Orally Administrable Therapeutic Nanoparticles for the Treatment of Colorectal Cancer
- 1The First Affiliated Hospital, Zhejiang University School of Medicine, Hangzhou, China
- 2National Health Commission (NHC), Key Laboratory of Combined Multi-Organ Transplantation, Hangzhou, China
- 3Key Laboratory of Organ Transplantation, Research Center for Diagnosis and Treatment of Hepatobiliary Diseases, Hangzhou, China
- 4Department of Medical Oncology, Sir Run Run Shaw Hospital, School of Medicine, Zhejiang University, Hangzhou, China
- 5Department of Colorectal Surgery, Sir Run Run Shaw Hospital, School of Medicine, Zhejiang University, Hangzhou, China
- 6Department of Oncology, The Second Affiliated Hospital of Soochow University, Suzhou, China
- 7Department of Oral Implantology and Prosthodontics, The Affiliated Hospital of Stomatology, School of Stomatology, Zhejiang University School of Medicine, Hangzhou, China
- 8Key Laboratory of Oral Biomedical Research of Zhejiang Province, Hangzhou, China
Colorectal cancer (CRC) is one of the most common and lethal human malignancies worldwide; however, the therapeutic outcomes in the clinic still are unsatisfactory due to the lack of effective and safe therapeutic regimens. Orally administrable and CRC-targetable drug delivery is an attractive approach for CRC therapy as it improves the efficacy by local drug delivery and reduces systemic toxicity. Currently, chemotherapy remains the mainstay modality for CRC therapy; however, most of chemo drugs have low water solubility and are unstable in the gastrointestinal tract (GIT), poor intestinal permeability, and are susceptible to P-glycoprotein (P-gp) efflux, resulting in limited therapeutic outcomes. Orally administrable nanoformulations hold the great potential for improving the bioavailability of poorly permeable and poorly soluble therapeutics, but there are still limitations associated with these regimes. This review focuses on the barriers for oral drug delivery and various oral therapeutic nanoparticles for the management of CRC.
Introduction
Colorectal cancer (CRC) is the third leading cause of cancer incidence and the second leading cause of cancer-related mortality, with 1.80 million new cases and approximately 900,000 deaths annually (Bray et al., 2018). Approximately 74% of CRC cases occur in the colon whereas 26% of CRC cases are reported in the rectum (Liu Y. et al., 2018). CRC is a malignant tumor derived from glandular cells and epithelial cells of the colon or rectum. Treatment approaches for CRC include surgical resection, local ablation, neoadjuvant chemotherapy, intra-arterial chemotherapy, adjuvant chemotherapy, radiotherapy, targeted therapy and immunotherapy (Punt et al., 2017; Dekker et al., 2019; Franke et al., 2019).
Chemotherapy remains a mainstay regimen for the management of CRC. In particular, the use of nanoparticles (NPs) has been widely successful for pharmaceutical delivery of active compounds (Pavitra et al., 2019). This approach enables higher intratumoral drug delivery and reduced systemic toxicity than free drug dosages (Omar et al., 2007; Pinto, 2010). Previous studies have explored various nanoparticle-based systems, such as liposomes, micelles, polymeric NPs, phytosomes, dendrimers, gold NPs, and magnetic NPs with the aim of improving drug aqueous solubility and achieving disease-specific drug delivery (Cisterna et al., 2016; Wong K. E. et al., 2019).
The oral route of drug administration is one of the most preferred approaches because of several advantages including: (1) It is the simplest, most convenient and safest way of drug administration; (2) It is painless and can be self-administered, thus improving patient compliance; (3) It is convenient for patients to repeat the medication for a long time; (4) No special knowledge or special supplies are required; (5) No sterile precautions are required; (6) Extra economic cost of adjuvant drugs is low; and (7) An acute reaction of the drug is less likely to happen (Wang S. et al., 2015; Griffin et al., 2016; Jain and Jain, 2016; Alavian and Shams, 2020). Furthermore, a wide range of drug molecules, from small molecular compounds to large biomacromolecules, are tolerable in this administration route. More importantly, oral route is highly attractive for local drug delivery, especially for many diseases localized in the gastrointestinal tract (GIT).
Despite significant advances made in this field, oral drug administration for CRC therapy remains a big challenge. The efficacy of oral formulations has been generally compromised by several limitations, such as drug poor solubility, poor permeability, rapid degradation in the GIT, and the inability to penetrate and low adsorption across the mucosal barrier (Hua, 2014). In this review, we provide an updated summary and prospects of oral drug delivery systems for treatment of CRC.
Background of Colorectal Cancer
Colorectal cancer (CRC) is a common and one of the leading causes of cancer-related deaths. High incidence of CRC is associated with lifestyle changes (Brody, 2015). Advances in colorectal cancer screening approaches significantly reduce the incidence and mortality of CRC in developed countries. In addition, the mortality and incidence of CRC can be significantly reduced by implementing population-based screening programs (Feng et al., 2019). However, in some developing countries, incidence and death rates of CRC continue to rise due to westernized lifestyles, intake of low-fiber and high fat diet, smoking, heavy alcohol intake, obesity and reduced physical activity among the population (Wong M. C. et al., 2019; Siegel et al., 2020). CRC is characterized by late diagnosis and poor treatment due to high rates of metastatic burden as well as chemoresistance (Arvelo et al., 2015).
Colon and rectum play important roles in digestion by aiding absorption of water, minerals, nutrients and storage of wastes. CRC develops in a stepwise manner. Mutations of critical genes in neoplastic cells acquire survival advantage over normal epithelial cells (Vermeulen et al., 2013). These mutations induce the precancerous tissue to evolve to adenomas and then to carcinomas. Molecular mechanisms involving the occurrence and development of CRC are summarized previously (Markowitz and Bertagnolli, 2009). These mutations happen sequentially during progression of CRC and are associated with the stages of tumor development and histopathology (Fearon and Vogelstein, 1990). Notably, chronic intestinal inflammation is a major risk factor. Figure 1 summarizes the relationship between inflammation and CRC (West et al., 2015). Inflammation has many opposing effects on the development of CRC. Inflammation can induce anti-tumor effect in intestines, which is mediated by several immune cells including dendritic cells that recognize and present antigens, B cells and T cells. In addition, non-specific immunity orchestrated by natural killer cells, neutrophils, Treg cells and γδ T cells contributes to anti-tumor effects of inflammation. On the other hand, inflammation can promote tumor development. Tumor-associated immune cells in the tumor microenvironment (TME) trigger the production of several inflammatory factors which promote the proliferation, invasion, epithelial-mesenchymal transition, metastasis, angiogenesis and other processes in tumor cells hence enhancing the progression of tumors. In addition to environmental and genetic factors, studies showed that gut microbiota play a key role in shaping the inflammatory environment and promoting development of CRC (Brennan and Garrett, 2016).
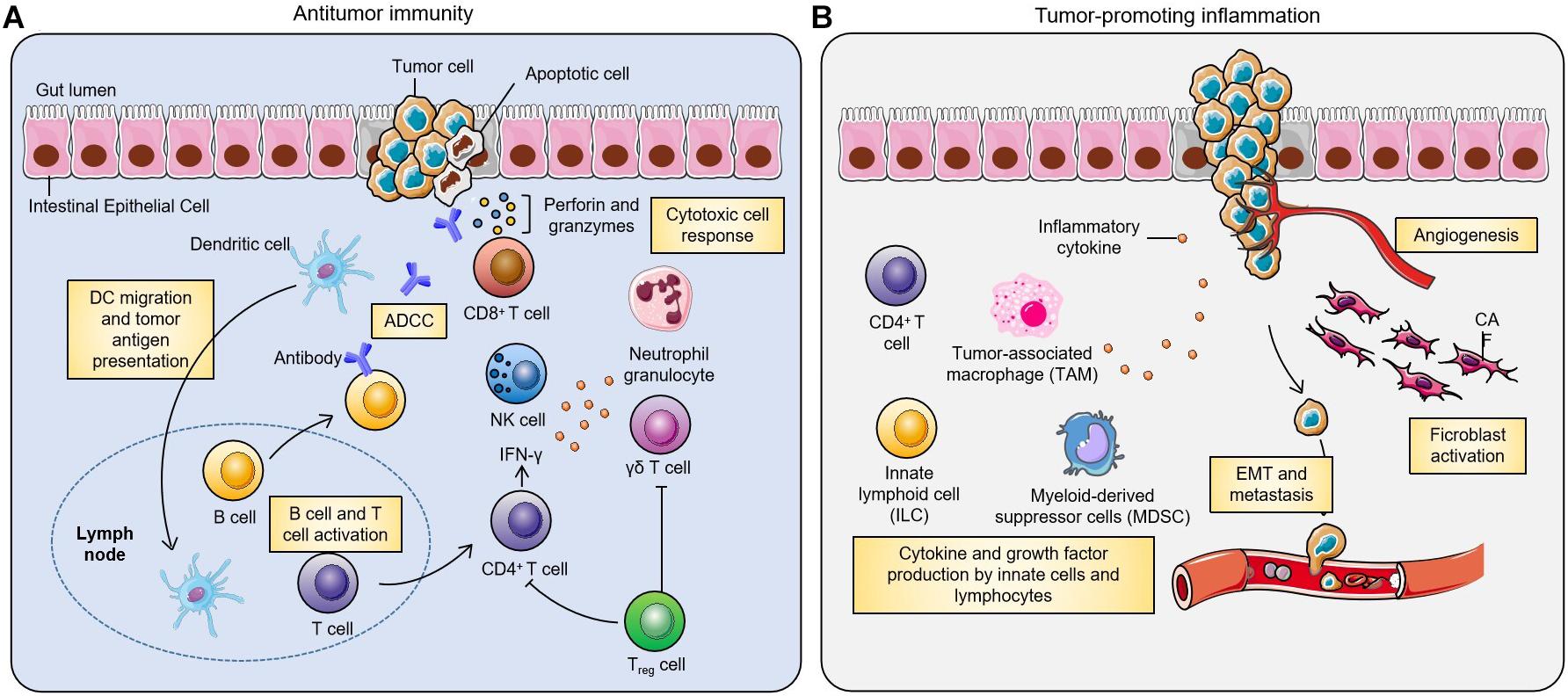
Figure 1. Inflammation is a double-edged sword in development of colorectal cancer (West et al., 2015). Inflammation plays a critical role in the pathogenesis of CRC. (A) In anti-tumor immunity, dendritic cells can recognize and present tumor antigens and activate tumor-specific B cells and T cells. Traditionally, effective anti-tumor immunity is mainly mediated by CD4+ T cells, CD8+ T cells and natural killer cells (NK cells). There is growing evidence that γδ T cells are also involved in anti-tumor immunity, and that Treg cells can restrain this process. (B) In addition, inflammation can also promote tumor development. CD4+ T cells, innate lymphoid cells and tumor-associated macrophages in the tumor microenvironment can induce the production of a variety of inflammatory cytokines, which lead to tumor proliferation, invasion, angiogenesis, epithelial-mesenchymal transition and metastasis.
Traditional chemotherapy for treatment of CRC has limited efficacy due to its toxicity and side effects on normal tissues (Prados et al., 2013). Therefore, CRC treatments are in urgent need of safe and effective new therapies. NP-mediated oral systems can enhance the drug efficacy and reduce side effects over their free drug forms (Hu et al., 2010). Use of NPs as delivery vehicles holds several advantages: (1) NP encapsulation can improve drug solubility and stability under harsh GIT conditions; (2) NP formulation could extend the half-life of drug payloads in the blood circulation (Fearon and Vogelstein, 1990); (3) NPs have enhanced permeability and retention (EPR) effect in tumor lesions, hence passive targeting capacity compared with free drugs, which is expected to augment intratumoral drug accumulation which is essential for high efficacy (Wang et al., 2012); (4) some NP strategies have been developed to subvert drug resistance mechanisms in cancer cells, thus significantly reducing the concentration of drugs required for treatment (Beretta and Cavalieri, 2016); (5) some therapeutics such as nucleic acids can be formulated in NPs to improve the stability and avoid in vivo degradation (Mokhtarzadeh et al., 2019); (6) NPs can be designed to target specific sites and encapsulate multiple drugs to produce good therapeutic effects (Banerjee and Sengupta, 2011; Blanco et al., 2011); and (7) toxicity can be reduced by reducing systemic drug exposure. Currently, numerous NPs that target cancer cells or TME have been examined for efficacy testing against preclinical CRC models (Table 1); however, most of them are not clinically approved.
Barriers for Oral Administration of Anticancer Nanotherapeutics
Drugs previously used for CRC treatment are either invasive or non-selective. Some nanosystems that allow oral administration have been developed. Oral administration is able to increase patient compliance and decreases side effects. An ideal orally administered drug for CRC therapy should reach the colon and rectum, specifically accumulate at the lesion sites, and also be deliverable to metastatic lesions through systemic circulation (Hua, 2014). However, NPs take long time before approaching the colon and rectum, including gastropore, which limits oral efficacy (Wahlgren et al., 2019).
Oral drug delivery poses three barriers occurred in the GIT (O’Driscoll et al., 2019): enzymatic barrier that may cause degradation of drug molecules, mucus barrier that may trap hydrophilic molecules in the mucus, and membrane barrier that may prevent drugs from entering enterocytes. In this section, we discuss the limitations regarding the NP delivery. The transit time of NPs along the GIT is influenced by several physiological factors including pH, mucosal layer, and microbiota (Figure 2; Hua, 2020). These factors also affect drug adsorption.
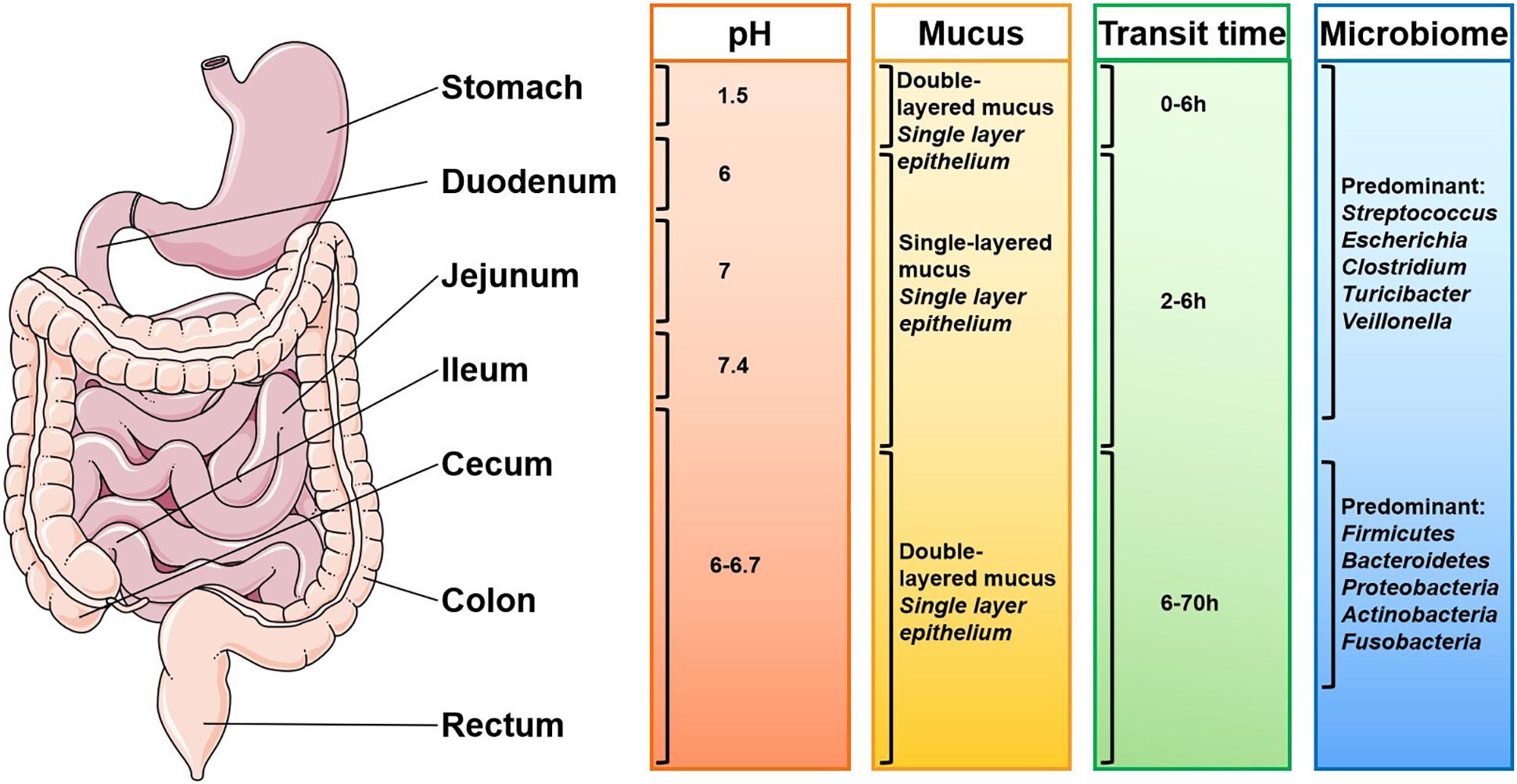
Figure 2. Gastrointestinal physiological factors that influence oral drug delivery (adapted from Hua, 2020). The complex gastrointestinal (GI) environment of human beings and the differences in different individuals bring challenges to the delivery of nanoparticles. The pH of the GI tract, composition of the mucosa, microorganisms and enzymes, and the residence time of the drugs in different parts of the GI tract all affect the drug delivery effect.
Stability
Upon orally administered, the stability of NPs and relevant drug payloads remains a crucial factor because of the acidic pH conditions in the stomach (1.0–2.5). Ideal oral NPs should be stable in the gastric fluid for effective delivery to the small intestine, colon and rectum (Homayun et al., 2019). After leaving the stomach, NPs are transported along the duodenum, jejunum, and ileum (pH value changes from 6 to 7.4), then through the caecum (pH = 5.7) and rectum (pH = 6.7) (Fallingborg, 1999). In addition, gastrointestinal protease and lipase act on drugs, thus affecting their activity. Liposomes are susceptible to combined effects of gastric acid, bile salt and pancreatic lipase in the GIT, resulting in reduced concentration of intact liposomes and payload leakage (Hu et al., 2013). Moreover, the gut especially the colon is rich in microbiome which may lower drug activity. Furthermore, hydrolases and metabolic enzymes in bacteria may degrade drugs and affect their stability (Rowland, 1988; Sinha and Kumria, 2001).
Permeability
NPs are absorbed from the intestinal lumen into the bloodstream of target tissues through endocytosis/pinocytosis or via carrier-mediated transport. Thus, the gastrointestinal mucosal barrier is an additional obstacle that impairs the effective uptake of NPs. Small intestine is the most permeable region of GIT because of its larger absorptive surface and leaky paracellular channels (Ritschel, 1991). Therefore, oral NPs need to be targeted to the small intestine for maximum absorption. In addition, drugs encounter hepatic barrier after entering blood vessels of intestinal epithelium (Homayun et al., 2019).
Transport
NPs ensures specific drug delivery to the lesion site of the colon and rectum. There are three major pathways for NPs to move from the apical side to the basal side of cells, including: paracellular pathway through tight junctions, transenterocytic pathway and M-cell-mediated pathway (Yu et al., 2016). Transenterocytic pathway comprises uptake, endocytosis, transport within the cell and exocytosis from the interior of the cell, thus it is the most effective pathway (Reinholz et al., 2018). However, in the endolysosomal uptake pathway, NPs are trapped successively in endosomes and lysosomes, and they are degraded by enzymes or infinitely accumulated in the lysosomes, thus affecting their transport.
To overcome these barriers, traditional NPs need be rationally re-engineered. For example, coating the particle surface with a suitable layer or storing them in an airtight container may help to augment the stability of therapeutic NPs against degradation by the harsh conditions in GIT (Xu L. et al., 2018). Moreover, surface modification of nanoparticles not only increases their stability, adhesion and permeability, but also improves their sustained and controllable drug release properties.
Another aspect through which the transport of NPs is impaired is via the degradation by lysosomes in epithelial cells. Some strategies have been developed to prevent endo/lysosomal damage (Martens et al., 2014; Selby et al., 2017), such as surface modification of NPs with endosomal escape agents (HA2 peptides) (Xu Y. et al., 2018). Furthermore, to achieve targeted transport and drug release from NPs, many multifunctional NPs with improved properties such as active targeting, good aggregation, cellular uptake into lesion sites, pH sensitivity and thermal sensitivity have been developed (Blanco et al., 2015). Recent advances in material science have led to some new strategies balancing the degradation and drug release kinetics. Moreover, the size, shape and charge of NPs can be manipulated to improve the stability and efficiency of drug delivery systems.
Applications of Oral Anti-CRC Nanotherapeutics
Several oral NPs are under development or in clinical trials. NPs are characterized by high solubility, high stability, controlled release, improved pharmacokinetics, and preferential accumulation in tumor tissues (Dadwal et al., 2018). The following section summarizes oral NPs developed for the treatment of CRC and classifies them into several types: lipid-based NPs, polymeric NPs, inorganic NPs, ligand-conjugated NPs, plant-derived NPs and stimuli-responsive NPs.
Lipid-Based Nanoparticles
Lipid-based nanoparticles (LNPs), especially liposomes, are promising platforms for cancer therapy, due to their good biodegradability, biocompatibility, structural simplicity, and tailorable functionality (Tang W. L. et al., 2018). LNPs can be basically divided into the following seven categories: liposome, core-shell NP, micelle, solid lipid nanoparticle (SLN), nanodisc and cubosome. The surface of liposomes can be modified by incorporating various functional motifs. LNPs are widely used not only because of their varieties, but also because they can be loaded with a variety of hydrophobic and hydrophilic therapeutic agents including chemotherapeutics, peptides, proteins, DNA, and RNA as shown in Figure 3 (Yang and Merlin, 2020). In this section, we focused on the current advances and applications of solid lipid NPs and liposomes.
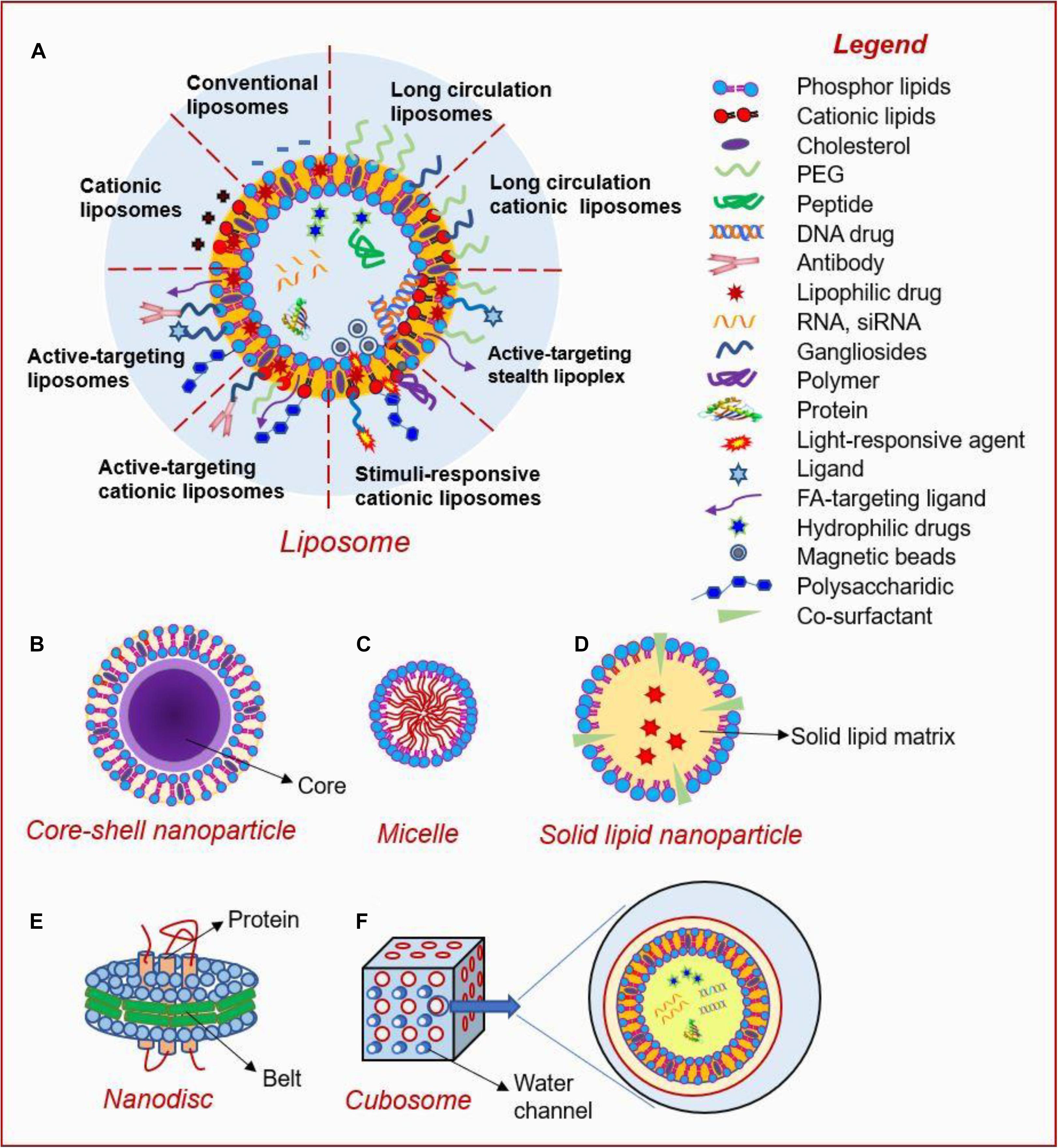
Figure 3. Types and structures of lipid-based nanoparticles (Yang and Merlin, 2020). Current lipid-based nanoplatforms include: (A) liposome, (B) core-shell nanoparticle, (C) micelle, (D) solid lipid nanoparticle, (E) nanodisc, and (F) cubosome.
Solid lipid NPs comprise lipids with low melting point, and varieties of surfactants and/or co-surfactants (Tapeinos et al., 2017). Selection and ratio of lipid and surfactant types determine the size, potential, stability, and drug loading and release profile of lipid NPs.
Solid lipid NPs are used to improve delivery of drugs with poor water solubility. For example, quercetin, an antioxidant found in onions, shows potent anti-tumor activities against CRC; however, it has poor water solubility. Li et al. (2009) designed quercetin-loaded solid lipid nanoparticles (QT-SLNs) using emulsification and low-temperature solidification method. The QT-SLNs showed longer Tmax and mean residence time (MRT), and improved relative bioavailability, implying that SLNs are effective oral delivery carriers for these hydrophobic agents.
Similarly, Shen et al. (2019) developed doxorubicin and superparamagnetic iron oxide NPs-loaded SLN delivery system and decorated the surface of SLNs with folate (FA) and dextran. Modification of these SLNs improved targeting capacity to cancer cells and facilitated its cellular uptake. In vivo experiments showed that the SLNs effectively inhibited primary tumor and metastatic burden, with lower systemic toxicity.
Liposomes can be classified into three types based on size and lamellarity. These classes include: small unilamellar vesicles (SUVs), large unilamellar vesicles (LUVs), and multilamellar vesicles (MLVs) (Yingchoncharoen et al., 2016). Particle sizes of SUVs range from 25 to 50 nm, and LUVs are larger than 100 nm in size, whereas sizes of MLVs ranges between 0.05 and 10 μm and are composed of multilayer phospholipid bilayer. In general, hydrophobic drugs are located in the phospholipid bilayer of the liposomes, whereas hydrophilic drugs are non-covalently trapped in the liposome cavity.
Batist et al. (2009) designed a novel liposome-encapsulated formulation of irinotecan and floxuridine, named CPX-1, and optimized the drug ratios to achieve therapeutic synergy. The codelivery system not only effectively maintained the high drug concentrations in blood circulation after systemically administered, but also showed high anti-tumor activity in CRC patients. Currently, a phase II trial for evaluating efficacy and safety of CPX-1 for CRC treatment is ongoing.
The therapeutic outcomes of several liposome-based chemotherapies are currently under clinical trials. OSI-7904L, a liposomal formulation of a non-competitive thymidylate synthase inhibitor (TSI), was designed for use in patients with advanced solid tumors (Beutel et al., 2005; Clamp et al., 2008; Ricart et al., 2008). In addition, liposomal DACH platinum L-NDDP, a liposomal formulation of cis-bis-neodecanoato-trans-R, R-1,2-diaminocyclohexane platinum (II) showed excellent anti-tumor activity in patients (Dragovich et al., 2006).
In addition, some natural lipid molecules have been used for drug derivatization and lipid nanoparticle preparation. For example, covalent conjugation of squalene (a natural lipid precursor) with different biologically active molecules enabled the squalenoylated prodrugs to self-assemble in aqueous media. Using this technology, Kotelevets et al. (2017) developed squalene-based nanoparticles loaded with cisplatin (SQ-CDDP NP) for oral delivery, thus significantly improving cisplatin efficacy. Treatment of CRC with cisplatin is associated with severe adverse effects and high risk of drug-resistance (Saber et al., 2018). The use of SQ-CDDP NPs enhanced the drug activity relative to its free drug form and circumvented the drug resistance mechanisms, partly due to facilitated cellular uptake. Inspired by these works, our group developed “PUFAylation” technology (Wu et al., 2020). Briefly, this strategy linked chemical drugs to polyunsaturated fatty acids (PUFAs). Such prodrug could self-assemble in aqueous media without exogenous excipients, hence provided effective anti-tumor effects.
In conclusion, lipid-based NPs are among the most promising drug delivery platforms given their biocompatibility, biocompatibility and extended functionality. They improve drug solubility, delivery efficiency, safety and efficacy making them ideal for cancer treatment.
Polymeric Nanoparticles
Polymeric materials have been used in various pharmaceutical and biotechnology products for over 50 years. Polymer NPs for drug delivery have several advantages including: (1) high in vitro and in vivo stability, (2) ability to achieve targeted release in different parts of the body, (3) ability to load DNA, RNA and proteins and prevent their degradation, (4) ease of modification by ligands (Elsabahy and Wooley, 2012; Kamaly et al., 2012; Bao et al., 2013; Banik et al., 2016). Some physiochemical properties of therapeutic delivery systems such as, stealth, charge, targeting, stimuli-responsiveness, particle size, morphology, aspect ratio core vs. shell and length vs. diameter, assembly vs. unimolecular and stability can be tailored to make them more effective and reduce their toxic effects (Figure 4; Elsabahy and Wooley, 2012). For instance, in terms of charge, cationic NPs which exhibit high cellular uptake, high cytotoxicity, and low specificity have been developed. In this section, several classes of polymer materials are described.
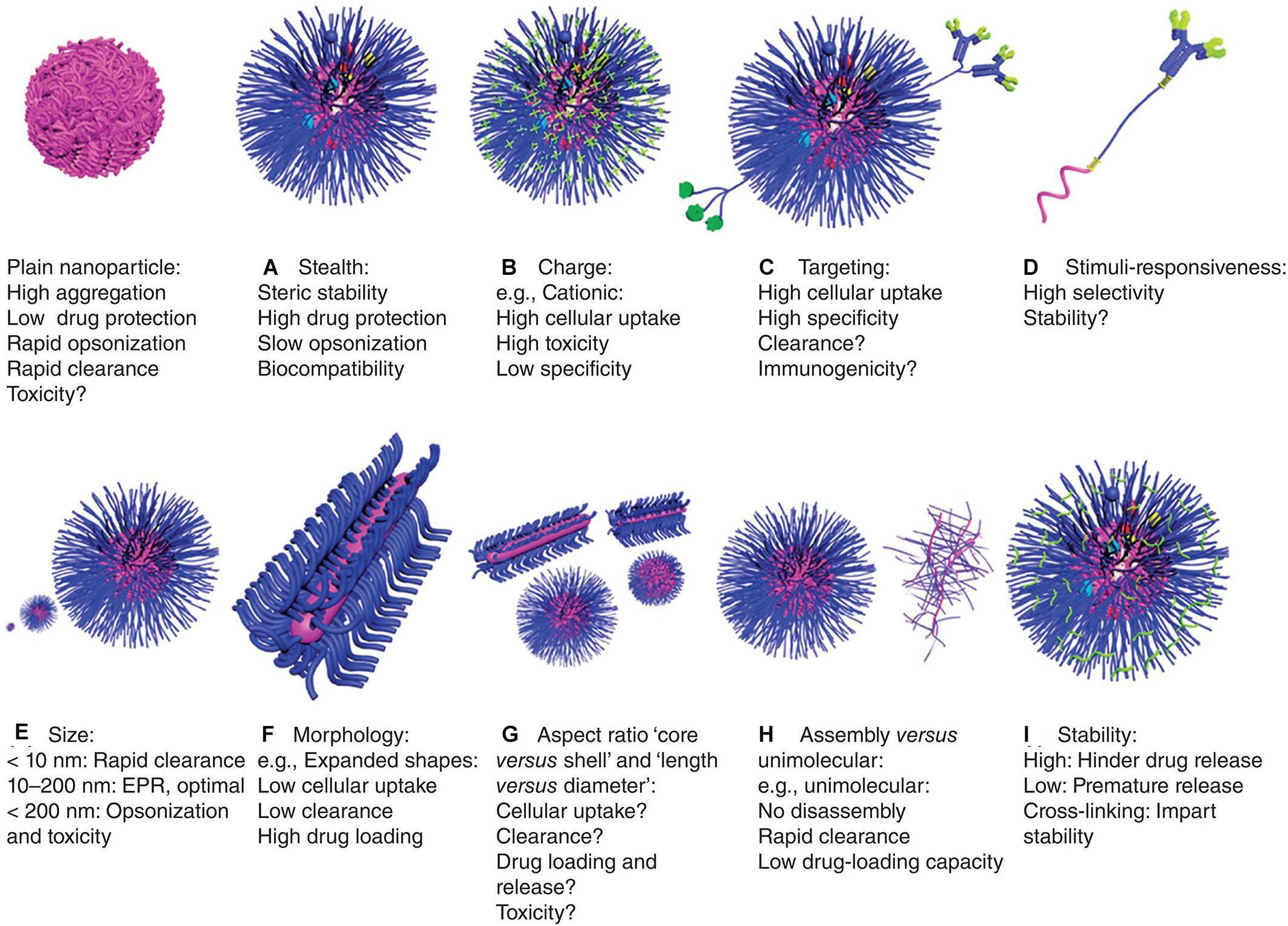
Figure 4. Characteristics of polymeric nanoparticles (Elsabahy and Wooley, 2012). The characteristics of polymer nanoparticles have great impact on their behavior in vitro and in vivo, including: (A) stealth: they have biocompatibility and stability, encapsulating drugs, which protect the nanoparticles from clearance by cells, but it may also reduce cell uptake and endosomal escape, (B) charge: cationic property can enhance cell uptake and endosomal escape, but their tissue distribution and toxicity are uncontrolled, (C) targeting: they have enhanced cellular uptake and specificity, but sometimes with accelerated clearance and/or immunogenicity, (D) stimuli-responsiveness: they can release drugs selectively at specific sites, but the stability and responsiveness of the materials may differ in physiological and pathological conditions, leading to premature drug release, (E) size: a size of about 100 nm is optimal for drug delivery, which is large enough to avoid being cleared by the kidneys, and small enough to reduce cell clearance and toxicity, (F) morphology: expanded morphology improves drug-loading capacity and reduces cellular uptake and clearance, (G) aspect ratio: the volume, length and diameter ratio of shell vs. core can greatly affect drug delivery efficiency and toxicity, (H) assembly vs. unimolecular structures: unimolecular structures are relatively more stable, but have poor drug-loading capacity and can be easily cleared, and (I) stability: stability of nanoparticles in circulation and physiological barriers and release at specific sites can be achieved by means of cross-linking.
Chitosan is a bioactive polymer, which is produced by alkaline deacetylation of chitin, a component of the exoskeleton of crustaceans (Prabaharan, 2015). It is widely used for drug conjugation or particle surface cloaking due to its high biocompatibility, biodegradability, cell membrane penetrability, low immunogenicity and low toxicity characteristics. Chitosan NPs are positively charged and can easily contact with cell membranes, which may facilitate the NP internalization into cancer cells (Narayanan et al., 2014; Zhang E. et al., 2019). Urbanska et al. (2012) encapsulated oxaliplatin in pH sensitive alginate microspheres with mucoadhesive chitosan coated. Oxaliplatin was loaded into NPs composed of lipid like polymeric molecules, and then these NPs were encapsulated in micro-sized alginate-based particles. The microparticles effectively passed through the stomach and targeted the intestinal tract, thus effectively alleviating tumor progression and morbidity. Hosseinzadeh et al. (2012) developed gemcitabine-loaded NPs through ionic gelation method using chitosan and Pluronic® F-127 as a carrier. The gemcitabine-loaded NPs showed controlled release profile and had significantly higher cytotoxicity effect compared with free drugs. Apart from these chemotherapeutic agents, chitosan NPs can also be used to deliver natural molecules. For example, curcumin-loaded NPs were previously prepared in a similar manner as gemcitabine-loaded NPs (Chuah et al., 2013). Besides, Wen et al. (2018) developed core-sheath electrospun fiber mat containing quercetin-loaded chitosan NPs (Q-loaded EFM), which improved oral bioavailability and colonic targeting of quercetin, a bioactive flavonoid which can inhibit the progression of cancers (Rauf et al., 2018).
The development of CRC is often associated with chronic inflammation. Therefore, chitosan NPs can also be used to simultaneously co-deliver anti-inflammatory drugs and chemotherapies. Han et al. (2019) chemically engineered curcumin (CUR) and 7-ethyl-10-hydroxycamptothecin (SN38) into hydrophilic mucoadhesive chitosan to generate chitosan-drug amphiphiles for treatment of inflammatory bowel diseases (IBDs) and colitis-associated colorectal cancer (CAC) as shown in Figure 5. In this study, CUR and SN38 were attached to carboxylated chitosan by the hydrolyzable ester bond. The attachment of hydrophobic agents rendered the conjugates with the ability to self-assemble into stable and bioadhesive NPs for oral administration. Upon oral gavage, these NPs effectively accumulated in inflamed tissues and tumor sites, and adhered closely to intestinal villi. Subsequently, the NPs released CUR and SN38, exerting anti-inflammatory and anti-tumor effects, respectively.
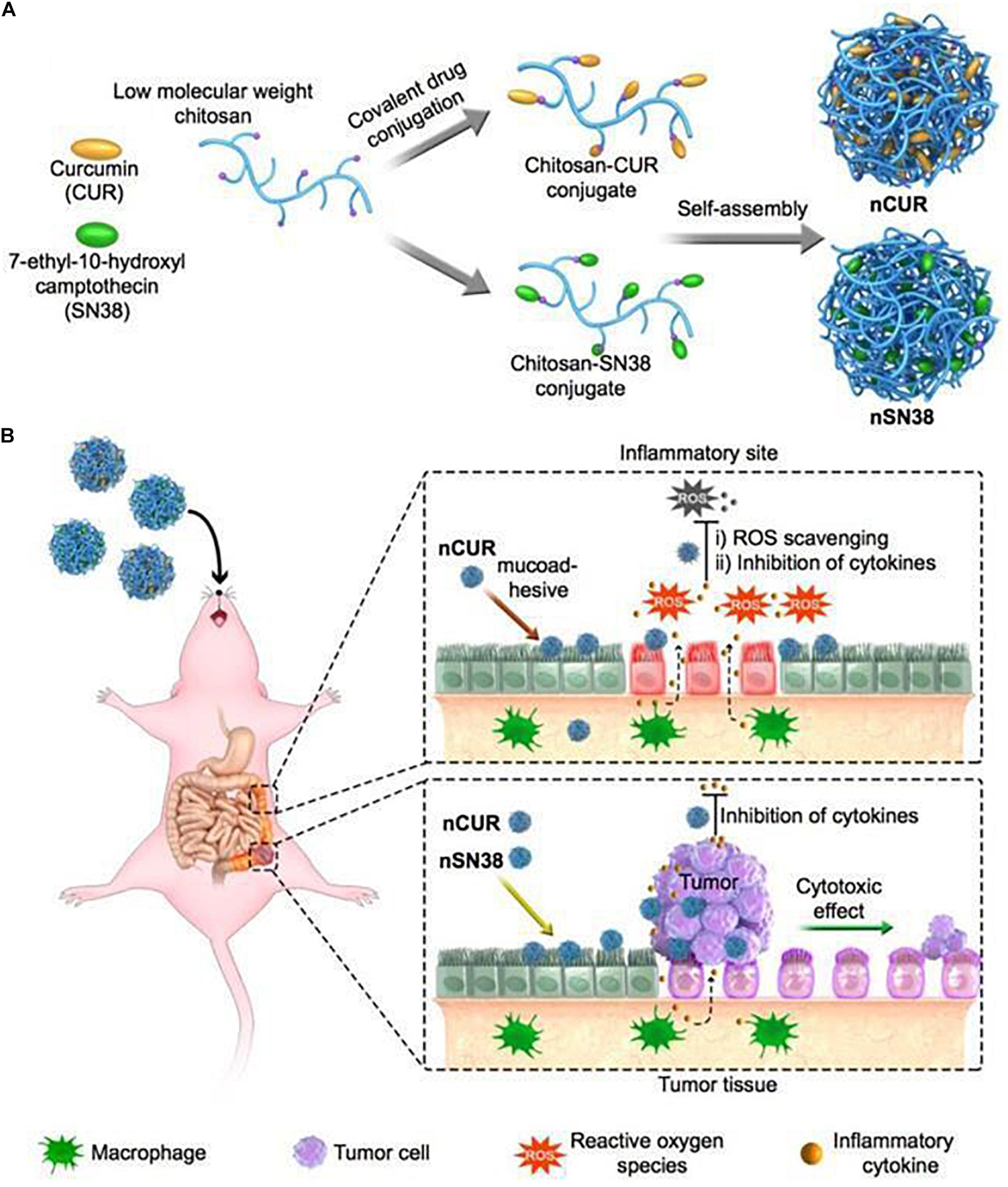
Figure 5. A scheme of self-assembly of chitosan-drug conjugates to form NPs and their oral administration for CAC treatment (Han et al., 2019). (A) Curcumin and SN38 were individually tethered to carboxylated chitosan by a self-hydrolyzable ester linkage. This formed amphiphile construct could be self-assembled in aqueous media and was suitable for oral administration. (B) Using a preclinical CAC mouse model, the anti-inflammatory nCUR and cytotoxic nSN38 nanoparticles could accumulate and release drugs in inflamed intestinal tissues and tumor tissues after oral administration, acting as an inhibitor of inflammation and tumor growth.
Poly (D,L-lactic-co-glycolic acid) (PLGA)-based nanocarriers have been used for drug delivery. PLGA-based NPs are characterized by good biocompatibility and controlled biodegradation for effective delivery of drugs and macromolecules (Dechy-Cabaret et al., 2004). Yang et al. (2020) prepared PLGA/PLA-PEG-FA NPs (NP-PEG-FA/17-AAG) which improved the oral bioavailability of 17-AAG, an inhibitor of HSP that effectively treats ulcerative colitis and CAC. Results from the study indicated that NP-PEG-FA/17-AAG was effectively internalized by the inflamed bowel tissue of the mice, resulting in higher therapeutic efficacy, even at low doses.
Poly(ethylene glycol)-block-poly(D,L-lactic acid) (PEG-PLA) is a di-block copolymer. Many drugs can be tethered to the polymers and form polymer-drug conjugates. The amphiphilicity endowed by this approach makes them self-assembling in aqueous medium. TNP-470, an analog of fumagillin, is one of the first antiangiogenic drugs to undergo clinical trials. Clinical use of TNP-470 is limited due to poor oral availability and extremely short plasma half-life. Benny et al. (2008) developed an oral formulation of TNP-470, named Lodamin. TNP-470 was conjugated to PEG-PLA, forming polymeric nanomicelles, which had anti-tumor and anti-metastasis effects. Cell experiments showed that Lodamin was taken up by endothelial cells through endocytosis and retained its original anti-angiogenesis activity.
Several other kinds of materials have also been developed for preparation of orally deliverable NPs. For example, eudragit S100 is a pH-responsive enteric material used for site-specific delivery. 5-fluorouracil (5-FU) is one of the first-line drugs used for the treatment of CRC. 5-FU was originally administered intravenously; however, it is degraded rapidly in systemic circulation. In some studies, 5-FU was modified in different platforms for oral delivery to improve its anti-tumor effect and reduce toxicity (Miura et al., 2017). In a study designed by Subudhi et al. (2015), 5-FU was encapsulated into Eudragit S100 coated Citrus Pectin Nanoparticles (E-CPNs) to improve oral delivery of 5-FU. The E-CPNs released 5-FU in a pH-dependent manner and showed high safety, specificity and effectiveness in vivo. Moreover, citrus pectin, a ligand of galectin-3 receptors that is overexpressed on CRC cells, enhances the ability to target cancer cells. Additionally, irinotecan-loaded microbeads were designed using folic acid-grafted solid lipid nanoparticles and coated with Eudragit S100 to achieve pH-responsive drug release (Rajpoot and Jain, 2020).
Poly(acrylic acid) (PAA) is another pH-responsive polymer commonly used to improve water solubility of materials. Tian et al. (2017) capped mesoporous silica SBA-15 with PAA through a facile graft-onto approach. In this delivery system, SBA-15 acted as a gatekeeper which controlled drug transport in and out of the pore channels. By loading DOX into PAA/SBA-15, water solubility of DOX was significantly increased by loading DOX into PAA/SBA-15. In the stomach (pH = 2.0), DOX molecules were encapsulated in the pore channels because the pore outlets were capped with collapsed PAA. However, in the colon (pH = 7.6), it showed rapid release due to removal of the capping.
Inorganic Nanoparticles
Inorganic NPs, such as Metal NPs and Silica NPs, can be used as drugs, imaging agents, gene carriers, sensors and antiseptics (Sekhon and Kamboj, 2010). Advances in inorganic NPs in biomedicines provides a promising way to develop novel imaging and drug delivery systems as these NPs can be used for a variety of applications (Tee et al., 2019). Based on materials used and shapes, inorganic nanoparticles can be classified into: spherical gold, gold nanorod, gold nanocage, gold nanoshell, silver NPs, hafnium oxide, gadolinium oxide, mesoporous silica NPs, quantum dots, iron oxide, carbon nanotubes and carbon dots. A typical inorganic NP can be divided into three parts: an inorganic core, the engineered surface coating and a shell of adsorbed biological molecules. The fate of typical inorganic NPs after being administrated is shown in Figure 6 (Feliu et al., 2016). In short, plain inorganic cores, if not coated with an organic layer, will agglomerate under physiological environments. NPs coated with organic layers exhibit better biocompatibility and have good cell adhesion and cellular uptake properties. Once internalized, the physical and morphological characteristics of NPs are altered and their inorganic core is degraded. The organic layer can also be cleared by intracellular degradation or protein corona modification. In this section, we described the design and modification of metal NPs and silica NPs in detail.
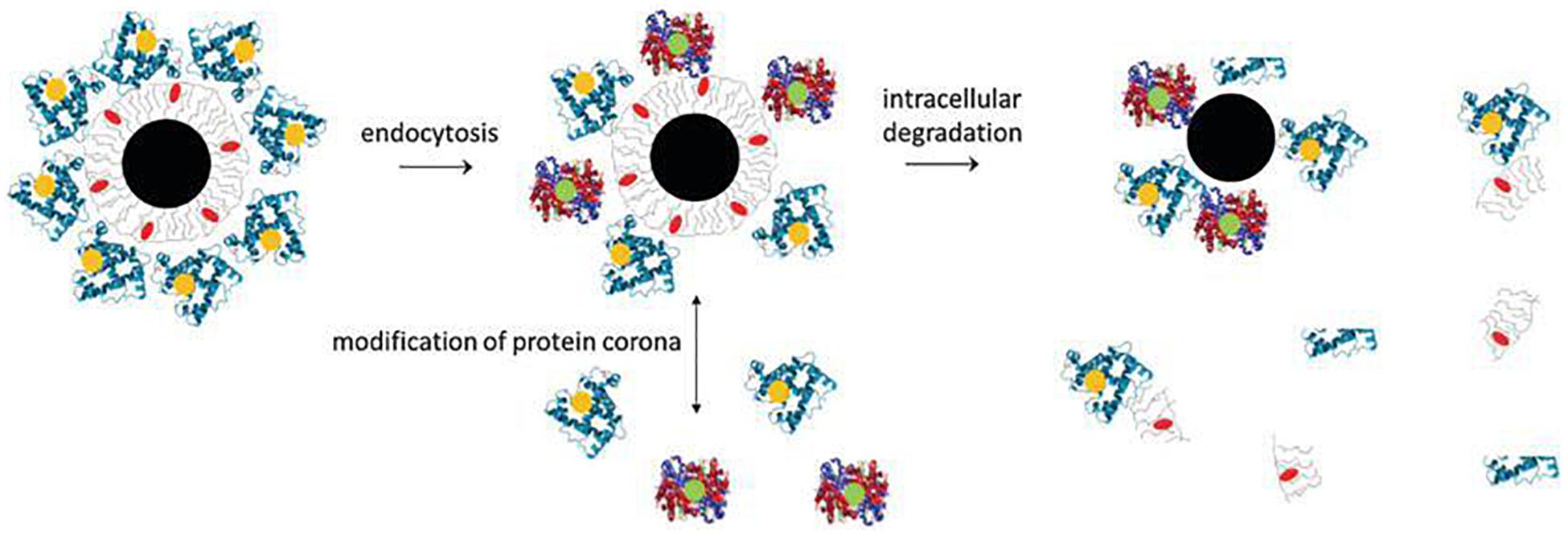
Figure 6. Composition and in vivo degradation of a tupical inorganic NP (Feliu et al., 2016). A typical inorganic NP comprises of an inorganic core (black), a engineered surface (gray) and a shell of adsorbed biological molecules (blue). During the degradation process, the nanoparticles may break down into individual components. The inorganic core may begin to decompose first, changing its physical and morphological characteristics. The engineered organic coating can also be partly removed by intracellular degradation.
Metal based NPs are widely used for treatment of CRC, as they are characterized by high stability and possibility of large-scale production avoiding organic solvents (Klebowski et al., 2018). Silver-based nanoparticles (AgNPs) are widely used in various fields, including medicine, food, health management, consumption and industry, due to their unique optical, electrical, and thermal and biological properties (Li W. R. et al., 2011; Zhang X. F. et al., 2016). Ranjan et al. (2013) synthesized starch-capped silver-based NPs. These NPs promoted apoptosis of HCT116 cells in a p53-dependent manner, by inducing increase in p53, p21 and Caspases 3, 8, and 9, and decrease in AKT and NF-κB (Satapathy et al., 2013). These metal-based NPs can be modified to improve effectiveness in drug delivery. Ghalandari et al. (2014) developed β-lactoglobulin (β-LG) NPs for oral delivery of oxali-palladium as a metal-based drug against colon cancer. This study reports that β-LG NPs containing oxali-palladium complexed with low methoxyl pectin (LMP) is a promising candidate for improving oral drug delivery for colon cancer treatment.
Furthermore, silica is commonly used in making inorganic NPs. Mesoporous silica nanoparticles (MSNs) were first reported in early 1900s (Kresge et al., 1992), and they are promising candidates for drug delivery in biomedical field. They effectively treats cancer cells due to the following properties: (1) they have large surface area and pore volume thus drugs can be adsorbed and loaded effectively, (2) they have mesoporous structure and adjustable pore size ensuring controlled release of drugs, (3) they have a surface that can be easily modified to achieve targeted therapy, (4) they are associated with good biosafety, biodegradability, distribution and excretion evaluations characteristics, (5) they allow combination with magnetic and/or luminescent materials, therefore bioimaging can be performed during drug administration (Wang Y. et al., 2015).
Liu W. et al. (2018) developed 5-FU@MSN-NH2/GC, galactosylated chitosans (GCs) based on mesoporous silica nanoparticle (MSN-NH2) which was loaded with 5-fluorouracil (5-FU) and functionalized with amino. These NPs showed high specificity in recognizing and binding to galactin receptors on cancer cells, thus promoting apoptosis of SW620 cells (Liu W. et al., 2018). In addition, these MSNs can be further modified. Kumar et al. (2017) developed a MSN-based enzyme-responsive materials which showed specific targeting of colon cancer cells. Guar gum, a natural carbohydrate polymer, was also used to cap drugs (GG-MSN). The NPs showed good anti-tumor effect in colon cancer cell lines. Moreover, Nguyen et al. (2017) loaded prednisone into 3-aminopropyl-functionalized MSN (MCM-NH2) and then wrapped it with succinylated ε-polylysine (SPL). SPL is a polymer obtained by modification of ε-polylysine (EPL). SPL was used for the first time to control drug release at pH 5.5–7.4 (the pH of the colon), with high stability of NPs in the stomach and small intestine. In addition, SPL may interact with intestinal and cancer cells through electrostatic interactions thus increasing drug residence time at the target site.
Ligand-Conjugated Nanoparticles
Targeting NPs to the lesion sites of CRC is important for their successful use. Many targeting ligands including small molecules, peptides, polysaccharides, antibodies, receptors, DNA, RNA have been identified and explored to facilitate active targeting of NPs, as shown in Figure 7 (Azharuddin et al., 2019; Yoo et al., 2019; Graczyk et al., 2020). These ligands can bind to specific receptors on the surface of the target cells, thus increasing the uptake of the NPs by the cells and ultimately improve therapeutic efficacy (Muhamad et al., 2018). In this section, we classified nanoparticles according to the types of modified ligands.
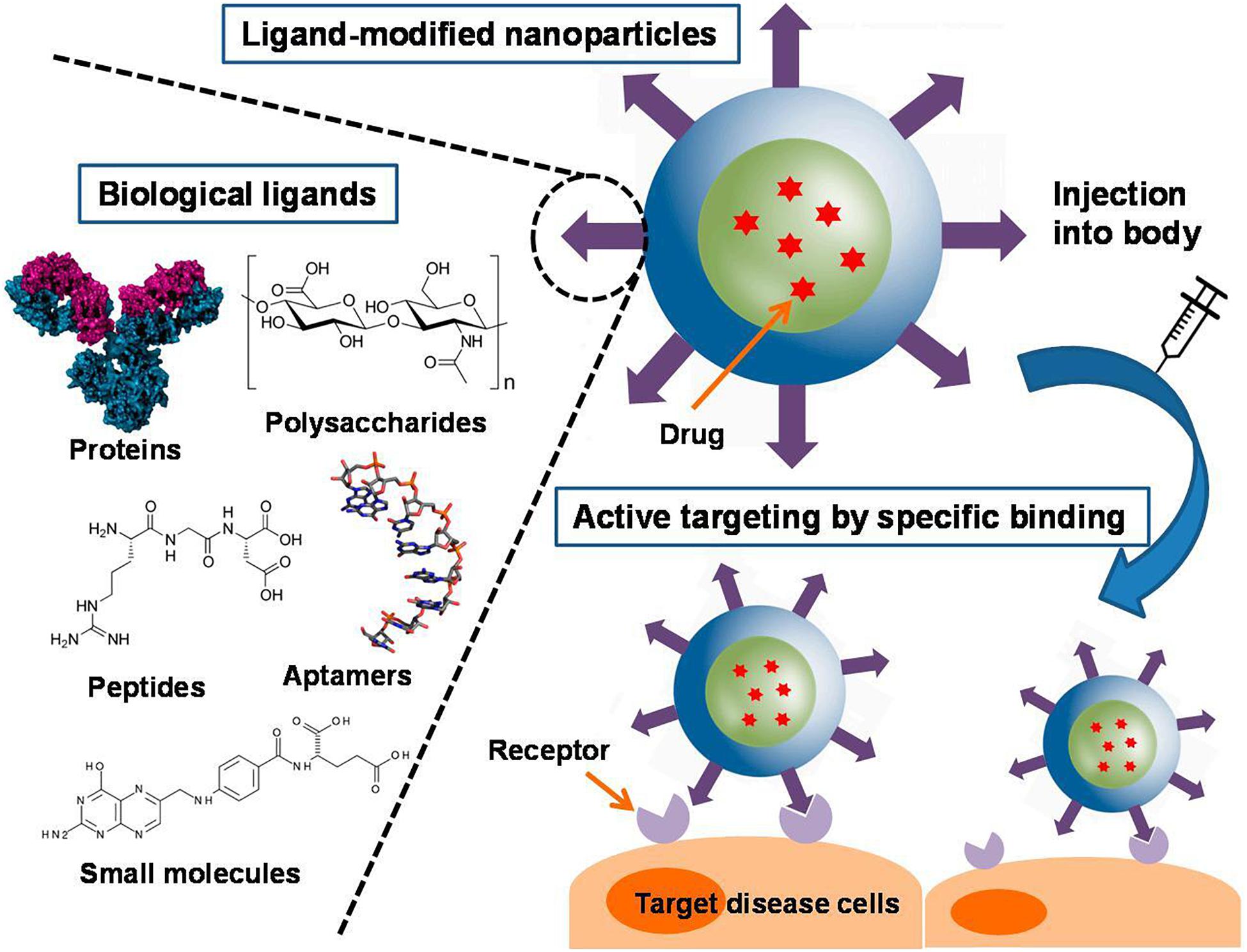
Figure 7. Different types of ligand-conjugated nanoparticles with various active molecules and their targeting mechanisms (Yoo et al., 2019). An increase in ligand density is advantageous for enhanced binding and cellular uptake. A variety of ligands, including proteins, polysaccharides, peptides, aptamers, and small molecules, are employed in functionalized nanoparticles. They can be physically adsorbed or chemically conjugated to the nanoparticles, or they can be tethered to the components of the nanoparticles before formation.
Hyaluronan (HA) is a natural polysaccharide consisting of N-acetylglucosamine and a β-glucuronic acid, which is one of the components of the extracellular matrix (Beldman et al., 2017). Its receptor, CD44, is highly expressed in several cancer types, therefore it is a potential target for cancer-targeting NPs. Xu et al. (2019) developed HA-NPs-PTC209, a colon cancer-targeted system to deliver the BMI-1 inhibitor, PTC209. PTC209 reverses stemness of CRC, thus reducing recurrence and metastasis of CRC. These HA-modified NPs have high affinity to CRC cells with high expression of CD44/CD168, and show effective targeting of tumor site. In addition, HA-CPT/CUR-NPs is a HA-modified NP developed by loading camptothecin (CPT)/curcumin (CUR) with HA-functionalized polymeric NPs (Xiao et al., 2015). This NP-based combination chemotherapy is efficient for colon cancer targeting.
Folic acid (FA) is used to modify anti-cancer drugs to improve specificity for human cancers which overexpress folate receptors (FRs). Moreover, folate binding protein, a glycosylphosphatidylinositol (GPI) that anchors cell surface receptor for folate, is overexpressed in some kinds of human cancers (Yoo and Park, 2004; Tang Y. et al., 2018). Desai et al. (2016) linked mesoporous silica NPs (MSNs) with poly(ethylene glycol) (PEG), poly(ethylene imine) (PEI) and FA in different combinations. The resulting MSNs were loaded with γ-secretase inhibitors of the Notch pathway for treatment of colon cancer. These modified MSNs specifically targeted the intestine and were easily internalized by intestinal epithelial cells, maintaining structural and functional integrity in the gastrointestinal environment. Rajpoot, Kuldeep, and Jain developed oxaliplatin-loaded FA-coupled solid lipid nanoparticles (SLNs) (OPSLNFs) formulations (Rajpoot and Jain, 2018). These OPSLNFs showed high entrapment efficiency, sustained drug release and high anti-cancer activity on HT-29 cell line.
Wheat germ agglutinin (WGA) is a dimer composed of two subunits and has four carbohydrate binding sites. It is derived from cereals and is a low immunogenic lectin (Xie et al., 2007). WGA recognizes certain types of cancer cells, therefore it is used to deliver NPs (Mo and Lim, 2005; Gao et al., 2007; Shen et al., 2011; Kuo et al., 2019). Wang and Huang (2019) integrated 5-fluorouracil (5-FU) and (−)-epigallocatechin-3-gallate (EGCG) into NPs, and then conjugated WGA to the surface of the nanoparticle through glutaraldehyde cross-linking (Wang R. et al., 2019). This colon cancer-targeted nanoparticle was named WGA-EF-NP. Glutaraldehyde cross-linking and positive charge of WGA-EF-NP results in smaller particle size and better stability in vitro and in vivo compared with EF-NP. Akl et al. (2019) designed curcumin-loaded PLGA NPs (C-PLGA NPs), and coated them with chitosan (CS), WGA and EGF analog peptides (GE11). Chitin promoted interaction of nanoparticles with cancer cells through non-specific electrostatic interactions, whereas WGA and GE11 promoted active targeting and specific recognition of cancer cells.
Song et al. (2019) developed Gd3+ -doped mesoporous hydroxyapatite NPs with polyacrylic acid (PAA) and anchored it to CS. Gadolinium was used as an MRI contrast agent in these NPs, PAA was a switch in response to pH, and PAA and CS ensured specific delivery of drugs to colon cancer tissues to avoid early release of drugs. In addition, these NPs could be loaded with chemotherapy drugs 5-FU and gefeitinib for localized targeted treatment of colon cancer. Zu et al. (2019) developed CS-functionalized camptothecin (CPT)-loaded polymeric NPs, with better pro-apoptotic ability and anti-colon cancer efficiency compared with non-targeted NPs. These CS-CPT-NPs showed a desirable hydrodynamic particle size, excellent monodispersity, narrow particle size distribution, electro-neutral surface, and good stability in biological environment, which improved their anti-cancer activities.
Alginic acid is a natural linear anionic polysaccharide which is formed from α-l-guluronic acid and β-d-mannuronic acid (Rhim et al., 2006; Zare et al., 2015). Alginic acid is characterized by good biodegradability, biocompatibility, non-toxicity and mild gelation properties, therefore, it is widely used in biomedical applications, such as surface modification of biomedical implants and drug delivery systems (Lee et al., 2009; Kolambkar et al., 2011; Srivastava et al., 2012). Victor et al. (2016) developed a nanoplatform with near-infrared fluorescence capability based on neodymium doped hydroxyapatite (HAN). The surface of these NPs was modified using alginic acid, resulting in NPs with appropriate particle size, needle shape, negative zeta potential, pH response, oral colonic targeting and were conducive to cell internalization. These NPs can be used to achieve early diagnosis of cancers, targeted cancer therapy and surveillance of colon cancer after oral administration.
Proteins and peptides are characterized by good biodegradability, biocompatibility and low immunogenicity properties, therefore, they are widely used to modify the surface of NPs. Modified proteins and peptides promote targeting of cancer tissues through receptor-ligand interaction. Colon cancer patients highly expresses CD98, therefore, Xiao et al. (2018) developed CD98 Fab’-functionalized NPs for co-delivery of camptothecin (CPT) and CD98 siRNA, which were highly effective in drug delivery to cancer tissues and showed improved anti-cancer effects as shown in Figure 8. Briefly, they coated siCD98/CPT-NPs with MAL-PEG-NHS to create MAL-siCD98/CPT-NPs. The resultant complex was reacted with Fab’-SH generated from CD98 antibodies, leading to the formation of Fab’-siCD98/CPT-NPs. The particle size, morphology, stability and cellular uptake profiles of the nanoparticles were characterized. The results showed that both nanoparticles exhibited good particle size distribution, and the PEG-siCD98/CPT-NPs were slightly smaller than Fab’-siCD98/CPT-NPs. Both nanoparticles showed solid spherical morphology as determined by transmission electron microscopy and were relatively stable in deionized water and in simulated colonic fluid. In terms of cell internalization, the cellular uptake efficiency of Fab’-CPT-NPs (II) was significantly lower in CD98-knockdown Colon-26 cells, demonstrating that CD98 might play important functions in cells. They further prepared NP-embedded hydrogel, which precisely and systematically controlled specific release of NPs in the colonic lumen and their internalization by target cancer cells.
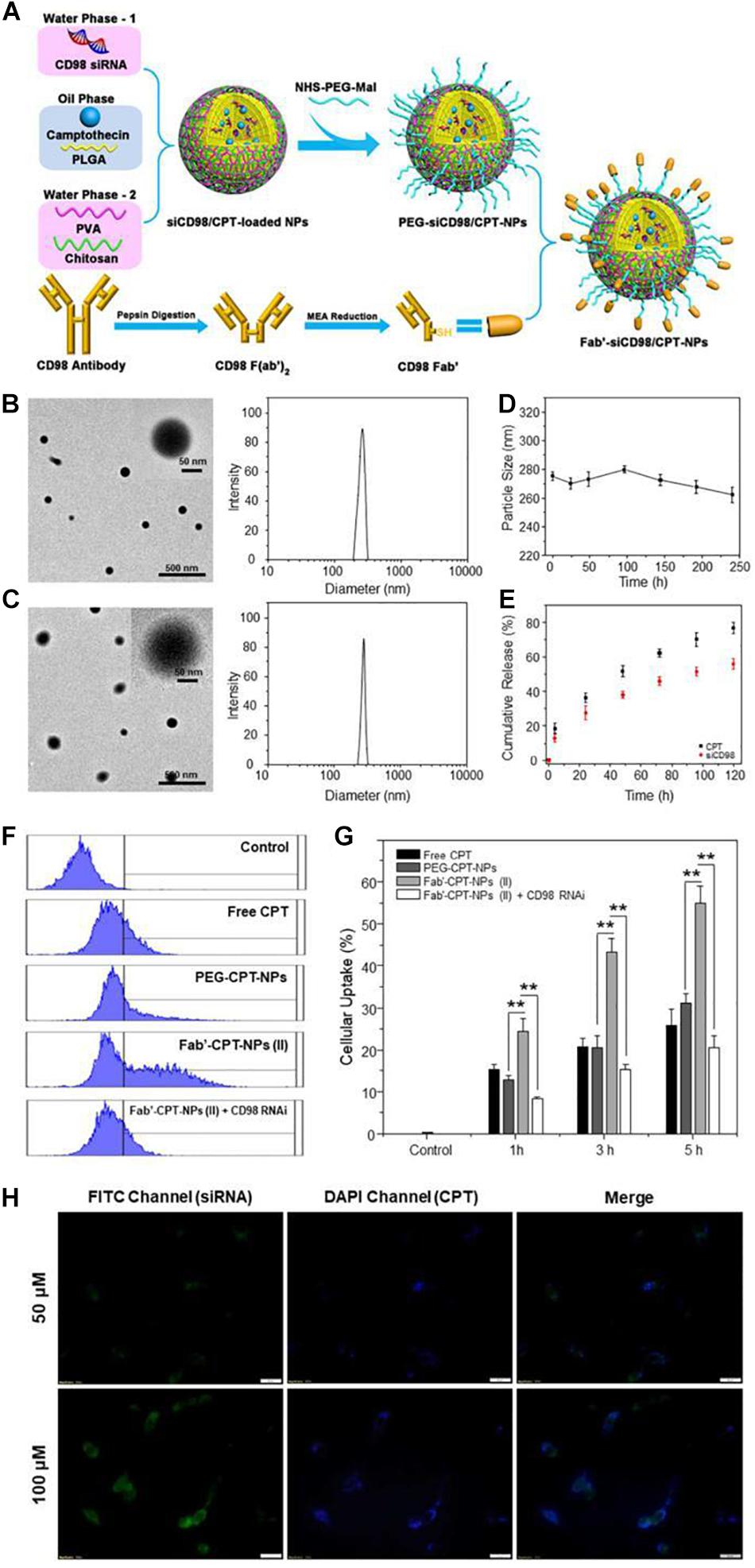
Figure 8. Fabrication, physicochemical characterization and in vitro targeting capacity of Fab’-functionalized nanoparticles (Elsabahy and Wooley, 2012). (A) A schematic illustration of preparation of Fab’-siCD98/CPT-NPs. Initially, Fab’-SH was generated from CD98 antibodies. Then MAL-siCD98/CPT-NPs were yielded by coating siCD98/CPT-NPs with MAL-PEG-NHS. Finally, MAL-siCD98/CPT-NPs were reacted with Fab’-SH to obtain II. (B,C) Representative transmission electron microscope images and size distribution of I and II, respectively. (D) The average particle size of II in deionized water after 240 h of storage at room temperature. (E) In vitro drug release profiles of siCD98 and CPT from II at 37°C. (F) Representative FCM histograms of fluorescence intensity of cells that were treated with I and II with same CPT concentration (32 μM) for 5 h. (G) Proportion of CPT-containing cells after being treated 1, 3, or 5 h as (F). (H) Fluorescence microscopy images of Fab’-FITC-siRNA/CPT-NPs cellular uptake profiles in Colon-26 cells after 5 h treatment, with a scale bar of 20 μm. Note: (I)PEG-siCD98/CPT-NPs, (II)Fab’-siCD98/CPT-NPs.
Nucleic acids can also be attached to the surface of NPs to act as ligands. Aptamers, also known as chemical antibodies, are short-stranded RNA or single-stranded DNA that bind to targets with high specificity and affinity (Alshaer et al., 2018). They are characterized by low toxicity, low immunogenicity and ease of production (Zhou and Wang, 2006). Xie et al. (2016) developed mesoporous silica NPs (MSNs) with doxorubicin (DOX) loaded and modified with aptamer, which targets epithelial cell adhesion molecule (EpCAM), and named the NP Ap-MSN-DOX. These NPs specifically bound to EpCAM over-expressing SW620 colon cancer cells, thus enhancing cellular uptake and increasing cytotoxicity to SW620 cells.
Plant-Derived Nanoparticles
Plant-derived natural NPs are used to overcome off-target cytotoxicity and high cost of mass production of traditional NPs. Nanocarriers derived from edible plants, such as ginger, grapefruit, broccoli, and lemon, can be used for drug delivery (Wang et al., 2013; Zhang et al., 2016a, b; Yang et al., 2018; Zhang M. et al., 2019). These nanocarriers have exosomal-like traits and can be applied in exosome-based drug delivery systems. Exosomes are cell-derived and widely distributed, therefore, exosome-based NPs are relatively non-toxic, non-immunogenic, highly efficient, highly specific and can be used as a powerful nanocarrier to deliver drugs. Some exosome-based NPs can be delivered orally to treat CRC (Wang J. et al., 2016).
Zhang et al. (2016a) isolated nanoparticles from edible ginger, which has several anti-inflammatory, anti-oxidative, and anti-tumor active constituents. These isolated NPs were exosome-like, with high levels of lipids, some proteins, miRNAs, and several bioactive constituents. This natural delivery mechanism ensures low toxicity and mass production of NPs. In a previous study, doxorubicin (Dox) was loaded with a nanovector made from ginger-derived lipids (GDNVs) (Zhang et al., 2016b). The drug-loaded nanovectors were effectively taken up by colon cancer cell lines, leading to the inhibition of cell proliferation and enhancement of cell apoptosis. In addition, conjugation of GDNVs and folic acid may enhance targeting of drugs to colon-26 tumors, hence improve efficacy.
Stimuli-Responsive Nanoparticles
Tumor microenvironment consists of extracellular matrix (ECM), tumor-related immune cells, neuroendocrine (NE) cells, adipose cells, blood and lymphatic vascular networks, cytokines, stroma, and other signaling molecules (Chen F. et al., 2015; Wang et al., 2017). Some bone marrow-derived progenitor cells are recruited to the TME and differentiate into endothelial cells, pericytes, fibroblasts and other stromal cells, thus promoting malignant progression of the tumor (Joyce and Pollard, 2009; Hanahan and Weinberg, 2011). In addition, TME plays an important factor in regulation of cancer cell metabolism (Cairns et al., 2011). Therefore, a better understanding of the interaction between TME, cancer cells and drugs is important in treatment of tumors.
Tumor microenvironment is characterized by acidity, hypoxia and thermal stability properties, which are conducive for development of stimuli-responsive nanoparticles. Therefore, these NPs ensure tumor targeted release as they are inactive in blood circulation and under normal physiological conditions (Yu et al., 2014; Du et al., 2015; An et al., 2016; Kang et al., 2017; Wu and Dai, 2017); once they arrive at the tumor site through passive or active targeting, they can be activated to release drugs in response to the characteristics of TME, thus achieving specific drug release and reducing adverse reactions. Interactions between stimuli-responsive nanocarriers and TME are shown in Figure 9 (Cairns et al., 2011). Stimuli-responsive nanocarriers can be used in drug delivery systems, imaging, therapy and theranostics. These carriers respond to internal stimuli (low pH, hypoxia, ATP, enzyme, redox, etc.) and external stimuli (magnetic field, electronic field, thermal, light, ultrasound, etc.). Given these properties, they can control drug release, drug and probe activation, ligand exposure, structure and size conformation, charge conversion, and response to specific biological molecules, thereby improving site-specific delivery.
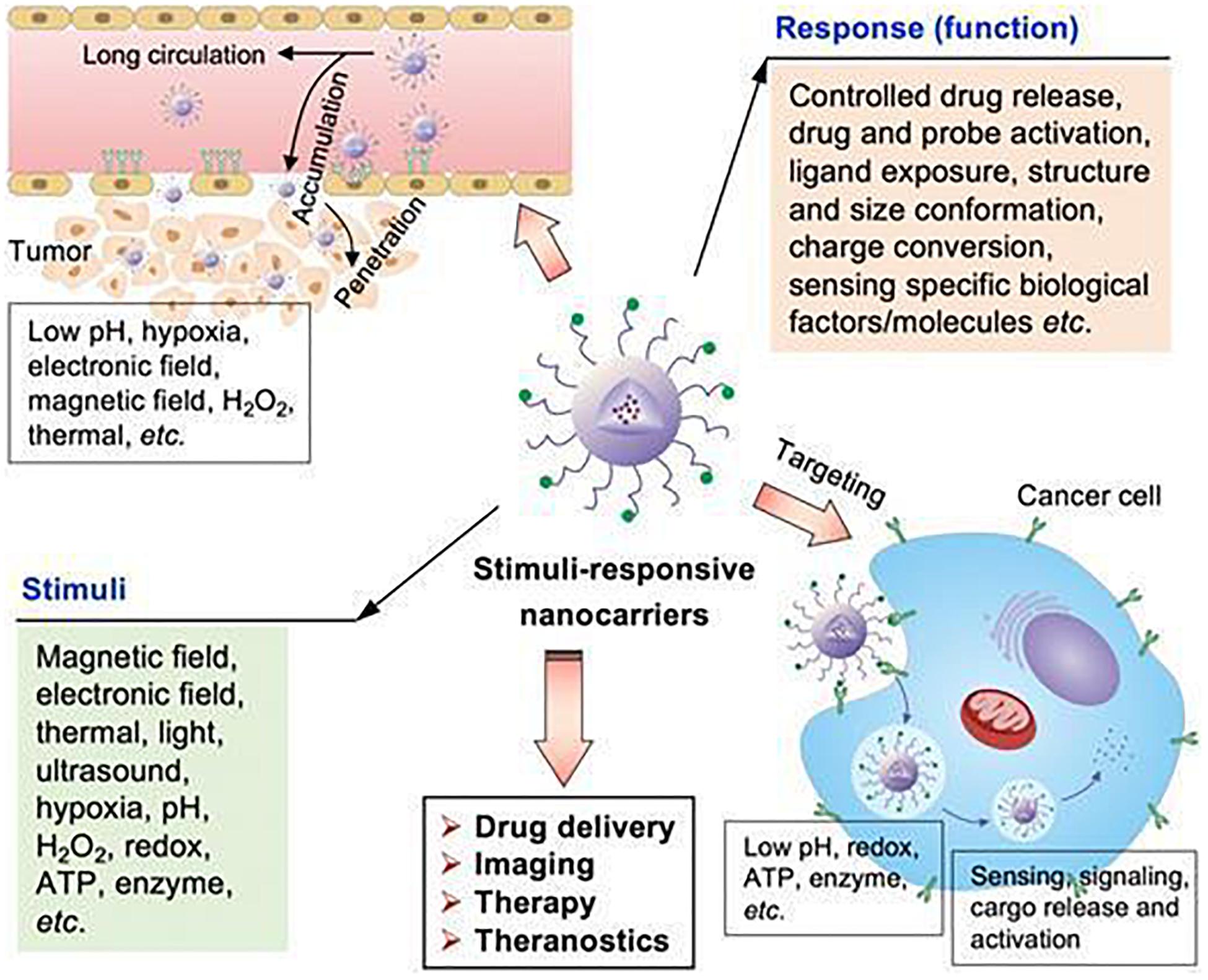
Figure 9. The stimuli-sensitive functions of stimuli-responsive nanocarriers (Cairns et al., 2011). Nanoparticles can accumulate in tumor sites, responding to internal and external stimuli. Internal stimuli include: pH, redox, ATP, enzyme, and hypoxia etc., while external stimuli include electronic field, magnetic field, thermal, light and ultrasound. The stimuli-responsive characteristic promotes the release of drugs in tumor sites to achieve accurate diagnosis and treatment of tumors.
Reactive oxygen species (ROS)-responsive NPs are common types of stimuli-responsive NPs. Vong et al. (2015) developed an oral nanotherapy using a redox nanoparticle RNP(O) by self-assembly of MeO-PEG-b-PMOT. In the core of RNP(O) is nitroxide radicals, acting as ROS scavengers. Oral administration of RNP(O) and combination with irinotecan significantly improved therapeutic efficacy for CAC in mice. Furthermore, orally administered RNP(O) was effectively internalized in cancer cells compared with normal cells, preventing undesired adverse effects and toxicity.
Several other redox nanoparticles have been developed for treatment of CRC. Vong et al. (2017) designed silica-containing redox nanoparticle (siRNP) with the ability to scavenge ROS. They also used BNS-22, a hydrophobic anticancer compound, as a novel oral nanocarrier for treatment of colitis-associated colorectal cancer.
OCD is also an oxidation-responsive material, obtained by hydrophobic linkage of β-cyclodextrin (β-CD) with an oxidation-labile group (Zhang et al., 2020). Zhang Q. et al. (2019) loaded a chemotherapeutic agent CPT-11 into OCD NP, called CPT-11/OCD NP. These NPs significantly reduced oxidative stress and inhibited inflammation in cancer cells. After oral administration, angiogenesis and tumor growth of colitis-associated colon cancer were significantly inhibited, especially in the inflammatory phase.
In addition, NPs also respond to pH and thermal changes in the intestinal environment to achieve specific drug release which improves CRC treatment. The research by Xiguang Chen designed DAHBCs NPs which were pH- and thermo-responsive. Hydroxybutyl chitosan (HBC) was prepared by conjugating hydroxybutyl groups to the backbone of chitosan, which served as a thermo-responsive polymer. HBC was then modified with deoxycholic acid (DOCA) to form an amphiphilic polymer named DAHBCs NPs with the ability to self-assemble in aqueous medium (Yang et al., 2013). Curcumin (CUR) was then loaded into DAHBCs NPs, resulting in optimal drug loading ratio (Wang F. et al., 2016). These NPs exhibited pH-responsive expansion and thermo-responsive shrinkage at low pH and 37°C in the stomach, thus remaining stable (Wang T. et al., 2019). In the intestinal environment, only a thermo-responsive shrinkage effect was observed, therefore, the drug was released in large quantities in the intestinal tract, thus exerting excellent anti-tumor effect.
Summary and Perspectives
The incidence and mortality rates of CRC have been increasing annually. This necessitates the search for highly efficient and less toxic treatment options for CRC patients. Conventional treatment regimens have limited efficacy, low targeting efficiency, and numerous side effects. Nanotherapeutics has emerged as a suitable alternative for successful treatment of CRC, and various formulations of nanoparticles have been used to design drug delivery systems. Of all drug delivery strategies, oral administration is considered to be the most common and acceptable form, due to their advantages of reduced pain, ease of ingestion and high patient compliance. For drugs with poor stability, solubility and bioavailability, encapsulating them into nanoparticles might make them suitable for oral administration. In this review, we discussed the types, composition, and effects of oral nano-drugs used for CRC treatment.
Numerous studies have attempted to improve the medicinal properties of insoluble anti-tumor drugs. Some of the approaches used to achieve this include strategies to avoiding acid or enzymatic degradation of drugs in the gastric environment, increasing drug retention in the gastrointestinal tract and absorption in intestinal epithelial cells. Currently, several nano-drugs are available for clinical use. Some researchers have reviewed the nanodrugs approved for clinical use and clinical trials testing their efficacy in the treatment of CRC (Bobo et al., 2016; Caster et al., 2017; Ventola, 2017; Anselmo and Mitragotri, 2019). Table 2 summarizes the oral nanotherapeutics undergoing clinical trials for the treatment of CRC.
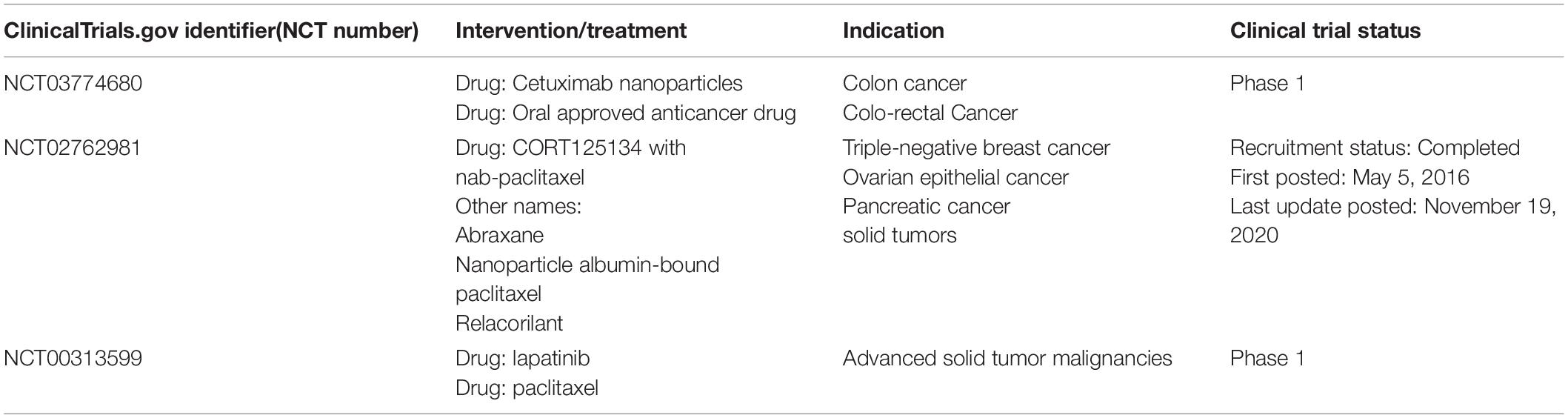
Table 2. Nanoparticles that are not clinically approved but are in clinical trials (including not yet recruiting, recruiting, or active) for treatment of CRC by oral administration.
However, compelling evidence indicates that the available nano-drug delivery systems are not sufficiently effective. Apart from biosafety and pharmacokinetics, the clinical application of nanoparticles can also be limited by various issues, including intellectual property, mass manufacturing, and overall cost-effectiveness compared with current therapies. Therefore, it is necessary to develop strategies to improve the critical characteristics of NPs so that they can be used in mainstream clinical practice to treat CRC. For example, numerous studies have shown that the adhesion of NPs to the mucus barrier limits the performance of nano-drugs. Hence, NPs that effectively penetrate the loosely adherent mucus layer and reach the firmly adherent layer have longer retention time which improves efficacy of the drugs. To simulate the absorption and transport of NPs in the intestinal tract, some cell and tissue models have been established. For example, Caco-2 cell, a human colon cancer cell line, is commonly used as a model of human intestinal epithelium without a mucus layer, with a relatively lower endocytotic activity. The Ussing chamber, an advanced in vitro technique used to study the transepithelial transport of the gut, allows us to investigate permeability and other physiological properties of the intestinal epithelium. The Ussing chamber can also be used as a model to study intestinal epithelial absorption of small molecule drugs in vivo. To accelerate the clinical application of nanoparticles, future researches should develop strategies to reduce the complexity of nanoparticle synthesis and select the optimal dosage form and formulations that are most suitable for clinical use.
Author Contributions
KY, HW, and BX wrote and revised the manuscript. BB, XG, and YX helped collect materials. HW and BX supervised and finalized this manuscript.
Funding
This work was supported by the Zhejiang Provincial Natural Science Foundation of China (grants nos. LR19H160002 to HW and LQ21H160027 to BX) and National Natural Science Foundation of China (grants nos. 82073296, 81773193, and 81571799 to HW).
Conflict of Interest
The authors declare that the research was conducted in the absence of any commercial or financial relationships that could be construed as a potential conflict of interest.
Acknowledgments
We are grateful to Home for Researchers editorial team (www.home-for-researchers.com) for language polishing and corrections on this manuscript.
References
Akl, M. A., Kartal-Hodzic, A., Suutari, T., Oksanen, T., Montagner, I. M., Rosato, A., et al. (2019). Real-time label-free targeting assessment and in vitro characterization of curcumin-loaded poly-lactic-co-glycolic acid nanoparticles for oral colon targeting. ACS Omega 4, 16878–16890. doi: 10.1021/acsomega.9b02086
Alavian, F., and Shams, N. (2020). Oral and intra-nasal administration of nanoparticles in the cerebral ischemia treatment in animal experiments: considering its advantages and disadvantages. Curr. Clin. Pharmacol. 15, 20–29. doi: 10.2174/1574884714666190704115345
Alshaer, W., Hillaireau, H., and Fattal, E. (2018). Aptamer-guided nanomedicines for anticancer drug delivery. Adv. Drug Deliv. Rev. 134, 122–137. doi: 10.1016/j.addr.2018.09.011
An, X., Zhu, A., Luo, H., Ke, H., Chen, H., and Zhao, Y. (2016). Rational design of multi-stimuli-responsive nanoparticles for precise cancer therapy. ACS Nano 10, 5947–5958. doi: 10.1021/acsnano.6b01296
Anselmo, A. C., and Mitragotri, S. (2019). Nanoparticles in the clinic: an update. Bioeng. Transl. Med. 4:e10143.
Arvelo, F., Sojo, F., and Cotte, C. (2015). Biology of colorectal cancer. Ecancermedicalscience 9:520.
Azharuddin, M., Zhu, G. H., Das, D., Ozgur, E., Uzun, L., Turner, A., et al. (2019). A repertoire of biomedical applications of noble metal nanoparticles. Chem. Commun. 55, 6964–6996. doi: 10.1039/c9cc01741k
Banerjee, D., and Sengupta, S. (2011). Nanoparticles in cancer chemotherapy. Prog. Mol. Biol. Transl. Sci. 104, 489–507.
Banik, B. L., Fattahi, P., and Brown, J. L. (2016). Polymeric nanoparticles: the future of nanomedicine. Wiley Interdiscip. Rev. Nanomed. Nanobiotechnol. 8, 271–299. doi: 10.1002/wnan.1364
Bao, G., Mitragotri, S., and Tong, S. (2013). Multifunctional nanoparticles for drug delivery and molecular imaging. Annu. Rev. Biomed. Eng. 15, 253–282.
Batist, G., Gelmon, K. A., Chi, K. N., Miller, W. J., Chia, S. K., Mayer, L. D., et al. (2009). Safety, pharmacokinetics, and efficacy of CPX-1 liposome injection in patients with advanced solid tumors. Clin. Cancer Res. 15, 692–700. doi: 10.1158/1078-0432.ccr-08-0515
Beldman, T. J., Senders, M. L., Alaarg, A., Perez-Medina, C., Tang, J., Zhao, Y., et al. (2017). Hyaluronan nanoparticles selectively target plaque-associated macrophages and improve plaque stability in atherosclerosis. ACS Nano 11, 5785–5799. doi: 10.1021/acsnano.7b01385
Benny, O., Fainaru, O., Adini, A., Cassiola, F., Bazinet, L., Adini, I., et al. (2008). An orally delivered small-molecule formulation with antiangiogenic and anticancer activity. Nat. Biotechnol. 26, 799–807. doi: 10.1038/nbt1415
Beretta, G. L., and Cavalieri, F. (2016). Engineering nanomedicines to overcome multidrug resistance in cancer therapy. Curr. Med. Chem. 23, 3–22. doi: 10.2174/0929867322666151006094559
Beutel, G., Glen, H., Schoffski, P., Chick, J., Gill, S., Cassidy, J., et al. (2005). Phase I study of OSI-7904L, a novel liposomal thymidylate synthase inhibitor in patients with refractory solid tumors. Clin. Cancer Res. 11, 5487–5495. doi: 10.1158/1078-0432.ccr-05-0104
Blanco, E., Hsiao, A., Mann, A. P., Landry, M. G., Meric-Bernstam, F., and Ferrari, M. (2011). Nanomedicine in cancer therapy: innovative trends and prospects. Cancer Sci. 102, 1247–1252. doi: 10.1111/j.1349-7006.2011.01941.x
Blanco, E., Shen, H., and Ferrari, M. (2015). Principles of nanoparticle design for overcoming biological barriers to drug delivery. Nat. Biotechnol. 33, 941–951. doi: 10.1038/nbt.3330
Bobo, D., Robinson, K. J., Islam, J., Thurecht, K. J., and Corrie, S. R. (2016). Nanoparticle-based medicines: a review of FDA-approved materials and clinical trials to date. Pharm. Res. 33, 2373–2387. doi: 10.1007/s11095-016-1958-5
Bray, F., Ferlay, J., Soerjomataram, I., Siegel, R. L., Torre, L. A., and Jemal, A. (2018). Global cancer statistics 2018: GLOBOCAN estimates of incidence and mortality worldwide for 36 cancers in 185 countries. CA Cancer J. Clin. 68, 394–424. doi: 10.3322/caac.21492
Brennan, C. A., and Garrett, W. S. (2016). Gut microbiota, inflammation, and colorectal cancer. Annu. Rev. Microbiol. 70, 395–411.
Brioschi, A., Zara, G. P., Calderoni, S., Gasco, M. R., and Mauro, A. (2008). Cholesterylbutyrate solid lipid nanoparticles as a butyric acid prodrug. Molecules 13, 230–254. doi: 10.3390/molecules13020230
Cabello, C. M., Bair, W. R., Bause, A. S., and Wondrak, G. T. (2009). Antimelanoma activity of the redox dye DCPIP (2,6-dichlorophenolindophenol) is antagonized by NQO1. Biochem. Pharmacol. 78, 344–354. doi: 10.1016/j.bcp.2009.04.016
Cairns, R. A., Harris, I. S., and Mak, T. W. (2011). Regulation of cancer cell metabolism. Nat. Rev. Cancer 11, 85–95.
Caster, J. M., Patel, A. N., Zhang, T., and Wang, A. (2017). Investigational nanomedicines in 2016: a review of nanotherapeutics currently undergoing clinical trials. Wiley Interdiscip. Rev. Nanomed. Nanobiotechnol. 9:e1416.
Cevenini, A., Celia, C., Orru, S., Sarnataro, D., Raia, M., Mollo, V., et al. (2020). Liposome-embedding silicon microparticle for oxaliplatin delivery in tumor chemotherapy. Pharmaceutics 12:559. doi: 10.3390/pharmaceutics12060559
Chen, F., Zhuang, X., Lin, L., Yu, P., Wang, Y., Shi, Y., et al. (2015). New horizons in tumor microenvironment biology: challenges and opportunities. BMC Med. 13:45. doi: 10.1186/s12916-015-0278-7
Chen, L., She, X., Wang, T., He, L., Shigdar, S., Duan, W., et al. (2015). Overcoming acquired drug resistance in colorectal cancer cells by targeted delivery of 5-FU with EGF grafted hollow mesoporous silica nanoparticles. Nanoscale 7, 14080–14092. doi: 10.1039/c5nr03527a
Chen, W., and Hu, S. (2011). Suitable carriers for encapsulation and distribution of endostar: comparison of endostar-loaded particulate carriers. Int. J. Nanomed. 6, 1535–1541. doi: 10.2147/ijn.s21881
Cheng, G., Zhang, X., Chen, Y., Lee, R. J., Wang, J., Yao, J., et al. (2019). Anticancer activity of polymeric nanoparticles containing linoleic acid-SN38 (LA-SN38) conjugate in a murine model of colorectal cancer. Colloids Surf. B Biointerfaces 181, 822–829. doi: 10.1016/j.colsurfb.2019.06.020
Chuah, L. H., Billa, N., Roberts, C. J., Burley, J. C., and Manickam, S. (2013). Curcumin-containing chitosan nanoparticles as a potential mucoadhesive delivery system to the colon. Pharm. Dev. Technol. 18, 591–599. doi: 10.3109/10837450.2011.640688
Cisterna, B. A., Kamaly, N., Choi, W. I., Tavakkoli, A., Farokhzad, O. C., and Vilos, C. (2016). Targeted nanoparticles for colorectal cancer. Nanomedicine 11, 2443–2456.
Clamp, A. R., Schoffski, P., Valle, J. W., Wilson, R. H., Marreaud, S., Govaerts, A. S., et al. (2008). A phase I and pharmacokinetic study of OSI-7904L, a liposomal thymidylate synthase inhibitor in combination with oxaliplatin in patients with advanced colorectal cancer. Cancer Chemother. Pharmacol. 61, 579–585. doi: 10.1007/s00280-007-0509-5
Dadwal, A., Baldi, A., and Kumar, N. R. (2018). Nanoparticles as carriers for drug delivery in cancer. Artif. Cells Nanomed. Biotechnol. 46(Suppl. 2), 295–305.
de Haan, L. H., Pot, G. K., Aarts, J. M., Rietjens, I. M., and Alink, G. M. (2006). In vivo relevance of two critical levels for NAD(P)H:quinone oxidoreductase (NQO1)-mediated cellular protection against electrophile toxicity found in vitro. Toxicol. In Vitro 20, 594–600. doi: 10.1016/j.tiv.2005.10.005
Dechy-Cabaret, O., Martin-Vaca, B., and Bourissou, D. (2004). Controlled ring-opening polymerization of lactide and glycolide. Chem. Rev. 104, 6147–6176. doi: 10.1021/cr040002s
Dehvari, K., Chen, Y., Tsai, Y. H., Tseng, S. H., and Lin, K. S. (2016). Superparamagnetic iron oxide nanorod carriers for paclitaxel delivery in the treatment and imaging of colon cancer in mice. J. Biomed. Nanotechnol. 12, 1734–1745. doi: 10.1166/jbn.2016.2283
Dekker, E., Tanis, P. J., Vleugels, J., Kasi, P. M., and Wallace, M. B. (2019). Colorectal cancer. Lancet 394, 1467–1480.
Desai, D., Prabhakar, N., Mamaeva, V., Karaman, D. S., Lahdeniemi, I. A., Sahlgren, C., et al. (2016). Targeted modulation of cell differentiation in distinct regions of the gastrointestinal tract via oral administration of differently PEG-PEI functionalized mesoporous silica nanoparticles. Int. J. Nanomed. 11, 299–313. doi: 10.2147/ijn.s94013
Dragovich, T., Mendelson, D., Kurtin, S., Richardson, K., Von Hoff, D., and Hoos, A. (2006). A Phase 2 trial of the liposomal DACH platinum L-NDDP in patients with therapy-refractory advanced colorectal cancer. Cancer Chemother. Pharmacol. 58, 759–764. doi: 10.1007/s00280-006-0235-4
Du, J., Lane, L. A., and Nie, S. (2015). Stimuli-responsive nanoparticles for targeting the tumor microenvironment. J. Control. Release 219, 205–214. doi: 10.1016/j.jconrel.2015.08.050
Duan, X., Chan, C., Han, W., Guo, N., Weichselbaum, R. R., and Lin, W. (2019). Immunostimulatory nanomedicines synergize with checkpoint blockade immunotherapy to eradicate colorectal tumors. Nat. Commun. 10:1899.
Elsabahy, M., and Wooley, K. L. (2012). Design of polymeric nanoparticles for biomedical delivery applications. Chem. Soc. Rev. 41, 2545–2561. doi: 10.1039/c2cs15327k
Entezar-Almahdi, E., Mohammadi-Samani, S., Tayebi, L., and Farjadian, F. (2020). Recent advances in designing 5-fluorouracil delivery systems: a stepping stone in the safe treatment of colorectal cancer. Int. J. Nanomed. 15, 5445–5458. doi: 10.2147/ijn.s257700
Fallingborg, J. (1999). Intraluminal pH of the human gastrointestinal tract. Dan. Med. Bull. 46, 183–196.
Fearon, E. R., and Vogelstein, B. (1990). A genetic model for colorectal tumorigenesis. Cell 61, 759–767. doi: 10.1016/0092-8674(90)90186-i
Feliu, N., Docter, D., Heine, M., Del, P. P., Ashraf, S., Kolosnjaj-Tabi, J., et al. (2016). In vivo degeneration and the fate of inorganic nanoparticles. Chem. Soc. Rev. 45, 2440–2457.
Feng, R. M., Zong, Y. N., Cao, S. M., and Xu, R. H. (2019). Current cancer situation in China: good or bad news from the 2018 Global Cancer Statistics? Cancer Commun. 39:22. doi: 10.1186/s40880-019-0368-6
Franke, A. J., Skelton, W. P., Starr, J. S., Parekh, H., Lee, J. J., Overman, M. J., et al. (2019). Immunotherapy for colorectal cancer: a review of current and novel therapeutic approaches. J. Natl. Cancer Inst. 111, 1131–1141. doi: 10.1093/jnci/djz093
Gao, X., Wu, B., Zhang, Q., Chen, J., Zhu, J., Zhang, W., et al. (2007). Brain delivery of vasoactive intestinal peptide enhanced with the nanoparticles conjugated with wheat germ agglutinin following intranasal administration. J. Control. Release 121, 156–167. doi: 10.1016/j.jconrel.2007.05.026
Ghalandari, B., Divsalar, A., Saboury, A. A., and Parivar, K. (2014). The new insight into oral drug delivery system based on metal drugs in colon cancer therapy through beta-lactoglobulin/oxali-palladium nanocapsules. J. Photochem. Photobiol. B 140, 255–265. doi: 10.1016/j.jphotobiol.2014.08.003
Graczyk, A., Pawlowska, R., Jedrzejczyk, D., and Chworos, A. (2020). Gold Nanoparticles in conjunction with nucleic acids as a modern molecular system for cellular delivery. Molecules 25:204. doi: 10.3390/molecules25010204
Griffin, B. T., Guo, J., Presas, E., Donovan, M. D., Alonso, M. J., and O’Driscoll, C. M. (2016). Pharmacokinetic, pharmacodynamic and biodistribution following oral administration of nanocarriers containing peptide and protein drugs. Adv. Drug Deliv. Rev. 106(Pt. B), 367–380. doi: 10.1016/j.addr.2016.06.006
Guo, J., Yu, Z., Das, M., and Huang, L. (2020). Nano Codelivery of Oxaliplatin and Folinic Acid Achieves Synergistic Chemo-Immunotherapy with 5-Fluorouracil for Colorectal Cancer and Liver Metastasis. ACS Nano 14, 5075–5089. doi: 10.1021/acsnano.0c01676
Gurunathan, S., Qasim, M., Park, C., Yoo, H., Kim, J. H., and Hong, K. (2018). Cytotoxic potential and molecular pathway analysis of silver nanoparticles in human colon cancer cells HCT116. Int. J. Mol. Sci. 19:2269. doi: 10.3390/ijms19082269
Han, W., Xie, B., Li, Y., Shi, L., Wan, J., Chen, X., et al. (2019). Orally deliverable nanotherapeutics for the synergistic treatment of colitis-associated colorectal cancer. Theranostics 9, 7458–7473. doi: 10.7150/thno.38081
Hanahan, D., and Weinberg, R. A. (2011). Hallmarks of cancer: the next generation. Cell 144, 646–674. doi: 10.1016/j.cell.2011.02.013
Homayun, B., Lin, X., and Choi, H. J. (2019). Challenges and recent progress in oral drug delivery systems for biopharmaceuticals. Pharmaceutics 11:129. doi: 10.3390/pharmaceutics11030129
Hosseinzadeh, H., Atyabi, F., Dinarvand, R., and Ostad, S. N. (2012). Chitosan-Pluronic nanoparticles as oral delivery of anticancer gemcitabine: preparation and in vitro study. Int. J. Nanomed. 7, 1851–1863. doi: 10.2147/ijn.s26365
Hu, C. M., Aryal, S., and Zhang, L. (2010). Nanoparticle-assisted combination therapies for effective cancer treatment. Ther. Deliv. 1, 323–334. doi: 10.4155/tde.10.13
Hu, S., Niu, M., Hu, F., Lu, Y., Qi, J., Yin, Z., et al. (2013). Integrity and stability of oral liposomes containing bile salts studied in simulated and ex vivo gastrointestinal media. Int. J. Pharm. 441, 693–700. doi: 10.1016/j.ijpharm.2012.10.025
Hua, S. (2014). Orally administered liposomal formulations for colon targeted drug delivery. Front. Pharmacol. 5:138. doi: 10.3389/fphar.2014.00138
Hua, S. (2020). Advances in oral drug delivery for regional targeting in the gastrointestinal tract - influence of physiological, pathophysiological and pharmaceutical factors. Front. Pharmacol. 11:524. doi: 10.3389/fphar.2020.00524
Huang, J. R., Lee, M. H., Li, W. S., and Wu, H. C. (2019). Liposomal irinotecan for treatment of colorectal cancer in a preclinical model. Cancers 11:281. doi: 10.3390/cancers11030281
Huh, J. W., Park, Y. A., Lee, K. Y., and Sohn, S. K. (2009). Heterogeneity of adenosine triphosphate-based chemotherapy response assay in colorectal cancer–secondary publication. Yonsei Med. J. 50, 697–703. doi: 10.3349/ymj.2009.50.5.697
Jain, A. K., and Jain, S. (2016). Advances in oral delivery of anti-cancer prodrugs. Expert Opin. Drug Deliv. 13, 1759–1775. doi: 10.1080/17425247.2016.1200554
Jain, A., and Jain, S. K. (2008). In vitro and cell uptake studies for targeting of ligand anchored nanoparticles for colon tumors. Eur. J. Pharm. Sci. 35, 404–416. doi: 10.1016/j.ejps.2008.08.008
Jain, A., Jain, S. K., Ganesh, N., Barve, J., and Beg, A. M. (2010). Design and development of ligand-appended polysaccharidic nanoparticles for the delivery of oxaliplatin in colorectal cancer. Nanomedicine 6, 179–190. doi: 10.1016/j.nano.2009.03.002
Joyce, J. A., and Pollard, J. W. (2009). Microenvironmental regulation of metastasis. Nat. Rev. Cancer 9, 239–252. doi: 10.1038/nrc2618
Juang, V., Chang, C. H., Wang, C. S., Wang, H. E., and Lo, Y. L. (2019). pH-Responsive PEG-shedding and targeting peptide-modified nanoparticles for dual-delivery of irinotecan and microRNA to enhance tumor-specific therapy. Small 15:e1903296.
Kamaly, N., Xiao, Z., Valencia, P. M., Radovic-Moreno, A. F., and Farokhzad, O. C. (2012). Targeted polymeric therapeutic nanoparticles: design, development and clinical translation. Chem. Soc. Rev. 41, 2971–3010. doi: 10.1039/c2cs15344k
Kamel, K. M., Khalil, I. A., Rateb, M. E., Elgendy, H., and Elhawary, S. (2017). Chitosan-coated cinnamon/oregano-loaded solid lipid nanoparticles to augment 5-fluorouracil cytotoxicity for colorectal cancer: extract standardization, nanoparticle optimization, and cytotoxicity evaluation. J. Agric. Food Chem. 65, 7966–7981. doi: 10.1021/acs.jafc.7b03093
Kang, T., Li, F., Baik, S., Shao, W., Ling, D., and Hyeon, T. (2017). Surface design of magnetic nanoparticles for stimuli-responsive cancer imaging and therapy. Biomaterials 136, 98–114. doi: 10.1016/j.biomaterials.2017.05.013
Klebowski, B., Depciuch, J., Parlinska-Wojtan, M., and Baran, J. (2018). Applications of Noble metal-based nanoparticles in medicine. Int. J. Mol. Sci. 19:4031.
Kolambkar, Y. M., Dupont, K. M., Boerckel, J. D., Huebsch, N., Mooney, D. J., Hutmacher, D. W., et al. (2011). An alginate-based hybrid system for growth factor delivery in the functional repair of large bone defects. Biomaterials 32, 65–74. doi: 10.1016/j.biomaterials.2010.08.074
Kotelevets, L., Chastre, E., Caron, J., Mougin, J., Bastian, G., Pineau, A., et al. (2017). A squalene-based nanomedicine for oral treatment of colon cancer. Cancer Res. 77, 2964–2975. doi: 10.1158/0008-5472.can-16-1741
Kresge, C. T., Leonowicz, M. E., Roth, W. J., Vartuli, J. C., and Beck, J. S. (1992). Ordered mesoporous molecular sieves synthesized by a liquid-crystal template mechanism. Nature 359, 710–712. doi: 10.1038/359710a0
Kumagai, M., Sarma, T. K., Cabral, H., Kaida, S., Sekino, M., Herlambang, N., et al. (2010). Enhanced in vivo magnetic resonance imaging of tumors by PEGylated iron-oxide-gold core-shell nanoparticles with prolonged blood circulation properties. Macromol. Rapid Commun. 31, 1521–1528. doi: 10.1002/marc.201000341
Kumar, B., Kulanthaivel, S., Mondal, A., Mishra, S., Banerjee, B., Bhaumik, A., et al. (2017). Mesoporous silica nanoparticle based enzyme responsive system for colon specific drug delivery through guar gum capping. Colloids Surf. B Biointerfaces 150, 352–361. doi: 10.1016/j.colsurfb.2016.10.049
Kuo, Y. C., Chang, Y. H., and Rajesh, R. (2019). Targeted delivery of etoposide, carmustine and doxorubicin to human glioblastoma cells using methoxy poly(ethylene glycol)poly(epsiloncaprolactone) nanoparticles conjugated with wheat germ agglutinin and folic acid. Mater. Sci. Eng. C Mater. Biol. Appl. 96, 114–128. doi: 10.1016/j.msec.2018.10.094
Lee, J., Tan, C. Y., Lee, S. K., Kim, Y. H., and Lee, K. Y. (2009). Controlled delivery of heat shock protein using an injectable microsphere/hydrogel combination system for the treatment of myocardial infarction. J. Control. Release 137, 196–202. doi: 10.1016/j.jconrel.2009.04.008
Lei, S., Chien, P. Y., Sheikh, S., Zhang, A., Ali, S., and Ahmad, I. (2004). Enhanced therapeutic efficacy of a novel liposome-based formulation of SN-38 against human tumor models in SCID mice. Anticancer Drugs 15, 773–778. doi: 10.1097/00001813-200409000-00006
Li, C., Cai, G., Song, D., Gao, R., Teng, P., Zhou, L., et al. (2019). Development of EGFR-targeted evodiamine nanoparticles for the treatment of colorectal cancer. Biomater. Sci. 7, 3627–3639. doi: 10.1039/c9bm00613c
Li, H., Zhao, X., Ma, Y., Zhai, G., Li, L., and Lou, H. (2009). Enhancement of gastrointestinal absorption of quercetin by solid lipid nanoparticles. J. Control. Release 133, 238–244. doi: 10.1016/j.jconrel.2008.10.002
Li, P., Wang, Y., Zeng, F., Chen, L., Peng, Z., and Kong, L. X. (2011). Synthesis and characterization of folate conjugated chitosan and cellular uptake of its nanoparticles in HT-29 cells. Carbohydr. Res. 346, 801–806. doi: 10.1016/j.carres.2011.01.027
Li, S., Wang, A., Jiang, W., and Guan, Z. (2008). Pharmacokinetic characteristics and anticancer effects of 5-fluorouracil loaded nanoparticles. BMC Cancer 8:103. doi: 10.1186/1471-2407-8-103
Li, W. R., Xie, X. B., Shi, Q. S., Duan, S. S., Ouyang, Y. S., and Chen, Y. B. (2011). Antibacterial effect of silver nanoparticles on Staphylococcus aureus. Biometals 24, 135–141. doi: 10.1007/s10534-010-9381-6
Li, Y., Gao, Y., Gong, C., Wang, Z., Xia, Q., Gu, F., et al. (2018). A33 antibody-functionalized exosomes for targeted delivery of doxorubicin against colorectal cancer. Nanomedicine 14, 1973–1985. doi: 10.1016/j.nano.2018.05.020
Liu, Q., Li, R. T., Qian, H. Q., Yang, M., Zhu, Z. S., Wu, W., et al. (2012). Gelatinase-stimuli strategy enhances the tumor delivery and therapeutic efficacy of docetaxel-loaded poly(ethylene glycol)-poly(varepsilon-caprolactone) nanoparticles. Int. J. Nanomed. 7, 281–295. doi: 10.2147/ijn.s26697
Liu, W., Zhu, Y., Wang, F., Li, X., Liu, X., Pang, J., et al. (2018). Galactosylated chitosan-functionalized mesoporous silica nanoparticles for efficient colon cancer cell-targeted drug delivery. R. Soc. Open Sci. 5:181027. doi: 10.1098/rsos.181027
Liu, X., Jiang, J., Chan, R., Ji, Y., Lu, J., Liao, Y. P., et al. (2019). Improved efficacy and reduced toxicity using a custom-designed irinotecan-delivering silicasome for orthotopic colon cancer. ACS Nano 13, 38–53. doi: 10.1021/acsnano.8b06164
Liu, Y., Sethi, N. S., Hinoue, T., Schneider, B. G., Cherniack, A. D., Sanchez-Vega, F., et al. (2018). Comparative molecular analysis of gastrointestinal adenocarcinomas. Cancer Cell 33, 721–735.e8.
Marill, J., Mohamed, A. N., and Paris, S. (2019). DNA damage enhancement by radiotherapy-activated hafnium oxide nanoparticles improves cGAS-STING pathway activation in human colorectal cancer cells. Radiother. Oncol. 141, 262–266. doi: 10.1016/j.radonc.2019.07.029
Markowitz, S. D., and Bertagnolli, M. M. (2009). Molecular origins of cancer: molecular basis of colorectal cancer. N. Engl. J. Med. 361, 2449–2460.
Martens, T. F., Remaut, K., Demeester, J., De Smedt, S. C., and Braeckmans, K. (2014). Intracellular delivery of nanomaterials: how to catch endosomal escape in the act. Nano Today 9, 344–364. doi: 10.1016/j.nantod.2014.04.011
Minelli, R., Serpe, L., Pettazzoni, P., Minero, V., Barrera, G., Gigliotti, C., et al. (2012). Cholesteryl butyrate solid lipid nanoparticles inhibit the adhesion and migration of colon cancer cells. Br. J. Pharmacol. 166, 587–601. doi: 10.1111/j.1476-5381.2011.01768.x
Miura, K., Shima, H., Takebe, N., Rhie, J., Satoh, K., Kakugawa, Y., et al. (2017). Drug delivery of oral anti-cancer fluoropyrimidine agents. Expert Opin. Drug Deliv. 14, 1355–1366. doi: 10.1080/17425247.2017.1316260
Mo, Y., and Lim, L. Y. (2005). Preparation and in vitro anticancer activity of wheat germ agglutinin (WGA)-conjugated PLGA nanoparticles loaded with paclitaxel and isopropyl myristate. J. Control. Release 107, 30–42. doi: 10.1016/j.jconrel.2004.06.024
Mokhtarzadeh, A., Vahidnezhad, H., Youssefian, L., Mosafer, J., Baradaran, B., and Uitto, J. (2019). Applications of spherical nucleic acid nanoparticles as delivery systems. Trends Mol. Med. 25, 1066–1079. doi: 10.1016/j.molmed.2019.08.012
Muhamad, N., Plengsuriyakarn, T., and Na-Bangchang, K. (2018). Application of active targeting nanoparticle delivery system for chemotherapeutic drugs and traditional/herbal medicines in cancer therapy: a systematic review. Int. J. Nanomed. 13, 3921–3935. doi: 10.2147/ijn.s165210
Narayanan, D., Jayakumar, R., and Chennazhi, K. P. (2014). Versatile carboxymethyl chitin and chitosan nanomaterials: a review. Wiley Interdiscip. Rev. Nanomed. Nanobiotechnol. 6, 574–598. doi: 10.1002/wnan.1301
Nguyen, C. T., Webb, R. I., Lambert, L. K., Strounina, E., Lee, E. C., Parat, M. O., et al. (2017). Bifunctional succinylated epsilon-polylysine-coated mesoporous silica nanoparticles for pH-responsive and intracellular drug delivery targeting the colon. ACS Appl. Mater. Interfaces 9, 9470–9483. doi: 10.1021/acsami.7b00411
Ning, S. T., Lee, S. Y., Wei, M. F., Peng, C. L., Lin, S. Y., Tsai, M. H., et al. (2016). Targeting Colorectal cancer stem-like cells with Anti-CD133 antibody-conjugated SN-38 nanoparticles. ACS Appl. Mater. Interfaces 8, 17793–17804. doi: 10.1021/acsami.6b04403
O’Driscoll, C. M., Bernkop-Schnurch, A., Friedl, J. D., Preat, V., and Jannin, V. (2019). Oral delivery of non-viral nucleic acid-based therapeutics - do we have the guts for this? Eur. J. Pharm. Sci. 133, 190–204. doi: 10.1016/j.ejps.2019.03.027
Omar, S., Aldosari, B., Refai, H., and Gohary, O. A. (2007). Colon-specific drug delivery for mebeverine hydrochloride. J. Drug Target 15, 691–700. doi: 10.1080/10611860701603281
Pavitra, E., Dariya, B., Srivani, G., Kang, S. M., Alam, A., Sudhir, P. R., et al. (2019). Engineered nanoparticles for imaging and drug delivery in colorectal cancer. Semin. Cancer Biol. 69, 293–306. doi: 10.1016/j.semcancer.2019.06.017
Pinto, J. F. (2010). Site-specific drug delivery systems within the gastro-intestinal tract: from the mouth to the colon. Int. J. Pharm. 395, 44–52. doi: 10.1016/j.ijpharm.2010.05.003
Prabaharan, M. (2015). Chitosan-based nanoparticles for tumor-targeted drug delivery. Int. J. Biol. Macromol. 72, 1313–1322. doi: 10.1016/j.ijbiomac.2014.10.052
Prados, J., Melguizo, C., Ortiz, R., Perazzoli, G., Cabeza, L., Alvarez, P. J., et al. (2013). Colon cancer therapy: recent developments in nanomedicine to improve the efficacy of conventional chemotherapeutic drugs. Anticancer Agents Med. Chem. 13, 1204–1216. doi: 10.2174/18715206113139990325
Punt, C. J., Koopman, M., and Vermeulen, L. (2017). From tumour heterogeneity to advances in precision treatment of colorectal cancer. Nat. Rev. Clin. Oncol. 14, 235–246. doi: 10.1038/nrclinonc.2016.171
Rajpoot, K., and Jain, S. K. (2018). Colorectal cancer-targeted delivery of oxaliplatin via folic acid-grafted solid lipid nanoparticles: preparation, optimization, and in vitro evaluation. Artif. Cells Nanomed. Biotechnol. 46, 1236–1247. doi: 10.1080/21691401.2017.1366338
Rajpoot, K., and Jain, S. K. (2020). Oral delivery of pH-responsive alginate microbeads incorporating folic acid-grafted solid lipid nanoparticles exhibits enhanced targeting effect against colorectal cancer: a dual-targeted approach. Int. J. Biol. Macromol. 151, 830–844. doi: 10.1016/j.ijbiomac.2020.02.132
Rauf, A., Imran, M., Khan, I. A., Ur-Rehman, M., Gilani, S. A., Mehmood, Z., et al. (2018). Anticancer potential of quercetin: a comprehensive review. Phytother. Res. 32, 2109–2130. doi: 10.1002/ptr.6155
Reinholz, J., Landfester, K., and Mailander, V. (2018). The challenges of oral drug delivery via nanocarriers. Drug Deliv. 25, 1694–1705. doi: 10.1080/10717544.2018.1501119
Rhim, J. W., Hong, S. I., Park, H. M., and Ng, P. K. (2006). Preparation and characterization of chitosan-based nanocomposite films with antimicrobial activity. J. Agric. Food Chem. 54, 5814–5822. doi: 10.1021/jf060658h
Ricart, A. D., Berlin, J. D., Papadopoulos, K. P., Syed, S., Drolet, D. W., Quaratino-Baker, C., et al. (2008). Phase I, pharmacokinetic and biological correlative study of OSI-7904L, a novel liposomal thymidylate synthase inhibitor, and cisplatin in patients with solid tumors. Clin. Cancer Res. 14, 7947–7955. doi: 10.1158/1078-0432.ccr-08-0864
Ritschel, W. A. (1991). Microemulsions for improved peptide absorption from the gastrointestinal tract. Methods Find. Exp. Clin. Pharmacol. 13, 205–220.
Rowland, I. R. (1988). Factors affecting metabolic activity of the intestinal microflora. Drug Metab. Rev. 19, 243–261. doi: 10.3109/03602538808994135
Saber, M. M., Al-Mahallawi, A. M., Nassar, N. N., Stork, B., and Shouman, S. A. (2018). Targeting colorectal cancer cell metabolism through development of cisplatin and metformin nano-cubosomes. BMC Cancer 18:822.
Satapathy, S. R., Mohapatra, P., Preet, R., Das, D., Sarkar, B., Choudhuri, T., et al. (2013). Silver-based nanoparticles induce apoptosis in human colon cancer cells mediated through p53. Nanomedicine 8, 1307–1322. doi: 10.2217/nnm.12.176
Sauraj, Kumar, V., Kumar, B., Deeba, F., Bano, S., Kulshreshtha, A., et al. (2019). Lipophilic 5-fluorouracil prodrug encapsulated xylan-stearic acid conjugates nanoparticles for colon cancer therapy. Int. J. Biol. Macromol. 128, 204–213. doi: 10.1016/j.ijbiomac.2019.01.101
Sekhon, B. S., and Kamboj, S. R. (2010). Inorganic nanomedicine–part 2. Nanomedicine 6, 612–618. doi: 10.1016/j.nano.2010.04.003
Selby, L. I., Cortez-Jugo, C. M., Such, G. K., and Johnston, A. (2017). Nanoescapology: progress toward understanding the endosomal escape of polymeric nanoparticles. Wiley Interdiscip. Rev. Nanomed. Nanobiotechnol. 9:e1452. doi: 10.1002/wnan.1452
Shen, M. Y., Liu, T. I., Yu, T. W., Kv, R., Chiang, W. H., Tsai, Y. C., et al. (2019). Hierarchically targetable polysaccharide-coated solid lipid nanoparticles as an oral chemo/thermotherapy delivery system for local treatment of colon cancer. Biomaterials 197, 86–100. doi: 10.1016/j.biomaterials.2019.01.019
Shen, Y., Chen, J., Liu, Q., Feng, C., Gao, X., Wang, L., et al. (2011). Effect of wheat germ agglutinin density on cellular uptake and toxicity of wheat germ agglutinin conjugated PEG-PLA nanoparticles in Calu-3 cells. Int. J. Pharm. 413, 184–193. doi: 10.1016/j.ijpharm.2011.04.026
Siegel, R. L., Miller, K. D., Goding, S. A., Fedewa, S. A., Butterly, L. F., Anderson, J. C., et al. (2020). Colorectal cancer statistics, 2020. CA Cancer J. Clin. 70, 145–164. doi: 10.1016/j.clcc.2020.07.001
Sinha, V. R., and Kumria, R. (2001). Polysaccharides in colon-specific drug delivery. Int. J. Pharm. 224, 19–38. doi: 10.1016/s0378-5173(01)00720-7
Smith, T., Affram, K., Bulumko, E., and Agyare, E. (2018). Evaluation of in-vitro cytotoxic effect of 5-FU loaded-chitosan nanoparticles against spheroid models. J. Nat. Sci. 4:e535.
Song, Q., Jia, J., Niu, X., Zheng, C., Zhao, H., Sun, L., et al. (2019). An oral drug delivery system with programmed drug release and imaging properties for orthotopic colon cancer therapy. Nanoscale 11, 15958–15970. doi: 10.1039/c9nr03802g
Srivastava, M., Singh, J., Yashpal, M., Gupta, D. K., Mishra, R. K., Tripathi, S., et al. (2012). Synthesis of superparamagnetic bare Fe(3)O(4) nanostructures and core/shell (Fe(3)O(4)/alginate) nanocomposites. Carbohydr. Polym. 89, 821–829. doi: 10.1016/j.carbpol.2012.04.016
Subudhi, M. B., Jain, A., Jain, A., Hurkat, P., Shilpi, S., Gulbake, A., et al. (2015). Eudragit S100 coated citrus pectin nanoparticles for colon targeting of 5-Fluorouracil. Materials 8, 832–849. doi: 10.3390/ma8030832
Tang, W. L., Tang, W. H., and Li, S. D. (2018). Cancer theranostic applications of lipid-based nanoparticles. Drug Discov. Today 23, 1159–1166. doi: 10.1016/j.drudis.2018.04.007
Tang, Y., Li, Y., Xu, R., Li, S., Hu, H., Xiao, C., et al. (2018). Self-assembly of folic acid dextran conjugates for cancer chemotherapy. Nanoscale 10, 17265–17274. doi: 10.1039/c8nr04657c
Tapeinos, C., Battaglini, M., and Ciofani, G. (2017). Advances in the design of solid lipid nanoparticles and nanostructured lipid carriers for targeting brain diseases. J. Control. Release 264, 306–332. doi: 10.1016/j.jconrel.2017.08.033
Tee, J. K., Yip, L. X., Tan, E. S., Santitewagun, S., Prasath, A., Ke, P. C., et al. (2019). Nanoparticles’ interactions with vasculature in diseases. Chem. Soc. Rev. 48, 5381–5407. doi: 10.1039/c9cs00309f
Tian, B., Liu, S., Wu, S., Lu, W., Wang, D., Jin, L., et al. (2017). pH-responsive poly (acrylic acid)-gated mesoporous silica and its application in oral colon targeted drug delivery for doxorubicin. Colloids Surf. B Biointerfaces 154, 287–296. doi: 10.1016/j.colsurfb.2017.03.024
Tran, P., Wang, T., Yin, W., Tran, T., Nguyen, T., Lee, B. J., et al. (2019). Aspirin-loaded nanoexosomes as cancer therapeutics. Int. J. Pharm. 572:118786. doi: 10.1016/j.ijpharm.2019.118786
Udofot, O., Affram, K., Israel, B., and Agyare, E. (2015). Cytotoxicity of 5-fluorouracil-loaded pH-sensitive liposomal nanoparticles in colorectal cancer cell lines. Integr. Cancer Sci. Ther. 2, 245–252.
Urbanska, A. M., Karagiannis, E. D., Guajardo, G., Langer, R. S., and Anderson, D. G. (2012). Therapeutic effect of orally administered microencapsulated oxaliplatin for colorectal cancer. Biomaterials 33, 4752–4761. doi: 10.1016/j.biomaterials.2012.03.023
Venkatesan, P., Puvvada, N., Dash, R., Prashanth, K. B., Sarkar, D., Azab, B., et al. (2011). The potential of celecoxib-loaded hydroxyapatite-chitosan nanocomposite for the treatment of colon cancer. Biomaterials 32, 3794–3806. doi: 10.1016/j.biomaterials.2011.01.027
Ventola, C. L. (2017). Progress in nanomedicine: approved and investigational nanodrugs. P T 42, 742–755.
Vermeulen, L., Morrissey, E., van der Heijden, M., Nicholson, A. M., Sottoriva, A., Buczacki, S., et al. (2013). Defining stem cell dynamics in models of intestinal tumor initiation. Science 342, 995–998. doi: 10.1126/science.1243148
Victor, S. P., Paul, W., Vineeth, V. M., Komeri, R., Jayabalan, M., and Sharma, C. P. (2016). Neodymium doped hydroxyapatite theranostic nanoplatforms for colon specific drug delivery applications. Colloids Surf. B Biointerfaces 145, 539–547. doi: 10.1016/j.colsurfb.2016.05.067
Vong, L. B., Kimura, S., and Nagasaki, Y. (2017). Newly designed silica-containing redox nanoparticles for oral delivery of novel TOP2 catalytic inhibitor for treating colon cancer. Adv. Healthc. Mater. 6:1700428. doi: 10.1002/adhm.201700428
Vong, L. B., Yoshitomi, T., Matsui, H., and Nagasaki, Y. (2015). Development of an oral nanotherapeutics using redox nanoparticles for treatment of colitis-associated colon cancer. Biomaterials 55, 54–63. doi: 10.1016/j.biomaterials.2015.03.037
Wahlgren, M., Axenstrand, M., Hakansson, A., Marefati, A., and Lomstein, P. B. (2019). In vitro methods to study colon release: state of the art and an outlook on new strategies for better in-vitro biorelevant release media. Pharmaceutics 11:95. doi: 10.3390/pharmaceutics11020095
Wang, A. Z., Langer, R., and Farokhzad, O. C. (2012). Nanoparticle delivery of cancer drugs. Annu. Rev. Med. 63, 185–198.
Wang, C., Ho, P. C., and Lim, L. Y. (2010). Wheat germ agglutinin-conjugated PLGA nanoparticles for enhanced intracellular delivery of paclitaxel to colon cancer cells. Int. J. Pharm. 400, 201–210. doi: 10.1016/j.ijpharm.2010.08.023
Wang, F., Xia, G., Lang, X., Wang, X., Bao, Z., Shah, Z., et al. (2016). Influence of the graft density of hydrophobic groups on thermo-responsive nanoparticles for anti-cancer drugs delivery. Colloids Surf. B Biointerfaces 148, 147–156. doi: 10.1016/j.colsurfb.2016.08.042
Wang, J., Zheng, Y., and Zhao, M. (2016). Exosome-based cancer therapy: implication for targeting cancer stem cells. Front. Pharmacol. 7:533. doi: 10.3389/fphar.2016.00533
Wang, M., Zhao, J., Zhang, L., Wei, F., Lian, Y., Wu, Y., et al. (2017). Role of tumor microenvironment in tumorigenesis. J. Cancer 8, 761–773.
Wang, Q., Zhuang, X., Mu, J., Deng, Z. B., Jiang, H., Zhang, L., et al. (2013). Delivery of therapeutic agents by nanoparticles made of grapefruit-derived lipids. Nat. Commun. 4:1867.
Wang, R., Huang, J., Chen, J., Yang, M., Wang, H., Qiao, H., et al. (2019). Enhanced anti-colon cancer efficacy of 5-fluorouracil by epigallocatechin-3- gallate co-loaded in wheat germ agglutinin-conjugated nanoparticles. Nanomedicine 21:102068. doi: 10.1016/j.nano.2019.102068
Wang, S., Liu, H., Zhang, X., and Qian, F. (2015). Intranasal and oral vaccination with protein-based antigens: advantages, challenges and formulation strategies. Protein Cell 6, 480–503. doi: 10.1007/s13238-015-0164-2
Wang, T., Wang, F., Sun, M., Tian, M., Mu, Y., Chen, X., et al. (2019). Gastric environment-stable oral nanocarriers for in situ colorectal cancer therapy. Int. J. Biol. Macromol. 139, 1035–1045. doi: 10.1016/j.ijbiomac.2019.08.088
Wang, Y., Zhao, Q., Han, N., Bai, L., Li, J., Liu, J., et al. (2015). Mesoporous silica nanoparticles in drug delivery and biomedical applications. Nanomedicine 11, 313–327.
Wang, Z. H., Liu, J. M., Li, C. Y., Wang, D., Lv, H., Lv, S. W., et al. (2019). Bacterial biofilm bioinspired persistent luminescence nanoparticles with gut-oriented drug delivery for colorectal cancer imaging and chemotherapy. ACS Appl. Mater. Interfaces 11, 36409–36419. doi: 10.1021/acsami.9b12853
Wen, P., Zong, M. H., Hu, T. G., Li, L., and Wu, H. (2018). Preparation and characterization of electrospun colon-specific delivery system for quercetin and its antiproliferative effect on cancer cells. J. Agric. Food Chem. 66, 11550–11559. doi: 10.1021/acs.jafc.8b02614
West, N. R., McCuaig, S., Franchini, F., and Powrie, F. (2015). Emerging cytokine networks in colorectal cancer. Nat. Rev. Immunol. 15, 615–629. doi: 10.1038/nri3896
Wong, K. E., Ngai, S. C., Chan, K. G., Lee, L. H., Goh, B. H., and Chuah, L. H. (2019). Curcumin nanoformulations for colorectal cancer: a review. Front. Pharmacol. 10:152. doi: 10.3389/fphar.2019.00152
Wong, M. C., Ding, H., Wang, J., Chan, P. S., and Huang, J. (2019). Prevalence and risk factors of colorectal cancer in Asia. Intest. Res. 17, 317–329. doi: 10.5217/ir.2019.00021
Wu, L., Zhang, F., Chen, X., Wan, J., Wang, Y., Li, T., et al. (2020). Self-assembled gemcitabine prodrug nanoparticles show enhanced efficacy against patient-derived pancreatic ductal adenocarcinoma. ACS Appl. Mater. Interfaces 12, 3327–3340. doi: 10.1021/acsami.9b16209
Wu, T., and Dai, Y. (2017). Tumor microenvironment and therapeutic response. Cancer Lett. 387, 61–68. doi: 10.1016/j.canlet.2016.01.043
Xiao, B., Han, M. K., Viennois, E., Wang, L., Zhang, M., Si, X., et al. (2015). Hyaluronic acid-functionalized polymeric nanoparticles for colon cancer-targeted combination chemotherapy. Nanoscale 7, 17745–17755. doi: 10.1039/c5nr04831a
Xiao, B., Viennois, E., Chen, Q., Wang, L., Han, M. K., Zhang, Y., et al. (2018). Silencing of intestinal glycoprotein CD98 by orally targeted nanoparticles enhances chemosensitization of colon cancer. ACS Nano 12, 5253–5265. doi: 10.1021/acsnano.7b08499
Xie, H. Y., Xie, M., Zhang, Z. L., Long, Y. M., Liu, X., Tang, M. L., et al. (2007). Wheat germ agglutinin-modified trifunctional nanospheres for cell recognition. Bioconjug. Chem. 18, 1749–1755. doi: 10.1021/bc060387g
Xie, X., Li, F., Zhang, H., Lu, Y., Lian, S., Lin, H., et al. (2016). EpCAM aptamer-functionalized mesoporous silica nanoparticles for efficient colon cancer cell-targeted drug delivery. Eur. J. Pharm. Sci. 83, 28–35. doi: 10.1016/j.ejps.2015.12.014
Xu, J., Zhang, Y., Xu, J., Wang, M., Liu, G., Wang, J., et al. (2019). Reversing tumor stemness via orally targeted nanoparticles achieves efficient colon cancer treatment. Biomaterials 216:119247. doi: 10.1016/j.biomaterials.2019.119247
Xu, L., Liang, H. W., Yang, Y., and Yu, S. H. (2018). Stability and reactivity: positive and negative aspects for nanoparticle processing. Chem. Rev. 118, 3209–3250. doi: 10.1021/acs.chemrev.7b00208
Xu, Y., Zheng, Y., Wu, L., Zhu, X., Zhang, Z., and Huang, Y. (2018). Novel solid lipid nanoparticle with endosomal escape function for oral delivery of insulin. ACS Appl. Mater. Interfaces 10, 9315–9324. doi: 10.1021/acsami.8b00507
Yang, C., and Merlin, D. (2020). Lipid-based drug delivery nanoplatforms for colorectal cancer therapy. Nanomaterials 10:1424. doi: 10.3390/nano10071424
Yang, C., Liu, H. Z., Fu, Z. X., and Lu, W. D. (2011). Oxaliplatin long-circulating liposomes improved therapeutic index of colorectal carcinoma. BMC Biotechnol. 11:21. doi: 10.1186/1472-6750-11-21
Yang, C., Zhang, M., and Merlin, D. (2018). Advances in plant-derived edible nanoparticle-based lipid nano-drug delivery systems as therapeutic nanomedicines. J. Mater. Chem. B 6, 1312–1321. doi: 10.1039/c7tb03207b
Yang, K., Gao, T., Bao, Z., Su, J., and Chen, X. (2013). Preparation and characterization of a novel thermosensitive nanoparticle for drug delivery in combined hyperthermia and chemotherapy. J. Mater. Chem. B 1, 6442–6448. doi: 10.1039/c3tb20772b
Yang, M., Zhang, F., Yang, C., Wang, L., Sung, J., Garg, P., et al. (2020). Oral targeted delivery by nanoparticles enhances efficacy of an Hsp90 inhibitor by reducing systemic exposure in murine models of colitis and colitis-associated cancer. J. Crohns Colitis 14, 130–141. doi: 10.1093/ecco-jcc/jjz113
Yingchoncharoen, P., Kalinowski, D. S., and Richardson, D. R. (2016). Lipid-based drug delivery systems in cancer therapy: what is available and what is yet to come. Pharmacol. Rev. 68, 701–787. doi: 10.1124/pr.115.012070
Yoo, H. S., and Park, T. G. (2004). Folate-receptor-targeted delivery of doxorubicin nano-aggregates stabilized by doxorubicin-PEG-folate conjugate. J. Control. Release 100, 247–256. doi: 10.1016/j.jconrel.2004.08.017
Yoo, J., Park, C., Yi, G., Lee, D., and Koo, H. (2019). Active targeting strategies using biological ligands for nanoparticle drug delivery systems. Cancers 11:640. doi: 10.3390/cancers11050640
Yoon, T. J., Kim, J. S., Kim, B. G., Yu, K. N., Cho, M. H., and Lee, J. K. (2005). Multifunctional nanoparticles possessing a “magnetic motor effect” for drug or gene delivery. Angew. Chem. Int. Ed Engl. 44, 1068–1071. doi: 10.1002/anie.200461910
Yu, J., Chu, X., and Hou, Y. (2014). Stimuli-responsive cancer therapy based on nanoparticles. Chem. Commun. 50, 11614–11630. doi: 10.1039/c4cc03984j
Yu, M., Yang, Y., Zhu, C., Guo, S., and Gan, Y. (2016). Advances in the transepithelial transport of nanoparticles. Drug Discov. Today 21, 1155–1161. doi: 10.1016/j.drudis.2016.05.007
Zare, E. N., Lakouraj, M. M., Mohseni, M., and Motahari, A. (2015). Multilayered electromagnetic bionanocomposite based on alginic acid: characterization and biological activities. Carbohydr. Polym. 130, 372–380. doi: 10.1016/j.carbpol.2015.05.020
Zhang, E., Xing, R., Liu, S., Qin, Y., Li, K., and Li, P. (2019). Advances in chitosan-based nanoparticles for oncotherapy. Carbohydr. Polym. 222:115004. doi: 10.1016/j.carbpol.2019.115004
Zhang, M., Viennois, E., Prasad, M., Zhang, Y., Wang, L., Zhang, Z., et al. (2016a). Edible ginger-derived nanoparticles: a novel therapeutic approach for the prevention and treatment of inflammatory bowel disease and colitis-associated cancer. Biomaterials 101, 321–340. doi: 10.1016/j.biomaterials.2016.06.018
Zhang, M., Xiao, B., Wang, H., Han, M. K., Zhang, Z., Viennois, E., et al. (2016b). Edible ginger-derived nano-lipids loaded with doxorubicin as a novel drug-delivery approach for colon cancer therapy. Mol. Ther. 24, 1783–1796. doi: 10.1038/mt.2016.159
Zhang, M., Yang, C., Yan, X., Sung, J., Garg, P., and Merlin, D. (2019). Highly biocompatible functionalized layer-by-layer ginger lipid nano vectors targeting p-selectin for delivery of doxorubicin to treat colon cancer. Adv. Ther. 2:1900129. doi: 10.1002/adtp.201900129
Zhang, Q., Zhang, F., Li, S., Liu, R., Jin, T., Dou, Y., et al. (2019). A multifunctional nanotherapy for targeted treatment of colon cancer by simultaneously regulating tumor microenvironment. Theranostics 9, 3732–3753. doi: 10.7150/thno.34377
Zhang, R., Liu, R., Liu, C., Pan, L., Qi, Y., Cheng, J., et al. (2020). A pH/ROS dual-responsive and targeting nanotherapy for vascular inflammatory diseases. Biomaterials 230:119605. doi: 10.1016/j.biomaterials.2019.119605
Zhang, X. F., Liu, Z. G., Shen, W., and Gurunathan, S. (2016). Silver nanoparticles: synthesis, characterization, properties, applications, and therapeutic approaches. Int. J. Mol. Sci. 17:1534. doi: 10.3390/ijms17091534
Zhao, X., Pan, J., Li, W., Yang, W., Qin, L., and Pan, Y. (2018). Gold nanoparticles enhance cisplatin delivery and potentiate chemotherapy by decompressing colorectal cancer vessels. Int. J. Nanomed. 13, 6207–6221. doi: 10.2147/ijn.s176928
Zhao, Y., Xu, J., Le, V. M., Gong, Q., Li, S., Gao, F., et al. (2019). EpCAM Aptamer-functionalized cationic liposome-based nanoparticles loaded with miR-139-5p for targeted therapy in colorectal cancer. Mol. Pharm. 16, 4696–4710. doi: 10.1021/acs.molpharmaceut.9b00867
Zhong, Y., Su, T., Shi, Q., Feng, Y., Tao, Z., Huang, Q., et al. (2019). Co-Administration Of iRGD enhances tumor-targeted delivery and anti-tumor effects Of paclitaxel-loaded PLGA nanoparticles for colorectal cancer treatment. Int. J. Nanomed. 14, 8543–8560. doi: 10.2147/ijn.s219820
Zhou, B., and Wang, B. (2006). Pegaptanib for the treatment of age-related macular degeneration. Exp. Eye Res. 83, 615–619. doi: 10.1016/j.exer.2006.02.010
Keywords: oral administration, drug delivery, nanoparticles, colorectal cancer, cancer therapy
Citation: Ying K, Bai B, Gao X, Xu Y, Wang H and Xie B (2021) Orally Administrable Therapeutic Nanoparticles for the Treatment of Colorectal Cancer. Front. Bioeng. Biotechnol. 9:670124. doi: 10.3389/fbioe.2021.670124
Received: 20 February 2021; Accepted: 14 May 2021;
Published: 07 July 2021.
Edited by:
Filippo Rossi, Politecnico di Milano, ItalyReviewed by:
Bruno A. Cisterna, Andres Bello University, ChileJianbo Jia, Guangzhou University, China
Copyright © 2021 Ying, Bai, Gao, Xu, Wang and Xie. This is an open-access article distributed under the terms of the Creative Commons Attribution License (CC BY). The use, distribution or reproduction in other forums is permitted, provided the original author(s) and the copyright owner(s) are credited and that the original publication in this journal is cited, in accordance with accepted academic practice. No use, distribution or reproduction is permitted which does not comply with these terms.
*Correspondence: Hangxiang Wang, wanghx@zju.edu.cn; Binbin Xie, xieBB@zju.edu.cn