- 1Department of Chemical Engineering and Applied Chemistry, University of Toronto, Toronto, ON, Canada
- 2Institute of Biomedical Engineering, University of Toronto, Toronto, ON, Canada
Microbial production of chemicals using renewable feedstocks such as glucose has emerged as a green alternative to conventional chemical production processes that rely primarily on petroleum-based feedstocks. The carbon footprint of such processes can further be reduced by using engineered cells that harness solar energy to consume feedstocks traditionally considered to be wastes as their carbon sources. Photosynthetic bacteria utilize sophisticated photosystems to capture the energy from photons to generate reduction potential with such rapidity and abundance that cells often cannot use it fast enough and much of it is lost as heat and light. Engineering photosynthetic organisms could enable us to take advantage of this energy surplus by redirecting it toward the synthesis of commercially important products such as biofuels, bioplastics, commodity chemicals, and terpenoids. In this work, we review photosynthetic pathways in aerobic and anaerobic bacteria to better understand how these organisms have naturally evolved to harness solar energy. We also discuss more recent attempts at engineering both the photosystems and downstream reactions that transfer reducing power to improve target chemical production. Further, we discuss different methods for the optimization of photosynthetic bioprocess including the immobilization of cells and the optimization of light delivery. We anticipate this review will serve as an important resource for future efforts to engineer and harness photosynthetic bacteria for chemical production.
Introduction
Societal dependence on fossil fuels is extensive and commonplace. This can be inferred from their widespread use in diverse applications including power, transportation, heating, and in the manufacture of plastics and beauty products. In an analysis using 2013 data, it was estimated that over 800 megatons of the chemical products we use annually are produced using petrochemical feedstocks (Levi and Cullen, 2018). Considering fossil fuels as a resource are finite and its extraction and use is a known contributor to the accumulation of greenhouse gases, finding viable alternative energy sources and chemical feedstocks is a challenge facing this generation.
Solar energy, as the most abundant energy source available on Earth (Blankenship et al., 2011), usually elicits images of large expanses of buzzing, black panels of photovoltaic cells which convert the energy from solar rays into electricity. However, the world’s oldest photovoltaic cells evolved within living organisms— the photosystems of photosynthetic bacteria capture photons to provide themselves the energy required for survival. Harnessing their ability to harvest solar energy and using it to produce the chemicals humans use daily could turn these bacteria into solar-powered microbial cell factories. If chemicals could be produced through this method at high enough yields and rates to be economically viable, a new era of consumerism could be ushered in— one that is both carbon-neutral and completely renewable.
Finding new pathways in photosynthetic organisms to convert substrates to valuable products is an area that has garnered a great deal of interest in recent years (Scholes et al., 2011; Bensaid et al., 2012; Carroll et al., 2018; Knoot et al., 2018; Hitchcock et al., 2020; Tiang et al., 2020). While processes using alternative substrates are also being developed in non-photosynthetic microbes, the most widely used carbon feedstock industrially is glucose from molasses or starch (Wendisch et al., 2016). Feedstock costs constitute most of the operating costs of a biological process and, on a large scale, this can be prohibitive unless the product value is high (Connelly et al., 2015). Alternatively, anthropogenic CO2 or wastewater streams could be used as the feedstock to synthesize chemicals using photosynthetic organisms. Considering large companies pay for the wastewater treatment of their processes and for the offset of their carbon emissions, using them as inputs to bioprocesses could have the potential to be both economically and ecologically lucrative in that they would both treat waste and output a product.
Typically, “photosynthesis” refers to oxygenic photosynthesis: the process performed by plants, algae, and cyanobacteria that generates oxygen through water splitting. This process is credited to having caused the oxygenation of Earth that made multicellular life possible over 500 million years ago (Madigan et al., 2010a) and continues to do so to this day. However, this process is theorized to have evolved 3.5 billion years ago from the lesser-known anoxygenic photosynthesis, a form that does not produce oxygen (Blankenship et al., 2007; Madigan et al., 2010a; Allen and Williams, 2011). Cyanobacteria are the only bacteria that perform oxygenic photosynthesis and they are an appealing chassis for biotechnological applications due to their genetic tractability particularly when compared to organisms like plants and algae (Jensen and Leister, 2014; Knoot et al., 2018; Hitchcock et al., 2020). Purple non-sulfur bacteria (PNSB) perform anoxygenic photosynthesis and are attractive for engineering because of their anaerobicity, metabolic versatility, and ability to naturally produce hydrogen (Tiang et al., 2020). Also, because they have been used as a model for the study of photosynthesis, their photosystems are generally well-understood (Yang et al., 2017; Fixen et al., 2019).
This review will look at both oxygenic and anoxygenic photosynthesis focusing on cyanobacteria and PNSB and consider how the different photosynthetic systems are being used and engineered both on a genetic level and a bioprocess level to overproduce native chemicals or to divert electrons toward heterologous products. When developing a bioprocess, an engineer must take a holistic approach: from organism selection based on biological strengths, to considerations of internal cellular processes, and finally to the design of the overall process. The electron transport chains of oxygenic and anoxygenic photosynthesis are similar but have several distinctions that would serve different applications. The complexes that drive photosynthesis can be engineered for increased electron flux to downstream cellular processes such as for the production of chemicals through the introduction of heterologous pathways. Additionally, external factors such as photobioreactor design can affect production as cells react to their environment and, as such, is a significant consideration. This review will examine each of these areas with the overarching goal of bioprocess design for solar-powered cell factories.
A Comparison of Oxygenic and Anoxygenic Photosynthesis
From a high-level perspective, photosystems can be likened to photovoltaic cells: solar energy packets known as quanta (Madigan et al., 2010b) are converted into electrical energy within photosystems, transferred along the electron transport chain (ETC), and ultimately stored in chemical bonds in energy carriers like ATP and NADH or NADPH for later use. The photosystem consists of light-harvesting complexes arranged around a reaction center similar in appearance to petals around a sunflower and in function to an antenna (Figure 1A). The reaction center is where solar energy is used to excite an electron and initiate the action of the ETC, but the majority of energy needed to do this is transferred from the antenna, which increases the cross-section for light absorption by approximately 100 times (Scholes et al., 2011).
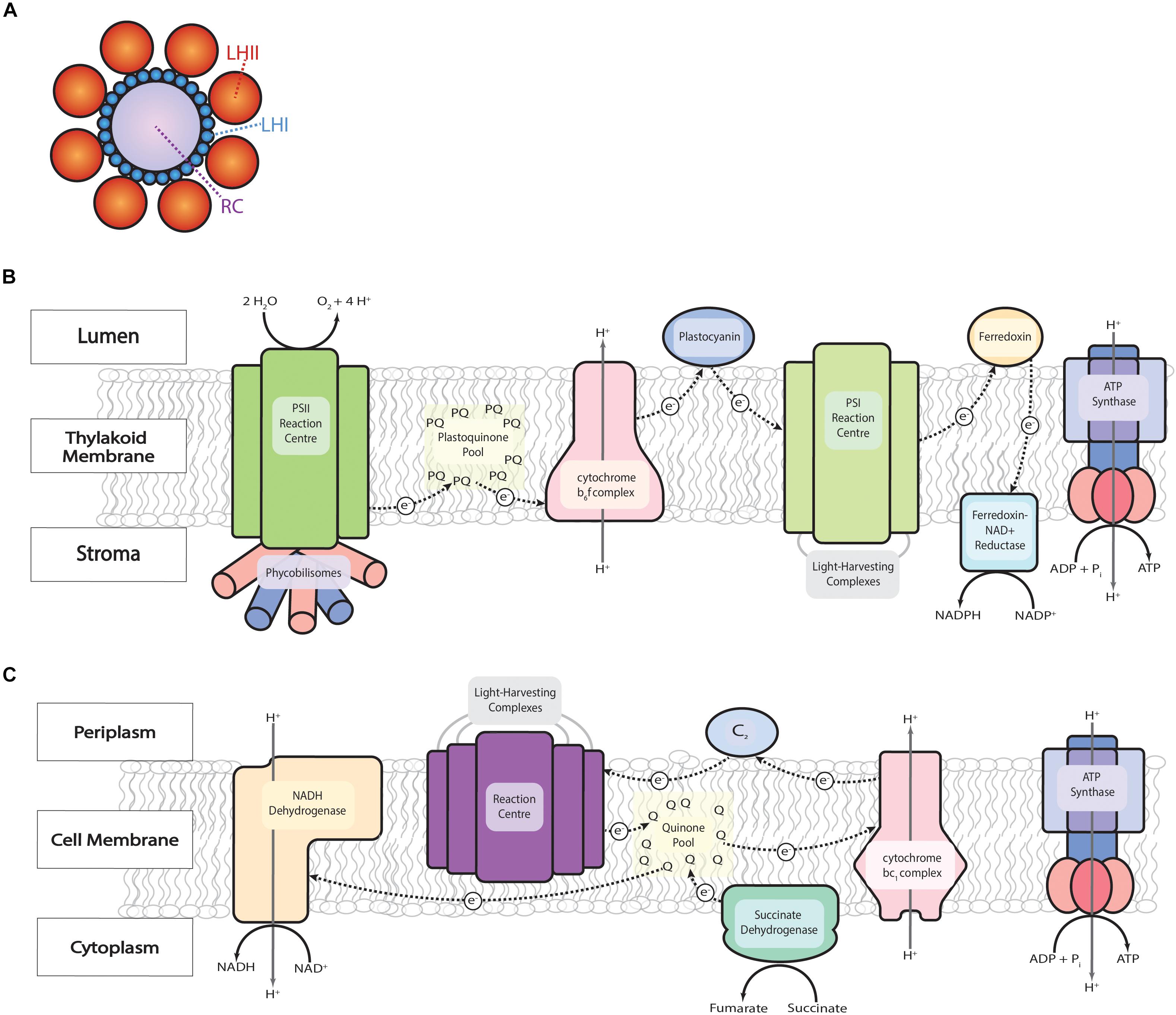
Figure 1. The ETC of oxygenic and anoxygenic photosynthesis. (A) Arrangement of light harvesting complexes (LH) around the reaction center (RC) of photosynthetic bacteria with two types of light harvesting complexes. Based on an atomic force micrograph of Phaeospirillum molischianum (Fassioli et al., 2009). (B) In oxygenic photosynthesis, as in cyanobacteria, there is a two-stage process of electron excitation. Solar energy is absorbed by the light-harvesting antenna complexes that surround the reaction center of Photosystem II (PSII) and transferred by resonance energy transfer to its reaction center where water is split, oxygen is released, hydrogen protons create a proton gradient across the membrane, and electrons begin to travel down the ETC first to the plastoquinone (PQ) pool then to the cytochrome b6f complex. The cytochrome b6f complex pumps more protons across the membrane and transfers electrons to Photosystem I (PSI) via plastocyanine and then transferred to ferredoxin- NADP+ reductase via ferredoxin to reduce NADP+ to NADPH. (C) Anoxygenic photosynthesis, as in PNSB, is simpler than that of oxygenic photosynthesis as there is only one reaction center for the excitation of electrons (e–) and the flow of these electrons is cyclic. The light-harvesting complexes accept photons and transfer energy to the reaction center where electron excitation occurs and begins to travel along the ETC to quinone (Q), cytochrome bc1, and cytochrome c2 (c2) generating a proton motive force whereby ATP can be synthesized via ATP synthase. PNSB benefits from a large buildup of an electrochemical energy that creates a reverse electron flow from the quinone pool to NADH dehydrogenase to produce NADH from NAD+.
Each reaction center is surrounded by a variety of nearly 200 choromophores (colored pigments like carotenoids, phycobilins, and chlorophyll or bacteriochlorophyll) bound in light harvesting complexes (Scholes et al., 2011). Different organisms contain different combinations of chromophores and, therefore, different ranges of absorbable light wavelengths, depending on their evolutionary history (Arp et al., 2020). In addition to their functionality in energy absorption for photosynthesis, these pigments are marketable products for dyes and as health products. For instance, carotenoids are brightly colored red and orange pigments that give foods such as tomatoes and carrots their characteristic color. Photosynthetic cells use them for the absorption of short wavelength, high energy light, protection from light damage, and as antioxidants (Hashimoto et al., 2016). Carotenoids also function as antioxidants when consumed by humans, making them marketable health supplements and beauty products, of which the most well-known is β-carotene (Stahl and Sies, 2003).
Oxygenic photosynthesis (Figure 1B) has a two-stage process of electron excitation found in plants, algae, and cyanobacteria. Solar energy is absorbed by the antenna-like light-harvesting complexes that surround the reaction center of Photosystem II (PSII) and transferred by resonance energy transfer to its reaction center where this energy is used to break the O-H bond in water, releasing molecules of dioxygen (O2), protons (H+), and electrons (e–). The electrons begin to travel down the ETC and the protons create an electrochemical gradient across the thylakoid membrane. First, the electrons are transferred to a pool of plastoquinone (PQ), reducing it to plastoquinol (PQH2). PQH2 can then diffuse through the membrane to transfer electrons to the cytochrome b6f complex. Ultimately, one electron ends up being transferred to photosystem I (PSI) by diffusion of either cytochrome c6 or plastocyanine depending on the organism expression (Lea-Smith et al., 2016). Once in PSI, the electron is re-excited by the absorption of solar energy. The electron is then transferred by diffusion of ferredoxin to reduce NADP+ via the enzyme ferredoxin-NADP+ reductase (Lea-Smith et al., 2016).
Anoxygenic photosynthesis (Figure 1C) such as that performed by PNSB is arguably a simpler process with only one stage of electron excitation and cyclic electron flow to generate the proton motive force necessary for ATP production. Just as in the oxygenic process, solar energy is absorbed by the light-harvesting complexes and transferred to the reaction center to excite an electron, but this electron is then transferred to the quinone pool. From there, electron pairs are transferred to the cytochrome bc1 complex to pump protons across the membrane, then on to cytochrome c2, and back to the reaction center in a cyclic process to generate a proton motive force that drives ATP production. Another differentiation from oxygenic photosynthesis is that there can be reverse electron flow in anoxygenic photosynthesis when there is sufficient build-up in the quinone pool: electrons travel against the thermodynamic gradient, driven by the energy of the proton motive force toward NADH dehydrogenase to generate NADH from NAD+ (Madigan et al., 2010b; Adessi and De Philippis, 2013).
Anoxygenic photosynthesis may only have one stage of light harvesting but it requires less ubiquitous, more reduced molecules than water as an electron donor, the donor for oxygenic photosynthesis. However, PNSB are widely considered among the most metabolically diverse groups of bacteria (Larimer et al., 2004; Pechter et al., 2016; Imhoff, 2017). Using light as their energy source and organic carbons as both the electron donor and carbon source, photoheterotrophy is the preferred mode of growth but PNSB can switch between any of the metabolic modes that support life: photoautotrophy, photoheterotrophy, chemoautotrophy, and chemoheterotrophy (Imhoff, 2017). This metabolic versatility is one aspect that makes it attractive for engineering not only because of the breadth of opportunities for products but also because of the possibilities for feedstocks. PNSB have been studied extensively for wastewater cleanup (Adessi et al., 2017) including in olive mill wastewater (Eroglu et al., 2010; Pintucci et al., 2013), beet molasses (Keskin and Hallenbeck, 2012), dairy wastewater (Seifert et al., 2010b), soy sauce wastewater (Anam et al., 2012), brewery wastewater (Seifert et al., 2010a), a swine wastewater ditch (Okubo et al., 2006), and crude glycerol (Sabourin-Provost and Hallenbeck, 2009; Ghosh et al., 2012a, b). It has also been shown to be effective for the bioremediation of aromatic compounds (Harwood and Gibson, 1986, 1988; Sasikala and Ramana, 1998; Zengler et al., 1999; Gibson and Harwood, 2006) including lignin monomer degradation (Harwood and Gibson, 1988; Salmon et al., 2013).
The origin of photosynthesis and how it has evolved is a subject that has been studied for decades and has yet to reach definitive conclusions (Hohmann-Marriott and Blankenship, 2011; Cardona, 2019). Based on phylogenetic analysis, the current consensus is that anoxygenic photosynthesis preceded oxygenic photosynthesis (Xiong and Bauer, 2002) and that oxygenic photosynthesis evolved from anoxygenic photosynthesis (Dismukes et al., 2001). Whichever way photosynthesis evolved, the organisms operating on it often evolved in the environment together (Cogdell et al., 2006). In lakes, cyanobacteria and algae are often aggregated near the surface where the water is aerobic and where their chlorophyll absorb a large portion of the blue and red light. Whereas purple bacteria can be found beneath them in the microaerobic to anaerobic depths and where the solar spectrum has been filtered by the upper level microbes leaving the longer wavelength green and red light to be absorbed by carotenoids and bacteriochlorophyll, respectively (Cogdell et al., 2006). The specific pigments and carotenoids that make up the antenna complexes of photosynthetic organisms evolved based on light availability in their environmental niche. Using a network model, the ideal absorption wavelengths of bacterial photosystems was accurately predicted with just the incident light wavelengths typical of their environment as inputs (Arp et al., 2020).
Knowing the origins and motives behind these organisms operating the way they do is important in biological process development, particularly when engineering an organism. Selecting an organism that functions on either oxygenic or anoxygenic photosynthesis depends upon the application an engineer is designing for. For example, if a product or process is sensitive to oxygen, such as enzymes used in the production of butanol (Lan and Liao, 2011), perhaps the process would be better suited to the anoxygenic PNSB. Or, if a process is being designed around the consumption of CO2 and it is not an ideal situation to provide a separate electron donor [which is required for PNSB to reduce CO2 (Larimer et al., 2004)], then perhaps the process would be better suited to cyanobacteria. In any case, photosynthetic organisms have evolved to sustain their own growth and metabolic processes necessary for survival not to maximize chemical production. They would, thus, need to be engineered to maximize their capacity for industrial process scales starting with solar energy capture.
Engineering Photosynthesis
In photosynthetic organisms, it is estimated that as much as 75% of incident photon flux is in excess (Lüttge, 2011). As a result, they have evolved methods like safety valves to regulate, reroute, and dissipate the excessive electrons excited within their reaction centers (Scholes et al., 2011). If these electrons could be redirected toward an alternative pathway or if the bottleneck to their usage could be removed, there could be an uptick in the potential for commercial viability.
One of the most notorious bottlenecks in photosynthesis is the first enzyme in the carbon fixation cycle: ribulose-1,5-bisphosphate carboxylase-oxygenase, also known as RuBisCO. RuBisCo is a large enzyme that can only process about three molecules of CO2 every second, which is a fraction of the substrate a typical enzyme can process (Alberts et al., 2014). Additionally, oxygen competes for its active site, which inactivates it for carbon fixation. Consequently, the energy of many excited electrons must be dissipated because they cannot be used fast enough. Many studies have, thus, been conducted to improve the efficiency of photosynthesis by improving the efficiency of RuBisCO using site-directed mutagenesis or directed evolution (Cai et al., 2014; Cotton et al., 2015). Efforts include increasing the selectivity of CO2 over oxygen as well as increasing the speed with which RuBisCO catalyzes its reactions (Cai et al., 2014; Cotton et al., 2015; Cummins et al., 2018). A recent analysis suggested that there is a trade-off between this specificity and turnover rate caused by selection pressures of the enzyme’s activity and stability (Cummins et al., 2018). Further understanding of this competition is needed in order to improve the constraints of RuBisCO. If carbon fixation is upstream of the target product production pathway, this step will be rate-limiting. Alternatively, incorporating an additional carbon-fixation enzyme can also reduce this bottleneck. In a recent study, phosphoenolpyruvate carboxylase, a carbon-fixation enzyme common in C4 and CAM plants, was inserted into the cyanobacterium Synechocystis PCC 6803 and resulted in an increase of ethylene production (Durall et al., 2020).
Bypassing carbon fixation by providing electrons with an alternative pipeline directly from the ETC is also a tactic being investigated. An example demonstrating this is the insertion of the mammalian cytochrome P450 CYP1A1 into cyanobacterium Synechococcus PCC 7002 as an artificial electron sink for excess electrons (Berepiki et al., 2016). In photoheterotrophic PNSB, such as Rhodopseudomonas palustris (R. palustris), hydrogen production is a natural alternative pathway to carbon fixation used as an electron sink (Figure 2). When the nitrogenase enzyme is obligately expressed, flux from the ETC preferentially flows toward hydrogen production over carbon fixation (McKinlay and Harwood, 2010, 2011). And, under certain circumstances, having an alternative pathway to carbon fixation is even a necessity for redox balancing. It was shown that mutant strains where RuBisCO was deleted could not grow at all due to a redox imbalance unless there was the alternative pathway toward hydrogen production the organism could use as an electron sink (Gordon and McKinlay, 2014). This shows that excess electrons can travel down this alternative pathway to hydrogen production.
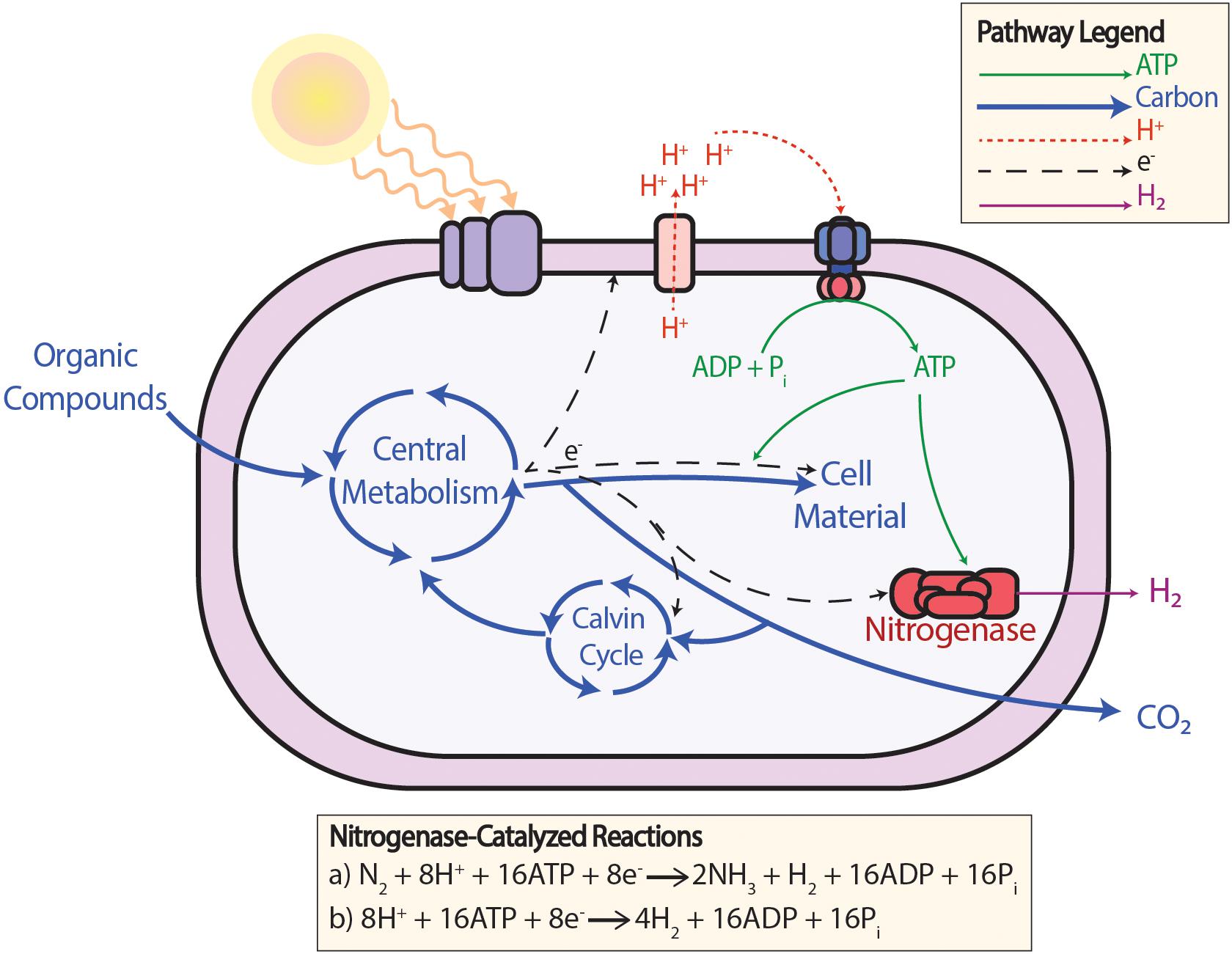
Figure 2. The production of H2 gas from the nitrogenase enzyme in PNSB under photoheterotrophic conditions. In wild-type PNSB, (a) the nitrogenase enzyme is expressed for N2 fixation when a more reduced nitrogen compound is not available. In mutant PNSB with the nitrogenase enzyme obligately expressed and more reduced nitrogen compounds are provided, (b) N2 does not need to be fixed and additional H2 can be produced using the same amount of ATP.
The ETC itself has also been demonstrated to be a potential bottleneck to increased electron flux capacity. Investigating the difference between the cyanobacteria Synechococcus 7942 and the faster growing Synechococcus 2973, researchers concluded that there is a bottleneck following PSII: there are insufficient electron carriers to continue down the ETC in the slower growing strain— the faster strain had a higher expression of PSI, cytochrome b6f, and plastocyanin on a per cell basis (Ungerer et al., 2018). Similarly, in PNSB, using a computational genome scale metabolic model, it was shown that the quinol oxidation rate was a determining factor for energy production from the ETC (Alsiyabi et al., 2019). Thus, conceivably, the overexpression of complexes downstream of PSI would reduce the bottleneck in PNSB.
Another method being used to engineer organisms for industrial applications is adjusting the photochemistry of photosynthesis (i.e., engineering the complexes and antennae of light absorption). As mentioned previously, most of the photon flux incident on the light harvesting complexes are in excess. However, only a small portion of those photons are within the range of wavelengths absorbable by the antenna. In cyanobacteria, PSII absorbs wavelengths around 680 nm and PSI absorbs around 700 nm, which means they often compete for photons of similar wavelength (Blankenship et al., 2011). Thus, it is suggested that if they were absorbing from different regions of the electromagnetic spectrum with a wider electrical potential difference, efficiency would increase, which has been observed in photovoltaic cells (Hanna and Nozik, 2006; Blankenship et al., 2011). By introducing antennae from other organisms (Hitchcock et al., 2016), one can expand the range of different light wavelengths that can be used in oxygenic photosynthesis into the lower energy infrared region (Blankenship et al., 2011; Chen and Blankenship, 2011). Similarly, in anoxygenic organisms where there is only one photosystem, this could be achieved by modifying the antenna proteins surrounding the reaction center (Swainsbury et al., 2017). Increasing energy capture in photosystems could increase the potential for desirable chemical production in these organisms. The development of tools for the modification of photosystems could reduce rigidity in the wavelength of light necessary for function of these organisms (Swainsbury et al., 2017), such as in the context of artificial growth systems like a photobioreactor or perhaps in a mixed community of photosynthetic organisms that could be modified to absorb the same wavelength of light for simplicity of lighting design. With these tools, organisms could be decoupled from their environmental niche and adjusted with process design in mind.
Increasing the electron flux down the ETC increases the capacity for solar harvesting in photosynthetic bacteria and maximizes the generation of reducing equivalents— essentially optimizing the upstream process of a photosynthetic organism. Ultimately, this would be for naught if the downstream process is not also optimized. Whether generating a new pathway for product formation or increasing the formation of natural products, metabolic bottlenecks in the transfer of electrons in the upstream process to ultimate product formation need to be resolved.
Engineering Electron Pathways for Product Formation
Conventionally, genetic modifications used a combination of random mutations and phenotypic screening methods (Nielsen, 2001) but numerous sophisticated tools and procedures have since been developed and the field has expanded. Using synthetic biology tools like regulating transcription or translation and modifying genetic material through the insertion or deletion of genes (Cheng and Lu, 2012), one can repurpose microorganisms to produce or overproduce native and non-native chemicals in a completely renewable process. An engineer may wish to think of a cell as a chemical plant as illustrated in Figure 3. Parallels can be drawn between an operator opening and closing valves in the pipelines between reactors in a chemical plant to genetic modifications in a cell. The carbon sources and electrons of metabolism can be imagined as being transferred in a pipeline to a reactor to produce a specific product. In a cell, this reactor is an enzyme, whose expression can be controlled genetically.
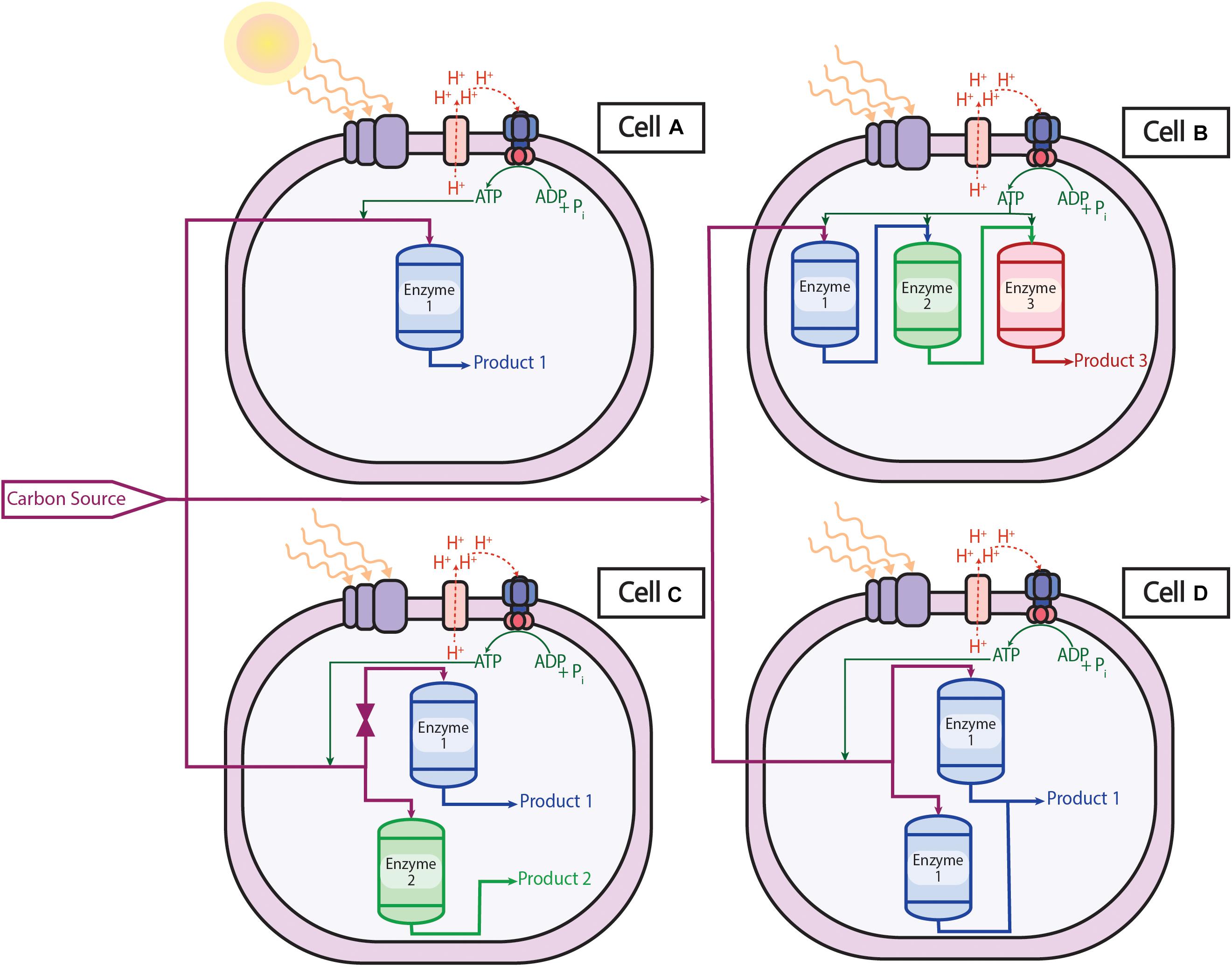
Figure 3. Solar-powered microbial cell factories: Cell A is the wild type organism that takes the carbon source, energy, and electrons and converts them to Product 1 via Enzyme 1. Cell B has had two heterologous enzymes (Enzyme 2 and Enzyme 3) inserted into the cell that produce Product 3 via the original Product 1. Cell C has silenced Enzyme 1 such that no or minimal Product 1 is produced in favor of Product 2 via the inserted non-native Enzyme 2. Cell D has overexpressed Enzyme 1 such that native Product 1 is overproduced.
The model organisms of metabolic engineering are the prokaryotic bacteria Escherichia coli (E. coli) and the eukaryotic yeast Saccharomyces cerevisiae (S. cerevisiae). They became model organisms mainly due to the speed at which they grow and the ease with which they can be handled but also because use begets more use. The more they were used for this application as the field was developing in the 1970s and 1980s, the more was known about them and the further technology was developed around them (Woolston et al., 2013). Other bacteria have also been used on a smaller scale for metabolic engineering for reasons such as their ability to grow at higher temperatures for faster reaction rates (e.g., thermophiles) or to use alternative feedstocks (e.g., CO2) (Woolston et al., 2013). However, in other organisms, synthetic biology tools have been reported to behave differently than they do in E. coli (Huang et al., 2010). Thus, since tool development in unconventional organisms has not been as rapid, the ability to use them in biological process development has also not been as rapid.
The engineering of photosynthetic organisms to produce heterologous products involves redirecting the electrons and energy generated from photosynthesis away from native pathways and toward more industrially advantageous products. Among photosynthetic microbes, cyanobacteria are the model organisms for metabolic engineering due to their genetic tractability and ability to produce valuable products using only CO2 and light (Jensen and Leister, 2014; Knoot et al., 2018; Xia et al., 2019; Hitchcock et al., 2020; Luan et al., 2020). As summarized in Table 1, the most common cyanobacterial strains used in metabolic engineering are Synechococcus 7942, Synechococcus 7002, and Synechocystis 6803, which have been used as chemical production chassis for such chemicals as biofuels, commodity chemicals, and flavoring compounds. One of the first successful heterologous pathways demonstrated in cyanobacteria was that of the biofuel ethanol (Deng and Coleman, 1999). A titer of 0.23 g/l was achieved by diverting the electron pathway from pyruvate for heterologous expression of the enzymes pyruvate decarboxylase and alcohol dehydrogenase from the organism Zymomonas mobilis (Deng and Coleman, 1999). As shown in Table 1, the titers of ethanol have since been improved 20-fold by a combination of gene overexpression, replacing native genes with better performing mutants, and improving cofactor availability along with some media optimization. Alternatively, pyruvate can be diverted to the production of different chemicals, such as the plastic precursor 2,3-butanediol by inserting the galactose-proton symporter and the enzymes G6P dehydrogenase and 6PG dehydrogenase (Kanno et al., 2017). However, electrons can be diverted from any point in the metabolism of an organism. Diverting electrons from further up the glycolytic pathway than pyruvate, such as from glucose, products such as sucrose can be produced (Ducat et al., 2012) or further down the pathway, such as from acetyl-CoA, fatty acids can be synthesized (Liu et al., 2011). While there have been advances in the development of tools for cyanobacteria (Wang et al., 2017), production of some of these chemicals are several orders of magnitude lower than they have been demonstrated to be in E. coli, due to relatively fewer synthetic biology tools that are effective in cyanobacteria, scarcity of plasmid libraries for expression of genes in cyanobacteria, and cyanobacterial polyploidy (Hitchcock et al., 2020; Luan et al., 2020).
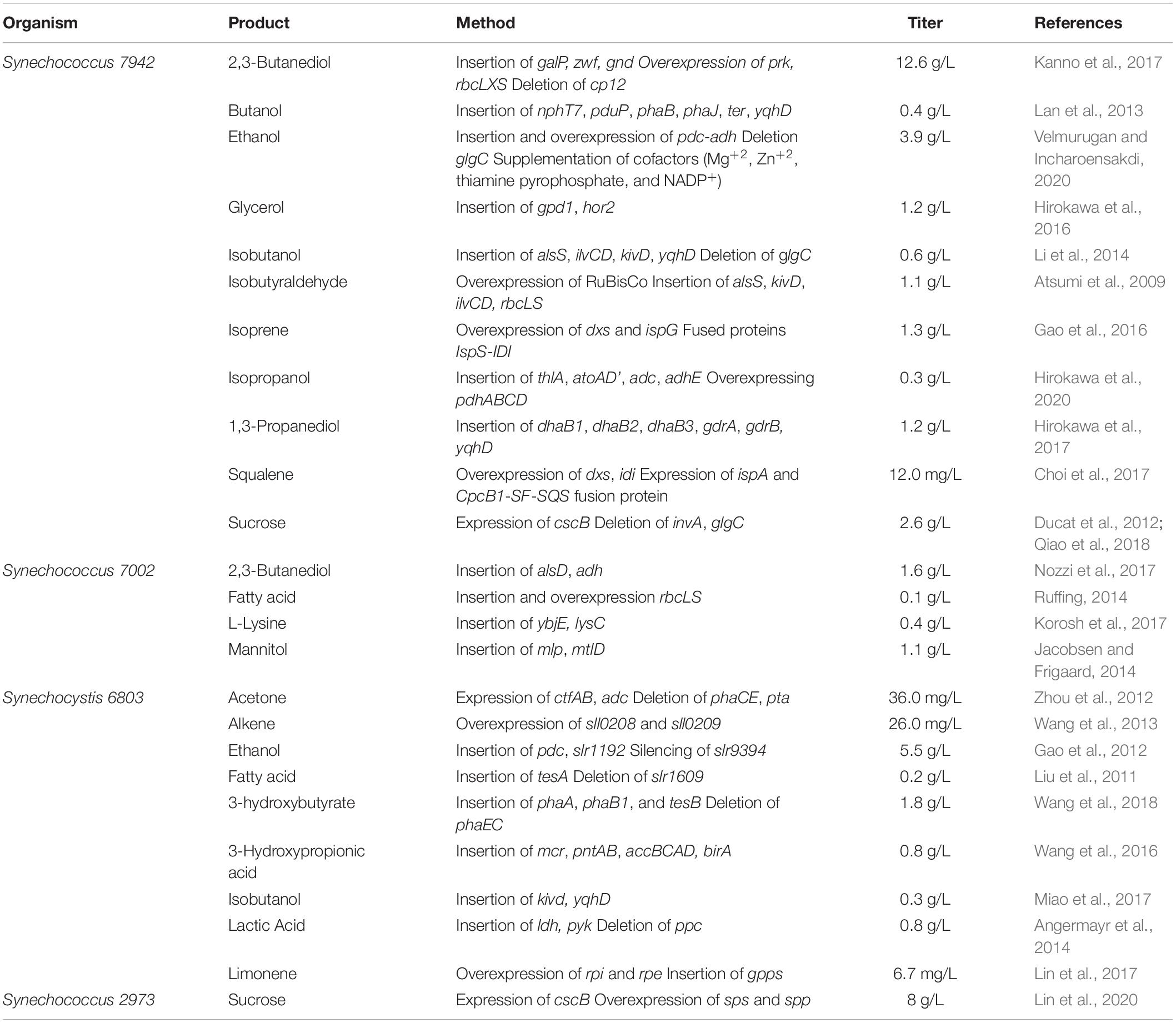
Table 1. Cyanobacteria as cell factories: the production of heterologous products via genetic modification and the production titers thereof.
Facing similar difficulties, engineering the anoxygenic organism PNSB is an even more recent endeavor. However, interest in PNSB for its intrinsic ability to produce a commercially important product precedes efforts to engineer the organism itself. PNSB are naturally able to produce hydrogen gas as a by-product of nitrogen fixation and as an alternative pathway for an electron sink when there is an excess of electrons from photosynthesis (Gordon and McKinlay, 2014). This cellular process is shown in Figure 2. The initial efforts at metabolic engineering PNSB were mainly directed toward increasing hydrogen production through the deletion of competing pathways such as carbon dioxide assimilation (Joshi and Tabita, 1996), or through the obligate expression of nitrogenases such that hydrogen is produced even in the absence of nitrogen gas (Rey et al., 2007). Another product that both PNSB and cyanobacteria produce naturally is poly(3-hydroxybutyrate) or PHB (Ansari and Fatma, 2016; Ranaivoarisoa et al., 2019). PHB is a lipid used for energy storage in many prokaryotic bacteria but, as a marketable product, PHB is a polymer precursor for biodegradable plastics (Ranaivoarisoa et al., 2019). Engineering of these microbes resulted in titers of PHB as high as 1.9 g/l in PNSB (Kobayashi and Kondo, 2019) and 1.8 g/l in cyanobacteria (Wang et al., 2018).
More recently, interest in PNSB has shifted to other marketable products that are heterologous such as terpenoids, non-native carotenoids, and biofuels (Table 2). The sesquiterpenoids valencene (citrus flavor) and patchoulol (patchouli scent) are being produced in Rhodobacter sphaeroides on an industrial scale (Schempp et al., 2018). Carotenoids are commonly used as pigments, but they are also antioxidants that are used in health supplements (Fardoux et al., 2008; Wang et al., 2019). Since photosynthetic organisms naturally produce some carotenoids, much of the pathway is naturally present, from which carbon can be easily diverted to produce non-natural products of commercial importance such as lycopene and β-carotene (Giraud et al., 2018). Cyanobacteria are also used to produce carotenoids but since they are oxygen sensitive (Krinsky and Deneke, 1982), using anoxygenic organisms may be beneficial. Research in the production of biofuels other than hydrogen is a new area for PNSB with butanol being one of the most recent producing titers of 0.1 g/L from a butyrate feedstock (Doud et al., 2017).
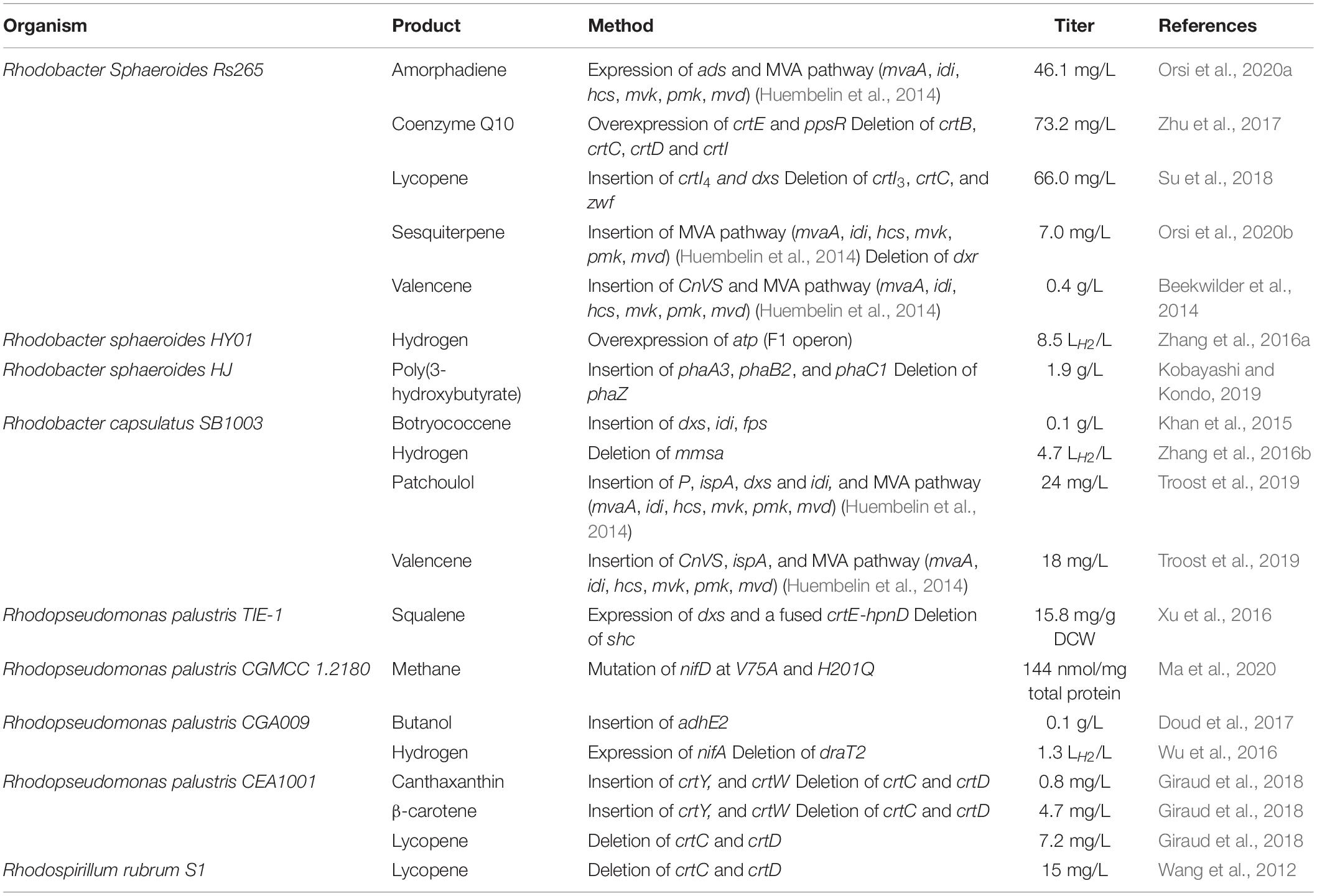
Table 2. Purple non-sulfur bacteria as cell factories: the production of heterologous products and overexpression of native products via genetic modification and the production titers thereof.
The ability to produce marketable products on a small scale is an important step in the development of an industrial process but this process must be scalable. A common issue with failed process scale-ups is the development of a microbial strain without consideration for how suitable it would be for industrial production (Crater and Lievense, 2018). While there are several tactics for scale-up in conventional microbial systems based on oxygen mass transfer or volumetric power consumption (Marques et al., 2010), photosynthetic bacteria introduce additional bioprocess considerations.
Engineering Light Delivery Systems
Engineering the internal chemistry of an organism to produce heterologous compounds or to increase the production of natural compounds is certainly essential in the development of economically viable bioprocesses. However, the external environment, in the form of reactor design and process optimization, also has a significant impact on product formation. Factors including light intensity and penetration as well as cell immobilization affect the metabolism of cells and, therefore, product formation.
One of the most important considerations in terms of reactor design for photosynthetic organisms is that of light delivery. Due to cell shading and light scattering, there is attenuation of light from the source into the depths of a reactor, particularly when the process liquid is turbid. Hence, delivering light uniformly and at an effective intensity can be a challenge. This challenge is one that the algae industry has been facing for many years. The most common system for algal growth is the outdoor raceway pond and other such open systems as they are the most economical and simple to operate (Nwoba et al., 2019). However, in addition to the issue of light attenuation, open systems are more difficult to scale up. Moreover, it is difficult to implement systems for pH or temperature control and maintain sterility in such systems (McBride and Merrick, 2014). When developing a process around an engineered organism, these factors are important to account for, and an open system would likely not be a practical or, ultimately, economical option if the engineered organism is outcompeted by wild bacteria. Developing methods for improving light delivery in a closed system has been a focus in the field of algal technology and one which has extended to use for other photosynthetic organisms (Heimann, 2016; Nwoba et al., 2019).
One of the techniques proposed for uniform light distribution is to grow cells on the surface on which light is incident using waveguide technology (Jung et al., 2012; Doud and Angenent, 2014; Genin et al., 2015; Delamare-Deboutteville et al., 2019; Nwoba et al., 2019). As the name suggests, a waveguide guides light from a light source and down the span of its surface allowing light to penetrate to the depths of a dark reactor and can also provide a surface to which cells can adhere. In addition to providing cells with light more effectively, using cell immobilization can be quite advantageous from an operational standpoint. Since biomass is already separate from the process fluid, downstream separation costs can be reduced through this method. Moreover, the immobilized biomass can be transferred to fresh media in a modular manner. For example, in a study using engineered yeast immobilized in a manufactured polymer, it was shown that cells could be reused for over a year of production (Johnston et al., 2020). In PNSB, Rhodobacter capsulatus cells immobilized on agar produced hydrogen stably for over 70 days (Elkahlout et al., 2019). In cyanobacteria engineered to produced ethylene, cells immobilized in an artificial biofilm matrix produced ethylene for up to 38 days (Vajravel et al., 2020). The reuse of cells without a growth phase could reduce yield losses incurred during the growth of the organisms.
Most bacterial cells naturally aggregate in a biofilm by excreting an extracellular polymeric matrix to adhere to surfaces and to each other (Halan et al., 2012). In nature, biofilms are one of the most widely distributed modes of life (Flemming et al., 2016). There are many benefits to biofilm cultivation such as the facilitation of intercellular interaction, resource capture, protection from external stressors like pH or temperature variations, and resistance to toxins (Flemming et al., 2016) that could prove advantageous while designing a robust host system for bioprocesses. This type of cellular immobilization has even been suggested as a means of improved reactor productivity because a higher density of cells can be concentrated into a smaller space than with suspended cultures and also because chemical products can diffuse out reducing toxicity of high chemical concentrations for the cell (Karagöz et al., 2009; Winkelhausen et al., 2010). This would mean increased product from the same reactor size as a suspended culture and has been shown to be the case for hydrogen production from PNSB (Chen and Chang, 2006; Adessi and De Philippis, 2014; Tsygankov and Kosourov, 2014; Wu et al., 2016; Adessi et al., 2017). It has also been suggested that, when in a microbial mat aggregate, cyanobacterial cells can export excess electrons to other cells in order to prevent over-reduction of their own photosystems ensuring that even cells with access to only lower energy wavelengths have access to electrons (Babauta et al., 2014; Lea-Smith et al., 2016). This suggests that cellular immobilization on the surface of a waveguide light source could encourage using neighboring cells as electron sinks for excess electrons in the event of production saturation, which would perhaps result in reduced photodamage and loss of energy as heat.
In addition to providing energy, light can be used as an engineering technique. Considering that the full spectrum of light is not absorbed by a photosynthetic organism and that different photosystems have evolved to preferentially absorb certain wavelengths and light intensities (Arp et al., 2020), light of different wavelengths can be used to modulate gene expression. Using monochromatic LED lights emitting wavelengths at the absorption maxima of a specific chromophore, one could select for different components of a photosystem. In the cyanobacteria Arthrospira maxima, more chlorophyll a can be formed under red light while phycobiliprotein reaches a maximum in blue light (Park and Dinh, 2019). Comparably, in the PNSB R. palustris, carotenoids can be produced maximally under blue light (Kuo et al., 2012).
Similar to the effects of wavelength, light intensity also affects gene expression in a photosynthetic organism. PNSB grow additional light harvesting complexes (pigments and carotenoids) around their photosynthetic reaction centers in the presence of low light intensity (Fassioli et al., 2009; Scholes et al., 2011). These additional chromophores increase the cross-section of light absorbable by the antenna and, thus, the energy which can be transferred to the reaction center for electron excitation. For example, it has been shown that in low light intensity (4 μmol photons/m2/s), R. palustris upregulates expression of different light harvesting complexes than it does under high light intensity (30 μmol photons/m2/s) (Fixen et al., 2019). This is caused by a change in the redox potential of the electron transport chain: under conditions of low light intensity, the quinone pool is more oxidized, which causes a redox signal (Fixen et al., 2019). Redox signals caused by a change in light intensity have also been observed in other PNSB (Swem et al., 2006; Wu and Bauer, 2010). Similar results are observed in cyanobacteria: in high light intensities, a higher growth rate is observed, and, in low light intensities, there is increased expression of light harvesting complexes (Jahn et al., 2018). From a practical perspective, one could potentially use this in process development: if the goal of the process is to produce pigments, one could ensure the photobioreactor has a low light intensity to increase their concentration. Conversely, increasing light intensity could result in an abundance of electrons that could be used for downstream chemical production. In PNSB, this has been studied with its native hydrogen production pathway where an increase in hydrogen is indeed observed with increased light intensity until saturation is reached (Uyar et al., 2007; Adessi and De Philippis, 2014). However, while an increased light intensity would increase electron availability, it is also associated with increased photodamage (Mellis, 1999). Increasing the rate of photodamage would result in reduced photosynthetic efficiency while the cell works to repair itself, so this is a consideration to make in the process design.
While bioreactors are often considered merely vessels for cellular growth, the way they are designed and operated affect the internal processes of the cell through upregulation or downregulation of genes in response to their environment. The availability, intensity, and wavelength of light inside a photobioreactor affect the structure and quantity of solar harvesting complexes for photosynthesis, which impacts the energy and electrons available for downstream cellular processes. Likewise, cellular immobilization can make cells more resilient to sudden changes in temperature, pH, and nutrient availability by creating a microenvironment separate from the reactor bulk fluid and where there is a division of labor among the individual cells (Flemming and Wingender, 2010). All aspects in the design of a bioprocess affect each other and, while this interconnectedness increases complexity, it also implies countless opportunities for optimization and improvement as well as possibilities for product formation.
Conclusion
To “begin with the end in mind” is an approach recommended when the overall goal is large-scale production (Crater and Lievense, 2018). More specifically, it is the idea that the scale-up of a process into industrial production will influence research from the beginning in the selection of the product and microbe and throughout the research process. In the case of solar-powered microbial cell factories, the selection of a photosynthetic organism depends largely on the chemical desired as a product and the feedstocks available as inputs. This necessitates an understanding of the internal cellular processes from photosynthesis to central metabolism and the effects of environmental factors on both.
A simple explanation of the differences between oxygenic and anoxygenic photosynthetic organisms is that oxygenic photosynthesis, such as that performed by cyanobacteria, evolves oxygen from the splitting of water molecules whereas anoxygenic photosynthesis, such as that performed by purple bacteria, does not evolve oxygen. Additional distinctions include the differing complexes of the ETC, whether the flow of electrons within the ETC is cyclic or not, and differences in feedstock preferences: some organisms more efficiently consume CO2 while others specialize in the uptake of organic carbons in wastewater. However, there is more complexity to it when considering the internal processes and bottlenecks in the ETC.
With much of the energy incident on photosynthetic organisms being dissipated as waste, there is considerable opportunity to increase the flux of electrons through the ETC and toward the production of chemicals. One bottleneck is RuBisCO, which is both slow and lacking in selectivity for the fixation of carbon. Another is the complexes in the ETC itself, where increasing expression of electron carriers can improve electron flux capacity. A further prospect to augment electron flux through the ETC is to modify the antennae of photosynthesis to increase the spectrum of absorbable energy. With more energy and electron flux available from the ETC, there is an enhanced ability to produce chemicals.
The production of chemicals using strategies such as the expression of heterologous genes, the deletion of native genes, and the overexpression of genes has been demonstrated in both oxygenic and anoxygenic photosynthetic organisms, but on significantly lower scales than with model organisms such as E. coli and yeast. Development and improvement of the library of tools available for use in unconventional organisms will be needed to advance this field, as will be the development of photobioreactors practical for this application on a large scale.
Considerations for light availability inside a photobioreactor is a challenge for effective distribution and for setpoint optimization both for wavelength and intensity. These affect the structure and availability of photosynthetic complexes and thus the efficiency of energy harvesting. The development of strategies for effective light distribution is an area requiring advancement. Another technology that requires further development is the coupling of light to solar energy whether through waveguides that guide solar light or through solar-powered lighting systems.
Both oxygenic and anoxygenic photosynthetic organisms have vast potential to change the course of consumerism to one that is environmentally conscious. However, diverting electrons and energy stored from photosynthesis toward chemical production pathways is no small feat. The production of chemicals from photosynthetic organisms has been demonstrated but, for the most part, currently lacks the titers and the equipment necessary for large-scale production. While microbial cell factories have been in development for more than 40 years and some have reached industrial quantities (Yim et al., 2011), the advancement of solar-powered microbial cell factories has been delayed largely by a lack of genetic tools and challenges associated with photobioreactors such as the delivery of light. Considering all these factors in a holistic approach will likely be necessary to reach industrial scales of production. The potential to use solar energy to power the production of chemicals from anthropogenic waste will be critical to address net zero emissions in most advanced economies.
Author Contributions
SS compiled the literature and drafted the manuscript. RM and DGA revised and edited the manuscript. All authors contributed to the article and approved the submitted version.
Funding
Funding has been provided by the Natural Sciences and Engineering Research Council of Canada (NSERC).
Conflict of Interest
The authors declare that the research was conducted in the absence of any commercial or financial relationships that could be construed as a potential conflict of interest.
References
Adessi, A., Corneli, E., and De Philippis, R. (2017). “Photosynthetic purple non sulfur bacteria in hydrogen producing systems: new approaches in the use of well known and innovative substrates,” in Modern Topics in the Phototrophic Prokaryotes: Environmental and Applied Aspects, ed. P. C. Hallenbeck (Berlin: Springer International Publishing Switzerland), 321–350. doi: 10.1007/978-3-319-46261-5_10
Adessi, A., and De Philippis, R. (2013). Purple Bacteria: Electron Acceptors and Donors, 2nd Edn. Amsterdam: Elsevier Inc, doi: 10.1016/B978-0-12-378630-2.00371-6
Adessi, A., and De Philippis, R. (2014). “Photosynthesis and hydrogen production in purple non sulfur bacteria: fundamental and applied aspects,” in Microbial BioEnergy: Hydrogen Production, eds D. Zannoni and R. Philippis (Netherlands: Springer), 269–290. doi: 10.1007/978-94-017-8554-9
Alberts, B., Johnson, A., Lewis, J., Raff, M., Roberts, K., and Walter, P. (2014). “Energy conversion: mitochondria and chloroplasts,” in Molecular Biology of the Cell (New York. NY: Garland Science), 753–812. doi: 10.1201/9780203833445-14
Allen, J. P., and Williams, J. C. (2011). The evolutionary pathway from anoxygenic to oxygenic photosynthesis examined by comparison of the properties of photosystem II and bacterial reaction centers. Photosynth. Res. 107, 59–69. doi: 10.1007/s11120-010-9552-x
Alsiyabi, A., Immethun, C. M., and Saha, R. (2019). Modeling the interplay between photosynthesis, CO2 fixation, and the quinone pool in a purple non-sulfur bacterium. Sci. Rep. 9, 1–9. doi: 10.1038/s41598-019-49079-z
Anam, K., Habibi, M. S., Harwati, T. U., and Susilaningsih, D. (2012). Photofermentative hydrogen production using Rhodobium marinum from bagasse and soy sauce wastewater. Int. J. Hydrogen Energy 37, 15436–15442. doi: 10.1016/j.ijhydene.2012.06.076
Angermayr, S. A., Van Der Woude, A. D., Correddu, D., Vreugdenhil, A., Verrone, V., and Hellingwerf, K. J. (2014). Exploring metabolic engineering design principles for the photosynthetic production of lactic acid by Synechocystis sp. PCC6803. Biotechnol. Biofuels 7:99. doi: 10.1186/1754-6834-7-99
Ansari, S., and Fatma, T. (2016). Cyanobacterial polyhydroxybutyrate (PHB): screening, optimization and characterization. PLoS One 11:e0158168. doi: 10.1371/journal.pone.0158168
Arp, T. B., Kistner-morris, J., Aji, V., Cogdell, R., and Van, R. (2020). Quieting a noisy antenna reproduces photosynthetic light harvesting spectra. Science 368, 1490–1495. doi: 10.1126/science.aba6630
Atsumi, S., Higashide, W., and Liao, J. C. (2009). Direct photosynthetic recycling of carbon dioxide to isobutyraldehyde. Nat. Biotechnol. 27, 1177–1180. doi: 10.1038/nbt.1586
Babauta, J. T., Atci, E., Ha, P. T., Lindemann, S. R., Ewing, T., Call, D. R., et al. (2014). Localized electron transfer rates and microelectrode-based enrichment of microbial communities within a phototrophic microbial mat. Front. Microbiol. 5:11. doi: 10.3389/fmicb.2014.00011
Beekwilder, J., van Houwelingen, A., Cankar, K., van Dijk, A. D. J., de Jong, R. M., Stoopen, G., et al. (2014). Valencene synthase from the heartwood of Nootka cypress (Callitropsis nootkatensis) for biotechnological production of valencene. Plant Biotechnol. J. 12, 174–182. doi: 10.1111/pbi.12124
Bensaid, S., Centi, G., Garrone, E., Perathoner, S., and Saracco, G. (2012). Towards artificial leaves for solar hydrogen and fuels from carbon dioxide. ChemSusChem 5, 500–521. doi: 10.1002/cssc.201100661
Berepiki, A., Hitchcock, A., Moore, C. M., and Bibby, T. S. (2016). Tapping the unused potential of photosynthesis with a heterologous electron sink. ACS Synth. Biol. 5, 1369–1375. doi: 10.1021/acssynbio.6b00100
Blankenship, R. E., Sadekar, S., and Raymond, J. (2007). “The evolutionary transition from anoxygenic to oxygenic photosynthesis,” in Evolution of Primary Producers in the Sea, eds P. G. Falkowski and A. H. Knoll (Amsterdam: Elsevier Academic Press), 21–35. doi: 10.1016/B978-012370518-1/50004-7
Blankenship, R. E., Tiede, D. M., Barber, J., Brudvig, G. W., Fleming, G., Ghirardi, M., et al. (2011). Comparing photosynthetic and photovoltaic efficiencies and recognizing the potential for improvement. Science 332, 805–809. doi: 10.1126/science.1200165
Cai, Z., Liu, G., Zhang, J., and Li, Y. (2014). Development of an activity-directed selection system enabled significant improvement of the carboxylation efficiency of Rubisco. Protein Cell 5, 552–562. doi: 10.1007/s13238-014-0072-x
Cardona, T. (2019). Thinking twice about the evolution of photosynthesis. Open Biol. 9:180246. doi: 10.1098/rsob.180246
Carroll, A. L., Case, A. E., Zhang, A., and Atsumi, S. (2018). Metabolic engineering tools in model cyanobacteria. Metab. Eng. 50, 47–56. doi: 10.1016/j.ymben.2018.03.014
Chen, C. Y., and Chang, J. S. (2006). Enhancing phototropic hydrogen production by solid-carrier assisted fermentation and internal optical-fiber illumination. Process Biochem. 41, 2041–2049. doi: 10.1016/j.procbio.2006.05.005
Chen, M., and Blankenship, R. E. (2011). Expanding the solar spectrum used by photosynthesis. Trends Plant Sci. 16, 427–431. doi: 10.1016/j.tplants.2011.03.011
Cheng, A., and Lu, T. K. (2012). Synthetic biology: an emerging engineering discipline. Annu. Rev. Biomed. Eng. 14, 155–178. doi: 10.1146/annurev-bioeng-071811
Choi, S. Y., Wang, J. Y., Kwak, H. S., Lee, S. M., Um, Y., Kim, Y., et al. (2017). Improvement of squalene production from CO2 in Synechococcus elongatus PCC 7942 by metabolic engineering and scalable production in a photobioreactor. ACS Synth. Biol. 6, 1289–1295. doi: 10.1021/acssynbio.7b00083
Cogdell, R. J., Gall, A., and Köhler, J. (2006). The architecture and function of the light-harvesting apparatus of purple bacteria: from single molecules to in vivo membranes. Q. Rev. Biophys. 39, 227–324. doi: 10.1017/S0033583506004434
Connelly, T. M. Jr., Change, M. C., Clarke, L., Hillson, N. J., Johnson, R. A., Keasling, J. D., et al. (2015). Industrialization of Biology: A Roadmap to Accelerate the Advanced Manufacturing of Chemicals. Washington, DC: National Research Council, doi: 10.17226/19001
Cotton, C. A. R., Douglass, J. S., De Causmaecker, S., Brinkert, K., Cardona, T., Fantuzzi, A., et al. (2015). Photosynthetic constraints on fuel from microbes. Front. Bioeng. Biotechnol. 3:36. doi: 10.3389/fbioe.2015.00036
Crater, J. S., and Lievense, J. C. (2018). Scale-up of industrial microbial processes. FEMS Microbiol. Lett. 365:fny138. doi: 10.1093/femsle/fny138
Cummins, P. L., Kannappan, B., and Gready, J. E. (2018). Directions for optimization of photosynthetic carbon fixation: RuBisCO’s efficiency may not be so constrained after all. Front. Plant Sci. 9:183. doi: 10.3389/fpls.2018.00183
Delamare-Deboutteville, J., Batstone, D. J., Kawasaki, M., Stegman, S., Salini, M., Tabrett, S., et al. (2019). Mixed culture purple phototrophic bacteria is an effective fishmeal replacement in aquaculture. Water Res. X 4:100031. doi: 10.1016/j.wroa.2019.100031
Deng, M.-D., and Coleman, J. R. (1999). Ethanol Synthesis by Genetic Engineering in Cyanobacteria. Available online at: http://aem.asm.org/ (accessed August 9, 2020).
Dismukes, G. C., Klimov, V. V., Baranov, S. V., Kozlov, Y. N., Dasgupta, J., and Tyryshkin, A. (2001). The origin of atmospheric oxygen on earth: the innovation of oxygenic photosynthesis. Proc. Natl. Acad. Sci. U.S.A. 98, 2170–2175. doi: 10.1073/pnas.061514798
Doud, D. F. R., and Angenent, L. T. (2014). Toward electrosynthesis with uncoupled extracellular electron uptake and metabolic growth: enhancing current uptake with Rhodopseudomonas palustris. Environ. Sci. Technol. Lett. 1, 351–355. doi: 10.1021/ez500244n
Doud, D. F. R., Holmes, E. C., Richter, H., Molitor, B., Jander, G., and Angenent, L. T. (2017). Metabolic engineering of Rhodopseudomonas palustris for the obligate reduction of n-butyrate to n-butanol. Biotechnol. Biofuels 10, 1–11. doi: 10.1186/s13068-017-0864-3
Ducat, D. C., Avelar-Rivas, J. A., Way, J. C., and Silver, P. A. (2012). Rerouting carbon flux to enhance photosynthetic productivity. Appl. Environ. Microbiol. 78, 2660–2668. doi: 10.1128/AEM.07901-11
Durall, C., Lindberg, P., Yu, J., and Lindblad, P. (2020). Increased ethylene production by overexpressing phosphoenolpyruvate carboxylase in the cyanobacterium Synechocystis PCC 6803. Biotechnol. Biofuels 13:16. doi: 10.1186/s13068-020-1653-y
Elkahlout, K., Sagir, E., Alipour, S., Koku, H., Gunduz, U., Eroglu, I., et al. (2019). Long-term stable hydrogen production from acetate using immobilized Rhodobacter capsulatus in a panel photobioreactor. Int. J. Hydrogen Energy 44, 18801–18810. doi: 10.1016/j.ijhydene.2018.10.133
Eroglu, E., Gunduz, U., Yucel, M., and Eroglu, I. (2010). Photosynthetic bacterial growth and productivity under continuous illumination or diurnal cycles with olive mill wastewater as feedstock. Int. J. Hydrogen Energy 35, 5293–5300. doi: 10.1016/j.ijhydene.2010.03.063
Fardoux, J., Giraud, E., Hannibal, L., and Verméglio, A. (2008). Method for Producing Carotenoids and Bacteria Used Therefor. Washington, DC: U.S. Patent and Trademark Office.
Fassioli, F., Olaya-Castro, A., Scheuring, S., Sturgis, J. N., and Johnson, N. F. (2009). Energy transfer in light-adapted photosynthetic membranes: from active to saturated photosynthesis. Biophys. J. 97, 2464–2473. doi: 10.1016/j.bpj.2009.08.033
Fixen, K. R., Oda, Y., and Harwood, C. S. (2019). Redox regulation of a light-harvesting antenna complex in an anoxygenic phototroph. mBio 10:e02838-19. doi: 10.1128/mBio.02838-19
Flemming, H. C., and Wingender, J. (2010). The biofilm matrix. Nat. Rev. Microbiol. 8, 623–633. doi: 10.1038/nrmicro2415
Flemming, H. C., Wingender, J., Szewzyk, U., Steinberg, P., Rice, S. A., and Kjelleberg, S. (2016). Biofilms: an emergent form of bacterial life. Nat. Rev. Microbiol. 14, 563–575. doi: 10.1038/nrmicro.2016.94
Gao, X., Gao, F., Liu, D., Zhang, H., Nie, X., and Yang, C. (2016). Engineering the methylerythritol phosphate pathway in cyanobacteria for photosynthetic isoprene production from CO2. Energy Environ. Sci. 9, 1400–1411. doi: 10.1039/c5ee03102h
Gao, Z., Zhao, H., Li, Z., Tan, X., and Lu, X. (2012). Photosynthetic production of ethanol from carbon dioxide in genetically engineered cyanobacteria. Energy Environ. Sci. 5, 9857–9865. doi: 10.1039/c2ee22675h
Genin, S. N., Aitchison, J. S., and Allen, D. G. (2015). Novel waveguide reactor design for enhancing algal biofilm growth. Algal Res. 12, 529–538.
Ghosh, D., Sobro, I. F., and Hallenbeck, P. C. (2012a). Stoichiometric conversion of biodiesel derived crude glycerol to hydrogen: response surface methodology study of the effects of light intensity and crude glycerol and glutamate concentration. Bioresour. Technol. 106, 154–160. doi: 10.1016/j.biortech.2011.12.021
Ghosh, D., Tourigny, A., and Hallenbeck, P. C. (2012b). Near stoichiometric reforming of biodiesel derived crude glycerol to hydrogen by photofermentation. Int. J. Hydrogen Energy 37, 2273–2277. doi: 10.1016/j.ijhydene.2011.11.011
Gibson, J., and Harwood, C. S. (2006). “Degradation of aromatic compounds by nonsulfur purple bacteria,” in Anoxygenic Photosynthetic Bacteria, eds R. E. Blankenship, Madigan, T. Michael, and C. E. Bauer (Dordrecht: Kluwer Academic Publishers), 991–1003. doi: 10.1007/0-306-47954-0_46
Giraud, E., Hannibal, L., Chaintreuil, C., Fardoux, J., and Verméglio, A. (2018). “Synthesis of carotenoids of industrial interest in the photosynthetic bacterium Rhodopseudomonas palustris: bioengineering and growth conditions,” in Microbial Carotenoids: Methods and Protocols, Methods in Molecular Biology eds C. Barreiro and J. -L. Barredo (Totowa, NJ: Humana Press Inc), 211–220. doi: 10.1007/978-1-4939-8742-9_12
Gordon, G. C., and McKinlay, J. B. (2014). Calvin cycle mutants of photoheterotrophic purple nonsulfur bacteria fail to grow due to an electron imbalance rather than toxic metabolite accumulation. J. Bacteriol. 196, 1231–1237. doi: 10.1128/JB.01299-13
Halan, B., Buehler, K., and Schmid, A. (2012). Biofilms as living catalysts in continuous chemical syntheses. Trends Biotechnol. 30, 453–465. doi: 10.1016/j.tibtech.2012.05.003
Hanna, M. C., and Nozik, A. J. (2006). Solar conversion efficiency of photovoltaic and photoelectrolysis cells with carrier multiplication absorbers. J. Appl. Phys. 100:074510. doi: 10.1063/1.2356795
Harwood, C. S., and Gibson, J. (1986). Uptake of Benzoate by Rhodopseudomonas palustris grown anaerobically in light. J. Bacteriol. 165, 504–509.
Harwood, C. S., and Gibson, J. (1988). Anaerobic and aerobic metabolism of diverse aromatic compounds by the photosynthetic bacterium Rhodopseudomonas palustris. Appl. Environ. Microbiol. 54, 712–717.
Hashimoto, H., Uragami, C., and Cogdell, R. J. (2016). “Carotenoids and photosynthesis,” in Carotenoids in Nature, Subcellular Biochemistry, ed. C. Stange (New York, NY: Springer), 111–139. doi: 10.1007/978-3-319-39126-7_4
Heimann, K. (2016). Novel approaches to microalgal and cyanobacterial cultivation for bioenergy and biofuel production. Curr. Opin. Biotechnol. 38, 183–189. doi: 10.1016/j.copbio.2016.02.024
Hirokawa, Y., Kubo, T., Soma, Y., Saruta, F., and Hanai, T. (2020). Enhancement of acetyl-CoA flux for photosynthetic chemical production by pyruvate dehydrogenase complex overexpression in Synechococcus elongatus PCC 7942. Metab. Eng. 57, 23–30. doi: 10.1016/j.ymben.2019.07.012
Hirokawa, Y., Maki, Y., and Hanai, T. (2017). Improvement of 1,3-propanediol production using an engineered cyanobacterium, Synechococcus elongatus by optimization of the gene expression level of a synthetic metabolic pathway and production conditions. Metab. Eng. 39, 192–199. doi: 10.1016/j.ymben.2016.12.001
Hirokawa, Y., Maki, Y., Tatsuke, T., and Hanai, T. (2016). Cyanobacterial production of 1,3-propanediol directly from carbon dioxide using a synthetic metabolic pathway. Metab. Eng. 34, 97–103. doi: 10.1016/j.ymben.2015.12.008
Hitchcock, A., Hunter, C. N., and Canniffe, D. P. (2020). Progress and challenges in engineering cyanobacteria as chassis for light-driven biotechnology. Microb. Biotechnol. 13, 363–367. doi: 10.1111/1751-7915.13526
Hitchcock, A., Jackson, P. J., Chidgey, J. W., Dickman, M. J., Hunter, C. N., and Canniffe, D. P. (2016). Biosynthesis of chlorophyll a in a purple bacterial phototroph and assembly into a plant chlorophyll-protein complex. ACS Synth. Biol. 5, 948–954. doi: 10.1021/acssynbio.6b00069
Hohmann-Marriott, M. F., and Blankenship, R. E. (2011). Evolution of photosynthesis. Annu. Rev. Plant Biol. 62, 515–548. doi: 10.1146/annurev-arplant-042110-103811
Huang, H. H., Camsund, D., Lindblad, P., and Heidorn, T. (2010). Design and characterization of molecular tools for a synthetic biology approach towards developing cyanobacterial biotechnology. Nucleic Acids Res. 38, 2577–2593. doi: 10.1093/nar/gkq164
Huembelin, M., Beekwilder, M. J., and Kierkels, J. G. T. (2014). Rhodobacter for Preparing Terpenoids. Pat. WO 2014014339 A2 111111. Available online at: https://patentimages.storage.googleapis.com/cb/fc/28/187a051f51da61/WO2014014339A2.pdf (accessed August 11, 2020).
Imhoff, J. F. (2017). “Diversity of anaerobic anoxygenic phototrophic purple bacteria,” in Modern Topics in the Phototrophic Prokaryotes, ed. P. C. Hallenbeck (Cham: Springer), doi: 10.1007/978-3-319-46261-5
Jacobsen, J. H., and Frigaard, N. U. (2014). Engineering of photosynthetic mannitol biosynthesis from CO2 in a cyanobacterium. Metab. Eng. 21, 60–70. doi: 10.1016/j.ymben.2013.11.004
Jahn, M., Vialas, V., Karlsen, J., Maddalo, G., Edfors, F., Forsström, B., et al. (2018). Growth of cyanobacteria is constrained by the abundance of light and carbon assimilation proteins. Cell Rep. 25, 478.e8–486.e8. doi: 10.1016/j.celrep.2018.09.040
Jensen, P. E., and Leister, D. (2014). Cyanobacteria as an experimental platform for modifying bacterial and plant photosynthesis. Front. Bioeng. Biotechnol. 2:7. doi: 10.3389/fbioe.2014.00007
Johnston, T. G., Yuan, S. F., Wagner, J. M., Yi, X., Saha, A., Smith, P., et al. (2020). Compartmentalized microbes and co-cultures in hydrogels for on-demand bioproduction and preservation. Nat. Commun. 11:563. doi: 10.1038/s41467-020-14371-4
Joshi, H. M., and Tabita, F. R. (1996). A global two component signal transduction system that integrates the control of photosynthesis, carbon dioxide assimilation, and nitrogen fixation. Proc. Natl. Acad. Sci. U.S.A. 93, 14515–14520. doi: 10.1073/pnas.93.25.14515
Jung, E. E., Kalontarov, M., Doud, D. F. R., Ooms, M. D., Angenent, L. T., Sinton, D., et al. (2012). Slab waveguide photobioreactors for microalgae based biofuel production. Lab Chip 12, 3740–3745. doi: 10.1039/c2lc40490g
Kanno, M., Carroll, A. L., and Atsumi, S. (2017). Global metabolic rewiring for improved CO2 fixation and chemical production in cyanobacteria. Nat. Commun. 8, 1–11. doi: 10.1038/ncomms14724
Karagöz, P., Erhan, E., Keskinler, B., and Özkan, M. (2009). The use of microporous divinyl benzene copolymer for yeast cell immobilization and ethanol production in packed-bed reactor. Appl. Biochem. Biotechnol. 152, 66–73. doi: 10.1007/s12010-008-8336-7
Keskin, T., and Hallenbeck, P. C. (2012). Hydrogen production from sugar industry wastes using single-stage photofermentation. Bioresour. Technol. 112, 131–136. doi: 10.1016/j.biortech.2012.02.077
Khan, N. E., Nybo, S. E., Chappell, J., and Curtis, W. R. (2015). Triterpene hydrocarbon production engineered into a metabolically versatile host-Rhodobacter capsulatus. Biotechnol. Bioeng. 112, 1523–1532. doi: 10.1002/bit.25573
Knoot, C. J., Ungerer, J., Wangikar, P. P., and Pakrasi, H. B. (2018). Cyanobacteria: promising biocatalysts for sustainable chemical production. J. Biol. Chem. 293, 5044–5052. doi: 10.1074/jbc.R117.815886
Kobayashi, J., and Kondo, A. (2019). Disruption of poly (3-hydroxyalkanoate) depolymerase gene and overexpression of three poly (3-hydroxybutyrate) biosynthetic genes improve poly (3-hydroxybutyrate) production from nitrogen rich medium by Rhodobacter sphaeroides. Microb. Cell Fact. 18:40. doi: 10.1186/s12934-019-1088-y
Korosh, T. C., Markley, A. L., Clark, R. L., McGinley, L. L., McMahon, K. D., and Pfleger, B. F. (2017). Engineering photosynthetic production of L-lysine. Metab. Eng. 44, 273–283. doi: 10.1016/j.ymben.2017.10.010
Krinsky, N. I., and Deneke, S. M. (1982). Interaction of oxygen and oxy-radicals with carotenoids. J. Natl. Cancer Inst. 69, 205–210. doi: 10.1093/jnci/69.1.205
Kuo, F. S., Chien, Y. H., and Chen, C. J. (2012). Effects of light sources on growth and carotenoid content of photosynthetic bacteria Rhodopseudomonas palustris. Bioresour. Technol. 113, 315–318. doi: 10.1016/j.biortech.2012.01.087
Lan, E. I., and Liao, J. C. (2011). Metabolic engineering of cyanobacteria for 1-butanol production from carbon dioxide. Metab. Eng. 13, 353–363. doi: 10.1016/j.ymben.2011.04.004
Lan, E. I., Ro, S. Y., and Liao, J. C. (2013). Oxygen-tolerant coenzyme A-acylating aldehyde dehydrogenase facilitates efficient photosynthetic n-butanol biosynthesis in cyanobacteria. Energy Environ. Sci. 6, 2672–2681. doi: 10.1039/c3ee41405a
Larimer, F. W., Chain, P., Hauser, L., Lamerdin, J., Malfatti, S., Do, L., et al. (2004). Complete genome sequence of the metabolically versatile photosynthetic bacterium Rhodopseudomonas palustris. Nat. Biotechnol. 22, 55–61. doi: 10.1038/nbt923
Lea-Smith, D. J., Bombelli, P., Vasudevan, R., and Howe, C. J. (2016). Photosynthetic, respiratory and extracellular electron transport pathways in cyanobacteria. Biochim. Biophys. Acta Bioenerg. 1857, 247–255. doi: 10.1016/j.bbabio.2015.10.007
Levi, P. G., and Cullen, J. M. (2018). Mapping global flows of chemicals: from fossil fuel feedstocks to chemical products. Environ. Sci. Technol. 52, 1725–1734. doi: 10.1021/acs.est.7b04573
Li, X., Shen, C. R., and Liao, J. C. (2014). Isobutanol production as an alternative metabolic sink to rescue the growth deficiency of the glycogen mutant of Synechococcus elongatus PCC 7942. Photosynth. Res. 120, 301–310. doi: 10.1007/s11120-014-9987-6
Lin, P. C., Saha, R., Zhang, F., and Pakrasi, H. B. (2017). Metabolic engineering of the pentose phosphate pathway for enhanced limonene production in the cyanobacterium Synechocystis sp. PCC 6803. Sci. Rep. 7, 1–10. doi: 10.1038/s41598-017-17831-y
Lin, P. C., Zhang, F., and Pakrasi, H. B. (2020). Enhanced production of sucrose in the fast-growing cyanobacterium Synechococcus elongatus UTEX 2973. Sci. Rep. 10: 390. doi: 10.1038/s41598-019-57319-5
Liu, X., Sheng, J., and Curtiss, R. (2011). Fatty acid production in genetically modified cyanobacteria. Proc. Natl. Acad. Sci. U.S.A. 108, 6899–6904. doi: 10.1073/pnas.1103014108
Luan, G., Zhang, S., and Lu, X. (2020). Engineering cyanobacteria chassis cells toward more efficient photosynthesis. Curr. Opin. Biotechnol. 62, 1–6. doi: 10.1016/j.copbio.2019.07.004
Lüttge, U. (2011). “Regulation of photosynthetic light energy capture,” in Ecophysiology of Photosynthesis, eds E.-D. Schulze and M. M. Caldwell (Berlin: Springer Berlin Heidelberg), 17–44. doi: 10.1017/CBO9781139051477.008
Ma, L., Fang, Z., Wang, Y. Z., Zhou, J., and Yong, Y. C. (2020). Photo-driven highly efficient one-step CO2 biomethanation with engineered photo-synthetic bacteria Rhodopseudomonas palustris. ACS Sustain. Chem. Eng. 8, 9616–9621. doi: 10.1021/acssuschemeng.0c02703
Madigan, M. T., Martinko, J. M., Bender, K. S., Buckley, D. H., and Stahl, D. A. (2010a). “Evolution and the extent of microbial life,” in Brock Biology of Microorganisms, ed. M. T. Madigan (London: Pearson), 5–7.
Madigan, M. T., Martinko, J. M., Bender, K. S., Buckley, D. H., and Stahl, D. A. (2010b). “Phototrophy, chemolithotrophy, and major biosyntheses,” in Brock Biology of Microorganisms, ed. M. T. Madigan (London: Pearson), 340–371.
Marques, M. P. C., Cabral, J. M. S., and Fernandes, P. (2010). Bioprocess scale-up: quest for the parameters to be used as criterion to move from microreactors to lab-scale. J. Chem. Technol. Biotechnol. 85, 1184–1198. doi: 10.1002/jctb.2387
McBride, R. C., and Merrick, D. S. (2014). Catalyzing innovation: innovations in open pond algae agriculture for biofuel production. Ind. Biotechnol. 10, 162–163. doi: 10.1089/ind.2013.1614
McKinlay, J. B., and Harwood, C. S. (2010). Carbon dioxide fixation as a central redox cofactor recycling mechanism in bacteria. Proc. Natl. Acad. Sci. U.S.A. 107, 11669–11675. doi: 10.1073/pnas.1006175107
McKinlay, J. B., and Harwood, C. S. (2011). Calvin cycle flux, pathway constraints, and substrate oxidation state together determine the H2 biofuel yield in photoheterotrophic bacteria. mBio 2:e00323-10. doi: 10.1128/mBio.00323-10
Mellis, A. (1999). Photosystem-II damage and repair cycle in chloroplasts: what modulates the rate of photodamage in vivo? Trends Plant Sci. 4, 130–135. doi: 10.1016/S1360-1385(99)01387-4
Miao, R., Liu, X., Englund, E., Lindberg, P., and Lindblad, P. (2017). Isobutanol production in Synechocystis PCC 6803 using heterologous and endogenous alcohol dehydrogenases. Metab. Eng. Commun. 5, 45–53. doi: 10.1016/j.meteno.2017.07.003
Nielsen, J. (2001). Metabolic engineering. Appl. Microbiol. Biotechnol. 55, 263–283. doi: 10.1007/s002530000511
Nozzi, N. E., Case, A. E., Carroll, A. L., and Atsumi, S. (2017). Systematic approaches to efficiently produce 2,3-butanediol in a marine cyanobacterium. ACS Synth. Biol. 6, 2136–2144. doi: 10.1021/acssynbio.7b00157
Nwoba, E. G., Parlevliet, D. A., Laird, D. W., Alameh, K., and Moheimani, N. R. (2019). Light management technologies for increasing algal photobioreactor efficiency. Algal Res. 39:101433. doi: 10.1016/j.algal.2019.101433
Okubo, Y., Futamata, H., and Hiraishi, A. (2006). Characterization of phototrophic purple nonsulfur bacteria forming colored microbial mats in a swine wastewater ditch. Appl. Environ. Microbiol. 72, 6225–6233. doi: 10.1128/AEM.00796-06
Orsi, E., Beekwilder, J., Peek, S., Eggink, G., Kengen, S. W. M., and Weusthuis, R. A. (2020a). Metabolic flux ratio analysis by parallel 13C labeling of isoprenoid biosynthesis in Rhodobacter sphaeroides. Metab. Eng. 57, 228–238. doi: 10.1016/j.ymben.2019.12.004
Orsi, E., Beekwilder, J., van Gelder, D., van Houwelingen, A., Eggink, G., Kengen, S. W. M., et al. (2020b). Functional replacement of isoprenoid pathways in Rhodobacter sphaeroides. Microb. Biotechnol. 13, 1082–1093. doi: 10.1111/1751-7915.13562
Park, J., and Dinh, T. B. (2019). Contrasting effects of monochromatic LED lighting on growth, pigments and photosynthesis in the commercially important cyanobacterium Arthrospira maxima. Bioresour. Technol. 291:121846. doi: 10.1016/j.biortech.2019.121846
Pechter, K. B., Gallagher, L., Pyles, H., Manoil, C. S., and Harwood, C. S. (2016). Essential genome of the metabolically versatile alphaproteobacterium Rhodopseudomonas palustris. J. Bacteriol. 198, 867–876. doi: 10.1128/JB.00771-15
Pintucci, C., Giovannelli, A., Traversi, M. L., Ena, A., Padovani, G., and Carlozzi, P. (2013). Fresh olive mill waste deprived of polyphenols as feedstock for hydrogen photo-production by means of Rhodopseudomonas palustris 42OL. Renew. Energy 51, 358–363. doi: 10.1016/j.renene.2012.09.037
Qiao, C., Duan, Y., Zhang, M., Hagemann, M., Luo, Q., and Lu, X. (2018). Effects of reduced and enhanced glycogen pools on salt-induced sucrose production in a sucrose-secreting strain of Synechococcus elongatus PCC 7942. Appl. Environ. Microbiol. 84, 2023–2040. doi: 10.1128/AEM.02023-17
Ranaivoarisoa, T. O., Singh, R., Rengasamy, K., Guzman, M. S., and Bose, A. (2019). Towards sustainable bioplastic production using the photoautotrophic bacterium Rhodopseudomonas palustris TIE-1. J. Ind. Microbiol. Biotechnol. 46, 1401–1417. doi: 10.1007/s10295-019-02165-7
Rey, F. E., Heiniger, E. K., and Harwood, C. S. (2007). Redirection of metabolism for biological hydrogen production. Appl. Environ. Microbiol. 73, 1665–1671. doi: 10.1128/AEM.02565-06
Ruffing, A. M. (2014). Improved free fatty acid production in cyanobacteria with Synechococcus sp. PCC 7002 as host. Front. Bioeng. Biotechnol. 2:17. doi: 10.3389/fbioe.2014.00017
Sabourin-Provost, G., and Hallenbeck, P. C. (2009). High yield conversion of a crude glycerol fraction from biodiesel production to hydrogen by photofermentation. Bioresour. Technol. 100, 3513–3517. doi: 10.1016/j.biortech.2009.03.027
Salmon, R. C., Cliff, M. J., Rafferty, J. B., and Kelly, D. J. (2013). The CouPSTU and TarPQM transporters in Rhodopseudomonas palustris: redundant, promiscuous uptake systems for lignin-derived aromatic substrates. PLoS One 8:59844. doi: 10.1371/journal.pone.0059844
Sasikala, C., and Ramana, C. V. (1998). Biodegradation and metabolism of unusual carbon compounds by anoxygenic phototrophic bacteria. Adv. Microb. Physiol. 39, 368–377. doi: 10.1016/s0065-2911(08)60020-x
Schempp, F. M., Drummond, L., Buchhaupt, M., and Schrader, J. (2018). Microbial cell factories for the production of terpenoid flavor and fragrance compounds. J. Agric. Food Chem. 66, 2247–2258. doi: 10.1021/acs.jafc.7b00473
Scholes, G. D., Fleming, G. R., Olaya-Castro, A., and Van Grondelle, R. (2011). Lessons from nature about solar light harvesting. Nat. Chem. 3, 763–774. doi: 10.1038/nchem.1145
Seifert, K., Waligorska, M., and Laniecki, M. (2010a). Brewery wastewaters in photobiological hydrogen generation in presence of Rhodobacter sphaeroides O.U. 001. Int. J. Hydrogen Energy 35, 4085–4091. doi: 10.1016/j.ijhydene.2010.01.126
Seifert, K., Waligorska, M., and Laniecki, M. (2010b). Hydrogen generation in photobiological process from dairy wastewater. Int. J. Hydrogen Energy 35, 9624–9629. doi: 10.1016/j.ijhydene.2010.07.015
Stahl, W., and Sies, H. (2003). Antioxidant activity of carotenoids. Mol. Aspects Med. 24, 345–351. doi: 10.1016/S0098-2997(03)00030-X
Su, A., Chi, S., Li, Y., Tan, S., Qiang, S., Chen, Z., et al. (2018). Metabolic redesign of rhodobacter sphaeroides for lycopene production. J. Agric. Food Chem. 66, 5879–5885. doi: 10.1021/acs.jafc.8b00855
Swainsbury, D. J. K., Martin, E. C., Vasilev, C., Parkes-Loach, P. S., Loach, P. A., and Neil Hunter, C. (2017). Engineering of a calcium-ion binding site into the RC-LH1-PufX complex of Rhodobacter sphaeroides to enable ion-dependent spectral red-shifting. Biochim. Biophys. Acta Bioenerg. 1858, 927–938. doi: 10.1016/j.bbabio.2017.08.009
Swem, L. R., Gong, X., Yu, C. A., and Bauer, C. E. (2006). Identification of a ubiquinone-binding site that affects autophosphorylation of the sensor kinase RegB. J. Biol. Chem. 281, 6768–6775. doi: 10.1074/jbc.M509687200
Tiang, M. F., Fitri Hanipa, M. A., Abdul, P. M., Jahim, J. M. D., Mahmod, S. S., Takriff, M. S., et al. (2020). Recent advanced biotechnological strategies to enhance photo-fermentative biohydrogen production by purple non-sulphur bacteria: an overview. Int. J. Hydrogen Energy 45, 13211–13230. doi: 10.1016/j.ijhydene.2020.03.033
Troost, K., Loeschcke, A., Hilgers, F., Özgür, A. Y., Weber, T. M., Santiago-Schübel, B., et al. (2019). Engineered Rhodobacter capsulatus as a phototrophic platform organism for the synthesis of plant sesquiterpenoids. Front. Microbiol. 10:1998. doi: 10.3389/fmicb.2019.01998
Tsygankov, A., and Kosourov, S. (2014). “Immobilization of photosynthetic microorganisms for efficient hydrogen production,” in Microbial BioEnergy: Hydrogen Production, Advances in Photosynthesis and Respiration, eds D. Zannoni and R. De Philippis (Berlin: Springer), 321–347. doi: 10.1007/978-94-017-8554-9_14
Ungerer, J., Lin, P. C., Chen, H. Y., and Pakrasi, H. B. (2018). Adjustments to photosystem stoichiometry and electron transfer proteins are key to the remarkably fast growth of the Cyanobacterium Synechococcus elongatus UTEX 2973. mBio 9:e02327-17. doi: 10.1128/mBio.02327-17
Uyar, B., Eroglu, I., Yücel, M., Gündüz, U., and Türker, L. (2007). Effect of light intensity, wavelength and illumination protocol on hydrogen production in photobioreactors. Int. J. Hydrogen Energy 32, 4670–4677. doi: 10.1016/j.ijhydene.2007.07.002
Vajravel, S., Sirin, S., Kosourov, S., and Allahverdiyeva, Y. (2020). Towards sustainable ethylene production with cyanobacterial artificial biofilms. Green Chem. 22, 6404–6414. doi: 10.1039/d0gc01830a
Velmurugan, R., and Incharoensakdi, A. (2020). Heterologous expression of ethanol synthesis pathway in glycogen deficient Synechococcus elongatus PCC 7942 resulted in enhanced production of ethanol and exopolysaccharides. Front. Plant Sci. 11:74. doi: 10.3389/fpls.2020.00074
Wang, B., Eckert, C., Maness, P.-C., and Yu, J. (2017). A genetic toolbox for modulating the expression of heterologous genes in the cyanobacterium Synechocystis sp. PCC 6803. ACS Synth Biol 7, 276–286. doi: 10.1021/acssynbio.7b00297
Wang, B., Xiong, W., Yu, J., Maness, P.-C., and Meldrum, D. R. (2018). Unlocking the photobiological conversion of CO2 to (R)-3-hydroxybutyrate in cyanobacteria. Green Chem. 20:3772. doi: 10.1039/c8gc01208c
Wang, C., Zhao, S., Shao, X., Park, J., Bin, Jeong, S. H., et al. (2019). Challenges and tackles in metabolic engineering for microbial production of carotenoids. Microb. Cell Fact. 18, 1–8. doi: 10.1186/s12934-019-1105-1
Wang, G. S., Grammel, H., Abou-Aisha, K., Sägesser, R., and Ghosh, R. (2012). High-level production of the industrial product Lycopene by the photosynthetic Bacterium rhodospirillum rubrum. Appl. Environ. Microbiol. 78, 7205–7215. doi: 10.1128/AEM.00545-12
Wang, W., Liu, X., and Lu, X. (2013). Engineering cyanobacteria to improve photosynthetic production of alka(e)nes. Biotechnol. Biofuels 6, 1–9. doi: 10.1186/1754-6834-6-69
Wang, Y., Sun, T., Gao, X., Shi, M., Wu, L., Chen, L., et al. (2016). Biosynthesis of platform chemical 3-hydroxypropionic acid (3-HP) directly from CO2 in cyanobacterium Synechocystis sp. PCC 6803. Metab. Eng. 34, 60–70. doi: 10.1016/j.ymben.2015.10.008
Wendisch, V. F., Brito, L. F., Gil Lopez, M., Hennig, G., Pfeifenschneider, J., Sgobba, E., et al. (2016). The flexible feedstock concept in industrial biotechnology: metabolic engineering of Escherichia coli, Corynebacterium glutamicum, Pseudomonas, Bacillus and yeast strains for access to alternative carbon sources. J. Biotechnol. 234, 139–157. doi: 10.1016/j.jbiotec.2016.07.022
Winkelhausen, E., Velickova, E., Amartey, S. A., and Kuzmanova, S. (2010). Ethanol production using immobilized saccharomyces cerevisiae in lyophilized cellulose gel. Appl. Biochem. Biotechnol. 162, 2214–2220. doi: 10.1007/s12010-010-8995-z
Woolston, B. M., Edgar, S., and Stephanopoulos, G. N. (2013). Metabolic engineering: past and future. Annu. Rev. Chem. Biomol. Eng. 4, 259–288. doi: 10.1146/annurev-chembioeng-061312-103312
Wu, J., and Bauer, C. E. (2010). RegB kinase activity is controlled in part by monitoring the ratio of oxidized to reduced ubiquinones in the ubiquinone pool. mBio 1:e00272-10. doi: 10.1128/mBio.00272-10
Wu, X. M., Zhu, L. Y., Zhu, L. Y., and Wu, L. (2016). Improved ammonium tolerance and hydrogen production in nifA mutant strains of Rhodopseudomonas palustris. Int. J. Hydrogen Energy 41, 22824–22830. doi: 10.1016/j.ijhydene.2016.10.093
Xia, P. F., Ling, H., Foo, J. L., and Chang, M. W. (2019). Synthetic biology toolkits for metabolic engineering of cyanobacteria. Biotechnol. J. 14, 1–11. doi: 10.1002/biot.201800496
Xiong, J., and Bauer, C. E. (2002). Complex evolution of photosynthesis. Annu. Rev. Plant Biol. 53, 503–521. doi: 10.1146/annurev.arplant.53.100301.135212
Xu, W., Chai, C., Shao, L., Yao, J., and Wang, Y. (2016). Metabolic engineering of Rhodopseudomonas palustris for squalene production. J. Ind. Microbiol. Biotechnol. 43, 719–725. doi: 10.1007/s10295-016-1745-7
Yang, J., Yin, L., Lessner, F. H., Nakayasu, E. S., Payne, S. H., Fixen, K. R., et al. (2017). Genes essential for phototrophic growth by a purple alphaproteobacterium. Environ. Microbiol. 19, 3567–3578. doi: 10.1111/1462-2920.13852
Yim, H., Haselbeck, R., Niu, W., Pujol-Baxley, C., Burgard, A., Boldt, J., et al. (2011). Metabolic engineering of Escherichia coli for direct production of 1,4-butanediol. Nat. Chem. Biol. 7, 445–452. doi: 10.1038/nchembio.580
Zengler, K., Heider, J., Rosselló-Mora, R., and Widdel, F. (1999). Phototrophic utilization of toluene under anoxic conditions by a new strain of Blastochloris sulfoviridis. Arch. Microbiol. 172, 204–212. doi: 10.1007/s002030050761
Zhang, Y., Yang, H., Feng, J., and Guo, L. (2016a). Overexpressing F0/F1 operon of ATPase in Rhodobacter sphaeroides enhanced its photo-fermentative hydrogen production. Int. J. Hydrogen Energy 41, 6743–6751. doi: 10.1016/j.ijhydene.2016.03.061
Zhang, Y., Yang, H., and Guo, L. (2016b). Enhancing photo-fermentative hydrogen production performance of Rhodobacter capsulatus by disrupting methylmalonate-semialdehyde dehydrogenase gene. Int. J. Hydrogen Energy 41, 190–197. doi: 10.1016/j.ijhydene.2015.09.122
Zhou, J., Zhang, H., Zhang, Y., Li, Y., and Ma, Y. (2012). Designing and creating a modularized synthetic pathway in cyanobacterium Synechocystis enables production of acetone from carbon dioxide. Metab. Eng. 14, 394–400. doi: 10.1016/j.ymben.2012.03.005
Keywords: purple bacteria, cyanobacteria, metabolic engineering, biofilm, photosynthesis, bioprocess, waveguide, solar energy
Citation: Stephens S, Mahadevan R and Allen DG (2021) Engineering Photosynthetic Bioprocesses for Sustainable Chemical Production: A Review. Front. Bioeng. Biotechnol. 8:610723. doi: 10.3389/fbioe.2020.610723
Received: 27 September 2020; Accepted: 01 December 2020;
Published: 08 January 2021.
Edited by:
Jian-Ming Liu, Technical University of Denmark, DenmarkReviewed by:
Jianping Yu, National Renewable Energy Laboratory (DOE), United StatesNamita Khanna, Birla Institute of Technology and Science, India
Copyright © 2021 Stephens, Mahadevan and Allen. This is an open-access article distributed under the terms of the Creative Commons Attribution License (CC BY). The use, distribution or reproduction in other forums is permitted, provided the original author(s) and the copyright owner(s) are credited and that the original publication in this journal is cited, in accordance with accepted academic practice. No use, distribution or reproduction is permitted which does not comply with these terms.
*Correspondence: Radhakrishnan Mahadevan, a3Jpc2huYS5tYWhhZGV2YW5AdXRvcm9udG8uY2E=; D. Grant Allen, ZGdyYW50LmFsbGVuQHV0b3JvbnRvLmNh