Writing – review & editing, Conceptualization, Funding acquisition, Resources. PR: Writing – review & editing, Conceptualization, Funding acquisition, Methodology, Resources. AS: Investigation, Writing – review & editing, Conceptualization, Formal analysis, Funding acquisition, Methodology, Project administration, Supervision, Validation, Visualization.
Funding
The author(s) declare that financial support was received for the research and/or publication of this article. This project was funded by the Agriculture and Food Research Initiative, project award #2022-68016-38665 from the U.S. Department of Agriculture’s National Institute of Food and Agriculture, and an Institutional Development Award (IDeA Network) from the National Institute of General Medical Sciences of the National Institutes of Health (Grant#: P20GM103408). The authors do not have any conflicts of interest to disclose.
Acknowledgments
The authors would like to extend their gratitude to the collaborators and staff at the University of Idaho Dairy Center and commercial farm in South Dakota. Thank you to the NOAA Air Resource Laboratories for use of HYSPLIT modeling system, and TANTUS technologies for providing access to AirNow Tech Navigator technology. Hematology analyses for CON calves were performed at the South Dakota State Animal Disease Research and Diagnostic Laboratory. Chemical analysis of feed samples was performed by Dairy One Forage Testing Laboratory. Thank you to Dr. Lucelia de Moura Pereira, Victoria Akwamaa Yeboah, as well as our undergraduate students, veterinarians, and volunteers who assisted on various aspects of this project. The authors are grateful for the statistical consultations provided by Dr. Julia Piaskowski at the University of Idaho College of Agricultural and Life Sciences.
Conflict of interest
The authors declare that the research was conducted in the absence of any commercial or financial relationships that could be construed as a potential conflict of interest.
Generative AI statement
The author(s) declare that no Generative AI was used in the creation of this manuscript.
Publisher’s note
All claims expressed in this article are solely those of the authors and do not necessarily represent those of their affiliated organizations, or those of the publisher, the editors and the reviewers. Any product that may be evaluated in this article, or claim that may be made by its manufacturer, is not guaranteed or endorsed by the publisher.
Supplementary material
The Supplementary Material for this article can be found online at: https://www.frontiersin.org/articles/10.3389/fanim.2025.1580654/full#supplementary-material
References
Abatzoglou J. T., Williams A. P. (2016). Impact of anthropogenic climate change on wildfire across western US forests. Proc. Natl. Acad. Sci. U. S. A 113, 11770–11775. doi: 10.1073/pnas.1607171113
Abdo M., Ward I., O’Dell K., Ford B., Pierce J. R., Fischer E. V., et al. (2019). Impact of wildfire smoke on adverse pregnancy outcomes in colorado 2007–2015. Int. J. Environ. Res. Public Health 16, 3720. doi: 10.3390/ijerph16193720
Ackermann M. R., Derscheid R., Roth J. A. (2010). Innate immunology of bovine respiratory disease. Vet. Clin. North Am. Food Anim. Pract. 26, 215–228. doi: 10.1016/j.cvfa.2010.03.001
Anderson A., Rezamand P., Skibiel A. L. (2022). Effects of wildfire smoke exposure on innate immunity, metabolism, and milk production in lactating dairy cows. J. Dairy. Sci. 105, 7047–7060. doi: 10.3168/jds.2022-22135
Barker D. J. (1990). The fetal and infant origins of adult disease. BMJ 301, 1111. doi: 10.1136/bmj.301.6761.1111
Barker D. J. P. (2007). The origins of the developmental origins theory. J. Internal Med. 261, 412–417. doi: 10.1111/j.1365-2796.2007.01809.x
Barker D. J. P., Osmond C. (1986). Infant mortality, childhood nutrition, and ischaemic heart disease in England and Wales. Lancet 327, 1077–1081. doi: 10.1016/S0140-6736(86)91340-1
Basilio E., Chen R., Fernandez A. C., Padula A. M., Robinson J. F., Gaw S. L. (2022). Wildfire smoke exposure during pregnancy: A review of potential mechanisms of placental toxicity, impact on obstetric outcomes, and strategies to reduce exposure. IJERPH 19, 13727. doi: 10.3390/ijerph192113727
Basilio E., Ozarslan N., Buarpung S., Benmarhnia T., Padula A. M., Robinson J. F., et al. (2023). Gestational age-dependent decrease in fetal Hofbauer cells in placentas from pregnancies exposed to wildfire smoke in California. medRxiv. doi: 10.1101/2023.01.11.23284125
Beaupied B., Martinez H., Martenies S., McConnel C., Pollack I., Giardina D., et al. (2021). Cows as canaries: The effects of ambient air pollution exposure on milk production and somatic cell count in dairy cows. Environ. Res. 207, 112197. doi: 10.1016/j.envres.2021.112197
Black C., Gerriets J. E., Fontaine J. H., Harper R. W., Kenyon N. J., Tablin F., et al. (2017). Early life wildfire smoke exposure is associated with immune dysregulation and lung function decrements in adolescence. Am. J. Respir. Cell Mol. Biol. 56, 657–666. doi: 10.1165/rcmb.2016-0380OC
Bongaerts E., Bové H., Lambrichts I., Saenen N. D., Gyselaers W., Plusquin M., et al. (2021). Reply to ‘Fetal side’ of the placenta: Anatomical mis-annotation of carbon particle ‘transfer’ across the human placenta. Nat. Commun. 12, 7050. doi: 10.1038/s41467-021-26438-x
Bové H., Bongaerts E., Slenders E., Bijnens E. M., Saenen N. D., Gyselaers W., et al. (2019). Ambient black carbon particles reach the fetal side of human placenta. Nat. Commun. 10, 3866. doi: 10.1038/s41467-019-11654-3
Breton C., Park C., Wu J. (2011). Effect of prenatal exposure to wildfire-generated PM2.5 on birth weight. Epidemiology 22, S66. doi: 10.1097/01.ede.0000391864.79309.9c
Brown A. P., Cai L., Laufer B. I., Miller L. A., LaSalle J. M., Ji H. (2022). Long-term effects of wildfire smoke exposure during early life on the nasal epigenome in rhesus macaques. Environ. Int. 158, 106993. doi: 10.1016/j.envint.2021.106993
Burke M., Driscoll A., Heft-Neal S., Xue J., Burney J., Wara M. (2021). The changing risk and burden of wildfire in the United States. Proc. Natl. Acad. Sci. U.S.A. 118, e2011048118. doi: 10.1073/pnas.2011048118
Cadaret C. N., Merrick E. M., Barnes T. L., Beede K. A., Posont R. J., Petersen J. L., et al. (2019). Sustained maternal inflammation during the early third-trimester yields intra-uterine growth restriction, impaired skeletal muscle glucose metabolism, and diminished β-cell function in fetal sheep1,2. J. Anim. Sci. 97, 4822–4833. doi: 10.1093/jas/skz321
Camacho L. E., Chen X., Hay W. W., Limesand S. W. (2017). Enhanced insulin secretion and insulin sensitivity in young lambs with placental insufficiency-induced intra-uterine growth restriction. Am. J. Physiol. Regul. Integr. Comp. Physiol. 313, R101–R109. doi: 10.1152/ajpregu.00068.2017
Capitanio J. P., Del Rosso L. A., Gee N., Lasley B. L. (2022). Adverse biobehavioral effects in infants resulting from pregnant rhesus macaques’ exposure to wildfire smoke. Nat. Commun. 13, 1774. doi: 10.1038/s41467-022-29436-9
Chen M., Liang S., Qin X., Zhang L., Qiu L., Chen S., et al. (2018). Prenatal exposure to diesel exhaust PM2.5 causes offspring β cell dysfunction in adulthood. Am. J. Physiology-Endocrinol. Metab. 315, E72–E80. doi: 10.1152/ajpendo.00336.2017
Chen H., Samet J. M., Bromberg P. A., Tong H. (2021). Cardiovascular health impacts of wildfire smoke exposure. Particle. Fiber. Toxicol. 18, 2. doi: 10.1186/s12989-020-00394-8
Christensen R. D., Yoder B. A., Baer V. L., Snow G. L., Butler A. (2015). Early-onset neutropenia in small-for-gestational-age infants. Pediatrics 136, e1259–e1267. doi: 10.1542/peds.2015-1638
Ciriza J., Thompson H., Petrosian R., Manilay J. O., García-Ojeda M. E. (2013). The migration of hematopoietic progenitors from the fetal liver to the fetal bone marrow: Lessons learned and possible clinical applications. Exp. Hematol. 41, 411–423. doi: 10.1016/j.exphem.2013.01.009
Cooke R. F., Arthington J. D. (2013). Concentrations of haptoglobin in bovine plasma determined by ELISA or a colorimetric method based on peroxidase activity. J. Anim. Physiol. Anim. Nutr. 97, 531–536. doi: 10.1111/j.1439-0396.2012.01298.x
Cox B., Gasparrini A., Catry B., Fierens F., Vangronsveld J., Nawrot T. S. (2016). Ambient air pollution-related mortality in dairy cattle: does it corroborate human findings? Epidemiology 27, 779. doi: 10.1097/EDE.0000000000000545
Dabass A., Talbott E. O., Rager J. R., Marsh G. M., Venkat A., Holguin F., et al. (2018). Systemic inflammatory markers associated with cardiovascular disease and acute and chronic exposure to fine particulate matter air pollution (PM2.5) among US NHANES adults with metabolic syndrome. Environ. Res. 161, 485–491. doi: 10.1016/j.envres.2017.11.042
Dado-Senn B., Field S. L., Davidson B. D., Casarotto L. T., Marrero M. G., Ouellet V., et al. (2021). Late-gestation in utero heat stress limits dairy heifer early-life growth and organ development. Front. Anim. Sci. 2. doi: 10.3389/fanim.2021.750390
Davidson B. D., Dado-Senn B., Ouellet V., Dahl G. E., Laporta J. (2021). Effect of late-gestation heat stress in nulliparous heifers on postnatal growth, passive transfer of immunoglobulin G, and thermoregulation of their calves. JDS. Commun. 2, 165–169. doi: 10.3168/jdsc.2020-0069
Deelen S. M., Leslie K. E., Steele M. A., Eckert E., Brown H. E., DeVries T. J. (2016). Validation of a calf-side β-hydroxybutyrate test and its utility for estimation of starter intake in dairy calves around weaning. J. Dairy. Sci. 99, 7624–7633. doi: 10.3168/jds.2016-11097
Deyssenroth M. A., Rosa M. J., Eliot M. N., Kelsey K. T., Kloog I., Schwartz J. D., et al. (2021). Placental gene networks at the interface between maternal PM2.5 exposure early in gestation and reduced infant birthweight. Environ. Res. 199, 111342. doi: 10.1016/j.envres.2021.111342
Dickinson G. N., Miller D. D., Bajracharya A., Bruchard W., Durbin T. A., McGarry J. K. P., et al. (2022). Health risk implications of volatile organic compounds in wildfire smoke during the 2019 FIREX-AQ campaign and beyond. GeoHealth 6, e2021GH000546. doi: 10.1029/2021GH000546
Dikmen S. (2008). The effect of temperature-humidity index (THI) on the dairy cows in summer. J. Dairy Sci. 91, 2624–2633. doi: 10.3168/jds.2008-1072
Draxler R. R. (1999).HYSPLIT4 user’s guide. In: NOAA Tech. Memo. ERL ARL-230 (NOAA Air Resources Laboratory). Available online at: https://www.arl.noaa.gov/wparl/wp-content/uploads/documents/reports/arl-230.pdf (Accessed Jan. 21, 2021).
Draxler R. R., Hess G. D. (1997).Description of the HYSPLIT_4 modeling system. In: NOAA Tech. Memo. ERL ARL-224 (NOAA Air Resources Laboratory). Available online at: https://www.arl.noaa.gov/documents/reports/arl-224.pdf (Accessed Jan. 21, 2021).
Draxler R. R., Hess G. D. (1998). An overview of the HYSPLIT_4 modeling system of trajectories, dispersion, and deposition. Aust. Meteorol. Mag. 47, 295–308.
Egberts V., van Schaik G., Brunekreef B., Hoek G. (2019). Short-term effects of air pollution and temperature on cattle mortality in the Netherlands. Prev. Vet. Med. 168, 1–8. doi: 10.1016/j.prevetmed.2019.03.021
García-Serna A. M., Hernández-Caselles T., Jiménez-Guerrero P., Martín-Orozco E., Pérez-Fernández V., Cantero-Cano E., et al. (2021). Air pollution from traffic during pregnancy impairs newborn’s cord blood immune cells: The NELA cohort. Environ. Res. 198, 110468. doi: 10.1016/j.envres.2020.110468
García-Serna A. M., Martín-Orozco E., Jiménez-Guerrero P., Hernández-Caselles T., Pérez-Fernández V., Cantero-Cano E., et al. (2022). Cytokine profiles in cord blood in relation to prenatal traffic-related air pollution: The NELA cohort. Pediatr. Allergy Immunol. 33, e13732. doi: 10.1111/pai.13732
Hammon H. M., Steinhoff-Wagner J., Schönhusen U., Metges C. C., Blum J. W. (2012). Energy metabolism in the newborn farm animal with emphasis on the calf: endocrine changes and responses to milk-born and systemic hormones. Domest. Anim. Endocrinol. 43, 171–185. doi: 10.1016/j.domaniend.2012.02.005
Hansen C. A., Barnett A. G., Pritchard G. (2008). The effect of ambient air pollution during early pregnancy on fetal ultrasonic measurements during mid-pregnancy. Environ. Health Perspect. 116, 362–369. doi: 10.1289/ehp.10720
Harris L., Taylor A. H. (2015). Topography, fuels, and fire exclusion drive fire severity of the rim fire in an old-growth mixed-conifer forest, yosemite national park, USA. Ecosystems 18, 1192–1208. doi: 10.1007/s10021-015-9890-9
Heaney A., Stowell J. D., Liu J. C., Basu R., Marlier M., Kinney P. (2022). Impacts of fine particulate matter from wildfire smoke on respiratory and cardiovascular health in California. GeoHealth 6, e2021GH000578. doi: 10.1029/2021GH000578
Hocquette J.-F., Sauerwein H., Higashiyama Y., Picard B., Abe H. (2006). Prenatal developmental changes in glucose transporters, intermediary metabolism and hormonal receptors related to the IGF/insulin-glucose axis in the heart and adipose tissue of bovines. Reprod. Nutr. Dev. 46, 257–272. doi: 10.1051/rnd:2006014
Holstius D. M., Reid C. E., Jesdale B. M., Morello-Frosch R. (2012). Birth Weight following Pregnancy during the 2003 Southern California Wildfires. Environ. Health Perspect. 120, 1340–1345. doi: 10.1289/ehp.1104515
Holzhausen E. A., Chalifour B. N., Tan Y., Young N., Lurmann F., Jones D. P., et al. (2024). Prenatal and early life exposure to ambient air pollutants is associated with the fecal metabolome in the first two years of life. Environ Sci Technol. 58, 14121–14134. doi: 10.1021/acs.est.4c02929
Hsiao E. Y., Patterson P. H. (2012). Placental regulation of maternal-fetal interactions and brain development. Dev. Neurobiol. 72, 1317–1326. doi: 10.1002/dneu.22045
Kaur K., Lesseur C., Deyssenroth M. A., Kloog I., Schwartz J. D., Marsit C. J., et al. (2022). PM2.5 exposure during pregnancy is associated with altered placental expression of lipid metabolic genes in a US birth cohort. Environ. Res. 211, 113066. doi: 10.1016/j.envres.2022.113066
Lan Q., Weinberger K., Luke S., Lavigne E., Weichenthal S., Henderson S. B. (2024). Prenatal exposure to wildfire-related PM2.5 and respiratory infections by age 1 year: A population-based case-control analysis of critical developmental windows. ACS EST Air 1, 1483–1494. doi: 10.1101/2023.09.09.23295304. 2023.09.09.23295304.
Laporta J., Fabris T. F., Skibiel A. L., Powell J. L., Hayen M. J., Horvath K., et al. (2017). In utero exposure to heat stress during late gestation has prolonged effects on the activity patterns and growth of dairy calves. J. Dairy. Sci. 100, 2976–2984. doi: 10.3168/jds.2016-11993
Li D., Li Y., Li G., Zhang Y., Li J., Chen H. (2019). Fluorescent reconstitution on deposition of PM2.5 in lung and extrapulmonary organs. Proc. Natl. Acad. Sci. 116, 2488–2493. doi: 10.1073/pnas.1818134116
Li R., Sun Q., Lam S. M., Chen R., Zhu J., Gu W., et al. (2020). Sex-dependent effects of ambient PM2.5 pollution on insulin sensitivity and hepatic lipid metabolism in mice. Part Fiber. Toxicol. 17, 14. doi: 10.1186/s12989-020-00343-5
Limesand S. W., Camacho L. E., Kelly A. C., Antolic A. T. (2018). Impact of thermal stress on placental function and fetal physiology. Anim. Reprod. 15, 886–898. doi: 10.21451/1984-3143-AR2018-0056
Lindower J. B. (2017). Water balance in the fetus and neonate. Semin. Fetal. Neonatal. Med. 22, 71–75. doi: 10.1016/j.siny.2017.01.002
Littell J., McKenzie D., Peterson D., Westerling A. (2009). Climate and wildfire area burned in western U.S. ecoprovinces 1916–2003. Ecol. Appl. 19, 1003–1021. doi: 10.1890/07-1183.1
Ma Y., Zang E., Liu Y., Wei J., Lu Y., Krumholz H. M., et al. (2024). Long-term exposure to wildland fire smoke PM2.5 and mortality in the contiguous United States. Proc. Natl. Acad Sci. USA 121, e2403960121. doi: 10.1073/pnas.2403960121
Maresca S., Lopez Valiente S., Rodriguez A. M., Long N. M., Pavan E., Quintans G. (2018). Effect of protein restriction of bovine dams during late gestation on offspring postnatal growth, glucose-insulin metabolism and IGF-1 concentration. Livestock. Sci. 212, 120–126. doi: 10.1016/j.livsci.2018.04.009
McGuirk S. M. (2008). Disease management of dairy calves and heifers. Vet. Clin. North Am. Food Anim. Pract. 24, 139–153. doi: 10.1016/j.cvfa.2007.10.003
National Interagency Coordination Center (NICC) (2022). Wildland Fire Summary and Statistics Annual Report 2022. Available online at: https://www.nifc.gov/sites/default/files/NICC/2-Predictive%20Services/Intelligence/Annual%20Reports/2022/annual_report.2.pdf (Accessed 16 September, 2024).
O’Donnell M. H., Behie A. M. (2013). Effects of bushfire stress on birth outcomes: A cohort study of the 2009 Victorian Black Saturday bushfires. Int. J. Disaster. Risk Reduction. 5, 98–106. doi: 10.1016/j.ijdrr.2013.08.002
Ollivett T. L., Buczinski S. (2016). On-farm use of ultrasonography for bovine respiratory disease. Vet. Clin. North Am. Food Anim. Pract. 32, 19–35. doi: 10.1016/j.cvfa.2015.09.001
Pace A., K. M. Mirkin, P. Rezamand, A. L. Skibiel (2024). Seeing through the smoke: The effects of wildfire fine particulate matter (PM2.5) exposure on standing and lying behavior in Holstein heifer calves. JDS Commun. 5 (5), 490–494.
Pace A., Villamediana P., Rezamand P., Skibiel A. L. (2023). Effects of wildfire smoke PM2.5 on indicators of inflammation, health, and metabolism of preweaned Holstein heifers. J. Anim. Sci. 101, skad246. doi: 10.1093/jas/skad246
Peek S. F., Ollivett T. L., Divers T. J. (2018). “Respiratory diseases,” in Rebhun’s Diseases of Dairy Cattle (St. Louis, Missouri: Elsevier), 94–167. doi: 10.1016/B978-0-323-39055-2.00004-8
Pessa-Morikawa T., Niku M., Iivanainen A. (2012). Fetal bovine bone marrow is a rich source of CD34+ hematopoietic progenitors with myelo–monocytic colony-forming activity. Dev. Comp. Immunol. 36, 572–577. doi: 10.1016/j.dci.2011.09.014
Quigley J. D., Smith Z. P., Heitmann R. N. (1991). Changes in plasma volatile fatty acids in response to weaning and feed intake in young calves. J. Dairy. Sci. 74, 258–263. doi: 10.3168/jds.S0022-0302(91)78168-X
Skibiel A. L., Peñagaricano F., Amorín R., Ahmed B. M., Dahl G. E., Laporta J. (2018). In utero heat stress alters the offspring epigenome. Sci. Rep. 8, 14609. doi: 10.1038/s41598-018-32975-1
Skibiel A. L., Fabris T. F., Corrá F. N., Torres Y. M., McLean D J., Chapman J. D., et al (2017). Effects of feeding an immunomodulatory supplement to heat-stressed or actively cooled cows during late gestation on postnatal immunity, health, and growth of calves. J. Dairy Sci. 100, 7659–7668.
Stein A. F., Draxler R. R., Rolph G. D., Stunder B. J. B., Cohen M. D., Ngan F. (2015). NOAA’s HYSPLIT atmospheric transport and dispersion modeling system. Bull. Am. Meteorol. Soc 96, 2059–2077. doi: 10.1175/BAMS-D-14-00110.1
Tao S., Monteiro A. P. A., Thompson I. M., Hayen M. J., Dahl G. E. (2012). Effect of late-gestation maternal heat stress on growth and immune function of dairy calves. J. Dairy. Sci. 95, 7128–7136. doi: 10.3168/jds.2012-5697
Taylor R. K., LeMaster C. T., Mangrum K. S., Ricks R. E., Long N. M. (2018). Effects of maternal nutrient restriction during early or mid-gestation without realimentation on maternal physiology and fetal growth and development in beef cattle. Animal 12, 312–321. doi: 10.1017/S175173111700163X
Tinling M. A., West J. J., Cascio W. E., Kilaru V., Rappold A. G. (2016). Repeating cardiopulmonary health effects in rural North Carolina population during a second large peat wildfire. Environ. Health 15, 12. doi: 10.1186/s12940-016-0093-4
Tsai C. Y. (2021). The relationship between nutrient metabolism and health measures during the periparturient period in Pacific Northwest dairy herds. PhD Dissertation. Animal and Veterinary Science, University of Idaho.
United States Environmental Protection Agency (2024). Download Daily Data. Available online at: https://www.epa.gov/outdoor-air-quality-data/download-daily-data (Accessed December 9th, 2024).
USDA National Agricultural Statistics Service (2022). Census of Agriculture, Dairy and Milk Production. ACH22-16. Available online at: https://www.nass.usda.gov/Publications/Highlights/2024/Census22_HL_Dairy.pdf:~:text=California%20led%20the%20country%20in%20both%20milk,cow%20inventory%20and%2053%%20of%20milk%20sales (Accessed 16 September, 2024).
van Leenen K., Jouret J., Demeyer P., Vermeir P., Leenknecht D., Van Driessche L., et al. (2021). Particulate matter and airborne endotoxin concentration in calf barns and their association with lung consolidation, inflammation, and infection. J. Dairy. Sci. 104, 5932–5947. doi: 10.3168/jds.2020-18981
Wathes D. C. (2022). Developmental Programming of fertility in cattle—Is it a cause for concern? Animals 12, 2654. doi: 10.3390/ani12192654
Xu R., Li S., Wu Y., Yue X., Wong E. M., Southey M. C., et al. (2023). Wildfire-related PM2.5 and DNA methylation: An Australian twin and family study. Environ. Int. 171, 107704. doi: 10.1016/j.envint.2022.107704
Yue H., Ji X., Li G., Hu M., Sang N. (2020). Maternal exposure to PM2.5 affects fetal lung development at sensitive windows. Environ. Sci. Technol. 54, 316–324. doi: 10.1021/acs.est.9b04674
Yue H., Ji X., Zhang Y., Li G., Sang N. (2019). Gestational exposure to PM2.5 impairs vascularization of the placenta. Sci. Total. Environ. 665, 153–161. doi: 10.1016/j.scitotenv.2019.02.101
Zhang B., Gong X., Han B., Chu M., Gong C., Yang J., et al. (2022). Ambient PM2.5 exposures and systemic inflammation in women with early pregnancy. Sci. Total. Environ. 829, 154564. doi: 10.1016/j.scitotenv.2022.154564
Zhao N., Qiu J., Ma S., Zhang Y., Lin X., Tang Z., et al. (2018). Effects of prenatal exposure to ambient air pollutant PM10 on ultrasound-measured fetal growth. Int. J. Epidemiol. 47, 1072–1081. doi: 10.1093/ije/dyy019
Zhou S., Li T., Han N., Zhang Y., Chen G., Ji Y., et al. (2022). The associations of prenatal exposure to PM2.5 and its constituents with fetal growth: A prospective birth cohort in Beijing, China. Environ. Res. 214, 114196. doi: 10.1016/j.envres.2022.114196
Keywords: intra-uterine, in utero growth restriction, developmental programming, catch-up growth, air quality
Citation: Pace A, Villamediana P, Rovai M, Ponce G, Ellis N, Stegeman A, Larson M, Konetchy DE, Rezamand P and Skibiel AL (2025) Prenatal wildfire smoke PM2.5 exposure has carryover effects on the postnatal calf. Front. Anim. Sci. 6:1580654. doi: 10.3389/fanim.2025.1580654
Received: 20 February 2025; Accepted: 14 April 2025;
Published: 20 May 2025.
Edited by:
Vishwajit S. Chowdhury, Kyushu University, JapanReviewed by:
Hatem Eltahan, Agricultural Research Center, EgyptOlajide Mark Sogunle, Federal University of Agriculture, Abeokuta, Nigeria
Copyright © 2025 Pace, Villamediana, Rovai, Ponce, Ellis, Stegeman, Larson, Konetchy, Rezamand and Skibiel. This is an open-access article distributed under the terms of the Creative Commons Attribution License (CC BY). The use, distribution or reproduction in other forums is permitted, provided the original author(s) and the copyright owner(s) are credited and that the original publication in this journal is cited, in accordance with accepted academic practice. No use, distribution or reproduction is permitted which does not comply with these terms.
*Correspondence: Amy L. Skibiel, YXNraWJpZWxAdWlkYWhvLmVkdQ==