The Research Advance of Cell Bridges in vitro
- 1College of Sericulture, Textile and Biomass Sciences, Southwest University, Chongqing, China
- 2State Key Laboratory of Silkworm Genome Biology, Southwest University, Chongqing, China
- 3Hunan Provincial Key Laboratory of Controllable Preparation and Functional Application of Fine Polymers, School of Chemistry and Chemical Engineering, Hunan University of Science and Technology, Xiangtan, China
The microenvironment in which cells reside in vivo dictates their biological and mechanical functioning is associated with morphogenetic and regenerative processes and may find implications in regenerative medicine and tissue engineering. The development of nano- and micro-fabricated technologies, three-dimensional (3D) printing technique, and biomimetic medical materials have enabled researchers to prepare novel advanced substrates mimicking the in vivo microenvironment. Most of the novel morphologies and behaviors of cells, including contact guidance and cell bridges which are observed in vivo but are not perceived in the traditional two-dimensional (2D) culture system, emerged on those novel substrates. Using cell bridges, cell can span over the surface of substrates to maintain mechanical stability and integrity of tissue, as observed in physiological processes, such as wound healing, regeneration and development. Compared to contact guidance, which has received increased attention and is investigated extensively, studies on cell bridges remain scarce. Therefore, in this mini-review, we have comprehensively summarized and classified different kinds of cell bridges formed on various substrates and highlighted possible biophysical mechanisms underlying cell bridge formation for their possible implication in the fields of tissue engineering and regenerative medicine.
Introduction
Cell–cell contact (Ilkka et al., 2018), bioactive factors (Jiali et al., 2019) and extra-cellular matrix (ECM) (Ramirez-San et al., 2017) provide biomechanical and biochemical cues that are crucial for cell phenotype and function. The interaction of extracellular matrix (ECM) with cells is predominantly mediated through focal adhesion (Ray et al., 2017; Yu et al., 2020). However, cells suspend easily in the absence of attachment to ECM due to the discontinuity of adhesion sites and the complexity and heterogeneity of ECM geometrical cues, which varies with the cell type and the Rac activation (Guillou et al., 2008). Particularly, cells have the capacity to sense and transduce the chemical and physical properties of ECM to their cytoskeletal structures to bridge across the non-adhesion areas and maintain the mechanical stability and integrity of tissue (Thery, 2010), as observed in wound healing (Vedula et al., 2015), regeneration (Goldshmit et al., 2012) and development (Whong, 1931).
Decades of research have indicates that the microenvironment of cells plays a crucial role in regulating its behaviors and function both in vivo and in vitro (Carrel and Burrows, 1911). Since the 1980s, the introduction of photolithographical techniques, initially developed for the microelectronic industry, into the cell culturing field has led the development of patterned substrates for cell analysis and culture (Curtis and Varde, 1964). The majority of cell types can adapt their adhesion, morphology, migration, cytoskeleton and/or genome according to the chemical and topographic patterns on artificial substrates mimicking the native ECM microenvironment (Ahmed and Ffrench-Constant, 2016; Ma et al., 2018). Notably, some novel cell morphologies which in part dictates the cell such as contact guidance (Nguyen et al., 2016; Ray et al., 2017) and cell bridges (Goldner et al., 2006; Zhang et al., 2015b), have been observed on the patterned substrates at micro and nano scale. While contact guidance has been extensively investigated in regeneration medicine and tissue engineering (Kamyar et al., 2018; Tonazzini et al., 2019), studies on cell bridges observed on chemical pattern substrates and some nano/micro topographical substrates remain scarce.
Although the traditional two-dimension (2D) culture system is extensively used as a valuable tool for cell-based studies, it exhibits several limitations (Liu et al., 2018), such as abnormal structural characteristics, mechanical constraints, not being able to accurately mimic the natural microenvironment where cells reside in tissues and interactions between cells. Thus, as an alternative to the technical limitations of the 2D culture system, a 3D culture system with the advantage of mimicking the microenvironment of natural ECM is preferred for regenerative medicine and tissue engineering research (Joyce et al., 2018; Lin et al., 2019). Besides, 3D scaffolds can provide a better representation of the natural tissue architecture, chemical composition, and mimic cell communication between the cell and microenvironment (Hassani et al., 2020; Hou et al., 2020). More recently, scaffold-based 3D assembly of cells has been applied and used as a supporting structure to guide cell adhesion, growth, differentiation, and arrangement in a manner analogous to developmental processes or tissue repair (Liu et al., 2018). Thus, 3D culture systems overcoming the obstacles arising from traditional 2D culture systems have emerged as pioneering methodology and extensively utilized for stem cell research, regenerative medicine studies, and tissue engineering (Yuan et al., 2018). On different types of 3D substrates, such as microspheres, porous scaffolds or 3D printing scaffolds (Jamal et al., 2010), various cell types span across different surfaces of scaffold and form cell bridges with single-cell or collective cells (Alvim Valente et al., 2018).
With the recent advancement of tissue engineering, cell biology, and biomaterials science, various substrates or scaffolds with more defined topographic (Zhao et al., 2018) and chemical (Na et al., 2017) pattern are prepared to mimic the natural cell niche in tissue in order to guide the cells behavior. Contact guidance (Zhang et al., 2015a; Tonazzini et al., 2019), cell adhesion (Vasiliki and Triantafyllos, 2018), differentiation (Yao et al., 2016; Zhang et al., 2019) and gene expression (Qiongfang et al., 2018) have been paid much attention and investigated extensively; however, cell bridges, a phenomenon as consistent as contact guidance remain to be completely elucidated. As an essential behavior of cells, cell bridges may play crucial role in organization of cells, migration and development. Therefore, particular attention should be paid to these cell bridges in cell biology, regenerative medicine and embryonic development. In this mini-review, we comprehensively summarized and classified different kinds of cell bridges formed on various substrates; besides, the process of formation, possible mechanism and cues of cell bridges will be outlined and highlighted. The effects of the formation of cell bridges on cell proliferation and/or differentiation will be reviewed. We will also discuss the need for investigations of cell bridges. This article will provide critical overview of cell bridges for their possible implications in the fields of tissue engineering, biology and regenerative medicine and will be helpful in expanding our understanding of cell behaviors.
Approaches of Cell Bridging
Cells can span across non-adhesion areas or concave and suspend their body in air irrespective of chemical pattern or topography both in vivo and in vitro. In this section, the approaches of cell bridging in vitro will be summarized, discussed, and classified.
Topography, as a critical factor of microenvironment, impose specific mechanical boundary conditions to cells, has engrossed increasing attentions over a few decades due to its influence on cell mechanics, function, polarity, and architecture (Abagnale et al., 2017). Various topographies with different morphology and size (from nanometer, sub-micrometer to micrometers) have been prepared to investigate the phenomenon and mechanism of cell behavior and function (Nguyen et al., 2016; Ribeiro et al., 2019). Several kinds of cells spreading and morphology have been reported on diverse topographic substrates and can be roughly classified into three patterns: (1) growing along the topography, as represented by Xenopus tissue on micropost arrays (Song et al., 2015) and neurons on ring-shaped nanopillar arrays (Xie et al., 2010); (2) engulfing the topography, as shown by neurons on gold-spine electrode (Hai et al., 2010); (3) spanning across topography, as depicted by primary hippocampal neurons on pillar arrays (Park et al., 2016a,b). Furthermore, cell bridges containing a single cell or multiple cells on groove substrates have been summarized and described by five strategies (Figures 1AI–V,B–V) depending on the interaction between cell/cell and topographic substrates, as illustrated in Figure 1A (sketch maps) and Figure 1B (scanning electron microscope photos) (Zhang et al., 2015b). In the first strategy as shown in Figures 1AI,BI, cells located at the bottom of grooves with other parts of their body adhering the two side walls. In the second strategy as shown in Figures 1AII,BII, cells suspended in air with their body adhering the bottom of grooves and one side wall. In other strategies, cells bridged across grooves with their body grasping the two side walls (Figures 1AIII,BIII), one side wall and the other side plateau (Figures 1AIV,BIV) or two side plateau (Figures 1AV,BV). Sometimes, cells combined together to bridge grooves as shown in Figure 1BVI. In this section, cell bridges constituting different kinds of cells, i.e., mesenchymal stem cells (MSCs), neurons, on a variety of topographic surfaces with different structure and biochemical compositions, will be classified according to the strategies shown in Figures 1A,B, and will be summarized as presented in Table 1. Typical cell bridges between two neighboring microspheres, two surfaces of a scaffold, and a cavity structure are shown in Figures 1C–E, individually (Jamal et al., 2010; Nikkhah et al., 2010; Cheng et al., 2014).
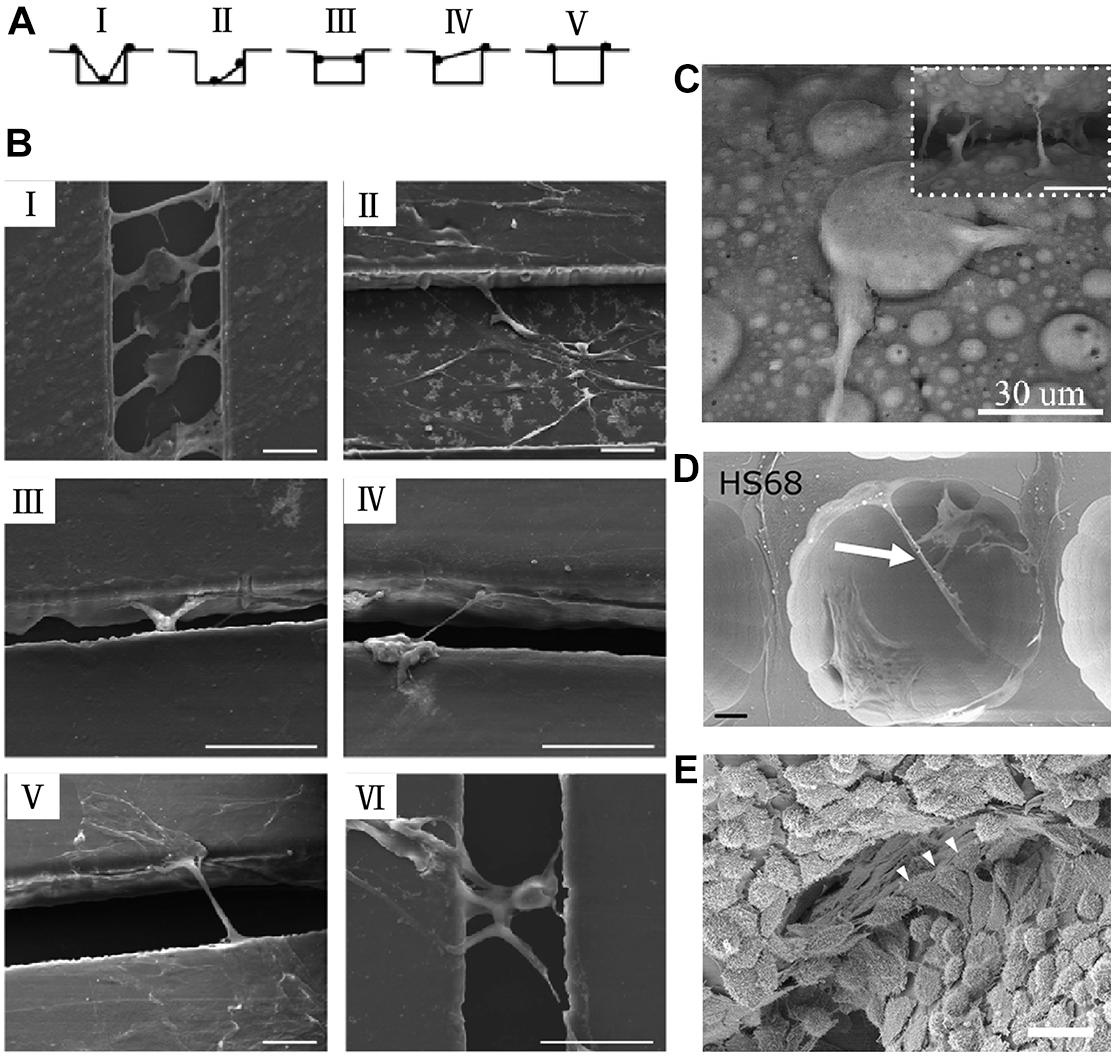
Figure 1. Various morphologies of cell bridges resulting from topographic cues. The sketch maps (A) and SEM photos (B) of various types of human mesenchymal stem cells (hMSCs) bridges observed on micro-grooved substrates. In panels (AI,BI), cells contacted with substrates at bottom and two side walls. In panels (AII,BII), cells adhered bottom and one side wall. In panels (AIII–V,BIII–V), cells suspended above grooves by climbing two side walls, one side wall and the other side plateau or two side plateau, respectively. Collective cells bridges crossing grooves as shown in panel (BVI). From Zhang et al. (2015b). Copyright 2015 RSC. (C) mouse mesenchymal stem cells (mMSCs) bridges spanning over two microspheres. From Cheng et al. (2014). Copyright 2014 RSC. (D) Fibroblasts bridges stretching inside the etched features. From Nikkhah et al. (2010). Copyright 2010 Elsevier. (E) Fibroblasts bridges across the gap between two neighboring rigid panels. From Jamal et al. (2010). Copyright 2010 Elsevier. Scale bars represent 50 μm in panel (B), 20 μm in panel (D), and 30 μm in panel (E).
The adhesion sites, mediating the attachment of cells to ECM, are not continuous in the microenvironment of cells, and its spatial distribution is predominantly dependent on the biochemical composition pattern of the microenvironment (chemical microenvironment), which is dictated by the location and orientation of ECM fibers and the positions of adjacent cells (Thery, 2010). Consistent with the topographic microenvironment, chemical cues also play a crucial role in the regulation of cellular differentiation, gene expression, and functions (Xing et al., 2019). Chemical micro-patterns are prepared to mimic the chemical microenvironment in vivo to accurately investigate the cell behavior and mechanism based on cell adhesions (Tu et al., 2017). On these artificial chemical micro-patterns, in addition to being confined to adhesion micro-areas, cells can also span across the non-adhesion area and grow into cell bridges identical to axon (Sorkin et al., 2006) and epithelium bridges (Vedula et al., 2014b) emerged during wound healing in vivo. Three key patterns have been identified for the formation of cell bridgs on chemical micropattern (Figure 2): (1) single-cell spreading on specific chemical micropattern with parts of cell body suspended on non-adhesion areas (Théry et al., 2010; Figure 2A); (2) multiple-cells spanning over a non-adhesion area while communicating each other (Vedula et al., 2014b; Figure 2C); (3) axon and dendrite spanning over non-adhesion areas without suspending cell bodies (Sorkin et al., 2006; Figure 2B). Cell bridges arising on chemical micropattern have been summarized in Table 2.
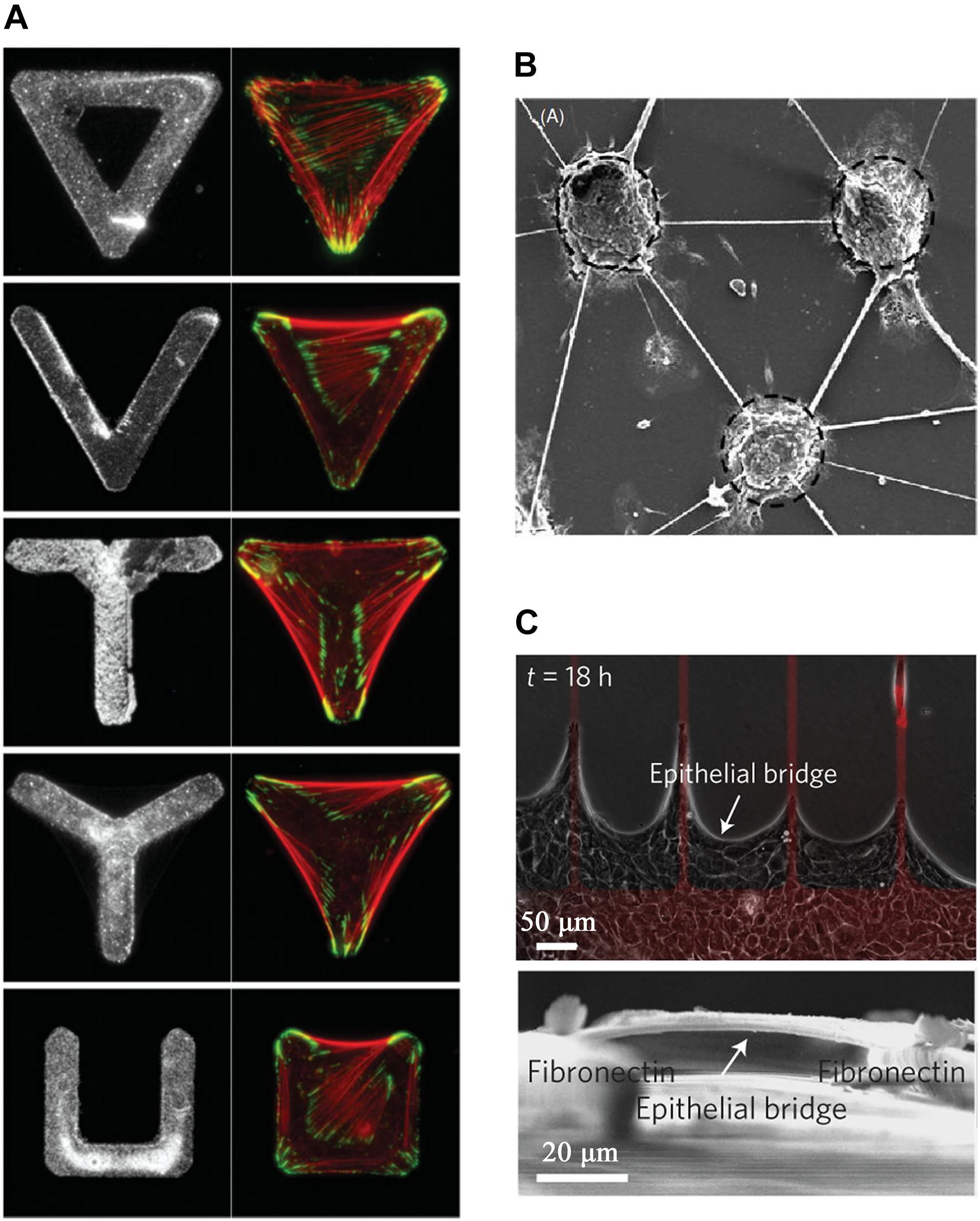
Figure 2. Various morphologies of cell bridges spanning non-adhesive areas on chemical micropatterns. (A) Single cell bridges were directed by various adhesive shapes (triangle, “V,” “T,” “Y,” and “⊔”) coated by homogeneous fibronectin for cell adhesion. The edge length of the triangle is 46 μm. From Théry et al. (2010). Copyright 2006 Wiley-Liss. (B) Axons and dendrites bridges connecting 100 μm adhesive islands on which neurons clusters adhered. From Sorkin et al. (2006). Copyright 2006 Institute of Physics Publishing. (C) Epithelial collective cells bridges suspending over non-adhesive areas with a part of cells adhesion on fibronectin strips. From Vedula et al. (2014b). Copyright 2013 Nature Publish Group.
The Key Cues for Initiation of Cell Bridges Formation and the Extremities of Cell Bridges
Cellular morphology and organization are crucial for migration and differentiation of cells and tissue microarchitecture. Therefore, it becomes imperative to identify cues that initiate the formation of some typical morphology and organization of cells within the tissue for implication in tissue regeneration, morphogenesis, and engineering. In this section, some key cues for initiation of cell bridges formation and the extremities of cell bridges will be summarized and discussed. All content will be presented in two parts according to cell bridges directed by topography or chemical patterns.
According to the papers published until now, three primary cues have been identified to affect the formation of cell bridges on topographic substrates: (1) the characteristics of cells, including size, source, age of donor; (2) the features of topographic substrates, including size, porous structure, roughness, and pattern; (3) the wettability of substrates. Noticeably, cells naturally suspend parts of their body and adhere to substrates with the other parts of their body when they are cultured on topographic substrates with spacing between pillars or ridges of smaller (such as nano or sub-micro meter) than cell size, because cells prevent bending themselves to adapt to the uneven of topography (Ohara and Buck, 1979). Of note, the mechanical stress exerted on cells increases with increasing pattern size (Sunami et al., 2014). In contrast, the persistence length of cell filopodia bridging perpendicular to the grooves decreases with the increasing age of donors (Sales et al., 2019). If the space between pillars or ridges is comparable to the size of cells, then cells may span between neighboring pillars or ridges or be confined to the bottom of pillars, or a single ridge or groove. Nevertheless, on the deeper and narrower groove, it is easier for cells to bridge neighboring ridges (Braber et al., 1996, 2010; Curtis and Wilkinson, 1997). Furthermore, the ratio of groove width to depth remains crucial for forming cell bridges, and hMSCs only bridge across neighboring ridges when encountering grooves with a ratio of width to depth of less than two within the range of testing (Zhang et al., 2015b). The largest distance for cells to bridge can vary with the cell type and substrate size [ranging from 50 μm (Stevenson and Donald, 2009) to 200 μm (Zhang et al., 2015b)]. Cell bridges spanning across large distance areas are usually composed of multi cells or cell collective. Furthermore, the formation of multicellular epithelial bridges across negative curvature grooves with dozens of micrometers width has been found to be associated with the ratio of the cosine and sine of cell–cell and cell-substrate contact angle and cell type (Broaders et al., 2015). Noticeably, cancer cells prevent bridging and tend to adapt to the curved surfaces of the chamber due to their easy distortion caused by impaired cytoskeleton compared to fibroblast cells (Nikkhah et al., 2010). Moreover, the wettability of the material surface may trigger the transformation from spreading on top of the pillars to spreading on the bottom. Cell bridges forms over pillars with 4.5 μm height and 5 μm spacing on the PDMS surface; however, spread on the bottom of the surface with the same pillars on poly(ethylene oxide)/poly(butyleneterephtalate; PEOT/PBT) and poly L-lactic acid (PLLA) surfaces (Papenburg et al., 2010).
Similar to the formation of cell bridges on topography substrates, there are major three factors that affect the formation of cell bridges on chemical micropattern: (1) the characteristics of chemical micropattern, including size, shape, and distance; (2) the characteristics of cells, such as cell type, the contact force between each other; (3) the initial sites of cell localization. In general, a single cell is able to bridge across the non-adhesion areas on various chemical micropatterns, which usually enable the cell to adhere to specific areas on substrates. In this condition, cells usually suspend parts of their body and adhere to substrates with the other parts of their body when they are cultured on “T”- or “V”-shaped adhesion micropatterns; however, they adhere to round or square adhesion micropatterns with their whole body and turn to be round or square shape accordingly. In addition, cells can form a multicellular bridge to span across a non-adhesion area with a considerable distance. The adhesion force between cells, the distance between adhesion strips, and the width of adhesion strips remains crucial for the building of these multicellular bridges (Vedula et al., 2014b). Moreover, the increasing adhesion strip width and the decreasing spacing between adhesion strips are both beneficial to the formation of multicellular bridges. The critical distance between adhesion strips at which multicellular bridges form is limited to 200 μm (Vedula et al., 2014b). According to a study of artificial neural networks reported by Hanein et al., cell density influences the formation of the bridges composed of dendrites and axons (Sorkin et al., 2006). In this system, the maximal spanning distance of a bridge was reported to be as long as 400 μm (Sorkin et al., 2006). In contrast, Lehnert et al. (2003) reported that cells could span 3∼4 adhesive sites on adhesive square islands substrates if they are initially located non-adherent areas between adhesive squares, but not bridge across non-adhesive gaps if they initially centered on adhesive squares (Dirk, 2004).
The Progress and Mechanism of Cell Bridges Formation
The characteristics of cells, such as cell membrane elasticity (Ohara and Buck, 1979), tensile forces from pulling cells (Vedula et al., 2014b), substantially affect the formation of cell bridges. Possible processes involved in the formation of cell bridges have been speculated by various investigators based on their results. These processes will be discussed separately, depending on the substrates (topography or chemical micropattern) on which cell bridges formed.
As the radical bending of the cell body is prevented by cell membrane elasticity or rigidity, so cells prefer bridging over the top of nanofeatures, neighboring ridges, or neighboring faces of a scaffold to adapt to the morphology of topographic substrates (Jie et al., 2014). Three different processes have been described to reveal the process of cell bridges formations (Figure 3). Jie et al. (2014) prepared a string of gratings to investigate the effect of the depth of micro-grating on the spreading of murine neural progenitor cells. Furthermore, to identify the relationship of neuronal elongation and alignment with grating depth, a quantitative model was established to explain the depth-sensing mechanism of the cells and predict bridging over the neighboring gratings (Figure 3A). Based on their hypothesis, when cells encounter a grating edge they first initiate filopodia to randomly probe the potential adhesion sites, then cells extend along with the gratings, adhere to the bottom of the groove, or bridge across the gap between neighboring gratings depending on the features of topography. Their results suggested that if the cytoskeleton contractility caused by the adhesion of filopodia to a substrate is strong enough to balance the microtubule bending resistance, cells will adhere to the bottom of the groove; if not strong enough, cells will favor extending along with gratings or bridging over grooves when they form long and sufficient number of horizontal filopodia. Different from this hypothesis, other researchers suggested the formation of cell bridges was driven by the higher site of cell which climbed along the groove walls to the adjacent plateaus (Goldner et al., 2006; Zhang et al., 2015b). Cells initially located at the bottom suspended themselves by the contractile force during their climbing along the wall to a higher site, as represented in Figure 3B(a). Previously, Gartner et al. reported that in epithelial Madin-Darby canine kidney (MDCK) cells, the majority of detachments occurred in the areas most significant concavity (center of channels and the convex ridges) (Broaders et al., 2015). According to their hypothesis (Figure 3C), the lateral contractile force generated by the surrounding tissue exhibited the potential to resist the adhesive forces centered at areas of greatest concavity areas raised the cells up.
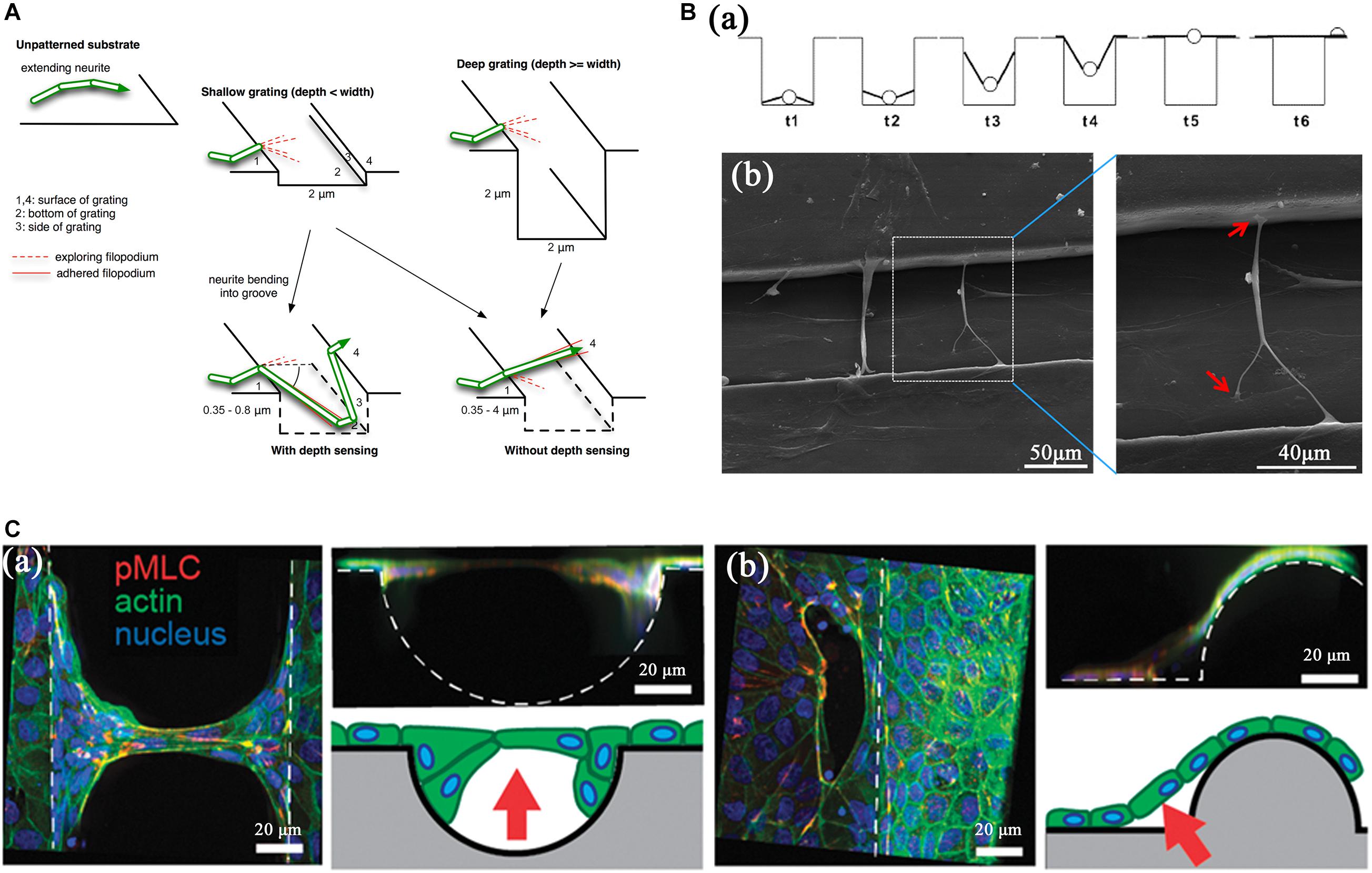
Figure 3. Various processes for the formation of cell bridges caused by topographic cues. (A) A schematic diagram of neurite bridges formation process with depth sensing, during which cells stretched themselves across the groove when depth ≥ width. From Jie et al. (2014). Copyright 2014 Elsevier. (B) Cells initially at the bottom, extended to the groove walls and bridged across two adjacent plateaus. Panel B(a) is a diagrammatic drawing of this process and panel B(b) is SEM photos about this strategy. Panel B(a) from Goldner et al. (2006). Copyright 2006 Elsevier. Panel B(b) from Zhang et al. (2015b). Copyright 2015 RSC. (C) Tissues raised from negative curvature regions by the contractility force of neighboring cells. Lifted tissue at channels and bridges were shown in panel C(a,b) individually. From Broaders et al. (2015). Copyright 2015 Oxford University Press.
Consistent with topographic substrates, the formation of cell bridges on chemical micropatterns was resulted from the migration and interaction of cells. Sorkin et al. (2006) demonstrated a patterned neural network consisting of cell clusters and non-adherent bundles between them. Cells after being seeded on adhesion micropattern substrates formed cell clusters; subsequently, the cell clusters migrated and anchored at specific positions, which triggered the formation of dendrites and axons bridges spanning non-adherent areas and an artificial neural network, as shown in Figure 4B. Furthermore, epithelial bridges were easily formed when cell ensemble migrated on heterogeneous substrates (Albert and Schwarz, 2016a). More recently, Ladoux et al. reported multicellular bridges to span across non-adherent areas when human keratinocytes were allowed to migrate along surfaces micropatterned with alternating strips of non-adherent polymer and fibronection, as shown in Figure 4A (Vedula et al., 2014b). They hypothesized that the tensile forces transferred from the traction of cells on the fibronectin strips drove the formation of epithelial bridges. For a single cell bridging on “V” or “T” shapes, the geometry of adhesive substrates forces cells to reorganize their internal cytoskeleton and bridge non-adhesion site, which is characterized by reinforcement of peripheral actin bundles and formation of RhoA-dependent stress fibers (James et al., 2010; Théry et al., 2010; Rossier et al., 2014).
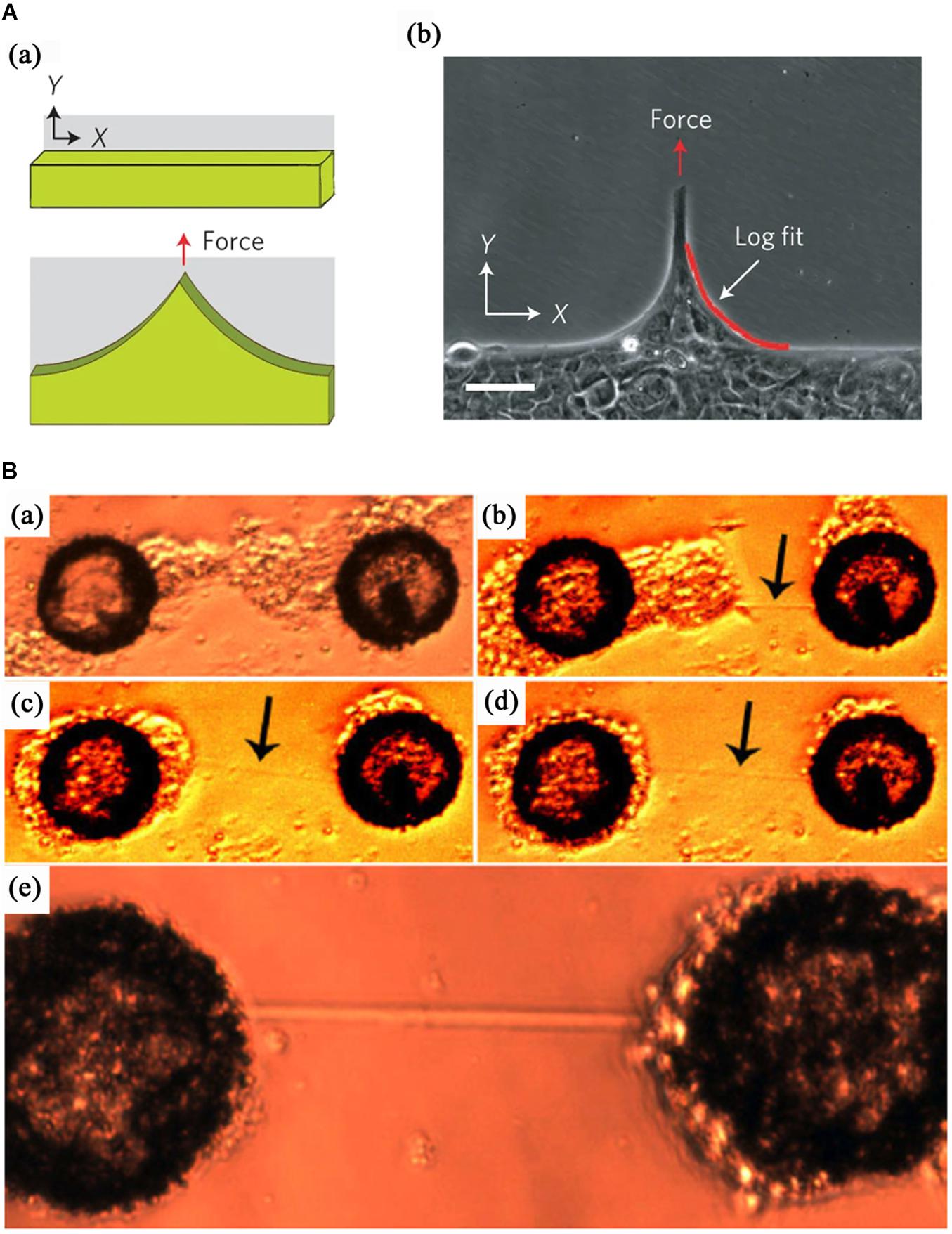
Figure 4. Two processes for the formation of cell bridges on chemical micropatterns. (A) The formation of multicellular bridges driven by the traction of cell migration along adhesive strips separated by non-adhesive regions. From Vedula et al. (2014b). Copyright 2013 Nature Publish Group. (B) Bridges composed of axons and dendrites resulting from the self-assembly of cells into clusters on separated adhesive islands. From Sorkin et al. (2006). Copyright 2006 Institute of Physics Publishing.
As mentioned earlier, some ambiguous hypotheses were raised to explain the process and mechanism of cell bridges formation, few of which consider and explain the underlying molecular and mechanotransduction mechanisms. In contrast, common theories, including polarization of F-actin due to mechanical restriction (Dunn and Heath, 1976), maximization of focal adhesion areas (Ohara and Buck, 1979), and actin polymerization resulting from discontinuities of substrates (Curtis and Clark, 1990), have also been proposed justify contact guidance. Recently, Deshpande and Bouten et al. presented further two theories of entropic forces (Buskermolen et al., 2019) and gap avoidance (Buskermolen et al., 2020) to explain the contact guidance. In the “gap avoidance” theory, they considered the contact guidance as the result of “the energetic penalty of cell adhesions on non-adhesive gaps” (Buskermolen et al., 2020). Conceivably, contact guidance and cell bridges emerged simultaneously in the same culture system comprising of topographic and micropattern substrates. The molecular and biophysical mechanisms underlying these theories of cell bridges may complement the hypotheses about contact guidance; and hence, can be referred for a molecular and mechanistic understanding.
The Crucial Factors Involved in the Maintenance of Cell Bridges
Actin edge-bundles are utilized in the cells’ maintenance and morphology (Zand and Albrecht-Buehler, 1989). Concave actin edge-bundles are constantly observed in suspended cell bridges. Various tissue- and cell-level variables are tightly coupled during the stabilization of cell bridges, such as geometry of ECM, inherent mechanical characteristics of cell monolayer, cross-talk between cell–cell and cell-ECM adhesions, and transmission and generation of force. For the stabilization of cell bridges on chemical micropatterns, Rossier et al. (2014) suggested that: (1) at the concave edges of single-cell bridges, the myosin-IIA triggers the assembly of actin filament at adhesion and (2) in the body of these bridges, myosin cross-links actin filaments and promote the healing of acto-myosin network when breaks occur. Furthermore, the actomyosin contractile force required in the bridged regions of cells spreading over non-adhesion areas involves the activation of the Rho-ROCK (Rho: a member of the Ras super family of low molecular weight GTPases; ROCK: Rho associated coiled coil forming protein kinase) pathway (Suffoletto et al., 2015). Conversely, myosin contractility is not necessary for the multicellular bridges, and the intermediate pool of E-cadherins is responsible for the stabilization of epithelial bridges on fibronectin micro-trips (Vedula et al., 2014b). Mitosis can also induce the destruction of epithelial bridges integrity in part (Vedula et al., 2014b). For multicellular bridges on topographic substrates with negative curvature, tissue contractility plays a crucial role in the detachment and formation of epithelial bridges, which involves ROCK, Rho GEFs (guanine nucleotide-exchange factors), the Ras (any of a family of genes that undergo mutation to oncogenes and especially to some commonly linked to human cancers)–Raf (a member of mitogen-activated protein kinase kinase kinase)–MEK (ERK kinase)–ERK (extracellular signal-regulated kinase) and Ras–PI3K (phosphatidylinositol-3- kinase) pathways (Broaders et al., 2015).
The Influence of Detachment on Cells
An adhesive substrate must be approved for the anchorage-dependent cell to support its growth. Cells have to span over non-adhesive gaps due to the heterogeneity of ECM and microenvironment. Various cell bridges on chemical micropattern and topographic substrates have been observed and investigated. The impact of detachment and suspended status on the behavior and fate of cells is crucial for embryonic development, tissue healing, and scaffold preparation. A single cell or multicellular bridges are held by adhesive forces and are subjected to enormous tensions, and the force have been measured by researchers through various approaches. In 2010, using a PDMS pillar device, Rossier et al. (2014) measured the force generated by single-cell bridges and reported the largest traction forces of 5∼20 nN/pillar located in the vicinity of the concave edges. Hua et al. also revealed higher tension in bridging portions of cells using fluorescence resonance energy transfer (FRET) (Suffoletto et al., 2015). Vedula et al. (2014b) demonstrated the homogeneous landscapes of both velocity and vorticity in keratinocytes sheets with pluricellular bridges and heterogeneous landscapes in MDCK cells sheet with multicellular bridges by using particle image velocimetry. The results reflected the differences in traction force fields of both cell sheets and suggested that the keratinocytes exhibited elastic-like behavior, and MDCK indicated fluid-like behavior. Simultaneously, the stiffness of hMSCs bridges was roughly measured and compared by atomic force microscopy (AFM), and there was no difference among cell bridges across grooves with different width and normal spreading cells (Zhang et al., 2015b). In contrast, the lateral direction of epithelial bridges showed higher modulus in a diverging channel (Hu et al., 2017). Furthermore, cells exhibit decreased spreading and proliferation when they span over nanogratings with a height of 560 nm or partially attached on the bottom compared with cells cultured on 150 nm height pillars; however, the influence of height can be alleviated by the increasing nanogratings space (Song et al., 2016). Furthermore, the bridging behavior did not trigger the expression of desmin, osteocalcin, alkaline phosphatase (ALP), and alizarin in hMSCs (Zhang et al., 2015b). Notably, the cell bridges are tightly coupled with cell migration (Albert and Schwarz, 2016b) and wound repair (Hu et al., 2017). Although chemical micropattern and topographic cues on substrates can regulate cell fate (Anselme et al., 2010; Jing et al., 2016) through stressing effect on focal adhesion (Abagnale et al., 2015), cell shape, cytoskeletal tension, and RhoA signaling (McBeath et al., 2004), there is a paucity of studies on the mechanism and effect of cell bridges phenomenon on properties of cells, such as stiffness, proliferation, and differentiation.
Conclusion and Outlook
Recent advances in the development of microtechnologies led to an increased understanding of cell bridges; however, similar to contact guidance, cell bridging as an optimum alignment of the cell should be investigated thoroughly. In our view, further aspects to consider includes the following: (1) a comprehensive investigation of cell bridges on topographic or chemical surface patterning should be performed to probe the effect of variable factors on cell bridges formations; (2) cell bridges and contact guidance usually co-exist, therefore, their interaction and synergies should be investigated carefully; (3) most of work on cell bridges are based on epithelial cells or keratinocytes, thus, cell bridges constitution in other cell types should be paid more attention, particularly stem cell on scaffolds, which is crucial for the regeneration medicine; (4) mechanistic studies of cell bridges at molecular-, subcellular-, cellular- and tissue-level should be performed using advanced techniques, such as traction force microscopy, particle image velocimtry (Vedula et al., 2014b), FRET (Suffoletto et al., 2015), complementary microscopic techniques (Fritzsche et al., 2017), second harmonic generation, and vibrational microscopy (Liu et al., 2017); (5) theoretical models should be adopted to describe and predict cell bridges, which will be helpful in understanding the role of cell bridges in cell–cell arrangement and organization, tissue regeneration, and shape problems in biological systems.
Author Contributions
The author confirms being the sole contributor of this work and has approved it for publication.
Funding
This work was financially supported by the National Natural Science Foundation of China (grant no. 51703196), Fundamental Research Funds for the Central Universities (grant no. SWU119057), and Open Research Fund of Hunan Provincial Key Laboratory of Controllable Preparation and Functional Application of Fine Polymers (grant no. E22028).
Conflict of Interest
The author declares that the research was conducted in the absence of any commercial or financial relationships that could be construed as a potential conflict of interest.
References
Abagnale, G., Sechi, A., Steger, M., Zhou, Q., Kuo, C.-C., Aydin, G., et al. (2017). Surface topography guides morphology and spatial patterning of induced pluripotent stem cell colonies. Stem. Cell Rep. 9, 654–666. doi: 10.1016/j.stemcr.2017.06.016
Abagnale, G., Steger, M., Nguyen, V. H., Hersch, N., Sechi, A., Joussen, S., et al. (2015). Surface topography enhances differentiation of mesenchymal stem cells towards osteogenic and adipogenic lineages. Biomaterials 61, 316–326. doi: 10.1016/j.biomaterials.2015.05.030
Ahmed, M., and Ffrench-Constant, C. (2016). Extracellular matrix regulation of stem cell behavior. Curr. Stem Cell Rep. 2, 197–206. doi: 10.1007/s40778-016-0056-2
Albert, P. J., and Schwarz, U. S. (2016a). Dynamics of cell ensembles on adhesive micropatterns: bridging the gap between single cell spreading and collective cell migration. PLoS Comput. Biol. 12:e1004863. doi: 10.1371/journal.pcbi.1004863
Albert, P. J., and Schwarz, U. S. (2016b). Optimizing micropattern geometries for cell shape and migration with genetic algorithms. Integr. Biol. 8, 741–750. doi: 10.1039/c6ib00061d
Albert, P. J., and Schwarz, U. S. (2016c). Modeling cell shape and dynamics on micropatterns. Cell Adh. Migr. 10, 516–528. doi: 10.1080/19336918.2016.1148864
Alvim Valente, C., Cesar Chagastelles, P., Fontana Nicoletti, N., Ramos Garcez, G., Sgarioni, B., Herrmann, F., et al. (2018). Design and optimization of biocompatible polycaprolactone/poly (l-lactic-co-glycolic acid) scaffolds with and without microgrooves for tissue engineering applications. J. Biomed. Mater. Res. Part A 6, 1522–1534. doi: 10.1002/jbm.a.36355
Anselme, K., Ploux, L., and Ponche, A. (2010). Cell/Material interfaces: influence of surface chemistry and surface topography on cell adhesion. J. Adhes. Technol. 24, 831–852. doi: 10.1163/016942409X12598231568186
Aufan, M. R., Sumi, Y., Kim, S., and Lee, J. Y. (2015). Facile synthesis of conductive polypyrrole wrinkle topographies on polydimethylsiloxane via a swelling–deswelling process and their potential uses in tissue engineering. ACS Appl. Mater. Interfaces 7, 23454–23463. doi: 10.1021/acsami.5b09355
Baranes, K., Hibsh, D., Cohen, S., Yamin, T., Efroni, S., Sharoni, A., et al. (2019). Comparing transcriptome profiles of neurons interfacing adjacent cells and nanopatterned substrates reveals fundamental neuronal interactions. Nano Lett. 19, 1451–1459. doi: 10.1021/acs.nanolett.8b03879
Braber, E. T. D., Ruijter, J. E. D., Ginsel, L. A., Recum, A. F. V., and Jansen, J. A. (1996). Quantitative analysis of fibroblast morphology on microgrooved surfaces with various groove and ridge dimensions. Biomaterials 17, 2037–2044. doi: 10.1016/0142-9612(96)00032-4
Braber, E. T. D., Ruijter, J. E. D., Smits, H. T. J., Ginsel, L. A., Recum, A. F. V., and Jansen, J. A. (2010). Effect of parallel surface microgrooves and surface energy on cell growth. J. Biomed. Mater. Res. 29, 511–518. doi: 10.1002/jbm.820290411
Broaders, K. E., Cerchiari, A. E., and Gartner, Z. J. (2015). Coupling between apical tension and basal adhesion allow epithelia to collectively sense and respond to substrate topography over long distances. Integr. Biol. 7, 1611–1621. doi: 10.1039/c5ib00240k
Buskermolen, A. B. C., Ristori, T., Mostert, D., van Turnhout, M. C., Shishvan, S. S., Loerakker, S., et al. (2020). Cellular contact guidance emerges from gap avoidance. Cell Rep. Phys. Sci. 1:100055. doi: 10.1016/j.xcrp.2020.100055
Buskermolen, A. B. C., Suresh, H., Shishvan, S. S., Vigliotti, A., and Deshpande, V. S. (2019). Entropic forces drive cellular contact guidance. Biophys. J. 116, 1994–2008. doi: 10.1016/j.bpj.2019.04.003
Carrel, A., and Burrows, M. T. (1911). Cultivation in vitro of malignant tumors. J. Exp. Med. 13, 571–575. doi: 10.1084/jem.13.5.571
Cheng, D., Cao, X., Gao, H., Ye, X., Li, W., and Wang, Y. (2014). Engineering PLGA doped PCL microspheres with a layered architecture and an island–sea topography. Rsc. Adv. 4, 9031–9038. doi: 10.1039/C3RA45274C
Curtis, A., and Wilkinson, C. (1997). Topographical control of cells. Biomaterials 18, 1573–1583. doi: 10.1016/s0142-9612(97)00144-0
Curtis, A. S., and Varde, M. (1964). Control of cell behaviror: topological factors. J. Natl. cancer Inst. 33, 15–26. doi: 10.1016/0014-4827(64)90035-7
Curtis, A. S. G., and Clark, P. (1990). The effects of topographic and mechanical-properties of materials on cell behavior. Crit. Rev. Biocomp. 5, 343–362. doi: 10.1016/0010-4825(90)90026-L
D. Lehnert et al. D., L. (2004). Cell behaviour on micropatterned substrata: limits of extracellular matrix geometry for spreading and adhesion. J. Cell Sci. 117, 41–52. doi: 10.1242/jcs.00836
Dunn, G. A., and Heath, J. P. (1976). A new hypothesis of contact guidance in tissue cells. Exp. Cell Res. 101, 1–14. doi: 10.1016/0014-4827(76)90405-5
Fritzsche, M., Li, D., Colin-York, H., Chang, V. T., Moeendarbary, E., Felce, J. H., et al. (2017). Self-organizing actin patterns shape membrane architecture but not cell mechanics. Nat. Commun. 8:14347. doi: 10.1038/ncomms14347
Ghassemi, S., Meacci, G., Liu, S., Gondarenko, A. A., Mathur, A., Roca-Cusachs, P., et al. (2012). Cells test substrate rigidity by local contractions on submicrometer pillars. Proc. Natl. Acad. Sci. U.S.A. 109, 5328–5332. doi: 10.1073/pnas.1119886109
Ghibaudo, M., Trichet, L., Le Digabel, J., Richert, A., Hersen, P., and Ladoux, B. (2009). Substrate topography induces a crossover from 2D to 3D behavior in fibroblast migration. Biophys. J. 97, 357–368. doi: 10.1016/j.bpj.2009.04.024
Goldner, J. S., Bruder, J. M., Li, G., Gazzola, D., and Hoffman-Kim, D. (2006). Neurite bridging across micropatterned grooves. Biomaterials 27, 460–472. doi: 10.1016/j.biomaterials.2005.06.035
Goldshmit, Y., Tang, J. K. K. Y., Siegel, A. L., Nguyen, P. D., Kaslin, J., Currie, P. D., et al. (2012). Fgf-dependent glial cell bridges facilitate spinal cord regeneration in Zebrafish. J. Neurosci. 32, 7477–7492. doi: 10.1523/JNEUROSCI.0758-12.2012
Guillou, H., Depraz-Depland, A., Planus, E., Vianay, B., Chaussy, J., Grichine, A., et al. (2008). Lamellipodia nucleation by filopodia depends on integrin occupancy and downstream Rac1 signaling. Exp. Cell Res. 314, 478–488. doi: 10.1016/j.yexcr.2007.10.026
Hai, A., Shappir, J., and Spira, M. E. (2010). In-cell recordings by extracellular microelectrodes. Nat. Methods 7, 200–202. doi: 10.1038/nmeth.1420
Hassani, F. A., Shi, Q., Wen, F., He, T., Haroun, A., Yang, Y., et al. (2020). Smart materials for smart healthcare moving from sensors and actuators to self-sustained nanoenergy nanosystems. Smart Mater. Med. 1, 92–124. doi: 10.1016/j.smaim.2020.07.005
Hou, Y., Deng, X., and Xie, C. (2020). Biomaterial surface modification for underwater adhesion. Smart Mater. Med. 1, 77–91. doi: 10.1016/j.smaim.2020.07.003
Hu, B., Leow, W. R., Amini, S., Nai, B., Zhang, X., Liu, Z., et al. (2017). Orientational coupling locally orchestrates a cell migration pattern for re-epithelialization. Adv. Mater. 29:1700145. doi: 10.1002/adma.201700145
Ilkka, P., LoC, S., Minkyoung, L., Lagendijk, A. K., Daniel, H., Cora, W., et al. (2018). Junction-based lamellipodia drive endothelial cell rearrangements in vivo via a VE-cadherin-F-actin based oscillatory cell-cell interaction. Nat. Commun. 9:3545. doi: 10.1038/s41467-018-05851-9
Jamal, M., Bassik, N., Cho, J.-H., Randall, C. L., and Gracias, D. H. (2010). Directed growth of fibroblasts into three dimensional micropatterned geometries via self-assembling scaffolds. Biomaterials 31, 1683–1890. doi: 10.1016/j.biomaterials.2009.11.056
James, J., Goluch, E. D., Hu, H., Liu, C., and Mrksich, M. (2010). Subcellular curvature at the perimeter of micropatterned cells influences lamellipodial distribution and cell polarity. Cell Motil Cytoskeleton 65, 841–852. doi: 10.1002/cm.20305
Jiali, T., Zhang, M., Hai, Z., Wu, C., Lin, J., Kuang, W., et al. (2019). Sustained release of two bioactive factors from supramolecular hydrogel promotes periodontal bone regeneration. ACS Nano 13, 5616–5622. doi: 10.1021/acsnano.9b00788
Jie, C. S., Chng, C. P., Moe, A. A., Tann, J. Y., Goh, E. L., Chiam, K. H., et al. (2014). Extending neurites sense the depth of the underlying topography during neuronal differentiation and contact guidance. Biomaterials 35, 7750–7761. doi: 10.1016/j.biomaterials.2014.06.008
Jing, H., Mei, T., and Cheng-Gee, K. (2016). The regulation of cellular responses to mechanical cues by Rho GTPases. Cells 5:17. doi: 10.3390/cells5020017
Joyce, C. H., Paula, M., and Shuler, M. L. (2018). A pumpless body-on-a-chip model using a primary culture of human intestinal cells and a 3D culture of liver cells. Lab on a Chip 18, 2036–2046. doi: 10.1039/c8lc00111a
Kamyar, E. P., Edgar, C. D. L. H., Cohen, A. R., and Bojana, G. (2018). Contact guidance is cell cycle-dependent. APL Bioeng 2:031904. doi: 10.1063/1.5026419
Kim, H. N., Jang, K. J., Shin, J. Y., Kang, D., Kim, S. M., Koh, I., et al. (2017). Artificial slanted nanocilia array as a mechanotransducer for controlling cell polarity. ACS Nano 11, 730–741. doi: 10.1021/acsnano.6b07134
Klein, F., Striebel, T., Fischer, J., Jiang, Z., Franz, C. M., Freymann, G. V., et al. (2010). Elastic fully three-dimensional microstructure scaffolds for cell force measurements. Adv. Mater. 22, 868–871. doi: 10.1002/adma.200902515
Lauria, I., Kramer, M., Schröder, T., Kant, S., Hausmann, A., Ke, F. B., et al. (2016). Inkjet printed periodical micropatterns made of inert alumina ceramics induce contact guidance and stimulate osteogenic differentiation of mesenchymal stromal cells. Acta Biomater. 44, 85–96. doi: 10.1016/j.actbio.2016.08.004
Lin, S., Yang, G., Jiang, F., Zhou, M., Yin, S., Tang, Y., et al. (2019). A magnesium enriched 3D culture system that mimics the bone development microenvironment for vascularized bone regeneration. Adv. Sci. 6:1900209. doi: 10.1002/advs.201900209
Liu, A. P., Ovijit, C., and Parekh, S. H. (2017). New advances in probing cell–extracellular matrix interactions. Integr. Biol. 9, 383–405. doi: 10.1039/c6ib00251j
Liu, Z., Tang, M., Zhao, J., Chai, R., and Kang, J. (2018). Looking into the future: toward advanced 3D biomaterials for stem-cell-based regenerative medicine. Adv. Mater. 30:e1705388. doi: 10.1002/adma.201705388
Ma, Y., Lin, M., Huang, G., Li, Y., Wang, S., Bai, G., et al. (2018). 3D spatiotemporal mechanical microenvironment: a hydrogel-based platform for guiding stem cell fate. Adv. Mater. 30:1705911. doi: 10.1002/adma.201705911
Park, M., Oh, E., Seo, J., Kim, M. H., Cho, H., Choi, J. Y., et al. (2016). Control over neurite directionality and neurite elongation on anisotropic micropillar arrays. Small 12, 1148–1152. doi: 10.1002/smll.201501896
McBeath, R., Pirone, D. M., Nelson, C. M., Bhadriraju, K., and Chen, C. S. (2004). Cell shape, cytoskeletal tension, and RhoA regulate stem cell lineage commitment. Dev. Cell 6, 483–495. doi: 10.1016/s1534-5807(04)00075-9
Na, J., Heo, J. S., Han, M., Lim, H., Kim, H. O., and Kim, E. (2017). Harvesting of living cell sheets by the dynamic generation of diffractive photothermal pattern on PEDOT. Adv. Funct. Mater. 27:1604260. doi: 10.1002/adfm.201604260
Nguyen, A. T., Sathe, S. R., and Yim, E. K. F. (2016). From nano to micro: topographical scale and its impact on cell adhesion, morphology and contact guidance. J. Phys. Condens Matter. 28:183001. doi: 10.1088/0953-8984/28/18/183001
Nier, V., Deforet, M., Duclos, G., Yevick, H. G., Cochet-Escartin, O., Marcq, P., et al. (2015). Tissue fusion over nonadhering surfaces. Proc. Natl. Acad. Sci. U.S.A. 112, 9546–9551. doi: 10.1073/pnas.1501278112
Nikkhah, M., Strobl, J. S., Vita, R. D., and Agah, M. (2010). The cytoskeletal organization of breast carcinoma and fibroblast cells inside three dimensional (3-D) isotropic silicon microstructures. Biomaterials 31, 4552–4561. doi: 10.1016/j.biomaterials.2010.02.034
Ohara, P. T., and Buck, R. C. (1979). Contact guidance in vitro. A light, transmission, and scanning electron microscopic study. Exp. Cell Res. 121, 235–249. doi: 10.1016/0014-4827(79)90002-8
Papenburg, B. J., Rodrigues, E. D., Wessling, M., and Stamatialis, D. (2010). Insights into the role of material surface topography and wettability on cell-material interactions. Soft. Matter. 6, 4377–4388. doi: 10.1039/B927207K
Park, M., Oh, E., Seo, J., Kim, M. H., Cho, H., Choi, J. Y., et al. (2016a). Control over neurite directionality and neurite elongation on anisotropic micropillar arrays. Small 12, 1148–1152. doi: 10.1002/smll.201501896
Park, M., Oh, E., Seo, J., Kim, M.-H., Cho, H., Choi, J. Y., et al. (2016b). Neurites: control over neurite directionality and neurite elongation on anisotropic micropillar arrays. Small 12:1147. doi: 10.1002/smll.201670044
Qiongfang, L., Bo, Z., Naresh, K., Jinmin, M., Aidong, Y., Zhanfeng, C., et al. (2018). Differential and interactive effects of substrate topography and chemistry on human mesenchymal stem cell gene expression. Int. J. Mol. Sci. 19:2344. doi: 10.3390/ijms19082344
Ramirez-San, G. R., Juan, P., Oakes, W., and Gardel, M. L. (2017). Contact guidance requires spatial control of leading-edge protrusion. Mol. Biol. Cell 28, 1043–1053. doi: 10.1091/mbc.e16-11-0769
Ray, A., Lee, O., Win, Z., Edwards, R. M., Alford, P. W., Kim, D. H., et al. (2017). Anisotropic forces from spatially constrained focal adhesions mediate contact guidance directed cell migration. Nat. Commun. 8:14923. doi: 10.1038/ncomms14923
Ribeiro, V., Pina, S., Costa, J. B., Cengiz, I. F., García-Fernández, L., Fernández-Gutiérrez, M. D. M., et al. (2019). Enzymatically cross-linked silk fibroin-based hierarchical scaffolds for osteochondral regeneration. ACS Appl. Mater. Interfaces 11, 3781–3799. doi: 10.1021/acsami.8b21259
Rossier, O. M., Gauthier, N., Biais, N., Vonnegut, W., and Sheetz, M. P. (2014). Force generated by actomyosin contraction builds bridges between adhesive contacts. EMBO J. 29, 1055–1068. doi: 10.1038/emboj.2010.2
Rother, J., Buchsenschutz-Gobeler, M., Noding, H., Steltenkamp, S., Samwer, K., and Janshoff, A. (2015). Cytoskeleton remodelling of confluent epithelial cells cultured on porous substrates. J. R. Soc. Interface 12:20141057. doi: 10.1098/rsif.2014.1057
Sales, A., Picart, C., and Kemkemer, R. (2019). Age-dependent migratory behavior of human endothelial cells revealed by substrate microtopography. Exp. Cell Res. 374, 1–11. doi: 10.1016/j.yexcr.2018.10.008
Song, J., Shawky, J. H., Kim, Y. T., Hazar, M., Leduc, P. R., Sitti, M., et al. (2015). Controlled surface topography regulates collective 3D migration by epithelial–mesenchymal composite embryonic tissues. Biomaterials 58, 1–9. doi: 10.1016/j.biomaterials.2015.04.021
Song, L., Wang, K., Li, Y., and Yang, Y. (2016). Nanotopography promoted neuronal differentiation of human induced pluripotent stem cells. Colloids Surfaces B Biointerfaces. 148, 49–58. doi: 10.1016/j.colsurfb.2016.08.041
Sorkin, R., Gabay, T., Blinder, P., Baranes, D., Ben-Jacob, E., and Hanein, Y. (2006). Compact self-wiring in cultured neural networks. J. Neural Eng. 3, 95–101. doi: 10.1088/1741-2560/3/2/003
Stevenson, P. M., and Donald, A. M. (2009). Identification of three regimes of behavior for cell attachment on topographically patterned substrates. Langmuir 25, 367–376. doi: 10.1021/la802859v
Suffoletto, K., Ye, N., Meng, F., Verma, D., and Hua, S. Z. (2015). Intracellular forces during guided cell growth on micropatterns using FRET measurement. J. Biomech. 48, 627–635. doi: 10.1016/j.jbiomech.2014.12.051
Sunami, H., Yokota, I., and Igarashi, Y. (2014). Influence of the pattern size of micropatterned scaffolds on cell morphology, proliferation, migration and F-actin expression. Biomater. Sci. 2, 399–409. doi: 10.1039/C3BM60237K
Teixeira, A. I., Mckie, G. A., Foley, J. D., Bertics, P. J., Nealey, P. F., and Murphy, C. J. (2006). The effect of environmental factors on the response of human corneal epithelial cells to nanoscale substrate topography. Biomaterials 27, 3945–3964. doi: 10.1016/j.biomaterials.2006.01.044
Thery, M. (2010). Micropatterning as a tool to decipher cell morphogenesis and functions. J. Cell Sci. 123(Pt 24), 4201–4213. doi: 10.1242/jcs.075150
Théry, M., Pépin, A., Dressaire, E., Chen, Y., and Bornens, M. (2010). Cell distribution of stress fibres in response to the geometry of the adhesive environment. Cell Motil Cytoskeleton 63, 341–355. doi: 10.1002/cm.20126
Tonazzini, I., Woerden, G. M. V., Masciullo, C., Mientjes, E. J., and Cecchini, M. (2019). The role of ubiquitin ligase E3A in polarized contact guidance and rescue strategies in UBE3A-deficient hippocampal neurons. Mol.Autism 10:202. doi: 10.1186/s13229-019-0293-1
Tu, Y., Nakamoto, H., Ichii, T., Utsunomiya, T., Khatri, O. P., and Sugimura, H. (2017). Fabrication of reduced graphene oxide micro patterns by vacuum-ultraviolet irradiation: from chemical and structural evolution to improving patterning precision by light collimation. Carbon 119, 82–90. doi: 10.1016/j.carbon.2017.04.008
Vasiliki, G., and Triantafyllos, S. (2018). Cell adhesion and matrix stiffness: coordinating cancer cell invasion and metastasis. Front. Oncol. 8:145. doi: 10.3389/fonc.2018.00145
Vedula, S. R. K., Hirata, K., Hn, Nai, Brugués, A., Toyama, Y., and Trepat, X. (2014a). Epithelial bridges maintain tissue integrity during collective cell migration. Nat. Mater. 13, 87–96. doi: 10.1038/nmat3814
Vedula, S. R. K., Hirata, H., Nai, M. H., Brugues, A., Toyama, Y., Trepat, X., et al. (2014b). Epithelial bridges maintain tissue integrity during collective cell migration. Nat. Mater. 13, 87–96.
Vedula, S. R. K., Peyret, G., Cheddadi, I., Chen, T., and Ladoux, B. (2015). Mechanics of epithelial closure over non-adherent environments. Nat. Commun. 6:6111. doi: 10.1038/ncomms7111
Webb, A. V., Clark, P., Skepper, J., Compston, A., and Wood, A. T. (1995). Guidance of oligodendrocytes and their progenitors by substratum. J. Cell Ence. 108(Pt 8), 2747–2760. doi: 10.1083/jcb.130.4.1005
Whong, S. H. (1931). On the formation of cell bridges in the development of the egg of dendraster eccentricus. Protoplasma 12, 123–128. doi: 10.1007/BF01618704
Xie, C., Hanson, L., Xie, W., Lin, Z., Cui, B., and Cui, Y. (2010). Noninvasive neuron pinning with nanopillar arrays. Nano Lett. 10, 4020–4024. doi: 10.1021/nl101950x
Xing, F., Li, L., Zhou, C., Long, C., and Zhang, X. (2019). Regulation and directing stem cell fate by tissue engineering functional microenvironments: scaffold physical and chemical cues. Stem Cells Int. 2019, 1–16. doi: 10.1155/2019/2180925
Yang, J., Liu, A., and Zhou, C. (2011). Proliferation of mesenchymal stem cell on chitosan films associated with convex micro-topography. J. Biomater. Sci. Polym. Ed. 22, 919–929. doi: 10.1163/092050610X496396
Yao, Q., Cosme, J. G. L., Xu, T., Miszuk, J. M., Picciani, P. H. S., Fong, H., et al. (2016). Three dimensional electrospun PCL/PLA blend nanofibrous scaffolds with significantly improved stem cells osteogenic differentiation and cranial bone formation. Biomaterials 115, 115–127. doi: 10.1016/j.biomaterials.2016.11.018
Yu, L., Hou, Y., Xie, W., Camacho, J. L. C., Cheng, C., and Holle, A. (2020). Ligand diffusion enables force-independent cell adhesion via activating α5β1 integrin and initiating Rac and RhoA signaling. Adv. Mater. 32:2002566.
Yuan, H., Xing, K., and Hsu, H. Y. (2018). Trinity of three-dimensional (3D) scaffold, vibration, and 3D printing on cell culture application: a systematic review and indicating future direction. Bioengineering 5:57. doi: 10.3390/bioengineering5030057
Zand, M. S., and Albrecht-Buehler, G. (1989). What structures, besides adhesions, prevent spread cells from rounding up Cell Motility 13, 195–211. doi: 10.1002/cm.970130307
Zhang, Q., Dong, H., Li, Y., Zhu, Y., Zeng, L., Gao, H., et al. (2015a). Microgrooved polymer substrates promote collective cell migration to accelerate fracture healing in an in vitro model. ACS Appl. Mater. Interfaces. 7, 23336–23345. doi: 10.1021/acsami.5b07976
Zhang, Q., Li, Y. L., Sun, H., Zeng, L., Li, X., Yuan, B., et al. (2015b). hMSCs bridging across micro-patterned grooves. RSC Adv. 5, 47975–47982. doi: 10.1039/C5RA06414G
Zhang, Q., Lu, M., Zheng, S., Wang, Y., Feng, M., Duan, B., et al. (2019). Air-plasma treatment promotes bone-like nano-hydroxylapatite formation on protein films for enhanced in vivo osteogenesis. Biomater. Sci. 7, 2326–2334. doi: 10.1039/c9bm00020h
Keywords: regeneration medicine, chemical pattern, cell bridges, topography, tissue engineering
Citation: Zhang Q (2020) The Research Advance of Cell Bridges in vitro. Front. Bioeng. Biotechnol. 8:609317. doi: 10.3389/fbioe.2020.609317
Received: 23 September 2020; Accepted: 02 November 2020;
Published: 24 November 2020.
Edited by:
Qiang Wei, Sichuan University, ChinaReviewed by:
Hua Dong, South China University of Technology, ChinaWenqian Feng, Max Planck Institute for Medical Research, Germany
Copyright © 2020 Zhang. This is an open-access article distributed under the terms of the Creative Commons Attribution License (CC BY). The use, distribution or reproduction in other forums is permitted, provided the original author(s) and the copyright owner(s) are credited and that the original publication in this journal is cited, in accordance with accepted academic practice. No use, distribution or reproduction is permitted which does not comply with these terms.
*Correspondence: Qing Zhang, zhq20190810@swu.edu.cn; zhq0508@126.com