- 1Department of Materials, Loughborough University, Loughborough, United Kingdom
- 2Department of Civil Engineering, University of Sheffield, Sheffield, United Kingdom
Surface properties of biomaterials, such as chemistry and morphology, have a major role in modulating cellular behavior and therefore impact on the development of high-performance devices for biomedical applications, such as scaffolds for tissue engineering and systems for drug delivery. Opportunely-designed micro- and nanostructures provides a unique way of controlling cell-biomaterial interaction. This mini-review discusses the current research on the use of electrospinning (extrusion of polymer nanofibers upon the application of an electric field) as effective technique to fabricate patterns of micro- and nano-scale resolution, and the corresponding biological studies. The focus is on the effect of morphological cues, including fiber alignment, porosity and surface roughness of electrospun mats, to direct cell migration and to influence cell adhesion, differentiation and proliferation. Experimental studies are combined with computational models that predict and correlate the surface composition of a biomaterial with the response of cells in contact with it. The use of predictive models can facilitate the rational design of new bio-interfaces.
Introduction
The natural regeneration process of human tissues is strongly regulated by the interaction of cells with the extracellular matrix (ECM) (Lutolf and Hubbell, 2005; Liu and Wang, 2014). ECM is a dynamic and complex fibrous network of proteins and polysaccharides, such as collagen, elastin, fibronectin, laminin, proteoglycans and glycosaminoglycans. Cells interact with ECM by transmembrane receptors, known as integrins, that ligate with specific motifs of ECM proteins, for example arginine, glycine and arginylglycylaspartic acid (RGD) peptides (Anderson et al., 2016; Dalby et al., 2018). Cells continuously remodel the ECM environment, which, in turn, influences cell behavior and fate (differentiation, proliferation and migration) by biochemical, physical and mechanical signals (Geiger et al., 2001), and provides structural support to cells. Recent studies have investigated the effects of ECM physical properties, particularly porosity, topography and hierarchical 3D architecture, on cellular functions, and extrapolated rules to design structures for effective tissue regeneration (Li et al., 2017; Marino et al., 2017; Lin et al., 2018).
One of the technologies that is widely used to produce ECM-mimicking structures and particularly to replicate the fibrillar architecture of ECM is electrospinning (Khorshidi et al., 2016). The electrospinning technique allows the production of networks of fibers with a diameter in the range of few nm to few μm via the application of electrical forces to polymer solutions or melts (Bhardwaj and Kundu, 2010; Mele, 2016; Zhang et al., 2016). Structural modifications of electrospun nanofibres, such as altering topographical characteristics and inducing porosity, can be achieved by controlling and varying the process parameters (polymer concentration, applied voltage, evaporation rate of the solvent used). Similarly, changes to the final makeup of the fibrous network, such as alignment and patterning of fibers, can be obtained by modifications of the electrospinning apparatus or post-processing.
This mini review analyses a selection of recent works on the use of solution electrospinning to create nanofibres with engineered surface topography (random, aligned and patterned fibers) for controlling adhesion, differentiation, and migration of different cells lines. The mini review is divided in two main sections: the first one will focus on experimental studies on electrospun fibers that provide physical cues for cell growth and differentiation; the second section will discuss computational models to predict cell behavior on micropatterns. Although mathematical models that simulate cell behavior on electrospun fibers are not currently available, the computational approaches here discussed can be adapted, in the future, to electrospun scaffolds and used to elucidate the underlying mechanisms responsible for cell-fiber interaction.
Effects of FIBER Topography and Micro-Patterning on Cellular Response
Multiple studies have demonstrated that the morphology and roughness of fibers produced by electrospinning influence cell adhesion, proliferation, and orientation (Sill and von Recum, 2008; Xie et al., 2008; Bergmeister et al., 2013; Cirillo et al., 2014; Zhu et al., 2015; Sun et al., 2018). All factors that are imperative for successful tissue regeneration (Agarwal et al., 2008). Cells can sense topographical structures on a surface by filipodia that are actin-rich protrusions (0.1–0.3 μm in diameter) of the cell membrane and are involved in cell contact guidance (Mattila and Lappalainen, 2008; Dalby et al., 2014). If nanoscale aligned features are present onto a surface, filopodia tend to orient along the direction of the features and determine cytoskeleton orientation. Focal adhesions at the cell membrane mediate the initial cell-biomaterial interaction, with integrin ligands in direct contact with the substrate and connected to the actin micro-filaments of the cell cytoskeleton by a 40-nm stratum, which includes focal adhesion kinase (FAK), paxillin, talin, and vinculin (Kanchanawong et al., 2010).
This section of the review will discuss how electrospun mats with controlled porosity and surface morphology have been used to influence the behavior of mesenchymal stem cells (MSCs) (Jiang et al., 2015; Yin et al., 2015; Baudequin et al., 2017; Lin et al., 2017; Liu et al., 2017; Nedjari et al., 2017; Su et al., 2017; Zhang et al., 2017; Ghosh et al., 2018; Jin et al., 2018; Rahman et al., 2018; Sankar et al., 2018) and human umbilical vein endothelial cells (HUVECs) (Fioretta et al., 2014; Xu et al., 2015; Shin et al., 2017; Taskin et al., 2017; Yan et al., 2017; Ahmed et al., 2018). The literature on other cell lines, such as on myoblasts (Mele et al., 2015; Jun et al., 2016; Park et al., 2016; Tallawi et al., 2016; Abarzúa-Illanes et al., 2017; Yang et al., 2017) and neuron-like cells (Binan et al., 2014; Xie et al., 2014; Malkoc et al., 2015; Xue et al., 2017; Hajiali et al., 2018; Xia and Xia, 2018), will not be analyzed in detail here but a summary of it is reported in Table 1.
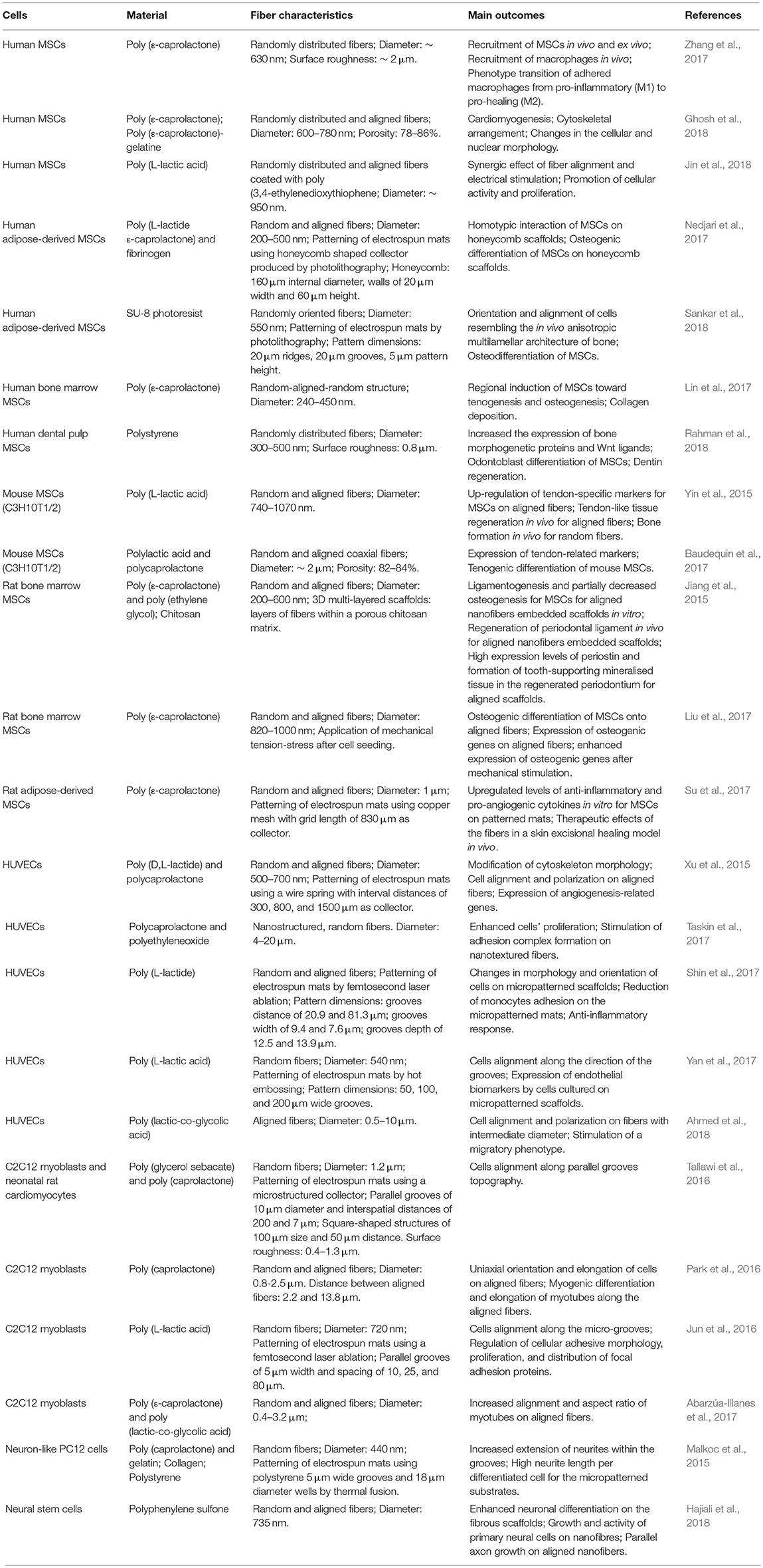
Table 1. Summary of the recent literature on the use of electrospun fibers to control morphology, alignment and differentiation of diverse cell lines.
Mesenchymal Stem Cells
MSCs are multipotent stem cells that are primarily isolated from bone marrow, but they can also be found in adipose tissue, dental pulp, placenta, umbilical cord and other vascularized tissues throughout the body (Lv et al., 2014; Tartarini and Mele, 2015). MSCs are of great interest in regenerative medicine, because of their therapeutic effects, such as: ability to differentiate into various cell types and therefore promote regeneration of a wide range of tissues (bone, cartilage, muscle, marrow, tendon, ligament, nervous tissue, and skin); secretion of bioactive molecules for tissue repair; migration to inflamed tissues and modulation of local inflammation; immunomodulatory functions (Sharma et al., 2014).
In a recent research, Zhang et al. have studied how the topography and fibrillar organization of electrospun poly (ε-caprolactone) (PCL) fibers influences the recruitment of MSCs in vivo and ex vivo (Zhang et al., 2017). PCL mats (randomly distributed fibers) were implanted into the subcutaneous tissue of rats and the results were compared with solid PCL films (not electrospun). It was observed that, during the initial post-implantation period (1 day), a great number of macrophages with M1 phenotype (pro-inflammatory) were recruited to the PCL fibers, differently from solid PCL. This was attributed to the high surface area of the fibers and the porosity of the electrospun mats that promoted protein adsorption from the surrounding tissue, such as complement C3a (a chemo-attractant responsible to activate and recruit immune cells), fibronectin and vitronectin. After 4 and 7 days of implantation, the PCL fibers attracted host MSCs and modulated macrophages polarization with an increased number of cells exhibiting M2 (pro-healing) phenotype. While the number of M2 cells continuously increased over the entire period of implantation for PCL fibers, this was not the case for solid PCL where a large population of M1 cells was retained. Migration of MSCs was also observed in ex vivo experiments conducted with the implanted PCL samples. It was found that the macrophages at the implanted PCL mats secreted high levels of SDF-1, a chemokine that mediates MSCs recruitment by interacting with CXC chemokine receptors on the MSCs membrane. The study concluded that the physical organization of the PCL electrospun network induced the phenotype M1-to-M2 transition of macrophages that attracted MSCs at the implantation site by releasing SDF-1. This cascade of events was beneficial to stimulate tissue repair. PCL electrospun fibers have been used also to stimulate the production of pro-angiogenic and anti-inflammatory paracrine factors in rat adipose-derived MSCs (Ad-MSCs) (Su et al., 2017) and in skin excisional wound-healing model in rats (Table 2). Ad-MSCs were seeded on three types of electrospun PCL fibers, random (REF), aligned (AEF) and with a mesh pattern (MEF). It was observed that scaffolds with oriented fibers (AEF and MEF) promoted the expression of PGE2 (Prostaglandin E2, a potent inflammatory mediator), iNOS (inducible Nitric Oxide Synthase), VEGF (vascular endothelial growth factor) and HGF (hepatocyte growth factor), compared to REF scaffolds. In order to elucidate the molecular signaling mechanism responsible for the paracrine secretion of Ad-MSCs, the cells were treated with an inhibitor of NF-kB (a transcription factor that induces the expression of pro-inflammatory genes) and this significantly reversed the paracrine response of MSCs to the electrospun scaffolds. The authors therefore speculated that, in the presence of the scaffolds, MSCs behaved as if they were exposed to an external inflammatory stimulus. Similar results have been recently reported for MSCs cultured on electrospun fibers of PCL/polytetrahydrofuran (PTHF) urethane (P fibers) and PCL-PTHF urethane/collagen I (PC fibers) (Jiang et al., 2018). In this case, down-regulation of genes that contribute to inflammation and suppression of the NF-kB pathway signaling pathway were achieved by changing the mechanical properties of the fibers. PC fibers with a Young's modulus of 4.3 MPa were able to suppress inflammation, differently from P fibers (Young's modulus of 6.8 MPa).
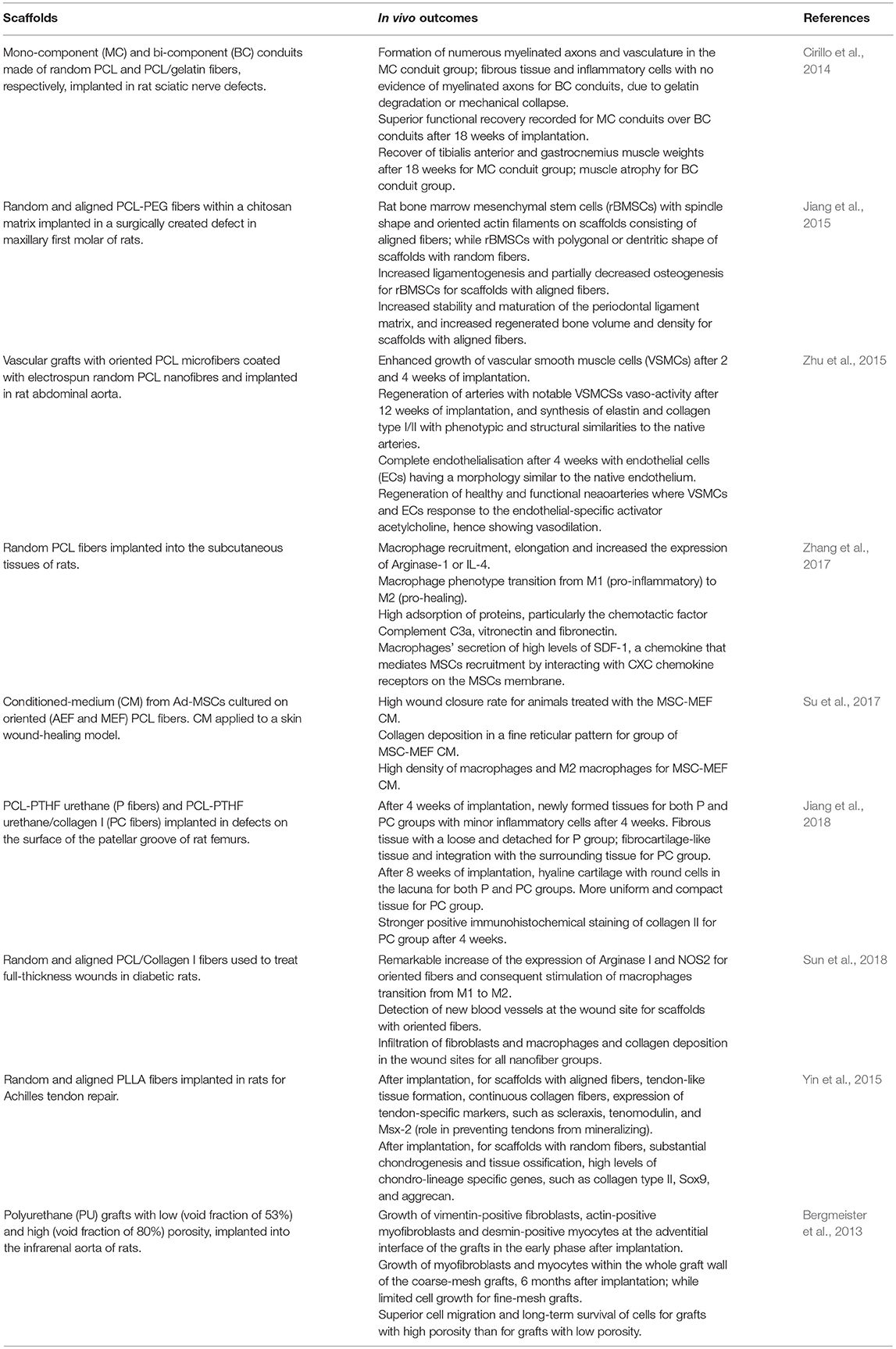
Table 2. Summary of main results reported in selected recent papers on electrospun scaffolds used in vivo experiments.
Another study has investigated how electrospun PCL scaffolds with a novel random-aligned-random structure can be used to mimic bone-ligament connections and native ligaments (Lin et al., 2017). The authors designed a fiber collecting device for the fabrication of electrospun scaffolds with a controlled spatial distribution of random and aligned fibers. The regions of the scaffold with random fibers were then mineralized with Ca-P. In vitro tests on human bone marrow MSCs (hBMSCs) revealed that fiber anisotropy modified cells' morphology: polygonal, round-shaped cells without alignment were detected in the random, mineralized regions of the scaffold; while elongated spindle-shaped cells aligned along the fiber direction were visible in the aligned region. Moreover, the aligned fibers significantly up-regulated tendon-specific and tendon-related markers (Tnmd, Mkx) and therefore guided tenogenic phenotypes of hBMSCs; while, the regions with random, mineralized fibers determined the expression of bone-specific markers (Runx-2, Ocn, Opn) and consequently hBMSCs osteogenic phenotypes. Although the authors have not elucidated the underlying cell signaling mechanisms, this work demonstrates that electrospun scaffolds with engineered fiber anisotropy are advantageous to achieve region-specific distribution of tendon- and bone-related genes and find potential application in ligament repair and regeneration of bone-ligament connections.
The possibility to mediate the expression of signaling biomolecules by electrospun fibers and hence guide MSCs differentiation has been demonstrated also by Rahman and co-workers (Rahman et al., 2018). They investigated the odontoblastic differentiation of human dental pulp MSCs (DP-MSCs) on polystyrene (PS) random fibers. The cells cultured on PS mats strongly increased the expression of bone morphogenetic proteins (BMPs) and Wnt ligands that are essential in tooth development: Wnt3a transcript expression was more than 50 folds higher after 4 days of culturing on PS fibers than on standard petri dishes. The levels of odontoblast/osteoblast markers, such as dentin sialophosphoprotein (DSPP), osteocalcin, and bone sialoprotein, were also higher for DP-MSCs cultured on electrospun fibers. The results of this study indicate that nanofibres mimicking the in vivo microenvironment are crucial to stimulate the differentiation of DP-MSCs into odontoblasts (specialized cells responsible for dentin formation) by mediating the production of signaling molecules including Wnt3a, and to promote dentinogenesis. Osteogenesis of MSCs has been reported also on random Poly-L-lactic acid (PLLA) fibers, due to cytoskeletal rearrangements and tensions, which in turn influence intracellular mechanotransductive pathways (Yin et al., 2015). In fact, when the cells were treated with Rho kinase (ROCK) inhibitor Y-27632 (inhibitor of myosin-generated cytoskeletal tension), loss of lineage commitment was detected, and cells' morphology was not affected by the fibers topography.
The works here summarized and the others conducted on the interaction of MSCs with electrospun substrates (Tables 1, 2) demonstrate that networks of polymer fibers (random, aligned and hierarchical) are effective in providing topographical and physical cues to guide differentiation of stem cells. These observations have led to the development of bioinspired scaffolds with potential future implications in diverse clinical areas, including the regeneration and repair of bone, tendon, ligament, dentin, and skin.
Human Umbilical Vein Endothelial Cells
Vascular endothelial cells are of fundamental importance for the entire circulatory system, because they are involved in fluid filtration, homeostasis and prevention of thrombosis (Rajendran et al., 2013). Endothelial cells and particularly HUVECs, which are isolated from human umbilical cord veins, are widely used to study cardiovascular diseases and develop biomedical devices for vascular tissue engineering (Lei et al., 2016). One important aspect to consider when designing scaffolds for endothelial cells is the role played by surface topography, at micro- and nano-scale, on cell adhesion, proliferation and migration, to create a physiological environment that stimulates the formation of a functional endothelium.
A recent work of Ahmed and co-workers has reported on the influence of the diameter of electrospun fibers on HUVECs migration (Ahmed et al., 2018). Aligned poly(lactic-co-glycolic acid) (PLGA) fibers with five different diameters (0.5, 1, 2, 4, and 10 μm) were analyzed. The greater cell displacement in a scratch wound assay was measured for HUVECs seeded on fibers with intermediate diameter (1 and 2 μm) with peak migration velocity of 24 μm/h after 12 h of cell culture. HUVECs were able also to move on scaffolds with 0.5 μm size fibers but at lower migration rates. On these scaffolds cell alignment and polarization, and higher levels of FAK expression were detected. FAK is a non-receptor tyrosine kinase that regulates cell shape, adhesion and motility. The fiber diameter influenced the focal adhesion of HUVECs but not their metabolism or the formation of cell-matrix anchorage points. At 12 h, a significant increase in phosphorylated FAK (pFAK, associated with actin regulation and adhesion dynamics) was detected, which is linked to the peak migration velocity. On the contrary, limited cell motility was observed for scaffolds with 4 and 10 μm fibers. Investigations of the spatial distribution of pFAK revealed that pFAK was localized in the HUVECs cytosol for 0.5, 1.0, and 2.0 μm fibers, and at the cell periphery for 4 and 10 μm fibers (non-uniform distribution). This promoted uniaxial cell morphology and stimulated the migratory process to occur preferentially along the fiber longitudinal direction for scaffolds with intermediate fiber diameter. A similar conclusion has been drawn by other researchers working on HUVECs cultured on micropatterned scaffolds with spatially heterogeneous alignment of poly(D,L-lactide) (PDLLA)/PCL electrospun fibers of 0.5–1 μm size (Xu et al., 2015). Fibrous scaffolds with patterns of random and well-aligned PDLLA/PCL fibers were prepared using a wire spring as template collector. It was observed that the micropatterned scaffolds induced the proliferation of HUVECs and modifications to their cytoskeleton morphology (Figure 1). The lowest values of mean cell body shape index (a parameter indicating the degree of cell polarization) were measured for cells cultured on patterned scaffolds having the longest distance (1,500 μm) between regions with random and aligned fibers, indicating the highest degree of cell polarization and alignment. Furthermore, those scaffolds stimulated the cells to express high levels of angiogenesis-related genes and therefore they have potential applications in vascular tissue engineering. The combination of electrospinning and micro-pattering techniques has proven to be effective for creating hierarchical bio-interfaces that direct the arrangement of endothelial cells and their biological functions (Shin et al., 2017; Yan et al., 2017).
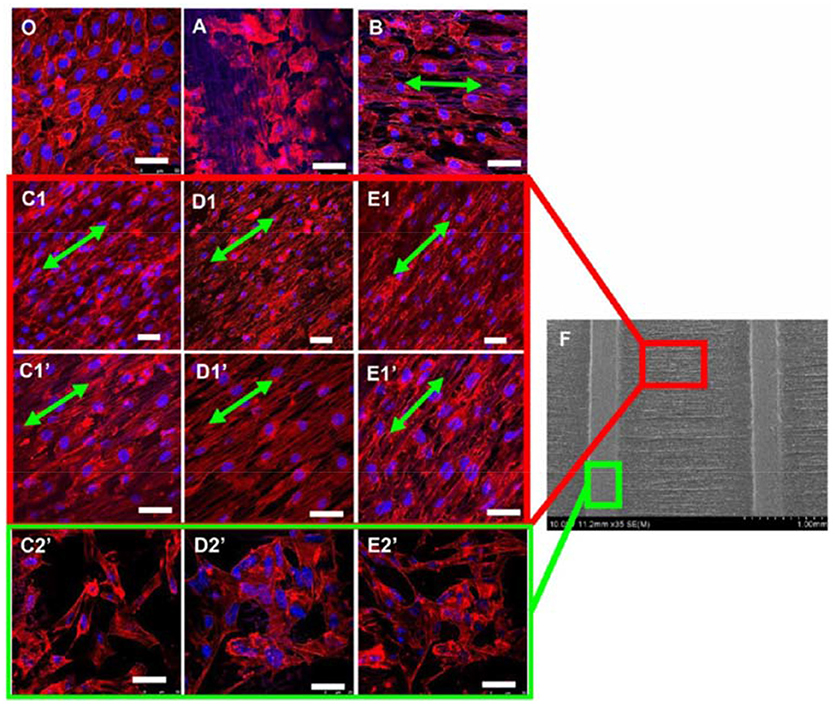
Figure 1. Confocal images of HUVECs (actin filaments in red and nuclei in blue) cultured for 7 days on (O) standard petri dish (typical cobblestone-like structure) and electrospun scaffolds with different patterns: (A) nonwoven (cells with flat, round shape morphology); (B) single directionally aligned pattern (cell alignment along fibers direction, green arrows); (C1–E1) anisotropic aligned patterns with interval distances of 300, 800, and 1500 μm, respectively; (C1′-E1′) anisotropic aligned patterns with interval distances of 300, 800, and 1500 μm (high magnification images), respectively (spindle shape along the long fiber axes for cells between the embossments) (C2′- E2′) anisotropic aligned patterns with interval distances of 300, 800, and 1500 μm, respectively (polygonal shape with random stretching for cells on the embossments). (F) SEM image of anisotropic aligned pattern. Scale bar = 50 μm. Reprinted with permission from Xu et al. (2015). Copyright 2018 of the American Chemical Society.
Computational Models
The literature that has been discussed so far in this review provides experimental evidences that the surface topography of biomaterials influences cellular behavior, including cells' alignment, elongation, migration, phenotype transitions and differentiation. In vitro and in vivo studies are incredibly beneficial to collect data and results on how artificially created micro- and nano-features perform in realistic applications (Tables 1, 2). The underlying mechanisms of cell-material interactions are only partially understood and further investigations are required to define the best scaffold design for promoting the regeneration of a target tissue (Kennedy et al., 2017; Paim et al., 2018). However, time and cost requirements for in vitro and in vivo tests pose limitations on the use of and reliance on experimental studies alone, together with ethical issues when animal models are concerned. Computational modeling has the significant advantage of facilitating research by conducting thousands of simulated trials with a wide range of variations and for a plethora of complex biological systems (Geris et al., 2018).
Albert and Schwarz have developed mathematical models to predict the dynamics of cell shape and forces on micropatterned substrates (Albert and Schwarz, 2014, 2016a,b). Their models are based on the cellular Potts model (CPM) that allows to simulate the behavior of single or interacting cells by describing them as internally structureless but spatially extended objects on a regular lattice (Voss-Böhme, 2012; Tartarini and Mele, 2015). The number of lattice sites belonging to a single cell defines the area occupied by the cell. By changing the lattice resolution and the indices of the lattice sites, cells with arbitrary shape and shape evolutions can be represented. Initially, the authors compared simulations with experimental data on single cell attached on crossbow, Y and H patterns (Albert and Schwarz, 2014). The model well described how the cell contour adapted to the pattern's geometry and reconstructed the traction forces in agreement with experiments. The forces were higher at the extremities of the patterns (adhesive edges of the contour) and increased with the curvature of the contour depending on the availability of receptors for focal adhesion. The CPM-based model was then used to predict the collective behavior of cells on micropatterns, including cell division, cell-cell contacts and migration (Albert and Schwarz, 2014). The model predicted, for example, that for a cell dividing on a L shaped pattern, the two daughter cells were most likely to be located on the two arms of the L, as confirmed by experimental results. In order to identify the optimal adhesive patterns to control cell functions, CPM was combined with genetic algorithms (GAs) (Albert and Schwarz, 2016c) (Figure 2), which are computational techniques inspired by natural evolution for the heuristic search of problem solutions (McCall, 2005). The migration of cells on rachet micropatterns in a linear arrangement was analyzed and the algorithm predicted that a triangular shape was ideal to guide cell migration in the direction of the tip of the triangle, as also demonstrated experimentally. Differently from what expected though, the most effective pattern to achieve unidirectional migration of cells consisted of asymmetric triangles that were rotated and connected to one another to form a pattern with an almost straight horizontal edge. The computational model developed is a useful tool to predict cell interactions with structured scaffolds and it can be adapted to simulate diverse cellular processes.
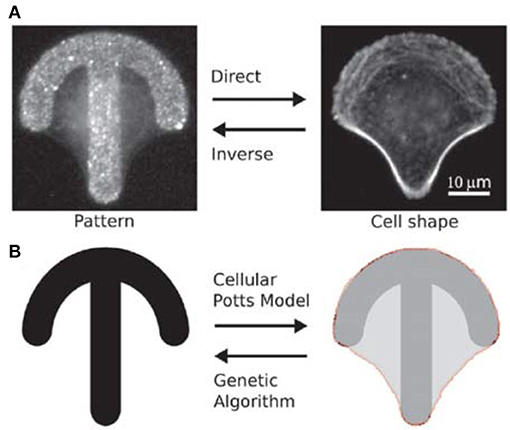
Figure 2. (A) Fluorescence images of a HeLa cell stained for actin on a crossbow microstructure coated with fibronectin. Given a micro-pattern, cell shape can be observed with optical microscopy (direct problem). Give a cell shape, it is not always straightforward to experimentally identify the original pattern (inverse problem). (B) CPM can be used to predict cell shape on a microstructure, and genetic algorithms can help to define pattern geometry. Reproduced with permission from Albert and Schwarz (2016c). Copyright 2016 of the Royal Society of Chemistry.
With a distinct lack of literature on computational/numerical modeling that predicts how cells interact with electrospun nanofibrous structures (role of roughness and topography), there is a clear gap in the field which has great potential if correctly pursued. This will open even more possibilities to design and create novel fibrous scaffolds with engineered surface structures (Ziebert and Aranson, 2016). For example, computer aided characterization of complex biointerfacial interactions of specific polymer fibers could be created. Computational algorithms and numerical solutions could be formulated to generate a method of predicting the most suitable surface topography of electrospun mats for specific cells and to prompt tissue regeneration processes. In the development of computational models that describe how cell behavior is affected by the surface properties of electrospun scaffolds, geometrical parameters to be considered include fibers diameter, fibers organization and degree of alignment, porosity of the mat, presence of nanostructures or nanopores on single fiber surface, overall roughness of the electrospun mat. All these aspects have been evaluated experimentally, as discussed in the previous section of this mini review.
Conclusions
Electrospun nanofibers have become vital structures for a plethora of different applications, with the field of biomedical engineering being, arguably, one of the most important. Thanks to the versatility of electrospinning, nanofibrous scaffolds can be tailored and modified to improve their biocompatibility for applications such as tissue engineering, drug delivery and wound dressings. For example, electrospun mats have been used in clinical studies for the treatment of arterial occlusive disease, skin cancer and diabetic foot (Table 3). As discussed in this review, fiber alignment, micropatterning, and controlled porosity of nanofibrous mats have all been found to have significant effect on cellular behavior, inducing cell attachment, migration and differentiation. Extensive research has been conducted on exploring morphological cues provided by 2D electrospun mats, and only recently fibrous 3D scaffolds have been proposed to closely mimic the ECM structure (Cai et al., 2013; Lee et al., 2014; Cho et al., 2016; Hwang et al., 2018; Unnithan et al., 2018). The studies conducted so far have demonstrated that a fine tuning of the 3D porosity of the electrospun scaffolds is crucial to promote cell infiltration. Future research in the field should combine experimental studies with numerical and computational modeling for the design and fabrication of novel micro- and nanostructured 3D scaffolds. Computer aided simulations could not only be used to predict cell interaction with specific topography but be formulated in a manner which then advises on the most suitable functional group (or biological molecule) that ought to be immobilized on the surface or embedded within the scaffold. This would require taking in consideration a complex combination of parameters that include the chemical composition of the scaffold (exposed chemical groups, wetting properties, and biodegradation), micro- and nano-porosity, organization of the fibrous network (random or aligned fibers) and mechanical properties of the scaffold.
Author Contributions
AD and EM contributed to the conception and design of the study. AD and EM wrote the first draft of the manuscript. DT wrote sections of the manuscript. All authors contributed to manuscript revision, read and approved the submitted version.
Conflict of Interest Statement
The authors declare that the research was conducted in the absence of any commercial or financial relationships that could be construed as a potential conflict of interest.
References
Abarzúa-Illanes, P. N., Padilla, C., Ramos, A., Isaacs, M., Ramos-Grez, J., Olguín, H. C., et al. (2017). Improving myoblast differentiation on electrospun poly(ε-caprolactone) scaffolds. J. Biomed. Mater. Res. A. 105, 2241–2251. doi: 10.1002/jbm.a.36091
Agarwal, S., Wendorff, J., and Greiner, A. (2008). Use of electrospinning technique for biomedical applications. Polymer 49, 5603–5621. doi: 10.1016/j.polymer.2008.09.014
Ahmed, M., Ramos, T., Wieringa, P., Blitterswijk, C. V., Boer, J., and Moroni, L. (2018). Geometric constraints of endothelial cell migration on electrospun fibres. Sci. Rep. 8:6386. doi: 10.1038/s41598-018-24667-7
Albert, P. J., and Schwarz, U. S. (2014). Dynamics of cell shape and forces on micropatterned substrates predicted by a cellular Potts model. Biophys. J. 106, 2340–2352. doi: 10.1016/j.bpj.2014.04.036
Albert, P. J., and Schwarz, U. S. (2016a). Modeling cell shape dynamics on micropatterns. Cell Adh. Migrat. 10, 516–528. doi: 10.1080/19336918.2016.1148864
Albert, P. J., and Schwarz, U. S. (2016b). Dynamics of cell ensembles on adhesive micropatterns: bridging the gap between single cell spreading collective cell migration. PLoS Comput. Biol. 12:e1004863. doi: 10.1371/journal.pcbi.1004863
Albert, P. J., and Schwarz, U. S. (2016c). Optimizing micropattern geometries for cell shape migration with genetic algorithms. Integr. Biol. 11, 741–750. doi: 10.1039/c6ib00061d
Anderson, H. J., Sahoo, J. K., Ulijn, R. V., and Dalby, M. J. (2016). Mesenchymal stem cell fate: applying biomaterials for control of stem cell behaviour. Front. Bioeng. Biotechnol. 4:38. doi: 10.3389/fbioe.2016.00038
Baudequin, T., Gaut, L., Mueller, M., Huepkes, A., Glasmacher, B., Duprez, D., et al. (2017). The osteogenic and tenogenic differentiation potential of C3H10T1/2 (mesenchymal stem cell model) cultured on PCL/PLA electrospun scaffolds in the absence of specific differentiation medium. Materials 10:E1387. doi: 10.3390/ma10121387
Bergmeister, H., Schreiber, C., Grasl, C., Walter, I., Plasenzotti, R., Stoiber, M., et al. (2013). Healing characteristics of electrospun polyurethane grafts with various porosities. Acta Biomater. 9, 6032–6040. doi: 10.1016/j.actbio.2012.12.009
Bhardwaj, N., and Kundu, S. C. (2010). Electrospinning: a fascinating fiber fabrication technique. Biotechnol. Adv. 28, 325–347. doi: 10.1016/j.biotechadv.2010.01.004
Binan, L., Tendey, C., De Crescenzo, G., El Ayoubi, R., Ajji, A., and Jolicoeur, M. (2014). Differentiation of neuronal stem cells into motor neurons using electrospun poly-L-lactic acid/gelatin scaffold. Biomater 35, 664–674. doi: 10.1016/j.biomaterials.2013.09.097
Cai, S., Xu, H., Jiang, Q., and Yang, Y. (2013). Novel 3D electrospun scaffolds with fibers oriented randomly and evenly in three dimensions to closely mimic the unique architectures of extracellular matrices in soft tissues: fabrication and mechanism study. Langmuir 29, 2311–2318. doi: 10.1021/la304414j
Cho, M., Kim, S. H., Jin, G., Park, K. I., and Jang, J. H. (2016). Salt-induced electrospun patterned bundled fibers for spatially regulating cellular responses. ACS Appl. Mater. Interfaces 8, 13320–13331. doi: 10.1021/acsami.6b03848
Cirillo, V., Clements, B. A., Guarino, V., Bushman, J., Kohn, J., and Ambrosio, L. (2014). A comparison of the performance of mono- and bi-component electrospun conduits in a rat sciatic model. Biomaterials 35, 8970–8982. doi: 10.1016/j.biomaterials.2014.07.010
Dalby, M. J., Gadegaard, N., and Oreffo, R. O. C. (2014). Harnessing nanotopography and integrin–matrix interactions to influence stem cell fate. Nat. Mater. 13, 558–569. doi: 10.1038/nmat3980
Dalby, M. J., García, A. J., and Salmeron-Sanchez, M. (2018). Receptor control in mesenchymal stem cell engineering. Nat. Rev. Mater. 3:17091. doi: 10.1038/natrevmats.2017.91
Fioretta, E. S., Simonet, M., Smits, A. I., Baaijens, F. P., and Bouten, C. V. (2014). Differential response of endothelial and endothelial colony forming cells on electrospun scaffolds with distinct microfiber diameters. Biomacromolecules 15, 821–829. doi: 10.1021/bm4016418
Geiger, B., Bershadsky, A., Pankov, R., and Yamada, K. M. (2001). Transmembrane extracellular matrix-cytoskeleton crosstalk. Nat. Rev. Molec. Cell Biol. 2, 793–805. doi: 10.1038/35099066
Geris, L., Lambrechts, T., Carlier, A., and Papantoniou, I. (2018). The future is digital: in silico tissue engineering. Curr. Opinion Biomed. Eng. 6, 92–98. doi: 10.1016/j.cobme.2018.04.001
Ghosh, L. D., Jain, A., Sundaresan, N. R., and Chatterjee, K. (2018). Elucidating molecular events underlying topography mediated cardiomyogenesis of stem cells on 3D nanofibrous scaffolds. Mater. Sci. Eng. C 88, 104–114. doi: 10.1016/j.msec.2018.03.012
Hajiali, H., Contestabile, A., Mele, E., and Athanassiou, A. (2018). Influence of topography of nanofibrous scaffolds on functionality of engineered neural tissue. J. Mater. Chem. B 6, 930–939. doi: 10.1039/C7TB02969A
Hwang, T. I., Maharjan, B., Tiwari, A. P., Lee, S., Joshi, M. K., Park, C. H., et al. (2018). Facile fabrication of spongy nanofibrous scaffold for tissue engineering applications. Mater. Lett. 219, 119–122. doi: 10.1016/j.matlet.2018.02.040
Jiang, T., Kai, D., Liu, S., Huang, X., Heng, S., Zhao, J., et al. (2018). Mechanically cartilage-mimicking poly(PCL-PTHF urethane)/collagen nanofibers induce chondrogenesis by blocking NF-kappa B signalling pathway. Biomaterials 178, 281–292. doi: 10.1016/j.biomaterials.2018.06.023
Jiang, W., Li, L., Zhang, D., Huang, S., Jing, Z., Wu, Y., et al. (2015). Incorporation of aligned PCL-PEG nanofibers into porous chitosan scaffolds improved the orientation of collagen fibers in regenerated periodontium. Acta Biomater. 25, 240–252. doi: 10.1016/j.actbio.2015.07.023
Jin, L., Hu, B., Li, Z., Li, J., Gao, Y., Wang, Z., et al. (2018). Synergistic effects of electrical stimulation and aligned nanofibrous microenvironment on growth behavior of mesenchymal stem cells. ACS Appl. Mater. Interfaces 10, 18543–18550. doi: 10.1021/acsami.8b04136
Jun, I., Chung, Y. W., Heo, Y. H., Han, H. S., Park, J., Jeong, H., et al. (2016). Creating hierarchical topographies on fibrous platforms using femtosecond laser ablation for directing myoblasts behaviour. ACS Appl. Mater. Interfaces 8, 3407–3417. doi: 10.1021/acsami.5b11418
Kanchanawong, P., Shtengel, G., Pasapera, A. M., Ramko, E. B., Davidson, M. W., Hess, H. F., et al. (2010). Nanoscale architecture of integrin-based cell adhesions. Nature 468, 580–584. doi: 10.1038/nature09621
Kennedy, K. M., Bhaw-Luximon, A., and Jhurry, D. (2017). Cell-matrix mechanical interaction in electrospun polymeric scaffolds for tissue engineering: implications for scaffold design and performance. Acta Biomater. 50, 41–55. doi: 10.1016/j.actbio.2016.12.034
Khorshidi, S., Solouk, A., Mirzadeh, H., Mazinani, S., Lagaron, J. M., Sharifi, S., et al. (2016). A review of key challenges of electrospun scaffolds for tissue engineering applications. J. Tissue Eng. Regen. Med. 10, 715–738. doi: 10.1002/term.1978
Lee, S., Cho, S., Kim, M., Jin, G., Jeong, U., and Jang, J. H. (2014). Highly moldable electrospun clay-like fluffy nanofibers for three-dimensional scaffolds. ACS Appl. Mater. Interfaces 6, 1082–1091. doi: 10.1021/am404627r
Lei, J., Peng, S., Samuel, S. B., Zhang, S., Wu, Y., Wang, P., et al. (2016). A simple and biosafe method for isolation of human umbilical vein endothelial cells. Anal. Biochem. 508, 15–18. doi: 10.1016/j.ab.2016.06.018
Li, Y., Xiao, Y., and Liu, C. (2017). The horizon of materiobiology: a perspective on material-guided cell behaviours and tissue engineering. Chem. Rev. 117, 4376–4421. doi: 10.1021/acs.chemrev.6b00654
Lin, J., Zhou, W., Han, S., Bunpetch, V., Zhao, K., Liu, C., et al. (2018). Cell-material interactions in tendon tissue engineering. Acta Biomater. 70, 1–11. doi: 10.1016/j.actbio.2018.01.012
Lin, Z., Zhao, X., Chen, S., and Du, C. (2017). Osteogenic and tenogenic induction of hBMSCs by an integrated nanofibrous scaffold with chemical and structural mimicry of the bone-ligament connection. J. Mater. Chem. B 5, 1015–1027. doi: 10.1039/C6TB02156E
Liu, X., and Wang, S. (2014). Three-dimensional nano-biointerface as a new platform for guiding cell fate. Chem. Soc. Rev. 43, 2385–2401. doi: 10.1039/C3CS60419E
Liu, Y., Yang, G., Ji, H., Xiang, T., Luo, E., and Zhou, S. (2017). Synergetic effect of topological cue and periodic mechanical tension-stress on osteogenic differentiation of rat bone mesenchymal stem cells. Colloids Surf. B Biointerfaces 154, 1–9. doi: 10.1016/j.colsurfb.2017.02.035
Lutolf, M. P., and Hubbell, J. A. (2005). Synthetic biomaterials as instructive extracellular microenvironments for morphogenesis in tissue engineering. Nat. Biotech. 23, 47–55. doi: 10.1038/nbt1055
Lv, F. J., Tuan, R. S., Cheung, K. M., and Leung, V. Y. (2014). Concise review: the surface markers and identity of human mesenchymal stem cells. Stem Cells 32, 1408–1419. doi: 10.1002/stem.1681
Malkoc, V., Gallego-Perez, D., Nelson, T., Lannutti, J. J., and Hansford, D. J. (2015). Controlled neuronal cell patterning and guided neurite growth on micropatterned nanofiber platforms. J. Micromech. Microeng. 25:125001. doi: 10.1088/0960-1317/25/12/125001
Marino, A., Genchi, G. G., Sinibaldi, E., and Ciofani, G. (2017). Piezoelectric effects of materials on bio-interfaces. ACS Appl. Mater. Interfaces 9, 17663–17680. doi: 10.1021/acsami.7b04323
Mattila, P. K., and Lappalainen, P. (2008). Filopodia: molecular architecture and cellular functions. Nat. Rev. Mol. Cell Biol. 9, 446–454. doi: 10.1038/nrm2406
McCall, J. (2005). Genetic algorithms for modelling and optimisation. J. Computat. Appl. Mathemat. 184, 205–222. doi: 10.1016/j.cam.2004.07.034
Mele, E. (2016). Electrospinning of natural polymers for advanced wound care: towards responsive and adaptive dressings. J. Mater. Chem. B 4, 4801–4812. doi: 10.1039/C6TB00804F
Mele, E., Heredia-Guerrero, J. A., Bayer, I. S., Ciofani, G., Genchi, G. G., Ceseracciu, L., et al. (2015). Zwitterionic nanofibers of super-glue for transparent and biocompatible multi-purpose coatings. Sci. Rep. 5:14019. doi: 10.1038/srep14019
Nedjari, S., Awaja, F., and Altankov, G. (2017). Three dimensional honeycomb patterned fibrinogen based nanofibers induce substantial osteogenic response of mesenchymal stem cells. Sci Rep. 7:15947. doi: 10.1038/s41598-017-15956-8
Paim, Á., Tessaro, I. C., Cardozo, N. S. M., and Pranke, P. (2018). Mesenchymal stem cell cultivation in electrospun scaffolds: mechanistic modeling for tissue engineering. J. Biol. Phys. 44, 245–271. doi: 10.1007/s10867-018-9482-y
Park, S. H., Kim, M. S., Lee, B., Park, J. H., Lee, H. J., Lee, N. K., et al. (2016). Creation of a hybrid scaffold with dual configuration of aligned and random electrospun fibers. ACS Appl. Mater. Interfaces 8, 2826–2832. doi: 10.1021/acsami.5b11529
Rahman, S. U., Oh, J. H., Cho, Y. D., Chung, S. H., Lee, G., Baek, J. H., et al. (2018). Fibrous topography-potentiated canonical Wnt signaling directs the odontoblastic differentiation of dental pulp-derived stem cells. ACS Appl. Mater. Interfaces 10, 17526–17541. doi: 10.1021/acsami.7b19782
Rajendran, P., Rengarajan, T., Thangavel, J., Nishigaki, Y., Sakthisekaran, D., Sethi, G., et al. (2013). The vascular endothelium and human diseases. Int. J. Biol. Sci. 9, 1057–1069. doi: 10.7150/ijbs.7502
Sankar, S., Kakunuri, M. D., Eswaramoorthy, S., Sharma, C. S., and Rath, S. N. (2018). Effect of patterned electrospun hierarchical structures on alignment and differentiation of mesenchymal stem cells: biomimicking bone. J. Tissue Eng. Regen. Med. 12, e2073–e2084. doi: 10.1002/term.2640
Sharma, R. R., Pollock, K., Hubel, A., and McKenna, D. (2014). Mesenchymal stem or stromal cells: a review of clinical applications and manufacturing practices. Transfusion 54, 1418–1437. doi: 10.1111/trf.12421
Shin, Y. M., Shin, H. J., Heo, Y., Jun, I., Chung, Y. W., Kim, K., et al. (2017). Engineering an aligned endothelial monolayer on a topologically modified nanofibrous platform with a micropatterned structure produced by femtosecond laser ablation. J. Mater. Chem. B 5, 318–328. doi: 10.1039/C6TB02258H
Sill, T., and von Recum, H. A. (2008). Electrospinning: applications in drug delivery and tissue engineering. Biomater 29, 1989–2006. doi: 10.1016/j.biomaterials.2008.01.011
Su, N., Gao, P. L., Wang, K., Wang, J. Y., Zhong, Y., and Luo, Y. (2017). Fibrous scaffolds potentiate the paracrine function of mesenchymal stem cells: a new dimension in cell-material interaction. Biomater 141, 74–85. doi: 10.1016/j.biomaterials.2017.06.028
Sun, L., Gao, W., Fu, X., Shi, M., Xie, W., Zhang, W., et al. (2018). Enhanced wound healing in diabetic rats by nanofibrous scaffolds mimicking the basket weave pattern of collagen fibrils in native skin. Biomater. Sci. 6, 340–349. doi: 10.1039/C7BM00545H
Tallawi, M., Dippold, D., Rai, R., D'Atri, D., Roether, J. A., Schubert, D. W., et al. (2016). Novel PGS/PCL electrospun fiber mats with patterned topographical features for cardiac patch applications. Mater. Sci. Eng. C Mater. Biol. Appl. 69, 569–576. doi: 10.1016/j.msec.2016.06.083
Tartarini, D., and Mele, E. (2015). Adult stem cell therapies for wound healing: biomaterials and computational models. Front. Bioeng. Biotechnol. 3:206. doi: 10.3389/fbioe.2015.00206
Taskin, M. B., Xia, D., Besenbacher, F., Dong, M., and Chen, M. (2017). Nanotopography featured polycaprolactone/polyethyleneoxide microfibers modulate endothelial cell response. Nanoscale 9, 9218–9229. doi: 10.1039/C7NR03326E
Unnithan, A. R., Sasikala, A. R. K., Thomas, S. S., Nejad, A. G., Cha, Y. S., Park, C. H., et al. (2018). Strategic design and fabrication of biomimetic 3d scaffolds: unique architectures of extracellular matrices for enhanced adipogenesis and soft tissue reconstruction. Sci Rep. 8:5696. doi: 10.1038/s41598-018-23966-3
Voss-Böhme, A. (2012). Multi-scale modeling in morphogenesis: a critical analysis of the cellular Potts model. PLoS ONE 7:e42852. doi: 10.1371/journal.pone.0042852
Xia, H., and Xia, Y. (2018). An in vitro study of non-aligned or aligned electrospun poly(methyl methacrylate) nanofibers as primary rat astrocytes-loading scaffold. Mater. Sci. Eng. 91, 228–235. doi: 10.1016/j.msec.2018.05.050
Xie, J., Li, X., and Xia, Y. (2008). Putting electrospun nanofibers to work for biomedical research. Macromol. Rapid Comm. 29, 1775–1792. doi: 10.1002/marc.200800381
Xie, J., Liu, W., MacEwan, M. R., Bridgman, P. C., and Xia, Y. (2014). Neurite outgrowth on electrospun nanofibers with uniaxial alignment: the effects of fiber density, surface coating, and supporting substrate. ACS Nano. 8, 1878–1885. doi: 10.1021/nn406363j
Xu, H., Li, H., Ke, Q., and Chang, J. (2015). An anisotropically and heterogeneously aligned patterned electrospun scaffold with tailored mechanical property and improved bioactivity for vascular tissue engineering. ACS Appl. Mater. Interfaces 7, 8706–8718. doi: 10.1021/acsami.5b00996
Xue, J., Yang, J., O'Connor, D. M., Zhu, C., Huo, D., Boulis, N. M., et al. (2017). Differentiation of bone marrow stem cells into schwann cells for the promotion of neurite outgrowth on electrospun fibers. ACS Appl. Mater. Interfaces 9, 12299–12310. doi: 10.1021/acsami.7b00882
Yan, S., Zhang, X., Zhang, L., Liu, H., Wang, X., and Li, Q. (2017). Polymer scaffolds for vascular tissue engineering fabricated by combined electrospinning and hot embossing. Biomed. Mater. 13:015003. doi: 10.1088/1748-605X/aa8a81
Yang, G. H., Jeon, H., and Kim, G. (2017). Alternately plasma-roughened nanosurface of a hybrid scaffold for aligning myoblasts. Biofabrication 9:025035. doi: 10.1088/1758-5090/aa77ba
Yin, Z., Chen, X., Song, H. X., Hu, J. J., Tang, Q. M., Zhu, T., et al. (2015). Electrospun scaffolds for multiple tissues regeneration in vivo through topography dependent induction of lineage specific differentiation. Biomaterials 44, 173–185. doi: 10.1016/j.biomaterials.2014.12.027
Zhang, L.-H., Duan, X.-P., Yan, X., Yu, M., Ning, X., Zhao, Y., et al. (2016). Recent advances in melt electrospinning. RSC Adv. 6, 53400–53414. doi: 10.1039/C6RA09558E
Zhang, Q., Hwang, J. W., Oh, J. H., Park, C. H., Chung, S. H., Lee, Y. S., et al. (2017). Effects of the fibrous topography-mediated macrophage phenotype transition on the recruitment of mesenchymal stem cells: an in vivo study. Biomater 149, 77–87. doi: 10.1016/j.biomaterials.2017.10.007
Zhu, M., Wang, Z., Zhang, J., Wang, L., Yang, X., Chen, J., et al. (2015). Circumferentially aligned fibers guided functional neoartery regeneration in vivo. Biomater 61, 85–94. doi: 10.1016/j.biomaterials.2015.05.024
Keywords: bio-interfaces, surface topography, electrospinning, micro-patterning, mathematical modeling
Citation: Denchai A, Tartarini D and Mele E (2018) Cellular Response to Surface Morphology: Electrospinning and Computational Modeling. Front. Bioeng. Biotechnol. 6:155. doi: 10.3389/fbioe.2018.00155
Received: 29 August 2018; Accepted: 08 October 2018;
Published: 24 October 2018.
Edited by:
Gianni Ciofani, Politecnico di Torino, ItalyReviewed by:
Elia Ranzato, Università degli Studi del Piemonte Orientale, ItalySimona Martinotti, Università degli Studi del Piemonte Orientale, Italy
Copyright © 2018 Denchai, Tartarini and Mele. This is an open-access article distributed under the terms of the Creative Commons Attribution License (CC BY). The use, distribution or reproduction in other forums is permitted, provided the original author(s) and the copyright owner(s) are credited and that the original publication in this journal is cited, in accordance with accepted academic practice. No use, distribution or reproduction is permitted which does not comply with these terms.
*Correspondence: Elisa Mele, ZS5tZWxlMkBsYm9yby5hYy51aw==