- Beijing Key Laboratory of Resource-oriented Treatment of Industrial Pollutants, International Science and Technology Cooperation Base for Environmental and Energy Technology of Ministry of Science and Technology of People's Republic of China, School of Energy and Environmental Engineering, University of Science and Technology Beijing, Beijing, China
Previous studies showed that adding zero valent iron (ZVI) can increase the methane production and degradation rate of organic waste by improving the performance of anaerobic digester. However, our study firstly found that ZVI (37 μm, 10 g/L) inhibited the anaerobic digestion (AD) of cow manure and lignocellulose. ZVI significantly increased the methanogenic rate of cow manure in the first 6 days, but decreased the accumulative methane yield and volatile fatty acids yield by 10.3 and 12%, respectively. The effect of ZVI on AD of liquid biomass separated from cow manure was positive, but the effect on solid biomass was negative. These results indicated that ZVI enhanced the AD of easily biodegradable organics but inhibited the biodegradation of refractory organics (lignocellulose). By analyzing the varying effects of ZVI in diverse anaerobic systems, it was found that the effects were influenced by the characteristics of substrate and inoculum-substrate ratio. This study suggested that only proper ZVI addition can improve the AD process depending on the feeding materials.
Introduction
Anaerobic digestion (AD), with the merits of reducing organic pollution, energy recovery, and low operation cost, has become a widespread pathway in converting organic waste to energy (Romero-Güiza et al., 2016). Agricultural solid waste, including livestock manure and crop straw, is regarded as a reused biomass resource due to its high content of biodegradable organic matters and nutrients (Lu et al., 2016). Among them, cow manure (one of the main livestock manure) contains not only easily degradable organics such as proteins, lipids, and soluble polysaccharides, but also refractory organics such as lignocellulose. As a major carbon source of agricultural solid waste in AD system, lignocellulose is difficult to be biodegraded because of its highly resistant and recalcitrant biomass structure (Sawatdeenarunat et al., 2015). To improve the biodegradability of substrate and the efficiency of AD, several approaches have been suggested and evaluated in many studies, such as mechanical or chemical pretreatment (Hendriks and Zeeman, 2009), co-digestion with several mixed substrates (Wang et al., 2018), and introduction of additives (Romero-Güiza et al., 2016).
Zero valent iron (ZVI)—a strong reductive material—is among the most prominent additive to enhance the AD of wastewater and waste activated sludge due to its non-toxicity, abundance, low cost, and easy manufacturing (Hwang et al., 2019). ZVI promotes the hydrogenotrophic methanogenesis by providing electrons or hydrogen evolution from the iron corrosion, which results in increased CH4 production from the consumption of CO2, as shown in Equations (1)–(3) (Hu et al., 2015; Xu et al., 2019).
In addition, ZVI also accelerates the hydrolysis process of sludge by converting particulate matter to soluble substrates, and enhances the conversion of propionate to acetate (Meng et al., 2013). ZVI (20 g/L) increased the accumulative methane production by 43.5% of waste activated sludge, promoted the decomposition of protein and polysaccharide, and accelerated both methanogenesis and hydrolysis–acidification processes (Feng et al., 2014). However, a recent report suggested that ZVI has a positive effect on the AD of waste activated sludge, but has no, or little effect on the solubilization, hydrolysis, and acidification processes (Zhao et al., 2018). Moreover, the effect of microscale ZVI on the biochemical methane potential (BMP) of blackwater is negligible (Xu et al., 2019). Therefore, ZVI has a positive effect on AD of some organic wastes, especially waste activated sludge, and has diverse effects on AD in various systems.
Many studies have investigated the role of ZVI in the AD of various organic wastes, but the effect on cow manure is still unknown. In the anaerobic co-digestion process of cow manure and Phragmites straw, Fe2+ increased the accumulative biogas yield and methane content by 18.1 and 8.3%, respectively, and extended the gas production peak stage by improving the cellulase activities (Zhang et al., 2016). Compared with Fe2+, ZVI can decrease oxidative–reductive potential of the anaerobic digestion media, serve as electron donor for hydrogenotrophic methanogens, and provide a more favorable environment for anaerobic digestion. However, waste iron powder had no effect on digestion performances of cow manure (Andriamanohiarisoamanana et al., 2018). Therefore, it is inconclusive that whether ZVI can enhance the AD of cow manure and accelerate the hydrolysis, acidification and methanogenesis process.
This study investigated the effects of ZVI on the hydrolysis–acidification processes and methanogenesis of cow manure. Liquid and solid biomass separated from cow manure were used for BMP tests to study the mechanism of ZVI inhibition. Besides, this study investigated the effects of ZVI on AD of two model substrates (starch and cellulose) and cow manure under varying inoculum-substrate ratio (ISR). ZVI played diverse roles in different AD systems depending on the components of substrate, which was studied and summarized to provide a reference for the practical application of ZVI in AD system.
Materials and Methods
Materials
Cow manure and untreated inoculum were collected from the same dairy farm of Beijing, China. Waste activated sludge was collected from a municipal wastewater treatment plant of Beijing, China. The cow manure was stored in a sealed bag and placed in a refrigerator at −20°C before experiments. The inoculum was incubated with glucose for 2 weeks, and removed the supernatant before use. Waste activated sludge was stored in a serum bottle and placed in a refrigerator at 4°C before experiments. Microcrystalline cellulose (90 μm) and starch (AR) were purchased from Shandong Xiya Reagent Co., Ltd. and Sinopharm Chemical Reagent Co., Ltd., respectively. ZVI (purity > 98%, 37 μm in diameter) was purchased from Aladdin Reagent Co., Ltd., China.
Cow manure was washed with an equal weight of deionized water and extruded by a sieve (0.2 mm) for solid–liquid separation. The separated liquid was labeled as liquid cow manure, and the separated solid was labeled as residue. The main characteristics of cow manure, liquid cow manure and residue are listed in Table 1. The contents of total solid (TS) of untreated inoculum, inoculum, and waste activated sludge were 4.96, 4.44, and 4.84%, respectively. The contents of volatile solid (VS) of untreated inoculum, inoculum, and waste activated sludge were 55.64, 53.14, and 51.18%, respectively. The content of total soluble iron of inoculum was 2.29 mg/L.
Experimental Design
The effects of ZVI on AD of several substrates were investigated using BMP tests. BMP tests were conducted by an Automatic Methane Potential Test System II (Bioprocess Control AB, Sweden), as described below. The mixture (VSinoculum:VSsubstrate = 2:1) of substrate and inoculum in a 500 mL serum bottle (working volume = 400 mL) was placed in a water bath at 36 ± 1°C. All serum bottles were sealed with butyl rubber stoppers after flushing with nitrogen, and stirred for 1 min every 5 min at 120 rpm by an automatic stirring rod. A serum bottle (working volume = 80 mL) containing 3M NaOH was connected to the reactor through a natural latex tube to fix CO2. Biogas volume (without CO2) was automatically recorded after entering the gas volume measuring device through a natural latex tube.
ZVI particles were added to reach the final concentration of 10 g/L in the 500 mL serum bottle, which was selected on the base of the effective dosage range in literature. Each batch experiment included a control (without ZVI, labeled as C) and a ZVI addition group (labeled as Z). Six sets of BMP tests under ISR of 2 were set up as follows: cow manure (C-CM1, Z-CM1), liquid cow manure (C-LCM, Z-LCM), residue (C-R, Z-R), microcrystalline cellulose (C-MC, Z-MC), starch soluble (C-S, Z-S) and cow manure inoculated with untreated inoculum (C-UI, Z-UI). To explored the effects of ZVI on the hydrolysis–acidification of cow manure, 50 mM sodium 2-bromoethanesulfonate (SBES) was added in C-CM2 and Z-CM2 to remove methanogens (Feng et al., 2014), and the mass ratio of substrate to inoculum was fixed at 1:1 (on the basis of VS). All trials were conducted in triplicate.
Chemical Analysis
TS and VS were determined by differential weighing after drying at 105 °C overnight and by subsequent incineration at 550°C, respectively, according to standard methods. pH was directly determined with a pH meter (HQ30d, Hach, USA). Cellulose, hemicellulose, and lignin contents of cow manure and residue were determined by Van Soest. Starch content was determined by the sulfonic acid ketone method. Carbon and nitrogen contents in dried biomass materials were detected by an elemental analyzer (vario EL cube, Elementar, Germany). For determination of the total metallic iron, cow manure and residue were digested with HNO3, then the digested liquid was filtered by 0.45 μm membrane and quantified for total soluble iron. Liquid samples were centrifuged at 8,000 rpm for 20 min at 4°C and filtered through a 0.45 μm polyether sulfone membrane to quantify the soluble product. The concentration of total soluble iron was measured by inductively coupled plasma-optical emission spectroscopy (725 ES, Agilent, USA). Soluble polysaccharide and soluble proteins were measured by using phenol–sulfuric acid method and Bradford Protein Assay Kit, respectively, with a microplate spectrophotometer (SpectraMax Plus384, Molecular Devices, USA). Volatile fatty acids (VFAs) were analyzed by a gas chromatograph (GC-8600, Beijing) equipped with a flame ionization detector. The operating temperatures of the oven, injection port, and detector were 130, 220, and 250°C, respectively. The injection volume was 1 μL, and He was the carrier gas. The composition of biogas was analyzed using the same gas chromatograph equipped with a thermal conductivity detector. The temperature of the detector was 70°C.
Statistical Analysis
SPSS (SPSS 24.0) was used for t-test analysis and p < 0.05 was considered to be statistically different.
Data Analysis
The theoretical moles of methane for each mole of the substrate was calculated by the following stoichiometric equation (Buswell and Mueller, 1952):
The theoretical methane potential (YTh, mL/g VS) of the substrate was calculated using the theoretical moles of methane by the ideal gas law, as shown in Equation (5):
The level of anaerobic biodegradability (BDCH4, %) was calculated by the accumulative methane yield (YExp,mL/g VS) under the BMP test in comparison with its theoretical value as follows (Elbeshbishy et al., 2012):
Results and Discussion
Effects of ZVI on the AD of Cow Manure
During the 25 days digestion, biogas and supernatant were measured every day to analyze the effect of ZVI on AD of cow manure. Figure 1A shows the changes in accumulative methane. Both digesters started to produce methane quickly after a short period of adaptation, and the accumulative methane yield increased quicker in Z-CM1 than C-CM1 during the first 10 days. Compared with the control group, ZVI increased the methane yield in the initial stage, but gradually decreased it in the subsequent stages. After digestion, the groups treated with 10 g/L ZVI had significantly lower accumulative methane yield than the control, which were 196.6 ± 2.9 mL/g VS and 219.2 ± 7.8 mL/g VS, respectively. This result was inconsistent with the previous studies that ZVI played an active role and increased the methane production in some AD system (Hao et al., 2017; Kong et al., 2018). However, cow manure was not used as a substrate in previous studies, which might be the reason for the difference. There existed two methanogenesis periods (days 1–7 and days 7–25) of the daily methane yield (Figure 1B). During the first period, the higher daily methane yield was observed in Z-CM1. Then, the daily methane yield of Z-CM1 rapidly decreased from day 7 and remained at a much lower value than that of C-CM1 till the end. ZVI enhanced the methanogenic process of cow manure in first few days but reduced methane yield in following days and played a negative role. Easily degradable organics produced CH4 in the first period, and poorly biodegradable organics contributed to CH4 production in the second period (Zhang et al., 2019). Cow manure is a mixture of organic components with varying biodegradability, and contains plenty of lignocellulose (516.1 g/kg TS), which is difficult to be biodegraded. Thus, ZVI had opposite effects during the two periods probably because ZVI facilitated the AD process of easily degradable organics in cow manure but inhibited the AD process of refractory organics.
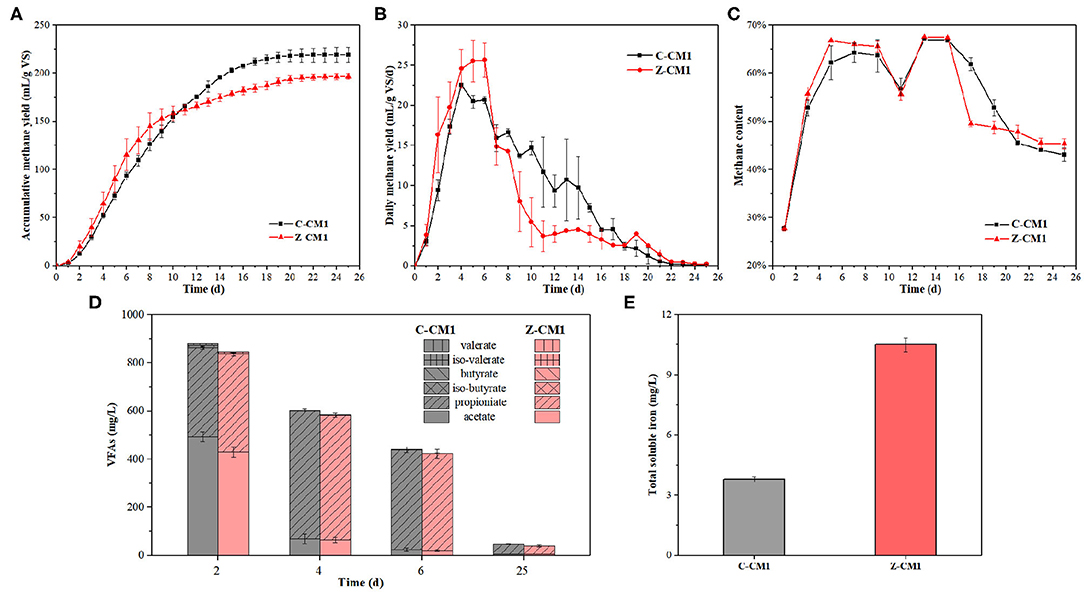
Figure 1. Changes in accumulative methane yield (A), daily methane yield (B), methane content (C), and concentration and composition of VFAs (D) in AD of cow manure by BMP tests. (E) The content of total soluble iron in two reactors on day 25. C-CM1: reactors (500 mL) without ZVI; Z-CM1: reactors (500 mL) with 10 g/L ZVI.
The VFAs concentrations of digestate from both groups are shown in Figure 1D. Acetic acid and VFAs concentration in C-CM1 on day 2 were 492.6 and 880.8 mg/L, respectively, which were higher than those of Z-CM1 with 429.1 and 845.9 mg/L, respectively. The lower VFAs concentration in Z-CM1 indicated that ZVI facilitated the conversion of VFAs by improving the metabolic activity of anaerobic microbes (Yuan et al., 2020). Subsequently, VFAs concentration dropped as methanogen consumed acetic acid, and ZVI had no significant effect on the formation of acetic acid and VFAs at the same time (p > 0.05). During the AD of cow manure, VFAs concentration was always at a lower level compared with that in other studies, and remained far below the inhibitory levels, which indicated that methanogens were sufficient in both groups to convert acetic to CH4 in time, thus hydrolysis process was the rate-limiting step in AD of cow manure. ZVI had no effect on VFAs production from blackwater (Xu et al., 2019), protein, and carbohydrates (Zhao et al., 2018) but effectively increased the total VFAs yield from swine manure (Yang et al., 2018) and waste activated sludge (Feng et al., 2014). Therefore, the effect of ZVI on VFAs concentration in AD system associated with the composition of substrate and the organic loading rate of reactor. Most of the iron introduced into the anaerobic system is not readily accessible to microorganisms. Therefore, adding iron to iron-deficient digestion system can increase soluble iron content, which can increase the growth and metabolism of microorganisms (Cai et al., 2018). It could be observed from Figure 1E that the total soluble iron in Z-CM1 at significantly higher compared to that in C-CM1 at the end of the digestion, due to the iron corrosion. As the digestion progressed, available iron was gradually released from organic matters decomposition and adsorbed by intracellular enzymes in microorganisms or by suspended solids and microbial surfaces (Cai et al., 2019). The final total soluble iron of C-CM1 was 3.80 ± 0.13 mg/L, higher than the original total soluble iron at the first day. These results showed that the soluble iron was enough in this system for microorganisms and enzymes, as ingested iron from animal feed was excreted in cow manure (Yang et al., 2018), which again confirmed that the effect of ZVI in AD system was affected by the characteristics of the substrate.
Figure 1C shows the methane content of biogas in two groups. The methane content rapidly increased at the beginning, and reached the first peak on day 2, followed by a transient drop on day 11, and rebounded to the second peak on day 13. The reactors with ZVI addition had higher methane content of biogas than the control in the first 9 days. Methane was either produced via the cleavage of acetate (aceticlastic pathway) or by the reduction of CO2 with hydrogen (hydrogenotrophic pathway) and from methylated C1 compounds to a minor extent. ZVI sustained a high syntrophic hydrogenotrophic methanogenesis activity (Yang et al., 2018), which contributed to the high utilization efficiency of CO2 to produce CH4, thereby resulting in a high methane content. Zhao et al. (2018) also confirmed that ZVI remarkably promoted hydrogenotrophic and syntrophic methanogenesis. The dominant metabolic pathway for CH4 production at the startup phase was hydrogenotrophic methanogenesis, and then turned into aceticlastic methanogenesis in the latter steady phase (Huang et al., 2017). Therefore, ZVI increased the methane content at the early stage by enhancing the hydrogenotrophic methanogenesis.
Effect of ZVI on the Acidification Phase of Cow Manure
During the acidification process, complicated organics were first hydrolyzed into simpler and soluble organic compounds and then converted to VFAs by the biotransformation process referring to acidogenesis (De La Rubia et al., 2009). The decomposition rate of protein and polysaccharide typically limited the efficiency of AD. Thus, the concentrations of soluble protein and polysaccharide were measured to assess the solubilization of cow manure. Figure 2A shows that the concentrations of soluble protein and polysaccharide in Z-CM2 represented no significant difference compared with the control. The low concentrations of soluble protein and polysaccharide in both groups indicated that most of easily degradable organics were hydrolyzed into VFAs after 2 days. Under the addition of SBES, ZVI had no effect on the hydrolysis of protein and polysaccharide from easily degradable organics. Consistent with this study, ZVI had no or minimal effect on the hydrolysis process of bovine serum albumin and dextran, and drove hydrolysis forward in thermodynamics by enhancing methanogenesis (Zhao et al., 2018).
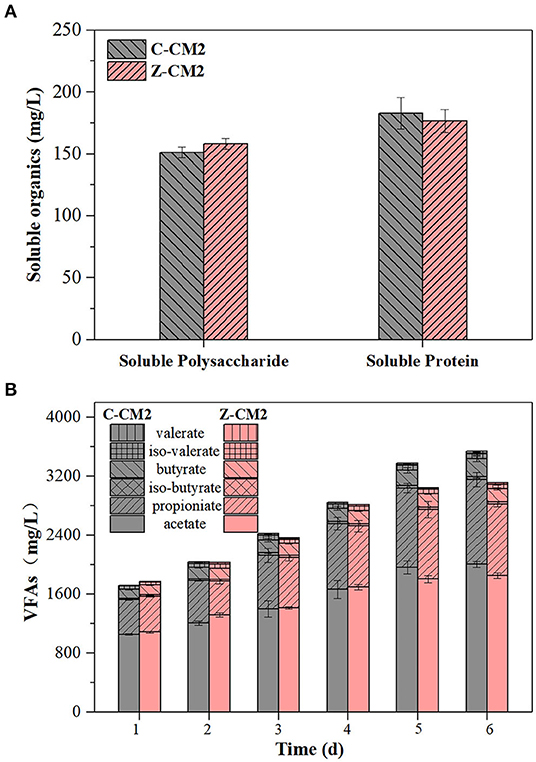
Figure 2. Effect of ZVI on the acidification phase of cow manure with SBES: (A) the soluble protein and polysaccharide concentrations on day 2; (B) the concentration and composition of VFAs. C-CM2: reactors (500 mL) without ZVI; Z-CM2: reactors (500 mL) with 10 g/L ZVI.
The most crucial product in the acidification phase is VFAs, because it is converted to acetate, H2, and CO2, which can further serve as a direct nutrient source for methanogenesis (Lu et al., 2018). SBES was added to reactors in order to prevent the VFAs from being consumed by methanogens. Figure 2B shows the concentrations of five types of VFAs, which contains acetate, propionate, iso-butyrate, butyrate, iso-valerate, and valerate. Total amounts of VFAs in two groups were basically non-distinctive in the first 4 days. Easily degradable substances (e.g., starch, proteins, and lipid) were degraded and converted into VFAs at this period. This result reconfirmed that ZVI had no or minimal effect on the hydrolysis process of easily degradable organics. The paired samples t-test indicated that ZVI significantly influenced the accumulation of VFAs on days 5 and 6 (p < 0.05). At the end of the experimental time, the VFAs concentration in Z-CM2 was 3539.8 mg/L, which was 12.0% lower than that of C-CM2. The higher accumulative VFAs production in Z-CM2 from day 5 indicated that ZVI suppressed the hydrolysis and acidification of refractory organic substances, such as lignocellulose. Therefore, ZVI decreased VFAs production by inhibiting the hydrolysis–acidification processes of cow manure, thereby decreasing the accumulative methane production.
Effects of ZVI on the AD of Substrates With Different Biodegradability
According to the analysis of anaerobic performance in four reactors, ZVI had different effects on AD of various organics due to their varying biodegradability. Therefore, cow manure was separated to liquid part (liquid cow manure) containing easily degradable organics (e.g., protein, lipid, and soluble organics) and solid part (residue) containing refractory organics for BMP tests. Lignocellulose was the main refractory organic component of cow manure in this study—up to 51.61%, and difficult to be degraded due to its complex structure (Wang et al., 2018). Figure 3 shows the changes in accumulative methane yields and daily methane yields of liquid cow manure and residue during 22 days. ZVI showed a sustained promotion or inhibition effect on the AD of liquid cow manure and residue, respectively. The accumulative methane yield of liquid cow manure (Z-LCM) reached 125.4 ± 7.4 mL/g VS, which was 15.2% higher than that of C-LCM. And the accumulative methane yield of residue (Z-R) was 50.8 ± 3.5 mL/g VS, which was 20.4% lower than that of C-R. The higher methanation rate of liquid cow manure indicated that it was more accessible for the microorganisms to degradation than the residue. Because unbalanced C/N ratio of two substrates and inefficient anaerobic systems, the accumulative methane yields of both groups were lower than that of cow manure. Besides, ZVI had no significant influence on the AD of residue in the first few days but gradually decreased the accumulative methane yield from day 7, which suggests that ZVI mainly inhibited the degradation of residue at the later stage.
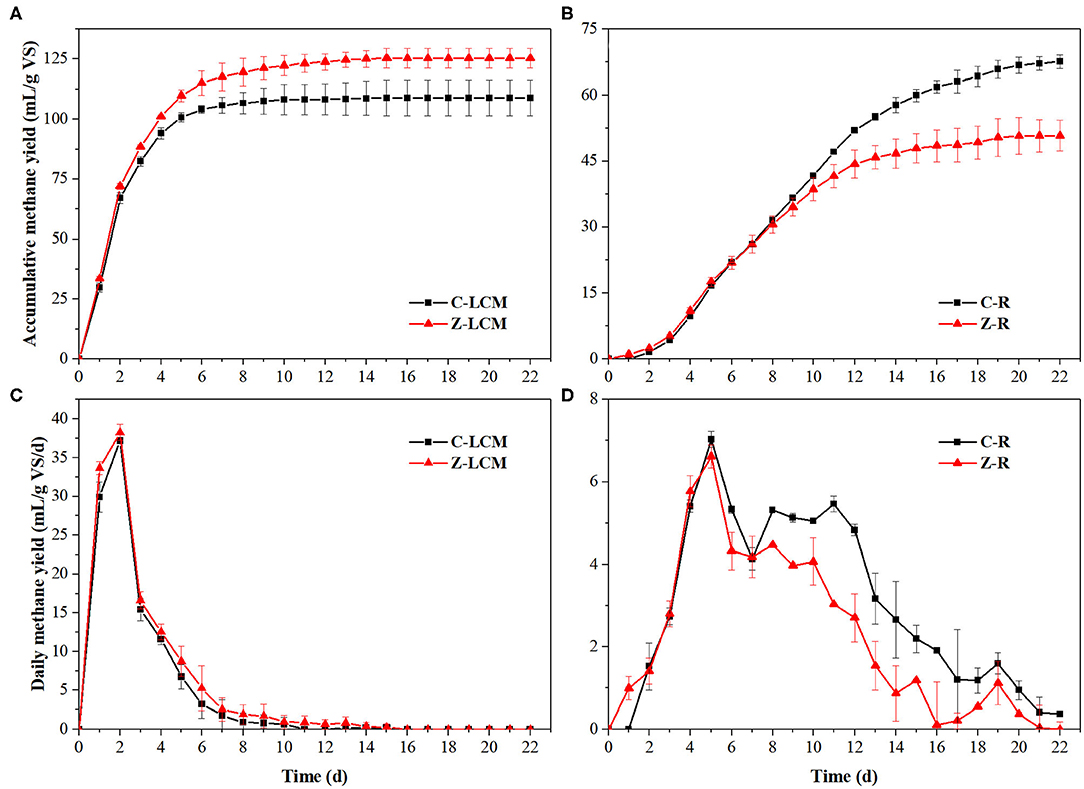
Figure 3. Changes in accumulative methane yield (A,B) and daily methane yield (C,D) in AD by BMP tests. C-R, AD of residue (solid part from solid–liquid separation of cow manure) without ZVI; Z-R, AD of residue with 10 g/L ZVI; C-LCM, AD of liquid cow manure (liquid part from solid–liquid separation of cow manure) without ZVI; Z-LCM, AD of liquid cow manure with 10 g/L ZVI.
Microbes needed several days to decompose the lignocellulose into monomers, whereas the duration of hydrolysis of soluble carbohydrates was only a few hours. From Figure 3C, the daily methane yields of C-LCM and Z-LCM rapidly increased and reached maximum methane yield rate on day 2, respectively, then rapidly decreased to below 1 mL/g VS/day. Z-R began to produce biogas on day 1, whereas C-R started on day 2, which suggested that microorganisms in Z-R adapted to the environment better. The daily methane yields of residue were much lower than that of liquid cow manure, and reached the first peak of 7.0 ± 0.2 and 6.6 ± 0.9 mL/g VS/day on day 5, then reached the second peak on days 9–12. In the two methanogenesis stages, the difference between Z-R and C-R was negligible in the first stage but C-R performed better in the second stage, which indicated that ZVI mainly inhibited the AD of lignocellulose in residue.
ZVI decreased the methane yield of residue in this study, but Fe3+ showed no effect on methane yield of rice straw (Mancini et al., 2019) and Fe2+ increased the methane yield of another rice straw (Cai et al., 2017). As another major agriculture solid waste, rice straw also contained lots of lignocellulose, up to 63.13% (Cai et al., 2017). The reason for the difference might be related to different particle sizes: rice straw was cut down to a particle size smaller than 4 mm (Mancini et al., 2019); another rice straw was pulverized with a high-speed grinder and passed through a 1 mm mesh filter (Cai et al., 2017). Because milling (cutting the lignocellulosic biomass into smaller pieces) decreased particle size and crystallinity, which increased the available specific surface of substrate and decreased the degree of polymerization, contributing to an increase in hydrolysis yield of lignocellulose by 5–25% in most cases (Hendriks and Zeeman, 2009). Methane production was increased as the hydrolysis–acidification process was enhanced. Therefore, the methane production decrement could be offset by decreasing the particle size or promoting the hydrolysis. These results suggested that complex lignocellulosic biomass required an enhancement of the hydrolysis process by pretreatment rather than ZVI alone. For example, alkali treatment can destroy the structure of lignocellulose, thereby making it easier to be decomposed, which is beneficial to the digestion with Fe (Khatri et al., 2015).
BMP Tests of Model Substrates
The main carbon sources in the residue were starch and lignocellulose, with the contents of 8.69 and 68.85%, respectively. Because ZVI had no effect on AD of residue in the beginning, and had negative effect on AD of lignocellulose at a later stage, starch—the uppermost renewable carbon source in residue besides lignocellulose—was used as a substrate for BMP test. Starch was synthesized in semi-crystalline granular structures polymerized by glucose, which was easily to be degraded (Wang et al., 2016). Figure 4 shows that the accumulative methane yield of Z-S was slightly higher than that of control in the first 2 days, and the daily methane yield of Z-S was significantly higher than that of C-S on day 2. This happened because ZVI promoted hydrogenotrophic and syntrophic methanogenesis, thereby increasing the rate of methanogenesis (Zhao et al., 2018). The daily methane yield of two groups began to drop from day 3 with the consumption of substrate, and then Z-S produced lower daily methane yield than control due to the faster consumption of substrate at the beginning. As shown in Figure 4A, there is no significant difference between the accumulative methane yields of Z-S and C-S, that is, ZVI had no effect on the BMP of starch, resulting in the alike accumulative methane yield of Z-R and C-R in the first few days (Figure 2A).
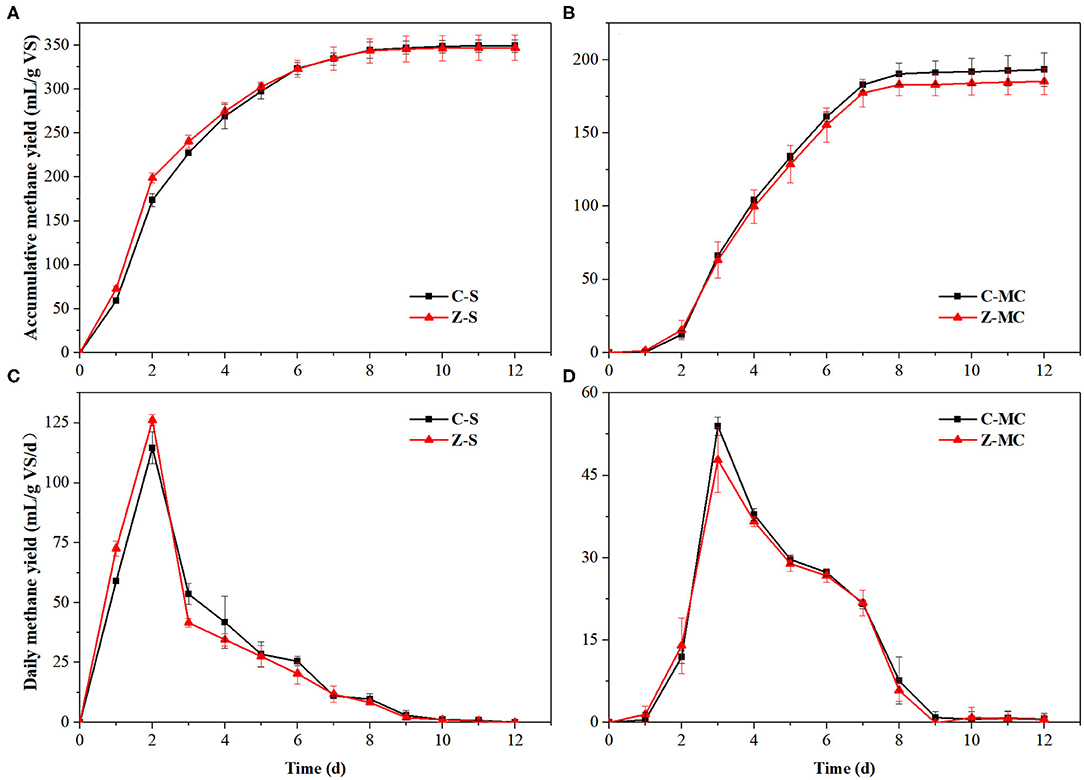
Figure 4. Changes in accumulative methane yield (A,B) and daily methane yield (C,D) in AD by BMP tests. C-MC, AD of microcrystalline cellulose without ZVI; Z-MC, AD of microcrystalline cellulose with 10 g/L ZVI; C-S, AD of starch without ZVI. Z-S, AD of starch with 10 g/L ZVI.
Lignocellulose primarily consists of cellulose, hemicellulose, and lignin. Among them, cellulose is composed of polymerized glucose molecules and has the highest organic carbon level in the residue (31.23%). Compared with lignin and xylose, cellulose could be degraded to produce a considerably higher accumulative methane production per gram, and contributed to most methane production in lignocellulose (Wyman et al., 2019). Both starch and cellulose are polysaccharides of glucose, with the same chemical formula—(C6H10O5)n, whose theoretical methane potential was 414.81 mL/g VS. According to Equation (6), the level of anaerobic biodegradability of starch was 84.19%, which is much higher the cellulose of 46.59%. Daily methane yield in Z-MC was 6.0 mL/g VS/day on the first day, whereas it was 0.9 mL/g VS/day in C-MC, demonstrating that ZVI could shorten the start-up process of AD. The comparison of accumulative methane yield in Z-MC and C-MC showed that ZVI had no effect on the anaerobic degradation of cellulose. Microcrystalline cellulose was almost completely degraded on day 9, whereas the daily methane production in Z-R was gradually lower than that of C-R from day 8 (Figure 3D). These results suggested that ZVI mostly suppressed the disintegration and degradation of the recalcitrant lignocellulosic structure rather than cellulose. The lignocellulosic structure was formed by cross-linking among cellulose, hemicellulose, and lignin, which was a barrier for liquid penetration and enzyme access, thereby impeding the digestion of lignocellulosic biomass (Yang et al., 2015).
The disintegration and degradation of the lignocellulosic biomass was limited by several factors, including crystallinity of cellulose, available surface area, degree of polymerization, moisture content and lignin content (Hendriks and Zeeman, 2009). In anaerobic system, ZVI reacted with water to form hydrogen, which could simply be expressed by Equation (7). As this reaction proceeded, the precipitation of Fe(OH)2 became favorable, and then Fe(OH)2 might be transformed to magnetite according to Equation (8) (Wei et al., 2018):
Majority of nanoscale ZVI was precipitated in the form of FeOOH or iron oxide via the reaction with H2O (He et al., 2017). Any excess iron could precipitate as iron carbonate as long as the pH is 6.4 or above, and the pH values in all BMP tests was above 7.0 in this study. As ZVI was gradually corroded, Fe(II) and Fe(III) solids formed by ZVI would be adsorbed on the surface of lignocellulose, which reduced the available surface area of lignocellulose and the accessibility of cellulose, thereby inhibiting of the hydrolysis of lignocellulosic biomass.
Effects of ZVI on the AD of Cow Manure Under Different Condition of Inoculum
Figure 5A shows the accumulative methane yield of untreated inoculum by BMP tests, which was collected from anaerobic fermentation tank in the dairy farm. The fresh inoculum was incubated at 36 ± 1°C for 2 weeks before use to consume excess substrate. The characteristic of inoculum is a crucial factor affecting the biochemical reactions during AD. As shown in Figure 5B, ZVI increased the accumulative methane yield of cow manure inoculated with untreated inoculum, which is contrary from the result described above. Because the anaerobic fermentation tank was feed with liquid cow manure, untreated inoculum contains more easily degradable organics. The increase of accumulative methane yield from easily degradable organics was higher than the decrease of accumulative methane yield from lignocellulose aroused by ZVI.
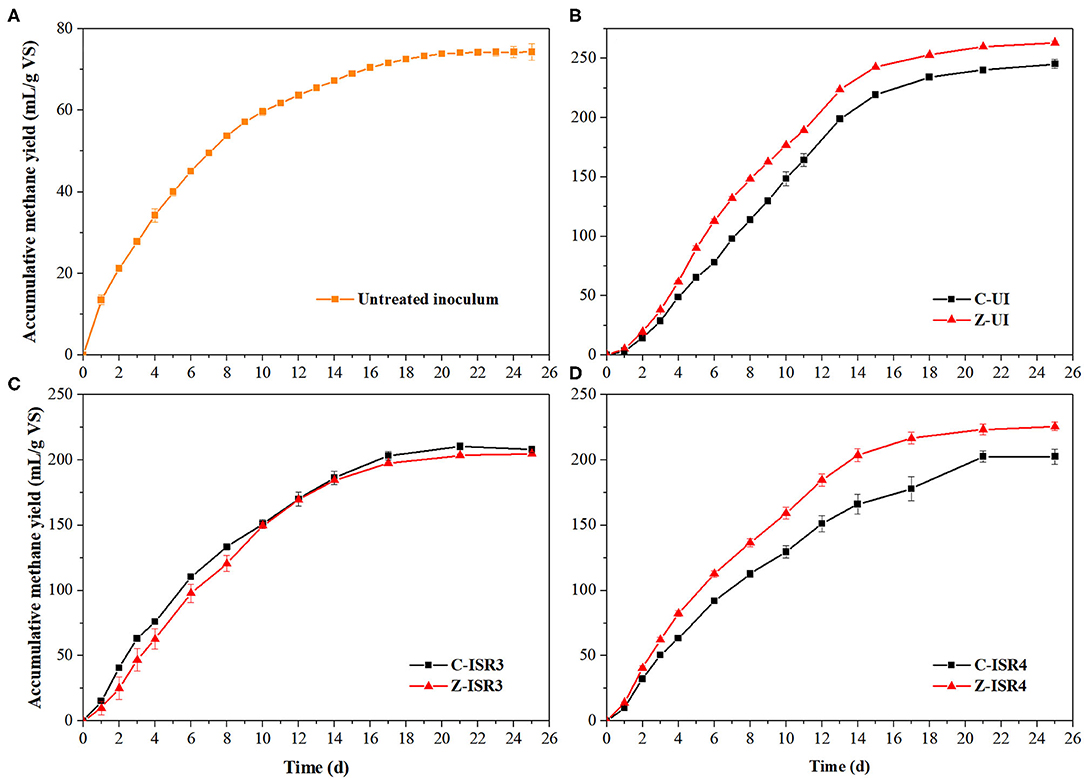
Figure 5. (A) Accumulative methane yield of untreated inoculum by BMP tests. And the effects of 10 g/L ZVI on accumulative methane yield of cow manure in various systems: (B) Inoculation with untreated inoculum; (C) ISR = 3; (D) ISR = 4.
ISR is an important factor for the start of a balanced microbial population in anaerobic system, and an appropriate ISR is beneficial to balance the bacteria and archaea associated with the acidification and methanogenic process (Li et al., 2018; Zhang et al., 2020). A lower ISR means fewer methanogens and higher risk of VFAs inhibition, resulting in decreased biogas and methane yields (Latifi et al., 2019). A higher ISR can increases microbial populations and buffering capacity, but the excessive inoculum takes space and reduces the reactor utilization efficiency (Li et al., 2018). The effects of ZVI on the AD of cow manure under ISR of 3 and 4 were shown in Figures 5C,D, respectively. There was no significant difference among the accumulative methane yield of cow manure without ZVI under varying ISR of 2, 3, and 4, which indicated that there were enough microbes in three anaerobic systems to efficiently consume the substrates. At the same time, ZVI increased the accumulative methane yield of Z-ISR4 by 11.4%, and showed no effect on Z-ISR3, which meant that increasing ISR could alleviate even change the ZVI inhibition. With the increasing ISR, more microbes and easily degradable organics were introduced to anaerobic system, resulting in higher hydrolytic degree and biodegradability of total organics, thereby increasing the increment of methane production within methanogenesis process caused by ZVI.
Comparison of the Effects of ZVI on the AD of Different Substrates
Table 2 summarizes diverse effects of Fe on the AD system performance of various substrates. The influences of Fe2+ or Fe3+ was adopted because few studies investigated the effects of ZVI on AD of cow manure or lignocellulosic biomass. When the substrate was 30 g VSsubstrate/L organic fraction of municipal solid waste, reactors experienced a period of long-term excessive acidification from day 2.5, whereas ZVI could alleviate excessive acidification (Kong et al., 2018). The methane productivity of waste activated sludge at ZVI of 20 g/L increased by 43.5%, in which protein and polysaccharide accounted for 63.0 and 12.3% of organic matter, respectively (Feng et al., 2014). As shown in Figure 6, more (p < 0.05) methane was produced from waste activated sludge than the control during the whole period by adding ZVI at the studied six levels (i.e., 2, 4, 6, 8, 10, and 15 g/L), which was consistent with the results of waste activated sludge treatment in other studies (Feng et al., 2014; Zhao et al., 2018). The maximal accumulative methane yield of 160.0 ± 0.6 mL/g VS was achieved at ZVI dosage of 10 g/L, while only 100.7 ± 1.8 mL/g VS was produced from waste activated sludge without ZVI addition, representing a relative increase of 58.9%. If the substrate was much easily degraded, reactors could be overloaded with high organic loading rate, especially in batch systems, causing an imbalance between the acidogenesis/acetogenesis and methanogenesis steps, resulting in a large accumulation of VFAs. Substantial accumulation of VFAs decreased alkalinity and pH values, then inhibited methanogenesis (Braz et al., 2019). ZVI could accelerate the consumption of VFAs by enhancing methanogenesis, thereby driving hydrolysis and acidification forward in thermodynamics and increasing methane production (Zhao et al., 2018). Based on the previous reported studies, the mechanisms of iron enhancement on methanogenesis are summarized as three possible pathways: i) hydrogen evolution from iron corrosion could enhance hydrogenotrophic methanogenesis; ii) as an essential trace element for anaerobes, iron contributed to the growth of microbes responsible for CH4 production; iii) iron could stimulate the activities of enzymes involved in methanogenesis (Wei et al., 2018; Zhao et al., 2018). Therefore, with easily degraded substrate or high organic loading rates, ZVI could accelerate the conversion of excess VFAs to CH4, resulting in a stable and favorable condition for microorganism and methane production increase.
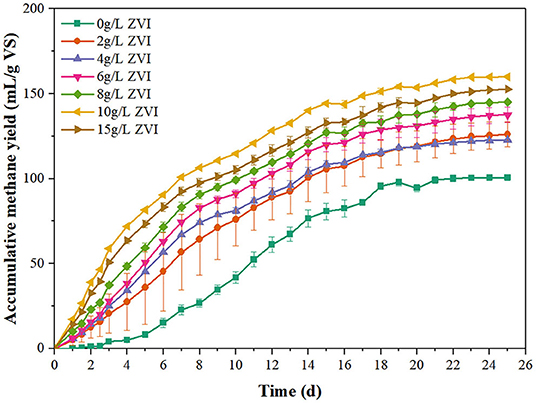
Figure 6. Accumulative methane yield from waste activated sludge during AD with and without ZVI by BMP tests.
By contrast, the main carbon source in the AD of cow manure and residue was crop straw, which was difficult to be degraded because of its complex lignocellulosic structure. According to the experimental results of this study in Table 2, ZVI increased the accumulative methane yield of liquid cow manure, but reduced the accumulative methane yield of cow manure and residue. It was concluded that Fe(II) and Fe(III) solids formed by ZVI were adsorbed on the surface of lignocellulose and reduced the available surface area of lignocellulose and the accessibility of cellulose, thereby inhibiting of the hydrolysis of lignocellulosic biomass. Besides, ZVI had potential risks of inhibition effect on bacterial activity, which could be attributed to the accumulation of solid iron particulates on the cell surface or overproduction of free radical species, thereby causing cellular injury (Wu et al., 2015). The detections of microbial community structure and enzyme activities offer a way to further explore the mechanism of ZVI inhibition in future studies. In conclusion, ZVI addition is not suitable for every AD system, which requires serious consideration.
Conclusions
This study firstly investigated the effect of ZVI on the AD of cow manure. The accumulative methane and VFAs yield decreased by 10.3 and 12% with adding 10 g/L ZVI, respectively. ZVI decreased the methane yield of residue by 20.4%, while increased that of liquid cow manure by 15.2%, and did not change that of starch and microcrystalline cellulose. Experimental results indicated that ZVI promoted the AD of easily degradable organics, such as protein, lipid, and soluble carbohydrate, but inhibited the hydrolysis–acidification process of lignocellulose by reducing the available surface area and the accessibility of cellulose. With the increasing ISR, the effect of ZVI on methane production of cow manure changed from negative to positive. Further investigation indicated that the pretreatment of lignocellulosic biomass may be required before adding ZVI to enhance hydrolysis process, and then to increase methane yield.
Data Availability Statement
The raw data supporting the conclusions of this article will be made available by the authors, without undue reservation.
Author Contributions
ZL, LZha, XZ, and SC conceptualized the study. YM, XW, and WB completed the experiment and data analysis. YM, ZL, LZhe, and XW wrote and revised the manuscript. All authors listed have made a substantial, direct and intellectual contribution to the work, and approved it for publication.
Funding
This work was supported by the International Scientific and Technological Cooperation and Exchange Projects (2016YFE0115600), the Fundamental Research Funds for the Central Universities (FRF-IC-19-001), and the Beijing Science and Technology Project (Z181100002418016). This study was also supported by the Beijing Key Laboratory of Resource-oriented Treatment of Industrial Pollutants, International Science and Technology Cooperation Base for Environmental and Energy Technology of MOST.
Conflict of Interest
The authors declare that the research was conducted in the absence of any commercial or financial relationships that could be construed as a potential conflict of interest.
Abbreviations
AD, anaerobic digestion; BMP, biochemical methane potential; ISR, inoculum-substrate ratio; SBES, sodium 2-bromoethanesulfonate; TS, total solid; VS, volatile solid; VFAs, volatile fatty acids; ZVI, zero valent iron.
References
Andriamanohiarisoamanana, F. J., Shirai, T., Yamashiro, T., Yasui, S., Iwasaki, M., Ihara, I., et al. (2018). Valorizing waste iron powder in biogas production: hydrogen sulfide control and process performances. J. Environ. Manage. 208, 134–141. doi: 10.1016/j.jenvman.2017.12.012
Braz, G. H. R., Fernandez-Gonzalez, N., Lema, J. M., and Carballa, M. (2019). Organic overloading affects the microbial interactions during anaerobic digestion in sewage sludge reactors. Chemosphere 222, 323–332. doi: 10.1016/j.chemosphere.2019.01.124
Buswell, A. M., and Mueller, H. F. (1952). Mechanisms of methane fermentation. Indus. Eng. Chem. 44, 550–552. doi: 10.1021/ie50507a033
Cai, Y., Hu, K., Zheng, Z., Zhang, Y., Guo, S., Zhao, X., et al. (2019). Effects of adding EDTA and Fe2+ on the performance of reactor and microbial community structure in two simulated phases of anaerobic digestion. Bioresource Technol. 275, 183–191. doi: 10.1016/j.biortech.2018.12.050
Cai, Y., Hua, B., Gao, L., Hu, Y., Yuan, X., Cui, Z., et al. (2017). Effects of adding trace elements on rice straw anaerobic mono-digestion: focus on changes in microbial communities using high-throughput sequencing. Bioresource Technol. 239, 454–463. doi: 10.1016/j.biortech.2017.04.071
Cai, Y., Zhao, X., Zhao, Y., Wang, H., Yuan, X., Zhu, W., et al. (2018). Optimization of Fe2+ supplement in anaerobic digestion accounting for the Fe-bioavailability. Bioresource Technol. 250, 163–170. doi: 10.1016/j.biortech.2017.07.151
De La Rubia, M. A., Raposo, F., Rincón, B., and Borja, R. (2009). Evaluation of the hydrolytic–acidogenic step of a two-stage mesophilic anaerobic digestion process of sunflower oil cake. Bioresource Technol. 100, 4133–4138. doi: 10.1016/j.biortech.2009.04.001
Elbeshbishy, E., Nakhla, G., and Hafez, H. (2012). Biochemical methane potential (BMP) of food waste and primary sludge: Influence of inoculum pre-incubation and inoculum source. Bioresource Technol. 110, 18–25. doi: 10.1016/j.biortech.2012.01.025
Feng, Y., Zhang, Y., Quan, X., and Chen, S. (2014). Enhanced anaerobic digestion of waste activated sludge digestion by the addition of zero valent iron. Water Res. 52, 242–250. doi: 10.1016/j.watres.2013.10.072
Hao, X., Wei, J., van Loosdrecht, M. C. M., and Cao, D. (2017). Analysing the mechanisms of sludge digestion enhanced by iron. Water Res. 117, 58–67. doi: 10.1016/j.watres.2017.03.048
He, C., He, P., Yang, H., Li, L., Lin, Y., Mu, Y., et al. (2017). Impact of zero-valent iron nanoparticles on the activity of anaerobic granular sludge: from macroscopic to microcosmic investigation. Water Res. 127, 32–40. doi: 10.1016/j.watres.2017.09.061
Hendriks, A. T. W. M., and Zeeman, G. (2009). Pretreatments to enhance the digestibility of lignocellulosic biomass. Bioresource Technol. 100, 10–18. doi: 10.1016/j.biortech.2008.05.027
Hu, Y., Hao, X., Zhao, D., and Fu, K. (2015). Enhancing the CH4 yield of anaerobic digestion via endogenous CO2 fixation by exogenous H2. Chemosphere 140, 34–39. doi: 10.1016/j.chemosphere.2014.10.022
Huang, W., Zhao, Z., Yuan, T., Huang, W., Lei, Z., and Zhang, Z. (2017). Low-temperature hydrothermal pretreatment followed by dry anaerobic digestion: a sustainable strategy for manure waste management regarding energy recovery and nutrients availability. Waste Manage. 70, 255–262. doi: 10.1016/j.wasman.2017.09.011
Hwang, Y., Sivagurunathan, P., Lee, M., Yun, Y., Song, Y., and Kim, D. (2019). Enhanced hydrogen fermentation by zero valent iron addition. Int. J. Hydr. Energ. 44, 3387–3394. doi: 10.1016/j.ijhydene.2018.06.015
Khatri, S., Wu, S., Kizito, S., Zhang, W., Li, J., and Dong, R. (2015). Synergistic effect of alkaline pretreatment and Fe dosing on batch anaerobic digestion of maize straw. Appl. Energ. 158, 55–64. doi: 10.1016/j.apenergy.2015.08.045
Kong, X., Yu, S., Xu, S., Fang, W., Liu, J., and Li, H. (2018). Effect of Fe0 addition on volatile fatty acids evolution on anaerobic digestion at high organic loading rates. Waste Manage. 71, 719–727. doi: 10.1016/j.wasman.2017.03.019
Latifi, P., Karrabi, M., and Danesh, S. (2019). Anaerobic co-digestion of poultry slaughterhouse wastes with sewage sludge in batch-mode bioreactors (effect of inoculum-substrate ratio and total solids). Renew. Sustain. Energy Rev. 107, 288–296. doi: 10.1016/j.rser.2019.03.015
Li, Y., Wang, Y., Yu, Z., Lu, J., Li, D., Wang, G., et al. (2018). Effect of inoculum and substrate/inoculum ratio on the performance and methanogenic archaeal community structure in solid state anaerobic co-digestion of tomato residues with dairy manure and corn stover. Waste Manage. 81, 117–127. doi: 10.1016/j.wasman.2018.09.042
Lu, X., Wang, H., Ma, F., Zhao, G., and Wang, S. (2016). Enhanced anaerobic digestion of cow manure and rice straw by the supplementation of an iron oxide–zeolite system. Energ. Fuel 31, 599–606. doi: 10.1021/acs.energyfuels.6b02244
Lu, X., Wang, H., Ma, F., Zhao, G., and Wang, S. (2018). Improved process performance of the acidification phase in a two-stage anaerobic digestion of complex organic waste: effects of an iron oxide-zeolite additive. Bioresource Technol. 262, 169–176. doi: 10.1016/j.biortech.2018.04.052
Mancini, G., Papirio, S., Lens, P., and Esposito, G. (2019). A preliminary study of the effect of bioavailable fe and co on the anaerobic digestion of rice straw. Energies 12:577. doi: 10.3390/en12040577
Meng, X., Zhang, Y., Li, Q., and Quan, X. (2013). Adding Fe0 powder to enhance the anaerobic conversion of propionate to acetate. Biochem. Eng. J. 73, 80–85. doi: 10.1016/j.bej.2013.02.004
Romero-Güiza, M. S., Vila, J., Mata-Alvarez, J., Chimenos, J. M., and Astals, S. (2016). The role of additives on anaerobic digestion: a review. Renew. Sustain. Energy Rev. 58, 1486–1499. doi: 10.1016/j.rser.2015.12.094
Sawatdeenarunat, C., Surendra, K. C., Takara, D., Oechsner, H., and Khanal, S. K. (2015). Anaerobic digestion of lignocellulosic biomass: challenges and opportunities. Bioresource Technol. 178, 178–186. doi: 10.1016/j.biortech.2014.09.103
Wang, X., Li, Z., Bai, X., Zhou, X., Cheng, S., Gao, R., et al. (2018). Study on improving anaerobic co-digestion of cow manure and corn straw by fruit and vegetable waste: methane production and microbial community in CSTR process. Bioresource Technol. 249, 290–297. doi: 10.1016/j.biortech.2017.10.038
Wang, X., Li, Z., Zhou, X., Wang, Q., Wu, Y., Saino, M., et al. (2016). Study on the bio-methane yield and microbial community structure in enzyme enhanced anaerobic co-digestion of cow manure and corn straw. Bioresource Technol. 219, 150–157. doi: 10.1016/j.biortech.2016.07.116
Wei, J., Hao, X., van Loosdrecht, M. C. M., and Li, J. (2018). Feasibility analysis of anaerobic digestion of excess sludge enhanced by iron: a review. Renew. Sustain. Energy Rev. 89, 16–26. doi: 10.1016/j.rser.2018.02.042
Wu, D., Zheng, S., Ding, A., Sun, G., and Yang, M. (2015). Performance of a zero valent iron-based anaerobic system in swine wastewater treatment. J. Hazard. Mater. 286, 1–6. doi: 10.1016/j.jhazmat.2014.12.029
Wyman, V., Serrano, A., Fermoso, F. G., and Villa Gomez, D. K. (2019). Trace elements effect on hydrolytic stage towards biogas production of model lignocellulosic substrates. J. Environ. Manage. 234, 320–325. doi: 10.1016/j.jenvman.2019.01.015
Xu, R., Xu, S., Zhang, L., Florentino, A. P., Yang, Z., and Liu, Y. (2019). Impact of zero valent iron on blackwater anaerobic digestion. Bioresource Technol. 285, 121351. doi: 10.1016/j.biortech.2019.121351
Yang, L., Xu, F., Ge, X., and Li, Y. (2015). Challenges and strategies for solid-state anaerobic digestion of lignocellulosic biomass. Renew. Sustain. Energy Rev. 44, 824–834. doi: 10.1016/j.rser.2015.01.002
Yang, Y., Yang, F., Huang, W., Huang, W., Li, F., Lei, Z., et al. (2018). Enhanced anaerobic digestion of ammonia-rich swine manure by zero-valent iron: with special focus on the enhancement effect on hydrogenotrophic methanogenesis activity. Bioresource Technol. 270, 172–179. doi: 10.1016/j.biortech.2018.09.008
Yuan, T., Ko, J. H., Zhou, L., Gao, X., Liu, Y., Shi, X., et al. (2020). Iron oxide alleviates acids stress by facilitating syntrophic metabolism between Syntrophomonas and methanogens. Chemosphere 247:125866. doi: 10.1016/j.chemosphere.2020.125866
Zhang, H., Tian, Y., Wang, L., Mi, X., and Chai, Y. (2016). Effect of ferrous chloride on biogas production and enzymatic activities during anaerobic fermentation of cow dung and Phragmites straw. Biodegradation 27, 69–82. doi: 10.1007/s10532-016-9756-7
Zhang, H., Wang, L., Dai, Z., Zhang, R., Chen, C., and Liu, G. (2020). Effect of organic loading, feed-to-inoculum ratio, and pretreatment on the anaerobic digestion of tobacco stalks. Bioresource Technol. 298:122474. doi: 10.1016/j.biortech.2019.122474
Zhang, J., Wang, Z., Lu, T., Liu, J., Wang, Y., Shen, P., et al. (2019). Response and mechanisms of the performance and fate of antibiotic resistance genes to nano-magnetite during anaerobic digestion of swine manure. J. Hazard. Mater. 366, 192–201. doi: 10.1016/j.jhazmat.2018.11.106
Keywords: anaerobic digestion, methane, zero valent iron, cow manure, lignocellulose
Citation: Men Y, Zheng L, Zhang L, Li Z, Wang X, Zhou X, Cheng S and Bao W (2020) Effects of Adding Zero Valent Iron on the Anaerobic Digestion of Cow Manure and Lignocellulose. Front. Bioeng. Biotechnol. 8:590200. doi: 10.3389/fbioe.2020.590200
Received: 31 July 2020; Accepted: 25 September 2020;
Published: 28 October 2020.
Edited by:
Chunbao (Charles) Xu, University of Western Ontario, CanadaReviewed by:
Wenming Zhang, Nanjing Tech University, ChinaYejun Han, Institute of Process Engineering (CAS), China
Copyright © 2020 Men, Zheng, Zhang, Li, Wang, Zhou, Cheng and Bao. This is an open-access article distributed under the terms of the Creative Commons Attribution License (CC BY). The use, distribution or reproduction in other forums is permitted, provided the original author(s) and the copyright owner(s) are credited and that the original publication in this journal is cited, in accordance with accepted academic practice. No use, distribution or reproduction is permitted which does not comply with these terms.
*Correspondence: Lei Zheng, emhlbmdsQHVzdGIuZWR1LmNu; Zifu Li, emlmdWxpQHVzdGIuZWR1LmNu