- 1North Queensland Algal Identification Facility, Aquaculture, College of Science and Engineering, James Cook University, Townsville, QLD, Australia
- 2Departamento de Biología, Universidad Autónoma de Madrid, Madrid, Spain
- 3Australian Institute of Marine Science (AIMS), Townsville, QLD, Australia
- 4Centre for Marine Bioproducts Development (CMBD), College of Medicine and Public Health, Flinders University, Bedford Park, SA, Australia
Rising CO2 levels, associated climatic instability, freshwater scarcity and diminishing arable land exacerbate the challenge to maintain food security for the fast growing human population. Although coal-fired power plants generate large amounts of CO2 emissions and wastewater, containing environmentally unsafe concentrations of metals, they ensure energy security. Nitrogen (N2)-fixation by cyanobacteria eliminate nitrogen fertilization costs, making them promising candidates for remediation of waste CO2 and metals from macronutrient-poor ash dam water and the biomass is suitable for phycocyanin and biofertilizer product development. Here, the effects of CO2 and metal mixtures on growth, bioproduct and metal removal potential were investigated for the self-flocculating, N2-fixing freshwater cyanobacterium Tolypothrix sp. Tolypothrix sp. was grown outdoors in simulated ash dam wastewater (SADW) in 500 L vertical bag suspension cultures and as biofilms in modified algal-turf scrubbers. The cultivation systems were aerated with air containing either 15% CO2 (v/v) or not. CO2-fertilization resulted in ∼1.25- and 1.45-fold higher biomass productivities and ∼40 and 27% increased phycocyanin and phycoerythrin contents for biofilm and suspension cultures, respectively. CO2 had no effect on removal of Al, As, Cu, Fe, Sr, and Zn, while Mo removal increased by 37% in both systems. In contrast, Ni removal was reduced in biofilm systems, while Se removal increased by 73% in suspension cultures. Based on biomass yields and biochemical data obtained, net present value (NPV) and sensitivities analyses used four bioproduct scenarios: (1) phycocyanin sole product, (2) biofertilizer sole product, (3) 50% phycocyanin and 50% biofertilizer, and (4) 100% phycocyanin and 100% biofertilizer (residual biomass) for power station co-located and not co-located 10 ha facilities over a 20-year period. Economic feasibility for the production of food-grade phycocyanin either as a sole product or with co-production of biofertilizer was demonstrated for CO2-enriched vertical and raceway suspension cultures raised without nitrogen-fertilization and co-location with power stations significantly increased profit margins.
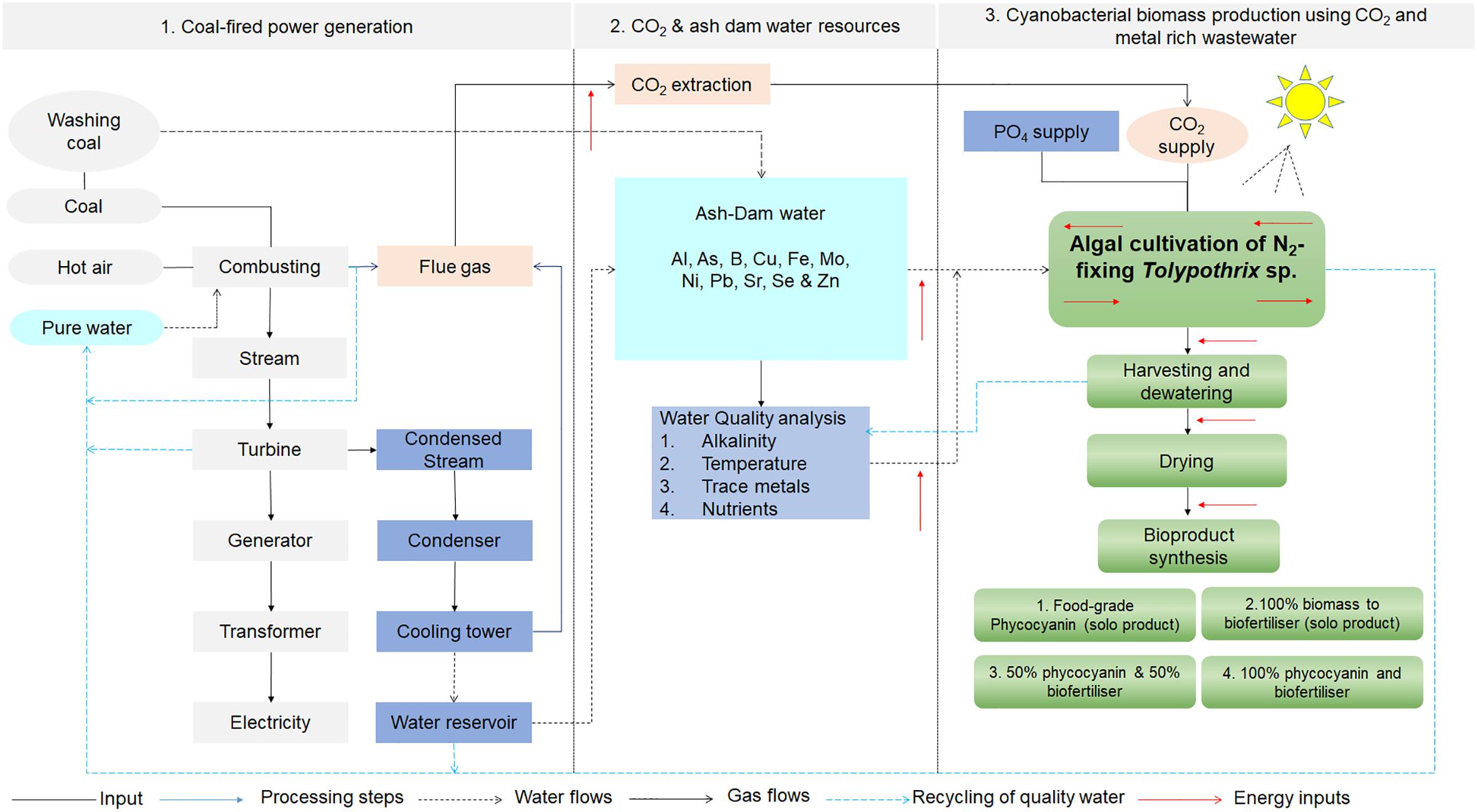
Graphical Abstract. Framework for an integrated bio-economic model for co-locating cyanobacterial cultivation with coal-fired power station based on a biorefinery approach.
Introduction
Anthropogenic emissions of carbon dioxide (CO2) account for 68% of total emissions (Ho et al., 2011), posing a threat to the global climatic equilibrium. At present, coal-powered electricity generation is still required in Australia and globally to meet energy requirements and – security (Stock, 2014). Flue gas from coal-fired power plants contain 10–15% CO2 (v/v) and generate wastewater enriched with heavy metals (Artanto et al., 2014). Biological fixation of CO2 and absorption of nutrients/metals from wastewaters by photosynthetic organisms such as microalgae and cyanobacteria is gaining industrial interest, as the biomass produced can yield a variety of high- and low-value renewable products (Wang et al., 2008). Wastewater generated at coal-fired power plants (ash dam water) cannot be discharged due to its potential toxicity and is therefore stored in ash dams (Roberts et al., 2015; Velu et al., 2019). The ash dam water contains metals, many of which serve as micronutrients important for plant growth, but macro-nutrients, such as nitrogen and phosphate, are lacking (Saunders et al., 2012). As the cultivation of eukaryotic photosynthetic organisms requires nitrogen and phosphate for growth, ash dam water needs to be supplemented with these macro-nutrients, increasing the cost of bioremediation and biomass production (Velu et al., 2019). In contrast, the cultivation of diazotrophic cyanobacteria does not require nitrogen fertilization, as these organisms can fix atmospheric nitrogen (N2), making them an ideal choice for bioremediation of metals from nitrogen-limited wastewaters (Markou and Georgakakis, 2011). This is a clear advantage, as globally 85 million tons of nitrogenous fertilizer were used in 2000 for food production. Synthetic nitrogenous fertilizers are projected to not meet the demands of the ever growing human population in the near future (Singh et al., 2016). In addition, the current exploitation of chemically derived fertilizers have been shown to contribute to environmental problems, such as pollution, reduced soil fertility and adverse impacts on the ozone layer (Benemann, 1979; Singh et al., 2016). In an Australian context, agricultural productivity is declining in regions with marginal/leached soils, as microbial consortia, essential for soil fertility, are negatively affected by declining soil carbon contents. Soil fertility cannot be improved by provision of nitrogen without the addition of large amounts of carbon (QLD, 2016). Despite the realization of adverse impacts of synthetic nitrogenous fertilizers, ∼5.3 million tonnes of chemical fertilizers were used on 49.1 million ha agricultural land in Australia between 2014–2015 (ABS, 2015). Thus, there is a pressing need for the sustainable production of innovative fertilizers that are effective, renewable, environmentally friendly, cost-efficient, and improve soil fertility to ensure food security in the future. In this context, fertilizers derived from biological nitrogen fixation and through recycling and re-use of nitrogen contained in various wastewaters offer great potential benefits (Benemann, 1979; Singh et al., 2016).
Nitrogen fixation is carried out by the oxygen-sensitive, iron and molybdenum-containing nitrogenase complex (Abed et al., 2009), resulting in higher iron and molybdenum requirements, elements that are present at elevated concentrations in ash dam wastewater (Saunders et al., 2012). While excessive metal concentrations can retard cyanobacterial growth (Pereira et al., 2011), species such as Anabaena subcylindrica, Aphanocapsa sp., Calothrix sp., Microcystis sp., Oscillatoria salina, Plectonema terebrans, and Synechococcus sp. can be used for the treatment of domestic and industrial wastewater (Dubey et al., 2011). Biomineralisation of metals by cyanobacteria occurs via intracellular bioaccumulation and/or passive biosorption, the latter is mediated by the presence of an exopolysaccharide layer (EPS) on the outside of many cyanobacterial species (Pereira et al., 2011). The EPS consists of complex heteropolysaccharides on a glucosamine backbone, providing an accumulation of negative charges that play an essential role in the chelation of metal ions (De Philippis et al., 2011). On a dry weight basis, Calothrix scopulorum and C. marchica chelated 0.7 and 6.4% of lead (Weckesser et al., 1988; Ruangsomboon et al., 2007). Nostoc muscorum chelated 22.5, 11.8, 26.4, and 32% of copper, cobalt, lead and manganese, while Anabaena subcylindrica performed much better (81.8. 33.7, 100, and 100%, respectively) (El-Sheekh et al., 2005). The large differences in the biosorption of metals indicates that the choice of species is an important criterion to consider, especially when the reutilization of large volumes of wastewater is an important aspect of the industrial process. As CO2-fertilization enhances cyanobacterial growth (Velu et al., 2015, 2019), EPS content will increase simultaneously, which should enhance metal chelation capacity of the cultures and, hence, remediation capacity. The presence of complex metals mixtures in industrial wastewaters may, however, result in competition for the same binding sites, which can result in reduced adsorption efficiencies (Pereira et al., 2011). The diazotrophic filamentous freshwater cyanobacterium, Tolypothrix sp. has been used for treatment of domestic and industrial wastewaters and T. ceytonica achieved an 86 and 64.4% efficiency for the removal of zinc and total suspended solids (El-Bestawy, 2008).
In addition to the exploitation of environmental services (CO2 and metal remediation), the prokaryotic cyanobacteria show additional advantages for biotechnological applications, such as strain-dependent wide environmental tolerances, e.g., marine to freshwater, acid and/or alkaline conditions (Gupta et al., 2013), rapid growth and high photosynthetic activities (Hall et al., 1995). Furthermore, the produced biomass has multiple commercial applications through bioproduct development. In general, potential algal bioproducts include medicinal compounds, food and feed supplements (restricted to CO2 enriched grown species without inclusion of metal- or other potentially toxic compound-containing wastewater treatment), pigments (e.g., β-carotene, astaxanthin, fucoxanthin, lutein, phycocyanin, phycoerythrin, the latter two from cyanobacteria), protein, carbohydrate, biofuel and biohydrogen, and biofertilizers (Setta et al., 2017; von Alvensleben and Heimann, 2019). Specific cyanobacterial bioproducts could be protein, mineral and unsaturated fatty acid supplements and the pigments phycocyanin and phycoerythrin from Arthrospira platensis or Limnospira maxima (formerly Spirulina platensis and S. maxima), where the protein content of the biomass can reach 74% (Cohen, 1997), which can be extracted through biorefining (Borowitzka, 2013). It might be argued that cyanobacterial biomass produced using metal-rich wastewater is not suitable for high-value phycocyanin product development. Indeed some binding of iron and mercury, the latter not present in ash dam water of coal-fired power plants used in this study, and less efficient binding of some other metals to phycocyanin has been described (Bermejo et al., 2008; Gelagutashvili and Tsakadze, 2013; Bhayani et al., 2016). It is, however, unclear how much binding would occur and how irreversible the binding would be. In addition, affinity of the metals present in ash dam water can be expected traditional metal chelating proteins, such as metallothioneins, and the highly negatively charged EPS, both present in cyanobacterial including Tolypothrix sp. biomass, would be more efficient binding sites. In addition, as proposed in this study, phycocyanin extracted from Tolypothrix sp. biomass will be purified to upgrade the product to food-grade phycocyanin to obtain a higher sales price, which would further remove any metals bound to phycocyanin. It will nonetheless be essential to analyze the final product for metal contents for quality assurance.
While light, temperature and CO2 supplies can be easily controlled at laboratory scale, therefore producing best biomass yields, biomass productivities are typically reduced in large volume suspension-based systems, due to light and carbon limitation, particularly in raceway pond cultivation (Pierobon et al., 2018). Under outdoor large-scale cultivation conditions, improved solar and carbon supplies can be achieved in closed bioreactors, but this adds energy and infrastructure costs, limiting suitability to high-value product development (Pierobon et al., 2018). In contrast, cyanobacterial biofilm reactors are better suited for cost-effective biomass production and are frequently used for wastewater treatment (Hoh et al., 2016). Cyanobacterial biofilm cultivation requires minimal water supplies, gas exchange (CO2 absorption and O2 venting) is more efficient and harvesting is energy-efficient (Heimann, 2016). Recently developed porous substrate biofilm reactors show efficient light –, carbon – and water utilization and scale-up of this technology is easily possible, making them a promising technology for economical microalgal/microbial biomass production (Pierobon et al., 2018). For example, cyanobacterial biomass productivity was greater in rotating biofilm reactors without aeration or additional CO2-supplementation compared to suspension reactors (Gross and Wen, 2014), but the adhesion process for mat establishment is sensitive to shear forces, and species- and substrate-dependent.
Integrated biomass production with wastewater and CO2 emission-generating industries has many advantages, i.e., use of non-arable land, non-potable water and provision of trace metals, and CO2 to support biomass and bioproduct productivities (Roberts et al., 2015; Moheimani, 2016; Aslam et al., 2019). Nonetheless, economic feasibility still needs to be demonstrated on a case-by-case basis, as outcomes are dependent on the value of the bioproduct(s) and yields. In addition, in the case of ash dam water generated at coal-fired power plants, metal toxicity may occur, reducing yields and application potential of generated bioproducts (Velu et al., 2019). Previous research established that the diazotrophic Tolypothrix sp. (isolated from tropical Australia) efficiently self-flocculates, reducing energy requirements for harvesting/dewatering of biomass by 90% (Heimann et al., 2013; Velu et al., 2015). Furthermore, no metal toxicity was observed for Tolypothrix sp. biomass production in simulated ash dam water (SADW) and growth performance was independent of nitrogen supply, yet costs for phosphate fertilization are incurred (Velu et al., 2019). The graphical abstract illustrates the integrated production of Tolypothrix sp. biomass and potential bioproducts when co-located at a coal-fired power plant.
Therefore, this study used the Australian isolate of Tolypothrix sp. to contrast biomass productivities, metal removal capacity and bioproduct potential for biomass cultivated in simulated ash dam water (SADW) between a traditional bubble column reactor and a modified algal turf scrubber with and without CO2 supplementation under outdoor conditions. Additionally, the economic feasibility for bioproduct development was estimated, considering four scenarios: (1) production of food-grade phycocyanin as a sole product, (2) biofertilizer as a sole product, (3) use of half the biomass for biofertilizer and food-grade phycocyanin production and (4) biorefining of the high-value phycocyanin with the residue being used as biofertilizer. This study modeled net present value (NPV) and sensitivity analysis for these four scenarios under conditions of co-location with coal-fired power plants and traditional cultivation (not co-located) for a 10 ha plant using suspension bubble columns and raceways for biomass production.
Materials and Methods
Culture Collection and Strain Characterization
The diazotrophic, filamentous, freshwater cyanobacterial strain Tolypothrix sp. NQAIF319 was isolated and maintained as described in Velu et al. (2015).
Synthetic Ash Dam Wastewater Preparation
Synthetic ash dam wastewater was prepared as described in Velu et al. (2019) based on concentrations obtained for ash dam water of a Queensland coal-fired power plant, Australia (Saunders et al., 2012).
Outdoor Cultivation Set Up, Growth Estimation and Biochemical Profiling
Outdoor cultivation of Tolypothrix sp. NQAIF319 occurred in SADW in four meso-scale open bioreactor prototypes: (1) two algal turf scrubbers (ATS) of 2.2 m2 each (2.2 m long × 1 m wide) (Supplementary Figure S1 and Supplementary Table S1); (2) two suspension vertical bags of 500 L each with a 0.3 m2 area footprint, designed and assembled at James Cook University Australia (Supplementary Figure S2 and Supplementary Table S2). The cultivation area was shaded with a UV-shade cloth (Coolaroo, 3.66 m wide, 60% shading) to control photon flux density between 500 and 900 μmol m–2 s–1 at the Freshwater Compound at James Cook University, Townsville, Australia (19.33 S, 146.76 E). The plastic trays of the ATSs were lined with polystyrene for attachment of Tolypothrix sp. and had a slope of 7%. Water flow conditions were continuous at 66 L min–1 delivered from a sump with a 500 L fill volume beneath the ATS. The 500 L vertical bubbled suspension culture systems were constructed from PVC bag material contained in a wire cage with a plastic keeled footing of 0.3 m2. A tap was fitted to the bottom corner of the vertical bag for harvesting, while aeration was provided by a suspended diffuser delivering 0.05 L air L–1 min–1 from the main compressor.
The two ATS tanks and two suspension vertical bags were filled with 500 L SADW. Outdoor culture inoculi (starter cultures) were grown in 20 L polycarbonate carboys in BG11 medium without nitrogen [BG11(-N)] at 28°C and a photon flux density of 100 μmol m–2 s–1 until cultures reached stationary phase. For inoculation of the vertical bag system, the starter cultures were centrifuged (8,000 × g, 20 min; Beckman Avanti® J-26XP, Australia) and an adequate volume of the cell pellet was resuspended into 500 L of fresh SADW medium to reach an initial biomass concentration of 0.1 g dry weight L–1. The ATS were inoculated by spreading the centrifuged Tolypothrix sp. biomass to an initial concentration of 5 g DW m–2 without water flow. After an overnight attachment period, water was supplied to the top of the system via a baffle.
A total of 16-day growth experiments were simultaneously performed in both systems in two consecutive runs, during September 2016 (run 1) and October 2016 (run 2). In both runs, one set of each cultivation system was supplemented with CO2-enriched air (15% v/v), while the other sets were supplied with air at atmospheric CO2 levels (non-CO2 controls). CO2-enriched air and air were baffled in both cultivation systems. Gases were 99.9% pure, ISO certified and supplied by BOC, a member of the Linde Group, Townsville, Australia. Temperature and pH were monitored twice daily (WP-81, TPS Instruments, Australia) and irradiance was measured at the time of sampling with a LI-250A photosynthetic active radiation (PAR) light probe (LiCor, Biosciences, United States). The incident sun light at sample time varied between 500 and 700 μmol m–2 s–1. Due to infrastructure and space limitations, replication for each system was carried out sequentially. Thus changes in the outdoor environmental parameters, such as light, temperature, and humidity, the latter especially affecting the biofilm cultivation system, is expected to additionally influence biomass production and metabolic profiles, rendering formal statistical analysis of the data inappropriate.
Samples were collected via a tap for the vertical suspension bag systems and by scraping a 100 cm–2 biofilm square (10 × 10 cm) using a silicone rubber cell scraper (IWAKI, Japan) every 4 days. Biomass growth (g DW m–2) was determined gravimetrically. Biomass productivities (g DW m–2 day–1) were calculated as per von Alvensleben et al. (2013), while doubling rate (k) and doubling time (T2) calculations followed Gour et al. (2014). Water samples for nutrient and metal analyses were collected from the ATS sumps and the supernatant of the centrifuged samples of the vertical suspension culture systems. Culture media phosphate and metal concentrations followed procedures described in detail in Velu et al. (2019), and biomass growth (g DW m–2) and productivity (g DW m–2 day–1) was determined gravimetrically (von Alvensleben et al., 2013) on days 0, 4, 8, 12, and 16. Biomass-specific growth rates (μ1–3), doubling rate (k) and doubling time (T2) were calculated as per Gour et al. (2014) and von Alvensleben et al. (2013).
Biomass was harvested on day 16 and biomass pellets were freeze-dried (Dynavac model Fd12, Australia) and stored in the dark at −80°C (Sanyo MDF-U33V, Japan) until analyses. Procedures for analyses of pigments (phycocyanin and phycoerythrin), fatty acids (fatty acid methyl esters (FAME), total lipid, alkane/alkene, protein, carbohydrate and carbon, hydrogen, sulphur, phosphorous, potassium (CHNSPK) contents followed Velu et al. (2019).
All chemicals and solvents were obtained from Sigma-Aldrich, Sydney, Australia.
Net Present Value (NPV) and Sensitivity Analyses
A techno-economic cost assessment was used to evaluate the economic feasibility for production of food-grade phycocyanin and biofertilizer from Tolypothrix sp. biomass, assuming either co-location with a coal-fired power plant or traditional cultivation, which is classified here as biomass production solely for bioproduct development without co-location with either an industrial wastewater producer and/or CO2 emitter. The following boundaries were set: (1) The algae cultivation plant has a 10 ha cultivation area and employs suspension-based open cultivation systems with an annual biomass production period of 300 days year–1. (2) The operating life was set to 20 years. (3) Production data generated in this study in vertical suspension culture systems in SADW and supplied with 15% CO2 (v/v) and average biomass productivities achieved for Nannochloropsis oculata in large-scale outdoor raceways formed the basis of the analyses. (4) All costs were adjusted to increase by 5% annually, while production sale prices were not adjusted. (5) Food-grade phycocyanin production assumed an extraction/purification efficiency of 67% (Chaiklahan et al., 2018) and a sale price for total phycocyanin of US$ 500 kg–1 (Querques et al., 2015), while the sale price for biofertilizer was estimated to be US$ 500 t–1.
The NPV was calculated by difference between the present value of cash income and the present value of all cash expenditures following the equation
where Ct is the net cash flow during the period t, C0 is the total initial investment cost, t is the number of time periods (years) and r is the discount rate.
The weighted average costs of capital (WACC) was set to 10% and sensitivity analyses were performed for two scenarios: (1) a reduced biofertilizer price of 25% of the current value (US$ 125 t–1) and (2) a reduced sales price for food-grade phycocyanin at 25% of today’s price (US$ 125 kg–1).
Results
Effect of Culture System and CO2-Supplementation on Growth and Phosphate Uptake of SADW-Grown Tolypothrix sp.
CO2-supplementation enhanced growth of Tolypothrix sp. in both types of outdoor cultivation systems. A 1.15- and 1.26-times higher final biomass yield of 34 and 42 g DW m–2 was obtained for biofilm cultures (Figure 1A1,2), while a 1.2- and 1.3-times higher yield in vertical suspension-based systems achieved a final biomass yield of 870 and 1310 g DW m–2 for runs 1 and 2, respectively (Figure 1B1,2). Growth over the 16-day time course could be divided into two phases of specific growth rate (μ1,2) (Table 1). μ1 was not affected by CO2-supplementation for any of the cultivation systems, whereas μ2 was ∼21 and 30% higher for biofilm cultures and ∼11 and 32% higher for vertical bag suspension cultures for runs 1 and 2, respectively, when CO2 was supplied. The difference in μ2 between the two systems is not surprising, as CO2 requirements of the biofilms are supplemented by direct access to CO2 from the atmosphere. As such, growth in the bubble column suspension system appears to be light- and CO2-limited in dense cultures. CO2-supplementation had no effect on doubling rates (k) in either cultivation system, but doubling time was generally reduced by 15% for suspension-grown Tolypothrix sp. Despite positive outcomes of CO2-fertilization on biomass productivity (Figures 1Ap, Bp) and system-dependent differences in growth performance, phosphate removal from the medium were slightly higher when supplemented with CO2, showing rapid uptake for the first 3 days of cultivation (Figures 2A,B). In contrast, phosphate removal rates (mg PO43– g–1 DW d–1) were higher for CO2-supplemented suspension cultures, while no trends were discernible for biofilms (Figures 2A1,B1). Growth of Tolypothrix sp. was phosphate-limited from day 8, and systems were devoid of phosphate from days 16 and 12 for CO2-supplemented cultures and non-CO2 controls of biofilm and suspension cultures, respectively. Biomass-standardized phosphate uptake rates were 0.2 to 0.3 for biofilms, but only 0.0065 to 0.0074 mg PO43– g–1 DW d–1 for suspension cultures and uptake rates were much lower for both cultivation systems from days 8 to 16, reflecting phosphate depletion from the SADW medium.
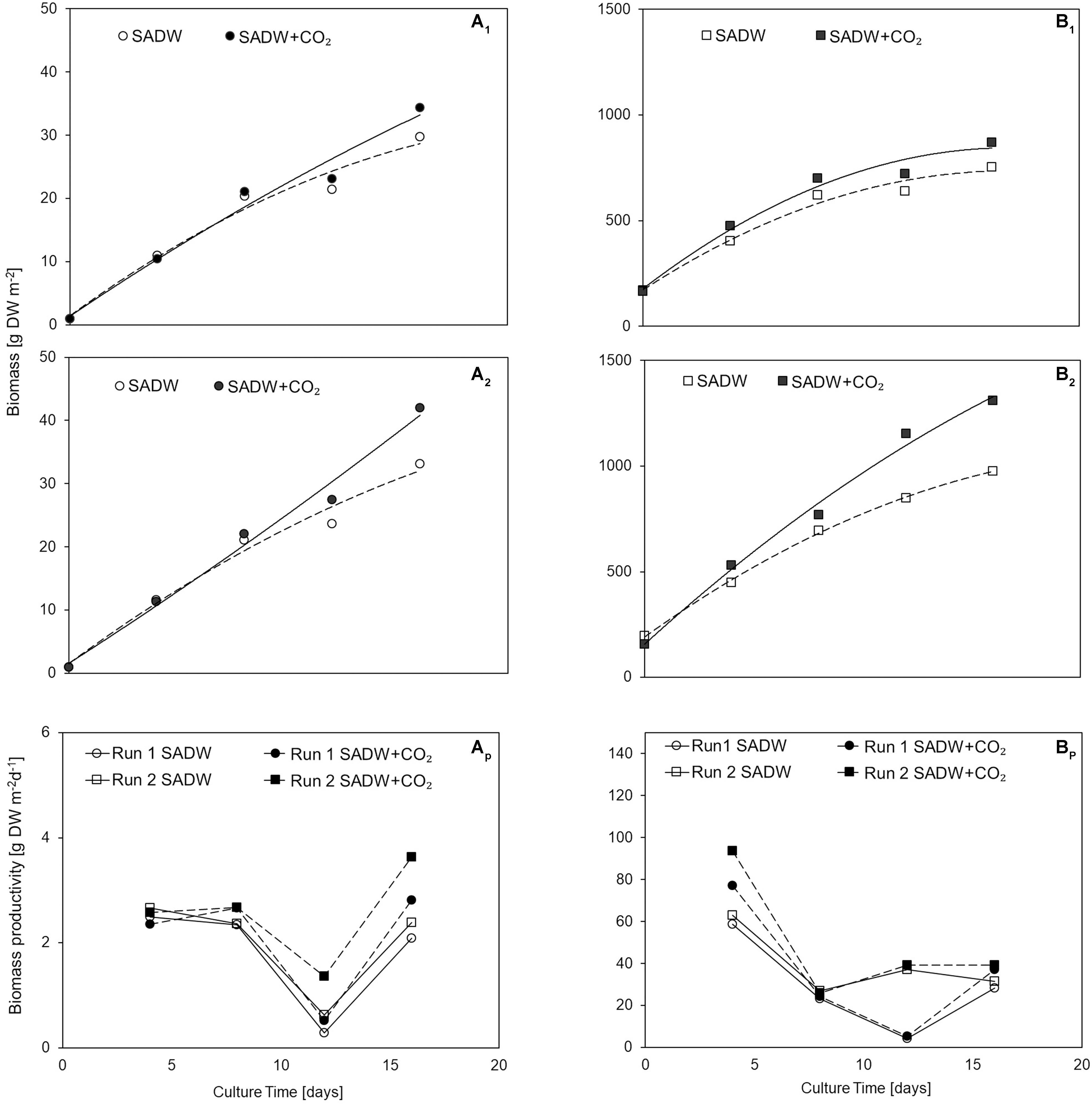
Figure 1. Effect of heavy metal and CO2 on Tolypothrix sp. growth in outdoor cultivation (A1) ATS run 1, (A2) ATS run 2, (Ap) ATS biomass productivity, (B1) V. Bag run 1, (B2) V. Bag run 2, (Bp) V. Bag biomass productivity.
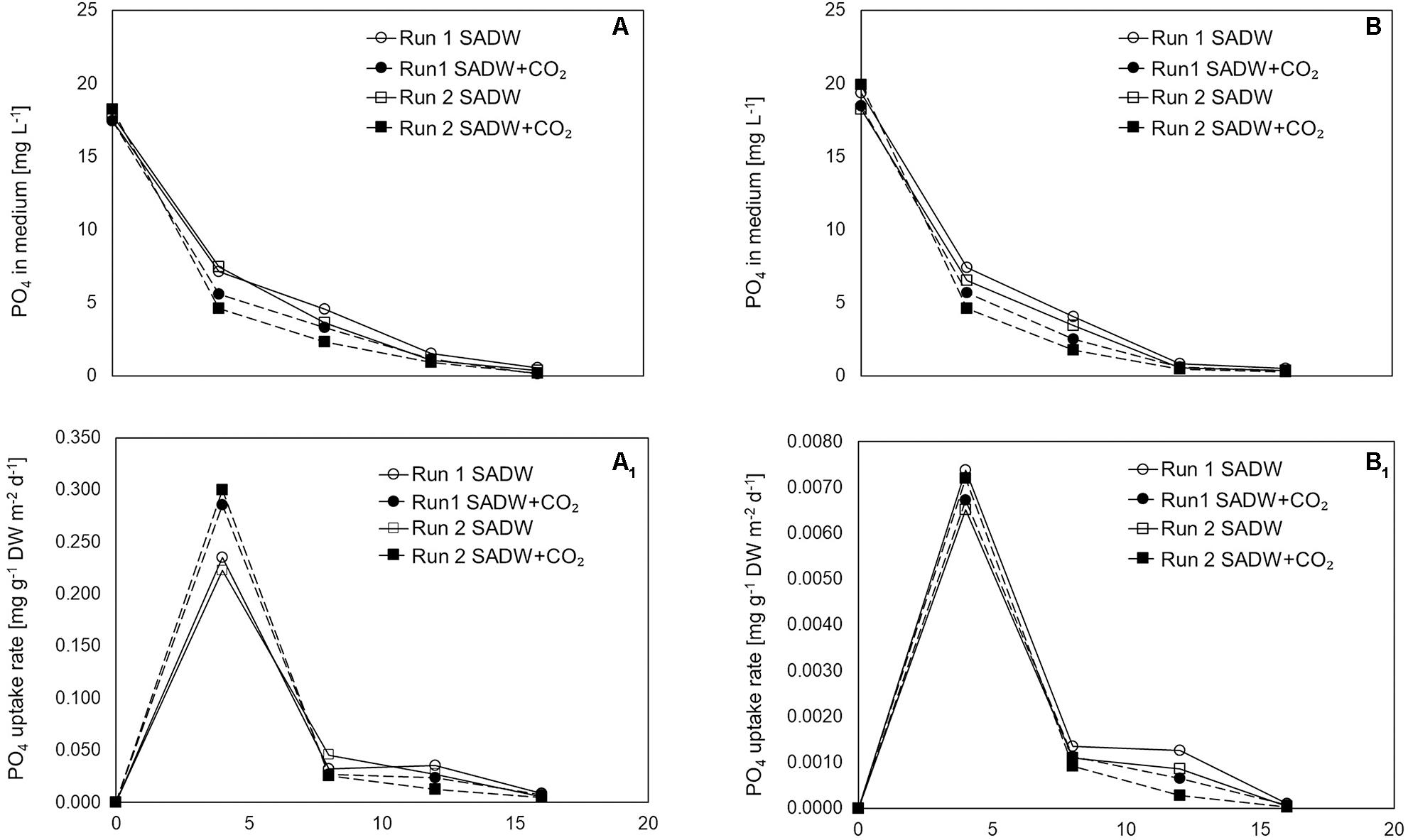
Figure 2. Effect of heavy metal and CO2 on culture medium phosphate levels and uptake rate of Tolypothrix sp. in outdoor cultivation. (A,A1) ATS and (B,B1) V. Bag.
Effect of Culture System and CO2-Supplementation on the Biochemical Profile of SADW-Grown Tolypothrix sp.
Carbohydrate, Protein, Lipid, Phycocyanin and Phycoerythrin Contents
CO2-supplementation increased carbohydrate and lipid contents of Tolypothrix sp. by 16 and 25% for biofilms and 26 and 38% for suspension cultures for runs 1 and 2, respectively (Figures 3A1,B1). In contrast, effects of CO2-supplementation on protein contents were marginal (Figures 3A1,B1). Maximal carbohydrate, protein and lipid contents were ∼49.2, 25.1, and 12.4% of cell dry weight (DW) for Tolypothrix sp. biofilms and ∼54.7, 26.0, and 14.8% of DW for suspension cultures when fertilized with CO2 (Figures 3A1,B1). Similarly, fertilization with 15% CO2 (v/v) increased phycobiliprotein (phycocyanin, phycoerythrin) contents (% w/w) by ∼40 and 27% for Tolypothrix sp. biofilms and suspension cultures, respectively. Maximal phycocyanin and phycoerythrin productivities were 0.3, 0.2, 3.6, and 2.9 g m–2 d–1 for biofilms and suspension cultures, respectively (Figures 3A,B).
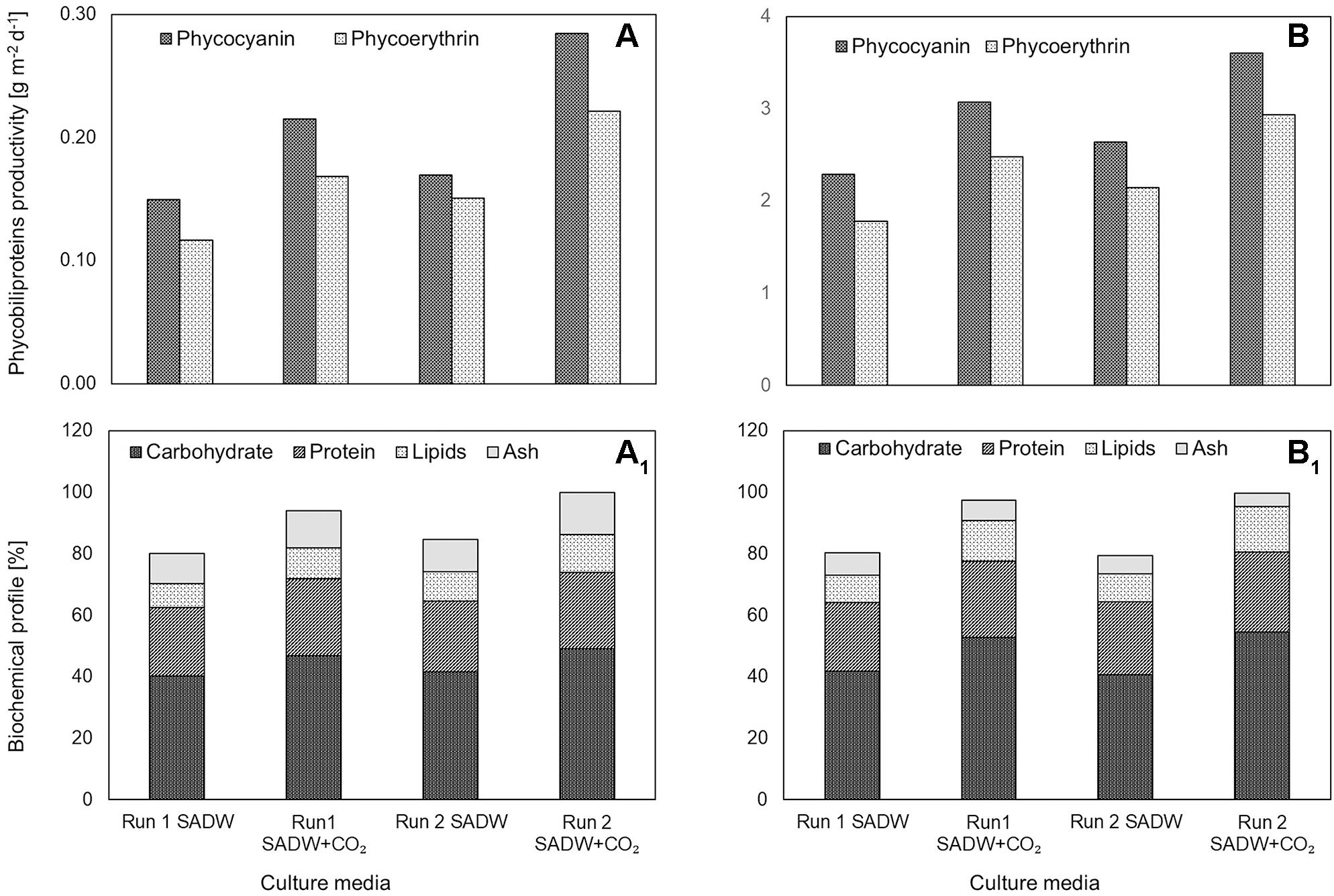
Figure 3. Effect of heavy metal and CO2 on pigments and biochemical profile of Tolypothrix sp. in outdoor cultivation. (A,A1) ATS and (B,B1) V. Bag.
Fatty Acid and Elemental Composition
As growth, lipid and phycobiliprotein contents were increased under CO2-fertilization, potential effects on fatty acid profiles and elemental composition (C, H, N, S, P, and K) and C/N ratios were investigated. Total fatty acid (TFA) contents and TFA productivities were ∼19 and 12% higher under CO2 supply for Tolypothrix sp. biofilms and suspension cultures, respectively. Maximal TFA yields were ∼75 mg g–1 DW for biofilms and ∼38 and 47 mg g–1 DW for runs 1 and 2, respectively (Table 2).
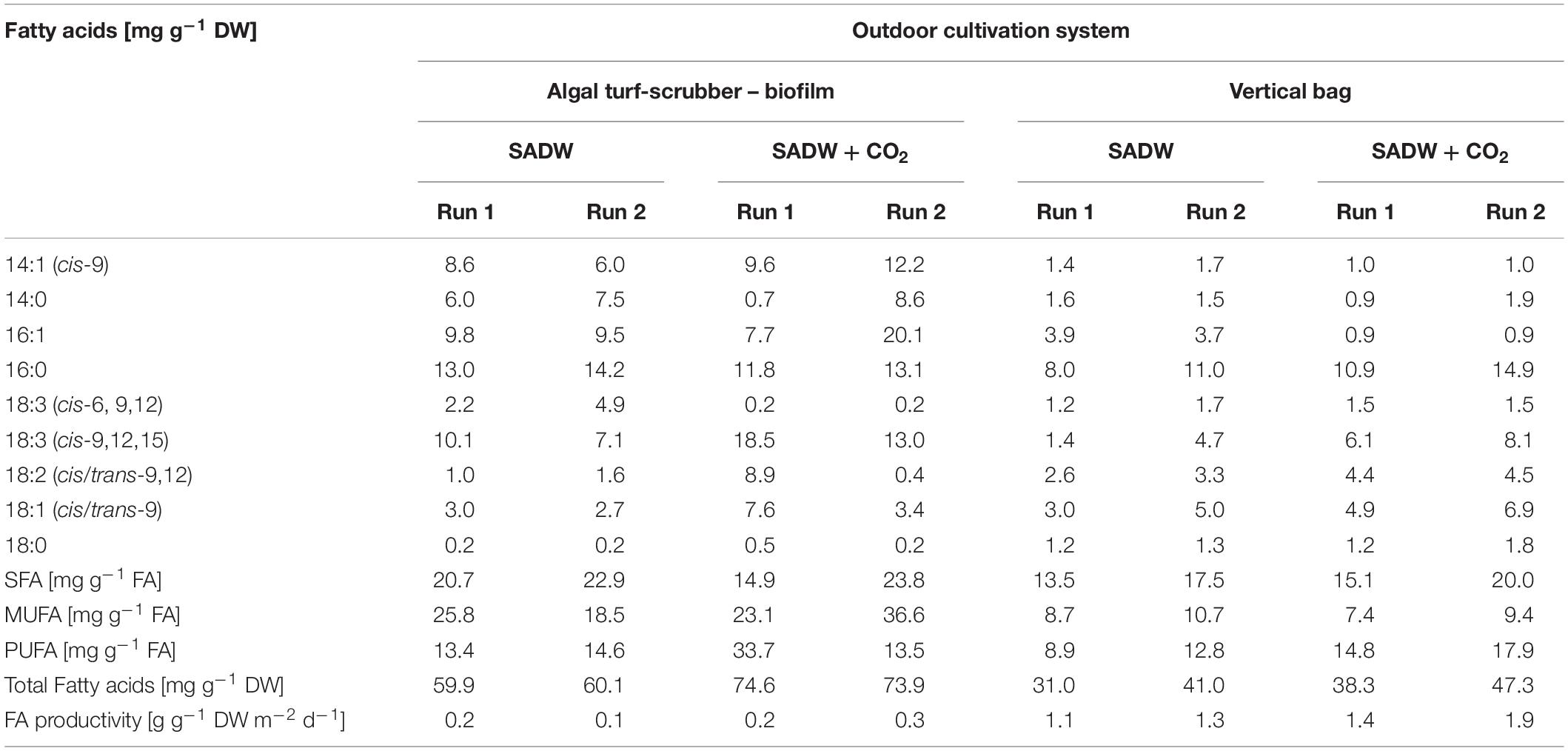
Table 2. Effect of CO2 and heavy metals on fatty acid profiles of Tolypothrix sp. in outdoor cultivation.
A positive effect of CO2-supplementation on saturated – (SFA), mono-unsaturated – (MUFA) and polyunsaturated fatty acid (PUFA) contents was noticeable for suspension-grown Tolypothrix sp., where the fatty acid profile was dominated by SFA, followed by PUFA and MUFA (Table 2). In contrast, responses to CO2-fertilization varied between both runs for Tolypothrix sp. biofilms, especially for MUFA and PUFA (Table 2), possibly due to variations in co-habiting bacterial communities, which are present and required for biofilm establishment and stabilization. The most abundant fatty acids were palmitic (hexadecanoic) acid (C16:0), followed by the ω-3-group of fatty acids α-linolenic acid [C18:3 (cis 9. 12. 15)], myristoleic acid (C14:1), the SFA myristic acid (C14:0), linoleic acid [C18:2 (cis/trans 9, 12)], the ω-9 oleic acid (C18:1) and the ω-6 γ-linolenic acid [C18:3 (cis 6, 9, 12)] (Table 2).
CO2-fertilization increased contents of C14:1, palmitoleic acid (C16:1), α-linolenic acid [C18:3 (cis 9. 12. 15)], C18:2 and C18:1 by 33, 31, 45, 72 and 48% in Tolypothrix sp. biofilms, respectively, while contents of C16:0 and C18:0 were unaffected. In contrast, CO2-supplementation increased C16:0, C18:3, C18:2, and C18:1 by 25, 57, 33 and 32% for Tolypothrix sp. suspension cultures, respectively (Table 2).
Culture system and CO2-supplementation did not result in large differences in C, H, N, S, P, and K contents. Carbon [∼45 and 47, and ∼47% (w/w)], K [∼0.79, 0.99, 0.68, and 0.79% (w/w)], and S [0.5, 0.7, and 0.7% (w/w)] were higher when supplemented with CO2 for Tolypothrix sp. biofilms and suspension cultures in runs 1 and 2, respectively (Table 3). A small positive effect of CO2-fertilization on nitrogen content and C/N ratios was also evident for Tolypothrix sp. biofilms, but not for suspension cultures (Table 3). In contrast, CO2 supply had a positive effect on P content of suspension cultures, but not biofilms (Table 3).
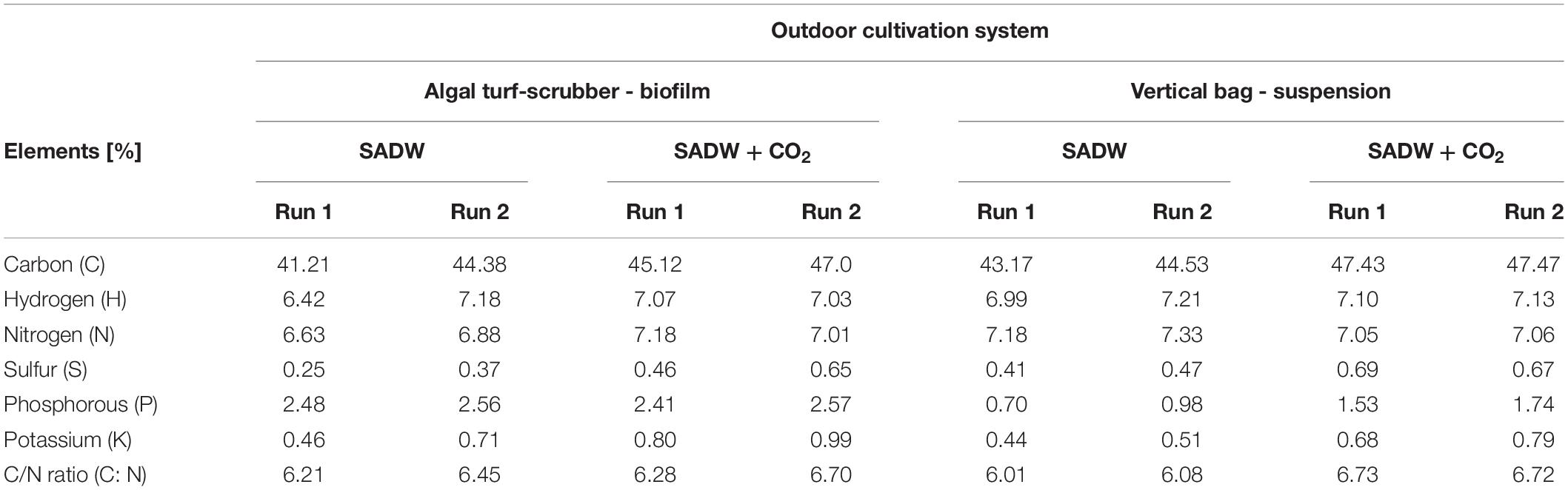
Table 3. Effect of CO2 and heavy metals on the elemental composition of Tolypothrix sp. in outdoor cultivation.
Metal Removal
A total of 16-day time course experiments investigated the effect of cultivation system and CO2 on metal removal from SADW, containing metals and concentrations typically found in ash dam water of coal-fired power plants, by Tolypothrix sp. (Table 4). Cultivation system and CO2-fertilization had no effect on maximal cumulative metal removal (Al, Sr, and Zn (≥90%), followed by Cu and Fe (∼70–80%), and As [∼65–75%)] for both biofilms and suspension cultures. In contrast, CO2-supplementation increased Mo removal by 37% in both cultivation systems, but an additional cultivation system effect was evident under CO2-fertilization, i.e., maximal Mo removal was ∼98% for biofilms but only ∼60% for suspension cultures. Conversely, a cultivation system effect was evident under CO2 supply for Se removal, with a 73% increase but a slight decrease for suspension-cultivated Tolypothrix sp. and biofilms, respectively. An even stronger cultivation effect was observed for Ni, where CO2-supplementation negatively affected removal in biofilm cultures, but not suspension cultures of Tolypothrix sp.
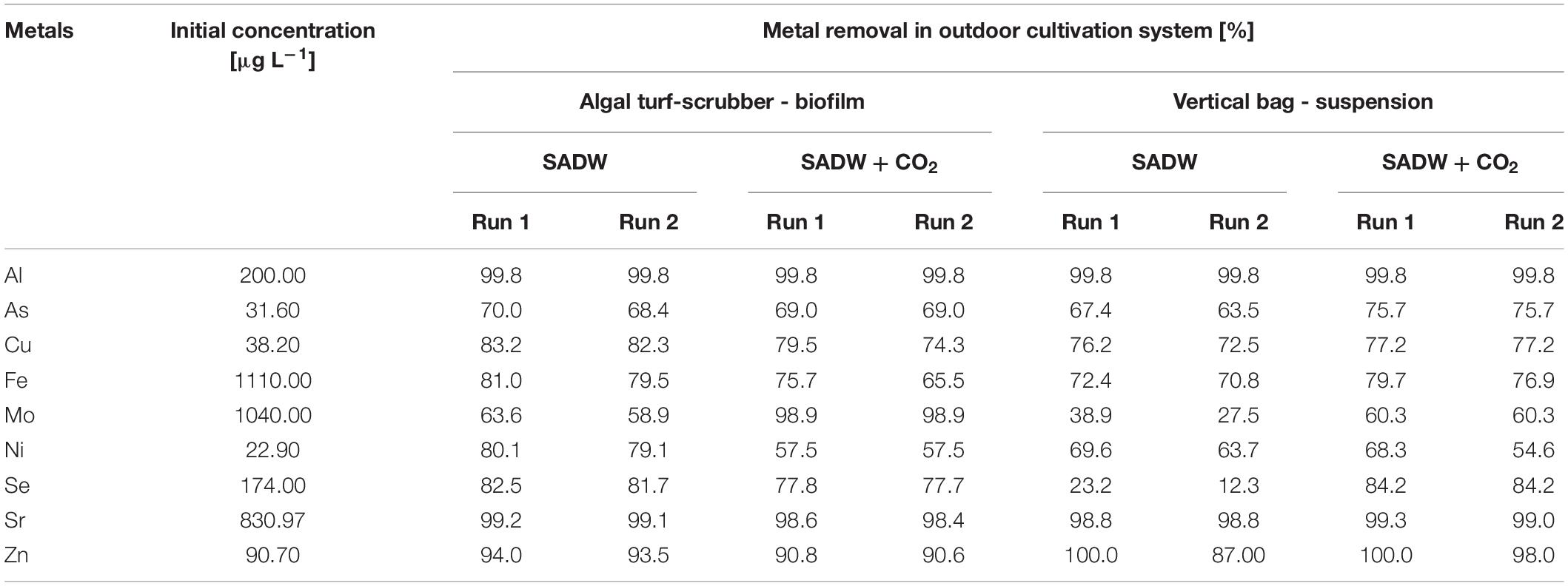
Table 4. Effect of CO2 on cumulative metal removal from SADW medium by Tolypothrix sp. over a 16-day time course.
Economic Viability Assessment of Bioproduct Commercialization Derived From SADW-Produced Tolypothrix sp. Biomass – Effect of Co-location With Coal-Fired Power Plants
Direct and Indirect Capital and Operational Costs
Based on the biomass productivities and biochemical profiles achieved with CO2 supplementation of SADW-grown Tolypothrix sp. in vertical bubble column suspension cultures, NPV analyses assessed the economic viability of four bioproduct scenarios under co-location with coal-fired power stations and traditional cultivation (no co-location) in raceway ponds, commonly used for production at commercial scale. The four bioproduct scenarios modeled were: (1) food-grade phycocyanin as the sole product, (2) biofertilizer as the sole product, (3) 50% of biomass used each for food-grade phycocyanin and biofertilizer production, and (4) biorefining of phycocyanin (100%) and use of the extracted biomass as a biofertilizer (Table 5). All capital costs and operating costs for Tolypothrix sp. cultivation (Table 5) were derived from published data for production of microalgal biomass (Davis et al., 2011; Griffin and Batten, 2013; Heimann et al., 2015; Schenk, 2016; Doshi, 2017; Fornarelli et al., 2017). Direct and indirect capital costs (engineering fees set at 15% of capital and contingency set at 5% of capital) for building a 10 ha production facility and some operational expenses were considered to be unavoidable. The co-location scenario considered savings on capital costs (land acquisition) and operational costs for maintenance and insurance, water (ash dam wastewater) and CO2 supply (flue gas) (Table 5), but did not apply potential income generated through CO2 emission reduction and wastewater treatment, as these were deemed covered by the savings made. Irrespective of location scenario, the lack of nitrogen-fertilization and the benefits of the self-settling properties of Tolypothrix sp. on energy saving for harvesting were considered by applying no costs for nitrogen fertilization and a 90% saving on dewatering costs (Table 5). Accordingly, total capital costs for constructing the 10 ha facility not co-located with a coal-fired power plant were estimated at US$ 853,442 for pond construction, CO2, nutrient supply, water-recirculation, and harvest/dewatering systems and land acquisition. The only direct capital cost avoided by co-location was for land acquisition (US$ 147,526), reducing the direct capital cost to US$ 705,916 (Table 5). Standardized indirect capital costs for engineering fees (including land acquisition), set at 15% and applying a 5% contingency were estimated to be US$ 170,688 for a not co-located facility, of which US$ 29,505 were avoided through free land provided in the co-location scenario, reducing indirect capital costs to US$ 141,183 (Table 5). A working capital of 5% of the total costs did not consider any of the benefits derived through co-location. Total annual operating costs for a not co-located facility was estimated to be US$ 2,440,633 for the 10 ha facility and included cost for salaries, maintenance and insurance, phosphate fertilizer and water requirements, CO2, energy for cultivation, dewatering and drying, and pigment extraction and purification (Table 5). Co-location resulted in significant operational savings of ∼70%, particularly through avoiding water and CO2 supply costs and a 90% saving on maintenance and insurance, and energy expenditure; the latter considered that energy would be purchased from the power station at 10% of the sales price to ordinary customers, and maintenance/insurance costs would also be 10% of ordinary costs. Therefore, annual operational costs were estimated to be US$ 758,241 when co-located (Table 5).
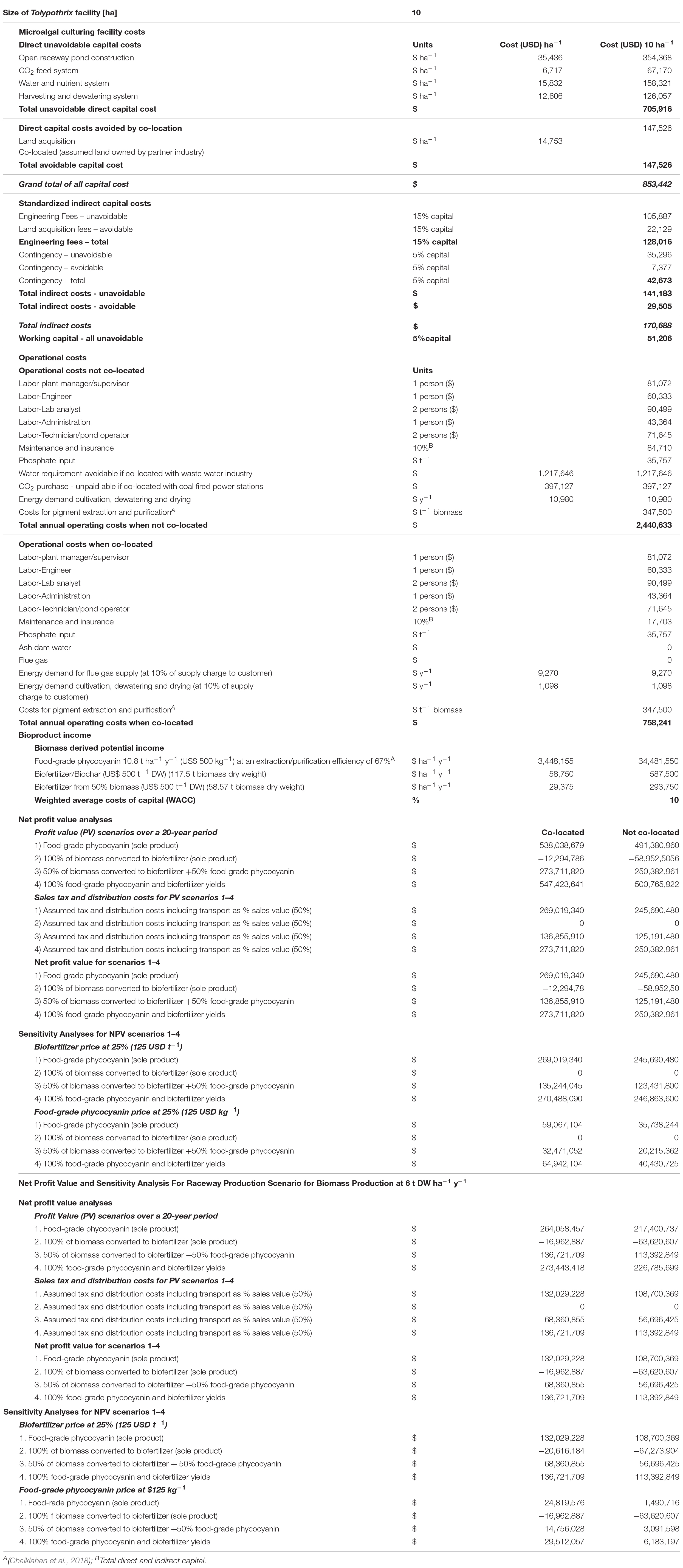
Table 5. Microalgal culturing facility capital and operating costs, product income potential, net profit value and sensitivity analyses.
Bioproduct-Generated Income, Net Present Value and Sensitivity Analyses
Bioproduct yields were estimated from biomass productivity and bioproduct productivities obtained in this study and for average productivities achieved for Nannochloropsis occulata in large-scale outdoor raceway cultivation. Based on this, it is estimated that a 10 ha facility could produce 117.5 and 60 t Tolypothrix sp. biomass ha–1 y–1 for a 300-day production period, respectively. The average phycocyanin content of Tolypothrix sp. used in this study is 8.8% (w/w), although as shown here, higher yields are possible. In order to put the NPV on a realistic footing, the average yields were used to calculate the yields of food-grade phycocyanin (A620 nm/A280 nm: 0.7), which can be extracted and purified with an efficiency of 67% (Chaiklahan et al., 2018). This equates to a production of 10.3 t unpurified phycocyanin ha–1 y–1, which yields 6.9 t food-grade phycocyanin ha–1 y–1, valued at US$ 3,448,155 ha–1 y–1 based on a sales price at US$ 500 kg–1 (Table 5). The final purified product will require metal analysis for quality assurance, which has not been considered as a cost, as costs for these analyses are expected to be absorbed by the coal-fired power plant the production would be collocated with. Furthermore, production of the food-grade phycocyanin produced when not collocated would not be subject to such analyses, i.e., in this scenario no metal analyses costs would be incurred. Biofertilizer/biochar can fetch a sales price of US$ 500 t–1, which, based on biomass productivities achieved here, equates to US$ 58,750 ha–1 y–1 (Table 5). Based on this, the predicted values for the modeled bioproduct scenarios are highest for co-located production for phycocyanin extraction in a biorefinery approach and conversion of the extracted biomass to biofertilizer (scenario 4, US$ 547,423,641), closely followed by producing food-grade phycocyanin as the sole product (scenario 1, US$ 538,038,679) (Table 5). Net present values were 50% lower based on assumed tax and distribution costs (Table 5).
In order to decide whether a project remains commercially viable, sensitivity analyses are essential. Accordingly, the weighted average costs of capital (WACC) is 10% and the sensitivity analyses modeled two scenarios: (1) reduction of the biofertilizer price to 25% of the current value (US$ 125 t–1) and (2) a food-grade phycocyanin price to 25% of the current value due to market saturation (US$ 125 kg–1). This showed that producing biofertilizer as the sole product (scenario 2) is not commercially viable even when the production facility is co-located. It is assumed that income for environmental services provided at the coal-fired power plant would not provide a strong business incentive. In contrast, producing phycocyanin as the sole product (scenario 1) remains to be of commercial interest (Table 5).
Discussion
Effect of CO2-Supplementation on Biomass Production
Successful cultivation of microalgae and cyanobacteria in wastewaters arising from energy -, mining - and mineral-processing industries would provide for a sustainable platform for production of algal biomass and bioproducts, whilst simultaneously providing for efficient bioremediation of potentially harmful nutrients and metals (Roberts et al., 2013). Significant growth challenges, hampering overall productivities and economics when using wastewater for production, must be addressed first for the realization of the true commercial potential.
This study demonstrated that CO2 supplementation significantly improved final phycobiliprotein and biomass yields, as well as biomass productivities of the diazotrophic cyanobacterium Tolypothrix sp. grown in SADW under outdoor conditions as biofilms of suspension cultures. Similar increases of up to 60% have been reported for biomass productivities using the non-diazotrophic cyanobacterium Arthrospira platensis for cultures supplemented with 1% CO2 (v/v) (Ravelonandro et al., 2011). In contrast, growth of Tolypothrix sp. was reduced by 60% under outdoor (this study) compared to indoor suspension cultivation under the same CO2-supplemented – and wastewater conditions (Velu et al., 2019). Growth of outdoor cultures is often generally lower due to difficulties in controlling cultivation and environmental conditions, such as hydrodynamics, temperature, UV irradiation and irradiance within optimal ranges (Chen et al., 2011). Light intensity is one of the major factors affecting cyanobacterial and microalgal growth. The optimal light intensity for growth of Tolypothrix sp. is ∼500 μmol photons m–2 s–1, however, observed light intensities at noon varied from 500 to 900 μmol photons m–2 s–1 (Supplementary Table S3). This could suggest that periodic photoinhibition might have occurred, especially in younger cultures with low cell concentrations. It must be emphasized that the reported CO2-supplemented growth performance was comparable to other microalgae and cyanobacteria grown in various cultivation systems but was achieved without nitrogen-fertilization (Table 6). In addition, biomass growth was phosphate-limited after ∼3 to 8 days of cultivation. It is therefore conceivable that phosphate fertilization at appropriate intervals should improve biomass yields and productivities further. High temperature and irradiance experienced in the ATS were the most likely factors impeding growth performance of biofilms of Tolypothrix sp. (Del Campo et al., 2007; Chen et al., 2011), a conclusion supported by higher growth performance of indoor-cultivated microalgal biofilms (Table 6).
The observed higher growth performance of Tolypothrix sp. when supplemented with CO2 could be attributable to positive effects on nitrogen – and photosynthesis-linked carbon fixation. For example, supplementation of cultures of the marine diazotrophic cyanobacterium Trichodesmium sp. with large amounts of CO2 resulted in a 20% increase in nitrogen fixation rates (Levitan et al., 2007). In addition, CO2 supplementation could have allowed for the reallocation of energy required for inorganic carbon (Ci) uptake and scavenging of O2. For instance, CO2-supplementation reduced the energy requirements for Ci uptake in Trichodesmium sp. by suppressing the energy-intensive carbon concentrating mechanism (CCM), which are employed under carbon-limiting conditions, freeing up this energy for the fixation of atmospheric nitrogen, another metabolic pathway with large energy requirements (Levitan et al., 2007). In contrast, despite a large effect of CO2-supplementation on biomass productivity, no effect was observed for phosphate requirements in biofilm and suspension-grown Tolypothrix sp., which was also observed for indoor-cultivated suspension cultures (Velu et al., 2019). This could represent a direct result of phosphate limitation after large uptake over the first 3 days of the growth period for replenishing internal phosphate stores, providing sufficient energy for CO2 fixation. In the context of deploying industrial cultivation of Tolypothrix sp. for the bioremediation of macronutrient-poor ash dam wastewaters at coal-fired power plants, the ability of sustained growth without requirements for nitrogen-fertilization offers a distinctive economical advantage. For example, nitrate provision for large-scale production of the non-diazotrophic Arthrospira platensis (synonym Spirulina platensis) was estimated to account for 50% of the overall production costs (Vonshak and Richmond, 1988). Energy savings provided by the self-settling ability of Tolypothrix sp. is another significant advantage for wastewater-utilizing large-scale cultivation, as costly dewatering infrastructure and energy requirements, that apply to the commonly used microalgal genera Chlorella spp. and Scenedesmus spp. (Silva and Silva, 2007), is abolished. For example, biofilms and self-flocculated biomass of Tolypothrix sp. were 80- and 53-fold more concentrated than the original suspension culture (Velu et al., 2015). Taken together, these properties reduce the need for finite chemical fertilizers and improve the overall economics of cultivation in macronutrient-limited ash dam wastewaters.
Effect of CO2-Supplementation on Biochemical Profiles, Metal Removal Capacity and Bioproduct Potential
CO2-fertilization of outdoor-grown biofilms and suspension cultures of Tolypothrix sp. resulted in increased total carbohydrate and lipid contents, as has also been reported for indoor cultivated suspension cultures (Velu et al., 2019). Similarly, an increase in CO2 supply from 5 to 25% (v/v) increased total carbohydrate and lipid contents of Scenedesmus bajacalifornicus by up to 20 and 10%, respectively (Patil and Kaliwal, 2017). Elevated CO2 supplies typically result in increased carbohydrate contents in microalgae, and are likely a result of enhanced photosynthetic efficiencies (Giordano, 2001) or CO2-induced low pH stress (Dragone et al., 2011). In contrast, 15% CO2 (v/v) supplementation led to an increase in pH from 6.0 to 9.0 and 7.0 to 9.0 for CO2-supplemented and non-CO2 controls under outdoor cultivation of Tolypothrix sp. biofilms and suspension cultures (Table 6), suggesting strongly that cultures were still carbon-limited (Coleman and Colman, 1981). Accounting for the fact that nitrogen-requirements for growth had to be met solely through nitrogen fixation, it is not surprising that CO2-supplementation had no effect on protein content for biomass cultivated in either system, which differs from other reported outcomes, where CO2-supplementation has been shown to correlate with improved nitrate uptake and thus higher protein production (Xia and Gao, 2005). As demonstrated by significant CO2-induced increases in the nitrogen-containing pigments phycocyanin and phycoerythrin, nitrogen supply through nitrogen fixation must have been sufficient under outdoor cultivation of Tolypothrix sp. The increase in the content of these pigments could have been also responsible for improved growth performance under CO2-supplementation, as they are accessory pigments for the capture of light in the light harvesting complexes of the photosystems and protect the photosynthetic apparatus from excess light and reactive oxygen damage (Chakdar and Pabbi, 2016).
Similar to the increase in phycobiliprotein contents, i.e., phycocyanin and phycoerythrin, CO2-supplementation resulted in 19 and 12% higher TFA contents in Tolypothrix sp. biofilms and suspension-grown biomass, which is similar to results obtained in indoor cultivation (Velu et al., 2019) and with eukaryotic microalgae (Tsuzuki et al., 1990), but no significant effect on MUFA or PUFA content was evident. α- (C18:3 ω-3) and γ-Linolenic acid (C18:3 ω-6) are important dietary supplements with critical health benefits, and the latter is also an ingredient in cosmetics (Ryckebosch et al., 2012). Similar to indoor suspension cultivation (Velu et al., 2019), Tolypothrix sp. produced 25% C18:3 ω-6 or 18.5 mg g–1 TFA, which is higher than reported for Arthrospira (Spirulina) sp. (11–16%) (De Oliveira et al., 1999). In contrast to phycocyanin, yields of (C18:3 ω-6), however, remained insufficient for consideration as a main target product in a biorefinery approach, due to low TFA contents characteristic for cyanobacteria.
Irrespective of cultivation system and CO2-fertilization, metal bioremediation of Tolypothrix sp. from macronutrient-poor ash dam wastewater, containing concentrations of Al, As, Cd, Ni, and Zn that exceed the ANZECC guidelines (Roberts et al., 2013), showed that levels were lowered to acceptance thresholds at the end of the cultivation period. This demonstrated that Tolypothrix sp. is a suitable organism for the bioremediation of metals from complex mixtures, under macronutrient-limiting conditions. The produced Tolypothrix sp. biomass was rich in carbon (45%) and nitrogen (7%), resulting in a C/N ratio of 6.58, similar to results obtained in indoor cultivation (Velu et al., 2019). In addition, the elemental composition and concentrations were comparable to those found in other cyanobacteria, previously reported as suitable for biofertilizer applications (Osman et al., 2010). Importantly, biomass of Tolypothrix sp. remained suitable at application rates required for the fertilization of wheat, supplying in addition to nitrogen and carbon also essential trace elements, such as Cu, Fe, Mo, and Zn (Velu et al., 2019). Diazotrophic cyanobacteria, such as Tolypothrix sp., are natural and renewable sources of biological nitrogen, contributing up to 30 kg N ha–1 and providing large quantities of organic matter and important plant hormones (i.e., gibberellin, auxin and cytokines) to soils, thereby improving soil fertility (Issa et al., 2014), supporting plant development and protecting against pathogens (Singh et al., 2016). Diazotrophic cyanobacteria, such as Tolypothrix sp., are commonly employed as biofertilizers in rice fields (Karthikeyan et al., 2007) and applications of Tolypothrix sp. specifically resulted in a 25% increase in crop yields in poorly drained rice fields (Watanabe et al., 1951). Long-term applications improved nitrogenous fertility of soils, attributable to the increase in soil carbon and nitrogen through accumulation of decomposing and live biomass, respectively (Watanabe, 1962). In summary, the above application potential, together with CO2-enhanced growth responses and phycocyanin yields, makes Tolypothrix sp. biomass production for use as a biofertilizer a real potential in regions, where agricultural production is located near freshwater-using coal-fired power plants, as is the case for the Tarong power station in Queensland, Australia.
To test and substantiate the commercial viability of Tolypothrix sp.-derived food-grade phycocyanin and biofertilizer, net present value and sensitivity analyses evaluated four bioproduct scenarios for the production of Tolypothrix sp. biomass under coal-fired power plant co-location and non-colocation of the production facility. Production in traditional raceway ponds was chosen, as no published data on the construction costs of large-scale production facilities using bubble columns could be found and a comparison of average microalgal and cyanobacterial maximal biomass productivities was not strongly influenced by cultivation system when operated under outdoor conditions (Heimann et al., 2015). In addition, biofilm cultivation was not considered in the modeled scenarios for several reasons. (1) Systems used in the study have a very large areal production footprint. (2) The areal productivity is low. (3) The systems are more prone to contamination by other microalgae when used for extended periods under outdoor conditions (Velu et al., 2015). (4) Establishment costs for large-scale production cannot be applied with any certainty, as biofilm cultivation systems vary significantly in design (Heimann, 2016). (5) As the biofilms were not harvested at regular intervals, it is impossible to determine the true yield potential of the systems at this stage, as regrowth of the remaining turf may have vastly different biomass production characteristics compared to freshly seeded turfs.
The modeled NPV and sensitivity analyses showed that the production of food-grade phycocyanin is advantageous for commercial viability, whether or not the facility would be co-located, whereas biofertilizer production as a sole product was not commercially viable in any of the modeled scenarios. Outcomes for biofertilizer income were similar to the commercial production of Azospirillum, a nitrogen-fixing bacterium, simulated for liquid biofertilizer production in Cuba, but the production scale for the plant was 4-fold larger in terms of product volume (Pérez Sánchez et al., 2018) than for the Tolypothrix sp. plant in the presented study. In that analysis, salary costs accounted for >50% of the production cost, as the process is labor-intensive, requiring 29 employees to man 24 h shifts (Pérez Sánchez et al., 2018), while production of Tolypothrix sp. biomass represented only 10% of the overall production costs. An NPV analysis for the commercial production of dried microalgal biomass (US$625 t–1) using dairy effluent as a nutrient and water supply also concluded that the process is commercially feasible for a plant size treating 1 million liter of dairy effluent over a 20-year period (Kumar et al., 2020). The sensitivity analyses using one quarter of today’s food-grade phycocyanin sales price demonstrated that facilities producing phycocyanin as a sole product or phycocyanin and biofertilizer remain commercially viable whether co-located or not. Instead of using product sales prices, reduction of biomass yields are an alternative parameter in sensitivity analyses. Reduction of biomass yields to one quarter of the original tonnage therefore had a comparable effect on NPV outcomes (Kumar et al., 2020). An obvious worst-case scenario for commercial production would be reduced yearly biomass yields (reduced to 33%) and reduced product sales prices (at 25% each of today’s sales prices). Applying this situation to the Tolypothrix sp. production scenario proposed here over the entire 20-year period determined that production of food-grade phycocyanin as a sole product, 50% of biomass extraction for each food-grade phycocyanin and biofertilizer, and 100% food-grade phycocyanin with the residual biomass converted to biofertilizer (100%) remain commercially feasible irrespective of co-location or not for the bubble column production scenario, but only when co-located for the raceway production simulation. Therefore, with regards to a decision whether co-location offers significant benefits, the profit difference predicted here would be as large as ∼US$ 23 million over 20 years for production of 100% food-grade phycocyanin at a quarter of today’s sale price with 100% co-production of biofertilizer. This provides a significant incentive for co-locating production facilities with CO2-polluting and metal-rich wastewater generating industries, for the simultaneous application of the environmental services of diazotrophic cyanobacteria and bioproduct development.
Conclusion
This study demonstrated significantly enhanced biomass and phycocyanin yields and productivities in response to CO2-fertilization and excellent metal removal capacity of Tolypothrix sp. cultivated under outdoor conditions in meso-scale systems without supply of nitrogen fertilization. This makes Tolypothrix sp. an outstanding candidate for bioremediation of CO2 and metals at freshwater-utilizing coal-fired power plants. Obtained growth performance suggests that 10.91 and 117.5 t dry biomass ha–1 can be produced in a year set at 300 days of cultivation in biofilms and vertical bag suspension cultures, respectively. The NPV and sensitivity analyses performed with production data obtained in this study and modeled for a simulated raceway production scenario, taking the organism’s self-settling ability into account, demonstrated that co-location with coal-fired power plants was not essential for commercial viability, but significantly increased achievable net present values for all modeled product scenarios, making it an attractive proposition, if freshwater-utilizing plants are in close proximity to agricultural land. The most profitable scenario was production of food-grade phycocyanin (100%) coupled with co-production of biofertilizer (100%), followed by food-grade phycocyanin as the sole product, and 50% of each phycocyanin and biofertilizer production. In contrast, production of biofertilizer as a sole product was not commercially viable under any of the modeled scenarios. Based on the above, cultivation of Tolypothrix sp. in vertical suspension cultures with CO2 supply, but without nitrogen-fertilization is recommended for the production of food-grade phycocyanin either as a sole product or with co-production of biofertilizer.
Data Availability Statement
The raw data supporting the conclusions of this article will be made available by the authors, without undue reservation, to any qualified researcher.
Author Contributions
KH, SC, and CV were responsible for the experimental design of this study and analyzed all the data. CV carried out the experimental work of the outdoor growth experiments, protein – and phycobiliprotein contents. DB and CV conducted the analysis and quantification of biomass lipid content and fatty acid profiles. KH and CV performed the NPV and sensitivity analyses. KH, CV, SC, and DB jointly assembled and critically interpreted these data for publication. All authors contributed to writing the manuscript, provide approval for publication, and agreed to be accountable for all aspects of the work.
Funding
KH acknowledges grant funding by the Advanced Manufacturing Co-operative Research Centre (AMCRC), Melbourne, Australia, grant number 2.3.4. CV gratefully acknowledges receipt of an AMCRC Ph.D. scholarship and JCU tuition fee waiver, receipt of project funding through the Graduate Research School at James Cook University (JCU) and Essential Aquaculture.
Conflict of Interest
The authors declare that the research was conducted in the absence of any commercial or financial relationships that could be construed as a potential conflict of interest.
Acknowledgments
The authors thank the Advanced Analytical Centre at James Cook University for metal analysis, the Australian Institute of Marine Science for fatty acid, and hydrocarbon analyses and OEA Labs Ltd., for elemental analysis.
Supplementary Material
The Supplementary Material for this article can be found online at: https://www.frontiersin.org/articles/10.3389/fbioe.2020.00051/full#supplementary-material
References
Abed, R. M. M., Dobretsov, S., and Sudesh, K. (2009). Applications of cyanobacteria in biotechnology. J. Appl. Microbiol. 106, 1–12. doi: 10.1111/j.1365-2672.2008.03918.x
Artanto, Y., Jansen, J., Pearson, P., Puxty, G., Cottrell, A., Meuleman, E., et al. (2014). Pilot-scale evaluation of AMP/PZ to capture CO2 from flue gas of an Australian brown coal–fired power station. Int. J. Greenhous Gas Convers. 20, 189–195. doi: 10.1016/j.ijggc.2013.11.002
Aslam, A., Thomas-Hall, S. R., Mughal, T., Zaman, Q.-U., Ehsan, N., Javied, S., et al. (2019). Heavy metal bioremediation of coal-fired flue gas using microalgae under different CO2 concentrations. J. o Environ. Manag. 241, 243–250. doi: 10.1016/j.jenvman.2019.03.118
Benemann, J. R. (1979). Production of nitrogen fertilizer with nitrogen-fixing blue-green algae. Enzyme Microbial Technol. 1, 83–90. doi: 10.1016/0141-0229107990103-90100
Bermejo, P., Piñero, E., and Villar, ÁM. (2008). Iron-chelating ability and antioxidant properties of phycocyanin isolated from a protean extract of Spirulina platensis. Food Chem. 110, 436–445. doi: 10.1016/j.foodchem.2008.02.021
Bhayani, K., Mitra, M., Ghosh, T., and Mishra, S. (2016). C-Phycocyanin as a potential biosensor for heavy metals like Hg2+ in aquatic systems. RSC Adv. 6, 111599–111605. doi: 10.1039/C6RA22753H
Borowitzka, M. A. (2013). High-value products from microalgae—their development and commercialisation. J. Appl. Phycol. 25, 743–756. doi: 10.1007/s10811-013-9983-9
Chaiklahan, R., Chirasuwan, N., Loha, V., Tia, S., and Bunnag, B. (2018). Stepwise extraction of high-value chemicals from Arthrospira (Spirulina) and an economic feasibility study. Biotechnol. Rep. 20:e00280. doi: 10.1016/j.btre.2018.e00280
Chakdar, H., and Pabbi, S. (2016). “Cyanobacterial phycobilins: production, purification, and regulation,” in Frontier Discoveries and Innovations in Interdisciplinary Microbiology, 1 Edn, ed. P. Shukla, (New York, NY: Springer), 45–69. doi: 10.1007/978-81-322-2610-9_4
Chen, C.-Y., Yeh, K.-L., Aisyah, R., Lee, D.-J., and Chang, J.-S. (2011). Cultivation, photobioreactor design and harvesting of microalgae for biodiesel production: a critical review. Bioresour. Technol. 102, 71–81. doi: 10.1016/j.biortech.2010.06.159
Cheng, P., Ji, B., Gao, L., Zhang, W., Wang, J., and Liu, T. (2013). The growth, lipid and hydrocarbon production of Botryococcus braunii with attached cultivation. Bioresour. Technol. 138, 95–100. doi: 10.1016/j.biortech.2013.03.150
Chiang, C.-L., Lee, C.-M., and Chen, P.-C. (2011). Utilization of the cyanobacteria Anabaena sp. CH1 in biological carbon dioxide mitigation processes. Bioresour. Technolo. 102, 5400–5405. doi: 10.1016/j.biortech.2010.10.089
Cohen, Z. (1997). “The chemicals of Spirulina.,” in Spirulina platensis (Arthrospira): Physiology, cell Biology and Biotechnology, ed. A. Vonshak, (London: Taylor and Francis), 175–204.
Coleman, J. R., and Colman, B. (1981). Inorganic carbon accumulation and photosynthesis in a blue-green alga as a function of external pH. Plant Physiol. 67, 917–921. doi: 10.1104/pp.67.5.917
Davis, R., Aden, A., and Pienkos, P. T. (2011). Techno-economic analysis of autotrophic microalgae for fuel production. Appl. Energy 88, 3524–3531. doi: 10.1016/j.apenergy.2011.04.018
De Morais, M. G., and Costa, J. A. V. (2007). Biofixation of carbon dioxide by Spirulina sp. and Scenedesmus obliquus cultivated in a three-stage serial tubular photobioreactor. J. Biotechnol. 129, 439–445. doi: 10.1016/j.jbiotec.2007.01.009
De Oliveira, M. A. C. L., Monteiro, M. P. C., Robbs, P. G., and Leite, S. G. F. (1999). Growth and chemical composition of Spirulina maxima and Spirulina platensis biomass at different temperatures. Aquac. Int. 7, 261–275.
De Philippis, R., Colica, G., and Micheletti, E. (2011). Exopolysaccharide-producing cyanobacteria in heavy metal removal from water: molecular basis and practical applicability of the biosorption process. Appl Microbiol. Biotechnol. 92, 697–708. doi: 10.1007/s00253-011-3601-z
Del Campo, J. A., García-González, M., and Guerrero, M. G. (2007). Outdoor cultivation of microalgae for carotenoid production: current state and perspectives. Appl. Microbiol. Biotechnol. 74, 1163–1174. doi: 10.1007/s00253-007-0844-9
Dineshbabu, G., Uma, V. S., Mathimani, T., Deviram, G., Ananth, D. A., Prabaharan, D., et al. (2017). On-site concurrent carbon dioxide sequestration from flue gas and calcite formation in ossein effluent by a marine cyanobacterium Phormidium valderianum BDU 20041. Energy Convers. Manag. 141, 315–324. doi: 10.1016/j.enconman.2016.09.040
Doshi, A. (2017). Economic Analyses of Microalgae Biofuels and Policy Implications in Australia. Brisbane: Queensland University of Technology.
Dragone, G., Fernandes, B. D., Abreu, A. P., Vicente, A. A., and Teixeira, J. A. (2011). Nutrient limitation as a strategy for increasing starch accumulation in microalgae. Appl. Energy 88, 3331–3335. doi: 10.1016/j.apenergy.2011.03.012
Dubey, S. K., Dubey, J., Mehra, S., Tiwari, P., and Bishwas, A. J. (2011). Potential use of cyanobacterial species in bioremediation of industrial effluents. Afr. J. Biotechnol, 10, 1125–1132. doi: 10.1007/s10295-008-0452-4
El-Bestawy, E. (2008). Treatment of mixed domestic–industrial wastewater using cyanobacteria. J. Indust. Microbiol. Biotechnol. 35, 1503–1516. doi: 10.1007/s10295-008-0452-4
El-Sheekh, M. M., El-Shouny, W. A., Osman, M. E. H., and El-Gammal, E. W. E. (2005). Growth and heavy metals removal efficiency of Nostoc muscorum and Anabaena subcylindrica in sewage and industrial wastewater effluents. Environ. Toxicol. Pharmacol. 19, 357–365. doi: 10.1016/j.etap.2004.09.005
Fornarelli, R., Bahri, P. A., and Moheimani, N. (2017). Utilization of Microalgae to Purify Waste Streams and Production of Value Added Products. North Sydney: Australian Meat Processor Corporation.
Gelagutashvili, E., and Tsakadze, K. (2013). Effect of Hg(II) and Pb(II) ions on C-phycocyanin (Spirulina platensis). Opt. Photonics J. 3, 122–127. doi: 10.4236/opj.2013.31020
Giordano, M. (2001). Interactions between C and N metabolism in Dunaliella salina cells cultured at elevated CO2 and high N concentrations. J Plant Physiol. 158, 577–581. doi: 10.1078/0176-1617-00234
Gour, R., Kant, A., and Singh Chuahan, R. (2014). Screening of micro algae for growth and lipid accumulation properties. J. Algal Biomass Util. 5, 38–46.
Griffin, G. J., and Batten, D. F. (2013). The economics of producing biodiesel from micro-algae in the Asua Pacific region. Int. J. Renew. Energy Deve. 2, 105–113.
Gross, M., and Wen, Z. (2014). Yearlong evaluation of performance and durability of a pilot-scale revolving algal biofilm (RAB) cultivation system. Bioresour. Technol. 171, 50–58. doi: 10.1016/j.biortech.2014.08.052
Gupta, V., Ratha, S. K., Sood, A., Chaudhary, V., and Prasanna, R. (2013). New insights into the biodiversity and applications of cyanobacteria (blue-green algae)—Prospects and challenges. Algal Res, 2, 79–97. doi: 10.1016/j.algal.2013.01.006
Hall, D. O., Markov, S. A., Watanabe, Y., and Rao, K. K. (1995). The potential applications of cyanobacterial photosynthesis for clean technologies. Photosynth. Res. 46, 159–167. doi: 10.1007/BF00020426
Heimann, K. (2016). Novel approaches to microalgal and cyanobacterial cultivation for bioenergy and biofuel production. Curr. Opini. Biotechnol. 38, 183–189. doi: 10.1016/j.copbio.2016.02.024
Heimann, K., Cires, S., and Karthikeyan, O. P. (2015). “Cultivation of microalgae: Implications for the carbon footprint of aquaculture and agriculture industries,” in The Carbon Footprint Handbook, ed. S. S. Muthu, (Boca Raton: CRC Press), 406–447.
Heimann, K., Karthikayan, O. P., and Cirés, S. (2013). Turning bad gas into good solids. Qld. Min. Energy Bull. (Spring) 92–96.
Ho, S.-H., Chen, C.-Y., Lee, D.-J., and Chang, J.-S. (2011). Perspectives on microalgal CO2-emission mitigation systems—a review. Biotechnol. Adv. 29, 189–198. doi: 10.1016/j.biotechadv.2010.11.001
Hoh, D., Watson, S., and Kan, E. (2016). Algal biofilm reactors for integrated wastewater treatment and biofuel production: a review. Chem. Eng. J. 287, 466–473. doi: 10.1016/j.biotechadv.2011.05.015
Issa, A. A., Abd-Alla, M. H., and Ohyama, T. (2014). “Nitrogen fixing cyanobacteria: future prospect,” in Advances in Biology and Ecology of Nitrogen Fixation, ed. T. Ohyama, (london: e-book: InTech).
Ji, B., Zhang, W., Zhang, N., Wang, J., Lutzu, G. A., and Liu, T. (2014). Biofilm cultivation of the oleaginous microalgae Pseudochlorococcum sp. Bioprocess Biosyst. Eng 37, 1369–1375. doi: 10.1007/s00449-013-1109-x
Kao, C.-Y., Chiu, S.-Y., Huang, T.-T., Dai, L., Wang, G.-H., Tseng, C.-P., et al. (2012). A mutant strain of microalga Chlorella sp. for the carbon dioxide capture from biogas. Biomass Bioenergy 36, 132–140. doi: 10.1016/j.biombioe.2011.10.046
Karthikeyan, N., Prasanna, R., Nain, L., and Kaushik, B. D. (2007). Evaluating the potential of plant growth promoting cyanobacteria as inoculants for wheat. Eur. J. Soil Biol. 43, 23–30. doi: 10.1016/j.ejsobi.2006.11.001
Kumar, A. K., Sharma, S., Dixit, G., Shah, E., and Patel, A. (2020). Techno-economic analysis of microalgae production with simultaneous dairy effluent treatment using a pilot-scale High Volume V-shape pond system. Renew. Energy 145, 1620–1632. doi: 10.1016/j.renene.2019.07.087
Levitan, O., Rosenberg, G., Setlik, I., Setlikova, E., Grigel, J., Klepetar, J., et al. (2007). Elevated CO2 enhances nitrogen fixation and growth in the marine cyanobacterium Trichodesmium. Global Change Biol. 13, 531–538. doi: 10.1104/pp.110.159145
López, C. G., Fernández, F. A., Sevilla, J. F., Fernández, J. S., García, M. C., and Grima, E. M. (2009). Utilization of the cyanobacteria Anabaena sp. ATCC 33047 in CO2 removal processes. Bioresour. Technol. 100, 5904–5910. doi: 10.1016/j.biortech.2009.04.070
Markou, G., and Georgakakis, D. (2011). Cultivation of filamentous cyanobacteria (blue-green algae) in agro-industrial wastes and wastewaters: a review. Appl. Energy 88, 3389–3401. doi: 10.1016/j.apenergy.2010.12.042
Moheimani, N. R. (2016). Tetraselmis suecica culture for CO2 bioremediation of untreated flue gas from a coal-fired power station. J. Appl. Phycol. 28, 2139–2146. doi: 10.1007/s10811-015-0782-3
Moreno, J., Vargas, M. A., Olivares, H., Rivas, J., and Guerrero, M. G. (1998). Exopolysaccharide production by the cyanobacterium Anabaena sp. ATCC 33047 in batch and continuous culture. J. Biotechnol. 60, 175–182. doi: 10.1016/s0168-1656(98)00003-0
Osman, M. E. H., El-Sheekh, M. M., El-Naggar, A. H., and Gheda, S. F. (2010). Effect of two species of cyanobacteria as biofertilizers on some metabolic activities, growth, and yield of pea plant. Biol. Fertil. Soils 46, 861–875. doi: 10.1007/s00374-010-0491-7
Patil, L., and Kaliwal, B. (2017). Effect of CO2 concentration on growth and biochemical composition of newly isolated indigenous microalga Scenedesmus bajacalifornicus BBKLP-07. Appl. Biochemi. Biotechnol. 182, 335–348. doi: 10.1007/s12010-016-2330-2
Pereira, S., Micheletti, E., Zille, A., Santos, A., Moradas-Ferreira, P., Tamagnini, P., et al. (2011). Using extracellular polymeric substances (EPS)-producing cyanobacteria for the bioremediation of heavy metals: do cations compete for the EPS functional groups and also accumulate inside the cell? Microbiology 157, 451–458. doi: 10.1099/mic.0.041038-0
Pérez Sánchez, A., Singh, S., Pérez Sánchez, E. J., and Segura Silva, R. M. (2018). Techno-economic evaluation and conceptual design of a liquid biofertilizer plant. Rev. Colomb. Biotecnol. 20, 6–18.
Pierobon, S. C., Cheng, X., Graham, P. J., Nguyen, B., Karakolis, E. G., and Sinton, D. (2018). Emerging microalgae technology: a review. Sustain. Energy Fuels 2, 13–38. doi: 10.1039/c7se00236j
Querques, N., Cesta, M., Santos, R. M., and Chiang, Y. W. (2015). Microalgal phycocyanin productivity: strategies for phyco-valorization. J. Chemi. Technol. Biotechnol. 90, 1968–1982. doi: 10.1002/jctb.4796
Ravelonandro, P. H., Ratianarivo, D. H., Joannis-Cassan, C., Isambert, A., and Raherimandimby, M. (2011). Improvement of the growth of Arthrospira (Spirulina) platensis from Toliara (Madagascar): effect of agitation, salinity and CO2 addition. Food Bioprod. Proce. 89, 209–216. doi: 10.1016/j.fbp.2010.04.009
Roberts, D. A., Paul, N. A., Bird, M. I., and de Nys, R. (2015). Bioremediation for coal-fired power stations using macroalgae. J f Environ. Manag. 153, 25–32. doi: 10.1016/j.jenvman.2015.01.036
Roberts, G. W., Fortier, M.-O. P., Sturm, B. S. M., and Stagg-Williams, S. M. (2013). Promising pathway for algal biofuels through wastewater cultivation and hydrothermal conversion. Energy Fuels 27, 857–867. doi: 10.1021/ef3020603
Ruangsomboon, S., Chidthaisong, A., Bunnag, B., Inthorn, D., and Narumon, W. H. (2007). Lead (Pb2+) adsorption characteristics and sugar composition of capsular polysaccharides of cyanobacterium Calothrix marchica. J. Sci. Technol. 29, 529–541.
Ryckebosch, E., Bruneel, C., Muylaert, K., and Foubert, I. (2012). Microalgae as an alternative source of omega-3 long chain polyunsaturated fatty acids. Lipid Technol. 24, 128–130. doi: 10.1002/lite.201200197
Saunders, R. J., Paul, N. A., Hu, Y., and de Nys, R. (2012). Sustainable sources of biomass for bioremediation of heavy metals in waste water derived from coal-fired power generation. PLoS One 7:e36470. doi: 10.1371/journal.pone.0036470
Schenk, P. (2016). On-farm Algal Ponds to Provide Protein for Northern Cattle. North Sydney NSW: Meat and Lifestock Australia.
Schultze, L. K. P., Simon, M., Li, T., Langenbach, D., Podola, B., and Melkonian, M. (2015). High light and carbon dioxide optimize surface productivity in a twin-layer biofilm photobioreactor. Algal Res. 8, 37–44. doi: 10.1016/j.algal.2015.01.007
Setta, B. R. S., Barbarino, E., Passos, F. B., and Lourenço, S. O. (2017). An assessment of the usefulness of the cyanobacterium Synechococcus subsalsus as a source of biomass for biofuel production. Latin Am. J. Aqu. Res. 42, 364–375. doi: 10.3856/vol42-issue2-fulltext-7
Silva, P. G., and Silva, H. J. (2007). Effect of mineral nutrients on cell growth and self-flocculation of Tolypothrix tenuis for the production of a biofertilizer. Bioresour. Technol. 98, 607–611. doi: 10.1016/j.biortech.2006.02.026
Singh, J. S., Kumar, A., Rai, A. N., and Singh, D. P. (2016). Cyanobacteria: a precious bio-resource in agriculture, ecosystem, and environmental sustainability. Front. Microbiol. 7:529. doi: 10.3389/fmicb.2016.00529
Stock, A. (2014). “Australia’s electricity sector,” in Ageing, Inefficient and Unprepared, ed. C. Council, (Hobart: Australia Climate Council of Australian Ltd).
Tsuzuki, M., Ohnuma, E., Sato, N., Takaku, T., and Kawaguchi, A. (1990). Effects of CO2 concentration during growth on fatty acid composition in microalgae. Plant Physiol. 93, 851–856. doi: 10.1104/pp.93.3.851
Velu, C., Cirés, S., Alvarez-Roa, C., and Heimann, K. (2015). First outdoor cultivation of the N2-fixing cyanobacterium Tolypothrix sp. in low-cost suspension and biofilm systems in tropical Australia. J. Appli. Phycol. 27, 1743–1753. doi: 10.1007/s10811-014-0509-x
Velu, C., Cirés, S., Brinkman, D. L., and Heimann, K. (2019). Effect of CO2 and metal-rich waste water on bioproduct potential of the diazotrophic freshwater cyanobacterium. Tolypothrix sp. Heliyon 5:e01549. doi: 10.1016/j.heliyon.2019.e01549
von Alvensleben, N., and Heimann, K. (2019). “The potential of microalgae for biotechnology: a focus on carotenoids,” in Blue Biotechnology: Production and Use of Marine Molecules, eds S. La Barre and S. Bates, (London: Wiley & Sons), 117–142. doi: 10.1002/9783527801718.ch4
von Alvensleben, N., Stookey, K., Magnusson, M., and Heimann, K. (2013). Salinity tolerance of Picochlorum atomus and the use of salinity for contamination control by the freshwater cyanobacterium Pseudanabaena limnetica. PloS One 8:e63569. doi: 10.1371/journal.pone.0063569
Vonshak, A., and Richmond, A. (1988). Mass production of the blue-green alga Spirulina: an overview. Biomass 15, 233–247. doi: 10.1016/0144-4565(88)90059-5
Wang, B., Li, Y., Wu, N., and Lan, C. Q. (2008). CO2 bio-mitigation using microalgae. Appl. Microbiol. Biotechnol. 79, 707–718. doi: 10.1007/s00253-008-1518-y
Watanabe, A. (1962). Effect of nitrogen-fixing blue-green alga: Tolypothrix tenuis on the nitrogenous fertility of paddy soil and on the crop yield of rice plant. J. Gen. Appl. Microbiol. 8, 85–91. doi: 10.2323/jgam.8.85
Watanabe, A., Nishigaki, S., and Konishi, C. (1951). Effect of nitrogen-fixing blue-green algae on the growth of rice plants. Nature 168:748. doi: 10.1038/168748b0
Weckesser, J., Hofmann, K., Jürgens, U. J., Whitton, B. A., and Raffelsberger, B. (1988). Isolation and chemical analysis of the sheaths of the filamentous cyanobacteria Calothrix parietina and C. scopulorum. Microbiology 134, 629–634. doi: 10.1099/00221287-134-3-629
Xia, J. R., and Gao, K. S. (2005). Impacts of elevated CO2 concentration on biochemical composition, carbonic anhydrase, and nitrate reductase activity of freshwater green algae. J. Integ. Plant Biol. 47, 668–675. doi: 10.1111/j.1744-7909.2005.00114.x
Zhang, K., Kurano, N., and Miyachi, S. (1999). Outdoor culture of a cyanobacterium with a vertical flat-plate photobioreactor: effects on productivity of the reactor orientation, distance setting between the plates, and culture temperature. Appl. Microbiol. Biotechnol. 52, 781–786. doi: 10.1007/s002530051591
Keywords: biofertilizer, bioremediation, metals, biorefinery, coal-fired power, phycobiliproteins, nitrogen-fixing cyanobacteria, economics
Citation: Velu C, Cirés S, Brinkman DL and Heimann K (2020) Bioproduct Potential of Outdoor Cultures of Tolypothrix sp.: Effect of Carbon Dioxide and Metal-Rich Wastewater. Front. Bioeng. Biotechnol. 8:51. doi: 10.3389/fbioe.2020.00051
Received: 29 October 2019; Accepted: 22 January 2020;
Published: 11 February 2020.
Edited by:
Manfred Zinn, HES-SO Valais-Wallis, SwitzerlandReviewed by:
Sergio Revah, Autonomous Metropolitan University, MexicoChanghong Yao, Sichuan University, China
Copyright © 2020 Velu, Cirés, Brinkman and Heimann. This is an open-access article distributed under the terms of the Creative Commons Attribution License (CC BY). The use, distribution or reproduction in other forums is permitted, provided the original author(s) and the copyright owner(s) are credited and that the original publication in this journal is cited, in accordance with accepted academic practice. No use, distribution or reproduction is permitted which does not comply with these terms.
*Correspondence: Kirsten Heimann, a2lyc3Rlbi5oZWltYW5uQGZsaW5kZXJzLmVkdS5hdQ==