Toward Biomimetic Scaffolds for Tissue Engineering: 3D Printing Techniques in Regenerative Medicine
- 1Center for Biomaterials, Korea Institute of Science and Technology, Seoul, South Korea
- 2KU-KIST Graduate School of Converging Science and Technology, Korea University, Seoul, South Korea
- 3Department of Orthopedic Surgery, Korea University Anam Hospital, Seoul, South Korea
- 4School of Electrical and Electronic Engineering, Yonsei University, Seoul, South Korea
Three-dimensional (3D) printing technology allows fabricating complex and precise structures by stacking materials layer by layer. The fabrication method has a strong potential in the regenerative medicine field to produce customizable and defect-fillable scaffolds for tissue regeneration. Plus, biocompatible materials, bioactive molecules, and cells can be printed together or separately to enhance scaffolds, which can save patients who suffer from shortage of transplantable organs. There are various 3D printing techniques that depend on the types of materials, or inks, used. Here, different types of organs (bone, cartilage, heart valve, liver, and skin) that are aided by 3D printed scaffolds and printing methods that are applied in the biomedical fields are reviewed.
Introduction
Three-dimensional (3D) printing, also known as additive manufacturing, is a method that can fabricate objects with complex structures by depositing materials, i.e., metals, polymers, and ceramics, layer by layer (He et al., 2014; Hung et al., 2014). A 3D object can be produced through 3D scanning technology, such as computed tomography (CT) and computer-aided design (CAD) software (Hollister, 2005; Melchels et al., 2010). After image file of an object is acquired; it is converted to an STL file format that can be sliced into layers to create a 3D model (Melchels et al., 2010; Zorlutuna et al., 2012). Charles W. Hull, the president of 3D SYSTEMS, invented the first 3D printer, which was based on a stereolithography apparatus (SLA) technique (Melchels et al., 2010; Murphy and Atala, 2014; Schubert et al., 2014). The SLA printing method obtained issued a patent in 1986 (Gross et al., 2014). In 1990, a fused deposition modeling (FDM)-type printer was developed by Scott Crump, chairman of STRATASYS (Rengier et al., 2010; Gross et al., 2014). These 3D printing techniques sent shockwaves throughout multiple industries, such as automotive, aerospace, architecture, fashion, as well as bio-medicine (Duoss et al., 2014; Gross et al., 2014), since complex 3D structures can be precisely controlled and easily produced compared to subtractive methods (He et al., 2014; Choi and Kim, 2015).
In the medical fields, 3D printing technology is a promising tool for personalized treatments (Choi and Kim, 2015; Jakus et al., 2015; Kim et al., 2016). 3D models of a patient’s damaged organ can be produced to serve as a visual aid for the surgeons and to help the patient to understand his or her conditions (Melchels et al., 2010; Gross et al., 2014). Additionally, 3D printing techniques are applied to produce scaffolds and implants for regenerative medicine (He et al., 2014; Torres-Rendon et al., 2015; Kim et al., 2018, Lee et al., 2019). For example, Mankovich et al. (1994) used additive manufacturing to fabricate calvarial bone grafts, and Morrison et al. (2015) designed 3D printed scaffolds to treat tracheobronchomalacia. There are various 3D printing techniques that are classified by the types of materials and printing methods that are used to create an object (Table 1; Sachlos and Czernuszka, 2003; Childers et al., 2015). FDM is the most common 3D printing method. Thermoplastic filaments are melted by a heating block, and then a nozzle head directs the extrusion of the melted filaments to deposit thin layers (Azari and Nikzad, 2009; Gross et al., 2014). One of the advantages of FDM is that there are wide ranges of biodegradable and biocompatible materials, or filaments, which can be printed. Additionally, toxic organic solvents are not required to dissolve the polymeric filaments for printing (Yang et al., 2002; Leong et al., 2003). For example, Hutmacher et al. (2001) fabricated poly(ε-caprolactone) (PCL) scaffolds with a honeycomb-like porous structure using an FDM-type printer. Fibroblasts were able to proliferate and differentiate on the scaffold. However, the high temperature applied during the melt-extrusion stage can change the inherent material properties, and high-resolution printing is challenging (Yang et al., 2002). SLA type is based on solidification of liquid resin through photo-crosslinking (Melchels et al., 2010). A stage, or a base plate, for an object is immersed in liquid resin, and then the laser beam is applied to cure the resin on the stage. After the first layer is produced, the stage moves downward and the second layer is cured to deposit on the first layer (Gross et al., 2014). A selective laser sintering (SLS) printer follows a similar process to the SLA type, but the high-powered laser is applied to sinter solid powders (Leong et al., 2003). SLA-type printers can produce objects with high resolution and design more precise structures compared to the FDM technique. However, they are limited to photo-polymerizing resins, and the resins are often toxic for biomedical applications. The SLS method does not require liquid resins or toxic organic solvents to dissolve polymers, yet the sintering process can damage materials that are biodegradable (Yang et al., 2002; Williams et al., 2005). 3D plotting is an extrusion-based technology, which expels materials from a chamber by pneumatics (Sachlos and Czernuszka, 2003). Typically, plotting pastes and viscose materials are used as printing inks (Luo et al., 2013), which are either directly printed or melted in a feeding channel before they are extruded by a pneumatic pump (Ragaert et al., 2010). Due to the mild printing conditions, various soft materials (i.e., hydrogels, biocompatible polymers, and cell spheroids) can be printed with the 3D plotting method, and it is also referred to as bioprinting when cells are printed with hydrogel inks (Murphy and Atala, 2014). Haberstroh et al. (2010) were able to fabricate 3D plotted cell-seeded scaffolds of poly(L-lactide-co-glycolide) (PLGA), tricalcium phosphate (TCP)/collagen, and TCP/collagen/chitosan successfully for bone regeneration. The bioactivity of scaffolds and bone formation in calvarial defect model were evaluated. Lee et al. (2019) developed a new type of 3D bioprinting method, which allows printing a more precise and complex organ structure using hydrogel inks. The collagen ink was printed within a thermo-reversible support bath of gelatin microparticles to reproduce patient-specific cardiac ventricles. Inkjet printing is also widely used in regenerative medicine. It is a droplet-based extrusion printing technique, where droplets from the supplied fluid are deposited layer by layer, or patterned to desired shapes with biomolecules (Xu et al., 2013). Inkjet printing methods are cost-effective and applied in various fields from drug screening to tissue engineering (Boland et al., 2006); however, it is challenging to print viscous materials and cells (Koch et al., 2010). The laminated object manufacturing (LOM) process builds polymeric and metallic layers that are sequentially fed from a roller (Park et al., 2000). Laminates are cut with CO2 laser, and then layers are bonded by a heated roller. This rapid prototyping process can fabricate large objects with low cost (Murr(ed.)., 2015); however, it is challenging to make small and precise structures (Yang et al., 2002).
Every year, millions of patients are waiting organ donors and suffer from long transplant waiting lists. Tissue engineering has a potential not only to solve the current complications in organ shortage but also to improve the current level of the biomedical technology (Khademhosseini et al., 2006; Slaughter et al., 2009). Vacanti et al. (1988) established seminal work in the field of tissue engineering in the 1980s, and it is still one of the most researched fields. Conventionally, most scaffolds for tissue engineering were fabricated through a “top-down” approach, where scaffolds are designed with biocompatible polymeric materials in porous structures to biomimic the host tissue. However, for cell attachment and proliferation, the scaffolds were coated with bioactive substances, or surface modification was necessary. In contrast, the “bottom-up” approach aims to encapsulate cells in hydrogels to allow self-assembly of cell aggregation and 3D print cells directly in the form of a scaffold (Figure 1). An ideal scaffold has to possess a surface that is suitable for cell attachment and 3D inter-connected porous structures for extracellular matrix (ECM) formation and vascularization. 3D printing allows fabricating scaffolds with more controlled and precise structures (Derby, 2012; Do et al., 2015) compared to electro-spinning (Poologasundarampillai et al., 2011), foaming (Mahony et al., 2010), freeze-drying (Connell et al., 2014), and salt-leaching (Woodard et al., 2017) techniques. Here, we review 3D printing technologies for regenerative medicine, from 3D printed polymeric scaffolds to bio-artificial tissues, and promising outlooks for advanced treatments through 3D printing.
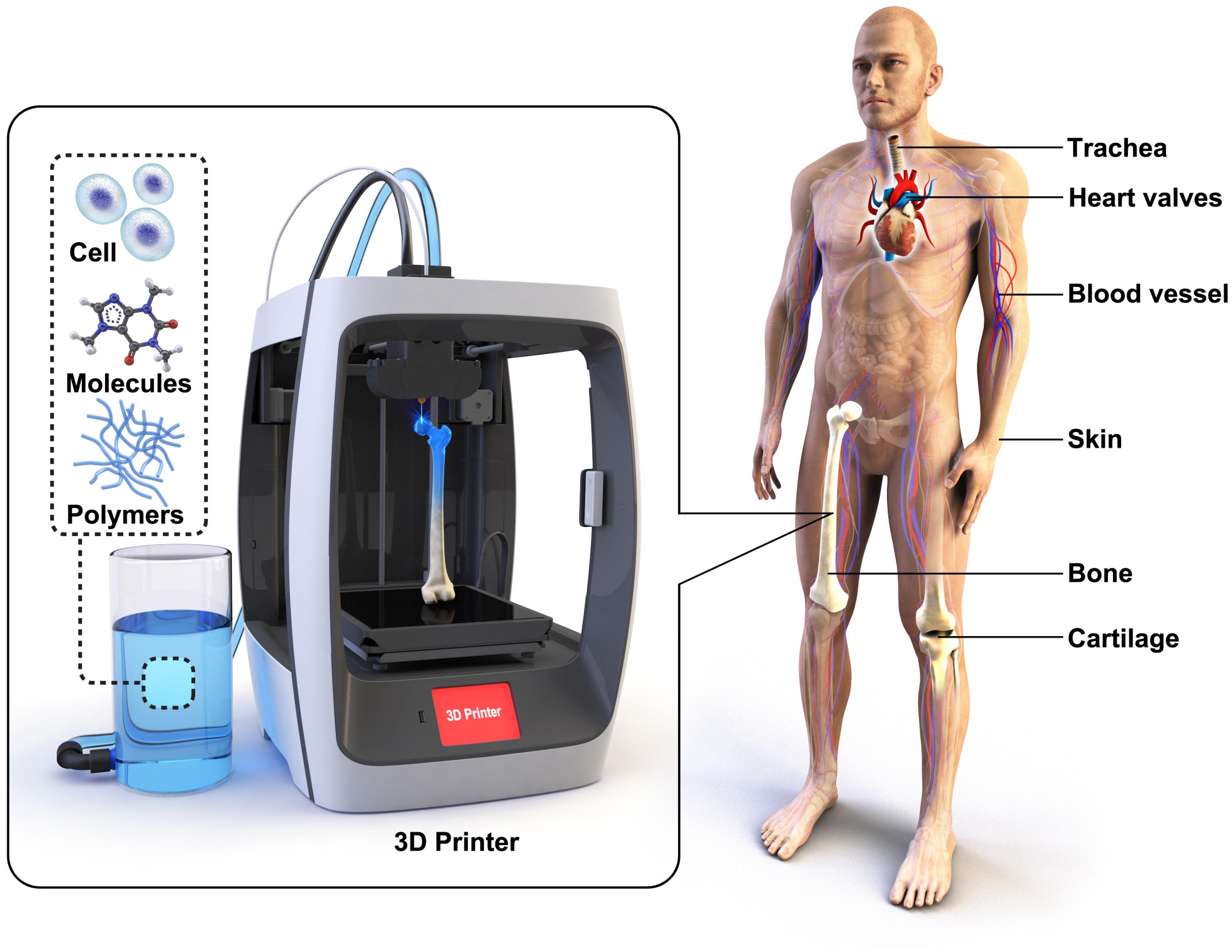
Figure 1. Various scaffolds for tissues and organs have been 3D printed in the regenerative medicine field. 3D scaffolds are typically made of biocompatible polymers. Cells and bioactive molecules are often incorporated with the scaffolds to enhance bioactive properties.
3D Printed Scaffolds for Tissue Engineering
There have been numerous types of 3D printing techniques used and developed by researchers in the field of tissue engineering. In this section, 3D printing techniques and 3D printed biomaterials are categorized into subsections by tissues and organs that they were designed to aid. They are also summarized in Table 2.
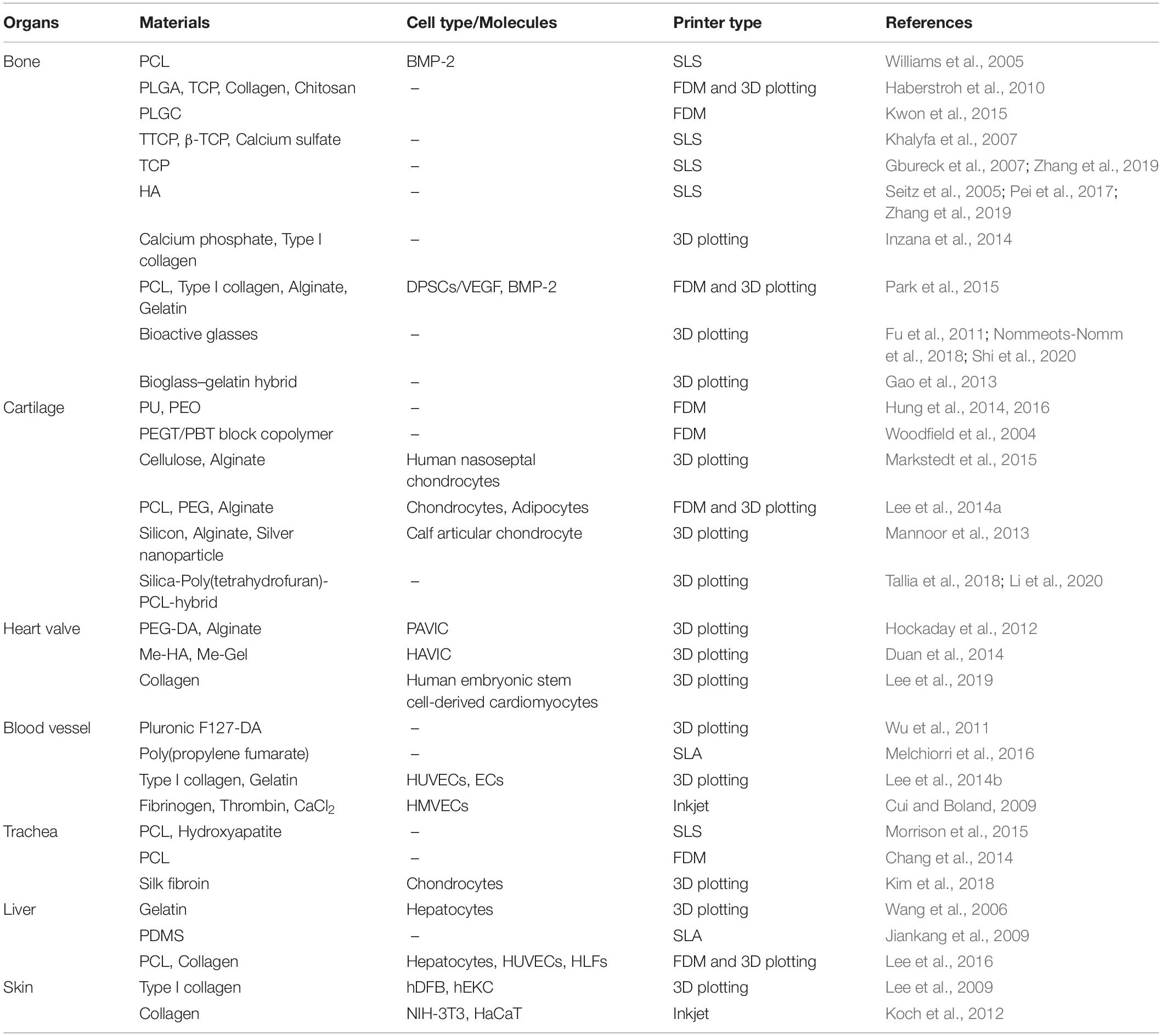
Table 2. Summary of materials, cell types, and molecules used for 3D printed scaffolds for tissue engineering.
Bone
The bone regeneration process involves migration and recruitment of osteoprogenitor cells to a defect region, which will then differentiate to osteoblasts to form bone minerals or hydroxyapatite (HA). There are various methods to enhance bioactive properties to bone tissue engineering scaffolds, such as incorporating growth factors and gene/drug deliveries. An ideal bone scaffold should be made with biocompatible materials that act as a temporary template to withstand mechanical forces in the defect site until the host tissue is fully recovered. Specifically, biodegradation rate should be similar to the duration of bone formation process, and inter-connected porous structure for vascularization is essential for the scaffold design (Bose et al., 2012; Jones, 2013).
Synthetic biodegradable polymers, such as poly(caprolactone) (PCL), poly(glycolic acid) (PGA), poly(lactic acid) (PLA), and their copolymers, have received high attention in the biomedical field since ester linkages can be degraded by hydrolysis and their by-products are non-toxic. These biodegradable polymers have also been 3D printed to produce scaffolds for tissue engineering (Figure 2A). Williams et al. (2005) fabricated PCL scaffold with similar mechanical properties to that of human trabecular bone using the SLS printing technique. Bone morphogenetic protein-7 (BMP-7) was seeded to enhance the bioactivity of the scaffold, and subcutaneous implantation has shown bone formation within 4 weeks. Additionally, the scaffold was fabricated to replicate a CT-scanned minipig condyle structure, which showed a possibility of producing patient-specific scaffolds (Figures 2B,C). Kwon et al. (2015) synthesized PCL containing PLGC [methoxy poly(ethylene glycol)-co-L-lactide-co-glycolide-co-ε-caprolactone] copolymer scaffold through the FDM process. Human dental pulp stem cell (hDPSC)-loaded PLGC scaffold instigated bone regeneration, and the degradation rate of the scaffold was comparable to the bone formation rate.
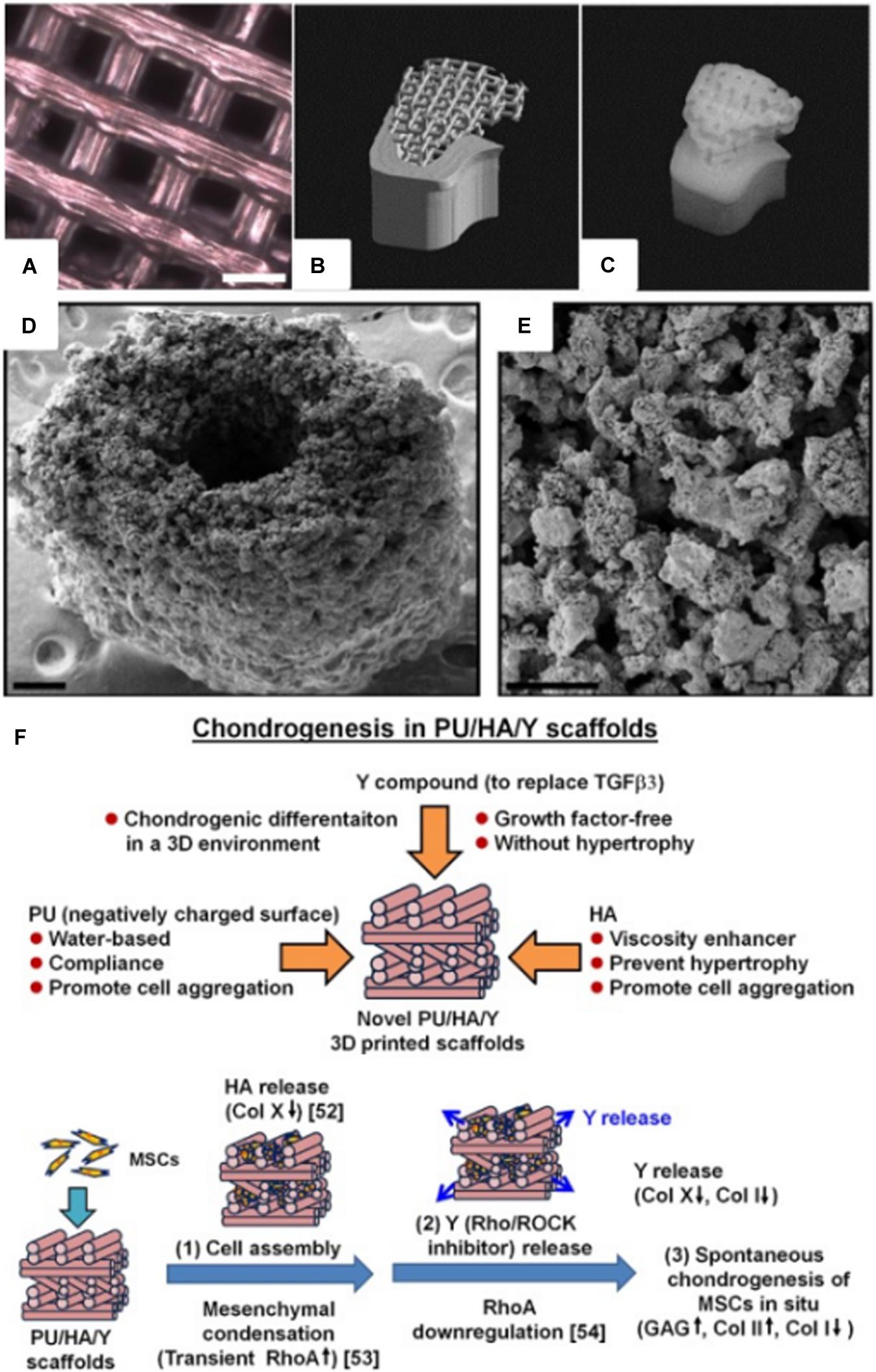
Figure 2. (A) Optical microscope image of a 3D printed poly(l-lactide-co-ε-caprolactone) scaffold for adipose tissue engineering (courtesy of YJ, scale bar = 2 mm). (B) STL image of a pig condyle scaffold. (C) Front view of the 3D printed PCL scaffold (Williams et al., 2005). (D) SEM image of a 3D printed murine-sized scaffold for femoral mid-diaphysis regeneration (scale bar = 250 μm). (E) Micro-porosity of the calcium phosphate-collage composite scaffold with pore sizes of 20–50 μm (scale bar = 100 μm) (Inzana et al., 2014). (F) 3D printed PU/HA-based scaffold design, and possible mechanism of spontaneous chondrogenesis in situ (Hung et al., 2016) (Reproduced with permission from Williams et al., 2005; Inzana et al., 2014; Hung et al., 2016).
Calcium phosphate-based ceramics have similar compositions to bone mineral; therefore, they have been widely used as bone substitutes. Since bioceramics are in powder form, the SLS method is often used to produce grid-like scaffold structures (Seitz et al., 2005; Gbureck et al., 2007; Khalyfa et al., 2007). 3D plotting is also practiced with a post-sintering process when binders and sacrificial polymers are mixed with bioceramics to produce printable inks (Pei et al., 2017; Zhang et al., 2019). Although bioactive glasses are not commercially successful as bioceramics, they are known to be more bioactive, and 45S5 composition (Bioglass®) was the first artificial material that formed a chemical bond to bone (Hench, 2006; Jones, 2013; Cai et al., 2018). Various compositions of bioactive glasses were also 3D printed to biomimic the porous structure of bone (Fu et al., 2011; Nommeots-Nomm et al., 2018; Shi et al., 2020). However, inorganic scaffolds (i.e., bioceramics and bioactive glasses) are too brittle for repairing defect sites that are exposed to constant loading. Flexibility and toughness of bioactive glasses can be enhanced by introducing flexible polymers to the silica network with covalent bonding, termed inorganic–organic hybrids. This is possible since glasses can be fabricated through a sol-gel process that prevents oxidization of polymers (Sanchez et al., 2005; Jones et al., 2006; Valliant and Jones, 2011; Chung et al., 2017). Gao et al. (2013) were able to 3D print a gelatin–bioactive glass hybrid to a grid-like structure. MC3T3-E1 osteoblast precursor cells were able to adhere and proliferate on the printed hybrid scaffold.
Bone is a nanocomposite composed of HA (50–70%) and organic matrix (20–40%), which is primarily composed of type I collagen (Clarke, 2008; Jones, 2013). Collagen is widely used as biomaterials for tissue engineering in skin (Powell et al., 2008), bone (Rodrigues et al., 2003), tendon (Young et al., 1998), and blood vessel (Zorlutuna et al., 2008) applications due to its tough mechanical properties and biocompatibility. Inzana et al. (2014) 3D printed collagen and calcium phosphate composite for bone regeneration. The scaffold was implanted in a murine femur with critical defect size, and osteoconductivity was confirmed (Figures 2D,E). 3D bioprinting technique is one of the most recent methods of printing biomaterials; it renders 3D tissue constructs with cells embedded in hydrogels (Mironov et al., 2009; Kang et al., 2013; Levato et al., 2014). Park et al. (2015) were able to demonstrate the multi-head bioprinting method. Vascular endothelial growth factor (VEGF) and BMP-2 were loaded to various blends of hydrogels with hDPSC. The hydrogels were 3D plotted to a PCL scaffold framework, and this fabrication method was shown to produce large volume scaffolds, which is one of the major limitations in the tissue engineering field.
Cartilage
The ECM of cartilage is composed of type II collagen and glycosaminoglycan (GAG), which allows to regulate expression of chondrocyte phenotype and instigate chondrogenesis (Suh and Matthew, 2000). In contrast to bone, cartilage has limited ability to naturally heal itself, since avascular structure inhibits nutrients and progenitor cells to migrate toward the defect region. Articular cartilage covers end of bones in synovial joints, which allows the bones to glide over each other; therefore, it should withstand load-bearing forces while providing low-friction surfaces. Osteoarthritis and high-impact injuries can cause articular cartilage defects, and it is one of the most challenging tissues to repair (Temenoff and Mikos, 2000; Huey et al., 2012).
Poly(ethylene glycol) terephthalate/poly(butylene terephthalate) (PEGT/PBT) block copolymer was 3D printed through the FDM technique to fabricate grid-like structured scaffold. The scaffold was seeded with bovine articular chondrocytes (bACs), which developed cartilage-like tissue in vivo while having mechanical properties similar to the native articular cartilage (Woodfield et al., 2004). Hung et al. (2014, 2016) were able to 3D print cartilage scaffolds with water as a printing ink solvent, which allowed incorporation of biomolecules, i.e., growth factors, with higher biocompatibility compared to inks that require organic solvents. Polyurethane (PU) particles, hyaluronic acid (HA), and TGFβ3 containing ink were 3D printed through a customized low-temperature FDM-type printer. Then, the scaffold was seeded with mesenchymal stem cells (MSCs) to improve cartilage regeneration in vivo (Figure 2F). Markstedt et al. (2015) developed a nanocellulose-alginate scaffold using the 3D bioprinting technique. The rheological properties of the composite bioink, which required low pressure to extrude at room temperature, allowed the production of precise 3D grid, disc, human ear, and sheep meniscus constructs. The cytotoxicity and live/dead cell-imaging assay confirmed that the scaffold was suitable for cartilage regeneration.
Other researchers have also reported 3D printing of ear-shaped structures for cartilage regeneration. Lee et al. (2014a) fabricated a human ear scaffold by printing both articular cartilage and fat tissue. Poly(ethylene glycol) (PEG) was used as a sacrificial layer since it is soluble in aqueous solutions, and PCL was printed as a main framework of the scaffold. Alginate hydrogel was used as a bioink to print chondrocyte and adipocyte. It was printed along with PCL to fabricate an ear scaffold with two distinct regions, which included a main ear part (chondrocyte) and an earlobe area (adipocyte). Additionally, co-printing of cell-seeded alginate scaffold confirmed that gene expression of both chondrocyte and adipocyte were remarkably enhanced compared to that of the control group. Mannoor et al. (2013) were able to integrate 3D biological tissue and electronic components. Alginate hydrogel with chondrocyte and silver nanoparticles was printed to structurally mimic human ears. Then, cochlea-shaped electrodes for hearing were inserted into the hydrogel construct, which was referred to as “cyborg ears.” Cell viability of the cyborg ear was 91.3 ± 3.9%, which is an adequate biocompatibility for the application.
Recently, an inorganic–organic hybrid of silica-poly(tetrahydrofuran)/PCL was 3D printed to fabricate scaffolds for articular cartilage regeneration (Tallia et al., 2018; Li et al., 2020). The silica network and organic component was forming co-networks via covalent bonding, which displayed elasticity, self-healing ability, and bioactivity. The scaffold with a grid-like structure mimicked the compressive behavior of cartilage, and in vitro chondrogenic differentiation was observed.
Heart Valve
Heart is one of the essential organs in human physiology, which consists of various muscles to pump blood in the circulatory system. Heart valves, two in atria and the other two in ventricle chambers, are important for the blood circulation since they prevent backward flow (Dasi et al., 2009). In 2000, the American Heart Association announced that 87,000 replacement surgeries occurred (Flanagan and Pandit, 2003). Specifically, aortic valve disease is one of the serious cardiovascular diseases that are usually treated by replacement of the valves. Many researchers have studied artificial heart valves using various polymeric materials, such as PGA, PLA, collagen, and fibrin. Similar to the scaffolds described in the previous sections, inherent structures and mechanical properties are also important for designing heart valve conduits. Therefore, a 3D printing technique has been applied to this research field for several years (Hockaday et al., 2012). For heart valve engineering, hydrogels are promising materials due to their physicochemical and mechanical stability while they are hydrated. Furthermore, hydrogels are permeable for nutrients and waste transportation. Duan et al. (2014) fabricated human aortic valvular interstitial cell (HAVIC)-encapsulated heart valve conduits with photo-crosslinkable methacrylated hyaluronic acid (Me-HA) and methacrylated gelatin (Me-Gel) hydrogels. The viscosity of the hydrogel conduits was optimized and tuned by applying different hydrogel concentrations. The 3D bioprinted hydrogel conduits confirmed cell viability and remodeling potential for initial collagen and glycosaminoglycan matrix formation (Figures 3A–G). Hockaday et al. (2012) printed heart valve scaffolds with photo-crosslinkable poly(ethylene glycol)-diacrylate (PEG-DA). The scaffold was fabricated with two types of PEG-DA with different molecular weights in order to meet the heterogeneous mechanical properties of aortic valves. The conduit had high elastic modulus and nearly 100% cell viability (Figures 3H–J).
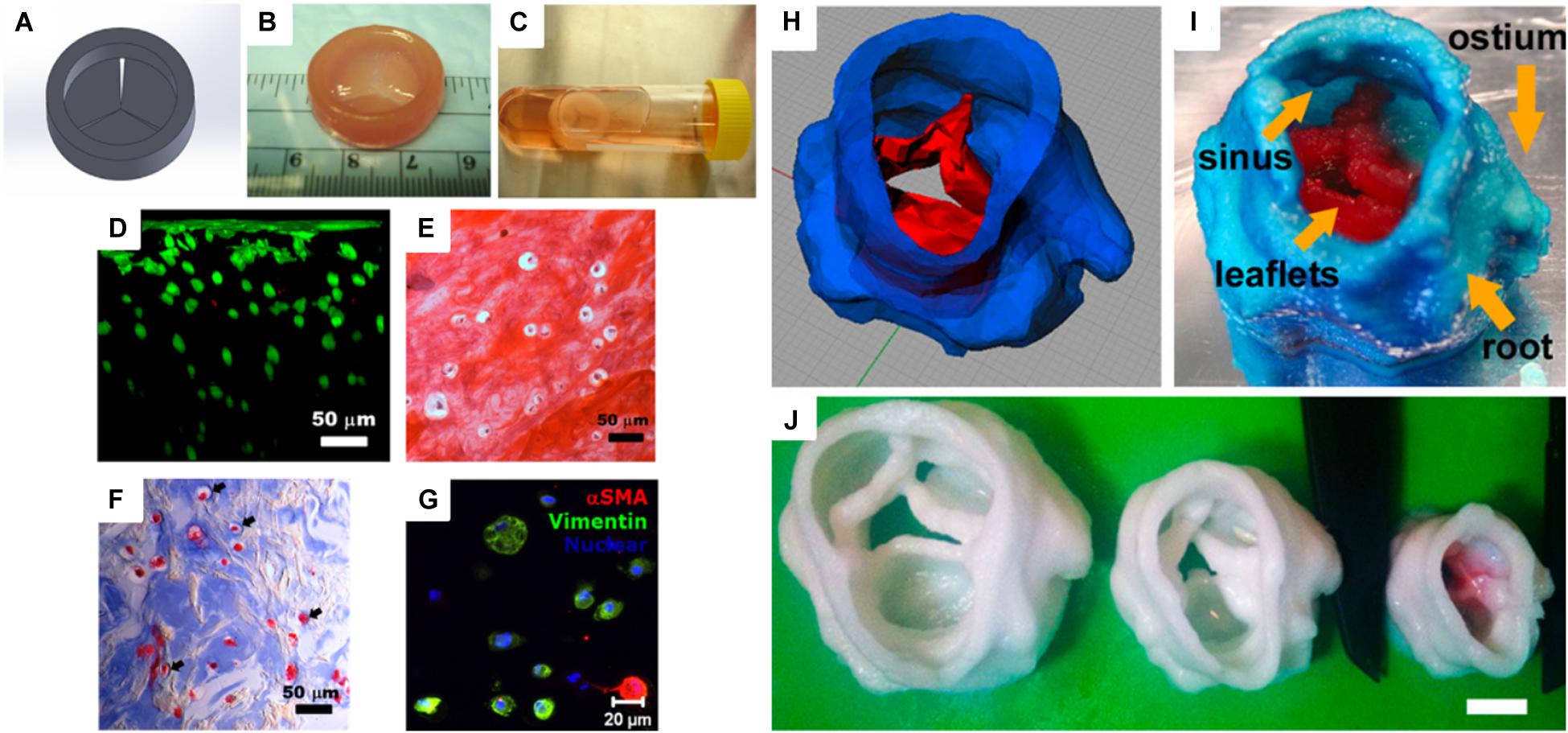
Figure 3. (A) Computer-aided design (CAD) model of a heart valve. (B) Bioprinted methacrylated hyaluronic acid/gelatin heart valve conduit. (C) The hydrogel hybrid conduit after 7 days of static culture, and (D) cross-sectional view of a live/dead cell viability assay. (E) Safranin-O staining image and (F) Masson’s Trichrome staining images showed that the heart valve conduit was composed of collagen type II and GAG. (G) Representative immunohistochemical staining image of αSMA, vimentin, and nuclei (Duan et al., 2014). (H) Porcine aortic valve model and (I) 3D printed scaffold with two types of PEG-DA inks [root: 700 molecular weight (MW) PEG-DA and leaflets: 700/8000 MW PEG-DA]. (J) Scaffolds were printed with 700 MW PEG-DA at different scales for fidelity analysis. The inner diameters (ID) were 22, 17, and 12 mm. Scale bar = 1 cm (Hockaday et al., 2012) (Reproduced with permission from Hockaday et al., 2012; Duan et al., 2014).
Blood Vessel/Trachea
In the United States, coronary artery bypass grafting surgeries are performed more than 400,000 per year. Critical drawbacks of the surgeries are graft damages during harvesting procedure, poor long-term patency, and donor morbidity. Therefore, there are high demands for the development of artificial blood vessels that can overcome the current shortcomings. An ideal artificial blood vessel should be biocompatible, anti-thrombogenic, and durable, and have comparable compliance with structural density to that of the native blood vessels (Mosadegh et al., 2015). Wu et al. (2011) fabricated a biomimetic 3D microvascular network based on hydrogel matrix. The blood vessel’s branching pattern was designed by omnidirectional printing of sacrificial ink in a photo-crosslinkable hydrogel matrix. The authors suggested that this technique can be applied to 3D cell culture; however, there were no cell tests performed. Similar work was performed by Lee et al. (2014b); functional vascular channels with perfused open lumen were fabricated through 3D bioprinting of collagen matrix with liquefying fugitive ink. Gelatin with endothelial cell (EC) was used as a fugitive ink, which protected from plasma protein and dextran molecule. Additionally, human umbilical vein endothelial cells (HUVECs) were cultured in the vascular channel, which was successfully aligned along the flow direction. The gene expression analysis confirmed that the 3D printed vascular channels had high potential for tissue engineering application.
Fibrin is a natural polymer formed by polymerization of fibrinogen and thrombin. It is present in human blood and involved in the wound healing process. Cui and Boland (2009) 3D printed human microvascular endothelial cell (HMVEC)-seeded bioink of thrombin and Ca2+ solution into a fibrinogen substrate. The scaffold was composed of fibrin channels with aligned HMVECs, and 21 days of cell culture confirmed tubular structure formation inside the channels. Poly(propylene fumarate)-based aorta graft was synthesized via digital light stereolithography technique (Melchiorri et al., 2016). The biodegradable polymer was 3D printed to an MRI/CT scanned structure, which showed a possibility to design patient-specific aorta grafts. Additionally, the scaffold was able to confirm bioactive properties in vivo with comparable mechanical strength to that of human aorta.
Tracheal structure restoration and scaffold fabrications are also in great demand. Forty-three percent of pediatric patients who went through tracheostomy experience respiratory arrest due to tube occlusion (Carr et al., 2001; Berry et al., 2010). However, if the pediatric patients are supported by a temporary scaffold for 24 to 36 months, the airway growth can naturally resolve the disease. Morrison et al. (2015) were able to produce a personalized and biodegradable tracheal splint through the SLS technique. PCL powders were mixed with hydroxyapatite, which was used as a flowing agent for the laser sintering process. PCL splints were successfully implanted to pediatric patients, and they were able to expand over time with airway growth (Figures 4A,B). Chang et al. (2014) 3D printed PCL scaffolds through an FDM-type printer for tracheal regeneration. The scaffold was coated with MSC-seeded fibrin to enhance bioactivity. In vivo study confirmed that the scaffolds were mechanically stable and able to reconstruct trachea within 8 weeks of implantation. Kim et al. (2018) produced a ring-like cartilaginous trachea scaffold through 3D bioprinting with digital light processing technique. Chondrocytes were encapsulated in methacrylated silk fibroin, which made cross-linking possible through UV light exposure. This cell-loaded hydrogel scaffold showed homogeneously distributed cells and cartilage tissue formation in vitro. The chemically modified silk fibroin ink was also printed to heart, lung, and vascular shapes, which confirmed that the bioink and printing method can be applied to various tissue engineering applications.
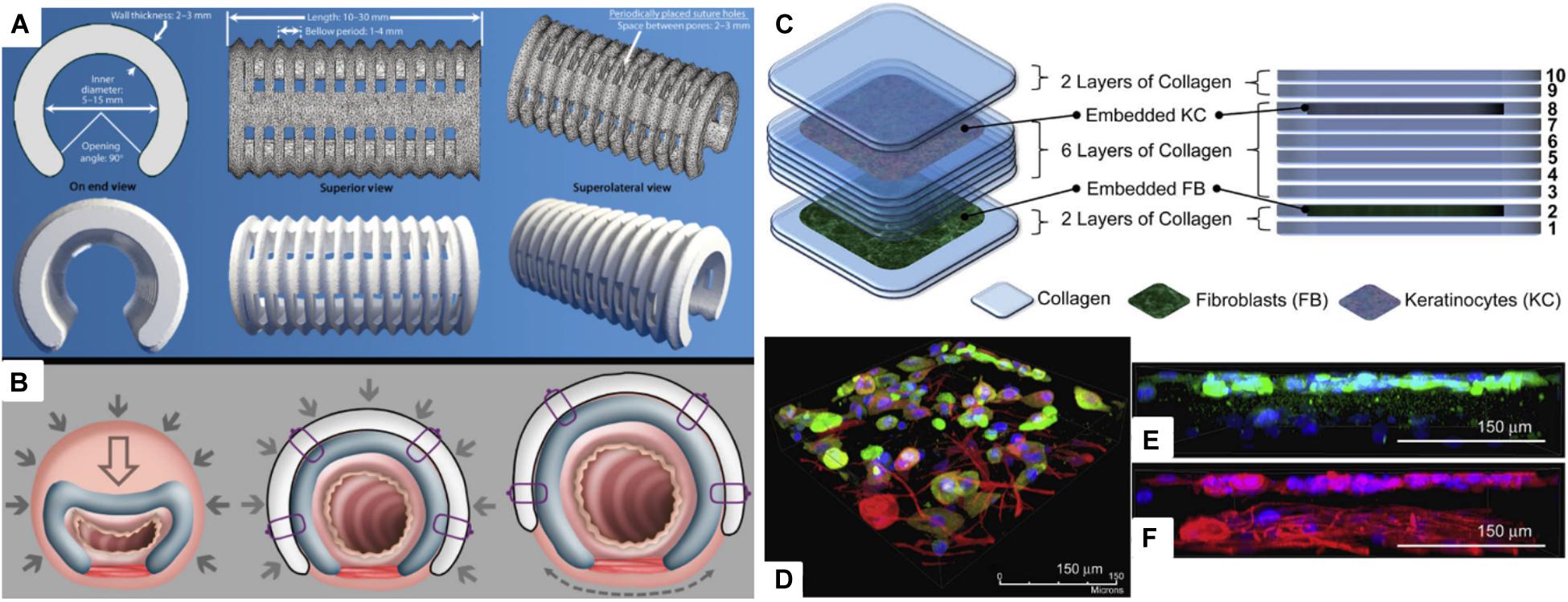
Figure 4. (A) Virtual rendering of tracheobronchial splint STL file in top, bottom, and side views. Inner diameter, length, thickness, and suture hole spacing were patient-specifically designed, and then it was placed over an airway through the 90° opening angle. (B) The mechanism of the tracheobronchial splint. Filled arrows signify intrathoracic pressure when breathing out, and empty arrows represent reducing vector values. Dashed arrow indicates the vector movement of a splint according to the airway growth (Morrison et al., 2015). (C) Representative scheme of a multi-layered collagen scaffold for tissue regeneration. Primary adult human dermal fibroblast-seeded collagen is printed in the 2nd layer, and primary adult human epidermal keratinocyte embedded collagen layer is deposited in the 8th layer. (D) Immunofluorescent image of the 3D printed multi-layered scaffold with fibroblast and keratinocyte on a tissue culture dish. (E) Keratinocyte layer with keratin, and (F) keratinocyte and fibroblast layer with β-tubulin (Lee et al., 2009) (Reproduced with permission from Lee et al., 2009; Morrison et al., 2015).
Liver
The liver is a fundamental organ that is responsible for multifunctional metabolic activities. Although liver transplant has been practiced for a long time, the procedure is costly, patient survival rate is poor, and there is a shortage of organ donors (Wang et al., 2007). Wang et al. (2006) made a 3D construct of gelatin hydrogel with hepatocyte as an ECM and 2.5% glutaraldehyde as a cross-linking agent. They were able to confirm that hepatocytes in gelatin construct can survive over 2 months in in vitro cell culture and retain their 3D structure for a month. A chitosan–gelatin hybrid scaffold was developed to biomimic the architecture of natural liver. A highly porous and well-organized structure was fabricated by a combination of 3D printing, micro-replication, and freeze-drying techniques. The novel scaffold was also composed of intrinsic fluidic channels and hepatic chambers. Firstly, a resin mold was fabricated by the SLA technique to cast polydimethylsiloxane (PDMS) for creating a micro-replication mold. Chitosan–gelatin solution was cast in the PDMS mold followed by freeze-drying to produce a porous structure. Biodegradability and hepatocyte growth were confirmed through 7-day cell culture. More importantly, albumin secretion and urea synthesis were evident, which are representative evaluation for hepatocyte functionality (Jiankang et al., 2009). Lee et al. (2016) 3D printed PCL to a grid-like structure as a primary framework to mechanically support collagen bioinks. HUVECs, human lung fibroblasts (HLFs), and hepatocytes were encapsulated in collagen inks, printed in between PCL struts for angiogenesis. The authors also evaluated albumin secretion and urea synthesis for confirming hepatocytes functionality and angiogenesis. Specifically, hepatocyte-, HLF-, and HUVEC-containing constructs showed highest albumin secretion and urea formation compared to that of hepatocytes only and hepatocyte- and HLF-containing groups.
Skin
Skin is the largest organ in our body that is responsible for various functions, such as preventing loss of body fluid, acting as a barrier against pathogenic bacterium and thermotaxis, and regulating body temperature (Bonvallet et al., 2015; Ojeh et al., 2015). Severe acute and chronic wounds (i.e., burns, diabetic ulcer, pressure sores, and lesion) effect loss of dermal tissues. Skin grafts have limitations in antigenicity and shortage of transplantable tissues; therefore, there are high demands for skin regeneration (Tchemtchoua et al., 2011; Wang et al., 2013). Lee et al. (2009) used a 3D bioprinter with four-channel dispensers to print stratified skin layers. PDMS substrate was first coated with sodium bicarbonate, a pH-altering cross-linking agent. Then, collagen was printed layer by layer to fabricate a multi-layered skin construct. Specifically, among the 10 layers of skin construct, the 2nd and 8th collagen layers were embedded with cells, fibroblast and keratinocyte, respectively. Several advantages of this fabrication method include the following: the scaffold can be made on irregular surfaces, as long as cross-linking agent coating is possible, and other types of hydrogels can substitute collagen, if they are cross-linkable. Additionally, this was the first study to 3D print both keratinocyte and fibroblast for skin regeneration (Figures 4C–F). Koch et al. (2010, 2012) also 3D printed keratinocyte- and fibroblast-embedded collagen for skin tissue engineering. Laser-assisted bioprinting (LaBP) with laser-induced forward transfer technique was used to produce 3D scaffolds. The LaBP method is advantageous over other bioprinting techniques since higher-resolution cell printing is possible with greater cell density. Also, various hydrogels can be printed regardless of their viscosity. The skin scaffold, which was printed in micro-scale, confirmed that each cell layer did not blend into each other. Ten days of culture confirmed the cell vitality of the cells embedded in the scaffold, and collagen layers did not intermix with each other.
Conclusion and Perspectives
Three-dimensional printing is one of the most promising technologies in tissue engineering and regenerative medicine to fabricate advanced 3D scaffolds. It allows to produce more defined and biomimetic scaffolds with bioactive factors to enhance their functionalities. Although there have been numerous studies in 3D printing for biomedical applications, there are still much room for improvement (Jakus et al., 2016; Zhu et al., 2016). Optimization of printable inks, standardization of printing methods, and higher reproducibility with mass production are major challenges. 4D printing, an integration of 3D printing with time, has emerged recently. This technology allows the printed materials to change their physical forms or functionalities when excited by an external stimulus, such as temperature, water, magnetism, and pH (Gao et al., 2016; Gladman et al., 2016). Since human body is a complex environment with various stimuli, 4D printing technology is receiving a lot of attention for medical implant surgeries. 3D bioprinting of stem cells has shown unprecedented possibilities for producing tissue constructs from bone to skin. As bioprinting technology advances, printing induced pluripotent stem (iPS) cells could take current bioactive scaffolds a step closer to regenerate patient-specific tissues and organs. iPS cells are known to have advantages over embryonic stem cells since they can be derived from patients for autologous cell treatment. However, the reprogramming process is not fully understood so far (Yamanaka, 2009). 3D bioprinting is an encouraging technology for future regenerative medicine. It allows to deliver, or mount, cells and physicochemical factors that are essential for tissue regeneration. Furthermore, patient-specific therapies are one of the essential technologies for hospital factory, where damaged organ rendering is produced by medical imaging, and defect regenerating construct is printed with patient’s cells, plasma, and tissues in the operating theater. The authors are confident that the progress in 3D printing technology will foster and enhance personalized regenerative medicine.
Author Contributions
JC and HI drafted the initial manuscript with guidance by YJ. SK, YJ, and JP made contributions and modifications according to their field of expertise. All authors reviewed and approved the manuscript.
Funding
This research was supported by the KIST Institutional Program (2V08550). This work was partially supported by the Nano-Material Technology Development Program (NRF-2018M3A7B4071106) through the National Research Foundation of Korea and a National Research Foundation of Korea grant (NRF-2020R1C1C1012881) funded by the Ministry of Science and ICT (MSIT).
Conflict of Interest
The authors declare that the research was conducted in the absence of any commercial or financial relationships that could be construed as a potential conflict of interest.
References
Azari, A., and Nikzad, S. (2009). The evolution of rapid prototyping in dentistry: a review. Rapid Prototyp. J. 15, 216–225. doi: 10.1108/13552540910961946
Berry, J. G., Graham, R. J., Roberson, D. W., Rhein, L., Graham, D. A., Zhou, J., et al. (2010). Patient characteristics associated with in-hospital mortality in children following tracheotomy. Arch. Dis. Child. 95, 703–710. doi: 10.1136/adc.2009.180836
Boland, T., Xu, T., Damon, B., and Cui, X. (2006). Application of inkjet printing to tissue engineering. Biotechnol. J. 1, 910–917. doi: 10.1002/biot.200600081
Bonvallet, P. P., Schultz, M. J., Mitchell, E. H., Bain, J. L., Culpepper, B. K., Thomas, S. J., et al. (2015). Microporous dermal-mimetic electrospun scaffolds pre-seeded with fibroblasts promote tissue regeneration in full-thickness skin wounds. PLoS One 10:e0122359. doi: 10.1371/journal.pone.0122359
Bose, S., Roy, M., and Bandyopadhyay, A. (2012). Recent advances in bone tissue engineering scaffolds. Trends Biotechnol. 30, 546–554. doi: 10.1016/j.tibtech.2012.07.005
Bose, S., Vahabzadeh, S., and Bandyopadhyay, A. (2013). Bone tissue engineering using 3D printing. Mater. Today 16, 496–504. doi: 10.1016/j.mattod.2013.11.017
Cai, L., Zhang, J., Qian, J., Li, Q., Li, H., Yan, Y. G., et al. (2018). The effects of surface bioactivity and sustained-release of genistein from a mesoporous magnesium-calcium-silicate/PK composite stimulating cell responses in vitro, and promoting osteogenesis and enhancing osseointegration in vivo. Biomater. Sci. 6, 842–853. doi: 10.1039/c7bm01017f
Carr, M. M., Poje, C. P., Kingston, L., Kielma, D., and Heard, C. (2001). Complications in pediatric tracheostomies. Laryngoscope 111(11 Pt 1), 1925–1928. doi: 10.1097/00005537-200111000-00010
Chang, J. W., Park, S. A., Park, J. K., Choi, J. W., Kim, Y. S., Shin, Y. S., et al. (2014). Tissue-engineered tracheal reconstruction using three-dimensionally printed artificial tracheal graft: preliminary report. Artif. Organs 38, E95–E105. doi: 10.1111/aor.12310
Childers, E. P., Wang, M. O., Becker, M. L., Fisher, J. P., and Dean, D. (2015). 3D printing of resorbable poly(propylene fumarate) tissue engineering scaffolds. MRS Bull. 40, 119–126. doi: 10.1557/mrs.2015.2
Choi, J. W., and Kim, N. (2015). Clinical application of three-dimensional printing technology in craniofacial plastic surgery. Arch. Plast. Surg. 42, 267–277. doi: 10.5999/aps.2015.42.3.267
Chung, J. J., Sum, B. S. T., Li, S., Stevens, M. M., Georgiou, T. K., and Jones, J. R. (2017). Effect of comonomers on physical properties and cell attachment to silica-methacrylate/acrylate hybrids for bone substitution. Macromol. Rapid Commun. 28:1700168. doi: 10.1002/marc.201700168
Clarke, B. (2008). Normal bone anatomy and physiology. Clin. J. Am. Soc. Nephrol. 3(Suppl. 3), S131–S139. doi: 10.2215/CJN.04151206
Connell, L. S., Romer, F., Suarez, M., Valliant, E. M., Zhang, Z. Y., Lee, P. D., et al. (2014). Chemical characterisation and fabrication of chitosan-silica hybrid scaffolds with 3-glycidoxypropyl trimethoxysilane. J. Mater. Chem. B 2, 668–680. doi: 10.1039/c3tb21507e
Cornejo, I., McNulty, T. F., Lee, S., Bianchi, E., Danforth, S. C., and Safari, A. (2000). “Development of bioceramic tissue scaffolds via fused deposition of ceramics,” in Bioceramics: Materials and Applications III, ed. L. George (Westerville, OH: American Ceramic Society), 183–195.
Cui, X. F., and Boland, T. (2009). Human microvasculature fabrication using thermal inkjet printing technology. Biomaterials 30, 6221–6227. doi: 10.1016/j.biomaterials.2009.07.056
Dasi, L. P., Simon, H. A., Sucosky, P., and Yoganathan, A. P. (2009). Fluid mechanics of artificial heart valves. Clin. Exp. Pharmacol. Physiol. 36, 225–237. doi: 10.1111/j.1440-1681.2008.05099.x
Derby, B. (2012). Printing and prototyping of tissues and scaffolds. Science 338, 921–926. doi: 10.1126/science.1226340
Do, A. V., Khorsand, B., Geary, S. M., and Salem, A. K. (2015). 3D printing of scaffolds for tissue regeneration applications. Adv. Healthc. Mater. 4, 1742–1762. doi: 10.1002/adhm.201500168
Duan, B., Kapetanovic, E., Hockaday, L. A., and Butcher, J. T. (2014). Three-dimensional printed trileaflet valve conduits using biological hydrogels and human valve interstitial cells. Acta Biomater. 10, 1836–1846. doi: 10.1016/j.actbio.2013.12.005
Duoss, E. B., Weisgraber, T. H., Hearon, K., Zhu, C., Small, W., Metz, T. R., et al. (2014). Three-dimensional printing of elastomeric, cellular architectures with negative stiffness. Adv. Funct. Mater. 24, 4905–4913. doi: 10.1002/adfm.201400451
Flanagan, T. C., and Pandit, A. (2003). Living artificial heart valve alternatives: a review. Eur. Cell Mater. 6, 28–45; discussion 45.
Fu, Q. A., Saiz, E., and Tomsia, A. P. (2011). Bioinspired strong and highly porous glass scaffolds. Adv. Funct. Mater. 21, 1058–1063. doi: 10.1002/adfm.201002030
Gao, B., Yang, Q., Zhao, X., Jin, G., Ma, Y., and Xu, F. (2016). 4D bioprinting for biomedical applications. Trends Biotechnol. 34, 746–756. doi: 10.1016/j.tibtech.2016.03.004
Gao, C., Rahaman, M. N., Gao, Q., Teramoto, A., and Abe, K. (2013). Robotic deposition and in vitro characterization of 3D gelatin-bioactive glass hybrid scaffolds for biomedical applications. J. Biomed. Mater. Res. A 101, 2027–2037. doi: 10.1002/jbm.a.34496
Gbureck, U., Hozel, T., Klammert, U., Wurzler, K., Muller, F. A., and Barralet, J. E. (2007). Resorbable dicalcium phosphate bone substitutes prepared by 3D powder printing. Adv. Funct. Mater. 17, 3940–3945. doi: 10.1002/adfm.200700019
Gladman, A. S., Matsumoto, E. A., Nuzzo, R. G., Mahadevan, L., and Lews, J. A. (2016). Biomimetic 4D printing. Nat. Mater. 15, 413–418. doi: 10.1038/Nmat4544
Gross, B. C., Erkal, J. L., Lockwood, S. Y., Chen, C., and Spence, D. M. (2014). Evaluation of 3D printing and its potential impact on biotechnology and the chemical sciences. Anal. Chem. 86, 3240–3253. doi: 10.1021/ac403397r
Haberstroh, K., Ritter, K., Kuschnierz, J., Bormann, K. H., Kaps, C., Carvalho, C., et al. (2010). Bone repair by cell-seeded 3D-bioplotted composite scaffolds made of collagen treated tricalciumphosphate or tricalciumphosphate-chitosan-collagen hydrogel or PLGA in ovine critical-sized calvarial defects. J. Biomed. Mater. Res. B Appl. Biomater. 93, 520–530. doi: 10.1002/jbm.b.31611
He, Y., Xue, G. H., and Fu, J. Z. (2014). Fabrication of low cost soft tissue prostheses with the desktop 3D printer. Sci. Rep. 4:6973. doi: 10.1038/srep06973
Hench, L. L. (2006). The story of bioglass (R). J. Mater. Sci. Mater. Med. 17, 967–978. doi: 10.1007/s10856-006-0432-z
Hockaday, L. A., Kang, K. H., Colangelo, N. W., Cheung, P. Y. C., Duan, B., Malone, E., et al. (2012). Rapid 3D printing of anatomically accurate and mechanically heterogeneous aortic valve hydrogel scaffolds. Biofabrication 4:035005. doi: 10.1088/1758-5082/4/3/035005
Hollister, S. J. (2005). Porous scaffold design for tissue engineering. Nat. Mater. 4, 518–524. doi: 10.1038/nmat1421
Huey, D. J., Hu, J. C., and Athanasiou, K. A. (2012). Unlike bone, cartilage regeneration remains elusive. Science 338, 917–921. doi: 10.1126/science.1222454
Hung, K. C., Tseng, C. S., Dai, L. G., and Hsu, S. H. (2016). Water-based polyurethane 3D printed scaffolds with controlled release function for customized cartilage tissue engineering. Biomaterials 83, 156–168. doi: 10.1016/j.biomaterials.2016.01.019
Hung, K. C., Tseng, C. S., and Hsu, S. H. (2014). Synthesis and 3D printing of biodegradable polyurethane elastomer by a water-based process for cartilage tissue engineering applications. Adv. Healthc. Mater. 3, 1578–1587. doi: 10.1002/adhm.201400018
Hutmacher, D. W., Schantz, T., Zein, I., Ng, K. W., Teoh, S. H., and Tan, K. C. (2001). Mechanical properties and cell cultural response of polycaprolactone scaffolds designed and fabricated via fused deposition modeling. J. Biomed. Mater. Res. 55, 203–216. doi: 10.1002/1097-4636(200105)55:2<203::aid-jbm1007>3.0.co;2-7
Inzana, J. A., Olvera, D., Fuller, S. M., Kelly, J. P., Graeve, O. A., Schwarz, E. M., et al. (2014). 3D printing of composite calcium phosphate and collagen scaffolds for bone regeneration. Biomaterials 35, 4026–4034. doi: 10.1016/j.biomaterials.2014.01.064
Jakus, A. E., Rutz, A. L., and Shah, R. N. (2016). Advancing the field of 3D biomaterial printing. Biomed. Mater. 11:014102. doi: 10.1088/1748-6041/11/1/014102
Jakus, A. E., Secor, E. B., Rutz, A. L., Jordan, S. W., Hersam, M. C., and Shah, R. N. (2015). Three-dimensional printing of high-content graphene scaffolds for electronic and biomedical applications. ACS Nano 9, 4636–4648. doi: 10.1021/acsnano.5b01179
Jiankang, H., Dichen, L., Yaxiong, L., Bo, Y., Hanxiang, Z., Qin, L., et al. (2009). Preparation of chitosan-gelatin hybrid scaffolds with well-organized microstructures for hepatic tissue engineering. Acta Biomater. 5, 453–461. doi: 10.1016/j.actbio.2008.07.002
Jones, J. R. (2013). Review of bioactive glass: from Hench to hybrids. Acta Biomater. 9, 4457–4486. doi: 10.1016/j.actbio.2012.08.023
Jones, J. R., Ehrenfried, L. M., and Hench, L. L. (2006). Optimising bioactive glass scaffolds for bone tissue engineering. Biomaterials 27, 964–973. doi: 10.1016/j.biomaterials.2005.07.017
Kang, K. H., Hockaday, L. A., and Butcher, J. T. (2013). Quantitative optimization of solid freeform deposition of aqueous hydrogels. Biofabrication 5:035001. doi: 10.1088/1758-5082/5/3/035001
Khademhosseini, A., Langer, R., Borenstein, J., and Vacanti, J. P. (2006). Microscale technologies for tissue engineering and biology. Proc. Natl. Acad. Sci. U.S.A. 103, 2480–2487. doi: 10.1073/pnas.0507681102
Khalyfa, A., Vogt, S., Weisser, J., Grimm, G., Rechtenbach, A., Meyer, W., et al. (2007). Development of a new calcium phosphate powder-binder system for the 3D printing of patient specific implants. J. Mater. Sci. Mater. Med. 18, 909–916. doi: 10.1007/s10856-006-0073-2
Kim, J. E., Kim, S. H., and Jung, Y. (2016). Current status of three-dimensional printing inks for soft tissue regeneration. Tissue Eng. Regen. Med. 13, 636–646. doi: 10.1007/s13770-016-0125-8
Kim, S. H., Yeon, Y. K., Lee, J. M., Chao, J. R., Lee, Y. J., Seo, Y. B., et al. (2018). Precisely printable and biocompatible silk fibroin bioink for digital light processing 3D printing. Nat. Commun. 9:1620. doi: 10.1038/s41467-018-03759-y
Koch, L., Deiwick, A., Schlie, S., Michael, S., Gruene, M., Coger, V., et al. (2012). Skin tissue generation by laser cell printing. Biotechnol. Bioeng. 109, 1855–1863. doi: 10.1002/bit.24455
Koch, L., Kuhn, S., Sorg, H., Gruene, M., Schlie, S., Gaebel, R., et al. (2010). Laser printing of skin cells and human stem cells. Tissue Eng. Part C Methods 16, 847–854. doi: 10.1089/ten.TEC.2009.0397
Kwon, D. Y., Kwon, J. S., Park, S. H., Park, J. H., Jang, S. H., Yin, X. Y., et al. (2015). A computer-designed scaffold for bone regeneration within cranial defect using human dental pulp stem cells. Sci. Rep. 5:12721.
Lee, A., Hudson, A. R., Shiwarski, D. J., Tashman, J. W., Hinton, T. J., and Yerneni, S. (2019). 3D bioprinting of collagen to rebuild components of the human heart. Science 365, 482–487. doi: 10.1126/science.aav9051
Lee, J. S., Hong, J. M., Jung, J. W., Shim, J. H., Oh, J. H., and Cho, D. W. (2014a). 3D printing of composite tissue with complex shape applied to ear regeneration. Biofabrication 6:024103. doi: 10.1088/1758-5082/6/2/024103
Lee, J. W., Choi, Y. J., Yong, W. J., Pati, F., Shim, J. H., Kang, K. S., et al. (2016). Development of a 3D cell printed construct considering angiogenesis for liver tissue engineering. Biofabrication 8:015007. doi: 10.1088/1758-5090/8/1/015007
Lee, V. K., Kim, D. Y., Ngo, H., Lee, Y., Seo, L., Yoo, S. S., et al. (2014b). Creating perfused functional vascular channels using 3D bio-printing technology. Biomaterials 35, 8092–8102. doi: 10.1016/j.biomaterials.2014.05.083
Lee, W., Debasitis, J. C., Lee, V. K., Lee, J. H., Fischer, K., Edminster, K., et al. (2009). Multi-layered culture of human skin fibroblasts and keratinocytes through three-dimensional freeform fabrication. Biomaterials 30, 1587–1595. doi: 10.1016/j.biomaterials.2008.12.009
Leong, K. F., Cheah, C. M., and Chua, C. K. (2003). Solid freeform fabrication of three-dimensional scaffolds for engineering replacement tissues and organs. Biomaterials 24, 2363–2378. doi: 10.1016/s0142-9612(03)00030-9
Levato, R., Visser, J., Planell, J. A., Engel, E., Malda, J., and Mateos-Timoneda, M. A. (2014). Biofabrication of tissue constructs by 3D bioprinting of cell-laden microcarriers. Biofabrication 6:035020. doi: 10.1088/1758-5082/6/3/035020
Li, S. W., Tallia, F., Mohammed, A. A., Stevens, M. M., and Jones, J. R. (2020). Scaffold channel size influences stem cell differentiation pathway in 3-D printed silica hybrid scaffolds for cartilage regeneration. Biomater. Sci. 8, 4458–4466. doi: 10.1039/c9bm01829h
Luo, Y. X., Lode, A., Sonntag, F., Nies, B., and Gelinsky, M. (2013). Well-ordered biphasic calcium phosphate-alginate scaffolds fabricated by multi-channel 3D plotting under mild conditions. J. Mater. Chem. B 1, 4088–4098. doi: 10.1039/c3tb20511h
Mahony, O., Tsigkou, O., Ionescu, C., Minelli, C., Ling, L., Hanly, R., et al. (2010). Silica-gelatin hybrids with tailorable degradation and mechanical properties for tissue regeneration. Adv. Funct. Mater. 20, 3835–3845. doi: 10.1002/adfm.201000838
Mankovich, N. J., Samson, D., Pratt, W., Lew, D., and Beumer, J. III (1994). Surgical planning using three-dimensional imaging and computer modeling. Otolaryngol. Clin. North Am. 27, 875–889. doi: 10.1016/s0030-6665(20)30614-9
Mannoor, M. S., Jiang, Z., James, T., Kong, Y. L., Malatesta, K. A., Soboyejo, W. O., et al. (2013). 3D printed bionic ears. Nano Lett. 13, 2634–2639. doi: 10.1021/nl4007744
Markstedt, K., Mantas, A., Tournier, I., Martinez Avila, H., Hagg, D., and Gatenholm, P. (2015). 3D bioprinting human chondrocytes with nanocellulose-alginate bioink for cartilage tissue engineering applications. Biomacromolecules 16, 1489–1496. doi: 10.1021/acs.biomac.5b00188
Melchels, F. P., Feijen, J., and Grijpma, D. W. (2010). A review on stereolithography and its applications in biomedical engineering. Biomaterials 31, 6121–6130. doi: 10.1016/j.biomaterials.2010.04.050
Melchiorri, A. J., Hibino, N., Best, C. A., Yi, T., Lee, Y. U., Kraynak, C. A., et al. (2016). 3D-printed biodegradable polymeric vascular grafts. Adv. Healthc. Mater. 5, 319–325. doi: 10.1002/adhm.201500725
Mironov, V., Trusk, T., Kasyanov, V., Little, S., Swaja, R., and Markwald, R. (2009). Biofabrication: a 21st century manufacturing paradigm. Biofabrication 1:022001. doi: 10.1088/1758-5082/1/2/022001
Morrison, R. J., Hollister, S. J., Niedner, M. F., Mahani, M. G., Park, A. H., Mehta, D. K., et al. (2015). Mitigation of tracheobronchomalacia with 3D-printed personalized medical devices in pediatric patients. Sci. Transl. Med. 7:285ra264. doi: 10.1126/scitranslmed.3010825
Mosadegh, B., Xiong, G., Dunham, S., and Min, J. K. (2015). Current progress in 3D printing for cardiovascular tissue engineering. Biomed. Mater. 10:034002. doi: 10.1088/1748-6041/10/3/034002
Murphy, S. V., and Atala, A. (2014). 3D bioprinting of tissues and organs. Nat. Biotechnol. 32, 773–785. doi: 10.1038/nbt.2958
Murr, L., (ed.). (2015). “Additive manufacturing additive manufacturing: changing the rules of manufacturing,” in Handbook of Materials Structures, Properties, Processing and Performance, (Cham: Springer International Publishing), 691–699. doi: 10.1007/978-3-319-01815-7_42
Nommeots-Nomm, A., Lee, P. D., and Jones, J. R. (2018). Direct ink writing of highly bioactive glasses. J. Eur. Ceram. Soc. 38, 837–844. doi: 10.1016/j.jeurceramsoc.2017.08.006
Ojeh, N., Pastar, I., Tomic-Canic, M., and Stojadinovic, O. (2015). Stem cells in skin regeneration, wound healing, and their clinical applications. Int. J. Mol. Sci. 16, 25476–25501. doi: 10.3390/ijms161025476
Park, J., Tari, M. J., and Hahn, H. T. (2000). Characterization of the laminated object manufacturing (LOM) process. Rapid Prototyp. J. 6, 36–50. doi: 10.1108/13552540010309868
Park, J. Y., Shim, J. H., Choi, S. A., Jang, J., Kim, M., Lee, S. H., et al. (2015). 3D printing technology to control BMP-2 and VEGF delivery spatially and temporally to promote large-volume bone regeneration. J. Mater. Chem. B 3, 5415–5425. doi: 10.1039/c5tb00637f
Pei, X., Ma, L., Zhang, B. Q., Sun, J. X., Sun, Y., Fan, Y. J., et al. (2017). Creating hierarchical porosity hydroxyapatite scaffolds with osteoinduction by three-dimensional printing and microwave sintering. Biofabrication 9:045008. doi: 10.1088/1758-5090/aa90ed
Poologasundarampillai, G., Yu, B. B., Jones, J. R., and Kasuga, T. (2011). Electrospun silica/PLLA hybrid materials for skeletal regeneration. Soft Matter 7, 10241–10251. doi: 10.1039/c1sm06171b
Powell, H. M., Supp, D. M., and Boyce, S. T. (2008). Influence of electrospun collagen on wound contraction of engineered skin substitutes. Biomaterials 29, 834–843. doi: 10.1016/j.biomaterials.2007.10.036
Ragaert, K., Cardon, L., Dekeyser, A., and Degrieck, J. (2010). Machine design and processing considerations for the 3D plotting of thermoplastic scaffolds. Biofabrication 2:014107. doi: 10.1088/1758-5082/2/1/014107
Rengier, F., Mehndiratta, A., von Tengg-Kobligk, H., Zechmann, C. M., Unterhinninghofen, R., Kauczor, H. U., et al. (2010). 3D printing based on imaging data: review of medical applications. Int. J. Comput. Assist. Radiol. Surg. 5, 335–341. doi: 10.1007/s11548-010-0476-x
Rimell, J. T., and Marquis, P. M. (2000). Selective laser sintering of ultra high molecular weight polyethylene for clinical applications. J. Biomed. Mater. Res. 53, 414–420. doi: 10.1002/1097-4636(2000)53:4<414::aid-jbm16>3.0.co;2-m
Rodrigues, C. V., Serricella, P., Linhares, A. B., Guerdes, R. M., Borojevic, R., Rossi, M. A., et al. (2003). Characterization of a bovine collagen-hydroxyapatite composite scaffold for bone tissue engineering. Biomaterials 24, 4987–4997. doi: 10.1016/s0142-9612(03)00410-1
Sachlos, E., and Czernuszka, J. T. (2003). Making tissue engineering scaffolds work. Review: the application of solid freeform fabrication technology to the production of tissue engineering scaffolds. Eur. Cell Mater. 5, 29–39; discussion 39–40.
Sanchez, C., Julian, B., Belleville, P., and Popall, M. (2005). Applications of hybrid organic-inorganic nanocomposites. J. Mater. Chem. 15, 3559–3592.
Schubert, C., van Langeveld, M. C., and Donoso, L. A. (2014). Innovations in 3D printing: a 3D overview from optics to organs. Br. J. Ophthalmol. 98, 159–161. doi: 10.1136/bjophthalmol-2013-304446
Seitz, H., Rieder, W., Irsen, S., Leukers, B., and Tille, C. (2005). Three-dimensional printing of porous ceramic scaffolds for bone tissue engineering. J. Biomed. Mater. Res. B Appl. Biomater. 74, 782–788. doi: 10.1002/jbm.b.30291
Shi, X., Nommeots-Nomm, A., Todd, N. M., Devlin-Mullin, A., Geng, H., Lee, P. D., et al. (2020). Bioactive glass scaffold architectures regulate patterning of bone regeneration in vivo. Appl. Mater. Today 20:100770. doi: 10.1016/j.apmt.2020.100770
Slaughter, B. V., Khurshid, S. S., Fisher, O. Z., Khademhosseini, A., and Peppas, N. A. (2009). Hydrogels in regenerative medicine. Adv. Mater. 21, 3307–3329. doi: 10.1002/adma.200802106
Suh, J. K., and Matthew, H. W. (2000). Application of chitosan-based polysaccharide biomaterials in cartilage tissue engineering: a review. Biomaterials 21, 2589–2598. doi: 10.1016/s0142-9612(00)00126-5
Tallia, F., Russo, L., Li, S. W., Orrin, A. L. H., Shi, X. M., Chen, S., et al. (2018). Bouncing and 3D printable hybrids with self-healing properties. Mater. Horiz. 5, 849–860. doi: 10.1039/c8mh00027a
Tchemtchoua, V. T., Atanasova, G., Aqil, A., Filee, P., Garbacki, N., Vanhooteghem, O., et al. (2011). Development of a chitosan nanofibrillar scaffold for skin repair and regeneration. Biomacromolecules 12, 3194–3204. doi: 10.1021/bm200680q
Temenoff, J. S., and Mikos, A. G. (2000). Review: tissue engineering for regeneration of articular cartilage. Biomaterials 21, 431–440. doi: 10.1016/s0142-9612(99)00213-6
Torres-Rendon, J. G., Femmer, T., De Laporte, L., Tigges, T., Rahimi, K., Gremse, F., et al. (2015). Bioactive gyroid scaffolds formed by sacrificial templating of nanocellulose and nanochitin hydrogels as instructive platforms for biomimetic tissue engineering. Adv. Mater. 27, 2989–2995. doi: 10.1002/adma.201405873
Tsang, V. L., and Bhatia, S. N. (2004). Three-dimensional tissue fabrication. Adv. Drug Deliv. Rev. 56, 1635–1647. doi: 10.1016/j.addr.2004.05.001
Vacanti, J. P., Morse, M. A., Saltzman, W. M., Domb, A. J., Perezatayde, A., and Langer, R. (1988). Selective cell transplantation using bioabsorbable artificial polymers as matrices. J. Pediatr. Surg. 23, 3–9. doi: 10.1016/s0022-3468(88)80529-3
Valliant, E. M., and Jones, J. R. (2011). Softening bioactive glass for bone regeneration: sol-gel hybrid materials. Soft Matter 7, 5083–5095. doi: 10.1039/c0sm01348j
Wang, H. M., Chou, Y. T., Wen, Z. H., Wang, C. Z., Chen, C. H., and Ho, M. L. (2013). Novel biodegradable porous scaffold applied to skin regeneration. PLoS One 8:e56330. doi: 10.1371/journal.pone.0056330
Wang, X., Yan, Y., Pan, Y., Xiong, Z., Liu, H., Cheng, J., et al. (2006). Generation of three-dimensional hepatocyte/gelatin structures with rapid prototyping system. Tissue Eng. 12, 83–90. doi: 10.1089/ten.2006.12.83
Wang, X., Yan, Y., and Zhang, R. (2007). Rapid prototyping as a tool for manufacturing bioartificial livers. Trends Biotechnol. 25, 505–513. doi: 10.1016/j.tibtech.2007.08.010
Williams, J. M., Adewunmi, A., Schek, R. M., Flanagan, C. L., Krebsbach, P. H., Feinberg, S. E., et al. (2005). Bone tissue engineering using polycaprolactone scaffolds fabricated via selective laser sintering. Biomaterials 26, 4817–4827. doi: 10.1016/j.biomaterials.2004.11.057
Woodard, L. N., Kmetz, K. T., Roth, A. A., Page, V. M., and Grunlun, M. A. (2017). Porous Poly(epsilon-caprolactone)-Poly(L-lactic acid) Semi-Interpenetrating networks as superior, defect-specific scaffolds with potential for cranial bone defect repair. Biomacromolecules 18, 4075–4083. doi: 10.1021/acs.biomac.7b01155
Woodfield, T. B., Malda, J., de Wijn, J., Peters, F., Riesle, J., and van Blitterswijk, C. A. (2004). Design of porous scaffolds for cartilage tissue engineering using a three-dimensional fiber-deposition technique. Biomaterials 25, 4149–4161. doi: 10.1016/j.biomaterials.2003.10.056
Wu, W., DeConinck, A., and Lewis, J. A. (2011). Omnidirectional printing of 3D microvascular networks. Adv. Mater. 23, H178–H183. doi: 10.1002/adma.201004625
Xu, T., Zhao, W., Zhu, J. M., Albanna, M. Z., Yoo, J. J., and Atala, A. (2013). Complex heterogeneous tissue constructs containing multiple cell types prepared by inkjet printing technology. Biomaterials 34, 130–139. doi: 10.1016/j.biomaterials.2012.09.035
Yang, S. F., Leong, K. F., Du, Z. H., and Chua, C. K. (2002). The design of scaffolds for use in tissue engineering. Part II. Rapid prototyping techniques. Tissue Eng. 8, 1–11. doi: 10.1089/107632702753503009
Young, R. G., Butler, D. L., Weber, W., Caplan, A. I., Gordon, S. L., and Fink, D. J. (1998). Use of mesenchymal stem cells in a collagen matrix for Achilles tendon repair. J. Orthop. Res. 16, 406–413. doi: 10.1002/jor.1100160403
Zein, I., Hutmacher, D. W., Tan, K. C., and Teoh, S. H. (2002). Fused deposition modeling of novel scaffold architectures for tissue engineering applications. Biomaterials 23, 1169–1185. doi: 10.1016/s0142-9612(01)00232-0
Zhang, B. Q., Sun, H., Wu, L. N., Ma, L., Xing, F., Xing, F., et al. (2019). 3D printing of calcium phosphate bioceramic with tailored biodegradation rate for skull bone tissue reconstruction. Biodes. Manuf. 2, 161–171. doi: 10.1007/s42242-019-00046-7
Zhu, W., Ma, X., Gou, M., Mei, D., Zhang, K., and Chen, S. (2016). 3D printing of functional biomaterials for tissue engineering. Curr. Opin. Biotechnol. 40, 103–112. doi: 10.1016/j.copbio.2016.03.014
Zorlutuna, P., Annabi, N., Camci-Unal, G., Nikkhah, M., Cha, J. M., Nichol, J. W., et al. (2012). Microfabricated biomaterials for engineering 3D tissues. Adv. Mater. 24, 1782–1804. doi: 10.1002/adma.201104631
Keywords: 3D printing, tissue engineering, bioink, scaffold, regenerative medicine
Citation: Chung JJ, Im H, Kim SH, Park JW and Jung Y (2020) Toward Biomimetic Scaffolds for Tissue Engineering: 3D Printing Techniques in Regenerative Medicine. Front. Bioeng. Biotechnol. 8:586406. doi: 10.3389/fbioe.2020.586406
Received: 23 July 2020; Accepted: 05 October 2020;
Published: 04 November 2020.
Edited by:
Hon Fai Chan, The Chinese University of Hong Kong, ChinaReviewed by:
Fatemeh Kabirian, KU Leuven, BelgiumJiacan Su, Second Military Medical University, China
Changchun Zhou, Sichuan University, China
Copyright © 2020 Chung, Im, Kim, Park and Jung. This is an open-access article distributed under the terms of the Creative Commons Attribution License (CC BY). The use, distribution or reproduction in other forums is permitted, provided the original author(s) and the copyright owner(s) are credited and that the original publication in this journal is cited, in accordance with accepted academic practice. No use, distribution or reproduction is permitted which does not comply with these terms.
*Correspondence: Youngmee Jung, winnie97@kist.re.kr
†These authors have contributed equally to this work